- 1Department of Biology, University of Massachusetts Dartmouth, Dartmouth, MA, United States
- 2Department of Biology, University of Illinois Urbana-Champaign, Urbana, IL, United States
Predators have a major influence on prey populations and broader ecosystem dynamics through both their consumptive and non-consumptive effects. Prey employ risk-induced trait responses such as shifts in habitat use or changes in foraging behavior in response to the presence of predators. Risk-induced changes in foraging depend upon both the predator community and the environmental context; however, the influence of these factors have rarely been concurrently examined in free-living animals. We investigated the interactive effects of habitat, refuge availability, and predator type on the foraging behavior of free-living white-footed mice, accounting for the abiotic factor moonlight. We used auditory calls of a local terrestrial cursorial predator and a local avian ambush predator to simulate predation risk in both a forest edge and a forest interior habitat, and measured the foraging of mice under different experimental refuge types. We found that, while mice had reduced foraging when exposed to predation risk, the degree of this response depended on an interaction among habitat, refuge use, and type of predator. Prey had the greatest reduction in foraging and used refuges the most when exposed to cursorial-hunting foxes at the open forest edge. The risk-induced reduction in foraging and the use of refuges was much weaker in the forest interior, but even here foxes elicited a greater response as compared to owls. Generally, foraging tended to decrease with increasing moonlight, but this was not significant. We suggest that it is the temporal nature of cursorial vs. ambush predators in our system that drives such effects as opposed to their hunting mode, and that prey responses to temporal hotspots of risk need further examination. Generally, our results show that wild small-mammal prey species have variable responses to predation risk depending on the environmental context in which risk occurs.
Introduction
Predators have a major influence on prey populations and broader ecosystem dynamics through both their consumptive (i.e., killing; Sih et al., 1985; Krebs et al., 2001) and non-consumptive effects (Lima and Dill, 1990; Werner and Peacor, 2003; Sheriff et al., 2020b). For example, the mere presence of predators can result in risk-induced trait responses in prey behavior, morphology, and physiology (Lima, 1998; Peacor et al., 2020). These responses can scale up to alter prey fitness (Sheriff et al., 2009; Zanette et al., 2011), with potential effects on prey population size and community dynamics (Sheriff et al., 2020b). These changes have been observed across a wide range of taxa, including mammals (e.g., Creel et al., 2007; Sheriff et al., 2011; Cherry et al., 2016), birds (e.g., Fontaine and Martin, 2006), fish (e.g., McGhee et al., 2020), and invertebrates (e.g., Hermann and Thaler, 2014). Importantly, these changes may depend upon the environment in which they are elicited. Thus, understanding risk-induced effects in free-living prey under different contexts is an important part of understanding risk effects in natural systems (Peacor et al., 2020; Sheriff et al., 2020b).
One of the most well-studied risk-induced trait response in prey is a change in behavior (Lima and Dill, 1990; Lima, 1998). Many studies show a reduction in activity and a shift in habitat use corresponding to reduced foraging and increased refuge use, respectively, in response to predation risk (e.g., Werner et al., 1983; Heithaus and Dill, 2002; Ferrari et al., 2009). Sih (1984) showed that greater risk induces greater refuge use by prey. Longland and Price (1991) showed that various rodent species alter habitat use in response to increased predation risk, preferentially foraging in habitats with greater refuge availability. Orrock and Fletcher (2014) found that deer mice reduced their foraging in response to increased predation risk by re-introduced foxes; however, the reduction in foraging was highly dependent on the availability of shelter and moonlight. On nights with low moon illumination, mice foraged less in patches that had no shelter and more in patches that had shelter as fox abundance increased, but the effect was opposite during nights of low moon illumination. This may be because shelter provided overhead cover, allowing mice to avoid being detected by avian predators on nights with lower moon illumination but perhaps providing less of a benefit on nights with higher moon illumination, when the mice were more vulnerable to fox attacks (Orrock and Fletcher, 2014). These results suggest that the effect of predation risk on refuge use is highly dependent on other contextual factors, including moonlight illumination.
Predator type (e.g., terrestrial vs. avian, which in some cases may influence hunting mode) has also been shown to affect the risk-induced behavioral responses of prey (Preisser et al., 2007; Schmitz, 2008). For example, Embar et al. (2014) found that gerbils reduced their foraging more in response to the presence of owls than they did in response to the presence of snakes. Predator type has also been shown to influence microhabitat and refuge use. Kotler et al. (1991) found that gerbils forage more under the cover of bushes than they do in the open when exposed to cues that signify the presence of owls. However, gerbils exposed to cues that signify the presence of snakes preferentially forage in open microhabitats and avoid foraging under bushes (Kotler et al., 1993). Although its impact is highly context-dependent, predator hunting mode has also been shown to affect the behavior of prey, with sit-and-wait ambush predators generally expected to elicit stronger prey responses when compared to actively hunting predators (Preisser et al., 2007). This is likely because the attacks of ambush predators are more predictable in space and time than those of cursorial predators, causing prey to exhibit anti-predator behavior on a more constant basis.
Thus, risk-induced changes in foraging depend upon refuge availability, the predator community, and the environment in which these interactions occur. Although many studies have examined the interactive effects of some of these factors (e.g., Preisser et al., 2007; Wilson and Cooper, 2007; Miller et al., 2013), the influence of all three of these factors have not been examined concurrently in free-living animals. In addition, the effects of refuge availability and microhabitat on foraging behavior can be influenced by environmental characteristics, such as moon phase. Generally, mammals tend to forage more actively when there is lower moonlight intensity, presumably because these conditions make it less likely that they will be spotted by a predator (Prugh and Golden, 2014; Loggins et al., 2019). For example, Kotler et al. (2010) showed that gerbils forage less and display more vigilant behavior during brighter moon phases. Also, white-footed mice have been shown to only forage under a certain threshold of ground-level moonlight intensity (Guiden and Orrock, 2019).
