- 1Department of Integrated Mathematical Oncology, H. Lee Moffitt Cancer Center and Research Institute, Tampa, FL, United States
- 2Department of Cancer Physiology, H. Lee Moffitt Cancer Center and Research Institute, Tampa, FL, United States
Dormancy is an inactive period of an organism’s life cycle that permits it to survive through phases of unfavorable conditions in highly variable environments. Dormancy is not binary. There is a continuum of dormancy phenotypes that represent some degree of reduced metabolic activity (hypometabolism), reduced feeding, and reduced reproduction or proliferation. Similarly, normal cells and cancer cells exhibit a range of states from quiescence to long-term dormancy that permit survival in adverse environmental conditions. In contrast to organismal dormancy, which entails a reduction in metabolism, dormancy in cells (both normal and cancer) is primarily characterized by lack of cell division. “Cancer dormancy” also describes a state characterized by growth stagnation, which could arise from cells that are not necessarily hypometabolic or non-proliferative. This inconsistent terminology leads to confusion and imprecision that impedes progress in interdisciplinary research between ecologists and cancer biologists. In this paper, we draw parallels and contrasts between dormancy in cancer and other ecosystems in nature, and discuss the potential for studies in cancer to provide novel insights into the evolutionary ecology of dormancy.
Introduction
“You keep using that word. I do not think it means what you think it means.” From the character Inigo Montoya, Princess Bride.
Most living organisms, from microbes to blue whales, experience temporal environmental fluctuations. These fluctuations induce periods of stress due to extreme temperatures, lack of resources, or disease. Dormancy, an evolutionary adaptation, enables organisms to survive through stressful conditions, in part by decreasing metabolic activity to conserve energy. However, there is a broad range of dormant states across taxa, which vary based on how long an organism remains in dormancy, whether dormancy is induced prior to or in response to stress, the magnitude of the response, etc. Despite attempts to devise a universal framework for animal dormancy (Wilsterman et al., 2020), precise definitions remain challenging. Dormancy is further complicated by diverging conceptualizations in other fields.
Cancer dormancy often refers to a period of time, from months, years, or even decades, between treatment of a primary tumor and metastatic relapse. This could be due to isolated non-proliferative cells that disseminated from the primary tumor (cancer cell dormancy) or small non-expanding populations of cancer cells (tumor mass dormancy). Although both of these categories could result in clinically undetectable cancer, they describe different biological mechanisms. This creates confusion within the field about what is meant by “cancer dormancy,” and between fields about how cancer dormancy relates to organismal dormancy in other species. Furthermore, additional terminology has been used to describe cancer cells that survive treatment or other cellular stresses such as drug-tolerant persisters, hypoxia-resistant cells, and polyaneuploid cancer cells (PACCs). These descriptors and cell states add to the confusion owing to their overlapping characteristics with dormant cancer cells, such as cellular quiescence. Furthermore, this babel of terms and cell states can stifle the exchange of ideas between cancer biologists and other evolutionary ecologists.
In this paper, we take a critical look at the concept of tumor cell dormancy, in its many guises. Although we are not the first to point out the confusion associated with tumor cell dormancy (Vallette et al., 2019; Phan and Croucher, 2020), we aim to help clarify terminology by comparing it to key characteristics of dormancy in nature. This is not to imply that cancer is not a part of nature. It is. Rather we will use “in nature” as shorthand to describe all natural systems other than cancer. In what follows, we first examine how the term dormancy and related concepts are used in organismal biology. We then examine the history of the terms in cancer biology, with a focus on how those uses compare to what the terms mean in the context of whole organism biology and ecology. Finally, we discuss the mutual benefit of studying dormancy as it applies to ecology and cancer biology, and how experiments in cancer may help provide novel insights into mechanisms that drive dormancy from cells to organisms. Overall, we hope this paper provides a starting point for ecologists to help understand the terminology used in cancer biology and facilitate cross-disciplinary work on dormancy, while simultaneously convincing cancer biologists of the benefits of conceptualizing cancer dormancy using insights from ecology.
Dormancy in Organismal Biology
Dormancy is often used as an “umbrella” term indicating a spectrum of inactive states characterized by reduced metabolism, or hypometabolism, as adaptations to survive periods of reduced resource availability or other adverse environmental conditions. Dormancy also refers to specific states of hypometabolism in both animals and plants (Table 1). The terms “dormancy” and “torpor” are often used interchangeably in the organismal literature. Dormancy encompasses many different hypometabolic states that have evolved across widely divergent taxa. These hypometabolic states exist along a continuum of metabolic expenditure (Figure 1). From the extreme state of essentially zero metabolic expenditure exhibited by bacterial endospores and cysts, dormant states include seed dormancy, estivation, diapause, quiescence, and hibernation. These diverse states are difficult to place into well-defined categories with sharp boundaries, and terminology can be confusing (Schmidt-Nielsen, 1997; Lee, 2009). Some of the characteristics that differentiate these states include (1) whether an organism enters dormancy prior to (obligatory) or in direct response to (facultative) an environmental stress, (2) the duration of the response (3) the reduction in metabolic activity, and (4) how resistant the state is to predation or stress. Dormancy may be of short duration (hours), as in the shallow or daily torpor of hummingbirds, or of long duration (days to weeks to months to years), as in the deep torpor associated with hibernation in mammals or seed dormancy in plants (Melvin and Andrews, 2009). While the manifestation of these states differs considerably among taxa, dormancy shares various core elements across numerous taxa (Melvin and Andrews, 2009; Villanueva-Cañas et al., 2014). Shared elements include an integrated down-regulation of cell functions, including cross-membrane transport, intermediary metabolism (biochemical reactions that provide the cell with metabolic intermediates), gene expression and protein synthesis, and utilization of stored energy reserves. In the following, we describe a subset of dormant states in organisms in order to give a broad overview of the terminology, and include a more complete set in Table 1. Although dormancy clearly is an adaptation to minimize energy expenditure during adverse environmental conditions, it comes with some costs, including the cost of arousal through endogenous heat production and vulnerability to predators (Withers and Cooper, 2010).
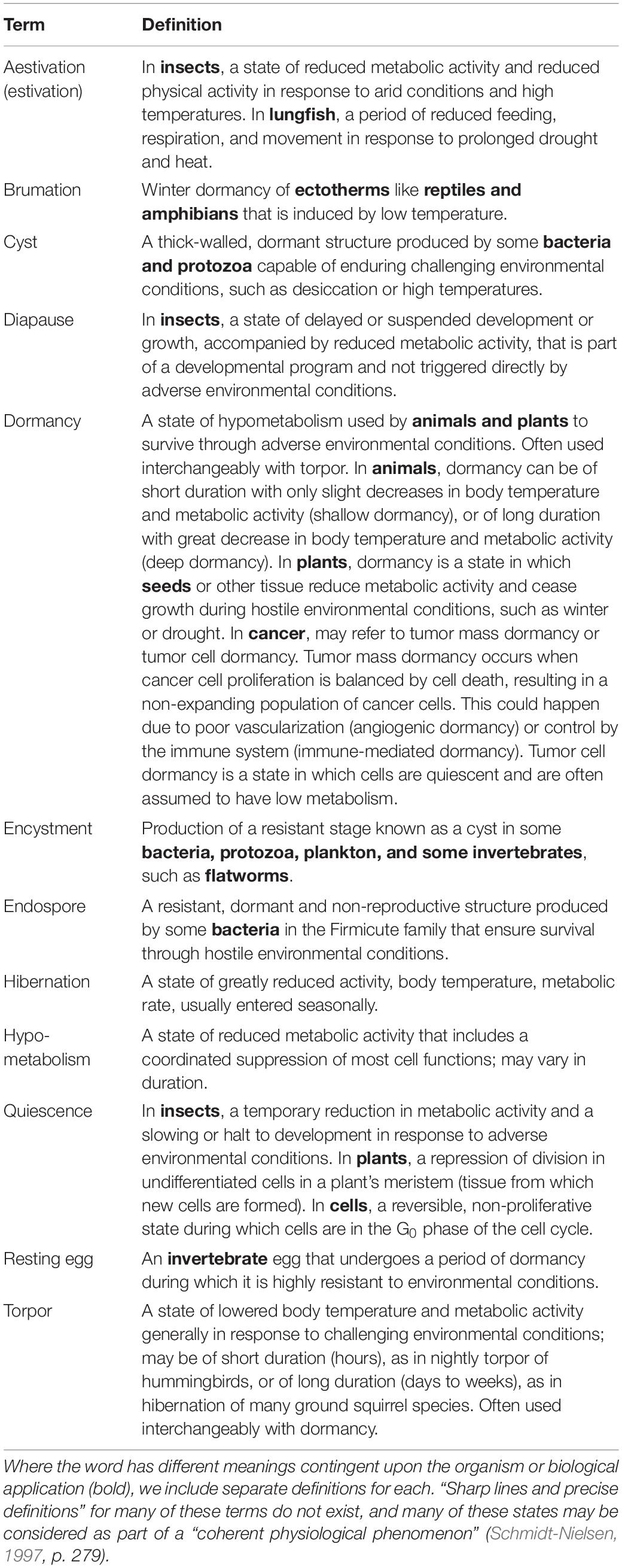
Table 1. Definitions of terms used in reference to states of reduced activity and/or metabolism in cancer, microbiology, animals, and plants.
