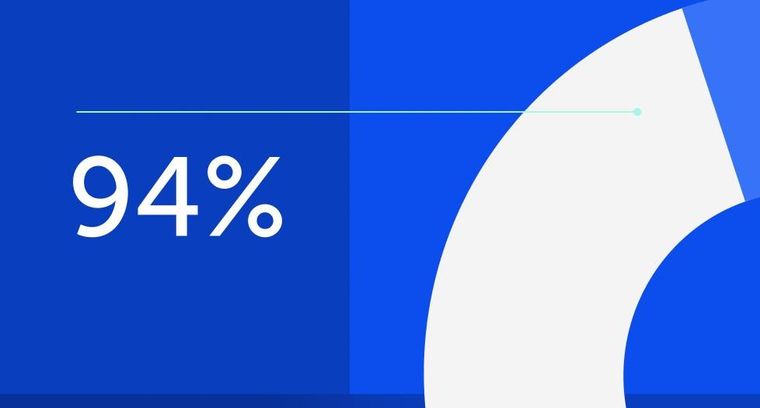
94% of researchers rate our articles as excellent or good
Learn more about the work of our research integrity team to safeguard the quality of each article we publish.
Find out more
ORIGINAL RESEARCH article
Front. Ecol. Evol., 07 December 2021
Sec. Paleoecology
Volume 9 - 2021 | https://doi.org/10.3389/fevo.2021.688512
This article is part of the Research TopicEarly Human Colonization of Remote Indian Ocean Islands and its Ecological ImpactsView all 11 articles
Madagascar’s biota underwent substantial change following human colonization of the island in the Late Holocene. The timing of human arrival and its role in the extinction of megafauna have received considerable attention. However, the impacts of human activities on regional ecosystems remain poorly studied. Here, we focus on reconstructing changes in the composition of terrestrial and aquatic ecosystems to evaluate the impact of human land use and climate variability. We conducted a paleoenvironmental study, using a sediment record that spans the last ∼1,145 years, collected from a lakebed in the Namonte Basin of southwest Madagascar. We examined physical (X-ray fluorescence and stratigraphy) and biotic indicators (pollen, diatoms and micro- and macro-charcoal particles) to infer terrestrial and aquatic ecosystem change. The fossil pollen data indicate that composition of grasslands and dry deciduous forest in the region remained relatively stable during an arid event associated with northward displacement of the Intertropical Convergence Zone (ITCZ) between ∼1,145 and 555 calibrated calendar years before present (cal yr BP). Charcoal particles indicate that widespread fires occurred in the region, resulting from a combination of climate drivers and human agency during the entire span covered by the paleorecord. Following settlement by pastoral communities and the disappearance of endemic megafauna ∼1,000 cal yr BP, grasslands expanded and the abundance of trees that rely on large animals for seed dispersal gradually declined. A reduction in the abundance of pollen taxa characteristic of dry forest coincided with an abrupt increase in charcoal particles between ∼230 and 35 cal yr BP, when agro-pastoral communities immigrated into the region. Deforestation and soil erosion, indicated by a relatively rapid sedimentation rate and high K/Zr and Fe/Zr, intensified between 180 and 70 cal yr BP and caused a consequent increase in lake turbidity, resulting in more rapid turnover of the aquatic diatom community. Land use and ongoing climate change have continued to transform local terrestrial and freshwater ecosystems during the last ∼70 years. The current composition of terrestrial and aquatic ecosystems reflects the legacy of extinction of native biota, invasion of exotic species, and diminished use of traditional land management practices.
Remote islands of the southwestern Indian Ocean were among the last regions worldwide to be permanently colonized by humans. Human settlement and land use practices, which invariably involve non-native plants and animals, may contribute to loss of endemic biota and transformation of native ecosystems (Douglass and Zinke, 2015; Wang et al., 2019). On Madagascar, people hunted endemic fauna (Perez et al., 2005; Anderson et al., 2018), introduced domesticated animals and plants (Crowther et al., 2016; Hixon et al., 2021a), and promoted the expansion of grasslands (Burney, 1987b; Burns et al., 2016). The timing of human arrival and contribution of hunting to faunal extinctions on Madagascar have received considerable attention (Crowley, 2010; Feranec et al., 2011; Douglass et al., 2019a). The direct and indirect impacts of anthropogenic activities on the composition and function of terrestrial and aquatic ecosystems are a subject of ongoing research. Only recently have studies begun to focus on the long-term consequences of human activities on Madagascar’s ecosystems (Virah-Sawmy et al., 2010, 2016; Burns et al., 2016). Integration of paleoecological, archaeological, and historical information provides a long-term perspective to assess the timing and socio-ecological consequences of human disturbance on such insular ecosystems (Douglass and Cooper, 2020).
The timing of the peopling of Madagascar has been widely debated (Crowley, 2010; Anderson et al., 2018; Hansford et al., 2018; Lawler, 2018; Douglass et al., 2019a; Mitchell, 2020; Hixon et al., 2021a). However, a growing body of archaeological research suggests that foraging communities had settled the island permanently by ∼1,500 cal yr BP, several centuries before a pulse of faunal extinctions that included giant lemurs, pygmy hippos, giant tortoises, and elephant birds (Douglass and Zinke, 2015; Douglass et al., 2019a). Human subsistence strategies later diversified, as evidenced by the coincident spread of southeast Asian crops and pastoralism, beginning by around 1,000 years ago (Crowther et al., 2016; Godfrey and Crowley, 2016; Hixon et al., 2021a). The emergence of agro-pastoral economies involved the introduction of animals such as zebu cattle (Bos taurus indicus), sheep (Ovis aries), and goats (Capra hircus), as well as cultigens like rice, greater yam, coconut, and Indian saffron during the last millennium (Burney et al., 2004; Kull et al., 2012; Beaujard, 2017).
Contemporary Malagasy people rely on a range of ecosystems. People typically use grasslands and succulent woodlands to fodder livestock and exploit forests for hunting and as sources of firewood, construction materials, and edible and medicinal plants (Bloesch, 1999; Kaufmann and Tsirahamba, 2006; Kull and Laris, 2009; Fritz-Vietta et al., 2017). Lakes and wetlands also constitute important sources of animal protein and drinking water, and anthropogenic disturbances have degraded these ecosystems and threatened aquatic biodiversity (Bamford et al., 2017). Although fire is the leading cause of past forest degradation and loss (Kull, 2000), paleoenvironmental data suggest that wildfires and fire-adapted vegetation were common even before the expansion of human populations on the island (Virah-Sawmy, 2009; Godfrey and Crowley, 2016). Today, Madagascar’s biodiversity is increasingly threatened by intensifying land use practices (e.g., deforestation, charcoal production, shifting cultivation, and industrial mining) and the climate crisis (Spencer et al., 2005; Hannah et al., 2008; Goodman et al., 2018). Interactions between land use and climate change have important socio-ecological impacts, including biodiversity loss, ecosystem degradation, pollution, disruption of traditional lifeways, and conflicts over natural resources (Scott et al., 2006; Virah-Sawmy et al., 2009; Harvey et al., 2014; Douglass and Cooper, 2020).
Climate change and human activities have also shaped Madagascar’s vegetation. Paleoecological reconstructions have shown, for example, that Erica-Myrica heath/grasslands replaced Uapaca woodlands during the last 950 years in coastal southeast Madagascar due to marine surges, reduced precipitation, and anthropogenic fires (Virah-Sawmy et al., 2009). In contrast, the vegetation around Andolonomby/Ambolisatra in the coastal southwest transitioned from a palm-savanna to depauperate, xerophytic bush ∼700 cal yr BP, during a period of intensified burning and a possible drought event (Virah-Sawmy et al., 2016). Multiple studies have shown that (1) northwest Madagascar was wetter and relatively forested during the Early and Middle Holocene, (2) grasslands expanded during the onset of drier conditions ∼1,000 years ago, and (3) human activities promoted the establishment of fire-adapted trees and ruderal herbs ∼500 years ago (Burney, 1987a,b; Matsumoto and Burney, 1994; Burney et al., 1997; Crowley and Samonds, 2013; Burns et al., 2016; Wang et al., 2019).
We assessed the long-term consequences of climate variability and anthropogenic impacts on the composition of terrestrial vegetation and aquatic ecosystems in southwest Madagascar. Specifically, we used a 1,145-year sediment record from a lake in the Namonte Basin to evaluate responses in aquatic and terrestrial ecosystems to Late Holocene drought, the spread of pastoralism, the extinction of endemic fauna, and recent intensification of forest clearance. We examined physical (X-ray fluorescence and stratigraphy) and biotic indicators (pollen, diatoms and micro- and macro-charcoal particles) in the sediment record to explore past terrestrial and aquatic ecosystem change. Integration of paleoecological, archaeological, and historical data is crucial for developing holistic perspectives on the timing and socio-ecological consequences of human land use in insular ecosystems (Braje et al., 2017; Douglass et al., 2019b). The following research questions were tested in this study: (a) Did increasing fire frequency during dry/wet periods induce changes in vegetation composition? (b) How did excess detrital and organic matter, produced by anthropogenic deforestation and forest burning, affect the aquatic ecosystem? and c) What were the impacts of extinction of large seed dispersers on vegetation?
The Namonte Basin (S 22.12513°, E 43.40634°; ∼30 m asl; Figure 1A) is comprised of a system of seasonal and permanent lakes and floodplains in the lowlands of the Mangoky-Ihotry complex in southwest Madagascar. The main chain of lake basins has an area of ∼13 km2 and a catchment area that is ∼2,000 km2. However, these lake basins are shallow (maximum water depth up to ∼1.4 m deep in September, 2017), and their areas fluctuate considerably with interannual variation in rainfall. For example, during a 1997–98 drought (100 mm total annual precipitation), the lakes approached desiccation, but during a wet interval in 1999–2000 (1,500 mm annual precipitation), the lakes expanded into the surrounding forest (Tucker, 2020). In 2017, the lake was fresh (conductivity of 261 μS/cm) and mesotrophic. The modern climate is semi-arid, with a mean temperature of 23°C and annual precipitation < 400 mm, which falls mostly during the austral summer (November – March) (Dewar and Richard, 2007). The sandy substrate of Quaternary alluvium in this area includes both active and vegetated dunes.