Here, we investigate the combined effects of refuge availability, predator type (which also influences predator hunting mode), and habitat on prey foraging behavior in free-living white-footed mice, Peromyscus leucopus, while accounting for the environmental covariate of moon illumination. White-footed mice are an ideal species to investigate the combined effects of contextual factors on risk-induced behavioral responses; they are preyed on by both avian and terrestrial predators, and studies have shown reduced foraging responses to both predator types (e.g., Orrock and Fletcher, 2014; Giordano et al., submitted1). They also occupy a wide range of habitats (which may be more or less risky depending on their predators; Witmer and Moulton, 2012). Using auditory playbacks of either avian (ambush sit-and-wait hunting) or mammalian (cursorial chase hunting) predators, we measured the foraging behavior of white-footed mice with access to various refuge types at the forest edge (open habitat, 10 m from a distinct forest edge) and forest interior (closed canopy with dense understory, 70 m from the same distinct forest edge). We tested the hypothesis that the risk-induce trait responses of prey can vary according to the risk perceived during a foraging attempt and the environment in which the attempt occurs. We expected that mice exposed to predation risk would forage less overall and would forage more under refuges compared to in patches without refuge. Furthermore, we expected that mice would forage more in the forest interior compared to at the forest edge. We predicted that:
(1) Mice would forage the most under the refuge that provided the greatest protection from the specific predator’s hunting mode. Specifically, we predicted that mice would forage most under the refuge that provided vertical cover (i.e., closed top but open sides) when exposed to owl playbacks, but forage most under the refuge that provided horizontal cover (i.e., closed sides but open top) when exposed to mammalian playbacks. In both cases, we predicted that mice would forage the least in habitats without refuges due to higher perceived predation risk in open habitats regardless of predator type.
(2) Mice would have the lowest foraging effort in response to the predator that posed the most risk in a given habitat. As such, we predicted that mice at the forest edge would reduce foraging the most in response to owl playbacks (given the more open habitat), and that mice in the forest interior would reduce foraging the most in response to mammalian playbacks (given the denser understory protecting them from owls but not as much from foxes, who are shorter than the understory canopy).
(3) Alternatively, prey may reduce foraging the most to owls regardless of habitat or refuge, given that they are sit-and-wait ambush predators, which are generally predicted to elicit a greater anti-predator response (Preisser et al., 2007; Schmitz, 2008).
Materials and Methods
Study Location and Study Species
This study occurred within a forested tract of land 2 km long and 0.65 km wide surrounded by low density rural housing and farms in Westport, Massachusetts from August 8th to September 20th, 2019. The study area was dominated by oak trees (Quercus sp.) and holly trees (Ilex sp.) with a thick understory of Vaccinum sp. The edge habitat also included various willow species. Deer, coyotes, bobcats, foxes, fishers, rabbits and small mammals are readily seen or heard. Based on observations from trapping and camera footage, the small mammal community in the area is dominated by white-footed mice (P. leucopus) and chipmunks (Tamias striatus) and includes few other species. Barred owls (Strix varia) and red-tailed hawks (Buteo jamaicensis) are the prevalent avian predators in the area, with great horned owls (Bubo virginianus) and eastern screech owls (Megascops asio) also present at a lower density. The study design aimed to primarily target white-footed deer mice (P. leucopus), a nocturnal small mammal species. To attempt to exclude diurnal species such as chipmunks (T. striatus), data was only collected between the hours of 7 pm and 7 am. To exclude larger seed-eating species, mice could only gain access to seeds through small openings in the feeding trays. Although footprints characteristic of white-footed mice were observed in trays over the course of the experiment, there were no footprints that suggested the presence of other species.
Predation Risk Manipulation
To manipulate predation risk, we used auditory calls/playbacks of local predators and non-predators (Zanette et al., 2011; Suraci et al., 2016). A speaker system was positioned approximately 40 m into the forest, such that it was equidistant from foraging trays at the forest edge (10 m into the forest) and forest interior (70 m into the forest) habitats. The speaker system played calls for 40% of the time between 7 pm and 7 am at approximately 70 dB (at the source of sound), which attenuated to approximately 68 dB at the foraging trays. In addition, given that predators do not call while they are attacking, the speaker system included motion detecting infrared sensors, such that any motion within 1 m of the foraging trays triggered the speaker system to stop playbacks for 30 s. Playbacks included: a barred owl (S. varia) avian predator treatment; a red fox (Vulpes vulpes) mammalian predator treatment; and a spring peeper (Pseudacris crucifer) noise control treatment.
Giving-Up Density Measurements
We measured foraging behavior using the giving-up density (GUD) technique (Brown et al., 1988). The GUD technique involves setting out trays filled with a set amount of food homogenously distributed in an inedible substrate like sand or soil. The amount of food that remains after a forager gives up on feeding from the tray is measured as its GUD. This indicates the point at which the benefits of foraging are equal to or less than the costs of continuing to forage, which include metabolic costs, missed-opportunity costs, and the risk of predation (Brown, 1988; Brown et al., 1997).
We placed three foraging trays (32 cm × 11 cm × 17.5 cm) 1 m apart both 10 m (forest edge) and 70 m (forest interior) into the forest (i.e., from the forest edge). In each group of three trays, two were enclosed by either a table-like refuge (30 cm tall with a 60 × 60 cm covered top and open sides) or an open-topped box refuge (30 cm tall with 60 cm wide closed sides and an open top, walls sloped inward at 30 degrees; mice could easily access the foraging tray under the sloping walls which remained at least a few inches above the substrate). One foraging tray was left in a patch without a refuge. Refuges were constructed with plywood and painted brown with non-toxic, non-scented paint. Two infrared sensors per group of foraging trays were embedded in 1 m tall PVC pipe stands, which were also painted brown. These sensors detected small mammal movement within 1 m of any foraging tray and triggered the speaker system to stop playbacks for 30 s. Each foraging tray contained 0.5 L sand and 2.5 g seeds. All trays were placed in the forest at 7 pm and collected at 7 am. Once collected, seeds were sifted out of the sand. The seeds were then dried at 60°C for 2 h to remove moisture accumulated in them from ambient humidity. Seeds were then weighed to estimate the GUD for each tray on each night.
