- 1Museum für Naturkunde Magdeburg, Magdeburg, Germany
- 2Museum für Naturkunde Berlin, Leibniz-Institut für Evolutions- und Biodiversitätsforschung, Berlin, Germany
- 3Institut für Biologie, Humboldt-Universität zu Berlin, Berlin, Germany
- 4Urweltmuseum GEOSKOP, Thallichtenberg, Germany
Among amniote and non-amniote tetrapod trackways from late Carboniferous to early Permian deposits, certain trackway measures vary notably. Some of this variability can be attributed to evolutionary changes in trackmaker anatomy and locomotion style close to the origin of amniotes. Here we demonstrate that steps in early amniote locomotion evolution can be addressed by applying methods of ancestral state reconstruction on trackway data – a novel approach in tetrapod ichnology. Based on (a) measurements of 186 trackways referred to the Carboniferous and early Permian ichnogenera Batrachichnus, Limnopus, Hylopus, Amphisauropus, Matthewichnus, Ichniotherium, Dimetropus, Tambachichnium, Erpetopus, Varanopus, Hyloidichnus, Notalacerta and Dromopus, (b) correlation of these ichnotaxa with specific groups of amphibian, reptiliomorph, synapsid, and reptilian trackmakers based on imprint morphology and (c) known skeletal-morphology-based phylogenies of the supposed trackmakers, we infer ancestral states for functionally controlled trackway measures in a maximum likelihood approach. The most notable finding of our analysis is a concordant change in trackway parameters within a series of ancestral amniote trackmakers, which reflects an evolutionary change in locomotion: In the ancestors of amniotes and diadectomorphs, an increase in body size was accompanied by a decrease in (normalized) gauge width and glenoacetabular length and by a change in imprint orientation toward a more trackway-parallel and forward-pointing condition. In the subsequent evolution of diadectomorph, synapsid and reptilian trackmakers after the diversification of the clades Cotylosauria (Amniota + Diadectomorpha) and Amniota, stride length increased whereas gauges decreased further or remained relatively narrow within most lineages. In accordance with this conspicuous pattern of evolutionary change in trackway measures, we interpret the body size increase as an underlying factor that triggered the reorganization of the locomotion apparatus. The secondary increase in stride length, which occurred convergently within distinct groups, is interpreted as an increase in locomotion capability when the benefits of reorganization came into effect. The track-trackmaker pair of Ichniotherium sphaerodactylum and Orobates pabsti from the early Permian Bromacker locality of the Thuringian Forest, proposed in earlier studies as a suitable ancestral amniote track-trackmaker model, fits relatively well with our modeled last common ancestor of amniotes – with the caveat that the Bromacker material is younger and some of the similarities appear to be due to convergence.
Introduction
Movement traces represent an additional source of information about the locomotion of a fossil trackmaker, amending the functional interpretation of body fossil morphology. In case of a long extinct group of tetrapods, the skeletal anatomy may allow for a wide range of hypothetical postures and movement patterns, whereas fossil trackways may point to a reduced set of probable locomotion styles. Moreover, evolutionary changes in locomotion can be inferred from the comparison of distinct trackway samples produced by related trackmakers. Especially the comparative study of trackways from episodes of evolutionary transition in lifestyle, ecology and environment potentially yield notable signals of locomotion evolution. Thus, we can expect to find pattern changes in the trackways of late Paleozoic Amniota and their anamniote relatives, which cover a crucial stage of terrestrialization that preceded the emergence of erect gaits in archosauromorphs and therapsids (Kemp, 2005; Nesbitt, 2011; Benton, 2020).
The earliest body fossils of synapsids and reptiles (sauropsids), the two principal groups of Amniota, have been described from the late Carboniferous of Europe and North America (e.g., Carroll, 1964, 1969; Reisz, 1972, 1977, 1981; Reisz and Dilkes, 2003; Reisz and Fröbisch, 2014; Modesto et al., 2015; Mann et al., 2019, 2020). Diadectomorpha, usually regarded as the sister group of Amniota (within the clade Cotylosauria sensu Laurin and Reisz, 1995), have their first occurrence in the same time interval (e.g., Berman et al., 2010; Kissel, 2010), suggesting a late Mississippian to early Pennsylvanian origin of amniotes (Tuinen and Hadly, 2004; Pardo et al., 2020; Didier and Laurin, 2020). Apart from diadectomorphs, several Paleozoic tetrapod groups have been classified as amniote relatives: Embolomeres, gephyrostegids, seymouriamorphs, and lepospondyls have been regarded as successively more closely related to Cotylosauria according to several morphology-based phylogenetic studies (Vallin and Laurin, 2004; Ruta and Coates, 2007; Clack et al., 2012; Witzmann and Schoch, 2017). For practical reasons, these groups are referred to as “reptiliomorphs” in this study – in agreement with the temnospondyl hypothesis of lissamphibian ancestry. If, however, embolomeres and their allies were more distantly related to amniotes than temnospondyls, Embolomeri would not be part of the clade Reptiliomorpha and if lepospondyls instead of temnospondyls were ancestral to lissamphibians, the amniote stem-group would be much smaller and various groups, including embolomeres, gephyrostegids, seymouriamorphs and lepospondyls would not be part of the Reptiliomorpha (see discussion in Marjanović and Laurin, 2019; it should be noted that lissamphibian ancestry and other aspects of tetrapod evolution after the Cisuralian are not of much relevance for our approach – apart from questions of terminology).
The Carboniferous body fossil record of amniotes and reptiliomorphs is supplemented by a sparse record of trackways and individual footprints (e.g., Haubold et al., 2005; Falcon-Lang et al., 2007; Voigt and Ganzelewski, 2010; Fillmore et al., 2012; Meade et al., 2016; Marchetti et al., 2020b). In the early Permian, the abundance and diversity of reptiliomorph, reptilian and synapsid body fossils and tracks increased (Figure 1). From the Thuringian Forest Basin records of more than ten trackways have been described for several distinct ichnotaxa (see Voigt, 2005: Batrachichnus salamandroides, Amphisauropus kablikae, Ichniotherium cottae, Ichniotherium sphaerodactylum and Dromopus lacertoides), but also from other deposits, such as the Choza, Robledo Mountains and Hermit formations multiple trackways of distinct ichnotaxa have been described (e.g., Haubold and Lucas, 2003; Voigt and Lucas, 2015; Marchetti et al., 2020a). Further records may include well-preserved couples and individual footprints of different ichnotaxa (e.g., Voigt and Haubold, 2015; Mujal et al., 2016; Zouicha et al., 2021), but not a larger sample of trackways (i.e., a succession of footprints that constitute at least one complete step cycle) as a reasonable requirement for functional interpretations.
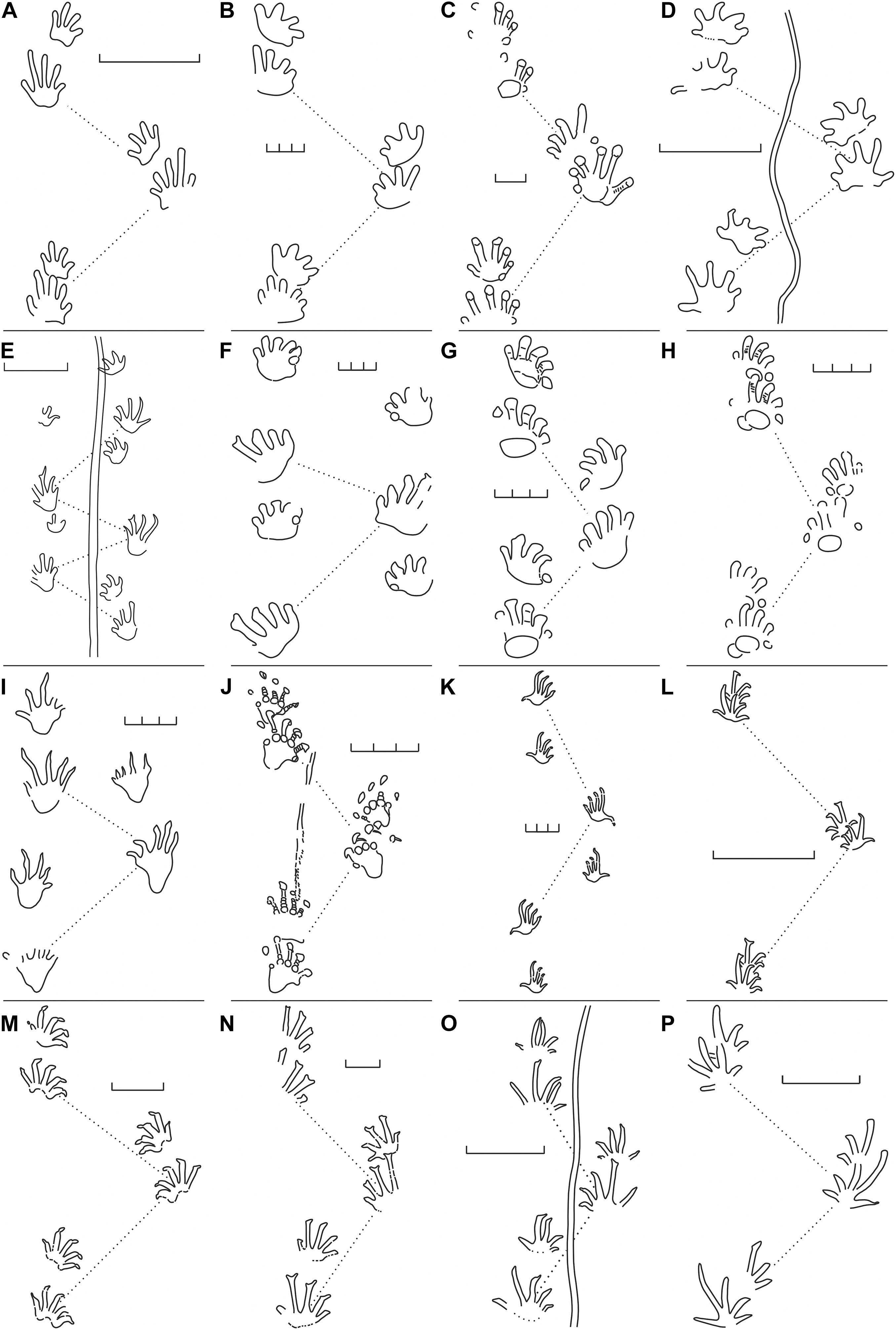
Figure 1. Trackway patterns of Carboniferous and early Permian tetrapod ichnotaxa. (A) Batrachichnus salamandroides, VF-4307/4314, Oberhof Fm, Thuringia/Germany; (B) Limnopus vagus, YPM 405, Howard Fm, Kansas/United States. (C) Hylopus isp., NMMNH P-64276, Mauch Chunk Fm, Pennsylvania/United States; (D) Amphisauropus kablikae, MNG-7813-84, Rotterode Fm, Thuringia/Germany; (E) Matthewichnus caudifer, NMMNH P-23511, Robledo Mts. Fm, New Mexico/United States; (F) Ichniotherium praesidentis, DBM-060003309001-04, Bochum Fm, Nordrhein-Westfalen/Germany; (G) Ichniotherium sphaerodactylum, MNG-1351, Tambach Fm, Thuringia/Germany; (H) Ichniotherium cottae, SSB-1, Tambach Fm, Thuringia/Germany; (I) Dimetropus isp., DBM-060003357001, Bochum Fm, Nordrhein-Westfalen/Germany; (J) Dimetropus leisnerianus MNG-13490, Tambach Fm, Thuringia/Germany; (K) Tambachichnium schmidti, Tambach Fm, Thuringia/Germany; (L) Erpetopus willistoni, NMMNH P–32411, Choza Fm, Texas/United States; (M) Varanopus curvidactylus, NMMNH P–32391, Choza Fm, Texas/United States; (N) Hyloidichnus bifurcatus, USNM 11518, Hermit Fm, Kansas/United States; (O) Notalacerta missouriensis NMMNH P–31746, McAlester Fm, Oklahoma/United States; (P) Dromopus lacertoides, JF-1, Oberhof Fm, Thuringia/Germany. Trackway patterns modified after Voigt (2005) and Voigt et al. (2013). Scale unit: 3 cm.
Since the approaches of Haubold (1970, 1971) and Fichter (1983a; 1983b), track-trackmaker relationships have been clarified for various groups of early amniotes and amniote relatives from the Carboniferous and early Permian (e.g., Voigt, 2005; Voigt et al., 2007; Marchetti et al., 2017, 2019a, 2020c; Mujal and Marchetti, 2020) and provide a robust framework for the biostratigraphic interpretation of tracks (Voigt and Lucas, 2018; Marchetti et al., 2019b; Schneider et al., 2020; Lucas et al., 2021). Some track types with a tetradactyl manus as in extant amphibians, such as Batrachichnus and Limnopus, have been assigned to temnospondyl producers (Haubold et al., 1995; Voigt, 2005), whereas the trackway pattern in Matthewichnus points to a lepospondyl trackmaker (Voigt and Lucas, 2015). Among tracks with a pentadactyl manus, certain morphotypes with blunt digit tips that lack distinct claw traces have been assigned to anamniote reptiliomorph producers. Based on digit proportions and the morphology of heel pad, the following ichnotaxa can be distinguished: Amphisauropus as the assumed track type of seymouriamorphs, Ichniotherium as an assumed diadectomorph track type and Hylopus as the presumable product of more distantly related reptiliomorph (“anthracosaur-grade”) trackmakers, such as embolomeres and gephyrostegids (e.g., Sundberg et al., 1990; Voigt et al., 2007; Marchetti et al., 2017). In alleged early amniote tracks, the manus imprints are pentadactyl, digit tips are pointed and they may display claw traces of varying distinctness. Early synapsid track types are marked by comparatively long heels and paw-like impressions, indicating a large tarsus and the so-called synapsid digital arcade (Kümmell and Frey, 2012; Marchetti et al., 2019a). These features are, however, more distinct in Dimetropus than in Tambachichnium, the alleged track type of varanopids, which shares certain similarities with reptilian (sauropsid) tracks (e.g., Voigt, 2005; some recent phylogenetic analyses place varanopids in the Reptilia, see Ford and Benson, 2020; Modesto, 2020). The earliest Pennsylvanian records of presumptive reptile tracks include Notalacerta, which can be distinguished from many later-occurring types by its continuous body drag trace and it is regarded as the product of basal eureptiles and protorothyridid eureptiles (Chesnut Jr., et al., 1994; Marchetti et al., 2020b). Early Permian track types assigned to reptiles vary considerably in their toe proportions, trackway patterns and in the morphology of the proximal part of their imprints. Common ichnotaxa include Erpetopus, which has been interpreted as parareptile track type (Marchetti et al., 2019b); Varanopus, which has been referred to captorhinid (Haubold, 1971; Fichter, 1983b; Voigt, 2005) or parareptile producers (Müller, 1954; Haubold, 1971; Haubold and Lucas, 2001; Schneider et al., 2020), Hyloidichnus as a presumable captorhinid track type (Voigt et al., 2010) and Dromopus, whose lacertoid imprint morphology has been mostly likened to araeoscelid diapsids (Haubold, 1971; but see Spindler et al., 2019 for a further interpretation as varanopid tracks). In this study, we cover all of the above-named ichnogenera, sometimes with samples from distinct time slices and localities, and include certain well-known ichnospecies of Ichniotherium as separate units (Table 1).
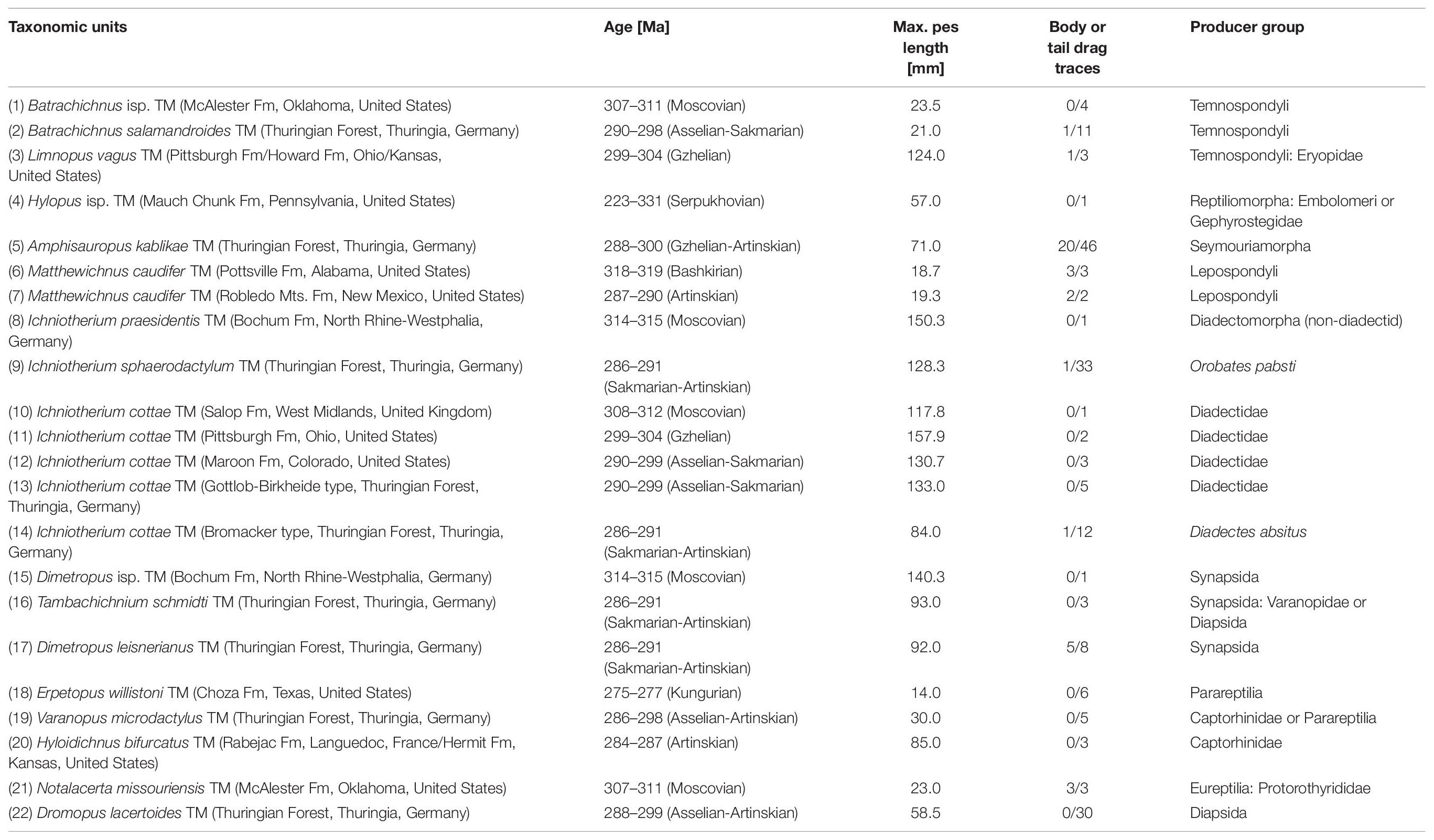
Table 1. List of trackmakers (TMs) used as taxonomic units for the ancestral state reconstruction approach and the respective age range, maximum pes length, abundance of body or tail drag traces and assumed producer group.
