- 1Animal Behaviour Group, Department of Psychology, Neuroscience and Behaviour, McMaster University, Hamilton, ON, Canada
- 2Department of Wildland Resources and Ecology Center, Utah State University, Logan, UT, United States
Cognition, defined as the processes concerned with the acquisition, retention and use of information, underlies animals’ abilities to navigate their local surroundings, embark on long-distance seasonal migrations, and socially learn information relevant to movement. Hence, in order to fully understand and predict animal movement, researchers must know the cognitive mechanisms that generate such movement. Work on a few model systems indicates that most animals possess excellent spatial learning and memory abilities, meaning that they can acquire and later recall information about distances and directions among relevant objects. Similarly, field work on several species has revealed some of the mechanisms that enable them to navigate over distances of up to several thousand kilometers. Key behaviors related to movement such as the choice of nest location, home range location and migration route are often affected by parents and other conspecifics. In some species, such social influence leads to the formation of aggregations, which in turn may lead to further social learning about food locations or other resources. Throughout the review, we note a variety of topics at the interface of cognition and movement that invite further investigation. These include the use of social information embedded in trails, the likely important roles of soundscapes and smellscapes, the mechanisms that large mammals rely on for long-distance migration, and the effects of expertise acquired over extended periods.
Introduction
The factors necessary for maximizing growth, survival and reproduction vary in time and space. To accommodate this temporal and spatial variation, most animals possess the physical means for moving toward beneficial resources and away from harm. In addition to the ability to move, however, animals must frequently decide about the timing, direction and duration of movement as well as its final destination. To make such decisions, animals rely on their cognitive system, which consists of the structures and processes concerned with the acquisition, retention and use of information (Dukas, 2004, 2017). Research in the past few decades has integrated mechanistic information on animal cognition with functional knowledge on animal ecology and evolution (Dukas, 1998; Dukas and Ratcliffe, 2009; Morand-Ferron et al., 2016; Ratcliffe and Phelps, 2019). Our contemporary understanding of animal cognitive ecology, however, is still not well incorporated within the field of movement ecology.
Scientists across a wide range of disciplines have engaged in insightful research on organismal movement for a long time. Examples range from laboratory analyses of movement in bacteria at the micrometer scale (Adler, 1976; Koshland, 1980; Eisenbach and Lengeler, 2004) to field work on whale migration over thousands of kilometers (Pike, 1962; Rasmussen et al., 2007). The young field of movement ecology adds to this knowledge by relying both on new technologies for monitoring natural animal movement over vast areas, and on modern computational tools for analyzing the large data sets acquired through automated tracking (Nathan et al., 2008; Abrahms et al., 2021). Only recently, however, movement ecology has increased the consideration of animal cognition (Fagan et al., 2013, 2017; Avgar et al., 2015; Lewis et al., 2021), an approach that typically requires controlled experimental settings.
Animal cognition can be divided into a few interconnected categories. The first component is perception, which involves capturing information from the environment and converting it into internal representations retained by neuronal networks. Information acquisition is carried out by receptors specialized to capture cue attributes emitted by or associated with relevant objects including patterns of reflected light, sound, odors, flavors and texture. Newly acquired information may either fade away immediately, remain for brief periods, or consolidate into long lasting internal representations that can persist for many years. The process of adding new representations into neuronal networks is termed learning, and the information retained is referred to as memory. The only utility of information acquisition and retention is to determine and execute action. To this end, individuals have to continuously assess relevant environmental features and their experience to decide about their subsequent action (Rolls, 2014; Anderson, 2015; Dukas, 2017).
The framework of movement ecology laid out by Nathan et al. (2008) clearly recognized the crucial role of cognition in general and navigational abilities in particular for the obvious reason that cognition underlies all animal decisions regarding when and where to travel. Although one can study movement while ignoring its underlaying internal mechanisms, a thorough understanding of individuals’ movement decisions requires us to quantify the cognitive processes that drive them. Chief among the cognitive abilities relevant to animal movement are the mechanisms that enable spatial orientation. Such mechanisms allow individuals to both navigate their local surrounding while engaging in their daily routines, and to undertake long-distance seasonal migrations.
To keep our review within the space constraints, we will focus here on experimental research in birds and mammals as these groups have been the subject of most studies in movement ecology. While we will aid our analyses with a few examples from insects, we cannot encompass here the rich body of research on insect navigation (Dyer, 1998; Collett and Collett, 2002; Wehner, 2020). Our review has five parts. In the first two sections, we focus on individual cognition and ignore social influences. First, we discuss the roles of learning and memory in movements within the local settings of one’s home range. Second, we take the broader perspective of the innate mechanisms, learning and memory involved in long-distance movements typically associated with seasonal migration. Most birds and mammals have parental care, many species live in groups (Wilson, 1975; Clutton-Brock, 2016), and even the ones classified as solitary show rich social interactions (Caro, 1994; Elbroch et al., 2017). Hence, our third section assesses the multiple effects of the social environment on the cognitive features that guide movement. The fourth part briefly integrates the previous three sections to address the understudied topic of animal expertise, defined as the traits that enable individuals to show superior performance after a long period of individual and social learning (Dukas, 2019). Finally, our prospects section focusses on a few suggestions for promising research at the interface of cognition and movement.
Individual Learning and Memory Within the Home Range
Most animals can benefit from learning about the attributes of relevant environmental settings, resources and individuals. Examples include food sources, shelters, temperature, predators and other hazards, kin, social partners, competitors, and prospective mates. Key features associated with such variables include unique, identifying cues such as odor, color, sound, taste, size and shape, and their location in space and time. It is thus not surprising that all animals subjected to critical experimental tests show learning when tested under the controlled conditions designed to distinguish learning from relevant alternatives (Dukas, 2008a, 2017). Critical evidence for learning, however, requires strict experimental protocols because learning can only be inferred indirectly through a change in behavior. This means that one has to carefully rule out non-learning alternatives including changes in perception, satiation, physiology, and motivation. For example, while GPS movement data on a single mule deer (Odocoileus hemionus) suggested reliance on spatial memory (Jakopak et al., 2019), the study could not critically rule out alternatives including the use of trails or other cues, or following other individuals. Nevertheless, evidence such as the ability of an individual to return to its summer range after moving about 100 km away is instructive regardless of the mechanism employed. That is, we encourage researchers to modulate their vocabulary based on their evidence where the settings and priorities do not allow for critical tests of learning. Additionally, future research may continue to develop protocols that allow critical tests of cognitive abilities in the field (Morand-Ferron et al., 2016). We provide examples of such field tests throughout our review.
Most relevant for movement ecology is animals’ abilities to learn and remember the spatial locations of resources and individuals. Spatial learning and memory merely means having the ability to acquire and later recall information about distances and directions among relevant objects. This allows individuals to navigate, i.e., find their way among these objects. Controlled laboratory studies indicate robust spatial learning and memory in key model systems including fruit flies (Drosophila melanogaster) (Ofstad et al., 2011) and rats (Rattus norvegicus) (O’Keefe and Dostrovsky, 1971; Moser et al., 2008). Many field studies over the past several decades, which included controlled experiments as well as observations using individually tagged individuals, have revealed exceptional navigational abilities in honey bees (Apis mellifera) (von Frisch, 1967; Seeley, 1996; Dyer, 1998; Menzel et al., 2005; Riley et al., 2005). In addition to routinely traveling to flower patches up to several km away from their nests, honey bees communicate to nestmates the direction and distance to both flower patches and prospective new nests (Dyer, 2002; Visscher, 2007). These skills allow honey bees to dynamically adjust to changes in the spatial and temporal distribution of floral rewards, and to locate the best locally available tree cavities for new nests (Visscher and Seeley, 1982; Beekman and Ratnieks, 2000; Steffan-Dewenter and Kuhn, 2003; Seeley, 2010).
It is fair to assume that all birds and mammals possess spatial learning and memory as good as or better than that experimentally demonstrated for honey bees in the field. Controlled laboratory and enclosure studies typically confined to up to several meters indeed demonstrate excellent spatial learning and memory in a variety of birds and mammals (e.g., Morris, 1981; Sherry et al., 1981; Balda and Kamil, 1992). Much of the field work is either limited or suggestive owing to the lack of a large body of controlled experiments. A notable exception is the homing pigeon (Columba livia domestica) discussed in the section below (Wallraff, 2005; Wiltschko and Wiltschko, 2015).
Consider the following example for study design that has enabled strong inference on cognitive processes. A well controlled field study (Edwards et al., 1996) tested spatial memory in domestic sheep (Ovis aries) in a 30 by 45 m pasture. There was a grid of 4 by 8 plastic bowls with randomly chosen 4 bowls containing food pellets (Figure 1A). The food could not be seen until a sheep was within 0.5 m of the bowl. Each sheep was tested individually 11 times over about a week. In trials 1–6, the position of the bowls containing food remained constant, and sheep reduced the number of bowl visits required to locate the four bowls containing food (Figure 1B). In trial 7, half the sheep had no food in any bowl, and half the sheep had food in four new randomly chosen bowls. This probe trial tested whether sheep relied on spatial memory or on cues emanating from the food. The sheep in both groups mostly searched first in the four bowls that had previously contained food and then searched randomly among the other bowls. This resulted in no change in the number of visits needed to locate the previously food-containing bowls in the no-food group, and in a large increase in the number of visits needed to locate the four new food containing bowls in the location-switching group (Figure 1B). Trials 8–10 consisted of retraining, where the no-food group from trial 7 received food again in the same bowls as in trials 1–6, while the location-switching group received food at the same bowls as in trial 7. Sheep from the previously no-food group maintained their small number of visits required to locate the four food bowls, while sheep from the location-switching group reduced again the number of visits required to find the new locations of the four food bowls (Figure 1B). Trial 11 tested spatial memory after longer than the retention period of 12 h used previously. Here half the sheep were tested after 24 h while the other half were tested after 72 h. Both groups showed the same high performance as in the earlier trials (Figure 1B). The sheep study illustrates how one can critically assess spatial memory in the field. Similar work may be conducted at larger spatial scales with a variety of wild animal populations that are habituated to feeding near humans.
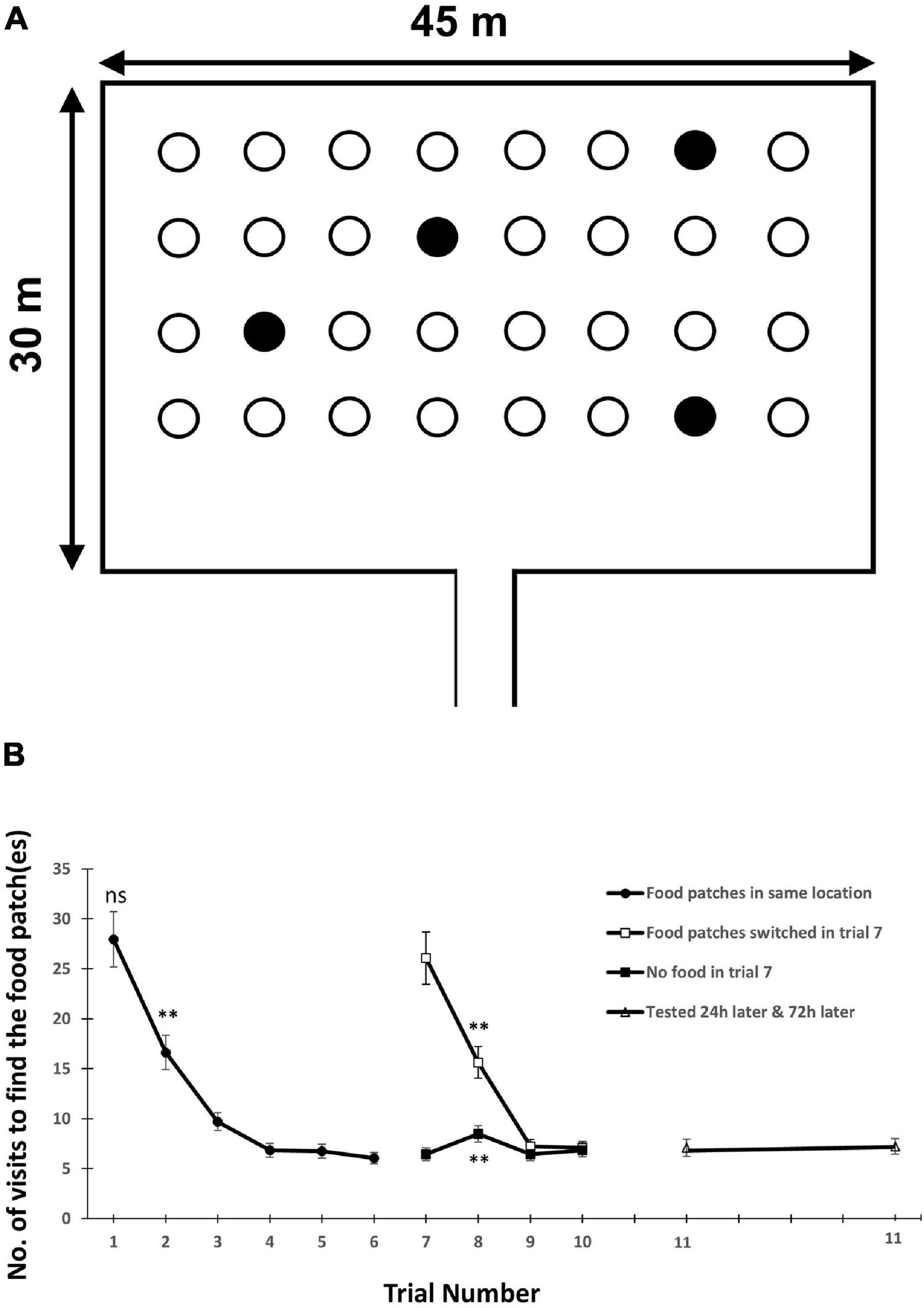
Figure 1. (A) The layout of food bowls in the sheep spatial memory experiment. Open circles illustrate empty bowls while filled circles depict the four bowls containing food. (B) The mean ± SE number of visits required to locate all food bowls. In trials 1–6, the food was always in the same 4 bowls. In trial 7, half the sheep encountered food in 4 new bowls (□) and these bowls also contained food in trials 8–11. The other half of the sheep encountered no food in any bowl in trial 7 (■) and had food in the same bowls as in trials 1–6 in trials 8–11. In trial 11, half the sheep were tested 24 h after trial 10, and the other half were tested 72 h after trial 10. The asterisks indicate visit numbers statistically different from random search in tests conducted in trials 1, 2, and 8. Data from Edwards et al. (1996).
Innate Behavior, Individual Learning and Memory in Seasonal Migration
Seasonal migration occurs in nearly all major animal groups. Traveling to exploit favorable conditions (e.g., food, warmth, or mates) and escaping adverse conditions (e.g., parasitism, predation, or competition) is a beneficial strategy that many animals adopt (Avgar et al., 2014; Somveille et al., 2015). To make navigational decisions during migration, animals use a combination of innate instructions, information they have previously learned either individually or gleaned from others, and cues they currently perceive (Spiegel and Crofoot, 2016). Seasonal migration consists of three phases in which animals use different cues to navigate (Mouritsen, 2018; Figure 2). During the long-distance phase, animals navigate using innate and learned information, and global/regional cues (Schmidt-Koenig, 1990; Gwinner, 1996). In the narrowing-in/homing phase, animals use compasses and landscape information (O’Keefe and Nadel, 1978; Toledo et al., 2020). For the pinpointing-the-goal phase, animals follow specific landmarks near the goal or the goal itself. We will focus on species that live long enough to partake in multiple migrations throughout their lives, providing good opportunity to discuss cognitive processes beyond innate instructions.
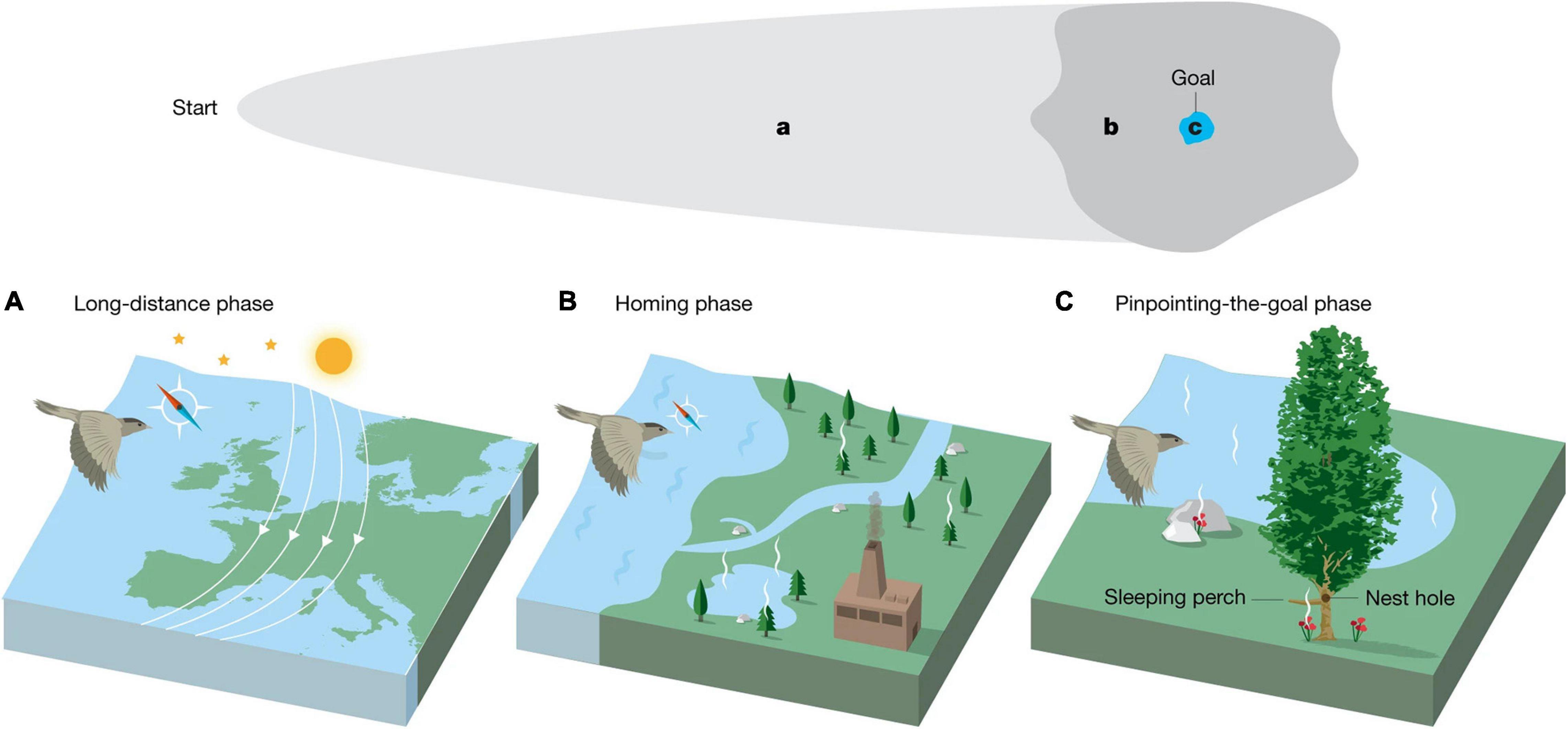
Figure 2. Illustrated phases of long-distance navigation. (A) During the long-distance phase, individuals rely on celestial and magnetic cues as well as on large landmarks such as mountains, lakes, and coastlines. (B) During the homing phase, gradients, landmarks, and compasses are important. (C) During the pinpointing-the-goal phase, residential cues including beacons and the goal itself are valuable. Figure from Mouritsen (2018) with permission.
The vast literature on animal migration has revealed a multitude of innate mechanisms and learned features that guide individuals toward their long-distance goals. The number and complexity of processes involved as well as the variation among species precludes simple generalizations. We thus detail below several key elements. We will first assume no social interactions and focus on the combination of innate mechanisms and individual learning that guide navigation. Then we will discuss in the subsequent section social influences and social learning, which are prevalent in many species.
Compass Orientation
In many cases, orientation toward a long-distance goal can be aided by a compass mechanism. The three compasses—magnetic, sun, and stars—provide simple directional information regardless of the current location (Wiltschko and Wiltschko, 2015). Migratory birds, especially inexperienced individuals, rely on compasses during the long-distance and homing phases of long-distance movement (Mouritsen, 2018). The avian magnetic compass is primarily innate (Wiltschko and Gwinner, 1974), while celestial compasses are primarily learned (Wiltschko and Wiltschko, 1980; Michalik et al., 2014). Birds typically use one compass mechanism to calibrate another (Pakhomov and Chernetsov, 2020). For example, night-migratory songbirds update their star compass using their magnetic compass as a reference (Wiltschko and Wiltschko, 1975). Subsequently, the calibrated star compass can be used independently (Wiltschko and Wiltschko, 2015).
Information from multiple compasses is usually available concurrently, depending on the season, time of day, weather, and magnetic anomalies. Currently, there are various conflicting theories regarding the hierarchy of the compasses used for orientation (Johnsen et al., 2020; Pakhomov and Chernetsov, 2020). When multiple cue types are available, birds likely have preferences for which one to follow based on individual experience, current environment, and distance to their goal (Munro and Wiltschko, 1995; Wiltschko and Wiltschko, 2015; Chernetsov, 2017). If an in-use compass becomes unreliable, birds switch to cues with more accurate readings. For example, pigeons initially rely on magnetic cues, then attempt to compensate for disorientation during magnetic anomalies or experimental disturbance using celestial cues (Keeton, 1971; Ioalé, 1984; Wiltschko and Wiltschko, 2001; Schiffner et al., 2011).