Study Design
The speaker system described above was set up at the study site. Each auditory call treatment was played for 2 consecutive nights with 1–2 nights of silence (no playbacks) in between. Separate trays were deployed on each of these consecutive nights for the purpose of collecting GUDs. There were six trays deployed on each of the two playback nights: three at the forest interior and three at the forest edge. At each location, these trays were spaced 1 m apart so that each of them was under a different refuge condition (1 under a table-like refuge, 1 under a box-like refuge, and 1 in a non-refuge patch). This resulted in the generation of 6 GUDs per night, and 12 GUDs per treatment round. This procedure was repeated for 10 rounds, resulting in 120 GUDs total (3 of which were discarded because they were improperly dried before weighing). The position of the refuge types was randomized between rounds. We semi-randomized the order of the treatments to control for potential variation due to the timing of the experiment. The treatments were run in the order of: Control (C), Owl (O), Control (C), Fox (F), O, C, F, O, F, C. No experiments were conducted concurrent with rainfall.
Statistical Analysis
GUDs (n = 117) were analyzed using a linear mixed regression model examining the interactive effects of predator treatment (control/fox/owl), habitat (forest interior/forest edge), and refuge (enclosed/non-refuge patch) on mice foraging behavior, thereby controlling for the block design of our study. The degrees of freedom (here: denominator-degrees-of-freedom) were adjusted using the Satterthwaite method. We found no statistical difference between the two different types of enclosed refuges (t = −0.179, p = 0.858), so we grouped them. To simplify the presentation of the data, each GUD was converted into the amount of food eaten by subtracting the GUD from the initial weight of the seed (2.5 g seed – GUD). These values were then log-transformed. One observation was excluded to obtain normality of model residuals. We included moon illumination as a covariate in the regression model (R package lunar v.0.1-04). Moon illumination was calculated from moon phase, which was determined by the date of data collection. A full moon was recorded as 100% illumination, whereas a new moon was recorded as 0% illumination. Day was also included as a fixed effect to control for potential differences between consecutive experiment days within experiments. Day could not be included as a random effect, as this variable only has two levels and the recommended minimum levels of a factor to be included as a random effect is 5 (Zuur et al., 2009). Experiment (defined as each set of 2 consecutive days in one replicate; N = 10) was included as a random effect to control for environmental differences between replicates, such as ambient temperature or seasonal change. Post hoc tests were conducted with the emmeans package (v.1.5.2-1), using the Holm-Bonferroni p-value correction for multiple testing. Model residuals were visually checked to conform to the assumption of homogeneity of variance; no patterns were observed in model residuals. All data were analyzed using R v.4.0.3 in RStudio v.1.3.1093. An all-pair comparison table of the ANOVA is presented in Supplementary Table 1.
Results
We found a significant effect of habitat and refuge (Table 1). Overall, mice ate 29% less food at the forest edge (Mean GUD = 0.936 g) compared to the forest interior (Mean GUD = 0.286 g; t117,1 = 11.834, p < 0.0001). Mice ate 7% less food at trays in patches without refuges (Mean GUD = 0.598 g) compared to those enclosed in refuges (Mean GUD = 0.448 g; t117,1 = −2.931, p = 0.004; Figure 1). Moon illumination and day were not significant (Table 1), although food eaten tended to decrease with increasing moon illumination (Figure 2). While treatment only tended to be significant (p = 0.064), the interaction between treatment and habitat was significant (Table 1). The fixed effects explained 48% of the variation in GUDs (marginal R2; R package MuMIn v.1.43.17; Nakagawa et al., 2017) while the full model explained 76% of the variation in GUDs (conditional R2).
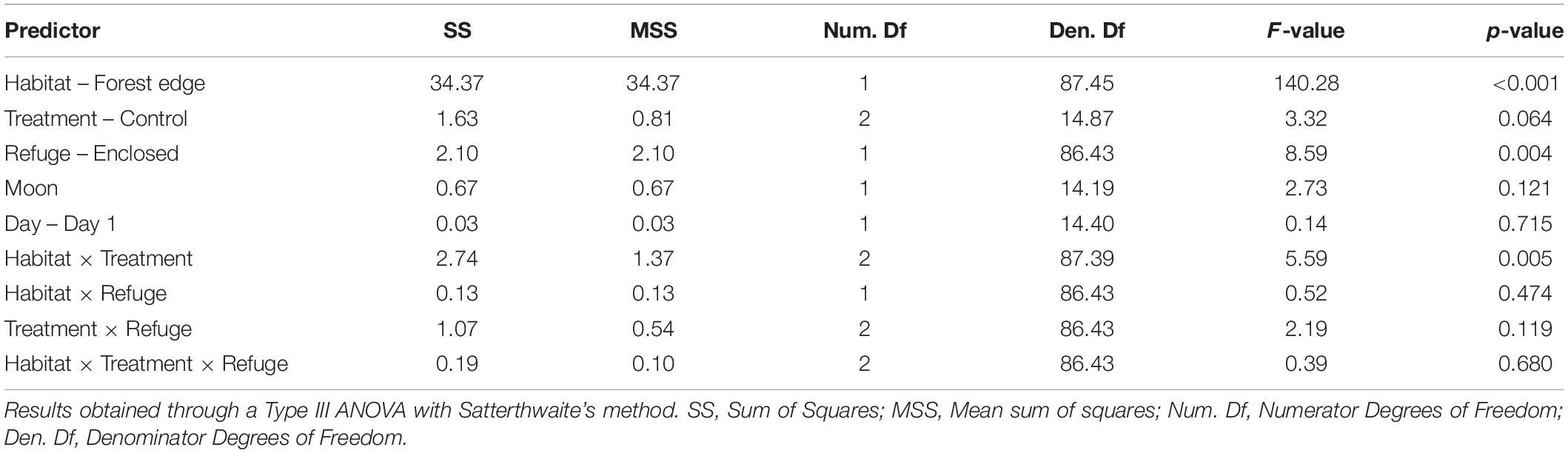
Table 1. Model output showing the effects of habitat, refuge, risk treatment, moon illumination, and day on the amount of food eaten by white-footed mice.