Aspects of locomotion and evolutionary change close to the origin of amniotes have been discussed based on individual trackway samples of diadectomorphs (e.g., Voigt and Haubold, 2000; Voigt et al., 2007; Voigt and Ganzelewski, 2010; Buchwitz and Voigt, 2018; Mujal and Marchetti, 2020), early reptiles (Haubold and Lucas, 2001, 2003; Haubold et al., 2005; Gand and Durand, 2006; Bernardi and Avanzini, 2011; Curth et al., 2014; Marchetti et al., 2014, 2020a, 2020b) and synapsids (Hunt and Lucas, 1998; Voigt, 2005; Gand and Durand, 2006; Voigt and Lucas, 2015; Lucas et al., 2016), documenting the occurrence of relatively narrow gauges and lack of belly or tail drag traces in many Paleozoic ichnotaxa. One conclusion from these approaches was that sprawling gaits of early amniotes and their closest relatives were not necessarily “primitive” but covered a certain spectrum and included semi-upright postures (Lucas, 2019). Especially the recent biomechanical models of Nyakatura et al. (2015, 2019) for the diadectid O. pabsti from the middle Cisuralian Bromacker locality as the trackmaker of the abundant co-occurring I. sphaerodactylum tracks represented a step forward toward a more comprehensive interpretation of tracks and trackmakers. Among others, their approach of simulating gaits by means of digital and robotic Orobates models (based on CT scans of fossil skeletons) – with the constraint that it shall fit the trackway pattern of I. sphaerodactylum – demonstrated that some postures and body movements, such as crawling, a high degree of sprawling or high trunk flexion, are energetically and structurally disadvantageous and thus can be ruled out. Comparison of the modeled Orobates walking cycles with those of extant model animals, such as salamanders, lizards and crocodiles, provided some indications to what degree their locomotion styles are comparable to those of the fossil trackmaker and, more generally, to what degree the actual variability of amphibian and reptile locomotion is reflected by the observed trackway pattern.
For Paleozoic tetrapod trackways, the same standardized measures as in most extant trackways are used (see Haubold, 1971; Leonardi, 1987), namely pace length, pace angulation, stride length, gauge width, orientation of the imprint with respect to the direction of progression, “apparent trunk length” (supposed glenoacetabular length), and distance between successive pes and manus imprints parallel and perpendicular to the direction of movement (Figure 2). The base of digit III represents the reference point to measure distances between footprints and the orientation of digit III close to the base represents the reference orientation, which is compared to the direction of progression (which can be inferred through reconstruction of a trackway midline). In the case of a homogeneous symmetric trackway pattern of a quadruped, there are no more than six degrees of freedom (not including imprint sizes) and several measures provide redundant information – among others, gauge width and stride length can be calculated from pace length and pace angulation. This kind of redundancy must be considered in the interpretation of trackway data, especially when data analysis methods are applied.
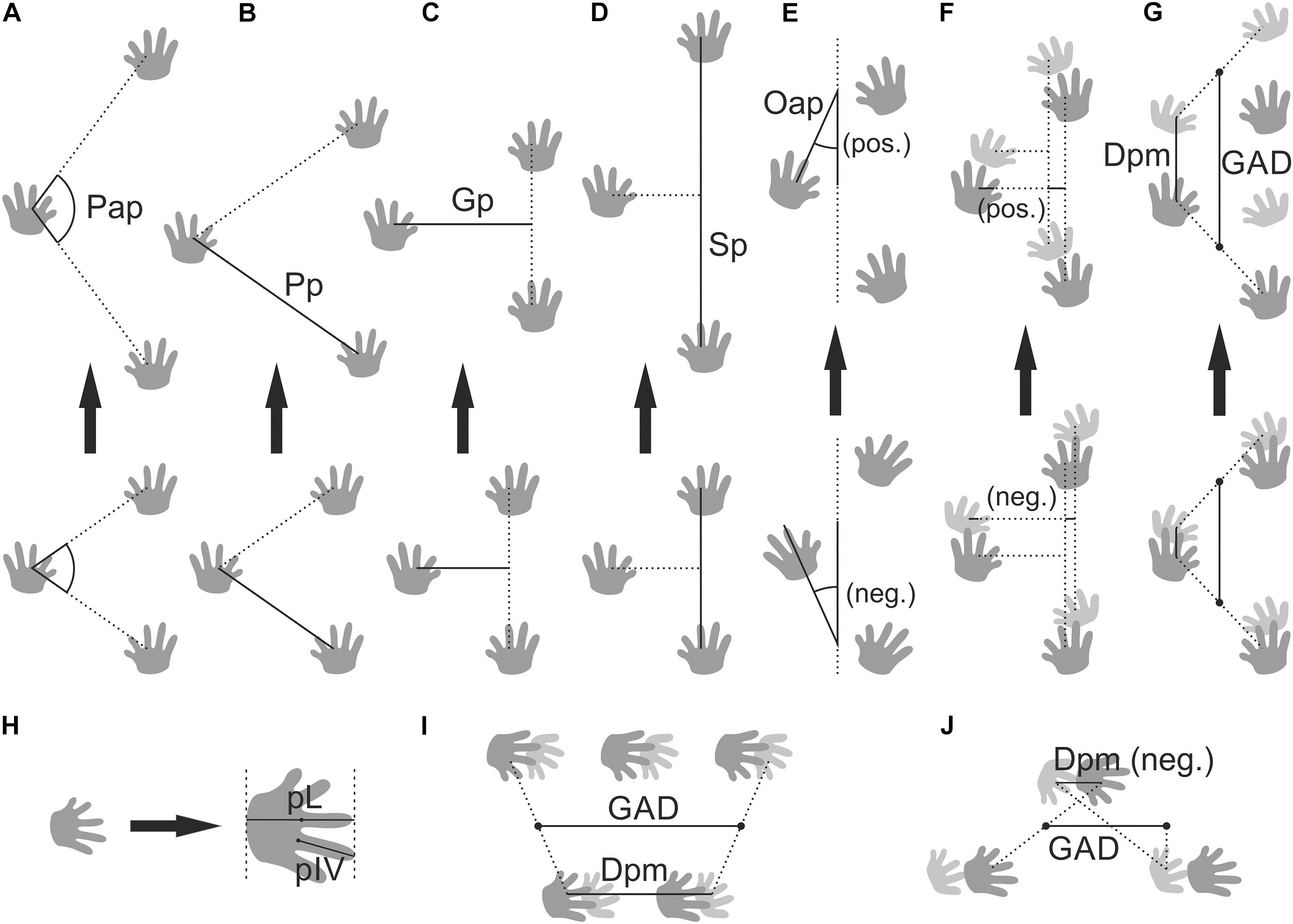
Figure 2. Trackway measures and footprint length. (A) Pedal pace angulation (Pap), (B) pedal pace length (Pp); (C) pedal gauge width (Gp); (D) pedal stride length (Sp); (E) orientation of the pes imprints with respect to trackway midline (Oap); (F) difference between manual and pedal gauge width; (G) along-track distance between pes and manus (Dpm) and supposed glenoacetabular distance (GAD) for “primitive” walking trot; (H) pes length (pL) and length of pedal digit IV (pIV); (I) pes/manus distance and supposed glenoacetabular distance as it has been measured for Matthewichnus caudifer (coupling value close to 2.0); (J) pes/manus distance and supposed glenoacetabular distance as it has been measured for Tambachichnium schmidti (lateral sequence walk, notable primary overlap).
Studies of trackway data that were aiming at the interpretation of evolutionary change at a larger scale are sparse: In a stratigraphic approach considering Permian and Triassic tetrapod trackways in their entirety, Kubo and Benton (2009) documented an increase in pace angulation from the Permian through the Triassic, which was interpreted as an indicator for the rise of derived gaits and erect postures in certain groups, such as therapsids and archosaurs. With a similar type of study – the comparison of a certain measure for trackway samples from different time slices, Kubo and Kubo (2013) found an increase in normalized stride, indicating speed increases, within certain archosauriforms trackmaker groups during the Triassic. Another example is the inference of gait and postural changes in the evolution of sauropod dinosaurs based on changes in gauge width and imprint orientation in sauropod trackways (Carrano and Wilson, 2001; Henderson, 2006; Lallensack et al., 2018). Trackway data have also been raised for the tracks of extant crocodiles and desert monitor lizards to assess their intraspecific and interspecific variability and compare them with the fossil record of reptile footprints and trackways (Farlow and Pianka, 2000; Farlow et al., 2018).
With the rise of quantitative phylogenetic approaches in vertebrate paleontology since the 1980s, individual assignments of ichnotaxa to certain fossil trackmaker groups has been amended by relating ichnotaxa and certain changes of ichnological features to phylogenetic trees and trackmaker taxa defined in accordance with phylogenetic systematics. Especially the tracks of Triassic archosaurs and their relatives, which underwent a rapid change in their locomotion apparatus including the multiple independent evolution of bipedalism, have been interpreted phylogenetically (Haubold and Klein, 2002; Brusatte et al., 2011; Bernardi et al., 2015). Another example are sauropodomorph dinosaurs, whose peculiar adaptation to gigantism in the course of their phylogeny is also visible in their tracks (Wilson, 2005). The idea that tetrapod footprint ichnotaxa can be sorted phylogenetically and assigned to certain clades of a skeletal-morphology-based phylogenetic tree according to the occurrence of certain diagnostic features in both, track and trackmaker morphology, has been termed “synapomorphy-based track-trackmaker correlation” by Carrano and Wilson (2001) and is discussed in several recent approaches on Paleozoic tracks (e.g., Voigt et al., 2007; Marchetti et al., 2017, 2019a, 2020c; Buchwitz and Voigt, 2018; Mujal and Marchetti, 2020).
Mapping features on a phylogenetic tree and inferring character states in common ancestors of terminal taxa, whose character states are known, represents a standard way of addressing character evolution and has been introduced to paleontology together with cladistic methods. Quantitative methods of ancestral state reconstruction include parsimony approaches, which may be used for characters with discrete character states, and maximum likelihood approaches, which are often used when character states are continuously distributed and ages of nodes and terminal taxa are known or can be estimated (Joy et al., 2016; Royer-Carenzi and Didier, 2016). Previous applications in vertebrate paleontology include inference of lifestyle (e.g., Kriloff et al., 2008) the reconstruction of ancestral basic metabolic rates and genome size based on osteohistological features (Organ et al., 2007; Legendre et al., 2016), soft body characteristics (e.g., Barrett et al., 2015; Yang et al., 2019), body size (e.g., Laurin, 2004; Benson et al., 2014; Lee et al., 2014; Brocklehurst and Brink, 2017), and paleogeography (e.g., Poropat et al., 2016; Borths and Stevens, 2017).
In this study, we employ ancestral character estimation based on maximum likelihood – a method, which has not yet been attempted in vertebrate ichnology. Based on (a) trackway measurement data taken from a sample of trackways assigned to a certain set of related footprint ichnotaxa, (b) track-trackmaker correlation based on imprint morphology and (c) a known skeletal-morphology-based phylogeny, we infer ancestral states for (1) maximum pes length (as an indicator for maximum body size), (2) abundance of body or tail drag traces and (3) standard trackway measures, which, taken together, constitute a trackway pattern (Figure 2). Given that ichnotaxa cannot evolve themselves but merely reflect evolution in their producers, we devise the concept of a hypothetical trackmaker (=TM) as a taxonomical unit, which is assumed to represent a monophyletic or paraphyletic group of track producers with a homogeneous locomotion (Figures 3A,B). Given the relative character paucity of tetrapod tracks, trackmaker phylogenies cannot be expected to reach resolutions comparable to those of trees based on skeletal morphology. For certain critical nodes that mark the origin of major clades, however, corresponding nodes do occur on a less well resolved trackmaker tree and for these nodes, the state of locomotion-dependent trackway measures is worth targeting. Certain outcomes of our analysis – e.g., distribution averages for certain trackway measures at certain nodes – can be depicted as a color ramp or gray scale imposed upon the trackmaker tree (Figure 3C) or as binary plots, which feature trackway parameter variability as a function of time (Figure 3D).
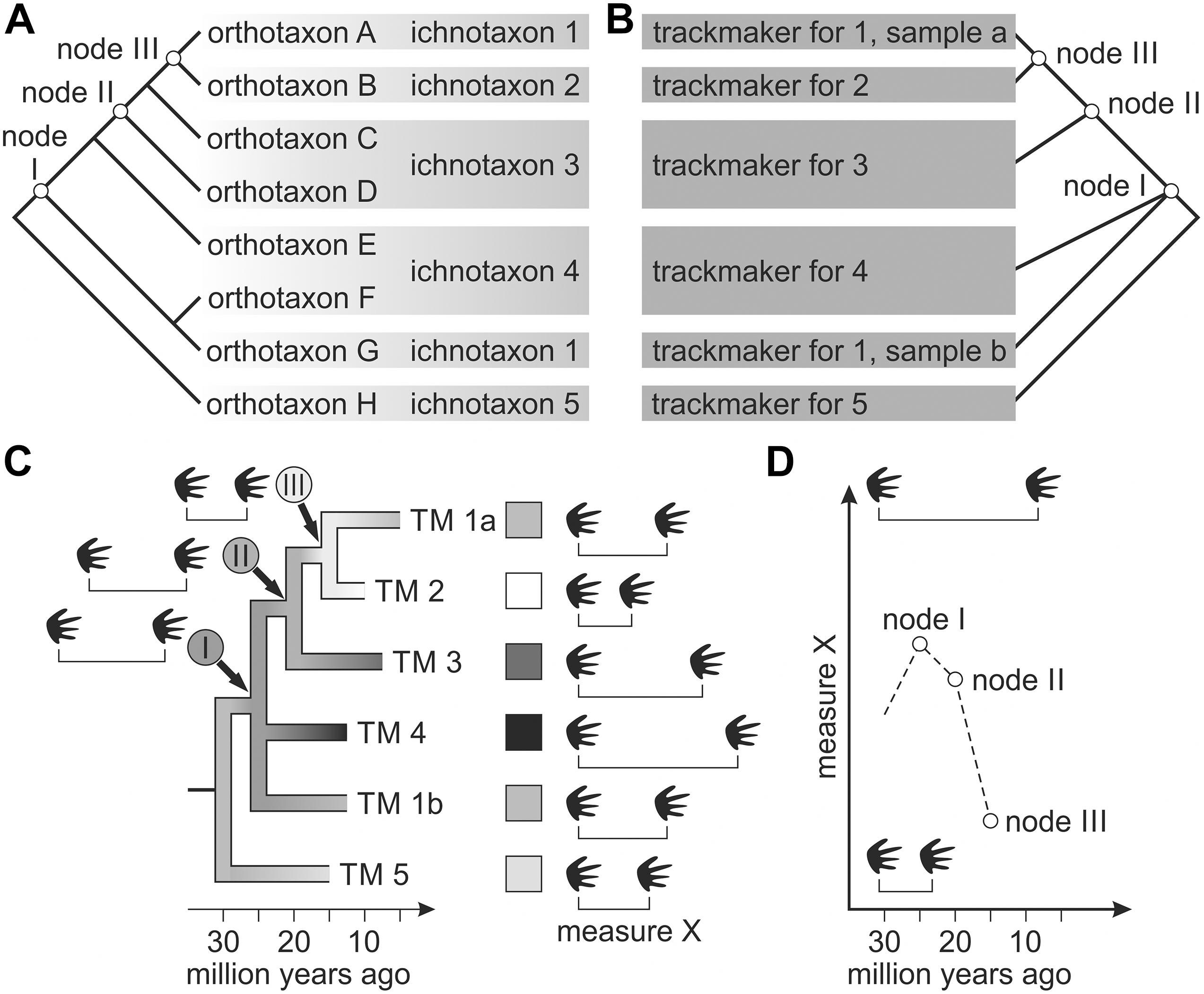
Figure 3. Methodological approach for phylogenetically informed analysis of tetrapod trackway data. (A) Correlation of ichnotaxa with certain orthotaxa; (B) trackmaker tree inferred from track-trackmaker correlation; (C) reconstruction of ancestral states for trackway measure X at nodes I-III; (D) reconstructed evolutionary changes in trackway measure X depicted as a function of time.
With a sample of trackways assigned to 13 ichnogenera that have been related to a skeletal-morphology-based trackmaker tree with 22 trackmakers as taxonomical units, covering the ancestry and early diversification of amniotes, our present ancestral state reconstruction approach addresses the following research questions:
(1) How much do trackway measures vary between common ancestors of early amniotes and what kinds of evolutionary change can be observed?
(2) Are the observed changes in the trackway pattern indicative for evolutionary changes of locomotion among amniote ancestors?
(3) What conclusions can be drawn regarding the locomotion in the last common ancestor of amniotes (LCAA)? Was it similar to certain trackmakers covered by our late Carboniferous to early Permian trackway sample?
(4) Which methodological implementations had an influence on the outcome and does that matter for future studies of early amniote tracks or other problems of vertebrate ichnology?
