- 1Quantitative and Systems Biology Graduate Group, School of Natural Sciences, University of California, Merced, Merced, CA, United States
- 2Center for Engaged Teaching and Learning, Teaching Commons, University of California, Merced, Merced, CA, United States
- 3Environmental Systems Graduate Group, School of Engineering, University of California, Merced, Merced, CA, United States
- 4Department of Life and Environmental Sciences, University of California, Merced, Merced, CA, United States
Earth is changing rapidly and so are many plant species’ ranges. Here, we synthesize eco-evolutionary patterns found in plant range studies and how knowledge of species ranges can inform our understanding of species conservation in the face of global change. We discuss whether general biogeographic “rules” are reliable and how they can be used to develop adaptive conservation strategies of native plant species across their ranges. Rules considered include (1) factors that set species range limits and promote range shifts; (2) the impact of biotic interactions on species range limits; (3) patterns of abundance and adaptive properties across species ranges; (4) patterns of gene flow and their implications for genetic rescue, and (5) the relationship between range size and conservation risk. We conclude by summarizing and evaluating potential species range rules to inform future conservation and management decisions. We also outline areas of research to better understand the adaptive capacity of plants under environmental change and the properties that govern species ranges. We advise conservationists to extend their work to specifically consider peripheral and novel populations, with a particular emphasis on small ranges. Finally, we call for a global effort to identify, synthesize, and analyze prevailing patterns or rules in ecology to help speed conservation efforts.
Sustained by previous discoveries, we can go forth into the future, and by foreseeing the consequences of phenomena, we can understand once and for all the laws to which nature subjected itself.
– Alexander von Humboldt and Aimé Bonpland (1807)
Introduction
A core component of ecology is to recognize and understand patterns in nature (MacArthur, 1972). Since the early studies of biogeography (e.g., von Humboldt and Bonpland, 1807), scientists have put forward a variety of ecological hypotheses, some of which have become entrenched or taken for granted enough to be considered paradigms or “rules,” and these efforts continue today (Sagarin and Gaines, 2002; Connallon and Sgrò, 2018; Liu et al., 2020). For example, there is recent interest in establishing “rules of life” to understand and predict how properties of living systems (i.e., environment, phenotype, evolution, etc.) interact (National Science Foundation, 2016; Midlands Integrative Biosciences Training Partnership, 2019) and how these rules can inform conservation (Kindsvater et al., 2016). A central issue is predicting how species will respond to climate change. Accelerated biodiversity loss (Urban, 2015) and disruptions to global patterns of community assembly (Trisos et al., 2020) are already underway. Thus, we urgently need to understand how species respond to rapid change at the geographic scale, and whether there are broad geographic patterns or phenomena that may lead to enhanced conservation and ecosystem management. Although many species are likely to become endangered or go extinct, targeted conservation measures can save many species from this fate. Every species has a story to tell, and its geographic range (e.g., range size, gene flow patterns, etc.) can provide important insights as to how it can be conserved, managed, and restored.
Whether or not ecological or evolutionary patterns can serve as reliable rules is debatable since few strict laws exist in ecology, but many general ones may (Lawton, 1999; Temperton et al., 2004; Dickey et al., 2021). Broadly, we consider rules to be effective, predictive hypotheses with strong empirical support. Like any good rule, they will be broken due to the idiosyncrasies among species and the vast variation life represents. Nevertheless, knowing whether species ranges provide generalizable ecological rules, such as in patterns of abundance, distribution, and interactions, or evolutionary rules, such as in patterns of selection, drift, and gene flow, would allow more informed management decisions at large geographic scales (Pelletier et al., 2018).
In this article, we evaluate various paradigms as potential “rules” within five eco-evolutionary realms of species ranges—some long-held—that are important for species conservation and biogeography. We highlight new and emerging findings throughout, including needed areas of future research, and we include conservation recommendations within each section. Although this topic applies to all forms of life, we focus our examples and conservation prescriptions primarily on plants. As primary producers, all ecosystems depend on their plant communities to influence a suite of essential ecological processes, including resource use efficiency, biomass production, and nutrient recycling (Cardinale et al., 2011). Therefore, managing for healthy, resilient plant communities is of primary concern for ecosystem conservation and restoration. We end the paper by summarizing our general findings for each rule and its associated conservation implications (Table 1).
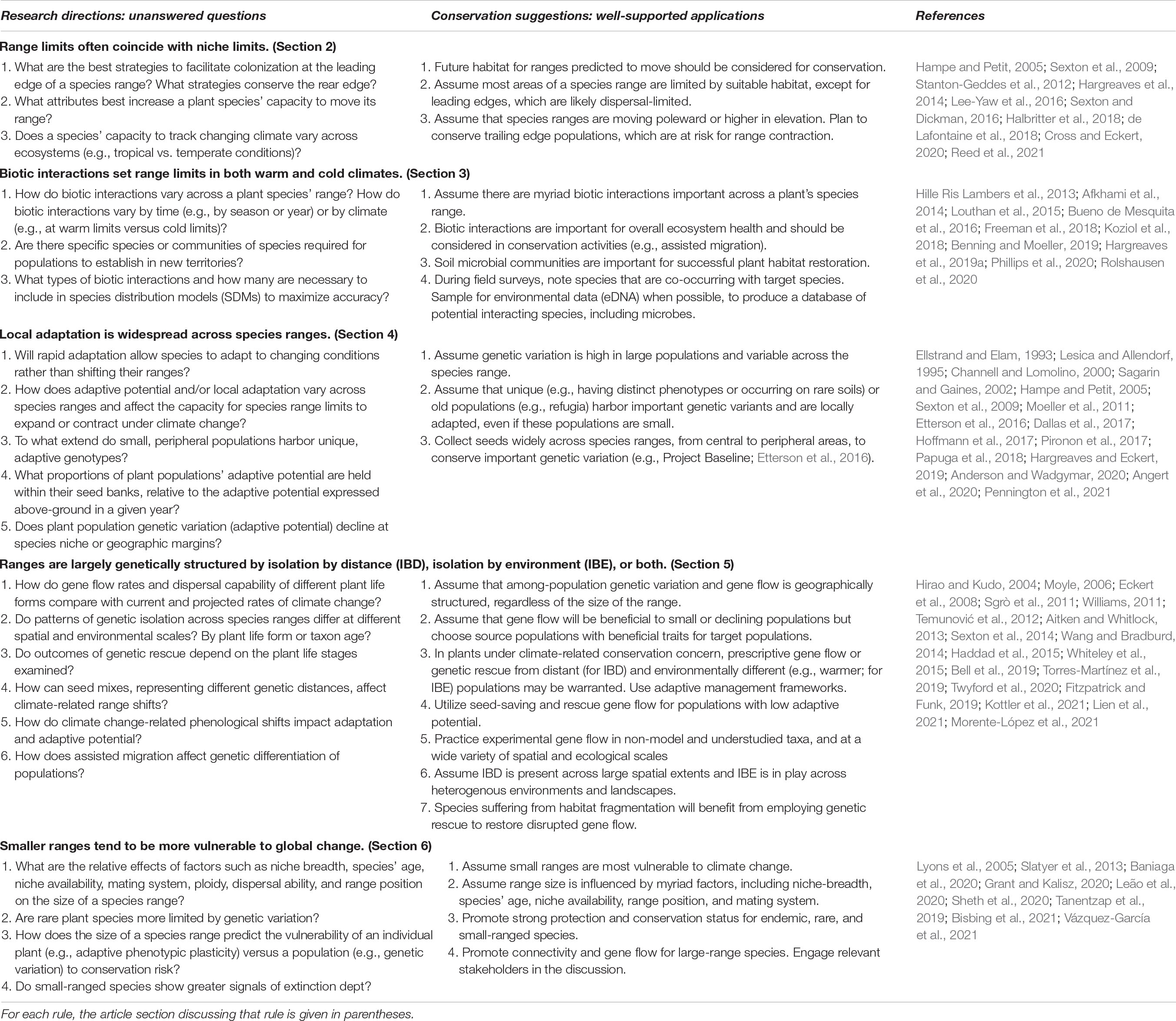
Table 1. Rules of plant species ranges, their open research questions, conservation applications, and supporting literature.
How are Species Ranges Defined and Tracked?
The Essence of Plant Species Ranges
Plants respond to stress and rapid environmental change through several mechanisms. As sessile organisms in terrestrial ecosystems, plants greatly employ a local scale of adaptation (Alpert and Simms, 2002; Palacio-López et al., 2015). However, there is also a large geographic scale at which plant populations vary in their attributes, environments, modes of communication among populations (i.e., gene flow), and interactions with abiotic and biotic factors (Darwin, 1859; Griggs, 1914; MacArthur, 1972; Brown, 1984). This scale is commonly known as the species range (Gaston, 2003), which is assumed to be a projection of niche availability in geographic space (Sexton et al., 2009). In this vein, a classic paradigm that has garnered enough support to be considered a rule is that range limits are niche limits, beyond which populations tend to decline along with their available niche attributes. Nevertheless, some edges are limited more by dispersal. Recent and current work has sought to identify the relative importance of niche vs. dispersal limitation for focal species, mainly through the use of transplant experiments and species distribution models (SDMs) (Hargreaves et al., 2014; Lee-Yaw et al., 2016; Connallon and Sgrò, 2018; Bayly and Angert, 2019; Ackerly et al., 2020). From these studies, we can form generalizations about what types of edges are most likely to fall into each of these two categories, or both. Transplant and modeling approaches have strengths and weaknesses (reviewed in Araújo and Peterson, 2012; Ehrlén and Morris, 2015; Greiser et al., 2020). Moreover, with respect to species ranges, climate edges may not always correlate with geographic edges due to climate heterogeneity and geographic scale effects on climate properties (Oldfather et al., 2020). Thus, range limits are perceptible and approximate niche limits at particular scales, but also shift as climates change (Sexton et al., 2009; Halbritter et al., 2018).
Plant Species Ranges on the Move in a Changing Climate
In response to global warming, plant species ranges are shifting, contracting, and expanding into new territories and into refugia characterized by milder climate conditions and greater water availability (Hampe and Petit, 2005; Lenoir et al., 2008(Feeley, 2012; de Lafontaine et al., 2018; Freeman et al., 2018; Meng et al., 2019; Miller et al., 2020; Mamantov et al., 2021; Reed et al., 2021; Zu et al., 2021). In most cases, plant species range limits are moving quickly. The general pattern for plant species ranges is to move to higher latitudes, elevations, and cooler environments with higher precipitation to escape rising temperatures and drought, often resulting in range contraction (Kelly and Goulden, 2008). This pattern of uphill range shifts is documented in many ecosystems, including deserts, such as the Newberry mountains of the Mojave Desert (NV, United States) (Guida et al., 2014), the tropical Andes mountains (Peru, South America) (Feeley et al., 2011; Feeley, 2012), the subtropical mountains of Mt. Gongga (Sichuan, China) (Zu et al., 2021), and other montane systems worldwide (Mamantov et al., 2021). However, tracking of climate change in montane species may be more pronounced in the tropics, where seasonality is reduced (Ghalambor et al., 2006), than in temperate zones (Freeman et al., 2018). Rapid plant community change is also a symptom of climate change. In coastal ecosystems, as sea levels rise, the globally distributed mangrove, Avicennia sp. (Acanthaceae), is replacing existing habitats (e.g., salt marsh ecosystems) as their distributions expand (Saintilan et al., 2014). Nevertheless, there are plant species with static ranges that are so far resilient to climate change, such as with some heat-adapted desert shrubs (Tielbörger and Salguero-Gómez, 2014).
Conclusions, Future Directions, and Conservation Applications
A general rule is that species range limits often coincide with niche limits and as a consequence, are shifting in response to climate change (Reed et al., 2021). Dispersal limitation can cause discordance between shifting niche limits and range limits (Stanton-Geddes et al., 2012; Lee-Yaw et al., 2016; Sexton and Dickman, 2016; Cross and Eckert, 2020). Range limits continue to offer a compelling spatial context for conservation research (Serra-Diaz and Franklin, 2019). Research on how to best facilitate new colonizations at the leading edges and conserve rear edges of species ranges is needed (Kottler et al., 2021; Table 1). Through the use of transplant experiments and robust SDMs, key drivers and patterns across species ranges can be uncovered (Franklin et al., 2017). Studies that identify and distinguish between the capacity for ranges to move, versus rapid adaptation that stabilizes range limits, will be useful in predicting future range shifts or lack of shifts, respectively. Further research into whether a species’ capacity to track climate varies across ecosystems (e.g., tropical vs. temperate conditions), or why some plant ranges (e.g., annuals, perennials, trees, etc.) are better than others at tracking conditions, is greatly needed.
Conservationists and managers should assume most areas outside of a species range are limited by suitable habitat, except for the leading edges, which are likely to be more limited by dispersal. The trailing edges of species ranges are, in many cases, at risk for contraction and should be another priority for conservation by saving seeds from warm-adapted regions to facilitate and enable genetic rescue (see section 5.5). Regions that represent future habitat for ranges that are moving should also be conserved and protected. In general, more research and protection are needed in tropical systems where biodiversity loss will be greatest and in the Southern Hemisphere where ecosystems are understudied.
How Do Biotic Interactions Influence Species Ranges?
Evidence for the Importance of Biological Interactions on Species Ranges
Biotic interactions are an integral component of a species’ realized niche (Peay, 2016; Phillips et al., 2020) and are essential to consider in range limit contexts (Hargreaves et al., 2014; Freeman et al., 2018) and species distributions (Hille Ris Lambers et al., 2013). For millennia, natural climate change events have altered the dynamic of these important interactions (Blois et al., 2013; Hamann et al., 2021), affecting their co-evolution (Parmesan, 2006), and influencing their role in facilitating range shifts (Hille Ris Lambers et al., 2013). Darwin (1859) predicted that negative biotic interactions (i.e., competition, predation, herbivory, and parasitism) establish range limits at warm edges where species diversity is higher. Since then, this paradigm has received extensive support (MacArthur, 1972; Brown et al., 1996; Gaston, 2003; Normand et al., 2009; Paquette and Hargreaves, 2021) and is the leading hypothesis for how biotic interactions influence species ranges (Barton, 1993; Bullock et al., 2000; Scheidel and Bruelheide, 2001; Holt and Barfield, 2009; Louthan et al., 2015).
