- 1Department of Physical Geography and Ecosystem Science, Lund University, Lund, Sweden
- 2Geospatial Data Solutions Center, University of California, Irvine, Irvine, CA, United States
Fire regimes across the world are expected to be altered by continuing variations in socio-economic conditions and climate. Current global fire-vegetation models are able to represent the present-day fire activity, but it is unclear how well they can simulate past or future scenarios. Here we use sedimentary charcoal-based biomass burning reconstructions to evaluate fire probability and total carbon flux emitted to the atmosphere per year simulated by the dynamic global vegetation model LPJ-GUESS with its incorporated fire model SIMFIRE-BLAZE across the boreal region during the last century. The analyses were run for the whole time period (1900–2000 CE), as well as for the intervals 1900–1950 CE and 1950–2000 CE. The data–model comparison for the 20th century reveals a general disagreement in trends between charcoal reconstructions (with decreasing or stable trends) and simulations (showing an overall increase) at both global (boreal forests) and continental scales (North America and Fennoscandia), as well as for most of the regional sub-areas (Canada, Norway and Sweden). The only exceptions are Alaska and Finland/Russia Karelia, where all the variables increase. Negative correlations between observations and model outputs are also recorded for the two different sub-periods, except for Alaska and North America during the time interval 1900–1950 CE, and Norway and Finland/Russia Karelia between 1950 and 2000 CE. Despite several uncertainties in charcoal records, main differences between modeled and observed fire activity are probably due to limitations in the representation of the human impact on fire regime (especially connected to forest management and landscape fragmentation) in the model simulations.
Introduction
Fire is a major disturbance which organizes the physical and biological features of many ecosystems (Goldammer, 2013) and affects the distribution, structure and diversity of vegetation communities and fuel load (Bowman et al., 2009). Simultaneously, the fire regime (defined as the frequency, intensity, magnitude and seasonality of a fire, Krebs et al., 2010) results from complex interactions between long-term trends in climate and local fuel availability (Heyerdahl et al., 2008) and is also influenced by anthropogenic impacts (Bowman et al., 2011). Additionally, forest fires play a critical role in land-atmosphere carbon exchange and land use emissions, and thus have significant impact on biogeophysical coupling and atmospheric aerosol loads (Sommers et al., 2014; Landry et al., 2015).
Despite the fact that satellite measurements of burned area provide relative good information regarding present-day fires (Giglio et al., 2010) and already show strong temporal trends (Andela et al., 2017), these high quality data only cover a relative short time interval and they do not encompass the range of climate variability expected for the next century. Much less is thus known about long-term fire dynamics, feedbacks and resilience of ecosystems to fire disturbances. By covering a broad range of climatic and environmental conditions and varying intensities of human impact, proxy-based past fire reconstructions are the most promising tools for better evaluating ecosystems’ resilience or vulnerability to fire impact and response over long time intervals (Willis et al., 2007). Documenting coherent changes in biomass burning, sedimentary charcoal records are unique among these data sources because of their broad temporal and spatial coverage, which includes reconstructions of fire history at local to global spatial scales and from decadal to millennial temporal scales (e.g., Power et al., 2010; Iglesias et al., 2015).
Charcoal-based studies are a primary source of information about long-term fire dynamics in most terrestrial ecosystems (Tolonen, 1978; Patterson et al., 1987). Charcoal is formed from the incomplete combustion of fuel under reducing conditions. Charcoal particles are carried aloft during the fire, transported by airborne and surficial processes, and incorporated into the sediments where they are preserved. Year by year, lake and bog sediments collect and bury charcoal from local and distant fires (Whitlock and Larsen, 2001; Conedera et al., 2009). Charcoal analysis is based on the assumption that stratigraphic intervals with abundant charcoal particles represent a primary contribution that is deposited mainly through aerial fallout during or shortly after a fire (Whitlock and Larsen, 2001).
Global wildfire models coupled to dynamic global vegetation models (DGVMs) have existed since the 1990s and have advanced rapidly during the last decades (Hantson et al., 2016). However, while these models are largely able to represent present day fire occurrence (Hantson et al., 2020) uncertainty remains regarding their skill at representing changes in fire regime through time (Andela et al., 2017; Forkel et al., 2019). Hence, there is a clear need to evaluate the performance of global fire models over longer periods, for which paleodata-model comparisons are well suited.
Discrepancies between observations and simulations have been underlined by several previous studies. For example, model simulations by Pechony and Shindell (2010) suggest that during the Pre-Industrial period the global fire regime was strongly driven by precipitation, shifting to an anthropogenic-driven regime with the Industrial Revolution. These findings contradict analyses of palaeodata, indicating temperature as the most important driver of long-terms changes in biomass burning (Daniau et al., 2012; Power et al., 2013). Additionally, Marlon et al. (2016) found a disagreement between area burned simulated by the CLIMBA model (Brücher et al., 2014) and sedimentary charcoal-based fire reconstructions over the last 6000 years. Furthermore, contrary to AeroCom 1750 (Dentener et al., 2006) and the Coupled Model Intercomparison Project (CMIP) phase 5 and 6 aerosol emission datasets (Lamarque et al., 2010; van Marle et al., 2017), palaeoenvironmental archives suggest that late Pre-Industrial Holocene (PI) fire activity was similar to present-day (PD) activity, if not higher (Hamilton et al., 2018). According to Hamilton et al. (2018), global aerosol model simulations of the PD/PI ratio in black carbon concentrations using LMfire and SIMFIRE-BLAZE fire emission models are more in line with the trends predicted from palaeofire proxy records than the global aerosol model simulation which uses the CMIP6 fire emissions scenario. Moreover, discrepancies in the Southern Hemisphere fire emissions’ trends over the past two centuries are presented by Liu et al. (2021) based on Antarctic and Andean ice records (suggesting that historical fire activity exceeded present-day levels) and the LPJ-LMfire dynamic global vegetation model (simulating an overall decline in fire emissions over the 20th century). Finally, the reconstruction of fire activity based on a 200-year charcoal record from Lake Lading (Indonesia) by Cheung et al. (2021) also contradict assumptions made in current fire emissions estimates and suggest an over-simplification of the spatiotemporal complexity of fire in Equatorial Asia before the 1960s. Despite both palaeofire reconstructions (Marlon et al., 2009b) and ecosystem fire-model studies (Kloster et al., 2012, Knorr et al., 2016a) suggest a rise in frequency and severity of future forest fires linked to rapid and extreme climate variations, a better understanding of the capability of global wildfire models to reproduce reconstructed trends in fire activity is thus needed. As a result of their location at climatically sensitive high northern latitudes, the boreal regions have been suggested to be significantly affected by the ongoing anthropogenic global warming more than other regions worldwide (IPCC., 2014), with a subsequent increase in the frequency, intensity and areal extent of fires (Flannigan et al., 2009b). Furthermore, the occurrence of dry hot summers characterized by high pressure followed by thunderstorms without accompanying rains might increase the chance of lightning, the only natural cause of fire ignition in boreal forests (Veraverbeke et al., 2017). Based on these assumptions, sedimentary charcoal-based fire reconstructions are here compared with natural and anthropogenic fire dynamics simulated by the LPJ-GUESS-SIMFIRE-BLAZE coupled fire-vegetation model for the 20th century (1900–2000 CE) in boreal forests.
LPJ-GUESS-SIMFIRE-BLAZE is a new-generation prognostic wildfire scheme that takes advantage of the detailed horizontal and vertical description of vegetation structure in the DGVM LPJ-GUESS (Smith et al., 2014). Recently the model has been developed in order to enable simulations of fire occurrence, impact and emissions (Rabin et al., 2017; Pellegrini et al., 2018). Different versions of the SIMFIRE model have been used to project past and future fire occurrence (Knorr et al., 2016b, c, 2017) and a better quantification of its skill at simulating longer-term trends is needed.
In order to underline spatial differences in the results, the study area was divided into continental (North American and Fennoscandian boreal forests) and regional (Alaska, Canada, Norway, Sweden, Finland/Russia Karelia) sub-areas. Furthermore, the analyses were run for the whole time period taken into consideration (1900–2000 CE), as well as for two different sub-periods corresponding to the intervals 1900–1950 CE and 1950–2000 CE. The evaluation of model projections that incorporate the behavior and ecosystem effects of forest fires based on proxy-based reconstructions enabled us to highlight the correlation between simulated and reconstructed past fire activity and investigate the possible reasons for differences and similarities between observations and model outputs.
Materials and Methods
Charcoal-Based Fire Reconstructions
Variations in biomass burning across the boreal biome (a transcontinental circumpolar band located between 50 and 70oN latitude) were analyzed based on 88 sedimentary charcoal records from lakes and peat bogs characterized by robust dating control. Even though some geographic areas are less well represented than others, a broad view of boreal forests’ fire activity during the investigated period is provided (Figure 1). Charcoal data were selected from the latest version of the Global Charcoal Database (GCD v4.0.6) compiled by the Global Palaeofire Working Group (GPWG1) or from a previous published synthesis (Molinari et al., 2020). Metadata including detailed information about each record are presented in Table 1.