Magnetic Compass
Geomagnetic fields stretching from poles to equator remain relatively consistent over animals’ lifetime, making them informative for determining direction. Birds may rely on a few features of magnetic fields including intensity (strength of the magnetic field), inclination (the angle between the magnetic field and earth surface), direction (polarity), and declination (the difference between true north and magnetic north) (Wallraff, 2005; Wiltschko and Wiltschko, 2005, 2015; Mouritsen, 2018). While much of the research on magnetic compasses has been done in birds, there is growing evidence that mammals, specifically rodents and bats, also possess a magnetic compass (Holland et al., 2006, 2010; Oliveriusová et al., 2012, 2014; Finn, 2021). Some large terrestrial mammals can spontaneously align their bodies with magnetic fields (Begall et al., 2013; Obleser et al., 2016; Painter et al., 2016; Červený et al., 2017), but it is unclear if they use an established magnetic compass for navigation.
Birds inherit their ability to sense magnetic inclination (Wiltschko and Wiltschko, 1972, 2005; Wiltschko and Gwinner, 1974). In some cases, they must calibrate this compass using celestial cues (Able and Able, 1990; Cochran et al., 2004), or vice versa (Muheim et al., 2007, 2009). Magnetic compass orientation is dependent on the presence and wavelength of light (Wiltschko W. and Wiltschko R., 1981; Muheim et al., 2002), although night-migratory songbirds require less light than diurnal birds (Wiltschko and Wiltschko, 2015). The avian magnetic compass works in a functional magnetic intensity window; increasing or decreasing the magnetic strength by 25–30% is disorienting, until birds establish a separate functional window (Wiltschko, 1978; Wiltschko and Wiltschko, 2015). Because magnetic field intensity changes through space, an adjustable compass is advantageous for long-distance movements (Wiltschko and Wiltschko, 2015).
Solar Compass
The temporal cycles and perceived movement of the sun make it an excellent guide for orientation (Guilford and Taylor, 2014). Solar cues are valuable during the long-distance phase of movement (Wiltschko and Wiltschko, 2015; Mouritsen, 2018). Birds may use polarized light cues or the azimuth of the sun itself (Munro and Wiltschko, 1995; Wiltschko and Wiltschko, 2015; Muheim et al., 2016). The sun compass requires learning in juvenile birds. Pigeons establish their sun compass before they are 12 weeks old, while early experience flying can accelerate learning to 8–10 weeks (Wiltschko and Wiltschko, 1981).
Birds must integrate the sun’s movements into their internal clock to orient themselves based on their perceived time-of-day (Wiltschko and Wiltschko, 1980, 1981; Schmidt-Koenig, 1990; Schmidt-Koenig et al., 1991; Åkesson et al., 2017). The integrated sun compass and internal clock must constantly be updated to account for daily and seasonal changes in the perceived location of the sun (Wiltschko and Wiltschko, 2015). Improper synchronization between the internal and sun compass is disorienting. For example, pigeons under experimental settings in which the light-dark cycles were shifted 6 h ahead flew 90 degrees counter-clockwise compared to control pigeons (Schmidt-Koenig, 1958). Once individuals recognize that their compass is shifted, they resynchronize their sun compass and internal clock. Such synchronization occurs naturally when birds travel to different locations (Schmidt-Koenig, 1958; Wiltschko et al., 1998). Yet in some cases, following the sun compass without updating it can be advantageous. Arctic shorebirds such as the American golden plover (Pluvialis dominica), semipalmated sandpiper (Calidris pusilla), pectoral sandpiper (C. melanotos), and the white-rumped sandpiper (C. fuscicollis) migrate without synchronizing their internal clock and sun compass. At high altitudes, non-stop flights attuned to the sun compass result in orthodomes, traveling the shortest distance between two points on a sphere (Alerstam et al., 2001).
Star Compass
The other celestial compass, which is based on stars, provides direction for nighttime navigation. Night-migratory songbirds learn to locate a north-south directional axis based on the fact that stars closer to the celestial axis move through smaller arcs (Emlen, 1970). In order for night-migratory songbirds to learn the compass, they require 2–3 weeks of exposure to a rotating star pattern (Able and Able, 1990; Michalik et al., 2014). Learning occurs during the pre-migratory period before autumn (Emlen, 1970, 1972), but can take place the following spring if needed (Zolotareva et al., 2021). Star patterns change seasonally because of the earth’s rotation around the sun, so migrating birds must regularly update their celestial information (Wiltschko and Wiltschko, 2015). On the other hand, because birds learn the celestial axis rather than the time-dependent celestial location of stars, shifting birds’ internal clock does not affect their star compass orientation (Emlen, 1970; Mouritsen and Larsen, 2001; Pakhomov et al., 2017). Once a star compass is established, it can be used independently of magnetic and solar cues.
Vector Navigation
Long-distance movement poses a considerable challenge for young, inexperienced individuals. First year avian migrants either follow experienced individuals (see Social Learning section below) or use vector navigation (Gwinner, 1996; Bingman and Cheng, 2005; Mouritsen, 2018). Vector navigation, also called the clock-and-compass strategy, uses at least one compass and a set of genetically encoded instructions for direction and distance rooted in their internal clock (Mouritsen et al., 2016). Garden warblers (Sylvia borin) deprived of any seasonal cues for a year displayed migratory restlessness only at the appropriate temporal windows for spring and fall migrations (Gwinner, 1996). The inherited migratory instructions are population specific. Crossbreeding individuals from separate populations with distinct migration routes results in hybrid offspring with intermediate migration patterns (Berthold and Querner, 1981; Helbig, 1991).
Circadian and circannual clocks are responsible for the onset, distance (duration), and direction of migration (Gwinner, 1996). Before learning alternative navigation strategies, naïve individuals rely on genetic instructions, effectively demonstrated by displacement experiments. Experienced birds can correct for displacement over extraordinary distances, while inexperienced juveniles typically fail to do this (Perdeck, 1958). For example, in an experiment involving the displacement of juvenile and adult white-crowned sparrows (Zonotrichia leucophrys gambelii), adults corrected for displacement by adjusting their route toward their usual wintering grounds. Juveniles neglected to reorient themselves, flying in the direction of the expected migration route (Figure 3; Thorup et al., 2007). Juveniles fail to correct for displacement not because they lack that ability, but because they lack information required for compensation (Wiltschko and Wiltschko, 2015).
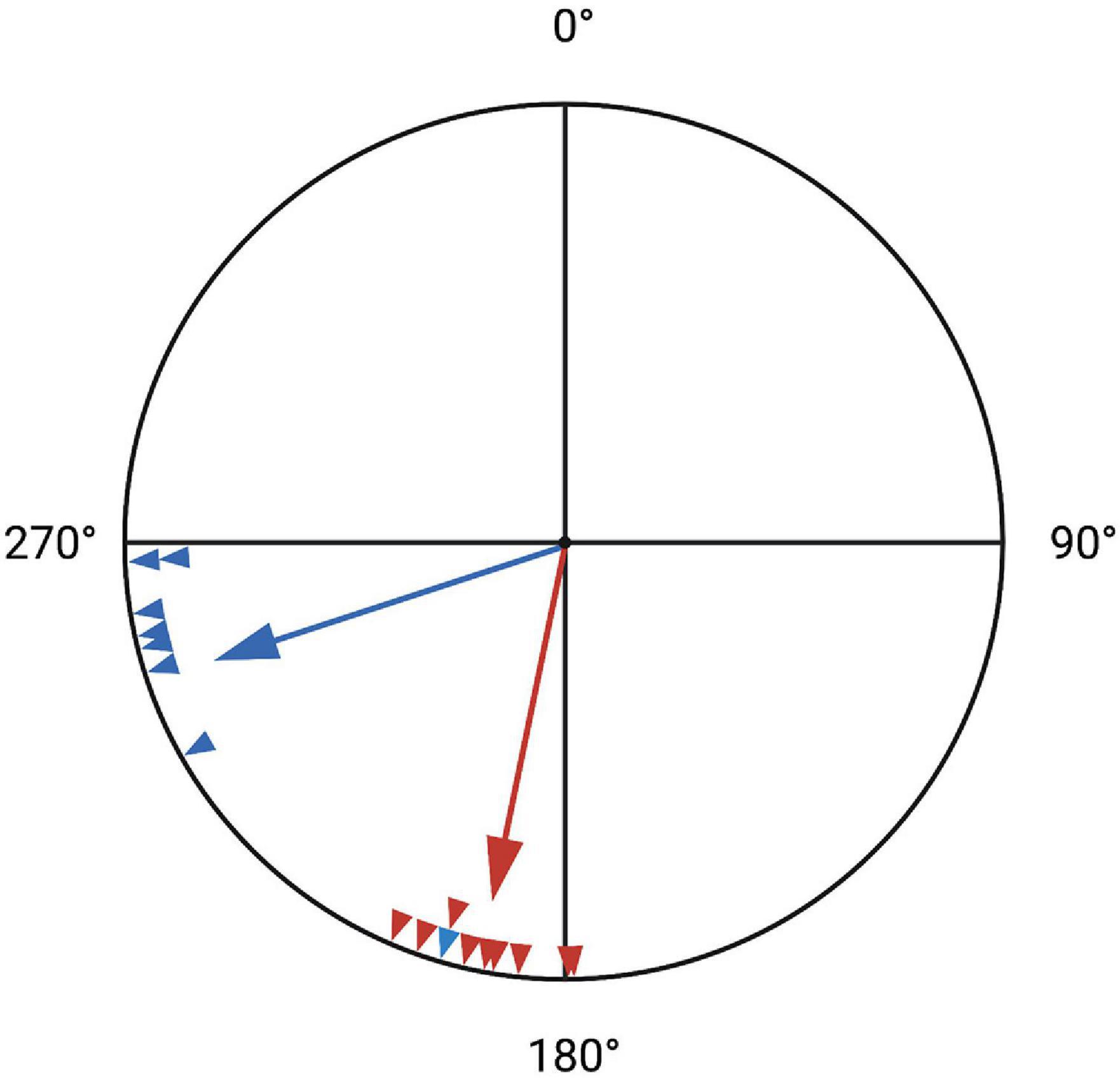
Figure 3. Last observed direction of white-crowned sparrows after experimental displacement east of their location. Adults (blue, large arrow is the average) correctly reoriented southwest toward their original wintering grounds, exhibiting true navigation. Juveniles (red, large arrow is the average) failed to reorient, continuing southward using vector navigation. Based on Thorup et al. (2007).
In some instances, juveniles can correct for displacement. Surprisingly, juvenile blackcaps (Sylvia atricapilla), willow warbles (Phylloscopus trochilus) and garden warblers could reorient themselves after experimental or natural displacement during their first migration to an unfamiliar goal (Thorup et al., 2011). Likewise, some juvenile common cuckoos (Cuculus canorus) corrected for experimental displacement at the same level as adults, traveling toward their expected wintering grounds (Thorup et al., 2020). These compensation mechanisms toward an unfamiliar goal remain unclear—juveniles may be following magnetic cues or using inherited signposts, which are discussed next (Thorup et al., 2011, 2020).
Signposts
Signposts are markers that trigger specific responses that aid in navigation (Wiltschko and Wiltschko, 2005; Freake et al., 2006). Behavioral responses to signposts can be genetically encoded or imprinted. Various species respond to signposts. These include birds (Beck and Wiltschko, 1988; Fransson et al., 2001), turtles (Lohmann et al., 2001), eels (Schabetsberger et al., 2016; Naisbett-Jones et al., 2017), salmon (Putman, 2015; Scanlan et al., 2018) and lobsters (Boles and Lohmann, 2003). Signature magnetic and physical properties act as signposts. Examples include region-specific magnetic intensity, temperature, odor, water salinity or currents (e.g., Fransson et al., 2001; Schabetsberger et al., 2016). For example, particular magnetic intensities can trigger animals to change directions during migration (Putman, 2015; Naisbett-Jones et al., 2017; Scanlan et al., 2018), reorient themselves to avoid ecological barriers and dangerous conditions (Beck and Wiltschko, 1988; Lohmann et al., 2001), or land at stopover sites for refueling (Fransson et al., 2001).
True Navigation
True navigators are individuals that can navigate to a goal after being displaced to an unknown location, at an unknown distance and direction (Griffin, 1952; Kramer, 1953; Keeton, 1974; Able, 2001; Thorup et al., 2007, 2020; Wikelski et al., 2015; Kishkinev et al., 2021). True navigation allows individuals to reach their goal when familiar landscape information is absent. Animals must determine their geographic location, then orient themselves toward the goal using a compass (Griffin, 1952). As mentioned in the vector navigation section above, displaced adult white-crowned sparrows flew toward their usual wintering grounds, exhibiting true navigation, while inexperienced juveniles relied on vector navigation, flying according to genetically encoded instructions (Thorup et al., 2007). Bi-coordinate position fixing, that is, navigation using at least two gradients, is a prerequisite for true navigation (Griffin, 1952; Freake et al., 2006). True navigation involves at least one compass and gradient-based or location-based navigation discussed in the section below.
Navigation Based on Learning and Memory
Animals may learn distinct information for guiding their navigation. Such learning may be egocentric, meaning that it is based on the animal’s own movement, or exocentric, implying that it is based on features of the landscape (Klatzky, 1998). Based on the type of information learned, one can distinguish among four non-mutually exclusive navigation strategies (Fagan et al., 2013) detailed in the four sub-sections below (Figure 4).
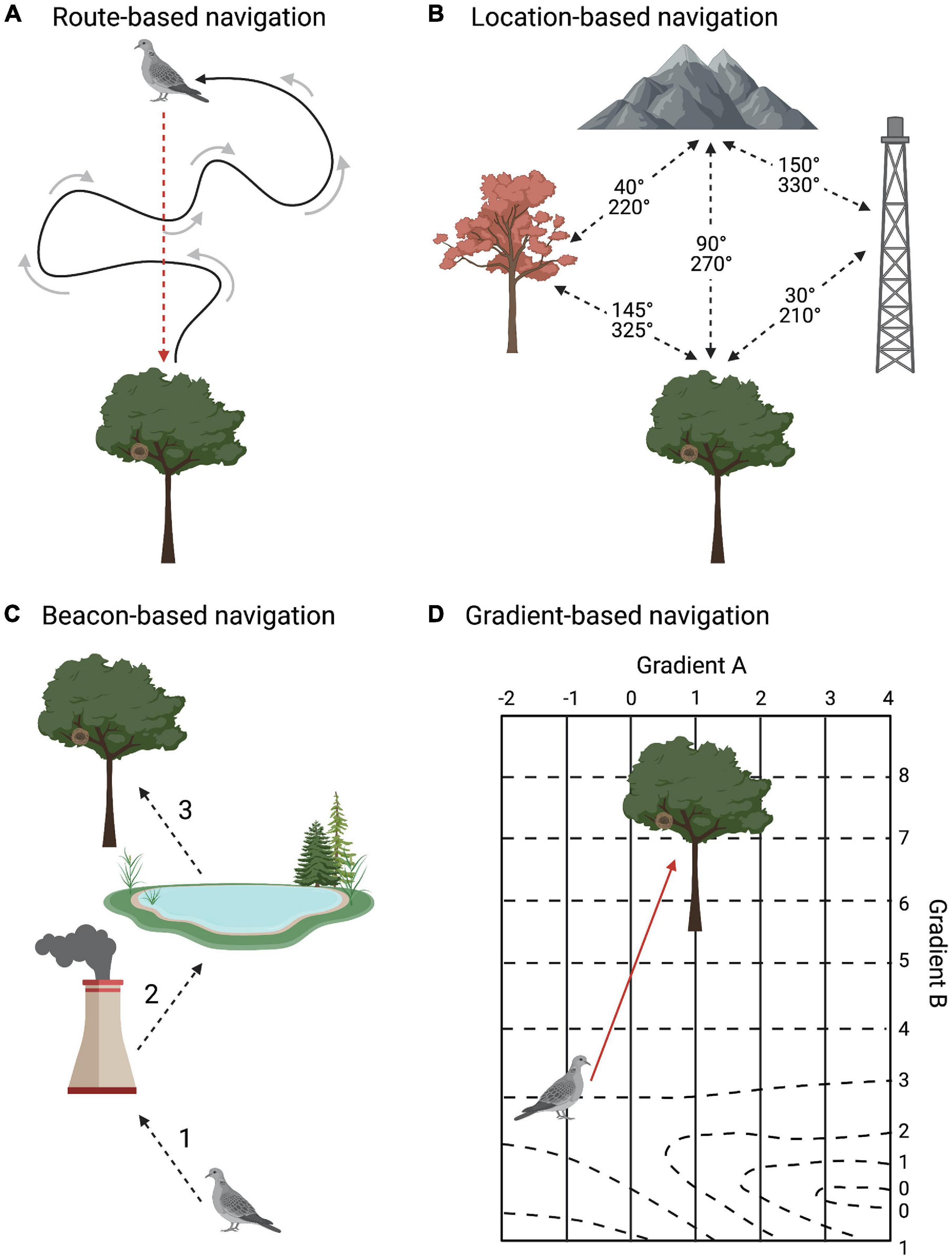
Figure 4. Types of memory-based navigation. (A) In route-based navigation, the individual sums the distance and direction of its outward movements to estimate its current position and take a direct path to its starting point. (B) In location-based navigation, the individual memorizes spatial relationships between landmarks and goals. (C) In beacon-based navigation, specifically pilotage, the individual follows sequential landmarks leading to the goal. (D) In gradient-based navigation, the individual navigates with memorized gradients. In this illustration, both gradients are magnetic (solid and dash lines), with a magnetic anomaly in the southeast corner, which could initially disorient the individual. The scale of D is over 1000 km. Created with BioRender.com.
Route-Based Navigation
During route-based navigation, also called path integration (Figure 4A), individuals record their movements relative to the starting point using a compass and return by reversing their net outward movements (Schmidt-Koenig, 1975; Wiltschko and Wiltschko, 2000, 2015; Wallraff, 2005; Fagan et al., 2013; Bidder et al., 2015). Learning is egocentric and structured around self-movement, thus the individual does not need to be familiar with the landscape because landmarks are not required (Wehner et al., 1996; Kimchi et al., 2004). Route-based navigation is a cognitively simple strategy that requires little memorization (Mittelstaedt and Mittelstaedt, 1982; Wehner and Wehner, 1986; Fagan et al., 2013). Additional distance and turns increase the cognitive demand of keeping track of the route, leaving more room for mistakes. Thus, we can expect route-based navigation to be used at smaller scales. During route-based navigation, individuals acquire information of the unfamiliar area en route to build their exocentric navigation strategies (Wiltschko and Wiltschko, 2015).
Location-Based Navigation
In location-based navigation (Figure 4B), one learns the spatial relationships between landmarks and goals (O’Keefe and Nadel, 1978; Bingman and Cheng, 2005; Wallraff, 2005; Fagan et al., 2013; Wiltschko and Wiltschko, 2015; Toledo et al., 2020). This strategy may use simple memory snapshots (Cartwright and Collett, 1982; Alert et al., 2015) or complex cognitive representations of space (Bingman and Cheng, 2005). A compass is required for learning geographical directions in relation to landmarks (Wiltschko and Wiltschko, 1982, 2015). Although learning spatial relationships between landmarks and goals can produce a heavy memory load, repeated experience moving throughout the landscape should reinforce these memories, reducing cognitive load. Migratory animals probably learn spatial information at a larger scale but lower acuity compared to non-migratory navigators (Bingman and Cheng, 2005). This navigation technique is valuable during the homing phase of long-distance movement.
Beacon-Based Navigation
Individuals using the beacon-based strategy (Figure 4C) are guided to their goal by at least one familiar beacon, which is a landmark near the goal (Papi, 1992; Biro et al., 2004; Wallraff, 2005; Fagan et al., 2013; Wiltschko and Wiltschko, 2015). This includes traveling toward the goal itself or following a series of landmarks to reach the goal (Collett et al., 1986, 1992; Steck et al., 2009; Guilford and Biro, 2014; Yovel and Ulanovsky, 2017). While exploring unfamiliar areas, individuals use a compass to navigate until they learn which landmark(s) lead them to their goal. Once they have established a route, compasses become unnecessary as they can follow the landmark(s) directly (Papi, 1992; Biro et al., 2007; Guilford and Biro, 2014). Beacon-based navigation is most applicable during the homing and pinpointing phases of long-distance movement, as landmarks are typically too small to perceive at long distances, with the exception of massive geographical features such as coastlines or mountains.
Gradient-Based Navigation
Individuals that rely on gradient-based navigation (Figure 4D) have to learn perceptual signatures of at least one feature that changes gradually over space (e.g., magnetic or olfactory gradients) (Bingman and Cheng, 2005; Wallraff, 2005; Fagan et al., 2013; Wiltschko and Wiltschko, 2015). Navigating using gradients requires a compass (Wiltschko and Wiltschko, 2015). Magnetic cues provide both compass orientation and gradient-based navigational information through different perceptual mechanisms—interfering with magnetic cues for one does not impair the other (Munro et al., 1997; Deutschlander et al., 2012; Holland and Helm, 2013; Chernetsov et al., 2017). Gradients are functional during the homing phase and can be projected to longer distances beyond an individual’s experience (Gagliardo et al., 2013; Wikelski et al., 2015). For example, birds learn the features of the magnetic field throughout their home or migratory range, then extrapolate that information to spatial scales beyond what they have experienced (Figure 5; Thorup et al., 2007; Kishkinev et al., 2021). Extrapolated gradients are not always accurate representations of nature (Wallraff, 2005). Individuals extrapolate to unknown locations based on memory of familiar gradients, leaving unfamiliar anomalies and gradient changes unaccounted for, which could result in navigation miscalculations. For instance, individuals displaced to the northwest corner of Figure 5A would move away from their true home because their extrapolated gradient is signaling them to fly in the opposite direction (Wallraff, 2005).