Over time, Darwin’s theory has been expanded to acknowledge that both positive (i.e., pollination, facilitation, and mutualism) and negative interactions influence plant species range limits at both warm and cold climate limits (Afkhami et al., 2014; Louthan et al., 2015; Benning et al., 2019; Benning and Moeller, 2021a). For example, in Clarkia xantiana ssp. xantiana (Onagraceae) fitness decreased beyond its cold limit due to a lack of positive interactions (e.g., pollinators) and the presence of negative interactions (e.g., herbivores) (Benning and Moeller, 2019). When pollen was supplemented and herbivores were removed, fitness beyond the range tripled, demonstrating the importance of these positive and negative interactions for range shifts and expansions. In a second example, Ettinger and Hille Ris Lambers (2017) found that competition between neighboring trees limited performance within ranges, whereas facilitative interactions between adults and juveniles demonstrated the potential to accelerate upward range expansion. Most studies focus on one or a limited set of interactions, often due to logistical constraints, and rarely have multiple interactions been studied simultaneously in range-limit contexts. Given their clear ecological importance, identifying and including an array of biotic interactions will increase accuracy when predicting species range shifts under climate change (Van der Putten et al., 2010).
Both Positive and Negative Interactions Matter in Setting Warm and Cold Range Limits
From the above, the prevailing paradigm is that negative interactions, particularly competition, drive warm-edge range limits (Schemske et al., 2009; Sexton et al., 2009; Louthan et al., 2015; Paquette and Hargreaves, 2021). Although this is often true, there are examples in which other negative interactions affect plant distributions. For example, seed predation is known to influence cold-edge expansion (Brown and Vellend, 2014; Hargreaves et al., 2019b) and herbivory-induced delays in phenology and subsequent reductions in fitness (e.g., biomass or height) limit the species range of susceptible plants (Louda, 1982; Lau et al., 2008; Benning et al., 2019). In California serpentine environments, Lau et al. (2008) found that increased herbivory was one factor that reduced survival and persistence in the native herb Collinsia sparsiflora (Plantaginaceae), restricting the species, realized niche to serpentine habitats (Lau et al., 2008). Depending on which species is under consideration, negative biotic interactions can limit or facilitate expansion of a plant species range; in some cases, herbivory of one plant is beneficial to another plant. For instance, in tundra experiencing climate warming, herbivory of competing species protected native plant populations (Eskelinen et al., 2017), allowing range expansion of the tundra community (Kaarlejärvi et al., 2017).
More recently, positive interactions have emerged as relevant and important for consideration in climate-range research. Mutualisms are abundant in stressful conditions (Callaway et al., 2002), affect plant fitness (Lau and Lennon, 2012), mitigate climate stress on species distributions (Bulleri et al., 2016), and influence local adaptation (Pickles et al., 2015). Facultative mutualisms can facilitate expansion of species ranges into stressful habitats (Afkhami et al., 2014; Millar and Bennett, 2016; Benning and Moeller, 2021b) in addition to novel environments (Crotty and Bertness, 2015; Bueno de Mesquita et al., 2020). A key example of a mutualism that expands the plant realized niche is pollination (Phillips et al., 2020). In general, pollinator species distributions are strongly linked to their visiting plant geographic ranges (Duffy and Johnson, 2017). In a four-year study of Clarkia xantiana ssp. xantiana (Onagraceae), pollinator availability declined with distance from the center of the plant range, contributing to the maintenance of the species range limit (Moeller et al., 2012). Climate change continues to reduce the quantity and quality of pollination services globally (Burkle et al., 2013; Gérard et al., 2020), leading to contractions and reductions of plant species ranges (Chalcoff et al., 2012; Moeller et al., 2012).
Microbes are also important plant mutualists. As such, microbes are an integral part of a plant’s habitat (Peay, 2016) and their absence contributes to defining suitable limits as well as hindering expansion (Benning and Moeller, 2019). In the endangered Hypericum cumulicola (Hypericaceae), soil microbes boosted population growth and persistence and allowed the plant to expand into previously uninhabitable environments (David et al., 2019). Similarly, soil microbes in the Rocky Mountains interacted with alpine bunchgrass, Deschampsia cespitosa (Poaceae), to allow establishment and growth in new, unvegetated areas beyond the range, suggesting the significance of microbes in climate-induced range expansions (Bueno de Mesquita et al., 2020). In the absence of mutualistic soil microbes beyond the species range edge, host plants experienced reduced fitness, limiting this expansion capacity (Benning and Moeller, 2021a). Climate change can alter plant-microbe interactions in a variety of ways, including changing microbial species ranges, community composition, functionality, fitness, and occurrence of host plant species (Rudgers et al., 2020). Plant genotype and root exudates affect microbial community composition (Bulgarelli et al., 2012), allowing plants to counter the impacts of climate-induced stress (Liu et al., 2015; Rodriguez and Durán, 2020). In turn, the spatial variation in microbial communities affects patterns of plant local adaptation (Pickles et al., 2015) and influences the location of a plant’s species range (Van der Putten et al., 2010). Understanding microbial community structure across species ranges will provide a better view of mutualist-mediated niche dynamics, especially as it relates to expansion in response to environmental pressures from climate (Rolshausen et al., 2020).
Conclusions, Future Directions, and Conservation Applications
From the above evidence, it is still difficult to say whether biotic interactions are more limiting at warm versus cold limits, but a general rule that can be gleaned is that biotic interactions often set range limits in both warm and cold climate extremes. More studies are needed to identify and understand important biotic interactions across plant species ranges (Wisz et al., 2013). Mutualisms are more important than classically appreciated for range limits in a changing climate, especially when introducing a plant to a new habitat or predicting future range shifts (Hille Ris Lambers et al., 2013; Freeman et al., 2018; Benning and Moeller, 2021a). Range limit research should aim at understanding interactions through species co-occurrence data (e.g., presence/absence data, field observations, etc.) to effectively model distributions and predict range shifts under climate change (Araújo and Luoto, 2007; Kissling et al., 2012; Bueno de Mesquita et al., 2016; Miele et al., 2021). To help track ecosystem biodiversity and change in biota over time, environmental samples from sediment, soil, air, or surfaces can be analyzed using metabarcoding and metagenomics and characterized through reference databases to more completely identify interacting taxa and communities (Moore et al., 2021). This process employs environmental DNA (eDNA) techniques and is known as ecological forensics, and this field has broad implications for conservation, especially for identifying associated soil microbial communities that confer ecosystem resistance and resilience to climate disruption (Koziol et al., 2018; Rudgers et al., 2020). Overall, species interactions are largely under-researched, especially across large biogeographic scales or in remote or unique habitats (Table 1).
Restoration efforts should consider the presence and significance of biotic interactions, including soil microbes. Assisted migration efforts are an important conservation strategy and can help plants occupy novel, habitable regions (Hällfors et al., 2017), but traditionally ignore biotic interactions and how they might influence transplanted populations (Bucharova, 2017). When protecting predicted range regions for transplantation or seeding, it is important to assess whether key biotic interactions can persist in these new territories, with special attention given to native soil communities. As we gain more perspective into positive and negative biotic interactions of conservation-targeted species, we can incorporate these occurrence data of interacting species into models (Giannini et al., 2013). For example, a recent model developed by Miele et al. (2021) combines species interaction data, environmental data, and species occurrences to disentangle the effects of abiotic and biotic interactions on species distributions (see ELGRIN model, Miele et al., 2021).
Do Different Regions of Species Ranges Hold Predictable Adaptive or Resilience Properties?
The Abundant Center Hypothesis Is Not a General Rule
Whether specific regions of species ranges (e.g., peripheral, central, warmer, older, etc.) differ in ecological and evolutionary properties is an essential question for guiding management of plant populations under global change. As discussed earlier, range limits are generally niche limits when ranges are in climate equilibrium, and the dynamics between and within different regions of plant species’ ranges have evoked several hypotheses to consider. For example, Lesica and Allendorf (1995) proposed that peripheral regions of species ranges should harbor genetically unique and isolated genotypes that are useful for conservation purposes. In agreement with this hypothesis, peripheral populations in shrinking species ranges are just as likely as central populations to serve as refugia (Channell and Lomolino, 2000).
A handful of paradigms have developed regarding the center of the species range. One classic paradigm is the abundant center hypothesis (ACH), which posits that populations are most abundant at the center of their range and will decrease in both size and density towards range margins (Brown, 1984); nevertheless, this does not appear to hold as a general rule (Sagarin and Gaines, 2002; Sexton et al., 2009; Dallas et al., 2017; Pironon et al., 2017). Another paradigm is the niche-distance-abundance (NDA) hypothesis, which proposes that species will be most abundant at the center of their niche (Dallas and Hastings, 2018; Osorio-Olvera et al., 2019); however, this has also received mixed support (Dallas et al., 2017; Weber et al., 2017; Dallas and Hastings, 2018; Jiménez-Valverde et al., 2021). A recent study of the endemic Iberian Peninsula snapdragon, Antirrhinum lopesianum (Plantaginaceae), found a negative relationship with abundance and distance from the species’ niche center (Hernández-Lambraño et al., 2020). Similarly, an analysis of European vascular plants found evidence of a negative niche distance-abundance relationship, but the relationship was weak and highly variable (Sporbert et al., 2020). There are many examples where range position, niche position, and abundance do not correlate (Sagarin and Gaines, 2002; Eckert et al., 2008; Sexton et al., 2009, 2016; Dallas et al., 2017; Pironon et al., 2017; Kennedy et al., 2020), and in some cases, plant population density actually increases towards range limits (e.g., Sexton et al., 2016). A growing body of research suggests that the history of a population is more indicative of its patterns of abundance and genetic variation than contemporary measures of the population’s size, its range position, or the species’ range size (Abeli et al., 2014; Koski et al., 2019; Cruz-Nicolás et al., 2020).
Genetic Variation Determines Adaptive Potential of Populations
To conserve threatened species, it is useful to identify populations that are most vulnerable and those that have the potential to help other populations of the species adapt to changing climate conditions. Adaptive potential is determined by genetic variation, which allows a population’s traits to change in response to changes in the environment (Pennington et al., 2021). Quantitative genetic variation (QGV) is a measure of genetically based phenotypic variation and, ultimately, the evolutionary adaptive potential of populations (Rice and Emery, 2003; Conner and Hartl, 2004). Populations with larger effective population sizes tend to be higher in QGV (Hoffmann et al., 2017), and so are important for conservation. Nevertheless, small populations, especially those in adverse conditions (e.g., stressful soils), may harbor unique variation that is also important for conservation (Ellstrand and Elam, 1993). Furthermore, older populations and populations that may have been glacial refugia may also retain important variation (Hampe and Petit, 2005), but may not have large numbers of individuals in their populations. Evidence suggests that larger, older, and unique populations are more likely to be important sources of QGV, and these may occur anywhere within species ranges, centrally or peripherally. Given that the ACH is not supported as a general rule, QGV, and therefore adaptability, may not be highest in central regions of species ranges. Overall, more research is needed in this area, including replicated sampling and comparison of peripheral and central regions within species ranges (Pennington et al., 2021).
Local Adaptation Follows Adaptive Potential
Local adaptation occurs throughout species ranges and is often driven by climate (Anderson and Song, 2020; Anderson and Wadgymar, 2020; Bontrager et al., 2021). Local adaptation has been observed in myriad species and results in differential responses to climate change across species ranges (Hargreaves et al., 2014; Harrison et al., 2019; Peterson et al., 2019; Torres-Martínez et al., 2019; Anderson and Wadgymar, 2020; Patsiou et al., 2020). Peripheral populations are critical when considering climate-driven fitness variation and conservation (Lesica and Allendorf, 1995; Channell and Lomolino, 2000; Macdonald et al., 2017; Papuga et al., 2018) because they are often locally adapted to more extreme habitats and are home to phenotypes that are not expressed in other areas of the range (Moeller et al., 2011; Papuga et al., 2018; Hargreaves and Eckert, 2019; Angert et al., 2020; Morente-López et al., 2021). For example, in a reciprocal transplant of the ‘ōhi‘a lehua tree (Metrosideros polymorpha) from tropical Hawaii, seedlings that are locally adapted to historically wet regions germinated less than seedlings adapted to drought in contemporary dry regions (Barton et al., 2020).
Further such studies are needed, including more studies that reveal patterns of population genetic variation and size across plant species ranges (Pennington et al., 2021). As climate change alters local adaptation (Anderson and Wadgymar, 2020), patterns of adaptive variation and abundance may change. Besides directly measuring QGV across species ranges, other methods to quantify differences in adaptive potential such as artificial selection and resurrection studies—in which prior generations are compared to contemporary populations for their trait values—are useful for contrasting historical patterns with contemporary patterns to understand how populations are responding to climate change. Recent plant resurrection studies have captured varying phenological change in response to climate change and illustrate that some degree of rapid adaptation is possible for many plant species (Franks et al., 2018; Dickman et al., 2019; Vtipil and Sheth, 2020; Wooliver et al., 2020; Anstett et al., 2021; Kooyers et al., 2021).
Conclusions, Future Directions, and Conservation Applications
Patterns of adaptive potential and local adaptation are in need of better understanding, especially at range edges where potential expansion or contraction may occur in response to rapidly changing climate. Nevertheless, peripheral populations are understudied and, as a result, underprotected (Caissy et al., 2020). A general rule is that local adaptation is widespread across species ranges. SDMs that incorporate local adaptation, such as ΔTraitSDMs (Garzón et al., 2019), should be considered when predicting species’ range responses to climate change. Small populations in unique environments and older populations may harbor important, but underexplored, genetic variation. The abundant center-hypothesis, although supported in some species, is not a rule and, instead, a niche-abundance relationship deserves further study. Both central and peripheral populations are equally important to consider in research and conservation contexts. Deeper explorations of the relationship between niche, range, and abundance patterns across plant species ranges will provide better predictions of important populations for conservation. Overall, these questions need to be explored in more systems as these patterns vary widely by species (Angert et al., 2020; Reed et al., 2021).
