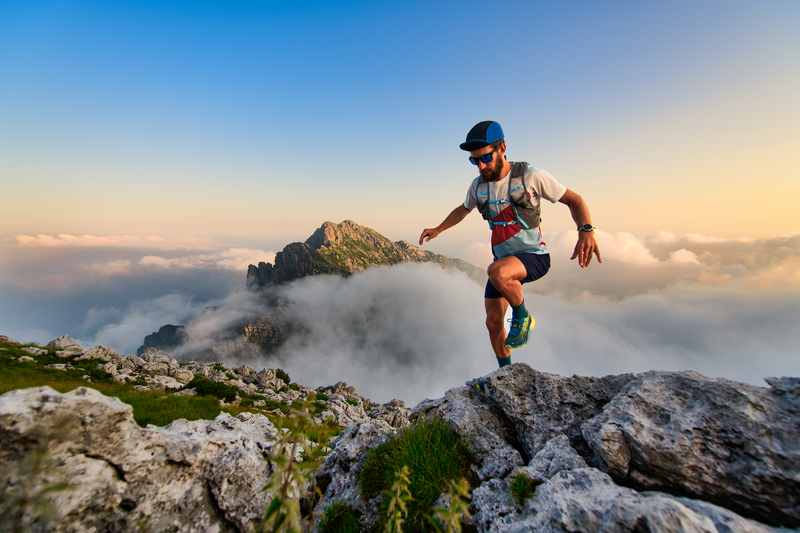
95% of researchers rate our articles as excellent or good
Learn more about the work of our research integrity team to safeguard the quality of each article we publish.
Find out more
ORIGINAL RESEARCH article
Front. Ecol. Evol. , 04 October 2021
Sec. Biogeography and Macroecology
Volume 9 - 2021 | https://doi.org/10.3389/fevo.2021.719741
This article is part of the Research Topic Temporal and Large-Scale Spatial Patterns of Plant Diversity and Diversification View all 10 articles
Apocynaceae (the dogbane and milkweed family) is one of the ten largest flowering plant families, with approximately 5,350 species and diverse morphology and ecology, ranging from large trees and lianas that are emblematic of tropical rainforests, to herbs in temperate grasslands, to succulents in dry, open landscapes, and to vines in a wide variety of habitats. Despite a specialized and conservative basic floral architecture, Apocynaceae are hyperdiverse in flower size, corolla shape, and especially derived floral morphological features. These are mainly associated with the development of corolline and/or staminal coronas and a spectrum of integration of floral structures culminating with the formation of a gynostegium and pollinaria—specialized pollen dispersal units. To date, no detailed analysis has been conducted to estimate the origin and diversification of this lineage in space and time. Here, we use the most comprehensive time-calibrated phylogeny of Apocynaceae, which includes approximately 20% of the species covering all major lineages, and information on species number and distributions obtained from the most up-to-date monograph of the family to investigate the biogeographical history of the lineage and its diversification dynamics. South America, Africa, and Southeast Asia (potentially including Oceania), were recovered as the most likely ancestral area of extant Apocynaceae diversity; this tropical climatic belt in the equatorial region retained the oldest extant lineages and these three tropical regions likely represent museums of the family. Africa was confirmed as the cradle of pollinia-bearing lineages and the main source of Apocynaceae intercontinental dispersals. We detected 12 shifts toward accelerated species diversification, of which 11 were in the APSA clade (apocynoids, Periplocoideae, Secamonoideae, and Asclepiadoideae), eight of these in the pollinia-bearing lineages and six within Asclepiadoideae. Wind-dispersed comose seeds, climbing growth form, and pollinia appeared sequentially within the APSA clade and probably work synergistically in the occupation of drier and cooler habitats. Overall, we hypothesize that temporal patterns in diversification of Apocynaceae was mainly shaped by a sequence of morphological innovations that conferred higher capacity to disperse and establish in seasonal, unstable, and open habitats, which have expanded since the Eocene-Oligocene climate transition.
The early diversification of flowering plants (angiosperms), a clade comprising approximately 90% of extant land plant species (Hernández-Hernández and Wiens, 2020), was marked by a rapid increase in lineage diversity, abundance, and distribution during the Late Cretaceous, 100–66 million years ago (Ma; Friis et al., 2011). More than half of angiosperm families diverged between 100 and 90 Ma (stem node ages) and diversified in the Cenozoic (66–2.6 Ma; crown node ages; Ramírez-Barahona et al., 2020). The abrupt collapse of ecosystems caused by the Cretaceous-Paleogene (K-Pg) mass extinction at ca. 65 Ma catalyzed drastic floristic changes and extirpated up to 57% of plant species, mostly affecting lineages dispersed and pollinated by animals (McElwain and Punyasena, 2007). The recovery of plant diversity in the early Paleocene coincided with the establishment and diversification of modern tropical rainforests (e.g., Morley, 2000; Wang et al., 2012; Meseguer et al., 2018) and, in the early Eocene climatic optimum (52–50 Ma), rainforests reached higher latitudes and formed a relatively continuous boreotropical flora (Wolfe, 1978). The global cooling and increased aridification that started mainly from the Eocene-Oligocene climatic transition (34 Ma) and intensified after the mid-Miocene climatic optimum (15 Ma), however, fostered the establishment and expansion of modern cold and dry biomes and narrowed rainforests to lower latitudes, which resulted in a collapse of intercontinental routes suitable for tropical plants (i.e., megathermal plants; Folk et al., 2020). While, in general, the recent temperate habitats show higher rates of speciation and turnover, older tropical rainforests have been less affected by extinction, show higher rates of net diversification (Igea and Tanentzap, 2020), and harbor more ancient families of angiosperms (Ramírez-Barahona et al., 2020). Although the accumulation of extant species increased mainly since the Pliocene (5 Ma) and, more intensively, during the Quaternary (2.6 Ma), the impact of Pleistocene climatic oscillations on the diversification of angiosperms, in particular within highly diverse tropical regions, such as the lowland Amazonian rainforests and campos rupestres highlands, remains debatable (Rapini et al., 2021).
Exceptionally diverse lineages are scattered across the angiosperm tree of life, but are also often concentrated in particular clades, such as the core asterids, which comprises six of the ten extremely species-rich orders of angiosperms (Magallón and Sanderson, 2001). Although a great variety of possible drivers of species diversification have been discussed, including, among others, biotic interactions, morphological key innovations, and whole-genome duplications (e.g., Soltis et al., 2019), shifts in diversification rates often depend on a complex and intricate net of interactions between intrinsic biological traits and extrinsic (biotic and abiotic) conditions (Davies et al., 2004; Vamosi and Vamosi, 2011; Bouchenak-Khelladi et al., 2015; Donoghue and Sanderson, 2015; Magallón et al., 2019; Nürk et al., 2020). Geographic range size is often positively associated with species diversity and was recently indicated as the trait that most explains overall diversification rates of orders and families within angiosperms (Hernández-Hernández and Wiens, 2020). Theory predicts that widely distributed lineages are less prone to extinction and more susceptible to fragmentation and ecological specialization, favoring higher diversification rates. In turn, higher diversification rates may generate key innovations that will boost range expansion under favorable conditions (Vamosi and Vamosi, 2011). In this relationship, the precedence between range size and key innovations is not clear-cut, encouraging spatiotemporal investigations with highly diverse groups of angiosperms (Hernández-Hernández and Wiens, 2020). Lineage-specific studies are essential to understand the inescapable idiosyncrasy associated with the evolution of biodiversity (Donoghue and Sanderson, 2015) and, here, we explore the historical biogeography and diversification of Apocynaceae (Gentianales), one of the ten largest families of angiosperms (Christenhusz and Byng, 2016).
Apocynaceae, the dogbane and milkweed family, consists of approximately 5,350 species and 378 genera (Endress et al., 2018-2019). The lineage is essentially pantropical, with centers of diversity in Southeast Asia, tropical America, and southern Africa (Ollerton et al., 2019), but otherwise distributed almost worldwide. It comprises large trees, treelets, shrubs, lianas (twining woody climbers), and more rarely epiphytes in tropical rainforests, succulents in dry, open landscapes, perennial herbs in temperate grasslands, to vines (herbaceous or succulent twiners) in a wide variety of habitats. The flowers are usually pentamerous (tetramerous in Leuconotis, and calyx tetramerous in Parahancornia and a few species of Aspidosperma), isostemonous, bicarpellate (tri- to pentacarpellate in Lepinia, Lepiniopsis, and some species of Pleiocarpa), and hyperdiverse in size and corolla shape (Figure 1), ranging from reflexed (e.g., Asclepias) or rotate, star-like (e.g., Stapelia) to campanulate (e.g., Beaumontia and Mandevilla), urceolate (e.g., Urceola and Dischidia), salverform (e.g., Carissa and Tabernaemontana), cylindrical pitfalls (Ceropegia), or even fig-like (Heterostemma ficoides A. Kidyoo; Kidyoo, 2019). They often produce corolline and/or staminal coronas and show a spectrum of integration between anthers and style-head that culminated with the formation of a gynostegium and five pollinaria, formed by a translator derived from gynoecium exudates and pollinia from the androecium (Endress, 2016; Endress et al., 2018-2019). Several species are planted as ornamentals, mainly because of their showy flowers, such as allamanda (Allamanda cathartica), Madagascar periwinkle (Catharanthus roseus), frangipani (Plumeria rubra), Easter lily vine (Beaumontia grandiflora), oleander (Nerium oleander), milkweeds (Asclepias spp.), and desert rose (Adenium obesum), but also due to a cactus-like habit, particularly the stapeliads.
Figure 1. Diversity of flowers in Apocynaceae. (A) Aspidosperma pyrifolium, Aspidospermateae; (B) Hancornia speciosa, Willughbeieae; (C) Couma rigida, Willughbeieae; (D) Tabernaemontana solanifolia, Tabernaemontaneae; (E) Condylocarpon isthmicum, Alyxieae; (F) Thevetia peruviana, Plumerieae; (G) Allamanda puberula, Plumerieae, (H) Wrightia coccinea, Wrightieae; (I) Adenium obesum, Nerieae; (J) Mandevilla atroviolacea, Mesechiteae; (K) Pentopetia grevei, Periplocoideae; (L) Secamone parviflora, Secamonoideae; (M) Ceropegia tihamana, Ceropegieae; (N) Orbea lutea, Ceropegieae; (O) Calotropis procera, Asclepiadinae; (P) Oxypetalum rusticum, Oxypetalinae [Photos of panels (A–G,I,J,O,P) by Rapini; panels (H,K–N) by Meve].
Most Apocynaceae species are pollinated by insects, such as butterflies, moths, bees, wasps, beetles, flies, even cockroaches (Xiong et al., 2020), and very rarely by birds (Pauw, 1998). Almost 20% of the species studied so far are insect generalists, i.e., pollinated by more than three of the seven big classes of insects listed above, and floral complexity is not paralleled by a specialization in pollinators (Ollerton et al., 2019). Anti-herbivory and medicinal compounds, particularly alkaloids and cardenolides, are widespread in the family (e.g., Agrawal et al., 2012) and are key in several fascinating plant-animal-animal interactions. The sequestration of glycosides from milkweeds (Asclepias spp.), for instance, causes the emblematic monarch butterfly (Danaus plexipus) to be unpalatable to birds (Agrawal, 2017), whereas other groups of Lepidoptera sequester pyrrolizidine alkaloids from various genera of apocynoids to produce pheromones for courtship displays (Edgar et al., 2007). The cardiac glycoside ouabain, extracted from the bark of Acokanthera, is used by the African crested rat (Lophiomys imhausi) against larger mammals, but also by traditional East African hunters to kill elephants (Kingdon et al., 2011). However, not all interactions with animals are made through phytochemicals. For example, leaves in some epiphytic species of Dischidia and Hoya are modified into “pitchers” that are inhabited by ants. These plants offer room for the ants to raise their young and in return, the ants bring nutrients through their debris and confer protection to the plant (Janzen, 1974; Peeters and Wiwatwitaya, 2014).
The species in Apocynaceae are classified in five major groups: three monophyletic subfamilies (Periplocoideae, Secamonoideae, and Asclepiadoideae) and two non-monophyletic groups informally treated as rauvolfioids and apocynoids (Endress et al., 2018-2019). These five divisions are defined by the direction of corolla lobe aestivation, type of pollen apertures, level of reproductive synorganization, presence of translators, and number and type of pollinia. Rauvolfioids and apocynoids do not produce a morphologically differentiated translator and were placed in the traditional Apocynaceae s.str. These two groups, however, form a grade toward the pollinia-bearing subfamilies: Periplocoideae, Secamonoideae, and Asclepiadoideae (e.g., Sennblad and Bremer, 1996; Fishbein et al., 2018; Antonelli et al., 2021). The pollinia-bearing subfamilies produce differentiated translators (acellular bodies derived from the hardening of style-head exudates), which apparently evolved more than once (Livshultz, 2010; Straub et al., 2014). The clade consisting of the apocynoids along with the two pollinia-bearing lineages Periplocoideae and Secamonoideae-Asclepiadoideae is known as the APSA clade (Livshultz et al., 2007; see also Endress et al., 2018-2019). The style-head is free from the anthers in rauvolfioids, but almost always adnate to the anthers, forming a gynostegium in the APSA clade. Besides a derived floral synorganization, the APSA clade is also mostly characterized by wind-dispersed seeds with a coma at the micropylar end, produced in follicles. Fruits of rauvolfioids, in contrast, are highly variable and include dehiscent fruits with ejected or wind-blown seeds, dehiscent fruits with arillate seeds dispersed by animals, indehiscent fleshy fruits with seeds also dispersed by animals, and indehiscent fruits adapted to disperse seeds by water, even sea water (Simões et al., 2016; Endress et al., 2018-2019; Figure 2).