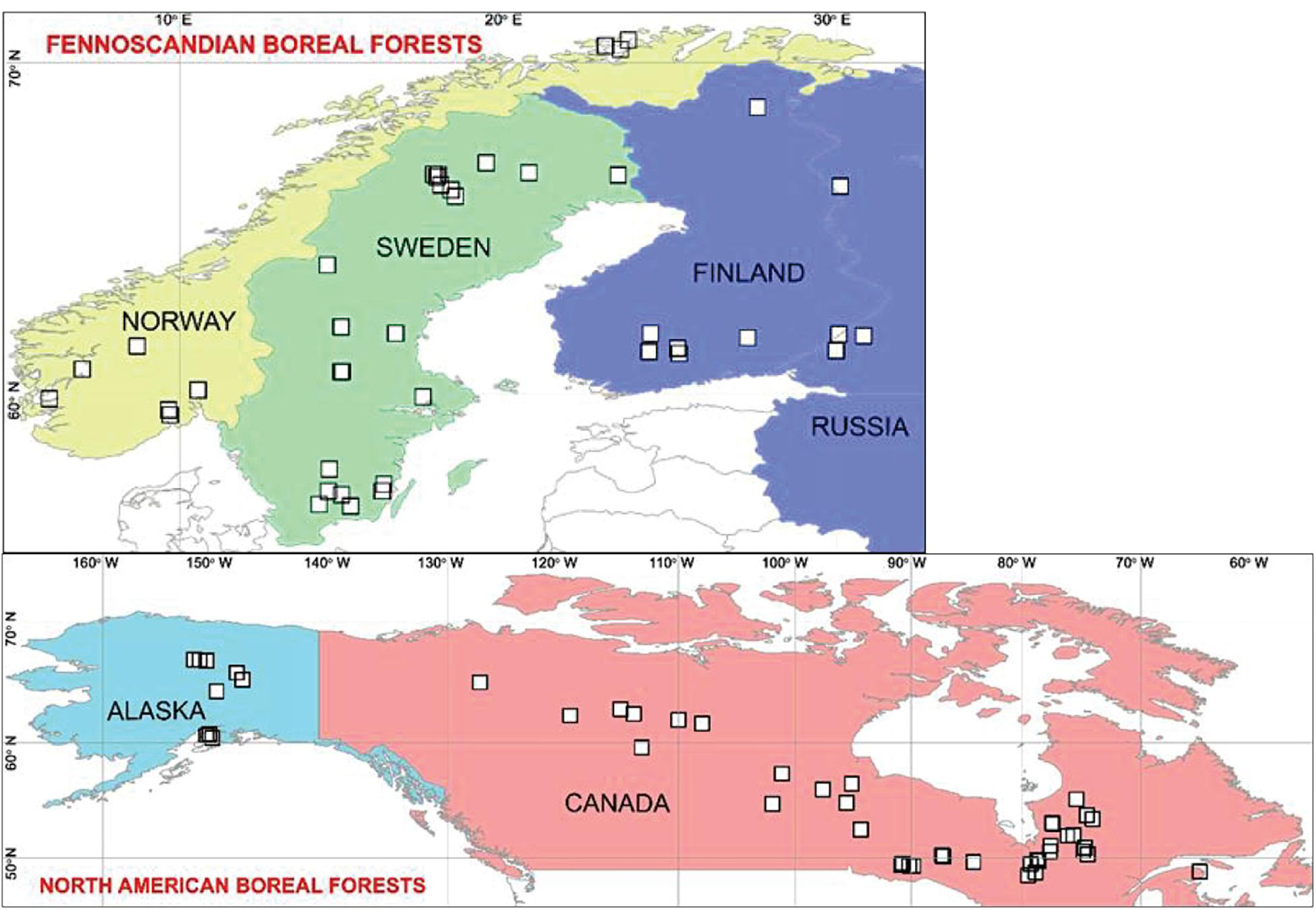
Figure 1. Location map of the selected charcoal records divided into the different continental and regional sub-areas identified for the present study. For the same sites the probability of a grid-cell to burn per year (Fp) and the total carbon flux emitted to the atmosphere per year (Ce) were simulated by LPJ-GUESS- SIMFIRE-BLAZE.
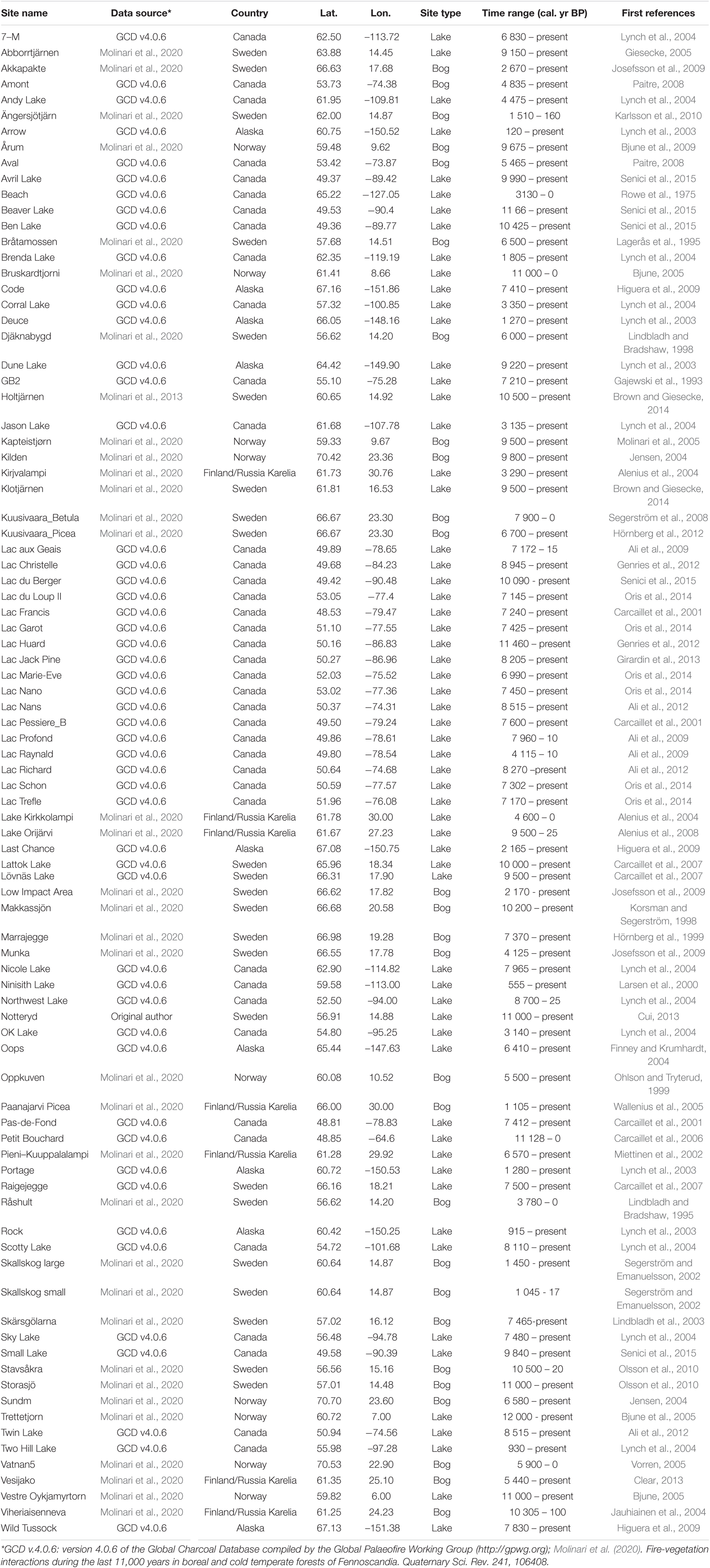
Table 1. Name, source, location, depositional context, time range, and first reference for individual records of Holocene biomass burning in boreal forests.
To reconstruct regional and continental composite curves of changes in fire activity over the last century, all charcoal data were first converted to charcoal accumulation rates (CHARs, i.e., the number, area or weight of particles cm-2 year-1). A standardization procedure was then applied in order to allow comparison within and between charcoal records obtained from various depositional environments and quantified with different laboratory techniques (Power et al., 2008). This technique includes: (1) a min-max rescaling of CHAR values, (2) a Box-Cox transformation to homogenize the within-record variance, and (3) a Z-score conversion using a base period between 1900 and 2000 CE. Palaeofire analyses generally omitted this time interval from the base period because of the large impacts of anthropogenic activities on ignitions and suppressions during the 19th and the 20th century (Power et al., 2008), which, instead, is what we want to capture in this study. Transformed charcoal data were then bootstrap re-sampled 1000 times with a moving window procedure using non-overlapping bins of 1-yr. Re-sampled time series were then smoothed using a LOcally WEighted regression Scatterplot Smoother (LOWESS) with a window half-width of 10 years. Charcoal composite series mean and 97.5% confidence intervals (hereafter CI) were calculated by averaging the smoothed and bootstrapped data series (Daniau et al., 2012). For each sub-area taken into consideration, composite curves were finally produced with the method implemented in the R (R Core Team, 2016) package “paleofire” version 1.2.4 (Blarquez et al., 2014).
LPJ-GUESS-SIMFIRE-BLAZE Fire Model Description
We used the LPJ-GUESS-SIMFIRE-BLAZE model to perform a transient simulation over the last century of vegetation and fire dynamics as influenced by changes in climate, land use and population density. Here, the empirical fire model SIMFIRE (SIMple FIREmodel, Knorr et al., 2014) produces an annual burned area for each grid-cell (0.5o x 0.5o) containing a charcoal record based on fire weather, fuel continuity, fire-biome and human population density. Using a monthly fire climatology, daily values of burned area (as fraction of grid-cell) are computed and used as a fire probability for the combustion model BLAZE (Blaze-Induced Land–Atmosphere Flux Estimator, Rabin et al., 2017). In LPJ-GUESS (Smith et al., 2001, 2014) natural vegetation is represented by a multitude of patches with different “time since last disturbance” to account for cohorts of different age and height. Each patch is tested against the aforementioned fire probability on a daily basis. In case of a successful ignition, the whole patch is affected by fire and BLAZE simulates fire driven fluxes within the biosphere (live to litter) and from the biosphere to the atmosphere. The combustion model computes survival-probabilities for stands of trees based on vegetation properties (like height or diameter-at-breast-height, DBH) and potential fire-line intensity [based on a revised set of FullCAM parameters following Surawski et al. (2012)] derived from available fuels and local meteorology. In the event of a fire, the stochastic survival of each tree is tested individually. Total carbon fluxes (the total amount of C emitted by fire to the atmosphere) are then computed from the amount of tree-deaths and as fractions of above-ground litter combusted based on the fire-line intensity. SIMFIRE-BLAZE incorporates the HYDE 3.1 population density dataset (Klein Goldewijk et al., 2011) within LPJ-GUESS.
Patterns and annual cycles of burned area and carbon emissions within the range of satellite-based observations have been previously demonstrated by Kloster et al. (2004) to be reasonably simulated by the Arora and Boer (2005) fire model implemented in the Community Land Model (CLM-CM).
Comparison Between Model Outputs and Charcoal Data
For each grid-cell containing a charcoal record, the probability to burn per year (fraction year–1, hereafter fire probability Fp) and the total carbon flux emitted to the atmosphere per year (kg C m–2 year–1, hereafter carbon emitted Ce) simulated by LPJ-GUESS-SIMFIRE-BLAZE during the period 1900–2000 CE were treated analogously to sedimentary charcoal data in order to estimate regional and continental fire activity. To reduce the high year-to-year variability, a 10-yr running mean filter was applied before the Z-scores were derived. This same data processing minimized inconsistences in the statistical analysis and maximize comparability between the different datasets. In this way, discrepancies in the data-model comparison can only be explained by model deficiencies.
