- 1Department of Integrative Biology, Oregon State University, Corvallis, OR, United States
- 2Department of Neurobiology, Physiology and Behavior, University of California, Davis, Davis, CA, United States
- 3Department of Biology, Southern Oregon University, Ashland, OR, United States
- 4School of Biological Sciences, Washington State University, Pullman, WA, United States
- 5Department of Biology, Eastern Michigan University, Ypsilanti, MI, United States
Physiological preparations for migration generally reflect migratory strategy. Migrant birds fuel long-distance flight primarily with lipids, but carrying excess fuel is costly; thus, the amount of fat deposited prior to departure often reflects the anticipated flight duration or distance between refueling bouts. Seasonal pre-migratory deposition of fat is well documented in regular seasonal migrants, but is less described for more facultative species. We analyze fat deposits of free-living birds across several taxa of facultative migrants in the songbird subfamily Carduelinae, including house finches (Haemorhous mexicanus), American goldfinches (Spinus tristis), pine siskins (Spinus pinus) and four different North American ecotypes of red crossbills (Loxia curvirostra), to evaluate seasonal fat deposition during facultative migratory periods. Our data suggest that the extent of seasonal fat deposits corresponds with migratory tendency in these facultative taxa. Specifically, nomadic red crossbills with a seasonally predictable annual movement demonstrated relatively large seasonal fat deposits coincident with the migratory periods. In contrast, pine siskins, thought to be more variable in timing and initiation of nomadic movements, had smaller peaks in fat deposits during the migratory season, and the partial migrant American goldfinch and the resident house finch showed no peaks coincident with migratory periods. Within the red crossbills, those ecotypes that are closely associated with pine habitats showed larger peaks in fat deposits coincident with autumn migratory periods and had higher wing loading, whereas those ecotypes associated with spruces, Douglas-fir and hemlocks showed larger peaks coincident with spring migratory periods and lower wing loading. We conclude that population averages of fat deposits do reflect facultative migration strategies in these species, as well as the winter thermogenic challenges at the study locations. A difference in seasonal fattening and wing loading among red crossbill ecotypes is consistent with the possibility that they differ in their migratory biology, and we discuss these differences in light of crossbill reproductive schedules and phenologies of different conifer species.
Introduction
Every year billions of birds migrate to track resources or to mitigate the impact of challenging environmental conditions on survival and reproduction. In most migratory birds, these movements occur largely through long periods of flapping flight (Hedenström, 1993) – an energetically demanding mode of locomotion that can drive the instantaneous costs of migration upward of 10 times the basal metabolic rate for extended periods of time [reviewed in Guglielmo (2018)]. Migratory flight is thus energetically expensive and requires substantial fuel stores to power the sustained muscle activity, especially given that most migratory birds cannot forage while flying.
The energetic demands of flight shape fueling strategies in migrant birds (McWilliams et al., 2004). Fats contain 8 to 10 times as much energy per unit mass as compared to carbohydrates and proteins, in large part due to their hydrophobic nature. Fatty acids are, thus, the primary fuel utilized during migratory flight in birds (Blem, 1980; Ramenofsky, 1990; Bairlein, 2002; Guglielmo, 2018). Birds store some fats directly in working myocytes [i.e., muscle cells; (Marsh, 1984; Napolitano and Ackman, 1990)], but much of the energy required for long distance flight is mobilized from triacylglycerides stored in adipocytes [i.e., fat storage cells; (Blem, 1976; Ramenofsky et al., 1999; Maillet and Weber, 2006)]. Adipocytes are concentrated in a variety of locations in the body, though subcutaneous fat depots are the primary area of storage (Blem, 1976; Maillet and Weber, 2006). The amount of fat stored in extra-muscular deposits is generally thought to reflect the perceived risk of failing to meet energy demands balanced by the risk of increased flight costs or decreased performance when carrying extra fat mass (Videler et al., 1988; McNamara and Houston, 1990; Witter and Cuthill, 1993; Kullberg et al., 1996). For example, birds that have recently experienced unstable food resources (Lehikoinen, 1986; Rogers, 1987; Houston and McNamara, 1993; Bednekoff and Krebs, 1995), high thermoregulatory costs (Helms and Drury, 1960; Newton, 1969; Ekman and Hake, 1990) or long periods of fasting (Kendeigh, 1969; Vincent and Bédard, 1976; Haftorn, 1989) may carry larger fat deposits, whereas high predator density can have the opposite effect (Blem, 1975; Lima, 1986; Pravosudov and Grubb, 1998; Gentle and Gosler, 2001; Lind and Cresswell, 2006; Pascual and Senar, 2015).
Premigratory fat deposits in migrant birds generally reflect the anticipated flight duration prior to refueling opportunities, but can also reflect other aspects of migratory ecology (Odum, 1960; Alerstam and Lindstrom, 1990; Weber et al., 1994). For example, migrants that fly over barriers without refueling opportunities must carry more fat than those that make frequent stopovers for refueling (Blem, 1980; Alerstam and Lindstrom, 1990; Newton, 2008) and migrants may carry more fat than is necessary to provide a buffer for coping with uncertain food resources during stopover or at the breeding grounds (Pettersson and Hasselquist, 1985; Nielsen and Rees, 2013). However, those migrants that face high predation pressure may carry less fat (Alerstam and Lindstrom, 1990) and take longer to replenish fat stores at stopover sites (Cimprich et al., 2005; Schmaljohann and Dierschke, 2005). Further, those birds under pressure for a timely arrival at breeding locations (e.g., high latitude breeders and territorial spring breeders, etc.) may also take more risks during fueling and depart with lower fat stores compared to those that instead take the time to optimize energy costs throughout the journey (Alerstam and Lindstrom, 1990; Wojciechowski et al., 2014). While there exists a relatively rich body of research investigating how ecological and physiological contexts affect fattening in traditional, seasonal to-and-fro migrants [i.e., obligate migrants, reviewed in Blem (1976, 1980), Ramenofsky (1990); Bairlein and Gwinner (1994), Biebach (1996); Jenni and Jenni-Eiermann (1998), Bairlein (2002); McWilliams et al. (2004), Newton (2008); Weber (2009), Guglielmo (2010, 2018), Price (2010), and Pierce and McWilliams (2014)], there are fewer accounts of how fat deposits fluctuate in facultative types of migrations that occur less predictably in space and/or time, as in nomadic and irruptive species and partial migrants.
Facultative migrants may deposit smaller fat stores prior to or during migration than most obligate migrants, though data are much more limited (Alerstam and Lindstrom, 1990). Irruptive and nomadic migrations are thought to occur largely in response to low food resources, often combined with high population density (Newton, 2006a). Yet these movements are often seasonal, as in many boreal seed-eating species (Newton, 2006b; Benkman and Young, 2020; Dawson, 2020), some insectivores [e.g., long-tailed tit (Aegithalos caudatus); (Bojarinova and Babushkina, 2015)] and some frugivores [e.g., waxwings (Bombycilla spp.); (Witmer, 2020)]. The responsiveness of facultative migrants to dwindling food resources has led some to speculate that facultative migrants may struggle to meet energy demands during migration or to store sufficient fat (Tiainen, 1980; Silverin, 2003), but others challenge that hypothesis given that facultative migrants often carry large fat deposits and maintain high body mass even during mass irruptions when resources should be most limiting (Bojarinova and Babushkina, 2010). Seasonal cycles in fat stores consistent with premigratory fattening have been observed in populations of facultative migrants under both natural conditions (Newton, 1972; Summers et al., 1996; Marquiss and Rae, 2002; Cornelius and Hahn, 2012); but see Alonso and Arizaga (2011) and controlled captive conditions with unlimited food (Berthold and Gwinner, 1978; Hahn, 1995; Babushkina and Bojarinova, 2011; Newton and Dawson, 2011; Cornelius and Hahn, 2012; Robart et al., 2018). Further, the timing of premigratory fattening in the irruptive long-tailed tit and pine siskin (Spinus pinus) responds to experimental manipulations of photoperiod in captivity (Babushkina and Bojarinova, 2011; Robart et al., 2018), although apparently not in Eurasian siskins (Spinus spinus) (Newton and Dawson, 2011). These studies suggest that even those species with highly flexible migratory behaviors may sometimes utilize endogenous programs and predictive cues to prepare for movements. Given that many facultative migrants do not have consistent migratory routes and destinations across years, fattening may reflect insurance against uncertainty about refueling opportunities. In partial migrant systems, movements may be programmed and consistent, but only in particular populations [e.g., more northerly populations: Linnets (Linaria cannabina; Stey et al., 2017); goldcrests (Regulus regulus; Bojarinova et al., 2008) or subsets of populations (e.g., females and juveniles in European blackbirds (Turdus merula; Lundberg, 1985; Fudickar et al., 2013) and dark-eyed juncos (Junco hyemalis; Holberton, 1993)]. The migratory individuals in these populations show more fattening during the migratory season than do their resident counterparts (Lundberg, 1985; Jahn et al., 2010; Fudickar et al., 2013), but see Holberton (1993). Facultative migrants may thus share some similarities in premigratory physiology with obligate migrants, but exhibit a higher degree of variation at the population level.
Hypotheses and Predictions
We hypothesize that the degree of seasonal fattening observed in a facultative species is associated with variation in timing or prevalence of facultative movements across individuals and populations. We test this hypothesis with long-term datasets from a subfamily of songbirds with variable facultative migration strategies, the cardueline finches, including: house finches (Haemorhous mexicanus), American goldfinches (Spinus tristis), pine siskins and four different North American ecotypes of red crossbills (Loxia curvirostra) to determine if average fat deposits change seasonally and match described migratory patterns.
House finches are resident and experience relatively mild winters at our study locations in California (Table 1), thus we predict little or no seasonal fluctuations in fat deposits. American goldfinches are partial migrants at our study locations and the birds that remain in winter experience cold conditions, thus we expect average fat deposits to be larger in wintering goldfinches than in goldfinches captured during the migratory season – which presumably includes migrants intent on traveling variable distances and those birds that remain resident. Pine siskins are irruptive and nomadic with highly variable migratory behavior but with movements centered in fall, peaking in September and October, and in spring, peaking in April and May (Dawson, 2020), although they may move at other times of year less regularly (Palmer, 1968). Moreover migrant and/or transient pine siskins can co-occur with individuals in a resident life cycle stage (e.g., breeding; Granlund et al., 1994; also observed in Eurasian siskins Senar et al., 1992). We therefore predict fat deposits to show high variability during the migratory season in pine siskins and for fat deposits to also be large and comparatively less variable during the cold winter months. Red crossbills are nomadic migrants that commonly initiate movements in May and June to locate newly developing cone crops (Bielefeldt and Rosenfield, 1994; Summers et al., 1996; Marquiss and Rae, 2002; Newton, 2006b; Brady et al., 2019; Gatter and Gatter, 2019; Nothdurft, 2019) and these movements can transition into irruptions later in the summer and fall if no sufficient cone crops are encountered (Newton, 1972, 2006b; Brady et al., 2019; Benkman and Young, 2020). Additional facultative movements commonly occur in the fall in North America (Brady et al., 2019; Benkman and Young, 2020) and have been observed in Europe (Gatter and Gatter, 2019) if seed crops disperse from cones at high rates or birds seek better conditions elsewhere between breeding seasons (Marquiss and Rae, 2002). We therefore predict fat deposits to be high during migratory periods in red crossbills. Winter fattening in crossbills may be complicated by opportunistic winter breeding, if breeding birds carry less fat than nonbreeders as in some other species (Freed, 1981; Krause et al., 2016). We therefore predict migratory fat to exceed winter fat, when evaluated on average across years.