To avoid losing unknown adaptive potential, plant conservationists should first assume that local adaptation is widespread, and that adaptive potential is equal across study species ranges, until shown otherwise. Populations with high adaptive potential and populations with unique genotypes are of particular interest to conservation. Small, young populations are likely to have lower adaptive potential and are, therefore, more vulnerable. Wherever possible, populations should be evaluated for their adaptive potential, especially in areas that are at risk to climate change. Small populations with high genetic variation or unique adaptations can be as important for a species’ conservation as large populations. Conservationists should collect seeds widely across species ranges, including edge populations to conserve genetic variation and adaptive potential (see Project Baseline, Etterson et al., 2016). Additionally, to our knowledge, it is an open question what proportions of plant populations’ adaptive potential are held within their seed banks, relative to the adaptive potential expressed above-ground in a given year. The proportion of genetic variation of a population contained within its soil seed bank should vary greatly by plant life form. Finally, measuring and mapping genetic variation and using approaches that estimate responses to selection such as resurrection studies are useful to assist in conservation and management of plant populations. These strategies can inform managers about which populations are most vulnerable to change, and whether certain areas of a species’ range should be prioritized for conservation.
Are There Predictable Patterns and Effects of Gene Flow on Adaptation Across Species Ranges?
Gene Flow Across Species Ranges
Gene flow is widely recognized for both its enhancement and inhibition of adaptation, and it is one of the best evolutionary tools for managing species range responses to climate change (Aitken and Whitlock, 2013; Sexton et al., 2014; Smith et al., 2014; Bontrager and Angert, 2019; Kottler et al., 2021). Thus, understanding rules of gene flow across plant species ranges is key for a conservation biologist. Prescribing gene flow is also a game of chance, of course (Bell et al., 2019), but it can be successful with good planning and strategy (Sgrò et al., 2011). Environmental, geographical (spatial), or temporal patterns of gene flow are prevalent across species ranges (Sexton et al., 2014; Peters and Weis, 2019). At species range limits, gene flow is theorized to enhance genetic variation to expand a species’ niche (Holt and Gomulkiewicz, 1997), or gene flow may potentially limit or collapse a range (Kirkpatrick and Barton, 1997). The lack of gene flow is also theorized to set range limits in marginal populations that have small population sizes and high rates of inbreeding depression (Antonovics, 1976; Hoffmann and Blows, 1994; Morente-López et al., 2021). Finally, different patterns of gene flow occur simultaneously and interact with each other to influence eco-evolutionary outcomes across species ranges (Sexton et al., 2014; Bontrager and Angert, 2019; Nadeau and Urban, 2019).
Isolation by Distance Is Prevalent in Plants
Dispersal and dispersal limitation are key features influencing plant ecology, evolution, and distributions. Selection or habitat adaptation notwithstanding, limited dispersal of both pollen or seeds can lead to decreased gene flow and increased genetic drift, resulting in increased genetic isolation with increased geographic distance across the species range, known as genetic isolation by distance (IBD) (Dobzhansky, 1937; Wright, 1943). IBD is the most prevalent pattern of gene flow observed in plants to date, likely due to their sessile nature (Moyle, 2006; Eckert et al., 2008; Orsini et al., 2013; Sexton et al., 2014; Torres-Martínez et al., 2019; Twyford et al., 2020). In this vein, high dispersal ability usually promotes high genetic variation in plants (Hamrick and Godt, 1996; Lander et al., 2021); nevertheless, this variation can affect evolutionary processes among populations differently. For example, in one plant family (Fagaceae), there are examples of little to no adaptive effects of gene flow from long-distance dispersal (Moracho et al., 2016) and large adaptive effects from short distances (Gauzere et al., 2020).
Habitat fragmentation (e.g., agriculture, urbanization, or harvesting of natural resources) can disrupt gene flow among contiguous populations and erode genetic diversity by decreasing the effective population size and increasing the spatial isolation of populations. This can result in genetically depauperate populations subject to increased genetic drift, inbreeding depression and reduced gene flow (Young et al., 1996; Couvet, 2002; Aguilar et al., 2019). IBD increases with habitat fragmentation. Where IBD is detected, even at small spatial scales (Gauzere et al., 2020), genetic variation necessary to respond to rapid environmental change may be limiting, requiring prescriptive or rescue gene flow (see section 5.5) from distant sources (Willi et al., 2007).
Isolation by Environment Is Also Common in Plants
The movement of alleles between populations from similar habitats or environments creates a pattern known as isolation by environment (IBE) or “ecological isolation” (Dobzhansky, 1937; Wang, 2013). IBE scenarios are driven by environmental heterogeneity across species ranges and are caused by natural selection or non-random mating among similar environments (Hirao and Kudo, 2004; Temunović et al., 2012); IBE and IBD are often correlated (Wang and Bradburd, 2014; Shafer and Wolf, 2013). IBE is the prevalent pattern of gene flow in the majority of non-plant species examined, and is nearly as prevalent as patterns of IBD in plants (Sexton et al., 2014; Wang and Bradburd, 2014; Morente-López et al., 2021). Recently, an IBE pattern was found in Asian temperate deserts across the range of the broad-leaved evergreen shrub, Ammopiptanthus mongolicus (Fabaceae). In this example, landscape heterogeneity in precipitation was associated with IBE (Jiang et al., 2019). Similar to scenarios with IBD, if plants under conservation consideration exhibit local adaptation and IBE, genetic variation necessary to respond to rapid environmental change may require prescriptive or rescue gene flow from different (i.e., warmer) environments (Sexton et al., 2014; Kottler et al., 2021).
Climate warming has, in most cases, led to an earlier shift in plant flowering phenology (Menzel et al., 2006; Wolkovich et al., 2012; Dai et al., 2014; Leinonen et al., 2020), which influences both plant distribution (Parmesan and Yohe, 2003; Chuine, 2010; Song et al., 2021) and gene flow patterns (Schuster et al., 1989; Wadgymar et al., 2015). Isolation by phenology (IBP) is a form of IBE and occurs when phenology differences (e.g., flowering time) divide populations into different mating pools (Peters and Weis, 2019). Climate warming is leading to more uniformity in phenology, reducing IBP (Franks and Weis, 2009; Chen et al., 2018; Vitasse et al., 2018). Unfortunately, genetically-based evolution of phenology may happen too slowly to rescue populations from rapid climate change (Vtipil and Sheth, 2020). Future work is needed to examine the impact of climate shifts on phenology across large geographic gradients and in assisted migration conservation efforts.
Currently, most studies find that plant genetic variation is explained by a combination of IBE and IBD (Sexton et al., 2014; Moran et al., 2017; Nadeau and Urban, 2019; Da Silva et al., 2021). For example, long distance seed dispersal prevented snowmelt-driven isolation in Salix herbacea (Salicaceae) in the Swiss Alps (Cortés et al., 2014). Future studies of gene flow patterns should combine and parse the effects of environment and distance and sample a wide range of environmental variables (biotic and abiotic) across species ranges to isolate drivers of IBE.
The Myth of Gene Swamping in the Creation of Range Limits
Maladaptive gene flow as a mechanism for stalling or degrading adaptation is known as gene swamping and has been invoked as a mechanism for creating range limits (Haldane and Ford, 1956; Kirkpatrick and Barton, 1997). This long-standing paradigm assumes that gene swamping reduces fitness and limits local adaptation at the range edge by flooding the region with genes adapted to different conditions in central populations, suppressing locally beneficial genes (Antonovics, 1976; García-Ramos and Kirkpatrick, 1997; Kirkpatrick and Barton, 1997; Kawecki, 2008; Lopez et al., 2008). Reduction in fitness from mating genetically divergent populations (i.e., outbreeding depression) has been observed in several plant species (Fenster and Galloway, 2000; Montalvo and Ellstrand, 2001; Oakley et al., 2015). For example, Montalvo and Ellstrand (2001) documented outbreeding depression as a result of crossing deerweed varieties (Lotus scoparius var. scoparius and L. s. var. brevialatus; Fabaceae) and recommended caution when crossing plants from very genetically divergent lines for restoration.
Although gene swamping can certainly stall adaptation, it does not appear to be a reliable rule for explaining range limits. A recent review found little evidence to support gene swamping in the evolution of range limits for two reasons (Kottler et al., 2021). First, gene flow is not universally asymmetrical from the center of a range to its peripheries, likely because the abundant center hypothesis is not a universal rule (see section 4), an assumption that range-wide gene swamping relies on. Second, in the few empirical cases where gene flow has been experimentally introduced to plant populations at the edge of a species range, the results are overwhelmingly positive for edge populations (Kottler et al., 2021). This is likely due to the fact that edge populations may suffer from reduced effective population sizes (drift) brought about by increased isolation and strong selection (Hoffmann and Blows, 1994; Eckert et al., 2008; Kottler et al., 2021; Pennington et al., 2021).
The Potential of Genetic Rescue in Conservation
An alternate hypothesis to gene swamping stalling adaptation is genetic rescue, where genetic variation from outside populations is beneficial to populations suffering from inbreeding depression (Tallmon et al., 2004; Hedrick et al., 2011). Gene flow can benefit depauperate populations through the introduction of environment-specific alleles that improve fitness (Sexton et al., 2011; Bontrager and Angert, 2019). When crossing monkeyflower plants (Mimulus laciniatus, Phrymaceae) between warm-limit edge populations, Sexton et al. (2011) found that plant fitness increased at the warm-limit. Similarly, Bontrager and Angert (2018) investigated gene flow effects across the Clarkia pulchella (Onagraceae) species range in the Pacific Northwest and found a fitness boost in cold-limit edge populations from central gene flow due to rescue effects of warm-adapted populations in a warm, dry climate year.
Small populations are particularly threatened by habitat fragmentation (Haddad et al., 2015) and restoring gene flow through genetic rescue is a viable option for protecting fragmented species ranges (Bell et al., 2019). Genetic rescue is an underappreciated and useful tool for conservation of endangered species (Whiteley et al., 2015). Nevertheless, although discussed often in the literature, genetic rescue is rarely used as a conservation strategy (Frankham et al., 2017; Robinson et al., 2020). The exploration and use of genetic rescue as a conservation and management tool is still in its infancy (Bell et al., 2019). Yet, this strategy shows great promise (Fitzpatrick and Funk, 2019) and should be used more often in range-wide contexts.
Conclusions, Future Directions, and Conservation Applications
Gene flow is important for adaptation across species ranges, and range limits can be positively influenced by gene flow events in plant systems. A general rule is that plant species ranges are largely genetically structured by IBD (driven by dispersal limitation and drift), IBE (driven by selection and non-random mating), or both. Because of the preponderance of some form of genetic isolation across plant species ranges, assisted gene flow is an important tool for increasing the adaptive potential of populations. IBP, as a form of IBE, is likely to be a common phenomenon in plants, however, it is still poorly understood for its ramifications under climate change. Gene swamping as a creator of range limits is not a rule, since gene flow often has beneficial effects on local adaptation in marginal populations. To better understand beneficial and harmful effects of gene flow in plant conservation contexts, more research is needed at different plant life stages, in non-model and understudied taxa, and at a wide variety of spatial and ecological scales (Table 1). Key areas of focus should include controlled cases of gene flow, measuring the effects of different types of gene flow (i.e., IBD and IBE) across ranges, and studying gene flow effects on rapid adaptation (Rehfeldt et al., 1999; Montalvo and Ellstrand, 2001; Sexton et al., 2011; Bontrager and Angert, 2018). Lastly, restoring gene flow through genetic rescue is a proven technique for combating habitat fragmentation and needs more focused application and research (Bell et al., 2019).
Assisted gene flow can be used as a strategy to facilitate local adaptation to climate change (Aitken and Whitlock, 2013). Plant conservation and restoration managers are encouraged to experiment with gene flow and to use prescriptive gene flow more often, employing adaptive management (e.g., Williams, 2011; Lien et al., 2021) with respect to gene flow levels and prescriptive population mixes (Sgrò et al., 2011). Collecting seeds and experimenting with seed mixes that represent different gene flow “distances” are important and sorely needed actions and are especially important to test now that range shifts related to climate are prominent.
Does Range Size Predict Vulnerability Under Global Change?
Range Size Matters
The question of why some plant species are widespread, with large ranges, and others are rare or have restricted ranges, has intrigued botanists for ages. For instance, the niche breadth-range size hypothesis (Brown, 1984; Slatyer et al., 2013) predicts that a species’ range size is a manifestation ultimately of its niche breadth and thus represents its ability to persist in more or fewer environments. Besides potentially having reduced niche breadth, small-ranged species may also have fewer individuals and thus lower effective population sizes. As a result, species with small ranges may be at greater risk under global change. We refer to this phenomenon as the range size vulnerability hypothesis.
Explanations for restricted distributions range from a lack of genetic variation, to species being newly evolved taxa, to species being very old and consisting of remnants of a past range (Stebbins, 1942, 1980; Leão et al., 2020). Recent research has supported the case that plant species generally begin small, “budding” from parental species, often sympatrically within the parent species range, and then expanding over time through niche evolution and/or dispersing more widely over time (Grossenbacher et al., 2014; Anacker and Strauss, 2014). Recent literature mainly sustains this view (Gastauer et al., 2015; Heydel et al., 2017; Skeels and Cardillo, 2018), but there is important variation, nuance, and exception, and a variety of forms of speciation and specialization in plants (Boucher et al., 2016; Rajakaruna, 2018; Salariato and Zuloaga, 2021). For example, a species may evolve through adaptation to a niche that is very widespread (e.g., ruderal plants), and so it has the potential to fill this niche quickly and will appear, geologically, as if it expanded its niche rapidly and exploded. Alternatively, clade radiations may fill unused habitats, creating sudden bursts of diversification, followed by gradual broadening of ecological niches and range sizes (Tanentzap et al., 2015; Folk et al., 2019). More diverse plant lineages may typically be comprised of species with smaller ranges (Leão et al., 2020).
What ultimately determines plant species range size can be determined by myriad factors. Sheth et al. (2020) performed a meta-analysis and review on this topic and found that niche breadth, species’ age, niche availability (i.e., how common a niche is), and range position (i.e., range characteristics such as latitudinal breadth) were consistently strong factors associated with range size, but concluded that much more research is needed to confirm these effects on plant range sizes plus other potentially important effects such as mating system, ploidy, and dispersal ability. Grossenbacher et al. (2015) found strong support that more highly selfing plants have larger range sizes, and Grant and Kalisz (2020) recently confirmed that selfing plants indeed generally possess greater niche breadth than more outcrossing plant species. Moreover, polyploid plants with higher numbers of chromosomes tend to differentiate their niches faster (Baniaga et al., 2020). Finally, although logistically challenging, very few studies exist testing whether rare plant species are limited by genetic variation, but research thus far suggests that they are (Sheth et al., 2020).
