- 1State Key Joint Laboratory of Environmental Simulation and Pollution Control, School of Environment, Beijing Normal University, Beijing, China
- 2School of Grassland, Beijing Forestry, Beijing, China
- 3College of Resource and Environment, Shanxi Agricultural University, Jinzhong, China
- 4School of Environmental and Chemical Engineering, Shanghai University, Shanghai, China
Nitrogen deposition and climate warming can alter soil bacterial communities. However, the response of soil bacteria in an alpine steppe to these changes is largely unknown. In this study, a field experiment was performed on the northeastern Qinghai-Tibetan Plateau to determine the changes in soil bacterial communities of alpine steppes in response to nitrogen application and warming. The experiment consisted of four treatments, namely no-N application with no-warming (CK), N application (8 kg N ha−1 year−1) with no-warming (N), warming with no-N application (W), and N application (8 kg N ha−1 year−1) with warming (W&N). This study aimed to investigate (1) the changes in soil bacterial diversity and community structure under simulated nitrogen deposition and warming conditions, and (2) the key environmental factors responsible for these changes. Based on the results, soil bacterial diversity and community composition did not change significantly in the short term. Warming had a significant effect on overall bacterial composition, rare species composition, and individual bacterial taxa. Besides, the interaction between nitrogen application and warming had a significant effect on community β-diversity. Above-ground plant variables were highly correlated with bacterial community characteristics. Nitrogen application and warming did not significantly alter the distribution range of the bacterial community. Overall, this study suggests that soil bacterial communities can remain relatively stable at the level of simulated nitrogen application and warming and that short-term climatic changes may have no significant impacts on soil bacterial communities.
Introduction
Ecosystems are profoundly affected by an ocean of human activities, including the usage of fossil fuels and changes in land use, resulting in an increased concentration of atmospheric carbon dioxide, increased deposition rates of nitrogen compounds, and increased average temperatures. Studies on the ecological responses to these global changes have shown that subsurface physical, chemical, and biological processes usually involve and are mediated by soil microbes (Hu et al., 1999). For example, subsurface microbial-mediated processes can facilitate the fixation and release of nutrients that limit primary production and may influence the response of ecosystems to global changes in the long term.
The increase in surface temperature and nitrogen deposition rate is more pronounced at higher elevations (Bodelier and Laanbroek, 2004), affecting highly sensitive biogeochemical processes that depend on cold systems (Zhou et al., 2016). The Qinghai-Tibetan Plateau (the QTP), also known as the “Third Pole of the Earth,” is highly vulnerable to climate change and therefore considered a “pre-warning region” for the world (Wang et al., 2002). Nitrogen deposition in this region ranges from 8.7 to 13.8 kg N ha−1 yr−1 (Lü and Tian, 2007), whereas warming is about three times the average global warming level of the past 50 years (Qiu, 2008). These more dramatic changes have significantly affected the biochemical processes of the alpine ecosystems on the QTP. Therefore, we speculated that the microbial community in alpine ecosystems of the QTP is highly sensitive and susceptible to climate change. Thus, the response of the soil microbial community to N deposition and warming in QTP grasslands could be used to predict future climate change, the carbon cycle, and ecosystem functions on the Tibetan Plateau.
The relationship between bacterial communities and environmental variables has been widely investigated. Although Boxman et al. (1998) and Frey et al. (2004) found insignificant effects of short-term N application on soil bacterial biomass, diversity and community composition, Xue et al. (2007) reported that short-term N application greatly increased the number of soil bacteria and thus changed bacterial community composition. This inconsistent result has been caused by the different nitrogen requirements of various bacterial species/taxa under a low soil nitrogen status, making it necessary to verify exactly how the soil bacterial community responds to short-term N addition. Also, N application has a certain acidifying effect on the soil, and some studies have shown that soil bacterial diversity, especially species richness, is significantly positively correlated with pH (Tripathi et al., 2012). This study also verified whether there is an indirect effect of such N application on the soil bacterial community. Meanwhile, Ratkowsky et al. (1982) found that temperature is an important factor for microbial growth. Also, warming likely affects soil bacterial communities indirectly by influencing water availability (Sheik et al., 2011; Waghmode et al., 2018) and above-ground vegetation (Chung et al., 2007). Previous studies have shown that warming significantly reduces the richness and diversity of bacterial communities (Waghmode et al., 2018) and substantially alters microbial community composition (DeAngelis et al., 2015). Other studies have reported a decrease in bacterial abundance and diversity in response to soil warming (Allison and Treseder, 2008; Castro et al., 2010; Hayden et al., 2012). In contrast, Zhang et al. (2005) showed that microbial community diversity increase with increasing temperature. Based on these contradictory findings, we aimed to further investigate how soil bacterial communities in alpine steppe on the QTP respond to warming treatments. Xiong et al. (2014) showed that short-term (15 months) warming does not significantly affect the α-diversity of bacterial communities, but it significantly shifted the soil bacterial community composition in the grasslands on the QTP. Xiong et al. (2016) found that the combined treatment of warming and nitrogen application significantly promotes soil microbial diversity and relative abundance, including Gram-positive bacteria and fungi, in a high-elevation alpine meadow. These observations highlight that microbial responses to N application and warming are complex and difficult to understand without knowing their potential interactive effects.
Soil bacteria represent a large fraction of the subsurface biomass and biome and regulate the biogeochemical cycling of organic matters. Therefore, the responses of soil bacterial diversity and composition to global climate change remain a major research interest. We performed field experiments to investigate how soil bacterial communities in an alpine steppe on the QTP respond to nitrogen deposition and warming. The experiment consisted of four treatments, including no-N application with no-warming (CK), N application (8 kg N ha−1 year−1) with no-warming (N), warming with no-N application (W), and N application (8 kg N ha−1 year−1) with warming (W&N). The experiments were conducted using ammonium nitrate (NH4NO3) and open-top chambers (OTC) in an alpine steppe (AS), one of the major types of alpine grasslands at Tiebujia Town on the QTP (Zhang Y. et al., 2016), during the growing season. This study aimed to determine: (1)the changes in soil bacterial diversity and community composition at different gradients of controlled nitrogen deposition and warming; (2) the key environmental factors responsible for these changes?
Materials and Methods
Study Sites
This study was conducted in Tiebujia Town of Gonghe County, Hainan Tibetan autonomous prefecture (37°02′ N, 99°35′ E, 3270 m ASL), Qinghai Province, China. The study site is located west of the Qinghai Lake, covered by the alpine steppe (AS). The mean annual temperature is approximately 0°C, with a yearly evaporation capacity of 1484 mm, and a mean annual precipitation of 380 mm (Zhao et al., 2017). The typical vegetation is alpine steppe, dominated by Leymus secalinus, Stipa capillata, Poa crymophila, and Agropyron cristatum. The soil is mostly composed of loam-clay (Zhao et al., 2017).
Experimental Design
We applied a two-way factorial design (N application and warming) with four treatments: control treatment (CK), only N application (N), only warming (W), and a combined treatment of warming and N deposition (W&N). In early 2015, six plots in the grassland part of the alpine steppe (AS) were fenced. Each plot had an area of 10 m2 (2 m × 5 m). We randomly selected three plots (replicates) for the control treatment and another three plots (replicates) for the nitrogen application treatment, with ammonium nitrate (NH4NO3) of 8 kg N ha−1 year−1. According to a previous study, 8 kg N ha−1 year−1 is the current level of annual N deposition in this area (Lü and Tian, 2007). Also, there were six open-top chambers (OTCs), where the temperature was about 2°C higher than on the outside. Three OTCs (replicates) were randomly placed in the fenced plots for only warming treatments (W), and the other three OTCs (replicates) were randomly placed in the fenced plots for a comprehensive treatment of warming and N deposition (8 kg N ha−1 year−1) (W&N). All the treatments had a similar land use history and topography. The N was applied in form of ammonium nitrate (NH4NO3) in early May, early July, and early September of 2015–2017. The ammonium nitrate was first dissolved in deionized water and sprayed evenly in the sample plots using a spray bottle.
Soil Sampling
Soil samples were collected in July, 2017, after 3 years of experimental N addition and warming. In each plot, three 3.5-cm diameter soil cores (0–20 cm in depth) were collected after fertilization in early July. The sample were mixed and sieved through a 2-mm mesh to remove roots and stones, then stored at −80°C until analysis.
