- 1Instituto de Ciencias Ambientales y Ecológicas, Universidad de Los Andes, Mérida, Venezuela
- 2Consorcio para el Desarrollo Sostenible de la Ecorregión Andina (CONDESAN), Quito, Ecuador
- 3Laboratorio de Dendrología, Facultad de Ciencias Ambientales y Forestales, Universidad de Los Andes, Mérida, Venezuela
- 4Instituto Jardín Botánico de Mérida, Universidad de Los Andes, Mérida, Venezuela
- 5Ecological Plant Geography, Faculty of Geography, Universityof Marburg, Marburg, Germany
- 6Grupo de Investigación en Biodiversidad, Medio Ambiente y Salud – BIOMAS, Universidad de las Américas, Quito, Ecuador
Analyzing plant phenology and plant–animal interaction networks can provide sensitive mechanistic indicators to understand the response of alpine plant communities to climate change. However, monitoring data to analyze these processes is scarce in alpine ecosystems, particularly in the highland tropics. The Andean páramos constitute the coldest biodiversity hotspot on Earth, and their species and ecosystems are among the most exposed and vulnerable to the effects of climate change. Here, we analyze for the first time baseline data for monitoring plant phenological dynamics and plant–pollinator networks along an elevation gradient between 4,200 and 4,600 m asl in three mountain summits of the Venezuelan Andes, which are part of the GLORIA monitoring network. We estimated the presence and density of plants with flowers in all the summits and in permanent plots, every month for 1 year. Additionally, we identified pollinators. We calculated a phenological overlap index between species. We summarized the plant–pollinator interactions as a bipartite matrix and represented a quantitative plant–pollinator network, calculating structural properties (grade, connectance, nestedness, and specialization). We also evaluated whether the overall network structure was influenced by differences in sampling effort, changes in species composition between summits, and phenology of the plant species. Finally, we characterized the pollination syndrome of all species. Flowering showed a marked seasonality, with a peak toward the end of the wet season. The overall phenological overlap index was low (0.32), suggesting little synchrony in flowering among species. Species richness of both plants and pollinators decreased along the elevation gradient. Flies, bumblebees, and hummingbirds were the most frequent pollinators in the network, while entomophily and anemophily were the prevailing pollination syndromes. The interaction network in all summits showed high connectance values, significant specialization (H2), and low nestedness. We did not find a significant effect of sampling effort, summit plant species composition, or plant phenology on network structure. Our results indicate that these high tropical alpine plant communities and their plant-pollination networks could be particularly vulnerable to the loss of species in climate change scenarios, given their low species richness and functional redundancy coupled with a high degree of specialization and endemism.
Introduction
Climate change is accelerating and is having important impacts on species range shifts, which are particularly well documented in cold biomes, where there is increasing evidence that alpine species distributions are shifting upwards (Lenoir and Svenning, 2015; Steinbauer et al., 2019). Long-term research within the framework of monitoring networks such as the Global Observation Research Initiative in Alpine Environment (GLORIA) has shown that global warming is affecting community structure (Steinbauer et al., 2019). For example, in European mountains, climate warming and changes in precipitation are having contrasting effects on the summit floras, increasing species richness in boreal-temperate summits but decreasing richness in Mediterranean sites, probably because of increased drought severity in the European south (Pauli et al., 2012; Lamprecht et al., 2021). Monitoring within the GLORIA–Andes network in northwestern Argentina has shown an increase in the abundance of small herbs and disperse grasses (Carilla et al., 2017). However, in the context of monitoring long-term vegetation dynamics in alpine ecosystems, much less is known about the impacts of climate change on plant reproduction (but see Tovar et al., 2020 in the case of plant dispersal strategies), including phenological responses, changes in plant-pollination, and the possible relation between these two processes.
In plants and crops from the temperate zone, evidence has shown that increases in temperature in recent years, can have a significant effect on phenological patterns, including the time for the beginning or the amplitude of the flowering or fruiting period, as well as significant disruptions of pollination interactions (Harrison, 2000; Chapman et al., 2005; Inouye, 2008). In diverse and/or generalist systems, the wide availability of different pollinators can provide a buffer against alterations in phenological patterns (Anderson et al., 2012). Moreover, in systems with a high degree of functional redundancy, pollinator species with strong preferences for certain floral traits have less need to synchronize their dynamics with the phenology of particular plant species (Benadi et al., 2014). However, in systems with less functional redundancy and/or with many specialists, there can be a greater susceptibility to species losses and changes in phenology, resulting in adverse effects on the stability of plant–pollinator networks (Bartomeus et al., 2011, 2013).
In alpine systems, pollinator’s diversity is generally low compared with lowland diversity (Ramirez and Brito, 1992). In fact, in mountain systems, the ratio of pollinator/plant abundance decreases with elevation (i.e., pollinators become increasingly scarce for plants), while generalist and specialized systems coexist, although generalist systems tend to dominate (Medan et al., 2002; Ramos-Jiliberto et al., 2010; Trøjelsgaard and Olesen, 2013). Species displacements from lower to higher elevations, changes in species abundance, or species extinctions linked with climate change could all cause alterations in mutualistic plant–pollinator systems. Hence, there is an urgent need for studies on this topic that encompass the wide spatial and temporal variability (within and between years) characteristic of alpine areas around the world and which can provide a baseline for future comparison of plant phenological dynamics and plant–pollinator interactions in global change scenarios (Forrest, 2014; Inouye, 2020).
In this context, the study of ecological interactions and complex networks should offer key insights to analyze primary ecological properties like the interdependence of the components of the network (Bascompte et al., 2006). For example, the analysis of connectance allows one to assess the degree of association between species; metrics such as the degree of nesting can help to evaluate the robustness of the system to species loss, while specialization indexes can provide a measure of the degree of species interdependence (Jordano, 1987; Bascompte et al., 2006; Blüthgen et al., 2006, 2007; Burgos et al., 2007; Memmott et al., 2007; Tylianakis et al., 2007; Almeida-Neto and Ulrich, 2011; Devoto et al., 2012; Dormann and Strauss, 2014). Moreover, the probability of alternative matrices of interactions can be computed to evaluate variables that could influence network structure on the basis of important ecological attributes that are thought to have explanatory value (e.g., species abundance patters and flowering phenology), which can then be compared to null models (Vázquez et al., 2009; Vázquez, 2010).
The available studies of plant–pollinator networks in temperate alpine ecosystems have generally reported a high degree of nestedness (Dupont et al., 2003) and low connectance values (Olesen and Jordano, 2002; Santamaría et al., 2014). However, the study of plant reproductive ecology and plant–animal interactions has received substantially less attention in the case of the tropical mountain ecosystems, with the few available studies concentrating on the páramos of the tropical Andes (Aguirre et al., 2011; Llambí and Rada, 2019). The páramos are diverse high tropical mountain ecosystems distributed between 3,000 and 4,800 m along the Northern Andes (Monasterio, 1980b; Josse et al., 2011). The high specialization of the flora to the conditions of the cold tropics suggests that these species are particularly vulnerable to global warming (Anthelme et al., 2014). In fact, recent continental scale research in long-term monitoring summits of the GLORIA–Andes network has shown that vegetation in páramo summits of the northern Andes is particularly vulnerable to habitat loss in climate change scenarios because of a high abundance of endemic species with narrow thermal niches (Cuesta et al., 2020).
The few available studies on the reproductive ecology and pollination in the high tropical Andes reinforce the idea that these systems could be sensitive to the loss of species due to global changes, given that they are relatively poor in pollinator species and show high endemism (Ramos-Jiliberto et al., 2010; González and Loiselle, 2016; Pelayo et al., 2019). Analyses on plant phenology and reproductive aspects in the páramos have focused on very emblematic species such as the giant rosettes (Espeletia spp.), as well as some exploratory studies on small herbs and cushions (Berry, 1986; Berry and Calvo, 1994; Fagua and Bonilla, 2005). Thus, we know that giant rosettes species are entomophilous and anemophilous, while in the case of herbs and cushions, self-fertilization seems to predominate.
One of the few available studies on plant phenology and pollination at a community scale in the alpine tropics was carried out in the Venezuelan páramos between 3,000 and 4,200 m (Pelayo et al., 2019). This study showed that flowering occurs gradually from the beginning to the end of the rainy season (from May to November), with a low overlap of flowering between species. Few species exhibited constant flowering throughout the year, and few species bloomed during the dry season (from December to April), which tends to be particularly harsh in the Venezuelan páramos (compared to other páramos in the Northern Andes). A high proportion of the studied species were pollinated by animals, including hummingbirds and bumblebees. Moreover, the structure of plant–pollinator networks showed significant specialization; low values of connectance, asymmetry, and nestedness; and high values of functional complementarity (Pelayo et al., 2019). However, this study did not fully cover the superpáramo or subnival belt (above 4,200 m asl), an ecological belt where more extreme climatic and edaphic conditions could be linked to a decrease in richness of plants and pollinators. In a more humid superpáramo in the Sierra Nevada de Mérida (4,155–4,220 m), Manrique et al. (2019) reported a network with significant specialization, which was also influenced by phenotypic and phenological adjustments.
In this context, the presence of three permanent monitoring summits of the GLORIA–Andes network in the Venezuelan superpáramos (between 4,200 and 4,600 m asl, see Cuesta et al., 2017; Gámez et al., 2020) offers a unique opportunity to (a) establish a baseline to analyze the potential impacts of climate change on the reproductive behavior of high elevation species in tropical mountain ecosystems; (b) develop a protocol for recording the phenology of annual flowering in the different summits and orientations; and (c) document the dynamics of the interactions between plants with flowers and their pollinators. Hence, the objective of this study was to analyze changes in the flowering phenology and the structure of the plant–pollinator network in three summits along an elevation gradient in a high belt of Venezuelan páramos.
Based on previous results from páramos at lower elevations (Pelayo et al., 2019), we evaluated the following predictions: (1) the phenological behavior of the plant community will be strongly influenced by the marked rainfall seasonality of the Venezuelan páramo, and the phenological overlap index could increase with elevation as the climatic filtering for flowering becomes more severe (i.e., more severe seasonal thermal and hydric stress at higher elevations); (2) we expect a higher incidence of pollinator visits in periods when flower availability is higher; (3) we expect a reduction in the species richness of both plants and pollinators and a shift in the relative importance of the different plant growth forms (increase in the predominance of small flowering herbs) and pollinator taxa (reduction in the importance of birds and bumblebees vs. flies); (4) these networks are expected to show high levels of specialization but low levels of connectance and nestedness compared to other systems at lower elevations; and (5) the network structure should be influenced by plant phenology and the patterns of change in plant species composition between summits. The assessment of these predictions should provide a more detailed and mechanistic understanding of the vulnerability to climate change of these unique high elevation tropical communities.
Materials and Methods
Study Area
This study was carried out in the Piedras Blancas páramo, in the northeast region of the Sierra de La Culata National Park, Venezuela. The region is influenced by the dry climate of the high Chama river basin, with a total annual precipitation of 860 mm (Pico El Águila weather station, 4,118 m asl). Less than 5% of this precipitation falls during the dry season, extending from December to March (Sarmiento, 1986). Mean monthly temperatures vary less than 2–3°C, and the growing season extends all year. However, there are large daily temperature oscillations, that increase in amplitude during the dry season, when air temperatures can reach 25°C during the day and drop at night below –5°C (Monasterio, 1986; Azócar and Rada, 2006).
The subnival belt (locally known as high Andean páramo or superpáramo) extends between 4,200 and 4,800 m asl. Within this belt, two types of vegetation can be distinguished: (a) the desert páramo, dominated by scattered giant rosettes (genus Espeletia) together with a lower stratum dominated by cushion plants, acaulescent rosettes, low shrubs, tussock grasses, and herbs (4,200–4,500 m asl); and (b) the periglacial desert, where plant cover is normally less than 10% and small grasses and herbs are dominant (typically above 4,500 m, Monasterio, 1980a).