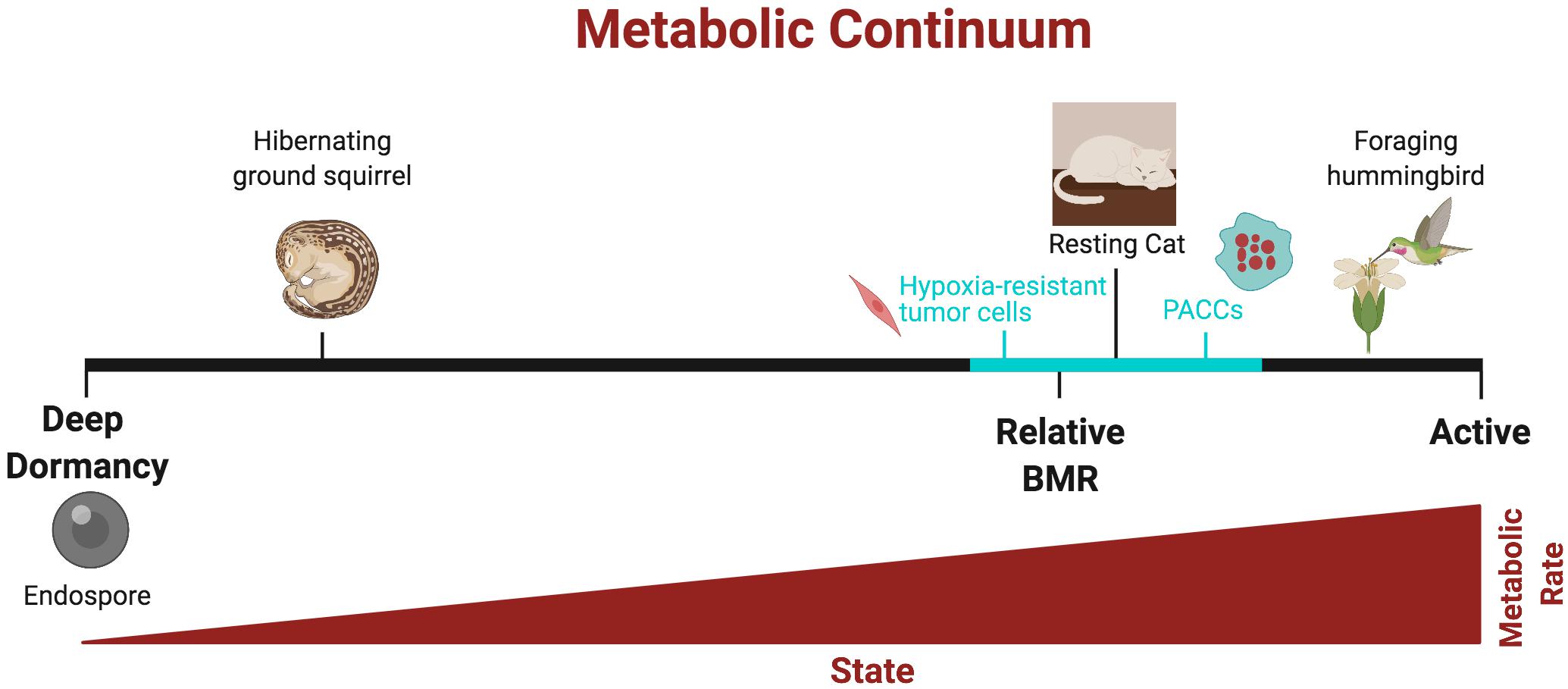
Figure 1. Continuum of metabolic states in organisms and cancer cells, from lowest (“deep dormancy”) to highest (“active”). The states below basal metabolic rate (BMR) represent hypometabolic states. The metabolic continuum of cancer cells (teal) likely falls within a narrower range of metabolic states in organisms (black). Created with BioRender.com.
Most plant species produce seeds that exhibit a period of complete metabolic dormancy following dispersal from the mother plant (Bewley, 1997; Baskin and Baskin, 2004). In temperate regions and deserts, many plants themselves enter a dormant stage (Rohde and Bhalerao, 2007), in which photosynthetic and other metabolic activity cease or are reduced to very low levels. In winter dormancy, woody plants like trees and shrubs typically drop their leaves, and many herbaceous plants survive the winter below ground as roots while letting their aboveground biomass whither.
Many microorganisms, including bacteria and protists, differentiate into a metabolically inactive and highly resistant state when faced with starvation or inhospitable environmental conditions (Sadoff, 1975). The most resistant state, produced by some bacteria, is known as an endospore. The endospore may exist for centuries, during which time they “exhibit complete metabolic dormancy and extreme resistance to multiple environmental insults” (Mury and Popham, 2014). A similar resistant state, known as a cyst, resting egg, or resting stage, is also common in a variety of protists (Corliss, 2001; Ross and Hallock, 2016), and phyto- (Ribeiro et al., 2011; Ellegaard and Ribeiro, 2018) and zooplankton (Gilbert, 1974; Ricci, 2001).
Among insects, estivation is a dormant state that manifests as quiescence, a short period of moderately depressed metabolic rate triggered by unfavorable environmental conditions (Masaki, 2009); or diapause, a prolonged period of suppressed metabolism and arrested development (Denlinger, 2009; Lee, 2009). Diapause may be facultative or obligatory, and generally is expressed in a particular life stage, which varies among taxa. Depending on the taxa, the egg, larval, or adult life history stage may undergo diapause.
Perhaps the type of dormancy that is most familiar among people generally is hibernation (deep torpor)— classically exhibited by ground squirrels (e.g., Punxsutawney Phil of the movie Groundhog Day) and carnivores like bears. Hibernation in this sense appears inextricably tied to endothermy, as hibernation involves a shallow to a deep decline in body temperature (Lyman et al., 1982). In its most extreme manifestation thus documented, the arctic ground squirrel (Urocitellus parryii) drops its core body temperature from 37 to −1.9°C during its nine month hibernation (Barnes, 1989). Because many species inhabiting subtropical and tropical regions exhibit hypometabolism generally associated with winter dormancy, many investigators now believe that hibernation is a flexible phenotypic response to scarce resources and energy conservation instead of a direct response to cold temperatures (Martin and Yoder, 2014).
Quiescence and Dormancy in Normal Cells
As with whole organisms, quiescence and dormancy are terms commonly used to describe a growth arrested state in mammalian cells including hematopoietic stem cells, lymphocytes, and fibroblasts. Quiescence is a reversible, non-proliferative state in response to nutrient deprivation (e.g., glucose, insulin, amino acids), mitogen or growth factor deprivation, loss of adhesion, or contact inhibition (Valcourt et al., 2012; Yao, 2014). Quiescence is essential for tissue homeostasis and regulation of the immune and wound healing response (Valcourt et al., 2012; Fiore et al., 2018). Gene expression, metabolism, and cell cycle re-entrance dynamics vary widely among quiescent clonal cell populations depending on the signal that initiated quiescence. As with dormancy in organisms, quiescence describes a collection of diverse states (Yao, 2014). However, cellular quiescence may not include hypometabolism, or a shutting down of metabolic functions outside of proliferation.
Hematopoietic stem cells (HSCs) regulate hematopoiesis, the production of billions of blood cells each day. In vivo mouse studies suggest that there are two populations of HSCs that control homeostasis and are maintained in adjacent “niches”. Short-term (“active”) HSCs are capable of self-renewal and divide frequently to replenish blood cells daily. Long-term HSCs may divide only five or so times per lifetime, or they can be activated to proliferate in response to injury. These long-term HSCs have been termed “dormant” (Wilson et al., 2008; Li and Clevers, 2010). Dormant HSCs exhibit a decreased metabolism as a result of reduced ribosomal biogenesis and DNA replication and are highly dependent on autophagy for survival (Wilson et al., 2008; Valcourt et al., 2012). Maintaining dormant HSCs is evolutionarily advantageous because it decreases the risk for oncogenic mutations and helps prevent stem cell depletion (Wilson et al., 2008).
Further down the hematopoietic lineage, lymphocytes, components of the adaptive immune response, are maintained in a quiescent state until activation by antigen presentation (Bryder et al., 2006). Quiescent lymphocytes are small in size and have few membrane glucose transporters, especially in the absence of growth factors (Valcourt et al., 2012). Quiescent lymphocytes depend on autophagy to obtain carbon sources for ATP production, which is synthesized by oxidative phosphorylation (Valcourt et al., 2012). Upon activation, glucose transporters increase and lymphocytes produce ATP by glycolysis (Valcourt et al., 2012). Lymphocyte quiescence prevents cell exhaustion and autoimmune disease.