Materials and Methods
Materials
Starting with a sample of 148 trackways assigned to early Permian ichnotaxa from the relatively large trackway record of the Thuringian Forest Basin (see list of Voigt, 2005, Appendix 21), we included further material to reach a better coverage of relevant tetrapod groups surrounding the origin of amniotes (Table 1 and Supplementary Material 1 includes the list of institutional abbreviations):
1. Ichniotherium trackways from the Pennsylvanian of Germany (DBM-060003309001-04), Britain (LMG-2471) and Ohio (OSU-16553, CMC-VP-3052) and a trackway sample from the Cisuralian of Colorado (DMNS-50618, 50622, 55056) (Carman, 1927; Schmidt, 1956; Haubold and Sarjeant, 1973; Voigt et al., 2005; Voigt and Ganzelewski, 2010; Buchwitz and Voigt, 2018);
2. Material of track types referred to other anamniote producers, namely Hylopus from the late Mississippian of Pennsylvania (NMMNH P-64276) the ichnotaxa Limnopus (CMC-VP-791, YPM-205, YPM-405), Batrachichnus (NMMNH-P-31676, NMMNH-P-31677, and NMMNH-P-31757) from Pennsylvanian deposits and Matthewichnus (UCM 060, 469, 1878, NMMNH P-23312, 23511) from Pennsylvanian and Cisuralian deposits of the United States (Baird, 1952; Lucas et al., 2004; Haubold et al., 2005; Fillmore et al., 2012; Voigt and Lucas, 2015);
3. Notalacerta missouriensis, the earliest occurring track type of eureptile tracks with a trackway sample from the Pennsylvanian of Oklahoma (NMMNH P-31705, 31746, 31759) and Dimetropus isp. (DBM-060003357001) from the Pennsylvanian of Germany as one of the earliest reported synapsid trackways (Lucas et al., 2004; Voigt and Ganzelewski, 2010; Marchetti et al., 2020b);
4. Samples of the relatively younger ichnotaxa Erpetopus willistoni (NMMNH P-32400, 32411, 32478, 32487, 40196, 46905) and Hyloidichnus bifurcatus (ML-54L, ML-not-assigned, USNM 11518) from the Cisuralian of the United States and France in order to cover parareptile and captorhinomorph producers (Haubold and Lucas, 2001, 2003; Gand and Durand, 2006; Marchetti et al., 2020a).
The age of the fossil-bearing units is based on the most recent chronostratigraphic interpretations (e.g., Schneider et al., 2020; Lützner et al., 2021). Our aim was to include at least three trackways per ichnotaxon and locality, but in some cases only one or two specimens were at hand. Sometimes not all available trackways were preserved well enough to be included in the quantitative approach.
Methods
The graphic documentations of trackways and measurements were carried out between 1996 and 2020 (by S.V.), following the procedure outlined in Voigt (2005). Accordingly, measurements of the supposed glenoacetabular length (“apparent trunk length”) were taken following the assumption of a walking trot (sensu Hildebrand, 1980; Figure 2G, “primitive alternate pace” in Leonardi, 1987, plate 8C), even though a low degree of primary overlap between manus and pes has been observed in some ichnotaxa. Exceptions were only made for extreme deviations in the trackway patterns of Tambachichnium schmidti and Matthewichnus caudifer (Figures 1C,J). In case of Matthewichnus, which was probably produced by lepospondyls with elongated trunks, a higher coupling value with occasional secondary overlap was assumed and for the measurement of glenoacetabular length one stride length was added (in comparison to primitive walking trot and a coupling value of 1.0; see Figure 2I). Assuming that the trackmaker of Tambachichnium placed the pes in front of the manus during the same step cycle, the glenoacetabular length was adapted according to the formula suggested by Soergel (1925) for lateral sequence walk (Figure 2J, see also plate 8D in Leonardi, 1987).
For all measured variables, we calculated trackway mean values (see Supplementary Material 2). Additionally, all of the (averaged) length measures describing the trackway pattern were normalized using (average) pes length as denominator. In case of three or more processable trackways per taxonomical unit, we have defined a centerpoint trackway (multivariate median) to represent the whole sample of trackways in the following ancestral state approach. The inference of this trackway is based on a combination six of trackway measures (normalized pedal stride length, normalized pedal gauge width, normalized difference between pedal and manual gauge width, normalized glenoacetabular length, imprint orientation of the pes with respect to trackway midline, imprint orientation of the manus with respect to the pes orientation). For all trackways of a given TM sample, the values for each of the six variables were centered and reduced (i.e., the mean is substracted from the value and the result is divided by the variance), after which the euclidean distance to the centroid (which is thus at zero) was measured for each complete trackway. The trackway specimen with the lowest distance to the centroid was then picked as representative of the ichnospecies.
The phylogeny of trackmakers is based on common hypotheses about amniote relationships (following Ruta et al., 2003a; Ruta and Coates, 2007; but see also revision of these datasets and analyses by Marjanović and Laurin, 2019) and the track-trackmaker correlations introduced above (see introduction and Table 1). Certain first occurrences of trackmaker groups are based on skeletons and/or tracks and assumed divergence ages for major clades. Representing the supposed trackmakers of Matthewichnus tracks, lepospondyls are included as a group more closely related to amniotes than embolomeres and seymouriamorphs (as discussed by Carroll, 1995; Laurin and Reisz, 1997; Ruta et al., 2003a), but less closely related than diadectomorphs, which form the sistergroup of amniotes within the Cotylosauria (Laurin and Reisz, 1995; Reisz, 2007). The divergence age for the clade Lepospondyli + Cotylosauria was set at 344 Ma, because some lepospondyl groups, such as adelospondyls and aïstopods, occur in the Viséan (Andrews and Carroll, 1991; Anderson et al., 2003). In accordance with the first occurrence of presumable diadectomorph and synapsid tracks in the earliest Moscovian (Voigt and Ganzelewski, 2010), the Bashkirian (early Langsettian), the age of the reptile Hylonomus and reptile footprint Notalacerta (e.g., Calder et al., 2006; Marchetti et al., 2020b) and previous assumptions about amniote origins (see discussion in Didier and Laurin, 2020; Pardo et al., 2020), the ages of the clades Cotylosauria and Amniota have been set close to the Mississippian/Pennsylvanian boundary at 326 and 323 Ma, respectively. Other major nodes (Table 2) have been set following a predefined age distance of three million years; for the time span between a node and the center of the ranges of terminal taxa, a minimum age difference of three million years has been implemented.
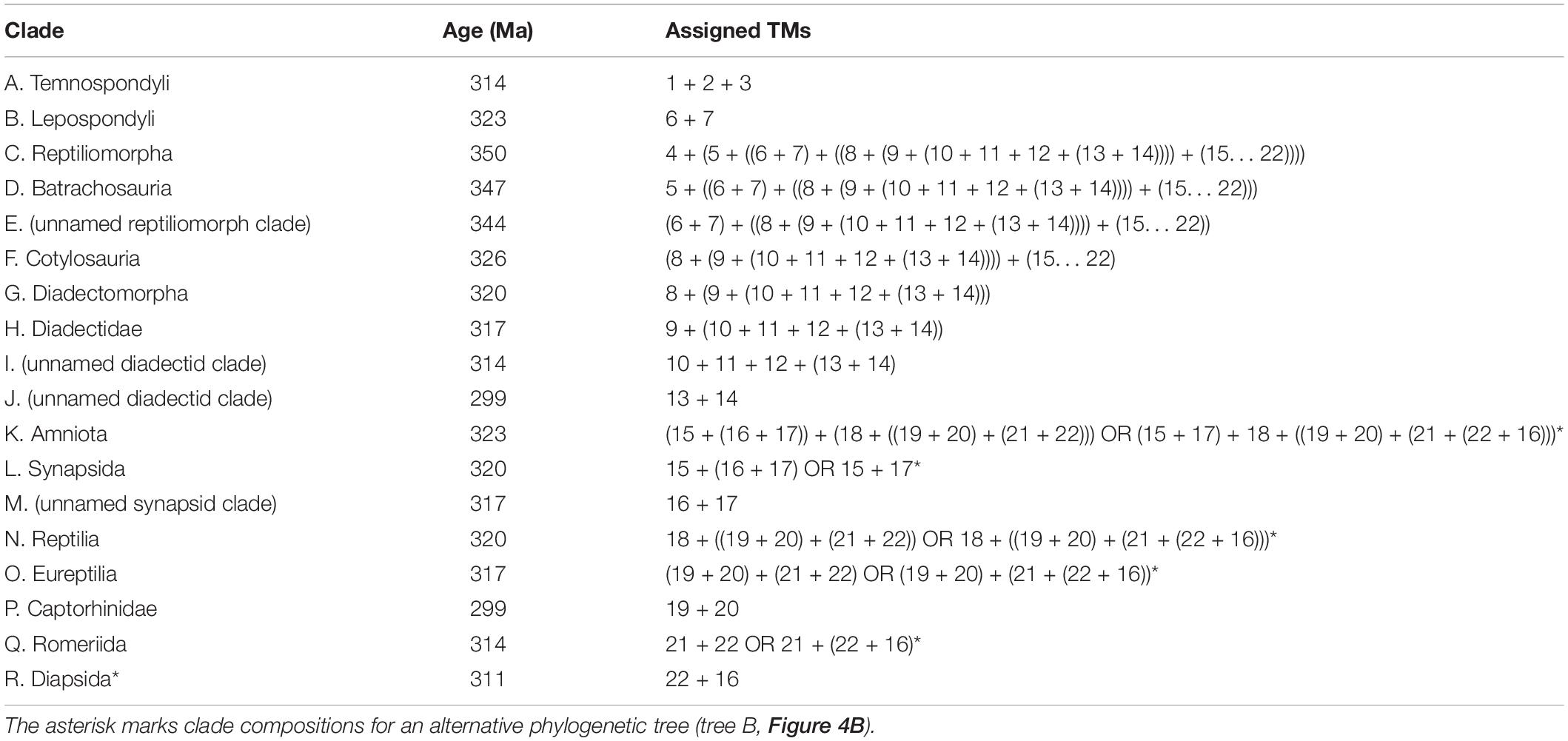
Table 2. Clades of the assumed phylogenetic tree of trackmakers with formulas for clade composition.
The trackmakers of the three temnospondyl trackway samples have been included as an unresolved trichotomy (node A, Temnospondyli). Internal relationships of the Ichniotherium trackmakers are based on Buchwitz and Voigt (2018, Figure 18), but Pennsylvanian Ichniotherium cottae trackways from Britain and Ohio are herein considered as separate units and a sample from the Maroon Formation of Colorado has been added to reach a better overall representation of diadectomorph tracks. The internal relationships of synapsid trackmakers have been set according to the assumption that T. schmidti and Dimetropus leisnerianus from the Tambach Formation of the Thuringian Forest were produced by more closely related synapsid groups than the earliest synapsid tracks from the Bochum Formation, which were referred to Dimetropus isp. Phylogenetic analyses of early reptiles have found the groups Parareptilia, Captorhinomorpha, and Protorothyrididae to be successively more closely related to Diapsida (e.g., Müller and Reisz, 2006; but see alternative placement of parareptiles in Laurin and Piñeiro, 2017; Ford and Benson, 2020) and the relationships of the trackmakers for Erpetopus, Varanopus, Hyloidichnus, Notalacerta, and Dromopus have been ordered accordingly. Considering the early occurrence of supposed basal eureptile and protorothyridid tracks (ichnogenus Notalacerta) and body fossils, the divergence ages of the clades Reptilia (Sauropsida), Eureptilia, and Romeriida fall in the range between 320 and 314 million years.
Given its small influence on the reconstruction of ancestral amniote character states, the alternative that Varanopus microdactylus represents a parareptile (e.g., Müller, 1954; Haubold, 1971; Haubold and Lucas, 2001) instead of captorhinomorph trackmaker (e.g., Fichter, 1983b; Voigt, 2005) has not been considered in the following analytical approach. Because some phylogenetic analyses found that varanopids were diapsid relatives instead of synapsids (Ford and Benson, 2020; Modesto, 2020) and Tambachichnium tracks share a certain similarity to presumable diapsid track types, such as Dromopus (e.g., Voigt, 2005), a second set of analyses has been performed based on a modified tree with Tambachichnium trackmakers as the sistergroup to Dromopus trackmakers (tree B, Table 2 and Figure 4B). Consideration of other alternatives, especially different hypotheses concerning diadectomorph relationships (e.g., Berman et al., 1992; Berman, 2013; Marjanović and Laurin, 2019) and certain alternative producer group assignments for the ichnotaxa Ichniotherium praesidentis, Hylopus, and Notalacerta, is not in the scope of this study and shall be addressed elsewhere.
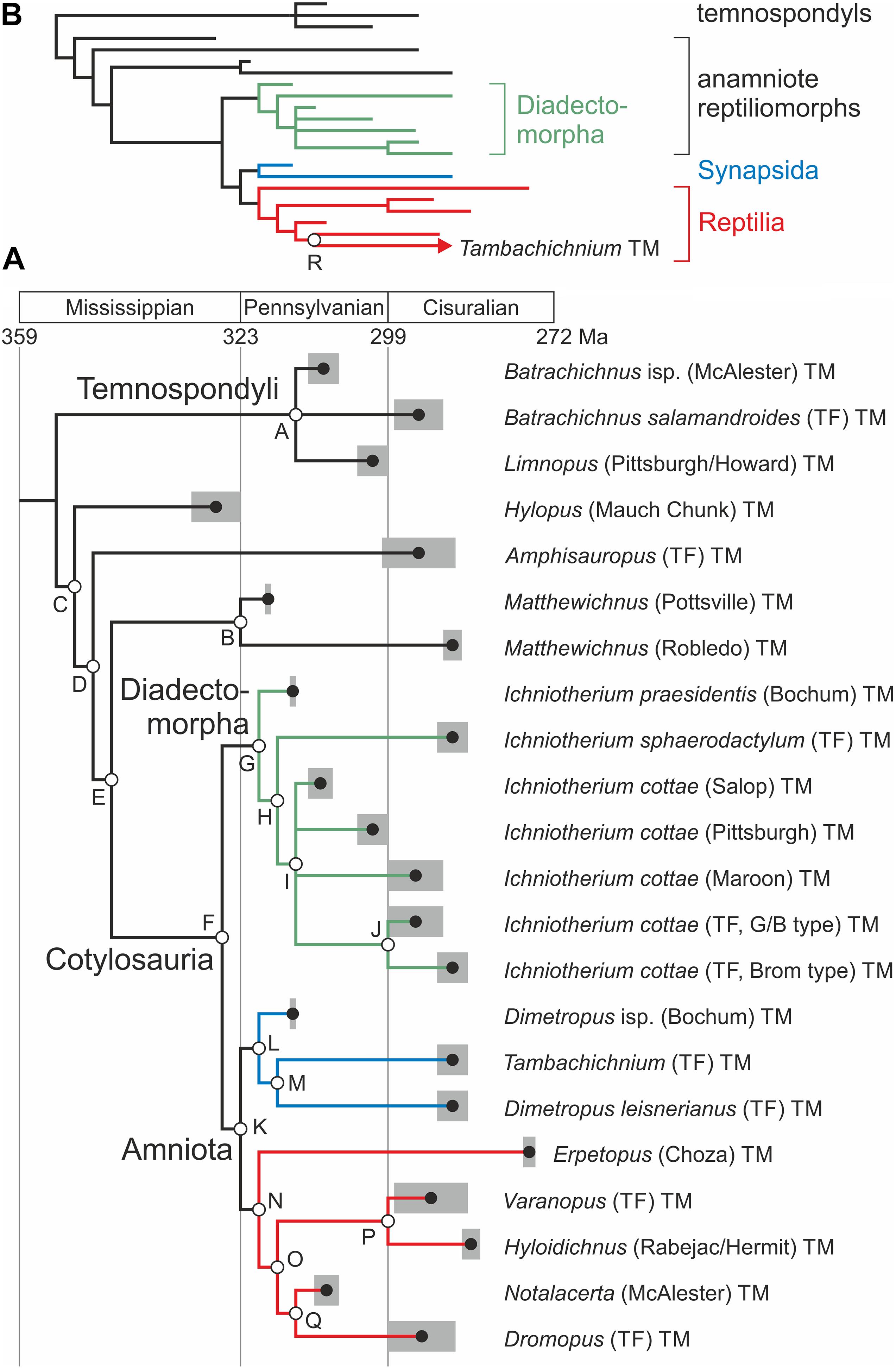
Figure 4. Trackmaker phylogeny used as input for the ancestral state reconstruction. (A) Time-calibrated tree with gray-colored age ranges and individual nodes labeled (A–Q). (B) Simplified alternative tree, including the Tambachichnium trackmaker within the group Diapsida (clade R).
Ancestral states were reconstructed for each character (apart from the abundance of medial tail/body drag traces, see below) based on the centerpoint track of each taxonomical unit using a Brownian motion model fitted by Maximum Likelihood (Felsenstein, 1973; Schluter et al., 1997), as implemented by function ‘reconstruct’ in the R package ‘ape’ (Paradis and Schliep, 2019). The pes length and the length of pedal digit IV were log-transformed before the computation. The overall likelihood was computed (by summing up the likelihood of the tree for each of the reconstructed characters) for each of the two tested trees. The trees were compared by means of the Akaike Information Criterion (AIC and ΔAIC; Akaike, 1974; Burnham and Anderson, 2002) in order to test, which of the two considered phylogenetic hypotheses fits best with the observed measurements. In order to assess the effect of polymorphism and, more generally, intraspecific variability on those reconstructions, we attempted a bootstrapping approach (Efron, 1979) in order to estimate the distribution of each character at each node: a random specimen was picked as representative for the ichnospecies, and the ancestral state of the character was recalculated using the same methodology as above based on that specimen. The process was repeated 10,000 times (results are presented as box-and-whisker plots in Figure 5; Tukey, 1977). Mean values of the resulting distributions were used for the graphic depiction of character evolution as color ranges on tree schemes (Figures 6, 7).
