- 1Beaverlodge Research Farm, Agriculture and Agri-Food Canada, Beaverlodge, AB, Canada
- 2Department of Entomology, Texas A&M University, College Station, TX, United States
- 3Department of Animal Science, Texas A&M University, College Station, TX, United States
Recent work demonstrated that honey bee (Apis mellifera L.) queens reared in pesticide-laden beeswax exhibit significant changes in the composition of the chemicals produced by their mandibular glands including those that comprise queen mandibular pheromone, which is a critical signal used in mating as well as queen tending behavior. For the present study, we hypothesized that pesticide exposure during development would alter other queen-produced chemicals, including brood pheromone in immature queens, thus resulting in differential feeding of queen larvae by nurse workers, ultimately impacting adult queen morphology. We tested these hypotheses by rearing queens in beeswax containing field-relevant concentrations of (1) a combination of tau-fluvalinate and coumaphos, (2) amitraz, or (3) a combination of chlorothalonil and chlorpyrifos. These pesticides are ubiquitous in most commercial beekeeping operations in North America. We observed nurse feeding rates of queen larvae grafted into pesticide-laden beeswax, analyzed the chemical composition of larval queen pheromones and measured morphological markers in adult queens. Neither the nurse feeding rates, nor the chemical profiles of immature queen pheromones, differed significantly between queens reared in pesticide-laden wax compared to queens reared in pesticide-free wax. Moreover, pesticide exposure during development did not cause virgin or mated adult queens to exhibit differences in morphological markers (i.e., body weight, head width, or thorax width). These results were unexpected given our previous research and indicate that future work is needed to fully understand how pesticide exposure during development affects honey bee queen physiology, as well as how various adult queen quality metrics relate to each other.
Introduction
Pesticide contamination is ubiquitous in honey bee colonies across the United States A comprehensive survey conducted a decade ago found that 98.4% of wax samples collected from commercial beekeeping operations in North America were contaminated with multiple pesticides (Mullin et al., 2010). Of the 259 samples analyzed in that survey, 83% contained both of the beekeeper-applied miticides tau-fluvalinate and coumaphos, which have been heavily used over the last three decades to control infestations by the ectoparasitic mite, Varroa destructor. Despite Varroa mites developing resistance to both products by the year 2000 (Elzen et al., 2000; Elzen and Westervelt, 2002; Rinkevich, 2020), these miticides have persisted and have accumulated in the beeswax matrix of commercial beekeeping operations over time (Wallner, 1999; Mullin et al., 2010; Traynor et al., 2016; Haber et al., 2019; Ostiguy et al., 2019). A more recently introduced miticide, amitraz, and its metabolites N-(2,4-dimethylphenyl)-N-methylformamidine (DMPF) and 2,4-dimethylaniline (DMA), were present in 60.5% of the wax samples analyzed by Mullin et al. (2010). In addition to these miticides, two agrochemicals, the fungicide chlorothalonil and the insecticide chlorpyrifos, were among the top five pesticides detected in beeswax samples (Mullin et al., 2010). They were found in 49.2 and 63.2% of all samples analyzed, respectively, and were likely introduced into the colonies by forager bees that visited crops that had been treated with those chemicals. More recent studies have confirmed that wax and other hive products are consistently being exposed to tau-fluvalinate, coumaphos and amitraz (and its metabolites), in addition to other agro-chemicals (Traynor et al., 2016, 2021a,b; Ostiguy et al., 2019; Milone et al., 2021).
Miticide exposure during development has been shown to cause sub-lethal effects on queen and drone reproductive quality. For example, tau-fluvalinate exposure causes increased queen mortality in the first 6 months after their introduction to new colonies (Pettis et al., 1991; Sokol, 1996). It also causes lower body weight (Haarmann et al., 2002) and increased supersedure rates (Sokol, 1996). Coumaphos exposure during development also causes lower body and ovary weight in queens (Haarmann et al., 2002), lower survival (Pettis et al., 2004) and has been reported to cause a 90% decrease in queen acceptance (Fell and Tignor, 2001). Most strikingly, coumaphos exposure at the label application rate of one strip per five frames of bees can cause high mortality in developing queen larvae 24 h after exposure (Haarmann et al., 2002), in addition to as much as a 75% decrease in adult survival during the first 6 months after exposure (Collins et al., 2004; Pettis et al., 2004).
Recently, Walsh et al. (2020b) showed that exposure to pesticide-containing wax during development affects several aspects of queen physiology and worker behavior. In particular, adult queens that were reared in beeswax contaminated with field-relevant concentrations of miticides showed significant differences in the chemical composition of their mandibular gland contents compared to queens reared in pesticide-free wax. These differences were discernable by queen tending workers (around 1 week of age), who were significantly more attracted to the glandular contents of queens reared in pesticide-free wax compared to those reared in pesticide-laden wax. Furthermore, pesticide exposure during development caused mated queens to have lower egg-laying rates and smaller queen retinues (Walsh et al., 2020b).
Most honey bee queens are reared by nurse workers throughout the reproductive season, which typically occurs in the spring when colonies are populous and healthy (Seeley, 1996). During this time, a colony can rear a dozen or more queens that develop asynchronously inside large, protected cells made of thick layers of wax (Winston, 1987). After finishing their 16-day developmental period, virgin queens announce their impending emergence by vocalizing the queen “piping” signal (Simpson, 1963), whereby the first emerging queen typically walks around the nest and kills other queens, especially those that are more mature (Gilley, 2001; Harano and Obara, 2004; Harano et al., 2008). Sororicide of immature queens is accomplished when an adult queen chews a small hole at the top of a developing queen’s cell (where the queen’s abdomen is located) and stings the rival to death through the hole. If a rival queen emerges before the first emerged queen can kill her, the queens duel each other to death until there is usually only one queen left standing (Gilley, 2001). In a study of 27 queens in artificial fighting arenas, the heaviest queen won duels against other queens approximately 75% of the time (Tarpy and Mayer, 2009), indicating that body size may be associated with a queen’s competitive ability. External morphological features, including body weight, head width and thorax width are therefore often considered markers of queen reproductive potential (Eckert, 1937; Haarmann et al., 2002; Delaney et al., 2011; Rangel et al., 2013; De Souza et al., 2019).
Ovary weight and number of ovarioles per ovary are also considered measures of queen reproductive quality. The number of ovarioles per ovary typically ranges between 100 and 180 (Delaney et al., 2011; Jackson et al., 2011). Each ovariole is made of a germarium, a mass of undifferentiated cells that eventually forms oocytes, nurse cells and follicular cells. Nurse cells nourish the oocytes during early growth, while follicular cells function in enlarging oocytes with an epithelium and chorion as the oocytes ripen into eggs. Once the queen has mated, follicular cells become vitellogenic and grow in size (Tanaka and Hartfelder, 2004; Hartfelder et al., 2018). Eckert (1937) found a correlation between ovary weight and the number of capped brood cells produced by a queen. However, ovary weight and ovariole number are not always predictive indicators of a queen’s egg-laying capacity. For example, environmental variables can cause workers to remove eggs from the brood area when the colony is stressed (Ratnieks, 1993), which would underestimate the number of eggs laid by the queen over time. In contrast, workers can also activate their ovaries and lay unfertilized eggs while mimicking queen-laid egg signals (Katzav-Gozansky et al., 2003), which would overestimate the queen’s egg-laying rate. For example, a study by Fine (2020) found that exposure to insect growth regulator pesticides in queen monitoring cages decreased egg survival without impacting queen egg-laying rates. Additionally, exposure of pollen to combinations of pesticides at field-relevant concentrations caused significant brood loss in exposed colonies 1 month post-exposure (Traynor et al., 2021b). Moreover, ovary weight and ovariole number do not always correlate with other measures of queen body size including thorax width, head width and body weight (Eckert, 1934; Jackson et al., 2011). More recently, when queens were stressed by being exposed to neonicotinoid insecticides during development, they had more ovarioles than control queens but lower rates of oviposition and survival 4 weeks after emergence (Williams et al., 2015).