Human dermal fibroblasts are maintained in a quiescent state that is characterized by their secretion of extracellular matrix. Wounding induces fibroblast activation and proliferation to coordinate wound-healing. Quiescent and activated dermal fibroblasts have similar metabolic rates, which suggests that hypometabolism is not necessarily associated with cellular quiescence (Valcourt et al., 2012). Unlike HSCs and lymphocytes, quiescent fibroblasts are not dependent on autophagy for survival and uptake glucose at rates comparable to proliferating fibroblasts (Valcourt et al., 2012). Multiple external cues, including contact inhibition and mitogen withdrawal, induce rat embryonic fibroblast to enter a non-proliferative quiescent state (Kwon et al., 2017). Fibroblasts that remain quiescent for longer move into a deeper quiescence and require greater stimulation or more time to reenter the cell cycle following serum stimulation (Kwon et al., 2017). As in organismal dormancy, quiescence in fibroblasts is heterogeneous and may entail a reactivation cost in deeply quiescent cells. However, unlike in organismal dormancy, deeply quiescent cells may remain metabolically active.
Quiescence and Dormancy in Cancer Cells
Mechanisms that regulate quiescence in normal cells provide insights into the pathways that promote quiescence in cancer cells. Cancer cells simply use or repurpose the processes, epigenetics, and genetics of normal cells. In normal cells, the trigger to divide or go quiescent is regulated by the availability of mitogenic growth factors, nutrients, and space. Loss of sensitivity to anti-growth signals is a hallmark of cancer (Hanahan and Weinberg, 2000) and a key aspect of tumorigenesis. Yet, the natural history of carcinogenesis and cancer eco-evolutionary dynamics do not always conform to continuous monotonic growth. The cancer cells making up a tumor live within a highly dynamic and interactive microenvironment consisting of fibroblasts, endothelial and inflammatory cells, growth factors, cytokines, vasculature, and lymph vessels. Collectively these constitute the tumor ecosystem. Fluctuations or interruptions in blood flow across a tumor or within regions of a tumor, lack of nutrients or space, or adverse interactions with normal cells can force cancer cells, and sometimes the tumor population as a whole, to pause rapid proliferation (Zahl et al., 2008; Almog, 2013; Hahnfeldt, 2013). Following treatment, a patient’s cancer may remain clinically undetectable for months, years, and perhaps even decades (Aguirre-Ghiso, 2018). The disease seems to persist in a cell-arrested or non-proliferative state that is often referred to as “dormancy.”
In 1934, Willis (1934) suggested “dormant” tumor cells as those that disseminated from the primary tumor and remain in a growth-arrested state. Twenty years later, Hadfield (1954) proposed that dormant tumor cells are in a temporary state of mitotic arrest. More recently, experimental models of dormancy have revealed that tumor dormancy may result from a balance between cell proliferation and death so that the tumor mass (i.e., small population of cancer cells) maintains a constant size. This balance may result from poor vascularization that limits nutrient availability to cells (Gimbrone et al., 1972; Wheelock et al., 1981) or from control by the immune system (Weinhold et al., 1979; Wheelock et al., 1981).
Cancer dormancy is thus divided into two categories (see Figure 2): (1) non-proliferative cancer cells persisting over a long period of time without dying (cellular dormancy) and (2) populations of cancer cells with cell proliferation balanced by cell death (tumor mass dormancy) (Enderling et al., 2012; Aguirre-Ghiso, 2007). Both of these categories may result in clinically undetectable cancer. The mechanisms producing them, however, are distinct and may present unique therapeutic opportunities. While dormant cancer cells are often assumed to have a lower metabolism, few studies have empirically quantified their metabolic activity (Endo et al., 2014; Carcereri de Prati et al., 2017). On the other hand, population-level dormancy includes metabolically active, proliferating cells. Because this concept of tumor population-level dormancy diverges from organismal dormancy, we do not further discuss it herein.
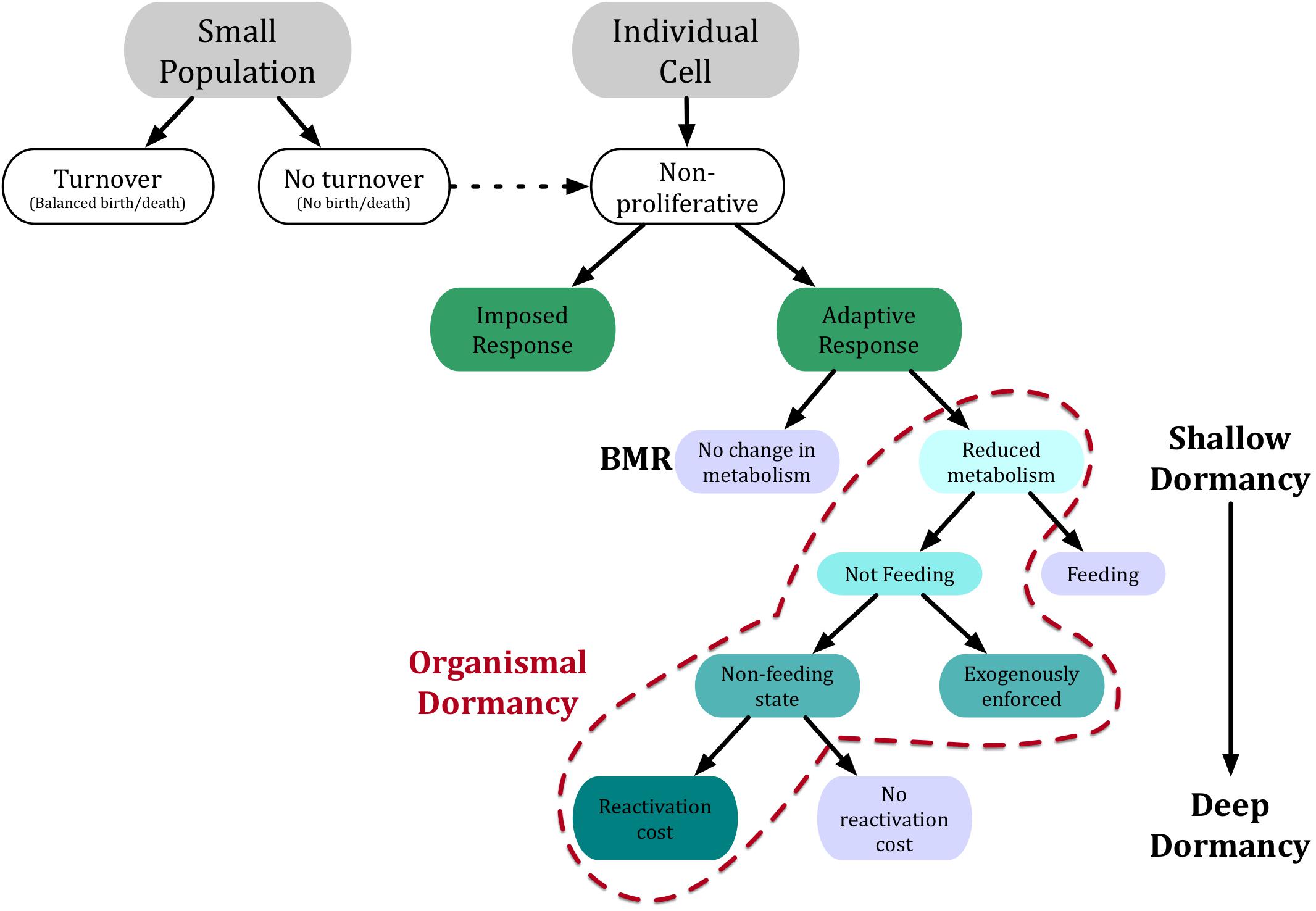
Figure 2. Characteristics of cancer dormancy. Cancer dormancy describes either a small population of cells or individual cell dormancy. Small population level dormancy could either imply that the tumor mass maintains a constant size through balanced birth and death (turnover), or the tumor mass consists of a group of dormant cells (no turnover). Each dormant cell in the group may have the same characteristics of individual cell dormancy (black dotted arrow). Imposed response represents a form of dormancy that results from the absence of an environmental factor (a germination cue) required to break dormancy (e.g., the absence of oxygen in hypoxic environments, like waterlogged soils). Parallels between organismal dormancy and cancer dormancy exist at the individual level in which dormancy is an adaptive response. Organismal dormancy (red dashed ring) is a state characterized by reduced metabolism, no feeding, and a reactivation cost. In organisms, depth of dormancy increases as metabolic activity, body temperature, and sensitivity to growth signals decreases (deeper dormancy = darker teal). Cancer cells may have additional traits associated with quiescence or dormancy (purple boxes). Exogenous factors that prevent cancer cells from feeding include lack of resources or glucose transporters. The different aspects of dormancy depicted do not imply a series of physiological processes but the different depths of dormancy attained by different organisms.
While the concept of cellular dormancy is often used in the context of disseminated cancer cells, drug-tolerant persisters and PACCs also exhibit a state that is stress resistant, quiescent, or dormant (Vallette et al., 2019; Pienta et al., 2020a). Because of their potential parallels to organismal dormancy, we consider these cells in our discussion below.