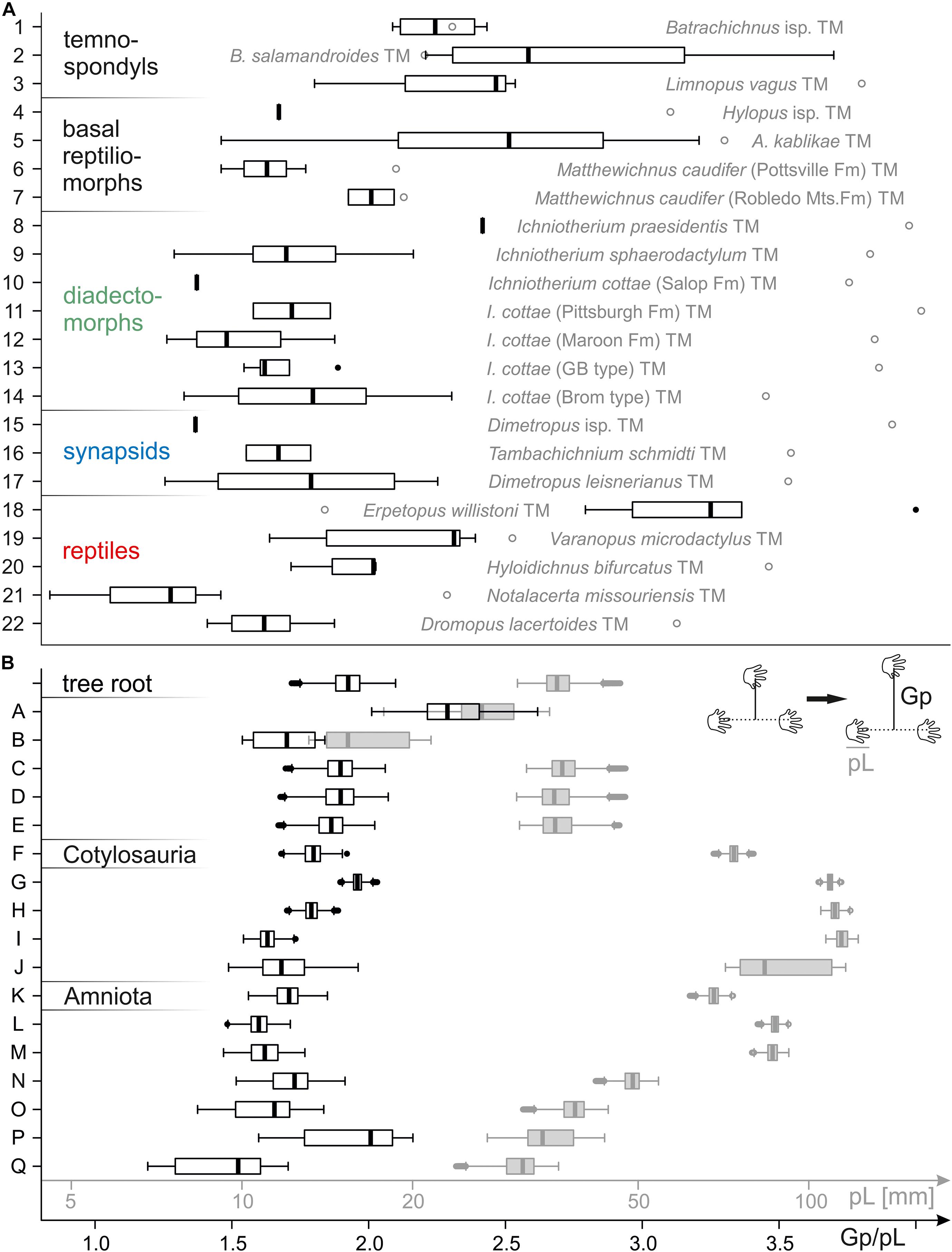
Figure 5. Box-and-whisker plots depicting variation in normalized pedal gauge width (Gp/pL, black) and pes length (pL, gray). (A) Plots for all taxonomical units (TMs 1–22). Instead of a pes length distribution, only maximum pes length is indicated by a gray empty circle. (B) Plots depicting the distributions inferred for nodes A–Q of trackmaker tree A (see Figure 4A) in a bootstrapping approach (based on nodal reconstructions with consideration of random specimens for TMs 1–22).
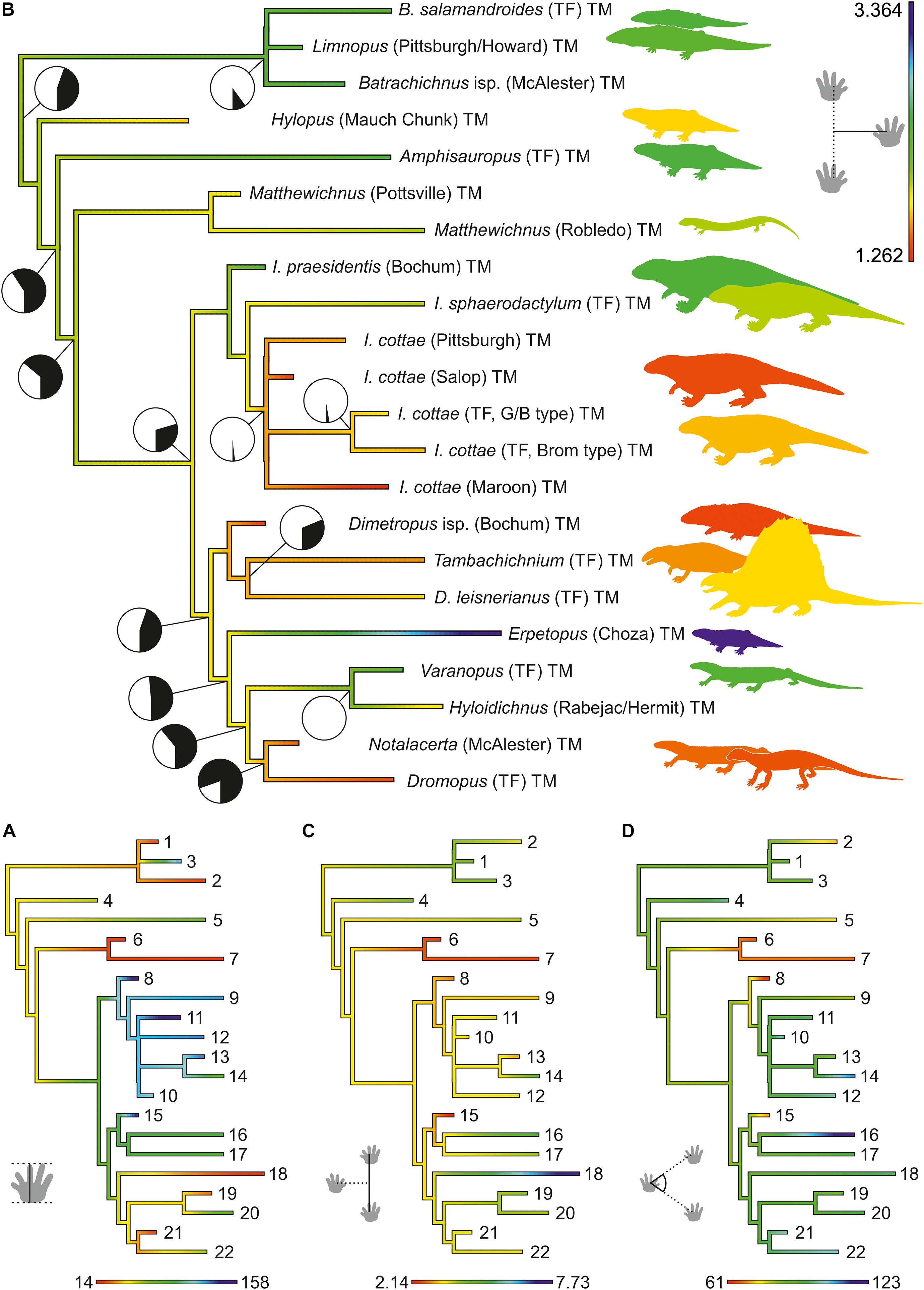
Figure 6. Evolutionary change in estimated ancestral character states superimposed on a phylogenetic tree of trackmakers (tree A; Figure 4A). By means of a bootstrapping approach, character distributions have been inferred for all nodes and characters (see distribution ranges in Supplementary Material 2). The schemes depict changes in the mean values for following characters: (A) normalized pedal gauge width (color range and silhouettes) and abundance of medial drag traces (pie charts); (B) maximum pes length (in mm); (C) normalized stride length; (D) pace angulation for the pes imprints (in degree). Numbering of terminal taxa as in Table 1; data are listed in Table 3.
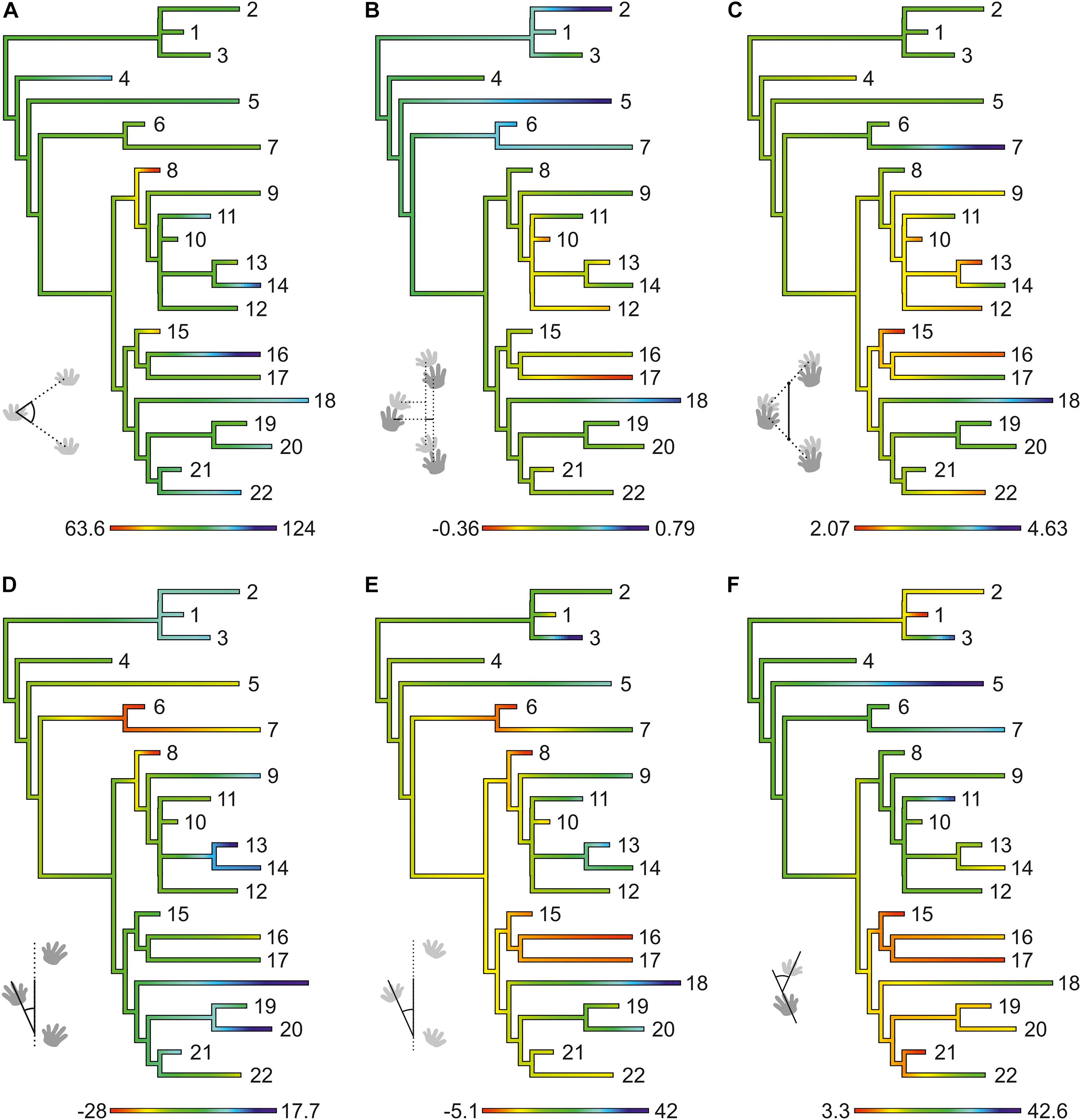
Figure 7. Evolutionary change in estimated ancestral character states superimposed on a phylogenetic tree of trackmakers (tree A). By means of a bootstrapping approach, character distributions have been inferred for all nodes and characters (see distribution ranges in Supplementary Material 2). The schemes depict changes in the mean values for following characters: (A) pace angulation for the pes imprints (in degree); (B) difference between normalized gauge width for the manus and pes imprints (positive values = inward-positioned manus), (C) normalized glenoacetabular length; (D,E) imprint orientations of the pes and manus imprints (in degree; positive values = inward rotation); (F) deviation between manus and pes orientation (in degree). Data are listed in Table 4.
The percentage of observed specimens with drag traces per taxonomical unit has been considered as indicative for the probability of finding such a trace in common ancestors and accordingly the occurrence probabilities are reconstructed (for individual nodes of the trackmaker tree) using independent phylogenetic contrast (Zaneveld and Thurber, 2014), as implemented in the R package ‘castor’ (Louca and Doebeli, 2017).
In order to find trackmakers with a locomotion similar to the supposed LCAA, we compare all trackway patterns used as input data to the ancestral state modeled for the node Amniota by measuring the Euclidean distance between each included specimen and the reconstructed character set for the node Amniota and rank the specimens from closest to furthest (Supplementary Material 2).
Results
Mean values of the character distributions, which have been inferred for all trackway characters and nodes based on nodal reconstructions (and a bootstrapping approach; see distribution ranges in Figure 5 and Supplementary Material 2), can be compared in order describe patterns that may be related to evolutionary changes in locomotion: In the amniote stem-group, maximum pes length increases toward the last common ancestor of cotylosaurs, remains on a high level during the divergence of diadectomorphs, synapsids and reptiles and then appears to decrease independently within certain clades of these groups (Table 3 and Figures 6A, 8A), most notably within reptiles. A similar pattern has been found for the other two imprint measures correlating with body size (pes length of the centerpoint specimen and length of pedal digit IV, Supplementary Material 2). The occurrence of medial belly or tail drag traces becomes less probable toward the last common ancestor of amniotes and diadectomorphs (decrease from >60 to 30% probability between nodes E and F), but the modeled abundance of such traces re-increases and ranges between 45 and 50% in the LCAA (node K; Table 3 and Figures 6B, 8B).
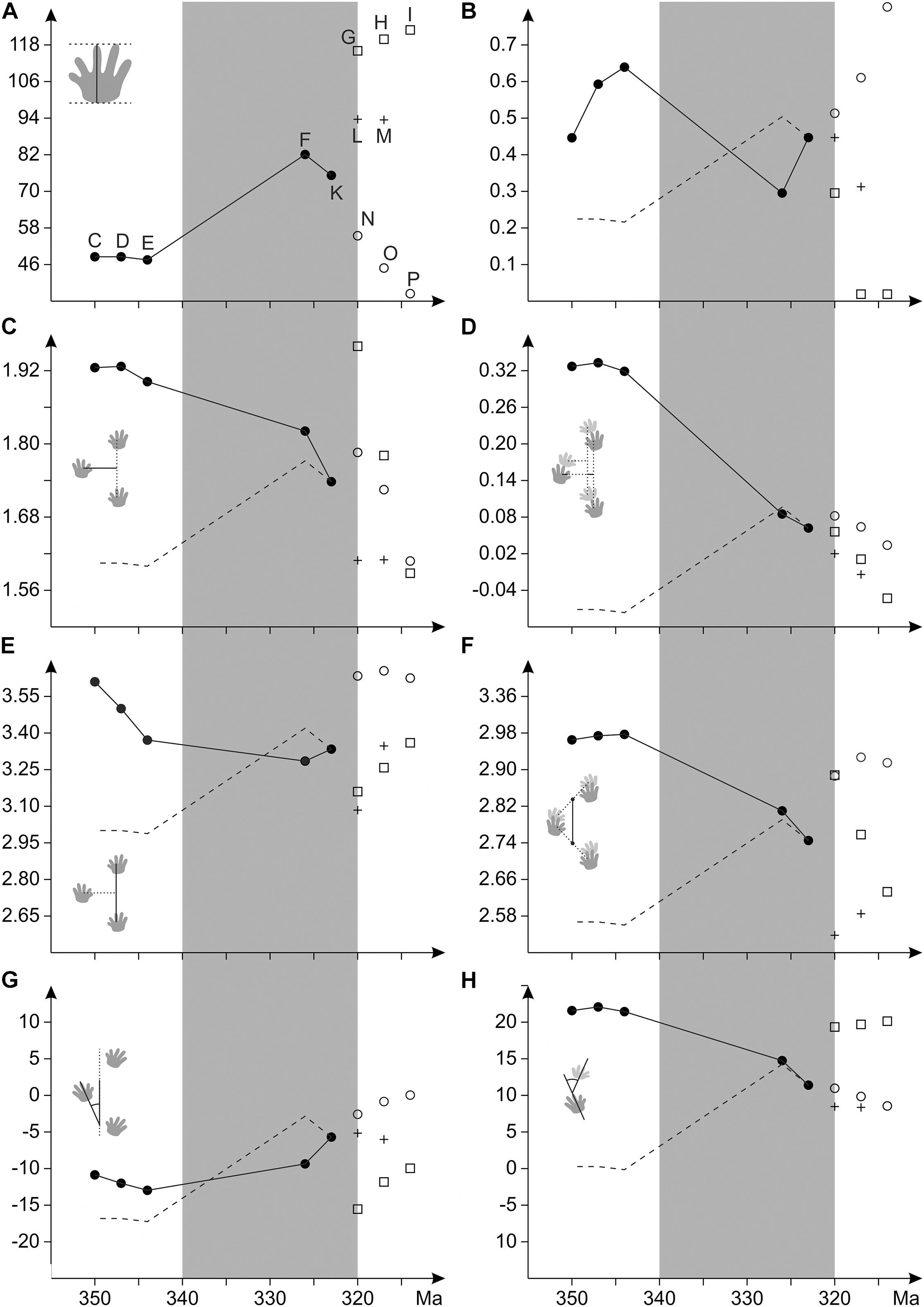
Figure 8. Evolutionary change in estimated ancestral states of trackway characters as a function of time. By means of a bootstrapping approach, character distributions have been inferred for all nodes and characters (see distribution ranges in Supplementary Material 2). The plots depict changes the in mean values for following characters: (A) maximum pes length (mm); (B) abundance of medial drag traces; (C) normalized pedal gauge width (G/pL); (D) difference between normalized gauge width for the manus and pes imprints [(Gp-Gm)/pL]; (E) normalized pedal stride length (Sp/pL); (F) normalized glenoacetabular length (GAD/pL); (G) orientation of the pes imprints (Oap in degree; negative values = outward orientation); (H) deviation between manus and pes orientation (Oam-Oap, in degree). The dashed line shows the trend in maximum pes length (as body size proxy). The time span between 340 and 320 Ma is gray-shaded. Nodes of the trackmaker tree (Figure 4A) are labeled in (A); full circle – amniote stem group, square – Diadectomorpha, cross – Synapsida, empty circle – Reptilia.
