- 1Department of Biosciences, Centre for Ecological and Evolutionary Synthesis (CEES), University of Oslo, Oslo, Norway
- 2School of Biological Sciences, Victoria University of Wellington, Wellington, New Zealand
- 3McDonald Institute for Archaeological Research, Department of Archaeology, University of Cambridge, Cambridge, United Kingdom
- 4Department of Archaeology, Cultural Heritage Agency of the Netherlands, Amersfoort, Netherlands
- 5Department of Historical and Classical Studies, Norwegian University of Science and Technology (NTNU), Trondheim, Norway
- 6Department of Archaeology and Cultural History, Norwegian University of Science and Technology (NTNU) University Museum, Norwegian University of Science and Technology, Trondheim, Norway
- 7Archaeology Institute, University of the Highlands and Islands, Orkney, United Kingdom
- 8Oxford Archaeology, Oxford, United Kingdom
- 9Department of Archaeology & Anthropology, Bournemouth University, Poole, United Kingdom
- 10Marine Scotland Science, Aberdeen, United Kingdom
- 11Centre for Baltic and Scandinavian Archaeology (ZBSA), Schleswig, Germany
- 12BioArCh, Department of Archaeology, University of York, York, United Kingdom
- 13Department of Natural History, The University Museum, University of Bergen, Bergen, Norway
Ancient DNA (aDNA) approaches have been successfully used to infer the long-term impacts of climate change, domestication, and human exploitation in a range of terrestrial species. Nonetheless, studies investigating such impacts using aDNA in marine species are rare. Atlantic cod (Gadus morhua), is an economically important species that has experienced dramatic census population declines during the last century. Here, we investigated 48 ancient mitogenomes from historical specimens obtained from a range of archeological excavations in northern Europe dated up to 6,500 BCE. We compare these mitogenomes to those of 496 modern conspecifics sampled across the North Atlantic Ocean and adjacent seas. Our results confirm earlier observations of high levels of mitogenomic variation and a lack of mutation-drift equilibrium—suggestive of population expansion. Furthermore, our temporal comparison yields no evidence of measurable mitogenomic changes through time. Instead, our results indicate that mitogenomic variation in Atlantic cod reflects past demographic processes driven by major historical events (such as oscillations in sea level) and subsequent gene flow rather than contemporary fluctuations in stock abundance. Our results indicate that historical and contemporaneous anthropogenic pressures such as commercial fisheries have had little impact on mitogenomic diversity in a wide-spread marine species with high gene flow such as Atlantic cod. These observations do not contradict evidence that overfishing has had negative consequences for the abundance of Atlantic cod and the importance of genetic variation in implementing conservation strategies. Instead, these observations imply that any measures toward the demographic recovery of Atlantic cod in the eastern Atlantic, will not be constrained by recent loss of historical mitogenomic variation.
Introduction
Continuous human activities and a changing climate have influenced terrestrial and marine ecosystems for millennia (Venter et al., 2016; Rodrigues et al., 2019; Mitchell and Rawlence, 2021), impacting the evolutionary potential and population demography of a range of species (Seersholm et al., 2018). Ancient mitochondrial DNA (mtDNA) has been widely used to understand long-term genomic consequences of such impacts (Shapiro et al., 2004; Nyström et al., 2006; Stiller et al., 2010; Paijmans et al., 2013; Fortes and Paijmans, 2015; Casas-Marce et al., 2017). Nonetheless, most ancient mtDNA studies have focused on terrestrial species, and studies that investigate the impacts of long-term human activities and/or climatic variation on fish, using whole genome sequencing approaches, are relatively rare. Long-term commercial fisheries—covering many centuries—have contributed to the decline of economically and ecologically important marine species (Exadactylos et al., 2007; Pinnegar and Engelhard, 2008; Barrett, 2019). The consequences of intensive fishing in recent times may be difficult to assess as this requires an understanding of historical population dynamics (Selim et al., 2016). The analysis of long-term biological and demographic fluctuations can therefore help to improve guidelines for sustainable fisheries management and optimal conservation measures (Barrett, 2019). In order to provide a long-term perspective on fishing exploitation impacts, the use of archeological evidence, such as fish bone remains, is essential for those periods for which little or no historical data are available. Recent developments in whole genome aDNA methods now allow the inference of demographic histories and the estimation of genetic fluctuations over time from fishbone samples (Oosting et al., 2019; Ferrari et al., 2021). Such combined molecular analyses of historical and modern samples can potentially provide an understanding of the association between human-environmental impact and population declines (Hofman et al., 2015).