The rate at which nurse bees feed queen larvae, as well as the amount and quality of food that the larvae are fed, drastically impacts adult queen morphology. A recent study showed that rearing queen larvae on supplemental diets with added nutrients and higher levels of juvenile hormone increased adult queen weight, head size and thorax width, but not ovary weight (De Souza et al., 2019). Brouwers et al. (1986) reported that nurse bees typically spend less than 50 s each time they visit a developing worker larva during its first two instars and the visits are gradually lengthened (> 50 s) during subsequent instars. More recent work has utilized video analyses to quantify nursing “events,” or visits to larval cells more precisely. They discovered that on the third day of larval development, nurses visited individual cells 2,461 ± 154 times per day, with the general trend of more nursing visits during the first larval developmental day and fewer visits on the last day of larval development (Siefert et al., 2021). Furthermore, under nutritional stress, nurses preferentially feed starved larvae over well-fed larvae (Sagili et al., 2018), suggesting that nurses could be sensitive to changes caused by environmental and changing nutritional cues emitted by developing queens (e.g., larval pheromones). Honey bee brood pheromone comprises a suite of compounds including methyl palmitate, methyl oleate, methyl stearate, methyl linoleate, methyl linolenate, ethyl palmitate, ethyl oleate, ethyl stearate, ethyl linoleate, ethyl linolenate and E-β-ocimene. The composition of this pheromone changes drastically throughout larval development and signals larval presence, age and nutritional status to nurse bees (Le Conte et al., 1990; Trouiller, 1993; Maisonnasse et al., 2010). While all of these brood-produced compounds consistently elicit nursing responses, there is still much to learn about brood pheromones, as demonstrated by the recent identification of a new compound, allo-ocimene, which may be an additional brood pheromone component (Wu et al., 2019). It is clear that brood pheromones play key roles in determining the resources foraged for by a colony (Traynor et al., 2015; Ma et al., 2016) and in modulating nurse bee physiology and behavior (Traynor et al., 2014, 2017; Ma et al., 2018, 2019). Although the signals that elicit feeding of queen larvae done by nurse workers has not been thoroughly examined, the duration of nurse visits to feed worker larvae and the signals that mediate the amount and type of feeding are probably similar to those used by nurses to feed queen larvae.
In this study, we examined whether exposure to wax contaminated with field-relevant concentrations of tau-fluvalinate, coumaphos, amitraz, chlorpyrifos and chlorothalonil during development affected the rate at which queen larvae are fed by nurse bees, their brood pheromone chemical composition and the morphology of emerged virgin and mature queens exposed to these chemicals during development. We hypothesized that pesticide exposure would negatively affect the variables measured (e.g., change feeding rates by nurses, change queen larval pheromones, and decrease queen morphometrics), thus affecting overall queen fitness.
Materials and Methods
Bee Source and Queen Rearing
The colonies used in this study were kept at the Janice and John G. Thomas Honey Bee Facility of Texas A&M University in Bryan, TX (N 30°38′31.037″ W 96°27′39.495″) and the Windy Hill Apiary in Watertown, WI (N 43°7′9.79″ W 88°44′30.562). All colonies were headed by queens of Italian stock procured from Olivarez Honey Queens Inc. (Orland, CA, United States). Mated queens for the morphology experiments were reared in 2016 and 2019, while queens for the feeding, larval pheromones, and virgin morphology experiments were reared in 2019. Experimental queens were reared by grafting, a standard queen-rearing procedure (Laidlaw and Eckert, 1964) whereby first-instar worker larvae were transferred into plastic cups (JZBZ Honey Co., Santa Cruz, CA, United States). Each cup had been coated with ≈200 mg of molten beeswax (certified, pesticide-free wax pellets, Koster Keunen Inc., Watertown, CT, United States) that was either kept untreated, or mixed separately with (a) a combination of 204 ppm of tau-fluvalinate and 91.9 ppm of coumaphos (> 98% purity, Thermo Fisher), (b) 43 ppm of amitraz (> 98% purity, Sigma-Aldrich), or (c) a combination of 9.8 ppm of chlorpyrifos and 53.7 ppm of chlorothalonil (> 98% purity, Thermo Fisher) while the wax was molten. While we are confident that our initial wax was pesticide-free, we did not confirm our final wax pesticide concentrations with laboratory analyses. We chose these pesticides and their concentrations based on the reported values found in wax samples collected from commercial beekeeping operations in North America (Mullin et al., 2010). We also used adult queens from a concurrent study (Walsh et al., 2020b) for the mated queen morphological measurements.
All grafted larvae used for morphological assessments were placed into queenless nucleus colonies, or “cell builders” (Laidlaw and Eckert, 1964), so that nurse bees could care for queens during larval and pupal development. Approximately 2 days before the expected queen emergence, each capped cell was put into a queen-holding cage. We collected two subsets of queens for morphological comparisons: mated queens and virgin queens. To obtain mated queens (i.e., those grafted in 2016), once adult queens emerged in the holding cages we placed them individually into queenless five-frame mating nucleus colonies or mating “nucs” composed of approximately one thousand workers, two frames containing brood, one frame containing nectar and pollen, one empty frame and one frame feeder with sugar syrup for bees to feed ad libitum. The queens were then released from their cages, marked and allowed to mate naturally (Winston, 1987). Successful queen mating was verified by examining the mating nucs for the presence of both the queen and diploid offspring ten to 15 days after a queen was released into the nuc. Once the queens mated, they were caged again and used for two separate studies (Walsh et al., 2020a, b) before they were collected and frozen at −20°C for subsequent for morphological measurements. To obtain virgin queens (i.e., those grafted in 2019) for comparison against the mated queens, once adult queens emerged in the holding cages, they were anesthetized by freezing at −20°C for 3–5 min to conduct morphological analyses (see below).
Feeding Rates of Developing Queens
In the spring of 2019, we grafted first-instar larvae into wax-coated plastic cups to examine the feeding behavior of nurses toward developing queens in the different treatment groups. Three trials were conducted, each using larvae grafted on the same day. A total of 20 larvae, or five larvae per experimental group, were grafted per trial. Of the 60 grafts that were done overall, only 30 were initiated and survived until the end of the data collection period. For each trial, the grafts were put into a glass-walled observation hive that was kept closed for 3 days. Each observation hive was provisioned with one frame of emerging brood, one frame of honey and approximately 4,000 nurse bees. It also had two wooden grafting bars holding 10 cups each, for a total of 20 cups (N = 5 per treatment group) containing the grafted larvae, which were positioned on the bars at random to eliminate any location effects.
To measure the rate at which nurse bees fed royal jelly to queen larvae, we counted the number of bees that stuck their heads and thoraces in the queen cups for 3 s or more, following methods previously published (Metz et al., 2010). These counts were repeated for five consecutive minutes until each surviving larva in the observation colony had been observed for ten separate 5-min periods on the morning of the third day post-graft. The observation colony was fed 1:1 weight by volume sugar syrup ad libitum and kept in a dark room during this assay. All nurse bee feeding assessments were conducted under a red light to avoid disturbance.
Chemical Analysis of Queen Larval Pheromones
We reared additional larvae in the summer of 2019 to analyze the chemical composition of pheromones produced by developing queens. To do this, we used forceps to gently remove larvae or pupae from their queen cups, starting on the third larval instar (2 days after grafting) and collected them in individual microcentrifuge tubes daily (N = 114 individuals: control = 32; fluvalinate and coumaphos = 25; amitraz = 28; and chlorothalonil and chlorpyrifos = 29). The samples were kept at −80°C in glass vials until use. To conduct the chemical analysis of brood-produced compounds, we added 0.5–0.75 mL of hexane to each glass vial for 9 min. We then transferred each sample extract to a 2 mL glass autosampler vial containing a 300 μL glass conical insert. Hexane extracts were dried completely under a nitrogen stream and resuspended with 15 μL of hexane mixed with 1 μL of octadecanoic acid as an internal standard. To ensure resuspension, samples were heated to 50°C, vortexed for 20 s and allowed to sit for 3 min.
We used a 7890B gas chromatograph (GC) system (AgilentTM) equipped with a 30 m × 250 μm × 0.25 μm film thickness column (AgilentTM) coupled to a 5977B mass selective detector (MSD, AgilentTM) for separation, identification and quantification of the compounds of interest. Helium was the carrier gas and 2 μL of sample was injected using a 7693 autosampler (AgilentTM), while the inlet was kept at 300°C and operated in splitless mode. The column temperature was kept at 40°C for 1 min, heated to 200°C at 15°C/min, then to 315°C at 6°C/min, until it reached 315°C and maintained at the final temperature for 2 min. The mass selective detector (MSD) was operated using electron impact ionization and a scan range from 40 to 350 m/z.
When performing the gas chromatography-mass spectrometry analysis, we utilized analytical grade standards to confirm the identity of selected peaks. These included methyl palmitate, methyl oleate, methyl stearate, methyl linoleate, methyl linolenate, ethyl palmitate, ethyl oleate, ethyl stearate, ethyl linoleate, ethyl linolenate and E-β-ocimene (all sourced from Sigma-Aldrich, Inc.), as done previously (Le Conte et al., 1990; Maisonnasse et al., 2010). E-β-ocimene was a blend of both isomers, which we differentiated after analysis. We compared the retention indices and mass spectra from our samples to the standards and published values (NIST 17) and integrated the identified peaks for quantification, which were manually integrated when needed. All identified peak values were normalized to the peak area value of the internal standard, octadecane. We determined the relative proportions of the compounds in each sample by calculating the percent normalized peak area in reference to the total normalized peak area.