Disseminated Tumor Cells
Disseminated tumor cells (DTCs) are cancer cells that have detached from the primary tumor and spread to other locations in the body through the circulatory system. DTCs may exist, undetected, in a non-proliferative state for extended periods, referred to as cancer cell dormancy. The microenvironment within the target organ in which the DTCs survive appears to play a critical role both in regulating the apparently dormant state of the DTCs, and their re-awakening into a proliferative state (Linde et al., 2016). Dormant DTCs may elude detection and attack by the adaptive immune system, and later be “reawakened by innate immune cells (neutrophils) responding to non-tumor inflammation” (Aguirre-Ghiso, 2018). Following reawakening, the active DTCs proliferate into metastases. Whether DTCs detach early or late in the evolution of the primary tumor could also impact their potential to respond to dormancy cues, with late DTCs having greater metastatic growth potential either due to their later stage of evolution or the creation of pre-metastatic niches by early DTCs (Sosa et al., 2014). The processes of dissemination, dormancy, and reawakening may have critical clinical and therapeutic relevance.
The metastatic spread of cancer bears great resemblance to seed dispersal in plants or spores of microbes. The “seed and soil” hypothesis proposed by Paget (1889) suggests that the pattern of spread of the DTCs (“the seeds”) within the body of a patient is due to the preferential growth and survival of DTCs within certain microenvironments (“the soil”), which could explain why particular cancers only metastasize in certain organs (e.g., prostate-to-bone). In plants, seeds (or microbial spores) disperse over short or long distances. Dispersed seeds can either immediately germinate or remain dormant. Delayed germination is beneficial to the plant. When seeds do not germinate at the same time they reduce competition, sib-sib competition, and spread the risk. Furthermore, dormancy and dispersal allow seeds to escape from unfavorable conditions or arrive at favorable conditions in time and space.
Similar to plant seeds, DTCs colonize microenvironments that may be favorable or unfavorable for growth. Like many cases of seed or spore dispersal, the vast majority of circulating tumor cells die and never become DTC’s, but a tiny fraction may (Luzzi et al., 1998; Chambers et al., 2002; Lloyd et al., 2017). Microenvironments colonized by DTCs that are “non-permissive” for growth (e.g., hypoxic regions), activate stress signaling pathways that induce the DTC to enter quiescence (Aguirre-Ghiso, 2007). DTCs also colonize microenvironments where stem cells are found (stem cell niches) where signals that control HSC dormancy induce their dormancy. Because dormant HSCs can be found in hypoxic regions, these microenvironments may not be mutually exclusive (Lévesque et al., 2010). Although dormant DTCs are often assumed to be in a hypometabolic state, confirming this assumption is hampered by limited in vivo models. Insights into the metabolic state of the subset of DTCs located in hypoxic regions may be inferred from the metabolism of hypoxia resistant tumor cells.
Hypoxia Resistant Tumor Cells
While DTCs may become dormant in certain regions of an organ that are hypoxic, cancer cells of actively growing tumors also experience hypoxia through temporal variations in intratumoral blood flow and instability of vasculature (Gillies et al., 2018). Although many tumor cells die in hypoxic environments, some survive by entering a state referred to as dormancy. Because few in vitro models of tumor cell dormancy exist, understanding the properties of these dormant cells is challenging. Existing evidence suggests that these cells undergo cell-cycle arrest in the G0/G1 phases or greatly reduce proliferation (Carcereri de Prati et al., 2017).
Hypoxia-resistant cells have a lower metabolism, as indicated by an 80% decrease in glucose consumption and lower pyruvate and lactate production (Endo et al., 2014; Carcereri de Prati et al., 2017). During chronic hypoxia, hypoxia-resistant cells upregulate autophagy to obtain nutrients despite the lower consumption of glucose (Carcereri de Prati et al., 2017). When hypoxia-resistant cells are reoxygenated, their proliferation rate returns to normal after a short delay. The reversibility of their decreased proliferation (Endo et al., 2014; Carcereri de Prati et al., 2017) thus requires metabolic activation and cell remodeling. Hypoxia-resistant cells are more resistant to chemotherapy, either because of their low proliferation rate or because the drug cannot reach the hypoxic regions of the tumor. While some cancer cells enter quiescence or proliferate more slowly under chronic hypoxia, cancer cells may utilize anaerobic glycolysis under acute hypoxia, increasing glucose uptake and lactate production (Endo et al., 2014). Hence, the duration of hypoxia influences the metabolic activity of cancer cells in hypoxic environments.
Polyaneuploid Cancer Cells
Polyaneuploid cancer cells are aneuploid (have abnormal number of chromosomes) and undergo whole genome doubling in response to stress (Pienta et al., 2020a). These correspond to what others have described as polyploid giant cancer cells and persister cells (Illidge et al., 2000; Puig et al., 2008). They form from the fusion of 2N cells or from failed cytokinesis resulting in poly- or mono-nucleated polyaneuploids. This reversible state is also characterized by G0 cell cycle arrest (quiescence), increased cell size, and increased metabolic activity (distinguishing it from other quiescent states that are hypometabolic) (Pienta et al., 2020a). Once the stress is removed, and this can be months, PACCs re-enter the cell cycle and bud off non-polyploid (2N) progeny. They themselves do not proliferate as PACCs begetting PACCs. Such highly metabolic, resource uptaking, non-proliferative life history states have been found in a variety of taxa including bacteria (Valderrama et al., 2019), protists (Parfrey and Katz, 2010), fungi (Anderson et al., 2015), and plants and robustly permit survival to microenvironmental and therapeutic stress (Pienta et al., 2020a). It has been proposed that PACCs serve a decisive ecological role of allowing for increased storage, cell function, metabolic rate, and protection from stressors such as hypoxia, pH, metabolites, oxidative stress, and therapeutics. Evolutionarily, they increase heritable variation, permit self-genetic modification, and new functionality (see Table 1 from Pienta et al., 2021). The stress response in PACCs is reminiscent of organisms that undergo facultative sex such as Daphnia magna (“water fleas”), which can reproduce sexually and asexually based on environmental conditions. In good conditions, D. magna reproduce asexually to create clones, whereas under adverse conditions (e.g., cold or dry) they reproduce sexually to produce resting/diapausing eggs that are wrapped in a tough protective shell until conditions improve (Gerber et al., 2018). Sexual reproduction during adverse conditions permits genetic recombination, which, by increasing genetic diversity, may increase survival in a possibly altered environment following “awakening” from diapause.
Drug-Tolerant Persisters
In cancer biology, “drug-tolerant persisters” refer to a subpopulation of cancer cells that are reversibly tolerant to treatment due to non-mutational mechanisms such as epigenetic reprogramming (Sharma et al., 2010). This terminology is analogous to bacterial “persister cells,” a small subset of antibacterial tolerant cells. Bacteria persisters form either stochastically or in response to antibiotic treatment, are slow dividing or growth arrested, and resume growth and drug sensitivity once the antibiotic is removed (Fisher et al., 2017). While there is no single definition of drug-tolerant persisters, four properties distinguish this state from cancer cell dormancy: (1) slow proliferation, (2) decreased sensitivity to treatment, (3) restoration of drug sensitivity and cell proliferation following treatment, and (4) contribution to genetic resistance (Shen S. et al., 2020). Slow-proliferation may not be sufficient for persister cells to survive therapy; evasion of therapy may also necessitate minimizing glucose consumption, changing their cell identity via the epithelial-to-mesenchymal transition, or interacting with other cell types in the tumor microenvironment (Shen S. et al., 2020). Most of these studies use 2D cultures, where drug-tolerant persisters are rare and result from stochastic epigenetic states (Sharma et al., 2010). However, recent studies suggest that in 3D cultures, treatment persistent residual tumors emerge and adopt a program similar to embryonic diapause, a reversible state of paused development in epiblasts that is triggered by adverse conditions (Dhimolea et al., 2021; Rehman et al., 2021). Similar to embryonic diapause, these “treatment persistent organoids” are characterized by quiescence or slow-cycling, downregulated metabolic and biosynthetic activity, increased cell adhesion, and increased autophagy (Dhimolea et al., 2021; Rehman et al., 2021). Thus, treatment persistent organoids may use an evolutionarily conserved mechanism that promotes survival under stress. An open research question concerns the degree to which persister cells in cancer and other microbial systems are polyaneuploid and vice-versa. Possible differences relate to whether these non-reproductive cells are polyploid or not, whether such cells are hyper- or hypo-metabolic, and whether such cells facilitate surviving unfavorable conditions as well as accelerating evolutionary changes such as drug resistance (Pienta et al., 2020b). For those studying these phenomena in yeast and bacteria, cancer may provide an ideal complementary experimental model organism (see section “Comparison of Dormancy in Other Organisms and in Cancer”).
