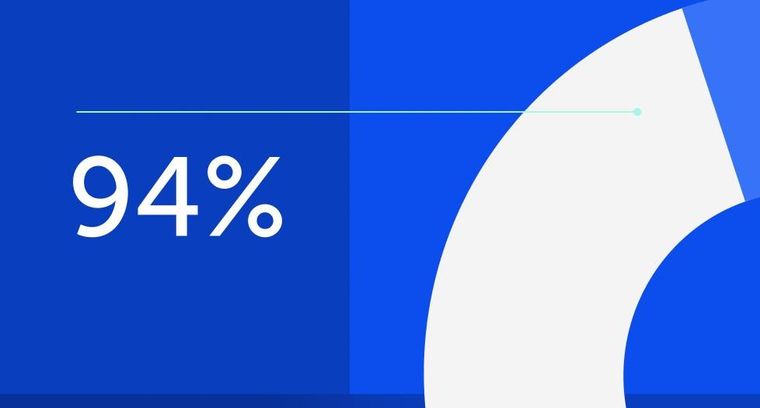
94% of researchers rate our articles as excellent or good
Learn more about the work of our research integrity team to safeguard the quality of each article we publish.
Find out more
ORIGINAL RESEARCH article
Front. Ecol. Evol., 15 June 2021
Sec. Ecophysiology
Volume 9 - 2021 | https://doi.org/10.3389/fevo.2021.704105
This article is part of the Research TopicEcophysiological Analysis of Vulnerability to Climate Warming in EctothermsView all 15 articles
Global warming has led to an increase in the frequency, duration, and intensity of heat waves in the summer, which can cause frequent and acute heat stress on ectotherms. Thus, determining how ectothermic animals respond to heat waves has been attracting growing interest among ecologists. However, the physiological and biochemical responses to heat waves in reptiles, especially aquatic reptiles, are still poorly understood. The current study investigated the oxidant physiology, immunity, and expression levels of heat shock proteins (HSP) mRNA after exposure to a simulated heat wave (1 week, 35 ± 4°C), followed by a recovery period (1 week, 28 ± 4°C) in juvenile Asian yellow pond turtle (Mauremys mutica), a widely farmed aquatic turtle in East Asia. The contents of malondialdehyde (MDA) in the liver and muscle were not significantly affected by the heat wave or recovery. Of all antioxidant enzymes, only the activity of glutathione peroxidase (GSH-Px) in muscles increased after heat wave, while the total superoxide dismutase (T-SOD), catalase activity (CAT), and total antioxidant capacity (T-AOC) did not change during the study. The organo-somatic index for the liver and spleen of M. mutica decreased after the heat wave but increased to the initial level after recovery. In contrast, plasma lysozyme activity and serum complement C4 levels increased after the heat wave, returning to the control level after recovery. In addition, heat waves did not alter the relative expression of HSP60, HSP70, and HSP90 mRNA in the liver. Eventually, heat wave slightly increased the IBR/n index. Therefore, our results suggested that heat waves did not lead to oxidative damage to lipids in M. mutica, but deleteriously affected the turtles’ immune organs. Meanwhile, the constitutive levels of most antioxidative enzyme activities, HSPs and enhanced blood immune functions might protect the turtles from the threat of heat waves under the current climate scenarios.
The global average air temperature has increased by approximately 0.85°C over the past century and may increase by a further 0.3–4.8°C by the end of this century (IPCC, 2014). Such climate change has dramatically affected fauna, causing problems, such as advances in phenology, shifts in distribution, and increased risk of species extinction (Sinervo et al., 2010; Hoegh-Guldberg et al., 2019). In addition to the mean temperature, the impacts of climate warming on animals are also associated with the increasing frequency, duration, and intensity of extreme temperature events, such as summer heat waves (Bozinovic et al., 2011; IPCC, 2014). Indeed, there is increasing awareness on heat wave events that may pose greater threats to animals than gradual warming (Thompson et al., 2013; Vasseur et al., 2014; Pansch et al., 2018), as heat waves can induce more severe impacts on animals than increasing mean temperatures, including on morphology, physiology, reproduction and even survival (Bauerfeind and Fischer, 2014; Zhang et al., 2018; Breitenbach et al., 2020). Therefore, investigating how animals respond to heat wave events is essential to enrich our understanding of the vulnerability of animals to climate warming.
For ectotherms, thermal environments can strongly influence their biological processes, including behavior, metabolic rates, growth, and reproduction (Angiletta, 2009; Adamo and Lovett, 2011; Goessling et al., 2019), as their body temperatures are highly dependent on the ambient temperature. The body temperatures of ectotherms usually fluctuate to some extent during the day or season, under natural conditions (Christian and Weavers, 1996; Angiletta, 2009). Thus, ectotherms are well adapted to daily and seasonal temperature variations. Nevertheless, dramatic temperature variation would have a negative impact on ectotherms at several levels, including molecular, biochemical, and physiological (Stahlschmidt et al., 2017; Ferreira-Rodríguez et al., 2018; Gao et al., 2021). For example, acute thermal stress can stimulate the formation of reactive oxygen species (ROS) and in turn induce oxidative damage in ectotherms (Ben Ameur et al., 2012; Zhang et al., 2019). To clear ROS and maintain the oxidative balance, antioxidant enzyme systems, including superoxide dismutase (SOD), glutathione peroxidase (GSH-Px), and catalase (CAT), are activated (Zhang et al., 2019; Baker et al., 2020). Additionally, immune functions in ectotherms are sensitive to acute temperature changes (Goessling et al., 2019; Gao et al., 2021). For example, innate immunity of common musk turtles (Sternotherus odoratus) compensated for new temperature when exposed to a 5 or 10°C temperature change for 48 h (Goessling et al., 2019). Moreover, acute heat exposure increases the expression of heat shock proteins (HSPs), which act as molecular chaperones to protect cells from heat damage and enhance thermal resistance in many ectotherms, including (Zhao and Jones, 2012), fish (Narum et al., 2013), and reptiles (Tedeschi et al., 2015).