Soil and Vegetation Property Measurements
To measure nitrate nitrogen (NO3-N) and ammonium nitrogen (NH4-N), the soil was extracted with a 0.01 mol/L CaCl2 solution at a soil-solution ratio of 1:2.5, shaken in a shaker at 180 rpm for 30 min and then removed and left to stand. Subsequently, the supernatant was filtered through a filter membrane, and NO3-N and NH4-N were determined using a flow injection auto-analyzer (AACE, Germany). For the measurement of available potassium (AK) and available phosphorus (AP), the soil was air-dried and ground, passed through a100-mesh sieve, weighed with an accuracy of 0.1 g, and placed in a Teflon jar. We then added 5 mL of nitric acid, 2 mL of hydrofluoric acid, and 1 mL of perchloric acid. The jar was then placed in an automatic digestion apparatus and heated according to the following protocol: 120°C 1 h, 140°C 1 h, 160°C 1 h, 180°C 45 min. Next, the reflux funnel was removed, and the acid was droved to nearly dry. After cooling, the volume was brought to 25 mL with ultra-pure water, and AP and AK were determined using inductively coupled plasma spectrometers (ICP) (SPECTRO ARCOS EOP, Germany). For the measurement of total carbon (TC), the soil was passed through a100-mesh sieve. About 0.2 mg of the sieved soil was taken and wrapped in a tin cup. TC was measured using an element analyzer (EA 3000, Italy). Soil pH was determined from the filtered supernatant using a pH meter (Mettler Toledo). Soil moisture (SM) was measured using the wet soil and dry soil weight. To obtain dry soil, a small portion of the wet soil from each sample was placed in an aluminum specimen box, and oven-dried at 105°C for 24 h. To estimate vegetation variables, we randomly selected a 1 m × 1 m square area in each plot and recorded vegetation type, abundance, and average height according to the method of Wang et al. (2012). Subsequently, a 25 cm × 25 cm area of vegetation was trimmed, dried, and weighed to obtain above-ground biomass data (AGB). Vegetation diversity was measured by the Shannon-Wiener diversity index (SW).
DNA Extraction and PCR and DNA Sequencing
We performed DNA extraction and polymerase chain reaction (PCR), and DNA sequencing as described previously (Li et al., 2016). The DNA was extracted from 0.5 g of soil using a soil-specific FastDNA spin kit according to the manufacturer’s instructions. The DNA quality and quantity were assessed using a Nano-Drop ND-1000 spectrophotometer. Subsequently, the DNA samples were diluted to 10 ng/μL and stored at −20°C. Before high-throughput sequencing, the DNA samples were subjected to PCR amplification with bacteria-specific primers targeting the V4-V5 regions of the 16S rRNA: forward 515F (5′-GTGCCAGCMGCCGCGG-3′) and reverse 907R (5-CCGTCAATTCMTTTRAGTTT-3).
The sequences in the V4 of 16S rRNA region provide comprehensive coverage (Sul et al., 2011) with the highest for taxonomical accuracy (Wang et al., 2007). Briefly, 50 μL of the amplification reaction system contained 1 × buffer, 0.2 μM of each primer, 1.5 mM MgCl2, 10 ng of template, and 1 unit of Pfu polymerase (BioVision, Mountain View, CA, United States). The amplification procedure was as follows: 2 min at 94°C, followed by 25 cycles of denaturation at 94°C for 30 s, primer annealing at 55°C for 30 s, extension at 72°C for 1 min and a final extension step for 10 min. After three repetitions of the reaction procedure, the resulting products were combined and separated by gel electrophoresis. The separated PCR products were extracted from the gel using the QIAquick gel extraction kit and purified using the Qiagen PCR purification kit. High-throughput sequencing was performed with the Miseq Sequencer (Illumina).
Sequence Analysis
Sequence analysis was conducted as described previously (Li et al., 2016). Sequence processing and diversity estimation were performed using the microbial data processing platform QIIME (Quantitative Insights into Microbial Ecology). As suggested by Huse et al. (2007), sequences containing ambiguous base calls, less than 380 nt or more than 10 homologs were eliminated. A chimera check was performed with QIIME via ChimeraSlayer. There were four replicate datasets for each treatment (i.e., CK, N, W, and W&N). The pairing distance between the sequences was calculated with the distant neighbor algorithm, and the OTU was clustered by 97% sequence similarity. Singleton OTUs (with only one read) were removed, and the remaining sequences were sorted into each sample based on OTUs (Zhou et al., 2012). Subsequently, the most abundant sequence was selected from each OTU as the representative sequence. The Basic Local Alignment Search Tool (BLAST) was used for the taxonomic assignment of each representative sequence with a subset of the Silva database, and the bacterial OTU was classified. Based on a previous study, 2,000 denoised sequences per sample could interpret more than 80% of the trends in α-diversity and 95% in β-diversity in samples where 15,000–20,000 bacterial sequences were observed (Lundin et al., 2012). When sampling up to 2,100 denoised sequences, the rarified data set should be acceptable. Rarefaction was repeated 30 times, and each subsequent analysis was based on the average of 30 random trials.
Data Analysis
Statistical analysis was performed using the software packages of vegan, picante, car and cardata in R v3.6.4. The sample was rarified to 21,000 sequences because the number of sequences between soil cores was not equal. Samples with less than 21000 sequences were not included. Samples of plots 1 (CK), 2 (N), 3 (W), and 4 (W&N) were up to this standard and were excluded. Bacterial α-diversity was characterized using the Shannon-Wiener Index (SW). Species richness (SR, calculated as OTU numbers), and Pielou’s evenness, and bacterial β-diversity was determined by the average pairwise community differences in each treatment using the Bray-Curtis distance matrix (Rodrigues et al., 2013). The distribution of OTUs in each soil core was calculated based on the number of soil cores that the OTUs evenly distributed in Rodrigues et al. (2013), whereas the distribution of each OTU was calculated as the number of soil cores in which it was detected. Tukey’s HSD test was used to perform multiple comparisons of the bacterial phyla, α-diversity, β-diversity, and relative abundance across the four treatments. Two-way analysis of variance (ANOVA) was performed to examine the effects of N application, warming, and their interactions on bacterial diversity.
Non-metric multidimensional scaling (NMDS) was used to estimate the differences in overall community composition across the treatments. A total of 16 soil and plant variables were tested to evaluate possible links between bacteria and soil and vegetation variables. Stepwise regression analysis was performed to determine the variables influencing bacterial α- and β-diversity. For bacterial composition, the most important factors were selected based on variance inflation factors (VIF < 10) with 999 Monte Carlo permutations, as well as Mantel test and biology (Zhou et al., 2011). Finally, we fitted nine variables as vectors onto the NMDS ordination graphic to clarify the relationships among soil, vegetation and the bacterial community.
Results
Effects of N Application and Warming on Soil Physicochemical and Plant Properties
There were no significant changes in soil pH, moisture, NH4+-N, AK, AP, and TC caused by N addition, warming and their interaction. Nitrogen addition alone caused a significant increase in NO3–-N and AGB by 44.17 and 45.07%, respectively. Warming caused a significant increase in AGB by 81.37%, but it induced a significant decrease in plant diversity by 28.55%. Significant positive interaction effects between N addition and warming were found on AGB (123.50%). A significant decrease in plant diversity by 26.49% was found caused by interactive plots (Supplementary Table 1).
Effects of N Application and Warming on Bacterial Diversity
The interaction between nitrogen application and warming had no significant effects on bacterial α-diversity (i.e., SW, SR, and Pielou’s evenness) (Table 1). Sole N application or sole warming did not induce significant changes in bacterial α-diversity (Figure 1). The responses of bacterial α-diversity to N application and warming were significantly correlated with some soil (e.g., TC) and plant (plant SR and AGB) factors, which explained more than 30% of the variation in bacterial α-diversity (Table 2).