Trackway measures that describe the succession of pes imprints display the following pattern: normalized gauge width decreases in the amniote stem-group toward the LCAA and independently within the Diadectomorpha. Synapsids are marked by narrow gauges, whereas wide gauges appear to re-occur in some early Permian reptile groups (Figures 6A, 8C). Stride length, pace length and pace angulation display concurrent patterns: after a decrease in the amniote stem group toward the last common ancestor of cotylosaurs (between nodes C and F), the values re-increase toward the LCAA and later they appear to rise independently within diadectomorph, synapsid and reptile trackmakers (Table 3 and Figures 6C,D).
The position of the manus imprint with respect to the pes imprint shows only minor variation in the amniote stem-group: Unlike the rather medial position in amphibian and most reptiliomorph trackmakers, the manus is more laterally positioned toward the LCAA, a tendency which continues within diadectomorphs and synapsids whereas reptile trackways also include track types with medially positioned manus (Table 4 and Figures 7B, 8D). The glenoacetabular length (“apparent trunk length”) decreases toward the LCAA and independently within the Diadectomorpha. Within different groups of amniotes, however, higher glenoacetabular lengths re-occur (Figures 7C, 8E). Among some morphotypes of Ichniotherium and in certain amniote track types, such as Erpetopus and Dromopus, primary partial overstep between pes and manus footprints occurs regularly, which is reflected by short normalized manus-pes distances for certain diadectid and amniote clades (values of 1.0 or lower, Table 4). With its very high normalized pace length, stride length, gauge width and glenoacetabular length, the late occurring Erpetopus represents a notable exception to other reptilian track types and significantly enlarges the ranges represented in our sample, but it has limited influence on estimated values for Carboniferous nodes (Figures 6, 7 and Supplementary Material 2).
The orientation of the pes imprints does not vary much on the amniote stem (Figures 7D, 8F). Toward the LCAA, the pes imprint is less outward- and more parallel-oriented, a tendency, which becomes more pronounced in some reptile tracks and is paralleled by the occurrence of more parallel-oriented footprints in early Permian Ichniotherium tracks (Figures 7D, 8G). The distribution pattern for the orientation of the manus imprints looks quite different: toward amniotes, the manus becomes successively less inward-oriented, a trend not seen in diadectomorphs. In some reptile track types a more inward orientation of the manus re-occurs (Figures 7E,F, 8H).
Among the 22 taxonomical units used in our phylogenetic approach, some represent trackway patterns similar to the modeled LCAA pattern. I. sphaerodactylum trackways dominate the list of specimens with the lowest distance values (Table 5). If all specimens of a taxonomical unit are considered and an average rank is calculated, the trackmakers of (1) Hylopus isp., (2) I. sphaerodactylum (3) I. cottae from the Pittsburgh Formation, (4) N. missouriensis and (5) I. cottae (Birkheide-Gottlob type) come out as the most amniote-ancestor-like types (Supplementary Material 2). Apart from the single trackway of Hylopus, some trackways of I. sphaerodactylum and of I. cottae from the locality Birkheide also have pes lengths close to the value inferred for the amniote ancestor, implying a similar body size of the respective trackmaker.
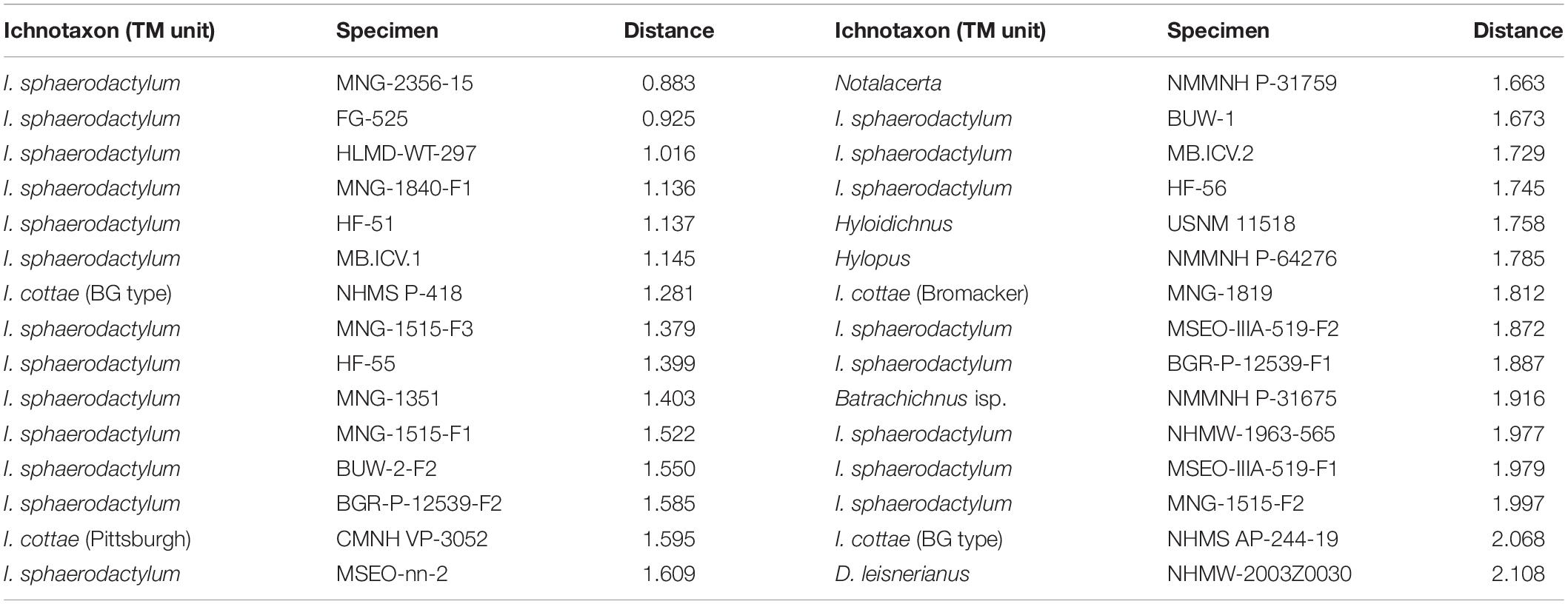
Table 5. Individual trackways ordered according to their similarity with the last common ancestor of amniotes (distance to node K, result for tree A) based on six trackway measures (complete list in Supplementary Material 2).
For an alternative trackmaker phylogeny (tree B) that includes the Tambachichnium trackmaker as a close relative of the alleged diapsid producers of Dromopus (Figure 4B), only minor differences in the reconstructed ancestral states were found (Tables 3, 4). According to the Akaike Information Criterion calculated for both trees based on 11 characters, the alternative tree is significantly more likely (ΔAIC = 146,1656629) – a result which reflects the similarity of Dromopus and Tambachichnium trackway patterns.
Discussion
Evolutionary Change of the Trackway Pattern in Relation to Body Size and Functional Implications
According to the succession of ancestral states inferred in this approach, the Carboniferous evolution of reptiliomorph trackmakers is characterized by a conspicuous pattern of transition toward the last common ancestor of amniotes and diadectomorphs (Clade Cotylosauria, in our analysis node F has been set at 326 Ma). From earlier reptiliomorphs to cotylosaurs an increase in maximum pes length occurs, which is coeval to a continuous change in various trackway measures: gauges become narrower, strides shorter, the glenoacetabular length decreases, manus and pes imprints turn toward a more trackway-parallel orientation and are set at a similar distance from the trackway midline. We interpret these changes as causally linked and the increase in body size as a crucial factor driving evolutionary changes in the locomotion system: to support higher weights, limb postures become more vertical-oriented, i.e., the axis between hip and ankle joint reached a higher angle toward the ground. Accordingly, the degree of sprawling decreased – a change that is visible in the trackways as a transition to narrower gauges.
Higher weights also effect higher damaging loads, especially in the case of elongated and flexible trunks. Thus, morphological changes in the axial skeleton of cotylosaurs could have led to a shorter and stiffer trunk and to the decrease of forward propulsion through lateral axial bending (see e.g., discussion of changes in the axial skeleton of amniote relatives by Sumida, 1990). In the trackway pattern, such changes in the skeletal morphology appear as a decrease in glenoacetabular length and a low deviation in the orientation of manus and pes. But, as noted above, the supposed glenoacetabular length of a trackway is not only controlled by the actual trunk length of the trackmaker, but also by the type of gait and while a decrease in presacral vertebral number and shortening of the trunk has been observed within the Diadectomorpha (e.g., Müller et al., 2010), such a reduction did not necessarily happen in the earlier evolution of reptiliomorphs toward the origin of amniotes. In agreement with a more rigid trunk, the predominant use of a type of lateral sequence walk with less ground contact instead of a “primitive” walking trot could explain the observation that manus and pes are set parallel to each other and to the direction of movement. That the arms and shoulder girdle of amniote and diadectomorph ancestors played a more active role in weight support and/or forward propulsion, could be a further explanation for the more lateral position of manus imprints and their more forward-pointing orientation.
Toward the LCAA – in our analysis it is assumed to have an age of 323 Ma – large imprint sizes persist, and trends observed in the trackway pattern of earlier amniote ancestors continue, namely a decrease in gauge width and the change in manus and pes imprint orientation toward midline-parallel. However, unlike the trend in earlier reptiliomorphs, normalized stride length and pace angulation are rising. The increase in stride lengths appears to persist within separate producer groups after the initial divergence of amniotes and is paralleled by a similar trend in Ichniotherium trackmakers (see also Buchwitz and Voigt, 2018). We interpret these stride increases as indicative for benefits of the reorganization of the locomotion system – enabling more efficient walk with higher speeds – coming into effect.
Apart from the study of trackway measurements, the constraining effect of body size on locomotion style can also be deduced from the presence or absence of medial drag traces associated with footprints, which were produced through ground contact of the belly and/or tail. Such drag traces were not common in late Paleozoic tetrapod tracks (Table 1 and Figure 1) and continuous drag traces appear to be almost absent in trackways of larger trackmakers (see Supplementary Material 1), which is unsurprising, considering the mechanical disadvantage of high friction in case of belly dragging in combination with high weights. While the estimated maximum pes length and the modeled abundance of drag traces show the expected negative correlation (Figure 8B), the lack of sufficient sample sizes (3 or more) for Carboniferous Ichniotherium and Dimetropus tracks has a notable influence and leads to higher estimates for the amniote stem lineage. The estimated abundance of drag marks accounts for ca. 30% in the common ancestor of cotylosaurs and re-increases to >45% in the LCAA (Table 3 and Figure 6B).
Following the origin and early diversification of amniotes, pes length (as a body size proxy), appears to decrease toward romeriid eureptiles and the occurrence of a continuous medial drag mark in early reptile tracks assigned to the ichnotaxon Notalacerta indicates a notably modified locomotion system. If body size increases acted as a trigger or underlying factor and were driving locomotion changes in the ancestors of amniotes, certain changes in the ecology and body size of their reptilian descendants apparently had a somewhat contrary effect on the locomotion system and led to a re-occurrence of gaits characterized by ground contact of the belly and/or tail. With their extremely high gauge width, stride length, pace length and glenoacetabular length in combination with a primary overstep (from partial to total) of pes on manus imprints, the late Cisuralian (Kungurian) trackways of Erpetopus are an exception among early reptilian ichnotaxa. They arguably represent a derived type of broad sprawling gait, which is different from that observed in anamniote tracks, such as Amphisauropus, Matthewichnus, and I. praesidentis. Considering the relatively narrow gauges and rarity of medial drag marks in the alleged diapsid track type Dromopus, “crawling” locomotion as the eponymous feature of the group Reptilia, present in certain extant diapsids, is likely to be a secondary or tertiary adaptation that evolved from a distinct type of posture and gait.
Given the comparatively small dataset of twelve synapsid trackways included in our analysis, evolution trends within early synapsid trackmakers cannot be reliably inferred in this approach. Dimetropus isp. from the Bochum Formation and the Tambach Formation tracks assigned to D. leisnerianus and T. schmidti were made by relatively large individuals. They share the narrow gauge and the forward or slightly laterally pointing manus, but differ in most of the other characters. According to the results of our maximum likelihood approach, the alternative phylogenetic tree with Tambachichnium as a diapsid track type (in accordance with a revised placement of its varanopid trackmakers, e.g., Ford and Benson, 2020) is in much better agreement with the trackway data and arguably hypotheses about early synapsid locomotion should not rely too much on the inclusion of this ichnotaxon. Whether the presence of discontinuous medial drag marks in several Dimetropus trackways from the Tambach Formation is typical for a specific synapsid producer group can only be solved with a more comprehensive sample.
Evolutionary Stages of Ancestral Amniote Locomotion and Trackmaker Model for the LCAA
Based upon the results of our ancestral state modeling approach and their interpretation in terms of locomotion and functional morphology, we devise stages of locomotion evolution for the earliest amniotes and their ancestors (Figure 9):
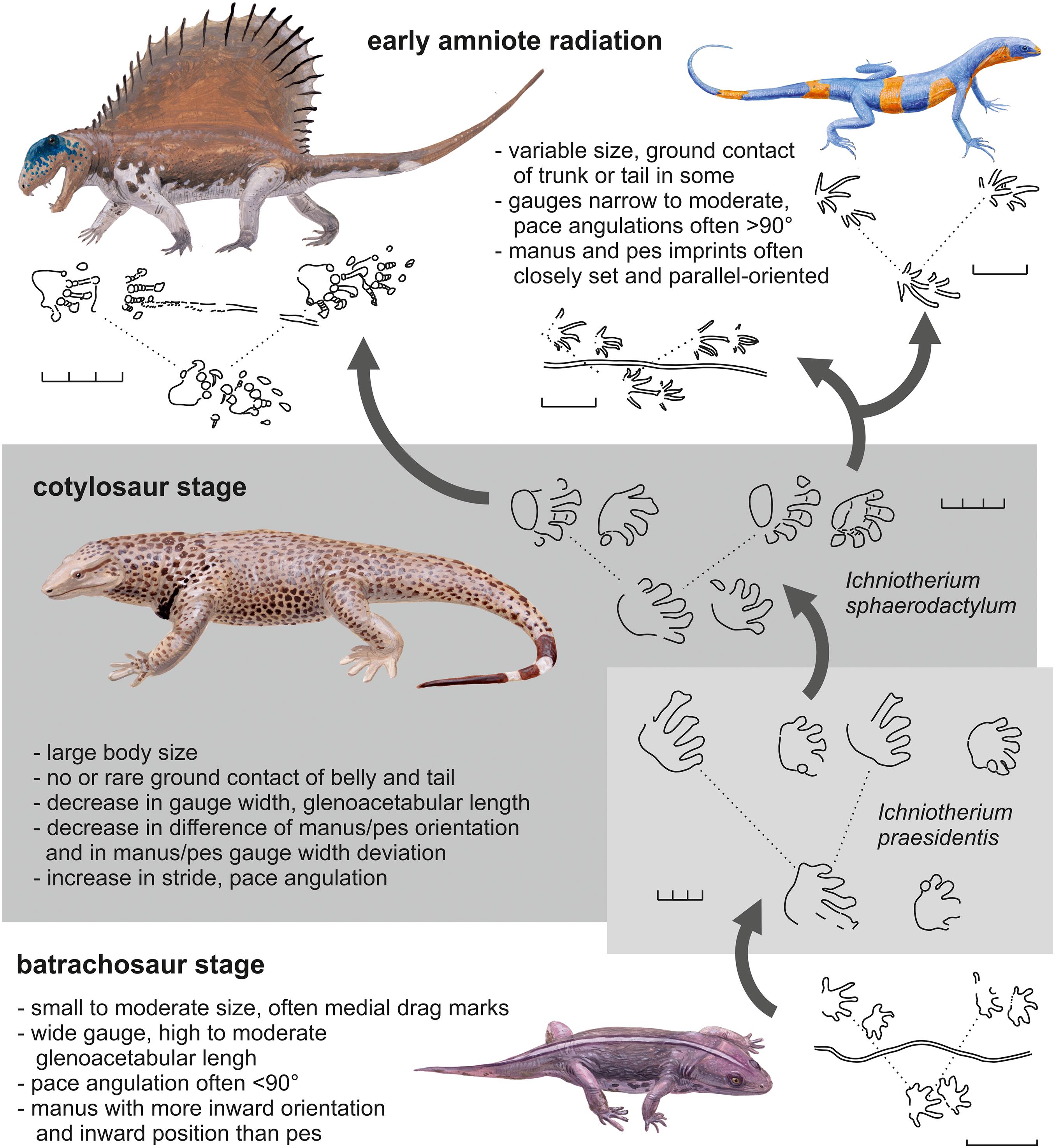
Figure 9. Stages of locomotion evolution toward early amniotes and notable trackway characteristics. Seymouria, Orobates, Dimetrodon, and Araeoscelis are depicted as possible trackmakers for Amphisauropus (bottom), Ichniotherium, Dimetropus (top left), and Dromopus (top right). Except for Ichniotherium praesidentis, the examples come from the early Permian of Germany and North America, illustrating that some variability representing the dynamics of locomotion evolution in the earliest amniotes and their relatives was still present by that time. Scale unit: 3 cm. (Drawings by J. Knüppe, Ibbenbühren).