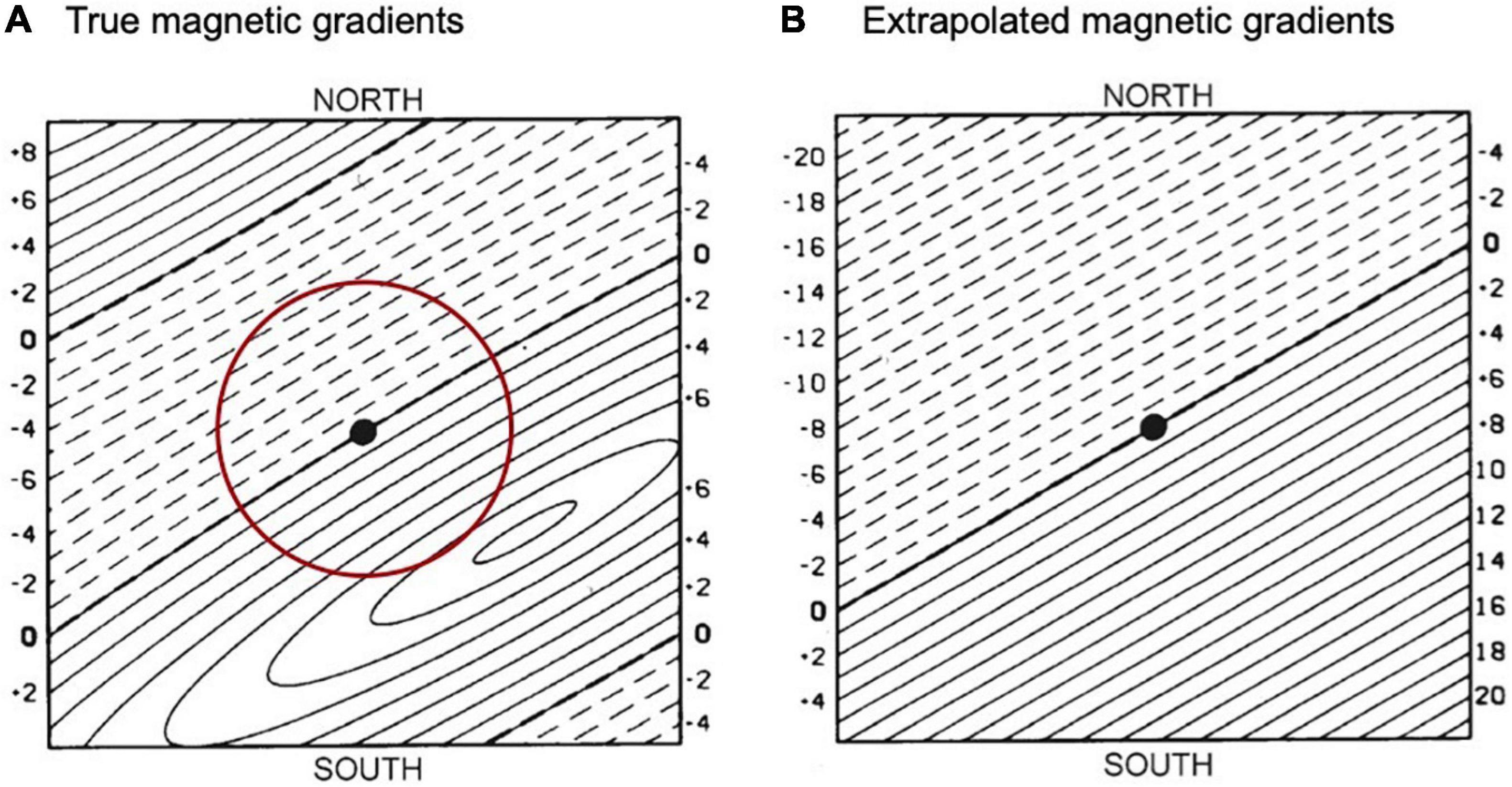
Figure 5. An example of true and extrapolated magnetic gradients. A single gradient is showed for simplicity with positive (dashed line) and negative (solid line) values, on a scale over 1000 km. (A) True magnetic gradient values surrounding the individual’s home (black dot) and its familiar range (red circle). (B) The individual extrapolates gradients based on experience, and incorrectly anticipates the gradient in the northwest and southeast corners. Based on Wallraff (1985).
Selecting a Navigation Strategy
Strategies of navigation vary among species. In species that employ multiple strategies, their use depend on individual experience, preference, available information, distance from the goal, and energy expenditure (Filannino et al., 2014; Green et al., 2020). Furthermore, animals may use multiple navigation strategies simultaneously (Wiltschko and Wiltschko, 2015). Navigation strategies driven by landscape familiarity (location-, beacon-, or gradient-based, Figure 4) are generally preferred over route-based navigation (Wiltschko and Wiltschko, 2015), likely because these strategies are less prone to errors, and allow for short cuts and course corrections. Pigeons using gradient-based navigation modified their route depending on their current motivational state. Food deprived pigeons released at an unknown location flew to a known food source, while satiated pigeons released at the same location flew to their home loft (Blaser et al., 2013). Contrarily, route-based navigation does not allow individuals to revise their goal, and displacing individuals is disorienting, as their reversed net outward movements no longer lead to the return point (Müller and Wehner, 1988; Andel and Wehner, 2004). If information necessary for a preferred strategy is unavailable, animals revert to a simpler feasible navigation technique. For instance, rats revert to route-based navigation when beacons are unavailable (Shettleworth and Sutton, 2005).
Synthesis
Notwithstanding our precautionary note that both the multitudes of complex processes involved in navigation and the variation among species preclude simple generalizations, we wish to end this section with a synthesis. In many species, naïve individuals traveling alone can successfully execute long-distance migration based on innate instructions and one or more compasses. All three compasses typically include innate as well as learned information. Overall, navigation is greatly enhanced by learning, which may be solely based on an individuals’ own movement, but typically also on a variety of environmental features including landmarks and gradients. Furthermore, individuals in many species acquire information related to navigation from others, a topic we discuss next.
Social Influences and Social Learning
The traditional separation of animals into solitary and social species is rapidly fading as we learn to appreciate the sophisticated social skills of animals historically classified as non-social (Costa, 2006; Durisko and Dukas, 2013; Elbroch et al., 2017). It is fair to assume that individuals in most species can gain from information gleaned from conspecifics, and that such information may guide their movements. Social influence merely means that a focal’s behavior is affected by the presence, activity, or cues left by other individuals. Social learning means that a focal acquires novel information based on the presence, activity, or cues left by other individuals. As we discuss below, a dominant source of information that young individuals should attend to is parents and other old individuals. While we focus here on species with parental care, direct or indirect cues left by parents are highly relevant in species in which young do not encounter their parents (Dukas, 2010). We begin by discussing philopatry and the importance of trails. We then review collective navigation, and the use of social learning for decisions regarding home range and migratory movement.
Philopatry
In birds and mammals, newborn rely on their parents for food and protection. When young are sufficiently mature, they typically travel farther from their nest or shelter and often follow their parents. The duration of parental feeding of fledgling varies widely among birds and is positively associated with the duration of practice required for reaching some threshold of foraging proficiency (Ashmole and Tovar, 1968; Heinsohn, 1991; Hunt et al., 2012). In mammals, post-weaning maternal care is brief in short-lived species but can last for years in long lived species (Clutton-Brock, 2016). At least in carnivores, the long duration of post-weaning maternal care is related to the low learning rate associated with challenging hunting skills (Caro, 1994; Wachter et al., 2017). While there is wide variation among species, in the vast majority of birds and mammals, young have ample opportunities to learn many features of their natal environment including the food types preferred by their parents, foraging skills, and the spatial locations of food, shelters, and hazards (Slagsvold and Wiebe, 2007, 2011).
In most species, a large proportion of individuals do not reach reproductive maturity. Of those that reproduce, a large proportion of parents fail to lead their offspring to independence owing to either predation or starvation (Clutton-Brock, 1988). Hence the fact that young have reached independence is a solid evidence that their parents have chosen well their natal environment. Given their likely lack of knowledge about alternative sites and the costs of acquiring such information, the young should copy their parents’ choice and show philopatry where possible (Stamps et al., 2009). Several other factors that promote philopatry include advantages of familiarity with biotic and abiotic features of the natal habitat, improvements to the environment by previous generations such as reusable burrow systems, tree cavities and trails, and the mortality risk associated with exploration of novel areas (Waser and Jones, 1983). Indeed, philopatry is prevalent in both birds and mammals (Greenwood, 1980; Waser and Jones, 1983).
A large cross fostering study tested the effects of early social experience on natal habitat preference in pied flycatchers (Ficedula hypoleuca) in Spain. Pied flycatchers are long-distance migrants who breed throughout Europe but spend the winter south of the Sahara. The experiment involved cross fostering nestlings between nests in a coniferous habitat and nests in a deciduous habitat one km away and, as a control, cross fostering nestling within each of the two distinct habitats. Most returning young birds came to the forest patch from which they had fledged, regardless of whether they had been cross-fostered within or between patches (Figure 6A). These results indicate a strong effect of early experience on habitat choice (Camacho et al., 2016), which is consistent with many other bird studies (Greenwood, 1980; Weatherhead and Forbes, 1994). The results also illustrate remarkable navigational and spatial memory abilities, which allowed 1 year old birds to relocate the small patch of their natal forest after a round trip migration of thousands of km. While the young birds receive no guidance from their parents (Mouritsen and Larsen, 1998), some reliance on social information cannot be ruled out.
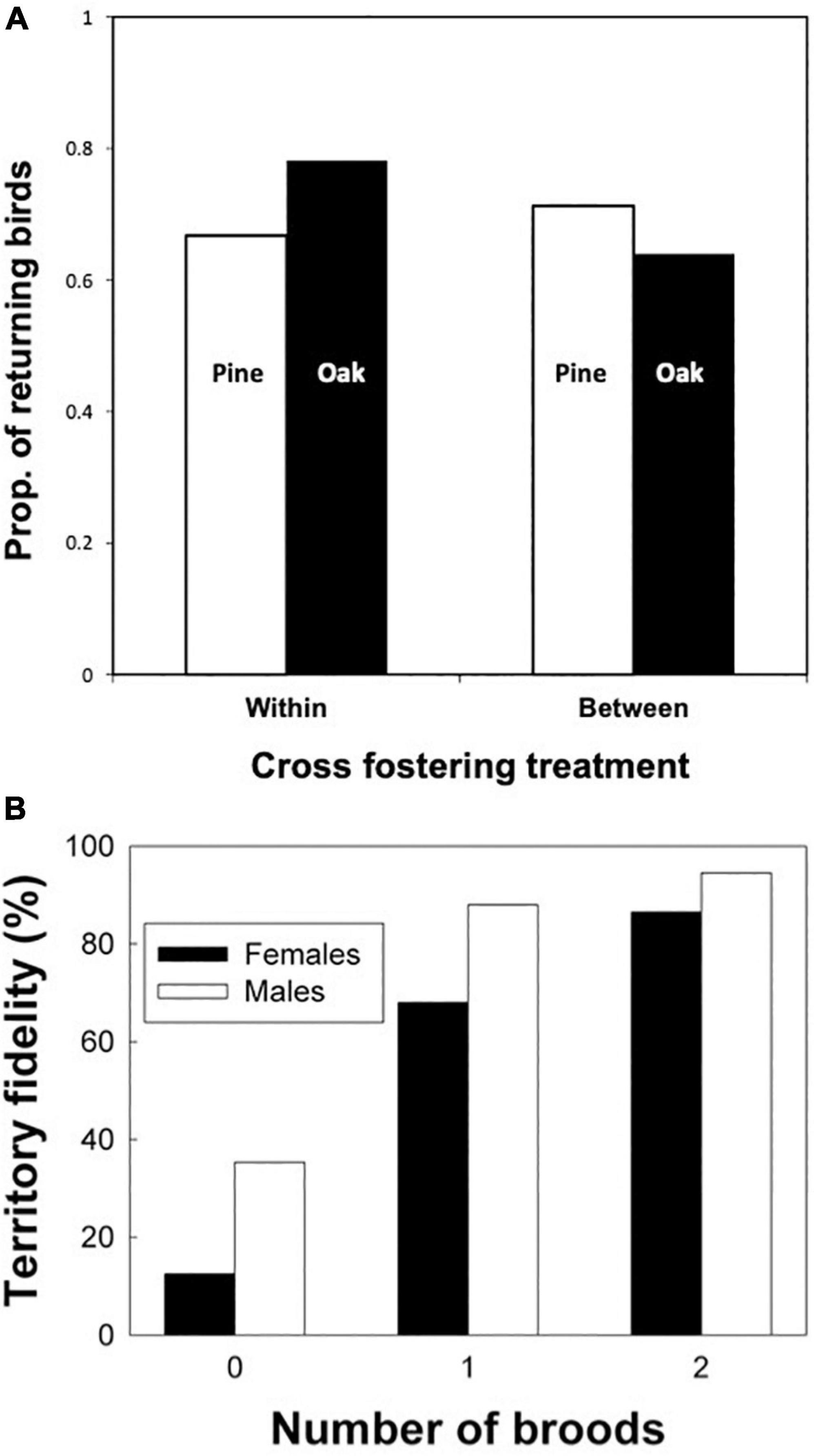
Figure 6. (A) The proportions of pied flycatcher nestlings that returned to breed in the same patch where they had fledged after being cross-fostered within or between habitats containing either pines or oaks. Data from Camacho et al. (2016). (B) Territory fidelity of female and male prothonotary warblers that were experimentally manipulated to have 0, 1, or 2 successful broods in the previous year. Data from Hoover (2003).
Similar effects of early social influence were observed in a study involving 57 radio-collard moose (Alces alces) in Sweden, which revealed strong philopatry by calves. Ten of the 14 radio collard calves returned with their mothers to their summer range, and 9 out of the 10 subsequently separated from the mothers but remained within 2 km from them. The female offspring kept returning to that range in subsequent summers (Cederlund et al., 1987).
In both birds and mammals, site fidelity is even stronger in breeding adults than in young. The same reasons listed above can readily explain why adults remain in their current home range, or keep returning to it in species that show seasonal migration. Indeed, adult philopatry tends to increase with the temporal consistency in conditions, risks, and resources, and particularly their predictability from year to year (Riotte-Lambert and Matthiopoulos, 2020; Morrison et al., 2021). The factor of experience becomes stronger over time, because individuals can keep learning site-specific relevant information as discussed in the expertise section below (Dukas, 2019). Individuals, however, can rely on their experience to decide whether it pays to show site fidelity. Indeed in many bird species, individuals are more likely to show site fidelity if they succeed than fail in reproduction (Greenwood and Harvey, 1982). For example, an elegant experiment manipulated the nesting success of prothonotary warblers (Protonotaria citrea) that used nest boxes in southern Illinois (Hoover, 2003). Birds were randomly assigned into rearing zero, one or two successful broods within a season, with the failures manipulated via predation. Success rates strongly affected the return rates of birds in the following season, after their long-distance migration to Central or South America (Figure 6B). Intriguingly, males whose nesting attempts failed were three times more likely to show site fidelity if they had successful rather than unsuccessful neighbors. Because the males can monitor and even father offspring in neighboring territories through extrapair copulations, it is likely that neighbors’ success affected their subsequent site fidelity (Hoover, 2003). In addition to their remarkable navigational and spatial memory abilities, the prothonotary warblers also showed sophisticated skills in optimizing decisions affecting future reproductive success based on both their own and their neighbors’ experience.
To synthesize, while there are clear benefits to philopatry and obvious costs to dispersal, the social and genetic trade-offs vary among species, leading to a large within and between species variation in the overall and sex-specific patterns of philopatry (Greenwood, 1980; Waser and Jones, 1983; Smale et al., 1997; Clutton-Brock, 2016; Morrison et al., 2021). There are many unresolved issues ripe for investigation, which can take advantage of modern movement ecology tools. Specifically, the causes and consequences of within species variation in philopatry provide intriguing questions. On the cognitive ecology side, in species where one sex remains and the other sex disperses, are there between sex differences in cognitive traits such as spatial abilities, tendencies to explore, attachment to kin, and openness to new experiences? On the movement ecology side, current tracking techniques can help us quantify the sex-specific trade-offs that underlie philopatry versus dispersal.
Trails
Trails can be perceived as social information left from previous generations owing to their usefulness in connecting multiple sites containing resources such as food, water, minerals and shelters while minimizing effort and perhaps danger. Similarly, trails may be used for migratory movement. Trails are also a rich source of contemporary social information, as olfactory and visual cues left by previous trail users can provide information on their identity, number, reproductive state, condition, and the time and direction of travel (Mutinda et al., 2011). Finally, established and well maintained trails allow fast, efficient travel between feeding areas, and between feeding patches and shelters. Indeed, as noted above, an established trail system is one factor that can promote site fidelity in walking species. For example, elephant shrews (Elephantulus rufescens) restrict much of their travel to a network of trails from which they regularly remove plant material and other obstacles. It has been suggested that their rapid running along these trails is an effective anti-predatory strategy (Rankin, 1965; Rathbun, 1979). In larger animals, trails have been studied primarily in elephants (Loxodonta africana) (Vanleeuwe and Gautier-Hion, 1998; Mutinda et al., 2011) and mentioned in a few other studies (Di Fiore and Suarez, 2007; Noyce and Garshelis, 2014; Trapanese et al., 2019). Despite the prevalence and potential importance of animal trails in shaping animal movement, however, they remain understudied.
Aggregations, Information and Individual Movement
A fair number of animals live their whole or part of their lives in aggregations. Examples include bird and bat roosts, and nesting aggregations in solitary bees and birds (Allee, 1931; Michener et al., 1958; Rolland et al., 1998; Beauchamp, 1999; Fenton and Simmons, 2015). Philopatry, discussed above, can readily lead to aggregation. Additional factors include the rarity of appropriate sites, anti-predatory advantages and social information about food and predators (Galef and Giraldeau, 2001; Danchin et al., 2004; Evans et al., 2016). The most likely effect of social information on movement is via local enhancement, whereby individuals searching for food join others whom they observe feeding (Thorpe, 1963; Krebs et al., 1972; Thiebault et al., 2014). Another possibility is that individuals from the aggregation follow departing, apparently informed individuals to food patches. Some field observations agree with this possibility while others do not (Brown, 1986; Mock et al., 1988; Marzluff et al., 1996; Danchin and Richner, 2001; Sonerud et al., 2001; Harel et al., 2017; Urmy, 2021). It is fair to assume, however, that socially biased movement occurs in many species.
Both philopatry and the rarity of satisfactory aggregation sites should lead to large spatial variation in the distribution of aggregating species. Because members of the aggregation can save time and energy as well as incur lower mortality by foraging closer to the aggregation, one would expect lower individual densities farther from the aggregation (Figure 4 in Dukas and Edelstein-Keshet, 1998). Bumblebees in the field indeed showed such pattern of spatial distribution (Figures 3, 4 in Osborne et al., 2008). Reliance on social information would further increase the spatial variation in individual densities. This can lead to cascading spatial effects on other trophic levels. For example, bumblebee wolves (Philanthus bicinctus), sphecid wasps that prey on bumblebees, nest in rare, large aggregations that persist over decades as indicated by the fact that an aggregation studied in the early 1960s (Armitage, 1965) still existed in 2004 (Dukas, 2005). Bumblebee densities at flowers were much lower within 4 km than farther than 5 km from the bumblebee wolf aggregation (Figure 7A). Consequently, fewer flowers of the bumblebee pollinated plant, western monkshood (Aconitum columbianum), set fruit within a few hundred m from the bumblebee wolf aggregation than 6 km away from the aggregation (Figure 7B; Dukas, 2005).