No Range Should Be Left Behind in Conservation
There are clear cases finding strong support for rarity predicting increased vulnerability or conservation risk for plants under climate change. For example, Zettlemoyer et al. (2019) found that rare, more specialized plants are more likely to go extinct in a study in Michigan, United States. Aspinwall et al. (2019) demonstrated experimentally that Eucalyptus (Myrtaceae) trees with smaller range sizes were more susceptible to experimental heat waves. Many studies have found positive associations between niche breadth and species range sizes based on SDMs (see Slatyer et al., 2013). Such correlative species distribution models (cSDMs) show potentially causal relationships between range size and species performance. Nevertheless, an important caveat is that spatial autocorrelation between the number of possible environments sampled and larger geographic extents can mask or overemphasize causal relationships (Moore et al., 2018; Journé et al., 2020).
Another caveat is that such observational studies base patterns on the observed, or realized niche, rather than the fundamental niche, which is of primary interest for understanding environmental tolerances (Sexton et al., 2017; Liu et al., 2020), but see above discussions on biotic interactions and the realized niche. Nevertheless, experimental data can confirm true relationships between vulnerability and range size. Historical considerations may also be quite strong. For example, Rapoport’s Rule states that species at higher latitudes should have larger ranges due to the greater stress and variability of those environments (Brown et al., 1996). Thus, more tropical species may be driven or boxed into smaller ranges than their higher-latitude relatives due to evolutionary history. Huang et al. (2021) recently presented evidence supporting this hypothesis in plants: greater climate variability has a large potential effect on the evolution of large range sizes.
There are also clear cases and considerations in contrast to the range size vulnerability hypothesis, or cases with mixed findings (Lacher and Schwartz, 2016; Hirst et al., 2017; Cai et al., 2021). Micro-habitats, local, or sub-surface factors can buffer plants under climate change stress (Franklin et al., 2013; Gremer et al., 2015; Denney et al., 2020), and so small-ranged species that occupy highly heterogeneous landscapes may be able to weather rapid global change through more accessible escape environments. For example, rarity does not appear to limit genetic variation or preclude subpopulation structure in the geographically restricted desert forb, Astragalus lentiginosus var. piscinensis (Fabaceae) (Harrison et al., 2019). Indeed, adaptation and diversification in rare, stressful environments can cause cradles or hotspots of diversity of taxa with smaller range sizes (Buira et al., 2021). Moreover, microhabitat variation may buffer populations via “portfolio effects,” but such effects may not be enough to save rare species from extirpation under rapid climate change (Abbott et al., 2017). In this vein, a species’ realized niche may be vastly smaller than their fundamental niche. In such cases, a plant with a very small range may be able to weather a great variety of climates experienced outside of its current realized niche. Finally, a complex and nuanced reality likely exists for many species regarding this question. For example, Hirst et al. (2017) found only mixed results in support of the niche-breadth range size hypothesis in Australian alpine daisies; rarer daisy species showed evidence of increased tolerance of stressful, specialized environments at the cost of lower growth rates in low-stress environments, but their seeds were also resilient to a wider range of germination environments. Thus, species may have reduced performance in a critical stage only and such limiting stages may take a fair amount of experimentation to confirm.
Conclusions, Future Directions, and Conservation Applications
Generally, the range size vulnerability hypothesis holds as a rule: smaller ranges tend to be more vulnerable to global change, but exceptions and patterns can vary greatly by taxon. For example, Tanentzap et al. (2019) found range size to be more strongly associated with extinction risk in conifers than in palms. Thus, we recommend that special status species with smaller geographic ranges receive high conservation priority, including reserve establishment in regions having many restricted endemics plants. Rare species are also important for conservation and evolutionary study for a variety of reasons (Stebbins, 1979) and should be assumed to be of high value, including for ecosystem function and services (Lyons et al., 2005). Nevertheless, larger-ranged species are no less important as conservation targets and can be vulnerable from falling through the cracks of political boundaries (Bisbing et al., 2021; Vázquez-García et al., 2021). For such species, we recommend greater focus on connectivity, dispersal habitat corridors, and multi-stakeholder and intergovernmental conservation plans. In this vein, local adaptation is likely to be a mechanism by which widespread species maintain their distributions (see section 4) and thus population conservation of populations in unique environments is critical.
Regarding future research, several avenues can be explored to uncover the conservation risk associated with range size (Table 1). More experimental assessments of plant performance at different life stages, under variable conditions, and between different taxa with varying range sizes are required to better assess the range size vulnerability hypothesis. Although a challenging area of research, tests of plant species range size vulnerability at the population and individual level are lacking (Slatyer et al., 2013). For example, metrics such as heat shock protein response can be used to assess the vulnerability of rare versus common plant taxa to predicted climate change stress (Al-Whaibi, 2011; Aspinwall et al., 2019). In order to determine if smaller ranges are indeed more at-risk from modern habitat alterations, extinction debt (Kuussaari et al., 2009) should be assessed in taxa varying in range size (Jamin et al., 2020; Makishima et al., 2021). Questions concerning the relationship between range size and particular plant groups, or life histories, should be investigated. For example, as stated earlier, highly selfing species are expected to have larger geographic ranges and greater niche breadth, but this was not found for Epipactis (Orchidaceae) species in Europe (Evans and Jacquemyn, 2020).
Concluding Remarks
Our world is in a constant state of flux, exacerbated by rapid climate change, and researchers, managers, and stakeholders would benefit from adopting goals that attend to the impact of these changes and develop methods that can accommodate uncertainty (Rollinson et al., 2021). We have rejected some long-standing paradigms as plant species range rules, provided an initial list of rules for consideration, identified gaps in the research, and outlined tasks to enhance our understanding of how ranges are governed and how they will change (Table 1). We strongly encourage researchers to create eco-evolutionary projects that focus on native plant taxa that have not been studied or have been traditionally understudied. Whenever possible, citizen science efforts can support these initiatives and provide educational opportunities to excite the next generation of botanists and plant conservation biologists. We want to acknowledge that many patterns observed among species ranges are not independent of each other and can overlap. We also acknowledge that there may be additional rules of species ranges not considered in this review, and we encourage the field to shine light on them, especially as they relate to conservation. Finally, we encourage the scientific community at large to continue to evaluate patterns and potential rules across disciplines in order to inform effective conservation and ecosystem management. In the case of ecological or biogeographical patterns, rules can be judged or weighted by importance factors, such as phylogenetic, geographical, or environmental parameters, etc. We trust that future ecologists will finish uncovering the laws by which nature can be conserved.
Author Contributions
All authors contributed to the development, writing, and approval of the final version of the manuscript.
Conflict of Interest
The authors declare that the research was conducted in the absence of any commercial or financial relationships that could be construed as a potential conflict of interest.
Publisher’s Note
All claims expressed in this article are solely those of the authors and do not necessarily represent those of their affiliated organizations, or those of the publisher, the editors and the reviewers. Any product that may be evaluated in this article, or claim that may be made by its manufacturer, is not guaranteed or endorsed by the publisher.
Acknowledgments
The authors thank Dave Ardell, Gordon Bennett, A. Carolin Frank, Brian Perry, and the reviewers for their comments and suggestions that improved the manuscript. The authors acknowledge support from the National Science Foundation under Grant No. IOS-1558035, US Fish and Wildlife Service and Bureau of Reclamation under CESU – R17AC00044, the University of California President’s Research Catalyst Award received by the UC Conservation Genomics Consortium, and the California Conservation Genomics Project.
References
Abbott, R. E., Doak, D. F., and Peterson, M. L. (2017). Portfolio effects, climate change, and the persistence of small populations: analyses on the rare plant saussurea weberi. Ecology 98, 1071–1081. doi: 10.1002/ecy.1738
Abeli, T., Gentili, R., Mondoni, A., Orsenigo, S., and Rossi, G. (2014). Effects of marginality on plant population performance. J. Biogeogr. 41, 239–249. doi: 10.1111/jbi.12215
Ackerly, D. D., Kling, M. M., Clark, M. L., Papper, P., Oldfather, M. F., Flint, A. L., et al. (2020). Topoclimates, refugia, and biotic responses to climate change. Front. Ecol. Environ. 18:288–297. doi: 10.1002/fee.2204
Afkhami, M. E., McIntyre, P. J., and Strauss, S. Y. (2014). Mutualist-mediated effects on species’ range limits across large geographic scales. Ecol. Lett. 17, 1265–1273. doi: 10.1111/ele.12332
Aguilar, R., Cristóbal-Pérez, E. J., Balvino-Olvera, F. J., de Jesús Aguilar-Aguilar, M., Aguirre-Acosta, N., Ashworth, L., et al. (2019). Habitat fragmentation reduces plant progeny quality: a global synthesis. Ecol. Lett. 22, 1163–1173. doi: 10.1111/ele.13272
Aitken, S. N., and Whitlock, M. C. (2013). Assisted gene flow to facilitate local adaptation to climate change. Annu. Rev. Ecol. Evol. Syst. 44, 367–388. doi: 10.1146/annurev-ecolsys-110512-135747
Alpert, P., and Simms, E. L. (2002). The relative advantages of plasticity and fixity in different environments: when is it good for a plant to adjust? Evol. Ecol. 16, 285–297. doi: 10.1023/A:1019684612767
Al-Whaibi, M. H. (2011). Plant heat-shock proteins: a mini review. J. King Saud Univ. Sci. 23, 139–150. doi: 10.1016/j.jksus.2010.06.022
Anacker, B. L., and Strauss, S. Y. (2014). The geography and ecology of plant speciation: range overlap and niche divergence in sister species. Proc. R. Soc. B Biol. Sci. 281:20132980. doi: 10.1098/rspb.2013.2980
Anderson, J. T., and Song, B. (2020). Plant adaptation to climate change—where are we? J. Syst. Evol. 58, 533–545. doi: 10.1111/jse.12649
Anderson, J. T., and Wadgymar, S. M. (2020). Climate change disrupts local adaptation and favours upslope migration. Ecol. Lett. 23, 181–192. doi: 10.1111/ele.13427
Angert, A. L., Bontrager, M. G., and Ågren, J. (2020). What do we really know about adaptation at range edges? Annu. Rev. Ecol. Evol. Syst. 51, 341–361. doi: 10.1146/annurev-ecolsys-012120-091002
Anstett, D. N., Branch, H. A., and Angert, A. L. (2021). Regional differences in rapid evolution during severe drought. Evol. Lett. 5, 130–142. doi: 10.1002/evl3.218
Antonovics, J. (1976). The nature of limits to natural selection. Ann. Missouri Bot. Garden 63, 224–247. doi: 10.2307/2395303
Araújo, M. B., and Luoto, M. (2007). The importance of biotic interactions for modelling species distributions under climate change. Global Ecol. Biogeogr. 16, 743–753. doi: 10.1111/j.1466-8238.2007.00359.x
Araújo, M. B., and Peterson, A. T. (2012). Uses and misuses of bioclimatic envelope modeling. Ecology 93, 1527–1539. doi: 10.1890/11-1930.1
Aspinwall, M. J., Pfautsch, S., Tjoelker, M. G., Vårhammar, A., Possell, M., Drake, J. E., et al. (2019). Range size and growth temperature influence eucalyptus species responses to an experimental heatwave. Global Change Biol. 25, 1665–1684. doi: 10.1111/gcb.14590
Baniaga, A. E., Marx, H. E., Arrigo, N., and Barker, M. S. (2020). Polyploid plants have faster rates of multivariate niche differentiation than their diploid relatives. Ecol. Lett. 23, 68–78. doi: 10.1111/ele.13402
Barton, A. M. (1993). Factors controlling plant distributions: drought, competition, and fire in montane pines in Arizona. Ecol. Monogr. 63, 367–397. doi: 10.2307/2937151
Barton, K. E., Jones, C., Edwards, K. F., Shiels, A. B., and Knight, T. (2020). Local adaptation constrains drought tolerance in a tropical foundation tree. J. Ecol. 108, 1540–1552. doi: 10.1111/1365-2745.13354
Bayly, M. J., and Angert, A. L. (2019). Niche models do not predict experimental demography but both suggest dispersal limitation across the northern range limit of the scarlet monkeyflower (Erythranthe Cardinalis). J. Biogeogr. 46, 1316–1328. doi: 10.1111/jbi.13609
Bell, D. A., Robinson, Z. L., Funk, W. C., Fitzpatrick, S. W., Allendorf, F. W., Tallmon, D. A., et al. (2019). The exciting potential and remaining uncertainties of genetic rescue. Trends Ecol. Evol. 34, 1070–1079. doi: 10.1016/j.tree.2019.06.006
Benning, J. W., Eckhart, V. M., Geber, M. A., and Moeller, D. A. (2019). Biotic interactions contribute to the geographic range limit of an annual plant: herbivory and phenology mediate fitness beyond a range margin. Am. Natural. 193, 786–797. doi: 10.1086/703187