Mated and Virgin Queen Morphology
Cohorts of grafted queens from each experimental group were used for external morphological measurements once they reached adulthood. We used a total of 49 mated queens that had been previously reared and frozen in 2016 (control = 18; fluvalinate and coumaphos = 10; amitraz = 11; and chlorothalonil and chlorpyrifos = 10) and 30 virgin queens reared in the spring of 2019 (control = 10; fluvalinate and coumaphos = 8; amitraz = 6; and chlorothalonil and chlorpyrifos = 8). We measured each queen’s thorax and head width twice using digital calipers (FisherbrandTM, Waltham, MA, United States) and used the average of the two values. We also recorded her body weight.
Adult Queen Ovary Size
A total of 41 additional queens (control = 16; fluvalinate and coumaphos = 9; amitraz = 7; chlorothalonil and chlorpyrifos = 9) were reared in the spring of 2019 for the ovary size assessment. After adult queens matured and mated successfully (as confirmed by the presence of worker brood inside the mating nucs) we collected each queen, anesthetized her by freezing at −20°C for 3–5 min and dissected her ovaries. The ovaries were fixed in 10% neutral buffered formalin solution, dehydrated in ethanol solution and then taken to the Texas A&M University Histology Laboratory (College Station, TX) where they were individually embedded in paraffin. Each ovary was cross-sectioned into 5-μm thick slices using a microtome. Each slice was mounted onto a slide and then stained with hematoxylin and eosin (H&E) stain. The slides were assigned numbers at random as a mechanism to avoid observer biases while performing the ovariole counts and each slide was counted twice by two different people. The person counting the number of ovarioles in each slide did so blindly and did not know the treatment group to which each queen belonged. We used a microscope with a mounted MotoX camera (Motic, Xiamen, China) to take high quality images of the ovary cross-sections, which were uploaded to the counting software ImageJ® (NIH, Madison, WI). This provided two technical replicate counts per ovary and the average value was used for analysis.
Statistical Analyses
We conducted the analyses after verifying that all statistical assumptions were met, including testing for normality using Shapiro-Wilk tests and equal variances with Brown-Forsythe tests. We performed a repeated measures ANOVA test to determine whether exposure to pesticides in wax during queen development affected nurse feeding rates toward queen larvae. We also conducted ANOVA tests to determine if pesticide exposure during development affected the external morphological measurements (i.e., body weight, head width and thorax width) of virgin queens. Furthermore, we compared queen ovariole count and mated queen external morphological measurements by performing full factorial ANOVA tests across treatment groups, using the location and year in which queens were reared as non-random variables. We present all descriptive statistics as the mean ± S.E.M. and set the level of significance for all tests at α = 0.05. All ANOVA tests were conducted with the statistical software JMP v.13 (SAS Institute Inc., NC). Brood pheromone data were analyzed with a permutational multivariate analysis of variance using Bray-Curtis distance matrices in R Studio and graphically displayed using Non-metric Multidimensional Scaling (NMDS) (Oksanen et al., 2019), which can be publicly found using the “vegan” citation code in R Studio.
Results
Immature Queen Feeding Rate and Brood Pheromones
We counted an average of 5.34 ± 0.05 nurse bee visits to queen larvae per 5-min interval for queens reared in pesticide-free wax (Figure 1). Queen larvae reared in wax containing tau-fluvalinate and coumaphos had an average of 5.05 ± 0.01 nurse visits per 5-min interval, larvae reared in amitraz-laden wax had 4.14 ± 0.71 nurse visits per 5-min interval and those reared in wax containing chlorothalonil and chlorpyrifos had 4.71 ± 0.80 nurse visits per 5-min interval. There was no statistical difference in the rate at which nurse bees visited queen larvae between the control and pesticide treatment groups [F(3,29) = 1.64, p = 0.20].
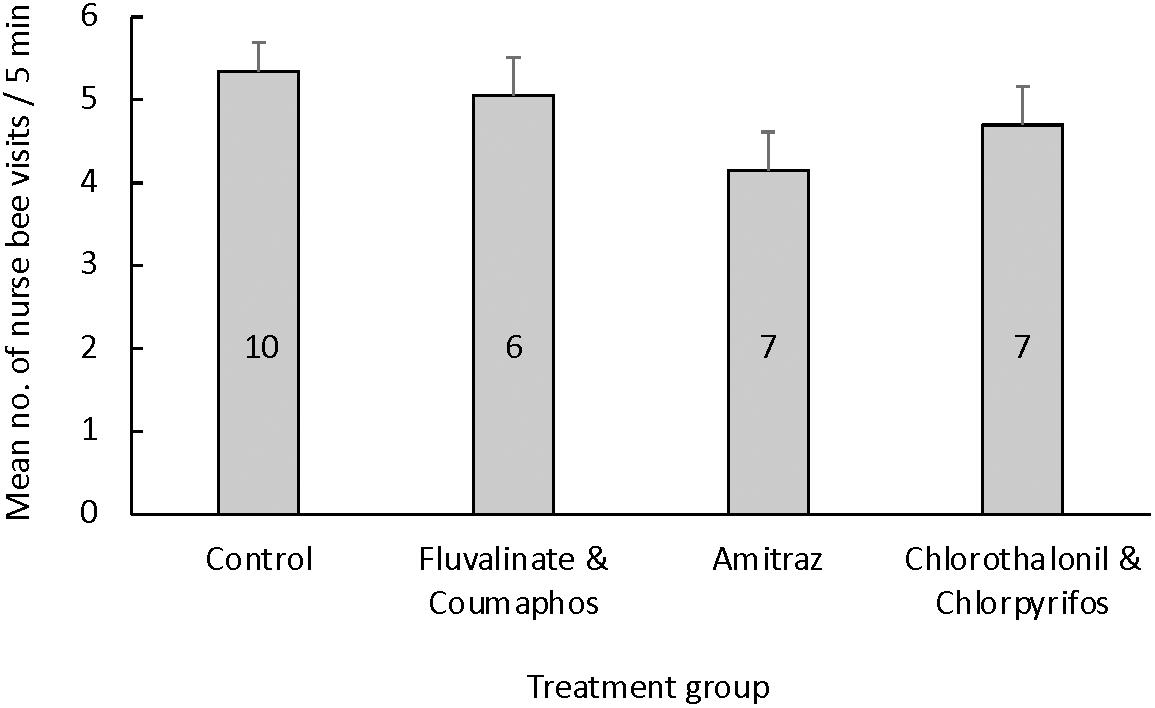
Figure 1. Mean (±S.E.M.) number of nurse feeding events per queen larva during 5-min intervals based on the queen’s rearing environment. Queen larvae were reared in cups coated with molten wax that was either pesticide-free (control group) or contaminated with field-relevant concentrations of either (a) a combination of tau-fluvalinate and coumaphos, (b) amitraz alone, or (c) a combination of chlorothalonil and chlorpyrifos. The number of queens used per treatment group is given inside each bar. There were no differences in the nurse feeding rate toward queen larvae based on the treatment group [ANOVA: F(3, 29) = 1.41, p = 0.26].
Regardless of the queens’ developmental stage, we found no difference in brood pheromone composition for larvae alone [PERMANOVA F(3,54) = 0.99, R2 = 0.05, p = 0.44; Supplementary Figure 2] or pupae alone [PERMANOVA F(3,55) = 0.81, R2 = 0.04, p = 0.64; Supplementary Figure 3]. Furthermore, brood pheromone compositions of queens reared in pesticide-free wax did not differ from those of queens reared in pesticide-laden wax when larval and pupal samples were combined for analysis [PERMANOVA F(3, 110) = 0.86, R2 = 0.02, p = 0.58; Supplementary Figure 1].
Adult Queen Morphometric Measurements
Mated queens reared in cups coated with pesticide-free wax had statistically similar body weight regardless of rearing location (TX vs. WI, p = 0.82) or year (2016 vs. 2019, p = 0.84). Average body weight for mated queens reared in pesticide-free wax was 237.14 ± 3.61 mg, which was not significantly different from that of any of the pesticide treatment groups [F(3,48) = 1.28, p = 0.27]. The average mated queen body weight for queens reared in cups contaminated with either tau-fluvalinate and coumaphos, amitraz, or chlorothalonil and chlorpyrifos was 237.52 ± 2.68 mg, 227.93 ± 3.60 mg and 226.84 ± 2.95 mg, respectively (Figure 2A). Mated queens reared in pesticide-free wax also had statistically similar head width, regardless of the rearing location (TX vs. WI, p = 0.72) or year (2016 vs. 2019, p = 0.78). There was no difference in head width for queens based on treatment [F(3,48) = 0.99, p = 0.50; Figure 2B]. Similarly, we found no difference in the thorax width of mated queens based on the rearing location (TX vs. WI, p = 0.64) or the year (2016 vs. 2019, p = 0.84) or treatment [F(3,48) = 1.23, p = 0.30; Figure 2C].