In order to evaluate the agreement between reconstructed and modeled fire dynamics in boreal forests since the beginning of the 20th century, we calculated the Spearman’s rank correlation coefficient (Spearman, 1904) between Z-scores of transformed charcoal influxes (hereafter tCHAR Z-scores) and Z-scores of transformed model output of fire probability (hereafter tFp Z-scores) or carbon emitted (hereafter tCe Z-scores) for the boreal forests as well as for each region and continent. The Spearman correlation measures the strength of the monotonic relation between two variables, is robust to the presence of outliers, especially when there is sufficient sample size, and is applicable for any continuous or ordinal scale (Sheskin, 2007). The statistical analyses were run for the whole time period taken into consideration (1900–2000 CE), as well as for the two different sub-periods (1900–1950 CE and 1950–2000 CE).
Results
Observed Boreal Forest Fire Dynamics
Reconstructions of fire activity across the boreal forests based on sedimentary charcoal records during the period 1900–2000 CE show different trajectories (Figure 2 and Table 2).
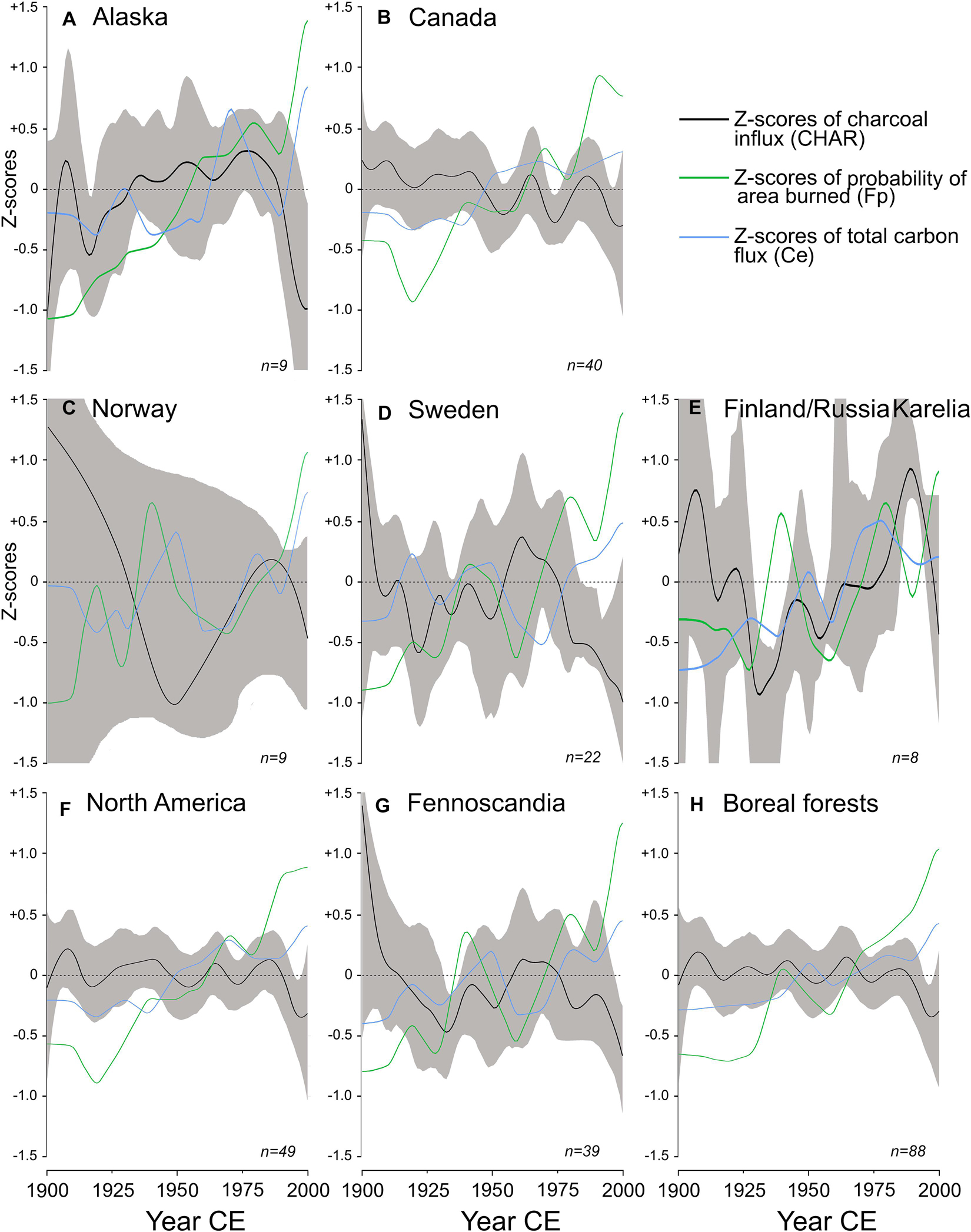
Figure 2. Reconstructed charcoal-based and modeled (probability of annual area burned and total carbon flux emitted per year) boreal forests fire dynamics for the 20th century for (A) Alaska, (B) Canada, (C) Norway, (D) Sweden, (E) Finland/Russia Karelia, (F) North America, (G) Fennoscandia, and (H) boreal forests, with the number of site contributing to the analysis for each sub-area. The composite curves have been smoothed using a 10-year window half width. Bootstrap 97.5% CI from resampling by charcoal records are shown as gray shadings.
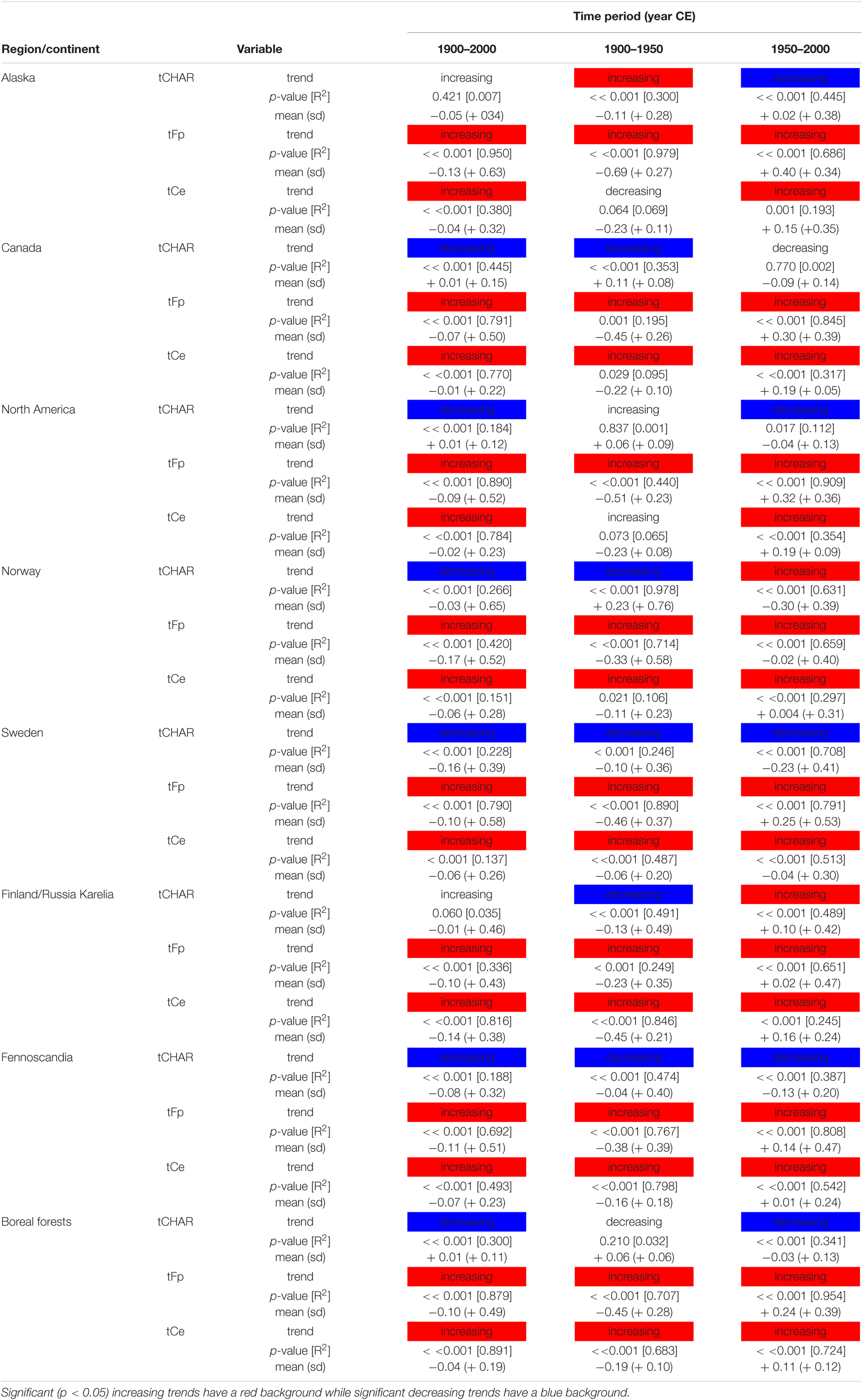
Table 2. Trends of fire activity in boreal forests for the 20th century expressed by mean (standard deviation, sd) Z-score of transformed charcoal influx (tCHAR), mean (standard deviation, sd) Z-score of transformed fire probability (tFp) and mean (standard deviation, sd) Z-score of carbon emitted (tCe).
In Alaska (Figure 2A), despite a downturn between 1910 and 1920 CE, a general increasing trend is recorded until 1980 CE. Then tCHAR Z-scores progressively decrease, with only a slight increase between 1998 and 2000 CE. In Canada (Figure 2B), charcoal values show a decrease during the period 1900–1955 CE, while two peaks (followed by downturns) are registered around 1965 and 1985 CE. An increase is recorded between 1998 and 2000 CE. The charcoal composite curves for North America (Figure 2F) and for the whole boreal forests (Figure 2H) record values around the long-term mean between 1900 and 1990 CE. A decreasing trend characterize the following years, with a little increase between 1998 and 2000 CE.