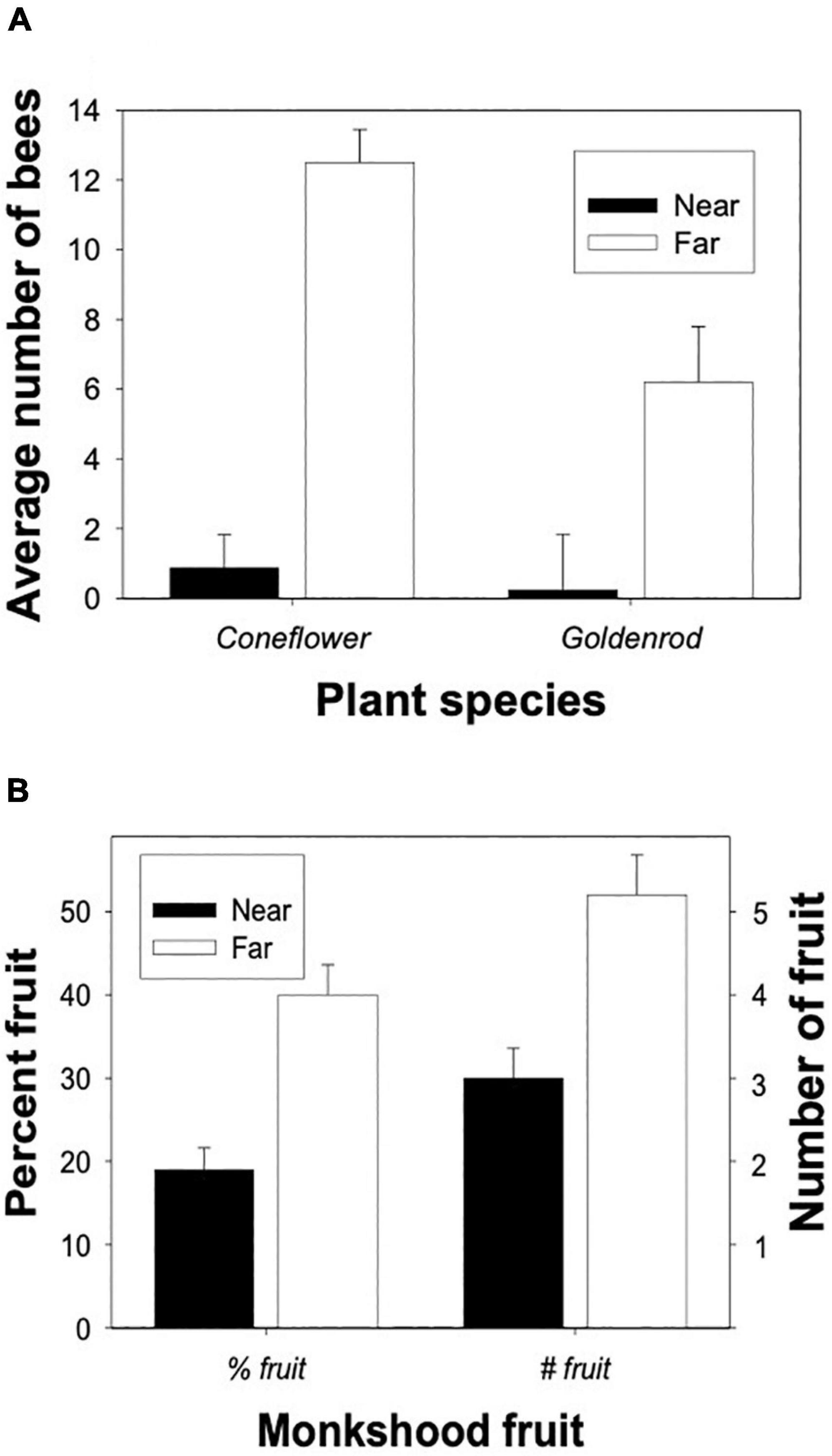
Figure 7. (A) The mean ± SE number of bumble bees observed at six matched pairs of coneflower (Rudbeckia occidentalis) and goldenrod (Solidago spp.) within 4 km and farther than 5 km from a large bumble bee wolf aggregation. (B) Fruit-set in the bumble bee pollinated flower, western monkshood (Aconitum columbianum), within 0.5 km and farther than 5 km from a large bumble bee wolf aggregation. Left bars: the percentage (mean ± SE) of marked monkshood flowers that produced fruits. Right bars: the total number (mean ± SE) of fruits on haphazardly chosen monkshood plants. Data from Dukas (2005).
Collective Navigation and Social Learning
Group-living animals balance individually acquired information with social information to make navigational decisions for home range and migratory movement. When individual information is insufficient, social information can reduce uncertainty (Bergman and Donner, 1964; Hamilton, 1967; Grünbaum, 1998; Couzin, 2018). As we discuss below, collective decision-making mechanisms consist of sharing information among group members or following a subset of directed individuals. Concurrently, knowledge regarding movement patterns can propagate within the group, reducing individual learning costs and improving movement efficiency (Mueller et al., 2013; Sasaki and Biro, 2017; Jesmer et al., 2018).
Collective Navigation Using Shared Information
Collective navigational accuracy can be increased by comparing information through social cues (emergent sensing), pooling information (many wrongs), or communicating preferences (voting).
Emergent Sensing
In emergent sensing (Figure 8A), group members respond to environmental gradients. This results in collective navigation even if all individuals are naïve (Berdahl et al., 2018; Couzin, 2018). Theoretical models suggest that this is a simple collective decision-making strategy that does not require either memory or complex cognition (Torney et al., 2009; Berdahl et al., 2013; Hein et al., 2015). Individuals within the group respond to environmental information and subsequent social cues. For example, golden shiners (Notemigonous crysoleucas) were tested in environments with varying light patches. Golden shiners prefer dark environments and increase swimming speed as a function of light, causing individuals to reduce speed in dark patches, resulting in the group collectively navigating toward dark areas (Berdahl et al., 2013). There is a trade-off between sensitivity to environmental gradients and social information. Greater ability to perceive environmental gradients lessens the need for social interaction, which could decrease group cohesion (Puckett et al., 2018). Therefore, an appropriate balance between environmental cues and social information can be achieved by weighing incoming information (Puckett et al., 2018).
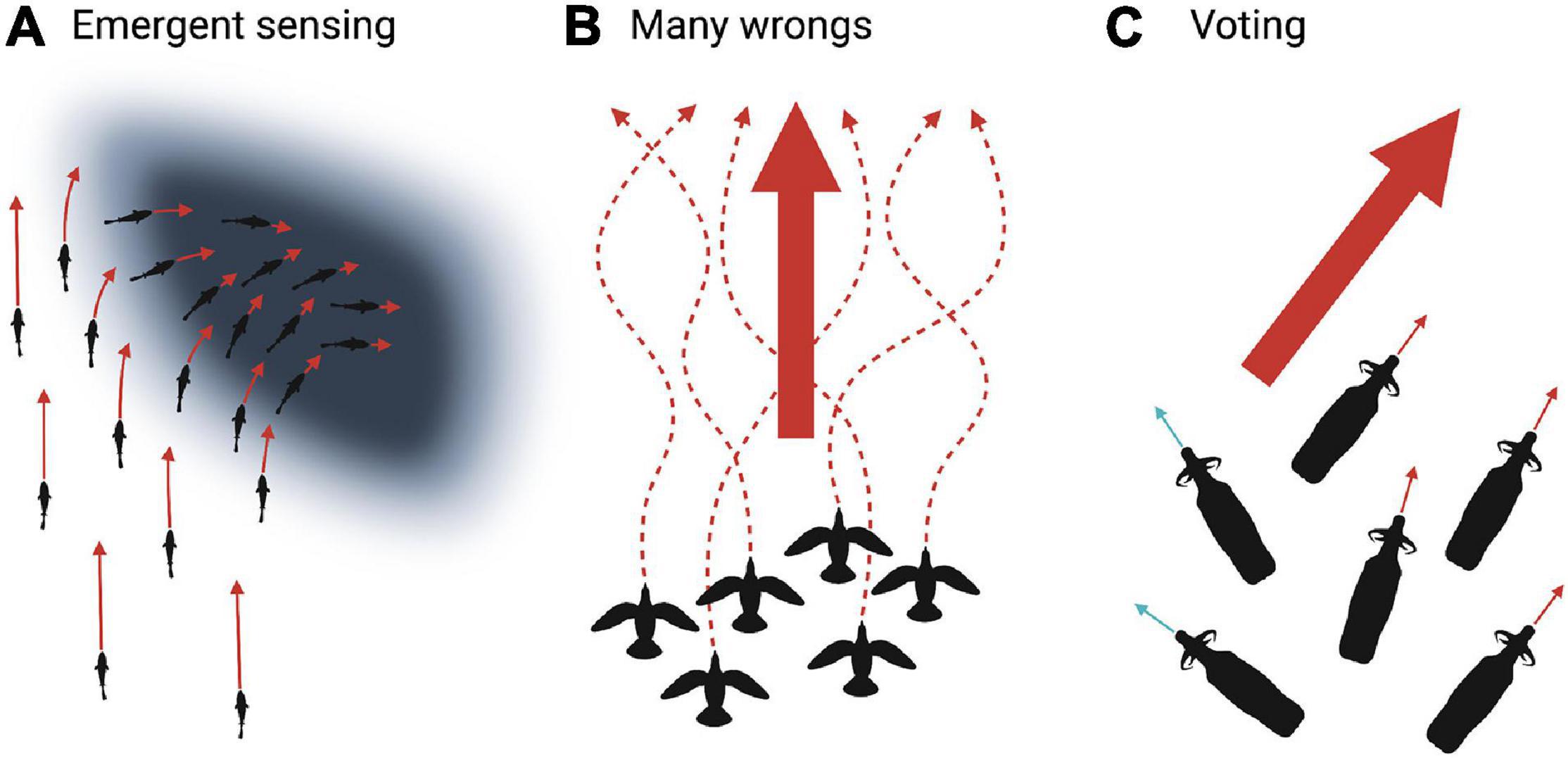
Figure 8. Illustrated examples of collective decision-making strategies. (A) Emergent sensing in which individuals respond to environmental gradients (e.g., golden shiners increase swimming speed as a function of light) and social cues (e.g., responding to movement of neighbors). This results in collective navigation toward favorable dark areas. (B) Many wrongs in which the collective pooling of estimates suppresses individual error (e.g., indirect homing routes in pigeons; dashed arrows) and increases collective navigational accuracy (large arrow). (C) Voting occurs when individuals express their preference for initiation or direction of movement (e.g., African buffalo orienting toward a proposed movement path; small blue and red arrows). Voting prompts the group to choose the majority (large red arrow) or average the proposed paths. Created with BioRender.com.
Many Wrongs
The many wrongs principle (Figure 8B) emerges from individuals pooling each imperfect estimate of direction to improve accuracy, in which group cohesion suppresses individual noise (Bergman and Donner, 1964; Tamm, 1980; Simons, 2004; Biro et al., 2006; Codling and Bode, 2014; Nesterova et al., 2014; Berdahl et al., 2018). For example, homing pigeons released with a small flock flew faster and more direct routes compared to their routes when released alone, even in familiar areas (Figure 9; Dell’Ariccia et al., 2008). When individuals are uncertain about navigational decisions, averaging group information reduces error. However, if the difference between individual estimates becomes too large, the group may split or adopt a leader (Biro et al., 2006; Nesterova et al., 2014). For example, homing pigeons with distinct individually established route preferences were released in pairs. When the distance between the two routes was small, pairs typically averaged their paths. But if the distance between each individual’s established route grew beyond a threshold, pigeons either followed one of the established routes or split to pursue their own route (Biro et al., 2006). For both emergent sensing and many wrongs, directional accuracy increases as group size increases, notably in groups with few individuals (Bergman and Donner, 1964; Wallraff, 1978; Berdahl et al., 2013). We can expect these strategies to be especially advantageous when knowledge among the group members is low and homogeneous, e.g., a group moving through a novel landscape or consisting of inexperienced juveniles.
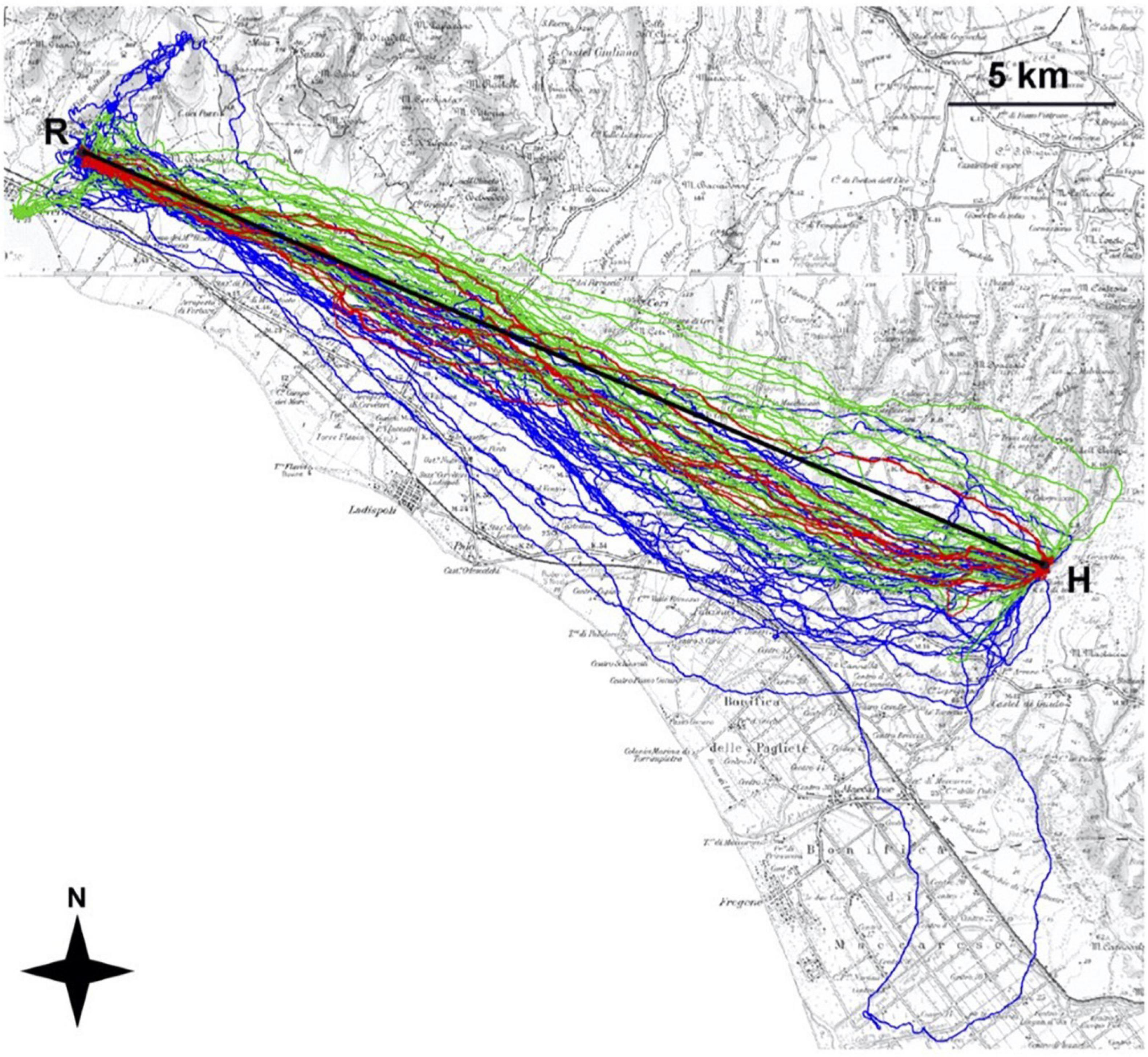
Figure 9. GPS-tracked pigeon routes between a release location (R) and home loft (H). Six pigeons were released six times individually (blue), then six times as a flock (red; one track per flock release), then pigeons were released another six times individually (green). Pigeons released with a small flock flew faster and more direct routes compared to their routes when released alone. Figure from Dell’Ariccia et al. (2008) with permission.
Voting
During the voting strategy (Figure 8C), individuals advertise their preference and then the group selects the majority or averages the choices (Norton, 1986; Black, 1988; Sueur et al., 2010, 2011; Walker et al., 2017). For example, it has been suggested that adult female African buffalo (Syncerus caffer) indicate their preferred foraging patch by orienting themselves toward a certain direction while grazing. The herd then departs in the average direction of individual preferences (Prins, 1996). This widely cited example requires critical tests. Similarly, individuals in troops of olive baboons (Papio anubis) propose a movement path. If the difference between the various prospective paths is above a threshold, the group follows the majority. But, when the angle between proposed routes is below the threshold, the group compromises to average the paths (Strandburg-Peshkin et al., 2015). Emergent sensing, many wrongs, and voting are not mutually exclusive. Furthermore, they are frequently combined with leadership and social learning discussed in the next sections.
Leadership
Group members can have varying degrees of influence on navigational decisions. Commonly, animal groups consist of a subset of individuals called “leaders” which guide the remaining group members deemed “followers” (Chance, 1967; Squires and Daws, 1975; Wallraff, 1978; Wilson, 2000; Van Vugt, 2006). Leadership can be distributed among multiple animals or centered around a sole individual (Garland et al., 2018; Strandburg-Peshkin et al., 2018). Leaders may be aware of their status, are recognized by the group as leaders, and can even produce overt signals to lead their group (Raveling, 1969; Poole et al., 1988; Boinski and Campbell, 1995; Lusseau and Conradt, 2009). Alternatively, leaders could be anonymous and unaware of their influence, thus followers rely on passive cues, such as the orientation of neighbors (Couzin et al., 2005; Rosenthal et al., 2015). The terms leader and follower are relative, as a leader in one situation may be a follower in another. For example, leadership may vacillate depending on the area. Homing pigeons can take turns leading during different segments of the same route (Biro et al., 2006). Additionally, leadership status depends on the identity of others in the group. For example, pigeons have leader hierarchies: if individual A leads B, and B leads C, A will also lead C (Biro et al., 2006; Nagy et al., 2010).
In some groups, knowledge or experience determines leadership. Leaders may possess and act on information that followers do not have, such as knowledge of a migratory route (Reebs, 2000; Olsen, 2001a, b; Chernetsov et al., 2004; Jesmer et al., 2018). However, followers are not always naïve. Followers may hold the same knowledge as leaders, but are more agreeable (Arnold, 1977; Smith et al., 2016), have lower route fidelity (Freeman et al., 2011), or are more receptive to social information (Guttal and Couzin, 2010). In homing pigeons, experience increases the chance of becoming a leader, but does not predict it (Flack et al., 2012, 2013; Watts et al., 2016). Factors such as social status (King et al., 2008), sex (Lusseau and Conradt, 2009), or age can also drive leadership. For example, pods of killer whales (Orcinus orca) are led by postreproductive females (Brent et al., 2015). Likewise, V-formations in families of greater white-fronted geese (Anser albifrons) are primarily led by the father (Kölzsch et al., 2020).
Old individuals may possess information on the most efficient migratory route or the location of a rarely visited site that provides limited resources such as food or water. Such a site may be crucial for the group’s success during either a local food shortage or drought. It has been suggested that old elephant matriarchs possess exclusive spatial information crucial to group fitness (Foley et al., 2008; Mutinda et al., 2011). There is indeed evidence for a positive association between matriarch age and her social knowledge, which translates into superior leadership by older matriarchs (McComb et al., 2001, 2011; Mutinda et al., 2011). The role of spatial information in that superior leadership, however, is unknown.
In some cases, leadership is much less systematic. For example, individuals that travel at high speeds (Pettit et al., 2015) or near the front of the group (Pettit et al., 2013b) can emerge as leaders. Further, group members may rotate leadership roles frequently. Alternating leadership roles is fittingly seen in juveniles with similar demographics and experience (Nesterova et al., 2014). For example, flocks of juvenile northern bald ibis (Geronticus eremita) take turns leading their V-formation during migration (Voelkl et al., 2015; Voelkl and Fritz, 2017). Additionally, leaders can emerge through simple behavioral rules by followers following the movements of their neighbors (Herbert-Read et al., 2011; King et al., 2011; Rosenthal et al., 2015; Torney et al., 2018; Sankey et al., 2021). Thus, the individuals that initiate movement may have a large influence on group navigation.
Although followers are less influential than leaders, followers can participate in and even initiate decisions. However, the threshold to reach a quorum is higher when followers propose decisions compared to leaders (Kummer, 1968; Bousquet et al., 2011; Walker et al., 2017). For example, families of Canada geese (Branta canadensis) perform vocalizations and head-tossing to evoke movement in the group, which ensures cohesion for take-off. If the calls are initiated by a family member other than the father, the number of calls required to elicit movement increases, as well as the period of time before departure (Raveling, 1969).
Cognitive abilities are not identical across group members, leaving some individuals predisposed to become leaders or followers. The shy–bold dimension of animal personality posits that bold individuals have a consistent tendency to explore unfamiliar areas and objects (Gosling and John, 1999; Sih et al., 2004; Réale et al., 2007). Bold individuals are more likely to be leaders than shy individuals (Kurvers et al., 2009; Found and St. Clair, 2016). Compared to shy individuals, leaders are more likely to explore while navigating (Flack et al., 2018), travel at faster speeds, and lead in both familiar and unfamiliar locations (Sasaki et al., 2018). In some cases, leaders may be responsible for immense changes in collective migratory behavior. For example, in elk (Cervus canadensis), bold individuals are three time less likely to migrate than shy individuals from the same population (Found and St. Clair, 2016), likely because they better adapt to changing environments (Found and St. Clair, 2019). Another dimension of personality, sociability, can also produce leaders. Chacma baboons (Papio ursinus) follow the movements of individuals that they are socially affiliated with (King et al., 2011). Thus, individuals that are highly social are more influential than their less social peers.
Social Learning
Information can diffuse throughout a population and across generations via social learning. Accumulated knowledge can act as a second, non-genetic, inheritance system (Whiten, 2005; Tennie et al., 2009; Jesmer et al., 2018). Many animals learn migratory behavior from their parents or older conspecifics in their population (Olsen, 2001a, b; Agostini, 2004; Chernetsov et al., 2004; Urbanek et al., 2005; Harrison et al., 2010; Palacín et al., 2011). Social influences may even override genetic instructions (Schüz, 1951; Perdeck, 1958; Ferrari et al., 2009; Mellone et al., 2016). Old individuals possess valuable information that has helped them survive thus far. Hence, juveniles profit by learning from old, experienced members of their group, even if juveniles possess adequate migratory information. For example, juvenile whooping cranes (Grus americana) migrate using more direct routes when flying with older individuals compared to groups consisting only of young birds (Figure 10; Mueller et al., 2013). It is unclear, however, if juveniles deviate from direct routes because of either error or exploration of unfamiliar territory (Mueller et al., 2013; Wolfson et al., 2020). Additionally, learned migratory behaviors are more flexible than genetic instructions, allowing changes in migratory patterns to spread through populations within the lifetimes of individuals. For example, populations containing older whooping cranes were more likely to modify their migratory routes by establishing new wintering grounds closer to their breeding grounds (Teitelbaum et al., 2016).