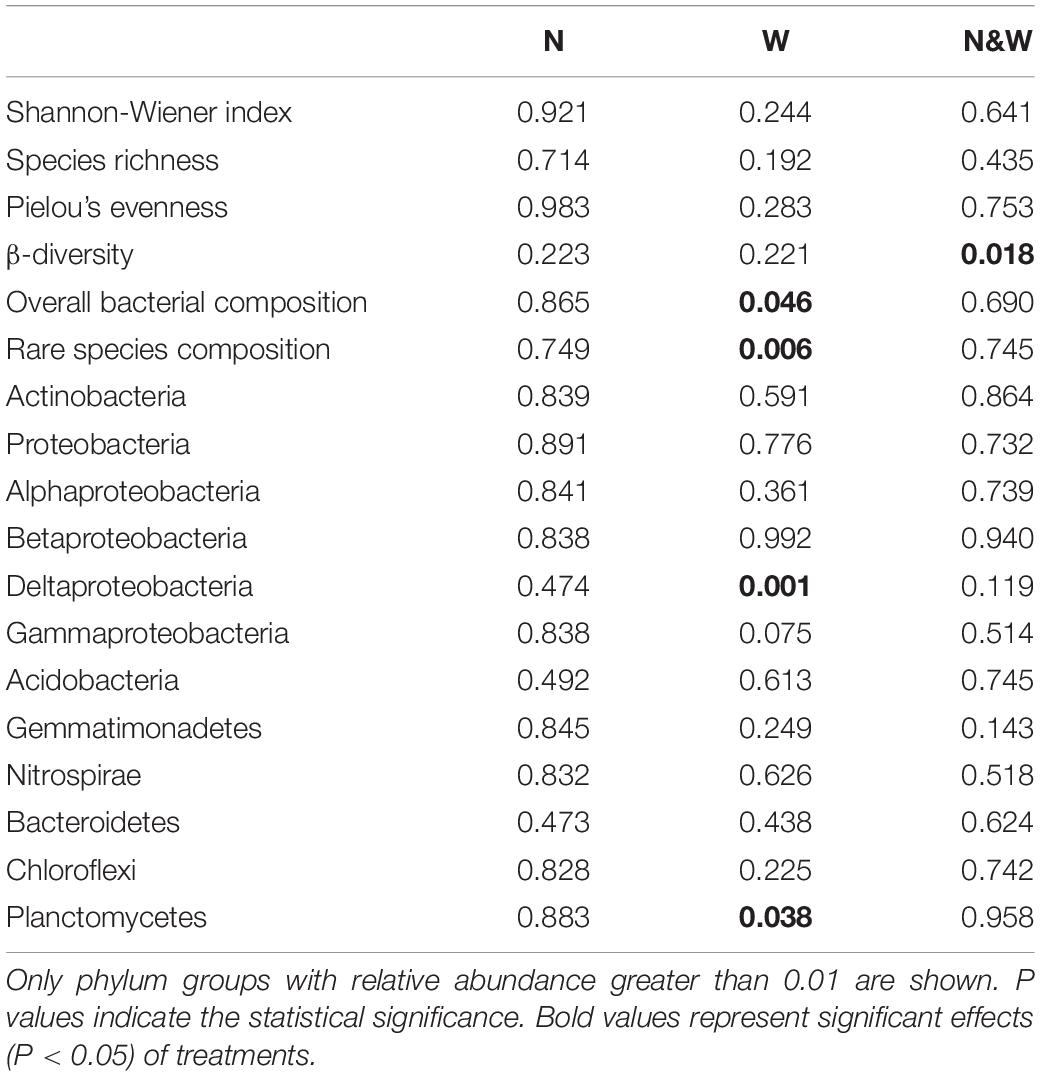
Table 1. Significance tests of the treatments on bacterial community diversity, overall community, and rare species (i.e., relative abundance <0.1%) composition and phylum groups using N application (N) and warming (W) as main factors Bacterial β-diversity was estimated as the average pairwise community dissimilarity within each treatment.
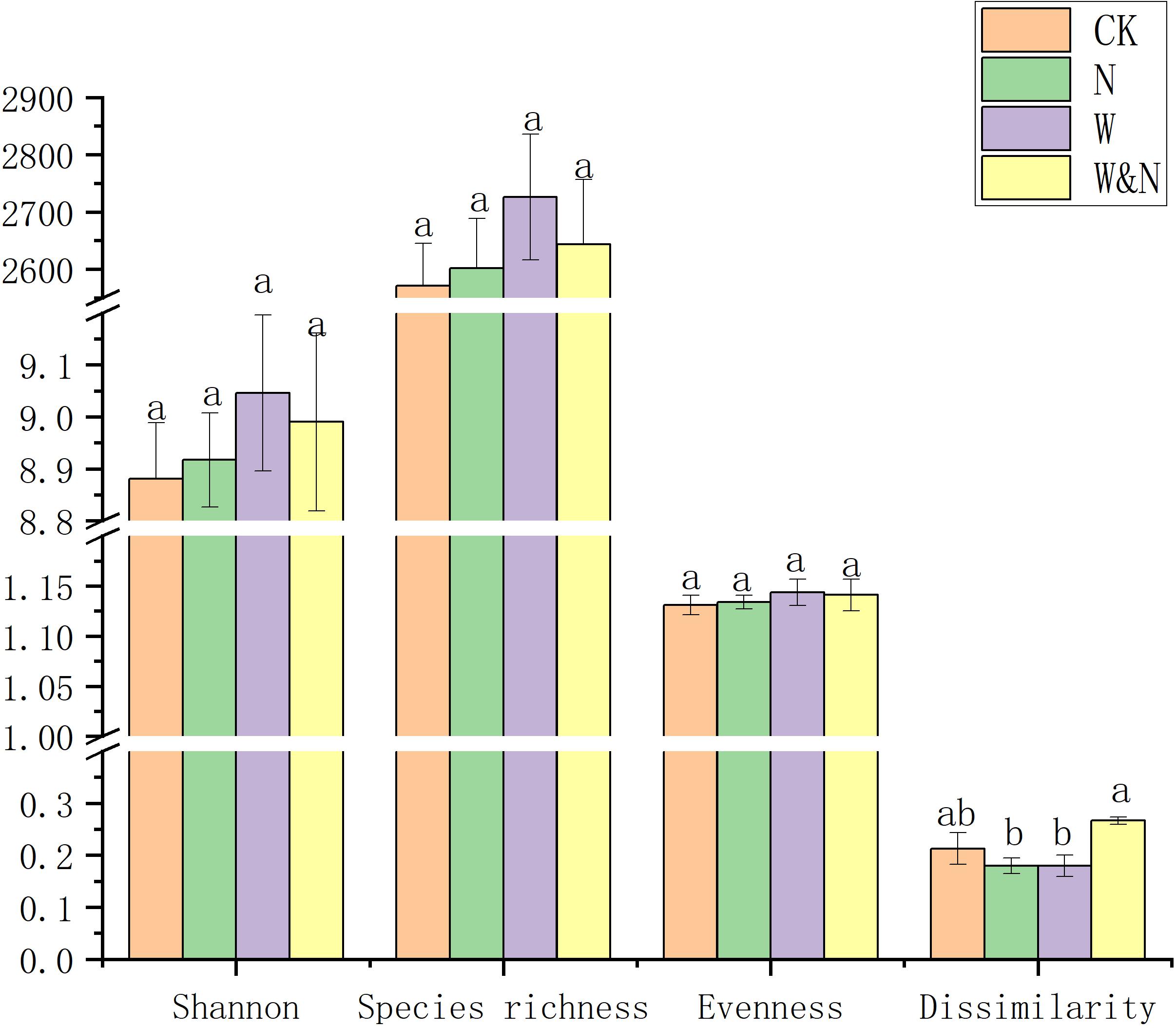
Figure 1. Bacterial Shannon-Wiener index, species richness, Pielou’s evenness and bacterial community dissimilarity for no-N application with no-warming (CK), N application with no-warming (N), no-N application with warming (W), and N application with warming (W&N) treatment. Different letters indicate significant differences at p < 0.05 level. Error bars represent standard error (n = 3).
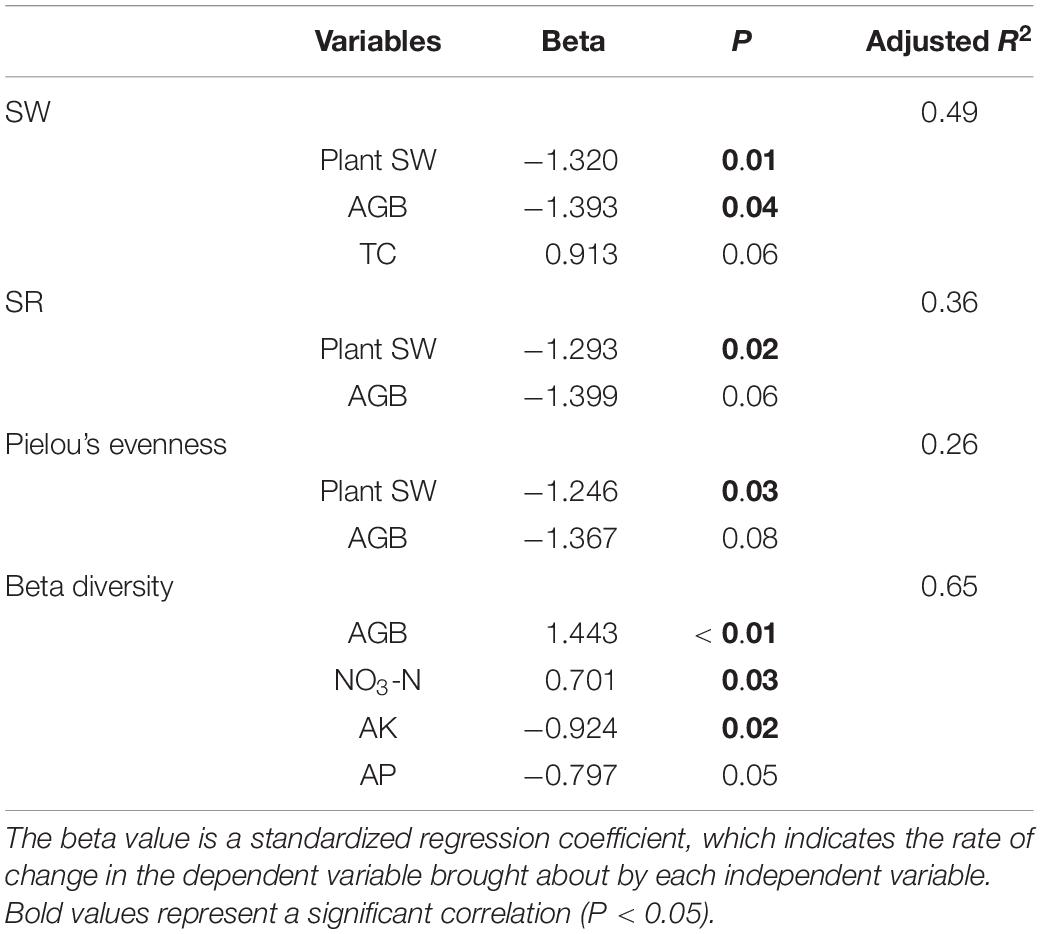
Table 2. Stepwise regression analysis of soil and vegetation factors influencing bacterial Shannon-Wiener index (SW), species richness (SR), Pielou’s evenness, and β-diversity.