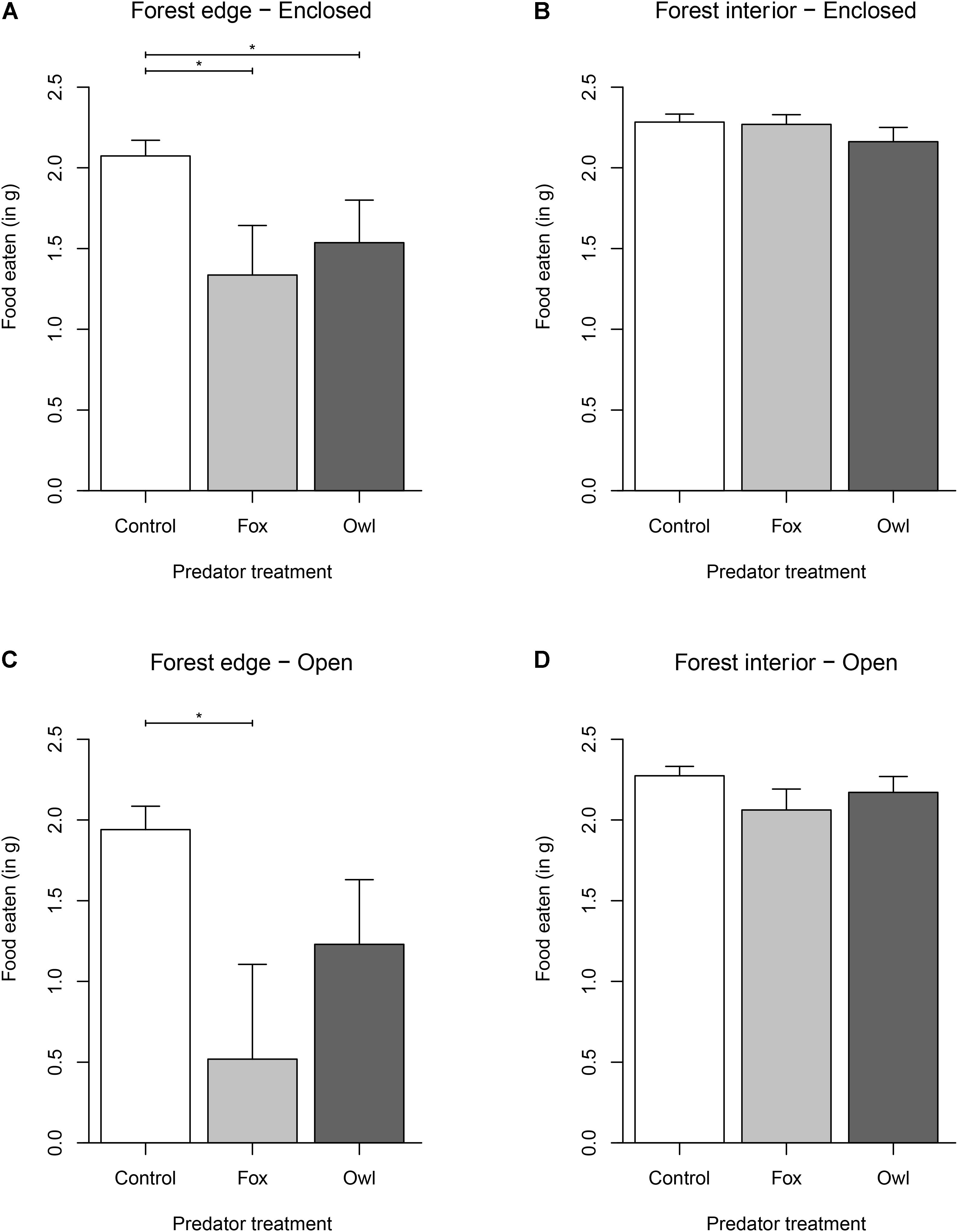
Figure 1. Effect of habitat [forest edge (A–C) vs. forest interior (B–D)], refuge [open (C,D) vs. enclosed (A,B)], and treatment [control (white) vs. fox (light gray) vs. owl (dark gray)] on the amount of food eaten (2.5 g – GUD) by free-living white-footed mice. Upper 95% confidence interval is shown as whiskers. Asterisks indicate significant differences (p < 0.05) among groups.
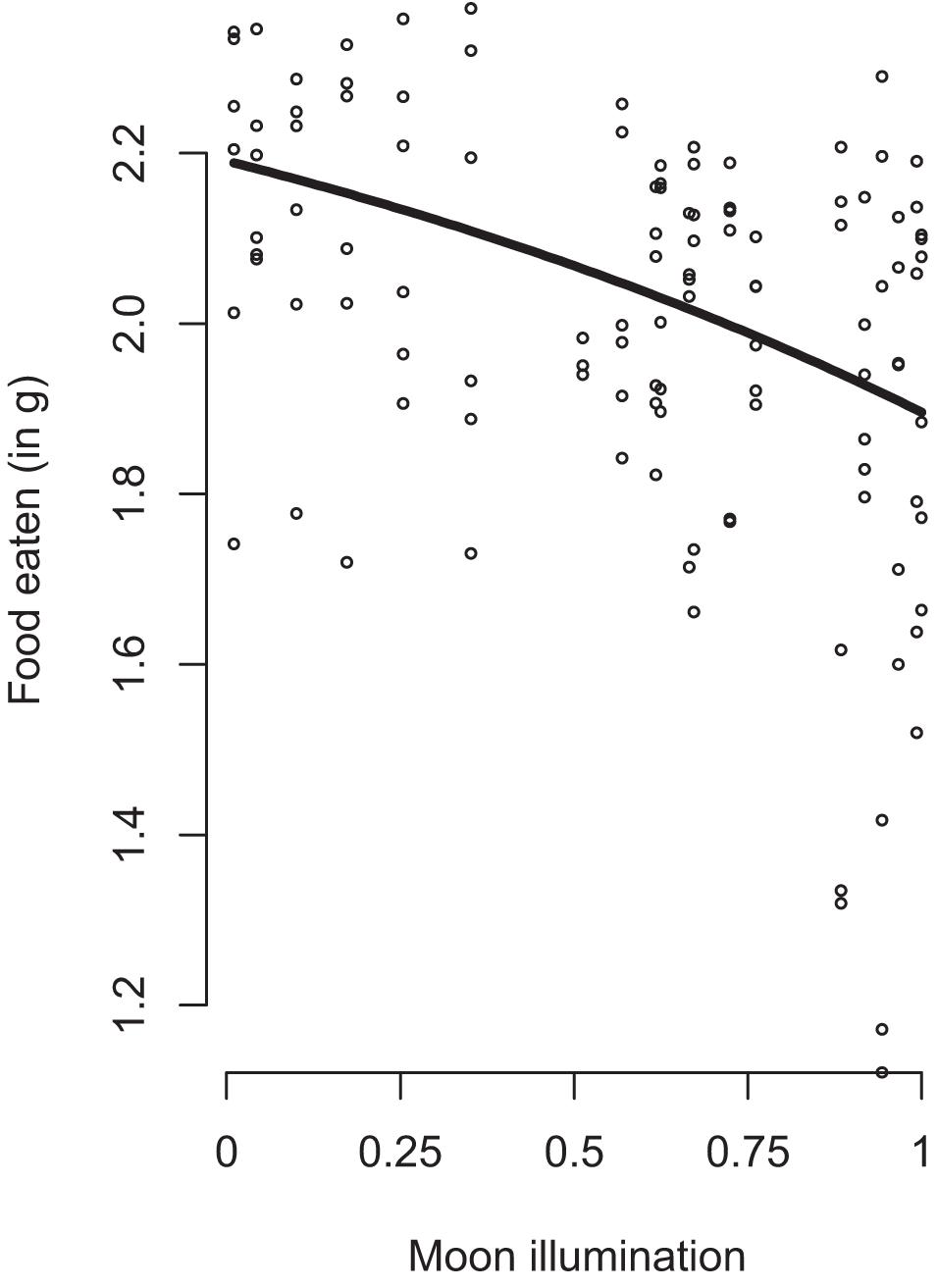
Figure 2. The effect of relative moon illumination (black line; 0 at new moon, 1 at full moon) on the amount of food eaten by free-living white-footed mice. 95% Confidence interval shown as gray area. Dots represent datapoints. Moon illumination was based on date of data collection and accessed through the R package lunar.