Benning, J. W., and Moeller, D. A. (2019). Maladaptation beyond a geographic range limit driven by antagonistic and mutualistic biotic interactions across an abiotic gradient. Evolution 73, 2044–2059. doi: 10.1111/evo.13836
Benning, J. W., and Moeller, D. A. (2021a). Microbes, mutualism, and range margins: testing the fitness consequences of soil microbial communities across and beyond a native plant’s range. New Phytol. 229, 2886–2900. doi: 10.1111/nph.17102
Benning, J. W., and Moeller, D. A. (2021b). Plant–soil interactions limit lifetime fitness outside a native plant’s geographic range margin. Ecology 102:e03254. doi: 10.1002/ecy.3254
Bisbing, S. M., Urza, A. K., Buma, B. J., Cooper, D. J., Matocq, M., and Angert, A. L. (2021). Can long-lived species keep pace with climate change? Evidence of local persistence potential in a widespread conifer. Diver. Distributions 27, 296–312. doi: 10.1111/ddi.13191
Blois, J. L., Zarnetske, P. L., Fitzpatrick, M. C., and Finnegan, S. (2013). Climate change and the past, present, and future of biotic interactions. Science 341, 499–504. doi: 10.1126/science.1237184
Bontrager, M., and Angert, A. L. (2018). Genetic differentiation is determined by geographic distance in clarkia pulchella. biorxiv [Preprint] doi: 10.1101/374454
Bontrager, M., and Angert, A. L. (2019). Gene flow improves fitness at a range edge under climate change. Evol. Lett. 3, 55–68. doi: 10.1002/evl3.91
Bontrager, M., Usui, T., Lee-Yaw, J. A., Anstett, D. N., Branch, H. A., Hargreaves, A. L., et al. (2021). Adaptation across geographic ranges is consistent with strong selection in marginal climates and legacies of range expansion. biorxiv [Preprint] doi: 10.1101/2020.08.22.262915 biorxiv 2020.08.22.262915,
Boucher, F. C., Zimmermann, N. E., and Conti, E. (2016). Allopatric speciation with little niche divergence is common among alpine primulaceae. J. Biogeogr. 43, 591–602. doi: 10.1111/jbi.12652
Brown, C. D., and Vellend, M. (2014). Non-climatic constraints on upper elevational plant range expansion under climate change. Proc. R. Soc. B Biol. Sci. 281, 20141779. doi: 10.1098/rspb.2014.1779
Brown, J. H. (1984). On the relationship between abundance and distribution of species. Am. Natural. 124, 255–279. doi: 10.1086/284267
Brown, J. H., Stevens, G. C., and Kaufman, D. M. (1996). The geographic range: size, shape, boundaries, and internal structure. Annu. Rev. Ecol. Evol. Syst. 27, 597–623. doi: 10.1146/annurev.ecolsys.27.1.597
Bucharova, A. (2017). Assisted migration within species range ignores biotic interactions and lacks evidence. Restoration Ecol. 25, 14–18. doi: 10.1111/rec.12457
Bueno de Mesquita, C. P., King, A. J., Schmidt, S. K., Farrer, E. C., and Suding, K. N. (2016). Incorporating biotic factors in species distribution modeling: are interactions with soil microbes important? Ecography 39, 970–980. doi: 10.1111/ecog.01797
Bueno de Mesquita, C. P., Sartwell, S. A., Schmidt, S. K., and Suding, K. N. (2020). Growing-season length and soil microbes influence the performance of a generalist bunchgrass beyond its current range. Ecology 101:e03095. doi: 10.1002/ecy.3095
Buira, A., Fernández-Mazuecos, M., Aedo, C., and Molina-Venegas, R. (2021). The contribution of the edaphic factor as a driver of recent plant diversification in a Mediterranean biodiversity hotspot. J. Ecol. 109, 987–999. doi: 10.1111/1365-2745.13527
Bulgarelli, D., Rott, M., Schlaeppi, K., Ver Loren van Themaat, E., Ahmadinejad, N., Assenza, F., et al. (2012). Revealing structure and assembly cues for Arabidopsis root-inhabiting bacterial microbiota. Nature 488, 91–95. doi: 10.1038/nature11336
Bulleri, F., Bruno, J. F., Silliman, B. R., and Stachowicz, J. J. (2016). Facilitation and the niche: implications for coexistence, range shifts and ecosystem functioning. Funct. Ecol. 30, 70–78. doi: 10.1111/1365-2435.12528
Bullock, J. M., Edwards, R. J., Carey, P. D., and Rose, R. J. (2000). Geographical separation of two Ulex species at three spatial scales: does competition limit species’ ranges? Ecography 23, 257–271. doi: 10.1111/j.1600-0587.2000.tb00281.x
Burkle, L. A., Marlin, J. C., and Knight, T. M. (2013). Plant-pollinator interactions over 120 years: loss of species, co-occurrence, and function. Science 339, 1611–1615. doi: 10.1126/science.1232728
Cai, Q., Welk, E., Ji, C., Fang, W., Sabatini, F. M., Zhu, J., et al. (2021). The relationship between niche breadth and range size of beech (Fagus) species worldwide. J. Biogeogr. 48, 1240–1253. doi: 10.1111/jbi.14074
Caissy, P., Klemet-N′Guessan, S., Jackiw, R., Eckert, C. G., and Hargreaves, A. L. (2020). High conservation priority of range-edge plant populations not matched by habitat protection or research effort. Biol. Conserv. 249:108732. doi: 10.1016/j.biocon.2020.108732
Callaway, R. M., Brooker, R. W., Choler, P., Kikvidze, Z., Lortie, C. J., Michalet, R., et al. (2002). Positive interactions among alpine plants increase with stress. Nature 417, 844–848. doi: 10.1038/nature00812
Cardinale, B. J., Matulich, K. L., Hooper, D. U., Byrnes, J. E., Duffy, E., Gamfeldt, L., et al. (2011). The functional role of producer diversity in ecosystems. Am. J. Bot. 98, 572–592. doi: 10.3732/ajb.1000364
Chalcoff, V. R., Aizen, M. A., and Ezcurra, C. (2012). Erosion of a pollination mutualism along an environmental gradient in a south Andean treelet, Embothrium coccineum (Proteaceae). Oikos 121, 471–480. doi: 10.1111/j.1600-0706.2011.19663.x
Channell, R., and Lomolino, M. V. (2000). Dynamic biogeography and conservation of endangered species. Nature 403, 84–86. doi: 10.1038/47487
Chen, L., Huang, J., Ma, Q., Hänninen, H., Rossi, S., Piao, S., et al. (2018). Spring phenology at different altitudes is becoming more uniform under global warming in europe. Global Change Biol. 24, 3969–3975. doi: 10.1111/gcb.14288
Chuine, I. (2010). Why does phenology drive species distribution? Philos. Trans. R. Soc. B Biol. Sci. 365, 3149–3160. doi: 10.1098/rstb.2010.0142
Connallon, T., and Sgrò, C. M. (2018). In search of a general theory of species’ range evolution. PLoS Biol. 16:e2006735. doi: 10.1371/journal.pbio.2006735
Conner, J. K., and Hartl, D. L. (2004). A Primer of Ecological Genetics. Sunderland, MA: Sinauer Associates Incorporated.
Cortés, A. J., Waeber, S., Lexer, C., Sedlacek, J., Wheeler, J. A., van Kleunen, M., et al. (2014). Small-scale patterns in snowmelt timing affect gene flow and the distribution of genetic diversity in the alpine dwarf shrub Salix herbacea. Heredity 113, 233–239. doi: 10.1038/hdy.2014.19
Couvet, D. (2002). Deleterious effects of restricted gene flow in fragmented populations. Conserv. Biol. 16, 369–376. doi: 10.1046/j.1523-1739.2002.99518.x
Cross, R. L., and Eckert, C. G. (2020). Integrated empirical approaches to better understand species’ range limits. Am. J. Bot. 107, 12–16. doi: 10.1002/ajb2.1400
Crotty, S. M., and Bertness, M. D. (2015). Positive interactions expand habitat use and the realized niches of sympatric species. Ecology 96, 2575–2582. doi: 10.1890/15-0240.1
Cruz-Nicolás, J., Giles-Pérez, G. I., Lira-Noriega, A., Martínez-Méndez, N., Aguirre-Planter, E., Eguiarte, L. E., et al. (2020). Using niche centrality within the scope of the nearly neutral theory of evolution to predict genetic diversity in a tropical conifer species-pair. J. Biogeogr. 47, 2755–2772. doi: 10.1111/jbi.13979
Da Silva, M. F., Cruz, M. V., Vidal Júnior, J. D., Zucchi, M. I., Mori, G. M., and De Souza, A. P. (2021). Geographical and environmental contributions to genomic divergence in mangrove forests. Biol. J. Linnean Soc. 132, 573–589. doi: 10.1093/biolinnean/blaa199
Dai, J., Wang, H., and Ge, Q. (2014). The spatial pattern of leaf phenology and its response to climate change in China. Int. J. Biometeorol. 58, 521–528. doi: 10.1007/s00484-013-0679-2
Dallas, T., Decker, R. R., and Hastings, A. (2017). Species are not most abundant in the centre of their geographic range or climatic niche. Ecol. Lett. 20, 1526–1533. doi: 10.1111/ele.12860
Dallas, T. A., and Hastings, A. (2018). Habitat suitability estimated by niche models is largely unrelated to species abundance. Global Ecol. Biogeogr. 27, 1448–1456. doi: 10.1111/geb.12820
Darwin, C. (1859). On The Origin of Species by Means of Natural Selection, or, the Preservation of Favoured Races in the Struggle for Life. J. Murray. London: Routledge.
David, A. S., Quintana-Ascencio, P. F., Menges, E. S., Thapa-Magar, K. B., Afkhami, M. E., and Searcy, C. A. (2019). Soil microbiomes underlie population persistence of an endangered plant species. Am. Natural. 194, 488–494. doi: 10.1086/704684
de Lafontaine, G., Napier, J. D., Petit, R. J., and Hu, F. S. (2018). Invoking adaptation to decipher the genetic legacy of past climate change. Ecology 99, 1530–1546. doi: 10.1002/ecy.2382
Denney, D. A., Jameel, M. I., Bemmels, J. B., Rochford, M. E., and Anderson, J. T. (2020). Small spaces, big impacts: contributions of micro-environmental variation to population persistence under climate change. AoB Plants 12:laa005. doi: 10.1093/aobpla/plaa005
Dickey, J. R., Swenie, R. A., Turner, S. C., Winfrey, C. C., Yaffar, D., Padukone, A., et al. (2021). The utility of macroecological rules for microbial biogeography. Front. Ecol. Evol. 9:633155. doi: 10.3389/fevo.2021.633155
Dickman, E. E., Pennington, L. K., Franks, S. J., and Sexton, J. P. (2019). Evidence for adaptive responses to historic drought across a native plant species range. Evol. Applic. 12, 1569–1582. doi: 10.1111/eva.12803
Duffy, K. J., and Johnson, S. D. (2017). Specialized mutualisms may constrain the geographical distribution of flowering plants. Proc. R. Soc. B Biol. Sci. 284:20171841. doi: 10.1098/rspb.2017.1841
Eckert, C. G., Samis, K. E., and Lougheed, S. C. (2008). Genetic variation across species’ geographical ranges: the central–marginal hypothesis and beyond. Mol. Ecol. 17, 1170–1188. doi: 10.1111/j.1365-294X.2007.03659.x
Ehrlén, J., and Morris, W. F. (2015). Predicting changes in the distribution and abundance of species under environmental change. Ecol. Lett. 18, 303–314. doi: 10.1111/ele.12410
Ellstrand, N. C., and Elam, D. R. (1993). Population genetic consequences of small population size: implications for plant conservation. Annu. Rev. Ecol. Evol. Syst. 24, 217–242. doi: 10.1146/annurev.es.24.110193.001245
Eskelinen, A., Kaarlejärvi, E., and Olofsson, J. (2017). Herbivory and nutrient limitation protect warming tundra from lowland species’ invasion and diversity loss. Global Change Biol. 23, 245–255. doi: 10.1111/gcb.13397
Etterson, J. R., Franks, S. J., Mazer, S. J., Shaw, R. G., Gorden, N. L. S., Schneider, H. E., et al. (2016). Project baseline: an unprecedented resource to study plant evolution across space and time. Am. J. Bot. 103, 164–173. doi: 10.3732/ajb.1500313
Ettinger, A., and Hille Ris Lambers, J. (2017). Competition and facilitation may lead to asymmetric range shift dynamics with climate change. Global Change Biol. 23, 3921–3933. doi: 10.1111/gcb.13649
Evans, A., and Jacquemyn, H. (2020). Impact of mating system on range size and niche breadth in epipactis (Orchidaceae). Ann. Bot. 126, 1203–1214. doi: 10.1093/aob/mcaa142
Feeley, K. J. (2012). Distributional migrations, expansions, and contractions of tropical plant species as revealed in dated herbarium records. Global Change Biol. 18, 1335–1341. doi: 10.1111/j.1365-2486.2011.02602.x
Feeley, K. J., Silman, M. R., Bush, M. B., Farfan, W., Cabrera, K. G., Malhi, Y., et al. (2011). Upslope migration of Andean trees. J. Biogeogr. 38, 783–791. doi: 10.1111/j.1365-2699.2010.02444.x
Fenster, C. B., and Galloway, L. F. (2000). Population differentiation in an annual legume: genetic architecture. Evolution 54, 1157–1172. doi: 10.1111/j.0014-3820.2000.tb00551.x
Fitzpatrick, S. W., and Funk, W. C. (2019). Genomics for Genetic Rescue. Population Genomics: Wildlife. (Cham: Springer), 437–471.
Folk, R. A., Stubbs, R. L., Mort, M. E., Cellinese, N., Allen, J. M., Soltis, P. S., et al. (2019). Rates of niche and phenotype evolution lag behind diversification in a temperate radiation. Proc. Natl. Acad. Sci. U.S.A. 116, 10874–10882. doi: 10.1073/pnas.1817999116
Frankham, R., Ballou, J. D., Ralls, K., Eldridge, M. D. B., Dudash, M. R., Fenster, C. B., et al. (2017). Genetic Management of Fragmented Animal and Plant Populations. Oxford: Oxford University Press.
Franklin, J., Davis, F. W., Ikegami, M., Syphard, A. D., Flint, L. E., Flint, A. L., et al. (2013). Modeling plant species distributions under future climates: how fine scale do climate projections need to be? Global Change Biol. 19, 473–483. doi: 10.1111/gcb.12051
Franklin, J., Serra-Diaz, J. M., Syphard, A. D., and Regan, H. M. (2017). Big data for forecasting the impacts of global change on plant communities. Global Ecol. Biogeogr. 26, 6–17. doi: 10.1111/geb.12501
Franks, S. J., Hamann, E., and Weis, A. E. (2018). Using the resurrection approach to understand contemporary evolution in changing environments. Evol. Applic. 11, 17–28. doi: 10.1111/eva.12528
Franks, S. J., and Weis, A. E. (2009). Climate change alters reproductive isolation and potential gene flow in an annual plant. Evol. Applic. 2, 481–488. doi: 10.1111/j.1752-4571.2009.00073.x
Freeman, B. G., Lee-Yaw, J. A., Sunday, J. M., and Hargreaves, A. L. (2018). Expanding, shifting and shrinking: the impact of global warming on species’ elevational distributions. Global Ecol. Biogeogr. 27, 1268–1276. doi: 10.1111/geb.12774
García-Ramos, G., and Kirkpatrick, M. (1997). Genetic models of adaptation and gene flow in peripheral populations. Evolution 51, 21–28. doi: 10.1111/j.1558-5646.1997.tb02384.x
Garzón, M. B., Robson, T. M., and Hampe, A. (2019). ΔTraitSDMs: species distribution models that account for local adaptation and phenotypic plasticity. New Phytol. 222, 1757–1765. doi: 10.1111/nph.15716
Gastauer, M., Saporetti-Junior, A. W., Silva Magnago, L. F., Cavender-Bares, J., and Meira-Neto, J. A. A. (2015). The hypothesis of sympatric speciation as the dominant generator of endemism in a global hotspot of biodiversity. Ecol. Evol. 5, 5272–5283. doi: 10.1002/ece3.1761
Gaston, K. J. (2003). The Structure and Dynamics of Geographic Ranges. Oxford: Oxford University Press.