Figure 2. Diversity of fruits and seeds in Apocynaceae. (A) Open follicle with winged seeds of Aspidosperma macrocarpon, Aspidospermateae; (B) Follicles of Alstonia macrophylla, Alstonieae; (C) One-seeded drupes of Kopsia pauciflora, Vinceae; (D) Berries of Couma rigida, Willughbeieae; (E) Berries of Hancornia speciosa, Willughbeieae; (F) Follicles of Tabaernaemontana pauciflora, Tabernaemontaneae; (G) Dehisced follicle with arillate seed of T. bovina, Tabernaemontaneae; (H) Berry with seeds of Spongiosperma grandiflorum, Tabernaemontaneae; (I) Torulose drupe with two articles of Alyxia siamensis, Alyxieae; (J) Torulose drupes with several articles of Condylocarpon isthmicum, Alyxieae; (K) Spiny capsule of Allamanda cathartica, Plumerieae; (L) Pair of fused immature follicles of Himatanthus drasticus, Plumerieae; (M) Open follicle with winged seeds of H. bracteatus, Plumerieae; (N) Drupaceous fruits of Thevetia peruviana, Plumerieae; (O) Berry of Carissa macrocarpa, Carisseae; (P) Open follicle with comose seeds of Wrightia pubescens subsp. lanitii, Wrightieae; (Q) Fused follicles of Temnadenia violacea, Echiteae; (R) Inflorescence with flowers and follicles of Hoya incrassata, Marsdenieae; (S) Comose seeds from an old fruit of Asclepias curassavica, Asclepiadinae [Photos of panels (A,D,E,J–O,Q,S) by Rapini; panels (B,G) by Middleton; panels (C,I) by Preecha Karaket, with permission from The Forest Herbarium Bangkok; panels (F,R) by Meve; panel (H) by Simões; panel (P) by Leonid Averyanov].
The oldest fossil assigned to the family seems to be the Alyxia-like pollen of Psilodiporites wolfendenii from the Paleocene of West Africa (Movshovich, 1975) and the Eocene of tropical Asia (Indonesia and Borneo; Muller, 1968; Morley, 1998). Comose seeds (seeds bearing a tuft of hairs at the end) remain the most convincing fossil evidence of Apocynaceae so far, indicating the presence of the APSA clade in Africa since the Eocene (47 Ma; Apocynospermum spp.; Collinson et al., 2010). However, most fossils from the Eocene to the Oligocene are restricted to the Northern Hemisphere, mainly to Europe and Asia (Martínez-Millán, 2010; Del Rio et al., 2020), such as the recently described fossil flower of the rauvolfioid Maryendressantha succinifera (Singh et al., 2021). American fossils assigned to Apocynaceae, such as the North American wood of Paraapocynaceoxylon barghoornii, dating from the late Cretaceous (Wheeler et al., 1987), and the well-preserved flower of the Neotropical Discoflorus neotropicus, dated to the mid-Tertiary (Poinar, 2017), are scant and taxonomically questionable. Pollen tetrads of Polyporotetradites laevigatus (Salard-Cheboldaeff, 1978) from the Oligocene-Miocene of Africa remain the oldest fossil evidence of Periplocoideae and comose seeds from the early Eocene were recently discovered in China and assigned to Asclepiadoideae (Asclepiadospermum spp.; Del Rio et al., 2020). Estimates for the Apocynaceae crown diversification based on molecular data are divergent, ranging from the early Eocene (Rapini et al., 2007) to the late Cretaceous (Fishbein et al., 2018; see also Ramírez-Barahona et al., 2020), with estimates exactly in between (Ribeiro et al., 2014; Pugliesi and Rapini, 2015). Although discrepant for ancient nodes, dating for intermediate nodes tends to be comparable among studies, as noted by Fishbein et al. (2018).
The biogeography of the family has been largely neglected from a phylogenetic perspective. Comprehensive inferences about the ancestral distributions of Apocynaceae and their major clades remain sparse and are mainly based on informal observations. Simões et al. (2007) suggested a tropical origin for the Apocynaceae, but did not postulate a place for their emergence, and Meve and Liede (2002b); Goyder (2006), Rapini et al. (2007), and Livshultz et al. (2007, 2011) suggested an African origin for the pollinia-bearing lineages. Pollinia provide additional protection against pollen desiccation, confer higher efficiency to pollination under low population densities, and may have reduced the extent of extinction in drier climate niches, contributing to the high diversity of milkweeds (Livshultz et al., 2011). However, formal biogeographic analyses in the family are few and restricted to particular asclepiad groups (e.g., Liede-Schumann et al., 2014, 2016), providing limited insights into a global understanding of the family’s diversification. Spatiotemporal patterns have not been formally investigated for the entire Apocynaceae, resulting in uncertainties regarding their place of origin and the emergence of the modern pantropical distribution.
Tropical plant families with a crown age younger than the continuing breakup of Gondwana, which started in the Jurassic, with greater peaks of continental fragmentation in early and late Cretaceous to Paleocene (McLoughlin, 2001), reached their ancestral distribution via two main historical routes: a southern Gondwanan (e.g., Axelrod and Raven, 1978; Linder and Crisp, 1995; van den Ende et al., 2017) or a northern Laurasian route (e.g., Davis et al., 2002; Ruhfel et al., 2016). Globally warmer climates, especially from the Paleocene–Eocene thermal maximum and early Eocene climatic optimum (ca. 56–48 Ma) to the Eocene-Oligocene climate transition (ca. 34 Ma; the terminal Eocene event, sensu Wolfe, 1978), allowed plants to disperse between the Old and the New World using more poleward routes and possibly land bridges to cross between continents in both Hemispheres (Wolfe, 1975, 1978; Axelrod and Raven, 1978; Tiffney, 1985b,a; McLoughlin, 2001; Morley, 2003). The global cooling and aridification after the Eocene-Oligocene climate transition closed these routes for megathermal plants and resulted in the expansion of temperate regions and the establishment of modern grasslands and deserts (Simon et al., 2009; Arakaki et al., 2011; Gagnon et al., 2019; Folk et al., 2020).
In this study, we provide the first attempt to integrate the most comprehensive time-calibrated phylogeny and morphological character evolution estimates in Apocynaceae (Fishbein et al., 2018) with an updated monographic work for the family (Endress et al., 2018-2019) to investigate (1) the historical biogeography of major lineages and (2) the dynamics of species diversification in the family. More specifically, we ask (1) whether a Gondwanan (Southern Hemisphere) or Laurasian (Northern Hemisphere) route was prevalent in shaping the distribution of Apocynaceae and (2) how variation in diversification rates underpinned the evolution of the family. First, we estimate ancestral areas under several models to produce new evidence on the origin of the family and the main routes of historical dispersals. Then, we estimate rates of species diversification across the Apocynaceae and test for shifts that may hint toward potential drivers of diversification. Finally, we associate estimates of diversification rates, historical biogeography, and morphological evolution (Endress et al., 2018-2019; Fishbein et al., 2018) to discuss the evolutionary history of Apocynaceae and to provide an overview of potential events that boosted the diversification of the family, from the uncertain origin to the uneven distribution of extant diversity.
We make use of the time-calibrated phylogenetic tree of Apocynaceae published in Fishbein et al. (2018) that covers all major lineages in the family and includes 1,041 species (ca. 20% of species richness) and 50% of the genera (ca. 180 out of 378 currently accepted sensu Endress et al., 2018-2019). The tree is a chronogram based on maximum likelihood analysis of 21 concatenated plastid loci (for more details, see Fishbein et al., 2018). Due to the incomplete and biased sampling, an inevitable limitation under such a broad coverage (e.g., Magallón et al., 2019; Mendel et al., 2019; Sun et al., 2020), we restricted our interpretations to Apocynaceae major clades, mainly above the genus level. Numbers of known species per clade and their distributions are recorded from Endress et al. (2018-2019) and updated according to new data and specialists’ knowledge (Supplementary Table 1; all data and scripts used in this study are available at our DRYAD repository).
Currently, three common biogeographic models are available for phylogenetic comparative inference of ancestral areas among species: dispersal and vicariance analysis (DIVA; Cronquist, 1997), the dispersal-extinction-cladogenesis (DEC; Ree et al., 2005; Ree and Smith, 2008), and BayArea models (Landis et al., 2013). The last model was the first to include a distance-dependent parameter, which can be set according to, for example, ecological distances, making possible direct comparison of biologically informed hypotheses (e.g., Pirie et al., 2019). These three biogeographic models are implemented in a likelihood framework in BioGeoBEARS (Matzke, 2013), which can also add the founder event (+J) parameter to all models, allowing speciation and dispersal to occur simultaneously. It has been argued that likelihoods under the classical models and their +J variants cannot be compared because +J models are biased toward having higher likelihoods (see discussion in Matzke, 2021). Since we found only minor differences in ancestral area estimates under the + J models compared to estimates without this parameter (Supplementary Tables 2A,B and Supplementary Figures 2–5, besides that model fit was generally much better for the + J models), we here present and discuss the estimates of the classical models (full results are available at DRYAD). For model comparison, we relied on log likelihoods and the sample size corrected Akaike information criterion (AICc), also calculating the ΔAICc (ΔAICc > 10 was taken as good evidence to reject a model in favor of the best one; Burnham and Anderson, 2002) and Akaike weights, which indicate the relative fit of a model in relation to all models in the set (i.e., cumulative Akaike weights of all models = 1).
We designed models to estimate ancestral areas during the diversification of Apocynaceae and to test specific hypotheses about (1) the geographical origin of the lineage and (2) macroevolutionary patterns of dispersal in BioGeoBEARS. Based on centers of species richness, relevant geological time periods, and theories of the major biogeographic realms, we divided the globe into six major terrestrial areas: Africa, including the Arabian Peninsula and Madagascar (A), temperate Eurasia (E), North America (N), Oceania, including Australasia and eastern Malesia (O), South America (S), and tropical Asia (T; justification, references, and maps are provided in Methods S1). We created a default model (M1), which allowed unconstrained and time homogeneous dispersal probabilities, and set the maximum number of areas to four (the maximum inhabited by extant species). To model shifts in dispersal probabilities over time due to plate movements, changing sea levels, and other major geological processes, we then defined a time-stratified model (M2) with four time periods (time slices: 0–30, 30–60, 60–80, 80–100 Ma; for details, see Supplementary Methods 1 and Supplementary Figure 1. N.B., the M2 model also restricts most dispersals between Hemispheres and is intended to illuminate the importance of such interhemispheric dispersal pathways in the biogeographic history of Apocynaceae). Additionally, in a “restricted range” modification of M1 and M2, we excluded unrealistic ancestral population distributions (e.g., Africa–North America) from the state space of geographic ranges (M1s, M2s models; for details, see R scripts at DRYAD). Additionally, to test hypotheses about the origin of Apocynaceae (i.e., the ancestral geographic range at the crown node of the family), we enforced the following constrained “test” models: South America (M3), Africa (M4), West Gondwana (M5), Gondwana (M6), tropical Asia (M7), East Gondwana (M8), Palaearctic (M9), Laurasia (M10), and two pantropical models, including West Gondwana plus tropical Asia (Pantropical 1, M11) and Gondwana plus tropical Asia (Pantropical 2, M12), respectively (for details, see Supplementary Table 3). At the time of crown group origin (85.6 Ma), these were the relevant landmasses according to plate tectonic reconstructions. We evaluated the test models with default (M3–M12) and restricted range (M3s–M12s) state spaces of geographic ranges. Each of the 24 models was run in BioGeoBEARS, using the DEC, BayArea-like, and DIVA-like models.
We estimated the frequency of dispersal events between areas with biogeographical stochastic mapping (BSM; Dupin et al., 2016; Matzke, 2016) using the model that best fits the data without constraining the area of the Apocynaceae crown node (M1s.dec model; see section “Results”; for BSM results including the + J parameter, see Supplementary Table 4). We conducted 1,000 MCMC stochastic mappings of occupied ranges and summarized dispersal events from the posterior distribution (R scripts and full analysis output are available at DRYAD).
To detect variation in species diversification rates among lineages in the Apocynaceae phylogeny, we used maximum likelihood (ML) and Bayesian approaches. First, we used ML in a character-free version of the HiSSE model (Beaulieu and O’Meara, 2016; Caetano et al., 2018), the so-called missing-state SSE (MiSSE) model, which implements averaging of rate estimates weighted according to goodness-of-fit of the models to the data (model averaging for short). We ran 52 MiSSE models varying in the number of “hidden” states (i.e., the number of different diversification regimes) with 1–26 regimes considered, and by setting the ratio of extinction to speciation (extinction fraction) to either 0.0 or 0.9, representing extreme diversification scenarios (Magallón and Sanderson, 2001). Sampling fraction was set to 20% for the entire tree, based on the empirical level of sampling. We used the functions generateMiSSEGreedyCombinations, MiSSEGreedy, and MarginReconMiSSE to obtain log likelihoods for the 52 models, and averaged rate estimates using Akaike weights based on the AICc (Burnham and Anderson, 2002).