Figure 1. (A) Vegetation map showing the Namonte Basin, circumscribed by the blue rectangle. Inset shows the island of Madagascar, with the red dot in the southwest indicating the study area location. The vegetation map was modified from the Madagascar Vegetation Mapping Project (www.vegmad.org). Extent of the Mikea National Park is marked with a dashed line and includes primarily dry forest (orange). (B) Sentinel 2 satellite image of the outlined blue area in (A), showing the region of Namonte, SW Madagascar. The coring site is marked with a red and yellow circle in a portion of the Namonte Basin known as Andramby.
The landscape is generally characterized by a mosaic of wooded grasslands, dry deciduous and riverine forests, and mangroves that grow near estuaries. The dry deciduous forest is dominated by several plant species, including the endemic family Didiereaceae, the fony baobab tree (Adansonia fony), several species of Euphorbia, and Pachypodium geayi (Grubb, 2003; Aronson et al., 2018). The region is also habitat for several endemic primates, including the ring-tailed lemur (Lemur catta), Verreaux’s sifaka (Propithecus verreauxi), and the reddish-gray mouse lemur (Microcebus griseorufus). Other endemic species with restricted distributions include Grandidier’s mongoose (Galidictis grandidieri) and the Madagascar radiated tortoise (Geochelone radiata) (Scott et al., 2006). The Mikea Forest is part of a vegetation mosaic in southwest Madagascar that refers specifically to the dry forest between the urban centers of Toliara and Morombe (Seddon et al., 2000). The northern portion of this forest surrounds the Namonte Basin and has been part of the Mikea National Park since 2012.
Mikea people descend from herding clans settled in the area during the 17th century to escape banditry and oppression by the Maroseragna and Andrevola kings (Tucker et al., 2015). In the late 19th century, the area came under French colonial control, and much of the coastal plains to the east have since been used to produce a variety of cash crops such as maize, cotton, and butterbeans (Blanc-Pamard et al., 2005). Currently, most Mikea living in the Namonte Basin reside in villages that are usually located near lake margins, where they fish, cultivate crops, and herd zebu and goats. Mikea complement their diet with a range of forest products, including wild tubers (Dioscorea, Colocasia, Ipomoea, and Tacca), honey, and wild meat (e.g., birds, tortoises, tenrecs and feral cats; Tucker, 2007; Douglass and Rasolondrainy, 2021). Deforestation has intensified greatly since the 1970s, with ∼55% of the primary forest lost between 1949 and 2004 (Blanc-Pamard et al., 2005).
In 2017, a 138-cm-long composite sediment core was retrieved in the deepest part of the Namonte basin (Figure 1B). A large-diameter (7 cm ID) mud-water interface piston-corer (Fisher et al., 1992) was used for the collection of upper deposits and a Colinvaux-Vohnout-type corer, modified to use clear polycarbonate core barrels (5.4 cm ID), was used for deeper deposits. Unconsolidated upper deposits were maintained in a vertical orientation and extruded in the field at 2-cm intervals. The sediment core was shipped to the University of Florida (UF), where the deeper portion was split lengthwise and photographed. Samples were then shipped to Pennsylvania State University (PSU) for X-ray fluorescence (XRF), pollen, and charcoal analysis, and to the University of Regina (UR) for diatom analysis. The stratigraphy of the sediment core was characterized based on field observations and lab-acquired digital photographs. Elemental data were produced from XRF scans at 3-cm core intervals, using an Olympus DeltaX model X-ray fluorescence core scanner and Geotek MSCL 7.9 Multi-sensor Core Scanner Innoux 1776 at PSU.
A core chronology was constructed using 14C accelerator mass spectrometry (AMS) dates on five samples of terrestrial plant material (i.e., seeds, charcoal fragments and fragments of plant remains). Samples were pretreated following the protocol of Kennett et al. (2014) and dated at the Radiocarbon Laboratory at PSU. Radiocarbon dates were calibrated using the Southern Hemisphere calibration curve SHCAL20 (Hogg et al., 2020). For the topmost (recent) section of the core, 14C ages were calibrated using the post-bomb (SH 1-2 zone) dataset (Hua et al., 2013). Two dates, PSUAMS-6324 and PSUAMS-6325, were excluded from the age-depth model, as they contain modern (post-bomb) carbon and likely indicate translocation of recent plant material to greater depth during the coring process. Radiocarbon dates were used to generate a Bayesian age-depth model with the rbacon package (v. 2 3.3) for R (Blaauw and Christen, 2011), using default parameters [accumulation rate (acc.mean) = 10 year cm–1, shape distribution of accumulation rate (acc.shape) = 1.5, memory mean (mem.mean) = 0.7, memory strength (mem.strength) = 4] to the full depth of the core. The model was built under the assumption that the top of the sequence corresponds to the year of core retrieval (2017 CE).
The sediment core was subsampled for pollen and spores, microcharcoal, and diatom analysis at 4-cm intervals. Pollen, spores, and microscopic charcoal sample preparation followed standard protocols, including treatment with hydrofluoric acid and acetolysis (Faegri and Iversen, 1989). A tablet of exotic Lycopodium spores was added to each sample to calculate pollen concentration and pollen influx. A total of 36 samples were analyzed for pollen and fungal spores. In each sample, we identified at least 300 pollen grains if possible, excluding aquatic taxa. Pollen grains and fungal spores were identified using the reference collection at PSU and palynological atlases and keys (Gosling et al., 2013; Schüler and Hemp, 2016). To assess temporal changes in pollen taxa that may be correlated with megafaunal extinctions, we assigned a seed dispersal mechanism to each terrestrial pollen taxon as defined by Albert-Daviaud et al. (2020). We grouped plant taxa based on their mode of seed dispersal (e.g., autochorous = self-dispersed by dehiscent fruits, anemochorous = wind-dispersed, or zoochorous = animal-dispersed) (Knowles et al., 1995).
Microcharcoal (15–150 μm) concentrations were determined in the same samples used for pollen analysis. Microcharcoal particles and Lycopodium spores were quantified by randomly selecting 200 fields of view in each sample under 40× magnification. Each particle was characterized as woody or herbaceous based on morphology, including presence of stomata, epidermal cells, and an elongated shape (Walsh et al., 2014). Microcharcoal particles are presented as microcharcoal influx, calculated using charcoal concentrations and bulk sedimentation rates derived from the age-depth model.
For macroscopic charcoal analysis from 30 cm to the bottom of the core, continuous sediment subsamples (0.5 cm3) were retrieved. Samples were processed following the protocol of Mooney and Radford (2001). The remaining fraction was photographed using a digital camera attached to an Olympus microscope. ImageJ software1 was used to process sample images, quantify the total number of charcoal particles, and estimate total area of each charcoal particle (Halsall et al., 2018). Macrocharcoal was expressed as macrocharcoal influx (particles cm–2 yr–1) and macrocharcoal area (mm2 cm–2 yr–1) based on concentration, total area of charcoal particles, and bulk sediment accumulation rate at specific depths.
For diatom analysis, 0.2–0.5 g of dry sediment was processed following the protocol of Battarbee (1986). Permanent slides were mounted in Zrax (RI ∼1.7 +). At least 400 valves in each sample were counted. Diatom species were grouped according to ecological guilds and motility into High Profile (HP), High Profile Planktonic (HPP), Low Profile (LP) and Motile Profile (MP), following Passy (2007) and Berthon et al. (2011) (Supplementary Material 4). Although most of the studies on diatom functional groups and disturbance have been conducted in rivers and streams, the multi-proxy analyses presented in Velez et al. (2021) showed that they can be used to identify anthropogenic disturbances, including drainage and deforestation, with the consequent changes in water level, water transparency and macrophyte cover. These guilds reflect the forms and life habits of diatoms that are adaptations to particular physical (currents, turbidity) and chemical (nutrients) conditions (Leira et al., 2015; Algarte et al., 2016). Large and colonial forms are included in the HP, short or low-stature species are included in LP, and MP include species that can move. Motile species are very useful to infer physical disturbance such as an increase in water turbidity, shading and burial from excess sediment input (Jones et al., 2014).
Total pollen sum, excluding aquatics, and total diatom sum were used to calculate the relative abundances of each taxon in each group, respectively, which are presented as percentages. Pollen and diatom diagrams were created using Tilia software (Grimm, 1991). Zones representing thresholds of change in pollen and diatom composition were identified using stratigraphically constrained incremental sum of square clustering (CONISS) (Grimm, 1987). To assess changes in the abundance of plant taxa assigned to a specific seed dispersal mechanism, pollen percentages were normalized by applying a min-max and box-cox transformation and rescaled to z-scores to reduce skewness caused by high variability among samples. Elemental profiles from XRF data were normalized to Zr, because measures of other lithogenic elements Al and Ti were discontinuous due to low abundance. A Principal Component Analysis (PCA) was used to assess changes in XRF data throughout the stratigraphic section. All statistical analyses were performed in R version 3.5.1 (R Core Team, 2013).