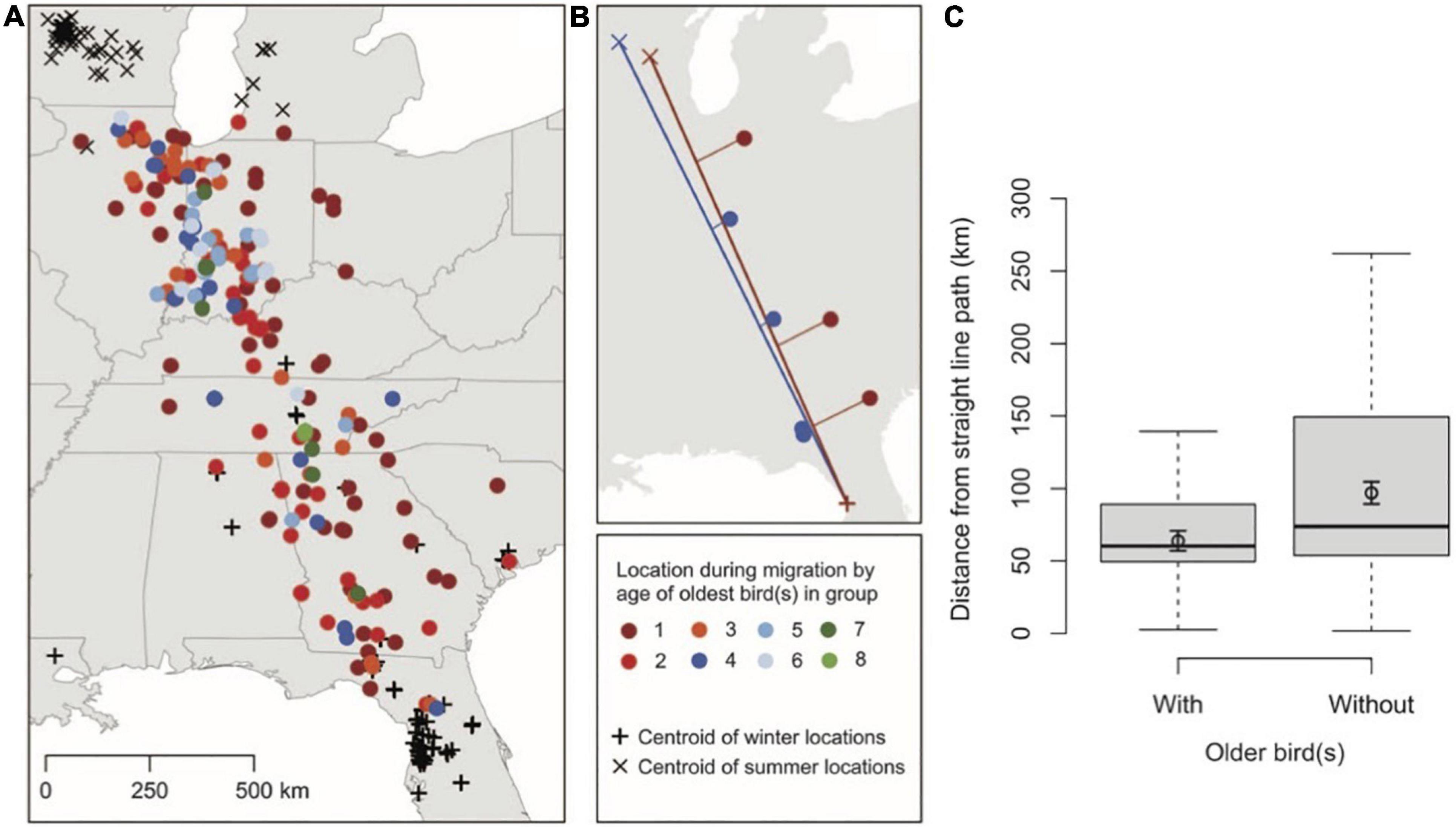
Figure 10. Groups of whooping cranes containing old individuals migrate using more direct paths compared to groups consisting of only juveniles. (A) Telemetry and visually observed locations of whooping cranes throughout their migratory journey. (B) Examples of migratory routes that juveniles performed with (blue) and without (red) the presence of older individuals. (C) Distance from straight line paths during migration of juvenile groups with and without older individuals. Figure from Mueller et al. (2013) with permission.
Individuals can update socially transmitted information to filter out inefficient routes. While homing, pigeon followers actively participate in navigation, learning more direct routes than that of their leader (Pettit et al., 2013a). Additional individuals incorporating their own information into the collective pool of knowledge can further improve group performance. An experiment by Sasaki and Biro (2017) investigated the effects of social learning and cumulative improvement. The experimental group consisted of chains of homing pigeon pairs, beginning with a single pigeon that had developed a homing route after 12 releases. The pigeon was then paired with a naïve individual for another 12 releases. The naïve individual learned the route throughout those trials, becoming experienced, then was paired with a new naïve individual for another 12 releases. This process of pairing a newly experienced pigeon with a naïve pigeon was repeated an additional two times for a total of 60 releases per chain. One control group consisted of solo pigeons and the other control group consisted of fixed pairs of pigeons. Pigeons in both control groups were released 60 times from the same site as the experimental group. By the end of the experiment, the experimental group outperformed both solo and fixed pair controls, which plateaued in efficiency. This suggests that naïve individuals learn route information via social learning and contribute to cumulative improvements in route efficacy, more so than solo or fixed pairs with the same total amount of experience.
Synthesis
Social groups adopt various strategies, typically coinciding, to move within and throughout a landscape. Groups can share information to improve navigational accuracy, during which opinions from some individuals have more influence than others, while valuable information can diffuse throughout the group via social learning. For example, leadership and voting coincide in packs of African wild dogs (Lycaon pictus) during pre-departure social rallies. Pack members increase the amount of abrupt nasal exhales (sneezes) prior to departure. Any pack member can propose a departure by initiating sneezing, although individuals other than the highest ranking dominant dogs must have higher signal frequency in order to succeed (Walker et al., 2017). Collective navigation, leadership and social learning are difficult to assess in nature through observation alone. Without controlled experiments, we can only estimate which process is occurring without firm conclusions.
Expertise and Movement
Individual learning, social influence and social learning are ubiquitous among animals. Their long term, combined effects lead to expertise, defined as the features that allow individuals with extensive experience on a given complex task to show superior performance on that task compared to novices (Dukas, 2019). While expertise has been studied primarily in humans (Ericsson and Lehmann, 1996; Ericsson et al., 2006; Vaci et al., 2019; Strittmatter et al., 2020), it is highly relevant for other species as it can manifest in many behaviors including movement. Two domains pertinent to movement ecology are first, mastery of an individual’s habitat, which may include a vast memory about the identity, location and occurrence of a variety of relevant items, events and individuals. Examples include the location of seasonal food plants, shelters, territorial neighbors, and predators. Second, individuals can improve their movement speed and efficiency with practice. This may be owing to motor learning within a small territory (Stamps, 1995), adopting optimal routes that avoid challenging terrain in a large home range (Green et al., 2020), or learning to better handle a variety of challenges throughout a long travel route. For example, a within individual comparison in black kites (Milvus migrans) indicated improvement in migratory performance with age. Part of this improvement was due to birds enhancing their abilities to exploit tailwinds and cope with wind drift (Sergio et al., 2014). Other studies also indicate that birds improve their abilities to handle winds and rising air currents with experience (Harel et al., 2016; Wynn et al., 2020).
Critical research on expertise is currently scarce as it requires comparisons of the same individuals over time while controlling for relevant alternatives that affect performance. These include correlated changes in anatomy, morphology, physiology and effort (Dukas, 2019). Furthermore, between individual analyses are insufficient as they commit selection bias owing to the likely higher mortality rates of inferior individuals. As far as we know, only a single program, which combined observations and controlled experiments in the field, has considered all factors by quantifying within individual foraging performance in natural settings, muscle physiology, and effort as functions of experience (Dukas and Visscher, 1994; Dukas, 2008b, c; Schippers et al., 2006, 2010). Many other species are amenable for long term research programs that add controlled experiments to information about within individual changes in performance with age (Clutton-Brock, 1988, 2016; Wooler et al., 1990; Sand et al., 2006; Daunt et al., 2007a, b; Leach and Sedinger, 2016).
Conclusion and Prospects
Our three main conclusions are first, that birds and mammals possess good spatial learning and memory, which enable them to find their way while engaging in their daily activities. Second, we have good understanding of the cognitive mechanisms that allow many species to navigate successfully over distances spanning up to thousands of km. Third, the movement ecology of many birds and mammals is heavily determined by social influence and social learning. While there is large variation among animals, researchers initiating work on a lesser studied species may assume that it has good spatial cognitive abilities that are influenced by social observations. Nevertheless, the strength of evidence for our three conclusions is rather mixed. Very few controlled experimental studies assessed spatial learning and memory in birds and mammals over a large area. On the other hand, owing to the conspicuousness and ubiquity of animal migration, we have known for a long time about animals’ abilities to orient well between their winter and summer grounds. Some of the mechanisms underlying these navigational skills are mostly understood, at least for a small selection of species, primarily birds. Finally, we know that social information influences some aspects of bird and mammal movement including, for example, philopatry and migration in some species. There are probably, however, many other aspects of movement that are under social influence but understudied. Examples include trails, long-lasting scents, and cues that indicate recent activity or successful reproduction by conspecifics.
While we focused on relatively well studied topics, our review can readily reveal subjects that invite future research. Most notably, we have not critically addressed specific issues of both perception and decisions even though they likely have strong effects on animal movement (Avgar et al., 2013). Some studies have addressed issues of perception relevant to movement ecology. For example, the topic of perceptual range, defined as the maximum distance from which one can detect relevant landscape features, has been recognized for some time (Zollner, 2000; Schooley and Wiens, 2003), but we still know relatively little about it. Similarly, while decisions have been implicitly included within the overall study of animal movement, there has not been a focus on the exact decision rules employed by individuals when considering, for example, when and where to go (Bauer et al., 2011). Finally, our review primarily encompassed issues related to movement either within a home range or during seasonal migration. We have not addressed the two other relevant categories of dispersal and nomadism (Baguette et al., 2014; Teitelbaum and Mueller, 2019) even though they can gain from a synthesis that takes a cognitive ecology approach.
There are various areas that would gain from further integration of methods and insights from cognitive ecology into the study of animal movement. First, there is a strong research bias toward the visual domain. It is thus crucial that we devote more research effort to the effects of understudied sensory domains on animal movement. Such domains include olfaction, electromagnetic radiation beyond the human perceptual range, and night vision. For example, we perceive the landscape primarily in the visual domain, but soundscape (Pijanowski et al., 2011; Van Oosterom et al., 2016) and smellscape (Wallraff and Andreae, 2000; Nevitt, 2008; Gagliardo et al., 2013; Henshaw, 2013; Buehlmann et al., 2015; Ackels et al., 2021) may be as or more important for many species. Particularly promising is the likely possibility that many animals perceive a rich scene of long-lasting olfactory cues, which inform them about the location, condition, age and sex of conspecifics, competitors and predators. Second, enlightening information gathered from GPS-collard animals has inspired increased interest in the spatial information that animals learn about, remember and employ to guide their movement. The GPS data, however, are merely observations on individuals’ locations over time. Hence they must be supplemented with controlled field studies that critically test for the spatial learning and memory of species of interest. Such work may modify protocols previously employed for critical tests of spatial memory in the field, such as the one detailed in Figure 1 (Edwards et al., 1996). Third, a few studies indicate that the large-scale spatial structure of animal movement affects species at other trophic levels through competition, predation, herbivory, pollination and seed dispersal (e.g., Dukas, 2005; Kohl et al., 2018). We think that further research on the effect of the movement patterns of one species on other species can be highly illuminating. Fourth, understandingly, a large share of movement ecology research has been devoted to large mammals. However, we have little experimental data on the mechanisms underlying seasonal migration in these species. For example, do they rely on all three compasses as birds do? Fifth, mechanisms of time keeping both within day and throughout the year have received significant attention in both animal cognition and physiology (Gallistel, 1989; Shettleworth, 2009; Kumar et al., 2010). While we know that animals possess excellent biological clocks that guide their short and long term movement decisions, we know less about how animals time their revisits to familiar places within and between days and years (Janmaat et al., 2013, 2014; Berger-Tal and Bar-David, 2015). Finally, there is growing appreciation that non-humans show long-term improvements in performance similar to those studied in human experts (see Expertise and Movement section above). Mechanisms contributing to the superior performance of experts include greater working and long-term memory, better allocation of attention among co-occurring tasks, and quicker and refined decisions (Dukas, 2019). Decisions regarding movement can benefit from expertise and should be subjected to future research. Similarly, evidence from humans indicates that people working together for a long time develop group expertise primarily owing to enhanced social dynamics (Argote and Epple, 1990; Tindale and Winget, 2017). Similar group expertise likely occurs in animal groups and probably contributes to superior movement performance.
Author Contributions
All authors listed have made a substantial, direct and intellectual contribution to the work, and approved it for publication.
Funding
Our research has been supported by the Natural Sciences and Engineering Research Council of Canada, Canada Foundation for Innovation, and Ontario Ministry of Research and Innovation, Utah Agricultural Experimental Station, and Utah State University’s Ecology Center.
Conflict of Interest
The authors declare that the research was conducted in the absence of any commercial or financial relationships that could be construed as a potential conflict of interest.
Publisher’s Note
All claims expressed in this article are solely those of the authors and do not necessarily represent those of their affiliated organizations, or those of the publisher, the editors and the reviewers. Any product that may be evaluated in this article, or claim that may be made by its manufacturer, is not guaranteed or endorsed by the publisher.
Acknowledgments
We thank L. Dukas for assistance, LR-L and two referees for thoughtful comments on the manuscript, and the Natural Sciences and Engineering Research Council of Canada for funding.
References
Able, K. P. (2001). The concepts and terminology of bird navigation. J. Avian Biol. 32, 174–183. doi: 10.1034/j.1600-048X.2001.320211.x
Able, K. P., and Able, M. A. (1990). Calibration of the magnetic compass of a migratory bird by celestial rotation. Nature 347, 378–380. doi: 10.1038/347378a0
Abrahms, B., Aikens, E. O., Armstrong, J. B., Deacy, W. W., Kauffman, M. J., and Merkle, J. A. (2021). Emerging perspectives on resource tracking and animal movement ecology. Trends Ecol. Evol. 26, 308–320. doi: 10.1016/j.tree.2020.10.018
Ackels, T., Erskine, A., Dasgupta, D., Marin, A. C., Warner, T. P. A., Tootoonian, S., et al. (2021). Fast odour dynamics are encoded in the olfactory system and guide behaviour. Nature 593, 558–563. doi: 10.1038/s41586-021-03514-2
Adler, J. (1976). The sensing of chemicals by bacteria. Sci. Am. 234, 40–47. doi: 10.1038/scientificamerican0476-40
Agostini, N. (2004). Additional observations of age-dependent migration behaviour in western honey buzzards Pernis apivorus. J. Avian Biol. 35, 469–470. doi: 10.1111/j.0908-8857.2004.03450.x
Åkesson, S., Ilieva, M., Karagicheva, J., Rakhimberdiev, E., Tomotani, B., and Helm, B. (2017). Timing avian long-distance migration: from internal clock mechanisms to global flights. Philos. Trans. R. Soc. B Biol. Sci. 372:20160252. doi: 10.1098/rstb.2016.0252
Alerstam, T., Gudmundsson, G. A., Green, M., and Hedenström, A. (2001). Migration along orthodromic sun compass routes by arctic birds. Science 291, 300–303. doi: 10.1126/science.291.5502.300
Alert, B., Michalik, A., Helduser, S., Mouritsen, H., and Güntürkün, O. (2015). Perceptual strategies of pigeons to detect a rotational centre—a hint for star compass learning? PLoS One 10:e0119919. doi: 10.1371/journal.pone.0119919
Allee, W. C. (1931). Animal Aggregations. A Study in General Sociology. Chicago: University of Chicago Press.
Andel, D., and Wehner, R. (2004). Path integration in desert ants, Cataglyphis: how to make a homing ant run away from home. Proc. R. Soc. B Biol. Sci. 271, 1485–1489. doi: 10.1098/rspb.2004.2749
Argote, L., and Epple, D. (1990). Learning curves in manufacturing. Science 247, 920–924. doi: 10.1126/science.247.4945.920
Armitage, K. B. (1965). Notes on the biology of Philanthus bicinctus (Hymenoptera: Sphecidae). J. Kansas Entomol. Soc. 38, 89–100. doi: 10.1111/j.1439-0310.1978.tb01825.x
Arnold, G. W. (1977). An analysis of spatial leadership in a small field in a small flock of sheep. Appl. Anim. Ethol. 3, 263–270. doi: 10.1016/0304-3762(77)90007-4
Ashmole, N. P., and Tovar, H. (1968). Prolonged parental care in royal terns and other birds. Auk 85, 90–100. doi: 10.2307/4083627
Avgar, T., Baker, J. A., Brown, G. S., Hagens, J. S., Kittle, A. M., Mallon, E. E., et al. (2015). Space-use behaviour of woodland caribou based on a cognitive movement model. J. Anim. Ecol. 84, 1059–1070. doi: 10.1111/1365-2656.12357
Avgar, T., Deardon, R., and Fryxell, J. M. (2013). An empirically parameterized individual based model of animal movement, perception, and memory. Ecol. Model. 251, 158–172. doi: 10.1016/j.ecolmodel.2012.12.002
Avgar, T., Street, G., and Fryxell, J. M. (2014). On the adaptive benefits of mammal migration. Can. J. Zool. 92, 481–490. doi: 10.1139/cjz-2013-0076
Baguette, M., Stevens, V. M., and Clobert, J. (2014). The pros and cons of applying the movement ecology paradigm for studying animal dispersal. Mov. Ecol. 2, 1–13.
Balda, R. P., and Kamil, A. C. (1992). Long-term spatial memory in Clark’s nutcracker, Nucifraga columbiana. Anim. Behav. 44, 761–769. doi: 10.1016/s0003-3472(05)80302-1
Bauer, S., Nolet, B. A., Giske, J., Chapman, J. W., Åkesson, S., Hedenström, A., et al. (2011). Cues and decision rules in animal migration. Anim. Migr. Synth. 68:87.
Beauchamp, G. (1999). The evolution of communal roosting in birds: origin and secondary losses. Behav. Ecol. 10, 675–687. doi: 10.1093/beheco/10.6.675
Beck, W., and Wiltschko, W. (1988). Magnetic factors control the migratory direction of pied flycatchers (Ficedula hypoleuca Pallas). Acta XIX Congr. Int. Ornithol. 2, 1955–1962.
Beekman, M., and Ratnieks, F. L. W. (2000). Long-range foraging by the honey-bee, Apis mellifera L. Funct. Ecol. 14, 490–496. doi: 10.1046/j.1365-2435.2000.00443.x
Begall, S., Malkemper, E. P., Červený, J., Němec, P., and Burda, H. (2013). Magnetic alignment in mammals and other animals. Mamm. Biol. 78, 10–20. doi: 10.1016/j.mambio.2012.05.005
Berdahl, A. M., Kao, A. B., Flack, A., Westley, P. A. H., Codling, E. A., Couzin, I. D., et al. (2018). Collective animal navigation and migratory culture: from theoretical models to empirical evidence. bioRxiv [Preprint] doi: 10.1101/230219
Berdahl, A., Torney, C. J., Ioannou, C. C., Faria, J. J., and Couzin, I. D. (2013). Emergent sensing of complex environments by mobile animal groups. Science 339, 574–576. doi: 10.1126/science.1225883
Berger-Tal, O., and Bar-David, S. (2015). Recursive movement patterns: review and synthesis across species. Ecosphere 6, 1–12.
Bergman, G., and Donner, K. (1964). An Analysis of the Spring Migration of the Common Scoter and the Long-Tailed Duck in Southern Finland. Helsinki: University of Helsinki.