Following the early diversification of reptiliomorphs between 350 and 340 Ma, amniote ancestors had small to moderate body sizes and sprawling postures that are reflected in the trackways by relatively wide gauges and moderate stride lengths. The trunks of these trackmakers were relatively long and flexible – these features are visible in the tracks as pes/manus overlap due to high coupling values, high to moderate glenoacetabular lengths, outward-oriented pes and inward-oriented and medially positioned manus imprints. Common belly or tail contact during progression resulted in the occurrence of furrow-like medial drag marks. Reptiliomorph trackmakers with these characteristics represent the “batrachosaur stage” of ancestral amniote locomotion, referring to Batrachosauria as a clade including seymouriamorphs and further groups that are more closely related to amniotes than embolomeres and other “anthracosaur-grade” reptiliomorphs (sensu Gauthier et al., 1988; Laurin and Reisz, 1995). With some reservations, late Carboniferous to early Permian trackmakers of Amphisauropus and Matthewichnus may be regarded as representatives of this stage. They are, however, considerably younger than the Mississippian diversification of reptiliomorphs in and it is unclear whether they are linked to their early relatives through a line of terrestrial ancestors (see e.g., discussion in Bazzana et al., 2020).
Between 340 and 320 Ma, members of the amniote stem group reached relatively large adult body sizes, which is indicated by a pes length comparable to that of smaller Ichniotherium and Dimetropus tracks (between 70 and 85 cm). As outlined above, a notable evolutionary transition in locomotion occurred during the “cotylosaur stage,” which led to a simultaneous change or quick succession of changes in multiple trackway parameters. Thereby trackmakers with longer and more flexible trunks, a pronounced sprawling posture and walking gaits that allowed only short strides and a low maximum speed evolved into more adept terrestrial dwellers with shorter trunks, less axial flexibility and gaits with a lower degree of sprawling that resulted in longer strides and higher maximum speed. In some aspects, this evolutionary trend is paralleled by the later transition within diadectomorphs (Figure 9) from trackmakers with an Ichniotherium-praesidentis-like trackway pattern to diadectid trackmakers of the late Cisuralian I. sphaerodactylum and I. cottae, which can be regarded as late survivors of “cotylosaur stage” amniote ancestors. Despite its plesiomorphic appearance with a high glenoacetabular length, a high normalized gauge width and a low pace angulation, the only known long trackway of I. praesidentis shows no indications of pattern variation due to axial bending and differs in this aspect from Matthewichnus and Amphisauropus tracks (e.g., Haubold et al., 2005; Voigt, 2005, 2015; Voigt and Lucas, 2015; Marchetti et al., 2017). This observation is concordant with the idea that stabilization of the axial skeleton and musculature to support the suspension of the trunk could have been one of the critical morphological changes related to gaining higher body masses.
According to our conceptual model of ancestral amniote locomotion, the time range of the cotylosaur stage and its spectrum of trackway patterns also includes the LCAA. Considering the combination of values found per ancestral state reconstruction, some trackways of I. sphaerodactylum display trackway patterns most similar to the LCAA (see similarity ranking in Table 5). Thus, our results support the hypothesis that the track-trackmaker pair of I. sphaerodactylum and Orobates pabsti from the Cisuralian Bromacker locality of the Thuringian Forest was close to the LCAA in terms of trackway pattern similarity and – in agreement with the approaches of Nyakatura et al. (2015, 2019) – represents a suitable ancestral amniote track-trackmaker model. Only the inward orientation of the manus in I. sphaerodactylum might have been untypical for the earliest amniote trackmakers and some of the similarities in the relatively young Bromacker material are probably due to convergence and do not represent surviving plesiomorphies. The seemingly derived pattern of the Hylopus trackway NMMNH P-64276, which is more similar to the LCAA than the average I. sphaerodactylum track, suggests that either an early cotylosaur relative instead of a more distantly related reptiliomorph was the trackmaker or that “amniote-like” trackway patterns were also produced by early reptiliomorphs or even more distantly related tetrapods in convergent episodes of terrestrialization (e.g., Smithson and Clack, 2017).
Between 320 Ma and the Carboniferous/Permian boundary amniotes diversified, which is also reflected by the morphological diversity, trackway patterns and size ranges of Cisuralian amniote tracks. Unlike most diadectomorph tracks and inferred track size for the cotylosaur stage, the late Pennsylvanian to Cisuralian reptile tracks were usually made by small-bodied trackmakers, while the synapsid track record also includes larger track types. Only few trackway characteristics are shared by most amniote track types of this stage and suggest a derived locomotion capability compared to the batrachosaur and cotylosaur stages: normalized stride lengths and pace angulations are usually moderate to high, exceeding 3.5 and 90°, respectively. With the exception of Erpetopus and Varanopus, gauge width is low. Noteworthy outward orientations of the pes and inward orientations of the manus occur in only a few track types. Most amniote trackway samples include one or several specimens with primary overstep of pes over manus (pes/manus distance < average footprint length), suggesting that a gait with non-simultaneous ground contact of manus and pes (i.e., a type of lateral sequence walk) was common.
Our results and conclusions are preliminary for various reasons. Most notably, all patterns discussed above merely consider the mean values of distributions that resulted from a bootstrapping approach, which was undertaken in order to assess the effects of variability among trackways assigned to the same trackmaker unit (and involves random trackway specimens of each unit in addition to nodal reconstructions based on the centerpoint specimens). Taking the high variances of these distributions and overlapping ranges for subsequent nodes into account (Figure 5B and Supplementary Material 2), the discussed mean values and deduced evolutionary trends involve a considerable degree of uncertainty, indicating that evolutionary shifts in imprint size and trackway parameters might be difficult to localize.
Apart from the effects of variability within trackmaker groups and the general uncertainty of nodal reconstructions (see also Laurin, 2004; Didier et al., 2019), ambiguous track-trackmaker correlations and the controversial phylogenetic relationships of the skeletal-morphology-based orthotaxa also contribute to the vagueness. We have compared results for two alternative trees, alternating the position of varanopids as supposed trackmakers of Tambachichnium – with only minor effects regarding the lineage of amniote ancestors. Other alternative tree topologies with potentially large consequences for reconstructed ancestral states include the following: diadectomorphs within Amniota as the sistergroup of all other synapsids (sensu Berman et al., 1992, Berman, 2013) or as a paraphyletic group (e.g., Ruta et al., 2003b; Pawley, 2006; Marjanović and Laurin, 2019) or deviant relationships of reptiliomorph groups with seymouriamorphs being more closely related to the clade Cotylosauria than lepospondyls (e.g., Klembara et al., 2014). Especially in the case that track types and trackmakers with large sizes were confined to a synapsid clade including diadectomorphs, the reconstructed trackway pattern for the LCAA would differ considerably and our interpretation of a “cotylosaur stage” encompassing large-bodied trackmakers that were ancestors to all amniotes, including reptiles, would be put into question. Thus, in future approaches alternative phylogenies should be addressed and, more generally, inferences about functional change and locomotion evolution should be tested regarding their robustness, i.e., whether they are plausible for different phylogenetic hypotheses.
Another issue leading to far-off conclusions could be sampling bias, e.g., underrepresentation of small or large track types of a trackmaker group, which would make separate processes appear to be a single event. If body size increases were a very common factor driving evolutionary changes in early tetrapod locomotion, an alternative to our model would be a parallel change in several lineages, e.g., a convergence in trackmaker anatomy that led to a decrease in gauge width, as soon as a certain group crosses a certain body size threshold. In this regard, more reliable conclusions regarding the ancestry of amniotes could be gained with a higher number of specimens per trackmaker sample for Carboniferous track types, but also through a generally improved coverage of trackway samples from the Carboniferous.
Considering body size as the main or sole trigger or driving factor of evolutionary change in locomotion among amniote ancestors, could also be a misconception based on the badly resolved early phylogeny of trackmakers. Especially the long timespan between the divergence of lepospondyls and cotylosaurs (node E) and the last common ancestor of cotylosaurs (node F) could include a series of subsequent changes that erroneously appear to be contemporaneous. Furthermore, the slight decrease in stride length and pace angulation prior to the change in body size and gauge width (see Figures 5B, 7E, from node C to E) is not explained by our hypothesis of cause and effect.
Methodological Aspects of Ancestral State Reconstructions to Pinpoint Evolutionary Changes in Fossil Trackmakers
In addition to the sources of uncertainty discussed above, further methodological aspects had an influence on our results and should be considered in other studies of trackway data that involve ancestral state reconstructions:
1. Choice of body size proxies: we chose pes length as a body size proxy for normalization of trackway measurements because our dataset includes a highly variable heel length and thus the length of pedal digit IV (usually the longest digit) would be a less reliable alternative proxy for total foot and body size. If increases in body weight and body volume can be correlated to the total imprint area of manus and pes, a combined measure based on length and width of manus and pes would be an even better body size indicator (see Buchwitz et al., 2020). In this study, however, completely preserved tracks with four measurable length and width values make up a considerably smaller part of the total sample, so we decided to use only the pes length instead.
2. Influence of postulated node ages and outliers: The proximity of node ages to certain ages of Carboniferous terminal taxa has a large influence on the modeled ancestral states and we have assumed a distance of at least 3 million years between the center of a trackmaker range and the closest node. Especially the two individual trackways of particularly large trackmakers from the Bochum Formation (TM 8 and TM 15 in Table 1, Voigt and Ganzelewski, 2010), which are close in age to the assumed origins of Amniota and Cotylosauria, had a large effect on the outcome of our analysis. In order to mitigate the influence of these outliers in our interpretations regarding body size evolution, we considered the maximum pes length instead of an average or mean pes length as body size proxy for an entire trackmaker sample. Given the somewhat higher likely age that Didier and Laurin (2020) inferred for the Amniota (synapsid/reptilian divergence at 322–340 Ma), it might be advisable to test the effect that different age assumptions can have on the reconstructions.
3. Inclusion of data from multiple localities: except for the Limnopus vagus and H. bifurcatus TM samples, all taxonomic units used in our phylogenetic approach represent one locality and stratigraphic unit or, in case of the Thuringian Forest data, a succession of strata including several units from the same depositional area. We suggest prioritizing homogeneity over sample size. If more than two individual samples pro ichnotaxon are available and track-trackmaker relationships cannot be resolved any further, we recommend to include these samples in an unresolved polytomy, e.g., a clade of trackmakers of the ichnotaxon Dromopus could include four taxonomic units that represent Dromopus samples from locality A/age I, locality B/age I, locality B/age II, and locality C/age II. The inclusion of several samples per ichnotaxon, which come from multiple localities, can mitigate the potentially distorting effect of substrate or taphonomic differences as a cause of variation between different ichnotaxa. With a rising coverage of distinct depositional settings, the possibility that coincidental differences in preservation or substrate (e.g., ichnotaxon X only from sandy substrates; ichnotaxon Y only from muddy substrates) and not actual locomotion differences are the cause of distinct trackway patterns, becomes increasingly unlikely.
4. Consideration of inhomogeneities in individual trackway samples: In this approach, trackways from the same locality assigned to the same ichnotaxon were considered as products of a homogeneous trackmaker group and variability within the sample as representing varying behavior, individual locomotion capability, minor anatomical differences and other factors that can also be observed in tracks produced by populations of closely related extant trackmakers with similar footprint morphology. In future studies, particularly large trackway samples could be searched for distribution patterns that indicate separable locomotion styles and/or trackmaker species (e.g., bimodal or multimodal trackway parameter distributions). If some of the (normalized) trackway measures correlate with footprint size, this pattern could be an indicator for either an ontogenetic change in locomotion or the mixing of a small and a large trackmaker species with similar footprint morphology but distinct locomotion. In the case that such an inhomogeneity is discovered, tracks of juvenile trackmakers could be exempted from the analysis or the sample could be split and the subsets referred to separate taxonomical units.
5. Handling of footprint ichnotaxa that have polyphyletic producer groups: trackway samples of an ichnotaxon that are likely to represent two or more unrelated groups, i.e., a polyphylum of trackmakers, should be avoided. If one of the alleged producer groups is stratigraphically or geographically restricted, it might be ruled out for at least some of the available trackway samples (that come from other regions or time ranges). Accordingly, the track-trackmaker correlation might be clarified for certain samples of an ichnotaxon (see ichnotaxon 1 in Figures 3A,B), which may be used in an ancestral state reconstruction approach, whereas other samples of the same ichnotaxon, which are equivocal in their trackmaker assignment, should be discarded. An alternative way to deal with ichnotaxa that have been correlated with distinct unrelated producer groups is the use of several different trackmaker trees – with the aim to compare the outcomes according to their likelihood and document whether patterns of evolutionary change in trackway measures differ significantly (see consideration of the Tambachichnium trackmaker in our analysis).
6. Use of alternative methods for the reconstruction of ancestral states and character evolution: A parsimony-based testing method introduced by Didier et al. (2019) for quantitative characters takes branch length into account and aims at the localization of trend changes on a phylogenetic tree. Whether such an approach can detect changes on the lineage connecting last common ancestor of tetrapods and the last common ancestor of amniotes – despite the comparatively low resolution of the trackmaker trees, high age differences between the supposed timing of divergence from the amniote stem lineage and the first occurrence of a trackmaker and poor sampling density for the Mississippian and early Pennsylvanian – remains to be tested.
Not all available footprint and trackway features have been used for ancestral state reconstruction in our present study. In the absence of a direct co-occurrence of tracks and trackmaker skeletons (i.e., on the same bedding plane), measures and non-numerical characters that describe footprint morphology, such as toe imprint proportions, toe divarication angles, shape and relative length of the heel imprint, usually form the basis of track-trackmaker correlation. Their ancestral states have not been pursued here, among others, because the results would be redundant to skeletal-data-based inference of evolutionary changes in certain characters (e.g., for toe proportions, reconstructions can be inferred with a higher precision based on the fossil record of foot skeletons instead of footprints). One of the ideas in this study was the documentation of changes in maximum body size, inferred from footprint length. Whether the discussed signal reflects an actual pattern could be counter-checked based on measurement data of trackmaker skeletons (e.g., skull length, long bone length, and total limb length). Accordingly, ancestral states could be reconstructed for a skeleton-based body size proxy – which would also have the advantage that it includes a more detailed phylogenetic tree that reflects body size changes more realistically (see Laurin, 2004; Didier et al., 2019 on skull length in amniotes and their relatives; Brocklehurst and Brink, 2017 on synapsid body size). Another idea to cover trackmaker body size with more detailedness would be the inclusion of the somewhat larger record of isolated tracks and couples (instead of only trackways), provided their preservation is good enough for trackmaker assignment. Apart from length and angle measures, a feature worth looking at in an ancestral state estimation approach could be the relative depth of footprints – whether the medial or lateral parts of a foot are deeper impressed is often controlled by function (e.g., articulations, posture, and type of gait) and follows a group-specific pattern (functional prevalence; Mujal et al., 2020).
In addition to amniote origins and locomotion evolution in early amniotes and their relatives, radiation events marked by fast change in footprint morphology and abundant appearance of new ichnotaxa may be approached through phylogenetically informed analysis of trackway data, including the evolution of synapsid trackmakers during the Permian (e.g., Marchetti et al., 2019a) and the rise of archosauromorph trackmakers in the Triassic (e.g., Haubold and Klein, 2002; Bernardi et al., 2015). Because sufficiently character-rich footprint morphologies are needed for detailed track-trackmaker correlations, not all tetrapod footprint ichnotaxa are equally well suited for phylogenetic considerations. Among others, trackways of a quadruped are more character-rich than those of a biped walker, footprints of a plantigrade foot display certain features of the sole/heel that are absent in the case of digitigrade foot morphologies and pentadactyl feet display more measurable sizes than tridactyl feet – in each case, the former mentioned morphologies are better suited for track-trackmaker correlation and ancestral state reconstruction approaches than the latter. As an alternative, large samples of character-poor trackways assigned to one or several ichnotaxa that were produced by a homogeneous trackmaker group, which cannot be further subdivided, may be studied in a time series approach (see discussion of such approaches in Kubo and Benton, 2009; Buchwitz and Voigt, 2018).
Data Availability Statement
The original contributions generated for this study are included in the article/Supplementary Material, further inquiries can be directed to the corresponding authors. The R script is deposited externally on Github and accessible via the following link: https://github.com/plannapus/tracks_asr.
Author Contributions
MB, SV, MJ, and JR conceived the approach. SV carried out all trackway measurements based on the graphic documentation of all trackway specimens on transparent sheets. MB sorted, selected, and processed the data. MJ and JR adapted the data for the ancestral character estimation approach, wrote the R script, carried out all related calculation steps, listed all results, and generated tree schemes with color ranges. LM did a literature search for ichnological and morphological data and studies that concern the origin of amniotes and locomotion evolution. MB, MJ, JR, LM, and SV discussed the results and wrote the manuscript. MB and MJ prepared the figures and tables. All authors contributed to the article and approved the submitted version.
Funding
The Bundesministerium für Bildung und Forschung (BMBF) provided funding for LM (BROMACKER Project 2020). We acknowledge support by the German Research Foundation (DFG) and the Open Access Publication Fund of Humboldt-Universität zu Berlin.
Conflict of Interest
The authors declare that the research was conducted in the absence of any commercial or financial relationships that could be construed as a potential conflict of interest.
Acknowledgments
The following institutions and collectors are acknowledged for providing access to specimens measured for this approach: Alabama Museum of Natural History Tuscaloosa, Bauhaus-Universität Weimar, Bundesanstalt für Geowissenschaften und Rohstoffe Berlin-Spandau, Cincinnati Museum Center, Denver Museum of Nature & Science Colorado, Deutsches Bergbau-Museum Bochum, Hans-Detlef Stober (Berlin), Hessisches Landesmuseum Darmstadt, Kletno Geological Museum, Lapworth Museum of Geology Birmingham, Martin-Luther-Universität Halle-Wittenberg, Marietta College, McWane Science Center Birmingham, Musée de Lodève, Museum der Natur Gotha, Museum für Mineralogie und Geologie Dresden, Museum für Naturkunde Berlin, Museum für Naturkunde Magdeburg, Museum Schloss Ehrenstein Ohrdruf, Naturhistorisches Museum Schloss Bertholdsburg Schleusingen, Naturhistorisches Museum Wien, New Mexico Museum of Natural History & Science Albuquerque, Orton Geological Museum, Phyletisches Museum Jena, Siegfried Severt (Magdeburg), Technische Universität Bergakademie Freiberg, United States National Museum of Natural History Washington, Universalmuseum Joanneum Graz, Urweltmuseum GEOSKOP Thallichtenberg, and Yale Peabody Museum of Natural History New Haven. We are thankful to the research group of Jörg Fröbisch (AG Fröbisch) at the Museum für Naturkunde Berlin for logistical support. Jens N. Lallensack, Michel Laurin, Jean-David Moreau and Abdelouahed Lagnaoui are gratefully acknowledged for critical comments on the first draft, which lead to a substantial improvement of our manuscript.