The sediment core from Namonte is characterized by dark silt (130–0 cm) that overlies primarily medium quartz sand at the base (138–131 cm). The chronology was established based on three AMS radiocarbon ages determined on plant remains. These dates indicate that the sediment record spans ∼1,145 years (Table 1 and Figure 2). Two radiocarbon ages (PSUAMS-6326 and PSUAMS-3520) have relatively wide calibrated 2σ ranges (∼280 and 180 years, respectively), which contributes to high uncertainty in the modeled ages of the lower core section. The distribution of AMS 14C dates with depth suggests two stages of deposition. Continuous deposition occurred from the base of the core at 130 cm to 85 cm depth (0.22 cm yr–1 on average between ∼1,145 and 270 cal yr BP). Following this, there was a period of more rapid sedimentation (0.55 cm yr–1 on average from ∼270 cal yr BP to present). However, given that the estimated sedimentation rate in the upper section of the core was based on only two AMS dates, we interpreted changes during the recent phase of the chronology with caution.
Table 1. AMS Radiocarbon dates used to construct the age-depth model for Namonte core 22-IX-17-1B, southwest Madagascar.
Figure 2. Age-depth model from Namonte sediment core 22-IX-17-1B, southwest Madagascar. All 14C dates pictured in blue were calibrated with SHCAL20 (Hogg et al., 2020), the gray line represents the 95% confidence interval, and the red dotted line is the best-fit model based on a weighted mean. 14C dates indicated in red were excluded from the age-depth model. The sediment surface (-67 cal yr BP) was included in the Bayesian modeling.
The evidence suggests no obvious major depositional hiatuses or sediment mixing in the sediment core. The inference is based on observations that (1) the sediment lithology of the core is relatively homogenous throughout the sediment core; (2) pollen preservation is good, as less than 5% broken or shriveled pollen grains were observed in each sample; (3) seeds of Carex and other Cyperaceae were common in the core and diatoms were found in all samples, suggesting that the coring site has been continuously covered with water.
Elemental analysis of the Namonte sediment core documents differences between sediments from 129 to 60 cm depth and those from the overlying section (Supplementary Material 1). The lower core section possesses relatively abundant Ni and Cd, whereas the overlying section (60–33 cm depth) includes more Ca, K, Cl, Fe, and Zr. The first two principal components of a PCA conducted on the correlation matrix of all elemental profiles explain nearly 65% of the variance and highlight the distinct geochemical compositions of the lower section (129–60 cm) and upper sections (60–33 cm) of the core (Supplementary Materials 1, 2). PC1 (46.4% variance explained) values generally increase up-core, despite peaks in S that occur at 91–81 cm and 59–39 cm. The change in sign of PC1 values up-core at ∼60 cm depth coincides with decreases in Ca/Zr and Si/Zr values and increases in Fe/Zr and K/Zr (Figure 3). These measures are sensitive to authigenic carbonate precipitation, deposition of biogenic silica, and weathering regime. Specifically, relatively more Ca deposition may have been associated with carbonate precipitation during dry conditions and low lake levels (Haberzettl et al., 2007; Kylander et al., 2011), or associated with higher primary production. Increased runoff could deposit detrital calcite and increase Ca/Zr values, but the alluvium around Namonte is almost entirely quartz sand. Deposition of biogenic silica, with only traces of lithogenic elements such as Zr, can drive increases in Si/Zr values (Agnihotri et al., 2008; Dickson et al., 2010). Here, we consider that changes in Si/Zr are driven mainly by changes in diatom productivity, given that the diatom analysis revealed good diatom preservation and a concomitant shift in the diatom assemblage over time. Mobile elements such as K, and sometimes Fe, are more likely to accumulate and drive high K/Zr and Fe/Zr values during times of relatively greater physical, as opposed to chemical weathering (Piva et al., 2008; Kylander et al., 2011; Aufgebauer et al., 2012). Given that the lake is shallow, we suspect that it has been consistently well-oxygenated and that changes in redox conditions have likely contributed very little to variation in Fe/Zr values.
Figure 3. Depth versus XRF-PC1 and XRF-derived element abundances normalized to Zr, from the Namonte sediment core, southwest Madagascar. Mean values are indicated by vertical lines and pollen and diatom zones are marked by different colors.
We identified 78 pollen taxa in the sediment core from Namonte. Pollen preservation was good as each sample contained less than 5% damaged or shriveled pollen grains. Pollen concentration was variable throughout the core (mean = 41,800 grains cm–3), likely attributable to depth-associated variability in overall pollen preservation and/or bulk sedimentation rate (Zhao et al., 2009). Cluster analysis identified five major pollen zones (P1, P-2a and 2b, P-3, P-4) within the sequence (Figure 4). Poaceae was the most abundant pollen type through the entire record, followed by Euphorbia and Ephedra, suggesting that grasslands and the dry deciduous forest dominated the landscape for at least the last ∼1,145 years (Figure 4 and Table 2). Taxa characteristic of drylands (Ephedra, Uapaca, Anacardiaceae) were relatively common between ∼1,145 and 200 cal yr BP, followed by a transition period, between ∼190 and 20 cal yr BP. This transition was characterized by a decline in Ephedra and Euphorbia and sporadic appearance of Arecaceae, Ficus, Ericaceae, Pandanus, and Syzygium. Most recent zone P-4 is differentiated by a dramatic decrease in abundance of Ephedra and an increase in Prosopis, an indicator of soil disturbance.
Figure 4. Pollen relative abundance and algae concentration versus depth and calibrated radiocarbon age in the sediment core from Namonte, southwest Madagascar. CONISS analysis on the right side of the diagram shows the four major pollen zones, with zone P-2 divided into two subzones.
Charcoal particles were found in all the samples analyzed throughout the core, and numerous charcoal peaks were observed, indicating past fire activity (Figure 5). A macrocharcoal peak was recorded ∼1,040 cal yr BP and a second peak of macrocharcoal area occurred between ∼470 and 400 cal yr BP. Between ∼240 and 125 cal yr BP, both macrocharcoal influx and macrocharcoal area increased abruptly, followed by sharp decreases between 120 and 70 cal yr BP.
Figure 5. Microcharcoal influx (particles cm–2 yr–1), macrocharcoal influx (particles cm–2 yr–1), and macrocharcoal area (mm2 cm–2 yr–1) from the Namonte sediment core, southwest Madagascar.
Our analyses also revealed differential trends regarding relative abundances of taxa with distinct seed-dispersal pathways throughout the core. Pollen of plants with wind-dispersed seeds (anemochory) dominate the pollen assemblage and tend to increase over time (Figure 6). In contrast, pollen of taxa with seeds dispersed by animals (zoochory) fluctuate over time. Pollen from plant taxa dispersed only by lemurs show a relative decrease in more recent pollen zones P-3 and P-4, in comparison to earlier zones P-1, P-2a, and P-2b. Pollen of plants with seeds dispersed by lemurs and other animals show a relative increase in abundance during pollen P-2a, decreases in P-2a and P-3, and a slight increase in P-4.
Figure 6. Box-and-whisker plots of the normalized abundances (z-score) for pollen types, distinguished by seed dispersal mechanism, in the sediment core from Namonte, southwest Madagascar.
We identified 62 diatom species and grouped them according to functional groups. We identified three main diatom zones (D1-3), with zone D1 divided into sub-zones D1-a and D1-b (Figure 7) based on the cluster analysis, as described in Table 3.
Figure 7. Diatom relative abundance and functional group sums versus depth and calibrated radiocarbon age in the sediment core from Namonte, southwest Madagascar. CONISS analysis on the right side of the diagram shows the three major diatom zones, with zone D-1 divided into two subzones.
This multi-proxy environmental reconstruction enabled us to assess the role of Late Holocene climate change, human occupation, and megafaunal extinctions on terrestrial and aquatic ecosystems of southwest Madagascar (Figure 8). The pollen record shows that grasslands and dry deciduous forests dominated the vegetation for at least the last ∼1,145 years and that a major turnover in the plant community took place between ∼195 and 20 cal yr BP, when grasslands expanded and elements of the dry deciduous forest declined. The decline of forest taxa (Euphorbia and Ephedra) was accompanied by a relative increase in fire-resistant vegetation (Prosopis and Ericaceae), along with plants that thrive in disturbed soils (Amaranthaceae and Ambrosia) (Supplementary Material 3). The last ∼60 years were characterized by continued degradation of regional dry forests.
Figure 8. Abbreviated paleoecological diagram for the last ∼1,145 years, inferred from the Namonte core, southwest Madagascar. (A) Pollen percentages of disturbance taxa, grasslands, and dry forest, (B) percentages of diatom guilds, (C) PCA1 and PCA2 of XRF analysis, (D) Influx of macrocharcoal and microcharcoal particles, (E) Precipitation reconstruction from the Rodrigues Island, Indian Ocean, speleothem δ18O record (Li et al., 2020), and (F) chronology of faunal turnover (based on classical confidence intervals from Hixon et al., 2021a) and approximate human history based on archaeological, written and oral records (Douglass et al., 2019b).
Alterations to the aquatic environment are reflected by changes in the diatom assemblage. Between ∼270 and 70 cal yr BP, HP species were replaced by MP taxa, and C. meneghiniana became more abundant. This turnover in the composition of the diatom community suggests an increase in disturbance, likely associated with increasing water turbidity resulting from greater influx of soil particles from the watershed, increased organic input from cattle excreta, and/or from people, cattle, and other domestic animals resuspending sediments along the littoral zone of the lake.