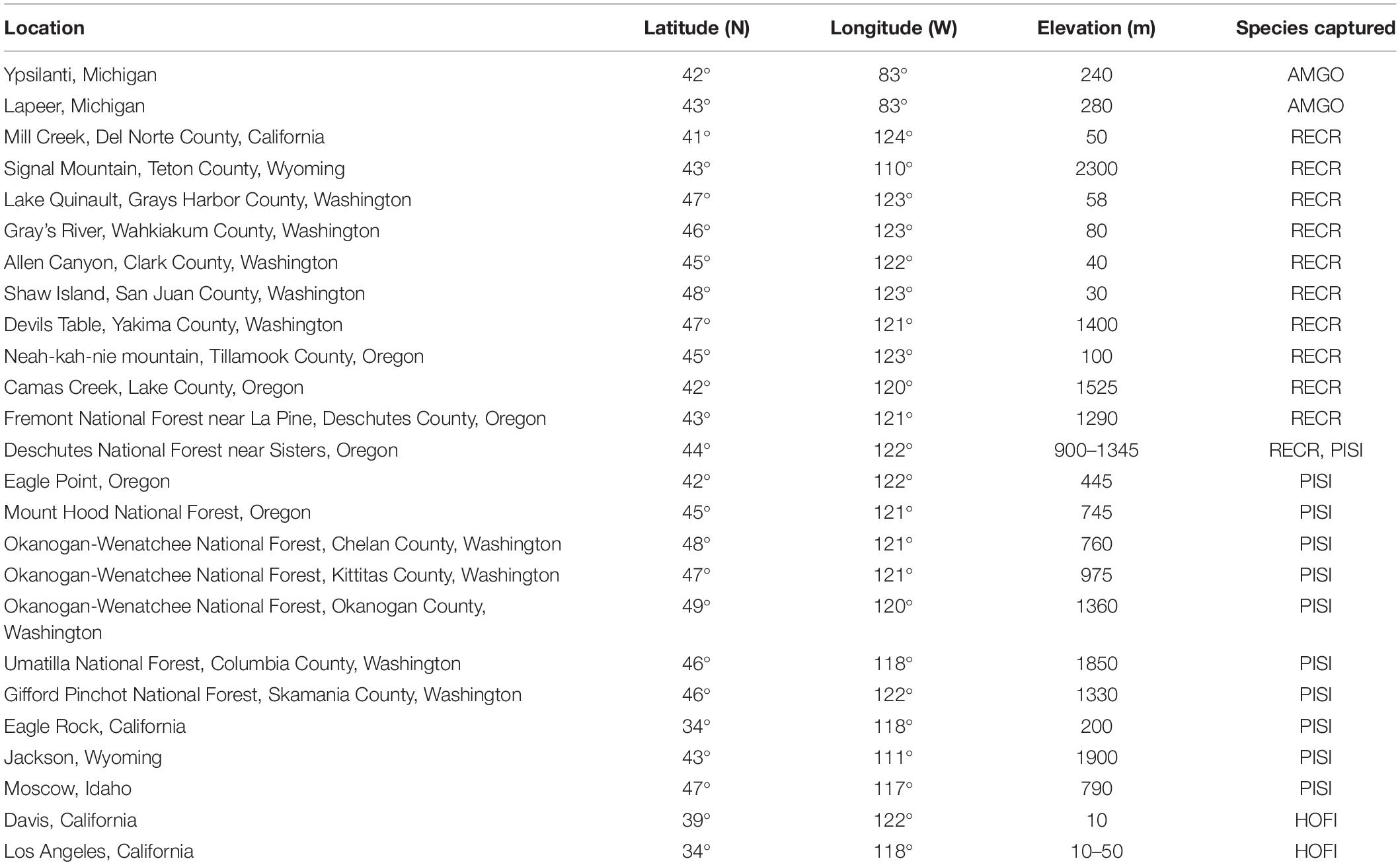
Table 1. Primary capture locations in the United States for American goldfinches (AMGO), red crossbills (RECR), pine siskins (PISI), and house finches (HOFI).
Red crossbills occur as different ecotypes that appear to be adapted morphologically to different conifer species (Benkman, 1993). Differences in conifer seed phenology may therefore drive differences in migratory preparations and morphology in the crossbill ecotypes that feed on them. Thus, we predict ecotypes that forage efficiently on conifers with more consistent annual seed production and retention to show relatively less fattening and ecotypes that forage primarily on species with less annual consistency to show relatively greater fattening. In our study area, lodgepole pine (Pinus contorta) has relatively consistent annual production of cone crops in the spring and like other pines has hard cones that open relatively slowly and later, whereas the softer cones of spruces, Douglas firs and hemlocks are more prone to rapid seed shedding (Ruth and Berntsen, 1955; Fowells, 1968; Smith and Balda, 1979; Benkman, 1987; Hahn, 1998; Marquiss and Rae, 2002; Mezquida et al., 2018). We therefore predict that red crossbills will show seasonal peaks in fat deposits in late spring and again in the fall that are more defined in ecotype 3 (closely associated with hemlocks and spruces) and ecotype 4 (closely associated with Douglas-fir and spruces) than in ecotype 2 (closely associated with ponderosa pine) and ecotype 5 (closely associated with lodgepole pine) (Benkman, 1993; Kelsey, 2008). As a further test of whether any ecotype differences in fattening likely reflect differences in migratory strategies, we also examine mass to wing chord quotients – as migrants are expected to have lower wing loading to reduce flight costs (Norberg, 1995; Bowlin and Wikelski, 2008). Thus, we expect that more nomadic ecotypes will have longer wings (and smaller mass to wing chord quotients). We evaluate seasonal patterns in fat deposits in relation to described annual schedules of each species and ecotype to better understand how fat deposits relate to facultative patterns of migration.
Materials and Methods
Study Sites
Sites were chosen based on availability of good capture locations within favorable habitat and the presence of target species (Figure 1 and Table 1). American goldfinches were captured at study sites throughout Michigan, with the majority of captures occurring at sites in southeast Michigan. Red crossbills and pine siskins were captured at study sites in Washington, Oregon, Wyoming, and California, with additional pine siskin captures in Idaho. House finches were captured in California, thus house finches were generally captured at more southerly locations than the other species.
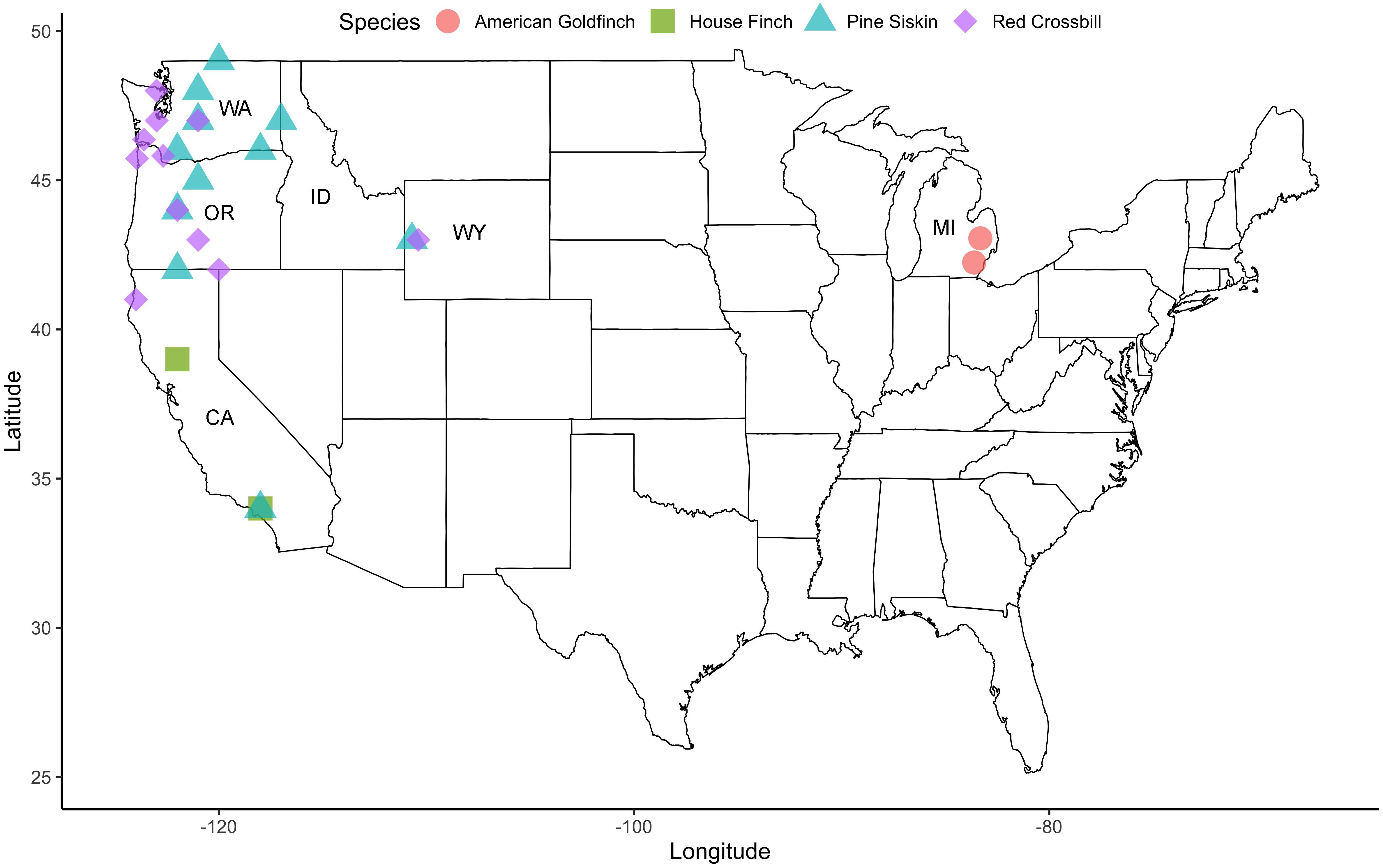
Figure 1. Map of main capture locations for each study species in the United States of America. State borders are shown with states where birds were captured labeled (WA, Washington; OR, Oregon; CA, California; ID, Idaho; WY, Wyoming; MI, Michigan).
Capture and Sampling
Data were collected from adult male and female birds captured at our field sites between 1987 and 2021. During this time we lured 1,352 adult red crossbills, 418 adult pine siskins, 272 adult American goldfinches and 148 adult house finches into mist nets using live decoys or into feeder traps baited with thistle or sunflower seed (Supplementary Table 1 describes captures by year and month). Juveniles were excluded to avoid age-related complications in timing or propensity of migratory movements in partial migrant species (Prescott and Middleton, 1990), because of potential age-related differences in fat deposits among first year birds, and because it is possible that fattening is limited by foraging ability in juvenile red crossbills during bill development (Jamie M. Cornelius, unpublished observations). We had no pine siskin recaptures during this study and only one house finch recapture (note that approximately 40% of the house finches captured in this study were retained for captive experiments). Twenty-four red crossbills were recaptured once during this study (i.e., approximately 1% of all captures) and 16 American goldfinches were recaptured between one and three times each (i.e., approximately 8% of all captures). We excluded data from recaptured birds given that there were few recaptures and the majority of these occurred within several weeks of the initial capture. For red crossbills, vocalizations were recorded to identify call type either when the bird was approaching the net or when it called upon release (see Supplementary Table 2 for sample sizes of ecotypes across life cycle stages). Spectrograms were created to assist with assigning type if there was uncertainty. Crossbills that did not vocalize were not assigned a call type and were excluded from type-specific analyses (N = 84). All birds were sexed and aged according to plumage, bill and skull characteristics or reproductive characteristics (Pyle et al., 1997). Birds for which characteristics were not sexually dimorphic at the time of capture (e.g., non-breeding pine siskins) were either molecularly sexed (as in Robart et al., 2018) or categorized as unknown sex and excluded from these analyses.