Comparison of Dormancy in Other Organisms and in Cancer
Although direct parallels are few, many characteristics of quiescent cancer cells overlap with characteristics of dormant states in organisms (Figure 2). One similarity is that the duration of the response varies, with the longest duration associated with DTCs in cancer (months to decades) and endospores in organisms (centuries). While few studies in cancer have quantified some of the key traits of organismal dormancy including hypometabolism and reactivation cost, there is some evidence of these characteristics in hypoxia-resistant tumor cells (Endo et al., 2014; Carcereri de Prati et al., 2017). Like cysts and endospores, cancer cells may morphologically change in response to stress: hypoxia-resistant cells can have a longer shape and higher volume of cytoplasm compared to cells in non-resistant populations (Carcereri de Prati et al., 2017), drug-tolerant persisters may change their cell identity through epithelial-to-mesenchymal transition, and PACCs morph from a 2N state into a polyploid state (Pienta et al., 2020b).
On the other hand, even though hypoxia-resistant tumor cells have a lower metabolism, they may still be taking up and metabolizing nutrients since there is not a complete depletion of glucose consumption. This is in contrast to organisms, which are in a non-feeding state when they are dormant. Furthermore, some quiescent cancer cells such as PACCs may not be in a hypometabolic state. Lastly, in comparison to hibernators, whether cancer cells acquire and store energy prior to dormancy is not known, though they often rely on autophagy for survival (Carcereri de Prati et al., 2017). While the cardinal characteristic of organismal dormancy is hypometabolism, the primary feature of cancer cell dormancy is non-proliferation. Dormancy in cancer is only loosely associated with hypometabolism and lack of feeding, and the continuum of metabolic states in non-proliferative or slowly dividing cancer cells likely falls within a narrower range compared to organisms (Figure 1).
Yeast (Saccharomyces sp.) may provide some of the most direct comparisons between cancer cell and microbial cell dormancy (Hohmann and Mager, 2007). For instance when used for producing ethanol, the yeast must be able to tolerate and respond to temperature, oxidative, ethanol, and osmotic stressors (Saini et al., 2018). In response, yeast can exhibit the continuum of maintaining proliferation and activity under stress, reducing proliferation or switching to sexual reproduction (form haploid spores through meiosis that can combine to form the diploid state), changing metabolic state (i.e., via activation of heat shock proteins), forming PACC-like polyploids, or reducing metabolic activity and engaging in autophagy.
Evolutionary Ecology of Dormancy Strategies
Variability in environmental conditions is common to all ecosystems, creating favorable and unfavorable periods for growth and survival. Natural ecosystems outside of cancer frequently experience temporal fluctuations in temperature and precipitation; in cancer, unpredictable patterns of blood flow cause temporal variations in nutrients, growth factors, pH, oxygen, and immune infiltration. Under these circumstances, dormancy is an adaptation that generally serves four possible functions: (1) bet-hedging, (2) avoiding over-crowding, (3) avoiding sib-sib competition, and (4) hunkering down and surviving unfavorable times (Simpson, 2007; Shefferson et al., 2018). The first three functions can select for a dormancy fraction where some of the population remain in an active state while others remain dormant. The fourth represents predictive dormancy where the organism or cancer cells have time to respond to the unfavorable conditions, and furthermore, can assess when conditions have improved. Cancer cells do exhibit predictive dormancy, may exhibit bet-hedging, and at present, there have been no experiments, to our knowledge, that would show whether cancer cells engage in dormancy to avoid over-crowding or sib-sib competition.
All of the above are in response to temporal variation. What of spatiotemporal variation? Venable and Brown (1988) used a mathematical model to predict how dormancy and dispersal traits in the seeds of annual plants co-adapt. As temporal autocorrelations decrease dormancy should be favored. In the absence of spatial autocorrelations and when spatial variability is on a smaller scale dispersal is favored over dormancy. Dormancy and dispersal can complement or substitute for each other, though not entirely. Snyder (2006) explores whether the presence of dormancy reduces the need for dispersal (Snyder, 2006). In terms of dispersal, cancer cells do exhibit stochastic and directed movement (chemotaxis) (Roussos et al., 2011; Sung and Weaver, 2017). But these movements, while large in relation to normal cells, are virtually sedentary compared to motile protists with cancer cells showing migration speeds of 0.4 μm per minute (Shen Q. et al., 2020), close to a body length per hour. This is one to three orders of magnitude slower than the speed of amoeboid cells (Van Haastert, 2011; Ildefonso et al., 2019). Dissemination in the blood as circulating tumor cells represents a long range dispersal that leads to highly improbable success. The extent to which cancers rely on dispersal as an adaptation to avoid spatiotemporal variability should be investigated in the context of dormancy. Regardless, dormancy should provide a prime adaptation for managing environmental uncertainty in cancer cells.
Most of our examples from nature and cancer have involved the use of predictive dormancy to respond to predictable or stochastic environmental variability. Ample evidence in terms of arrested cell cycles, PACCs, persister cells, and shifts toward autophagy show that cancer cells will cease proliferation and enter into some form of dormancy under harsh conditions. What of bet hedging? Bet-hedging is a strategy that evolves in unpredictably varying environments where expected fitness (arithmetic mean fitness) is sacrificed to reduce the temporal variance in fitness (geometric mean fitness) (Brockmann, 1987; Philippi and Seger, 1989) and was first proposed to explain why some plant seeds immediately germinate while others lie dormant (Cohen, 1966).
There can be “diversifying bet-hedging” where fractions of the population remain in different states regardless of current conditions. These states (e.g., fixed dormancy and germination fractions in annual plants) tradeoff fitness during good times and fitness during bad times (Childs et al., 2010; Starrfelt and Kokko, 2012). The bacteria Bacillus subtilus, like desert annuals, shows some stochastic sporulation regardless of nutrient conditions (Grimbergen et al., 2015). Similar examples can be found for the social amoeba Dictyostelium discoideum (Martínez-García and Tarnita, 2017), a marine amoeba Flabellula baltica (Fenchel, 2010), and in budding yeast Saccharomyces cerevisiae (Bagamery et al., 2020).
Diversifying bet-hedging in cancer does occur. PACCs have been identified as a small but ever-present fraction of the cancer cell population even in the absence of a major stress such as therapy, nutrient deprivation, or hypoxia (Lin et al., 2020). Cell culture experiments of dormancy show that during serum deprivation, a small proportion of cancer cells remained proliferative. Furthermore, when serum was replenished, a minor (but non-zero) proportion of cells remained non-proliferative (Barney et al., 2020). Some of these differences could arise due to the stochasticity in gene expression which generates phenotypic differences in cells that have the same genotype (Viney and Reece, 2013). Protein synthesis can promote rapid divergence so that sister cells are no more similar to each other than randomly chosen cell pairs (Spencer et al., 2009). Simons and Johnston (1997) suggest developmental instabilities as a source of diversifying bet-hedging, and the genetic instability of cancer cells may provide for bet-hedging through offspring with diverse heritable traits. Miller et al. (2020) explore how diversifying bet-hedging might promote coexistence of different cancer cell types as has been suggested for microbes (Jones and Lennon, 2010).
Conservative bet-hedging strategies involve a single state for the population where its trait value enhances survival under bad conditions while sacrificing opportunities during favorable times (Haaland et al., 2019, 2020). While most organisms’ traits are a likely compromise between variable conditions, it can be hard to determine when such traits strictly tradeoff arithmetic with geometric mean fitness. Simons and Johnston (2003) provide an example with Indian tobacco Lobelia inflate where flowering time, while suboptimal in most years, serves well during bad years. Putative traits in cancer have not been studied in detail within the context of conservative bet-hedging. Pseudohypoxia has been proposed as such a case. Here, the cancer cells maintain high HIF-1 expression as a response to hypoxic conditions even under normoxia (Pressley et al., 2021). Other traits could include those associated with the maintenance of high levels of membrane pumps and metabolism to respond quickly to stochastic variation in the presence of toxins, low pH, damaging levels of oxygen free radicals, and even drug therapy. These traits are straying from dormancy per se but represent potentially critical forms of bet hedging. Dormancy strategies likely best fall into diversifying bet-hedging.
Discussion—From Ecology to Cancer and Back Again
Cancer cells exist in a highly dynamic ecosystem where they experience both competition and cooperation with nearby cells. Increased understanding of the ecology and evolution of cancer is leading to new treatment strategies, like adaptive therapies that exploit cancer cell competition (Gatenby et al., 2009; Silva et al., 2012; Zhang et al., 2017). Similarly, better understanding dormancy from an ecological perspective may help devise new approaches to target these cells by exploiting the mechanisms that promote their awakening, maintenance, or eradication. Many advances have been made through interdisciplinary approaches between ecologists and cancer biologists. For such efforts to be fruitful, however, collaborators must possess a mutual understanding of technical terms such as “dormancy” and speak a common language. We believe that if the cancer biology community adopted a more precise definition of dormancy that also includes issues of reduced feeding, metabolism, robustness, stress tolerance, and reactivation costs rather than just lack of proliferation, then it would ease the journey of ecologists trying to contribute to cancer research.