The sampling sites were located in the three highest monitoring summits of the GLORIA–Andes network in Venezuela (4,207, 4,402, and 4,604 m asl) in La Culata-Piedras Blancas site (VE-CPB, Cuesta et al., 2017; Gámez et al., 2020). This site was installed in 2012 and a first resampling campaign was implemented in 2017. The total richness of vascular plants in these summits is 71 species, distributed in 53 genera, and 24 families (the most common being Asteraceae and Poaceae). Vegetation cover and total species richness in these summits decrease from 59% and 59 spp. at 4,207 m to 9.1% and 14 species at 4,604 m asl (Table 1).
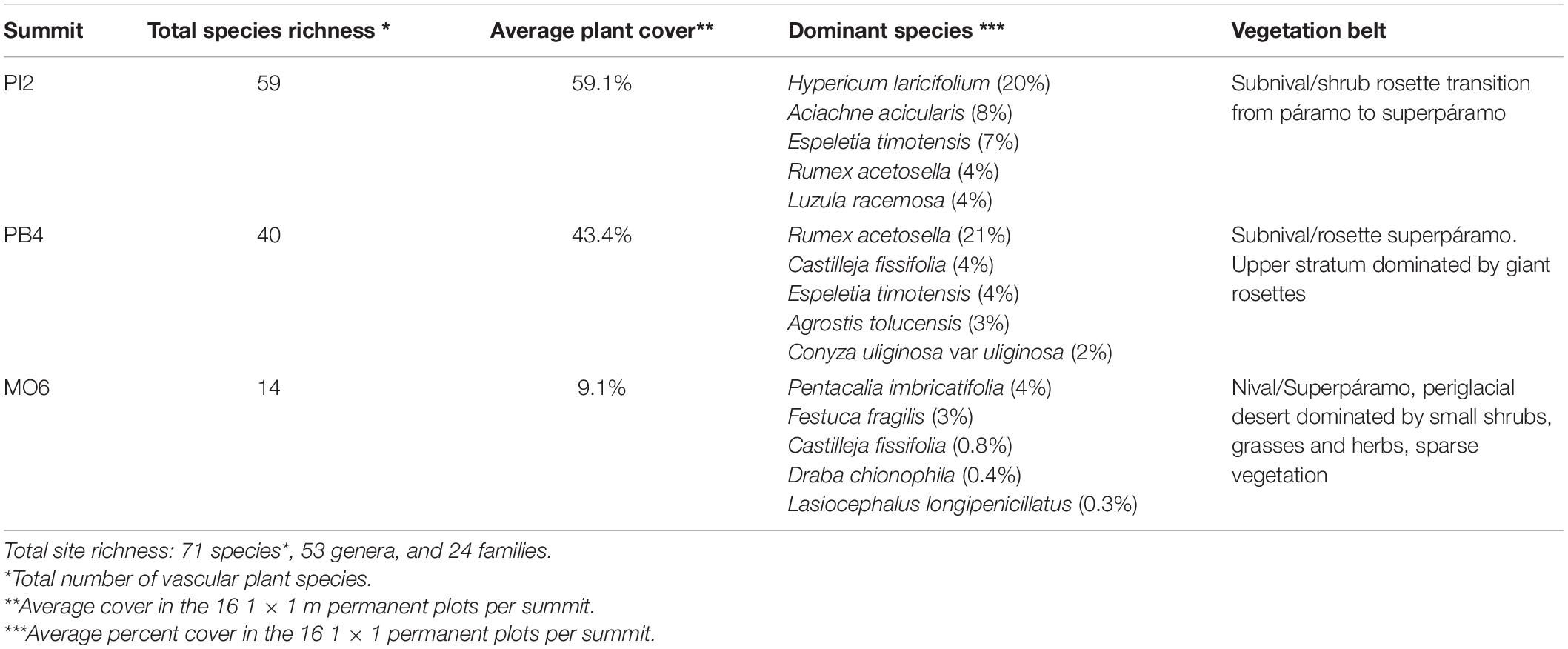
Table 1. Characteristics of the selected summits for monitoring the flowering phenology and plant–pollinators networks within the framework of the GLORIA–Andes network in Venezuela.
In the lowest summit (PI2), vegetation corresponds to a shrubland-rosette páramo; the upper stratum is dominated by the sclerophyllous shrub Hypericum laricifolium and the stem rosette Espeletia schultzii, while the lower stratum is dominated by the graminoids A. acicularis and L. racemosa and the exotic herb R. acetosella. In the intermediate summit at 4,402 m (PB4), vegetation corresponds to a giant rosette superpáramo; the giant stem rosette Espeletia timotensis is dominant in the upper stratum, while the lower stratum is dominated by herbs (R. acetosella, Castilleja fissifolia) and grasses (e.g., Agrostis tolucensis). Finally, the 4,604 summit (MO6) corresponds to a periglacial desert, with a single stratum dominated by small shrubs (Pentacalia imbricatifolia), grasses (Festuca fragilis), small herbs (C. fissifolia and Lasiocephalus longipenicillatus), and basal rosettes (D. chionophila) (Gámez et al., 2020).
Flowering Phenology
The quantitative analysis of plant phenology focused on the 3 × 3 m permanent plots (n = 4) located in the four cardinal orientations of each summit, established following the standard GLORIA network protocol (Pauli et al., 2015). During 1 year (December 2017–November 2018), we carried out 1-day visits between the last 2 weeks of each month to each summit, in order to count the number of individuals of vascular plants with flowers in the total area of each 3 × 3 m plot. We decided not to consider grasses, because this group is anemophilyc and relatively abundant. Hence, this allowed optimizing the time used for observing plants with flowers that could possibly receive visits from pollinators.
A complementary qualitative sampling was also implemented, registering the presence/absence of plants with flowers of each species in the whole summit area sections located in each cardinal orientation between the highest summit point and the 10-m-elevation contour line (see Pauli et al., 2015). Based on the qualitative information of presence/absence of plants with flowers for the whole summit each month, we represented the phenological patterns in graphs and calculated the overlap index (Primack, 1985) to analyze the degree of synchrony of flowering among species. This index ranges from 0 to 1, with 0 corresponding to a complete lack of synchrony and 1 to total synchrony. For this analysis, we integrated the information from the four orientations of each summit, and analyzed each summit separately or all summits together for a general synthesis of the three summits in the superpáramo belt. We also analyzed changes between the three summits in the proportion of species that exhibited different pollination syndromes, based on the morphology of their flowers, following the classification proposed by Faegri and van der Pijl (1979).
Plant–Pollinator Network
During each monthly visit to each of the summits, we recorded all the interactions observed between flowers and pollinators, while collecting the phenological data. For this, we used direct observation and binoculars (10 × 42), when necessary (a single expert carried out the visitation observations in all cases). In each case, the number of visits made by the pollinator was recorded, but only if it contacted the reproductive structures of the flowers. The unknown insects were collected for later identification at the species level, in the case of bumblebees and at the morphospecies and family level in the rest. They were deposited in the Entomological Collection at Universidad de Los Andes (Mérida, Venezuela). Bird pollinators were identified directly in the field by comparison with the Bird Guide of Venezuela (Hilty, 2003). The observation time varied between 2 and 6 h for each visit, depending on the weather conditions, as we were not able to register interactions during precipitation events (animal activity normally ceases). Simultaneous with direct observations, we placed 2 GOPRO White 3 cameras next to the plants with more abundant flowering during each visit (Pelayo, 2017). These normally focused on one or two individuals within the field of vision of the camera. The cameras took high-resolution pictures every 0.5 s. In total, during the year, we made a total sampling effort of 92 h of direct observations and 107 h of camera recordings and visually analyzed a total of 385,200 images. A limitation of the use of these cameras is that in many cases, the small insects are not observed in detail in the photographs, so it is very important and advisable to become familiar with the floral visitors in the field, for the subsequent analysis of the photographs.
Rarefaction curves and the Chao 2 richness index were calculated for the observed pollinators using EstimateS 9.1.0 (Colwell, 2013) to evaluate the completeness of our sampling effort. In the case of plants (excluding grasses) we observed 55 species with flowers across the year of observation, corresponding to 87.3% of the 63 species that we know to be present based on the full plant inventory in these summits from 2012 to 2017 (Gámez et al., 2020). Some of the few missing species (e.g., E. timotensis) are in fact known to flower every 2 or 3 years, and they are pollinized by wind, so these would not affect our analysis of the pollination network (Berry, 1986; Berry and Calvo, 1994).
We calculated the correlation (Spearman) between the number of monthly visits with (a) the total number of plants with flowers in all the 3 × 3 quadrants; and (b) number of species with flowers each month (for the whole summit).
With the information collected each month, we built matrixes for each summit (plant species in the rows and the pollinators in the columns), summarizing the total number of visits received by the flowers of each plant species by each type of pollinator during the sampling year. Based on these quantitative matrixes, we developed a plant–pollinator network for each summit and for the three summits together, and calculated their structural properties by means of the following metrics: (i) degree of a species (Bascompte et al., 2006), (ii) connectance (Jordano, 1987), (iii) weighted nestedness metric based on overlap and decreasing fill (NODF; Almeida-Neto and Ulrich, 2011; based on the quantitative interaction weights), and (iv) specialization index (H2; Blüthgen et al., 2006; based on quantitative interaction weights). Calculations of network metrics were conducted with the bipartite package (Dormann et al., 2008) in R 2.15.2 (R Core Team, 2016). Significance of weighted NODF and H2 was tested by comparing empirical values vs. a null model (10,000 repetitions of similar dimension network) in R 2.15.2.
The structure of the general plant–pollinator network was contrasted with alternative models based on attributes of the plant species (Vázquez et al., 2009), including phenology (qualitative and quantitative) and spatial distribution (presence–absence of each species in each of the three summits). We also evaluated if network structure could be explained by sampling effort (focal and video observation time per species). In some cases, combined models were also performed to explore the joint relationship of two variables, for example, phenology and plant distribution, focal and video hours. We carried out this analysis for the whole network based on the data for the three summits combined, because network sizes were too small to allow meaningful tests based on data from each individual summit taken separately. We did not implement models including variables related to fauna, since we only registered their visitation rates. Finally, a null model was created in which all interactions were assigned the same probability of occurrence [p = 1/(P ∗ A)] (where P and A were equal to the number of plant and animal species, respectively). Akaike’s information criterion (AIC) was calculated for each of these modeled matrices, which allowed to select the most plausible model (lowest AIC), to explain the observed matrix. Given a set of candidate models for the data, the preferred model is the one with the minimum value in the AIC and therefore p = 1, where p = exp [(AICmin - AICj)/2] and AICmin represents the lowest AIC of the four models explored (Burnham and Anderson, 2002). This analysis was carried out using the “mlik” function of the “ecolnet” package (Vázquez, 2010) of R 2.15.2.
Results
Flowering Phenology
In the three studied summits, we collected information on the phenology of flowering of 55 species that produced flowers during the study year, belonging to 19 families (excluding grasses). The families Asteraceae and Rosaceae presented the highest number of species with 26 spp. and 5 spp., respectively, followed by Brassicaceae, Caryophyllaceae, and Orobanchaceae, with three species each. Forty-seven species have characteristics related to entomophily, 2 to anemophily, 3 to ornithophily, and 3 exhibit mixed characteristics between entomophily and ornithophily (Figure 1).