Second, we used Bayesian analysis of macroevolutionary mixture (BAMM 2.5; Rabosky, 2014) to reconstruct the positions of significant shifts in diversification regimes during the evolution of Apocynaceae. Sampling fractions per clade were provided in order to account for uneven representation of species that are included in the tree (Supplementary Table 1). Appropriate priors were estimated in BAMMtools v2.1.7 (Rabosky et al., 2014) and a series of different priors on the expected number of shifts were tested in preliminary analyses (0.1–12; because results were stable among tested priors, we show and discuss results using a shift prior of 1; for comparison of the prior and posterior distributions of number of shifts, see Supplementary Figure 6). We ran four simultaneous Markov chain Monte Carlo (MCMC) chains for 20 million generations, saving estimates every 10 thousand generations and discarding the first 10% as burn-in before assessing convergence of chains; mixing of chains was assessed visually and effective sample sizes calculated using coda v0.19-4 (Plummer et al., 2006) were >200.
Of the 72 biogeographical models analyzed, the DEC model with restricted ranges and no constraint on the Apocynaceae ancestral area (M1s.dec) was the best fit by far, with an Akaike weight of 0.92 (Figure 3). Only one other model, the unrestricted DEC (M1.dec), had a ΔAICc < 10 (4.9), with an Akaike weight 0.08. All other models performed substantially worse (ΔAICc > 200; Supplementary Table 2A), including the time-stratified model M2 and M2s, which indicates the importance of interhemispheric dispersals in the history of the Apocynaceae. According to the M1s.dec model, the most recent common ancestor of Apocynaceae was most likely distributed in a geographic region that encompassed South America, Africa, and tropical Asia (S + A + T: state probability P = 0.62; Figure 3 and Supplementary Figure 2), whereas according to the M1.dec model, it would additionally include North America (S + A + T + N: P = 0.26; Supplementary Figure 3; state probabilities for all nodes are available at DRYAD). Uncertainties in ancestral ranges are apparent for other backbone nodes near the root, with South America, Africa, and/or tropical Asia as the most likely ranges (Figure 3 and Supplementary Figure 2). However, the crown node of early diverging clades was often associated with particular geographic regions generally with high degrees of certainty: e.g., the diversification of the Aspidospermateae (in South and North America, P = 0.94), Alstonieae and Vinceae (in tropical Asia, P = 0.91 and P = 0.81, respectively), Willughbeieae–Tabernaemontaneae (in South America, P = 0.24), and Melodineae–Asclepiadoideae (in Africa, P = 0.96).
Figure 3. Historical biogeography of Apocynaceae. Colored boxes at the tree nodes indicate the most probable ancestral geographic range inferred by the best biogeographic model (out of 72: M1s-DEC, Akaike weight 0.92; Supplementary Table 2A). Current distribution of species is given to the right of the tips following the color code in the map inset top right. Named clades according to Fishbein et al. (2018) are collapsed and sampling fraction is given after the clade name. The superimposed triangles in darker gray are scaled to the proportion of the sampling fraction per clade. The filled star indicates the APSA clade and the open star the pollinia-bearing lineages. (1) Results of biogeographic hypothesis tests of the ancestral area of Apocynaceae showing the model fit (Akaike weight): Pantropical 2, Pantropical 1, and Laurasia (Table 2). (2) The inferred ancestral distribution of Apocynaceae at around 72 Ma is indicated in the map. Pl., Pliocene; Quat., Quaternary; K-Pg, Cretaceous-Paleogene boundary; EECO, early Eocene climatic optimum; EOCT, Eocene-Oligocene climate transition; MMCO, middle Miocene climatic optimum.
Models constraining the ancestral range of Apocynaceae (M2–M12 and M2s–M12s) had much poorer fits than the less constrained models (M1 and M1s; Supplementary Table 2A). A comparison of the model fit among the 10 ancestral constrained “text” models (M3–M12) yields similar equivocal support for the range at the node of crown group (Table 1). Irrespective of the biogeographic model used (DEC, BayArea-like, or DIVA-like; Supplementary Table 5), only two constraints under the DEC model, the Pantropical model 2 (S + A + O + T; Akaike weight 0.50) and the Pantropical model 1 (S + A + T; Akaike weight 0.42), accounted for a cumulative AICc weight of 0.92 (Table 1 and Figure 3). Other constrained models with ΔAICc < 10 included the Laurasia model (N + E + T; Akaike weight 0.07, ΔAICc 4; Figure 3), and the Gondwana model (S + A + O; Akaike weight 0.01, ΔAICc 8; Table 1).
Table 1. Results of biogeographic hypothesis tests on the origin of Apocynaceae detailing fit to the data of the ten constrained models (M3–M12) using the dispersal-extinction-cladogenesis model (DEC).
Throughout the evolution of Apocynaceae, Africa is estimated by both ancestral area model fits (Figure 3 and Supplementary Figure 2) and BSM (Table 2) as the main source of dispersals to other areas with 64.2 events in total (ca. 0.8 events per million years; E/Myr), mainly to tropical Asia (ca. 0.3 E/Myr) and temperate Eurasia (ca. 0.2 E/Myr). The highest rates of lineage dispersals are estimated to have occurred between the two New World areas, with ca. 0.5 E/Myr from South to North America, and ca. 0.2 E/Myr for the reverse. North America is estimated as the main sink receiving ca. 0.5 E/Myr, closely followed by tropical Asia (Table 2).
Table 2. Numbers of dispersal events between the six biogeographic areas estimated by biogeographical stochastic mapping [BSM using M1s.dec; sum all dispersals (d) and range switching (a) events].
A model of constant diversification rate for the Apocynaceae was strongly rejected in both MiSSE and BAMM analyses. A single diversification regime (without a shift) was not included in the posterior of BAMM, and the MiSSE models with a single regime (one state in parameter “turnover”) were rejected by a ΔAICc of 776 for an extinction fraction (eps) of 0.9 and 1,236 for eps = 0.0 compared to the best model (Supplementary Table 6). Instead, the best-fitting MiSSE model assumed 18 diversification regimes and an extinction fraction of 0.9, with an Akaike weight of 0.13. Of the 52 MiSSE models tested, 16 models assuming 11–26 distinct diversification regimes received a ΔAICc < 10, all of which with eps = 0.9, with a cumulative AICc weight of 0.9998. The nine top models with ΔAICc < 2 assumed 14–22 distinct diversification rates with a cumulative Akaike weight of 0.8674 (Supplementary Table 6). The model-averaged MiSSE rate estimates of net diversification in Apocynaceae are given in Figure 4.
Figure 4. Species diversification in Apocynaceae. Colors on tree branches are averaged net diversification rate estimates inferred by the 52 models varying in the number of rate regimes (“hidden states”; 1–26) with extinction fraction (ratio of extinction to speciation) kept at 0.0 (26 models) or 0.9 (26 models) using MiSSE (rates > 1.35 species per million years are not shown in the color scale). Circles in grayscale superimposed on the tree indicate the nodes for which a shift in diversification rates has been inferred by BAMM and are scaled in gray and size to marginal likelihoods (shift probability; Table 1). Selected clade names and sampling fractions (spp. sampled/spp. known; for details see Figure 3 and Supplementary Table 1) are indicated. The ancient radiations (internodes with warmer colors) of rauvolfioids (close to R1) and apocynoids (close to R2) are indicated. The star indicates the APSA clade. Secamo., Secamonoideae; Periploco., Periplocoideae. EOM, clade comprising Echiteae, Odontadenieae, and Mesechiteae; MOG, clade comprising Metastelmatinae, Oxypetalinae, and Gonolobinae (plus other five smaller Neotropical subtribes). K-Pg, Cretaceous-Paleogene boundary; EECO, early Eocene climatic optimum; EOCT, Eocene-Oligocene climate transition; MMCO, middle Miocene climatic optimum. Insets top right are potential drivers of diversification: A, dry fruits with comose seeds; B, climbing habit (twining growth form) and; C. pollinarium with a pair of pollinia, indicating the two pollinia-bearing lineages as discussed in the text.
The BAMM analysis produced a single best shift configuration that includes 12 shifts toward accelerated species diversification (i.e., 13 different regimes; Figure 4 and Table 3). Shift configurations with 13–16 shifts in diversification rates are sampled with even higher frequencies in the posterior of the BAMM analysis (Supplementary Figure 4), which agree with our MiSSE results. Of the 12 shifts inferred in the best shift configuration (Figure 4 and Table 3), 11 occurred in the APSA clade: one in Wrightieae, two within the EOM clade and eight in pollinia-bearing lineages, including one leading to Periplocoideae, another to Secamonoideae, and the other six within Asclepiadoideae. Shifts toward higher net diversification rates occurred from the mid-Eocene onward, the oldest within the EOM clade (ca. 45 Ma) and the most recent within Mandevilla (ca. 3.3 Ma), with seven shifts during the Miocene. Although not detected as rate shifts by BAMM, the MiSSE results additionally indicate two older accelerations in species diversification rates, in the ancient radiations of rauvolfioids ca. 72–69 Ma (R1) and apocynoids ca. 55–52 Ma (R2; Figure 4).
Table 3. Shifts in net diversification rates inferred in the Bayesian analysis of macroevolutionary mixture (BAMM).
The complex breakup sequence of the southern supercontinent Gondwana started 180–150 Ma, involved approximately 90 fragments (including regions in Southeast Asia, Europe, and Florida), and caused disjunctions in lineages of angiosperms between land masses that currently correspond mainly to South America, Africa and Madagascar, Australia and New Zealand, and Antarctica (McLoughlin, 2001). Disjunct distributions in the Southern Hemisphere, therefore, are often interpreted as evidence of a Gondwanan origin followed by vicariance (e.g., Linder and Crisp, 1995), although this may not always be the most plausible explanation (e.g., Davis et al., 2002). Studies questioning the primacy of tectonic vicariance over oceanic dispersals in historical biogeography (e.g., Richardson et al., 2004; de Queiroz, 2005) and reinforcing the importance of dispersals on the distribution of tropical lineages since the late Cretaceous (e.g., Renner, 2004; Ruhfel et al., 2016) are increasingly being published. The predominantly austrotropical distribution of Apocynaceae fits the pattern classically associated with an initial diversification in tropical West Gondwana, i.e., South America-Africa in the late Cretaceous, ca. 85 Ma (Axelrod and Raven, 1978). Our results, however, refute the Gondwana or West Gondwana origin of Apocynaceae and did not support the prevalence of any route. Instead, they retrieved a tropical climatic belt in the equatorial region (the pantropical models), encompassing part of Gondwana (western landmasses) and part of Laurasia (Southeast Asia), as the most likely ancestral area of Apocynaceae, potentially also including Oceania (Figure 3). Uneven extinction rates and high rates of dispersal in plants obscure vicariance patterns and have often distorted biogeographic analyses of extant lineages, leading to unclear and equivocal reconstructions of ancestral areas especially at deeper nodes (Sanmartín and Ronquist, 2004; Meseguer et al., 2015, 2018; Rose et al., 2018; Larridon et al., 2020). An ancient pantropical distribution is shared by several other families (Procheş and Ramdhani, 2020) and the ancestral area estimated for Apocynaceae here reinforces the great importance of intercontinental dispersals in plant distribution since the late Cretaceous (e.g., Renner, 2004; de Queiroz, 2005).
Intercontinental dispersals occurred during the entire history of the family and, in a greenhouse world, we might expect that the ancestor of Apocynaceae was potentially widespread in austrotropical floras in South America, Africa, and Southeast Asia, with gene flow facilitated either through southern transoceanic land bridges (Morley, 2003) or through the boreotropical flora (Wolfe, 1975). The cool to warm temperate climate, with short periods of sunlight during winter months in Antarctica probably prevented most tropical lineages from using a southernmost route since the late Cretaceous (Sanmartín and Ronquist, 2004; van den Ende et al., 2017). North America, Europe, and Asia shared similar floristic compositions during the warmer early to mid-Eocene, suggesting a wide dispersal in tropical and subtropical floras of the Northern Hemisphere (Tiffney, 1985a, b; Su et al., 2020). The boreotropical flora reached paleolatitudes of approximately 60° N and persisted in a relatively uniform composition, from the late Cretaceous to mid-Eocene or later, with a shallow latitudinal temperature gradient (Wolfe, 1978). Eurasia was connected to eastern North America through the Northern Atlantic Land Bridge until the early Eocene and to western North America at higher latitudes by the Bering Strait, although with minor importance for megathermal plants after the early Eocene (Morley, 2003). These northern connections provided a pathway for disjunct austrotropical floras in South America, Africa, and Southeast Asia. Hence, the boreotropical flora represents an alternative hypothesis in place of oceanic long-distance dispersals to explain austrotropical floristic disjunctions that are too young to have been caused by West Gondwana fragmentation (Davis et al., 2002).