For the evolutionary ecologist interested in testing models and ideas pertaining to dormancy, cancer provides diverse experimental approaches. Cancer research can provide a rich spatial and temporal resolution of data not typically attainable in other natural or laboratory systems. In contrast to field studies, experiments can be replicated within the same cancer under a more controlled setting. Cancer biology may thus provide multiple avenues for testing both ecological and evolutionary theories of dormancy that are difficult to address otherwise. For example, cancer cell dormancy occurs de novo across a vast array of cancers, including multiple myeloma, prostate cancer metastasis to the bone, and breast cancer metastasis to the bone, lung, and brain (Phan and Croucher, 2020). This broad occurrence of dormancy could allow for the exploration of differences in how dormancy is regulated between cancers or metastatic sites, and whether those mechanisms resemble known regulation mechanisms found across taxa in organismal dormancy. Because cancers evolve rapidly, experimental cancer systems provide opportunities to test hypotheses regarding the environmental characteristics that select for the evolution of dormancy as an adaptation. Studying the evolution of cancer cell dormancy may provide novel insights into the evolution of dormancy at the organismal level.
Research on cancer brings an array of technologies for conducting experiments in mice (Lee et al., 2018), in organoids, as 3-D spheroids, or more traditional 2-D culture techniques. RNA sequencing (RNA-Seq) technologies permit measuring single cell expression of genes associated with cellular metabolism, proliferation, and cell membrane activity (Recasens and Munoz, 2019). Methylation profiling (Ferrer et al., 2020) and whole-genome or targeted genome sequencing can identify heritable differences between cancer cells within a cell line or between cell lines with diverse ecological properties (e.g., contrasts between breast cancer cells lines such as the highly glycolytic MDA-MB-231 and the non-glycolytic MCF-7). The Seahorse XF extracellular flux analyzer can measure single cell metabolism of cancer cells in different metabolic states, from different clones, or different cell lines (Bhatia et al., 2021). Immunohistochemical staining of cell cultures of histology preparations can identify metabolic markers of cell proliferation, metabolism, and other cellular properties that may be relevant to dormancy. Finally, selection experiments in the lab on cancer cell lines generally produce significant heritable changes within months. In this way, the technologies and resources available for cancer research may facilitate research on the evolutionary drivers of dormancy, just as understandings of dormancy in nature can add conceptual and terminological rigor to the insights gained from studying cancer dormancy.
Data Availability Statement
The original contributions presented in the study are included in the article/supplementary material, further inquiries can be directed to the corresponding author.
Author Contributions
JB and CW contributed to the conception and design of the study. AM, CW, and JB wrote the manuscript. DB provided funding. All authors contributed to manuscript revisions, read, and approved the submitted version.
Funding
DB and AM acknowledge funding from NCI (IMAG) U01CA202958 and PSON U01 (U01CA244101). JB acknowledges funding from NIH/NCI 1U54CA193489-01A1, Cancer as a Complex Adaptive System, NIH/NCI U54 Supplement, The tumor-host evolutionary arms race.
Conflict of Interest
The authors declare that the research was conducted in the absence of any commercial or financial relationships that could be construed as a potential conflict of interest.
Acknowledgments
We thank Andriy Marusyk and Mostafa Nasr for helpful discussions related to the content of this manuscript, and the peer reviewers for their constructive feedback. Figure 1 was created with ©BioRender—biorender.com as licensed by the Scientific Development Office of the Moffitt Cancer Center.
References
Aguirre-Ghiso, J. A. (2007). Models, mechanisms and clinical evidence for cancer dormancy. Nat. Rev. Cancer 7, 834–846. doi: 10.1038/nrc2256
Aguirre-Ghiso, J. A. (2018). How dormant cancer persists and reawakens. Science 361, 1314–1315. doi: 10.1126/science.aav0191
Almog, N. (2013). Genes and regulatory pathways involved in persistence of dormant micro-tumors. Adv. Exp. Med. Biol. 734, 3–17. doi: 10.1007/978-1-4614-1445-2_1
Anderson, C. A., Roberts, S., Zhang, H., Kelly, C. M., Kendall, A., Lee, C., et al. (2015). Ploidy variation in multinucleate cells changes under stress. Mol. Biol. Cell 26, 1129–1140. doi: 10.1091/mbc.e14-09-1375
Bagamery, L. E., Justman, Q. A., Garner, E. C., and Murray, A. W. (2020). A Putative Bet-hedging strategy buffers budding yeast against environmental instability. Curr. Biol. 30, 4563–4578. doi: 10.1016/j.cub.2020.08.092
Barnes, B. (1989). Freeze avoidance in a mammal: body temperatures below 0 degree C in an Arctic hibernator. Science 244, 1593–1595. doi: 10.1126/science.2740905
Barney, L. E., Hall, C. L., Schwartz, A. D., Parks, A. N., Sparages, C., Galarza, S., et al. (2020). Tumor cell-organized fibronectin maintenance of a dormant breast cancer population. Sci. Adv. 6:eaaz4157. doi: 10.1126/sciadv.aaz4157
Baskin, J. M., and Baskin, C. C. (2004). A classification system for seed dormancy. Seed Sci. Res. 14, 1–16. doi: 10.1079/SSR2003150
Bewley, J. D. (1997). Seed germination and dormancy. Plant Cell 96, 1055–1066. doi: 10.1105/tpc.9.7.1055
Bhatia, S., Thompson, E. W., and Gunter, J. H. (2021). “Studying the metabolism of epithelial-mesenchymal plasticity using the seahorse XFe96 extracellular flux analyzer,” in The Epithelial-to Mesenchymal Transition, eds K. Campbell and E. Theveneau (Berlin: Springer), 327–340. doi: 10.1007/978-1-0716-0779-4_25
Bryder, D., Rossi, D. J., and Weissman, I. L. (2006). Hematopoietic stem cells: the paradigmatic tissue-specific stem cell. Am. J. Pathol. 169, 338–346. doi: 10.2353/ajpath.2006.060312
Carcereri de Prati, A., Butturini, E., Rigo, A., Oppici, E., Rossin, M., Boriero, D., et al. (2017). Metastatic breast cancer cells enter into dormant state and express cancer stem cells phenotype under chronic hypoxia. J. Cell. Biochem. 118, 3237–3248. doi: 10.1002/jcb.25972
Chambers, A. F., Groom, A. C., and MacDonald, I. C. (2002). Dissemination and growth of cancer cells in metastatic sites. Nat. Rev. Cancer 2, 563–572. doi: 10.1038/nrc865
Childs, D. Z., Metcalf, C. J. E., and Rees, M. (2010). Evolutionary bet-hedging in the real world: empirical evidence and challenges revealed by plants. Proc. R. Soc. B Biol. Sci. 277, 3055–3064. doi: 10.1098/rspb.2010.0707
Cohen, D. (1966). Optimizing reproduction in a randomly varying environment. J. Theor. Biol. 12, 119–129. doi: 10.1016/0022-5193(66)90188-3
Denlinger, D. L. (2009). “Diapause,” in Encyclopedia of insects, eds V. Resh and R. Carde (Amsterdam: Elsevier), 267–271.
Dhimolea, E., de Matos Simoes, R., Kansara, D., Al’Khafaji, A., Bouyssou, J., Weng, X., et al. (2021). An embryonic diapause-like adaptation with suppressed myc activity enables tumor treatment persistence. Cancer Cell 39, 240.e11–256.e11. doi: 10.1016/j.ccell.2020.12.002
Ellegaard, M., and Ribeiro, S. (2018). The long-term persistence of phytoplankton resting stages in aquatic ‘seed banks.’. Biol. Rev. 93, 166–183. doi: 10.1111/brv.12338
Enderling, H., Almog, N., and Hlatky, L. (2012). Systems Biology of Tumor Dormancy. Berlin: Springer Science & Business Media.
Endo, H., Okuyama, H., Ohue, M., and Inoue, M. (2014). Dormancy of cancer cells with suppression of AKT activity contributes to survival in chronic hypoxia. PLoS One 9:e0098858. doi: 10.1371/journal.pone.0098858
Fenchel, T. (2010). The life history of Flabellula baltica Smirnov (Gymnamoebae, Rhizopoda): adaptations to a spatially and temporally heterogeneous environment. Protist 161, 279–287. doi: 10.1016/j.protis.2009.10.005
Ferrer, A. I., Trinidad, J. R., Sandiford, O., Etchegaray, J.-P., and Rameshwar, P. (2020). Epigenetic dynamics in cancer stem cell dormancy. Cancer Metastasis Rev. 39, 721–738. doi: 10.1007/s10555-020-09882-x
Fiore, A. P. Z. P., Ribeiro, P., de, F., and Bruni-Cardoso, A. (2018). Sleeping beauty and the microenvironment enchantment: microenvironmental regulation of the proliferation-quiescence decision in normal tissues and in cancer development. Front. Cell Dev. Biol. 6:59. doi: 10.3389/fcell.2018.00059
Fisher, R. A., Gollan, B., and Helaine, S. (2017). Persistent bacterial infections and persister cells. Nat. Rev. Microbiol. 15:453. doi: 10.1038/nrmicro.2017.42
Gatenby, R. A., Silva, A. S., Gillies, R. J., and Frieden, B. R. (2009). Adaptive therapy. Cancer Res. 69, 4894–4903.