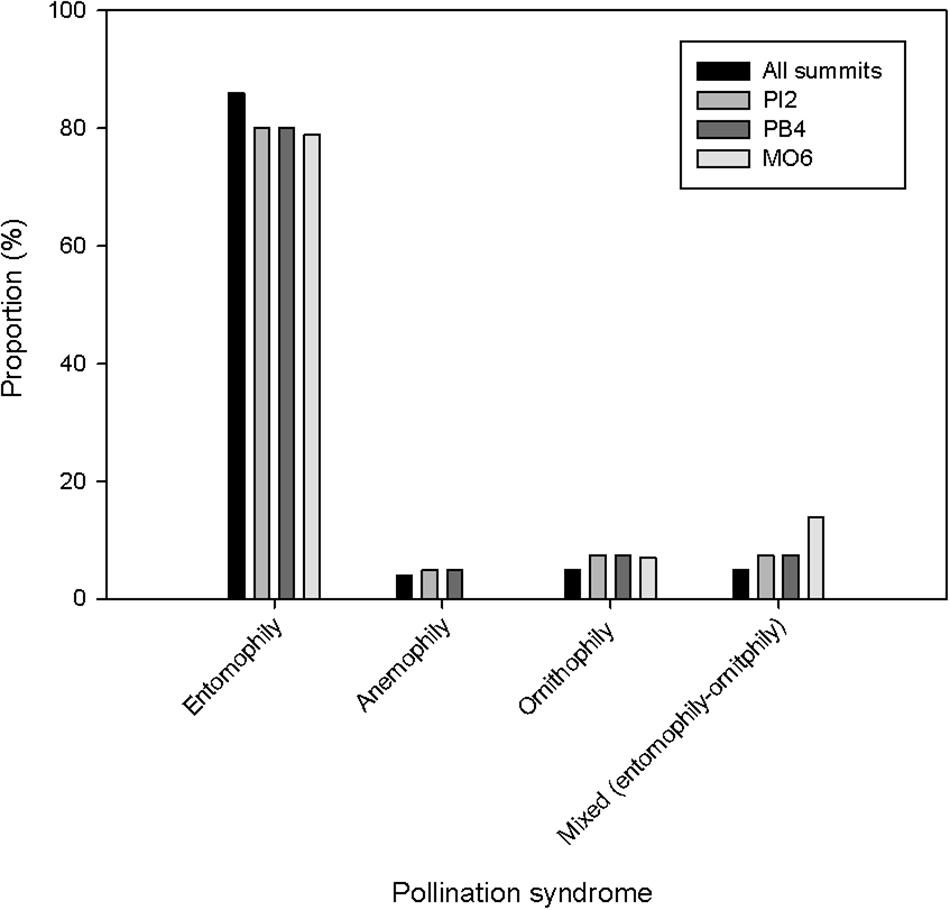
Figure 1. Proportion (%) of plant species that belong to each pollination syndrome in the three summits (PI2, PB4, and MO6) of the GLORIA–Andes network (VE-CPB site), at La Culata National Park, Piedras Blancas Páramo, Venezuela.
Most species flowered during the wet season (Figure 2), and tended to show limited overlap with each other during their flowering periods. However, a few species such as C. fissifolia, Draba pulvinata, H. laricifolium, Lachemilla polylepis, L. longipenicillatus, Conyza uliginosa var uliginosa, and Valeriana parviflora showed continuous flowering. There were also some species that were able to produce flowers during the dry season, some of them with periods of explosive blooming limited to a single month per year. The rest of the species showed a long bloom period. These general patterns were characteristic of the two lower summits (PI2 and PB4). However, in the highest summit (MO6), flowering began in the middle of the rainy season and lasted until the dry season (Figure 2). The overall phenological overlap index between plant species was low (0.32), suggesting little synchrony in flowering between species. This index increased with elevation (Table 2), suggesting that flowering could become more synchronic in the highest summit. For example, Senecio wedglacialis and P. imbricatifolia, which were abundant species present in the three summits, showed a tendency to bloom later in the year with increasing elevation, while their flowering period become shorter at higher altitudes (Figure 2).
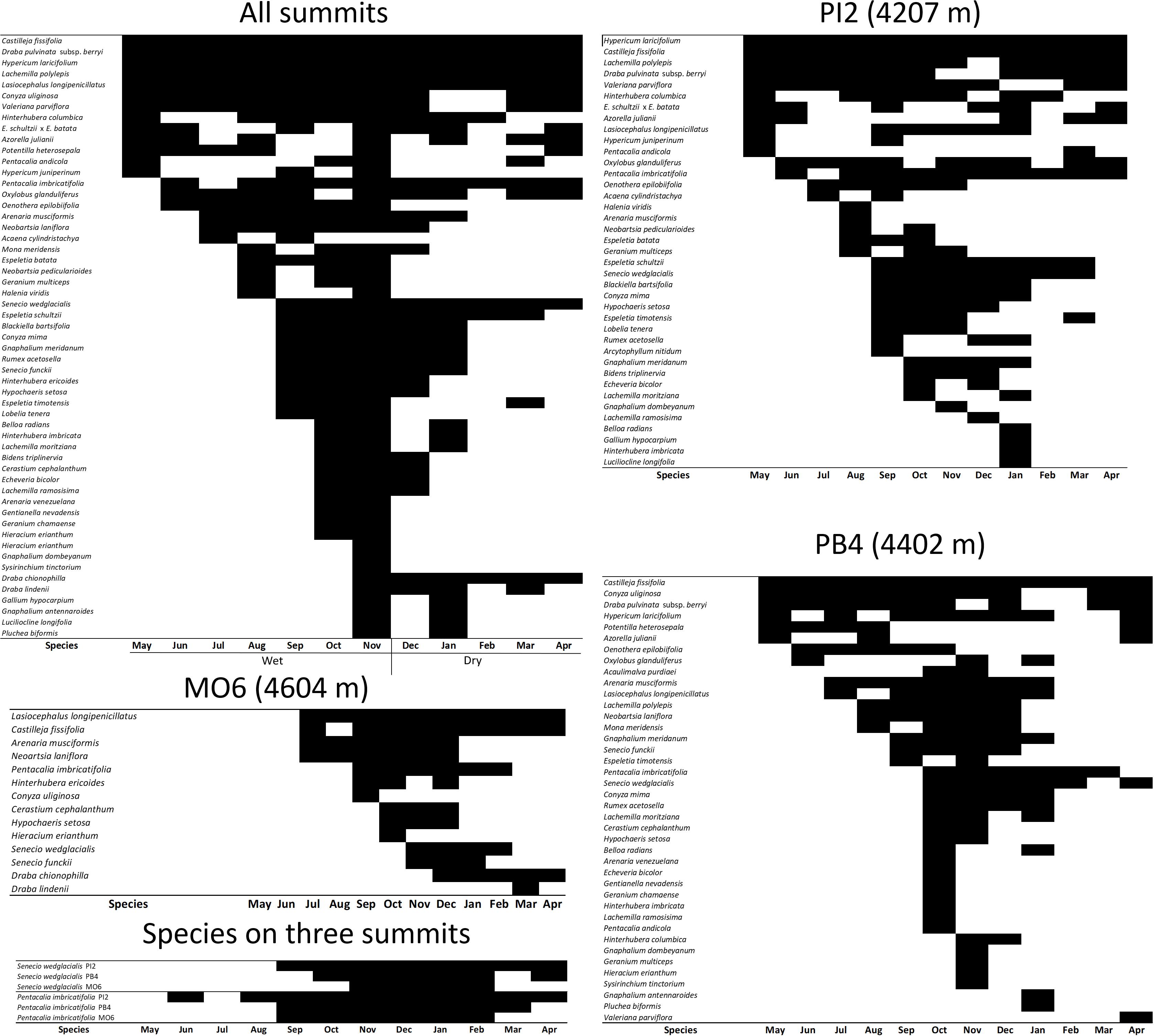
Figure 2. Qualitative phenology (presence in black-absence in white) of flowering of the 43 vascular plant species studied in three summits of the GLORIA–Andes network (VE-CPB site), at La Culata National Park, Piedras Blancas Páramo, Venezuela. Species are ordered first in decreasing order in terms of the duration of flowering, and secondly in terms of the coincidence of flowering with the beginning of the wet season (May).
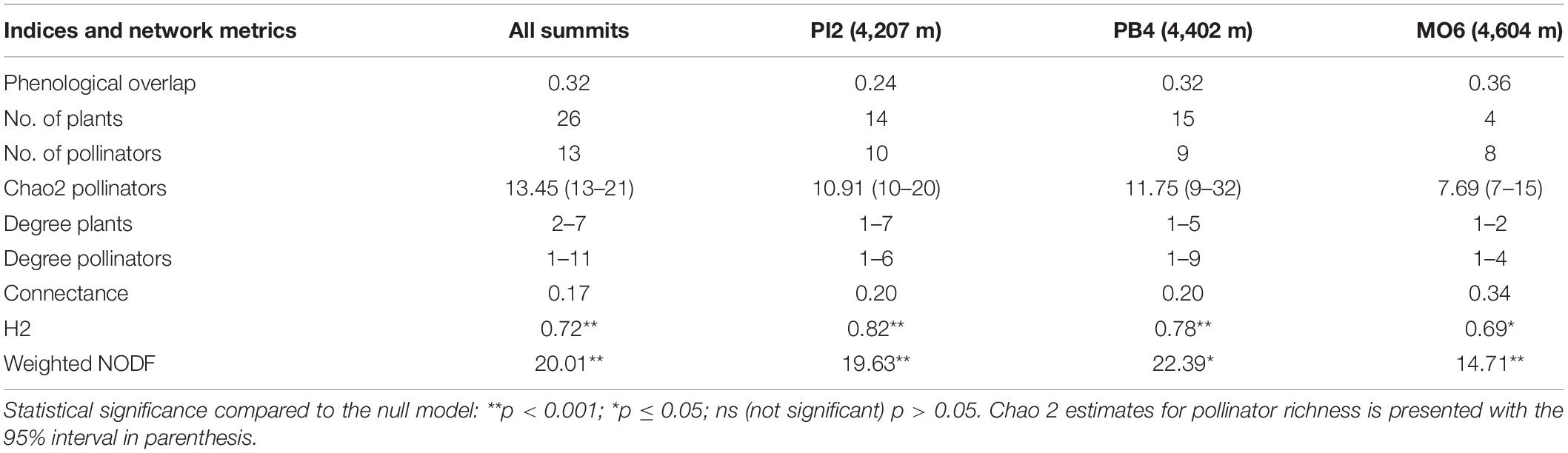
Table 2. Phenological overlap index between plants and structural properties of pollination networks in superpáramo summits (la Culata-Piedras Blancas site, GLORIA–Andes, Venezuela).
We found that the species with the highest density of plants with flowers changed with summit elevation (Figure 3). In PI2, the dominant species were the shrub H. laricifolium and the herbs Conyza mima and Oenothera epilobifolia. In PB4, the herbs C. fissifolia, C. mima, and Gnaphalium meridanum were dominant. Finally, in MO6, the herbs P. imbricatifolia, Bartsia laniflora, and L. longipenicillatus dominated. Regarding the effect of orientation within the summits, in the lowest summit (PI2), the highest number of individuals with flowers were recorded in the south and east orientations. In PB4 and MO6 summits, the highest density of plants with flowers was recorded in the north and west orientations (Figure 4).