Wing loading was estimated as the quotient of body mass to wing chord length in lean red crossbills (i.e., individuals with a fat score of 0; see below). Mass was measured by spring scale to the nearest 0.5 g and unflattened wing chord length was measured with dial calipers to the nearest 0.1 mm in red crossbills. While wing shape may be the ideal metric to measure wing loading in migrants (Minias et al., 2015; Buler et al., 2017), wing chord length can predict wing shape in some passerines (Blem, 1975; Lo Valvo et al., 1988), and relates to migratory ecology in many species (Lo Valvo et al., 1988; Marchetti et al., 1995; Boyle, 2008).
Fat Deposits
To assess fueling, we focus on the size of subcutaneous fat deposits. This provides a more direct measure of fuel stores than measures based on body mass, which is known to fluctuate seasonally independently of fat stores (e.g., due to changes in pectoralis muscle and heart size, egg laying or winter thermogenesis) (Ward and Jones, 1977; O’Connor, 1995; Liknes and Swanson, 2011). Subcutaneous fat deposits were scored in the furcular and abdominal cavities on a scale from 0.0 (no fat evident) to 5.0 (gross bulging fat deposits) based on descriptions in Helms and Drury (1960), and used previously in red crossbills and pine siskins specifically (Hahn, 1998; Cornelius and Hahn, 2012; Robart et al., 2018). Briefly, furcular (aka tracheal pit) fat deposits were scored as a 0 if no fat was visible in the pit, a 1 if there was a line of fat at the bottom of the pit, a 2 if fat covered the sides of the pit, a 3 if the fat deposits filled the pit, a 4 if the fat bulged above the pit, and a 5 if fat spilled across the pectoral muscle posterior to the furcular pit. Abdominal fat deposits were scored as a 0 if no fat was visible, a 1 if fat lined the posterior edge of the rib cage, a 2 if fat was visible amongst the intestinal tract, a 3 if fat covered the abdominal cavity, a 4 if fat bulged above the abdominal region and a 5 if fat spilled across the pectoral muscle anterior to the abdominal region. Fat scores from the two regions were summed for analysis. JC and HW were trained in fat scoring during long-term field and captive studies by TH. Other authors were subsequently trained by JC and HW. All participants were trained using the protocol described above; however, different species were generally scored by a subset of the authors on this article: red crossbill data were collected by JC and TH, pine siskin data were collected by HW, AR, BV, and JC. American goldfinch data were collected by JC, DZ., KG, and CN, and house finch data were collected by HW. Although most authors on this article score fat deposits to a much finer resolution than the whole number scale described above (i.e., to the 0.5 or 0.25 resolution), we round fat scores to the nearest whole number to minimize variability between observers. Systematic observer bias was also checked for in our data as described in the section “Statistical Analysis” below and by comparing observer scores of fat deposits collected from photographs of abdominal (N = 9) or furcular (N = 11) fat deposits of various species.
Fat Deposits in Relation to Timing of Breeding, Molt and Migration
Annual cycles of fat deposits were analyzed by calendar month, but we also assigned months to seasonal life cycle stages of breeding, molt, migration and overwintering based on published species accounts (Badyaev et al., 2020; Benkman and Young, 2020; Dawson, 2020; McGraw and Middleton, 2020; see section “Results”). While sure to introduce error, binning by life cycle stages allows us to condense our data into fewer bins and gives us the necessary power to include interaction terms between life cycle stage and sex in our models (see statistics below). Where possible we used morphological data collected in our study to evaluate the accuracy of the assignment of life stages within the annual schedules of each species (see below)
Reproductive timing was estimated using changes in cloacal protuberance (CP) length in males (Bailey, 1952; Wolfson, 1952). CP length increases in response to elevated testosterone from the testes and offers a non-invasive estimate of reproductive condition in male songbirds (Hegner and Wingfield, 1986). CP lengths were averaged and compared across months and breeding was determined to be prevalent in those months where average CP length exceeded the grand mean for CP length across the year. Use of CP length avoids seasonal biases that can occur when using brood patch prevalence if females are required to sit tighter on nests during colder conditions (e.g., winter breeding in red crossbills or early spring breeding in pine siskins).
Replacement of the primary flight feathers proceeds sequentially from wrist to wingtip during the complete, annual pre-basic molt in finches. We scored primary molt progress by recording the outermost feather that was actively growing at the time of capture for each individual, excluding the small tenth primary. The initial stages of primary molt can overlap with breeding in finches and the later stages of molt with migration (Cornelius et al., 2011). Thus, we assigned any months in which there was overlap as either breeding or migration and only assigned as molt those months in which primary molt occurred independently of any other stage.
Statistical Analysis
All statistical analyses were performed in JMP Professional v.14.0 and data and model residuals were analyzed to ensure that model assumptions were not violated. All models included random and fixed effects thus we used linear mixed models. Prior to statistical analyses we checked for inter-observer variability in fat scoring within a given species and month of capture by ANOVA and second by using a least squares model to determine if observer identification or photograph number described variability in fat scores. These tests suggested low inter-individual variability in fat scoring (see results below).
Seasonal Patterns of Fat Deposits Across Species
Seasonal patterns of fat deposits were analyzed by calendar month and by life cycle stage – which were constructed for each species based upon published data and capture data in this study (see section “Results”). To explore seasonal variation in fat deposits we first used a linear mixed model (LMM) fit by restricted maximum likelihood and constructed separately for each species with month (ordinal), sex (categorical), and time of day (continuous) as fixed effects. Fat deposits may vary by year or location in response to weather or other environmental fluctuations. We therefore also included year and location in the model as random effects. For red crossbills and pine siskins we had enough samples when binned by month to also include an interaction term between month and sex, but could not do so for house finches and American goldfinches without saturating the model due to smaller sample sizes. Significant month to month changes in the size of fat deposits were identified from the fixed effect parameter estimates which compared adjacent months (Supplementary Table 1) and peaks and valleys in fat deposits across the year were identified using Tukey pairwise comparison of means as the months with the highest or lowest mean fat scores that were significantly different from months with medial fat scores.
Second, we constructed LMMs as described above but replaced month with life cycle stage as the seasonal effect variable (categorical) and included a stage∗sex interaction term. Non-significant interaction terms were removed from models (as described below) to recover degrees of freedom and restore conditional effects to main effects. We again identified stages with different fat deposits using fixed effect parameter estimates with overwintering as the comparison group and identified peaks and valleys using Tukey comparison of means (Supplementary Table 2).
Seasonal Patterns of Fat Deposits Across Red Crossbill Ecotypes
We did not analyze type differences by month due to sample size limitations that prevented the inclusion of an interaction term between month and type in the model. To analyze seasonal patterns of fat deposits between crossbill types, we constructed a LMM with time of day, crossbill type, life cycle stage and an interaction between type and life cycle stage as fixed effects, and with year as a random effect. Location was not included as a random effect in this model because it is correlated with ecotype. Type-specific seasonal patterns were further compared by identifying peaks and valleys in fat deposits using Tukey comparisons of means.
Wing Loading Among Red Crossbill Ecotypes
Wing loading (i.e., lean body mass / wing chord length) was compared among red crossbill ecotypes using ANOVA, followed by post-hoc Tukey comparisons of means.
Results
Tests of Interobserver Variation in Fat Scoring
We found no significant differences between average observer scores of fat deposits in any species month (ANOVA, P > 0.05), except for the month of October in pine siskins (P < 0.0001). Fat scores of pine siskins captured in October were lower in 2020 when measured by BV than in 2018 or 2019 when measured by HW. However, average fat scores did not differ between these observers in any other months, indicating that the difference observed in October was more likely due to either year or location variability rather than systematic observer bias. Further, there was very low interindividual variation in the fat scores collected from photographs (average standard deviation = 0.35, range 0 – 0.6). A least squares model found that picture ID predicted fat scores with very high accuracy (F19,129 = 86.1, P < 0.0001, R2 = 0.93), and the addition of observer did not improve the fit (R2 = 0.93) (Supplementary Figure 1).
Determination of Life Cycle Stages in Each Species
A detailed description and discussion of the results for the determination of life cycles stages in each species is available in the Supplementary Material. Assignment of captures to life cycle stages for all species are summarized in Table 2.
Seasonal Patterns of Fat Deposits by Month
Month was a significant effect in models describing fat deposits in American goldfinches (F11,132 = 6.9, P < 0.0001), pine siskins (F9,5.3 = 9.0, P = 0.01), and red crossbills (F11,495 = 10.7, P < 0.0001), but not in house finches (F10,62 = 0.58, P = 0.83) (Figure 2 and Supplementary Table 3). Tukey comparisons of means suggest that fat deposits in American goldfinches peaked during February in the winter and were lowest in the spring, which was similar to the more subtle (and non-significant) seasonal pattern observed in house finches. Pine siskin fat deposits also peaked in winter, were lowest during breeding and were intermediate during the migratory periods. Red crossbills, on the other hand, had peak fat deposits in spring and fall, when movements typically occur, and the smallest fat deposits in the summer and winter, when breeding often occurs. Time of day significantly predicted fat deposits in all species (P < 0.0001 for all four species). Fat deposits increased linearly with time of day in all species (linear regression P < 0.0001), though variation was high around the best fit line (red crossbill r2 = 0.02; pine siskin r2 = 0.03; house finch r2 = 0.18; American goldfinch r2 = 0.08). The interaction term between sex and month was not significant in models explaining fat deposits in red crossbills or pine siskins (P = 0.2 and 0.3, respectively) and the interaction term was thus removed from the models. American goldfinches and red crossbills exhibited significant sex differences in fat deposits (American goldfinch F1,218 = 28.9, P < 0.0001; red crossbill F1,1069 = 27.5; P < 0.0001) but house finches and pine siskins did not (P = 0.8, and 0.3, respectively). Females had larger fat deposits than males in American goldfinches and red crossbills – though sex alone explained little of the variation (American goldfinch t1, 237 = –4.3; P < 0.0001, r2 = 0.07; red crossbill t1, 1203 = −6.0; P < 0.0001, r2 = 0.03).