Despite large uncertainties, tCHAR Z-scores for Norway (Figure 2C) show a decreasing trend during the first half of the 20th century, increasing values between 1950 and 1985 CE and a decrease at the end of the century. Sweden (Figure 2D) and Fennoscandia (Figure 2G) document decreasing charcoal trends between 1900 and 1930 CE. Increasing values are recorded during the period 1930–1960 CE, followed by a decrease with a minimum at 2000 CE. After increasing values at the beginning of the century, Finland/Russia Karelia (Figure 2E) registers a general decreasing trend between 1905 and ca 1930 CE. Increasing charcoal values are recorded until 1990 CE, followed by a decrease.
Simulated Boreal Forests Fire Dynamics
Changes in tFp and in tCe Z-scores simulated by LPJ-GUESS-SIMFIRE-BLAZE during the period 1900–2000 CE show a general progressive rise toward the end of the 20th century for all the areas taken into consideration (Figure 2 and Table 2). Large oscillations occur as a direct consequence of climatic drivers.
Comparison Between Observed and Simulated Boreal Forests Fire Dynamics
Observed and modeled boreal forest fire activity since the beginning of the 20th century are compared by investigating trends in tCHAR, tFp and tCe Z-scores transformed time series (Table 2). For the whole time period taken into consideration (1900–2000 CE) as well as for the two different sub-periods (1900–1950 CE, 1950–2000 CE), the Spearman rank correlation coefficient (Spearman, 1904) is also calculated.
In Alaska, based on 9 sites, an increasing trend in tCHAR (not-significant), tFp and tCe Z-scores is recorded when the whole 20th century is considered and, only in tCHAR and tFp, during the time interval 1900-1950 CE. These results are confirmed by the statistical analyses, indicating positive correlations (only significant among tCHAR and tFp, Table 3) for these time periods. Instead, during the second half of the 20th century the correlation between observations (decreasing) and simulations (increasing) is negative (Tables 2, 3).
For what concerns North America, on a total of 49 sites, an agreement between the different trends (all increasing but significant only for tFp) is recorded during the time interval 1900–1950 CE (Table 2). The statistical analyses confirm these results, showing a positive correlation (only significant among tCHAR and tFp) for charcoal data and model outputs during this period (Table 3). On the contrary, observations decrease and simulations increase between 1900 and 2000 CE and for the second half of the 20th century (Table 2), with a negative correlation between the variables (Table 3).
In Canada (40 sites), Sweden (22 sites), Fennoscandia (39 sites) and for the whole boreal forests (88 sites) observations (increasing, but non-significantly for Canada between 1950 and 2000 CE and for the whole boreal forests between 1900 and 1950 CE) and simulations (decreasing) follow opposite trends during the 20th century as well as for the two distinct periods (Table 2). Despite that, in Canada a positive non-significant correlation exists among tCHAR and tFp and tCe during the first half of the 20th century (Table 3), while in the whole boreal forests a positive non-significant correlation is registered between tCHAR and tFp for the period 1900–1950 CE.
In Norway, based on 9 sites, tCHAR, tFp, and tCe record an increasing trend during the second half of the 20th century. These results are confirmed by the statistical results, showing a positive relationship between the variables (only significant between tCHAR and tFp, Table 3). Instead, during the two different time intervals the correlations among observations (decreasing) and simulations (increasing) are negative (Tables 2, 3).
For Finland/Russia Karelia, based on 8 sites, observed and modeled data show an analogous (increasing, non-significantly for tCHAR between 1900 and 2000 CE) trend when the whole 20th century and during the second half of the 20th century (Table 2). This is in agreement with the Spearman rank correlation analyses indicating positive correlations (non-significant for tCHAR-tCe between 1900 and 2000 CE) among the variables during these periods (Table 3).
Discussion
Uncertainties in Observed Fire Dynamics
Granted all simplifications, generalizations and uncertainties in LPJ-GUESS-SIMFIRE-BLAZE structure, processes and input data (Hamilton et al., 2018), the use of sedimentary charcoal data to evaluate fire model simulations requires a careful consideration of the uncertainties connected to palaeofire data. In fact, even if time series of sedimentary charcoal abundance have become a widely used tool for reconstructing long-term trends in biomass burning (Whitlock and Bartlein, 2004), interpretation rests on understanding the source area of charcoal particles (i.e., the area burned and the distance to the site) and the ability to accurately infer other properties of the fire regime, such as frequency, intensity, and fuel type (Lynch et al., 2004). Constraining the charcoal source area is not a trivial task because charcoal abundance is influenced by vegetation, fire climate, and fire weather as well as site-specific processes related to charcoal deposition and burial (Clark, 1998). Despite advances in model-data integration (e.g. Higuera et al., 2007, 2011; Kelly et al., 2013) have helped validate charcoal-based fire reconstructions and identify uncertainties associated with natural (e.g., charcoal production, transport, and deposition) and analytical processes, reconstructing the area burned thus remains a challenge (Hennebelle et al., 2020). Furthermore, variability in the charcoal record comes from a variety of sources, including temporal variations in primary charcoal production, transport, and deposition; bioturbation and addition of secondary (remobilized) charcoal; differences in sampling, chemical treatment, and charcoal identification and quantification that can obscure the relationship between charcoal accumulation rates and fire (Iglesias et al., 2015). More specifically for our study, the uppermost sediments of the charcoal records might be less tightly constrained due to: (1) the difficulty to fully recover sediment/water interface during coring, (2) the high water content which tend to lower charcoal concentrations, and (3) the potential impact of human activities on catchment processes and sedimentation (Gale, 2009). Despite the recent development of Bayesian age-models (Blaauw et al., 2020), uncertainties in charcoal records can also be associated with a poor chronological control for recent times (where most of the dating are based on radiocarbon results) and to the different processes of charcoal production, transportation and deposition in the catchments, partially solved by field experiments that enable a better understanding of charcoal accumulation through time (Higuera et al., 2007; Hennebelle et al., 2020). Although charcoal is a proxy of carbon released by biomass burning, sedimentary-based reconstructions are currently insufficient for providing quantitative estimates of carbon released by fires because the links between stratigraphic charcoal and gaseous carbon fluxes are difficult to assess (Carcaillet et al., 2002). In boreal forests carbon release comes primarily from the ground layer (woody debris, humus, peat), which is more susceptible to becoming completely consumed than is the aboveground biomass (Kasischke et al., 2000). Finally, our analyses are based on charcoal records primarily collected from Canada (40 sites) and Sweden (22 sites), and this may have partly biased the results because some trends might be less robust than others.
Drivers of Observed and Simulated Fire Dynamics
The decline in fire activity over the 20th century shown by our charcoal-based reconstructions for most of the boreal regions taken into consideration reiterate the results of previous palaeoecological studies at the global scale (Marlon et al., 2009a), in Australia (Mooney et al., 2011), in Europe (Molinari et al., 2013) and in the Americas (Power et al., 2013). A number of causes have been suggested for this downturn, including: (1) land-use change, resulting in landscape fragmentation and a generally less flammable fuel (Pyne and World, 1995), (2) active fire suppression management policies (Granström and Niklasson, 2008; Marlon et al., 2009a), and (3) an economic and cultural transition from traditional fire practices (such as slash-and-burn cultivation) to modern agriculture and forestry activities, especially in Fennoscandia (Wallenius, 2011). On the contrary, the increase in fire probability and, thus, in carbon emitted simulated by LPJ-GUESS-SIMFIRE-BLAZE are the result of the factors which determine the probability of a grid-cell to burn per year, such as: (1) the shift of many grid-cells taken into consideration from tundra to boreal fire-biome with a different flammability due to a change in CO2-fertilization and climate (rise in both total annual precipitation and temperature), leading to an increase in the maximum annual fAPAR (fraction of Absorbed Photosynthetically Active Radiation, a proxy for the amount of vegetation growing in a grid-cell), and (2) a rise in the maximum annual Nesterov index, which is a proxy for fire-weather conditions and the curing of fuels. Both these factors lead to higher amounts of fuels and more fire-promoting weather conditions with a consequent increase of fire occurrence. So, when looking only into climatic drivers, an increase in boreal forest fires is expected under present and future climate change (Flannigan et al., 2005, 2009a; Ali et al., 2009). This is probably the reason of the agreement between trends in sedimentary charcoal data and model outputs during the 20th century in Alaska, a region where fire activity was mainly driven by warm temperatures and vegetation flammability (Hoecker and Higuera, 2019). The good correlation between observations and simulations detected in Finland/Russia Karelia is instead probably due to reworking of charcoal particles in the uppermost levels of the sequences due to ditching and clearcutting in the forests (Pitkänen and Huttunen, 1999).
Reasons for Data-Model Divergence
The influence of anthropogenic factors (e.g., fire suppression, forest management and ignition control) on boreal fires dynamics is likely to be the main cause of the data-model disagreement. In Canada, the decrease in charcoal-based reconstructions during the first half of the 20th century is mainly the result of forest management (Bergeron et al., 2001). For the same period, a gradual abandonment of slash-and-burn cultivations in Norway and Finland/Russia Karelia (Lehtonen and Kolström, 2000; Rolstad et al., 2017), and of an early use of fire management practices in Sweden (Zackrisson, 1977; Granström and Niklasson, 2008) resulted in a similar decrease in these regions. The good correspondence between fire dynamics observations and simulations in Alaska and in North America is instead connected to a rise in wildfire extent due to an earlier impact of climate warming in these northern latitude areas (Chapin et al., 2008; Kelly et al., 2016). Regarding the second half of the 20th century, the negative correlation between sedimentary charcoal trends and model projections recorded in Alaska and Canada is primarily related to the high efficiency of active fire suppression policies thanks to increased landscape fragmentation (Weir et al., 2000; Kasischke et al., 2010). For Canada this is in contrast with the data included in the Large Fire Database (LFDB, which includes information on fires larger than 200 ha in area) indicating, as suggested by LPJ-GUESS-SIMFIRE-BLAZE outputs, a general increase in area burned during the period 1959–1997 CE (Stocks et al., 2003). Discrepancies between charcoal-based reconstructions and simulations are also registered in Sweden, where the decline in forest fires is linked to both active fire suppression (Niklasson and Granstrom, 2000) and climate variations [particularly water availability as suggested by Drobyshev et al. (2012)]. Norway and Finland/Russia Karelia are the only sub-areas where both observations and model projections register an increase probably connected to a comeback of prescribed burning practices for biodiversity goals (Bleken et al., 1997; Lindberg et al., 2000) or to sediment remixing at the top of the sequences due to forest management (Pitkänen and Huttunen, 1999).