Increasingly common heat waves may cause frequent and acute heat stress on ectotherms. However, many studies have exposed animals to different acute constant temperature heat waves, which unfortunately neglects the diurnal fluctuations in temperature under natural conditions (Dittmar et al., 2014; Leicht et al., 2019). This lack of ecological relevance may lead to misleading interpretations, as the performance of ectotherms is not always identical under constant and fluctuating thermal conditions (Bozinovic et al., 2011; Moore et al., 2021). Moreover, the biochemical and physiological responses to heat waves are still poorly understood in ectotherms, especially in non-avian reptiles, which are particularly vulnerable to climate warming (Bauerfeind and Fischer, 2014; Zhang et al., 2018). Only a few studies have detected how heat waves affect reptile biochemistry and physiology, and no consistent patterns have been identified. For example, a simulated heat wave reduced oxidative damage in the corn snake (Pantherophis guttatus) (Stahlschmidt et al., 2017) but did not affect antioxidant capacity in a desert lizard (Phrynocephalus przewalskii) (Zhang et al., 2018); heat waves reduced immunity in rattlesnakes (Crotalus durissus) (Fabrício-Neto et al., 2019) but not in P. guttatus (Stahlschmidt et al., 2017).
The Asian yellow pond turtle (Mauremys mutica) is an aquatic turtle distributed in China, Japan, and Vietnam. It has been classified as “endangered” on the IUCN red list but is commonly cultured in southern China due to its high economic value as food, pets, and in traditional Chinese medicine (Lu et al., 2020; Wei et al., 2020). Recent studies have shown that extreme heat stress could downregulate the expression of immunity-associated genes in M. mutica, which may be the main reason underlying the increased morbidity and mortality rates associated with extreme heat events in turtle farms (Wei et al., 2020; Gao et al., 2021). However, like other turtles, the response of M. mutica to heat waves has not yet been investigated. In the current study, we conducted an experiment in M. mutica to reveal the effects of heat waves on its antioxidant responses, immune function, and gene expression. We exposed M. mutica to a simulated heat wave with subsequent recovery and measured malondialdehyde (MDA), total superoxide dismutase (T-SOD), GSH-Px, CAT activity, and total antioxidant capacity (T-AOC) in the liver and muscle. We also calculated the organo-somatic index (OSI) for the liver and spleen; quantified serum complement protein (C3, C4) levels, and lysozyme activity in the plasma; and expression of HSPs (HSP60, HSP70, HSP90) in the liver. At last, we used the integrated biomarker response (IBR) approach to evaluate the comprehensive effects of heat wave on M. mutica. We predicted that heat waves would induce oxidative stress and depress immune function in M. mutica, whereas antioxidant capacity and expression of HSPs would be enhanced to protect M. mutica from heat waves.
The animal study was reviewed and approved by Animal Ethical and Welfare Committee of Wenzhou University (Approval No. WZU-049).
In late July 2018, 30 yearling M. mutica (carapace length: 53.0 ± 0.6 mm; body weight: 30.4 ± 1.1 g; Supplementary Figure 1) were purchased from a turtle farm in Jiaxing, Zhejiang province (120.89°E, 30.77°N). Turtles were individually raised in plastic boxes (length∗ width∗height = 35 cm∗25 cm∗10 cm) with approximately 5-cm-depth water and housed in an incubator (KB400, Binder GmbH, Tuttlingen, Germany) under a fluctuating temperature of 28 ± 4°C for 1 week (set at a ten-step programmed ramp temperatures, see details in Supplementary Figure 2 and Supplementary Table 1). The acclimation temperature simulated the average fluctuation range of daily air temperatures in Jiaxing in July and August (1981--2010; data were obtained from ‘‘China Meteorological Data Service Center’’)1. Turtles were provided a 13 L:11D cycle by eight fluorescent lamps in the incubator (light on between 6:00 and 19:00) and fed a commercial diet at 10:00 every day. The water was changed every day when the light was turned off.
After 1-week acclimation, ten randomly selected turtles were left in the incubator and defined as the “control group” (n = 10), while the other 20 turtles were assigned to another incubator around 7:00 and were raised as described above, except for exposure to a simulated heat wave (35 ± 4°C, the average temperatures in Jiaxing when heat waves occurred, from July to August, 2011–2017, data were obtained from “https://lishi.tianqi.com”; set at a ten-step programmed ramp temperatures, see details in Supplementary Figure 2 and Supplementary Table 1). One week later, we sampled all the ten turtles from the control group, and randomly selected ten turtles which had been exposed to heat waves (heat wave group, n = 10) between 7:00 and 9:00 (Supplementary Figure 2). The remaining ten turtles were transferred back to 28 ± 4°C and also sampled between 7:00 and 9:00 after 1 week recovery (recovery group, n = 10; Supplementary Figure 2). Thus, the ten turtles from the recovery group experienced 1 week heat wave exposure and 1 week recovery. Overall, the thermal regime of the control group covered the set-point temperature range of M. mutica (25–29°C, unpublished data), and the thermal peak during the heat wave was approximately 2°C below the critical thermal maximum of M. mutica (approximately 41°C, according to Lu et al., 2020).
When sampled, turtles were immediately sacrificed with decapitation followed by pithing using heavy shears according to AVMA Guideline for the Euthanasia of Animals (2013 Edition), to separate the spleen, liver, and skeletal muscle on ice. Fresh liver and spleen were blotted free of blood, weighed (ML204, Mettler Toledo, Switzerland, ± 0.0001 g), and frozen in liquid nitrogen. Blood was collected from the carotid artery immediately and centrifuged at 3,000 rpm for 15 min (Fresco 21, Thermo Fisher Scientific, Waltham, MA, United States) to separate serum and plasma. All samples were stored in a –80°C refrigerator (CryoCube F570, Eppendorf AG, Barkhausenweg, Hamburg, Germany) before analysis.
Commercial kits (Nanjing Jiancheng Bioengineering Institute, Nanjing, China) were used to detect MDA content, T-AOC, and antioxidant enzyme activity (CAT, GSH-Px, and SOD) in the liver and muscle following the manufacturer’s instructions. MDA content was evaluated by measuring the absorbance of a red compound produced by thiobarbituric acid and malondialdehyde at 532 nm. T-AOC was measured based on the absorbance of the Fe2+-o-phenanthroline complex produced by o-phenanthroline reacted with Fe2+, from which Fe3+ was reduced by the reducing agents at 520 nm. CAT activity was estimated by measuring H2O2 consumption at 405 nm. GSH-Px activity was examined based on the GSH consumption rate at 412 nm during the enzymatic reaction of GSH-Px, which catalyzes dithiodinitrobenzoic acid. SOD activity was determined by monitoring the rate of cytochrome c reduction by superoxide ions at 550 nm in the xanthine-xanthine oxidase system.