Berthold, P., and Querner, U. (1981). Genetic basis of migratory behavior in European warblers. Adv. Sci. 212, 77–79. doi: 10.1126/science.212.4490.77
Bidder, O. R., Walker, J. S., Jones, M. W., Holton, M. D., Urge, P., Scantlebury, D. M., et al. (2015). Step by step: reconstruction of terrestrial animal movement paths by dead-reckoning. Mov. Ecol. 3, 1–16. doi: 10.1186/s40462-015-0055-4
Bingman, V. P., and Cheng, K. (2005). Mechanisms of animal global navigation: comparative perspectives and enduring challenges. Ethol. Ecol. Evol. 17, 295–318. doi: 10.1080/08927014.2005.9522584
Biro, D., Freeman, R., Meade, J., Roberts, S., and Guilford, T. (2007). Pigeons combine compass and landmark guidance in familiar route navigation. Proc. Natl. Acad. Sci. U.S.A. 104, 7471–7476. doi: 10.1073/pnas.0701575104
Biro, D., Meade, J., and Guilford, T. (2004). Familiar route loyalty implies visual pilotage in the homing pigeon. Proc. Natl. Acad. Sci. U.S.A. 101, 17440–17443. doi: 10.1073/pnas.0406984101
Biro, D., Sumpter, D. J. T., Meade, J., and Guilford, T. (2006). From compromise to leadership in pigeon homing. Curr. Biol. 16, 2123–2128. doi: 10.1016/j.cub.2006.08.087
Black, J. M. (1988). Preflight signalling in swans: a mechanism for group cohesion and flock formation. Ethology 79, 143–157. doi: 10.1111/j.1439-0310.1988.tb00707.x
Blaser, N., Dell’Omo, G., Dell’Ariccia, G., Wolfer, D. P., and Lipp, H. P. (2013). Testing cognitive navigation in unknown territories: homing pigeons choose different targets. J. Exp. Biol. 216, 3123–3131. doi: 10.1242/jeb.083246
Boinski, S., and Campbell, A. F. (1995). Use of trill vocalizations to coordinate troop movement among white-faced capuchins. Behaviour 132, 875–901. doi: 10.1163/156853995x00054
Boles, L. C., and Lohmann, K. J. (2003). True navigation and magnetic maps in spiny lobsters. Nature 421, 60–63. doi: 10.1038/nature01226
Bousquet, C. A. H., Sumpter, D. J. T., and Manser, M. B. (2011). Moving calls: a vocal mechanism underlying quorum decisions in cohesive groups. Proc. R. Soc. B Biol. Sci. 278, 1482–1488. doi: 10.1098/rspb.2010.1739
Brent, L. J. N., Franks, D. W., Foster, E. A., Balcomb, K. C., Cant, M. A., and Croft, D. P. (2015). Ecological knowledge, leadership, and the evolution of menopause in killer whales. Curr. Biol. 25, 746–750. doi: 10.1016/j.cub.2015.01.037
Brown, C. R. (1986). Cliff swallow colonies as information centers. Science 234, 83–85. doi: 10.1126/science.234.4772.83
Buehlmann, C., Graham, P., Hansson, B. S., and Knaden, M. (2015). Desert ants use olfactory scenes for navigation. Anim. Behav. 106, 99–105. doi: 10.1016/j.anbehav.2015.04.029
Camacho, C., Canal, D., and Potti, J. (2016). Natal habitat imprinting counteracts the diversifying effects of phenotype-dependent dispersal in a spatially structured population. BMC Evol. Biol. 16:158. doi: 10.1186/s12862-016-0724-y
Caro, T. M. (1994). Cheetahs of the Serengeti Plains: Group Living in an Asocial Species. Chicago: University of Chicago Press.
Cartwright, B. A., and Collett, T. S. (1982). How honey bees use landmarks to guide their return to a food source. Nature 295, 560–564. doi: 10.1038/295560a0
Cederlund, G., Sandegren, F., and Larsson, K. (1987). Summer movements of female moose and dispersal of their offspring. J. Wildl. Manage. 51, 342–352. doi: 10.2307/3801014
Červený, J., Burda, H., Ježek, M., Kušta, T., Husinec, V., Nováková, P., et al. (2017). Magnetic alignment in warthogs Phacochoerus africanus and wild boars Sus scrofa. Mamm. Rev. 47, 1–5. doi: 10.1111/mam.12077
Chance, M. R. A. (1967). Attention structure as the basis of primate rank orders author. Man 2, 503–518. doi: 10.2307/2799336
Chernetsov, N. (2017). Compass systems. J. Comp. Physiol. A Neuroethol. Sens. Neural Behav. Physiol. 203, 447–453. doi: 10.1007/s00359-016-1140-x
Chernetsov, N., Berthold, P., and Querner, U. (2004). Migratory orientation of first-year white storks (Ciconia ciconia): inherited information and social interactions. J. Exp. Biol. 207, 937–943. doi: 10.1242/jeb.00853
Chernetsov, N., Pakhomov, A., Kobylkov, D., Kishkinev, D., Holland, R. A., and Mouritsen, H. (2017). Migratory Eurasian reed warblers can use magnetic declination to solve the longitude problem. Curr. Biol. 27, 2647.e–2651.e. doi: 10.1016/j.cub.2017.07.024
Clutton-Brock, T. H. (1988). Reproductive Success: Studies of Individual Variation in Contrasting Breeding Systems. Chicago: University of Chicago Press.
Cochran, W. W., Mouritsen, H., and Wikelski, M. (2004). Migrating songbirds recalibrate their magnetic compass daily from twilight cues. Science 304, 405–408. doi: 10.1126/science.1095844
Codling, E. A., and Bode, N. W. F. (2014). Copycat dynamics in leaderless animal group navigation. Mov. Ecol. 2, 1–11. doi: 10.1186/2051-3933-2-11
Collett, T. S., and Collett, M. (2002). Memory use in insect visual navigation. Nat. Rev. Neurosci. 3, 542–552. doi: 10.1038/nrn872
Collett, T. S., Cartwright, B. A., and Smith, B. A. (1986). Landmark learning and visuo-spartial memories in gerbils. J. Comp. Physiol. A Sens. Neural Behav. Physiol. 158, 835–851. doi: 10.1007/bf01324825
Collett, T. S., Dillmann, E., Giger, A., and Wehner, R. (1992). Visual landmarks and route following in desert ants. J. Comp. Physiol. A 170, 435–442. doi: 10.1007/BF00191460
Couzin, I. D. (2018). Collective animal migration. Curr. Biol. 28, R976–R980. doi: 10.1016/j.cub.2018.04.044
Couzin, I. D., Krause, J., Franks, N. R., and Levin, S. A. (2005). Effective leadership and decision-making in animal groups on the move. Nature 433, 513–516. doi: 10.1038/nature03236
Danchin, E., and Richner, H. (2001). Viable and unviable hypotheses for the evolution of raven roosts. Anim. Behav. 61, F7–F11. doi: 10.1006/anbe.2000.1577
Danchin, E., Giraldeau, L. A., Valone, T. J., and Wagner, R. H. (2004). Public information: from nosy neighbors to cultural evolution. Science 305, 487–491. doi: 10.1126/science.1098254
Daunt, F., Afanasyev, V., Adam, A., Croxall, J. P., and Wanless, S. (2007a). From cradle to early grave: juvenile mortality in European shags Phalacrocorax aristotelis results from inadequate development of foraging proficiency. Biol. Lett. 3, 371–374. doi: 10.1098/rsbl.2007.0157
Daunt, F., Wanless, S., Harris, M. P., Money, L., and Monaghan, P. (2007b). Older and wiser: improvements in breeding success are linked to better foraging performance in European shags. Funct. Ecol. 21, 561–567. doi: 10.1111/j.1365-2435.2007.01260.x
Dell’Ariccia, G., Dell’Omo, G., Wolfer, D. P., and Lipp, H. P. (2008). Flock flying improves pigeons’ homing: GPS track analysis of individual flyers versus small groups. Anim. Behav. 76, 1165–1172. doi: 10.1016/j.anbehav.2008.05.022
Deutschlander, M. E., Phillips, J. B., and Munro, U. (2012). Age-dependent orientation to magnetically-simulated geographic displacements in migratory Australian Silvereyes (Zosterops l. lateralis). Wilson J. Ornithol. 124, 467–477. doi: 10.1676/11-043.1
Di Fiore, A., and Suarez, S. A. (2007). Route-based travel and shared routes in sympatric spider and woolly monkeys: cognitive and evolutionary implications. Anim. Cogn. 10, 317–329.
Dukas, R. (1998). Cognitive Ecology: The Evolutionary Ecology of Information Processing and Decision Making. Chicago: University of Chicago Press.
Dukas, R. (2004). Evolutionary biology of animal cognition. Annu. Rev. Ecol. Evol. Syst. 35, 347–374.
Dukas, R. (2005). Bumblebee predators reduce bumblebee density and plant fitness. Ecology 86, 1401–1406. doi: 10.1890/04-1663
Dukas, R. (2008a). Evolutionary biology of insect learning. Annu. Rev. Entomol. 53, 145–160. doi: 10.1146/annurev.ento.53.103106.093343
Dukas, R. (2008b). Life history of learning: performance curves of honeybees in the wild. Ethology 114, 1195–1200. doi: 10.1111/j.1439-0310.2008.01565.x
Dukas, R. (2008c). Life history of learning – short and long term performance curves of honeybees in settings that minimize the role of learning. Anim. Behav. 75, 1125–1130. doi: 10.1016/j.anbehav.2007.08.029
Dukas, R. (2010). “Insect social learning,” in Encyclopedia of Animal Behavior, eds M. Breed and J. Moore (Oxford: Academic Press), 176–179. doi: 10.1016/b978-0-08-045337-8.00058-9
Dukas, R. (2017). Cognitive innovations and the evolutionary biology of expertise. Philos. Trans. R. Soc. Lond. B 372:20160427. doi: 10.1098/rstb.2016.0427
Dukas, R. (2019). Animal expertise: mechanisms, ecology and evolution. Anim. Behav. 147, 199–210. doi: 10.1016/j.anbehav.2018.05.010
Dukas, R., and Edelstein-Keshet, L. (1998). The spatial distribution of colonial food provisioners. J. Theor. Biol. 190, 121–134. doi: 10.1006/jtbi.1997.0530
Dukas, R., and Visscher, P. K. (1994). Lifetime learning by foraging honey bees. Anim. Behav. 48, 1007–1012. doi: 10.1006/anbe.1994.1333
Durisko, Z., and Dukas, R. (2013). Attraction to and learning from social cues in fruit fly larvae. Proc. R. Soc. Lond. B Biol. Sci. 280:20131398. doi: 10.1098/rspb.2013.1398
Dyer, F. C. (1998). “Cognitive ecology of navigation,” in Cognitive Ecology, ed. R. Dukas (Chicago: University of Chicago Press), 201–260.
Edwards, G. R., Newman, J. A., Parsons, A. J., and Krebs, J. R. (1996). The use of spatial memory by grazing animals to locate food patches in spatially heterogeneous environments: an example with sheep. Appl. Anim. Behav. Sci. 50, 147–160. doi: 10.1016/0168-1591(96)01077-5
Elbroch, L. M., Levy, M., Lubell, M., Quigley, H., and Caragiulo, A. (2017). Adaptive social strategies in a solitary carnivore. Sci. Adv. 3:e1701218. doi: 10.1126/sciadv.1701218
Emlen, S. T. (1970). Celestial rotation: its importance in the development of migratory orientation. Science 170, 1198–1201.
Emlen, S. T. (1972). The ontogenetic development of orientation capabilities. NASA Special Publication 162, 191–210.
Ericsson, K. A., and Lehmann, A. C. (1996). Expert and exceptional performance: evidence of maximal adaptation to task constraints. Annu. Rev. Psychol. 47, 273–305. doi: 10.1146/annurev.psych.47.1.273
Ericsson, K. A., Charness, N., Feltovich, P. J., and Hoffman, R. R. (2006). The Cambridge Handbook of Expertise and Expert Performance. Cambridge: Cambridge University Press.
Evans, J. C., Votier, S. C., and Dall, S. R. X. (2016). Information use in colonial living. Biol. Rev. 91, 658–672. doi: 10.1111/brv.12188
Fagan, W. F., Gurarie, E., Bewick, S., Howard, A., Cantrell, R. S., and Cosner, C. (2017). Perceptual ranges, information gathering, and foraging success in dynamic landscapes. Am. Nat. 189, 474–489. doi: 10.1086/691099
Fagan, W. F., Lewis, M. A., Auger-Méthé, M., Avgar, T., Benhamou, S., Breed, G., et al. (2013). Spatial memory and animal movement. Ecol. Lett. 16, 1316–1329. doi: 10.1111/ele.12165
Fenton, M. B., and Simmons, N. B. (2015). Bats: A World of Science and Mystery. Chicago: University of Chicago Press.
Ferrari, E., Veschi, M., Guerzoni, V., Bergamini, L., Neviani, F., Manni, B., et al. (2009). A scale evaluating the emotivity and well-being of elderly (Italian acronym: SVEBA): analysis of psychometric characteristics. Arch. Gerontol. Geriatr. 49, 77–81. doi: 10.1016/j.archger.2009.09.015
Filannino, C., Armstrong, C., Guilford, T., and Gagliardo, A. (2014). Individual strategies and release site features determine the extent of deviation in clock-shifted pigeons at familiar sites. Anim. Cogn. 17, 33–43. doi: 10.1007/s10071-013-0635-x
Finn, K. T. (2021). Potential use of a magnetic compass during long-distance dispersal in a subterranean rodent. J. Mammal. 102(Pt 23), 250–257. doi: 10.1093/jmammal/gyaa163
Flack, A., Ákos, Z., Nagy, M., Vicsek, T., and Biro, D. (2013). Robustness of flight leadership relations in pigeons. Anim. Behav. 86, 723–732. doi: 10.1016/j.anbehav.2013.07.005
Flack, A., Nagy, M., Fiedler, W., Couzin, I. D., and Wikelski, M. (2018). From local collective behavior to global migratory patterns in white storks. Science 914, 911–914. doi: 10.1126/science.aap7781
Flack, A., Pettit, B., Freeman, R., Guilford, T., and Biro, D. (2012). What are leaders made of? The role of individual experience in determining leader-follower relations in homing pigeons. Anim. Behav. 83, 703–709. doi: 10.1016/j.anbehav.2011.12.018
Foley, C., Pettorelli, N., and Foley, L. (2008). Severe drought and calf survival in elephants. Biol. Lett. 4, 541–544. doi: 10.1098/rsbl.2008.0370
Found, R., and St. Clair, C. C. (2016). Behavioural syndromes predict loss of migration in wild elk. Anim. Behav. 115, 35–46. doi: 10.1016/j.anbehav.2016.02.007
Found, R., and St. Clair, C. C. (2019). Influences of personality on ungulate migration and management. Front. Ecol. Evol. 7:438. doi: 10.3389/fevo.2019.00438
Fransson, T., Jakobsson, S., Johansson, P., Kullberg, C., Lind, J., and Vallin, A. (2001). Bird migration: magnetic cues trigger extensive refuelling. Nature 414, 35–36. doi: 10.1038/35102115
Freake, M. J., Muheim, R., and Phillips, J. B. (2006). Magnetic maps in animals: a theory comes of age? Q. Rev. Biol. 81, 327–347. doi: 10.1086/511528
Freeman, R., Mann, R., Guilford, T., and Biro, D. (2011). Group decisions and individual differences: route fidelity predicts flight leadership in homing pigeons (Columba livia). Biol. Lett. 7, 63–66. doi: 10.1098/rsbl.2010.0627
Gagliardo, A., Bried, J., Lambardi, P., Luschi, P., Wikelski, M., and Bonadonna, F. (2013). Oceanic navigation in Cory’s shearwaters: evidence for a crucial role of olfactory cues for homing after displacement. J. Exp. Biol. 216, 2798–2805. doi: 10.1242/jeb.085738
Galef, B. G., and Giraldeau, L. A. (2001). Social influences on foraging in vertebrates: causal mechanisms and adaptive functions. Anim. Behav. 61, 3–15. doi: 10.1006/anbe.2000.1557
Gallistel, C. R. (1989). Animal cognition: the representation of space, time and number. Annu. Rev. Psychol. 40, 155–189. doi: 10.1146/annurev.ps.40.020189.001103
Garland, J., Berdahl, A. M., Sun, J., and Bollt, E. M. (2018). Anatomy of leadership in collective behaviour. Chaos 28:075308. doi: 10.1063/1.5024395
Gosling, S. D., and John, O. P. (1999). Personality dimensions in nonhuman animals: a cross-species review. Curr. Dir. Psychol. Sci. 8, 69–75. doi: 10.1111/1467-8721.00017
Green, S. J., Boruff, B. J., Bonnell, T. R., and Grueter, C. C. (2020). Chimpanzees use least-cost routes to out-of-sight goals. Curr. Biol. 30, 4528.e–4533.e. doi: 10.1016/j.cub.2020.08.076
Greenwood, P. J. (1980). Mating systems, philopatry and dispersal in birds and mammals. Anim. Behav. 28, 1140–1162. doi: 10.1016/s0003-3472(80)80103-5
Greenwood, P. J., and Harvey, P. H. (1982). The natal and breeding dispersal of birds. Annu. Rev. Ecol. Syst. 13, 1–21. doi: 10.1146/annurev.es.13.110182.000245
Grünbaum, D. (1998). Schooling as a strategy for taxis in a noisy environment. Evol. Ecol. 12, 503–522.
Guilford, T., and Biro, D. (2014). Route following and the pigeon’s familiar area map. J. Exp. Biol. 217, 169–179. doi: 10.1242/jeb.092908
Guilford, T., and Taylor, G. K. (2014). The sun compass revisited. Anim. Behav. 97, 135–143. doi: 10.1016/j.anbehav.2014.09.005
Guttal, V., and Couzin, I. D. (2010). Social interactions, information use, and the evolution of collective migration. Proc. Natl. Acad. Sci. U.S.A. 107, 16172–16177. doi: 10.1073/pnas.1006874107
Gwinner, E. (1996). Circadian and circannual programmes in avian migration. J. Exp. Biol. 199, 39–48. doi: 10.1242/jeb.199.1.39
Hamilton, W. J. (1967). “Social aspects of bird orientation mechanisms,” in Animal Orientation and Navigation, ed. R. M. Storm (Corvallis, OR: Oregon State University Press), 57–71.
Harel, R., Horvitz, N., and Nathan, R. (2016). Adult vultures outperform juveniles in challenging thermal soaring conditions. Sci. Rep. 6:27865. doi: 10.1038/srep27865
Harel, R., Spiegel, O., Getz, W. M., and Nathan, R. (2017). Social foraging and individual consistency in following behaviour: testing the information centre hypothesis in free-ranging vultures. Proc. R. Soc. B Biol. Sci. 284:20162654. doi: 10.1098/rspb.2016.2654
Harrison, X. A., Tregenza, T., Inger, R., Colhoun, K., Dawson, D. A., Gudmundsson, G. A., et al. (2010). Cultural inheritance drives site fidelity and migratory connectivity in a long-distance migrant. Mol. Ecol. 19, 5484–5496. doi: 10.1111/j.1365-294X.2010.04852.x
Hein, A. M., Rosenthal, S. B., Hagstrom, G. I., Berdahl, A., Torney, C. J., and Couzin, I. D. (2015). The evolution of distributed sensing and collective computation in animal populations. eLife 4:e10955. doi: 10.7554/eLife.10955
Heinsohn, R. G. (1991). Slow learning of foraging skills and extended parental care in cooperatively breeding white-winged choughs. Am. Nat. 137, 864–881. doi: 10.1086/285198
Helbig, A. J. (1991). Inheritance of migratory direction in a bird species: a cross-breeding experiment with SE- and SW-migrating blackcaps (Sylvia atricapilla). Behav. Ecol. Sociobiol. 28, 9–12. doi: 10.1007/BF00172133
Henshaw, V. (2013). Urban Smellscapes: Understanding and Designing City Smell Environments. Abingdon-on-Thames: Taylor & Francis.