Implications for Future Data-Model Improvements
As in most fire models (Hantson et al., 2016), the only anthropogenic driver mediating fire probability (and thus carbon emitted) in LPJ-GUESS-SIMFIRE-BLAZE is human population density which, in the high latitudes investigated by the present study, is at very low levels and its general mild increase toward the end of the century is outcompeted by the climatic drivers which promote a rise in fire probability. However, even under these low population densities, people can have large-scaled impacts on fire occurrence as indicated in the previous subsection. Detailed representation of forest management (and the associated stand structure) and landscape fragmentation (and their absence in the past) should be taken into account in high-latitudes fire-simulations for a better representation of the human impact on fire regime. Furthermore, a more detailed simulation of fires in the boreal vegetation, especially peatland and shrubs, with a special focus on soils, is needed to more accurately represent fires in these regions. The same goes for the representation of fire and forest management (e.g., fire prevention, prescription). While, in fact, the impact of such factors on the global scale is probably small, it is clear that in practise these can override the predominant climate signal currently simulated by LPJ-GUESS-SIMFIRE-BLAZE on a regional scale.
This study further indicates the need for additional sampling and high-resolution analyses of charcoal records from areas inadequately represented by the present dataset and covering the more recent years (i.e., the beginning of the 21st century). Due to the low number of charcoal records available for the last 20 years, it was in fact impossible to perform the statistical analyses beyond the end of the 20th century. Despite that, the rise in both charcoal-based reconstructions and model outputs at the end of the study period in Alaska, Canada, North America and for the whole boreal forests (Figures 2A,B,F,H) make us assuming increasing trends until the present time as already suggested by Turetsky et al. (2011), Sanford et al. (2015), and Tymstra et al. (2020). On the contrary, the decline in charcoal-based reconstructions register in Fennoscandia (Figures 2C–E,G), probably connected to the effectiveness of fire suppression policies in the modern forests to support a growing timber industry that makes large fire events rare in these regions (Pinto et al., 2020), is in contrast with an increasing fire risk in these areas raised by the outbreak of exceptionally large fires in Sweden in recent years, and especially during summers 2014 and 2018 (San-Miguel-Ayanz et al., 2019). A better understanding of the spatio-temporal variability of fire activity is thus needed in order to maximize the possibility of data-model comparison and better evaluate the ability of ecosystem models to more realistically simulate future biomass burning.
Conclusion
Our results show how palaeofire proxies can be used to evaluate fire projections made by global fire-vegetation models. A general disagreement is highlighted for the boreal region, where LPJ-GUESS-SIMFIRE-BLAZE simulates an increasing and sedimentary charcoal records a decreasing in fire activity over the 20th century. The present study demonstrates that socio-economical drivers of human behavior and forest management can override broad climate patterns, at least in these areas. Therefore, past and future projections in fire occurrence are still very uncertain and should take into account the specifics of the region (e.g., peatlands) and its particular vegetation. Additional improvements about these processes that are not yet completely understood are essential to better understand the complex interactions between climate, meteorology, vegetation, humans and fire. This enhanced understanding necessitates a closer collaboration between palaeoecologists and modelers.
Data Availability Statement
Sedimentary charcoal data used in this analysis are either stored in the Global Charcoal Database (http://gpwg.org) and accessible through the paleofire package (Blarquez et al., 2014) for R (R Core Team, 2016) or are available upon request. Model output is available upon request.
Author Contributions
CM conceived and designed the study, compiled and analyzed the charcoal data, made the figures, and drafted the manuscript. LN and SH performed the model simulation, helped interpreting the results, and contributed drafting the manuscript. All authors read and approved the final manuscript.
Funding
Financial support for CM and LN was provided by the Lund University Strategic Research Area (LU SRA) on Modeling the Regional and Global Earth system (MERGE). Simulations performed by LN were enabled by resources provided by the Swedish National Infrastructure for Computing (SNIC) at Lund University (LUNARC) partially funded by the Swedish Research Council through grant agreement no. 2018-05973.
Conflict of Interest
The authors declare that the research was conducted in the absence of any commercial or financial relationships that could be construed as a potential conflict of interest.
Publisher’s Note
All claims expressed in this article are solely those of the authors and do not necessarily represent those of their affiliated organizations, or those of the publisher, the editors and the reviewers. Any product that may be evaluated in this article, or claim that may be made by its manufacturer, is not guaranteed or endorsed by the publisher.
Acknowledgments
We thank our colleagues who have made this analysis possible through their contribution to the Global Charcoal Database.
Footnotes
References
Alenius, T., Grönlund, E., Simola, H., and Saksa, A. (2004). Land–use history of Riekkalansaari Island in the northern archipelago of Lake Ladoga, Karelian Republic, Russia. Veg. Hist. Archaeobot. 13, 23–31. doi: 10.1007/s00334-003-0030-7
Alenius, T., Mikkola, E., and Ojala, A. K. O. (2008). History of agriculture in Mikkeli Orijärvi, eastern Finland as reflected by palynological and archaeological data. Veg. Hist. Archaeobot. 17, 171–183. doi: 10.1007/s00334-007-0099-5
Ali, A. A., Blarquez, O., Girardin, M. P., Hély, C., Tinquaut, F., El Guellab, A., et al. (2012). Control of the multimillennial wildfire size in boreal North America by spring climatic conditions. Proc. Natl. Acad. Sci. U.S.A. 109, 20966–20970. doi: 10.1073/pnas.1203467109
Ali, A. A., Carcaillet, C., and Bergeron, Y. (2009). Long−term fire frequency variability in the eastern Canadian boreal forest: the influences of climate vs. local factors. Glob. Change Biol. 15, 1230–1241. doi: 10.1111/j.1365-2486.2009.01842.x
Andela, N., Morton, D. C., Giglio, L., Chen, Y., Van Der Werf, G. R., Kasibhatla, P. S., et al. (2017). A human-driven decline in global burned area. Science 356, 1356–1362. doi: 10.1126/science.aal4108
Arora, V. K. and Boer, G. J. (2005). Fire as an interactive component of dynamic vegetation models. J. Geophys. Res. 110:G02008. doi: 10.1029/2005JG000042
Bergeron, Y., Gauthier, S., Kafka, V., Lefort, P., and Lesieur, D. (2001). Natural fire frequency for the eastern Canadian boreal forest: consequences for sustainable forestry. Can. J. For. Res. 31, 384–391. doi: 10.1139/x00-178
Bjune, A. E. (2005). Holocene vegetation history and tree–line changes on a north–south transect crossing major climate gradients in southern Norway—evidence from pollen and plant macrofossils in lake sediments. Rev. Palaeobot. Palyno. 133, 249–275. doi: 10.1016/j.revpalbo.2004.10.005
Bjune, A. E., Bakke, J., Nesje, A., and Birks, H. J. B. (2005). Holocene mean july temperature and winter precipitation in western Norway inferred from palynological and glaciological lake-sediment proxies. Holocene 15, 177–189. doi: 10.1191/0959683605hl798rp
Bjune, A. E., Ohlson, M., Birks, H. J. B., and Bradshaw, R. H. W. (2009). The development and local stand–scale dynamics of a Picea abies forest in south eastern Norway. Holocene 19, 1073–1082. doi: 10.1177/0959683609341004
Blaauw, M., Christen, J. A., and Aquino-López, M. A. (2020). A review of statistics in palaeoenvironmental research. JABES 25, 17–31. doi: 10.1007/s13253-019-00374-2
Blarquez, O., Vannière, B., Marlon, J. R., Daniau, A. L., Power, M. J., Brewer, S., et al. (2014). Paleofire: an R package to analyse sedimentary charcoal records from the global charcoal database to reconstruct past biomass burning. Comput. Geosci. 72, 255–261. doi: 10.1016/j.cageo.2014.07.020
Bleken, E., Mysterud, I., and Mysterud, I. (1997). Forest Fire and Environmental Management: A Technical Report on Forest Fire as an Ecological Factor. Contracted Report. Directorate for Fire & Explosion Prevention and Department of Biology. Norway: University of Oslo.
Bowman, D. M., Balch, J. K., Artaxo, P., Bond, W. J., Carlson, J. M., Cochrane, M. A., et al. (2009). Fire in the earth system. Science 324, 481–484. doi: 10.1126/science.1163886
Bowman, D. M., Balch, J., Artaxo, P., Bond, W. J., Cochrane, M. A., D’Antonio, C. M., et al. (2011). The human dimension of fire regimes on earth. J. Biogeogr. 38, 2223–2236. doi: 10.1111/j.1365-2699.2011.02595.x
Brown, K. J., and Giesecke, T. (2014). Holocene fire disturbance in the boreal forest of central Sweden. Boreas 43, 639–651. doi: 10.1111/bor.12056
Brücher, T., Brovkin, V., Kloster, S., Marlon, J. R., and Power, M. J. (2014). Comparing modelled fire dynamics with charcoal records for the Holocene. Clim. Past 10, 811–824. doi: 10.5194/cp-10-811-2014
Carcaillet, C., Almquist, H., Asnong, H., Bradshaw, R. H., Carrión, J. S., Gaillard, M. J., et al. (2002). Holocene biomass burning and global dynamics of the carbon cycle. Chemosphere 49, 845–863. doi: 10.1016/s0045-6535(02)00385-5
Carcaillet, C., Bergeron, Y., Richard, P. J. H., Fréchette, B., Gauthier, S., and Prairie, Y. T. (2001). Change of fire frequency in the eastern Canadian boreal forests during the holocene: does vegetation composition or climate trigger the fire regime? J. Ecol. 89, 930–946. doi: 10.1111/j.1365-2745.2001.00614.x
Carcaillet, C., Bergman, I., Delorme, S., Hörnberg, G., and Zackrisson, O. (2007). Long–term fire frequency not linked to prehistoric occupations in a northern Swedish boreal forest. Ecology 88, 465–477. doi: 10.1890/0012-9658(2007)88[465:lffnlt]2.0.co;2
Carcaillet, C., Richard, P., Asnong, H., Capece, L., and Bergeron, Y. (2006). Fire and soil erosion history in East Canadian boreal and temperate forests. Quat. Sci. Rev. 25, 1489–1500. doi: 10.1016/j.quascirev.2006.01.004
Chapin, F. S., Trainor, S. F., Huntington, O., Lovecraft, A. L., Zavaleta, E., Natcher, D. C., et al. (2008). Increasing wildfire in Alaska’s boreal forest: pathways to potential solutions of a wicked problem. BioScience 58, 531–540. doi: 10.1641/B580609
Cheung, A. H., Vachula, R. S., Clifton, E., Sandwick, S., and Russell, J. M. (2021). Humans dominated biomass burning variations in Equatorial Asia over the past 200 years: evidence from a lake sediment charcoal record. Quat. Sci. Rev. 253:106778. doi: 10.1016/j.quascirev.2020.106778
Clark, J.S. (1988). Particle motion and the theory of charcoal analysis: source area, transport, deposition, and sampling. Quaternary Res. 30, 67–80. doi: 10.1016/0033-5894(88)90088-9
Clear, J. (2013). Holocene Fire and Vegetation Dynamics in the Northern European Forests. Doctorate Thesis. England: University of Liverpool.