Supplementary Material
The Supplementary Material for this article can be found online at: https://www.frontiersin.org/articles/10.3389/fevo.2021.674779/full#supplementary-material
Supplementary Material 1 | Specimen list and list of institutional abbreviations.
Supplementary Material 2 | Input data and results.
References
Akaike, H. (1974). A new look at the statistical model identification. IEEE Trans. Automatic Control 19, 716–723. doi: 10.1109/tac.1974.1100705
Anderson, J. S., Carroll, R. L., and Rowe, T. B. (2003). New information on Lethiscus stocki (Tetrapoda: lepospondyli: aistopoda) from high-resolution computed tomography and a phylogenetic analysis of Aistopoda. Can. J. Earth Sci. 40, 1071–1083. doi: 10.1139/e03-023
Andrews, S., and Carroll, R. (1991). The Order Adelospondyli: carboniferous lepospondyl amphibians. Transactions of the Royal Society of Edinburgh. Earth Sci. 82, 239–275. doi: 10.1017/S0263593300005332
Baird, D. (1952). Revision of the Pennsylvanian and Permian footprints Limnopus, Allopus and Baropus. J. Paleontol. 26, 832–840.
Barrett, P. M., Evans, D. C., and Campione, N. E. (2015). Evolution of dinosaur epidermal structures. Biol. Lett. 11:20150229. doi: 10.1098/rsbl.2015.0229
Bazzana, K. D., Gee, B. M., Bevitt, J. J., and Reisz, R. R. (2020). Postcranial anatomy and histology of Seymouria, and the terrestriality of seymouriamorphs. PeerJ 8:e8698. doi: 10.7717/peerj.8698
Benson, R. B. J., Campione, N. E., Carrano, M. T., Mannion, P. D., Sullivan, C., Upchurch, P., et al. (2014). Rates of Dinosaur Body Mass Evolution Indicate 170 Million Years of Sustained Ecological Innovation on the Avian Stem Lineage. PLoS Biol 12:e1001853. doi: 10.1371/journal.pbio.1001853
Benton, M. (2020). The origin of endothermy in synapsids and archosaurs and arms races in the Triassic. Gondwana Res. ∗[in press]
Berman, D. S. (2013). Diadectomorphs, amniotes or not? The Carboniferous-Permian Transition. Bull. New Mexico Museum. Nat. History Sci. 60, 22–35.
Berman, D. S., Sumida, S. S., and Lombard, R. E. (1992). Reinterpretation of the temporal and occipital regions in Diadectes and the relationships of diadectomorphs. J. Paleontol. 66, 481–499. doi: 10.1017/s0022336000034028
Berman, D. S., Reisz, R. R., and Scott, D. (2010). Redescription of the skull of Limnoscelis paludis Williston (Diadectomorpha: Limnoscelidae) from the Pennsylvanian of Cañon del Cobre, Northern New Mexico. New Mexico Museum of Natural History and Science Bulletin 49, 185–210.
Bernardi, M., and Avanzini, M. (2011). Locomotor behavior in early reptiles: insights from an unusual Erpetopus trackway. J. Paleontol. 85, 925–929. doi: 10.1666/10-080.1
Bernardi, M., Klein, H., Petti, F. M., and Ezcurra, M. D. (2015). The Origin and Early Radiation of Archosauriforms: integrating the Skeletal and Footprint Record. PLoS One 10:e0128449. doi: 10.1371/journal.pone.0128449
Borths, M. R., and Stevens, N. J. (2017). The first hyaenodont from the late Oligocene Nsungwe Formation of Tanzania: paleoecological insights into the Paleogene-Neogene carnivore transition. PLoS One 12:e0185301. doi: 10.1371/journal.pone.0185301
Brocklehurst, N., and Brink, K. S. (2017). Selection towards larger body size in both herbivorous and carnivorous synapsids during the Carboniferous. FACETS 2, 68–84. doi: 10.1139/facets-2016-0046
Brusatte, S. L., Niedźwiedzki, G., and Butler, R. J. (2011). Footprints pull origin and diversification of dinosaur stem-lineage deep into Early Triassic. Proc. R. Soc. London B 278, 1107–1113. doi: 10.1098/rspb.2010.1746
Buchwitz, M., Marchetti, L., Jansen, M., Falk, D., Trostheide, F., and Schneider, J. W. (2020). Ichnotaxonomy and trackmaker assignment of tetrapod tracks and swimming traces from the Middle Permian Hornburg Formation of Saxony-Anhalt (Germany). Annales Societatis Geologorum Poloniae 90, 291–320.
Buchwitz, M., and Voigt, S. (2018). On the morphological variability of Ichniotherium tracks and evolution of locomotion in the sistergroup of amniotes. PeerJ 6:e4346. doi: 10.7717/peerj.4346
Burnham, K. P., and Anderson, D. R. (2002). Model Selection And Multimodel Inference: A Practical Information-Theoretic Approach (2nd Ed.). New York: Springer-Verlag.
Calder, J. H., Gibling, M. R., Scott, A. C., Davies, S. J., Hebert, B. L., Greb, S. F., et al. (2006). A fossil lycopsid forest succession in the classic Joggins section of Nova Scotia: paleoecology of a disturbance-prone Pennsylvanian wetland. Special Papers Geol. Soc. Am. 399:169.
Carman, E. (1927). Fossil footprints from the Pennsylvanian System in Ohio. Bull. Geol. Soc. Am. 38, 385–396. doi: 10.1130/gsab-38-385
Carrano, M. T., and Wilson, J. A. (2001). Taxon distributions and the tetrapod track record. Paleobiology 27, 564–582. doi: 10.1666/0094-8373(2001)027<0564:tdattt>2.0.co;2
Carroll, R. L. (1964). The earliest reptiles. J. Linn. Soc. London Zool. 45, 61–83. doi: 10.1111/j.1096-3642.1964.tb00488.x
Carroll, R. L. (1969). A Middle Pennsylvanian Captorhinomorph, and the Interrelationships of Primitive Reptiles. J. Paleontol. 43, 151–170.
Carroll, R. L. (1995). Problems of the phylogenetic analysis of Paleozoic choanates. Bulletin du Muséum national d’Histoire naturelle, 4éme série-section C-Sciences de la Terre, Paléontologie, Géologie, Minéralogie, 17, 389–445.
Chesnut, D. R. Jr., Baird, D., Smith, J. H., and Lewis, R. Q. Sr. (1994). Reptile trackway from the Lee Formation (Lower Pennsylvanian) of south-central Kentucky. J. Paleontol. 68, 154–158. doi: 10.1017/s0022336000025671
Clack, J. A., Witzmann, F., Müller, J., and Snyder, D. (2012). A Colosteid-Like Early Tetrapod from the St. Louis Limestone (Early Carboniferous, Meramecian), St. Louis, Missouri, USA. Fieldiana Life Earth Sci. 2012, 17–39. doi: 10.3158/2158-5520-5.1.17
Curth, S., Fischer, M. S., and Nyakatura, J. A. (2014). Ichnology of an Extant Belly-Dragging Lizard—Analogies to Early Reptile Locomotion? Ichnos 21, 32–43. doi: 10.1080/10420940.2013.877006
Didier, G., Chabrol, O., and Laurin, M. (2019). Parsimony-based test for identifying changes in evolutionary trends for quantitative characters: implications for the origin of the amniotic egg. Cladistics 35, 576–599. doi: 10.1111/cla.12371
Didier, G., and Laurin, M. (2020). Exact distribution of divergence times from fossil ages and tree topologies. Syst. Biol. 69, 1068–1087. doi: 10.1093/sysbio/syaa021
Falcon-Lang, H. J., Benton, M. J., and Stimson, M. (2007). Ecology of early reptiles inferred from Lower Pennsylvanian trackways. J.Geol. Soc. London 164, 1113–1118. doi: 10.1144/0016-76492007-015
Farlow, J. O., and Pianka, E. R. (2000). Body form and trackway pattern in Australian desert monitors (Squamata: varanidae): comparing zoological and ichnological diversity. Palaios 15, 235–247. doi: 10.1669/0883-1351(2000)015<0235:bfatpi>2.0.co;2
Farlow, J. O., Robinson, N. J., Kumagai, C. J., Paladino, F. V., Falkingham, P. L., Elsey, R. M., et al. (2018). Trackways of the American crocodile (Crocodylus acutus) in northwestern Costa Rica: implications for crocodylian ichnology. Ichnos 25, 30–65. doi: 10.1080/10420940.2017.1350856
Felsenstein, J. (1973). Maximum likelihood estimation of evolutionary trees from continuous characters. Am. J. Hum. Genet. 25, 471–492.
Fichter, J. (1983a). Tetrapodenfährten aus dem saarpfälzischen Rotliegenden (Ober-Karbon – Unter-Perm; Südwest-Deutschland) I: Fährten der Gattungen Saurichnites, Limnopus, Amphisauroides, Protritonichnites, Gilmoreichnus, Hyloidichnus und Jacobiichnus. Mainzer Geowiss. Mitt. 12, 9–121.
Fichter, J. (1983b). Tetrapodenfährten aus dem saarpfälzischen Rotliegenden (Ober-Karbon – Unter-Perm; Südwest-Deutschland) II: Fährten der Gattungen Foliipes, Varanopus, Ichniotherium, Dimetropus, Palmichnus, Phalangichnus, cf. Chelichnus, cf. Laoporus und Anhomoiichnium. Mainzer Naturw. Archiv. 21, 125–186.
Fillmore, D. L., Lucas, S. G., and Simpson, E. L. (2012). Ichnology of the Mississippian Mauch Chunk Formation, eastern Pennsylvania. New Mexico Museum Nat. History Sci. Bull. 54, 1–136.
Ford, D. P., and Benson, R. B. (2020). The phylogeny of early amniotes and the affinities of Parareptilia and Varanopidae. Nat. Ecol. Evol. 4, 57–65.
Gand, G., and Durand, M. (2006). Tetrapod footprint ichno-associations from French Permian basins. Comparisons with other Euramerican ichnofaunas. Geol. Soc. London Spec. Publ. 265, 157–177. doi: 10.1144/gsl.sp.2006.265.01.07
Gauthier, J., Kluge, A. G., and Rowe, T. (1988). Amniote phylogeny and the importance of fossils. Cladistics 4, 105–209. doi: 10.1111/j.1096-0031.1988.tb00514.x
Haubold, H. (1970). Versuch einer Revision der Amphibien-Fährten des Karbon und Perm. Freiberger Forschungshefte Reihe C 260, 83–117.
Haubold, H., Allen, A., Atkinson, T. P., Buta, R. J., Lacefield, J. A., Minkin, S. C., et al. (2005). “Interpretation of the tetrapod footprints from the Early Pennsylvanian of Alabama,” in Pennsylvanian Footprints In The Black Warrior Basin Of Alabama, eds R. J. Buta, A. K. Rindsberg, and D. C. Kopaska-Merkel (Alabama: Alabama Paleontological Society Monograph. no. 1), 75–111.
Haubold, H., Hunt, A. P., Lucas, S. G., and Lockley, M. G. (1995). “Wolfcampian (Early Permian) vertebrate tracks from Arizona and New Mexico,” in Early Permian Footprints And Facies, eds S. G. Lucas and A. B. Heckert (New Mexico: New Mexico Museum of Natural History and Science Bulletin, no. 6), 135–165.
Haubold, H., and Klein, H. (2002). Chirotherien und Grallatoriden aus der Unteren bis Oberen Trias Mitteleuropas und die Entstehung der Dinosauria. Hallesches Jahrbuch für Geowissenschaften B 24, 1–22.
Haubold, H., and Lucas, S. G. (2001). Die Tetrapodenfährten der Choza-Formation (Texas) und das Artinsk-Alter der Redbed-Ichnofaunen des Unteren Perm. Hallesches Jahrbuch für Geowissenschaften B 23, 79–108.
Haubold, H., and Lucas, S. G. (2003). Tetrapod footprints of the lower Permian Choza Formation at Castle Peak. Texas. Paläontologische Zeitschrift 77, 247–261. doi: 10.1007/bf03006940
Haubold, H., and Sarjeant, W. A. S. (1973). Tetrapodenfährten aus den Keele und Enville Groups (Permokarbon: stefan und Autun) von Shropshire und South Staffordshire. Großbritannien. Zeitschrift für Geologische Wissenschaften 1, 895–933.
Henderson, D. M. (2006). Burly gaits: centers of mass, stability, and the trackways of sauropod dinosaurs. J. Vertebr. Paleontol. 26, 907–921. doi: 10.1671/0272-4634200626[907:BGCOMS]2.0.CO;2
Hildebrand, M. (1980). The adaptive significance of tetrapod gait selection. Am. Zool. 20, 255–267. doi: 10.1093/icb/20.1.255
Hunt, A. P., and Lucas, S. G. (1998). Vertebrate tracks and the myth of the belly-dragging, tail-dragging tetrapods of the late Paleozoic. New Mexico Museum Nat. History Sci. Bull. 12, 67–69.
Joy, J. B., Liang, R. H., McCloskey, R. M., Nguyen, T., and Poon, A. F. Y. (2016). Ancestral Reconstruction. PLoS Comput. Biol. 12:e1004763. doi: 10.1371/journal.pcbi.1004763
Kissel, R. A. (2010). Morphology, Phylogeny, And Evolution Of Diadectidae (Cotylosauria: Diadectomorpha). Ph. D thesis. ∗Canada: University of Toronto.
Klembara, J., Clack, J. A., Milner, A. R., and Ruta, M. (2014). Cranial anatomy, ontogeny, and relationships of the Late Carboniferous tetrapod Gephyrostegus bohemicus Jaekel, 1902. J. Vertebr. Paleontol. 34, 774–792. doi: 10.1080/02724634.2014.837055
Kriloff, A., Germain, D., Canoville, A., Vincent, P., Sache, M., and Laurin, M. (2008). Evolution of bone microanatomy of the tetrapod tibia and its use in palaeobiological inference. J. Evol. Biol. 21, 807–826. doi: 10.1111/j.1420-9101.2008.01512.x
Kubo, T., and Benton, M. J. (2009). Tetrapod postural shift estimated from Permian and Triassic trackways. Palaeontology 52, 1029–1037. doi: 10.1111/j.1475-4983.2009.00897.x
Kubo, T., and Kubo, M. (2013). Analysis of Triassic archosauriform trackways: difference in stride/footprint ratio between dinosauromorphs and other archosauriforms. Palaios 28, 259–265. doi: 10.2110/palo.2012.p12-099r
Kümmell, S. B., and Frey, E. (2012). Digital arcade in the autopodia of Synapsida: standard position of the digits and dorsoventral excursion angle of digital joints in the rays II–V. Palaeobio Palaeoenv 92, 171–196. doi: 10.1007/s12549-012-0076-6
Lallensack, J. N., Ishigaki, S., Lagnaoui, A., Buchwitz, M., and Wings, O. (2018). Forelimb orientation and locomotion of sauropod dinosaurs: insights from the ?Middle Jurassic Tafaytour tracksites (Argana Basin, Morocco). J. Vertebr. Paleontol. 38:e1512501. doi: 10.1080/02724634.2018.1512501
Laurin, M. (2004). The Evolution of Body Size, Cope’s Rule and The Origin of Amniotes. Syst. Biol. 53, 594–622. doi: 10.1080/10635150490445706
Laurin, M., and Piñeiro, G. H. (2017). A reassessment of the taxonomic position of mesosaurs, and a surprising phylogeny of early amniotes. Front. Earth Sci. 5:88. doi: 10.3389/feart.2017.00088
Laurin, M., and Reisz, R. R. (1995). A reevaluation of early amniote phylogeny. Zool. J.Linn. Soc. 113, 165–223. doi: 10.1111/j.1096-3642.1995.tb00932.x
Laurin, M., and Reisz, R. R. (1997). “A new perspective on tetrapod phylogeny,” in Amniote Origins: Completing the Transition to Land, eds S. S. Sumida and K. L. M. Martin (San Diego and London: Academic Press), 9–59.
Legendre, L. J., Guénard, G., Botha-Brink, J., and Cubo, J. (2016). Palaeohistological Evidence for Ancestral High Metabolic Rate in Archosaurs. Syst. Biol. 65, 989–996. doi: 10.1093/sysbio/syw033
Leonardi, G. (1987). Glossary And Manual Of Tetrapod Footprint Palaeoichnology. Brasilia: Ministerio Minas Energie, Departemento Nacional da Produçao Mineral.
Louca, S., and Doebeli, M. (2017). Efficient comparative phylogenetics on large trees. Bioinformatics 34, 1053–1055. doi: 10.1093/bioinformatics/btx701
Lucas, S. G. (2019). An ichnological perspective on some major events of Paleozoic tetrapod evolution. Boll. Soc. Paleontol. Ital. 58, 223–266.
Lucas, S. G., Kollar, A. D., Berman, D. S., and Henrici, A. C. (2016). Pelycosaurian-grade (Amniota: synapsida) footprints from the lower Permian Dunkard Group of Pennsylvania and West Virginia. Ann. Carnegie Mus. 83, 287–294. doi: 10.2992/007.083.0404
Lucas, S. G., Lerner, A. J., Bruner, M., and Shipman, P. (2004). Middle Pennsylvanian ichnofauna from eastern Oklahoma, USA. Ichnos 11, 45–55. doi: 10.1080/10420940490442322
Lucas, S. G., Stimson, M., King, O., Calder, J., Mansky, C., Hebert, B., et al. (2021). Carboniferous tetrapod footprint biostratigraphy, biochronology and evolutionary events. Geol. Soc. London Spec. Publ.∗ [in press].