Tropical lineages of angiosperms may have used the boreotropical pathway between the New and Old World until the Eocene-Oligocene climate transition (Wolfe, 1978). The decrease of temperatures and increase of seasonality after the early Eocene climatic optimum (55 Ma), but mainly since the Eocene-Oligocene climate transition (ca. 34 Ma), caused the deterioration of the boreotropical flora, which contracted to refugia in lower latitudes, including Southeast Asia (Tiffney, 1985a; Morley, 2003). Lineages not adapted to cooler conditions were extirpated from the Northern Hemisphere during the Neogene, but part of the microthermal flora adapted to cooler climates derived from the preceding Paleogene local flora (e.g., Wolfe, 1975; Tiffney, 1985a; Nürk et al., 2015, 2018; Meseguer et al., 2018). The dramatic floristic change in the Northern Hemisphere since the Eocene-Oligocene climate transition may have blurred and distorted biogeographic results (e.g., Meseguer and Condamine, 2020), but fossil records from the Eocene of Europe, North America, and Asia (Martínez-Millán, 2010; Endress et al., 2018-2019; Del Rio et al., 2020; Singh et al., 2021) support the presence of Apocynaceae in the Northern Hemisphere at least since approximately 52 Ma. Therefore, data available so far cannot confidently indicate a center of origin for the Apocynaceae. The regions encompassing tropical Asia, South America, and Africa recovered in the ancestral area reconstruction could be interpreted as “museums” (sensu Stebbins, 1974) of Apocynaceae as they have apparently retained the oldest extant lineages of the family since the earliest diversification.
The highest diversification rates in Apocynaceae are concentrated in relatively recent lineages, although ancient rauvolfioids and apocynoids also showed episodes of higher diversification rates in the MiSSE analysis, giving birth to lineages that are often recognized at tribe and subfamily levels (Figure 4). The oldest tribe of Apocynaceae (Aspidospermateae, 69 spp.) diversified exclusively in the New World, while the second oldest tribe (Alstonieae, 45 spp.) diversified exclusively in the Old World (Figure 3; for complete ancestral area estimates on the models with the best fit, see Supplementary Figures 2, 3). In contrast, another early diverging clade, Vinceae (156 spp.; Figure 3), apparently showed a higher capacity of dispersal and is distributed worldwide. Vinceae shows multiple intercontinental dispersal events and reached the Hawaiian Islands more than once, possibly from the southern and western Pacific and from the Caribbean islands (Simões et al., 2016). Although follicular fruits are found in some genera, drupaceous fruits are prevalent and the tribe is mainly dispersed by animals and water. Vinceae may have occupied boreo- and austrotropical forests during the Eocene and the largest genus, Rauvolfia, reached South America from the Paleotropics more than once according to our reconstruction (Supplementary Figure 2), starting in the Miocene, probably through transoceanic dispersals, and thence to North America, although Simões et al. (2016) supported only a single dispersal to the Neotropics. The sister clades Willughbeieae (144 spp.) and Tabernaemontaneae (168 spp.) share South American ancestral and current pantropical distributions (Figure 3). Both dispersed to the Paleotropics during the Oligocene-Miocene boundary, but a shift in diversification rates was detected only within Tabernaemontaneae (Figure 4). This is the only shift in a lineage of Apocynaceae consisting predominantly of trees and shrubs producing ecomose seeds. The shift may be associated with a change from tall trees to treelets and shrubs growing at the lower canopy and below but also with a change in the dispersal mode as it roughly coincided with a shift from berries to dehiscent follicles with arillate seeds, characteristic of most derived Tabernaemontaneae.
The remaining Apocynaceae began to diverge in Africa and a few small tribes appeared in quick succession during the ancient rauvolfioid radiation in the late Cretaceous (Figures 3, 4). Melodineae (I and II; 30 spp.) and Hunterieae (20 spp.) remained restricted to the Paleotropics. Conversely, Amsonieae (16 spp.) shows a Laurasian distribution (North America, Europe, and Japan), possibly representing a relict component of the Eocene boreotropical flora, adapted to the cooler Neogene in higher latitudes and able to disperse through the Bering Strait, still available for subtropical plants during the late Miocene (Graham, 2011, 2018). Alyxieae (138 spp.) dispersed to Southeast Asia and Oceania, reaching South America from the Paleotropics in the Eocene, Plumerieae (55 spp.) dispersed to South America in the Paleocene, showing independent diversifications in the New and Old Worlds since the late Eocene, and Carisseae (25 spp.) remained restricted to the Old World, with Carissa probably dispersing to Asia and Australia before the reduction of forests and expansion of grasslands, caused by an increase of aridity in northern and eastern India after the mid-Miocene climatic optimum (Chen et al., 2019).
The oldest apocynoid tribes also diverged in Africa (Figure 3). Wrightieae (31 spp.), sister to the rest of the APSA clade, diverged in the Paleocene, dispersed eastward to tropical Asia (and Australia), and showed a shift in diversification rates in the Miocene (Figure 4). Nerieae (71 spp.) remained mainly restricted to Africa, independently reaching Europe and Asia, whereas in Malouetieae (95 spp.), early diversification occurred in Africa and South America, during the Eocene, with later dispersals to tropical Asia and North America (see also Livshultz et al., 2007). Apart from the Paleocene appearance of Wrightieae, Nerieae, and Malouetieae, the ancient apocynoid radiation coincided with the early Eocene climatic optimum. The increase of diversification rate estimated by MiSSE during this warm period may be associated with a sequential expansion from Africa to South America and Southeast Asia followed by retractions (Figures 3, 4), giving rise to the EOM clade and Rhabdadenieae in the New World, as well as Apocyneae, Baisseeae, and the pollinia-bearing lineages in the Old World. The EOM clade (479 spp.) consists mainly of Neotropical lianas and comprises the tribes Echiteae, Odontadenieae, and Mesechiteae, although Odontadenieae was not recovered as monophyletic in the large Apocynaceae tree of Fishbein et al. (2018) used here. Outside Asclepiadoideae, this is the most diverse lineage of Apocynaceae in the New World. A lineage of the EOM clade dispersed to the Paleotropics between the Oligocene and Miocene and became especially diverse in Oceania (Artia-Parsonsia, ca. 85 spp.). The oldest and the most recent shifts in diversification rates are located in the EOM clade (Figure 4). The more recent and likely rate shift in the late Pliocene is located in a lineage of Mandevilla with flowers bearing only a pair of nectaries alternate with the carpels (Clade III p.p. in Simões et al., 2006). Apocyneae (115 spp.) originated and diversified mainly in tropical Asia, but became widespread in the Old World, reaching North America (Apocynum), whereas Rhabdadenieae (3 spp.) diversified in South America, dispersing northward, and Baisseeae (29 spp.) remained restricted to Africa and Madagascar.
Two pollinia-bearing lineages emerged in Africa, independently derived from the apocynoid ancient radiation (Straub et al., 2014), although they formed a clade in the Apocynaceae tree used here (Fishbein et al., 2018; Figures 3, 4). Livshultz et al. (2011) hypothesized that the more efficient pollination mechanism conferred by pollinia contributed to reduce the Allee effects caused by low population densities and fewer pollinators available in cooler and seasonally dry forests in Africa after the early Eocene climatic optimum (Jacobs, 2004). Periplocoideae (177 spp.) showed a shift in diversification rates during the early Miocene, and remained distributed mainly in Africa and Madagascar, reaching Asia, Australia, and Europe. The subfamily consists mainly of lianas, and pollinia evolved more than once in the group (Ionta and Judd, 2017). Secamonoideae (158 spp.) is sister to Asclepiadoideae and showed a shift in diversification rates during the Oligocene. The lineage remains restricted to the Old World, and an origin in Madagascar, which still harbors three relict genera (Calyptranthera, Pervillaea, and Secamonopsis), is most likely (Lahaye, 2005). From there, it probably dispersed to Africa and, subsequently, to Oceania as well as to Asia. Secamone (90 spp.), the largest genus of Secamonoideae, is also most diverse in Madagascar, possibly due to pulses of vicariance and ecological specializations (Lahaye et al., 2007).
Six of the 12 shifts in Apocynaceae diversification rates are reconstructed in Asclepiadoideae, the largest subfamily, with about 60% of the species (Figure 4); none of them in the oldest tribe Fockeeae (nine spp.). Two independent shifts were detected in the sister tribes Marsdenieae and Ceropegieae and four in Asclepiadeae, with one in Tylophorinae, one in the MOG clade, one in Cynanchinae, and one in Asclepiadinae. Marsdenieae (737 spp.) crown diversification is marked by a shift in diversification rates in the late Oligocene, associated with its expansion across the Paleotropics. The tribe shows more than one dispersal to Asia, with one of the lineages becoming highly diverse in tropical Asia and Oceania (Hoya-Dischidia, > 400 spp.). It also reached South America in the mid to late Miocene, giving rise to an exclusively American clade of Marsdenieae (ca. 120 spp., segregated as Ruehssia; Espírito Santo et al., 2019), which has the highest diversification rate among the four Neotropical asclepiad lineages (Rapini et al., 2007).
Within Ceropegieae (Stapeliinae + Anisotominae + Lepta- deniinae + Heterostemmatinae; 786 spp.), the unresolved relationships among more than 30 genera of Stapeliinae (stapeliads; Bruyns, 2000; Meve and Liede, 2002a) suggested a possible rapid diversification, resulting in a controversial proposal to include all species of the subtribe in a single genus (Bruyns et al., 2017). Prior to the availability of dated phylogenies of Apocynaceae, a Gondwanan distribution for some stapeliads was proposed due to their occurrence in Africa and India (Bruyns, 2000). However, the diversification of Stapeliinae has been estimated to be in the Miocene or later (Rapini et al., 2007; Bruyns et al., 2015; Fishbein et al., 2018), too recent to support that hypothesis. Meve et al. (2016) proposed an origin and early diversification of Ceropegieae in wet tropical forests of Southeast Asia, with at least two dispersals westward, to northeastern and eastern Africa. However, we reconstruct the origin and early diversification of Ceropegieae in Africa starting in the late Eocene, with two dispersals to Southeast Asia. The first gave rise to Heterostemma (30 spp.) and the second occurred within the Stapeliinae (705 spp.). The origin of Stapeliinae was associated with a shift in diversification rates soon after the mid-Miocene, with nested radiations in the Pliocene (Figure 4), associated with the establishment of the succulent biome (Arakaki et al., 2011; Gagnon et al., 2019). Ceropegia radiated in tropical Asia, while the remaining Stapeliinae, with succulent stems, diversified in a drier Africa (Bruyns et al., 2014, 2015; Meve et al., 2016), with several dispersals to Asia (Supplementary Figure 2). The appearance in Stapeliinae of fleshy and soft roots from ancestral hard and wiry roots provided an adaptation to drier habitats, which was followed by the characteristic stem succulence of the clade (Bruyns et al., 2017).
The remaining Apocynaceae fall into two clades, the depauperate, African Eustegieae (4 spp.) and its sister group, Asclepiadeae (1,820 spp.), the largest tribe of the family, which retains an ancestral African distribution, as does the early-diverging Astephaninae (15 spp.) (Figure 3). A dispersal from Africa to South America at the end of the Eocene gave rise to the MOG clade (1,053 spp.). As the North Atlantic Land Bridge broke up in the early Eocene and the Bering Strait at higher latitude had limited importance for evergreen plants (Tiffney, 1985b; Morley, 2003), a transatlantic dispersal may be the most likely mode of arrival in the New World (Rapini et al., 2007). The MOG lineage, the most species-rich of the Neotropical clades (about 20% of the family), consists of eight subtribes, the three largest giving the clade its designation: Metastelmatinae, Oxypetalinae, and Gonolobinae. A shift in diversification rates is inferred early in the radiation of the MOG clade during the Oligocene, and several rate increases are apparent within it (Figure 4). At least four dispersals to Mesoamerica were inferred starting in the Miocene: once each in Gonolobinae and Oxypetalinae (ca. 20 Ma), Metastelmatinae (ca 10 Ma), and Orthosiinae (after 3 Ma). Of these, only Gonolobinae shows significant diversity in North America with dispersal back to South America (Supplementary Figure 2).
Cynanchinae (252 spp.) consists of Schizostephanus (2 spp.) and Cynanchum (250 spp.). The subtribe is inferred to have originated and begun to diversify in Africa during the Oligocene (Figure 3). Cynanchum consists of two major lineages with strongly contrasting evolutionary trajectories. For the first, a brief period of rapid diversification in Africa was followed by low diversification rates, with one of its lineages dispersing northward to Eurasia and from there to the New Word, possibly by the Bering Strait, reaching South America at the end of the Oligocene (Supplementary Figure 2). The other major lineage of Cynanchum dispersed eastward, reaching Madagascar, Southeast Asia, and Oceania. It consists of succulent species previously assigned to different genera and radiated after a shift in diversification rates during the late Miocene (Figure 4). This diversification was relatively synchronous with the diversification of Stapeliinae in Africa and the diversification of other succulent plant lineages with the establishment of modern deserts (Arakaki et al., 2011). The succulent group comprises one-half of the 100 Madagascan species of Cynanchum (Liede and Täuber, 2002) and dispersed from there back to Africa and other Paleotropical regions (Meve and Liede, 2002b; Khanum et al., 2016).