Gauzere, J., Klein, E. K., Brendel, O., Davi, H., and Oddou-Muratorio, S. (2020). Microgeographic adaptation and the effect of pollen flow on the adaptive potential of a temperate tree species. New Phytol. 227, 641–653. doi: 10.1111/nph.16537
Gérard, M., Vanderplanck, M., Wood, T., and Michez, D. (2020). Global warming and plant–pollinator mismatches. Emerg. Top. Life Sci. 4, 77–86. doi: 10.1042/ETLS20190139
Ghalambor, C. K., Huey, R. B., Martin, P. R., Tewksbury, J. J., and Wang, G. (2006). Are mountain passes higher in the tropics? Janzen’s hypothesis revisited. Integr. Comp. Biol. 46, 5–17. doi: 10.1093/icb/icj003
Giannini, T. C., Chapman, D. S., Saraiva, A. M., Alves-dos-Santos, I., and Biesmeijer, J. C. (2013). Improving species distribution models using biotic interactions: a case study of parasites, pollinators and plants. Ecography 36, 649–656. doi: 10.1111/j.1600-0587.2012.07191.x
Grant, A., and Kalisz, S. (2020). Do selfing species have greater niche breadth? Support from ecological niche modeling. Evolution 74, 73–88. doi: 10.1111/evo.13870
Greiser, C., Hylander, K., Meineri, E., Luoto, M., and Ehrlén, J. (2020). Climate limitation at the cold edge: contrasting perspectives from species distribution modelling and a transplant experiment. Ecography 43, 637–647. doi: 10.1111/ecog.04490
Gremer, J. R., Bradford, J. B., Munson, S. M., and Duniway, M. C. (2015). Desert grassland responses to climate and soil moisture suggest divergent vulnerabilities across the Southwestern United States. Global Change Biol. 21, 4049–4062. doi: 10.1111/gcb.13043
Griggs, R. F. (1914). Observations on the behavior of some species at the edges of their ranges. Bull. Torrey Bot. Club 41, 25–49. doi: 10.2307/2479433
Grossenbacher, D., Runquist, R. B., Goldberg, E. E., and Brandvain, Y. (2015). Geographic range size is predicted by plant mating system. Ecol. Lett. 18, 706–713. doi: 10.1111/ele.12449
Grossenbacher, D. L., Veloz, S. D., and Sexton, J. P. (2014). Niche and range size patterns suggest that speciation begins in small, ecologically diverged populations in North American monkeyflowers (Mimulus Spp.). Evolution 68, 1270–1280. doi: 10.1111/evo.12355
Guida, R. J., Abella, S. R., Smith, W. J. Jr., Stephen, H., and Roberts, C. L. (2014). Climatic change and desert vegetation distribution: assessing thirty years of change in southern Nevada’s Mojave Desert. Prof. Geogr. 66, 311–322. doi: 10.1080/00330124.2013.787007
Haddad, N. M., Brudvig, L. A., Clobert, J., Davies, K. F., Gonzalez, A., Holt, R. D., et al. (2015). Habitat fragmentation and its lasting impact on Earth’s ecosystems. Sci. Adv. 1:e1500052. doi: 10.1126/sciadv.1500052
Halbritter, D. A., Teets, N. M., Williams, C. M., and Daniels, J. C. (2018). Differences in winter cold hardiness reflect the geographic range disjunction of Neophasia menapia and Neophasia terlooii (Lepidoptera: Pieridae). J. Insect Physiol. 107, 204–211. doi: 10.1016/j.jinsphys.2018.03.005
Haldane, J. B. S., and Ford, E. B. (1956). The relation between density regulation and natural selection. Proc. R. Soc. Lond. Ser. B Biol. Sci. 145, 306–308. doi: 10.1098/rspb.1956.0039
Hällfors, M. H., Aikio, S., and Schulman, L. E. (2017). Quantifying the need and potential of assisted migration. Biol. Conserv. 205, 34–41. doi: 10.1016/j.biocon.2016.11.023
Hamann, E., Blevins, C., Franks, S. J., Jameel, M. I., and Anderson, J. T. (2021). Climate change alters plant–herbivore interactions. New Phytol. 229, 1894–1910. doi: 10.1111/nph.17036
Hampe, A., and Petit, R. J. (2005). Conserving biodiversity under climate change: the rear edge matters. Ecol. Lett. 8, 461–467. doi: 10.1111/j.1461-0248.2005.00739.x
Hamrick, J. L., and Godt, M. J. W. (1996). Effects of life history traits on genetic diversity in plant species. Philos. Trans. R. Soc. Lond. Ser B Biol. Sci. 351, 1291–1298. doi: 10.1098/rstb.1996.0112
Hargreaves, A. L., and Eckert, C. G. (2019). Local adaptation primes cold-edge populations for range expansion but not warming-induced range shifts. Ecol. Lett. 22, 78–88. doi: 10.1111/ele.13169
Hargreaves, A. L., Germain, R. M., Bontrager, M., Persi, J., and Angert, A. L. (2019a). Local adaptation to biotic interactions: a meta-analysis across latitudes. Am. Natural. 195, 395–411. doi: 10.1086/707323
Hargreaves, A. L., Suárez, E., Mehltreter, K., Myers-Smith, I., Vanderplank, S. E., Slinn, H. L., et al. (2019b). Seed predation increases from the arctic to the equator and from high to low elevations. Sci. Adv. 5:eaau4403. doi: 10.1126/sciadv.aau4403
Hargreaves, A. L., Samis, K. E., and Eckert, C. G. (2014). Are species’ range limits simply niche limits writ large? A review of transplant experiments beyond the range. Am. Natural. 183, 157–173. doi: 10.1086/674525
Harrison, J. G., Forister, M. L., Mcknight, S. R., Nordin, E., and Parchman, T. L. (2019). Rarity does not limit genetic variation or preclude subpopulation structure in the geographically restricted desert forb Astragalus lentiginosus var. piscinensis. Am. J. Bot. 106, 260–269. doi: 10.1002/ajb2.1235
Hedrick, P. W., Adams, J. R., and Vucetich, J. A. (2011). Reevaluating and broadening the definition of genetic rescue. Conserv. Biol. 25, 1069–1070. doi: 10.1111/j.1523-1739.2011.01751.x
Hernández-Lambraño, R. E., Carbonell, R., and Sánchez-Agudo, J. A. (2020). Making the most of scarce data: mapping distribution range and variation in population abundance of a threatened narrow-range endemic plant. J. Nat. Conserv. 57:125889. doi: 10.1016/j.jnc.2020.125889
Heydel, F., Engels, J. G., Feigs, J. T., Vásquez, E., Rudolph, B., Rohwer, J. G., et al. (2017). Adaptation to tidal flooding and rapid genetic divergence between a narrow endemic grass species and its widespread congener lead to an early stage of ecological speciation. Perspect. Plant Ecol. Evol. Syst. 27, 57–67. doi: 10.1016/j.ppees.2017.05.001
Hille Ris Lambers, J., Harsch, M. A., Ettinger, A. K., Ford, K. R., and Theobald, E. J. (2013). How will biotic interactions influence climate change induced range shifts? Annals of the New York Academy of Sciences 1297, 112–125. doi: 10.1111/nyas.12182
Hirao, A. S., and Kudo, G. (2004). Landscape genetics of alpine-snowbed plants: comparisons along geographic and snowmelt gradients. Heredity 93, 290–298. doi: 10.1038/sj.hdy.6800503
Hirst, M. J., Griffin, P. C., Sexton, J. P., and Hoffmann, A. A. (2017). Testing the niche-breadth–range-size hypothesis: habitat specialization vs. performance in Australian alpine daisies. Ecology 98, 2708–2724. doi: 10.1002/ecy.1964
Hoffmann, A. A., and Blows, M. W. (1994). Species borders: ecological and evolutionary perspectives. Trends Ecol. Evol. 9, 223–227. doi: 10.1016/0169-5347(94)90248-8
Hoffmann, A. A., Sgrò, C. M., and Kristensen, T. N. (2017). Revisiting adaptive potential, population size, and conservation. Trends Ecol. Evol. 32, 506–517. doi: 10.1016/j.tree.2017.03.012
Holt, R. D., and Barfield, M. (2009). Trophic interactions and range limits: the diverse roles of predation. Proc. R. Soc. B Biol. Sci. 276, 1435–1442. doi: 10.1098/rspb.2008.1536
Holt, R. D., and Gomulkiewicz, R. (1997). The evolution of species’ niches: a population dynamic perspective. Case Stud. Math. Modell. Ecol. Physiol. Cell Biol. 25–50.
Huang, E., Chen, Y., Fang, M., Zheng, Y., and Yu, S. (2021). Environmental drivers of plant distributions at global and regional scales. Global Ecol. Biogeogr. 30, 697–709. doi: 10.1111/geb.13251
Jamin, A., Peintinger, M., Gimmi, U., Holderegger, R., and Bergamini, A. (2020). Evidence for a possible extinction debt in Swiss wetland specialist plants. Ecol. Evol. 10, 1264–1277. doi: 10.1002/ece3.5980
Jiang, S., Luo, M., Gao, R., Zhang, W., Yang, Y., Li, Y., et al. (2019). Isolation-by-environment as a driver of genetic differentiation among populations of the only broad-leaved evergreen shrub Ammopiptanthus mongolicus in Asian temperate deserts. Sci. Rep. 9:12008. doi: 10.1038/s41598-019-48472-y
Jiménez-Valverde, A., Aragón, P., and Lobo, J. M. (2021). Deconstructing the abundance–suitability relationship in species distribution modelling. Global Ecol. Biogeogr. 30, 327–338. doi: 10.1111/geb.13204
Journé, V., Barnagaud, J., Bernard, C., Crochet, P., and Morin, X. (2020). Correlative climatic niche models predict real and virtual species distributions equally well. Ecology 101:e02912. doi: 10.1002/ecy.2912
Kaarlejärvi, E., Eskelinen, A., and Olofsson, J. (2017). Herbivores rescue diversity in warming tundra by modulating trait-dependent species losses and gains. Nat. Commun. 8:419. doi: 10.1038/s41467-017-00554-z
Kawecki, T. J. (2008). Adaptation to marginal habitats. Annu. Rev. Ecol. Evol. Syst. 39, 321–342. doi: 10.1146/annurev.ecolsys.38.091206.095622
Kelly, A. E., and Goulden, M. L. (2008). Rapid shifts in plant distribution with recent climate change. Proc. Natl. Acad. Sci. U.S.A. 105, 11823–11826. doi: 10.1073/pnas.0802891105
Kennedy, J. P., Preziosi, R. F., Rowntree, J. K., and Feller, I. C. (2020). Is the central-marginal hypothesis a general rule? Evidence from three distributions of an expanding Mangrove species, Avicennia germinans (L.) L. Mol. Ecol. 29, 704–719. doi: 10.1111/mec.15365
Kindsvater, H. K., Mangel, M., Reynolds, J. D., and Dulvy, N. K. (2016). Ten principles from evolutionary ecology essential for effective marine conservation. Ecol. Evol. 6, 2125–2138. doi: 10.1002/ece3.2012
Kirkpatrick, M., and Barton, N. H. (1997). Evolution of a species’ range. Am. Natural. 150, 1–23. doi: 10.1086/286054
Kissling, W. D., Dormann, C. F., Groeneveld, J., Hickler, T., Kühn, I., McInerny, G. J., et al. (2012). Towards novel approaches to modelling biotic interactions in multispecies assemblages at large spatial extents. J. Biogeogr. 39, 2163–2178. doi: 10.1111/j.1365-2699.2011.02663.x
Kooyers, N. J., Morioka, K. A., Colicchio, J. M., Clark, K. S., Donofrio, A., Estill, S. K., et al. (2021). Population responses to a historic drought across the range of the common monkeyflower (Mimulus guttatus). Am. J. Bot. 108, 284–296. doi: 10.1002/ajb2.1589
Koski, M. H., Layman, N. C., Prior, C. J., Busch, J. W., and Galloway, L. F. (2019). Selfing ability and drift load evolve with range expansion. Evol. Lett. 3, 500–512. doi: 10.1002/evl3.136
Kottler, E. J., Dickman, E. E., Sexton, J. P., Emery, N. C., and Franks, S. J. (2021). Draining the swamping hypothesis: little evidence that gene flow reduces fitness at range edges. Trends Ecol. Evol. 36, 533–544. doi: 10.1016/j.tree.2021.02.004
Koziol, L., Schultz, P. A., House, G. L., Bauer, J. T., Middleton, E. L., and Bever, J. D. (2018). The plant microbiome and native plant restoration: the example of native mycorrhizal fungi. BioScience 68, 996–1006. doi: 10.1093/biosci/biy125
Kuussaari, M., Bommarco, R., Heikkinen, R. K., Helm, A., Krauss, J., Lindborg, R., et al. (2009). Extinction debt: a challenge for biodiversity conservation. Trends Ecol. Evol. 24, 564–571. doi: 10.1016/j.tree.2009.04.011
Lacher, I., and Schwartz, M. W. (2016). Empirical test on the relative climatic sensitivity between individuals of narrowly and broadly distributed species. Ecosphere 7:e01227. doi: 10.1002/ecs2.1227
Lander, T. A., Klein, E. K., Roig, A., and Oddou-Muratorio, S. (2021). Weak founder effects but significant spatial genetic imprint of recent contraction and expansion of European beech populations. Heredity 126, 491–504. doi: 10.1038/s41437-020-00387-5
Lau, J. A., and Lennon, J. T. (2012). Rapid responses of soil microorganisms improve plant fitness in novel environments. Proc. Natl. Acad. Sci. U.S.A. 109, 14058–14062. doi: 10.1073/pnas.1202319109
Lau, J. A., McCall, A. C., Davies, K. F., McKay, J. K., and Wright, J. W. (2008). Herbivores and edaphic factors constrain the realized niche of a native plant. Ecology 89, 754–762. doi: 10.1890/07-0591.1
Leão, T. C. C., Lughadha, E. N., and Reich, P. B. (2020). Evolutionary patterns in the geographic range size of Atlantic forest plants. Ecography 43, 1510–1520. doi: 10.1111/ecog.05160
Lee-Yaw, J. A., Kharouba, H. A., Bontrager, M., Mahony, C., Csergő, A. M., Noreen, A. M. E., et al. (2016). A synthesis of transplant experiments and ecological niche models suggests that range limits are often niche limits. Ecol. Lett. 19, 710–722. doi: 10.1111/ele.12604
Leinonen, P. H., Salmela, M. J., Greenham, K., McClung, C. R., and Willis, J. H. (2020). Populations are differentiated in biological rhythms without explicit elevational clines in the plant Mimulus laciniatus. J. Biol. Rhy. 35, 452–464. doi: 10.1177/0748730420936408
Lenoir, J., Gégout, J. C., Marquet, P. A., de Ruffray, P., and Brisse, H. (2008). A significant upward shift in plant species optimum elevation during the 20th century. Science 320, 1768–1771. doi: 10.1126/science.1156831
Lesica, P., and Allendorf, F. W. (1995). When are peripheral populations valuable for conservation? Conserv. Biol. 9, 753–760. doi: 10.1046/j.1523-1739.1995.09040753.x
Lien, A. M., Dew, T., Ruyle, G. B., Sherman, N. R., Perozzo, N., Miller, M., et al. (2021). Trust is essential to the implementation of adaptive management on public lands. Rangeland Ecol. Manag. 77, 46–56. doi: 10.1016/j.rama.2021.03.005
Liu, H., Ye, Q., and Wiens, J. J. (2020). Climatic-niche evolution follows similar rules in plants and animals. Nat. Ecol. Evol. 4, 753–763. doi: 10.1038/s41559-020-1158-x
Liu, Z., Li, Y., Wang, J., He, X., and Tian, C. (2015). Different respiration metabolism between mycorrhizal and non-mycorrhizal rice under low-temperature stress: a cry for help from the host. J. Agric. Sci. 153, 602–614. doi: 10.1017/S0021859614000434
Lopez, S., Rousset, F., Shaw, F. H., Shaw, R. G., and Ronce, O. (2008). Migration load in plants: role of pollen and seed dispersal in heterogeneous landscapes. J. Evol. Biol. 21, 294–309. doi: 10.1111/j.1420-9101.2007.01442.x
Louda, S. M. (1982). Distribution ecology: variation in plant recruitment over a gradient in relation to insect seed predation. Ecol. Monogr. 52, 25–41. doi: 10.2307/2937343
Louthan, A. M., Doak, D. F., and Angert, A. L. (2015). Where and when do species interactions set range limits? Trends Ecol. Evol. 30, 780–792. doi: 10.1016/j.tree.2015.09.011
Lyons, K. G., Brigham, C. A., Traut, B. H., and Schwartz, M. W. (2005). Rare species and ecosystem functioning. Conserv. Biol. 19, 1019–1024. doi: 10.1111/j.1523-1739.2005.00106.x
MacArthur, R. H. (1972). Geographical Ecology: patterns in the distribution of species. Princeton, NJ: Princeton University Press.