When we compared different predator effects within a single habitat, we found that mice at the forest edge reduced foraging by 51% when exposed to fox playbacks (Mean GUD = 1.518 g; t117,2 = −3.398, p = 0.009) and 31% when exposed to owl playbacks (Mean GUD = 1.106 g; t117,2 = −2.366, p = 0.0553; Figure 1) compared to the control (Mean GUD = 0.488 g). However, in the forest interior, predator playbacks of either fox or owl had no effect on foraging. Mice ate 20% less food at trays in refuge-less patches at the forest edge (Mean GUD = 1.121 g) in comparison to the amount they ate in enclosed trays at the forest edge (Mean GUD = 0.782 g; t117,1 = −2.529, p = 0.0132), but there was no effect of refuge in the forest interior.
Discussion
In this study, we examined foraging behavior in free-living white-footed mice to test the hypothesis that anti-predator responses depend on the environmental context under which they occur. Our results supported this hypothesis; in general, mice reduced foraging when exposed to predation risk, but the degree of this response differed based on habitat, refuge use, and predator type. Moonlight tended to reduce small mammal foraging, but not significantly as has been shown in other studies (Guiden and Orrock, 2019; Loggins et al., 2019; Figure 2). Overall, our results support the hypothesis that risk-induced trait responses of prey depend upon the context in which they occur.
Habitat has been shown to be a major factor influencing prey responses to predation risk. Studies have shown that prey will forage less in risky habitats and that, when exposed to predation risk, they will move from riskier to safer habitats (Lima and Dill, 1990). For example, granivorous rodents have been shown to preferentially forage in microhabitats that provide more cover (Brown, 1988). Similar effects have been found across taxa. In insects, for example, Kohler and McPeek (1989) found that baetis larvae avoided foraging in the top level of substrate (where predation risk is higher) when predator cues were present. Here, we found that mice ate significantly less at the forest edge as compared to the forest interior, regardless of refuge use or what predator type they were exposed to. In our study area there was a very distinct forest edge, delineated by sparse shrub and meadow habitat. Within the forest at the edge there was also less understory cover. Overall, this likely results in a riskier habitat with far less natural cover. Our finding that mice forage less in the risky habitat (forest edge) as compared to the safer habitat (forest interior) therefore corroborates previous research on prey anti-predator responses.
We also found that mice ate significantly more under refuges than they ate in patches without refuge. However, this result was confounded by habitat type—mice ate more under refuges only in the riskier forest edge habitat, while, in the safer forest interior habitat, there was no significant difference between the amount of food eaten under refuges in comparison to the amount of food eaten in patches without refuge. This is likely explained by the dense understory cover in the forest interior, which may have rendered the additional cover provided by refuges redundant. A similar effect has been found in some previous studies. Orrock et al. (2004), for example, showed that deer mice used refuges significantly more when exposed to predation risk. However, this effect was confounded by various aspects of environmental context which indirectly affect predation risk, such as foraging microhabitat, moon illumination, and precipitation. Donelan et al. (2017) found that snails increased the use of refuge and avoided foraging in open habitats in response to increases in predation risk. Thus, while studies have shown that prey utilize refuges in response to predation risk (Werner et al., 1983), the importance of such refuges in prey risk responses may be a consequence of the general habitat structure, increasing in importance as the habitat becomes riskier.
We also found that predator type, associated with different hunting modes, influenced prey foraging, and that this effect was also confounded by habitat type. Although it is generally assumed that sit-and-wait, ambush predators have a greater effect on prey, we found the opposite. At the forest edge, where we predicted owls to have the largest effect, we found mice reduced foraging by 51% in response to the fox treatment, whereas they only reduced foraging by 31% in response to the owl treatment. We found no effect of predation risk in the safer forest interior—however, fox playbacks overall (across both habitat types) reduced mouse foraging by much more than owls. We also found mice had a 3× increase in foraging under refuges when they were exposed to foxes at the forest edge, but refuges had little effect on mouse foraging when they were exposed to owls at the forest edge. This finding corroborates previous studies which have found differential prey responses to predation risk depending on predator identity. For example, Preisser et al. (2007) found that some predators elicit stronger non-consumptive effects than others, and Embar et al. (2014) found that prey use refuge differently in the presence of owls when compared to their refuge use in the presence of snakes.