Tylophorinae (154 spp.) consists of two genera, Pentatropis (4 spp.) and Vincetoxicum s.l. (150 spp.), including Tylophora and all other genera traditionally recognized in Tylophorinae (Liede-Schumann and Meve, 2018). The subtribe emerged and began to diversify in the Oligocene in Africa, although most of its diversification followed a rate shift near the mid-Miocene (Figures 3, 4). Although Liede-Schumann et al. (2012) estimated a younger origin for this group, the hypothesized major diversification of Vincetoxicum in Asia starting at the mid-Miocene climatic optimum agrees with the estimate obtained here. Both Pentatropis and Vincetoxicum are inferred to have dispersed to Asia, where Vincetoxicum radiated and subsequently dispersed to Europe (Liede-Schumann et al., 2012, 2016). The sequence of short branches with low support due to pulses of rapid diversification makes ancestral area reconstructions uncertain for recent events, but is consistent with this scenario.
Like Cynanchinae and Tylophorinae, Asclepiadinae (364 spp.) is inferred to have originated and begun to diversify in Africa, in this case more recently, in the late Miocene (Figure 3). Most of the species belong to the Asclepias s.l. radiation, which may consist of up to 400 species in Africa and the Americas. The origin of Asclepias s.l. coincides with a shift in diversification rates (Figure 4), which resulted in the largest temperate radiation in the family. The African and American species probably form sister clades restricted to each area (Fishbein et al., 2011; Chuba et al., 2017), but this dichotomy is not resolved in the large phylogeny of Fishbein et al. (2018) used here. The dispersal of Asclepiadinae to North America in the late Miocene and subsequent diversification were likely favored by the expansion of grasslands in Africa and North America (Fishbein et al., 2011). The lineage would have reached North America through the Bering Strait (Rapini et al., 2007; Fishbein et al., 2011), which would have been available as a route for subtropical plants to the late Miocene (Graham, 2011, 2018), suggesting that the lineage was present in Asia and became extinct during the cooler Quaternary, without leaving a trace of this dispersal route. After diversifying in North America, Asclepias dispersed to South America either by a stepping-stone route across the Central America Seaway (Rapini et al., 2007) or after the complete emergence of the Panama Isthmus and subsequent expansion of savannas (Bacon et al., 2016).
In Apocynaceae, only a diversification rate shift within Tabernaemontaneae (Figure 4) might be associated with a shift between biotic dispersal modes, from seeds embedded in the pulp of indehiscent fruits to exposed arillate seeds in dehiscent fruits (shift from fleshy to dry fruits in Fishbein et al., 2018). This shift is associated with a change in the dispersal propagule, which is the entire fruit in berries and each seed with the associated aril in follicles. Fruits and dispersal modes are heterogeneous in rauvolfioid lineages, but follicles producing wind-dispersed comose seeds are highly conservative in the APSA clade. Simões et al. (2007) recovered a dry dehiscent fruit as ancestral in Apocynaceae (see also Zhang et al., 2020), from which berries and drupes derived several times in rauvolfioid lineages. The apparent phylogenetic conservatism of dry fruits coupled with the great flexibility of fruits in rauvolfioids, however, led Fishbein et al. (2018) to estimate at least five origins of dry fruits from rauvolfioid ancestors with fleshy fruits. Among the several groups that produce dry fruits in the family, significant accelerations of diversification are concentrated in only one, the APSA clade. Eleven of the 12 rate shifts in the family occurred in this essentially anemochorous lineage (Figure 4). The 20-million-years lag between the appearance of comose seeds in the APSA clade, near the Cretaceous-Paleogene boundary, and diversification rate shifts after the mid-Eocene does not suggest a direct effect of dispersal modes in the Apocynaceae diversification. However, the impact of dispersal syndromes in diversification rates is circumstantial (Herrera, 1989; Tiffney and Mazer, 1995) and the higher capacity of dispersal of comose seeds outside wet forests might have pushed the expansion of the APSA clade in open habitats and indirectly favored its diversification in the drier Miocene.
The ancient apocynoid radiation in the Early Eocene was accompanied by a shift from self-supporting (trees) to climbing habit (lianas and vines) in the APSA clade (Fishbein et al., 2018). Anatomical evolutionary trends in wood, such as the reduction of vessel element length and formation of larger vessel clusters, marked the transition from erect to climbing habit and might have favored the occupation of drier habitats (Lens et al., 2008, 2009; Endress et al., 2018-2019). The wind-dispersed comose seeds, then, might have favored twining plants to disperse and occupy open and semi-arid habitats. Twining plants seem to have greater capacities for the uptake and transportation of water and are more competitive than trees in high-light, nutrient-rich environments, such as the seasonally dry forests (Medina-Vega et al., 2021). Xylem adaptations to drought and cold show similar trends and possibly contributed to rapid diversification events in several lineages of angiosperms in association with the global cooling and aridification, especially after the mid-Miocene climatic optimum (Folk et al., 2020).
The gynostegium is a synapomorphy of the APSA clade (Endress et al., 2018-2019) and a precursor (sensu Donoghue and Sanderson, 2015) of pollinaria (specialized pollen dispersal units; Endress, 2016). Each pollinarium carries the content of two or four pollen sacs in tetrads or, more often, packaged in pollinia. Otherwise known only in Orchidaceae, pollinia evolved more than once in the APSA clade (Straub et al., 2014) and there were no reversals (Fishbein et al., 2018). They confer higher pollen transfer efficiency to pollinia-bearing lineages, as a single pollinium transfer in this group can produce many more seeds than a pollination by means of single pollen grains (free monads). Pollinia-bearing lineages are thus thought to be less impacted by lower densities of mates and pollinators (mate-finding Allee effect), and to have been positively selected for during the African aridification (Livshultz et al., 2011).
Rather than a single key innovation, the high diversity of Apocynaceae can be mostly explained by a sequence of interacting innovations (i.e., a synnovation, sensu Donoghue and Sanderson, 2015) that conferred higher capacity to disperse and establish in dry, open, and unstable habitats, in agreement with Stebbins’ classic paper on aridity as a stimulus to evolution (Stebbins, 1952). Wind-dispersed comose seeds, a twining growth form, and pollinia appeared sequentially and probably work synergistically in the occupation of drier habitats, shaping most of the extant diversity of Apocynaceae. The long-term global decline of atmospheric CO2, temperature, and humidity after the Eocene-Oligocene climate transition promoted the expansion of temperate regions and the establishment of grass and succulent biomes during the Miocene (e.g., Schrire et al., 2005, 2009; Simon et al., 2009; Arakaki et al., 2011; Gagnon et al., 2019; Folk et al., 2020), and fostered the geographic expansion of the APSA clade. Wider distributions may then have fueled accelerations in diversification, for example, due to more opportunities for the occupation of new habitats and specialization or environmental changes and fragmentation (Vamosi and Vamosi, 2011; Nürk et al., 2020). Following the terminology of Bouchenak-Khelladi et al. (2015) and Donoghue and Sanderson (2015), we hypothesize that the diversification of the APSA clade was triggered by the key confluence of a background synnovation and the long-term environmental changes since the Eocene-Oligocene climatic transition, which might also have served as modulators of diversification in the family.
This is the first investigation of the biogeography and the tempo of diversification in Apocynaceae to consider all major lineages within the family. The cumulative noise caused by dispersals and extinctions may add uncertainty to ancestral area estimates at deep nodes, and uncertainties remain on the area of origin for Apocynaceae. However, tropical South America, Southeast Asia, and Africa have retained the oldest lineages since the early diversification (Figure 3) and can be considered museums of Apocynaceae diversity (Stebbins, 1974). Africa was confirmed as the cradle of pollinia-bearing lineages and the main source of Apocynaceae intercontinental dispersals (Figure 3 and Table 3). From Africa, Apocynaceae may have reached Asia by birds across the Indian Ocean (e.g., Petchia) or used land pathways (e.g., oceanic coastlines, as discussed for stem-succulent Cynanchum spp.; Meve and Liede, 2002b), either the boreotropical flora (before the Miocene) or the Arabian Peninsula (in the early and mid-Miocene for lineages inhabiting forests or in the late Miocene, after the expansion of savannas and semi-arid environments, for more xeric lineages; Chen et al., 2019). From tropical Asia, Apocynaceae dispersed to Oceania, temperate Eurasia (e.g., Vincetoxicum; Liede-Schumann et al., 2016), and North America, possibly through the Bering Strait. As discussed above, this northern subtropical to temperate route was probably used by Amsonia (Amsonieae), Apocynum (Apocyneae), Cynanchum (Cynanchinae), and Asclepias (Asclepiadinae). Most of the Apocynaceae diversity in the New World, however, dispersed from tropical Africa to South America, possibly via transatlantic dispersal or, before the mid-Eocene, also through the boreotropical flora. Two of the dispersals resulted in the largest American clades, the apocynoid EOM and the asclepiad MOG, but the rauvolfioid tribes Alyxieae and Plumerieae as well as the apocynoid tribes Malouetieae and Rhabdadenieae also reached South America from Africa (Supplementary Figure 2). Migrations from the New to Old World were rare, mostly restricted to the rauvolfioid tribes Willughbeieae and Tabernaemontaneae and the apocynoid EOM clade. Exchanges between South and North American Apocynaceae spanned most of the family history, at least since the Eocene, suggesting that the Greater and Lesser Antilles acted as plant sources for continental America (Liede-Schumann et al., 2014; Nieto-Blázquez et al., 2017) and that narrow seaways are not an effective barrier for plants (Joyce et al., 2021).
This Apocynaceae saga can be roughly divided in two major momenta ecologically driven by the increasingly dry Cenozoic climate and a sequence of key morphological innovations. Diversification rate shifts are concentrated after the Eocene-Oligocene climate transition, almost exclusively in lineages of the APSA clade, and mainly in groups with pollinia. From ancestors in closed tropical rainforests, the Apocynaceae phylogenetic backbone followed a general escalation toward the highly diverse wind-dispersed, twining, pollinia-bearing lineages in more stressful, unstable habitats. The increase of diversification rates in Apocynaceae, therefore, was probably stimulated by an evolutionary sequence of morphological innovations: (1) fixation of seeds with a micropylar coma (anemochory) and (2) transition from a self-supported to a climbing habit during the apocynoid early diversification, followed by (3) the advent of pollinia, first in Asclepiadoideae-Secamonoideae and, more recently, in Periplocoideae. From the Cretaceous through most of the Eocene, the climate was warmer, with a lower latitudinal gradient, allowing forests to spread over much of the Earth during the more humid Eocene. In this greenhouse world, Apocynaceae was mainly distributed in austro- and boreotropical rainforests. Following an abrupt decline of atmospheric CO2 after the Eocene-Oligocene climate transition, global temperature and humidity dropped, temperate and seasonally dry habitats expanded, while rainforests retracted to lower latitudes, mainly in the Southern Hemisphere. In this cooling world, the diversity of Apocynaceae was mainly dominated by the wind-dispersed, twining, pollinia-bearing lineages in more unstable seasonal and semi-arid habitats, whereas most rauvolfioid and ancient apocynoid lineages found refuge mainly in stable tropical forests.
The approaches assumed here are not immune to, for example, biases introduced by species sampling and model choice, which can affect results. In addition, traits apart from the evolutionary sequence toward pollinia-bearing lineages may also have effects on diversification of Apocynaceae, especially at more recent phylogenetic scales. Biotic interactions, in particular associated with the complex evolution of chemical defenses against herbivory (e.g., Agrawal et al., 2009; Livshultz et al., 2018), may be additionally involved in the diversification history of the family. Hence, although the big picture shown here may represent an oversimplification of Apocynaceae evolution, we believe that it nevertheless serves as a useful roadmap to highlight major biogeographic patterns and outline potential key drivers to higher diversification rates, which are now open to be tested.
The original contributions presented in the study are included in the article/Supplementary Material/DRYAD repository (https://doi.org/10.5061/dryad.vq83bk3sj), further inquiries can be directed to the corresponding author/s.
The research reported in this paper was exempt from ethical approval procedures.
CB and AR conceived the study and prepared the dataset, which was revised by all authors. CB and NN designed the analyses with contributions from AR. NN ran the analyses. CB, NN, and AR interpreted the results and prepared an initial draft. All authors revised the manuscript critically for intellectual content.
CB acknowledges the Coordenação de Aperfeiçoamento de Pessoal de Nível Superior—Brasil (CAPES)—Finance Code #1514632 (DS), an International Association of Plant Taxonomy (IAPT, 2016) grant, and the Neotropical Grasslands Conservancy Memorial (NGC, 2016) and Shirley A. Graham (Missouri Botanical Garden, 2018) Fellowships. AR is supported by CNPQ (Productivity Fellowship no. 307396/2019-3). NN acknowledges the support of the Deutsche Forschungsgemeinschaft (DFG grant number NU292/4-1). This publication was funded by the German Research Foundation (DFG) and the University of Bayreuth in the funding program Open Access Publishing.
The authors declare that the research was conducted in the absence of any commercial or financial relationships that could be construed as a potential conflict of interest.
All claims expressed in this article are solely those of the authors and do not necessarily represent those of their affiliated organizations, or those of the publisher, the editors and the reviewers. Any product that may be evaluated in this article, or claim that may be made by its manufacturer, is not guaranteed or endorsed by the publisher.