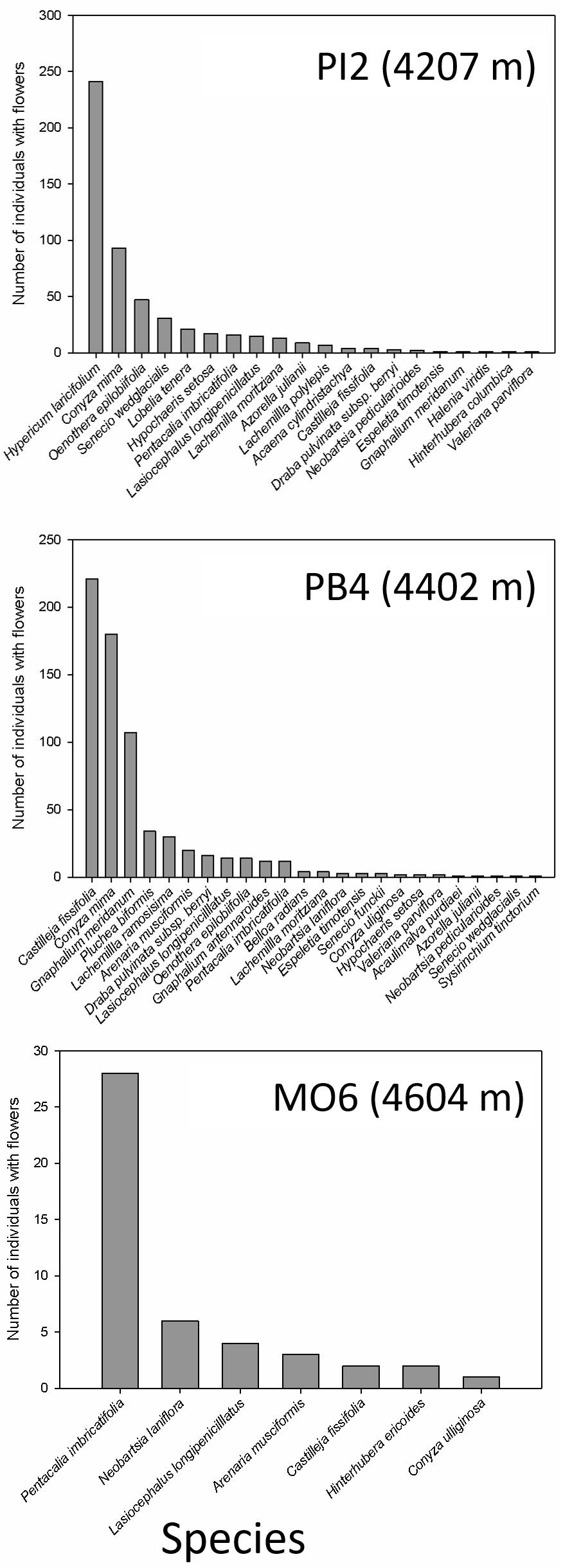
Figure 3. Total number of recorded individuals with flowers per species in the four 3 × 3 m permanent plots (36 m2) during 1 year, in each summit of the GLORIA–Andes network (VE-CPB site), at La Culata National Park, Piedras Blancas Páramo, Venezuela.
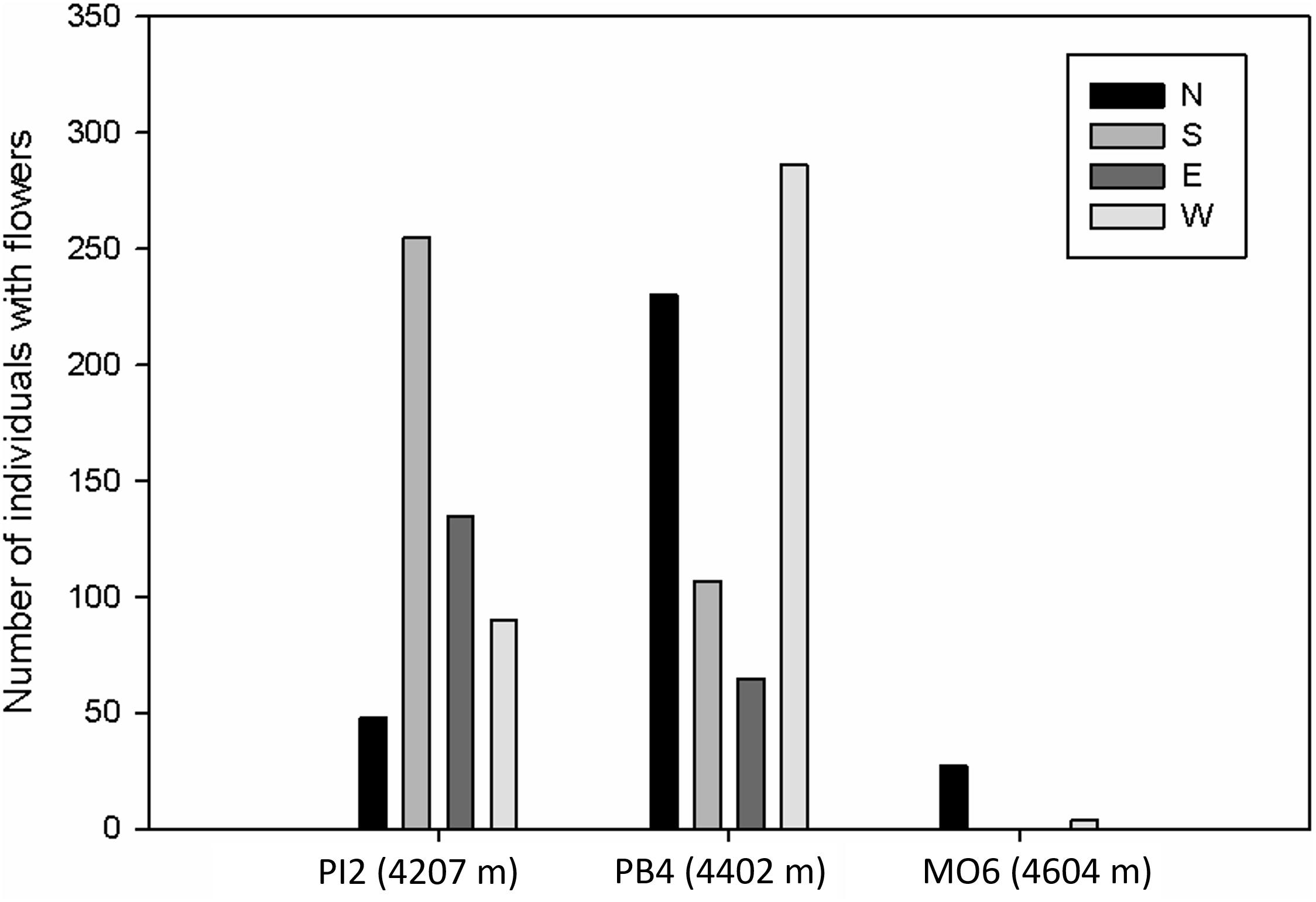
Figure 4. Number of individuals with flowers in each cardinal orientation in the summits of the GLORIA–Andes network (VE-CPB site), at La Culata National Park, Piedras Blancas Páramo, Venezuela.
Plant–Pollinator Networks
Of the 55 species that produced flowers during the year, 26 were included in the general pollination network (Figure 5), while the rest were not visited during our observations. We registered a total of 13 species of pollinators. This was within the 95% confidence interval of the Chao 2 richness index for pollinators (13–21 species), suggesting that the pollinator sampling was nearly complete (Table 2). The guild of floral visitors observed covered taxa as diverse as hummingbirds (2 species), flower piercers (1), flies (5), bumblebees (2), bees (1), wasp (1), and butterflies (1). With 47.68% of all of the interactions observed in the three summits (total frequency), flies were the most frequent floral visitors. Two bumblebees (Bombus rohweri and B. rubicundus) accounted for 35.31%; bees and the wasp, 9.80%; hummingbirds, 5.67%; flower piercer, 1.25%; and butterflies, 0.29% (Figure 5).
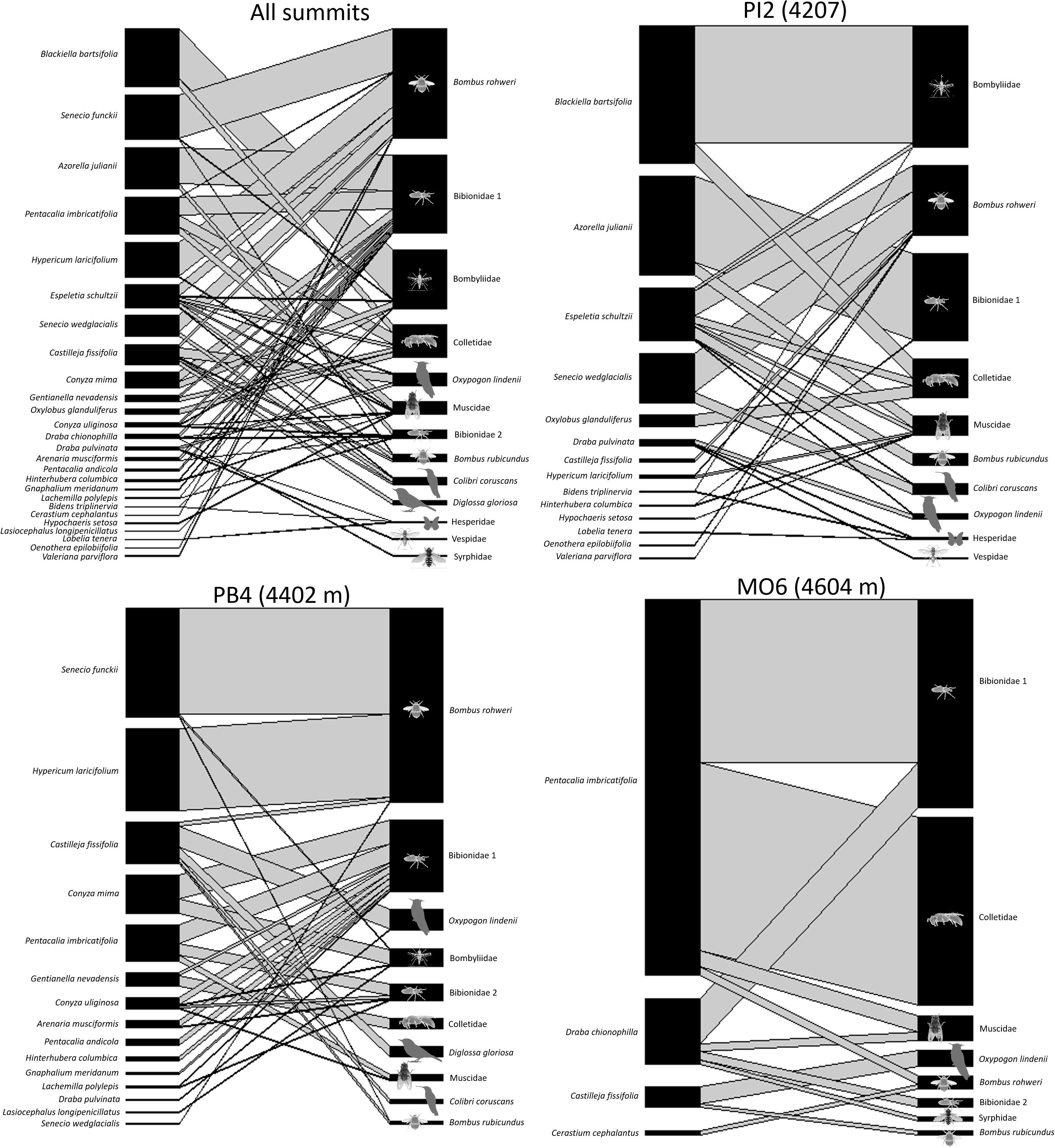
Figure 5. Plant–pollinator networks of the GLORIA–Andes network (VE-CPB site), at La Culata National Park, Piedras Blancas Páramo, Venezuela. The thickness of the links represents the total intensity of the interaction (visitation frequency). The size of the rectangles represents the relative importance of the species in the network (in terms of the number and intensity of its interactions with other species).