Gerber, N., Kokko, H., Ebert, D., and Booksmythe, I. (2018). Daphnia invest in sexual reproduction when its relative costs are reduced. Proc. R. Soc. B Biol. Sci. 285:20172176. doi: 10.1098/rspb.2017.2176
Gilbert, J. J. (1974). Dormancy in rotifers. Trans. Am. Microsc. Soc. 93, 490–513. doi: 10.2307/3225154
Gillies, R. J., Brown, J. S., Anderson, A. R. A., and Gatenby, R. A. (2018). Eco-evolutionary causes and consequences of temporal changes in intratumoural blood flow. Nat. Rev. Cancer 18, 576–585. doi: 10.1038/s41568-018-0030-7
Gimbrone, M. A. Jr., Leapman, S. B., Cotran, R. S., and Folkman, J. (1972). Tumor dormancy in vivo by prevention of neovascularization. J. Exp. Med. 136, 261–276. doi: 10.1084/jem.136.2.261
Grimbergen, A. J., Siebring, J., Solopova, A., and Kuipers, O. P. (2015). Microbial bet-hedging: the power of being different. Curr. Opin. Microbiol. 25, 67–72. doi: 10.1016/j.mib.2015.04.008
Haaland, T. R., Wright, J., and Ratikainen, I. I. (2020). Generalists versus specialists in fluctuating environments: a bet-hedging perspective. Oikos 129, 879–890. doi: 10.1111/oik.07109
Haaland, T. R., Wright, J., Tufto, J., and Ratikainen, I. I. (2019). Short-term insurance versus long-term bet-hedging strategies as adaptations to variable environments. Evolution 73, 145–157. doi: 10.1111/evo.13659
Hahnfeldt, P. (2013). “The host support niche as a control point for tumor dormancy: implications for tumor development and beyond,” in Systems Biology of Tumor Dormancy, eds H. Enderling, N. Almog, and L. Hlatky (Berlin: Springer), 19–35. doi: 10.1007/978-1-4614-1445-2_2
Hohmann, S., and Mager, W. H. (2007). Yeast Stress Responses. Berlin: Springer Science & Business Media.
Ildefonso, M., Bringas, C., Malaina, I., Regner, B., Pérez-Samartín, A., Boyano, M. D., et al. (2019). The nucleus does not significantly affect the migratory trajectories of amoeba in two-dimensional environments. Sci. Rep. 9, 1–15.
Illidge, T. M., Cragg, M. S., Fringes, B., Olive, P., and Erenpreisa, J. A. (2000). Polyploid giant cells provide a survival mechanism for p53 mutant cells after DNA damage. Cell Biol. Int. 24, 621–633. doi: 10.1006/cbir.2000.0557
Jones, S. E., and Lennon, J. T. (2010). Dormancy contributes to the maintenance of microbial diversity. Proc. Natl. Acad. Sci. 107, 5881–5886. doi: 10.1073/pnas.0912765107
Kwon, J. S., Everetts, N. J., Wang, X., Wang, W., Della Croce, K., Xing, J., et al. (2017). Controlling depth of cellular quiescence by an Rb-E2F network switch. Cell Rep. 20, 3223–3235. doi: 10.1016/j.celrep.2017.09.007
Lee, R. E. (2009). “Chapter 79 - Dormancy,” in Encyclopedia of insects, eds V. Resh and R. Carde (San Diego: Academic Press), 300–301.
Lee, S. H., Park, S. A., Zou, Y., Seo, S.-U., Jun, C.-D., Lee, W. J., et al. (2018). Real-time monitoring of cancer cells in live mouse bone marrow. Front. Immunol. 9:1681. doi: 10.3389/fimmu.2018.01681
Lévesque, J. P., Helwani, F. M., and Winkler, I. G. (2010). The endosteal ‘osteoblastic’ niche and its role in hematopoietic stem cell homing and mobilization. Leukemia 24, 1979–1992. doi: 10.1038/leu.2010.214
Li, N., and Clevers, H. (2010). Coexistence of quiescent and active adult stem cells in mammals. Science 327, 542–545. doi: 10.1126/science.1180794
Lin, K.-C., Sun, Y., Torga, G., Sherpa, P., Zhao, Y., Qu, J., et al. (2020). An in vitro tumor swamp model of heterogeneous cellular and chemotherapeutic landscapes. Lab. Chip 20, 2453–2464. doi: 10.1039/d0lc00131g
Linde, N., Fluegen, G., and Aguirre-Ghiso, J. A. (2016). The relationship between dormant cancer cells and their microenvironment. Adv. Cancer Res. 132, 45–71. doi: 10.1016/bs.acr.2016.07.002
Lloyd, M. C., Gatenby, R. A., and Brown, J. S. (2017). “Ecology of the metastatic process,” in Ecology and Evolution of Cancer, eds B. Ujvari, B. Roche, and F. Thomas (Amsterdam: Elsevier), 153–165. doi: 10.1016/b978-0-12-804310-3.00011-9
Luzzi, K. J., MacDonald, I. C., Schmidt, E. E., Kerkvliet, N., Morris, V. L., Chambers, A. F., et al. (1998). Multistep nature of metastatic inefficiency: dormancy of solitary cells after successful extravasation and limited survival of early micrometastases. Am. J. Pathol. 153, 865–873.
Lyman, C. P., Willis, J. S., Malan, A., and Wang, L. C. H. (1982). Hibernation and Torpor in Birds and Mammals. New York, NY: Aca-demic Press.
Martin, S. L., and Yoder, A. D. (2014). Theme and variations: heterothermy in mammals. Am. Zool. 54, 439–442. doi: 10.1093/icb/icu085
Martínez-García, R., and Tarnita, C. E. (2017). Seasonality can induce coexistence of multiple bet-hedging strategies in Dictyostelium discoideum via storage effect. J. Theor. Biol. 426, 104–116. doi: 10.1016/j.jtbi.2017.05.019
Masaki, S. (2009). “Aestivation,” in Encyclopedia of Insects, eds V. H. Resh and R. T. Cardé (Amsterdam: Elsevier), 2–4.
Melvin, R. G., and Andrews, M. T. (2009). Torpor induction in mammals: recent discoveries fueling new ideas. Trends Endocrinol. Metab. 20, 490–498. doi: 10.1016/j.tem.2009.09.005
Miller, A. K., Brown, J. S., Basanta, D., and Huntly, N. (2020). What is the storage effect, why should it occur in cancers, and how can it inform cancer therapy? Cancer Control 27, 1–9. doi: 10.1177/1073274820941968
Paget, S. (1889). The distribution of secondary growths in cancer of the breast. Lancet 133, 571–573. doi: 10.1016/s0140-6736(00)49915-0
Parfrey, L. W., and Katz, L. A. (2010). Genome dynamics are influenced by food source in Allogromia laticollaris strain CSH (Foraminifera). Genome Biol. Evol. 2, 678–685. doi: 10.1093/gbe/evq051
Phan, T. G., and Croucher, P. I. (2020). The dormant cancer cell life cycle. Nat. Rev. Cancer 20, 398–411. doi: 10.1038/s41568-020-0263-0
Philippi, T., and Seger, J. (1989). Hedging one’s evolutionary bets, revisited. Trends Ecol. Evol. 4, 41–44. doi: 10.1016/0169-5347(89)90138-9
Pienta, K. J., Hammarlund, E. U., Austin, R. H., Axelrod, R., Brown, J. S., and Amend, S. R. (2020a). Cancer cells employ an evolutionarily conserved polyploidization program to resist therapy. Semin. Cancer Biol. [Epub ahead of print].
Pienta, K. J., Hammarlund, E. U., Axelrod, R., Amend, S. R., and Brown, J. S. (2020b). Convergent evolution, evolving evolvability, and the origins of lethal cancer. Mol. Cancer Res. 18, 801–810. doi: 10.1158/1541-7786.MCR-19-1158
Pienta, K. J., Hammarlund, E. U., Brown, J. S., Amend, S. R., and Axelrod, R. M. (2021). Cancer recurrence and lethality are enabled by enhanced survival and reversible cell cycle arrest of polyaneuploid cells. Proc. Natl. Acad. Sci. U.S.A. 118:e2020838118. doi: 10.1073/pnas.2020838118
Pressley, M., Gallaher, J. A., Brown, J. S., Tomaszewski, M. R., Borad, P., Damaghi, M., et al. (2021). Cycling hypoxia selects for constitutive HIF stabilization. Sci. Rep. 11, 1–14.