This work is part of the Ph.D. thesis of CB, developed at Programa de Pós-Graduação em Botânica da Universidade Estadual de Feira de Santana. We would like to thank Moabe Fernandes for assistance with the dataset, Luciano Paganucci de Queiroz for suggesting our study as a potential contribution to this research topic, Danilo Neves for inviting us to join the article collection “Temporal and Large-Scale Spatial Patterns of Diversity and Diversification” at Frontiers in Ecology and Evolution, and the two reviewers for insightful suggestions.
The Supplementary Material for this article can be found online at: https://www.frontiersin.org/articles/10.3389/fevo.2021.719741/full#supplementary-material
Supplementary Table 1 | Species data: taxon names, clade assignment, species richness, sampling fractions, and species distribution.
Supplementary Table 2 | Biogeographic analyses: model comparison results (a: considered; b: incl. + J).
Supplementary Table 3 | Biogeographic test: model definition.
Supplementary Table 4 | BSM: dispersals estimated under the M1s.DEC + J model.
Supplementary Table 5 | Biogeographic tests: model comparison results (all test models).
Supplementary Table 6 | Diversification rates analysis (MiSSE): model comparison results.
Supplementary Methods 1 | Biogeographic analysis: Delimitation of areas; Paleogeographic model; References.
Supplementary Figure 1 | Paleogeographic model: used in the time-stratified analysis (M2, M2s models).
Supplementary Figure 2 | Biogeographic analyses: ancestral area estimates produced by the M1s.DEC model showing (A) the best estimates, and (B) the state probabilities.
Supplementary Figure 3 | Biogeographic analyses: ancestral area estimates produced by the M1.DEC model, showing (A) the best estimates, and (B) the state probabilities.
Supplementary Figure 4 | Biogeographic analyses: ancestral area estimates produced by the M1.DEC + j model, showing (A) the best estimates, and (B) the state probabilities.
Supplementary Figure 5 | Biogeographic analyses: ancestral area estimates produced by the M1s.DEC + j dec model, showing (A) the best estimates, and (B) the state probabilities.
Supplementary Figure 6 | Diversification rate shifts analysis (BAMM): Prior and posterior distributions of number of shifts.
Agrawal, A. A. (2017). Monarchs and Milkweed: A Migrating Butterfly, A Poisonous Plant, and Their Remarkable Story of Coevolution. Princeton, NJ: Princeton University Press.
Agrawal, A. A., Fishbein, M., Halitschke, R., Hastings, A. P., Rabosky, D. L., and Rasmann, S. (2009). Evidence for adaptive radiation from a phylogenetic study of plant defenses. Proc. Natl. Acad. Sci. U.S.A. 106, 18067–18072. doi: 10.1073/pnas.0904862106
Agrawal, A. A., Petschenka, G., Bingham, R. A., Weber, M. G., and Rasmann, S. (2012). Toxic cardenolides: chemical ecology and coevolution of specialized plant–herbivore interactions. New Phytol. 194, 28–45. doi: 10.1111/j.1469-8137.2011.04049.x
Antonelli, A., Clarkson, J. J., Kainulainen, K., Maurin, O., Brewer, G. E., Davis, A. P., et al. (2021). Settling a family feud: a high-level phylogenomic framework for the Gentianales based on 353 nuclear genes and partial plastomes. Am. J. Bot. 108, 1143–1165. doi: 10.1002/ajb2.1697
Arakaki, M., Christin, P.-A., Nyffeler, R., Lendel, A., Eggli, U., Ogburn, R. M., et al. (2011). Contemporaneous and recent radiations of the world’s major succulent plant lineages. Proc. Natl. Acad. Sci. U.S.A. 108, 8379–8384. doi: 10.1073/pnas.1100628108
Axelrod, D. I., and Raven, P. H. (1978). “Late cretaceous and tertiary vegetation history of Africa,” in Biogeography and Ecology of Southern Africa, eds M. J. A. Werger and A. C. van Bruggen (The Hague: Dr W. Junk bv Publishers), 77–130. doi: 10.1007/978-94-009-9951-0_5
Bacon, C. D., Molnar, P., Antonelli, A., Crawford, A. J., Montes, C., and Vallejo-Pareja, M. C. (2016). Quaternary glaciation and the Great American Biotic Interchange. Geology 44, 375–378. doi: 10.1130/G37624.1
Beaulieu, J. M., and O’Meara, B. C. (2016). Detecting hidden diversification shifts in models of trait-dependent speciation and extinction. Syst. Biol. 65, 583–601. doi: 10.1093/sysbio/syw022
Bouchenak-Khelladi, Y., Onstein, R. E., Xing, Y., Schwery, O., and Linder, H. P. (2015). On the complexity of triggering evolutionary radiations. New Phytol. 207, 313–326. doi: 10.1111/nph.13331
Bruyns, P. V. (2000). Phylogeny and biogeography of stapeliads. Plant Syst. Evol. 221, 199–226. doi: 10.1007/bf01089294
Bruyns, P. V., Klak, C., and Hanáèek, P. (2014). Evolution of the stapeliads (Apocynaceae – Asclepiadoideae) – repeated major radiation across Africa in an Old World group. Mol. Phylogen. Evol. 77, 251–263. doi: 10.1016/j.ympev.2014.03.022
Bruyns, P. V., Klak, C., and Hanáèek, P. (2015). Recent radiation of Brachystelma and Ceropegia (Apocynaceae) across the Old World against a background of climatic change. Mol. Phylogen. Evol. 90, 49–66. doi: 10.1016/j.ympev.2015.04.015
Bruyns, P. V., Klak, C., and Hanáèek, P. (2017). A revised, phylogenetically-based concept of Ceropegia (Apocynaceae). S. Afr. J. Bot. 112, 399–436. doi: 10.1016/j.sajb.2017.06.021
Burnham, K. P., and Anderson, D. R. (2002). Model Selection and Multimodel Inference: A Practical Information-Theoretic Approach. New York, NY: Springer-Verlag.
Caetano, D. S., O’Meara, B. C., and Beaulieu, J. M. (2018). Hidden state models improve state-dependent diversification approaches, including biogeographical models. Evolution 72, 2308–2324. doi: 10.1111/evo.13602
Chen, J., Thomas, D. C., and Saunders, R. M. K. (2019). Geographic range and habitat reconstructions shed light on palaeotropical intercontinental disjunction and regional diversification patterns in Artabotrys (Annonaceae). J. Biogeogr. 46, 2690–2705. doi: 10.1111/jbi.13703
Christenhusz, M. J. M., and Byng, J. W. (2016). The number of known plants species in the world and its annual increase. Phytotaxa 261, 201–217. doi: 10.11646/phytotaxa.261.3.1
Chuba, D., Goyder, D. J., Chase, J. M., and Fishbein, M. (2017). Phylogenetics of the African Asclepias complex (Apocynaceae) based on three plastid DNA regions. Syst. Bot. 42, 148–159. doi: 10.1600/036364417X694539
Collinson, M. E., Manchester, S. R., Wilde, V., and Hayes, P. (2010). Fruit and seed floras from exceptionally preserved biotas in the European Paleogene. Bull. Geosci. 85, 155–162. doi: 10.3140/bull.geosci.1155
Cronquist, F. (1997). Dispersal-vicariance analysis: a new approach to the quantification of historical biogeography. Syst. Biol. 46, 195–203. doi: 10.1093/sysbio/46.1.195
Davies, T. J., Barraclough, T. G., Chase, M. W., Soltis, P. S., Soltis, D. E., and Savolainen, V. (2004). Darwin’s abominable mystery: insights from a supertree of the angiosperms. Proc. Natl. Acad. Sci. U.S.A. 101, 1904–1909. doi: 10.1073/pnas.0308127100
Davis, C. C., Bell, C. D., Mathews, S., and Donoghue, M. J. (2002). Laurasian migration explains Gondwanan disjunctions: evidence from Malpighiaceae. Proc. Natl. Acad. Sci. U.S.A. 99, 6833–6837. doi: 10.1073/pnas.102175899
de Queiroz, A. (2005). The resurrection of oceanic dispersal in historical biogeography. Trends Ecol. Evol. 20, 68–73. doi: 10.1016/j.tree.2004.11.006
Del Rio, C., Wang, T.-X., Liu, J., Liang, S.-Q., Spicer, R. A., Wu, F.-X., et al. (2020). Asclepiadospermum gen. nov., the earliest fossil record of Asclepiadoideae (Apocynaceae) from the early Eocene of central Qinghai-Tibetan Plateau, and its biogeographic implications. Am. J. Bot. 107, 126–138. doi: 10.1002/ajb2.1418
Donoghue, M. J., and Sanderson, M. J. (2015). Confluence, synnovation, and depauperons in plant diversification. New Phytol. 207, 260–274. doi: 10.1111/nph.13367
Dupin, J., Matzke, N. J., Särkinen, T., Knapp, S., Olmstead, R., Bohs, L., et al. (2016). Bayesian estimation of the global biogeographic history of the Solanaceae. J. Biogeogr. 44, 887–899. doi: 10.1111/jbi.12898
Edgar, J. A., Boppré, M., and Kaufmann, E. (2007). Insect-synthesised retronecine ester alkaloids: precursors of the common arctiine (Lepidoptera) pheromone hydroxydanaidal. J. Chem. Ecol. 33, 2266–2280. doi: 10.1007/s10886-007-9378-y
Endress, M. E., Meve, U., Middleton, D. J., and Liede-Schumann, S. (2018-2019). “Apocynaceae,” in Flowering Plants. Eudicots. Apiales and Gentianales (Except Rubiaceae) Families and Genera of Vascular Plants, Vol. 15, eds J. W. Kadereit and V. Bittrich (Berlin: Springer), 208–411. doi: 10.1007/978-3-319-93605-5_3
Endress, P. K. (2016). Development and evolution of extreme synorganization in angiosperm flowers and diversity: a comparison of Apocynaceae and Orchidaceae. Ann. Bot. 117, 749–767. doi: 10.1093/aob/mcv119
Espírito Santo, F. S., Rapini, A., Ribeiro, P. L., Liede-Schumann, S., Goyder, D. J., and Fontella-Pereira, J. (2019). Phylogeny of the tribe Marsdenieae (Apocynaceae), reinstatement of Ruehssia and the taxonomic treatment of the genus in Brazil. Kew Bull. 74:30. doi: 10.1007/s12225-019-9807-4
Fishbein, M., Chuba, D., Ellison, C., Mason-Gamer, R. J., and Lynch, S. P. (2011). Phylogenetic relationships of Asclepias (Apocynaceae) inferred from non-coding chloroplast DNA sequences. Syst. Bot. 36, 1008–1023. doi: 10.1600/036364411x605010
Fishbein, M., Livshultz, T., Straub, S. C. K., Simões, A. O., Boutte, J., McDonnell, A., et al. (2018). Evolution on the backbone: Apocynaceae phylogenomics and new perspectives on growth forms, flowers, and fruits. Am. J. Bot. 105, 495–513. doi: 10.1002/ajb2.1067
Folk, R. A., Siniscalchi, C. M., and Soltis, D. E. (2020). Angiosperms at the edge: extremity, diversity, and phylogeny. Plant Cell Environ. 43, 2871–2893. doi: 10.1111/pce.13887
Friis, E. M., Crane, P., and Pedersen, K. (2011). Early Flowers and Angiosperm Evolution. Cambridge: Cambridge University Press.
Gagnon, E., Ringelberg, J. J., Bruneau, A., Lewis, G. P., and Hughes, C. E. (2019). Global succulent biome phylogenetic conservatism across the pantropical Caesalpinia group (Leguminosae). New Phytol. 222, 1994–2008. doi: 10.1111/nph.15633
Goyder, D. J. (2006). “An overview of asclepiad biogeography,” in Taxonomy and Ecology of African Plants, Their Conservation and Sustainable Use, eds S. A. Ghazanfar and H. J. Beentje (Kew: Royal Botanic Gardens), 205–214.
Graham, A. (2011). The age and diversification of terrestrial New World ecosystems through Cretaceous and Cenozoic time. Am. J. Bot. 98, 336–351. doi: 10.3732/ajb.1000353
Graham, A. (2018). Land Bridges: Ancient Environments, Plant Migration and New World Connections. Chicago, IL: Chicago Press.