The organo-somatic index (OSI) for the liver and spleen were calculated using the following formula:
OSI = organ weight (g)/body weight (g) × 100
Enzyme-linked immunosorbent assay (ELISA) kits (Shanghai MLBIO Biotechnology Co., LTD, Shanghai, China) were used to measure serum C3 and C4 levels, and plasma lysozyme activity according to the manufacturer’s instructions. Samples were combined with anti-fish horseradish peroxidase-linked antibody, and the color was developed using 3,3′,5,5′-tetramethylbenzidine after complete washing. The reaction was terminated by adding a sulfuric acid solution, and absorbance was measured at 450 nm.
Total RNA was extracted from the livers of four randomly selected individuals from each group using the TransZol Up Plus RNA kit (TransGen Biotech Co., Ltd., Beijing, China). RNA quality was evaluated by measuring the absorbance ratio at 260/280 nm using an ultraviolet spectrophotometer (NanoDrop 2000, Thermo Fisher Scientific, Waltham, MA, United States). The acceptable range was 1.8–2.1. cDNA was generated from the extracted RNA using a 2 × EasyTaq® PCR SuperMix kit (TransGen Biotech Co., Ltd., Beijing, China), according to the manufacturer’s instructions.
Real-time quantitative PCR was performed in duplicate for each sample to determine the relative expression of HSP60, HSP70, and HSP90 on a LightCycler 480 II (Roche Diagnostics Ltd., Forrentrasse, Switzerland), using the β-actin gene as an endogenous reference. All primers for RT-PCR (Table 1) were designed using Primer Premier 5.0 (PREMIER Biosoft International, United States). The reactions were carried out in a 20-μL reaction mixture containing 10 μL of TransStart Top Green qPCR SuperMix (TransGen Biotech Co., Ltd., Beijing, China), reverse-transcribed cDNA templates, and 0.4 μL of each primer. The relative mRNA level was calculated using the 2–ΔΔCt method (Livak and Schmittgen, 2001).
To combine all the measured biomarkers and evaluate the general responses, integrated biomarker response (IBR) index was calculated following the method of Beliaeff and Burgeot (2002) and modified by Marigómez et al. (2013): (1) calculation of mean (x) and standard deviation (s) for each biomarker from all the groups; (2) standardization of data for each biomarker: xi′ = (xi-x)/s, where xi′ is the standardized value of the biomarker, xi is the mean value of a biomarker from each group; (3) Z = xi′ or –xi′ in the case of biomarker responding to heat wave by activation or inhibition, then the minimum standardized value (xmin′) for all groups for each biomarker was obtained, and the absolute value of xmin′ was added to Z, yi = Z + | xmin′|; (4) yi was plotted on a star plot to display biomarker results, indicating the degree of biological effects for each biomarker in response to different thermal conditions; (5) star plot area connecting the ith and the (i + 1)th radius coordinates (Ai) was computed as Ai = [(yi × yi+1 × sin(2π/n)]/2, where yi and yi+1 represent individual standardized values of each biomarker and its next biomarker in the star plot, respectively, and n is the number of biomarkers; (6) IBR = ΣAi as IBR was the total area for each group, and finally IBR/n was presented in the results considering IBR was depend on the number of biomarkers.
Data were checked for normality and homogeneity of variance using the Shapiro-Wilk and Levene’s tests, respectively. One-way ANOVA was used to test the differences between groups, followed by the post hoc Tukey’s HSD test. The Kruskal-Wallis test followed by Mann-Whitney U post hoc test was used when the assumptions of one-way ANOVA were violated. Data are expressed as the mean ± SE. The significance level was set to α = 0.05. All analyses were performed with Statistica 6.0, and all graphs were drawn using SigmaPlot 12.5, except that calculation of IBR and star plot were performed by Excel (Microsoft 365).
MDA content in the liver and muscles was not significantly affected by heat waves, although MDA content tended to increase by more than 50% in the livers of turtles exposed to heat waves (Figure 1 and Table 2).
Figure 1. Effects of heat wave on MDA content in the liver and muscle of Mauremys mutica. Data were represented as mean ± standard error (SE).
Table 2. Statistical results of the effects of heat wave on oxidative stress indices in liver and muscle of Mauremys mutica.
For all antioxidant components, heat waves only significantly influenced the activity of GSH-Px in the muscle (Table 2), which increased after exposure for 1 week to the heat wave and decreased after 1 week of recovery (Figures 2A–D).
Figure 2. Effects of heat wave on TAC (A), CAT activity (B), GSH-Px activity (C), and SOD activity (D) in the liver and muscle of Mauremys mutica. Data were represented as mean ± standard error (SE).
Heat wave treatment significantly reduced the hepatosomatic index [F(2, 27) = 3.217, P = 0.056] and spleen somatic index [F(2,27) = 3.825, P = 0.034] but both organo-somatic indices slightly increased after 1-week recovery (Figure 3).
Figure 3. Effects of heat wave on organo-somatic index of Mauremys mutica. Data were represented as mean ± standard error (SE).
C3 levels did not significantly change during the experiment (C3: H = 5.544, P = 0.063, Figure 4B), while the lysozyme activity and C4 level increased significantly after the heat wave and decreased to the control level after 1 week of recovery [lysozyme: F(2,27) = 11.593, P < 0.001, Figure 4A; C4: F(2,27) = 11.137, P < 0.001, Figure 4C].
Figure 4. Effects of heat wave on the lysozyme activity (A), C3 level (B), and C4 level (C) of Mauremys mutica. Data were represented as mean ± standard error (SE).
Heat waves did not alter the relative expression levels of HSP60, HSP70, or HSP90 mRNA in the liver of M. mutica [HSP60: F(2, 9) = 0.04, P = 0.959; HSP70: F(2, 9) = 0.115, P = 0.989; HSP90: F(2, 9) = 0.108, P = 0. 898; Figure 5].