Lützner, H., Tichomirowa, M., Käßner, A., and Gaupp, R. (2021). Latest Carboniferous to early Permian volcano-stratigraphic evolution in Central Europe: U–Pb CA–ID–TIMS ages of volcanic rocks in the Thuringian Forest Basin (Germany). Int. J. Earth Sci. 110, 377–398. doi: 10.1007/s00531-020-01957-y
Mann, A., Gee, B. M., Pardo, J. D., Marjanović, D., Adams, G. R., Calthorpe, A. S., et al. (2020). Reassessment of historic ‘microsaurs’ from Joggins, Nova Scotia, reveals hidden diversity in the earliest amniote ecosystem. Pap. Palaeontol. 6, 605–625. doi: 10.1002/spp2.1316
Mann, A., McDaniel, E. J., McColville, E. R., and Maddin, H. C. (2019). Carbonodraco lundi gen. et sp. nov., the oldest parareptile, from Linton, Ohio, and new insights into the early radiation of reptiles. R. Soc. Open Sci. 6:191191. doi: 10.1098/rsos.191191
Marchetti, L., Klein, H., Buchwitz, M., Ronchi, A., Smith, R. M., De Klerk, W. J., et al. (2019a). Permian-Triassic vertebrate footprints from South Africa: ichnotaxonomy, producers and biostratigraphy through two major faunal crises. Gondwana Res. 72, 139–168. doi: 10.1016/j.gr.2019.03.009
Marchetti, L., Voigt, S., Lucas, S. G., Francischini, H., Dentzien-Dias, P., Sacchi, R., et al. (2019b). Tetrapod ichnotaxonomy in eolian paleoenvironments (Coconino and De Chelly formations, Arizona) and late Cisuralian (Permian) sauropsid radiation. Earth Sci. Rev. 190, 148–170. doi: 10.1016/j.earscirev.2018.12.011
Marchetti, L., Mujal, E., and Bernardi, M. (2017). An unusual Amphisauropus trackway and its implication for understanding seymouriamorph locomotion. Lethaia 50, 162–174. doi: 10.1111/let.12184
Marchetti, L., Santi, G., and Avanzini, M. (2014). The problem of small footprints in paleoichnology: remarks on the Early Permian ichnotaxon Erpetopus cassinisi, a local species from Southern Alps (northern Italy). Rivista Italiana di Paleontologia e Stratigrafia 120, 129–143.
Marchetti, L., Voigt, S., Lucas, S. G., Stimson, M. R., King, O. A., and Calder, J. H. (2020b). Footprints of the earliest reptiles: notalacerta missouriensis - ichnotaxonomy, potential trackmakers, biostratigraphy, palaeobiogeography and palaeoecology. Ann. Soc. Geol. Pol. 90, 271–290. doi: 10.14241/asgp.2020.13
Marchetti, L., Francischini, H., Lucas, S. G., Voigt, S., Hunt, A. P., and Santucci, V. L. (2020a). “Paleozoic Vertebrate Ichnology of Grand Canyon National Park,” in Grand Canyon National Park: Centennial Paleontological Resource Inventory (Non-Sensitive Version), Natural Resource Report Nps/Grca/Nrr—2020/2103, eds V. L. Santucci and J. S. Tweet (Fort Collins, Colorado: National Park Service), 333–379.
Marchetti, L., Voigt, S., Mujal, E., Lucas, S. G., Francischini, H., Fortuny, J., et al. (2020c). Extending the footprint record of Pareiasauromorpha to the Cisuralian: earlier appearance and wider palaeobiogeography of the group. Pap. Palaeontol.∗ doi: 10.1002/spp2.1342 [in press].
Marjanović, D., and Laurin, M. (2019). Phylogeny of Paleozoic limbed vertebrates reassessed through revision and expansion of the largest published relevant data matrix. PeerJ 6:e5565. doi: 10.7717/peerj.5565
Meade, L. E., Jones, A. S., and Butler, R. J. (2016). A revision of tetrapod footprints from the late Carboniferous of the West Midlands, UK. PeerJ 4:e2718. doi: 10.7717/peerj.2718
Modesto, S. P., Scott, D. M., MacDougall, M. J., Sues, H. D., Evans, D. C., and Reisz, R. R. (2015). The oldest parareptile and the early diversification of reptiles. Proc. R. Soc. B Biol. Sci. 282:20141912. doi: 10.1098/rspb.2014.1912
Mujal, E., Fortuny, J., Oms, O., Bolet, A., and Galobart, À, and Anadón, P. (2016). Palaeoenvironmental reconstruction and early Permian ichnoassemblage from the NE Iberian Peninsula (Pyrenean Basin). Geol. Mag. 153, 578–600. doi: 10.1017/s0016756815000576
Mujal, E., and Marchetti, L. (2020). Ichniotherium tracks from the Permian of France, and their implications for understanding the locomotion and palaeobiogeography of large diadectomorphs. Palaeogeogr. Palaeoclimatol. Palaeoecol. 547:109698. doi: 10.1016/j.palaeo.2020.109698
Mujal, E., Marchetti, L., Schoch, R. R., and Fortuny, J. (2020). Upper Paleozoic to Lower Mesozoic tetrapod ichnology revisited: photogrammetry and relative depth pattern inferences on functional prevalence of autopodia. Front. Earth Sci. 8:248. doi: 10.3389/feart.2020.00248
Müller, A. H. (1954). Zur Ichnologie und Stratonomie des Oberrotliegenden von Tambach (Thüringen). Paläontol. Z. 28, 189–203. doi: 10.1007/bf03041864
Müller, J., and Reisz, R. R. (2006). The phylogeny of early eureptiles: comparing parsimony and Bayesian approaches in the investigation of a basal fossil clade”. Syst. Biol. 55, 503–511. doi: 10.1080/10635150600755396
Müller, J., Scheyer, T. M., Head, J. J., Barrett, P. M., Werneburg, I., Ericson, P. G., et al. (2010). Homeotic effects, somitogenesis and the evolution of vertebral numbers in recent and fossil amniotes. Proc. Nat. Acad. Sci. 107, 2118–2123. doi: 10.1073/pnas.0912622107
Nesbitt, S. J. (2011). The Early Evolution of Archosaurs: relationships and the Origin of Major Clades. Bull. Am. Museum Nat. History 352, 1–292. doi: 10.1206/352.1
Nyakatura, J. A., Allen, V. R., Lauströer, J., Andikfar, A., Danczak, M., Ullrich, H.-J., et al. (2015). A Three-Dimensional Skeletal Reconstruction of the Stem Amniote Orobates pabsti (Diadectidae): analyses of Body Mass. Centre of Mass Position, and Joint Mobility. PLoS One 10:e0137284. doi: 10.1371/journal.pone.0137284
Nyakatura, J. A., Melo, K., Horvat, T., Karakasiliotis, K., Allen, V. R., Andikfar, A., et al. (2019). Reverse-engineering the locomotion of a stem amniote. Nature 565, 351–355. doi: 10.1038/s41586-018-0851-2
Organ, C., Shedlock, A., Meade, A., Pagel, M., and Edwards, S. V. (2007). Origin of avian genome size and structure in non-avian dinosaurs. Nature 446, 180–184. doi: 10.1038/nature05621
Paradis, E., and Schliep, K. (2019). Ape 5.0: an environment for modern phylogenetics and evolutionary analyses in R. Bioinformatics 35, 526–528. doi: 10.1093/bioinformatics/bty633
Pardo, J. D., Lennie, K., and Anderson, J. S. (2020). Can We Reliably Calibrate Deep Nodes in the Tetrapod Tree? Case Studies in Deep Tetrapod Divergences. Front. Genet. 11:506749. doi: 10.3389/fgene.2020.506749
Pawley, K. (2006). The Postcranial Skeleton Of Temnospondyls (Tetrapoda: Temnospondyli). Ph.D. thesis. Melbourne: La Trobe University.
Poropat, S., Mannion, P., Upchurch, P., Hocknull, S. A., Kear, B. P., Kundrát, M., et al. (2016). New Australian sauropods shed light on Cretaceous dinosaur palaeobiogeography. Sci. Rep. 6:34467. doi: 10.1038/srep34467
Reisz, R. R. (1972). Pelycosaurian reptiles from the Middle Pennsylvanian of North America. Bull. Mus. Comp. Zool. Harvard. Univ. 144, 27–62.
Reisz, R. R. (1977). Petrolacosaurus, the oldest known diapsid reptile. Science 196, 1091–1093. doi: 10.1126/science.196.4294.1091
Reisz, R. R. (1981). A diapsid reptile from the Pennsylvanian of Kansas. Spec. Publ. Mus. Nat. Hist. Univ. Kans. 7, 1–74.
Reisz, R. R. (2007). in The Cranial Anatomy Of Basal Diadectomorphs And The Origin Of Amniotes, eds J. S. Anderson and H. D. Sues (Bloomington: Indiana University Press), 228–252.
Reisz, R. R., and Dilkes, D. W. (2003). Archaeovenator hamiltonensis, a new varanopid (Synapsida: eupelycosauria) from the Upper Carboniferous of Kansas. Can. J. Earth Sci. 40, 667–678. doi: 10.1139/e02-063
Reisz, R. R., and Fröbisch, J. (2014). The oldest caseid synapsid from the late Pennsylvanian of Kansas, and the evolution of herbivory in terrestrial vertebrates. PLoS One 9:e94518. doi: 10.1371/journal.pone.0094518
Royer-Carenzi, M., and Didier, G. (2016). A comparison of ancestral state reconstruction methods for quantitative characters. J. Theor. Biol. 404, 126–142. doi: 10.1016/j.jtbi.2016.05.029
Ruta, M., and Coates, M. I. (2007). Dates, nodes and character conflict: addressing the Lissamphibian origin problem. J. Syst. Palaeontol. 5, 69–122. doi: 10.1017/s1477201906002008
Ruta, M., Coates, M. I., and Quicke, D. L. (2003a). Early tetrapod relationships revisited. Biol. Rev.Camb. Philos. Soc. 78, 251–345. doi: 10.1017/s1464793102006103
Ruta, M., Jeffery, J. E., and Coates, M. I. (2003b). A supertree of early tetrapods. Proc.R. Soc. B 270, 2507–2516. doi: 10.1098/rspb.2003.2524
Schluter, D., Price, T., Mooers, A. O., and Ludwig, D. (1997). Likelihood of ancestor states in adaptive radiation. Evolution 51, 1699–1711. doi: 10.1111/j.1558-5646.1997.tb05095.x
Schmidt, H. (1956). Die große Bochumer Oberkarbon-Fährte. Paläontol. Z. 30, 199–206. doi: 10.1007/bf03041784
Schneider, J. W., Lucas, S. G., Scholze, F., Voigt, S., Marchetti, L., Klein, H., et al. (2020). Late Paleozoic–early Mesozoic continental biostratigraphy — links to the Standard Global Chronostratigraphic Scale. Palaeoworld 29, 186–238. doi: 10.1016/j.palwor.2019.09.001
Smithson, T. R., and Clack, J. (2017). A new tetrapod from Romer’s gap reveals an early adaptation for walking. Earth Environ. Sci. Trans.R. Soc. Edinb. 108, 89–97. doi: 10.1017/s1755691018000075
Soergel, W. (1925). Die Fährten der Chirotheria. Eine paläobiologische Studie. Jena: Gustav Fischer Verlag.
Spindler, F., Werneburg, R., and Schneider, J. W. (2019). A new mesenosaurine from the lower Permian of Germany and the postcrania of Mesenosaurus: implications for early amniote comparative osteology. Paläontol. Z. 93, 303–344. doi: 10.1007/s12542-018-0439-z
Sumida, S. S. (1990). Vertebral morphology, alternation of neural spine height, and structure in Permo-Carboniferous tetrapods, and a reappraisal of primitive modes of terrestrial locomotion. Univ. Calif. Publ. Zool. 122, 1–144.
Sundberg, F. A., Bennington, J. B., Wizevich, M. C., and Bambach, R. K. (1990). Upper Carboniferous (Namurian) amphibian trackways from the Bluefield Formation. West Virginia, USA. Ichnos 1, 111–124. doi: 10.1080/10420949009386340
Tuinen, M. V., and Hadly, E. A. (2004). Error in Estimation of Rate and Time Inferred from the Early Amniote Fossil Record and Avian Molecular Clocks. J. Mo. Evol. 59, 267–276. doi: 10.1007/s00239-004-2624-9
Vallin, G., and Laurin, M. (2004). Cranial morphology and affinities of Microbrachis, and a reappraisal of the phylogeny and lifestyle of the first amphibians. J. Vertebr. Paleontol. 24, 56–72. doi: 10.1671/5.1
Voigt, S. (2005). Die Tetrapodenichnofauna Des Kontinentalen Oberkarbon Und Perm Im Thüringer Wald – Ichnotaxonomie, Paläoökologie Und Biostratigraphie. Göttingen: Cuvillier Verlag.
Voigt, S. (2015). Der Holotypus von Amphisauropus latus Haubold, 1980 – ein besonderes Objekt permischer Tetrapodenfährten im Naturhistorischen Museum Schloss Bertholdsburg Schleusingen. Semana 30, 39–49.
Voigt, S., Berman, D. S., and Henrici, A. C. (2007). First well-established track-trackmaker association of Paleozoic tetrapods based on Ichniotherium trackways and diadectid skeletons from the Lower Permian of Germany. J. Vertebr. Paleontol. 27, 553–570. doi: 10.1671/0272-4634(2007)27[553:fwtaop]2.0.co;2
Voigt, S., and Ganzelewski, M. (2010). Toward the origin of amniotes: diadectomorph and synapsid footprints from the early Late Carboniferous of Germany. Acta Palaeontol. Pol. 55, 57–72. doi: 10.4202/app.2009.0021
Voigt, S., and Haubold, H. (2000). Analyse zur Variabilität der Tetrapodenfährte Ichniotherium cottae aus dem Tambacher Sandstein (Rotliegend. U-Perm, Thüringen). Hallesches Jahrbuch für Geowissenschaften B 22, 17–58.
Voigt, S., and Haubold, H. (2015). Permian tetrapod footprints from the Spanish Pyrenees. Palaeogeogr. Palaeoclimatol. Palaeoecol. 417, 112–120. doi: 10.1016/j.palaeo.2014.10.038
Voigt, S., Hminna, A., Saber, H., Schneider, J. W., and Klein, H. (2010). Tetrapod footprints from the uppermost level of the Permian Ikakern Formation (Argana basin, western High Atlas, Morocco). J. Afr. Earth Sci. 57, 470–478. doi: 10.1016/j.jafrearsci.2009.12.003
Voigt, S., and Lucas, S. G. (2015). Permian tetrapod ichnodiversity of the Prehistoric Trackways National Monument (south-central New Mexico, USA). New Mexico Museum Nat. History Sci. Bull. 65, 153–167.
Voigt, S., and Lucas, S. G. (2018). Outline of a Permian tetrapod footprint ichnostratigraphy. Geol. Soc. London Spec. Publ. 450, 387–404. doi: 10.1144/sp450.10
Voigt, S., Lucas, S. G., Buchwitz, M., and Celeskey, M. D. (2013). Robledopus macdonaldi, a new kind of basal eureptile footprint from the early Permian of New Mexico. New Mexico Museum Nat. History Sci. Bull. 60, 445–459.
Voigt, S., Small, B., and Sanders, F. (2005). A diverse terrestrial ichnofauna from the Maroon formation (Pennsylvanian–Permian), Colorado: biostratigraphic and paleoecological significance. New Mexico Museum Nat. History Sci. Bull. 30, 342–351.
Wilson, J. A. (2005). Integrating ichnofossil and body fossil records to estimate locomotor posture and spatiotemporal distribution of early sauropod dinosaurs: a stratocladistic approach. Paleobiology 31, 400–423. doi: 10.1666/0094-8373(2005)031[0400:iiabfr]2.0.co;2
Witzmann, F., and Schoch, R. R. (2017). Skull and postcranium of the bystrowianid Bystrowiella schumanni from the Middle Triassic of Germany, and the position of chroniosuchians within Tetrapoda. J. Syst. Palaeontol. 16, 711–739. doi: 10.1080/14772019.2017.1336579
Yang, Z., Jiang, B., McNamara, M. E., Kearns, S. L., Pittman, M., Kaye, T. G., et al. (2019). Pterosaur integumentary structures with complex feather-like branching. Nat. Ecol. Evol. 3, 24–30. doi: 10.1038/s41559-018-0728-7
Zaneveld, J. R., and Thurber, R. L. V. (2014). Hidden state prediction: a modification of classic ancestral state reconstruction algorithms helps unravel complex symbioses. Front. Microbiol. 5:431. doi: 10.3389/fmicb.2014.00431
Keywords: tetrapod ichnology, functional morphology, Carboniferous, Permian, Cotylosauria
Citation: Buchwitz M, Jansen M, Renaudie J, Marchetti L and Voigt S (2021) Evolutionary Change in Locomotion Close to the Origin of Amniotes Inferred From Trackway Data in an Ancestral State Reconstruction Approach. Front. Ecol. Evol. 9:674779. doi: 10.3389/fevo.2021.674779
Received: 01 March 2021; Accepted: 13 April 2021;
Published: 07 May 2021.
Edited by:
Michel Laurin, UMR 7207 Centre de Recherche sur la Paléobiodiversité et les Paléoenvironnements (CR2P), FranceReviewed by:
Jean-David Moreau, UMR 6282 Biogéosciences, FranceAbdelouahed Lagnaoui, Hassan Premier University, Morocco
Copyright © 2021 Buchwitz, Jansen, Renaudie, Marchetti and Voigt. This is an open-access article distributed under the terms of the Creative Commons Attribution License (CC BY). The use, distribution or reproduction in other forums is permitted, provided the original author(s) and the copyright owner(s) are credited and that the original publication in this journal is cited, in accordance with accepted academic practice. No use, distribution or reproduction is permitted which does not comply with these terms.
*Correspondence: Michael Buchwitz, bWljaGFlbC5idWNod2l0ekBtdXNlZW4ubWFnZGVidXJnLmRl; Maren Jansen, bWFyZW4uamFuc2VuQG1mbi1iZXJsaW4uZGU=