Overall, the cursorial predator treatment clearly had a larger effect as compared to the ambush predator treatment. The greater effect of foxes (a terrestrial cursorial predator) as compared to owls (an avian ambush predator) may be driven by the possibility that foxes consume more mice as compared to owls and, thus, induce greater anti-predator responses. Our experimental design was unable to test whether foxes or owls are more lethal. However, it may not be as simple as the predator with the greater lethality inducing the greater response. Mice may have greater relative increases in proportional fitness with greater responses to foxes as compared to owls (Sheriff et al., 2020a); i.e., increasing the response to owls may provide no additional fitness benefits, whereas increasing the response to foxes does provide additional fitness benefits. Similarly, the greater response may be influenced by local densities and familiarity with each predator. Although we do not know the exact densities of either predator, prevalent signs of both predators have been seen and heard in the area.
Differences in predator hunting mode may also contribute to the differences in the responses of mice. Most studies that have examined the effects of predator hunting mode compare systems where ambush predators set up hotspots of high predation risk (creating a landscape of fear; Laundré et al., 2001; Gaynor et al., 2019; Smith et al., 2019) and prey are highly mobile. In such systems, prey can make spatiotemporal decisions across the landscape on where and when to forage. This contrasts systems where cursorial predators actively pursue prey (and as such do not set up hotspots of risk across the landscape in the same manner as ambush predators; Sih et al., 1998; Schmitz, 2008). In our system, owls often hunt in a given area over a certain number of nights, an area that is as large if not larger than a mouse’s entire home range. Thus, while owls are ambush style hunters swooping down to attack their prey unaware, owls do not set areas of localized hotspots that mice can avoid. Rather, a mouse’s entire home range becomes very risky when an owl is nearby. In contrast, foxes’ cursorial, search-and-pursue hunting style may result in them creating acute temporal periods (minutes to hours) of high risk when they are within a small mammal’s home range; i.e., we suggest that hearing a fox may indicate an acute localized threat, but hearing an owl may indicate a more constant unlocalized threat. Thus, small mammals may respond to predators as predicted by the risk allocation hypothesis, which predicts stronger risk responses during acute high-risk scenarios and reduced risk responses as those high-risk scenarios become prolonged (Lima and Bednekoff, 1999), rather than as predicted by predator-hunting-mode hypotheses (Schmitz, 2008).
In our study, we examined how prey responses were influenced by the interactive effects of habitat, refuge availability, and predator type. We found that, while mice generally foraged less when exposed to predation risk, the degree of this response depended upon an interaction among habitat, refuge use, and type of predator. Prey foraged the least and used refuges the most when exposed to cursorial-hunting foxes at the open forest edge. The risk-induced reduction in foraging and the preferential use of refuges was much weaker in the forest interior. Interestingly, we found that cursorial hunting foxes elicited a stronger effect than ambush hunting owls regardless of habitat type or refuge availability. We suggest that the temporal nature of cursorial predators (i.e., an acute localized threat as they move through an area) as compared to ambush predators in our system drives these results. More work needs to be done examining the effects of temporal hotspots on prey risk responses and what role these may play in hypotheses related to the landscape of fear. Together, our results offer support for the risk allocation hypothesis, and show that wild small mammal prey species can have considerably different foraging behavior in response to predation risk depending upon environmental context. This study, thus, addresses an important yet understudied aspect of predator-prey interactions—the effects of different interacting environmental factors on prey responses to predation risk.
Our findings have important implications for the way prey respond to predation risk in varying contexts. Our results may be explained by behavioral changes that individual mice express in response to cues of predation risk. However, it is possible that these findings could result from another mechanism. For example, individual mice may consistently occupy different microhabitats (i.e., niche specialization) rather than foraging both at the forest edge and at the forest interior (Schirmer et al., 2019). If this is the case, it is possible that habitat use may be correlated to foraging behavior (trait-related habitat matching; Edelaar et al., 2008). Some individuals may preferentially forage in open habitats and take less risks when foraging, while others may preferentially forage in closed habitats and take more risks when foraging. Therefore, although our results may be explained by flexibility in the behavior of individual mice, this conclusion would require further research with the added element of tracking the identities of individuals.
Data Availability Statement
The raw data supporting the conclusions of this article will be made available by the authors, without undue reservation.
Ethics Statement
The animal study was reviewed and approved by the IACUC, University of Massachusetts Dartmouth.
Author Contributions
MS and VK designed the study. VK carried out the study. LH and VK did the statistical analysis. VK wrote the manuscript with contributions from LH and MS. All authors contributed to the article and approved the submitted version.
Funding
The Office of Undergraduate Research at the University of Massachusetts Dartmouth helped to cover most research expenses.
Conflict of Interest
The authors declare that the research was conducted in the absence of any commercial or financial relationships that could be construed as a potential conflict of interest.
Publisher’s Note
All claims expressed in this article are solely those of the authors and do not necessarily represent those of their affiliated organizations, or those of the publisher, the editors and the reviewers. Any product that may be evaluated in this article, or claim that may be made by its manufacturer, is not guaranteed or endorsed by the publisher.
Acknowledgments
We would like to thank the Office of Undergraduate Research at the University of Massachusetts Dartmouth for their support in the execution of this study.
Supplementary Material
The Supplementary Material for this article can be found online at: https://www.frontiersin.org/articles/10.3389/fevo.2021.718887/full#supplementary-material
Footnotes
- ^ Giordano, A., Hunninck, L., and Sheriff, M. (2021). Prey Responses to Predation Risk Under Chronic Road Noise (Manuscript submitted for publication).
References
Brown, J. S. (1988). Patch use as an indicator of habitat preference, predation risk, and competition. Behav. Ecol. Sociobiol. 22, 37–47. doi: 10.1007/bf00395696
Brown, J. S., Kotler, B. P., and Mitchell, W. A. (1997). Competition between birds and mammals: a comparison of giving-up densities between crested larks and gerbils. Evol. Ecol. 11, 757–771.