Figure 5. Effect of heat wave on the relative expression levels of HSP60, HSP70 and HSP90 mRNA in liver of Mauremys mutica. Data were represented as mean ± standard error (SE).
All the 18 measured biomarkers were represented in star plot (Figure 6A). Most sensitive biomarkers to heat wave were GSH-Px in liver (GSH-Pxl), GSH-Px in muscle (GSH-Pxm), spleen somatic index (SSI), lysozyme activity (Lys), C4 level, HSP60 and HSP90, concerning the magnitude of differences between thermal treatments. The IBR/n index was marginally higher in heat wave group, comparing with control and recovery groups (Figure 6B).
Figure 6. Integrated biomarker response (IBR) star plot (A), and IBR/n values (B) for Mauremys mutica after thermal treatments. MDAl, MDA in liver; MDAm, MDA in muscle; TACl:TAC in liver; TACm:TAC in muscle; CATl, CAT in liver; CATm, CAT in muscle; SH-Pxl, GSH-Px in liver; GSH-Pxm, GSH-Px in muscle; SODl, SOD in liver; SODm, SOD in muscle; HIS, hepatosomatic somatic indices; SSI, spleen somatic indices; Lys, lysozyme.
The current study demonstrated that the effects of a simulated heat wave on the biochemistry and physiology of the aquatic turtle M. mutica were complex and transient. After exposure to a 1-week heat wave, no significant oxidative damage was found, and only GSH-Px activity of the antioxidant indices was enhanced in M. mutica. Heat wave exposure deleteriously affected the liver and spleen, but increased the blood lysozyme activity and C4 level of the turtle. Moreover, heat waves did not affect the relative expression of HSPs in the turtle liver. A slight increasing in IBR/n value was induced by heat wave. Following a 1-week recovery period, the effects of heat waves disappeared, indicating that the protection mechanism was effective in M. mutica.
As the end product of lipid peroxidation induced by reactive oxygen species (ROS), MDA content indirectly reflects the degree of oxidative damage to cell membrane lipids—especially phospholipids, polyunsaturated fatty acids (PUFA), glycolipids and cholesterol (McCay et al., 1976; Parrish, 2013; Ayala et al., 2014). In the present study, MDA content did not significantly increase in the liver or muscle of turtles after the heat wave and recovery, indicating that heat waves did not induce visible adverse effects on the lipids in the tissues of M. mutica. A similar pattern was reported in the Chinese soft-shell turtle (Pelodiscus sinensis) after exposure to acute heat stress and recovery (Zhang et al., 2019) and in two marine gastropods (Nerita atramentosa and Austrocochlea concamerata) during a 1-week heat wave exposure experiment (Leung et al., 2019). However, increased MDA content induced by heat waves has been observed in other ectotherms, such as a desert lizard (Eremias multiocellata) (Han et al., 2020) and marine gastropod (Austrocochlea constricta) (Leung et al., 2019). These conflicting results indicate that heat waves have complex effects on the oxidative physiology in ectotherms. Otherwise, M. mutica may be somewhat resistant to lipid peroxidation, like some long-living marine mollusks which are usually able to acclimate to new temperatures and/or maintain homeostasis upon exposure to changing temperatures (Munro and Blier, 2012).
In the present study, heat waves did not affect T-SOD activity, CAT activity, or T-AOC in M. mutica but did elevate GSH-Px activity in the muscle of M. mutica. One possible explanation for this is that GSH-Px activity is more sensitive to thermal fluctuations than SOD and CAT. Similarly, a simulated heat wave did not influence SOD activity in the desert lizard P. przewalskii (Zhang et al., 2018). Acute heat stress had similar effects on the antioxidant enzymes in ectotherms. For example, in the Chinese soft-shell turtle, acute heat shock only altered GSH-Px activity and had no impact on the enzymatic activity of CAT and SOD (Zhang et al., 2019), whereas the activity of all three enzymes in a marine fish (Cyprinodon variegatus) did not change after acute heat exposure (Baker et al., 2020). Furthermore, the response of antioxidant enzymes to temperature change varied among tissues; it has previously been observed in the Chinese soft-shell turtle that GSH-Px activity increased in the kidney but decreased in the liver (Zhang et al., 2019). In the marine fish Sparus aurata, SOD activity increased in the liver but decreased in the brain after heat stress (Madeira et al., 2016). Previous studies showed that GSH-Px could prevent lipid peroxidation in cell membranes (McCay et al., 1976; Marinho et al., 1997). Hence, the enhanced GSH-Px activity may be one possible reason for the unchanged MDA content after heat wave exposure in M. mutica.
In the current study, the hepatosomatic and spleen somatic indices decreased after a 1-week heat wave, implying potential damage in turtle immune organs to heat waves (Figure 3). The hepatosomatic index has also been widely used as a rough indicator of the overall health of aquatic animals (Ben Ameur et al., 2012; Blazer et al., 2018). Reduction of the hepatosomatic index may indicate poor health triggered by the stress of heat waves. This result contradicts a previous finding that repeated daily acute heat shock did not change the hepatosomatic index in cold-water fish (Salvelinus namaycush) (Guzzo et al., 2019). Considering the key role of the liver in metabolism, another possible explanation for the reduced hepatosomatic index is that heat waves may increase energy expenditure or reduce feeding rate in M. mutica; this has been recognized in two fiddler crab species raised under warmer conditions (Vianna et al., 2020). Also, the decline of hepatosomatic index may suggest heat wave caused an energetic trade off in the turtles, as most ectothermic animals switch from a physiological compensation strategy (i.e., allocation of energy to cellular stress responses, repair mechanisms and molecular adjustments) to a metabolic conservation strategy (i.e., blocking stress responses) (Marshall et al., 2011; Yao and Somero, 2013; Vianna et al., 2020). Thus, the lack of responses in antioxidant enzymes as described above may reflect an exhaustion of the cellular machinery has occurred and animals went into metabolic depression. Further research is needed to elucidate this phenomenon by detecting the response of energy metabolism and feeding behavior to heat waves, and determining the level of tissue damage that actually occurred through histopathological analyses in turtles, which was a possible limitation to our study. As the dominant secondary lymphoid organ, the spleen plays an important role in defending against infection by parasites, bacteria, and viruses in reptiles (Zimmerman et al., 2010). In the present study, heat waves decreased the relative size of the spleen and that may impair immunity in M. mutica, which could be a potential cause of severe disease infection in M. mutica facing frequent heat stress during late spring and early summer (Gao et al., 2021). Cooling strategies for turtles in the farms should be taken into account during heat wave events, such as providing more shelters or increasing water flow in the ponds for turtles. However, the relationship between disease outbreaks and the spleen somatic index remains unclear. The potential damage to the turtle liver and spleen was not permanent, as the two indices increased to control levels after 1-week recovery. But it was also unclear whether turtles’ recovery ability would be permanently suppressed when facing recurrent heat wave stress or not.