In terms of species, the largest number of visit records corresponded, in order of importance, to B. rohweri (Apidae, 33.3%), Bibionidae 1 (Diptera, 23.8%), Bombyliidae (Diptera, 17.83%), Colletidae (Hymenoptera, 9.65%), Oxypogon lindenii (Trochilidae, 3.7%), and Muscidae (Diptera, 3.61), while the rest of morphospecies together contributed to less than 8.1% of the visits (Figure 5). The general interaction network was dominated by B. rohweri and Bibionidae 1 with 11 and 9 connections (grade K), respectively; the remaining pollinators had between 7 and 1 connections. Regarding the plant species, E. schultzii and C. fissifolia were the most connected with seven and five connections each. The richness of plants and pollinators decreased with elevation. In all cases, the pollinator sampling was within the 95% confidence interval of the Chao 2 richness index (10–20 in PI2, 9–32 in PB4, and 7–15 in MO6), suggesting that the pollinator sampling was nearly complete (Table 2).
We registered 17.75% of all possible interactions. The interaction network showed a high and significant degree of specialization (H2), a high value of connectance compared to other systems, and a low value of nestedness, both at a general level and when analyzed separately in each summit (Table 2; Jordano et al., 2009). The two lowest summits (PI2 and PB4) had very similar plant and pollinator richness values and similar values for the degree of pollinators and plants. The two lowest summits also showed similar values of connectance, specialization, and nestedness. At the highest summit (MO6), species richness was lower for plants and pollinators, and the value for all the indices decreased, although this network was still highly specialized. The null model, which assumed the same probability of interaction between all plants and pollinators, presented the lowest AIC, compared with models in which interaction structure was related with plant phenology, plant species distribution between summits, or the sampling effort for each species of plant based on both camera recordings and direct observations (Table 3).
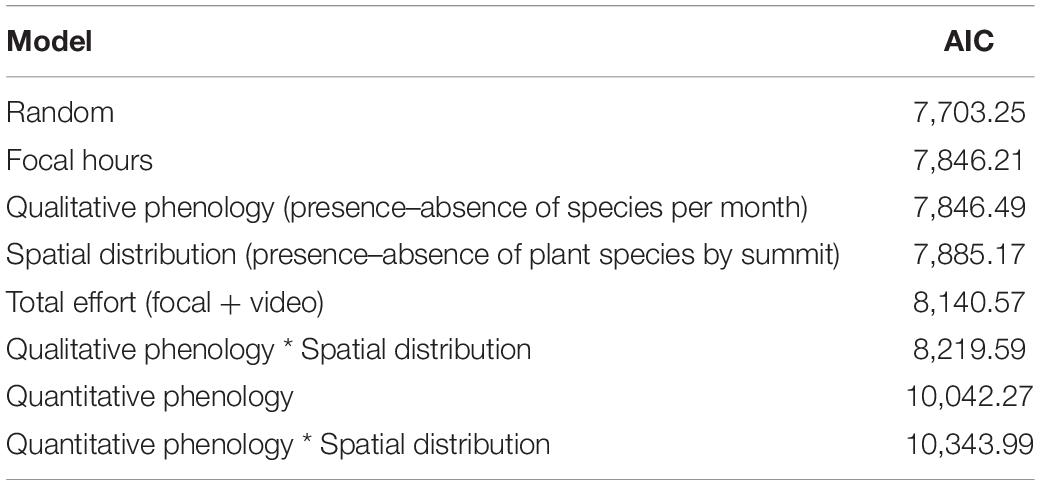
Table 3. Processes related to the network structure through Akaike’s information criterion (AIC) models; they are ordered from smallest to largest.
In the three summits, a marked seasonality in the total number of visits of pollinators was observed. In the lowest summit (PI2), visits concentrated between October and May, with a peak in the total number of visits in November. At the intermediate summit (PB4), visits occur between July and December, with a peak in the last 3 months. Finally, in the highest summit (MO6), visits occur from December to April, with a marked peak in December (Figure 6). The highest visitation frequencies seemed to coincide with the months when there were more plant individuals and plant species with flowers. However, the correlation (Spearman rank) between the monthly pollinator visits and the number of plants with flowers ranged between 0.10 and 0.51 for our three summits, while for the species richness of plants with flowers, the correlation ranged between 0.18 and 0.64 (with p > 0.05 in all cases, except for this last one in PB4; see details in Supplementary Table 1).
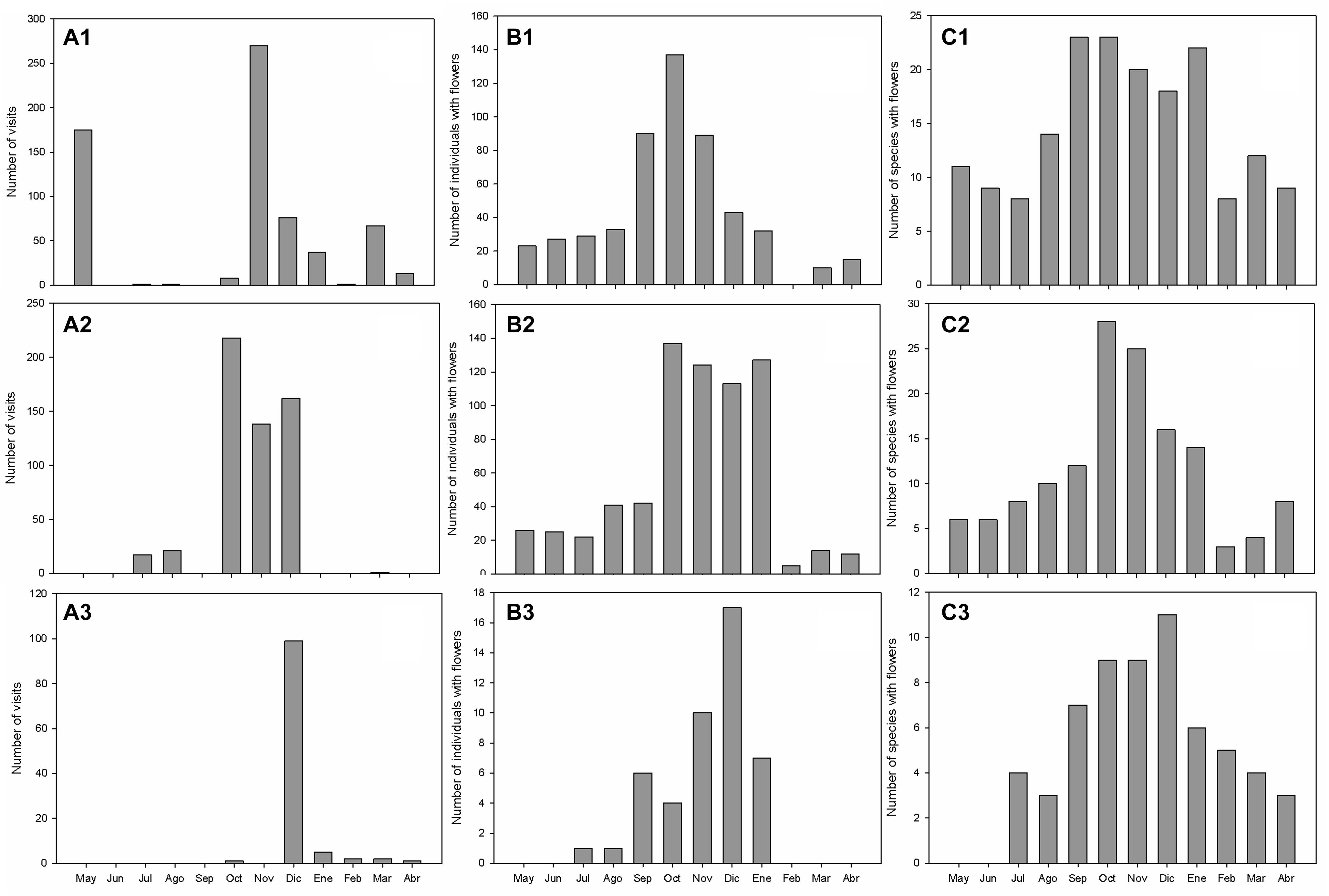
Figure 6. Dynamics of the total number of visits of pollinators (A1 = PI2, A2 = PB4, and A3 = MO6), total number of individuals with flowers in all the 3 × 3 quadrants (B1 = PI2, B2 = PB4, and B3 = MO6), and number of species with flowers (C1 = PI2, C2 = PB4, C3 = MO6) in summits of the GLORIA-Andes network (VE-CPB site), at La Culata National Park, Piedras Blancas Páramo, Venezuela.
Discussion
Our knowledge of the effects of global warming on mutualistic interactions is still limited (Visser and Both, 2005; Walther et al., 2005) and few empirical studies exist (Hegland et al., 2009). Long-term studies that encompass the temporal variability characteristic of alpine areas, and studies that provide a baseline for future comparison should provide particularly valuable contributions, particularly on tropical alpine systems, which comprise about 10% of global alpine areas but remain particularly understudied (Inouye, 2020).
The behavior of flowering phenology in the superpáramo summits studied was similar to the only previous report available at a whole-community scale in páramos at lower elevations (Pelayo et al., 2019), while it also agrees with the behavior of other species analyzed individually in Colombia and Venezuela (Berry and Calvo, 1994; Velez et al., 1998; Gutiérrez-Zamora et al., 2004; Fagua and Bonilla, 2005). Along the elevation gradient studied, the species with continuous flowering and with the highest number of individuals with flowers coincide with the species of non-graminoid plants that show the highest general abundance in these summits (Cuesta et al., 2017).
Common species in our study region with continuous flowering, such as C. fissifolia, D. pulvinata, and H. laricifolium, could be important to the maintenance of the assemblage of pollinators. These species have very wide altitudinal distributions in the páramos (Briceño and Morillo, 2002) and are considered as key elements in the ecology of these ecosystems. C. fissifolia is a hemiparasite (Steyermark, 1957) that provides floral resources for endemic pollinators throughout the year both in superpáramo and in páramo ecosystems (Pelayo et al., 2019, 2020). Moreover, H. laricifolium, which is an important ecosystem engineer in the superpáramo (with a positive effect on microhabitat conditions and the richness/abundance of other plants), showed high connectance values with pollinators (Cáceres et al., 2014; Ramírez et al., 2015; Manrique et al., 2019). D. pulvinata is an endemic species, pollinated by few species including O. lindenii, an endemic hummingbird from Mérida. Hence, it would be important to analyze in more detail the interdependence between this endemic pair of plant and pollinator species, both of which act as high elevation specialists that could be particularly vulnerable in climate change scenarios (Cuesta et al., 2020). Finally, E. schultzii, the more connected species in the network, is endemic from Venezuelan páramos and one of the more abundant and widely distributed species in the Cordillera de Mérida (Briceño and Morillo, 2002).
As indicated by our first prediction, the phenological behavior of the plant community seemed to be influenced by the marked rainfall seasonality of the Venezuelan páramo, with a decrease in the number of species flowering toward the end of the dry season and beginning of the wet season (February–May). However, there was a low value of the phenological overlap index across species in the three summits, which could be interpreted as a mechanism to minimize competition between plant species in conditions of low availability of pollinators and low visitation frequencies (Waser, 1983). In addition, as suggested by our first prediction, the index seemed to increase with elevation, suggesting that flowering could become more synchronic as conditions become harshest. However, further studies of inter-annual variability in plant phenology and its relationship with climatic variability would be required to evaluate the generality of these initial conclusions. In addition, it could be important to increase the sampling frequency within the year to be able to better estimate the phenological overlap index (although we did record flowering in 87.3% of the species with flowers we know to be present in these summits).