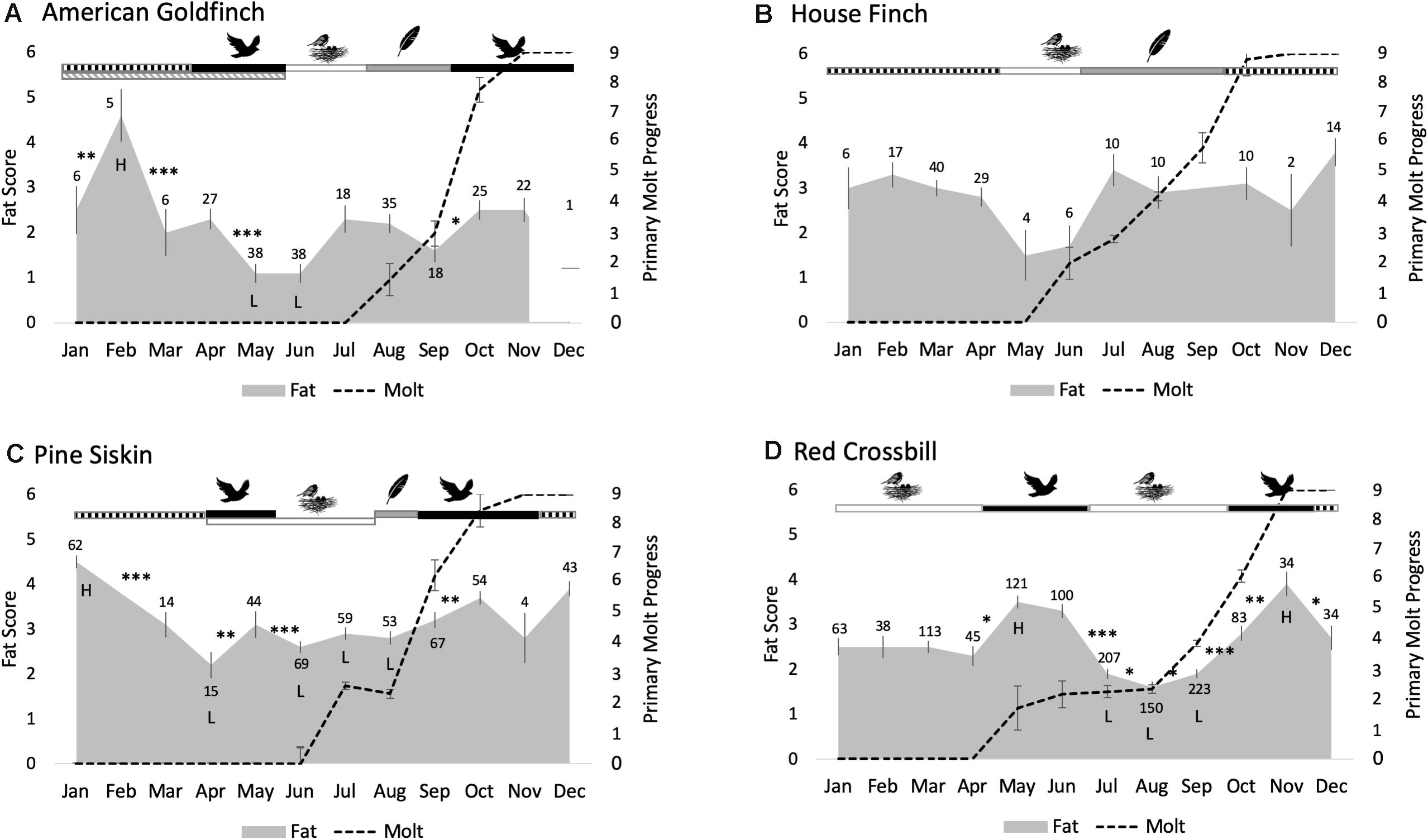
Figure 2. Changes in fat and molt progress across month and life cycle stages in free-living American goldfinches (A), house finches (B), pine siskins (C), and red crossbills (D). Average fat score (gray contour plots) +/– S.E.M. and average number of primaries that have been replaced (dashed line) +/– SEM given for each calendar month. Significant changes in fat score between consecutive months detected by the LMM are indicated by asterisks (∗P < 0.05, ∗∗P < 0.001, ∗∗∗P < 0.0001) and the highest and lowest levels are indicated by the letters H and L (identified by Tukey comparison of means P < 0.05). Dominant life cycle stages for each month are shown above the plots, including: overwintering (hatched black bars), migration (black bars), breeding (open bars), prealternate molt (hatched gray), and periods with exclusive primary molt (gray bars). Sample sizes are indicated above each monthly average.
Seasonal Patterns of Fat Deposits Across Species by Life Cycle Stages
Life cycle stage significantly predicted fat deposits in American goldfinches (F4,39 = 4.9, P = 0.003), pine siskins (F4,26 = 8.8, P = 0.0001), and red crossbills (F4,334 = 20.6, P < 0.0001), but not in house finches (F2,97 = 0.65, P = 0.52) (Supplementary Table 4). Tukey comparisons of means identified a peak in fat deposits in American goldfinches during the overwintering stage and a valley during the spring migratory and summer breeding stages. Again, this was similar to the more-subtle and non-significant pattern observed in house finches. Pine siskin fat deposits also peaked during the overwintering phase and were lowest in breeding. Fat deposits were intermediate during molt and both migratory stages, but fat deposits in spring migration were indistinguishable from breeding (P = 0.97). Red crossbills, on the other hand, had peak fat deposits during spring and fall migration stages and the smallest fat deposits during breeding – which included both summer and winter breeding. The overwintering stage included only the month of December for red crossbills and fat deposits during this stage were intermediate and weakly distinguishable from either migration (P = 0.06) or breeding stages (P = 0.09). Time of day again significantly predicted fat deposits in all species (P < 0.0001 for all four species; patterns are described above in month analysis). The interaction term between sex and life cycle stage was not significant and was removed from all models (American goldfinch P = 0.3; house finch P = 0.1; pine siskin P = 0.6 and red crossbill P = 0.3). Sex significantly predicted fat deposits in American goldfinches and red crossbills (P < 0.0001 for both species; patterns as described above in month analysis), but not in house finches and pine siskins (P > 0.5 for both species).
Seasonal Patterns of Fat Deposits Across Red Crossbill Ecotypes
The interaction between type and life cycle stage significantly predicted fat deposits in red crossbills (life cycle stage∗type F12,783 = 5.8; P < 0.0001; type F3,858 = 0.5; P = 0.7; life cycle stage F4,688 = 8.9; P < 0.0001). Fat deposits fluctuated seasonally with a peak during spring migration and a low during breeding in types 3 and 4 (P < 0.0001 and P = 0.0001; respectively), and the pattern was similar in type 2 – although fat deposits peaked during fall migration, and spring migration fat deposits were not significantly higher than breeding (P = 0.7) (Figure 3). Type 5s had lower fat deposits during spring migration compared to summer breeding (P = 0.01), winter breeding (P < 0.0001) and fall migration (P < 0.0001), but not overwintering (P = 0.9) (Figure 3).
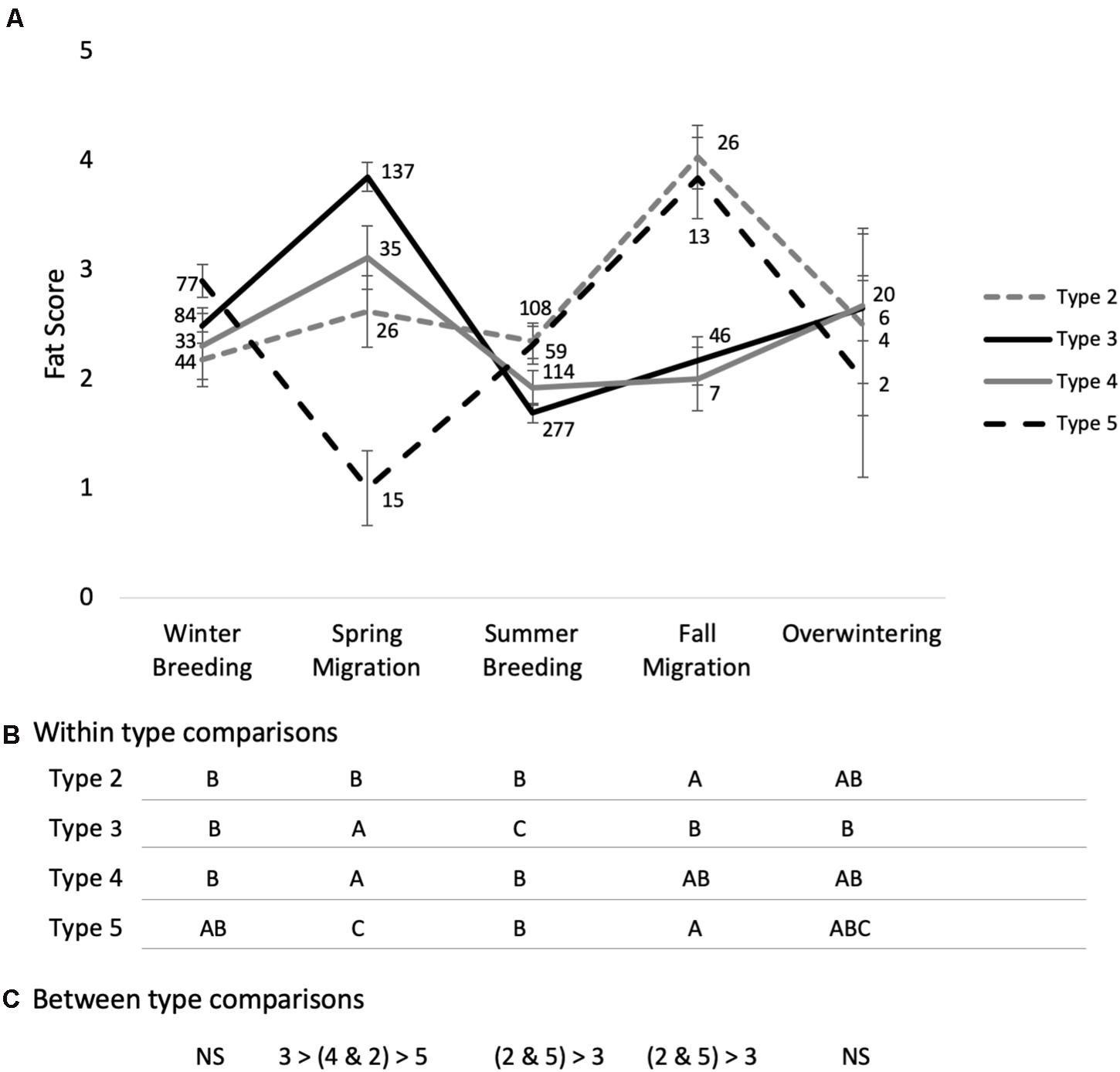
Figure 3. Fat deposits by life cycle stage in free-living red crossbill ecotypes 2, 3, 4, and 5. Fat deposits increased from winter breeding to spring migration in types 3 and 4, but declined or didn’t change in pine-associated types 5 and 2, respectively (A). The pattern flipped between summer breeding and fall migration, where pine-associated types showed larger fat deposits than types 3 and 4. There were no type differences in winter. Comparisons of life cycle stages within types (B). Different letters indicate life cycle stages that are significantly different based on Tukey comparison of means P < 0.05. Comparison between types within life cycle stages based on Tukey comparison of means P < 0.05 (C). Sample sizes given in (A), error bars denote SEM.
Wing-Loading in Red Crossbill Ecotypes
The quotient of body mass to wing chord length in lean red crossbills varied significantly among types (ANOVA F3,189 = 63.2, P < 0.0001, r2 = 0.5; Figure 4). Wing loading was lowest in type 3 ( = 0.319 + 0.002 SEM, N = 96), intermediate in type 4 ( = 0.347 + 0.003 SEM, N = 51) and type 5 ( = 0.349 + 0.006 SEM, N = 9) and highest in type 2 ( = 0.367+0.003 SEM, N = 34) (ordered differences by Tukey comparisons of means P < 0.05).