Puig, P., Guilly, M., Bouchot, A., Droin, N., Cathelin, D., Bouyer, F., et al. (2008). Tumor cells can escape DNA-damaging cisplatin through DNA endoreduplication and reversible polyploidy. Cell Biol. Int. 32, 1031–1043. doi: 10.1016/j.cellbi.2008.04.021
Recasens, A., and Munoz, L. (2019). Targeting Cancer Cell Dormancy. Trends Pharmacol. Sci. 40, 128–141. doi: 10.1016/j.tips.2018.12.004
Rehman, S. K., Haynes, J., Collignon, E., Goyal, S., Moffat, J., and Brien, C. A. O. (2021). Colorectal cancer cells enter a diapause-like DTP state to survive chemotherapy. Cell 184, 226.e21–242.e21. doi: 10.1016/j.cell.2020.11.018
Ribeiro, S., Berge, T., Lundholm, N., Andersen, T. J., Abrantes, F., and Ellegaard, M. (2011). Phytoplankton growth after a century of dormancy illuminates past resilience to catastrophic darkness. Nat. Commun. 2:311. doi: 10.1038/ncomms1314
Ricci, C. (2001). Dormancy patterns in rotifers. Hydrobiologia 446, 1–11. doi: 10.1007/978-94-010-0756-6_1
Rohde, A., and Bhalerao, R. P. (2007). Plant dormancy in the perennial context. Trends Plant Sci. 12, 217–223. doi: 10.1016/j.tplants.2007.03.012
Ross, B. J., and Hallock, P. (2016). Dormancy in the foraminifera: a review. J. Foraminifer. Res. 46, 358–368. doi: 10.2113/gsjfr.46.4.358
Roussos, E. T., Condeelis, J. S., and Patsialou, A. (2011). Chemotaxis in cancer. Nat. Rev. Cancer 11, 573–587. doi: 10.1038/nrc3078
Sadoff, H. L. (1975). Encystment and germination in Azotobacter vinelandii. Bacteriol. Rev. 39, 516–539. doi: 10.1128/mmbr.39.4.516-539.1975
Saini, P., Beniwal, A., Kokkiligadda, A., and Vij, S. (2018). Response and tolerance of yeast to changing environmental stress during ethanol fermentation. Process Biochem. 72, 1–12. doi: 10.1016/j.procbio.2018.07.001
Schmidt-Nielsen, K. (1997). Animal Physiology: Adaptation and Environment. Cambridge, MA: Cambridge university press.
Sharma, S. V., Lee, D. Y., Li, B., Quinlan, M. P., Takahashi, F., Maheswaran, S., et al. (2010). A chromatin-mediated reversible drug-tolerant state in cancer cell subpopulations. Cell 141, 69–80. doi: 10.1016/j.cell.2010.02.027
Shefferson, R. P., Kull, T., Hutchings, M. J., Selosse, M., Jacquemyn, H., Kellett, K. M., et al. (2018). Drivers of vegetative dormancy across herbaceous perennial plant species. Ecol. Lett. 21, 724–733. doi: 10.1111/ele.12940
Shen, Q., Niederstrasser, H., Barakat, R., Haddadin, Z., Miller, S. R., Posner, B., et al. (2020). Single-cell-level screening method for migratory cancer cells and its potential feasibility in high-throughput manner. Biofabrication 12:35019.
Shen, S., Vagner, S., and Robert, C. (2020). Persistent cancer cells: the deadly survivors. Cell 183, 860–874. doi: 10.1016/j.cell.2020.10.027
Silva, A. S., Kam, Y., Khin, Z. P., Minton, S. E., Gillies, R. J., and Gatenby, R. A. (2012). Evolutionary approaches to prolong progression-free survival in breast cancer. Cancer Res. 72, 6362–6370. doi: 10.1158/0008-5472.can-12-2235
Simons, A. M., and Johnston, M. O. (1997). Developmental instability as a bet-hedging strategy. Oikos 80, 401–406. doi: 10.2307/3546608
Simons, A. M., and Johnston, M. O. (2003). Suboptimal timing of reproduction in Lobelia inflata may be a conservative bet-hedging strategy. J. Evol. Biol. 16, 233–243. doi: 10.1046/j.1420-9101.2003.00530.x
Snyder, R. E. (2006). Multiple risk reduction mechanisms: can dormancy substitute for dispersal? Ecol. Lett. 9, 1106–1114. doi: 10.1111/j.1461-0248.2006.00962.x
Sosa, M. S., Bragado, P., and Aguirre-Ghiso, J. A. (2014). Mechanisms of disseminated cancer cell dormancy: an awakening field. Nat. Rev. Cancer 14, 611–622. doi: 10.1038/nrc3793
Spencer, S. L., Gaudet, S., Albeck, J. G., Burke, J. M., and Sorger, P. K. (2009). Non-genetic origins of cell-to-cell variability in TRAIL-induced apoptosis. Nature 459, 428–432. doi: 10.1038/nature08012
Starrfelt, J., and Kokko, H. (2012). Bet-hedging-a triple trade-off between means, variances and correlations. Biol. Rev. 87, 742–755. doi: 10.1111/j.1469-185X.2012.00225.x
Sung, B. H., and Weaver, A. M. (2017). Exosome secretion promotes chemotaxis of cancer cells. Cell Adh. Migr. 11, 187–195. doi: 10.1080/19336918.2016.1273307
Valcourt, J. R., Lemons, J. M. S., Haley, E. M., Kojima, M., Demuren, O. O., and Coller, H. A. (2012). Staying alive: metabolic adaptations to quiescence. Cell Cycle 11, 1680–1696. doi: 10.4161/cc.19879
Valderrama, J. A., Kulkarni, S. S., Nizet, V., and Bier, E. (2019). A bacterial gene-drive system efficiently edits and inactivates a high copy number antibiotic resistance locus. Nat. Commun. 10, 1–8.
Vallette, F. M., Olivier, C., Lézot, F., Oliver, L., Cochonneau, D., Lalier, L., et al. (2019). Dormant, quiescent, tolerant and persister cells: four synonyms for the same target in cancer. Biochem. Pharmacol. 162, 169–176. doi: 10.1016/j.bcp.2018.11.004
Van Haastert, P. J. M. (2011). Amoeboid cells use protrusions for walking, gliding and swimming. PLoS One 6:e27532. doi: 10.1371/journal.pone.0027532
Venable, D. L., and Brown, J. S. (1988). The selective interactions of dispersal, dormancy, and seed size as adaptations for reducing risk in variable environments. Am. Nat. 131, 360–384. doi: 10.1086/284795
Villanueva-Cañas, J. L., Faherty, S. L., Yoder, A. D., and Albà, M. M. (2014). Comparative genomics of mammalian hibernators using gene networks. Integr. Comp. Biol. 54, 452–462. doi: 10.1093/icb/icu048
Viney, M., and Reece, S. E. (2013). Adaptive noise. Proc. R. Soc. B Biol. Sci. 280:3. doi: 10.1098/rspb.2013.1104
Weinhold, K. J., Goldstein, L. T., and Wheelock, E. F. (1979). The tumor dormant state. Quantitation of L5178Y cells and host immune responses during the establishment and course of dormancy in syngeneic DBA/2 mice. J. Exp. Med. 149, 732–744. doi: 10.1084/jem.149.3.732
Wheelock, E. F., Weinhold, K. J., and Levich, J. (1981). The tumor dormant state. Adv. Cancer Res. 34, 107–140. doi: 10.1016/s0065-230x(08)60240-7
Wilson, A., Laurenti, E., Oser, G., van der Wath, R. C., Blanco-Bose, W., Jaworski, M., et al. (2008). Hematopoietic stem cells reversibly switch from dormancy to self-renewal during homeostasis and repair. Cell 135, 1118–1129. doi: 10.1016/j.cell.2008.10.048
Wilsterman, K., Ballinger, M. A., and Williams, C. M. (2020). A unifying, eco-physiological framework for animal dormancy. Funct. Ecol. 35, 11–31. doi: 10.1111/1365-2435.13718
Withers, P. C., and Cooper, C. E. (2010). Metabolic depression: a historical perspective. Prog. Mol. Subcell Biol. 49, 1–23. doi: 10.1007/978-3-642-02421-4_1
Yao, G. (2014). Modelling mammalian cellular quiescence. Interface Focus 4:20130074. doi: 10.1098/rsfs.2013.0074
Zahl, P.-H., Mæhlen, J., and Welch, H. G. (2008). The natural history of invasive breast cancers detected by screening mammography. Arch. Intern. Med. 168, 2311–2316. doi: 10.1001/archinte.168.21.2311
Keywords: dormancy, cancer, hypometabolism, quiescence, adaptation
Citation: Miller AK, Brown JS, Enderling H, Basanta D and Whelan CJ (2021) The Evolutionary Ecology of Dormancy in Nature and in Cancer. Front. Ecol. Evol. 9:676802. doi: 10.3389/fevo.2021.676802
Received: 06 March 2021; Accepted: 16 June 2021;
Published: 15 July 2021.
Edited by:
Sasha Raoul Xola Dall, University of Exeter, United KingdomReviewed by:
Thomas Haaland, University of Zurich, SwitzerlandE. Yagmur Erten, University of Zurich, Switzerland
Robert Noble, City University of London, United Kingdom
Copyright © 2021 Miller, Brown, Enderling, Basanta and Whelan. This is an open-access article distributed under the terms of the Creative Commons Attribution License (CC BY). The use, distribution or reproduction in other forums is permitted, provided the original author(s) and the copyright owner(s) are credited and that the original publication in this journal is cited, in accordance with accepted academic practice. No use, distribution or reproduction is permitted which does not comply with these terms.
*Correspondence: Anna K. Miller, QW5uYS5NaWxsZXJAbW9mZml0dC5vcmc=