Nitrogen application alone or warming alone caused no significant changes in bacterial β-diversity. The interaction between nitrogen application and warming also did not cause significant changes in bacterial β-diversity, which significantly increased in the interaction treatment compared to the nitrogen addition and warming treatments by 48.58 and 48.47%, respectively (Figure 1 and Supplementary Table 2). Interaction effects played a crucial role in altering the bacterial β-diversity (P = 0.018) (Table 1). The parameters AGB, NO3-N, and AK were critical factors influencing bacterial β-diversity, explaining 65% of the variation in bacterial β-diversity (Table 2).
Effects of N Application and Warming on Bacterial Community Composition
In our study site, the soil bacterial community mainly consisted of Actinobacteria followed in decreasing order of relative abundance by Proteobacteria, Acidobacteria, Chloroflexi, Gemmatimonadetes, and Planctomycetes (Figure 2). Warming significantly affected the overall and rare bacterial species composition (Table 1). The NMDS analysis showed that both nitrogen application and warming deviated the sample from the control in the same direction, and that the warming effect was greater. The interaction between warming and N application resulted in an increasing difference among the samples (Figure 3). At the phylum level, N application alone, warming alone, and interaction between N application and warming did not significantly change the relative abundance of phylum groups with relative abundance greater than 0.01 (Figure 2). There were significant effects of warming on the phylum/subphylum composition of Planctomycetes (p = 0.038) and Deltaproteobacteria (p = 0.001), although the differences of relative abundances among the four treatments were not significant (Table 1).
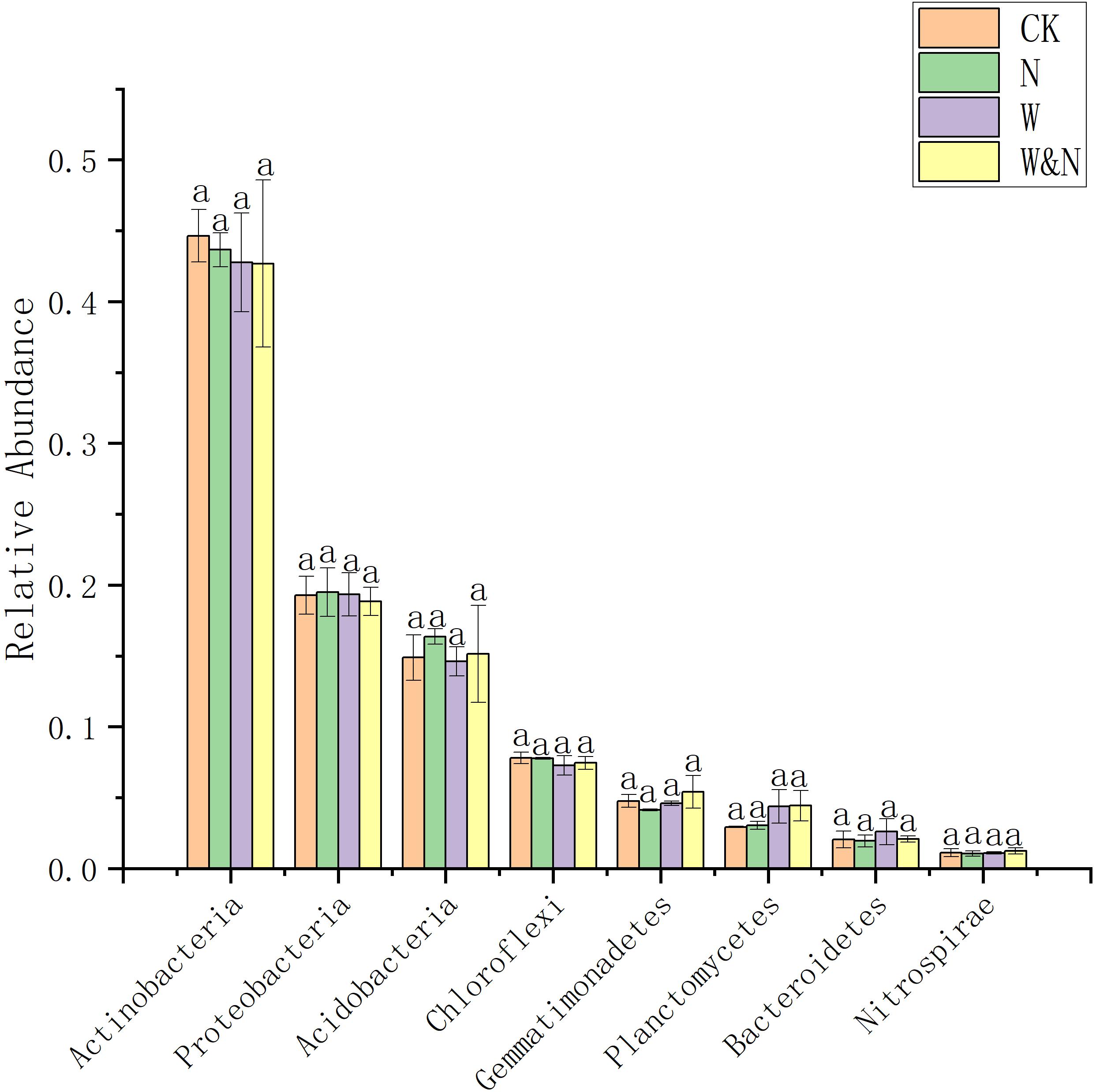
Figure 2. Relative abundance of bacterial phyla in no-N application with no-warming (CK), N application with no-warming (N), no-N application with warming (W), and N application with warming (W&N) treatments. Error bars represent standard error (n = 3).
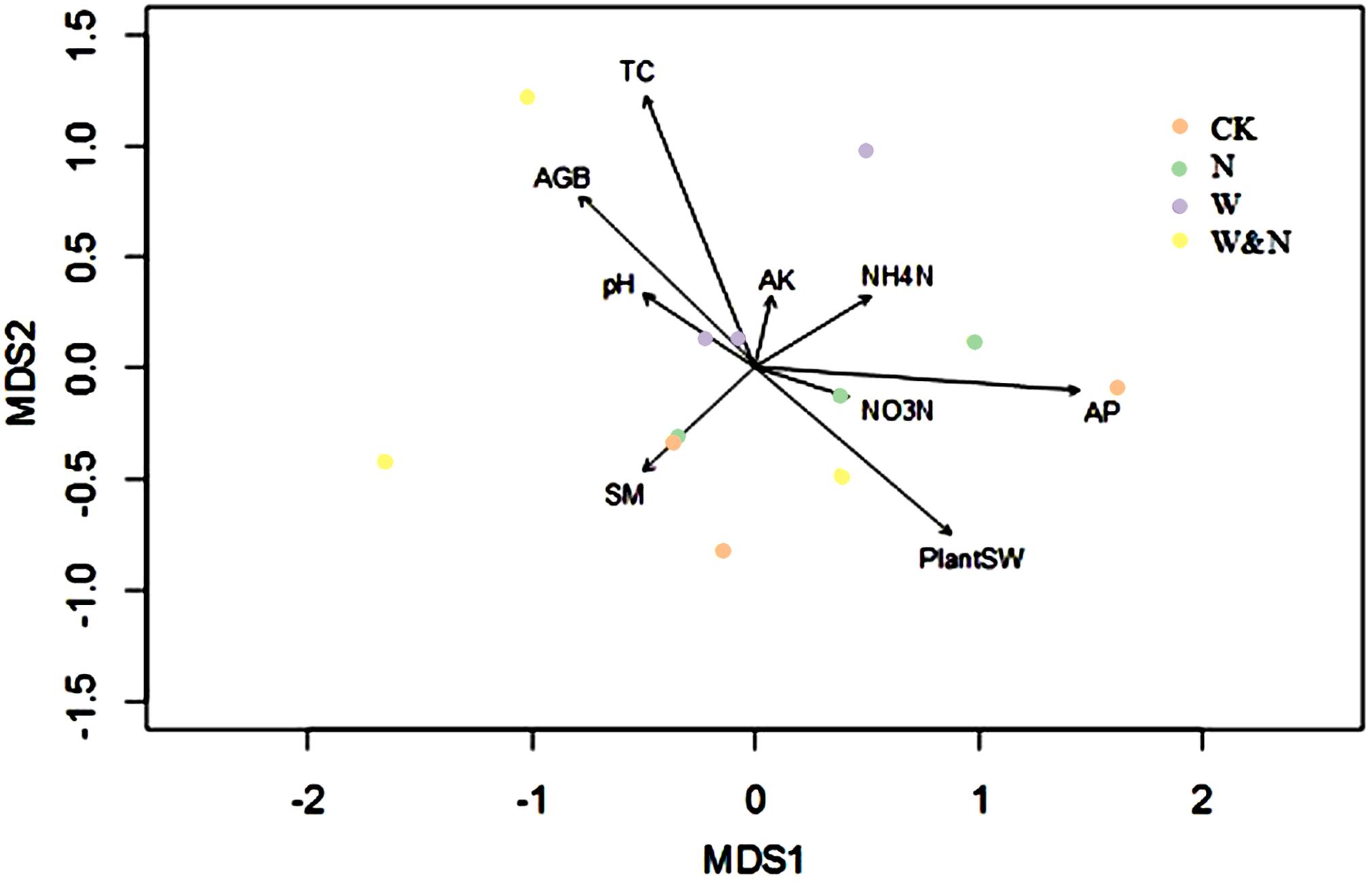
Figure 3. NMDS (non-metric multidimensional scaling) of bacterial community composition (A). Abbreviations: TC, total carbon; AK, available potassium; AP, available phosphorus; PlantSW, plant Shannon-Wiener index; SM, soil moisture; AGB, above-ground biomass. CK: No-N application with no-warming; N: N application with no-warming; W: no-N application with warming; W&N: N application with warming.