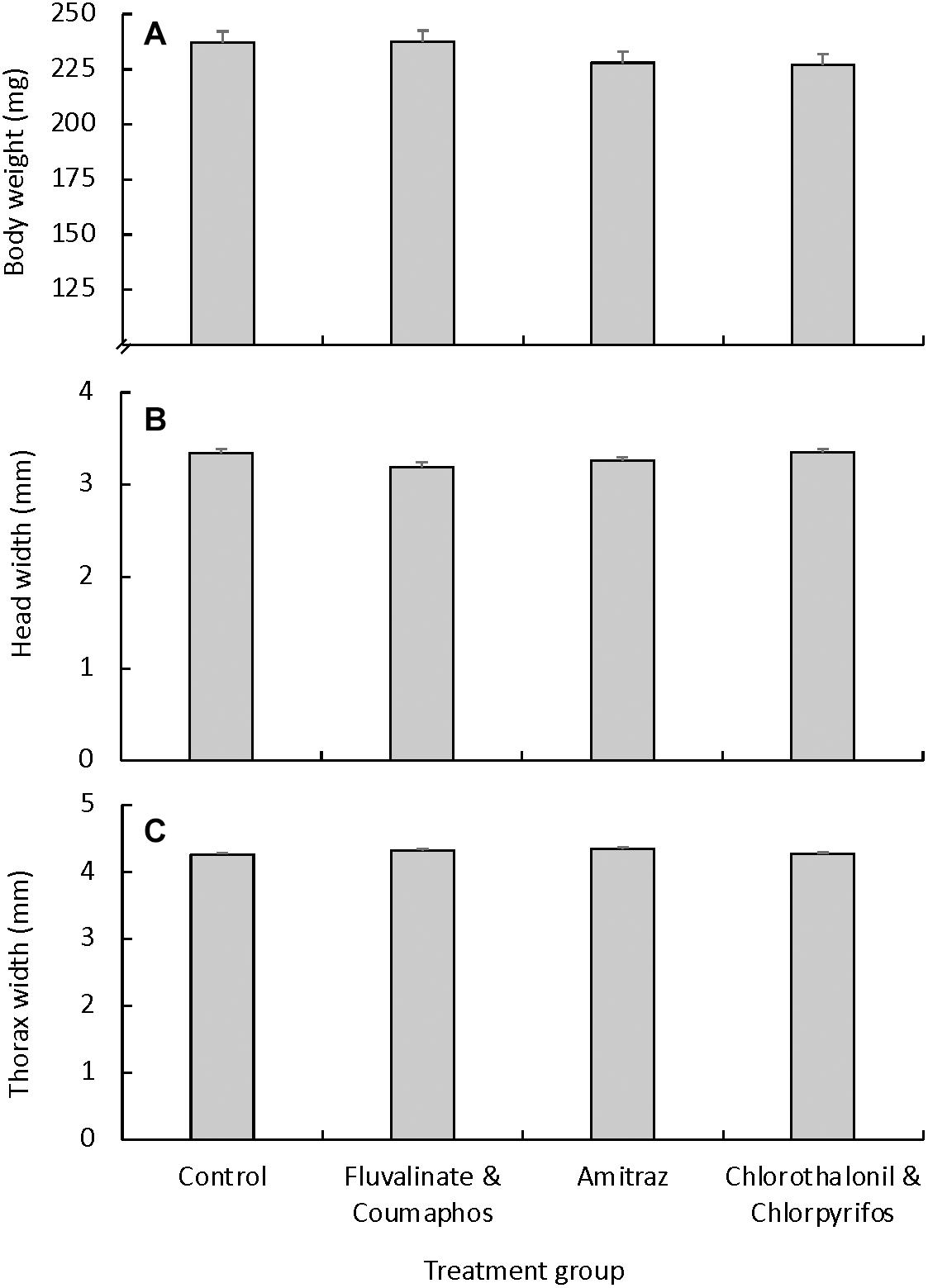
Figure 2. External morphology measurements (mean ± S.E.M.) for mated queens that were reared under different pesticide exposure conditions. Queens were grafted into plastic cups that were coated with pesticide-free molten wax (control group; n = 18), or wax containing either a combination of tau-fluvalinate and coumaphos (n = 10), amitraz alone (n = 11), or a combination of chlorothalonil and chlorpyrifos (n = 10). We found no differences across treatment groups for either (A) body weight [F(3, 48) = 0.99, p = 0.49], (B) head width [F(3, 48) = 1.28, p = 0.27] or (C) thorax width [F(3, 48) = 1.23, p = 0.30].
Similarly, we found no difference in virgin queen weight based on exposure to pesticides in wax during queen development [F(3, 29) = 0.88, p = 0.46]. On average, virgin queens reared in pesticide-free beeswax weighed 155.43 ± 5.40 mg. For the pesticide treatment groups, queens reared in wax containing tau-fluvalinate and coumaphos weighed an average of 153.87 ± 3.89 mg, those reared in amitraz-laden wax weighed 149.67 ± 4.07 mg and those reared in wax containing chlorothalonil and chlorpyrifos weighted 144.83 ± 7.94 mg (Figure 3A). We observed a similar trend for the other size measurements, as there were no differences in head or thorax width across treatment groups (Figures 3B,C).
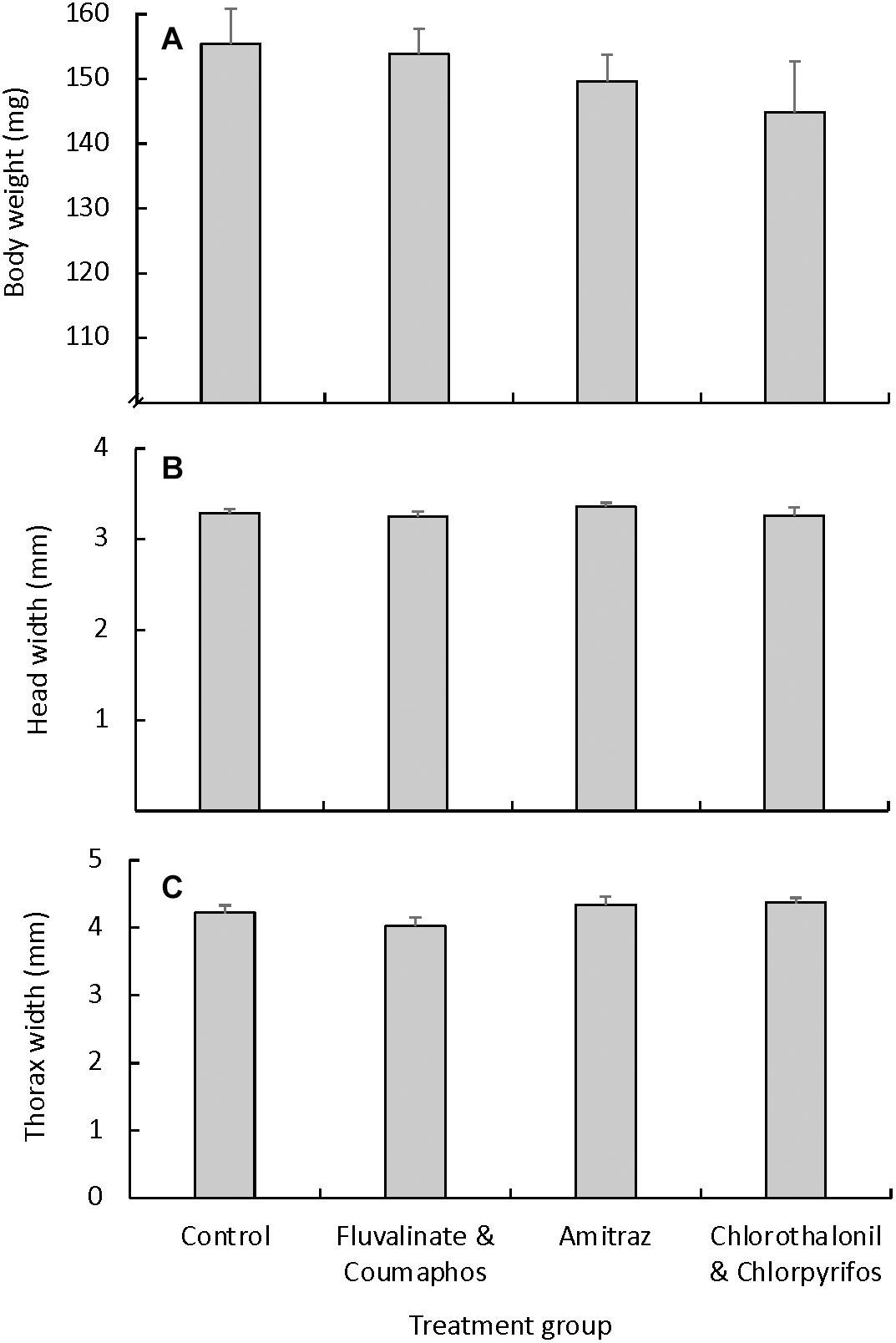
Figure 3. External morphology measurements (mean ± S.E.M.) for virgin queens that were reared under different pesticide exposure conditions. Queens were grafted into plastic cups that were coated with pesticide-free molten wax (control group; n = 10), or wax containing either a combination of tau-fluvalinate and coumaphos (n = 8), amitraz alone (n = 6), or a combination of chlorothalonil and chlorpyrifos (n = 6). We found no differences across treatment groups for either (A) body weight [F(3, 29) = 0.88, p = 0.46], (B) head width [F(3, 29) = 0.73, p = 0.54], or (C) thorax width [F(3, 29) = 2.17, p = 0.12].