Interestingly, total pollinator visitation frequency showed a marked seasonality (generally concentrating between October and December), which seemed to coincide with the months with the maximum richness or the total number of plants flowering in each of the summits (prediction 2). However, we observed no significant correlations between these two variables and visitation frequencies. This could be partly linked with the fact that flowering is much more widely spread during the year than pollinator visitation frequencies (see Figure 6), which is reflected in the low phenological overlap index observed.
The decline in pollinator richness and visitation frequencies with increasing elevation has also been reported at lower altitudes within the páramo belt (Pelayo et al., 2019) and at high elevations in extra-tropical regions (e.g., Arroyo et al., 1982; Totland, 2001). Additionally, the total density of plants with flowers decreased with elevation, although we found patches with relatively high density in some of the orientations, S and E in the lowest summit and N and W in the two highest summits. Additionally, an analysis of total plant cover in the three summits (data unpublished) reveals that the West and North orientation (generally more humid) always show the highest total cover when compared with South and East orientations (facing in intermountain dry enclave). Consequently, these differences could be due to microclimatic conditions associated with environmental heterogeneity, as has been described in other alpine ecosystems (Scherrer and Körner, 2011; Young et al., 2016). Differences in rock cover and the presence of rocky outcrops (i.e., act as thermal refuges, Rada et al., 2009), differential incidence of wind (which tends to be stronger in East and South faces, especially in the two highest summits), and the varying influence of humid vs. dry slope orientations could all contribute to generate these complex and patchy distribution of plants and flowers among the different summit orientations (with a possibly stronger influence of rock cover in the lower summit where there is less climatic contrast between orientations because of its geographic position inside a more humid watershed).
It should be noted that more than half of the plants with flowers registered in the summits had visits from pollinators, mainly from insects, indicating that, in these superpáramo ecosystems, entomophily is the predominant reproductive mechanism (prediction 3). This has also been documented in another superpáramo community in the Sierra Nevada in Mérida (Manrique et al., 2019). This contrasts with the results of previous studies in the Andean páramo at lower elevations, where pollination by hummingbirds were more important (Pelayo et al., 2019).
As expected in this ecosystem (e.g., Cuesta et al., 2017), we observed a decrease in both plants and pollinators’ richness along the elevation gradient. Moreover, there was a change in the composition and relative importance of both plants and animals (prediction 3). The increase with elevation in the relative importance of Diptera as pollinators observed here has also been reported in other studies along elevation gradients in tropical and temperate alpine regions (Arroyo et al., 1982; Manrique et al., 2019; Inouye, 2020). Interestingly, high elevation specialist plants such as D. chionophila showed a strong dependence on these flies, many of which we have only recorded in the two highest summits. However, hummingbirds and bumblebees with high metabolic rates and energy demands remained as important pollinators species, albeit they showed higher visitation frequencies and relative importance in the two lowest summits. In the case of the high elevation specialist hummingbirds O. lindenii, we have seen them foraging in these summits even during snowfalls, demonstrating their remarkable ability to adapt to these extreme environments (Figure 7).
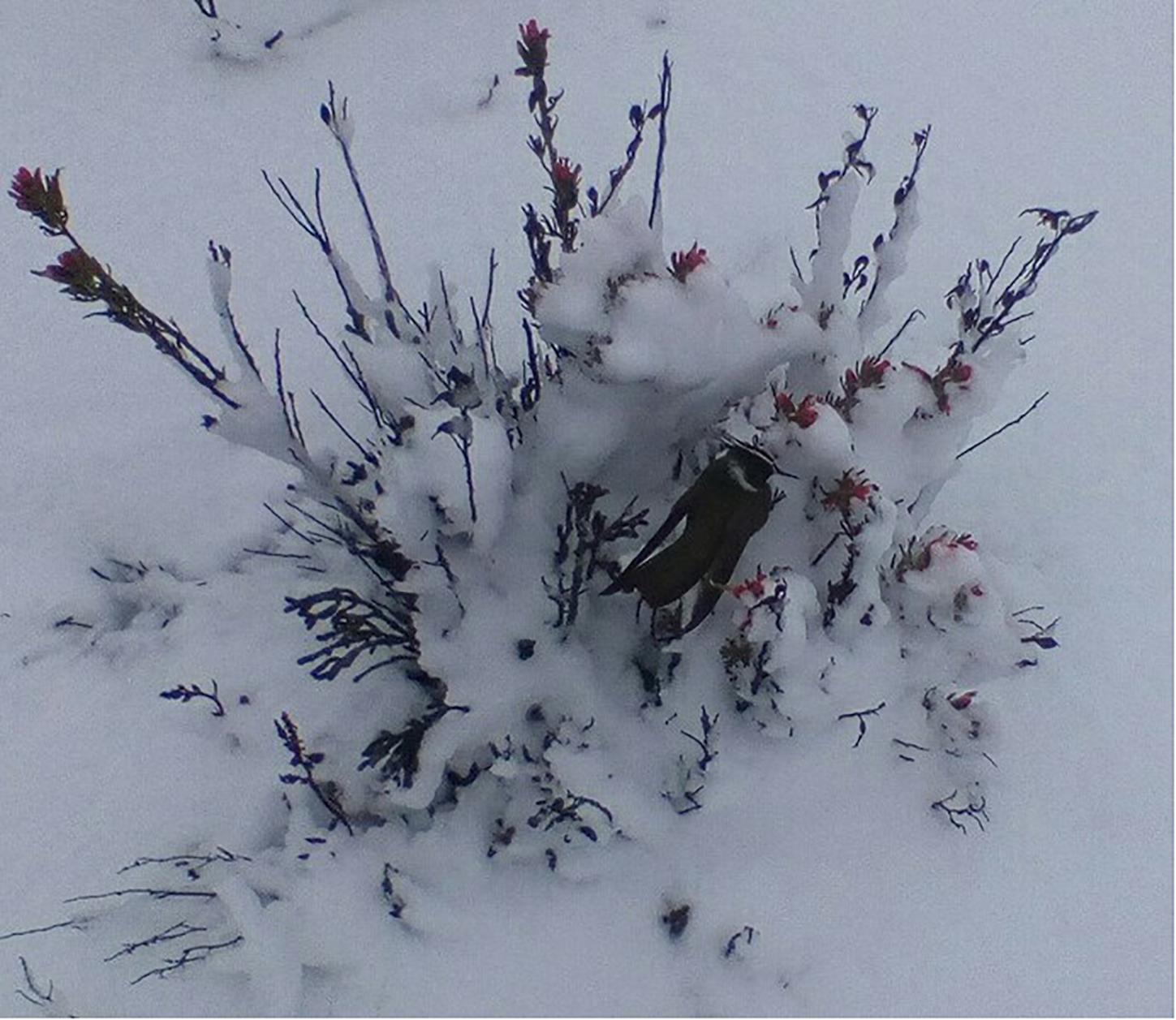
Figure 7. Oxypogon lindenii (Trochilidae) visiting flowers of Castilleja fissifolia (Orobanchaceae) during a snowfall event at 4,400 m in the Venezuelan superpáramo.
Our networks exhibited values of connectance similar to those found for alpine and temperate pollination networks (Olesen and Jordano, 2002; Santamaría et al., 2014) and a low nestedness, as has been reported in other páramos (prediction 4, Manrique et al., 2019; Pelayo et al., 2019). However, the high elevation networks studied here could be more vulnerable, as they showed the lower richness of plants and pollinators and higher values of specialization (prediction 4); they are also more exposed to fast warming and exhibit smaller populations (with less habitat in terms of area and climatic suitability, see Cuesta et al., 2020). Both a low nestedness and a high degree of specialization agree with what is expected for networks with a low richness of plant and animals (Bascompte et al., 2003).
In the superpáramo, self-reproducing and apomictic plants (see Berry, 1986; Samaniego et al., 2018) coexist with others with specialist pollination systems such as D. chionophila (which were visited by few species of flies) and with some generalists’ species such as E. schultzii and S. wedglacialis, which were visited by a wider range of insects and birds. However, these generalist species showed in our superpáramo summits a less rich pollinator assembly than previously reported in other páramos (Pelayo et al., 2015, 2019; Manrique et al., 2019). Hence, at higher elevations, even the more generalist plant species show a relatively poor assembly of pollinators (i.e., low functional redundancy for pollinators).
We found no relationship between plant phenological responses and the overall network structure in the studied summits (prediction 5). This could be partially due to the small size of these networks, which complicates the construction of robust models and detection of significant departures from randomness (see Dormann et al., 2014), preventing us for carrying out separate analysis of these relationships for each summit. In addition, it would be important to explore other plant and fauna attributes that could help to explain network structure, such as floral morphology, quality of rewards, phylogenetic relationships, or the patterns of change in the abundance pollinators (Fort et al., 2016; Manrique et al., 2019).
Even though the number of observed pollinators in the three studied summits was within the estimated values based on the Chao 2 estimator, the generally low visitation frequencies observed in the high páramos suggest that a higher sampling effort each month would be important to obtain a more exhaustive characterization of all the plant–pollinator interactions that could occur. However, given the remoteness of many sampling summits within the context of the GLORIA–Andes network, sampling effort could face important limitations for long-term monitoring purposes that need to be kept in mind.
Plant–pollinators systems with a high degree of specialization and with low functional redundancy like the ones studied here have been considered to be more sensitive to climate change (Gilman et al., 2010; Benadi et al., 2014; Miller-Struttmann et al., 2015; Valiente-Banuet et al., 2015). Moreover, comparative research across the GLORIA–Andes summits in South America has shown that the high elevation summits in the Northern páramo exhibit particularly high levels of endemism and are dominated by species with narrow thermal niches that are more prone to loss of niche space in future warming conditions (Cuesta et al., 2020). Together, all of these observations indicate that vegetation in superpáramo areas and their plant-pollination networks could be particularly vulnerable to species and functional diversity losses driven by climate change.
Longer-term monitoring of multiple pressures on pollinators and pollinator-dependent plants will help elucidate the mechanisms underpinning observed vegetation shifts, and inform the design and assessment of mitigation and adaptation options (Settele et al., 2016). Our preliminary analyses of plant community dynamics between the establishment of the baseline (2012) and the first resampling (2017) in the permanent plots of the GLORIA–Andes network showed an increase in species richness due to new arrivals of small herbs from the lower elevations, in agreement with reports by Carilla et al. (2017) in the northern Argentinian Andes. All of these new species were involved in our plant and pollinator networks (e.g., C. uliginosa var uliginosa, Oxylobus glanduliferus, Senecio funckii, and Luciliocline longifolia), showing in some cases very high visitation frequencies (e.g., S. funckii). These preliminary observations reinforce the idea that the GLORIA–Andes summits can function as an excellent natural laboratory for evaluating the long-term effects of climate change on interactions between plants and pollinators. However, a more detailed analysis of these patterns is required.
Conclusion
Most of the flowering plant species (excluding graminoids) recorded in the three summits received visits from insects and, to a lesser extent, from hummingbirds. These plants showed little synchrony in flowering among species, but synchrony increased with elevation. Flies and bumblebees were the most important pollinators in areas above 4,200 m asl with flies increasing in relative importance in the highest summit. The interaction networks showed a high and significant specialization (H2) and connectance but a low degree of nestedness. The baseline information collected here in the context of the GLORIA–Andes monitoring program should provide key information to evaluate changes in the reproductive behavior of plants, which could be more sensitive than species composition and abundance to climate change impacts. Moreover, this information could help understand the differential response of plant species in the High tropical Andes to climatic drivers along elevation gradients, proving a more process-oriented understanding of the climatic risks faced by these unique high elevation plant communities.
Data Availability Statement
The original contributions presented in the study are included in the article/Supplementary Material.