Herbert-Read, J. E., Perna, A., Mann, R. P., Schaerf, T. M., Sumpter, D. J. T., and Ward, A. J. W. (2011). Inferring the rules of interaction of shoaling fish. Proc. Natl. Acad. Sci. U.S.A. 108, 18726–18731. doi: 10.1073/pnas.1109355108
Holland, R. A., and Helm, B. (2013). A strong magnetic pulse affects the precision of departure direction of naturally migrating adult but not juvenile birds. J. R. Soc. Interface 10:20121047. doi: 10.1098/rsif.2012.1047
Holland, R. A., Borissov, I., and Siemers, B. M. (2010). A nocturnal mammal, the greater mouse-eared bat, calibrates a magnetic compass by the sun. Proc. Natl. Acad. Sci. U.S.A. 107, 6941–6945. doi: 10.1073/pnas.0912477107
Holland, R., Thorup, K., Vonhof, M. J., Cochran, W. W., and Wikelski, M. (2006). Bat orientation using Earth’s magnetic field. Nature 444, 702–702. doi: 10.1038/444702a
Hoover, J. P. (2003). Decision rules for site fidelity in a migratory bird, the prothonotary warbler. Ecology 84, 416–430. doi: 10.1890/0012-9658(2003)084[0416:drfsfi]2.0.co;2
Hunt, G. R., Holzhaider, J. C., and Gray, R. D. (2012). Prolonged parental feeding in tool-using New Caledonian crows. Ethology 118, 423–430. doi: 10.1111/j.1439-0310.2012.02027.x
Ioalé, P. (1984). Magnets and pigeon orientation. Monit. Zool. Ital. Ital. J. Zool. 18, 347–358. doi: 10.1080/00269786.1984.10736466
Jakopak, R. P., LaSharr, T. N., Dwinnell, S. P. H., Fralick, G. L., and Monteith, K. L. (2019). Rapid acquisition of memory in a complex landscape by a mule deer. Ecology 100:e02854. doi: 10.1002/ecy.2854
Janmaat, K. R. L., Ban, S. D., and Boesch, C. (2013). Chimpanzees use long-term spatial memory to monitor large fruit trees and remember feeding experiences across seasons. Anim. Behav. 86, 1183–1205. doi: 10.1016/j.anbehav.2013.09.021
Janmaat, K. R. L., Polansky, L., Ban, S. D., and Boesch, C. (2014). Wild chimpanzees plan their breakfast time, type, and location. Proc. Natl. Acad. Sci. U.S.A. 111, 16343–16348. doi: 10.1073/pnas.1407524111
Jesmer, B. R., Merkle, J. A., Goheen, J. R., Aikens, E. O., Beck, J. L., Courtemanch, A. B., et al. (2018). Is ungulate migration culturally transmitted? Evidence of social learning from translocated animals. Science 361, 1023–1025. doi: 10.1126/science.aat0985
Johnsen, S., Lohmann, K. J., and Warrant, E. J. (2020). Animal navigation: a noisy magnetic sense? J. Exp. Biol. 223, 1–7. doi: 10.1242/jeb.164921
Keeton, W. T. (1971). Magnets interfere with pigeon homing. Proc. Natl. Acad. Sci. U.S.A. 68, 102–106. doi: 10.1073/pnas.68.1.102
Keeton, W. T. (1974). “The orientational and navigational basis of homing in birds,” in Advances in the Study of Behavior, eds D. S. Lehrman, J. S. Rosenblatt, R. A. Hinde, and E. Shaw (Cambridge, MA: Academic Press), 47–132. doi: 10.1016/S0065-3454(08)60020-0
Kimchi, T., Etienne, A. S., and Terkel, J. (2004). A subterranean mammal uses the magnetic compass for path integration. Proc. Natl. Acad. Sci. U.S.A. 101, 1105–1109. doi: 10.1073/pnas.0307560100
King, A. J., Douglas, C. M. S., Huchard, E., Isaac, N. J. B., and Cowlishaw, G. (2008). Dominance and affiliation mediate despotism in a social primate. Curr. Biol. 18, 1833–1838. doi: 10.1016/j.cub.2008.10.048
King, A. J., Sueur, C., Huchard, E., and Cowlishaw, G. (2011). A rule-of-thumb based on social affiliation explains collective movements in desert baboons. Anim. Behav. 82, 1337–1345. doi: 10.1016/j.anbehav.2011.09.017
Kishkinev, D., Packmor, F., Zechmeister, T., Winkler, H. C., Chernetsov, N., Mouritsen, H., et al. (2021). Navigation by extrapolation of geomagnetic cues in a migratory songbird. Curr. Biol. 31, 1563–1569. doi: 10.1016/j.cub.2021.01.051
Klatzky, R. L. (1998). “Allocentric and egocentric spatial representations: definitions, distinctions, and interconnections,” in Spatial Cognition, eds C. Freksa, C. Habel, and K. F. Wender (Berlin: Springer), 1–17. doi: 10.1016/B978-0-12-385870-2.00045-7
Kohl, M. T., Stahler, D. R., Metz, M. C., Forester, J. D., Kauffman, M. J., Varley, N., et al. (2018). Diel predator activity drives a dynamic landscape of fear. Ecol. Monogr. 88, 638–652. doi: 10.1002/ecm.1313
Kölzsch, A., Flack, A., Müskens, G. J. D. M., Kruckenberg, H., Glazov, P., and Wikelski, M. (2020). Goose parents lead migration V. J. Avian Biol. 51, 1–8. doi: 10.1111/jav.02392
Krebs, J. R., MacRoberts, M. H., and Cullen, J. M. (1972). Flocking and feeding in the great tit Parus major–an experimental study. Ibis 114, 507–530. doi: 10.1111/j.1474-919x.1972.tb00852.x
Kumar, V., Wingfield, J. C., Dawson, A., Ramenofsky, M., Rani, S., and Bartell, P. (2010). Biological clocks and regulation of seasonal reproduction and migration in birds. Physiol. Biochem. Zool. 83, 827–835. doi: 10.1086/652243
Kummer, H. (1968). Social Organization of Hamadryas Baboons; A Field Study. Chicago: University of Chicago Press.
Kurvers, R. H. J. M., Eijkelenkamp, B., van Oers, K., van Lith, B., van Wieren, S. E., Ydenberg, R. C., et al. (2009). Personality differences explain leadership in barnacle geese. Anim. Behav. 78, 447–453. doi: 10.1016/j.anbehav.2009.06.002
Leach, A. G., and Sedinger, J. S. (2016). Male breeding experience, not mate familiarity, affects reproductive output in black brant geese. Behav. Ecol. 27, 1851–1858. doi: 10.1093/beheco/arw122
Lewis, M., Fagan, W., Auger-Methe, M., Frair, J., Fryxell, J., Gros, C., et al. (2021). Learning and animal movement. Front. Ecol. Evol. 9:681704. doi: 10.22541/au.159285496.64901842
Lohmann, K. J., Cain, S. D., Dodge, S. A., and Lohmann, C. M. F. (2001). Regional magnetic fields as navigational markers for sea turtles. Science 294, 364–366. doi: 10.1126/science.1064557
Lusseau, D., and Conradt, L. (2009). The emergence of unshared consensus decisions in bottlenose dolphins. Behav. Ecol. Sociobiol. 63, 1067–1077. doi: 10.1007/s00265-009-0740-7
Marzluff, J. M., Heinrich, B., and Marzluff, C. S. (1996). Raven roosts are mobile information centres. Anim. Behav. 51, 89–103. doi: 10.1006/anbe.1996.0008
McComb, K., Moss, C., Durant, S. M., Baker, L., and Sayialel, S. (2001). Matriarchs as repositories of social knowledge in African elephants. Science 292, 491–494. doi: 10.1126/science.1057895
McComb, K., Shannon, G., Durant, S. M., Sayialel, K., Slotow, R., Poole, J., et al. (2011). Leadership in elephants: the adaptive value of age. Proc. R. Soc. B Biol. Sci. 278, 3270–3276. doi: 10.1098/rspb.2011.0168
Mellone, U., Lucia, G., Mallìa, E., and Urios, V. (2016). Individual variation in orientation promotes a 3000-km latitudinal change in wintering grounds in a long-distance migratory raptor. Ibis 158, 887–893. doi: 10.1111/ibi.12401
Menzel, R., Greggers, U., Smith, A., Berger, S., Brandt, R., Brunke, S., et al. (2005). Honey bees navigate according to a map-like spatial memory. Proc. Natl. Acad. Sci. U.S.A. 102, 3040–3045. doi: 10.1073/pnas.0408550102
Michalik, A., Alert, B., Engels, S., Lefeldt, N., and Mouritsen, H. (2014). Star compass learning: how long does it take? J. Ornithol. 155, 225–234. doi: 10.1007/s10336-013-1004-x
Michener, C. D., Lange, R. B., Bigarella, J. J., and Salamuni, R. (1958). Factors influencing the distribution of bees’ nests in earth banks. Ecology 39, 207–217. doi: 10.2307/1931865
Mittelstaedt, H., and Mittelstaedt, M. L. (1982). “Homing by path integration,” in Avian Navigation. Proceedings in Life Sciences, eds F. Papi and H. G. Wallraff (Berlin: Springer), 290–297. doi: 10.1007/978-3-642-68616-0_29
Mock, D. W., Lamey, T. C., and Thompson, D. B. A. (1988). Falsifiability and the information centre hypothesis. Ornis Scand. 19, 231–248. doi: 10.2307/3676564
Morand-Ferron, J., Cole, E. F., and Quinn, J. L. (2016). Studying the evolutionary ecology of cognition in the wild: a review of practical and conceptual challenges. Biol. Rev. 91, 367–389. doi: 10.1111/brv.12174
Morris, R. G. M. (1981). Spatial localization does not require the presence of local cues. Learn. Motiv. 12, 239–260. doi: 10.1016/0023-9690(81)90020-5
Morrison, T. A., Merkle, J. A., Hopcraft, J. G. C., Aikens, E. O., Beck, J. L., Boone, R. B., et al. (2021). Drivers of site fidelity in ungulates. J. Anim. Ecol. 90, 955–966. doi: 10.1111/1365-2656.13425
Moser, E. I., Kropff, E., and Moser, M.-B. (2008). Place cells, grid cells, and the brain’s spatial representation system. Annu. Rev. Neurosci. 31, 69–89. doi: 10.1146/annurev.neuro.31.061307.090723
Mouritsen, H. (2018). Long-distance navigation and magnetoreception in migratory animals. Nature 558, 50–59. doi: 10.1038/s41586-018-0176-1
Mouritsen, H., and Larsen, O. N. (1998). Migrating young pied flycatchers Ficedula hypoleuca do not compensate for geographical displacements. J. Exp. Biol. 201, 2927–2934. doi: 10.1242/jeb.201.21.2927
Mouritsen, H., and Larsen, O. N. (2001). Migrating songbirds tested in computer-controlled Emlen funnels use stellar cues for a time-independent compass. J. Exp. Biol. 204, 3855–3865. doi: 10.1242/jeb.204.22.3855
Mouritsen, H., Heyers, D., and Güntürkün, O. (2016). The neural basis of long-distance navigation in birds. Annu. Rev. Physiol. 78, 133–154. doi: 10.1146/annurev-physiol-021115-105054
Mueller, T., O’Hara, R. B., Converse, S. J., Urbanek, R. P., and Fagan, W. F. (2013). Social learning of migratory performance. Science 341, 999–1002. doi: 10.1126/science.1237139
Muheim, R., Åkesson, S., and Phillips, J. B. (2007). Magnetic compass of migratory Savannah sparrows is calibrated by skylight polarization at sunrise and sunset. J. Ornithol. 148, 485–494. doi: 10.1007/s10336-007-0187-4
Muheim, R., Bäckman, J., and Åkesson, S. (2002). Magnetic compass orientation in European robins is dependent on both wavelength and intensity of light. J. Exp. Biol. 205, 3845–3856. doi: 10.1242/jeb.205.24.3845
Muheim, R., Phillips, J. B., and Deutschlander, M. E. (2009). White-throated sparrows calibrate their magnetic compass by polarized light cues during both autumn and spring migration. J. Exp. Biol. 212, 3466–3472. doi: 10.1242/jeb.032771
Muheim, R., Sjöberg, S., and Pinzon-Rodriguez, A. (2016). Polarized light modulates light-dependent magnetic compass orientation in birds. Proc. Natl. Acad. Sci. U.S.A. 113, 1654–1659. doi: 10.1073/pnas.1513391113
Müller, M., and Wehner, R. (1988). Path integration in desert ants, Cataglyphis fortis. Beyond Cogn. Map 85, 5287–5290. doi: 10.7551/mitpress/1571.003.0008
Munro, U., and Wiltschko, R. (1995). The role of skylight polarization in the orientation of a day-migrating bird species. J. Comp. Physiol. A 177, 357–362. doi: 10.1007/BF00192424
Munro, U., Munro, J. A., Phillips, J. B., Wiltschko, R., and Wiltschko, W. (1997). Evidence for a magnetite-based navigational “map” in birds. Naturwissenschaften 84, 26–28. doi: 10.1007/s001140050343
Mutinda, H., Poole, J. H., and Moss, C. J. (2011). “Decision making and leadership in using the ecosystem,” in The Amboseli Elephants: A Long-Term Perspective on a Long-Lived Mammal, eds C. J. Moss, H. Croze, and P. C. Lee (Chicago: University of Chicago Press), 246–259. doi: 10.7208/chicago/9780226542263.003.0016
Nagy, M., Ákos, Z., Biro, D., and Vicsek, T. (2010). Hierarchical group dynamics in pigeon flocks. Nature 464, 890–893. doi: 10.1038/nature08891
Naisbett-Jones, L. C., Putman, N. F., Stephenson, J. F., Ladak, S., and Young, K. A. (2017). A magnetic map leads Juvenile European Eels to the Gulf stream. Curr. Biol. 27, 1236–1240. doi: 10.1016/j.cub.2017.03.015
Nathan, R., Getz, W. M., Revilla, E., Holyoak, M., Kadmon, R., Saltz, D., et al. (2008). A movement ecology paradigm for unifying organismal movement research. Proc. Natl. Acad. Sci. U.S.A. 105:19052. doi: 10.1073/pnas.0800375105
Nesterova, A. P., Flack, A., van Loon, E. E., Marescot, Y., Bonadonna, F., and Biro, D. (2014). Resolution of navigational conflict in king penguin chicks. Anim. Behav. 93, 221–228. doi: 10.1016/j.anbehav.2014.04.031
Nevitt, G. A. (2008). Sensory ecology on the high seas: the odor world of the procellariiform seabirds. J. Exp. Biol. 211, 1706–1713. doi: 10.1242/jeb.015412
Norton, G. W. III (1986). 6 Leadership: decision processes of group movement in yellow. Primate Ecol. Conserv 2:145.
Noyce, K. V., and Garshelis, D. L. (2014). Follow the leader: social cues help guide landscape-level movements of American black bears (Ursus americanus). Can. J. Zool. 92, 1005–1017. doi: 10.1139/cjz-2014-0029
O’Keefe, J., and Dostrovsky, J. (1971). The hippocampus as a spatial map: preliminary evidence from unit activity in the freely-moving rat. Brain Res. 34, 171–175. doi: 10.1016/0006-8993(71)90358-1
O’Keefe, J., and Nadel, L. (1978). The Hippocampus as a Cognitive Map. Oxford: Clarendon Press. doi: 10.5840/philstudies19802725
Obleser, P., Hart, V., Malkemper, E. P., Begall, S., Holá, M., Painter, M. S., et al. (2016). Compass-controlled escape behavior in roe deer. Behav. Ecol. Sociobiol. 70, 1345–1355. doi: 10.1007/s00265-016-2142-y
Ofstad, T. A., Zuker, C. S., and Reiser, M. B. (2011). Visual place learning in Drosophila melanogaster. Nature 474, 204–207. doi: 10.1038/nature10131
Oliveriusová, L., Nìmec, P., Pavelková, Z., and Sedláček, F. (2014). Spontaneous expression of magnetic compass orientation in an epigeic rodent: the bank vole, Clethrionomys glareolus. Naturwissenschaften 101, 557–563. doi: 10.1007/s00114-014-1192-0
Oliveriusová, L., Pavel, N., and Králová, Z. (2012). Magnetic compass orientation in two strictly subterranean rodents: learned or species-specific innate directional preference? J. Exp. Biol. 215, 3649–3654. doi: 10.1242/jeb.069625
Olsen, G. H. (2001a). Of cranes and men: reintroduction of cranes to a migratory pathway: part I. J. Avian Med. Surg. 15, 133–137. doi: 10.1647/1082-6742(2001)015[0133:ocamro]2.0.co;2
Olsen, G. H. (2001b). Of cranes and men: reintroduction of cranes to a migratory pathway: part II. J. Avian Med. Surg. 15, 316–322. doi: 10.1647/1082-6742(2001)015[0316:ocamro]2.0.co;2
Osborne, J. L., Martin, A. P., Carreck, N. L., Swain, J. L., Knight, M. E., Goulson, D., et al. (2008). Bumblebee flight distances in relation to the forage landscape. J. Anim. Ecol. 77, 406–415. doi: 10.1111/j.1365-2656.2007.01333.x
Painter, M. S., Blanco, J. A., Malkemper, E. P., Anderson, C., Sweeney, D. C., Hewgley, C. W., et al. (2016). Use of bio-loggers to characterize red fox behavior with implications for studies of magnetic alignment responses in free-roaming animals. Anim. Biotelemetry 4, 1–19. doi: 10.1186/s40317-016-0113-8
Pakhomov, A., Anashina, A., and Chernetsov, N. (2017). Further evidence of a time-independent stellar compass in a night-migrating songbird. Behav. Ecol. Sociobiol. 71, 2–7. doi: 10.1007/s00265-017-2279-3
Pakhomov, A., and Chernetsov, N. (2020). A hierarchy of compass systems in migratory birds. Biol. Commun. 65, 262–276. doi: 10.21638/SPBU03.2020.306
Palacín, C., Alonso, J. C., Alonso, J. A., Magaña, M., and Martín, C. A. (2011). Cultural transmission and flexibility of partial migration patterns in a long-lived bird, the great bustard Otis tarda. J. Avian Biol. 42, 301–308. doi: 10.1111/j.1600-048X.2011.05395.x
Perdeck, A. C. (1958). Two types of orientation in migrating starlings, Sturnus vulgaris L., and chaffinches, Fringilla coelebs L., as revealed by displacement experiments. Ardea 46, 1–37. doi: 10.5253/arde.v1i2.p1
Pettit, B., Ákos, Z., Vicsek, T., and Biro, D. (2015). Speed determines leadership and leadership determines learning during pigeon flocking. Curr. Biol. 25, 3132–3137. doi: 10.1016/j.cub.2015.10.044
Pettit, B., Flack, A., Freeman, R., Guilford, T., and Biro, D. (2013a). Not just passengers: pigeons, Columba livia, can learn homing routes while flying with a more experienced conspecific. Proc. R. Soc. B Biol. Sci. 280:20122160. doi: 10.1098/rspb.2012.2160
Pettit, B., Perna, A., Biro, D., and Sumpter, D. J. T. (2013b). Interaction rules underlying group decisions in homing pigeons. J. R. Soc. Interface 10:20130529. doi: 10.1098/rsif.2013.0529
Pijanowski, B. C., Villanueva-Rivera, L. J., Dumyahn, S. L., Farina, A., Krause, B. L., Napoletano, B. M., et al. (2011). Soundscape ecology: the science of sound in the landscape. BioScience 61, 203–216.
Pike, G. C. (1962). Migration and feeding of the gray whale (Eschrichtius gibbosus). J. Fish. Board Canada 19, 815–838. doi: 10.1139/f62-051
Poole, J. H., Payne, K., Langbauer, W. R., and Moss, C. J. (1988). Behavioral ecology and sociobiology contexts of some very low frequency calls of African elephants. Behav. Ecol. Sociobiol. 22, 385–392. doi: 10.1007/bf00294975
Prins, H. (1996). Ecology and Behaviour of the African Buffalo: Social Inequality and Decision Making. Berlin: Springer Science & Business Media.
Puckett, J. G., Pokhrel, A. R., and Giannini, J. A. (2018). Collective gradient sensing in fish schools. Sci. Rep. 8:7587. doi: 10.1038/s41598-018-26037-9
Putman, N. F. (2015). Inherited magnetic maps in salmon and the role of geomagnetic change. Integr. Comp. Biol. 55, 396–405. doi: 10.1093/icb/icv020
Rankin, J. (1965). Notes on the ecology, capture and behaviour in captivity of the elephant shrew. Afr. Zool. 1, 73–80. doi: 10.1080/00445096.1965.11447300
Rasmussen, K., Palacios, D. M., Calambokidis, J., Saborío, M. T., Dalla Rosa, L., Secchi, E. R., et al. (2007). Southern Hemisphere humpback whales wintering off Central America: insights from water temperature into the longest mammalian migration. Biol. Lett. 3, 302–305. doi: 10.1098/rsbl.2007.0067
Ratcliffe, J. M., and Phelps, S. M. (2019). Twenty-five years of cognitive ecology. Anim. Behav. 147, 127–128. doi: 10.1016/j.anbehav.2018.10.017
Rathbun, G. B. (1979). The social structure and ecology of elephant-shrews. Z. Säugetierkd. Suppl. 20, l–76.
Raveling, D. G. (1969). Preflight and flight behavior of Canada geese. Auk 86, 671–681. doi: 10.2307/4083454
Réale, D., Reader, S. M., Sol, D., McDougall, P. T., and Dingemanse, N. J. (2007). Integrating animal temperament within ecology and evolution. Biol. Rev. 82, 291–318. doi: 10.1111/j.1469-185X.2007.00010.x
Reebs, S. G. (2000). Can a minority of informed leaders determine the foraging movements of a fish shoal? Anim. Behav. 59, 403–409. doi: 10.1006/anbe.1999.1314
Riley, J. R., Greggers, U., Smith, A. D., Reynolds, D. R., and Menzel, R. (2005). The flight paths of honeybees recruited by the waggle dance. Nature 435, 205–207. doi: 10.1038/nature03526
Riotte-Lambert, L., and Matthiopoulos, J. (2020). Environmental predictability as a cause and consequence of animal movement. Trends Ecol. Evol. 35, 163–174. doi: 10.1016/j.tree.2019.09.009
Rolland, C., Danchin, E., and de Fraipont, M. (1998). The evolution of coloniality in birds in relation to food, habitat, predation, and life-history traits: a comparative analysis. Am. Nat. 151, 514–529. doi: 10.2307/2463325
Rosenthal, S. B., Twomey, C. R., Hartnett, A. T., Wu, H. S., and Couzin, I. D. (2015). Revealing the hidden networks of interaction in mobile animal groups allows prediction of complex behavioral contagion. Proc. Natl. Acad. Sci. U.S.A. 112, 4690–4695. doi: 10.1073/pnas.1420068112
Sand, H., Wikenros, C., Wabakken, P., and Liberg, O. (2006). Effects of hunting group size, snow depth and age on the success of wolves hunting moose. Anim. Behav. 72, 781–789. doi: 10.1016/j.anbehav.2005.11.030
Sankey, D. W. E., O’Bryan, L. R., Garnier, S., Cowlishaw, G., Hopkins, P., Holton, M., et al. (2021). Consensus of travel direction is achieved by simple copying, not voting, in free-ranging goats. R. Soc. Open Sci. 8:201128. doi: 10.1098/rsos.201128
Sasaki, T., and Biro, D. (2017). Cumulative culture can emerge from collective intelligence in animal groups. Nat. Commun. 8:15049. doi: 10.1038/ncomms15049
Sasaki, T., Mann, R. P., Warren, K. N., Herbert, T., Wilson, T., and Biro, D. (2018). Personality and the collective: bold homing pigeons occupy higher leadership ranks in flocks. Philos. Trans. R. Soc. B Biol. Sci. 373:20170038. doi: 10.1098/rstb.2017.0038
Scanlan, M. M., Putman, N. F., Pollock, A. M., and Noakes, D. L. G. (2018). Magnetic map in nonanadromous Atlantic salmon. Proc. Natl. Acad. Sci. U.S.A. 115, 10995–10999. doi: 10.1073/pnas.1807705115
Schabetsberger, R., Miller, M. J., Dall’Olmo, G., Kaiser, R., Økland, F., Watanabe, S., et al. (2016). Hydrographic features of anguillid spawning areas: potential signposts for migrating eels. Mar. Ecol. Prog. Ser. 554, 141–155. doi: 10.3354/meps11824
Schiffner, I., Fuhrmann, P., and Wiltschko, R. (2011). Tracking pigeons in a magnetic anomaly and in magnetically “quiet” terrain. Naturwissenschaften 98, 575–581. doi: 10.1007/s00114-011-0802-3
Schippers, M. P., Dukas, R., and McClelland, G. B. (2010). Lifetime- and caste-specific changes in flight metabolic rate and muscle biochemistry of honeybees, Apis mellifera. J. Comp. Physiol. B 180, 45–55. doi: 10.1007/s00360-009-0386-9
Schippers, M.-P., Dukas, R., Smith, R. W., Wang, J., Smolen, K., and McClelland, G. B. (2006). Lifetime performance in foraging honeybees: behaviour and physiology. J. Exp. Biol. 209, 3828–3836. doi: 10.1242/jeb.02450
Schmidt-Koenig, K. (1958). Experimentelle Einflußnahme auf die 24-stunden-periodik bei brieftauben und deren auswirkungen unter besonderer berücksichtigung des heimfindevermögens. Z. Tierpsychol. 15, 301–331. doi: 10.1111/j.1439-0310.1958.tb00568.x
Schmidt-Koenig, K. (1990). The sun compass. Experientia 46, 336–342. doi: 10.1016/0016-0032(58)90628-8
Schmidt-Koenig, K., Ganzhorn, J. U., and Ranvaud, R. (1991). “The sun compass,” in Orientation in Birds. Experientia Supplementum, ed. P. Berthold (Basel: Birkhäuser Basel), 1–15. doi: 10.1007/978-3-0348-7208-9_1
Schooley, R. L., and Wiens, J. A. (2003). Finding habitat patches and directional connectivity. Oikos 102, 559–570. doi: 10.1034/j.1600-0706.2003.12490.x
Schüz, E. (1951). Überblick über die orientierungsversuche der vogelwarte rossitten (jetzt: vogelwarte radolfzell). Proc. Int. Ornithol. Congr. 10, 249–268.