Ovariole Count in Mated Queens
After confirming a lack of difference in the number of ovarioles between a queen’s right and left ovary using a subsample of seven queens (p = 0.36), we opted to use only a cross-section of the right ovary from each queen for analysis. Queens reared in pesticide-free wax had an average of 166.06 ± 18.75 ovarioles per ovary (Figure 4). Queens reared in wax containing either tau-fluvalinate and coumaphos, amitraz alone, or chlorpyrifos and chlorothalonil had an average of 211.50 ± 25.00, 161.86 ± 28.34 and 168.61 ± 25.00 ovarioles per ovary, respectively. There was no difference in the number of ovarioles per ovary based on treatment [F(3,40) = 0.88, p = 0.46].
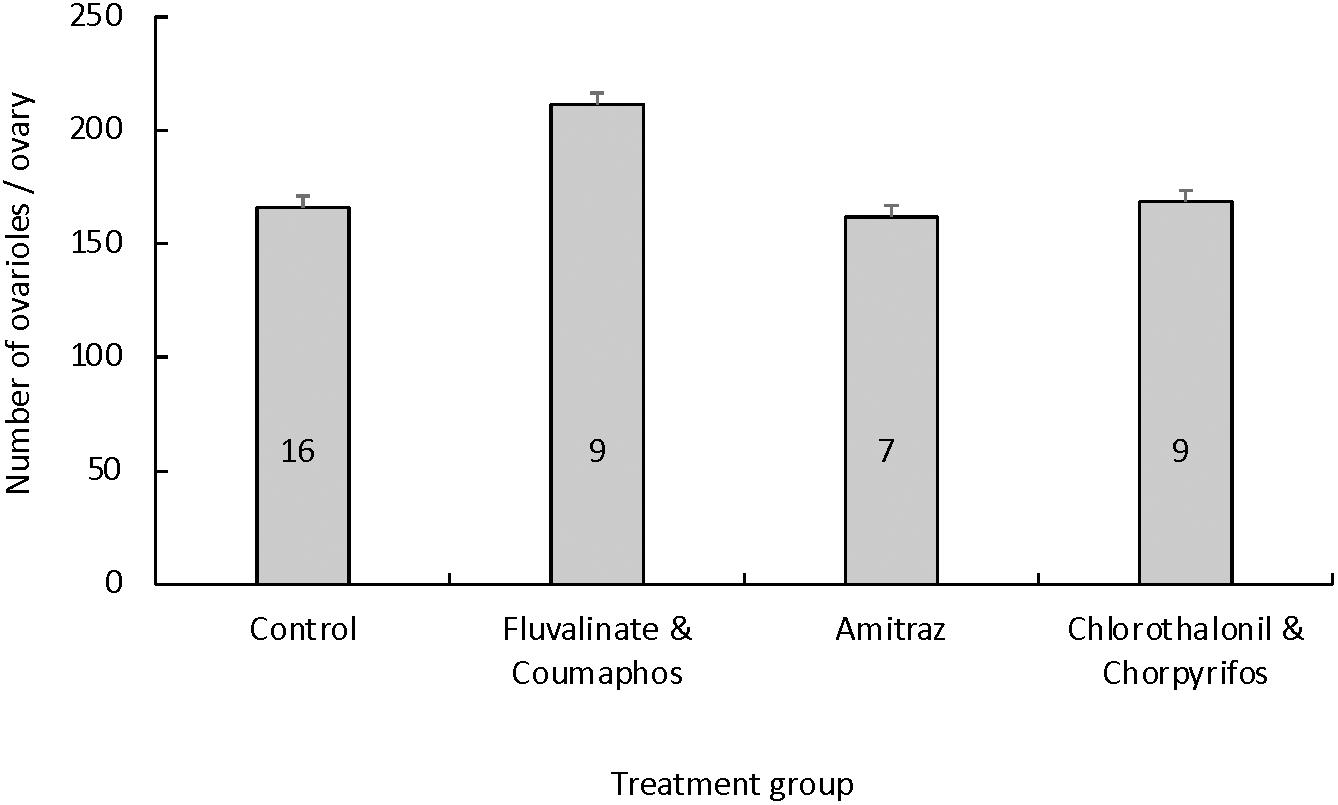
Figure 4. Mean (± S.E.M.) number of ovarioles per queen ovary based on the queen’s rearing environment. Queen larvae were reared in cups coated with wax that was pesticide-free (control group) or contaminated with either (a) a combination of tau-fluvalinate and coumaphos, (b) amitraz alone or (c) a combination of chlorothalonil and chlorpyrifos. The number of queens used per treatment group is given inside each bar. There was no difference in the number of ovarioles per queen ovary based on the treatment group [ANOVA: F(3, 40) = 0.88, p = 0.46].
Discussion
We examined the effects of honey bee queen developmental exposure to beeswax containing field-relevant concentrations of tau-fluvalinate, coumaphos, amitraz, chlorpyrifos and chlorothalonil on queen development and adult morphology. We chose those pesticides and combinations due to their widespread prevalence in commercial beekeeping operations across the United States (Mullin et al., 2010; Traynor et al., 2016; Ostiguy et al., 2019). We found that contrary to our predictions, contamination of the queen-rearing wax did not result in a significant difference in brood pheromone chemical composition, which likely explains why there was no difference in nurse feeding rates of queen larvae, and ultimately, the external morphology of virgin and mated queens. These results may be in part due to the relatively low sample sizes in our experimental groups. Grafting success was low throughout the experiments, with approximately 10% grafting success (whereby “success” is measured as adult queen emergence) throughout the experiments, although queens grafted into uncoated cups at the same locations, times, and by the same grafter had between 80% to 95% grafting success. It is possible that larger sample sizes would yield different results, but further studies should be done using either a different rearing method or different queen cups.
Despite these surprising results, previous research has found that honey bees exposed to these pesticides during development suffer developmental handicaps attributed to pesticide exposure. For example, queens that develop in wax containing tau-fluvalinate or coumaphos experience increased mortality, as well as reduced body and ovary weight (Haarmann et al., 2002; Pettis et al., 2004; Traynor et al., 2016). Furthermore, developing drones exposed to wax containing tau-fluvalinate and coumaphos at the same concentrations we used showed a significant decrease in adult drone sperm viability (Fisher and Rangel, 2018) and lower overall reproductive competitiveness (Rinderer et al., 1999; Burley, 2007; Shoukry et al., 2013). Moreover, sublethal exposure of developing worker bees to pesticides during development, including tau-fluvalinate, coumaphos, amitraz, chlorothalonil and chlorpyrifos causes developmental delays and shorter lifespan by an average of 4 days (Wu et al., 2011). Additionally, tau-fluvalinate, coumaphos, chlorothalonil, and chlorpyrifos all have high oral toxicities to honey bee larvae (Zhu et al., 2014). For instance, Dai et al. (2017) examined in vitro oral toxicity to honey bee larvae caused by exposure to the same pesticides we used and found that, from the most toxic to the least toxic pesticide, the order of toxicity was chlorpyrifos > tau-fluvalinate > coumaphos > amitraz. While these pesticides are all toxic to individual honey bees in different castes and life stages, another study showed that initial contamination of wax foundation with these pesticides neither slowed colony growth, nor individually increased overwintering mortality in newly established colonies (Payne et al., 2019).