Conedera, M., Tinner, W., Neff, C., Meurer, M., Dickens, A. F., and Krebs, P. (2009). Reconstructing past fire regimes: methods, applications, and relevance to fire management and conservation. Quat. Sci. Rev. 28, 555–576. doi: 10.1016/j.quascirev.2008.11.005
Cui, Q.-Y. (2013). Fire History in the Hemiboreal and Southern Boreal Zones of Southern Sweden During 11000 Years? Relationships With Past Vegetation Composition and Human Activities and Implications for Biodiversity Issues. PhD dissertation. Växjö: Linnaeus University.
Daniau, A.-L., Bartlein, P. J., Harrison, S. P., Prentice, I. C., Brewer, S., Friedlingstein, P., et al. (2012). Predictability of biomass burning in response to climate changes. Glob. Biogeochem. Cycles 26:GB4007. doi: 10.1029/2011GB004249
Dentener, F., Kinne, S., Bond, T., Boucher, O., Cofala, J., Generoso, S., et al. (2006). Emissions of primary aerosol and precursor gases in the years 2000 and 1750 prescribed data-sets for Aerocom. Atmos. Chem. Phys. 6, 4321–4344. doi: 10.5194/acp-6-4321-2006
Drobyshev, I., Niklasson, M., and Linderholm, H. W. (2012). Forest fire activity in Sweden: climatic controls and geographical patterns in 20th century. Agric. For. Meteorol. 154–155, 174–186. doi: 10.1016/j.agrformet.2011.11.002
Finney, B. P., and Krumhardt, A. P. (2004). Sediment, Pollen, and Charcoal Analyses of an Unnamed Lake in the White Mountains Recreational Area, Interior Alaska of Land Management Northern Field Office. Fairbanks, AK: Department of Forest Science.
Flannigan, M. D., Krawchuk, M., de Groot, W., Wotton, B., and Gowman, L. (2009a). Implications of changing climate for global wildland fire. Int. J. Wildland Fire 18, 483–507. doi: 10.1071/WF08187
Flannigan, M. D., Stocks, B., Turetsky, M., and Wotton, M. (2009b). Impacts of climate change on fire activity and fire management in the circumboreal forest. Glob. Change Biol. 15, 549–560. doi: 10.1111/j.1365-2486.2008.01660.x
Flannigan, M. D., Logan, K. A., Amiro, B. D., Skinner, W. R., and Stocks, B. J. (2005). Future area burned in Canada. Clim. Change 72, 1–16. doi: 10.1007/s10584-005-5935-y
Forkel, M., Dorigo, W., Lasslop, G., Chuvieco, E., Hantson, S., Heil, A., et al. (2019). Recent global and regional trends in burned area and their compensating environmental controls. Environ. Res. Commun. 1:051005. doi: 10.1088/2515-7620/ab25d2
Gajewski, K., Payette, S., and Ritchie, J. C. (1993). Holocene vegetation history at the boreal–forest —- shrub–tundra transition in north–western Québec. J. Ecol. 81, 433–443. doi: 10.2307/2261522
Gale, S. J. (2009). Event chronostratigraphy: a high-resolution tool for dating the recent past. Quat. Geochronol. 4, 391–399. doi: 10.1016/j.quageo.2008.12.003
Genries, A., Finsinger, W., Asnong, H., Bergeron, Y., Carcaillet, C., Garneau, M., et al. (2012). Local versus regional processes: can soil characteristics overcome climate and fire regimes by modifying vegetation trajectories? J. Quat. Sci. 27, 745–756. doi: 10.1002/jqs.2560
Giesecke, T. (2005). Holocene forest development in the central Scandes Mountains, Sweden. Veg. Hist. Archaeobot. 13, 133–147. doi: 10.1007/s00334-005-0070-2
Giglio, L., Randerson, J. T., van derWerf, G. R., Kasibhatla, P. S., Collatz, G. J., Morton, D. C., et al. (2010). Assessing variability and long-term trends in burned area by merging multiple satellite fire products. Biogeosciences 7, 1171–1186. doi: 10.5194/bg-7-1171-2010
Girardin, M. P., Ali, A. A., Carcaillet, C., Blarquez, O., Hély, C., Terrier, A., et al. (2013). Vegetation limits the impact of a warm climate on boreal wildfires. New Phytol. 199, 1001–1011. doi: 10.1111/nph.12322
Goldammer, J. G. (2013). Vegetation Fires and Global Change. Challenges for Concerted International Action. A White Paper Directed to the United Nations and International Organizations. Remagen-Oberwinter: Kessel Publishing House.
Granström, A., and Niklasson, M. (2008). Potentials and limitations for human control over historic fire regimes in the boreal forest. Philos. Trans. R. Soc. Lond. B Biol. Sci. 363, 2351–2356. doi: 10.1098/rstb.2007.2205
Hamilton, D. S., Carslaw, K. S., Hantson, S., Kaplan, J. O., Scott, C. E., Nieradzik, L. P., et al. (2018). Reassessment of pre-industrial fire emissions strongly affects anthropogenic aerosol forcing. Nat. Commun. 9:3182. doi: 10.1038/s41467-018-05592-9
Hantson, S., Arneth, A., Harrison, S. P., Kelley, D. I., Prentice, I. C., Rabin, S. S., et al. (2016). The status and challenge of global fire modelling. Biogeosciences 13, 3359–3375. doi: 10.5194/bg-13-3359-2016
Hantson, S., Kelley, D. I., Arneth, A., Harrison, S. P., Archibald, S., Bachelet, D., et al. (2020). Quantitative assessment of fire and vegetation properties in simulations with fire-enabled vegetation models from the fire model intercomparison project. Geosci. Model Dev. 13, 3299–3318. doi: 10.5194/gmd-13-3299-2020
Hennebelle, A., Aleman, J. C., Ali, A. A., Bergeron, I., Carcaillet, C., Grondin, P., et al. (2020). The reconstruction of burned area and fire severity using charcoal from boreal lake sediments. Holocene 30, 1400–1409. doi: 10.1177/0959683620932979
Heyerdahl, E. K., Morgan, P., and Riser, J. P. I. I. (2008). Multi-season climate synchronized historical fires in dry forests (1650-1900), northern Rockies, U.S.A. Ecology 89, 705–716. doi: 10.1890/06-2047.1
Higuera, P. E., Brubaker, L. B., Anderson, P. M., Hu, F. S., and Brown, T. A. (2009). Vegetation mediated the impacts of postglacial climate change on fire regimes in the south–central Brooks Range, Alaska. Ecol. Monogr. 79, 201–219. doi: 10.1890/07-2019.1
Higuera, P. E., Gavin, D. G., Bartlein, P. J., and Hallett, D. J. (2011). Peak detection in sediment–charcoal records: impacts of alternative data analysis methods on fire-history interpretations. Int. J. Wildland Fire 19, 996–1014. doi: 10.1071/WF09134
Higuera, P. E., Peters, M. E., Brubaker, L. B., and Gavin, D. G. (2007). Understanding the origin and analysis of sediment-charcoal records with a simulation model. Quat. Sci. Rev. 26, 1790–1809. doi: 10.1016/j.quascirev.2007.03.010
Hoecker, T. J., and Higuera, P. E. (2019). Forest succession and climate variability interacted to control fire activity over the last four centuries in an Alaskan boreal landscape. Landscape Ecol. 34, 227–241. doi: 10.1007/s10980-018-00766-8
Hörnberg, G., Östlund, L., Zackrisson, O., and Bergman, I. (1999). The genesis of two Picea–Cladina forests in northern Sweden. J. Ecol. 87, 800–814.
Hörnberg, G., Staland, H., Nordström, E.-M., Korsman, T., and Segerström, U. (2012). Fire as an important factor for the genesis of boreal Picea abies swamp forests in fennoscandia. Holocene 22, 203–214. doi: 10.1177/0959683611414936
Iglesias, V., Yospin, G., and Whitlock, C. (2015). Reconstruction of fire regimes through integrated paleoecological proxy data and ecological modelling. Front. Plant Sci. 5:785. doi: 10.3389/fpls.2014.00785
IPCC. (2014). “Climate change 2014: synthesis report,” in Contribution of Working Groups I, II and III to the Fifth Assessment Report of the Intergovernmental Panel on Climate Change, eds R. K. Pachauri and L. A. Meyer (Geneva: Intergovernmental Panel on Climate Change).
Jauhiainen, S., Pitkainen, A., and Vasanderl, H. (2004). Chemostratigraphy and vegetation in two boreal mires during the Holocene. Holocene 14, 769–779. doi: 10.1191/0959683604hl734rp
Jensen, C. (2004). The vegetation history of a coastal stone–age and iron–age settlement at 70oN, Norway. Veg. Hist. Archaeobot. 13, 269–284. doi: 10.1007/s00334-004-0046-7
Josefsson, T., Hörnberg, G., and Östlund, L. (2009). Long–term human impact and vegetation changes in a boreal forest reserve: implications for the use of protected areas as ecological references. Ecosystems 12, 1017–1036. doi: 10.1007/s10021-009-9276-y
Karlsson, H., Emanuelsson, M., and Segerström, U. (2010). The history of a farm–shieling system in the central Swedish forest region. Veg. Hist. Archaeobot. 19, 103–119. doi: 10.1007/s00334-009-0231-9
Kasischke, E. S., O’Neill, K. P., French, N. H. F., and Bourgeau-Chavez, L. L. (2000). “Controls on patterns of biomass burning in Alaskan boreal forests,” in Fire, Climate, and Carbon Cycling in the Boreal Forest, eds E. S. Kasischke and B. J. Stocks (New York, NY: Springer).