Macdonald, S. L., Llewelyn, J., Moritz, C., and Phillips, B. L. (2017). Peripheral isolates as sources of adaptive diversity under climate change. Front. Ecol. Evol. 5:88. doi: 10.3389/fevo.2017.00088
Makishima, D., Sutou, R., Goto, A., Kawai, Y., Ishii, N., Taniguchi, H., et al. (2021). Potential extinction debt due to habitat loss and fragmentation in subalpine moorland ecosystems. Plant Ecol. 222, 445–457. doi: 10.1007/s11258-021-01118-4
Mamantov, M. A., Gibson-Reinemer, D. K., Linck, E. B., and Sheldon, K. S. (2021). Climate-driven range shifts of montane species vary with elevation. Global Ecol. Biogeogr. 30, 784–794. doi: 10.1111/geb.13246
Meng, H., Zhou, S., Jiang, X., Gugger, P. F., Li, L., Tan, Y., et al. (2019). Are mountaintops climate refugia for plants under global warming? A lesson from high-mountain oaks in tropical rainforest. Alpine Bot. 129, 175–183. doi: 10.1007/s00035-019-00226-2
Menzel, A., Sparks, T. H., Estrella, N., Koch, E., Aasa, A., Ahas, R., et al. (2006). European phenological response to climate change matches the warming pattern. Global Change Biol. 12, 1969–1976. doi: 10.1111/j.1365-2486.2006.01193.x
Midlands Integrative Biosciences Training Partnership (2019). Understanding the Rules of Life. 2019. Available online at: https://warwick.ac.uk/fac/cross_fac/mibtp/areas_of_research/systems_approaches/. (accessed July 28, 2021).
Miele, V., Matias, C., Ohlmann, M., Poggiato, G., Dray, S., and Thuiller, W. (2021). Quantifying the overall effect of biotic interactions on species communities along environmental gradients. arxiv [Preprint] arxiv:2103.10433,
Millar, N. S., and Bennett, A. E. (2016). Stressed out symbiotes: hypotheses for the influence of abiotic stress on arbuscular mycorrhizal fungi. Oecologia 182, 625–641. doi: 10.1007/s00442-016-3673-7
Miller, T. E. X., Angert, A. L., Brown, C. D., Lee-Yaw, J. A., Lewis, M., Lutscher, F., et al. (2020). Eco-evolutionary dynamics of range expansion. Ecology 101:e03139. doi: 10.1002/ecy.3139
Moeller, D. A., Geber, M. A., Eckhart, V. M., and Tiffin, P. (2012). Reduced pollinator service and elevated pollen limitation at the geographic range limit of an annual plant. Ecology 93, 1036–1048. doi: 10.1890/11-1462.1
Moeller, D. A., Geber, M. A., and Tiffin, P. (2011). Population genetics and the evolution of geographic range limits in an annual plant. Am. Natural. 178, S44–S57. doi: 10.1086/661783
Montalvo, A. M., and Ellstrand, N. C. (2001). Nonlocal transplantation and outbreeding depression in the subshrub Lotus scoparius (Fabaceae). Am. J. Bot. 88, 258–269. doi: 10.2307/2657017
Moore, T., Gaynus, C., Levin, P. S., and Meyer, R. (2021). The Intersection of Forensic Techniques With Ecological Issues. Wildlife Biodiversity Conservation. (Cham: Springer), 147–161.
Moore, T. E., Bagchi, R., Aiello-Lammens, M. E., and Schlichting, C. D. (2018). Spatial autocorrelation inflates niche breadth–range size relationships. Global Ecol. Biogeogr. 27, 1426–1436. doi: 10.1111/geb.12818
Moracho, E., Moreno, G., Jordano, P., and Hampe, A. (2016). Unusually limited pollen dispersal and connectivity of pedunculate oak (Quercus robur) refugial populations at the species’ southern range margin. Mol. Ecol. 25, 3319–3331. doi: 10.1111/mec.13692
Moran, E. V., Reid, A., and Levine, J. M. (2017). Population genetics and adaptation to climate along elevation gradients in invasive Solidago canadensis. PLoS One 12:e0185539. doi: 10.1371/journal.pone.0185539
Morente-López, J., Lara-Romero, C., García-Fernández, A., Teso, M. L. R., Prieto-Benítez, S., and Iriondo, J. M. (2021). Gene flow effects on populations inhabiting marginal areas: origin matters. J. Ecol. 109, 139–153. doi: 10.1111/1365-2745.13455
Moyle, L. C. (2006). Correlates of genetic differentiation and isolation by distance in 17 congeneric silene species. Mol. Ecol. 15, 1067–1081. doi: 10.1111/j.1365-294X.2006.02840.x
Nadeau, C. P., and Urban, M. C. (2019). Eco-evolution on the edge during climate change. Ecography 42, 1280–1297. doi: 10.1111/ecog.04404
National Science Foundation (2016). NSF’s 10 Big Ideas: Understanding the Rules of Life. Special Reports. NSF’s 10 Big Ideas. Alexandria. VA: National Science Foundation. Available online at: https://www.nsf.gov/news/special_reports/big_ideas/life.jsp (accessed July 15, 2021).
Normand, S., Treier, U. A., Randin, C., Vittoz, P., Guisan, A., and Svennin, J. (2009). Importance of abiotic stress as a range-limit determinant for European plants: insights from species responses to climatic gradients. Global Ecol. Biogeogr. 18, 437–449. doi: 10.1111/j.1466-8238.2009.00451.x
Oakley, C. G., Ågren, J., and Schemske, D. W. (2015). Heterosis and outbreeding depression in crosses between natural populations of Arabidopsis thaliana. Heredity 115, 73–82. doi: 10.1038/hdy.2015.18
Oldfather, M. F., Kling, M. M., Sheth, S. N., Emery, N. C., and Ackerly, D. D. (2020). Range edges in heterogeneous landscapes: integrating geographic scale and climate complexity into range dynamics. Global Change Biol. 26, 1055–1067. doi: 10.1111/gcb.14897
Orsini, L., Vanoverbeke, J., Swillen, I., Mergeay, J., and De Meester, L. (2013). Drivers of population genetic differentiation in the wild: isolation by dispersal limitation, isolation by adaptation and isolation by colonization. Mol. Ecol. 22, 5983–5999. doi: 10.1111/mec.12561
Osorio-Olvera, L., Soberón, J., and Falconi, M. (2019). On population abundance and niche structure. Ecography 42, 1415–1425. doi: 10.1111/ecog.04442
Palacio-López, K., Beckage, B., Scheiner, S., and Molofsky, J. (2015). The ubiquity of phenotypic plasticity in plants: a synthesis. Ecol. Evol. 5, 3389–3400. doi: 10.1002/ece3.1603
Papuga, G., Gauthier, P., Pons, V., Farris, E., and Thompson, J. D. (2018). Ecological niche differentiation in peripheral populations: a comparative analysis of eleven Mediterranean plant species. Ecography 41, 1650–1664. doi: 10.1111/ecog.03331
Paquette, A., and Hargreaves, A. L. (2021). Biotic interactions are more important at species’ warm vs. cool range-edges: a synthesis. biorxiv [Preprint] doi: 10.1101/2021.04.07.438721 biorxiv 2021.04.07.438721,
Parmesan, C. (2006). Ecological and evolutionary responses to recent climate change. Annu. Rev. Ecol. Evol. Syst. 37, 637–669. doi: 10.1146/annurev.ecolsys.37.091305.110100
Parmesan, C., and Yohe, G. (2003). A globally coherent fingerprint of climate change impacts across natural systems. Nature 421, 37–42. doi: 10.1038/nature01286
Patsiou, T. S., Shestakova, T. A., Klein, T., di Matteo, G., Sbay, H., Chambel, M. R., et al. (2020). Intraspecific responses to climate reveal nonintuitive warming impacts on a widespread thermophilic conifer. New Phytol. 228, 525–540. doi: 10.1111/nph.16656
Peay, K. G. (2016). The mutualistic niche: mycorrhizal symbiosis and community dynamics. Annu. Rev. Ecol. Evol. Syst. 47, 143–164. doi: 10.1146/annurev-ecolsys-121415-032100
Pelletier, T. A., Carstens, B. C., Tank, D. C., Sullivan, J., and Espíndola, A. (2018). Predicting plant conservation priorities on a global scale. Proc. Natl. Acad. Sci. U.S.A. 115, 13027–13032. doi: 10.1073/pnas.1804098115
Pennington, L. K., Slatyer, R. A., Ruiz-Ramos, D. V., Veloz, S. D., and Sexton, J. P. (2021). How is adaptive potential distributed within species ranges? Evolution 75, 2152–2166.
Peters, M. A. E., and Weis, A. E. (2019). Isolation by phenology synergizes isolation by distance across a continuous landscape. New Phytol. 224, 1215–1228. doi: 10.1111/nph.16041
Peterson, M. L., Doak, D. F., and Morris, W. F. (2019). Incorporating local adaptation into forecasts of species’ distribution and abundance under climate change. Global Change Biol. 25, 775–793. doi: 10.1111/gcb.14562
Phillips, R. D., Peakall, R., van der Niet, T., and Johnson, S. D. (2020). Niche perspectives on plant–pollinator interactions. Trends Plant Sci. 25, 779–793. doi: 10.1016/j.tplants.2020.03.009
Pickles, B. J., Twieg, B. D., O’Neill, G. A., Mohn, W. W., and Simard, S. W. (2015). Local adaptation in migrated interior douglas-fir seedlings is mediated by ectomycorrhizas and other soil factors. New Phytol. 207, 858–871. doi: 10.1111/nph.13360
Pironon, S., Papuga, G., Villellas, J., Angert, A. L., García, M. B., and Thompson, J. D. (2017). Geographic variation in genetic and demographic performance: new insights from an old biogeographical paradigm. Biol. Rev. 92, 1877–1909. doi: 10.1111/brv.12313
Rajakaruna, N. (2018). Lessons on evolution from the study of edaphic specialization. Bot. Rev. 84, 39–78. doi: 10.1007/s12229-017-9193-2
Reed, P. B., Peterson, M. L., Pfeifer-Meister, L. E., Morris, W. F., Doak, D. F., Roy, B. A., et al. (2021). Climate manipulations differentially affect plant population dynamics within versus beyond northern range limits. J. Ecol. 109, 664–675. doi: 10.1111/1365-2745.13494
Rehfeldt, G. E., Ying, C. C., Spittlehouse, D. L., and Hamilton, D. A. (1999). Genetic responses to climate in Pinus contorta: niche breadth, climate change, and reforestation. Ecol. Monogr. 69, 375–407.
Rice, K. J., and Emery, N. C. (2003). Managing microevolution: restoration in the face of global change. Front. Ecol. Environ. 1:469–478.