The external morphological features of queens do not seem to be a reliable predictor of queen reproductive quality, despite the fact that body weight is a predictor of queen-queen duel winners in artificial fighting arenas (Tarpy and Mayer, 2009). This is likely in part because, in regular colonies, worker assistants tend to restrain losing queens in order for the winning queen to kill them, although the mechanism that workers use to determine what queen they will support is yet unknown (Gilley, 2001; Tarpy et al., 2004). Interestingly, the age at which queen larvae are grafted is highly associated with queen size and weight, whereby larvae grafted earlier have significantly larger size and weight upon adult queen emergence compared to larvae grafted days later (Rangel et al., 2013; De Souza et al., 2019). Therefore, it is possible that workers choose which queen to help during queen duels based on their assessment of a specific queen’s overall vigor and fitness. These decisions may (at least in part) depend on the genetic background or pheromonal signature of the queen larvae, both of which have been demonstrated to impact nursing behavior (Tilley and Oldroyd, 1997; Sagili et al., 2018; Withrow and Tarpy, 2018). Walsh et al. (2020b) recently found significant differences in the chemical composition of queen mandibular gland contents, mated queen retinue size and egg-laying rate between queens that were reared in pesticide-free wax and those reared in wax contaminated with pesticides, as done in the present study. Based on our study’s findings, it seems possible that the differences observed by Walsh et al. (2020b) among control and treatment queens in terms of the chemical composition of mandibular gland contents was indeed caused by pesticide exposure during queen development, rather than by differences in the nutritional care provided toward queen larvae by nurse bees.
While pesticide exposure does affect the mandibular gland composition of adult queens, it is remains unclear when these changes take place in a queen’s life and what other pheromone-producing glands, if any, are affected. Brood pheromones change throughout the maturation process of honey bees, allowing nurses to feed larvae the appropriate diet for each developmental stage (Trouiller et al., 1991; Siefert et al., 2021). Previous work has also shown that brood pheromones communicate the cast of immature bees to nurse workers, allowing the nurses to recognize both queen larvae and pupae by detecting the fatty acid esters in the cuticle of developing queen larvae and pupae (Le Conte et al., 1994). The chemical composition of brood pheromones also changes based on nutritional status, allowing nurses to differentiate between well-fed and starved larvae at various developmental instars (Free and Winder, 1983; Free et al., 1989; He et al., 2016; Siefert et al., 2021). This suggests that the rearing conditions of larvae can dramatically impact brood pheromone composition. However, we found that pesticide exposure during development did not affect developing queen brood pheromones at any developmental stage regardless of pesticide exposure. It is possible that, when a colony is nutritionally stressed, pesticide exposure during development may play a greater role for larval health and brood pheromone composition. However, this interaction remains to be tested.
A survey of 75 queens produced by commercial apiaries in North America showed that the median number of ovarioles per queen ovary is 160 (range = 116–219; Jackson et al., 2011). These values are similar to the number of ovarioles per ovary that we counted in this study. In Drosophila melanogaster, ovariole number in female flies is positively correlated with egg production (Boulétreau-Merle et al., 1982). Historically, this has also been assumed to be the case in honey bees, as there is a correlation between queen ovariole count and number of square inches of sealed brood produced by a colony (Eckert, 1937). However, there have been no recent reports of correlation between queen ovariole count and other morphological measurements such as thoracic width (Jackson et al., 2011) or egg-laying rate (Walsh et al., 2020b). There may be a correlation between ovariole number or ovary weight and body weight, but this trend has been inconsistent at best in the few studies that have tested this idea (Delaney et al., 2011; De Souza et al., 2019). In this study, we did not observe a significant difference in ovariole count based on pesticide contamination of wax during queen development, even though we previously found that this type of exposure causes a significant drop in queen egg-laying rate (Walsh et al., 2020b). This leads us to propose that queen egg-laying rate is not necessarily dependent on ovariole number, and instead might be associated with other variables, such as adult queen nutrition and/or care.
The larvae we examined had similar chemical composition of brood pheromones throughout their development and nurse feeding visits were also similar, regardless of whether or not the developing queens were reared in pesticide-free or pesticide-laden wax. We also found no differences in the morphological characteristics of virgin or mated queens based on whether or not they were reared in contaminated wax. While it is encouraging that we did not detect negative effects of exposure to field-relevant concentrations and combinations of these pesticides, it would be irresponsible to conclude that the pesticides are harmless to queens. This is particularly important to note in light of other studies, including some of our own, which have documented the negative impact that these pesticides cause to other aspects of queen reproductive quality (Burley, 2007; Haarmann et al., 2002; Collins et al., 2004; Traynor et al., 2016; Dai et al., 2017). Moreover, these pesticides are widespread in North American apiaries (Mullin et al., 2010; Ostiguy et al., 2019; Traynor et al., 2016, 2021a) and there are known synergistic capabilities among these pesticides, when combined (Johnson et al., 2010, 2013). Our results make it clear that we are still discovering new information about the impacts of beekeeper-applied pesticide contamination on queen morphology (including ovariole count) and what these measurements imply at both the individual queen and colony-wide levels. Non-significant but numerical trends in our data, such as higher ovarioles in fluvalinate and coumaphos queens compared to control queens, and fewer nursing visits for amitraz queens compared to control queens, may merit future exploration with larger sample sizes. Therefore, there is ample opportunity for further discovery, particularly regarding how pesticide exposure impacts the molecular and physiological aspects of queen health and how this further relates to colony-wide productivity and survival.
Data Availability Statement
The raw data supporting the conclusions of this article will be made available by the authors, without undue reservation.
Author Contributions
EW did all queen rearing, nurse feeding rates, and dissection. EW and OK conducted data collection for ovariole numbers and brood pheromones. JG did brood pheromone data analysis. AH provided equipment and additional training for EW and OK to conduct brood pheromone analysis with GC-MS. EW and JR wrote the manuscript. All authors participated in editing the manuscript and have approved the final manuscript.
Funding
This work was supported in part by the Herb Dean Scholarship to cover EW’s tuition, funding to JR and NI by a USDA-NIFA award (2015-67013-23170), a grant from the California State Beekeepers Association, and Texas A&M University’s Hatch Project (TEX09557). Funding for the pheromone analyses was obtained from a North American Pollinator Protection Campaign (NAPPC) grant written by EW and JR.
Conflict of Interest
The authors declare that the research was conducted in the absence of any commercial or financial relationships that could be construed as a potential conflict of interest.
Acknowledgments
We would like to thank E. T. Ash, as well as Joseph and Anne Walsh, for generously sharing their beekeeping assistance and resources throughout the project. We are also indebted to members of the Rangel Honey Bee Laboratory, both past and present, who provided insight into this work during all stages of planning, implementation, and completion of this project.
Supplementary Material
The Supplementary Material for this article can be found online at: https://www.frontiersin.org/articles/10.3389/fevo.2021.681506/full#supplementary-material
References
Boulétreau-Merle, J., Allemand, R., Cohet, Y., and David, J. R. (1982). Reproductive strategy in Drosophila melanogaster: significance of a genetic divergence between temperate and tropical populations. Oecologia 53, 323–329. doi: 10.1007/BF00389008
Brouwers, E. V. M., Ebert, R., and Beetsma, J. (1986). Behavioural and physiological aspects of nurse bees in relation to the composition of larval food during caste differentiation in the honeybee. J. Apicult. Res. 26, 11–23. doi: 10.1080/00218839.1987.11100729
Burley, L. M. (2007). The Effects of Miticides on the Reproductive Physiology of Honey Bee (Apis mellifera L.) Queens and Drones. Master’s Thesis. Blacksburg, VA: Virginia Polytechnic Institute.
Collins, A. M., Pettis, J. S., Wilbanks, R., and Feldlaufer, M. F. (2004). Performance of honey bee (Apis mellifera) queens reared in beeswax cells impregnated with coumaphos. J. Apicult. Res. 43, 128–134. doi: 10.1371/journal.pone.0076536
Dai, P., Jack, C. J., Mortensen, A. N., and Ellis, J. D. (2017). Acute toxicity of five pesticides to Apis mellifera larvae reared in vitro. Pest. Manag. Sci. 73, 2282–2286. doi: 10.1002/ps.4608
De Souza, D. A., Huang, M. H., and Tarpy, D. R. (2019). Experimental improvement of honey bee (Apis mellifera L.) queen quality through nutritional and hormonal supplementation. Apidologie 50, 14–27. doi: 10.1007/s13592-018-0614-y
Delaney, D. A., Keller, J. J., Caren, J. R., and Tarpy, D. R. (2011). They physical, insemination, and reproductive quality of honey bee queens (Apis mellifera L.). Apidologie 42, 1–13. doi: 10.1051/apido/2010027
Eckert, J. E. (1934). Studies in the number of ovarioles in queen honeybees in relation to body size. J. Econ. Ento. 27, 629–635. doi: 10.1093/jee/27.3.629
Eckert, J. E. (1937). Relation of size to fecundity in queen honey bees. J. Econ. Ento. 30, 646–648. doi: 10.1093/jee/30.4.646
Elzen, P., Baxter, J., Spivak, M., and Wilson, W. (2000). Control of Varroa jacobsoni Oud. Resistant to fluvalinate and amitraz using coumaphos. Apidologie 31, 437–441. doi: 10.1051/apido:2000134
Elzen, P. J., and Westervelt, D. (2002). Detection of coumaphos resistance in Varroa destructor in Florida. Am. Bee J. 142, 291–292.