Kasischke, E. S., Verbyla, D. L., Rupp, T. S., McGuire, A. D., Murphy, K. A., Jandt, R., et al. (2010). Alaska’s changing fire regime-implications for the vulnerability of its boreal forests. Can. J. Forest Res. 40, 1313–1324. doi: 10.1139/X10-098
Kelly, R., Chipman, M. L., Higuera, P. E., Stefanova, I., Brubaker, L. B., and Hu, F. S. (2013). Recent burning of boreal forests exceeds fire regime limits of the past 10,000 years. Proc. Natl. Acad. Sci. U.S.A. 110, 13055–13060. doi: 10.1073/pnas.1305069110
Kelly, R., Genet, H., McGuire, A. D., and Hu, F. S. (2016). Palaeodata-informed modelling of large carbon losses from recent burning of boreal forests. Nat. Clim. Change 6, 79–82. doi: 10.1038/nclimate2832
Klein Goldewijk, K., Beusen, A., van Drecht, G., and de Vos, M. (2011). The HYDE 3.1 spatially explicit database of human induced global land-use change over the past 12,000 years. Global Ecol. Biogeogr. 20, 73–86. doi: 10.1111/j.1466-8238.2010.00587.x
Kloster, S., Mahowald, N. M., Randerson, J. T., and Lawrence, D. M. (2010). Fire dynamics during the 20th century simulated by the Community Land Model. Biogeosciences 7, 1877–1902. doi: 10.5194/bg-7-1877-2010
Kloster, S., Mahowald, N. M., Randerson, J. T., and Lawrence, P. J. (2012). The impacts of climate, land use, and demography on fires during the 21st century simulated by CLM-CN. Biogeosciences 9, 509–525. doi: 10.5194/bg-9-509-2012
Knorr, W., Arneth, A., and Jiang, L. (2016a). Demographic controls of future global fire risk. Nat. Clim. Change 6, 781–785. doi: 10.1038/nclimate2999
Knorr, W., Dentener, F., Hantson, S., Jiang, L., Klimont, Z., and Arneth, A. (2016b). Air quality impacts of European wildfire emissions in a changing climate. Atmos. Chem. Phys. 16, 5685–5703. doi: 10.5194/acp-16-5685-2016
Knorr, W., Jiang, L., and Arneth, A. (2016c). Climate, CO2 and human population impacts on global wildfire emissions. Biogeosciences 13, 267–282. doi: 10.5194/bg-13-267-2016
Knorr, W., Dentener, F., Lamarque, J.-F., Jiang, L., and Arneth, A. (2017). Wildfire air pollution hazard during the 21st century. Atmos. Chem. Phys. 17, 9223–9236. doi: 10.5194/acp-17-9223-2017
Knorr, W., Kaminski, T., Arneth, A., and Weber, U. (2014). Impact of human population density on fire frequency at the global scale. Biogeosciences 11, 1085–1102. doi: 10.5194/bg-11-1085-2014
Korsman, T., and Segerström, U. (1998). Forest fire and lake–water acidity in a northern Swedish boreal area: holocene changes in lake–water quality at Makkassjön. J. Ecol. 86, 113–124. doi: 10.1046/j.1365-2745.1998.00239.x
Krebs, P., Pezzatti, G. B., Mazzoleni, S., Talbot, L. M., and Conedera, M. (2010). Fire regime: history and definition of a key concept in disturbance ecology. Theory Biosci. 129, 53–69. doi: 10.1007/s12064-010-0082-z
Lagerås, P., Jansson, K., and Vestbö, A. (1995). Land–use history of the Axlarp area in the Småland uplands, southern Sweden: palaeoecological and archaeological investigations. Veg. Hist. Archaeobot. 4, 223–234.
Lamarque, J.-F., Bond, T. C., Eyring, V., Granier, C., Heil, A., Klimont, Z., et al. (2010). Historical (1850–2000) gridded anthropogenic and biomass burning emissions of reactive gases and aerosols: methodology and application. Atmos. Chem. Phys. 10, 7017–7039. doi: 10.5194/acp-10-7017-2010
Landry, J.-S., Matthews, H. D., and Ramankutty, N. (2015). A global assessment of the carbon cycle and temperature responses to major changes in future fire regime. Clim. Change 133, 179–192. doi: 10.1007/s10584-015-1461-8
Larsen, C. P. S., Morris, W. A., and MacDonald, G. M. (2000). Records of geomagnetic secular variation since 1200 AD and the potential for chronological control of lake sediments in northern Alberta. Can. J. Earth Sci. 37, 1711–1722. doi: 10.1139/e00-052
Lehtonen, H., and Kolström, T. (2000). Forest fire history in Viena Karelia, Russia. Scand. J. Forest Res. 15, 585–590. doi: 10.1080/02827580050216833
Lindberg, H., Punttila, P., and Vanha-Majamaa, I. (2000). The challenge of combining variable retention and prescribed burning in Finland. Ecol. Process 9:4. doi: 10.1186/s13717-019-0207-3
Lindbladh, M., and Bradshaw, R. (1995). The development and demise of a Medieval forest-meadow system at Linnaeus’ birthplace in southern Sweden: implications for conservation and forest history. Veg. Hist. Archaeobot. 4, 153–160.
Lindbladh, M., and Bradshaw, R. (1998). The origin of present forest composition and pattern in southern Sweden. J. Biogeogr. 25, 463–477. doi: 10.1046/j.1365-2699.1998.2530463.x
Lindbladh, M., Niklasson, M., and Nilsson, S. G. (2003). Long–time record of fire and open canopy in a high biodiversity forest in southeast Sweden. Biol. Conserv. 114, 231–243. doi: 10.1016/s0006-3207(03)00043-0
Liu, P., Kaplan, J. O., Mickley, L. J., Li, Y., Chellman, N. Y., Arienzo, M. M., et al. (2021). Improved estimates of preindustrial biomass burning reduce the magnitude of aerosol climate forcing in the Southern Hemisphere. Sci. Adv. 7:eabc1379. doi: 10.1126/sciadv.abc1379
Lynch, A. J., Bigelow, A. B., Clark, J. S., Edwards, M., and Finney, B. (2003). Geographic and temporal variations in fire history in boreal ecosystems of Alaska. J. Geophys. Res. 108:8152.
Lynch, A. J., Clark, J. S., and Stocks, B. J. (2004). Charcoal production, dispersal, and deposition from the fort providence experimental fire: interpreting fire regimes from charcoal records in boreal forests. Can. J. Forest Res. 34, 1642–1656. doi: 10.1139/x04-071
Marlon, J. R., Bartlein, P. J., Carcaillet, C., Gavin, D. G., Harrison, S. P., Higuera, P. E., et al. (2009a). Climate and human influences on global biomass burning over the past two millennia. Nat. Geosci. 1, 697–701. doi: 10.1038/ngeo313
Marlon, J. R., Bartlein, P. J., Walsh, M. K., Harrison, S. P., Brown, K. J., Edwards, M. E., et al. (2009b). Wildfire responses to abrupt climate change in North America. Proc. Natl. Acad. Sci. U.S.A. 106, 2519–2524. doi: 10.1073/pnas.0808212106
Marlon, J. R., Kelly, R., Daniau, A.-L., Vannière, B., Power, M. J., Bartlein, P., et al. (2016). Reconstructions of biomass burning from sediment-charcoal records to improve data–model comparisons. Biogeosciences 13, 3225–3244. doi: 10.5194/bg-13-3225-2016
Miettinen, J., Grönlund, E., Simola, H., and Huttunen, P. (2002). Palaeolimnology of Lake Pieni–Kuuppalanlampi (Kurkijoki, Karelian Republic, Russia): isolation history, lake ecosystem development and long–term agricultural impact. J. Paleolimnol. 27, 29–44.
Molinari, C., Bradshaw, R. H. W., Risbøl, O., Lie, M., and Ohlson, M. (2005). Long–term vegetational history of a Picea abies stand in south–eastern Norway: implications for the conservation of biological values. Biol. Conserv. 126, 155–165. doi: 10.1016/j.biocon.2005.05.007
Molinari, C., Carcaillet, C., Bradshaw, R. H. W., Hannon, G. E., and Lehsten, V. (2020). Fire-vegetation interactions during the last 11,000 years in boreal and cold temperate forests of Fennoscandia. Quat. Sci. Rev. 241:106408. doi: 10.1016/j.quascirev.2020.106408
Molinari, C., Lehsten, V., Bradshaw, R. H. W., Power, M. J., Harmand, P., Arneth, A., et al. (2013). Exploring potential drivers of European biomass burning over the Holocene: a data-model analysis. Glob. Ecol. Biogeogr. 22, 1248–1260. doi: 10.1111/geb.12090
Mooney, S. D., Harrison, S. P., Bartlein, P. J., Daniau, A. L., Stevenson, J., Brownlie, K. C., et al. (2011). Late quaternary fire regimes of Australasia. Quat. Sci. Rev. 30, 28–46. doi: 10.1016/j.quascirev.2010.10.010
Niklasson, M., and Granstrom, A. (2000). Numbers and sizes of fires: long-term spatially explicit fire history in a Swedish boreal landscape. Ecology 81, 1484–1499. doi: 10.2307/177301
Ohlson, M., and Tryterud, E. (1999). Long-term spruce forest continuity–a challenge for a sustainable Scandinavian forestry. For. Ecol. Manag. 124, 27–34. doi: 10.1016/s0378-1127(99)00053-5
Olsson, F., Gaillard, M. J., Lemdahl, J., Greisman, A., Lanos, P., Marguerie, D., et al. (2010). A continuous record of fire covering the last 10,500 calendar years from southern Sweden–the role of climate and human activities. Palaeogeogr. Palaeocl. Palaeoecol. 291, 128–141. doi: 10.1016/j.palaeo.2009.07.013
Oris, F., Asselin, H., Finsinger, W., Hély, C., Blarquez, O., Ferland, M.-E., et al. (2014). Long-term fire history in northern Quebec: implications for the northern limit of commercial forests. J. Appl. Ecol. 51, 675–683. doi: 10.1111/1365-2664.12240
Paitre, C. (2008). Dynamique des Marges Forestières de Milieux Tourbeux du Haut–Boréal, Québec Nordique. PhD. thesis. Québec, QC: Université Laval.