Author Contributions
RP and LL conceived, designed the study, and wrote the first draft of the manuscript. RP, LL, JT, and LG collected field data. LG carried out all taxonomic identifications. LR manages the GLORIA-Venezuela database. RP, LL, and YB performed statistical analyses. All authors contributed to the discussion and interpretation of data, revised and complemented the manuscript, and approved the submitted version.
Funding
This work was partially funded by the Adaptation at Altitude and Andean Forest programs under CONDESAN coordination and with the financial support of the Swiss Agency for Development and Cooperation (SDC).
Conflict of Interest
The authors declare that the research was conducted in the absence of any commercial or financial relationships that could be construed as a potential conflict of interest.
Publisher’s Note
All claims expressed in this article are solely those of the authors and do not necessarily represent those of their affiliated organizations, or those of the publisher, the editors and the reviewers. Any product that may be evaluated in this article, or claim that may be made by its manufacturer, is not guaranteed or endorsed by the publisher.
Acknowledgments
We thank Manuel Fernández, Carmen Azócar, and Samantha Ruiz for assistance during field work and Nestor Sánchez for the taxonomic identification of insect species.
Supplementary Material
The Supplementary Material for this article can be found online at: https://www.frontiersin.org/articles/10.3389/fevo.2021.679045/full#supplementary-material
References
Aguirre, L. F., Anderson, E. P., Brehm, G., Herzog, S. K., Jørgensen, P. M., Kattan, G. H., et al. (2011). “Phenology and interspecific ecological interactions of Andean biota in the face of climate change,” in Climate Change and Biodiversity in the Tropical Andes, eds S. K. Herzog, R. Martínez, P. M. Jørgensen, and H. Tiessen (Paris: Scientific Committee on Problems of the Environment), 68–92.
Almeida-Neto, M., and Ulrich, W. (2011). A straightforward computational approach for measuringnestedness using quantitative matrices. Environ. Model. Softw. 26, 173–178. doi: 10.1016/j.envsoft.2010.08.003
Anderson, J. T., Inouye, D. W., McKinney, A. M., Colautti, R. I., and Mitchell, O. T. (2012). Phenotypic plasticity and adaptive evolution contribute to advancing flowering phenology in response to climate change. Proc. Biol. Sci. 279, 3843–3852. doi: 10.1098/rspb.2012.1051
Anthelme, F., Cavieres, L. A., and Dangles, O. (2014). Facilitation among plants in alpine environments in the face of climate change. Front. Plant Sci. 5:387. doi: 10.3389/fpls.2014.00387
Arroyo, M. T., Armesto, T., and Primack, R. (1982). Tendencias altitudinales en mecanismos de polinización en la zona andina de los Andes templados de Sudamérica. Rev. Chil. Hist. Nat. 56, 159–180.
Bartomeus, I., Ascher, J. S., Wagner, D., Danforth, D. N., Colla, S., Kornbluth, S., et al. (2011). Climate-associated phenological dvances in bee pollinators and bee-pollinated plants. Proc. Natl. Acad. Sci. U.S.A. 108, 20645–20649. doi: 10.1073/pnas.1115559108
Bartomeus, I., Park, M. G., Gibbs, J., Danforth, B. N., Lakso, A. N., and Winfree, R. (2013). Biodiversity ensures plant–pollinator phenological synchrony against climate change. Ecol. Lett. 16, 1331–1338. doi: 10.1111/ele.12170
Bascompte, J., Jordano, P., Melián, C. J., and Olesen, J. M. (2003). The nested assembly of plant-animal mutualistic networks. Proc. Natl. Acad. Sci. U.S.A. 100, 9383–9387. doi: 10.1073/pnas.1633576100
Bascompte, J., Jordano, P., and Olesen, J. M. (2006). Asymmetric coevolutionary networks facilitate biodiversity maintenance. Science 312, 431–433. doi: 10.1126/science.1123412
Benadi, G., Hovestadt, T., Poethke, H. C., and Blüthgen, N. (2014). Specialization and phenological synchrony of plant–pollinator interactions along an altitudinal gradient. J. Anim. Ecol. 83, 639–650. doi: 10.1111/1365-2656.12158
Berry, P. E. (1986). Los Sistemas Reproductivos y Mecanismos de Polinización del Género Espeletia en los Páramos Venezolanos. Anales del IV Congreso Latinoamericano de Botánica, vol. II. Simposio Ecología de la Reproducción e Interacciones Planta/Animal, Medellin, 25–33.
Berry, P. E., and Calvo, R. N. (1994). “An overview of the reproductive biology of Espeletia (Asteraceae) in the venezuelan andes,” in Tropical Alpine Environments: Plant Form and Function, eds P. W. Rundel and R. Meinzer (Cambridge: Cambridge University Press), 229–249. doi: 10.1017/cbo9780511551475.014
Blüthgen, N., Menzel, F., and Blüthgen, N. (2006). Measuring specialization in species interaction networks. BMC Ecol. 6:9. doi: 10.1186/1472-6785-6-9
Blüthgen, N., Menzel, F., Hovestadt, T., Fiala, B., and Blüthgen, N. (2007). Specialization, constraints, and conflicting interests in mutualistic networks. Curr. Biol. 17, 341–346. doi: 10.1016/j.cub.2006.12.039
Briceño, B., and Morillo, G. (2002). Catálogo abreviado de las plantas con flores de los páramos de Venezuela. Parte I. dicotiledóneas (Magnoliopsida). Acta Bot. Venez. 25, 1–46.
Burgos, E., Ceva, H., Perazzo, R. P. J., Devoto, M., Medan, D., Zimmermann, M., et al. (2007). Why nestedness in mutualistic networks? J. Theor. Biol. 249, 307–313. doi: 10.1016/j.jtbi.2007.07.030
Burnham, K. P., and Anderson, D. R. (2002). A Practical Information-Theoretic Approach. Model Selection and Multimodel Inference, 2nd Edn. New York, NY: Springer.
Cáceres, Y., Llambí, L. D., and Rada, F. (2014). Shrubs as foundation species in a high tropical alpine ecosystem: a multi-scale analysis of plant spatial interactions. Plant Ecol. Divers. 8, 147–161. doi: 10.1080/17550874.2014.960173
Carilla, J., Halloy, S., Cuello, S., Grau, A., Malizia, A., and Cuesta, F. (2017). Vegetation trends over eleven years on mountain summits in NW Argentina. Ecol. Evol. 8, 11554–11567. doi: 10.1002/ece3.4602
Chapman, C. A., Chapman, L. J., Struhsaker, T. T., Zanne, A. E., Clark, C. J., and Poulsen, J. R. (2005). A long-term evaluation of fruiting phenology: importance of climate change. J. Trop. Ecol. 21, 31–45. doi: 10.1017/s0266467404001993
Colwell, R. K. (2013). EstimateS, Version 9.1: Statistical Estimation of Species Richness and Shared Species from Samples. User’s Guide and Application. Available online at: http://viceroy.eeb.uconn.edu/estimates/ (accessed November 11, 2018).
Cuesta, F., Muriel, P., Llambí, L. D., Halloy, S., Aguirre, N., Beck, S., et al. (2017). Latitudinal and altitudinal patterns of plant community diversity on mountain summits across the tropical Andes. Ecography 40, 1381–1394. doi: 10.1111/ecog.02567
Cuesta, F., Tovar, C., Llambí, L. D., and Pauli, H. (2020). Thermal niche traits of high alpine plant species and communities across the tropical Andes and their vulnerability to global warming. J. Biogeogr. 47, 408–420. doi: 10.1111/jbi.13759
Devoto, M., Bailey, S., Craze, P., and Memmott, J. (2012). Understanding and planning ecological restoration of plant–pollinator networks. Ecol. Lett. 15, 319–328. doi: 10.1111/j.1461-0248.2012.01740.x
Dormann, C. F., and Strauss, R. (2014). A method for detecting modules in quantitative bipartite networks. Methods Ecol. Evol. 5, 90–98. doi: 10.1111/2041-210X.12139
Dormann, C. F., Fruend, J., Gruber, B., and Dormann, M. C. F. (2014). Package ‘Bipartite’. Visualizing Bipartite Networks and Calculating Some Ecological Indices (Version 2.04). Vienna: R Foundation for Statistical Computing.
Dormann, C. F., Gruber, B., and Fründ, J. (2008). Introducing the bipartite package: analysing ecological networks. R News 8, 8–11.
Dupont, Y. L., Hansen, D. M., and Olesen, J. M. (2003). Structure of a plant–flower-visitor network in the high-altitude sub-alpine desert of Tenerife, Canary Islands. Ecography 26, 301–310. doi: 10.1034/j.1600-0587.2003.03443.x
Faegri, K., and van der Pijl, L. (1979). The Principles of Pollination Ecology. New York, NY: Pergamon Press Ltd.
Fagua, J. C., and Bonilla, M. A. (2005). “Ecología de la polinización de Espeletia grandiflora,” in Estrategias Adaptativas de Plantas del Páramo y del Bosque alto Andino en la Cordillera Oriental de Colombia, ed. M. A. Bonilla (Bogotá: Universidad Nacional de Colombia), 246–271.
Forrest, J. R. K. (2014). Plant – pollinator interactions and phenological change: what can we learn about climate impacts from experiments and observations? Oikos 124, 4–13. doi: 10.1111/oik.01386
Fort, H., Vázquez, D. P., and Lan, B. L. (2016). Abundance and generalisation in mutualistic networks: solving the chicken-and-egg dilemma. Ecol. Lett. 19, 4–11. doi: 10.1111/ele.12535
Gámez, L. E., Llambí, L. D., Ramírez, L., Pelayo, R. C., Torres, J. E., Márquez, N., et al. (2020). Contribución al conocimiento de la vegetación altoandina: riqueza florística y clave para la identificación de plantas vasculares en cumbres de monitoreo de la red GLORIA-Andes en Venezuela. Pittieria 44, 76–103.
Gilman, S. E., Urban, M. C., Tewksbury, J., Gilchrist, G. W., and Holt, R. D. (2010). A framework for community interactions under climate change. Trends Ecol. Evol. 25, 325–331. doi: 10.1016/j.tree.2010.03.002
González, O., and Loiselle, B. A. (2016). Species interactions in an Andean bird flowering plant network: phenology is more important than abundance or morphology. PeerJ. 4:e2789. doi: 10.7717/peerj.2789
Gutiérrez-Zamora, A., Rojas-Nossa, S., and Stiles, G. (2004). Dinámica poblacional de la interacción colibrí-flor en ecosistemas altoandinos. Ornitol. Neotrop. 15, 205–213.
Harrison, R. D. (2000). Repercussions of El Niño: drought causes extinction and the breakdown of mutualism in Borneo. Proc. Biol. Sci. 267, 911–915.
Hegland, S. J., Nielsen, A., Lázaro, A., Bjerknes, A. L., and Totland, Ø (2009). How does climate warming affect plant-pollinator interactions? Ecol. Lett 12, 184–195. doi: 10.1111/j.1461-0248.2008.01269.x
Inouye, D. W. (2008). Effects of climate change on phenology, frost damage, and floral abundance of montane wildflowers. Ecology 89, 353–362. doi: 10.1890/06-2128.1
Inouye, D. W. (2020). Effects of climate change on alpine plants and their pollinators. Ann. N. Y. Acad. Sci. 1469, 26–37. doi: 10.1111/nyas.14104
Jordano, P. (1987). Patterns of mutualistic interactions in pollination and seed dispersal: connectance, dependence asymmetries, and coevolution. Am. Nat. 129, 657–677. doi: 10.1086/284665
Jordano, P., Vázquez, D., and Bascompte, J. (2009). Redes Complejas de Interacciones Mutualistas Planta-Animal. Ecología y Evolución de las Interacciones Planta-Animal: Conceptos y Aplicaciones. Santiago: Editorial Universitaria, 17–41.