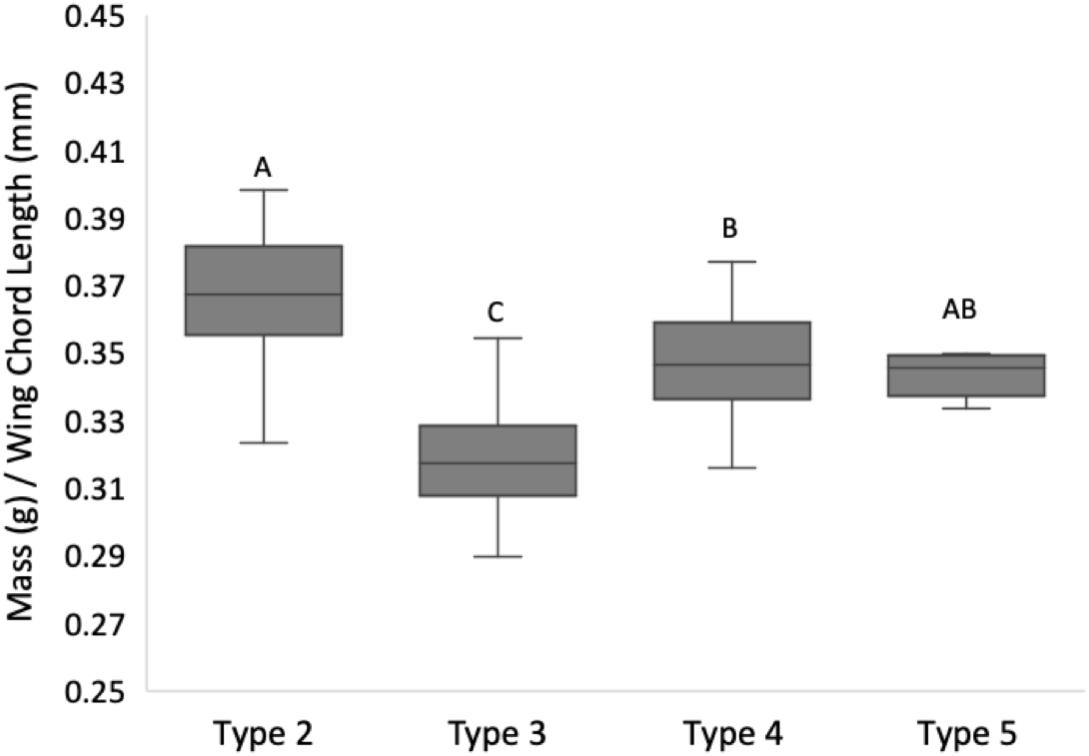
Figure 4. Wing loading in red crossbill ecotypes 2, 3, 4, and 5. The mass to wing chord length quotient in lean red crossbills was lowest in type 3 and highest in type 2. Box plots show inter-quartile range and median lines, whiskers give 95% CI. Box plots with different letters are significantly different based on Tukey comparisons of means (P < 0.0001). Sample sizes are 34 type 2, 96 type 3, 51 type 4, and 9 type 5 birds.
Discussion
Seasonal patterns of fat deposits in our long-term data set of four cardueline finch species generally support the hypothesis that population averages reflect different facultative migratory strategies and variable exposure to cold winter climates. The resident house finches captured in regions with mild winter climates can be considered a negative control in this analysis given that we predicted no pre-migratory or thermoregulatory fattening. In agreement, our models detected no significant seasonal variations in fat deposits in this species when analyzed by month or life cycle stage – although it should be noted that our sample sizes are low in spring and may preclude detection of a seasonal low during breeding. Pine siskins and American goldfinches, the two species with perhaps the most variable spring migratory behavior, had larger fat deposits in winter compared to the spring migratory period; whereas red crossbills, arguably the most consistent spring nomad, showed a distinct peak in fat deposits coincident with the late spring migratory period that exceeded winter fat deposits. Although winter breeding may cause adult crossbills to carry less fat as they invest in young (Cornelius et al., 2012; Jamie M. Cornelius, unpublished data), it is notable that fat deposits during the migratory phase in this species were also larger than the fat deposits carried during the cold winter month of December when winter breeding does not occur.
Cold winter temperatures may be both an ultimate and proximate driver of migration for some migrants. One continent-wide metaanalysis of banding records suggests that 11 of 12 partial migrant species migrate away from those areas with the coldest winter temperatures, including pine siskins and house finches (Bonnet-Lebrun et al., 2020). The exception was the American goldfinch – although it tended to overwinter in urban environments in cold places, as many other species do (Bonnet-Lebrun et al., 2020). Pine siskins and American goldfinches may need to carry comparatively more fat than red crossbills in winter due to their smaller size – as can be observed in sparrows of variable body size (Rogers, 2015). Rapidly declining fat deposits from January and February through March and April in these smaller species support that hypothesis. But whereas the partial migrant American goldfinch showed a continued decline in fat deposits through the spring migratory period, the pine siskin showed an elevation in May just after a nadir in fat deposits in April. This increase is consistent with results from captive pine siskins indicating spring migratory preparations of increased fat deposits, body mass, pectoral muscle size and hematocrit, with changes in physiology coincident with an increase in nocturnal migratory restlessness (Robart et al., 2018). Compared with diurnal migration, nocturnal migratory movements may limit the ability to locate foraging sites once underway (Alerstam, 2011) and increased nocturnal migratory behavior may be related to the elevation in fat deposits observed in pine siskins. The timing of migratory preparations in captive pine siskins also differed slightly than observed here, with preparations beginning earlier in captive birds (late March; Robart et al., 2018). This difference may reflect different suites of environmental cues experienced by captive and free-living birds (Calisi and Bentley, 2009).
Pine siskins, American goldfinches, and red crossbills showed fall increases in fat deposits. Pine siskins and goldfinches had a modest increase in the fall relative to the summer nadir, whereas this increase was much more pronounced in red crossbills. This difference between crossbills and siskins may be due to the smaller fat deposits carried by crossbills during breeding, rather than to the absolute levels carried during the fall migratory period, which were more similar between the species. On the other hand, given that pine siskins and goldfinches show more modest increases in fat deposits compared to crossbills during migratory periods, another possibility is that diet differences influence the need for preparatory fattening. Specifically, red crossbills have a more specialized diet compared to pine siskins or American goldfinches (Benkman and Young, 2020; Dawson, 2020; McGraw and Middleton, 2020) and thus may have fewer opportunities to refuel after departure – although insects are likely to play an important role for crossbills during spring migration and in early summer (Thomas P. Hahn and Jamie M. Cornelius, unpublished data).
The increases observed during the migratory periods in these facultative migrants were relatively modest compared to obligate migrants [e.g., the increase in fat score observed in red crossbills during spring migration is half as large as in two similar-sized North American buntings (Helms and Drury, 1960)]. Pine siskins in particular showed relatively small increases in fat during the spring migratory period, which agrees with other studies in free-living pine siskins where fat deposits were small in birds intercepted at banding stations during apparent migratory events in the fall (Yong and Finch, 2002; Seewagen and Newhouse, 2017). Crossbills nearly doubled fat reserves coincident with the period of fall migration and showed a slightly smaller increase during spring migration in this study. This agrees with data collected on crossbills during an irruption year in Britain that showed small but significant increases in body mass in October and then again just prior to departure in June (Summers et al., 1996), and with captive studies showing similar seasonal changes in mass and fat (Berthold, 1975; Cornelius and Hahn, 2012). Studies on crossbills thought to be more sedentary in pine forests of the Pyrenees found that month was a significant factor in models explaining changes in fat and mass, but post-hoc analysis failed to identify peaks or valleys (Alonso and Arizaga, 2011). Our type-specific analysis here also identified distinctions between crossbills that associate most strongly with spruces, Douglas-fir and hemlocks and crossbills that associate strongly with pines, especially in type 5, which is commonly associated with lodgepole pine.
Fat deposits during winter and summer breeding were not distinct between red crossbill ecotypes in this study but differed in interesting ways during the migratory periods. Fat deposits coincident with the annual nomadic migration in spring were as predicted: type 3 showed the largest increase in fat reserves, followed by type 4 and then type 2, which did not have significantly higher fat during the spring migratory period relative to breeding. This may reflect more consistent nomadic movements in those crossbill types that are associated with conifer species with large annual fluctuations in cone production (Ruth and Berntsen, 1955; Fowells, 1968; Smith and Balda, 1979). Wing loading in red crossbill ecotypes agrees with this hypothesis. Type 3 had the lowest wing loading, consistent with patterns in some other taxa where more migrant individuals (e.g., in partial migrants) or longer-distance migrants have lower wing loading (Pérez-Tris and Tellería, 2001; Nowakowski et al., 2014). Types 2 and 5 had the highest wing loading – suggesting less frequent or shorter-distance migrations and type 5 also showed a unique pattern in fat deposits, with lowest levels occurring during the spring migratory season. The data from types 2 and 5 agree, however, with data from crossbills captured in the Pyrenees that also feed on pines with consistent cone crops (Alonso and Arizaga, 2011), and suggests that crossbills foraging on more consistent food supplies may show less intensive migratory fattening. Alternatively, it is possible that differences in fat deposits during facultative migrations and wing loading reflect the average distance traveled, which is still relatively unknown for different crossbill ecotypes, or refueling options along the way.
Change in fat deposits across crossbill ecotypes did not follow our predictions during the fall migratory period. While fat deposits increased substantially during the fall migratory period in pine-associated crossbill ecotypes 2 and 5, they did not do so in types 3 and 4. One possible explanation is that pine-associated types are most likely to breed in summer on Douglas-fir (Psuedotsuga menziesii) and spruces before switching to pine in winter and spring when the seeds are more accessible. This has been documented in Europe (Marquiss and Rae, 2002) and may explain why pine-associated crossbills lay down more fat coincident with periods of fall movements than did the other types. Pine-associated ecotypes do not always move and switch diets, however, as we have observed type 5 crossbills using mostly blue spruce (Picea pungens) and Douglas-fir to support breeding in both summer and winter in several different years past in northwestern Wyoming [Jamie M. Cornelius, unpublished data; (Kelsey, 2008)].
American goldfinches are partial migrants and our study site was located toward the northern edge of their wintering range (McGraw and Middleton, 2020). We therefore expected to have captured at least some migrant and some resident birds during migratory months, though it remains possible that our sample was not balanced in this regard, precluding detection of migratory fattening. In many partial migrants the juveniles and females are more migratory and travel further than adult males (Chapman et al., 2011). American goldfinches may differ from this typical pattern in regards to age, but available data suggest that females winter further south than males on average (Prescott and Middleton, 1990). In our study, adult females carried more fat than adult males, but there was no interaction between sex and season, and fattening during spring migration was not apparent in either sex. Our finding of a February peak and a spring nadir in fat deposits is consistent with previous studies of American goldfinches in our study region in southeast MI (Dawson and Marsh, 1986), and with body composition analysis that found peak lipid content and body mass from December through February (Carey et al., 1978). These data support the importance of fat for small-bodied songbirds in winter and suggest that either migratory fueling is low in these diurnal partial migrants, as for other diurnal migrants (Bojarinova et al., 2008; Stey et al., 2017), or that our sample did not include enough active migrants to detect it.