At the 97% identity level, the resampled 34633 sequences were distributed into 4508 OTUs. A total of 85.69 and 88.58% of the OTUs (99.45 and 99.64% reads) were found in no-warming (CK and N)-warming (W and W&N), no-N application (CK and W)-N application (N and W&N) treatments (Figures 4A,D), respectively. The OTUs unique for no-warming and warming treatments accounted for 6.21% (0.20% of reads) and 8.10% (0.34% of reads) of the overall OTUs, respectively (Figure 4A). No-N application and N application treatments occupied 5.50% (0.17% reads) and 5.92% (0.19% reads) unique OTUs. Neither nitrogen application nor warming significantly affected the distribution range of shared and unique OTUs (Figures 4B,C,E,F). The two most abundant OTUs (OTU9 and OTU1) detected in the treatments belonged to the order Solirubrobacterales (class Thermoleophilia, phylum Actinobacteria). There were no significant effects of N application, warming, and their interaction on the relative abundances of the top 10 most abundant OTUs (Supplementary Table 3).
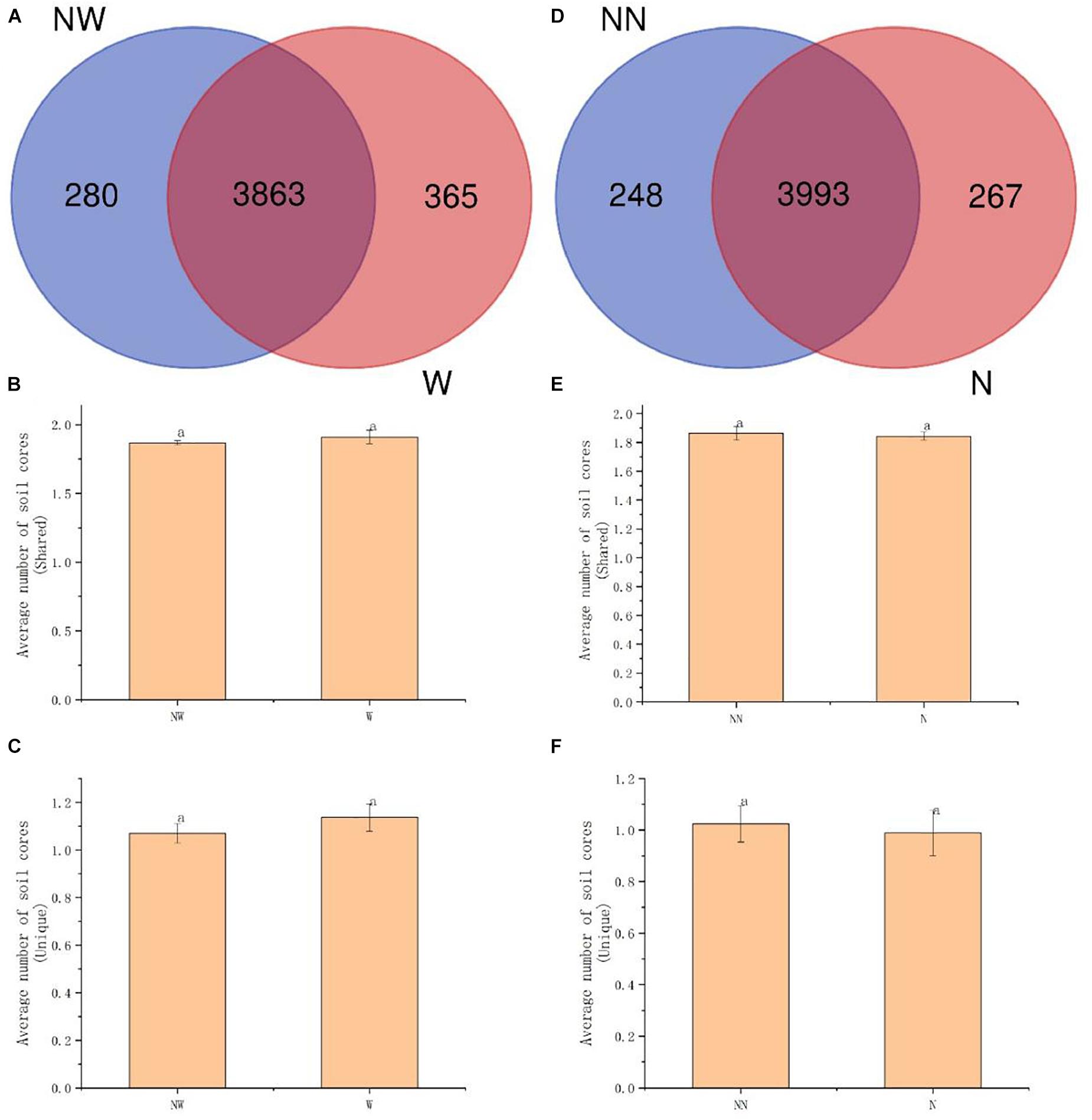
Figure 4. Distribution of bacterial OTUs across soil cores. (A) Venn diagrams showing the number of OTUs unique to no-warming (CK and N, NW) and warming (W and W&N, W) plots and those shared between them. (B) Number of soil cores that the shared OTUs were averagely distributed in for NW and W plots. Error bars indicate standard error (n = 3,863). (C) Number of soil cores that the unique OTUs were averagely distributed in for NW and W plots. (D) Venn diagrams showing the number of OTUs unique to no-N application (CK and W, NN) and N application (N and W&N, N) plots and those shared between them. (E) Number of soil cores that the shared OTUs were averagely distributed in for NN and N plots. Error bars indicate standard error of the mean (n = 3,993). (F) Number of soil cores that the unique OTUs were averagely distributed in for NN and N plots.
Relationship Between the Shift in Bacterial Community Composition and Environmental Variables
Based on the forward selection, five soil nutrient variables (pH, SM, TC, NO3-N, and NH4-N), two vegetation variables (plant SR and above-ground biomass), and two available soil nutrient variables (AK and AP) were selected to interpret the interrelationships between environmental variables and the bacterial community. The NMDS analysis showed that plant variables were significantly related to bacterial community composition (Figure 3), which was also supported by the results of the Mantel test (Table 3).
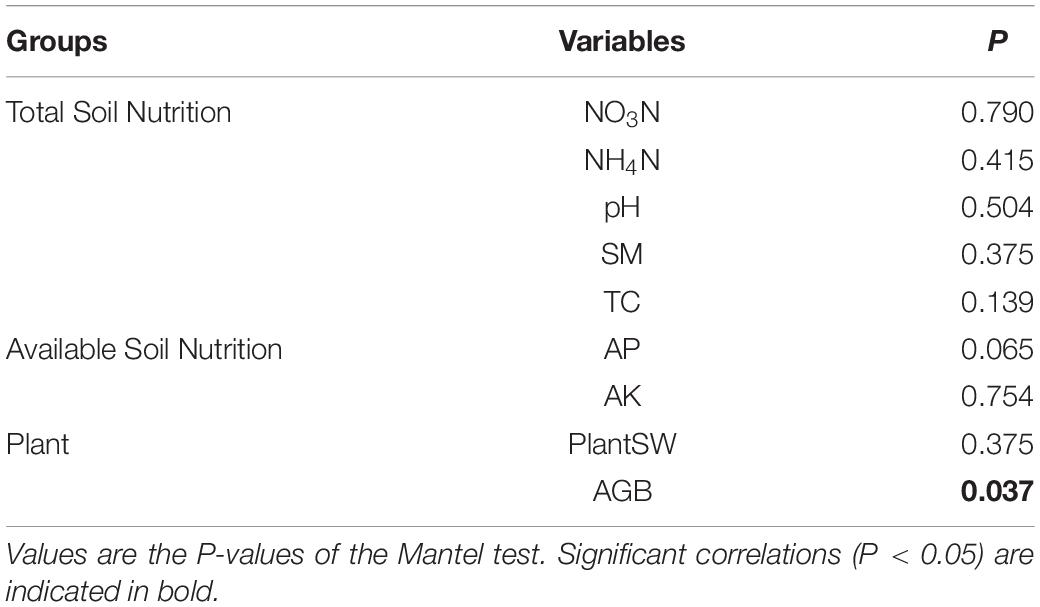
Table 3. Significance tests of the correlation between soil and vegetation variables and bacterial community composition as shown by Mantel tests.