Fell, R. D., and Tignor, K. (2001). Miticide effects on the reproductive physiology of queens and drones. Am. Bee J. 141, 888–889.
Fine, J. D. (2020). Evaluation and comparison of the effects of three insect growth regulators on honey bee queen oviposition and egg eclosion. Ecotoxicol. Environ. Saf. 205:111142. doi: 10.1016/j.ecoenv.2020.111142
Fisher, A. II, and Rangel, J. (2018). Exposure to pesticides during development negatively affects honey bee (Apis mellifera) drone sperm viability. PLoS One 13:e0208630. doi: 10.1371/journal.pone.0208630
Free, J. B., Ferguson, A. W., and Simpkins, J. R. (1989). The effects of different periods of brood isolation on subsequent brood-cell visits by worker honeybees (Apis mellifera L.). J. Apicult. Res. 28, 22–25. doi: 10.1080/00218839.1989.11100815
Free, J. B., and Winder, M. E. (1983). Brood recognition by honeybee (Apis mellifera) workers. Anim. Behav. 31, 539–545. doi: 10.1016/S0003-3472(83)80077-80073
Gilley, D. C. (2001). The behavior of honey bees (Apis mellifera ligustica) during queen duels. Ethology 107, 601–622. doi: 10.1046/j.1439-0310.2001.00692.x
Haarmann, T., Spivak, M., Weaver, D., Weaver, B., and Glenn, T. (2002). Effects of fluvalinate and coumaphos on queen honey bees (Hymenoptera: Apidae) in two commercial queen rearing operations. J. Econ. Entomol. 95, 28–35. doi: 10.1603/0022-0493-95.1.28
Haber, A. I., Steinhauer, N. A., and vanEngelsdorp, D. (2019). Use of chemical and nonchemical methods for the control of Varroa destructor (Acari: Varroidae) and associated winter colony losses in U.S. beekeeping operations. J. Econ. Entomol. 112, 1509–1525. doi: 10.1093/jee/toz088
Harano, K., and Obara, Y. (2004). Virgin queens selectively destroy fully matured queen cells in the honeybee Apis mellifera L. Insectes Sociaux 51, 253–258. doi: 10.1007/s00040-004-0735-3
Harano, K. I., Shibai, Y., Sonezaki, T., and Sasaki, M. (2008). Behavioral strategies of virgin honeybee (Apis mellifera) queens in sister elimination: different responses to unemerged sisters depending on maturity. Sociobiology 52, 31–46.
Hartfelder, K., Tiberio, G. J., Lago, D. C., and Dallacqua, R. P. (2018). The ovary and its genes—developmental processes underlying the establishment and function of a highly divergent reproductive system in the female castes of the honey bee, Apis mellifera. Apidologie 49, 49–70. doi: 10.1007/s13592-017-0548-549
He, X. J., Zhang, X. C., Jiang, W. J., Barron, A. B., Zhang, J. H., and Zeng, Z. J. (2016). Starving honey bee (Apis mellifera) larvae signal pheromonally to worker bees. Sci. Rep. 6:22359. doi: 10.1038/srep22359
Jackson, J. T., Tarpy, D. R., and Fahrbach, S. E. (2011). Histological estimates of ovariole number in honey bee queens, Apis mellifera, reveal lack of correlation with other queen quality measures. J. Insect. Sci. 11, 1–11. doi: 10.1673/031.011.8201
Johnson, R. M., Dahlgren, L., Siegfried, B. D., and Ellis, M. D. (2013). Acaricide, fungicide and drug interactions in honey bees (Apis mellifera). PLoS One 8:e54092. doi: 10.1371/journal.pone.0054092
Johnson, R. M., Ellis, M. D., Mullin, C. A., and Frazier, M. (2010). Pesticides and honey bee toxicity-USA. Apidologie 41, 312–331. doi: 10.1051/apido/2010018
Katzav-Gozansky, T., Soroker, V., Francke, W., and Hefetz, A. (2003). Honeybee egg-laying workers mimic a queen signal. Insectes Soc. 50:20. doi: 10.1007/s000400300003
Laidlaw, H. H., and Eckert, J. E. (1964). Queen Rearing. California: University of California Press.
Le Conte, Y., Arnold, G., Trouiller, J., and Masson, C. (1990). Identification of a brood pheromone in honeybees. Naturwissenschaften 77, 334–336. doi: 10.1007/BF01138390
Le Conte, Y., Sreng, L., Sacher, N., Trouiller, J., Dusticier, G., and Poitout, S. H. (1994). Chemical recognition of queen cells by honey bee workers Apis mellifera (Hymenoptera: Apidae). Chemoecology 5, 6–12. doi: 10.1007/BF01259967
Ma, R., Mueller, U. G., and Rangel, J. (2016). Assessing the role of β-ocimene in regulating foraging behavior of the honey bee, Apis mellifera. Apidologie 47, 135–144. doi: 10.1007/s13592-015-0382-x
Ma, R., Rangel, J., and Grozinger, C. M. (2019). Honey bee (Apis mellifera) larval pheromones may regulate gene expression related to foraging task specialization. BMC Genomics 20:592. doi: 10.1186/s12864-019-5923-5927
Ma, R., Villar, G., Grozinger, C. M., and Rangel, J. (2018). Larval pheromones act as colony-wide regulators of collective foraging behavior in honeybees. Behav. Ecol. 29, 1132–1141. doi: 10.1093/beheco/ary090
Maisonnasse, A., Lenoir, J.-C., Beslay, D., Crauser, D., and Le Conte, Y. (2010). E-β-ocimene, a volatile brood pheromone involved in social regulation in the honey bee colony (Apis mellifera). PLoS One 5:e13531. doi: 10.1371/journal.pone.0013531
Metz, B. N., Pankiw, T., Tichy, S. E., Aronstein, K. A., and Crew, R. M. (2010). Variation in and responses to brood pheromone of the honey bee (Apis mellifera L.). J. Chem. Ecol. 36, 432–440. doi: 10.1007/s10886-010-9775-9775
Milone, J. P., Chakrabarti, P., Sagili, R. R., and Tarpy, D. R. (2021). Colony-level pesticide exposure affects honey bee (Apis mellifera L.) royal jelly production and nutritional composition. Chemosphere 263:128183. doi: 10.1016/j.chemosphere.2020.128183
Mullin, C. A., Frazier, M., Frazier, J. L., Ashcraft, S., Simonds, R., Vanengelsdorp, D., et al. (2010). High levels of miticides and agrochemicals in North American apiaries: implications for honey bee health. PLoS One 5:e9754. doi: 10.1371/journal.pone.0009754
Oksanen, J., Guillaume Blanchet, F., Friendly, M., Kindt, R., and Legendre, P. (2019). vegan : Community Ecology Package. R package version 2.5-6.
Ostiguy, N., Drummond, F. A., Aronstein, K., Eitzer, B., Ellis, J. D., Spivak, M., et al. (2019). Honey bee exposure to pesticides: a four-year nationwide study. Insects 10:13. doi: 10.3390/insects10010013
Payne, A. N., Walsh, E. M., and Rangel, J. (2019). Initial exposure of wax foundation to agrochemicals causes negligible effects on the growth and winter survival of incipient honey bee (Apis mellifera) colonies. Insects 10:19. doi: 10.3390/insects10010019
Pettis, J., Collins, A. M., Wilbanks, R., and Feldaufer, M. F. (2004). Effects of coumaphos on queen rearing in the honey bee, Apis mellifera. Apidologie 35, 605–610. doi: 10.1051/apido:2004056
Pettis, J. S., Wilson, W. T., Shimanuki, H., and Teel, P. D. (1991). Fluvalinate treatment of queen and worker honey bees (Apis mellifera L) and effects on subsequent mortality, queen acceptance and supersedure. Apidologie 22, 1–7. doi: 10.1051/apido:19910101
Rangel, J., Keller, J. J., and Tarpy, D. R. (2013). The effects of honey bee (Apis mellifera L.) queen reproductive potential on colony growth. Insect. Soc. 60, 65–73. doi: 10.1007/s00040-012-0267-261
Ratnieks, F. L. (1993). Egg-laying, egg-removal, and ovary development by workers in queenright honey bee colonies. Behav. Ecol. Sociobiol. 32:191. doi: 10.1007/BF00173777
Rinderer, T. E., De Guzman, L. I., Lancaster, V. A., Delatte, G. T., and Stelzer, J. A. (1999). Varroa in the mating yard: I. The effects of Varroa jacobsoni and Apistan on drone honey bees. Am. Bee J. 139, 134–139.