The fossil pollen record shows that grasslands and dry forest were the dominant vegetation components between ∼1,145 and 545 cal yr BP (Zones P-1 and P-2a), when arid climate conditions prevailed in southwest Madagascar. The pollen assemblage was characterized by the presence of Poaceae, Euphorbia, Ephedra and other native taxa such as Cassia, Pandanus, Uapaca, and Syzygium. Regional speleothem records indicate that much of this period, from ∼900 to 400 cal yr BP, was characterized by successive megadrought episodes, attributed to northward shifts in the position of the Intertropical Convergence Zone (ITCZ) and changing phases of the Indian Ocean Dipole (IOD) (Figure 8, Virah-Sawmy et al., 2016; Li et al., 2020). Vegetation turnover was also documented during this period at other sites on the island, and it has typically been attributed to the displacement of the ITCZ. For example, near Lakes Mitsinjo and Anjohibe, grasslands expanded ∼1,000 cal yr BP (Matsumoto and Burney, 1994; Crowley and Samonds, 2013), and, near Andolonomby, xerophytic bush intermixed with palm trees expanded between ∼1,000 and 500 cal yr BP (Virah-Sawmy et al., 2016).
The XRF analysis of the core identified relatively high Ca/Zr values during the period between ∼1,145 and 400 cal yr BP, which likely was a consequence of drier conditions that resulted in CaCO3 precipitation (Haberzettl et al., 2007; Kylander et al., 2011). Aridity during the start of this interval is consistent with records from East Africa that document relatively dry conditions during the Medieval Warm Period (1000-700 cal yr BP), followed by a wetter interval coincident with the latter part of the Little Ice Age (∼300 cal yr BP) (Newton et al., 2006; Tierney et al., 2013).
The diatom assemblage also indicates relatively stable shallow lacustrine conditions between ∼1,145 and 270 cal yr BP (Figure 8, Sub-zones D-1a and D1b). Dominance of HP diatoms, especially S. construens, during that time suggests relatively little disturbance (Passy, 2007). Similar patterns have been observed in other records, including the one from Lake Tritrivakely, Madagascar (Gasse and Van Campo, 2001). Presence of S. construens var. venter, S. pinnata, P. parasitica, high abundance of pollen of sedges (Carex and other Cyperaceae) and high concentrations of the green alga Botryococcus, also suggest shallow and mesotrophic to eutrophic waters (Supplementary Material 4) (Wünnemann et al., 2010). An increase in Nymphaea (water lily) implies a slight increase in water levels between ∼400 and 200 cal yr BP. The persistence of taxa in the paleoecological record throughout this period suggests that both terrestrial and aquatic ecosystems were resistant in the face of a relative decrease in precipitation that impacted southern Madagascar.
A major change in terrestrial plant composition took place between ∼405 and 50 cal yr BP (P-2b and P-3). During that period, grasslands expanded and fire-resistant taxa (Ericaceae and Prosopis) and disturbance taxa (Amaranthaceae and Ambrosia) became more abundant. Vegetation turnover was associated with an increase in fire activity at both local and regional scales, as expressed by increases in both macro- and microcharcoal influx. This shift in vegetation and fire activity coincided with two dry periods recorded in speleothem δ18O and in Adansonia tree-ring δ13C values from ∼320 to 260 cal yr BP and ∼100 to 0 cal yr BP in northwest and southwest Madagascar, respectively (Scroxton et al., 2017; Razanatsoa, 2019). A similar vegetation trend was recorded at Lake Longiza, where grasslands expanded in association with an abrupt increase in charcoal particles and coprophilous fungi between 580 and 30 cal yr BP (Razanatsoa et al., 2021b). These results likely indicate that vegetation turnover was a consequence of changing climate conditions that favored ignition of vegetation and spread of fires, but changes in human activities may have also driven vegetation change and a decline in dry forest extent.
Changes in terrestrial ecosystems between ∼180 and 70 cal yr BP coincided with a dramatic turnover in the freshwater diatom community of the lake (D-2). MP diatoms increased in abundance during that period, which likely indicates increased physical disturbance of the aquatic environment, possibly by higher soil erosion in the watershed and increased detrital input to the lake (Jones et al., 2014). Motile diatoms fare relatively well during physical disturbance, because they possess the ability to move away from disturbed areas (Jones et al., 2014) and compete well for nutrients in eutrophic environments (Passy, 2007). Higher sediment input, most likely from soil erosion, is supported by relatively rapid sedimentation rates (0.54 cm yr–1) and high K/Zr and Fe/Zr values (Piva et al., 2008; Kylander et al., 2011; Aufgebauer et al., 2012).
The diatom assemblage also suggests increased variation in lake levels and water quality. C. meneghiniana increases in abundance ∼90 cal yr BP, likely associated with an increase in water turbidity (Hassan, 2013). Species of Eunotia and Pinnularia also become more common during that time, which could indicate periods of low water level (Gasse and Van Campo, 2001). The turnover in the diatom community suggests significant changes in the physical and chemical characteristics of the lake around ∼180 cal yr BP, which persisted until ∼70 cal yr BP. Relatively low Si/Zr values in sediment since ∼180 cal yr BP reflect relatively low deposition of biogenic silica, which may have resulted from a combination of declines in diatom production and increased detrital input (Agnihotri et al., 2008; Dickson et al., 2010). Further geochemical studies on the lake sediment will provide additional information regarding the factors that influence biogenic silica variation through time.
During the last ∼70 years, the dry deciduous forest declined, while grasslands expanded, and extra-local taxa increased. The presence of Amaranthaceae, Ambrosia, and Prosopis suggests degradation of dry forests associated with the establishment of species that are resistant to fire, trampling, and browsing pressures. The aquatic community of Namonte also displays evidence of considerable change during this time. Increases in abundance of colonizers S. pinnata, and A. minutissimum (Berthon et al., 2011) and LP and MP species, indicate intermittent physical disturbance, possibly associated with habitat degradation, highly variable lake levels, and environmental changes in the watershed (Passy, 2007; Berthon et al., 2011; Velez et al., 2021). High variation in the abundance of sedges and a relative increase in the abundance of Typha also support an inference for changes in lake level and periodic swampy conditions. These changes were previously linked to overgrazing and social conflict in the region, which forced local people into sedentism (Irwin et al., 2010). Ecosystem degradation today is likely exacerbated by aridification associated with climate change and abandonment of traditional land-management practices (Tucker et al., 2010; Virah-Sawmy et al., 2016; Razanatsoa et al., 2021a).
Charcoal particles are useful proxies for past fire activity. Macrocharcoal is usually considered evidence of local fires, and microcharcoal as evidence of more distant fires (Clark and Royall, 1996). The spatial scale reflected by charcoal particle size depends on the environmental characteristics of the site, such as topography and predominant wind direction (Whitlock and Larsen, 2002). In the case of Namonte, macrocharcoal particles could originate from burning within the small lake catchment or from fires on the coast, ∼11 km to the west. Fires that burn areas > 0.02 km2 can produce convective columns that extend > 1 km into the air and are capable of transporting macrocharcoal as much as ∼10 km (Clark, 1988). Thus, macrocharcoal recovered from Namonte probably came from the lake basin proper and/or the coast. In contrast, microcharcoal can travel several to > 100 km from its source, so microcharcoal in Namonte may have traveled from fires at considerable distances from the waterbody (Clark, 1988).
High values of charcoal particles suggest that fire activity was widespread around Namonte and in nearby areas during the last ∼1,145 years (average microcharcoal influx: 1,435,304 particles cm–2 yr–1). Macrocharcoal particles were common between 1,145 and 1,000 cal yr BP, at a time when hunter-gatherers and possibly early pastoralists were present in the region (Douglass et al., 2019a; Hixon et al., 2021a). Large concentrations of macrocharcoal (> 200 particles cm–3) during that early period indicate the occurrence of large fires near Namonte. Although it is possible that people used fire for hunting and possibly land clearing for livestock grazing, arid conditions likely favored the ignition and spread of natural fires in the fire-prone grasslands (Virah-Sawmy et al., 2009). The relatively great abundance of charcoal of all size classes suggests both high fire activity within the lake catchment and long-distance transport, perhaps from the coast, associated with foraging (Douglass et al., 2018).
Between ∼1,000 and 70 cal yr BP, macrocharcoal values increased dramatically, with a peak between ∼205 and 130 cal yr BP (macrocharcoal influx = 4,303 particles cm–2 yr–1, macrocharcoal area = 1,392 mm2 cm–2 yr–1), suggesting continued large-scale landscape transformation near Namonte. High charcoal influx overlaps with a decline in tree taxa, suggesting that forests were intentionally burned to expand grasslands for zebu and ovicaprids (Douglass et al., 2018; Hixon et al., 2021a,b). A peak in macrocharcoal area (average 1,896 mm2 cm–2 yr–1) between ∼460 and 425 cal yr BP could also indicate intense fire activity associated with vegetation clearing by local pastoralists and management of grasslands. The abundance of macrocharcoal particles (2,600 ± 4,207 particles cm–3) is similar to that found in sediments associated with large-scale landscape clearance (> 200 particles cm–3) in Mauritius (Gosling et al., 2017). Moreover, a similar increase in fire activity was observed ∼589 cal yr BP at Lake Longiza and ∼450 cal yr BP in southeast Madagascar, during a period of cultural transformation, when the size of settlements increased and cattle herding activity intensified (Razanatsoa et al., 2021b).
A large peak in woody and herbaceous microcharcoal influx (average 2,395,921 and 227,006 particles cm–2 yr–1, respectively) is observed between ∼230 and 35 cal yr BP. Intense fire activity during that period may have been the product of maintenance of grazing areas for herds of zebu. Microcharcoal influx decreased over time but remained relatively high through the period of French colonization (1897–1958 CE) and following the establishment of the Malagasy Republic (1958 to present). Despite colonial and post-Independence state-imposed sanctions designed to discourage burning and reduce deforestation and soil erosion (Kull and Laris, 2009), paleoecological and historic records show that fires remained common in the region. The period of French colonization was characterized by rapid and widespread resource exploitation, such as maize production, that involved changes in the local economy and impacted the livelihoods of native people (Wietzke, 2015). During the last 40 years, fire activity has decreased near Namonte. This could be the consequence of changes in pastoral practices and intensification of cash-cropping (Scales, 2011). Persistence of charcoal in the record, however, reflects continued use of fire to prepare fields for agriculture, produce fuel charcoal, control pests, mitigate the impacts of wildfires, and protest political and economic regimes (Kull, 2002; Kull and Laris, 2009).