Sergio, F., Tanferna, A., De Stephanis, R., Jimenez, L. L., Blas, J., Tavecchia, G., et al. (2014). Individual improvements and selective mortality shape lifelong migratory performance. Nature 515, 410–413. doi: 10.1038/nature13696
Sherry, D. F., Krebs, J. R., and Cowie, R. J. (1981). Memory for the location of stored food in marsh tits. Anim. Behav. 29, 1260–1266. doi: 10.1016/s0003-3472(81)80078-4
Shettleworth, S. J., and Sutton, J. E. (2005). Multiple systems for spatial learning: dead reckoning and beacon homing in rats. J. Exp. Psychol. Anim. Behav. Process. 31, 125–141. doi: 10.1037/0097-7403.31.2.125
Sih, A., Bell, A., and Johnson, J. C. (2004). Behavioral syndromes: an ecological and evolutionary overview. Trends Ecol. Evol. 19, 372–378. doi: 10.1016/j.tree.2004.04.009
Simons, A. M. (2004). Many wrongs: the advantage of group navigation. Trends Ecol. Evol. 19, 453–455. doi: 10.1016/j.tree.2004.07.001
Slagsvold, T., and Wiebe, K. (2007). Learning the ecological niche. Proc. R. Soc. B Biol. Sci. 274, 19–23. doi: 10.1098/rspb.2006.3663
Slagsvold, T., and Wiebe, K. L. (2011). Social learning in birds and its role in shaping a foraging niche. Philos. Trans. R. Soc. B Biol. Sci. 366:969. doi: 10.1098/rstb.2010.0343
Smale, L., Nunes, S., and Holekamp, K. E. (1997). Sexually dimorphic dispersal in mammals: patterns, causes, and consequences. Adv. Study Behav. 26, 181–251. doi: 10.1016/s0065-3454(08)60380-0
Smith, J. E., Gavrilets, S., Mulder, M. B., Hooper, P. L., Mouden, C. E., Nettle, D., et al. (2016). Leadership in mammalian societies: emergence, distribution, power, and payoff. Trends Ecol. Evol. 31, 54–66. doi: 10.1016/j.tree.2015.09.013
Somveille, M., Rodrigues, A. S. L., and Manica, A. (2015). Why do birds migrate? A macroecological perspective. Glob. Ecol. Biogeogr. 24, 664–674. doi: 10.1111/geb.12298
Sonerud, G. A., Smedshaug, C. A., and Bråthen, Ø (2001). Ignorant hooded crows follow knowledgeable roost-mates to food: support for the information centre hypothesis. Proc. R. Soc. Lond. Ser. B Biol. Sci. 268, 827–831. doi: 10.1098/rspb.2001.1586
Spiegel, O., and Crofoot, M. C. (2016). The feedback between where we go and what we know — information shapes movement, but movement also impacts information acquisition. Curr. Opin. Behav. Sci. 12, 90–96. doi: 10.1016/j.cobeha.2016.09.009
Squires, V. R., and Daws, G. T. (1975). Leadership and dominance relationships in Merino and Border Leicester sheep. Appl. Anim. Ethol. 1, 263–274. doi: 10.1016/0304-3762(75)90019-X
Stamps, J. (1995). Motor learning and the value of familiar space. Am. Nat. 146, 41–58. doi: 10.1086/285786
Stamps, J., Luttbeg, B., and Krishnan, V. V. (2009). Effects of survival on the attractiveness of cues to natal dispersers. Am. Nat. 173, 41–46. doi: 10.1086/593306
Steck, K., Hansson, B. S., and Knaden, M. (2009). Smells like home: desert ants, Cataglyphis fortis, use olfactory landmarks to pinpoint the nest. Front. Zool. 6:5. doi: 10.1186/1742-9994-6-5
Steffan-Dewenter, I., and Kuhn, A. (2003). Honeybee foraging in differentially structured landscapes. Proc. R. Soc. Lond. Ser. B Biol. Sci. 270, 569–575. doi: 10.1098/rspb.2002.2292
Strandburg-Peshkin, A., Farine, D. R., Couzin, I., and Crofoot, M. C. (2015). Shared decision-making drives collective movement in wild baboons. Science 348, 1358–1361. doi: 10.1126/science.aaa5099
Strandburg-Peshkin, A., Papageorgiou, D., Crofoot, M. C., and Farine, D. R. (2018). Inferring influence and leadership in moving animal groups. Philos. Trans. R. Soc. B Biol. Sci. 373:20170006. doi: 10.1098/rstb.2017.0006
Strittmatter, A., Sunde, U., and Zegners, D. (2020). Life cycle patterns of cognitive performance over the long run. Proc. Natl. Acad. Sci. U.S.A. 117:27255. doi: 10.1073/pnas.2006653117
Sueur, C., Deneubourg, J. L., and Petit, O. (2010). Sequence of quorums during collective decision making in macaques. Behav. Ecol. Sociobiol. 64, 1875–1885. doi: 10.1007/s00265-010-0999-8
Sueur, C., Deneubourg, J. L., and Petit, O. (2011). From the first intention movement to the last joiner: macaques combine mimetic rules to optimize their collective decisions. Proc. R. Soc. B Biol. Sci. 278, 1697–1704. doi: 10.1098/rspb.2010.2084
Tamm, S. (1980). Bird orientation: single homing pigeons compared with small flocks. Behav. Ecol. Sociobiol. 7, 319–322. doi: 10.1007/BF00300672
Teitelbaum, C. S., and Mueller, T. (2019). Beyond migration: causes and consequences of nomadic animal movements. Trends Ecol. Evol. 34, 569–581. doi: 10.1016/j.tree.2019.02.005
Teitelbaum, C. S., Converse, S. J., Fagan, W. F., Böhning-Gaese, K., O’Hara, R. B., Lacy, A. E., et al. (2016). Experience drives innovation of new migration patterns of whooping cranes in response to global change. Nat. Commun. 7:12793. doi: 10.1038/ncomms12793
Tennie, C., Call, J., and Tomasello, M. (2009). Ratcheting up the ratchet: on the evolution of cumulative culture. Philos. Trans. R. Soc. Lond. B Biol. Sci. 364, 2405–2415. doi: 10.1098/rstb.2009.0052
Thiebault, A., Mullers, R. H. E., Pistorius, P. A., and Tremblay, Y. (2014). Local enhancement in a seabird: reaction distances and foraging consequence of predator aggregations. Behav. Ecol. 25, 1302–1310. doi: 10.1093/beheco/aru132
Thorup, K., Bisson, I. A., Bowlin, M. S., Holland, R. A., Wingfield, J. C., Ramenofsky, M., et al. (2007). Evidence for a navigational map stretching across the continental U.S. in a migratory songbird. Proc. Natl. Acad. Sci. U.S.A. 104, 18115–18119. doi: 10.1073/pnas.0704734104
Thorup, K., Ortvad, T. E., Rabøl, J., Holland, R. A., Tøttrup, A. P., and Wikelski, M. (2011). Juvenile songbirds compensate for displacement to oceanic islands during autumn migration. PLoS One 6:e17903. doi: 10.1371/journal.pone.0017903
Thorup, K., Vega, M. L., Snell, K. R. S., Lubkovskaia, R., Willemoes, M., Sjöberg, S., et al. (2020). Flying on their own wings: young and adult cuckoos respond similarly to long-distance displacement during migration. Sci. Rep. 10:7698. doi: 10.1038/s41598-020-64230-x
Tindale, R. S., and Winget, J. R. (2017). “Learning while deciding in groups,” in The Oxford Handbook of Group and Organizational Learning, eds L. Argote and J. M. Levine (Oxford: Oxford University Press), 155–187. doi: 10.1093/oxfordhb/9780190263362.013.42
Toledo, S., Shohami, D., Schiffner, I., Lourie, E., Orchan, Y., Bartan, Y., et al. (2020). Cognitive map-based navigation in wild bats revealed by a new high-throughput tracking system. Science 369, 188–193. doi: 10.1126/science.aax6904
Torney, C. J., Lamont, M., Debell, L., Angohiatok, R. J., Leclerc, L. M., and Berdahl, A. M. (2018). Inferring the rules of social interaction in migrating caribou. Philos. Trans. R. Soc. B Biol. Sci. 373:20170385. doi: 10.1098/rstb.2017.0385
Torney, C., Neufeld, Z., and Couzin, I. D. (2009). Context-dependent interaction leads to emergent search behavior in social aggregates. Proc. Natl. Acad. Sci. U.S.A. 106, 22055–22060. doi: 10.1073/pnas.0907929106
Trapanese, C., Meunier, H., and Masi, S. (2019). What, where and when: spatial foraging decisions in primates. Biol. Rev. 94, 483–502. doi: 10.1111/brv.12462
Urbanek, R. P., Fondow, L. E. A., Satyshur, C. D., Lacy, A. E., and Zimorski, S. E. (2005). First cohort of migratory whooping cranes reintroduced to eastern North America: the first year after release. North Am. Crane Work. Proc. 9, 213–224.
Urmy, S. S. (2021). Visual trail following in colonial seabirds: theory, simulation, and remote observations. Ecol. Monogr. 91:e01429. doi: 10.1002/ecm.1429
Vaci, N., Edelsbrunner, P., Stern, E., Neubauer, A., Bilalić, M., and Grabner, R. H. (2019). The joint influence of intelligence and practice on skill development throughout the life span. Proc. Natl. Acad. Sci. U.S.A. 116, 18363–18369. doi: 10.1073/pnas.1819086116
Van Oosterom, L., Montgomery, J. C., Jeffs, A. G., and Radford, C. A. (2016). Evidence for contact calls in fish: conspecific vocalisations and ambient soundscape influence group cohesion in a nocturnal species. Sci. Rep. 6:19098.
Van Vugt, M. (2006). Evolutionary origins of leadership and followership. Pers. Soc. Psychol. Rev. 10, 354–371. doi: 10.1207/s15327957pspr1004_5
Vanleeuwe, H., and Gautier-Hion, A. (1998). Forest elephant paths and movement at the Odzala NP in Congo: the role of clearings (salt-licks) and Marantaceae on elephant distribution and movement. Afr. J. Ecol. 36, 174–182. doi: 10.1046/j.1365-2028.1998.00123.x
Visscher, P. K. (2007). Group decision making in nest-site selection among social insects. Annu. Rev. Entomol. 52, 255–275. doi: 10.1146/annurev.ento.51.110104.151025
Visscher, P. K., and Seeley, T. D. (1982). Foraging strategy of honeybee colonies in a temperate deciduous forest. Ecology 63, 1790–1801. doi: 10.2307/1940121
Voelkl, B., and Fritz, J. (2017). Relation between travel strategy and social organization of migrating birds with special consideration of formation flight in the northern bald ibis. Philos. Trans. R. Soc. B Biol. Sci. 372:20160235. doi: 10.1098/rstb.2016.0235
Voelkl, B., Portugal, S. J., Unsölde, M., Usherwood, J. R., Wilsond, A. M., and Fritz, J. (2015). Matching times of leading and following suggest cooperation through direct reciprocity during V-formation flight in ibis. Proc. Natl. Acad. Sci. U.S.A. 112, 2115–2120. doi: 10.1073/pnas.1413589112
von Frisch, K. (1967). The Dance Language and Orientation of Bees. Cambridge, MA: Harvard University Press.
Wachter, B., Broekhuis, F., Melzheimer, J., Horgan, J., Chelysheva, E. V., Marker, L., et al. (2017). “Behavior and communication of free-ranging cheetahs,” in Cheetahs: Biology and Conservation, eds P. J. Nyhus, L. Marker, L. K. Boast, and A. Schmidt-Kuentzel (Amsterdam: Elsevier), 121–134. doi: 10.1016/b978-0-12-804088-1.00009-5
Walker, R. H., King, A. J., McNutt, J. W., and Jordan, N. R. (2017). Sneeze to leave: African wild dogs (Lycaon pictus) use variable quorum thresholds facilitated by sneezes in collective decisions. Proc. R. Soc. B Biol. Sci. 284:20170347. doi: 10.1098/rspb.2017.0347
Wallraff, H. G. (1978). Social interrelations involved in migratory orientation of birds. Oikos 30, 401–404. doi: 10.2307/3543490
Wallraff, H. G. (1985). “Theoretical aspects of avian navigation,” in Proceedings of the XVIII Congressus internationalis Ornithologici, (Nauka), 284–292.
Wallraff, H. G. (2005). Avian Navigation: Pigeon Homin as a Pardigm. Berlin: Springer Science & Business Media.
Wallraff, H. G., and Andreae, M. O. (2000). Spatial gradients in ratios of atmospheric trace gases: a study stimulated by experiments on bird navigation. Tellus B Chem. Phys. Meteorol. 52, 1138–1157. doi: 10.1034/j.1600-0889.2000.00099.x
Waser, P. M., and Jones, W. T. (1983). Natal philopatry among solitary mammals. Q. Rev. Biol. 58, 355–390. doi: 10.1086/413385
Watts, I., Pettit, B., Nagy, M., de Perera, T. B., and Biro, D. (2016). Lack of experience-based stratification in homing pigeon leadership hierarchies. R. Soc. Open Sci. 3:150518. doi: 10.1098/rsos.150518
Weatherhead, P. J., and Forbes, M. R. L. (1994). Natal philopatry in passerine birds – genetic or ecological influences. Behav. Ecol. 5, 426–433. doi: 10.1093/beheco/5.4.426
Wehner, R. (2020). Desert Navigator: The Journey of the Ant. Cambridge, MA: Harvard University Press, doi: 10.4159/9780674247918
Wehner, R., and Wehner, S. (1986). Path integration in desert ants. Approaching a long-standing puzzle in insect navigation. Monit. Zool. Ital. Ital. J. Zool. 20, 309–331. doi: 10.1080/00269786.1986.10736505
Wehner, R., Michel, B., and Antonsen, P. (1996). Visual navigation in insects: coupling of egocentric and geocentric information. J. Exp. Biol. 199, 129–140. doi: 10.5167/uzh-634
Whiten, A. (2005). The second inheritance system of chimpanzees and humans. Nature 437, 52–55. doi: 10.1038/nature04023
Wikelski, M., Arriero, E., Gagliardo, A., Holland, R. A., Huttunen, M. J., Juvaste, R., et al. (2015). True navigation in migrating gulls requires intact olfactory nerves. Sci. Rep. 5:17061. doi: 10.1038/srep17061
Wiltschko, R., and Wiltschko, W. (1981). The development of sun compass orientation in young homing pigeons. Behav. Ecol. Sociobiol. 9, 135–141. doi: 10.1007/BF00293584
Wiltschko, R., and Wiltschko, W. (2000). A strategy for beginners! Reply to Wallraff (2000). Anim. Behav. 60, F37–F43. doi: 10.1006/anbe.2000.1494
Wiltschko, R., and Wiltschko, W. (2001). Clock-shift experiments with homing pigeons: a compromise between solar and magnetic information? Behav. Ecol. Sociobiol. 49, 393–400. doi: 10.1007/s002650000313
Wiltschko, R., and Wiltschko, W. (2015). Avian navigation: a combination of innate and learned mechanisms. Adv. Study Behav. 47, 229–310. doi: 10.1016/bs.asb.2014.12.002
Wiltschko, R., Haugh, C., Walker, M., and Wiltschko, W. (1998). Pigeon homing: sun compass use in the southern hemisphere. Behav. Ecol. Sociobiol. 43, 297–300. doi: 10.1007/s002650050494
Wiltschko, W. (1978). “Further analysis of the magnetic compass of migratory birds,” in Animal Migration, Navigation and Homing, eds K. Schmidt-Koenig and W. T. Keeton (Berlin: Springer Berlin Heidelberg), 302–310. doi: 10.1007/978-3-662-11147-5_29
Wiltschko, W., and Gwinner, E. (1974). Evidence for an innate magnetic compass in garden warblers. Naturwissenschaften 61, 406–407. doi: 10.1007/bf00622630
Wiltschko, W., and Wiltschko, R. (1972). Magnetic compass of European Robin. J. Chem. Inf. Model. 176, 62–64.
Wiltschko, W., and Wiltschko, R. (1975). The interaction of stars and magnetic field in the orientation system of night migrating birds: II. Spring experiments with European Robins (Erithacus rubecula). Z. Tierpsychol. 39, 265–282. doi: 10.1111/j.1439-0310.1975.tb00912.x
Wiltschko, W., and Wiltschko, R. (1980). The process of learning sun compass orientation in young homing pigeons. Naturwissenschaften 67, 512–514. doi: 10.1007/bf01047636
Wiltschko, W., and Wiltschko, R. (1981). Disorientation of inexperienced young pigeons after transportation in total darkness. Nature 291, 433–434. doi: 10.1038/291433a0
Wiltschko, W., and Wiltschko, R. (1982). “The role of outward journey information in the orientation of homing pigeons Bt – Avian navigation,” in Avian Navigation, eds F. Papi and H. G. Wallraff (Berlin: Springer Berlin Heidelberg), 239–252. doi: 10.1007/978-3-642-68616-0_24
Wiltschko, W., and Wiltschko, R. (2005). Magnetic orientation and magnetoreception in birds and other animals. J. Comp. Physiol. A Neuroethol. Sens. Neural Behav. Physiol. 191, 675–693. doi: 10.1007/s00359-005-0627-7
Wolfson, D. W., Fieberg, J. R., and Andersen, D. E. (2020). Juvenile Sandhill Cranes exhibit wider ranging and more exploratory movements than adults during the breeding season. Ibis 162, 556–562. doi: 10.1111/ibi.12786
Wooler, R. D., Bradley, J. S., Skira, I. J., and Serventy, D. L. (1990). Reproductive success of short-tailed shearwater Puffinus tenuirostris in relation to their age and breeding experience. J. Anim. Ecol. 59, 161–170. doi: 10.2307/5165
Wynn, J., Collet, J., Prudor, A., Corbeau, A., Padget, O., Guilford, T., et al. (2020). Young frigatebirds learn how to compensate for wind drift: frigatebirds and wind-drift compensation. Proc. R. Soc. B Biol. Sci. 287:20201970. doi: 10.1098/rspb.2020.1970rspb20201970
Yovel, Y., and Ulanovsky, N. (2017). Bat navigation. Curated Ref. Collect. Neurosci. Biobehav. Psychol. 1, 333–345. doi: 10.1016/B978-0-12-809324-5.21031-6
Zollner, P. A. (2000). Comparing the landscape level perceptual abilities of forest sciurids in fragmented agricultural landscapes. Landsc. Ecol. 15, 523–533.
Keywords: cognition, expertise, philopatry, spatial learning, social learning, navigation
Citation: Kashetsky T, Avgar T and Dukas R (2021) The Cognitive Ecology of Animal Movement: Evidence From Birds and Mammals. Front. Ecol. Evol. 9:724887. doi: 10.3389/fevo.2021.724887
Received: 14 June 2021; Accepted: 01 September 2021;
Published: 22 September 2021.
Edited by:
Louise Riotte-Lambert, University of Glasgow, United KingdomReviewed by:
Juan Manuel Morales, National University of Comahue, ArgentinaJerod Merkle, University of Wyoming, United States
Copyright © 2021 Kashetsky, Avgar and Dukas. This is an open-access article distributed under the terms of the Creative Commons Attribution License (CC BY). The use, distribution or reproduction in other forums is permitted, provided the original author(s) and the copyright owner(s) are credited and that the original publication in this journal is cited, in accordance with accepted academic practice. No use, distribution or reproduction is permitted which does not comply with these terms.
*Correspondence: Reuven Dukas, ZHVrYXNAbWNtYXN0ZXIuY2E=