Rinkevich, F. D. (2020). Detection of amitraz resistance and reduced treatment efficacy in the Varroa Mite, Varroa destructor, within commercial beekeeping operations. PLoS One 15:e0227264. doi: 10.1371/journal.pone.0227264
Sagili, R. R., Metz, B. N., Lucas, H. M., Chkrabarti, P., and Breece, C. R. (2018). Honey bees consider larval nutritional status rather than genetic relatedness when selecting larvae for emergency queen rearing. Sci. Rep. 8:7679. doi: 10.1038/s41598-018-25976-25977y
Shoukry, R. S., Khattaby, A. M., El-Sheakh, A. A., Abo-Ghalia, A. H., and Elbanna, S. M. (2013). Effect of some materials for controlling varroa mite on the honeybee drones (Apis mellifera L.). Egyp. J. Agric. Res. 91, 825–834. doi: 10.21608/ejar.2013.165098
Siefert, P., Buling, N., and Grünewald, B. (2021). Honey bee behaviours within the hive: insights from long-term video analysis. PLoS One 16:e0247323. doi: 10.1371/journal.pone.0247323
Simpson, J. (1963). The mechanism of honey bee queen piping. Z. Vergleichende Physiol. 48, 277–282. doi: 10.1007/BF00339456
Sokol, R. (1996). The influence of a multimonth persistence of Fluwarol in a hive of a honey bee colony. Medycyna Weterynaryjna 52, 718–720.
Tanaka, E. D., and Hartfelder, K. (2004). The initial stages of oogenesis and their relation to differential fertility in the honey bee (Apis mellifera) castes. Arthropod. Struct. Dev. 33, 431–442. doi: 10.1016/j.asd.2004.06.006
Tarpy, D. R., Gilley, D. C., and Seeley, T. D. (2004). Levels of selection in a social insect: a review of conflict and cooperation during honey bee (Apis mellifera) queen replacement. Behav. Ecol. Sociobiol. 55, 513–523. doi: 10.1007/s00265-003-0738-735
Tarpy, D. R., and Mayer, M. K. (2009). The effects of size and reproductive quality on the outcomes of duels between honey bee queens (Apis mellifera L.). Ethol. Ecol. Evol. 21, 147–153. doi: 10.1080/08927014.2009.9522503
Tilley, C. A., and Oldroyd, B. P. (1997). Unequal subfamily proportions among honey bee queen and worker brood. Anim. Behav. 54, 1483–1490. doi: 10.1006/anbe.1997.0546
Traynor, K. S., Le Conte, Y., and Page, R. E. (2014). Queen and young larval pheromones impact nursing and reproductive physiology of honey bee (Apis mellifera) workers. Behav. Ecol. Sociobiol. 68, 2059–2073. doi: 10.1007/s00265-014-1811-y
Traynor, K. S., Le Conte, Y., and Page, R. E. (2015). Age matters: pheromone profiles of larvae differentially influence foraging behavior in the honeybee, Apis mellifera. Anim. Behav. 99, 1–8. doi: 10.1016/j.anbehav.2014.10.009
Traynor, K. S., Pettis, J. S., Tarpy, D. R., Mullin, C. A., Frazier, J. L., Frazier, M., et al. (2016). In-hive pesticide exposome: assessing risks to migratory honey bees from in-hive pesticide contamination in the Eastern United States. Sci. Rep. 6:33207. doi: 10.1038/srep33207
Traynor, K. S., Tosi, S., Rennich, K., Steinhauer, N., Forsgren, E., Rose, R., et al. (2021a). Pesticides in honey bee colonies: establishing a baseline for real world exposure over seven years in the USA. Environ. Pollut. 279:1161566. doi: 10.1016/j.envpol.2021.116566
Traynor, K. S., vanEngelsdorp, D., and Lamas, Z. S. (2021b). Social disruption: sublethal pesticides in pollen lead to Apis mellifera queen events and brood loss. Ecotoxicol. Environ. Saf. 214:112105. doi: 10.1016/j.ecoenv.2021.112105
Traynor, K. S., Wang, Y., Brent, C. S., Amdam, G. V., and Page, R. E. (2017). Young and old honeybee (Apis mellifera) larvae differentially prime the developmental maturation of their caregivers. Anim. Behav. 124, 193–202. doi: 10.1016/j.anbehav.2016.12.019
Trouiller, J. (1993). La communication Chimique Inter-Et Intra-Specifique Chez L’abeille. Relations Abeille-Varroa, Couvain-Ouvriere et Reine-Ouvriere. Doctoral dissertation. Paris: Université de Paris 7.
Trouiller, J., Arnold, G., Le Conte, Y., Masson, C., and Chappe, B. (1991). Temporal pheromonal and kairomonal secretion in the brood of honeybees. Naturwissenschaften 78, 368–370. doi: 10.1007/BF01131612
Wallner, K. (1999). Varroacides and their residues in bee products. Apidologie. 30, 235–248. doi: 10.1051/apido:19990212
Walsh, E. M., Janowiecki, M. A., Zhou, K., Ing, N. H., Vargo, E. L., and Rangel, J. (2020a). Elevated mating frequency in honey bee (Hymenoptera: Apidae) queens exposed to the miticide amitraz during development. Ann. Entomol. Soc. Am. 20, 1–7. doi: 10.1093/aesa/saaa041
Walsh, E. M., Sweet, S., Knap, A., Ing, N., and Rangel, J. (2020b). Queen honey bee (Apis mellifera) pheromone and reproductive behavior are affected by pesticide exposure during development. Behav. Ecol. Sociobiol. 74, 1–14. doi: 10.3920/JIFF2020.0017
Williams, G., Troxler, A., Retschnig, G., Roth, K., Yañez, O., Shutler, D., et al. (2015). Neonicotinoid pesticides severely affect honey bee queens. Sci. Rep. 5:14621. doi: 10.1038/srep14621
Withrow, J. M., and Tarpy, D. R. (2018). Cryptic “royal” subfamilies in honey bee (Apis mellifera) colonies. PLoS One 13:e0199124. doi: 10.1371/journal.pone.0199124
Wu, F., Ma, C., Han, B., Meng, L., Hu, H., Fang, Y., et al. (2019). Behavioural, physiological and molecular changes in alloparental caregivers may be responsible for selection response for female reproductive investment in honey bees. Mol. Ecol. 28, 4212–4227. doi: 10.1111/mec.15207
Wu, J. Y., Anelli, C. M., and Sheppard, W. S. (2011). Sub-lethal effects of pesticide residues in brood comb on worker honey bee (Apis mellifera) development and longevity. PLoS One 6:e14720. doi: 10.1371/journal.pone.0014720
Keywords: reproductive fitness, queen health, pesticides, morphology, brood pheromones, honey bee, nurse behaviors
Citation: Walsh EM, Khan O, Grunseich J, Helms AM, Ing NH and Rangel J (2021) Pesticide Exposure During Development Does Not Affect the Larval Pheromones, Feeding Rates, or Morphology of Adult Honey Bee (Apis mellifera) Queens. Front. Ecol. Evol. 9:681506. doi: 10.3389/fevo.2021.681506
Received: 16 March 2021; Accepted: 07 May 2021;
Published: 09 June 2021.
Edited by:
Alison McAfee, North Carolina State University, United StatesReviewed by:
Pierre Giovenazzo, Laval University, CanadaKirsten Shoshanna Traynor, Arizona State University, United States
Copyright © 2021 Walsh, Khan, Grunseich, Helms, Ing and Rangel. This is an open-access article distributed under the terms of the Creative Commons Attribution License (CC BY). The use, distribution or reproduction in other forums is permitted, provided the original author(s) and the copyright owner(s) are credited and that the original publication in this journal is cited, in accordance with accepted academic practice. No use, distribution or reproduction is permitted which does not comply with these terms.
*Correspondence: Juliana Rangel, anJhbmdlbEB0YW11LmVkdQ==