Results from this study suggest that the landscape transformation was the product of fire intensification, likely associated with the management of grasslands for introduced cattle (Bloesch, 1999; Kull, 2002; Davis and Douglass, 2021). High concentrations of charcoal particles are associated with the concomitant increase of Poaceae (∼65%) over time in the region. A similar pattern was detected in sediment deposited between 1,250 and 850 cal yr BP in several water bodies across Madagascar, including Lakes Amparihibe, Kavitaha, Longiza, Mitsinjo, Ranobe, and Tritrivakely (Burney, 1987a; Matsumoto and Burney, 1994; Gasse and Van Campo, 1998; Burney et al., 2004; Hixon et al., 2021b; Razanatsoa et al., 2021b). This evidence indicates a close relationship between forest burning and grassland expansion and the important role that herders played in the transformation of the landscape during the last millennium.
Several megafauna (> 45 kg) species inhabited southwest Madagascar during the Late Holocene, including giant lemurs (e.g., Megaladapis edwardsi and Hadropithecus stenognathus), elephant birds (e.g., Mullerornis modestus and Aepyornis spp.), pygmy hippos (Hippopotamus spp.), and giant tortoises (Aldabrachelys spp.) (Crowley, 2010; Goodman and Jungers, 2014). These species played key ecological roles, including seed dispersal of native plants, maintenance of grasslands through grazing, and nutrient cycling in aquatic and terrestrial ecosystems (Godfrey et al., 2008; Godfrey and Crowley, 2016). Directly 14C-dated assemblages of megafauna bones from southwest Madagascar suggest that populations of large animals started to decline by ∼2,500 cal yr BP and disappeared from the record by ∼1000 cal yr BP (Crowley, 2010; Hansford et al., 2021; Hixon et al., 2021a). The long-term ecological consequences of these extinctions provide critical context for current conservation and reintroduction/rewilding efforts, yet they remain poorly understood (Pedrono et al., 2013; Godfrey et al., 2019).
Southwest Madagascar is inhabited by several tree species that rely on large-bodied animals for seed dispersal (e.g., species of Adansonia, Didiereaceae, Arecaceae) (Albert-Daviaud et al., 2020). Remnant lemur species, introduced bushpigs, and people may continue to disperse endemic seeds, yet multiple plant taxa are considered to have been “orphaned” by the extinction of their seed dispersers (Federman et al., 2016). In Namonte, the pollen record reveals the legacy of megafauna extinction on terrestrial ecosystem composition. The abundance of plant taxa dispersed exclusively by animals, particularly lemurs, decreased from ∼1,145 cal yr BP to present. In contrast, plants with wind-dispersed seeds became more abundant over time. Reduced coverage of dry forests may have been, in part, a consequence of megafauna extinctions, because: (1) several plant species lost their seed dispersers, which negatively impacted their life cycles by creating a bottleneck for seed germination and seedling recruitment; (2) the seed shadow pattern was altered, resulting in limited dispersal in both space and time; and (3) the population genetic structure of plant species was negatively affected because gene flow via seed movement was restricted (Guimarães et al., 2008; Pires et al., 2018). Plants that lost dispersers prior to 1,100 cal yr BP may today be more vulnerable to extinction in the face of climate change and land use intensification because they are unable to increase their geographic distribution.
Burney et al. (2003) suggested that a decline in megafauna probably led to proliferation of fires in the dry forest, but results from our study do not show a clear connection between fire prevalence and loss of large animals. Both the microcharcoal and macrocharcoal records from Namonte show that fires were widespread in the region during the last ∼1,145 years. The high incidence of fire over the last millennium was likely a consequence of arid climate conditions, combined with the settlement of agro-pastoralists, decline of megafauna, and expansion of grasslands in the region.
The paleoecological record in southwest Madagascar suggests that environmental impacts of anthropogenic activities were significant over the last ∼1,145 years. People have managed and modified this landscape over the last millennium, particularly following settlement of agro-pastoralists and the introduction of zebu and ovicaprids. Extensive cattle pastoralism evidently involved the use of fire to promote regrowth of palatable grasses at the expense of dry forest taxa. Pastoralism also impacted aquatic diatom communities, which probably responded to the effects of deforestation, soil erosion, and organic input to the lake. Interactions among humans, an increasingly arid climate, and disappearance of endemic megafauna, likely favored the establishment of fire-resistant vegetation and the reconfiguration of vegetation communities in the Malagasy drylands. This study highlights the advantages of integrating multiple paleoecological, archaeological and historical records to make inferences about long-term legacies of climate change and anthropogenic activities on terrestrial and aquatic ecosystems. It also highlights the vulnerability of insular ecosystems to intensifying anthropogenic impacts today.
The original contributions presented in the study are included in the article/Supplementary Material, further inquiries can be directed to the corresponding author.
SH, MB, JC, and DK designed the research and collected the samples. KD organized and managed fieldwork. AD, SH, MV, and BC analyzed the samples. AD, SH, and MV wrote the draft of the manuscript. SI and KD assisted with the analysis. All authors commented, provided input to the manuscript, and approved the submitted version.
Funding of this study was facilitated by National Science Foundation grants GRFP – 2015213455 (SH), Archaeology DDRI – 1838393 (DK and SH), and NSF-IES 1840968 (SI).
The authors declare that the research was conducted in the absence of any commercial or financial relationships that could be construed as a potential conflict of interest.
All claims expressed in this article are solely those of the authors and do not necessarily represent those of their affiliated organizations, or those of the publisher, the editors and the reviewers. Any product that may be evaluated in this article, or claim that may be made by its manufacturer, is not guaranteed or endorsed by the publisher.
First and foremost, we thank the communities of the Namonte area for allowing us to engage in this research and for providing support to the research team. We thank Laurie Eccles, Damy Delphin, George Manahira, Azaria Davis, and Madeline Helmbrecht and the Morombe Archaeological Project for their contributions to this project. Sampling was conducted with authorization of the Ministry of Mines (permit #001/2017), the Ministry of the Environment and Madagascar National Parks (permit #208/17/MEEF/SG/DGF/DSAP/SCB.Re), the National Office for the Environment (permit #728/17/MEEF/ONE/DG/DEE.rva), the Ministry of Higher Education and Scientific Research (permit #211/17-MESupReS/SG/DGRS), the Ministry to the Presidency in Charge of Mines & Petroleum (permit #277/MPMP/SG/DIR.A.A.A) and the University of Tulear’s CEDRATOM Museum.
The Supplementary Material for this article can be found online at: https://www.frontiersin.org/articles/10.3389/fevo.2021.688512/full#supplementary-material
Supplementary Material 1 | Principal component analysis of XRF data from the Namonte sediment core, southwest Madagascar, with loadings illustrated by vectors. The lower core section (129-60 cm depth), corresponds to diatom zone D-1 and pollen zones P-1 and P-2, has a geochemical composition that is distinct from the upper core section (60-33 cm depth), which corresponds to diatom zone D-2 and pollen zone Z-3.
Supplementary Material 2 | XRF data from the Namonte sediment core, southwest Madagascar.
Supplementary Material 3 | Ecology of the main plant taxa found in the Namonte sediment record, southwest Madagascar (Vincens et al., 2007).
Supplementary Material 4 | Ecology of the main diatoms found in the Namonte sediment record, southwest Madagascar.
Agnihotri, R., Altabet, M. A., Herbert, T. D., and Tierney, J. E. (2008). Subdecadally resolved paleoceanography of the Peru margin during the last two millennia. Geochem. Geophys. Geosyst. 9:Q05013.
Albert-Daviaud, A., Buerki, S., Onjalalaina, G. E., Perillo, S., Rabarijaona, R., Razafindratsima, O. H., et al. (2020). The ghost fruits of Madagascar: identifying dysfunctional seed dispersal in Madagascar’s endemic flora. Biol. Conserv. 242:108438. doi: 10.1016/j.biocon.2020.108438
Algarte, V. M., Dunck, B., Leandrini, J. A., and Rodrigues, L. (2016). Periphytic diatom ecological guilds in floodplain: ten years after dam. Ecol. Indic. 69, 407–414.
Anderson, A., Clark, G., Haberle, S., Higham, T., Nowak-Kemp, M., Prendergast, A., et al. (2018). New evidence of megafaunal bone damage indicates late colonization of Madagascar. PLoS One 13:e0204368. doi: 10.1371/journal.pone.0204368
Aronson, J. C., Phillipson, P. B., Le Floc’h, E., and Raminosoa, T. (2018). Dryland tree data for the southwest region of Madagascar: alpha-level data can support policy decisions for conserving and restoring ecosystems of arid and semiarid regions. Madag. Conserv. Dev. 13, 60–69.
Aufgebauer, A., Panagiotopoulos, K., Wagner, B., Schaebitz, F., Viehberg, F. A., Vogel, H., et al. (2012). Climate and environmental change in the Balkans over the last 17 ka recorded in sediments from Lake Prespa (Albania/FYR of Macedonia/Greece). Quat. Int. 274, 122–135.
Bamford, A. J., Razafindrajao, F., Young, R. P., and Hilton, G. M. (2017). Profound and pervasive degradation of Madagascar’s freshwater wetlands and links with biodiversity. PLoS One 12:e0182673. doi: 10.1371/journal.pone.0182673
Battarbee, R. W. (1986). “Diatom analysis,” in Handbook of Holocene Palaeoecology and Palaeohydrology, ed. B. E. Berglund (London: John Wiley and Sons Ltd), 527–570.