Patterson, W. A. III, Edwards, K. J., and Maguire, D. J. (1987). Microscopic charcoal as a fossil indicator of fire. Quat. Sci. Rev. 6, 3–23. doi: 10.1016/0277-3791(87)90012-6
Pechony, O., and Shindell, D. T. (2010). Driving forces of global wildfires over the past millennium and the forthcoming century. Proc. Natl. Acad. Sci. U.S.A. 107, 19167–19170. doi: 10.1073/pnas.1003669107
Pellegrini, A., Ahlström, A., Hobbie, S. E., Reich, P. B., Nieradzik, L. P., Staver, A. C., et al. (2018). Fire frequency drives decadal changes in soil carbon and nitrogen and ecosystem productivity. Nature 553, 194–198. doi: 10.1038/nature24668
Pinto, G. A. S. J., Niklasson, M., Ryzhkova, N., and Drobishev, I. (2020). A 500-year history of forest fires in Sala area, central Sweden, shows the earliest known onset of fire suppression in Scandinavia. Reg. Environ. Change 20:130. doi: 10.1007/s10113-020-01718-2
Pitkänen, A., and Huttunen, P. (1999). A 1300-year forest-fire history at a site in eastern Finland based on charcoal and pollen records in laminated lake sediment. Holocene 9, 311–320. doi: 10.1191/095968399667329540
Power, M. J., Marlon, J. R., Bartlein, P. J., and Harrison, S. P. (2010). Fire history and the global charcoal database: a new tool for hypothesis testing and data exploration. Palaeogeogr. Palaeocl. Palaeoecol. 291, 52–59. doi: 10.1016/j.palaeo.2009.09.014
Power, M. J., Marlon, J. R., Ortiz, N., Bartlein, P. J., Harrison, S. P., Mayle, F. E., et al. (2008). Changes in fire regimes since the last glacial maximum: an assessment based on a global synthesis and analysis of charcoal data. Clim. Dyn. 30, 887–907. doi: 10.1007/s00382-007-0334-x
Power, M. J., Mayle, F. E., Bartlein, P. J., Marlon, J. R., Anderson, R. S., Behling, H., et al. (2013). Climatic control of the biomass-burning decline in the Americas after AD 1500. Holocene 23, 3–13. doi: 10.1177/0959683612450196
Pyne, S., and World, J. (1995). Fire: The Culture of Fire on Earth. Seattle, DC: University of Washington Press.
R Core Team (2016). R: A Language and Environment for Statistical Computing. Vienna: R Foundation for Statistical Computing.
Rabin, S. S., Melton, J. R., Lasslop, G., Bachelet, D., Forrest, M., Hantson, S., et al. (2017). The fire modeling intercomparison project (FireMIP), phase 1: experimental and analytical protocols with detailed model descriptions. Geosci. Model Dev. 10, 1175–1197. doi: 10.5194/gmd-10-1175-2017
Rolstad, J., Blanck, Y.-I., and Storaunet, K. O. (2017). Fire history in a western Fennoscandian boreal forest as influenced by human land use and climate. Ecol. Monogr. 87, 219–245. doi: 10.1002/ecm.1244
Rowe, J. S., Spittlehouse, D., Johnson, E., and Jasieniuk, M. (1975). Fire Studies in the Upper Mackenzie Valley and Adjacent Precambrian Uplands. Ottawa, ON: Department of Indian and Northern Affairs.
Sanford, T., Wang, R., and Kenward, A. (2015). The Age of Alaskan Wildfires. Princeton NJ: Climate Central.
San-Miguel-Ayanz, J., Durrant, T., Boca, R., Liberta’, G., Branco, A., De Rigo, D., et al. (2019). Forest Fires in Europe, Middle East and North Africa 2018. Luxembourg: Publications Office of the European Union.
Segerström, U., and Emanuelsson, M. (2002). Extensive forest grazing, and hay–making on mires – vegetation changes in south–central Sweden due to land use since the Medieval Times. Veg. Hist. Archaeobot. 11, 181–190. doi: 10.1007/s003340200021
Segerström, U., von Stedingk, H., and Hörnberg, G. (2008). Long-term sustainability of a northern boreal deciduous swamp forest in northern Sweden: succession in the absence of fire. Holocene 18, 1113–1122. doi: 10.1177/0959683608093539
Senici, D., Chen, H. Y., Bergeron, Y., and Ali, A. A. (2015). The effects of forest fuel connectivity on spatiotemporal dynamics of Holocene fire regimes in the central boreal forest of North America. J. Quat. Sci. 30, 365–375. doi: 10.1002/jqs.2790
Sheskin, D. J. (2007). Spearman’s Rank-Order Correlation Coefficient. in Handbook of Parametric and Nonparametric Statistical Procedures. Florida, MIA: Chapman & Hall.
Smith, B., Prentice, I. C., and Sykes, M. T. (2001). Representation of vegetation dynamics in modelling of European ecosystems: comparison of two contrasting approaches. Global. Ecol. Biogeogr. 10, 621–637. doi: 10.1046/j.1466-822X.2001.t01-1-00256.x
Smith, B., Wårlind, D., Arneth, A., Hickler, T., Leadley, P., Siltberg, J., et al. (2014). Implications of incorporating N cycling and N limitations on primary production in an individual-based dynamic vegetation model. Biogeosciences 11, 2027–2054. doi: 10.5194/bg-11-2027-2014
Sommers, W. T., Loehman, R. A., and Hardy, C. C. (2014). Wildland fire emissions, carbon, and climate: science overview and knowledge needs. Forest Ecol. Manag. 317, 1–8. doi: 10.1016/j.foreco.2013.12.014
Spearman, C. (1904). The proof and measurement of association between two things. Am. J. Psychol. 15, 72–101. doi: 10.2307/1422689
Stocks, B. J., Mason, J. A., Todd, J. B., Bosch, E. M., Wotton, B. M., Amiro, B. D., et al. (2003). Large forest fires in Canada, 1959-1997. J. Geophys. Res. Atmos. 108:8149. doi: 10.1029/2001JD000484
Surawski, N. C., Sullivan, A. L., Roxburgh, S. H., and Cook, G. D. (2012). Review of FullCAM Forest Fire Event Parameters With Recommendations Supported by a Literature Review. Report for the Department of Climate Change and Energy Efficiency, Report No. EP 28061232. Canberra, ACT: Commonwealth Scientific and Industrial Research Organisation.
Tolonen, M. (1978). Palaeoecology of annually laminated sediments in Lake Ahvenainen, Southern Finland. I. Pollen and charcoal analyses and their relation to human impact. Annal. Bot. Fennici 15, 177–208.
Turetsky, M. R., Kane, E. S., Harden, J. W., Ottmar, R. D., Manies, K. L., Hoy, E., et al. (2011). Recent acceleration of biomass burning and carbon losses in Alaskan forests and peatlands. Nat. Geosci. 4, 27–31. doi: 10.1038/ngeo1027
Tymstra, C., Stocks, B. J., Cai, X., and Flannigan, M. D. (2020). Wildfire management in Canada: review, challenges and opportunities. Prog. Disaster Sci. 5:100045. doi: 10.1016/j.pdisas.2019.100045
van Marle, M. J. E., Kloster, S., Magi, B. I., Marlon, J. R., Daniau, A.-L., Field, R. D., et al. (2017). Historic global biomass burning emissions for CMIP6 (BB4CMIP) based on merging satellite observations with proxies and fire models (1750–2015). Geosci. Model Dev. 10, 3329–3357. doi: 10.5194/gmd-10-3329-2017
Veraverbeke, S., Rogers, B. M., Goulden, M. L., Jandt, R. R., Miller, C. E., Wiggins, E. B., et al. (2017). Lightning as a major driver of recent large fire years in North American boreal forests. Nat. Clim. Change 7, 529–534. doi: 10.1038/nclimate3329
Vorren, K. D. (2005). Stone age settlements at Sørøya, sub-arctic Norway: impact on the vegetation. Veg. Hist. Archaeobot. 14, 1–13. doi: 10.1007/s00334-004-0052-9
Wallenius, T. (2011). Major decline in fires in coniferous forests–reconstructing the phenomenon and seeking for the cause. Silva. Fenn. 45, 139–155. doi: 10.14214/sf.36
Wallenius, T. H., Pitkänen, A., Kuuluvainen, T., Pennanen, J., and Karttunen, H. (2005). Fire history and forest age distribution of an unmanaged Picea abies dominated landscape. Can. J. Forest Res. 35, 1540–1552. doi: 10.1139/x05-050
Weir, J. M. H., Johnson, E. A., and Miyanishi, K. (2000). Fire frequency and the spatial age mosaic of the mixed-wood boreal forest in western Canada. Ecol. Appl. 10, 1162–1177. doi: 10.1890/1051-07612000010[1162:FFATSA]2.0.CO;2
Whitlock, C. and Bartlein, P. J. (2004). “Holocene fire activity as a record of past environmental change,” in Developments in Quaternary Science, eds A. R. Gillespie, S. C. Porter and B. F. Atwater (Elsevier, Amsterdam).
Whitlock, C. and Larsen, C. (2001). “Charcoal as a fire proxy,” in Tracking Environmental Change Using Lake Sediments, eds J. P. Smol, H. J. B. Birks and W. M. Last (Dordrecht: Springer), 75–97.
Willis, K. J., Araújo, M. B., Bennett, K. D., Figueroa-Rangel, B., Froyd, C. A., and Myers, N. (2007). How can a knowledge of the past help to conserve the future? Biodiversity conservation and the relevance of long-term ecological studies. Philos. Trans. R. Soc. Lond. B. Biol. Sci. 362, 175–186. doi: 10.1098/rstb.2006.1977
Keywords: sedimentary charcoal record, biomass burning, fire model, burnt area, carbon flux, Spearman correlation coefficient
Citation: Molinari C, Hantson S and Nieradzik LP (2021) Fire Dynamics in Boreal Forests Over the 20th Century: A Data-Model Comparison. Front. Ecol. Evol. 9:728958. doi: 10.3389/fevo.2021.728958
Received: 22 June 2021; Accepted: 19 August 2021;
Published: 16 September 2021.
Edited by:
Angelica Feurdean, Goethe University Frankfurt, GermanyReviewed by:
Richard Vachula, College of William & Mary, United StatesJun Inoue, Osaka City University, Japan
Copyright © 2021 Molinari, Hantson and Nieradzik. This is an open-access article distributed under the terms of the Creative Commons Attribution License (CC BY). The use, distribution or reproduction in other forums is permitted, provided the original author(s) and the copyright owner(s) are credited and that the original publication in this journal is cited, in accordance with accepted academic practice. No use, distribution or reproduction is permitted which does not comply with these terms.
*Correspondence: Chiara Molinari, Y2hpYXJhLm1vbGluYXJpQG5hdGVrby5sdS5zZQ==