Contrary to the immune organs, plasma lysozyme activity and serum complement levels in M. mutica increased after exposure to the simulated heat wave and decreased to control levels after 1 week of recovery, whereas C3 levels did not show significant changes (Figure 4). This discrepancy reflected the varied response of the turtle immune system to temperature changes between organ and biochemical levels. Lysozymes and the complement system are key components in the reptile innate immune system, which mainly functions to kill bacteria or digest pathogens (Zimmerman et al., 2010; Baker et al., 2019). Accordingly, the elevated lysozyme activity and complement levels may indicate enhanced bacteria killing ability of M. mutica when exposed to heat waves, which was not directly measured in the current study. This result challenged our hypothesis and was in disagreement with previous studies that reported that heat waves inhibited immune functions of ectothermic animals, including snakes (Stahlschmidt et al., 2017), lizard (Han et al., 2020), fish (Dittmar et al., 2014), and freshwater snail (Leicht et al., 2013). Nonetheless, the current findings are not rare. For example, a previous study showed that a simulated heat wave increased the activity of immune-related (phenoloxidase and lysozyme-like) enzymes and resistance to bacterial infection in a cricket (Gryllus texensis) (Adamo and Lovett, 2011). Additionally, high temperature (37°C) did not weaken the immune function of the red-eared slider turtle (Trachemys scripta), whose critical thermal maximum was 40–42°C (Zimmerman et al., 2017). Overall, the diverse immune response of ectotherms to temperature change may be due to the variation of treatments from different research, or the varying thermal sensitivity of immune indices among taxa (Stahlschmidt et al., 2017; Zimmerman et al., 2017).
Expression of heat shock proteins was found to be upregulated after acute or gradual heat exposure in many ectotherms, including turtles, protecting organisms from physiological damage caused by high temperatures (Tedeschi et al., 2015; Dang et al., 2018; Harada and Burton, 2019). Unexpectedly, in the present study, heat waves did not induce significant upregulation in the expression of hsp gene in the liver of M. mutica, even though the turtles experienced 37–39°C for nearly 5 h daily during the heat wave (Supplementary Figure 2). A similar phenomenon was also observed in a montane lizard (Phrynosoma hernandesi) exposed to acute heat stress at 36°C for 4 h (Refsnider et al., 2021) and in a skink after 24 h exposure to 35°C (Plestiodon chinensis) (Dang et al., 2018). One possible explanation was exhaustion of the cellular stress responses indicated by the decreased hepatosomatic index as described above. Another possible reason may be that the heat wave climax or the duration of heat stress was insufficient to activate high expression of HSPs, and high-temperature resistance of hatchling M. mutica would be elevated after warm acclimation (Lu et al., 2020). Future work to explore whether 1-week heat waves would enhance turtle tolerance to high temperatures is warranted. Contrary interpretation also makes sense, since basal HSPs expression levels may maintain constantly high in the turtle to protect them against heat stress during summer. This has been previously observed in intertidal ectotherms exposed to steep temperature changes (Madeira et al., 2012). Alternatively, the response of HSPs to heat stress varies among tissues. For example, the expression of HSP60 was upregulated in the embryonic brain of loggerhead sea turtles (Caretta caretta) but was not altered in the heart after exposure to 36°C for 3 h (Tedeschi et al., 2015). In addition, the sampling time may be another cause. In the current study, turtles were sampled around 7:00–9:00 on the eighth day, and thus had adapted to moderate temperatures overnight. Previous research found that HSP70 in the liver decayed faster during 1-h post-stress adaptation than in other tissues, such as the lung and muscle in the Chinese soft-shell turtle (Li et al., 2012). Consequently, the expression of HSPs in the liver may not be a suitable biomarker for evaluating the stress of heat waves in turtles. To obtain a more comprehensive understanding of molecular responses to heat waves in turtles, further studies could introduce transcriptome analysis, which has been increasingly used to uncover transcriptional responses to heat stress in reptiles (Bentley et al., 2017; Gao et al., 2021), and assess the temporal and spatial variation in gene expression under heat waves.
Combining the responses of multiple biomarkers, IBR provided a valid tool to assess ecological risk and animal health status in relation to environmental stressors, including chemical contaminants, evaluated CO2 level and extreme temperatures (Beliaeff and Burgeot, 2002; Campos et al., 2019). In current work, seven biomarkers (GSH-Pxl, GSH-Pxm, SSI, Lys, C4, HSP60, and HSP90) seemed to be more sensitive to heat wave according to the star plot, which was consistent with the response patterns of these biomarkers to temperature treatments, even some did not alter significantly. Heat wave slightly increased the IBR/n index, indicating a mild stress on the turtles by heat wave. The IBR/n values of recovery group and control group were similar, suggesting the stress induced by heat wave diminished after 1 week recovery and the physiological and biochemical protection mechanism was effective in M. mutica.
In summary, the current study provides an initial insight into the complex physiological and biochemical effects of heat waves in an aquatic turtle. In M. mutica, a simulated heat wave did not induce significant oxidative stress, but may nevertheless cause damage to the immune organs. Meanwhile, the enhanced activity of antioxidant enzymes (GSH-Px) and immune functions of lysozyme activity and C4 levels might protect the turtles from the threat of heat waves. The influence of heat waves on the turtle might be temporary, as all the significant changes had returned to baseline after a 1-week recovery period. Taken together, these results suggest that M. mutica may tolerate heat waves under current climate scenarios. It should be noted that turtles used in current study are from a captive population in turtle farm, and further studies are needed to investigate whether wild populations would display similar responses to heat wave with the captive populations. Furthermore, future research should examine the physiological and biochemical responses to more frequent, extended, or intense heat waves, and investigate the long-lasting effects of heat waves on fitness-related traits (e.g., reproductive output and survival) in more ectothermic animals (Adamo and Lovett, 2011; Bauerfeind and Fischer, 2014; Stahlschmidt et al., 2017).