Brown, J. S., Kotler, B. P., Smith, R. J., and Wirtz, W. O. (1988). The effects of owl predation on the foraging behavior of heteromyid rodents. Oecologia 76, 408–415. doi: 10.1007/BF00377036
Cherry, M. J., Morgan, K. E., Rutledge, B. T., Conner, L. M., and Warren, R. J. (2016). Can coyote predation risk induce reproduction suppression in white-tailed deer? Ecosphere 7:e01481. doi: 10.1002/ecs2.1481
Creel, S., Christianson, D., Liley, S., and Winnie, J. A. (2007). Predation Risk Affects Reproductive Physiology and Demography of Elk. Science 315, 960–960. doi: 10.1126/science.1135918
Donelan, S. C., Grabowski, J. H., and Trussell, G. C. (2017). Refuge quality impacts the strength of nonconsumptive effects on prey. Ecology 98, 403–411. doi: 10.1002/ecy.1647
Edelaar, P., Siepielski, A. M., and Clobert, J. (2008). Matching Habitat Choice Causes Directed Gene Flow: a Neglected Dimension in Evolution and Ecology. Evolution 62, 2462–2472. doi: 10.1111/j.1558-5646.2008.00459.x
Embar, K., Raveh, A., Hoffmann, I., and Kotler, B. P. (2014). Predator facilitation or interference: a game of vipers and owls. Oecologia 174, 1301–1309. doi: 10.1007/s00442-013-2760-2
Ferrari, M. C. O., Sih, A., and Chivers, D. P. (2009). The paradox of risk allocation: a review and prospectus. Anim. Behav. 78, 579–585. doi: 10.1016/j.anbehav.2009.05.034
Fontaine, J. J., and Martin, T. E. (2006). Parent birds assess nest predation risk and adjust their reproductive strategies. Ecol. Lett. 9, 428–434. doi: 10.1111/j.1461-0248.2006.00892.x
Gaynor, K. M., Brown, J. S., Middleton, A. D., Power, M. E., and Brashares, J. S. (2019). Landscapes of fear: spatial patterns of risk perception and response. Trends Ecol. Evol. 34, 355–368. doi: 10.1016/j.tree.2019.01.004
Guiden, P. W., and Orrock, J. L. (2019). Invasive shrubs modify rodent activity timing, revealing a consistent behavioral rule governing diel activity. Behav. Ecol. 30, 1069–1075. doi: 10.1093/beheco/arz050
Heithaus, M. R., and Dill, L. M. (2002). Food Availability and Tiger Shark Predation Risk Influence Bottlenose Dolphin Habitat Use. Ecology 83, 480–491. doi: 10.1890/0012-9658(2002)083[0480:faatsp]2.0.co;2
Hermann, S. L., and Thaler, J. S. (2014). Prey perception of predation risk: volatile chemical cues mediate non-consumptive effects of a predator on a herbivorous insect. Oecologia 176, 669–676. doi: 10.1007/s00442-014-3069-5
Kohler, S. L., and McPeek, M. A. (1989). Predation Risk and The Foraging Behavior of Competing Stream Insects. Ecology 70, 1811–1825. doi: 10.2307/1938114
Kotler, B. P., Blaustein, L., and Dednam, H. (1993). The specter of predation: the effects of vipers on the foraging behavior of two gerbilline rodents. Isr. J. Ecol. Evol. 39, 11–21.
Kotler, B. P., Brown, J., Mukherjee, S., Berger-Tal, O., and Bouskila, A. (2010). Moonlight avoidance in gerbils reveals a sophisticated interplay among time allocation, vigilance and state-dependent foraging. Proc. R. Soc. B Biol. Sci. 277, 1469–1474. doi: 10.1098/rspb.2009.2036
Kotler, B. P., Brown, J. S., and Hasson, O. (1991). Factors Affecting Gerbil Foraging Behavior and Rates of Owl Predation. Ecology 72, 2249–2260. doi: 10.2307/1941575
Krebs, C. J., Boonstra, R., Boutin, S., and Sinclair, A. R. E. (2001). What Drives the 10-year Cycle of Snowshoe Hares? BioScience 51, 25–35. doi: 10.1641/0006-3568(2001)051[0025:wdtyco]2.0.co;2
Laundré, J. W., Hernández, L., and Altendorf, K. B. (2001). Wolves, elk, and bison: reestablishing the ‘landscape of fear’ in Yellowstone National Park, USA. Can. J. Zool. 79, 1401–1409. doi: 10.1139/z01-094
Lima, S. L. (1998). Nonlethal Effects in the Ecology of Predator-Prey Interactions. BioScience 48, 25–34. doi: 10.2307/1313225
Lima, S. L., and Bednekoff, P. A. (1999). Temporal Variation in Danger Drives Antipredator Behavior: the Predation Risk Allocation Hypothesis. Am. Nat. 153, 649–659. doi: 10.2307/2463621
Lima, S. L., and Dill, L. M. (1990). Behavioral decisions made under the risk of predation: a review and prospectus. Can. J. Zool. 68, 619–640. doi: 10.1139/z90-092
Loggins, A. A., Shrader, A. M., Monadjem, A., and McCleery, R. A. (2019). Shrub cover homogenizes small mammals’ activity and perceived predation risk. Sci. Rep. 9:16857. doi: 10.1038/s41598-019-53071-y
Longland, W. S., and Price, M. V. (1991). Observations of Owls and Heteromyid Rodents: can Predation Risk Explain Microhabitat Use? Ecology 72, 2261–2273.