Hernández-Hernández, T., and Wiens, J. J. (2020). Why are there so many flowering plants? A multiscale analysis of plant diversification. Am. Nat. 195, 948–963. doi: 10.1086/708273
Herrera, C. M. (1989). Seed dispersal by animals: a role in angiosperm diversification? Am. Nat. 133, 309–322. doi: 10.1086/284921
Igea, J., and Tanentzap, A. J. (2020). Angiosperm speciation cools down in the tropics. Ecol. Lett. 23, 692–700. doi: 10.1111/ele.13476
Ionta, G. M., and Judd, W. S. (2017). Phylogenetic relationships in Periplocoideae (Apocynaceae s.l.) and insights into the origin of pollinia. Ann. Missouri Bot. Gard. 94, 360–375. doi: 10.3417/0026-6493(2007)94[360:pripas]2.0.co;2
Jacobs, B. F. (2004). Palaeobotanical studies from tropical Africa: relevance to the evolution of forest, woodland and savannah biomes. Philos. Trans. R. Soc. Lond. B 359, 1573–1583. doi: 10.1098/rstb.2004.1533
Janzen, D. H. (1974). Epiphytic myrmecophytes in Sarawak: mutualism through the feeding of plants by ants. Biotropica 6, 237–259. doi: 10.2307/2989668
Joyce, E. M., Thiele, K. R., Slik, J. W. F., and Crayn, D. M. (2021). Plants will cross the lines: climate and available land mass are the major determinants of phytogeographical patterns in the Sunda–Sahul convergence zone. Biol. J. Linn. Soc. 132, 374–387. doi: 10.1093/biolinnean/blaa194
Khanum, R., Surveswaran, S., Meve, U., and Liede-Schumann, S. (2016). Cynanchum (Apocynaceae: Asclepiadoideae): a pantropical asclepiadoid genus revisited. Taxon 65, 467–486. doi: 10.12705/653.3
Kidyoo, A. (2019). Heterostemma ficoides (Apocynaceae: Asclepiadoideae), a new species with fig-like flowers from Northern Thailand. Kew Bull. 74:26. doi: 10.1007/S12225-019-9815-4
Kingdon, J., Agwanda, B., Kinnaird, M., O’Brien, T., Holland, C., Gheysens, T., et al. (2011). A poisonous surprise under the coat of the African crested rat. Proc. R. Soc. B 279, 675–680. doi: 10.1098/rspb.2011.1169
Lahaye, R. (2005). Phylogenie Moleculaire des Secamonoideae (Apocynaceae s.l.): Histoire Biogéographique et Évolution des Formes de Croissance. [Thèse de Doctorat en Systématique Végétale. Ecologie et Evolution]. Toulouse: Toulouse III.
Lahaye, R., Klackenberg, J., Källersjö, M., van Campo, E., and Civeyrel, L. (2007). Phylogenetic relationships between derived Apocynaceae s.l. and within Secamonoideae based on chloroplast sequences. Ann. Missouri Bot. Gard. 94, 376–391. doi: 10.3417/0026-6493(2007)94[376:prbdas]2.0.co;2
Landis, M. J., Matzke, N. J., Moore, B. R., and Huelsenbeck, J. P. (2013). Bayesian analysis of biogeography when the number of areas is large. Syst. Biol. 62, 789–804. doi: 10.1093/sysbio/syt040
Larridon, I., Díaz, J. G., Bauters, K., and Escudero, M. (2020). What drives diversification in a pantropical plant lineage with extraordinary capacity for long-distance dispersal and colonization? J. Biogeogr. 48, 64–77. doi: 10.1111/jbi.13982
Lens, F., Endress, M. E., Baas, P., Jansen, S., and Smets, E. (2008). Wood anatomy of Rauvolfioideae (Apocynaceae): a search for meaningful non-DNA characters at the tribal level. Am. J. Bot. 95, 1199–1215. doi: 10.3732/ajb.0800159
Lens, F., Endress, M. E., Baas, P., Jansen, S., and Smets, E. (2009). Vessel grouping patterns in subfamilies Apocynoideae and Periplocoideae confirm phylogenetic value of wood structure within Apocynaceae. Am. J. Bot. 96, 2168–2183. doi: 10.3732/ajb.0900116
Liede, S., and Täuber, A. (2002). Circumscription of the genus Cynanchum (Apocynaceae-Asclepiadoideae). Syst. Bot. 27, 789–800. doi: 10.1043/0363-6445-27.4.789
Liede-Schumann, S., and Meve, U. (2018). Vincetoxicum (Apocynaceae—Asclepiadoideae) expanded to include Tylophora and allies. Phytotaxa 369, 129–184. doi: 10.11646/phytotaxa.369.3.1
Liede-Schumann, S., Khanum, R., Mumtaz, A. S., Gherghel, I., and Pahlevani, A. (2016). Going west – a subtropical lineage (Vincetoxicum, Apocynaceae: Asclepiadoideae) expanding into Europe. Mol. Phylogen. Evol. 94, 436–446. doi: 10.1016/j.ympev.2015.09.021
Liede-Schumann, S., Kong, H.-H., Meve, U., and Thiv, M. (2012). Vincetoxicum and Tylophora (Apocynaceae: Asclepiadoideae: Asclepiadeae) – two sides of the same medal: independent shifts from tropical to temperate habitats. Taxon 61, 803–825. doi: 10.1002/tax.614007
Liede-Schumann, S., Nikolaus, M., Soares e Silva, U. C., Rapini, A., Mangelsdorff, R. D., and Meve, U. (2014). Phylogenetics and biogeography of the genus Metastelma (Apocynaceae-Asclepiadoideae-Asclepiadeae: Metastelmatinae). Syst. Bot. 39, 594–612. doi: 10.1600/036364414x680708
Linder, H. P., and Crisp, M. D. (1995). Nothofagus and Pacific biogeography. Cladistics 11, 5–32. doi: 10.1111/j.1096-0031.1995.tb00002.x
Livshultz, T. (2010). The phylogenetic position of milkweeds (Apocynaceae subfamilies Secamonoideae and Asclepiadoideae): evidence from the nucleus and chloroplast. Taxon 59, 1016–1030. doi: 10.1002/tax.594003
Livshultz, T., Kaltenegger, E., Straub, S. C. K., Weitemier, K., Hirsch, E., Koval, K., et al. (2018). Evolution of pyrrolizidine alkaloid biosynthesis in Apocynaceae: revisiting the defence de-escalation hypothesis. New Phytol. 218, 762–773. doi: 10.1111/nph.15061
Livshultz, T., Mead, J. V., Goyder, D. J., and Brannin, M. (2011). Climate niches of milkweeds with plesiomorphic traits (Secamonoideae; Apocynaceae) and the milkweed sister group link ancient African climates and floral evolution. Am. J. Bot. 98, 1966–1977. doi: 10.3732/ajb.1100202
Livshultz, T., Middleton, D. J., Endress, M. E., and Williams, J. (2007). Phylogeny of Apocynoideae and the APSA clade. Ann. Missouri Bot. Gard. 94, 323–361.
Magallón, S., and Sanderson, M. J. (2001). Absolute diversification rates in angiosperm clades. Evolution 55, 1762–1780. doi: 10.1554/0014-3820(2001)055[1762:adriac]2.0.co;2
Magallón, S., Sánchez-Reyes, L. L., and Gómez-Acevedo, S. L. (2019). Thirty clues to the exceptional diversification of flowering plants. Ann. Bot. 123, 491–503. doi: 10.1093/aob/mcy182
Martínez-Millán, M. (2010). Fossil record and age of the Asteridae. Bot. Rev. 76, 83–135. doi: 10.1007/s12229-010-9040-1
Matzke, N. J. (2013). BioGeoBEARS: BioGeography with Bayesian (and Likelihood) Evolutionary Analysis in R Scripts. R Package, Version 0.2.1. Available online at: http://CRAN.R-project.org/package=BioGeoBEARS (accessed July 27, 2013)
Matzke, N. J. (2016). Stochastic Mapping Under Biogeographical Models. Available online at: http://phylo.wikidot.com/bio-geobears#stochastic_mapping (accessed January 2, 2021)
Matzke, N. J. (2021). Statistical comparison of DEC and DEC+J is identical to comparison of two ClaSSE submodels, and is therefore valid. OSF [Preprints] doi: 10.31219/osf.io/vqm7r
McElwain, J. C., and Punyasena, S. W. (2007). Mass extinction events and the plant fossil record. Trends Ecol. Evol. 22, 548–557. doi: 10.1016/j.tree.2007.09.003
McLoughlin, S. (2001). The breakup history of Gondwana and its impact on pre-Cenozoic floristic provincialism. Aust. J. Bot. 49, 271–300. doi: 10.1071/bt00023
Medina-Vega, J. A., Bongers, F., Poorter, L., Schitzer, S. A., and Sterck, F. J. (2021). Lianas have more acquisitive traits than trees in a dry but not in a wet forest. J. Ecol. 109, 2367–2384. doi: 10.1111/1365-2745.13644
Mendel, J. R., Dikow, R. B., Siniscalchi, C. M., Thapa, R., Watson, L. E., and Funk, V. A. (2019). A fully resolved backbone phylogeny reveals numerous dispersals and explosive diversifications throughout the history of Asteraceae. Proc. Natl. Acad. Sci. U.S.A. 116, 14083–14088. doi: 10.1073/pnas.1903871116
Meseguer, A. S., and Condamine, F. L. (2020). Ancient tropical extinctions at high latitudes contributed to the latitudinal diversity gradient. Evolution 74, 1966–1987. doi: 10.1111/evo.13967
Meseguer, A. S., Lobo, J. M., Cornuault, J., Beerling, D., Ruhfel, B. R., Davis, C. C., et al. (2018). Reconstructing deep-time palaeoclimate legacies in the clusioid Malpighiales unveils their role in the evolution and extinction of the boreotropical flora. Global Ecol. Biogeogr. 27, 616–628. doi: 10.1111/geb.12724
Meseguer, A. S., Lobo, J. M., Ree, R. H., Beerling, D. J., and Sanmartin, I. (2015). Integrating fossils, phylogenies, and niche models into biogeography to reveal ancient evolutionary history: the case of Hypericum (Hypericaceae). Syst. Biol. 64, 215–232. doi: 10.1093/sysbio/syu088
Meve, U., and Liede, S. (2002a). A molecular phylogeny and generic rearrangement of the stapelioid Ceropegieae (Apocynaceae-Asclepiadoideae). Plant Syst. Evol. 234, 171–209. doi: 10.1007/s00606-002-0220-2
Meve, U., and Liede, S. (2002b). Floristic exchange between mainland Africa and Madagascar: case studies in Apocynaceae-Asclepiadoideae. J. Biogeogr. 29, 865–873. doi: 10.1046/j.1365-2699.2002.00729.x
Meve, U., Heiduk, A., and Liede-Schumann, S. (2016). Origin and early evolution of Ceropegieae (Apocynaceae-Asclepiadoideae). Syst. Biodivers. 15, 143–155. doi: 10.1080/14772000.2016.1238019
Morley, R. J. (1998). “Palynological evidence for tertiary plant dispersal in the SE Asian region in relation to plate tectonics and climate,” in Biogeography and Geological Evolution of SE Asia, eds R. Hall and J. D. Halloway (Leiden: Backhuys), 211–234.
Morley, R. J. (2003). Interplate dispersal paths for megathermal angiosperms. Perspect. Plant Ecol. Evol. Syst. 6, 5–20. doi: 10.1078/1433-8319-00039
Movshovich, E. B. (1975). Fundamentals of elaboration of a general classification for gas and oil traps. Izv. Akad. Nauk SSSR Ser. Geol. 5, 89–98.
Muller, J. (1968). Palynology of the Pedawan and Plateau sandstone formations (Cretaceous-Eocene) in Sarawak, Malaysia. Micropaleontology 14, 1–37. doi: 10.2307/1484763
Nieto-Blázquez, M. E., Antonelli, A., and Roncal, J. (2017). Historical biogeography of endemic seed plant genera in the Caribbean: did GAARlandia play a role? Ecol. Evol. 23, 10158–10174. doi: 10.1002/ece3.3521
Nürk, N. M., Linder, H., Onstein, R., Larcombe, M., Hughes, C., Piñeiro Fernández, L., et al. (2020). Diversification in evolutionary arenas: assessment and synthesis. Ecol. Evol. 10, 6163–6182. doi: 10.1002/ece3.6313
Nürk, N. M., Michling, F., and Linder, P. (2018). Are the radiations of temperate lineages in tropic-alpine ecosystems pre-adapted? Glob. Ecol. Biogeogr. 27, 334–345. doi: 10.1111/geb.12699
Nürk, N. M., Uribe-Convers, S., Gehrke, B., Tank, D. C., and Blattner, F. (2015). Oligocene niche shift, Miocene diversification – cold tolerance and accelerated speciation rates in the St. John’s Worts (Hypericum, Hypericaceae). BMC Evol. Biol. 15:80. doi: 10.1186/s12862-015-0359-4
Ollerton, J., Liede-Schumann, S., Endress, M. E., Meve, U., Rech, A. R., Shuttleworth, A., et al. (2019). The diversity and evolution of pollination systems in large plant clades: Apocynaceae as a case study. Ann. Bot. 123, 311–325. doi: 10.1093/aob/mcy127
Peeters, C., and Wiwatwitaya, D. (2014). Philidris ants living inside Dischidia epiphytes from Thailand. Asian Myrmecol. 6, 49–61.
Pirie, M. D., Kandziora, M., Nürk, N. M., Le Maitre, N. C., de Kuppler, A. M., Gehrke, B., et al. (2019). Leaps and bounds: geographical and ecological distance constrained the colonisation of the Afrotemperate by Erica. BMC Evol. Biol. 19:222. doi: 10.1186/s12862-019-1545-6
Plummer, M., Best, N., Cowles, K., and Vines, K. (2006). CODA: convergence diagnosis and output analysis for MCMC. R News 6, 7–11.