The raw data supporting the conclusions of this article will be made available by the authors, without undue reservation.
The animal study was reviewed and approved by Animal Ethical and Welfare Committee of Wenzhou University (Approval No. WZU-049).
SL and YZ designed the experiment, analyzed the data, and wrote the manuscript with comments from LX. SL, JL, WC, and ZX carried out the experiment. All authors contributed to the article and approved the submitted version.
This research was supported by grants from the National Natural Science Foundation of China (31801977 and 31971419) and Young Elite Scientists Sponsorship Program by CAST and ISZS for SL and Zhejiang Provincial Natural Science Foundation of China (LY16C030001).
The authors declare that the research was conducted in the absence of any commercial or financial relationships that could be construed as a potential conflict of interest.
We would like to thank Liuyang Jiang, Xiaoyu Hu, Xiaoyue Qu, and Jinghua Zhu for their assistance in turtle husbandry and data collection during the experiment.
The Supplementary Material for this article can be found online at: https://www.frontiersin.org/articles/10.3389/fevo.2021.704105/full#supplementary-material
Adamo, S. A., and Lovett, M. M. E. (2011). Some like it hot: the effects of climate change on reproduction, immune function and disease resistance in the cricket Gryllus texensis. J. Exp. Biol. 214, 1997–2004. doi: 10.1242/jeb.056531
Angiletta, M. J. Jr. (2009). Thermal Adaptation: A Theoretical and Empiricial Synthesis. Oxford: Oxford University Press.
Ayala, A., Muñoz, M. F., and Argüelles, S. (2014). Lipid peroxidation: production, metabolism and signaling mechanisms of malondialdehyde and 4-hydroxy-2-nonenal. Oxid. Med. Cell.Longev. 2014:360438. doi: 10.1155/2014/360438
Baker, B. P., Van Wie, I., Braun, E., and Jimenez, A. G. (2020). Thermal stability vs. variability: insights in oxidative stress from a eurytolerant fish. Comp. Biochem. Physiol. A Mol. Integr. Physiol. 249:110767. doi: 10.1016/j.cbpa.2020.110767
Baker, S. J., Kessler, E. J., and Merchant, M. E. (2019). Antibacterial activities of plasma from the common (Chelydra serpentina) and alligator snapping turtle (Macrochelys temminckii). J. Exp. Zool. Part A Ecol. Integr. Physiol. 331, 85–92. doi: 10.1002/jez.2237
Bauerfeind, S. S., and Fischer, K. (2014). Simulating climate change: temperature extremes but not means diminish performance in a widespread butterfly. Popul. Ecol. 56, 239–250. doi: 10.1007/s10144-013-0409-y
Beliaeff, B., and Burgeot, T. (2002). Integrated biomarker response: a useful tool for ecological risk assessment. Environ. Toxicol. Chem. 21, 1316–1322. doi: 10.1002/etc.5620210629
Ben Ameur, W., De Lapuente, J., El Megdiche, Y., Barhoumi, B., Trabelsi, S., Camps, L., et al. (2012). Oxidative stress, genotoxicity and histopathology biomarker responses in mullet (Mugil cephalus) and sea bass (Dicentrarchus labrax) liver from Bizerte Lagoon (Tunisia). Mar. Pollut. Bull. 64, 241–251. doi: 10.1016/j.marpolbul.2011.11.026
Bentley, B. P., Haas, B. J., Tedeschi, J. N., and Berry, O. (2017). Loggerhead sea turtle embryos (Caretta caretta) regulate expression of stress response and developmental genes when exposed to a biologically realistic heat stress. Mol. Ecol. 26, 2978–2992. doi: 10.1111/mec.14087
Blazer, V. S., Walsh, H. L., Braham, R. P., and Smith, C. (2018). Necropsy-based wild fish health assessment. J. Vis. Exp 139:e57946. doi: 10.3791/57946
Bozinovic, F., Bastías, D. A., Boher, F., Clavijo-Baquet, S., Estay, S. A., and Angilletta, M. J. Jr. (2011). The mean and variance of environmental temperature interact to determine physiological tolerance and fitness. Physiol. Biochem. Zool. 84, 543–552. doi: 10.1086/662551
Breitenbach, A. T., Carter, A. W., Paitz, R. T., and Bowden, R. M. (2020). Using naturalistic incubation temperatures to demonstrate how variation in the timing and continuity of heat wave exposure influences phenotype. Proc. R. Soc. B Biol. Sci. 287:20200992. doi: 10.1098/rspb.2020.0992
Campos, D. F., Braz-Mota, S., Val, A. L., and Almeida-Val, V. M. F. (2019). Predicting thermal sensitivity of three Amazon fishes exposed to climate change scenarios. Ecol. Indic. 101, 533–540. doi: 10.1016/j.ecolind.2019.01.051
Christian, K. A., and Weavers, B. W. (1996). Thermoregulation of monitor lizards in Australia: an evaluation of methods in thermal biology. Ecol. Monogr. 66, 139–157. doi: 10.2307/2963472
Dang, W., Xu, N., Zhang, W., Gao, J., Fan, H. D., and Lu, H. L. (2018). Differential regulation of Hsp70 expression in six lizard species under normal and high environmental temperatures. Pak. J. Zool. 50, 1043–1051. doi: 10.17582/journal.pjz/2018.50.3.1043.1051
Dittmar, J., Janssen, H., Kuske, A., Kurtz, J., Scharsack, J. P., and Ardia, D. (2014). Heat and immunity: an experimental heat wave alters immune functions in three–spined sticklebacks (Gasterosteus aculeatus). J. Anim. Ecol. 83, 744–757. doi: 10.1111/1365-2656.12175
Fabrício-Neto, A., Madelaire, C. B., Gomes, F. R., and Andrade, D. V. (2019). Exposure to fluctuating temperatures leads to reduced immunity and to stress response in rattlesnakes. J. Exp. Biol. 222:jeb208645. doi: 10.1242/jeb.208645