McGhee, K. E., Paitz, R. T., Baker, J. A., Foster, S. A., and Bell, A. M. (2020). Effects of predation risk on egg steroid profiles across multiple populations of threespine stickleback. Sci. Rep. 10:5239. doi: 10.1038/s41598-020-61412-5
Miller, J. R. B., Ament, J. M., and Schmitz, O. J. (2013). Fear on the move: predator hunting mode predicts variation in prey mortality and plasticity in prey spatial response. J. Anim. Ecol. 83, 214–222. doi: 10.1111/1365-2656.12111
Nakagawa, S., Johnson, P. C. D., and Schielzeth, H. (2017). The coefficient of determination R2 and intra-class correlation coefficient from generalized linear mixed-effects models revisited and expanded. J. R. Soc. Interface 14:20170213. doi: 10.1098/rsif.2017.0213
Orrock, J. L., Danielson, B. J., and Brinkerhoff, R. J. (2004). Rodent foraging is affected by indirect, but not by direct, cues of predation risk. Behav. Ecol. 15, 433–437. doi: 10.1093/beheco/arh031
Orrock, J. L., and Fletcher, R. J. (2014). An island-wide predator manipulation reveals immediate and long-lasting matching of risk by prey. Proc. R. Soc. B Biol. Sci. 281:20140391. doi: 10.1098/rspb.2014.0391
Peacor, S. D., Barton, B. T., Kimbro, D. L., Sih, A., and Sheriff, M. J. (2020). A framework and standardized terminology to facilitate the study of predation-risk effects. Ecology 101:e03152. doi: 10.1002/ecy.3152
Preisser, E. L., Orrock, J. L., and Schmitz, O. J. (2007). Predator Hunting Mode and Habitat Domain Alter Nonconsumptive Effects in Predator–Prey Interactions. Ecology 88, 2744–2751. doi: 10.1890/07-0260.1
Prugh, L. R., and Golden, C. D. (2014). Does moonlight increase predation risk? Meta-analysis reveals divergent responses of nocturnal mammals to lunar cycles. J. Anim. Ecol. 83, 504–514. doi: 10.1111/1365-2656.12148
Schirmer, A., Herde, A., Eccard, J. A., and Dammhahn, M. (2019). Individuals in space: personality-dependent space use, movement and microhabitat use facilitate individual spatial niche specialization. Oecologia 189, 647–660. doi: 10.1007/s00442-019-04365-5
Schmitz, O. J. (2008). Effects of Predator Hunting Mode on Grassland Ecosystem Function. Science 319, 952–954. doi: 10.1126/science.1152355
Sheriff, M. J., Krebs, C. J., and Boonstra, R. (2009). The sensitive hare: sublethal effects of predator stress on reproduction in snowshoe hares. J. Anim. Ecol. 78, 1249–1258. doi: 10.1111/j.1365-2656.2009.01552.x
Sheriff, M. J., Krebs, C. J., and Boonstra, R. (2011). From process to pattern: how fluctuating predation risk impacts the stress axis of snowshoe hares during the 10-year cycle. Oecologia 166, 593–605. doi: 10.1007/s00442-011-1907-2
Sheriff, M. J., Peacor, S. D., Hawlena, D., and Thaker, M. (2020b). Non-consumptive predator effects on prey population size: a dearth of evidence. J. Anim. Ecol. 89, 1302–1316. doi: 10.1111/1365-2656.13213
Sheriff, M. J., Orrock, J. L., Ferrari, M. C. O., Karban, R., Preisser, E. L., Sih, A., et al. (2020a). Proportional fitness loss and the timing of defensive investment: a cohesive framework across animals and plants. Oecologia 193, 273–283. doi: 10.1007/s00442-020-04681-1
Sih, A. (1984). The Behavioral Response Race Between Predator and Prey. Am. Nat. 123, 143–150. doi: 10.1086/284193
Sih, A., Crowley, P., McPeek, M., Petranka, J., and Strohmeier, K. (1985). Predation, Competition, and Prey Communities: a Review of Field Experiments. Annu. Rev. Ecol. Syst. 16, 269–311. doi: 10.1146/annurev.es.16.110185.001413
Sih, A., Englund, G., and Wooster, D. (1998). Emergent impacts of multiple predators on prey. Trends Ecol. Evol. 13, 350–355. doi: 10.1016/s0169-5347(98)01437-2
Smith, J. A., Donadio, E., Pauli, J. N., Sheriff, M. J., and Middleton, A. D. (2019). Integrating temporal refugia into landscapes of fear: prey exploit predator downtimes to forage in risky places. Oecologia 189, 883–890. doi: 10.1007/s00442-019-04381-5
Suraci, J. P., Clinchy, M., Dill, L. M., Roberts, D., and Zanette, L. Y. (2016). Fear of large carnivores causes a trophic cascade. Nat. Commun. 7:10698. doi: 10.1038/ncomms10698
Werner, E. E., Gilliam, J. F., Hall, D. J., and Mittelbach, G. G. (1983). An Experimental Test of the Effects of Predation Risk on Habitat Use in Fish. Ecology 64, 1540–1548. doi: 10.2307/1937508
Werner, E. E., and Peacor, S. D. (2003). A Review of Trait-Mediated Indirect Interactions in Ecological Communities. Ecology 84, 1083–1100. doi: 10.1890/0012-9658(2003)084[1083:arotii]2.0.co;2
Wilson, D., and Cooper, W. (2007). Beyond optimal escape theory: microhabitats as well as predation risk affect escape and refuge use by the phyrnosomatid lizard Sceloporus virgatus. Behaviour 144, 1235–1254. doi: 10.1163/156853907781890940
Witmer, G. W., and Moulton, R. S. (2012). Deer Mice (Peromyscus spp.) Biology, Damage and Management: a Review. Proc. Vertebr. Pest Conf. 25, 213–219.
Zanette, L. Y., White, A. F., Allen, M. C., and Clinchy, M. (2011). Perceived Predation Risk Reduces the Number of Offspring Songbirds Produce per Year. Science 334, 1398–1401. doi: 10.1126/science.1210908
Keywords: predation risk effects, risk-induced trait responses, non-consumptive effects (NCEs), giving-up-density (GUD), white-footed mice, Peromyscus leucopus
Citation: Kelleher V, Hunnick L and Sheriff MJ (2021) Risk-Induced Foraging Behavior in a Free-Living Small Mammal Depends on the Interactive Effects of Habitat, Refuge Availability, and Predator Type. Front. Ecol. Evol. 9:718887. doi: 10.3389/fevo.2021.718887
Received: 01 June 2021; Accepted: 28 September 2021;
Published: 20 October 2021.
Edited by:
Chiara Morosinotto, Novia University of Applied Sciences, FinlandReviewed by:
Jeanne Clermont, Université du Québec à Rimouski, CanadaDouglas Ferguson Makin, Ben-Gurion University of the Negev, Israel
Copyright © 2021 Kelleher, Hunnick and Sheriff. This is an open-access article distributed under the terms of the Creative Commons Attribution License (CC BY). The use, distribution or reproduction in other forums is permitted, provided the original author(s) and the copyright owner(s) are credited and that the original publication in this journal is cited, in accordance with accepted academic practice. No use, distribution or reproduction is permitted which does not comply with these terms.
*Correspondence: Michael J. Sheriff, bXNoZXJpZmZAdW1hc3NkLmVkdQ==