Discussion
Effect of N Application on Soil Bacterial Communities
In this study, 3 years of N application did not cause significant changes in soil microbial diversity and community structure at the experimental site. This is slightly different from the results of some previous studies. Frey et al. (2004) found that nitrogen application reduced soil microbial species richness and community diversity indices. Meanwhile, Xue et al. (2007) found that short-term nitrogen addition significantly increased soil bacterial abundance and richness. In this study, the amount of nitrogen applied at the site did not reach a threshold sufficient to cause changes in the soil bacterial community, and this could have contributed to the contradictory results. Boxman et al. (1998) found that lower nitrogen input levels had little effect on bacterial biomass and diversity, but De Vries et al. (2006) observed a significant increase in bacterial activity with the application of higher nitrogen amounts. In this study, 8 kg N year−1 ha−1 is similar to the global atmospheric nitrogen deposition level at this stage and may not be sufficient to produce shifts in the bacterial community. Meanwhile, this amount of N did not cause a significant decrease in soil pH. Some authors postulate that soil nitrogen fertilization may reduce microbial biomass due to the indirect effect of nitrogen fertilization on soil acidification (Janssens et al., 2010; Waring et al., 2013; Zhou et al., 2017). Other studies have shown that the species richness and diversity of soil bacteria were significantly positively correlated with pH (Tripathi et al., 2012; Shen et al., 2013). However, we did not observed similar effects in this study probably because the soil pH was high (about 6.76) and well buffered. We also found no significant change in soil pH due to nitrogen application, which may also contribute to the stability of the local bacterial community to some extent. Collectively, our results imply that the lower nitrogen inputs at our study site had no significant effect on soil bacteria in the short term.
Effect of Warming on Soil Bacterial Communities
The bacterial community composition in the alpine steppe was either resistant to warming or took longer to show a response, which is consistent with the results of Zheng et al. (2012) regarding QTP grassland, which exhibited an unchanged methanotrophic community composition under warming conditions. Many previous studies in different sites have shown that long-term warming can cause significant changes in the diversity and composition of soil bacterial communities (Zhang et al., 2005; Rinnan et al., 2007, 2009; DeAngelis et al., 2015). Thus, we speculated that the short-term warming in this study might have contributed to the insignificant changes in microbial diversity. Li et al. (2016) found that warming significantly improved the evenness and similarity of the bacterial communities and broadened the distribution range of the bacteria on the QTP after 3 years of experimental warming. However, we did not observe similar changes in the 3-year warming period. Zhang Y. et al. (2016) observed that neither the warming nor the precipitation reduction treatment alone had any significant effect on soil bacterial community structure on the QTP, in contrast to the combination of the two treatments. Sheik et al. (2011) observed that warming reduced soil species richness, evenness, and diversity during the non-drought period, whereas it increased soil species richness, evenness, and diversity during drought periods. These studies demonstrate that the effects of warming on microorganisms are dependent on water availability and that these two factors can potentially interact and affect the size and diversity of bacterial communities. Our experimental site was relatively dry, with an average annual precipitation of only 377 mm and average annual evaporation of almost four times the precipitation (1,484 mm): insignificant changes in soil water content were observed with warming. This may be attributed to the resistance of soil microbial diversity to warming. Further studies are required to discern how long this resistance will persist and which changes in bacterial community diversity and structure will eventually occur.
In this study, significant differences between the warming treatment and the CK were detected for the overall bacteria community composition and rare species composition of bacteria. However, insignificant changes were observed in the nitrogen application treatment alone or the interaction between nitrogen application and warming. This is consistent with the finding that warming significantly affected the communities of Planctomycetes and Deltaproteobacteria (Table 1), which could be attributed to the significant changes in biomass, relative abundance, activity, or other indicators of some of the more temperature-tolerant taxa in these two phyla. Planctomycetes are widespread in marine, hyper-saline, and even terrestrial soil habitats (Fuerst et al., 1997). Isosphaera pallida, a member of Planctomycetes, has moderately thermophilic physiology and can survive even at 55°C (Giovannoni et al., 1987). Deltaproteobacteria is an order of Proteobacteria, which is the most abundant taxon in the bacterial community and is divided into Alpha-, Beta-, Delta-, and Gammaproteobacteria. Previous studies have shown that taxa of this phylum and its sub-phylums are more susceptible to elevated temperatures (Sheik et al., 2011; Tuyet et al., 2015; Aydogan et al., 2018; Tao et al., 2020). These findings infer that increased temperatures induce the emergence and expansion of microbial communities that are more adapted to such conditions. Notably, increased temperature can enhance the competitive advantage of these communities, significantly altering the overall community composition and structure. The temperature-sensitive bacterial taxa are of particular interest for future study.
Interactive Effects of N Application and Warming on Soil Bacterial Communities
Against the background of climate change, previous studies have focused on the effects of individual climate change, such as nitrogen deposition, elevated temperature, and precipitation, among others, on soil microbes. However, it is still unclear how subsurface microbial communities respond to the interactions of these factors. NMDS analysis showed that the interaction of N-application and warming resulted in a significant difference among the samples, which corroborated the conclusion of Xiong et al. (2016) that the combined treatment of warming and nitrogen application can significantly promote soil bacterial diversity and relative abundance in a high-elevation alpine meadow. Two-way ANOVA showed that nitrogen application and warming had significant effects on the dissimilarity, i.e., β-diversity, of the bacterial community (Table 1). The β-diversity of the bacterial community was significantly changed by the interaction of nitrogen application and warming in comparison with nitrogen application and warming alone (Figure 1). Admittedly, the different responses of many taxa to nitrogen application and warming treatments may be attributed to the complex interactions among soil, plants, and microorganisms because of their different sensitivities to various environmental factors (Singh et al., 2010). The combination of nitrogen application and warming may induce indirect effects of soil and vegetation properties on soil bacterial communities, which are likely to be greater than the direct effect (Xiong et al., 2016). In this study, stepwise regression analysis (Table 2) and the Mantel test (Figure 3) verified that vegetation diversity and above-ground biomass potentially influence soil bacterial diversity and community composition, suggesting that above-ground vegetation significantly affects below-ground bacteria. Previous studies have reported a strong link between soil bacterial communities and above-ground biomass and diversity (Stephan et al., 2000; Chung et al., 2007; Li et al., 2013). These effects reflect changes in vegetation and suggest that plant and microbial responses to nitrogen addition and warming are closely coupled. Meanwhile, previous studies involving warming treatments on the alpine grasslands showed positive effects on bacterial biomass in spring and winter, but opposite effects in summer and autumn, indicating that the impact of warming on soil microbial communities are seasonal (Belay-Tedla et al., 2009; Liu et al., 2009; Schadt, 2010). In summer and early autumn (growing season), the vigorous plant growth at our experimental site could have affected the turnover and cycling of soil nutrient reservoirs, soil water availability, and other changes in the soil micro-environment, leading to intensified interactions between plants and microorganisms.
There is substantial evidence that climate change stressors significantly influence the composition and function of soil bacterial and even microbial communities in the grasslands of the QTP (Yang et al., 2014; Zhang et al., 2015). In this study, soil bacterial community in the alpine steppe showed some resistance to 3 years of nitrogen application and warming treatments, indicating that the soil bacterial community in the alpine steppe is stable under short-term nitrogen and warming treatments. Although we did not observe any significant changes in soil bacteria under short-term nitrogen addition and warming conditions, we cannot conclude that the soil microbial community will remain in this steady-state over the long term. Indeed, it may take longer to observe further changes in community composition and distribution in our experimental site. We did, however, observe a significant effect of warming on certain microbial taxa, suggesting that climate change potentially affects the soil microbial community structure by shifting species that can adapt to rapid environmental changes, increasing their dispersal opportunities, and, thus, changing ecosystem functions (Fierer et al., 2003; Gray et al., 2011). Therefore, it was crucial for us to further identify and study the roles and functions of rare microorganisms in the soil bacterial community. However, more in-depth and long-term studies are needed to examine the effects of nitrogen deposition and warming on soil microorganisms.
Data Availability Statement
The datasets presented in this study can be found in online repositories. The names of the repository/repositories and accession number(s) can be found below: BioProject: PRJDB11767/DDBJDRA012263.
Author Contributions
ZM, SD, and YL contributed to conception and design of the study. SL and ZZ organized the database. ZM performed the statistical analysis and wrote the first draft of the manuscript. HS, JZ, YH, and YX wrote sections of the manuscript. All authors contributed to manuscript revision, read, and approved the submitted version.