Berthon, V., Bouchez, A., and Rimet, F. (2011). Using diatom life-forms and ecological guilds to assess organic pollution and trophic level in rivers: a case study of rivers in south-eastern France. Hydrobiologia 673, 259–271.
Blaauw, M., and Christen, J. A. (2011). Flexible paleoclimate age-depth models using an autoregressive gamma process. Bayesian Anal. 6, 457–474. doi: 10.1214/ba/1339616472
Blanc-Pamard, C., Milleville, P., Grouzis, M., Lasry, F., and Razanaka, S. (2005). Une alliance de disciplines sur une question environnementale: la déforestation en forêt des Mikea (Sud-Ouest de Madagascar). Nat. Sci. Soc. 13, 7–20.
Bloesch, U. (1999). Fire as a tool in the management of a savanna/dry forest reserve in Madagascar. Appl. Veg. Sci. 2, 117–124. doi: 10.2307/1478888
Braje, T. J., Leppard, T. P., Fitzpatrick, S. M., and Erlandson, J. M. (2017). Archaeology, historical ecology and anthropogenic island ecosystems. Environ. Conserv. 44, 286–297. doi: 10.1017/s0376892917000261
Burney, D., James, H., Grady, F., Rafamantanantsoa, J.-G., Wright, H., and Cowart, J. (1997). Environmental change, extinction and human activity: evidence from caves in NW Madagascar. J. Biogeogr. 24, 755–767. doi: 10.1046/j.1365-2699.1997.00146.x
Burney, D. A. (1987b). Late Quaternary stratigraphic charcoal records from Madagascar. Quat. Res. 28, 274–280. doi: 10.1016/0033-5894(87)90065-2
Burney, D. A. (1987a). Late Holocene vegetational change in central Madagascar. Quat. Res. 28, 130–143. doi: 10.1016/0033-5894(87)90038-x
Burney, D. A., Burney, L. P., Godfrey, L. R., Jungers, W. L., Goodman, S. M., Wright, H. T., et al. (2004). A chronology for late prehistoric Madagascar. J. Hum. Evol. 47, 25–63. doi: 10.1016/j.jhevol.2004.05.005
Burney, D. A., Robinson, G. S., and Burney, L. P. (2003). Sporormiella and the late Holocene extinctions in Madagascar. Proc. Natl. Acad. Sci., 100, 10800–10805. doi: 10.1073/pnas.1534700100
Burns, S. J., Godfrey, L. R., Faina, P., McGee, D., Hardt, B., Ranivoharimanana, L., et al. (2016). Rapid human-induced landscape transformation in Madagascar at the end of the first millennium of the Common Era. Quat. Sci. Rev. 134, 92–99. doi: 10.1016/j.quascirev.2016.01.007
Clark, J. S. (1988). Particle motion and the theory of charcoal analysis: source area, transport, deposition, and sampling. Quat. Res. 30, 67–80. doi: 10.1016/0033-5894(88)90088-9
Clark, J. S., and Royall, P. D. (1996). Local and regional sediment charcoal evidence for fire regimes in presettlement north-eastern North America. J. Ecol. 84, 365–382. doi: 10.2307/2261199
Crowley, B. E. (2010). A refined chronology of prehistoric Madagascar and the demise of the megafauna. Quat. Sci. Rev. 29, 2591–2603. doi: 10.1016/j.quascirev.2010.06.030
Crowley, B. E., and Samonds, K. E. (2013). Stable carbon isotope values confirm a recent increase in grasslands in northwestern Madagascar. Holocene 23, 1066–1073. doi: 10.1177/0959683613484675
Crowther, A., Lucas, L., Helm, R., Horton, M., Shipton, C., Wright, H. T., et al. (2016). Ancient crops provide first archaeological signature of the westward Austronesian expansion. Proc. Natl. Acad. Sci. U.S.A. 113, 6635–6640. doi: 10.1073/pnas.1522714113
Davis, D., and Douglass, K. (2021). Remote sensing reveals lasting legacies of land use by small-scale communities in the southwestern Indian Ocean. Front. Ecol. Evol. 9:689399. doi: 10.3389/fevo.2021.689399
Dewar, R. E., and Richard, A. F. (2007). Evolution in the hypervariable environment of Madagascar. Proc. Natl. Acad. Sci. U.S.A. 104, 13723–13727. doi: 10.1073/pnas.0704346104
Dickson, A. J., Leng, M. J., Maslin, M. A., and Röhl, U. (2010). Oceanic, atmospheric and ice-sheet forcing of South East Atlantic Ocean productivity and South African monsoon intensity during MIS-12 to 10. Quat. Sci. Rev. 29, 3936–3947. doi: 10.1016/j.quascirev.2010.09.014
Douglass, K., Antonites, A. R., Quintana Morales, E. M., Grealy, A., Bunce, M., Bruwer, C., et al. (2018). Multi-analytical approach to zooarchaeological assemblages elucidates Late Holocene coastal lifeways in southwest Madagascar. Quat. Int. 471, 111–131. doi: 10.1016/j.quaint.2017.09.019
Douglass, K., and Cooper, J. (2020). Archaeology, environmental justice, and climate change on islands of the Caribbean and southwestern Indian Ocean. Proc. Natl. Acad. Sci. U.S.A. 117, 8254–8262. doi: 10.1073/pnas.1914211117
Douglass, K., Hixon, S., Wright, H. T., Godfrey, L. R., Crowley, B. E., Manjakahery, B., et al. (2019a). A critical review of radiocarbon dates clarifies the human settlement of Madagascar. Quat. Sci. Rev. 221:105878. doi: 10.1016/j.quascirev.2019.105878
Douglass, K., Walz, J., Quintana Morales, E., Marcus, R., Myers, G., and Pollini, J. (2019b). Historical perspectives on contemporary human–environment dynamics in southeast Africa. Conserv. Biol. 33, 260–274. doi: 10.1111/cobi.13244
Douglass, K., and Rasolondrainy, T. (2021). Social memory and niche construction in a hypervariable environment. Am. J. Hum. Biol. 33:e23557. doi: 10.1002/ajhb.23557
Douglass, K., and Zinke, J. (2015). Forging ahead by land and by sea: archaeology and paleoclimate reconstruction in Madagascar. Afr. Archeol. Rev. 32, 267–299. doi: 10.1007/s10437-015-9188-5
Federman, S., Dornburg, A., Daly, D. C., Downie, A., Perry, G. H., Yoder, A. D., et al. (2016). Implications of lemuriform extinctions for the Malagasy flora. Proc. Natl. Acad. Sci. U.S.A. 113, 5041–5046. doi: 10.1073/pnas.1523825113
Feranec, R. S., Miller, N. G., Lothrop, J. C., and Graham, R. W. (2011). The Sporormiella proxy and end-Pleistocene megafaunal extinction: a perspective. Quat. Int. 245, 333–338. doi: 10.1016/j.quaint.2011.06.004
Fisher, M. M., Brenner, M., and Reddy, K. R. (1992). A simple, inexpensive piston corer for collecting undisturbed sediment/water interface profiles. J. Paleolimnol. 7, 157–161.
Fritz-Vietta, N. V. M., Tahirindraza, H. S., and Stoll-Kleemann, S. (2017). Local people’s knowledge with regard to land use activities in southwest Madagascar – conceptual insights for sustainable land management. J. Environ. Manage. 199, 126–138. doi: 10.1016/j.jenvman.2017.05.034
Gasse, F., and Van Campo, E. (1998). A 40,000-yr pollen and diatom record from Lake Tritrivakely, Madagascar, in the southern tropics. Quat. Res. 49, 299–311. doi: 10.1006/qres.1998.1967
Gasse, F., and Van Campo, E. (2001). Late Quaternary environmental changes from a pollen and diatom record in the southern tropics (Lake Tritrivakely, Madagascar). Palaeogeogr. Palaeoclimatol. Palaeoecol. 167, 287–308. doi: 10.1016/s0031-0182(00)00242-x
Godfrey, L. R., and Crowley, B. E. (2016). Madagascar’s ephemeral palaeo-grazer guild: who ate the ancient C4 grasses? Proc. R. Soc. B Biol. Sci. 283:20160360. doi: 10.1098/rspb.2016.0360
Godfrey, L. R., Jungers, W. L., Schwartz, G. T., and Irwin, M. T. (2008). “Ghosts and orphans,” in Elwyn Simons: A Search for Origins, eds J. G. Fleagle and C. C. Gilbert (New York, NY: Springer), 361–395.
Godfrey, L. R., Scroxton, N., Crowley, B. E., Burns, S. J., Sutherland, M. R., Pérez, V. R., et al. (2019). A new interpretation of Madagascar’s megafaunal decline: the “Subsistence Shift Hypothesis.”. J. Hum. Evol. 130, 126–140. doi: 10.1016/j.jhevol.2019.03.002
Goodman, S. M., and Jungers, W. L. (2014). Extinct Madagascar: Picturing the Island’s Past. Chicago, IL: University of Chicago Press.
Goodman, S. M., Raherilalao, M. J., and Wohlhauser, S. (2018). The Terrestrial Protected Areas of Madagascar: Their History, Description, and Biota. Antananarivo: Assoc. Vahatra.
Gosling, W. D., de Kruif, J., Norder, S. J., de Boer, E. J., Hooghiemstra, H., Rijsdijk, K. F., et al. (2017). Mauritius on fire: tracking historical human impacts on biodiversity loss. Biotropica 49, 778–783. doi: 10.1111/btp.12490
Gosling, W. D., Miller, C. S., and Livingstone, D. A. (2013). Atlas of the tropical West African pollen flora. Rev. Palaeobot. Palynol. 199, 1–135. doi: 10.1016/j.revpalbo.2013.01.003
Grimm, E. C. (1987). CONISS: a FORTRAN 77 program for stratigraphically constrained cluster analysis by the method of incremental sum of squares. Comput. Geosci. 13, 13–35.