Ferreira-Rodríguez, N., Fernández, I., Cancela, M. L., and Pardo, I. (2018). Multibiomarker response shows how native and non-native freshwater bivalves differentially cope with heat-wave events. Aquat. Conserv. Mar. Freshw. Ecosyst. 28, 934–943. doi: 10.1002/aqc.2884
Gao, Y., Wei, Y., Cao, D., Ge, Y., and Gong, S. (2021). Transcriptome analysis reveals decreased immunity under heat stress in Mauremys mutica. Aquaculture 531:735894. doi: 10.1016/j.aquaculture.2020.735894
Goessling, J. M., Ward, C., and Mendonça, M. T. (2019). Rapid thermal immune acclimation in common musk turtles (Sternotherus odoratus). J. Exp. Zool. Part A Ecol. Integr. Physiol. 331, 185–191. doi: 10.1002/jez.2252
Guzzo, M. M., Mochnacz, N. J., Durhack, T., Kissinger, B. C., Killen, S. S., and Treberg, J. R. (2019). Effects of repeated daily acute heat challenge on the growth and metabolism of a cold water stenothermal fish. J. Exp. Biol. 222:jeb198143. doi: 10.1242/jeb.198143
Han, X., Hao, X., Wang, Y., Wang, X., Teng, L., Liu, Z., et al. (2020). Experimental warming induces oxidative stress and immunosuppression in a viviparous lizard, Eremias multiocellata. J. Therm. Biol. 90:102595. doi: 10.1016/j.jtherbio.2020.102595
Harada, A. E., and Burton, R. S. (2019). Ecologically relevant temperature ramping rates enhance the protective heat shock response in an intertidal ectotherm. Physiol. Biochem. Zool. 92, 152–162. doi: 10.1086/702339
Hoegh-Guldberg, O., Jacob, D., Taylor, M., Guillén Bolaños, T., Bindi, M., Brown, S., et al. (2019). The human imperative of stabilizing global climate change at 1.5°C. Science 365:eaaw6974. doi: 10.1126/science.aaw6974
IPCC (2014). Climate Change 2014: Synthesis Report. Contribution of Working Groups I, II and III to the Fifth Assessment Report of the Intergovernmental Panel on Climate Change. Geneva: IPCC.
Leicht, K., Jokela, J., and Seppälä, O. (2013). An experimental heat wave changes immune defense and life history traits in a freshwater snail. Ecol. Evol. 3, 4861–4871. doi: 10.1002/ece3.874
Leicht, K., Jokela, J., and Seppälä, O. (2019). Inbreeding does not alter the response to an experimental heat wave in a freshwater snail. PloS One 14:e0220669. doi: 10.1371/journal.pone.0220669
Leung, J. Y. S., Russell, B. D., and Connell, S. D. (2019). Adaptive responses of marine gastropods to heatwaves. One Earth 1, 374–381. doi: 10.1016/j.oneear.2019.10.025
Li, X. L., Kang, Y., Zhang, X. Y., Zhu, B. L., and Fang, W. H. (2012). Identification of a heat shock cognate protein 70 gene in Chinese soft-shell turtle (Pelodiscus sinensis) and its expression profiles under thermal stress. J. Zhejiang Univ.Sci. B 13, 465–477. doi: 10.1631/jzus.B1100309
Livak, K. J., and Schmittgen, T. D. (2001). Analysis of relative gene expression data using real-time quantitative PCR and the 2–ΔΔ CT method. Methods 25, 402–408. doi: 10.1006/meth.2001.1262
Lu, H. L., Hu, Y. C., Li, S. R., Dang, W., and Zhang, Y. P. (2020). Acclimatory responses of thermal physiological performances in hatchling yellow pond turtles (Mauremys mutica). Anim. Biol. 70, 55–65. doi: 10.1163/15707563-20191106
Madeira, D., Narciso, L., Cabral, H. N., Vinagre, C., and Diniz, M. S. (2012). HSP70 production patterns in coastal and estuarine organisms facing increasing temperatures. J. Sea Res. 73, 137–147. doi: 10.1016/j.seares.2012.07.003
Madeira, D., Vinagre, C., and Diniz, M. S. (2016). Are fish in hot water? Effects of warming on oxidative stress metabolism in the commercial species Sparus aurata. Ecol. Indic. 63, 324–331. doi: 10.1016/j.ecolind.2015.12.008
Marigómez, I., Garmendia, L., Soto, M., Orbea, A., Izagirre, U., and Cajaraville, M. P. (2013). Marine ecosystem health status assessment through integrative biomarker indices: a comparative study after the Prestige oil spill “Mussel Watch”. Ecotoxicology 22, 486–505. doi: 10.1007/s10646-013-1042-4
Marinho, H. S., Antunes, F., and Pinto, R. E. (1997). Role of glutathione peroxidase and phospholipid hydroperoxide glutathione peroxidase in the reduction of lysophospholipid hydroperoxides. Free Radic. Biol. Med. 22, 871–883. doi: 10.1016/S0891-5849(96)00468-6
Marshall, D. J., Dong, Y. W., Mcquaid, C. D., and Williams, G. A. (2011). Thermal adaptation in the intertidal snail Echinolittorina malaccana contradicts current theory by revealing the crucial roles of resting metabolism. J. Exp. Biol. 214, 3649–3657. doi: 10.1242/jeb.059899
McCay, P. B., Gibson, D. D., Kuo-Lan, F., and Hornbrook, K. R. (1976). Effect of glutathione peroxidase activity on lipid peroxidation in biological membranes. Biochim. Biophys. Acta-Lipids Lipid Metab. 431, 459–468. doi: 10.1016/0005-2760(76)90212-5
Moore, M. E., Hill, C. A., and Kingsolver, J. G. (2021). Differing thermal sensitivities in a host-parasitoid interaction: high, fluctuating developmental temperatures produce dead wasps and giant caterpillars. Funct. Ecol. 35, 675–685. doi: 10.1111/1365-2435.13748
Munro, D., and Blier, P. U. (2012). The extreme longevity of Arctica islandica is associated with increased peroxidation resistance in mitochondrial membranes. Aging Cell 11, 845–855. doi: 10.1111/j.1474-9726.2012.00847.x