American goldfinches are also unique in this study in that they are the only species to undergo a prealternate molt that peaks in early spring but is not complete in the population until June or July (Middleton, 1977). Many species separate prealternate molt from migration, presumably to reduce potential energetic trade-offs (Voelker and Rohwer, 1998; Danner et al., 2015). The clear overlap between the prealternate molt and the recorded periods of movement in goldfinches may drive lower fat reserves during the migratory period, as it does in Rusty blackbirds (Euphagus carolinus) and Pallas’s grasshopper warblers (Locustella certhiola) that exhibit variable molt and migration patterns and do not always avoid overlap (Wright et al., 2018; Eilts et al., 2021). However, the late breeding season in American goldfinches may also allow for a longer, slower prealternate molt (Middleton, 1977), which may reduce the costs of molt-migration overlap.
House finches are considered the least migratory species in our study sample and we captured them in regions with mild winter climates. Our finding that fat deposits did not change seasonally is consistent with a previous study of body composition in house finches in southern California (Dawson et al., 1983). However, similar examinations of house finches in areas with much colder winter climates have found seasonal elevations of body fat in the winter (Dawson et al., 1983; O’Connor, 1995). Thus, the absence of seasonal peaks in fat deposits, associated with either migratory or wintering stages, likely reflects both the lack of migratory behavior among house finches in California and a relatively mild climate or consistent food availability in the study locations.
In conclusion, we found support for seasonal fattening in facultative migrant finches coincident with migratory timing and consistent with annual and population-level variations observed in migratory behavior. We also identified ecotype-specific variation in the seasonal patterns of fattening in red crossbills that associate with different conifer species – suggesting that the degree of fattening may relate to probability of relocating to find new cone crops in a given season and/or the anticipated distance of nomadic movements. Remote tracking in this species and in other facultative migrant species will be illuminating in teasing apart these hypotheses further. Geolocators are particularly useful for demonstrating residence in more sedentary or site faithful populations of finches because they require recapture (Alonso et al., 2017) and radiotelemetry-based network systems such as the motus wildlife tracking network could be utilized in some localities where towers are installed (Taylor et al., 2017). The further miniaturization of satellite trackers, however, could be revolutionary for understanding patterns of movement in facultative migrants like Cardueline finches and such systems will allow for a much more accurate understanding of how premigratory preparations relate to actual migratory behavior in these facultative species.
Data Availability Statement
The datasets presented in this study can be found in online repositories. The names of the repository/repositories and accession number(s) can be found below: Data are available at ScholarsArchive@OSU at https://ir.library.oregonstate.edu/concern/datasets/9g54xr201.
Ethics Statement
The animal study was reviewed and approved by Institutional Animal Care and Use Committees at Oregon State University, Eastern Michigan University, University of Washington, University of California-Davis, Loyola Marymount University, and Washington State University.
Author Contributions
JC and HW conceived of the study. JC, TH, AR, BV, DZ, KG, CN, and HW collected the data. JC performed the statistical analysis and wrote the first draft of the manuscript. JC and BV generated figures. All authors contributed to manuscript editing and approved the submitted version.
Funding
This work was supported by NSF grants IBN-0988470 and IOB-0235911 to TH, IOS-1755227 to JC, as well as IOS-1456954, IOS-1756976, and IOS-1755245 to HW. JC was partially supported by an Eastern Michigan University faculty award, a Marjorie and Charles Elliott Fellowship, a UC Davis Dissertation Grant, a P.E.O. fellowship, an ARCS grant, and a Max Planck Institute of Ornithology post-doctoral stipend during the course of this work. HW received support from the Santa Monica Bay Audubon Society and a Ruth L. Kirschstein National Research Service Award from the NICHD (1F32HD056980) for portions of this work.
Conflict of Interest
The authors declare that the research was conducted in the absence of any commercial or financial relationships that could be construed as a potential conflict of interest.
Acknowledgments
We would like to thank J. C. Wingfield, M. Ramenofsky, H. Dingle, and M. Wikelski for guidance and support and for contributing to the development of ideas presented in the manuscript. K. Sewall, T. R. Kelsey, T. K. Chapple, E. M. Schultz, W. W. Dowd, D. Bradley, S. Chiparus, K. Campbell, M. Wurtz, and M. Bohanon assisted at times with fieldwork. We would also like to thank Grand Teton National Park, The Murie Center, Teton Science Schools, Columbia Land Trust, Phyllis Haehnle Memorial Sanctuary, and numerous Oregon, Washington and California State Parks and private landowners for access to trapping sites and logistical support throughout this study.
Supplementary Material
The Supplementary Material for this article can be found online at: https://www.frontiersin.org/articles/10.3389/fevo.2021.691808/full#supplementary-material
References
Alerstam, T. (2011). Optimal bird migration revisited. Journal of Ornithology 152, 5–23. doi: 10.1007/s10336-011-0694-1
Alerstam, T., and Lindstrom, A. (1990). “Optimal bird migration: the relative importance of time, energy, and safety,” in Bird migration, ed. E. Gwinner (Berlin: Springer), 331–351. doi: 10.1007/978-3-642-74542-3_22
Alonso, D., and Arizaga, J. (2011). Seasonal patterns of breeding, moulting, and body mass variation in Pyrenean Common Crossbills Loxia curvirostra curvirostra. Ringing & Migration 26, 64–70. doi: 10.1080/03078698.2011.587253
Alonso, D., Arizaga, J., Meier, C. M., and Liechti, F. (2017). Light-level geolocators confirm resident status of a Southern European Common Crossbill population. Journal of Ornithology 158, 75–81. doi: 10.1007/s10336-016-1388-5
Babushkina, O., and Bojarinova, J. (2011). Photoperiodically regulated cycle of locomotory activity and fat reserves during migration seasons in the irruptive bird species, the long-tailed tit Aegithalos c. caudatus. Journal of Avian Biology 42, 169–177. doi: 10.1111/j.1600-048x.2010.05149.x
Badyaev, A. V., Belloni, V., and Hill, G. E. (2020). “House Finch (Haemorhous mexicanus),” in Birds of the World, ed. A. F. Poole (Ithaca, NY: Cornell Lab of Ornithology).
Bailey, R. E. (1952). The incubation patch of passerine birds. The Condor 54, 121–136. doi: 10.2307/1365062
Bairlein, F. (2002). How to get fat: nutritional mechanisms of seasonal fat accumulation in migratory songbirds. Naturwissenschaften 89, 1–10. doi: 10.1007/s00114-001-0279-6
Bairlein, F., and Gwinner, E. (1994). Nutritional mechanisms and temporal control of migratory energy accumulation in birds. Annual review of nutrition 14, 187–215. doi: 10.1146/annurev.nu.14.070194.001155
Bednekoff, P., and Krebs, J. (1995). Great tit fat reserves: effects of changing and unpredictable feeding day length. Functional Ecology 9, 457–462. doi: 10.2307/2390009
Benkman, C. W. (1987). Food profitability and the foraging ecology of crossbills. Ecological Monographs 57, 251–267. doi: 10.2307/2937083
Benkman, C. W. (1993). Adaptation to single resources and the evolution of crossbill (Loxia) diversity. Ecological Monographs 63, 305–325. doi: 10.2307/2937103
Benkman, C. W., and Young, M. A. (2020). “Red Crossbill (Loxia curvirostra),” in Birds of the World, eds B. K. K. S. M. Billerman, P. G. Rodewald, and T. S. Schulenberg (Ithaca, NY: Cornell Lab of Ornithology).
Berthold, P. (1975). Migratory fattening endogenous control and interaction with migratory activity. Naturwissenschaften 62, 399–399. doi: 10.1007/bf00625361
Berthold, P., and Gwinner, E. (1978). Jahresperiodik der Gonadengrasse beim Fichtenkreuzschnabel (Loxia curvirostra). Journal of Ornithology 119, 338–339. doi: 10.1007/bf01643212
Biebach, H. (1996). “Energetics of winter and migratory fattening,” in Avian energetics and nutritional ecology, ed. C. Carey (Bostong: Springer), 280–323. doi: 10.1007/978-1-4613-0425-8_9
Bielefeldt, J., and Rosenfield, R. N. (1994). Summer birds of conifer plantations in southeastern Wisconsin. Passenger Pigeon 56, 123–135.
Blem, C. R. (1975). Geographic variation in wing-loading of the house sparrow. The Wilson Bulletin 87, 543–549.
Blem, C. R. (1976). Patterns of lipid storage and utilization in birds. American Zoologist 16, 671–684. doi: 10.1093/icb/16.4.671
Blem, C. R. (1980). “The energetics of migration,” in Animal migration, orientation, and navigation, ed. S. A. Gautreaux (New York, NY: Academic Press), 175–224. doi: 10.1016/b978-0-08-091833-4.50008-0
Bojarinova, J., and Babushkina, O. (2010). Are ecological differences of irruptive bird species from typical migrants real? (The example of the Long-tailed Tit Aegithalos c. caudatus). Russian Journal of Ecology 41, 422–427. doi: 10.1134/s1067413610050103
Bojarinova, J., and Babushkina, O. (2015). Photoperiodic conditions affect the level of locomotory activity during autumn migration in the Long-tailed Tit (Aegithalos c. caudatus). The Auk 132, 370–379. doi: 10.1642/auk-14-155.1
Bojarinova, J., Ilves, A., Chernetsov, N., and Leivits, A. (2008). Body mass, moult and migration speed of the Goldcrest Regulus regulus in relation to the timing of migration at different sites of the migration route. Ornis Fennica 85, 55–65.
Bonnet-Lebrun, A.-S., Manica, A., and Rodrigues, A. S. (2020). Effects of urbanization on bird migration. Biological Conservation 244, 108423. doi: 10.1016/j.biocon.2020.108423
Bowlin, M. S., and Wikelski, M. (2008). Pointed wings, low wingloading and calm air reduce migratory flight costs in songbirds. PLoS One 3:e2154. doi: 10.1371/journal.pone.0002154
Boyle, W. A. (2008). Partial migration in birds: tests of three hypotheses in a tropical lekking frugivore. Journal of Animal Ecology 77, 1122–1128. doi: 10.1111/j.1365-2656.2008.01451.x
Brady, R. S., Anich, N. M., and Young, M. A. (2019). Wisconsin’s Red Crossbill Irruption of 2017–18: Distribution, Abundance, and Breeding Behavior of Multiple Call Types. The Passenger Pigeon 81, 215–240.