Funding
This research was financially supported by the Second Tibetan Plateau Scientific Expedition and Research Program (2019QZKK0307), the National Key R&D Program of China (2016YFC0501906), and the Qinghai Provincial Key R&D Program in Qinghai Province (2018-NK-A2).
Conflict of Interest
The authors declare that the research was conducted in the absence of any commercial or financial relationships that could be construed as a potential conflict of interest.
Publisher’s Note
All claims expressed in this article are solely those of the authors and do not necessarily represent those of their affiliated organizations, or those of the publisher, the editors and the reviewers. Any product that may be evaluated in this article, or claim that may be made by its manufacturer, is not guaranteed or endorsed by the publisher.
Acknowledgments
We would like to thank the editor and reviewers for their constructive feedback. We are also grateful to Sir Deer band for their strong moral support.
Supplementary Material
The Supplementary Material for this article can be found online at: https://www.frontiersin.org/articles/10.3389/fevo.2021.709518/full#supplementary-material
References
Allison, S. D., and Treseder, K. K. (2008). Warming and drying suppress microbial activity and carbon cycling in boreal forest soils. Glob. Chang. Biol. 14, 2898–2909. doi: 10.1111/j.1365-2486.2008.01716.x
Aydogan, E. L., Moser, G., Müller, C., Kämpfer, P., and Glaeser, S. P. (2018). Long-term warming shifts the composition of bacterial communities in the phyllosphere of Galium album in a permanent grassland field-experiment. Front. Microbiol. 9:144. doi: 10.3389/fmicb.2018.00144
Belay-Tedla, A., Zhou, X., Su, B., Wan, S., and Luo, Y. (2009). Labile, recalcitrant, and microbial carbon and nitrogen pools of a tallgrass prairie soil in the US Great Plains subjected to experimental warming and clipping. Soil Biol. Biochem. 41, 110–116. doi: 10.1016/j.soilbio.2008.10.003
Bodelier, P. L., and Laanbroek, H. J. (2004). Nitrogen as a regulatory factor of methane oxidation in soils and sediments. FEMS Microbiol. Ecol. 47, 265–277. doi: 10.1016/s0168-6496(03)00304-0
Boxman, A. W., Blanck, K., Brandrud, T.-E., Emmett, B. A., Gundersen, P., Hogervorst, R. F., et al. (1998). Vegetation and soil biota response to experimentally-changed nitrogen inputs in coniferous forest ecosystems of the NITREX project. For. Ecol. Manag. 101, 65–79. doi: 10.1016/s0378-1127(97)00126-6
Castro, H. F., Classen, A. T., Austin, E. E., Norby, R. J., and Schadt, C. W. (2010). Soil microbial community responses to multiple experimental climate change drivers. Appl. Environ. Microbiol. 76, 999–1007. doi: 10.1128/aem.02874-09
Chung, H., Zak, D. R., Reich, P. B., and Ellsworth, D. S. (2007). Plant species richness, elevated CO2, and atmospheric nitrogen deposition alter soil microbial community composition and function. Glob Chang. Biol. 13, 980–989. doi: 10.1111/j.1365-2486.2007.01313.x
De Vries, F. T., Hoffland, E., van Eekeren, N., Brussaard, L., and Bloem, J. (2006). Fungal/bacterial ratios in grasslands with contrasting nitrogen management. Soil Biol. Biochem. 38, 2092–2103. doi: 10.1016/j.soilbio.2006.01.008
DeAngelis, K. M., Pold, G., Topçuoğlu, B. D., van Diepen, L. T., Varney, R. M., Blanchard, J. L., et al. (2015). Long-term forest soil warming alters microbial communities in temperate forest soils. Front. Microbiol. 6:104. doi: 10.3389/fmicb.2015.00104
Fierer, N., Schimel, J., and Holden, P. (2003). Influence of drying–rewetting frequency on soil bacterial community structure. Microbiol. Ecol. 45, 63–71. doi: 10.1007/s00248-002-1007-2
Frey, S. D., Knorr, M., Parrent, J. L., and Simpson, R. T. (2004). Chronic nitrogen enrichment affects the structure and function of the soil microbial community in temperate hardwood and pine forests. For. Ecol. Manag. 196, 159–171. doi: 10.1016/j.foreco.2004.03.018
Fuerst, J. A., Gwilliam, H. G., Lindsay, M., Lichanska, A., Belcher, C., Vickers, J. E., et al. (1997). Isolation and molecular identification of planctomycete bacteria from postlarvae of the giant tiger prawn. Penaeus monodon. Appl. Environ. Microbiol. 63, 254–262. doi: 10.1128/aem.63.1.254-262.1997
Giovannoni, S. J., Schabtach, E., and Castenholz, R. W. (1987). Isosphaera pallida, gen. and comb. nov., a gliding, budding eubacterium from hot springs. Arch.Microbiol. 147, 276–284. doi: 10.1007/bf00463488
Gray, S. B., Classen, A. T., Kardol, P., Yermakov, Z., and Mille, R. M. (2011). Multiple climate change factors interact to alter soil microbial community structure in an old- field ecosystem. Soil Sci. Soc. Am. J. 75, 2217–2226. doi: 10.2136/sssaj2011.0135
Hayden, H. L., Mele, P. M., Bougoure, D. S., Allan, C. Y., Norng, S., Piceno, Y. M., et al. (2012). Changes in the microbial community structure of bacteria, archaea and fungi in response to elevated co2 and warming in an australian native grassland soil. Environ. Microbiol. 14, 3081–3096. doi: 10.1111/j.1462-2920.2012.02855.x
Hu, S., Firestone, M. K., and Chapin, F. S. III (1999). Soil microbial feedbacks to atmospheric CO2 enrichment. Trends Ecol. Evol. 14, 433–437. doi: 10.1016/s0169-5347(99)01682-1
Huse, S. M., Huber, J. A., Morrison, H. G., Sogin, M. L., and Welch, D. M. (2007). Accuracy and quality of massively parallel DNA pyrosequencing. Genome Biol. 8, 1–9.
Janssens, I., Dieleman, W., Luyssaert, S., Subke, J.-A., Reichstein, M., Ceulemans, R., et al. (2010). Reduction of forest soil respiration in response to nitrogen deposition. Nature Geosci. 3, 315–322. doi: 10.1038/ngeo844
Li, J. J., Zheng, Y. M., Yan, J. X., Li, H. J., and He, J. Z. (2013). Succession of plant and soil microbial communities with restoration of abandoned land in the Loess Plateau. China. J. Soils Sediments 13, 760–769. doi: 10.1007/s11368-013-0652-z
Li, Y., Lin, Q., Wang, S., Li, X., Liu, W., Luo, C., et al. (2016). Soil bacterial community responses to warming and grazing in a Tibetan alpine meadow. FEMS Microbiol. Ecol. 92:fiv152. doi: 10.1093/femsec/fiv152
Liu, W., Zhang, Z., and Wan, S. (2009). Predominant role of water in regulating soil and microbial respiration and their responses to climate change in a semiarid grassland. Glob. Chang Biol. 15, 184–195. doi: 10.1111/j.1365-2486.2008.01728.x
Lü, C., and Tian, H. (2007). Spatial and temporal patterns of nitrogen deposition in China: synthesis of observational data. J. Geophys. Res. Atmosph. 112:D22S05.
Lundin, D., Severin, I., Logue, J. B., Östman, Ö, Andersson, A. F., and Lindström, E. S. (2012). Which sequencing depth is sufficient to describe patterns in bacterial α- and β-diversity? Environ. Microbiol. Rep. 4, 367–372. doi: 10.1111/j.1758-2229.2012.00345.x
Ratkowsky, D. A., Olley, J., McMeekin, T., and Ball, A. (1982). Relationship between temperature and growth rate of bacterial cultures. J. Bacteriol. 149, 1–5. doi: 10.1128/jb.149.1.1-5.1982
Rinnan, R., Michelsen, A., Bååth, E., and Jonasson, S. (2007). Fifteen years of climate change manipulations alter soil microbial communities in a subarctic heath ecosystem. Glob. Chang. Biol. 13, 28–39. doi: 10.1111/j.1365-2486.2006.01263.x
Rinnan, R., Stark, S., and Tolvanen, A. (2009). Responses of vegetation and soil microbial communities to warming and simulated herbivory in a subarctic heath. J. Ecol. 97, 788–800. doi: 10.1111/j.1365-2745.2009.01506.x
Rodrigues, J., Pellizari, V. H., Mueller, R., Baek, K., Jesus, E., Paula, F. S., et al. (2013). Conversion of the amazon rainforest to agriculture results in biotic homogenization of soil bacterial communities. Proc. Natl. Acad. Sci. U.S.A. 110, 988–993. doi: 10.1073/pnas.1220608110
Schadt, C. W. (2010). Soil microbial community responses to multiple experimental climate change drivers. Appl. Environ. Microbiol. 76, 999–1007.