Grubb, P. J. (2003). Interpreting some outstanding features of the flora and vegetation of Madagascar. Perspect. Plant Ecol. Evol. Syst. 6, 125–146. doi: 10.1078/1433-8319-00046
Guimarães, P. R. Jr., Galetti, M., and Jordano, P. (2008). Seed dispersal anachronisms: rethinking the fruits extinct megafauna ate. PLoS One 3:e1745. doi: 10.1371/journal.pone.0001745
Haberzettl, T., Corbella, H., Fey, M., Janssen, S., Lücke, A., Mayr, C., et al. (2007). Lateglacial and Holocene wet—dry cycles in southern Patagonia: chronology, sedimentology and geochemistry of a lacustrine record from Laguna Potrok Aike, Argentina. Holocene 17, 297–310.
Halsall, K. M., Ellingsen, V. M., Asplund, J., Bradshaw, R. H., and Ohlson, M. (2018). Fossil charcoal quantification using manual and image analysis approaches. Holocene 28, 1345–1353. doi: 10.1177/0959683618771488
Hannah, L., Dave, R., Lowry, P. P., Andelman, S., Andrianarisata, M., Andriamaro, L., et al. (2008). Climate change adaptation for conservation in Madagascar. Biol. Lett. 4, 590–594. doi: 10.1098/rsbl.2008.0270
Hansford, J., Wright, P. C., Rasoamiaramanana, A., Pérez, V. R., Godfrey, L. R., Errickson, D., et al. (2018). Early Holocene human presence in Madagascar evidenced by exploitation of avian megafauna. Sci. Adv. 4:eaat6925. doi: 10.1126/sciadv.aat6925
Hansford, J. P., Lister, A. M., Weston, E. M., and Turvey, S. T. (2021). Simultaneous extinction of Madagascar’s megaherbivores correlates with late Holocene human-caused landscape transformation. Quat. Sci. Rev. 263:106996. doi: 10.1016/j.quascirev.2021.106996
Harvey, C. A., Rakotobe, Z. L., Rao, N. S., Dave, R., Razafimahatratra, H., Rabarijohn, R. H., et al. (2014). Extreme vulnerability of smallholder farmers to agricultural risks and climate change in Madagascar. Philos. Trans. R. Soc. B Biol. Sci. 369:20130089. doi: 10.1098/rstb.2013.0089
Hassan, G. S. (2013). Diatom-based reconstruction of middle to late Holocene paleoenvironments in Lake Lonkoy, southern Pampas, Argentina. Diatom Res. 28, 473–486. doi: 10.1080/0269249x.2013.851118
Hixon, S. W., Douglass, K. G., Crowley, B. E., Rakotozafy, L. M. A., Clark, G., Anderson, A., et al. (2021a). Late Holocene spread of pastoralism coincides with megafaunal extinction on Madagascar. Proc. R. Soc. B Biol. Sci. 288:20211204. doi: 10.1098/rspb.2021.1204
Hixon, S. W., Curtis, J. H., Brenner, M., Douglass, K. G., Domic, A. I., Culleton, B. J., et al. (2021b). Drought coincided with, but does not explain, Late Holocene megafauna extinctions in SW Madagascar. Climate 9:138. doi: 10.3390/cli9090138
Hogg, A. G., Heaton, T. J., Hua, Q., Palmer, J. G., Turney, C. S., Southon, J., et al. (2020). SHCal20 Southern Hemisphere calibration, 0–55,000 years cal BP. Radiocarbon 62, 759–778. doi: 10.1017/rdc.2020.59
Hua, Q., Barbetti, M., and Rakowski, A. Z. (2013). Atmospheric radiocarbon for the period 1950–2010. Radiocarbon 55, 2059–2072. doi: 10.2458/azu_js_rc.v55i2.16177
Irwin, M. T., Wright, P. C., Birkinshaw, C., Fisher, B. L., Gardner, C. J., Glos, J., et al. (2010). Patterns of species change in anthropogenically disturbed forests of Madagascar. Biol. Conserv. 143, 2351–2362. doi: 10.1016/j.biocon.2010.01.023
Jones, J. I., Duerdoth, C. P., Collins, A. L., Naden, P. S., and Sear, D. A. (2014). Interactions between diatoms and fine sediment. Hydrol. Process. 28, 1226–1237. doi: 10.1002/hyp.9671
Kaufmann, J. C., and Tsirahamba, S. (2006). Forests and thorns: conditions of change affecting Mahafale pastoralists in southwestern Madagascar. Conserv. Soc. 4, 231–261.
Kennett, D. J., Culleton, B. J., Dexter, J., Mensing, S. A., and Thomas, D. H. (2014). High-precision AMS 14C chronology for gatecliff shelter, Nevada. J. Archaeol. Sci. 52, 621–632. doi: 10.1016/j.jas.2014.06.008
Kihara, Y., Sahashi, Y., Arita, S., and Ohtsuka, T. (2009). Diatoms of Yamakado Moor in Shiga prefecture, Japan. Diatom 25, 91–105.
Knowles, O. H., Knowles, D., Parrotta, J. A., and Parotta, J. (1995). Amazonian forest restoration: an innovative system for native species selection based on phenological data and field performance indices. Commonw. For. Rev. 74, 230–243.
Krammer, K., and Lange-Bertalot, H. (1991). “Bacillariophyceae. 3. Teil: Centrales, Fragilariaceae, Eunotiaceae,” in Süsswasserflora von Mitteleuropa, Band 2/3, eds J. Gerloff, J. Heynig, D. Mollenhauer, and H. Ettl (Stuttgart: Gustav Fischer Verlag).
Kull, C. A. (2000). Deforestation, erosion, and fire: degradation myths in the environmental history of Madagascar. Environ. Hist. 6, 423–450. doi: 10.3197/096734000129342361
Kull, C. A. (2002). Madagascar aflame: landscape burning as peasant protest, resistance, or a resource management tool? Polit. Geogr. 21, 927–953. doi: 10.1016/S0962-6298(02)00054-9
Kull, C. A., and Laris, P. (2009). “Fire ecology and fire politics in Mali and Madagascar,” in Tropical Fire Ecology, ed. M. A. Cochrane (Berlin: Springer), 171–226. doi: 10.1007/978-3-540-77381-8_7
Kull, C. A., Tassin, J., Moreau, S., Rakoto Ramiarantsoa, H., Blanc-Pamard, C., and Carrière, S. M. (2012). The introduced flora of Madagascar. Biol. Invasions 14, 875–888. doi: 10.1007/s10530-011-0124-6
Kylander, M. E., Ampel, L., Wohlfarth, B., and Veres, D. (2011). High-resolution X-ray fluorescence core scanning analysis of Les Echets (France) sedimentary sequence: new insights from chemical proxies. J. Quat. Sci. 26, 109–117. doi: 10.1002/jqs.1438
Lawler, A. (2018). Scarred bird bones reveal early settlement on Madagascar. Am. Assoc. Adv. Sci. 361:1059. doi: 10.1126/science.361.6407.1059
Leira, M., Filippi, M. L., and Cantonati, M. (2015). Diatom community response to extreme water-level fluctuations in two Alpine lakes: a core case study. J. Paleolimnol. 53, 289–307. doi: 10.1007/s10933-015-9825-7
Li, H., Sinha, A., André, A. A., Spötl, C., Vonhof, H. B., Meunier, A., et al. (2020). A multimillennial climatic context for the megafaunal extinctions in Madagascar and Mascarene Islands. Sci. Adv. 6:eabb2459. doi: 10.1126/sciadv.abb2459
Matsumoto, K., and Burney, D. A. (1994). Late Holocene environments at Lake Mitsinjo, northwestern Madagascar. Holocene 4, 16–24.
Mitchell, P. (2020). Settling Madagascar: when did people first colonize the world’s largest island? J. I. Coast. Archaeol. 15, 576–595. doi: 10.1080/15564894.2019.1582567
Mooney, S., and Radford, K. (2001). A simple and fast method for the quantification of macroscopic charcoal from sediments. Quat. Australas. 19, 43–46.
Moro, R. S., and Fürstenberger, C. B. (1997). Catálogo dos Principais Parâmetros Ecológicos de Diatomáceas Não-Marinhas. Parana: Editora UEPG.
Newton, A., Thunell, R., and Stott, L. (2006). Climate and hydrographic variability in the Indo-Pacific warm pool during the last millennium. Geophys. Res. Lett. 33, 1–5.
Passy, S. I. (2007). Diatom ecological guilds display distinct and predictable behavior along nutrient and disturbance gradients in running waters. Aquat. Bot. 86, 171–178. doi: 10.1016/j.aquabot.2006.09.018
Pedrono, M., Griffiths, O. L., Clausen, A., Smith, L. L., Griffiths, C. J., Wilmé, L., et al. (2013). Using a surviving lineage of Madagascar’s vanished megafauna for ecological restoration. Biol. Conserv. 159, 501–506. doi: 10.1016/j.biocon.2012.11.027
Perez, V. R., Godfrey, L. R., Nowak-Kemp, M., Burney, D. A., Ratsimbazafy, J., and Vasey, N. (2005). Evidence of early butchery of giant lemurs in Madagascar. J. Hum. Evol. 49, 722–742. doi: 10.1016/j.jhevol.2005.08.004
Pires, M. M., Guimarães, P. R., Galetti, M., and Jordano, P. (2018). Pleistocene megafaunal extinctions and the functional loss of long-distance seed-dispersal services. Ecography 41, 153–163. doi: 10.1111/ecog.03163
Piva, A., Asioli, A., Schneider, R. R., Trincardi, F., Andersen, N., Colmenero-Hidalgo, E., et al. (2008). Climatic cycles as expressed in sediments of the PROMESS1 borehole PRAD1-2, central Adriatic, for the last 370 ka: 1. Integrated stratigraphy. Geochem. Geophys. Geosyst. 9:Q01R01. doi: 10.1029/2007GC001713
R Core Team (2013). R: A Language and Environment for Statistical Computing. Vienna: R Foundation for Statistical Computing.