Buler, J. J., Lyon, R. J., Smolinsky, J. A., Zenzal, T. J., and Moore, F. R. (2017). Body mass and wing shape explain variability in broad-scale bird species distributions of migratory passerines along an ecological barrier during stopover. Oecologia 185, 205–212. doi: 10.1007/s00442-017-3936-y
Calisi, R. M., and Bentley, G. E. (2009). Lab and field experiments: are they the same animal? Hormones and behavior 56, 1–10. doi: 10.1016/j.yhbeh.2009.02.010
Carey, C., Dawson, W. R., Maxwell, L. C., and Faulkner, J. A. (1978). Seasonal acclimatization to temperature in cardueline finches. Journal of Comparative Physiology 125, 101–113. doi: 10.1007/bf00686746
Chapman, B. B., Brönmark, C., and Nilsson, J. Å, and Hansson, L. A. (2011). The ecology and evolution of partial migration. Oikos 120, 1764–1775. doi: 10.1111/j.1600-0706.2011.20131.x
Cimprich, D. A., Woodrey, M. S., and Moore, F. R. (2005). Passerine migrants respond to variation in predation risk during stopover. Animal Behaviour 69, 1173–1179. doi: 10.1016/j.anbehav.2004.07.021
Cornelius, J. M., and Hahn, T. P. (2012). Seasonal pre-migratory fattening and increased activity in a nomadic and irruptive migrant, the Red Crossbill Loxia curvirostra. Ibis 154, 693–702. doi: 10.1111/j.1474-919X.2012.01266.x
Cornelius, J. M., Breuner, C. W., and Hahn, T. P. (2012). Coping with the extremes: stress physiology varies between winter and summer in breeding opportunists. Biology letters 8, 312–315. doi: 10.1098/rsbl.2011.0865
Cornelius, J. M., Perfito, N., Zann, R. A., Breuner, C. W., and Hahn, T. P. (2011). Physiological trade-offs in self-maintenance: plumage molt and stress physiology in birds. Journal of Experimental Biology 214, 2768–2777. doi: 10.1242/jeb.057174
Danner, R. M., Greenberg, R. S., Danner, J. E., and Walters, J. R. (2015). Winter food limits timing of pre-alternate moult in a short-distance migratory bird. Functional Ecology 29, 259–267. doi: 10.1111/1365-2435.12322
Dawson, A. (2020). “Pine Siskin (Spinus pinus),” in Birds of the World, ed. A. F. Poole (Ithaca, NY: Cornell Lab of Ornithology). doi: 10.1016/j.ygcen.2012.04.023
Dawson, W. R., and Marsh, R. L. (1986). Winter fattening in the American Goldfinch and the possible role of temperature in its regulation. Physiological Zoology 59, 357–368. doi: 10.1086/physzool.59.3.30156107
Dawson, W. R., Marsh, R. L., Buttemer, W. A., and Carey, C. (1983). Seasonal and geographic variation of cold resistance in house finches Carpodacus mexicanus. Physiological Zoology 56, 353–369. doi: 10.1086/physzool.56.3.30152600
Eilts, H. J., Feuerbach, N., Round, P. D., Bourski, O., Allcock, J., Leader, P., et al. (2021). Complex postbreeding molt strategies in a songbird migrating along the East Asian Flyway, the Pallas’s Grasshopper Warbler Locustella certhiola. Ecology and evolution 11, 11–21. doi: 10.1002/ece3.7098
Ekman, J. B., and Hake, M. K. (1990). Monitoring starvation risk: adjustments of body reserves in greenfinches (Carduelis chloris L.) during periods of unpredictable foraging success. Behavioral Ecology 1, 62–67. doi: 10.1093/beheco/1.1.62
Fowells, H. A. (1968). Silvics of the United States. Agricultural Handbook No. 271. Washington, DC: USDA Forest Service.
Freed, L. A. (1981). Loss of mass in breeding wrens: Stress or adaptation? Ecology 62, 1179–1186. doi: 10.2307/1937282
Fudickar, A. M., Schmidt, A., Hau, M., Quetting, M., and Partecke, J. (2013). Female-biased obligate strategies in a partially migratory population. Journal of Animal Ecology 82, 863–871. doi: 10.1111/1365-2656.12052
Gatter, W., and Gatter, W. (2019). Der Fichtenkreuzschnabel Loxia curvirostra–ein regulärer Kurzstreckenzieher und Invasionsvogel mit Wegzug im Sommer, Heimzug im Herbst und Brut im Winter: Zugbeobachtungen aus fünf Jahrzehnten am Randecker Maar. Vogelwelt 139, 39–49.
Gentle, L. K., and Gosler, A. G. (2001). Fat reserves and perceived predation risk in the great tit, Parus major. Proceedings of the Royal Society of London. Series B 268, 487–491. doi: 10.1098/rspb.2000.1405
Granlund, J., McPeek, G. A., and Adams, R. J. (1994). The birds of Michigan. Bloomington, IN: Indiana University Press.
Guglielmo, C. G. (2010). Move that fatty acid: fuel selection and transport in migratory birds and bats. Integrative and Comparative Biology 50, 336–345. doi: 10.1093/icb/icq097
Guglielmo, C. G. (2018). Obese super athletes: fat-fueled migration in birds and bats. Journal of Experimental Biology 221(Suppl 1), jeb165753.
Haftorn, S. (1989). Seasonal and diurnal body weight variations in titmice, based on analyses of individual birds. The Wilson Bulletin 101, 217–235.
Hahn, T. P. (1995). Integration of photoperiodic and food cues to time changes in reproductive physiology by an opportunistic breeder, the red crossbill, Loxia-curvirostra (Aves, Carduelinae). Journal of Experimental Zoology 272, 213–226. doi: 10.1002/jez.1402720306
Hahn, T. P. (1998). Reproductive seasonality in an opportunistic breeder, the red crossbill, Loxia curvirostra. Ecology 79, 2365–2375. doi: 10.2307/176828
Hedenström, A. (1993). Migration by soaring or flapping flight in birds: the relative importance of energy cost and speed. Philosophical Transactions of the Royal Society of London. Series B 342, 353–361. doi: 10.1098/rstb.1993.0164
Hegner, R. E., and Wingfield, J. C. (1986). Behavioral and endocrine correlates of multiple brooding in the semicolonial house sparrow Passer domesticus II. Females. Hormones and behavior 20, 313–326. doi: 10.1016/0018-506x(86)90040-1
Helms, C. W., and Drury, W. H. J. (1960). Winter migratory weight and fat field studies on some North American buntings. Bird Banding 31, 1–40. doi: 10.2307/4510793
Holberton, R. L. (1993). An endogenous basis for differential migration in the dark-eyed junco. The Condor 95, 580–587. doi: 10.2307/1369602
Houston, A. I., and McNamara, J. M. (1993). A theoretical investigation of the fat reserves and mortality levels of small birds in winter. Ornis Scandinavica 24, 205–219. doi: 10.2307/3676736
Jahn, A. E., Levey, D. J., Farias, I. P., Mamani, A. M., Vidoz, J. Q., and Freeman, B. (2010). Morphological and genetic variation between migratory and non-migratory Tropical Kingbirds during spring migration in central South America. The Wilson Journal of Ornithology 122, 236–243. doi: 10.1676/09-086.1
Jenni, L., and Jenni-Eiermann, S. (1998). Fuel supply and metabolic constraints in migrating birds. Journal of Avian Biology 29, 521–528. doi: 10.2307/3677171
Kelsey, R. (2008). Biogeography, foraging ecology and population dynamics of red crossbills in North America. Ph.D.Thesis. Oakland, CA: University of California.
Kendeigh, S. C. (1969). Energy responses of birds to their thermal environments. The Wilson Bulletin 81, 441–449.
Krause, J. S., Pérez, J. H., Chmura, H. E., Meddle, S. L., Hunt, K. E., Gough, L., et al. (2016). The stress response is attenuated during inclement weather in parental, but not in pre-parental, Lapland longspurs (Calcarius lapponicus) breeding in the Low Arctic. Hormones and behavior 83, 68–74. doi: 10.1016/j.yhbeh.2016.05.018
Kullberg, C., Fransson, T., and Jakobsson, S. (1996). Impaired predator evasion in fat blackcaps (Sylvia atricapilla). Proceedings of the Royal Society of London. Series B 263, 1671–1675. doi: 10.1098/rspb.1996.0244
Lehikoinen, E. (1986). Dependence of winter survival on size in the great tit Parus major. Ornis Fennica 63, 10–16.
Liknes, E. T., and Swanson, D. L. (2011). Phenotypic flexibility of body composition associated with seasonal acclimatization in passerine birds. Journal of Thermal Biology 36, 363–370. doi: 10.1016/j.jtherbio.2011.06.010
Lima, S. L. (1986). Predation risk and unpredictable feeding conditions: determinants of body mass in birds. Ecology 67, 377–385. doi: 10.2307/1938580
Lind, J., and Cresswell, W. (2006). Anti-predation behaviour during bird migration; the benefit of studying multiple behavioural dimensions. Journal of Ornithology 147, 310–316. doi: 10.1007/s10336-005-0051-3
Lo Valvo, F., Lo Verde, G., and Lo Valvo, M. (1988). Relationships among wing length, wing shape and migration in Blackcap Sylvia atricapilla populations. Ringing & Migration 9, 51–54. doi: 10.1080/03078698.1988.9673923
Lundberg, P. (1985). Dominance behaviour, body weight and fat variations, and partial migration in European blackbirds Turdus merula. Behavioral Ecology and Sociobiology 17, 185–189.
Maillet, D., and Weber, J.-M. (2006). Performance-enhancing role of dietary fatty acids in a long-distance migrant shorebird: the semipalmated sandpiper. Journal of Experimental Biology 209, 2686–2695. doi: 10.1242/jeb.02299
Marchetti, K., Price, T., and Richman, A. (1995). Correlates of wing morphology with foraging behaviour and migration distance in the genus Phylloscopus. Journal of Avian Biology 26, 177–181. doi: 10.2307/3677316
Marquiss, M., and Rae, R. (2002). Ecological differentiation in relation to bill size amongst sympatric, genetically undifferentiated crossbills Loxia spp. Ibis 144, 494–508. doi: 10.1046/j.1474-919X.2002.00041.x
Marsh, R. L. (1984). Adaptations of the gray catbird Dumetella carolinensis to long-distance migration: flight muscle hypertrophy associated with elevated body mass. Physiological Zoology 57, 105–117. doi: 10.1086/physzool.57.1.30155973
McGraw, K. J., and Middleton, A. L. (2020). “American Goldfinch (Spinus tristus),” in Birds of the World, ed. P. G. Rodewald (Ithaca, NY: Cornell Lab of Ornithology).
McNamara, J. M., and Houston, A. I. (1990). The value of fat reserves and the tradeoff between starvation and predation. Acta biotheoretica 38, 37–61. doi: 10.1007/bf00047272
McWilliams, S. R., Guglielmo, C., Pierce, B., and Klaassen, M. (2004). Flying, fasting, and feeding in birds during migration: a nutritional and physiological ecology perspective. Journal of Avian Biology 35, 377–393. doi: 10.1111/j.0908-8857.2004.03378.x
Mezquida, E. T., Svenning, J. C., Summers, R. W., and Benkman, C. W. (2018). Higher spring temperatures increase food scarcity and limit the current and future distributions of crossbills. Diversity and Distributions 24, 473–484. doi: 10.1111/ddi.12694
Middleton, A. (1977). The molt of the American Goldfinch. The Condor 79, 440–444. doi: 10.2307/1367723
Minias, P., Meissner, W., Włodarczyk, R., Ożarowska, A., Piasecka, A., Kaczmarek, K., et al. (2015). Wing shape and migration in shorebirds: a comparative study. Ibis 157, 528–535. doi: 10.1111/ibi.12262
Napolitano, G. E., and Ackman, R. G. (1990). Anatomical distribution of lipids and their fatty acids in the semipalmated sandpiper Calidris pusilla L. from Shepody Bay, New Brunswick, Canada. J. Exp. Mar. Biol. Ecol. 144, 113–124. doi: 10.1016/0022-0981(90)90023-6
Newton, I. (1969). Winter fattening in the bullfinch. Physiological Zoology 42, 96–107. doi: 10.1086/physzool.42.1.30152470
Newton, I. (2006b). Movement patterns of common crossbills Loxia curvirostra in europe. Ibis 148, 782–788. doi: 10.1111/j.1474-919x.2006.00585.x
Newton, I., and Dawson, A. (2011). Seasonal changes in moult, body mass and reproductive condition in siskins Carduelis spinus exposed to daylength regimes simulating different latitudes. Journal of Avian Biology 42, 22–28. doi: 10.1111/j.1600-048x.2010.05249.x
Nielsen, B., and Rees, J. (2013). Fat accumulation and autumn migration strategy of Reed Warblers Acrocephalus scirpaceus and Sedge Warblers A. schoenobaenus in southern Sweden. Ringing & Migration 28, 69–76. doi: 10.1080/03078698.2013.869904
Norberg, U. M. (1995). How a long tail and changes in mass and wing shape affect the cost of flight in animals. Functional Ecology 9, 48–54. doi: 10.2307/2390089
Nothdurft, W. (2019). Der Fichtenkreuzschnabel Loxia curvirostra im Ulmer Raum: Bestandsfluktuationen, Ernährung und Brutaktivitäten. Ornithol. Anz. 58, 126–143. doi: 10.1159/000384974
Nowakowski, J. K., Szulc, J., and Remisiewicz, M. (2014). The further the flight, the longer the wing: relationship between wing length and migratory distance in Old World reed and bush Warblers (Acrocephalidae and Locustellidae). Ornis Fennica 91, 178–187.