Robinson, Z. L., Bell, D. A., Dhendup, T., Luikart, G., Whiteley, A. R., and Kardos, M. (2020). Evaluating the outcomes of genetic rescue attempts. Conserv. Biol. 35, 666–677. doi: 10.1111/cobi.13596
Rodriguez, R., and Durán, P. (2020). Natural holobiome engineering by using native extreme microbiome to counteract the climate change effects. Front. Bioeng. Biotechnol. 8:568. doi: 10.3389/fbioe.2020.00568
Rollinson, C. R., Finley, A. O., Alexander, M. R., Banerjee, S., Hamil, K. D., Koenig, L. E., et al. (2021). Working across space and time: nonstationarity in ecological research and application. Front. Ecol. Environ. 19:66–72. doi: 10.1002/fee.2298
Rolshausen, R., Hallman, U., Dal Grande, F., Otte, J., Knudsen, K., and Schmitt, I. (2020). Expanding the mutualistic niche: parallel symbiont turnover along climatic gradients. Proc. R. Soc. B Biol. Sci. 287:20192311. doi: 10.1098/rspb.2019.2311
Rudgers, J. A., Afkhami, M. E., Bell-Dereske, L., Chung, Y. A., Crawford, K. M., Kivlin, S. N., et al. (2020). Climate disruption of plant microbe interactions. Annu. Rev. Ecol. Evol. Syst. 51, 561–586. doi: 10.1146/annurev-ecolsys-011720-090819
Sagarin, R. D., and Gaines, S. D. (2002). The ‘abundant centre’ distribution: to what extent is it a biogeographical rule? Ecol. Lett. 5, 137–147. doi: 10.1046/j.1461-0248.2002.00297.x
Saintilan, N., Wilson, N. C., Rogers, K., Rajkaran, A., and Krauss, K. W. (2014). Mangrove expansion and salt marsh decline at mangrove poleward limits. Global Change Biol. 20, 147–157. doi: 10.1111/gcb.12341
Salariato, D. L., and Zuloaga, F. O. (2021). Ecological and spatial patterns associated with diversification of South American Physaria (Brassicaceae) through the general concept of species. Org. Diversity Evol. 21, 161–188. doi: 10.1007/s13127-021-00486-z
Scheidel, U., and Bruelheide, H. (2001). Altitudinal differences in herbivory on montane compositae species. Oecologia 129, 75–86. doi: 10.1007/s004420100695
Schemske, D. W., Mittelbach, G. G., Cornell, H. V., Sobel, J. M., and Roy, K. (2009). Is there a latitudinal gradient in the importance of biotic interactions? Annu. Rev. Ecol. Evol. Syst. 40, 245–269. doi: 10.1146/annurev.ecolsys.39.110707.173430
Schuster, W. S., Alles, D. L., and Mitton, J. B. (1989). Gene flow in limber pine: evidence from pollination phenology and genetic differentiation along an elevational transect. Am. J. Bot. 76, 1395–1403. doi: 10.1002/j.1537-2197.1989.tb15118.x
Serra-Diaz, J. M., and Franklin, J. (2019). What’s hot in conservation biogeography in a changing climate? Going beyond species range dynamics. Diversity Distrib. 25, 492–498.
Sexton, J. P., and Dickman, E. E. (2016). What can local and geographic population limits tell us about distributions? Am. J. Bot. 103, 129–139. doi: 10.3732/ajb.1500224
Sexton, J. P., Hangartner, S. B., and Hoffmann, A. A. (2014). Genetic isolation by environment or distance: which pattern of gene flow is most common? Evolution 68, 1–15. doi: 10.1111/evo.12258
Sexton, J. P., Hufford, M. B., Bateman, A. C., Lowry, D. B., Meimberg, H., Strauss, S. Y., et al. (2016). Climate structures genetic variation across a species’ elevation range: a test of range limits hypotheses. Mol. Ecol. 25, 911–928. doi: 10.1111/mec.13528
Sexton, J. P., McIntyre, P. J., Angert, A. L., and Rice, K. J. (2009). Evolution and ecology of species range limits. Annu. Rev. Ecol. Evol. Syst. 40, 415–436. doi: 10.1146/annurev.ecolsys.110308.120317
Sexton, J. P., Montiel, J., Shay, J. E., Stephens, M. R., and Slatyer, R. A. (2017). Evolution of ecological niche breadth. Annu. Rev. Ecol. Evol. Syst. 48, 183–206. doi: 10.1146/annurev-ecolsys-110316-023003
Sexton, J. P., Strauss, S. Y., and Rice, K. (2011). Gene flow increases fitness at the warm edge of a species’ range. Proc. Natl. Acad. Sci. U.S.A. 108, 11704–11709. doi: 10.1073/pnas.1100404108
Sgrò, C. M., Lowe, A. J., and Hoffmann, A. A. (2011). Building evolutionary resilience for conserving biodiversity under climate change. Evol. Applic. 4, 326–337. doi: 10.1111/j.1752-4571.2010.00157.x
Shafer, A. B. A., and Wolf, J. B. W. (2013). Widespread evidence for incipient ecological speciation: a meta-analysis of isolation-by-ecology. Ecol. Lett. 16, 940–950. doi: 10.1111/ele.12120
Sheth, S. N., Morueta-Holme, N., and Angert, A. L. (2020). Determinants of geographic range size in plants. New Phytol. 226, 650–665. doi: 10.1111/nph.16406
Skeels, A., and Cardillo, M. (2018). Reconstructing the geography of speciation from contemporary biodiversity data. Am. Natural. 193, 240–255. doi: 10.1086/701125
Slatyer, R. A., Hirst, M., and Sexton, J. P. (2013). Niche breadth predicts geographical range size: a general ecological pattern. Ecol. Lett. 16, 1104–1114. doi: 10.1111/ele.12140
Smith, T. B., Kinnison, M. T., Strauss, S. Y., Fuller, T. L., and Carroll, S. P. (2014). Prescriptive evolution to conserve and manage biodiversity. Annu. Rev. Ecol. Evol. Syst. 45, 1–22. doi: 10.1146/annurev-ecolsys-120213-091747
Song, Z., Fu, Y. H., Du, Y., and Huang, Z. (2021). Global warming increases latitudinal divergence in flowering dates of a perennial herb in humid regions across Eastern Asia. Agric. For. Meteorol. 296:108209. doi: 10.1016/j.agrformet.2020.108209
Sporbert, M., Keil, P., Seidler, G., Bruelheide, H., Jandt, U., Aćić, S., et al. (2020). Testing macroecological abundance patterns: the relationship between local abundance and range size, range position and climatic suitability among European vascular plants. J. Biogeogr. 47, 2210–2222. doi: 10.1111/jbi.13926
Stanton-Geddes, J., Tiffin, P., and Shaw, R. G. (2012). Role of climate and competitors in limiting fitness across range edges of an annual plant. Ecology 93, 1604–1613. doi: 10.1890/11-1701.1
Stebbins, G. L. (1942). The genetic approach to problems of rare and endemic species. Madroño 6, 241–258.
Stebbins, G. L. (1979). Rare species as examples of plant evolution. Great Basin Natural. Memoirs 3, 113–117.
Tallmon, D. A., Luikart, G., and Waples, R. S. (2004). The alluring simplicity and complex reality of genetic rescue. Trends Ecol. Evol. 19, 489–496. doi: 10.1016/j.tree.2004.07.003
Tanentzap, A. J., Brandt, A. J., Smissen, R. D., Heenan, P. B., Fukami, T., and Lee, W. G. (2015). When do plant radiations influence community assembly? The importance of historical contingency in the race for niche space. New Phytol. 207, 468–479. doi: 10.1111/nph.13362
Tanentzap, A. J., Igea, J., Johnston, M. G., and Larcombe, M. J. (2019). Does evolutionary history correlate with contemporary extinction risk by influencing range size dynamics? Am. Natural. 195, 569–576. doi: 10.1086/707207
Temperton, V. M., Hobbs, R. J., Nuttle, T., and Halle, S. (Eds.). (2004). Assembly Rules and Restoration Ecology: Bridging the Gap Between Theory and Practice (Vol. 5). Chicago: Island Press.
Temunović, M., Franjić, J., Satovic, Z., Grgurev, M., Frascaria-Lacoste, N., and Fernández-Manjarrés, J. F. (2012). Environmental heterogeneity explains the genetic structure of continental and Mediterranean populations of Fraxinus angustifolia vahl. PLoS One 7:e42764. doi: 10.1371/journal.pone.0042764
Tielbörger, K., and Salguero-Gómez, R. (2014). Some like it hot: are desert plants indifferent to climate change? Progress in Botany. Springer, Berlin, Heidelberg, 75, 377–400. doi: 10.1007/978-3-642-38797-5_12
Torres-Martínez, L., McCarten, N., and Emery, N. C. (2019). The adaptive potential of plant populations in response to extreme climate events. Ecol. Lett. 22, 866–874. doi: 10.1111/ele.13244
Trisos, C. H., Merow, C., and Pigot, A. L. (2020). The projected timing of abrupt ecological disruption from climate change. Nature 580, 496–501. doi: 10.1038/s41586-020-2189-9
Twyford, A. D., Wong, E. L. Y., and Friedman, J. (2020). Multi-level patterns of genetic structure and isolation by distance in the widespread plant Mimulus guttatus. Heredity 125, 227–239. doi: 10.1038/s41437-020-0335-7
Urban, M. C. (2015). Accelerating extinction risk from climate change. Science 348, 571–573. doi: 10.1126/science.aaa4984
Van der Putten, W. H., Macel, M., and Visser, M. E. (2010). Predicting species distribution and abundance responses to climate change: why it is essential to include biotic interactions across trophic levels. Philos. Trans. R. Soc. Lond. Ser B Biol. Sci. 365, 2025–2034. doi: 10.1098/rstb.2010.0037
Vázquez-García, J. A., Muñiz-Castro, M. A., Dahua-Machoa, A., Osorio-Muñoz, E. A., Hernández-Vera, G., Ortega-Peña, A. S., et al. (2021). How to save endangered magnolias? From population biology to conservation action: the case of allopatric radiation in western Mexico. Endangered Plants (London: IntechOpen), doi: 10.5772/intechopen.94346.
Vitasse, Y., Signarbieux, C., and Fu, Y. H. (2018). Global warming leads to more uniform spring phenology across elevations. Proc. Natl. Acad. Sci. U.S.A. 115, 1004–1008. doi: 10.1073/pnas.1717342115
von Humboldt, A. L., and Bonpland, A. (1807). Essai Sur La Géografie Des Plantes. Chez Levrault: Schoell.
Vtipil, E. E., and Sheth, S. N. (2020). A resurrection study reveals limited evolution of phenology in response to recent climate change across the geographic range of the scarlet monkeyflower. Ecol. Evol. 10, 14165–14177. doi: 10.1002/ece3.7011
Wadgymar, S. M., Cumming, M. N., and Weis, A. E. (2015). The success of assisted colonization and assisted gene flow depends on phenology. Global Change Biol. 21, 3786–3799. doi: 10.1111/gcb.12988
Wang, I. J. (2013). Examining the full effects of landscape heterogeneity on spatial genetic variation: a multiple matrix regression approach for quantifying geographic and ecological isolation. Evolution 67, 3403–3411. doi: 10.1111/evo.12134
Wang, I. J., and Bradburd, G. S. (2014). Isolation by environment. Mol. Ecol. 23, 5649–5662. doi: 10.1111/mec.12938
Weber, M. M., Stevens, R. D., Diniz-Filho, J. A. F., and Grelle, C. E. V. (2017). Is there a correlation between abundance and environmental suitability derived from ecological niche modelling? A meta-analysis. Ecography 40, 817–828. doi: 10.1111/ecog.02125
Whiteley, A. R., Fitzpatrick, S. W., Funk, W. C., and Tallmon, D. A. (2015). Genetic rescue to the rescue. Trends Ecol. Evol. 30, 42–49. doi: 10.1016/j.tree.2014.10.009
Willi, Y., van Kleunen, M., Dietrich, S., and Fischer, M. (2007). Genetic rescue persists beyond first-generation outbreeding in small populations of a rare plant. Proc. R. Soc. Biol. Sci. 274, 2357–2364. doi: 10.1098/rspb.2007.0768
Williams, B. K. (2011). Adaptive management of natural resources—framework and issues. J. Environ. Manag. Adaptive Manag. Natural Resour. 92, 1346–1353. doi: 10.1016/j.jenvman.2010.10.041
Wisz, M. S., Pottier, J., Kissling, D. W., Pellissier, L., Lenoir, J., Damgaard, C. F., et al. (2013). The role of biotic interactions in shaping distributions and realised assemblages of species: implications for species distribution modelling. Biol. Rev. 88, 15–30. doi: 10.1111/j.1469-185X.2012.00235.x
Wolkovich, E. M., Cook, B. I., Allen, J. M., Crimmins, T. M., Betancourt, J. L., Travers, S. E., et al. (2012). Warming experiments underpredict plant phenological responses to climate change. Nature 485, 494–497. doi: 10.1038/nature11014
Wooliver, R., Tittes, S. B., and Sheth, S. N. (2020). A resurrection study reveals limited evolution of thermal performance in response to recent climate change across the geographic range of the scarlet monkeyflower. Evolution 74, 1699–1710. doi: 10.1111/evo.14041
Young, A., Boyle, T., and Brown, T. (1996). The population genetic consequences of habitat fragmentation for plants. Trends Ecol. Evol. 11, 413–418. doi: 10.1016/0169-5347(96)10045-8
Zettlemoyer, M. A., McKenna, D. D., and Lau, J. A. (2019). Species characteristics affect local extinctions. Am. J. Bot. 106, 547–559. doi: 10.1002/ajb2.1266
Keywords: species range limits, biotic interactions, local adaptation, gene flow, range size, management, climate change
Citation: Shay JE, Pennington LK, Mandussi Montiel-Molina JA, Toews DJ, Hendrickson BT and Sexton JP (2021) Rules of Plant Species Ranges: Applications for Conservation Strategies. Front. Ecol. Evol. 9:700962. doi: 10.3389/fevo.2021.700962
Received: 27 April 2021; Accepted: 10 September 2021;
Published: 25 October 2021.
Edited by:
Amy L. Angert, University of British Columbia, CanadaReviewed by:
Megan Bontrager, University of Toronto, CanadaLesley Lancaster, University of Aberdeen, United Kingdom
Copyright © 2021 Shay, Pennington, Mandussi Montiel-Molina, Toews, Hendrickson and Sexton. This is an open-access article distributed under the terms of the Creative Commons Attribution License (CC BY). The use, distribution or reproduction in other forums is permitted, provided the original author(s) and the copyright owner(s) are credited and that the original publication in this journal is cited, in accordance with accepted academic practice. No use, distribution or reproduction is permitted which does not comply with these terms.
*Correspondence: Jackie E. Shay, anNoYXlAdWNtZXJjZWQuZWR1