Razanatsoa, E. (2019). Impact of Human Land use and Rainfall Variability in Tropical Dry Forests of Southwest Madagascar During the Late Holocene. Cape Town: University of Cape Town.
Razanatsoa, E., Gillson, L., Virah-Sawmy, M., and Woodborne, S. (2021b). Synergy between climate and human land use maintained open vegetation in southwest Madagascar over the last millennium. Holocene 09596836211041731. doi: 10.1177/09596836211041731
Razanatsoa, E., Virah-Sawmy, M., Woodborne, S., Callanan, C., and Gillson, L. (2021a). Adaptation of subsistence strategies of the southwestern Malagasy in the face of climate change. Malagasy Nat. 15, 1–30. doi: 10.1007/978-3-030-42091-8_204-1
Scales, I. R. (2011). Farming at the forest frontier: land use and landscape change in western Madagascar, 1896-2005. Environ. Hist. 17, 499–524. doi: 10.3197/096734011x13150366551481
Schüler, L., and Hemp, A. (2016). Atlas of pollen and spores and their parent taxa of Mt Kilimanjaro and tropical East Africa. Quat. Int. 425, 301–386. doi: 10.1016/j.quaint.2016.07.038
Scott, D. M., Brown, D., Mahood, S., Denton, B., Silburn, A., and Rakotondraparany, F. (2006). The impacts of forest clearance on lizard, small mammal and bird communities in the arid spiny forest, southern Madagascar. Biol. Conserv. 127, 72–87. doi: 10.1016/j.biocon.2005.07.014
Scroxton, N., Burns, S. J., McGee, D., Hardt, B., Godfrey, L. R., Ranivoharimanana, L., et al. (2017). Hemispherically in-phase precipitation variability over the last 1700 years in a Madagascar speleothem record. Quat. Sci. Rev. 164, 25–36. doi: 10.1016/j.quascirev.2017.03.017
Seddon, N., Tobias, J., Yount, J. W., Ramanampamonjy, J. R., Butchart, S., and Randrianizahana, H. (2000). Conservation issues and priorities in the Mikea Forest of south-west Madagascar. Oryx 34, 287–304. doi: 10.1046/j.1365-3008.2000.00134.x
Spencer, T., Laughton, A. S., Flemming, N. C., Ingram, J. C., and Dawson, T. P. (2005). Climate change impacts and vegetation response on the island of Madagascar. Philos. Trans. R. Soc. Math. Phys. Eng. Sci. 363, 55–59. doi: 10.1098/rsta.2004.1476
Tierney, J. E., Smerdon, J. E., Anchukaitis, K. J., and Seager, R. (2013). Multidecadal variability in East African hydroclimate controlled by the Indian Ocean. Nature 493, 389–392. doi: 10.1038/nature11785
Tucker, B. (2007). Applying behavioral ecology and behavioral economics to conservation and development planning: an example from the Mikea forest, Madagascar. Hum. Nat. 18, 190–208. doi: 10.1007/s12110-007-9017-x
Tucker, B. (2020). Où vivre sans boire revisited: water and political-economic change among Mikea hunter-gatherers of southwestern Madagascar. Econ. Anthropol. 7, 22–37. doi: 10.1002/sea2.12160
Tucker, B., Lill, E., Tombo, J., Lahiniriko, R., Rasoanomenjanahary, L., Razafindravelo, P. M., et al. (2015). Inequalities beyond the Gini: subsistence, social structure, gender, and markets in southwestern Madagascar. Econ. Anthropol. 2, 326–342. doi: 10.1002/sea2.12034
Tucker, B., Tsimitamby, M., Humber, F., Benbow, S., and Iida, T. (2010). Foraging for development: a comparison of food insecurity, production, and risk among farmers, forest foragers, and marine foragers in southwestern Madagascar. Hum. Organ. 69, 375–386. doi: 10.17730/humo.69.4.m1n76k5272632873
Van Dam, H., Mertens, A., and Sinkeldam, J. (1994). A coded checklist and ecological indicator values of freshwater diatoms from the Netherlands. Netherland J. Aquat. Ecol. 28, 117–133. doi: 10.1007/bf02334251
Velez, M. I., Salgado, J., Brenner, M., Hooghiemstra, H., Escobar, J., Boom, A., et al. (2021). Novel responses of diatoms in neotropical mountain lakes to indigenous and post-European occupation. Anthropocene 34:100294. doi: 10.1016/j.ancene.2021.100294
Vincens, A., Lézine, A.-M., Buchet, G., Lewden, D., and Le Thomas, A. (2007). African pollen database inventory of tree and shrub pollen types. Rev. Palaeobot. Palynol. 145, 135–141. doi: 10.1016/j.revpalbo.2006.09.004
Virah-Sawmy, M. (2009). Ecosystem management in Madagascar during global change. Conserv. Lett. 2, 163–170. doi: 10.1111/j.1755-263X.2009.00066.x
Virah-Sawmy, M., Gillson, L., Gardner, C. J., Anderson, A., Clark, G., and Haberle, S. (2016). A landscape vulnerability framework for identifying integrated conservation and adaptation pathways to climate change: the case of Madagascar’s spiny forest. Landsc. Ecol. 31, 637–654. doi: 10.1007/s10980-015-0269-2
Virah-Sawmy, M., Willis, K. J., and Gillson, L. (2009). Threshold response of Madagascar’s littoral forest to sea-level rise. Glob. Ecol. Biogeogr. 18, 98–110. doi: 10.1111/j.1466-8238.2008.00429.x
Virah-Sawmy, M., Willis, K. J., and Gillson, L. (2010). Evidence for drought and forest declines during the recent megafaunal extinctions in Madagascar. J. Biogeogr. 37, 506–519. doi: 10.1111/j.1365-2699.2009.02203.x
Walsh, M. K., Prufer, K. M., Culleton, B. J., and Kennett, D. J. (2014). A late Holocene paleoenvironmental reconstruction from Agua Caliente, southern Belize, linked to regional climate variability and cultural change at the Maya polity of Uxbenká. Quat. Res. 82, 38–50. doi: 10.1016/j.yqres.2014.01.013
Wang, L., Brook, G. A., Burney, D. A., Voarintsoa, N. R. G., Liang, F., Cheng, H., et al. (2019). The African Humid Period, rapid climate change events, the timing of human colonization, and megafaunal extinctions in Madagascar during the Holocene: evidence from a 2m Anjohibe cave stalagmite. Quat. Sci. Rev. 210, 136–153. doi: 10.1016/j.quascirev.2019.02.004
Wengrat, S., Bennion, H., de Lima Ferreira, P. A., Figueira, R. C. L., and Bicudo, D. C. (2019). Assessing the degree of ecological change and baselines for reservoirs: challenges and implications for management. J. Paleolimnol. 62, 337–357. doi: 10.1007/s10933-019-00090-4
Whitlock, C., and Larsen, C. (2002). “Tracking environmental change using lake sediments,” in Charcoal as a Fire Proxy, eds J. P. Smol, H. J. B. Birks, and W. M. Last (Dordrecht: Springer), 75–97.
Wietzke, F.-B. (2015). Long-term consequences of colonial institutions and human capital investments: sub-national evidence from Madagascar. World Dev. 66, 293–307. doi: 10.1016/j.worlddev.2014.08.010
Wünnemann, B., Demske, D., Tarasov, P., Kotlia, B. S., Reinhardt, C., Bloemendal, J., et al. (2010). Hydrological evolution during the last 15 kyr in the Tso Kar lake basin (Ladakh, India), derived from geomorphological, sedimentological and palynological records. Quat. Sci. Rev. 29, 1138–1155. doi: 10.1016/j.quascirev.2010.02.017
Keywords: agro-pastoralists, environmental change, dry deciduous forest, aquatic ecosystems, herbivore introductions, socio-ecological systems
Citation: Domic AI, Hixon SW, Velez MI, Ivory SJ, Douglass KG, Brenner M, Curtis JH, Culleton BJ and Kennett DJ (2021) Influence of Late Holocene Climate Change and Human Land Use on Terrestrial and Aquatic Ecosystems in Southwest Madagascar. Front. Ecol. Evol. 9:688512. doi: 10.3389/fevo.2021.688512
Received: 31 March 2021; Accepted: 29 October 2021;
Published: 07 December 2021.
Edited by:
Atholl John Anderson, ANU College of Arts & Social Sciences, AustraliaReviewed by:
Richard James Telford, University of Bergen, NorwayCopyright © 2021 Domic, Hixon, Velez, Ivory, Douglass, Brenner, Curtis, Culleton and Kennett. This is an open-access article distributed under the terms of the Creative Commons Attribution License (CC BY). The use, distribution or reproduction in other forums is permitted, provided the original author(s) and the copyright owner(s) are credited and that the original publication in this journal is cited, in accordance with accepted academic practice. No use, distribution or reproduction is permitted which does not comply with these terms.
*Correspondence: Sean W. Hixon, aGl4b25AdWNzYi5lZHU=
†These authors have contributed equally to this work
Disclaimer: All claims expressed in this article are solely those of the authors and do not necessarily represent those of their affiliated organizations, or those of the publisher, the editors and the reviewers. Any product that may be evaluated in this article or claim that may be made by its manufacturer is not guaranteed or endorsed by the publisher.
Research integrity at Frontiers
Learn more about the work of our research integrity team to safeguard the quality of each article we publish.