Narum, S. R., Campbell, N. R., Meyer, K. A., Miller, M. R., and Hardy, R. W. (2013). Thermal adaptation and acclimation of ectotherms from differing aquatic climates. Mol. Ecol. 22, 3090–3097. doi: 10.1111/mec.12240
Pansch, C., Scotti, M., Barboza, F. R., Al-Janabi, B., Brakel, J., Briski, E., et al. (2018). Heat waves and their significance for a temperate benthic community: a near-natural experimental approach. Glob. Change Biol. 24, 4357–4367. doi: 10.1111/gcb.14282
Parrish, C. C. (2013). Lipids in marine ecosystems. ISRN Oceanogr. 2013:604045. doi: 10.5402/2013/604045
Refsnider, J. M., Vazquez, T. K., Clifton, I. T., Jayawardena, D. M., and Heckathorn, S. A. (2021). Cellular and whole-organism effects of prolonged versus acute heat stress in a montane, desert lizard. J. Exp. Zool. Part A Ecol. Integr. Physiol. 335, 126–135. doi: 10.1002/jez.2426
Sinervo, B., Mendez-De-La-Cruz, F., Miles, D. B., Heulin, B., Bastiaans, E., Cruz, M. V. S., et al. (2010). Erosion of lizard diversity by climate change and altered thermal niches. Science 328, 894–899. doi: 10.1126/science.1184695
Stahlschmidt, Z. R., French, S. S., Ahn, A., Webb, A., and Butler, M. W. (2017). A simulated heat wave has diverse effects on immune function and oxidative physiology in the corn snake (Pantherophis guttatus). Physiol. Biochem. Zool. 90, 434–444. doi: 10.1086/691315
Tedeschi, J. N., Kennington, W. J., Berry, O., Whiting, S., Meekan, M., and Mitchell, N. J. (2015). Increased expression of Hsp70 and Hsp90 mRNA as biomarkers of thermal stress in loggerhead turtle embryos (Caretta Caretta). J. Therm. Biol. 47, 42–50. doi: 10.1016/j.jtherbio.2014.11.006
Thompson, R. M., Beardall, J., Beringer, J., Grace, M., and Sardina, P. (2013). Means and extremes: building variability into community-level climate change experiments. Ecol. Lett. 16, 799–806. doi: 10.1111/ele.12095
Vasseur, D. A., Delong, J. P., Gilbert, B., Greig, H. S., Harley, C. D. G., Mccann, K. S., et al. (2014). Increased temperature variation poses a greater risk to species than climate warming. Proc. R. Soc. B Biol. Sci. 281:20132612. doi: 10.1098/rspb.2013.2612
Vianna, B. D. S., Miyai, C. A., Augusto, A., and Costa, T. M. (2020). Effects of temperature increase on the physiology and behavior of fiddler crabs. Physiol. Behav. 215:112765. doi: 10.1016/j.physbeh.2019.112765
Wei, Y., Gao, Y., Cao, D., Ge, Y., Shi, H., and Gong, S. (2020). Effects of acute temperature stress on mRNA expression of transferrin in the yellow pond turtle Mauremys mutica. Asian Herpetol. Res. 11, 124–131. doi: 10.16373/j.cnki.ahr.200006
Yao, C. L., and Somero, G. N. (2013). Thermal stress and cellular signaling processes in hemocytes of native (Mytilus californianus) and invasive (M. galloprovincialis) mussels: cell cycle regulation and DNA repair. Comp. Biochem. Physiol. A Mol. Integr. Physiol. 165, 159–168. doi: 10.1016/j.cbpa.2013.02.024
Zhang, Q., Han, X., Hao, X., Ma, L., Li, S., Wang, Y., et al. (2018). A simulated heat wave shortens the telomere length and lifespan of a desert lizard. J. Therm. Biol. 72, 94–100. doi: 10.1016/j.jtherbio.2018.01.004
Zhang, W., Chen, B., Niu, C., Yuan, L., Jia, H., and Storey, K. B. (2019). Response of the Chinese soft-shelled turtle to acute heat stress: insights from the systematic antioxidant defense. Front. Physiol. 10:710. doi: 10.3389/fphys.2019.00710
Zhao, L., and Jones, W. A. (2012). Expression of heat shock protein genes in insect stress responses. ISJ Invertebr. Surviv. J. 9, 93–101. doi: 10.1155/2012/484919
Zimmerman, L. M., Carter, A. W., Bowden, R. M., and Vogel, L. A. (2017). Immunocompetence in a long-lived ectothermic vertebrate is temperature dependent but shows no decline in older adults. Funct. Ecol. 31, 1383–1389. doi: 10.1111/1365-2435.12867
Keywords: climate warming, heat wave, turtle, oxidative stress, immune function, heat shock proteins
Citation: Li S, Li J, Chen W, Xu Z, Xie L and Zhang Y (2021) Effects of Simulated Heat Wave on Oxidative Physiology and Immunity in Asian Yellow Pond Turtle (Mauremys mutica). Front. Ecol. Evol. 9:704105. doi: 10.3389/fevo.2021.704105
Received: 01 May 2021; Accepted: 24 May 2021;
Published: 15 June 2021.
Edited by:
Lin Zhang, Hubei University of Chinese Medicine, ChinaReviewed by:
Wenyi Zhang, Nanjing Normal University, ChinaCopyright © 2021 Li, Li, Chen, Xu, Xie and Zhang. This is an open-access article distributed under the terms of the Creative Commons Attribution License (CC BY). The use, distribution or reproduction in other forums is permitted, provided the original author(s) and the copyright owner(s) are credited and that the original publication in this journal is cited, in accordance with accepted academic practice. No use, distribution or reproduction is permitted which does not comply with these terms.
*Correspondence: Yongpu Zhang, emhhbmd5cEB3enUuZWR1LmNu
Disclaimer: All claims expressed in this article are solely those of the authors and do not necessarily represent those of their affiliated organizations, or those of the publisher, the editors and the reviewers. Any product that may be evaluated in this article or claim that may be made by its manufacturer is not guaranteed or endorsed by the publisher.
Research integrity at Frontiers
Learn more about the work of our research integrity team to safeguard the quality of each article we publish.