Sheik, C. S., Beasley, W. H., Elshahed, M. S., Zhou, X., Luo, Y., and Krumholz, L. R. (2011). Effect of warming and drought on grassland microbial communities. ISME J. 5, 1692–1700. doi: 10.1038/ismej.2011.32
Shen, C., Xiong, J., Zhang, H., Feng, Y., Lin, X., Li, X., et al. (2013). Soil pH drives the spatial distribution of bacterial communities along elevation on Changbai Mountain. Soil Biol. Biochem. 57, 204–211. doi: 10.1016/j.soilbio.2012.07.013
Singh, B. K., Bardgett, R. D., Smith, P., and Reay, D. S. (2010). Microorganisms and climate change: terrestrial feedbacks and mitigation options. Nat. Rev. Microbiol. 8, 779–790. doi: 10.1038/nrmicro2439
Stephan, A., Meyer, A. H., and Schmid, B. (2000). Plant diversity affects culturable soil bacteria in experimental grassland communities. J. Ecol. 88, 988–998. doi: 10.1046/j.1365-2745.2000.00510.x
Sul, W. J., Cole, J. R., Jesus, E. D. C., Wang, Q., Farris, R. J., Fish, J. A., et al. (2011). Bacterial community comparisons by taxonomy-supervised analysis independent of sequence alignment and clustering. Proc. Natl. Acad. Sci.U.S.A. 108, 14637–14642. doi: 10.1073/pnas.1111435108
Tao, X., Feng, J., Yang, Y., Wang, G., Tian, R., Fan, F., et al. (2020). Winter warming in Alaska accelerates lignin decomposition contributed by Proteobacteria. Microbiome 8, 1–12.
Tripathi, B. M., Kim, M., Singh, D., Lee-Cruz, L., Lai-Hoe, A., Ainuddin, A., et al. (2012). Tropical soil bacterial communities in Malaysia: pH dominates in the equatorial tropics too. Microbiol. Ecol. 64, 474–484. doi: 10.1007/s00248-012-0028-8
Tuyet, D. T. A., Tanaka, T., Sohrin, R., Nagaosa, K., and Kato, K. (2015). Effects of warming on microbial communities in the coastal waters of temperate and subtropical zones in the Northern Hemisphere, with a focus on Gammaproteobacteria. J. Oceanogr. 71, 91–103. doi: 10.1007/s10872-014-0264-2
Waghmode, T. R., Chen, S., Li, J., Sun, R., Liu, B., and Hu, C. (2018). Response of nitrifier and denitrifier abundance and microbial community structure to experimental warming in an agricultural ecosystem. Front. Microbiol. 9:474. doi: 10.3389/fmicb.2018.00474
Wang, G., Ju, Q., Cheng, G., and Lai, Y. (2002). Soil organic carbon pool of grassland soils on the qinghai-tibetan plateau and its global implication. Sci. Total Environ. 291, 207–217. doi: 10.1016/S0048-9697(01)01100-7
Wang, Q., Garrity, G. M., Tiedje, J. M., and Cole, J. R. (2007). Naive Bayesian classifier for rapid assignment of rRNA sequences into the new bacterial taxonomy. Appl. Environ. Microbiol. 73, 5261–5267. doi: 10.1128/aem.00062-07
Wang, S., Duan, J., Xu, G., Wang, Y., Zhang, Z., Rui, Y., et al. (2012). Effects of warming and grazing on soil n availability, species composition, and anpp in an alpine meadow. Ecology 93:1408. doi: 10.1890/11-1408.1
Waring, B. G., Averill, C., and Hawkes, C. V. (2013). Differences in fungal and bacterial physiology alter soil carbon and nitrogen cycling: insights from meta- analysis and theoretical models. Ecol. Lett. 16, 887–894. doi: 10.1111/ele.12125
Xiong, J., Sun, H., Peng, F., Zhang, H., Xue, X., Gibbons, S. M., et al. (2014). Characterizing changes in soil bacterial community structure in response to short-term warming. FEMS Microbiol. Ecol. 89, 281–292. doi: 10.1111/1574-6941.12289
Xiong, Q., Pan, K., Zhang, L., Wang, Y., Li, W., He, X., et al. (2016). Warming and nitrogen deposition are interactive in shaping surface soil microbial communities near the alpine timberline zone on the eastern Qinghai–Tibet Plateau, southwestern China. Appl. Soil Ecol. 101, 72–83. doi: 10.1016/j.apsoil.2016.01.011
Xue, J.-H., Mo, J.-M., Li, J., and Li, D.-J. (2007). The short-term response of soil microorganism number to simulated nitrogen deposition. Guihaia 27, 174–179.
Yang, Y., Gao, Y., Wang, S., Xu, D., Yu, H., Wu, L., et al. (2014). The microbial gene diversity along an elevation gradient of the Tibetan grassland. ISME J. 8, 430–440. doi: 10.1038/ismej.2013.146
Zhang, B., Chen, S., Zhang, J., He, X., Liu, W., Zhao, Q., et al. (2015). Depth-related responses of soil microbial communities to experimental warming in an alpine meadow on the Q inghai-T ibet P lateau. Eur. J. Soil Sci. 66, 496–504. doi: 10.1111/ejss.12240
Zhang, K., Shi, Y., Jing, X., He, J.-S., Sun, R., Yang, Y., et al. (2016). Effects of short-term warming and altered precipitation on soil microbial communities in alpine grassland of the Tibetan Plateau. Front. Microbiol. 7:1032. doi: 10.3389/fmicb.2016.01032
Zhang, W., Parker, K., Luo, Y., Wan, S., Wallace, L., and Hu, S. (2005). Soil microbial responses to experimental warming and clipping in a tallgrass prairie. Glob. Chang. Biol. 11, 266–277. doi: 10.1111/j.1365-2486.2005.00902.x
Zhang, Y., Dong, S., Gao, Q., Liu, S., Zhou, H., Ganjurjav, H., et al. (2016). Climate change and human activities altered the diversity and composition of soil microbial community in alpine grasslands of the Qinghai-Tibetan Plateau. Sci. Total Environ. 562, 353–363. doi: 10.1016/j.scitotenv.2016.03.221
Zhao, Z., Dong, S., Jiang, X., Liu, S., Ji, H., Li, Y., et al. (2017). Effects of warming and nitrogen deposition on CH4. CO2 and N2O emissions in alpine grassland ecosystems of the Qinghai-Tibetan Plateau. Sci. Total Environ. 592, 565–572. doi: 10.1016/j.scitotenv.2017.03.082
Zheng, Y., Yang, W., Sun, X., Wang, S.-P., Rui, Y.-C., Luo, C.-Y., et al. (2012). Methanotrophic community structure and activity under warming and grazing of alpine meadow on the Tibetan Plateau. Appl. Microbiol. Biotechnol. 93, 2193–2203. doi: 10.1007/s00253-011-3535-5
Zhou, J., Xue, K., Xie, J., Deng, Y., Wu, L., Cheng, X., et al. (2012). Microbial mediation of carbon-cycle feedbacks to climate warming. Nat. Clim. Chang. 2, 106–110. doi: 10.1038/nclimate1331
Zhou, J. Z., Kai, X., Xie, J. P., Ye, D., and Luo, Y. Q. (2011). Microbial mediation of carbon-cycle feedbacks to climate warming. Nat. Clim. Change 2, 106–110. doi: 10.1038/nclimate1331
Zhou, Y., Hagedorn, F., Zhou, C., Jiang, X., Wang, X., and Li, M.-H. (2016). Experimental warming of a mountain tundra increases soil CO 2 effluxes and enhances CH 4 and N 2 O uptake at Changbai Mountain. China. Sci. Rep. 6, 1–8.
Keywords: Qinghai-Tibetan Plateau, soil bacteria, diversity, community composition, nitrogen application, warming
Citation: Mu Z, Dong S, Li Y, Li S, Shen H, Zhang J, Han Y, Xu Y and Zhao Z (2021) Soil Bacterial Community Responses to N Application and Warming in a Qinghai-Tibetan Plateau Alpine Steppe. Front. Ecol. Evol. 9:709518. doi: 10.3389/fevo.2021.709518
Received: 14 May 2021; Accepted: 02 August 2021;
Published: 03 September 2021.
Edited by:
Jianshuang Wu, Institute of Environment and Sustainable Development in Agriculture, Chinese Academy of Agricultural Sciences, ChinaReviewed by:
Xuyang Lu, Institute of Mountain Hazards and Environment (IMHE), Chinese Academy of Sciences, ChinaJinsong Wang, Institute of Geographic Sciences and Natural Resources Research, Chinese Academy of Sciences, China
Copyright © 2021 Mu, Dong, Li, Li, Shen, Zhang, Han, Xu and Zhao. This is an open-access article distributed under the terms of the Creative Commons Attribution License (CC BY). The use, distribution or reproduction in other forums is permitted, provided the original author(s) and the copyright owner(s) are credited and that the original publication in this journal is cited, in accordance with accepted academic practice. No use, distribution or reproduction is permitted which does not comply with these terms.
*Correspondence: Shikui Dong, ZG9uZ3NoaWt1aUBiamZ1LmVkdS5jbg==; ZG9uZ3NoaWt1aUBzaW5hLmNvbQ==