Josse, C., Cuesta, F., Navarro, G., Barrena, V., Becerra, M. T., Cabrera, E., et al. (2011). “Physical geography and ecosystem in the tropical Andes,” in Climate Change and Biodiversity in the Tropical Andes, eds S. K. Herzog, R. Martínez, P. M. Jørgensen, and H. Tiessen (Brasilia: Scientific Committee on Problems of the Environment), 152–169.
Lamprecht, A., Pauli, H., Calzado, M. R. F., Lorite, J., Mesa, J. M., Steinbauer, K., et al. (2021). Changes in plant diversity in a water-limited and isolated high-mountain range (Sierra Nevada, Spain). Alp. Bot. 1311, 27–39. doi: 10.1007/s00035-021-00246-x
Lenoir, J., and Svenning, J. C. (2015). Climate-related range shifts: a global multidimensional synthesis and new research directions. Ecography 38, 15–28. doi: 10.1111/ecog.00967
Llambí, L. D., and Rada, F. (2019). Ecological research in the tropical alpine ecosystems of the Venezuelan páramo: past, present and future. Plant Ecol. Divers. 12, 519–538. doi: 10.1080/17550874.2019.1680762
Manrique, O., Barrios, Y., Sánchez, N., and Grande, J. (2019). “Red de interacciones planta-visitante floral en una comunidad de páramo altiandino (Parque Nacional Sierra Nevada, Mérida, Venezuela),” in Proceedings of the Latin American Conference 2.0 on Complex Networks; Aug 5-9. (Cartagena).
Medan, D., Montaldo, N. H., Devoto, M., Maniese, A., Vasellati, V., Roitman, G. G., et al. (2002). Plant-pollinator relationships at two altitudes in the Andes of Mendoza, Argentina. Arct. Antarct. Alp. Res. 34, 233–241. doi: 10.2307/1552480
Memmott, J., Craze, P. G., Waser, N. M., and Price, M. V. (2007). Global warming and the disruption of plant–pollinator interactions. Ecol. Lett 10, 710–717. doi: 10.1111/j.1461-0248.2007.01061.x
Miller-Struttmann, N. E., Geib, J. C., Franklin, J. D., Kevan, P. G., Holdo, R. M., Ebert-May, D., et al. (2015). Functional mismatch in a bumble bee pollination mutualism under climate change. Science 349, 1541–1544. doi: 10.1126/science.aab0868
Monasterio, M. (1980a). “Las formaciones vegetales de los páramos de Venezuela,” in Estudios Ecológicos en los Páramos, eds M. Andinos and M. Monasterio (Mérida: Editorial de la Universidad de Los Andes), 15–27.
Monasterio, M. (1980b). “Los páramos andinos como región natural. características biogeográficas generales y afinidad con otras regiones andinas,” in Estudios Ecológicos en los Páramos, eds M. Andinos and M. Monasterio (Mérida: Editorial de la Universidad de Los Andes), 47–92.
Monasterio, M. (1986). “Adaptive strategies of Espeletia in the Andeandesert paramo,” in High Altitude Tropical Biogeography, eds F. Vuilleumierand and M. Monasterio (New York, NY: Oxford University Press).
Olesen, J. M., and Jordano, P. (2002). Geographic patterns in plant–pollinator mutualistic networks. Ecology 83, 2416–2424. doi: 10.2307/3071803
Pauli, H., Gottfried, M., Dullinger, S., Abdaladze, O., Akhalkatsi, M., Alonso, J. L. B., et al. (2012). Recent plant diversity changes on europe’s mountain summits. Science 336, 353–355.
Pauli, H., Gottfried, M., Lamprecht, A., Niessner, S., Rumpf, S. B., Winkler, M., et al. (2015). The GLORIA Field Manual – Standard Multi-Summit Approach, Supplementary Methods and Extra Approaches. Vienna: GLORIA-Coordination.
Pelayo, R. C. (2017). Implicaciones Ecológicas y Evolutivas del Robo de Néctar en Ecosistemas Neotropicales Venezolanos. Ph. D. Thesis. España: Universidad de Vigo.
Pelayo, R. C., Márquez, N. J., Soriano, P. J., and Navarro, L. (2019). Phenological patterns and pollination network structure in a Venezuelan páramo: a community-scale perspective on plant-animal interactions. Plant Ecol. Divers. 12, 607–618. doi: 10.1080/17550874.2019.1675096
Pelayo, R. C., Rengifo, C., Ayala, M., Torres, J. E., and Torres, Y. (2020). Información adicional sobre la nidifi cación del Chivito de los Páramos Oxypogon lindenii en el norte de la Cordillera de Mérida, Venezuela. Rev. Venez. Ornitol. 10, 47–51.
Pelayo, R. C., Sánchez-Guillén, N., Sánchez, C. F., Jaimes, J. C., Ramírez, M., Villalba, E., et al. (2015). Cambios en la estructura de ensambles de visitantes florales en Espeletia schultzii Wedd y Senecio wedglacialis Cuatrec (Asteraceae) ante modificaciones artificiales de algunos caracteres florales. Ecotropicos 28, 14–26.
Primack, R. B. (1985). Patterns of Flowering Phenology in Communities, Populations, Individuals, and Single Flowers. In Population Structure of Vegetation. Berlin: Springer, 571–593.
Rada, F., García-Núñez, C., and Rangel, S. (2009). Low temperature resistance in saplings and ramets of Polylepis sericea in the Venezuelan Andes. Acta Oecol. 35, 610–613. doi: 10.1016/j.actao.2009.05.009
Ramírez, L. A., Rada, F., and Llambí, L. D. (2015). Linking patterns and processes through ecosystem engineering: effects of shrubs on microhabitat and water status of associated plants in the high tropical Andes. Plant Ecol. 216, 213–225. doi: 10.1007/s11258-014-0429-5
Ramirez, N., and Brito, Y. (1992). Pollination biology in a palm swamp community in the Venezuelan central plains. Bot. J. Linnaean Soc. 110, 277–302. doi: 10.1111/j.1095-8339.1992.tb00294.x
Ramos-Jiliberto, R., Domínguez, D., Espinoza, C., López, G., Valdovinos, F. S., Bustamante, R. O., et al. (2010). Topological change of Andean plant-pollinator networks along an altitudinal gradient. Ecol. Complex 7, 86–90. doi: 10.1016/j.ecocom.2009.06.001
R Core Team (2016). R Development Core Team [Internet], Vol. 55. Vienna: R: A Language and Environment for Statistical Computing, 2016.
Samaniego, F., Kolár, F., Urfus, T., Barragán, Á., and Romoleroux, K. (2018). Determination of apomixis by flow cytometry in two species of Lachemilla (Rosaceae) in Ecuador. Neotrop. Biodivers 4, 152–163. doi: 10.1080/23766808.2018.1542785
Santamaría, S., Galeano, J., Pastor, J. M., and Méndez, M. (2014). Robustness of alpine pollination networks: effects of network structure and consequences for endemic plants. Arct. Antarct. Alp. Res. 46, 568–580. doi: 10.1657/1938-4246-46.3.568
Sarmiento, G. (1986). “Ecological features of climate in high tropical mountains,” in High Altitude Tropical Biogeography, eds F. Vuilleumier and M. Monasterio (Oxford: Oxford University Press), 11–45.
Scherrer, D., and Körner, C. (2011). Topographically controlled thermal-habitat differentiation buffers alpine plant diversity against climate warming. J. Biogeogr. 38, 406–416. doi: 10.1111/j.1365-2699.2010.02407.x
Settele, J., Bishop, J., and Potts, S. G. (2016). Climate change impacts on pollination. Nat. Plants 2:16092. doi: 10.1038/NPLANTS.2016.92
Steinbauer, K., Lamprecht, A., Semenchuk, P., Winkler, M., and Pauli, H. (2019). Dieback and expansions: species-specific responses during 20 years of amplified warming in the high Alps. Alp. Bot. 130, 1–11. doi: 10.1007/s00035-019-00230-6
Totland, Ø (2001). Environment-dependent pollen limitation and selection on floral traits in an alpine species. Ecology 82, 2233–2244. doi: 10.1890/0012-9658(2001)082[2233:edplas]2.0.co;2
Tovar, C., Melcher, I., Kusumoto, B., Cuesta, F., Cleef, A., Meneses, R. I., et al. (2020). Plant dispersal strategies of high tropical alpine communities across the Andes. J. Ecol. 108, 1910–1922. doi: 10.1111/1365-2745.13416
Trøjelsgaard, K., and Olesen, J. M. (2013). Macroecology of pollination networks. Glob. Ecol. Biogeogr. 22, 149–162. doi: 10.1111/j.1466-8238.2012.00777.x
Tylianakis, J. M., Tscharntke, T., and Lewis, O. T. (2007). Habitat modification alters the structure of tropical host-parasitoid food webs. Nature 445, 202–205. doi: 10.1038/nature05429
Vázquez, D. (2010). Miscellaneous Functions for Analysis of Ecological Interaction Networks. Package Ecolnet v. 0, 1–4.
Valiente-Banuet, A., Aizen, M. A., Alcántara, J. M., Arroyo, J., Cocucci, A., Galetti, M., et al. (2015). Beyond species loss: the extinction of ecological interactions in a changing world. Funct. Ecol. 29, 299–307. doi: 10.1111/1365-2435.12356
Vázquez, D. P., Chacoff, N. P., and Cagnolo, L. (2009). Evaluating multiple determinants of the structure of mutualistic networks. Ecology 90, 2039–2046. doi: 10.1890/08-1837.1
Velez, V., Cavelier, J., and Devia, B. (1998). Ecological traits of the tropical treeline species Polylepis quadrijuga (Rosaceae) in the Andes of Colombia. J. Trop. Ecol. 14, 771–787. doi: 10.1017/s026646749800056x
Visser, M. E., and Both, C. (2005). Shifts in phenology due to global climate change: the need for a yardstick. Proc. R. Soc. B Biol. Sci. 272, 2561–2569. doi: 10.1098/rspb.2005.3356
Walther, G. R., Berger, S., and Sykes, M. T. (2005). An ecological ‘footprint’of climate change. Proc. R. Soc. B Biol. Sci. 272, 1427–1432.
Waser, N. M. (1983). The adaptive nature of floral traits: ideas and evidence. Pollin Biol. 1, 241–285. doi: 10.1016/b978-0-12-583980-8.50017-x
Keywords: plant reproduction, alpine ecosystems, GLORIA, animal-plant interactions, vegetation dynamics
Citation: Pelayo RC, Llambí LD, Gámez LE, Barrios YC, Ramirez LA, Torres JE and Cuesta F (2021) Plant Phenology Dynamics and Pollination Networks in Summits of the High Tropical Andes: A Baseline for Monitoring Climate Change Impacts. Front. Ecol. Evol. 9:679045. doi: 10.3389/fevo.2021.679045
Received: 10 March 2021; Accepted: 27 July 2021;
Published: 26 August 2021.
Edited by:
Isabel Marques, University of Lisbon, PortugalReviewed by:
Vincent Miele, UMR 5558 Biométrie et Biologie Evolutive (LBBE), FranceBrian Stucky, Florida Museum of Natural History, United States
Copyright © 2021 Pelayo, Llambí, Gámez, Barrios, Ramirez, Torres and Cuesta. This is an open-access article distributed under the terms of the Creative Commons Attribution License (CC BY). The use, distribution or reproduction in other forums is permitted, provided the original author(s) and the copyright owner(s) are credited and that the original publication in this journal is cited, in accordance with accepted academic practice. No use, distribution or reproduction is permitted which does not comply with these terms.
*Correspondence: Roxibell C. Pelayo, cm94aWJlbGxAdWxhLnZl