O’Connor, T. (1995). Metabolic characteristics and body composition in house finches: effects of seasonal acclimatization. Journal of Comparative Physiology B 165, 298–305. doi: 10.1007/bf00367313
Odum, E. P. (1960). Premigratory hyperphagia in birds. American Journal of Clinical Nutrition 8, 621–629. doi: 10.1093/ajcn/8.5.621
Palmer, R. S. (1968). “Spinus pinus (Wilson), Pine Siskin,” in Life histories of North American cardinals, grosbeaks, buntings, towhees, finches, sparrows, and allies, eds A. C. Bent and O. L. Austin Jr. (Washington, D.C: Bulletin of the United States National Museum), 424–447.
Pascual, J., and Senar, J. C. (2015). Resident but not transient Eurasian Siskins reduce body mass in response to increasing predation risk: a natural experiment. Journal of Ornithology 156, 451–456. doi: 10.1007/s10336-014-1143-8
Pérez-Tris, J., and Tellería, J. L. (2001). Age-related variation in wing shape of migratory and sedentary Blackcaps Sylvia atricapilla. Journal of Avian Biology 32, 207–213. doi: 10.1111/j.0908-8857.2001.320301.x
Pettersson, J., and Hasselquist, D. (1985). Fat deposition and migration capacity of robins Erithacus rebecula and goldcrests Regulus regulus at Ottenby, Sweden. Ringing & Migration 6, 66–76. doi: 10.1080/03078698.1985.9673859
Pierce, B. J., and McWilliams, S. R. (2014). The fat of the matter: how dietary fatty acids can affect exercise performance. American Zoologist 54, 903–912. doi: 10.1093/icb/icu098
Pravosudov, V. V., and Grubb, T. C. (1998). Management of fat reserves in tufted titmice Baelophus bicolorin relation to risk of predation. Animal Behaviour 56, 49–54. doi: 10.1006/anbe.1998.0739
Prescott, D. R., and Middleton, A. L. (1990). Age and sex differences in winter distribution of American Goldfinches in eastern North America. Ornis Scandinavica 21, 99–104. doi: 10.2307/3676804
Price, E. R. (2010). Dietary lipid composition and avian migratory flight performance: development of a theoretical framework for avian fat storage. Comparative Biochemistry and Physiology Part A 157, 297–309. doi: 10.1016/j.cbpa.2010.05.019
Pyle, P., Howell, S. N., and David, F. Y. (1997). Identification guide to North American birds: Part I Columbidae to PloceidaeSlate. Bolinas, CA: Creek Press.
Ramenofsky, M. (1990). “Fat storage and fat metabolism in relation to migration,” in Bird Migration, ed. E. Gwinner (Berlin: Springer-Verlag), 214–231. doi: 10.1007/978-3-642-74542-3_15
Ramenofsky, M., Savard, R., and Greenwood, M. (1999). Seasonal and diel transitions in physiology and behavior in the migratory dark-eyed junco. Comparative Biochemistry and Physiology Part A 122, 385–397. doi: 10.1016/s1095-6433(99)00013-6
Robart, A. R., McGuire, M. M., and Watts, H. E. (2018). Increasing photoperiod stimulates the initiation of spring migratory behaviour and physiology in a facultative migrant, the pine siskin. Royal Society Open Science 5, 180876. doi: 10.1098/rsos.180876
Rogers, C. M. (1987). Predation risk and fasting capacity – Do wintering birds maintain optimal body-mass. Ecology 68, 1051–1061. doi: 10.2307/1938377
Rogers, C. M. (2015). Testing optimal body mass theory: Evidence for cost of fat in wintering birds. Ecosphere 6, 1–12. doi: 10.1890/es14-00317.1
Ruth, R. H., and Berntsen, C. M. (1955). A 4-year record of sitka spruce and western hemlock seed fall on the Cascade Head Experimental Forest. USDA Forest Service PNW Old Series Research Paper No. 12: 1-13 12.∗∗∗q
Schmaljohann, H., and Dierschke, V. (2005). Optimal bird migration and predation risk: a field experiment with northern wheatears Oenanthe oenanthe. Journal of Animal Ecology 74, 131–138. doi: 10.1111/j.1365-2656.2004.00905.x
Seewagen, C. L., and Newhouse, M. (2017). Passage Dates, Energetic Condition, and Age Distribution of Irruptive Pine Siskins during Autumn Stopovers at a Reclaimed Landfill in the New Jersey Meadowlands. Northeastern Naturalist 24, 201–208. doi: 10.1656/045.024.0211
Senar, J., Burton, P., and Metcalfe, N. (1992). Variation in the nomadic tendency of a wintering finch Carduelis spinus and its relationship with body condition. Ornis Scandinavica 23, 63–72. doi: 10.2307/3676428
Silverin, B. (2003). “Behavioural and hormonal dynamics in a partial migrant—the willow tit,” in Avian migration, eds P. Berthold, E. Gwinner, and E. Sonnenschein (Berlin: Springer), 127–140. doi: 10.1007/978-3-662-05957-9_8
Smith, C. C., and Balda, R. P. (1979). Competition Among Insects, Birds and Mammals for Conifer Steeds. Integrative. and Comparative Biology 19, 1065–1083. doi: 10.1093/icb/19.4.1065
Stey, K., Röseler, D., and Bairlein, F. (2017). Endogenous migratory behaviour in a diurnally migrating songbird. Journal of Ornithology 158, 717–724. doi: 10.1007/s10336-017-1440-0
Summers, R., Jardine, D., Marquiss, M., and Proctor, R. (1996). The biometrics of invading Common Crossbills Loxia curvirostra in Britain during 1990–1991. Ringing & Migration 17, 1–10.
Taylor, P. D., Crewe, T. L., Mackenzie, S. A., Lepage, D., Aubry, Y., Crysler, Z., et al. (2017). The Motus Wildlife Tracking System: a collaborative research network to enhance the understanding of wildlife movement. Avian Conserv. Ecol. 12, art8.
Tiainen, J. (1980). Adaptedness of the willow tit, Parus montanus, to the migratory habit. Ornis Fenn 57, 77–81.
Videler, J., Groenewegen, A., Gnodde, M., and Vossebelt, G. (1988). Indoor flight experiments with trained kestrels: II. the effect of added weight on flapping flight kinematics. Journal of Experimental Biology 134, 185–199. doi: 10.1242/jeb.134.1.185
Vincent, J., and Bédard, J. (1976). Fat reserves in snow buntings. Canadian Journal of Zoology 54, 1051–1063. doi: 10.1139/z76-119
Voelker, G., and Rohwer, S. (1998). Contrasts in scheduling of molt and migration in Eastern and Western warbling-vireos. The Auk 115, 142–155. doi: 10.2307/4089119
Ward, P., and Jones, P. J. (1977). Pre-migratory fattening in three races of the Red-billed quelea Quelea quelea (Aves: Ploceidae), an intra-tropical migrant. Journal of Zoology 181, 43–56. doi: 10.1111/j.1469-7998.1977.tb04569.x
Weber, J.-M. (2009). The physiology of long-distance migration: extending the limits of endurance metabolism. Journal of Experimental Biology 212, 593–597. doi: 10.1242/jeb.015024
Weber, T. P., Houston, A. I., and Ens, B. J. (1994). Optimal departure fat loads and stopover site use in avian migration: an analytical model. Proceedings of the Royal Society of London. Series B 258, 29–34. doi: 10.1098/rspb.1994.0137
Witmer, M. C. (2020). “Bohemian Waxwing (Bombycilla garrulus,” in Birds of the World, ed. S. M. Billerman (Ithaca, NY: Cornell Lab of Ornithology).
Witter, M. S., and Cuthill, I. C. (1993). The ecological costs of avian fat storage. Philosophical Transactions of the Royal Society of London. Series B 340, 73–92. doi: 10.1098/rstb.1993.0050
Wojciechowski, M. S., Yosef, R., and Pinshow, B. (2014). Body composition of north and southbound migratory blackcaps is influenced by the lay-of-the-land ahead. Journal of Avian Biology 45, 264–272. doi: 10.1111/j.1600-048x.2013.00345.x
Wolfson, A. (1952). The cloacal protuberance- a means for determining breeding condition in live male passerines. Bird Banding 23, 159–165. doi: 10.2307/4510381
Wright, J. R., Tonra, C. M., and Powell, L. L. (2018). Prealternate molt-migration in Rusty Blackbirds and its implications for stopover biology. The Condor 120, 507–516. doi: 10.1650/condor-17-177.1
Keywords: annual cycle, Cardeuline, facultative movement, migration, fattening, fueling, finches, nomad
Citation: Cornelius JM, Hahn TP, Robart AR, Vernasco BJ, Zahor DL, Glynn KJ, Navis CJ and Watts HE (2021) Seasonal Patterns of Fat Deposits in Relation to Migratory Strategy in Facultative Migrants. Front. Ecol. Evol. 9:691808. doi: 10.3389/fevo.2021.691808
Received: 07 April 2021; Accepted: 04 June 2021;
Published: 29 June 2021.
Edited by:
Ivan Maggini, University of Veterinary Medicine Vienna, AustriaReviewed by:
Andrea Ferretti, University of Vienna, AustriaWieland Heim, University of Münster, Germany
Copyright © 2021 Cornelius, Hahn, Robart, Vernasco, Zahor, Glynn, Navis and Watts. This is an open-access article distributed under the terms of the Creative Commons Attribution License (CC BY). The use, distribution or reproduction in other forums is permitted, provided the original author(s) and the copyright owner(s) are credited and that the original publication in this journal is cited, in accordance with accepted academic practice. No use, distribution or reproduction is permitted which does not comply with these terms.
*Correspondence: Jamie M. Cornelius, amFtaWUuY29ybmVsaXVzQG9yZWdvbnN0YXRlLmVkdQ==; Heather E. Watts, aGVhdGhlci53YXR0c0B3c3UuZWR1
†These authors have contributed equally to this work