Poinar, G. O. (2017). Ancient termite pollinator of milkweed flowers in Dominican amber. Am. Entomol. 63, 52–56. doi: 10.1093/ae/tmx011
Procheş, Ş, and Ramdhani, S. (2020). A global regionalisation based on the present-day distribution of broad plant lineages. Phytotaxa 442, 20–26. doi: 10.11646/phytotaxa.442.1.3
Pugliesi, L., and Rapini, A. (2015). Tropical refuges with exceptionally high phylogenetic diversity reveal contrasting phylogenetic structures. Int. J. Biodivers. 2015:e758019. doi: 10.1155/2015/758019
Rabosky, D. L. (2014). Automatic detection of key innovations, rate shifts, and diversity-dependence on phylogenetic trees. PLoS One 9:e89543. doi: 10.1371/journal.pone.0089543
Rabosky, D. L., Grundler, M., Anderson, C., Title, P., Shi, J. J., Brown, J. W., et al. (2014). BAMMtools: an R package for the analysis of evolutionary dynamics on phylogenetic trees. Methods Ecol. Evol. 5, 701–707. doi: 10.1111/2041-210X.12199
Ramírez-Barahona, S., Sauquet, H., and Magallón, S. (2020). The delayed and geographically heterogeneous diversification of flowering plant families. Nat. Ecol. Evol. 4, 1232–1238. doi: 10.1038/s41559-020-1241-3
Rapini, A., Bitencourt, C., Luebert, F., and Cardoso, D. (2021). An escape-to-radiate model for explaining the high plant diversity and endemism in campos rupestres. Biol. J. Linn. Soc. 133, 481–498. doi: 10.1093/biolinnean/blaa179
Rapini, A., van den Berg, C., and Liede-Schumann, S. (2007). Diversification of Asclepiadoideae (Apocynaceae) in the New World. Ann. Missouri Bot. Gard. 94, 407–422. doi: 10.3417/0026-6493(2007)94[407:doaait]2.0.co;2
Ree, R. H., and Smith, S. A. (2008). Maximum likelihood inference of geographic range evolution by dispersal, local extinction, and cladogenesis. Syst. Biol. 57, 4–14. doi: 10.1080/10635150701883881
Ree, R. H., Moore, B. R., Webb, C. O., and Donoghue, M. J. (2005). A likelihood framework for inferring the evolution of geographic range on phylogenetic trees. Evolution 59, 2299–2311. doi: 10.1111/j.0014-3820.2005.tb00940.x
Renner, S. (2004). Plant dispersal across the tropical Atlantic by wind and sea currents. Int. J. Plant Sci. 165(4 Suppl.), S23–S33. doi: 10.1086/383334
Ribeiro, P. L., Rapini, A., Damascena, L. S., and van den Berg, C. (2014). Plant diversification in the Espinhaço range: insights from the biogeography of Minaria (Apocynaceae). Taxon 63, 1253–1264. doi: 10.12705/636.16
Richardson, J. E., Chatrou, L. W., Mols, J. B., Erkens, R. H. J., and Pirie, M. D. (2004). Historical biogeography of two cosmopolitan families of flowering plants: Annonaceae and Rhamnaceae. Philos. Trans. R. Soc. Lond. B 359, 1495–1508. doi: 10.1098/rstb.2004.1537
Rose, J. P., Kleist, T. J., Löfstrand, S. D., Drewd, B. T., Schönenberger, J., and Sytsma, K. J. (2018). Phylogeny, historical biogeography, and diversification of angiosperm order Ericales suggest ancient Neotropical and East Asian connections. Mol. Phylogen. Evol. 122, 59–79. doi: 10.1016/j.ympev.2018.01.014
Ruhfel, B. R., Bove, C. P., Philbrick, C. T., and Davis, C. C. (2016). Dispersal largely explains the Gondwanan distribution of the ancient tropical clusioid plant clade. Am. J. Bot. 103, 1117–1128. doi: 10.3732/ajb.1500537
Salard-Cheboldaeff, M. (1978). Sur la palynoflore maestrichtiene et tertiaires du Bassin sédimentaire littoral du Cameroun. Pollen Spores 20, 215–260.
Sanmartín, I., and Ronquist, F. (2004). Southern Hemisphere biogeography inferred by event-based models: plant versus animal patterns. Syst. Biol. 53, 216–243. doi: 10.1080/10635150490423430
Schrire, B. D., Lavin, M., Nigel, P. B., and Forest, F. (2009). Phylogeny of the tribe Indigofereae (Leguminosae – Papilionoideae): geographically structured more in succulent-rich and temperate settings than in grass-rich environments. Am. J. Bot. 96, 816–852. doi: 10.3732/ajb.0800185
Schrire, B. D., Lewis, G. P., and Lavin, M. (2005). “Biogeography of the Leguminosae,” in Legumes of the World, eds G. Lewis, B. Schrire, B. Mackinder, and M. Lock (Richmond, VA: Kew Publishing), 21–54.
Sennblad, B., and Bremer, B. (1996). The familial and subfamilial relationships of Apocynaceae and Asclepiadaceae evaluated data with rbcL data. Plant Syst. Evol. 202, 153–175. doi: 10.1007/bf00983380
Simões, A. O., Endress, M. E., van der Niet, T., Kinoshita, L. S., and Conti, E. (2006). Is Mandevilla (Apocynaceae, Mesechiteae) monophyletic? Evidence from five plastid DNA loci and morphology. Ann. Missouri Bot. Gard. 93, 565–591. doi: 10.3417/0026-6493(2006)93[565:imamme]2.0.co;2
Simões, A. O., Kinoshita, L. S., Koch, I., Silva, M. J., and Endress, M. E. (2016). Systematics and character evolution of Vinceae (Apocynaceae). Taxon 65, 99–122. doi: 10.12705/651.7
Simões, A. O., Livshultz, T., and Endress, M. E. (2007). Phylogeny and systematics of the Rauvolfioideae (Apocynaceae) based on molecular and morphological evidence. Ann. Missouri Bot. Gard. 94, 268–297. doi: 10.3417/0026-6493(2007)94[268:pasotr]2.0.co;2
Simon, M. F., Grether, R., Queiroz, L. P., Skema, C., Pennington, R. T., and Hughes, C. E. (2009). Recent assembly of the Cerrado, a neotropical plant diversity hotspot, by in situ evolution of adaptations to fire. Proc. Natl. Acad. Sci. U.S.A. 106, 20359–20364. doi: 10.1073/pnas.0903410106
Singh, H., Judd, W. S., Samant, B., Agnihotri, P., Grimaldi, D. A., and Manchester, S. R. (2021). Flowers of Apocynaceae in amber from the early Eocene of India. Am. J. Bot. 108, 1–10. doi: 10.1002/ajb2.1651
Soltis, P. S., Folk, R. A., and Soltis, D. E. (2019). Darwin review: angiosperm phylogeny and evolutionary radiations. Proc. R. Soc. B 286, e2019.0099. doi: 10.1098/rspb.2019.0099
Stebbins, G. L. (1952). Aridity as a stimulus to plant evolution. Am. Nat. 86, 33–44. doi: 10.1086/281699
Stebbins, G. L. (1974). Flowering Plants: Evolution above the Species Level. Cambridge, MA: Harvard University Press.
Straub, S. C., Moore, M. J., Soltis, P. S., Soltis, D. E., Liston, A., and Livshultz, T. (2014). Phylogenetic signal detection from an ancient rapid radiation: effects of noise reduction, long-branch attraction, and model selection in crown clade Apocynaceae. Mol. Phylogen. Evol. 80, 169–185. doi: 10.1016/j.ympev.2014.07.020
Su, T., Spicer, R. A., Wu, F.-X., Farnsworth, A., Huang, J., Del Rio, C., et al. (2020). A middle Eocene lowland humid subtropical “Shangri-La” ecosystem in central Tibet. Proc. Natl. Acad. Sci. U.S.A. 117, 32989–32995. doi: 10.1073/pnas.2012647117
Sun, M., Folk, R. A., Gitzendanner, M. A., Soltis, P. S., Chen, Z., Soltis, D. E., et al. (2020). Recent accelerated diversification in rosids occurred outside the tropics. Nat. Commun. 11:3333. doi: 10.1038/s41467-020-17116-5
Tiffney, B. H. (1985a). Perspectives on the origin of the floristic similarity between eastern Asia and eastern North America. J. Arnold Arbor. 66, 73–94. doi: 10.5962/bhl.part.13179
Tiffney, B. H. (1985b). The Eocene North Atlantic land bridge: its importance in Tertiary and modern phytogeography of the Northern Hemisphere. J. Arnold Arbor. 66, 243–273. doi: 10.5962/bhl.part.13183
Tiffney, B. H., and Mazer, S. J. (1995). Angiosperm growth habit, dispersal and diversification reconsidered. Evol. Ecol. 9, 93–117. doi: 10.1007/bf01237700
Vamosi, J. C., and Vamosi, S. M. (2011). Factors influencing diversification in angiosperms: at the crossroads of intrinsic and extrinsic traits. Am. J. Bot. 98, 460–471. doi: 10.3732/ajb.1000311
van den Ende, C., White, L. T., and van Welzen, P. C. (2017). The existence and break-up of the Antarctic land bridge as indicated by both amphi-Pacific distributions and tectonics. Gondwana Res. 44, 219–227. doi: 10.1016/j.gr.2016.12.006
Wang, W., Ortiz, R. C., Jacques, F. M. B., Xiang, X.-G., Li, H.-L., Lin, L., et al. (2012). Menispermaceae and the diversification of tropical rainforests near the Cretaceous-Paleogene boundary. New Phytol. 195, 470–478. doi: 10.1111/j.1469-8137.2012.04158.x
Wheeler, E., Lee, M., and Matten, L. C. (1987). Dicotyledonous woods from the Upper Cretaceous of southern Illinois. Bot. J. Linn. Soc. 95, 77–100. doi: 10.1111/j.1095-8339.1987.tb01990.x
Wolfe, J. A. (1975). Some aspects of plant geography of the Northern Hemisphere during the late Cretaceous and Tertiary. Ann. Missouri Bot. Gard. 62, 264–279. doi: 10.2307/2395198
Wolfe, J. A. (1978). A paleobotanical interpretation of Tertiary climates in the Northern Hemisphere. Am. Sci. 66, 694–703.
Xiong, W., Ollerton, J., Liede-Schumann, S., Zhao, W., Jiang, Q., Sun, H., et al. (2020). Specialized cockroach pollination in the rare and endangered plant Vincetoxicum hainanense in China. Am. J. Bot. 107, 1–11. doi: 10.1002/ajb2.1545
Zhang, C., Zhang, T., Luebert, F., Xiang, Y., Huang, C.-H., Hu, Y., et al. (2020). Asterid phylogenomics/phylotranscriptomics uncover morphological evolutionary histories and support phylogenetic placement for numerous whole-genome duplications. Mol. Biol. Evol. 37, 3188–3210. doi: 10.1093/molbev/msaa160
Keywords: apocynoids, APSA clade, Asclepiadoideae, biogeography, Gondwana, Laurasia, long-distance dispersal, rauvolfioids
Citation: Bitencourt C, Nürk NM, Rapini A, Fishbein M, Simões AO, Middleton DJ, Meve U, Endress ME and Liede-Schumann S (2021) Evolution of Dispersal, Habit, and Pollination in Africa Pushed Apocynaceae Diversification After the Eocene-Oligocene Climate Transition. Front. Ecol. Evol. 9:719741. doi: 10.3389/fevo.2021.719741
Received: 03 June 2021; Accepted: 27 August 2021;
Published: 04 October 2021.
Edited by:
Danilo M. Neves, Federal University of Minas Gerais, BrazilReviewed by:
Edeline Gagnon, Royal Botanic Garden Edinburgh, United KingdomCopyright © 2021 Bitencourt, Nürk, Rapini, Fishbein, Simões, Middleton, Meve, Endress and Liede-Schumann. This is an open-access article distributed under the terms of the Creative Commons Attribution License (CC BY). The use, distribution or reproduction in other forums is permitted, provided the original author(s) and the copyright owner(s) are credited and that the original publication in this journal is cited, in accordance with accepted academic practice. No use, distribution or reproduction is permitted which does not comply with these terms.
*Correspondence: Alessandro Rapini, cmFwaW5pYm90QHlhaG9vLmNvbS5icg==; Sigrid Liede-Schumann, c2lncmlkLmxpZWRlQHVuaS1iYXlyZXV0aC5kZQ==
†ORCID: Cássia Bitencourt, orcid.org/0000-0001-9141-2323; Nicolai M. Nürk, orcid.org/0000-0002-0471-644X; Alessandro Rapini, orcid.org/0000-0002-8758-9326; Mark Fishbein, orcid.org/0000-0003-3099-4387; André O. Simões, orcid.org/0000-0001-6555-8759; David J. Middleton, orcid.org/0000-0003-3754-1452; Ulrich Meve, orcid.org/0000-0001-7395-5199; Sigrid Liede-Schumann, orcid.org/0000-0003-2707-0335
‡Present address: Cássia Bitencourt, Royal Botanic Gardens, Kew, Richmond, United Kingdom
Disclaimer: All claims expressed in this article are solely those of the authors and do not necessarily represent those of their affiliated organizations, or those of the publisher, the editors and the reviewers. Any product that may be evaluated in this article or claim that may be made by its manufacturer is not guaranteed or endorsed by the publisher.
Research integrity at Frontiers
Learn more about the work of our research integrity team to safeguard the quality of each article we publish.