Several studies have shown the utility of temporal mtDNA analyses in the marine environment. For instance, ancient mitogenomes have investigated impacts of climate and hunting on the Atlantic walrus (Star et al., 2018; Keighley et al., 2019; Barrett et al., 2020), narwhals (Louis et al., 2020), and the extinct great auk (Thomas et al., 2019). In fish, such studies remain limited to partial mitogenome data. For example, a shift in sturgeon species distributions was detected during the Holocene in the North East Atlantic based on CytB amplicon data (Nikulina and Schmölcke, 2016). Moreover, impacts of habitat destruction and human activities during the 1800s were associated with a reduction of the mtDNA diversity of Chinook salmon from the Columbia River in the 12S and control region by comparing ancient and modern samples (Johnson et al., 2018). Similarly, impacts of human exploitation and climate oscillations were associated with losses of haplotypic CytB variation in Atlantic cod during the 15th to 16th centuries in Iceland (Olafsdottir et al., 2014). In contrast, comparable levels of ancient mtDNA genetic diversity were found between ancient and modern samples of herring specimens, despite continuous human exploitation (Speller et al., 2012). Notwithstanding these examples, human-environmental impacts and population declines remain unclear for a wide range of marine species and populations.
Atlantic cod (Gadus morhua L. 1758) is a benthopelagic predatory fish with high reproductive rates and with a fundamental ecological role in marine ecosystems (Barth et al., 2017; Edvardsson et al., 2019). It has been one of the most exploited fish species in the North Atlantic Ocean (Carr et al., 1995; Árnason et al., 2000; Nicholls et al., 2021). The distribution of this species extends through the cold waters of North America, across the continental shelves of Greenland and Iceland, and northern Europe (Lait et al., 2018). Relatively large population sizes have been characteristic throughout its entire distribution even during the expansion of long-distance fish trading during the 12th to 13th centuries in the eastern Atlantic and at the beginning of the 16th century in the western Atlantic (Barrett et al., 2004, 2011; Orton et al., 2014; Castañeda et al., 2020). However, intensive fishing activities during the 20th century (Mieszkowska et al., 2009; Jonsson et al., 2016; Brattey et al., 2018) resulted in the severe depletion of several stocks, for instance the North Sea stock, which was decimated from annual landings of 354,000 to 50,000 tons during this period (Bannister, 2004). In addition to past human exploitation, climatic events like the Little Ice Age—a cooling period that varied regionally in timing and duration but occurred between ca. 1300–1850 CE—may have caused large declines between the sixteenth and 17th centuries (Edvardsson et al., 2019).
The genomic consequences of such population dynamics and declines in Atlantic cod remain unclear. Based on partial and whole mtDNA data, Atlantic cod populations between the western and eastern Atlantic Ocean show significant structure (Árnason, 2004; Jørgensen et al., 2018; Lait et al., 2018), whereas low to no mtDNA differentiation has been found across a wide range of eastern Atlantic locations (Carr et al., 1995; Árnason and Palsson, 1996; Árnason et al., 1998, 2000; Sigurgíslason and Árnason, 2003). Here, we compared modern and ancient Atlantic cod mitogenomes—dated up to 6500 BCE—from different fishing locations in northern Europe. We evaluated whether Atlantic cod in the eastern Atlantic have experienced any loss of genetic variation, analyzed long term patterns of effective population size, and related any observed decline to the impact of commercial fisheries or climate change.
Materials and Methods
Sample Collection
Ancient samples of Atlantic cod (n = 48) were obtained from 11 excavation sites (Figure 1 and Supplementary Table 1) and were stored dry and unfrozen. The specimens were all supplied by the relevant archeological organizations, or sampled with permission on their premises. The shipment of Atlantic cod bones does not require CITES or other wildlife regulation permits for transport or analysis. Where practicable, only a subsample of bone was employed for the aDNA research, leaving material for other studies. Dating of the samples (Supplementary Table 1) was based on archeological context. Ancient samples were morphologically and genetically identified as Atlantic cod. A total of 472 available modern mitogenomes were obtained from Jørgensen et al. (2018), Lait et al. (2018), and Barth et al. (2019). Novel mtDNA sequence data from modern specimens sampled in 2016 in Orkney, United Kingdom (n = 24) were also included (Figure 1 and Supplementary Table 2). The collection of the Orkney specimens complied with the Nagoya Protocol and Convention on Biological Diversity, which the United Kingdom signed up to in 2016. All specimens were deceased when the fin clip was collected.
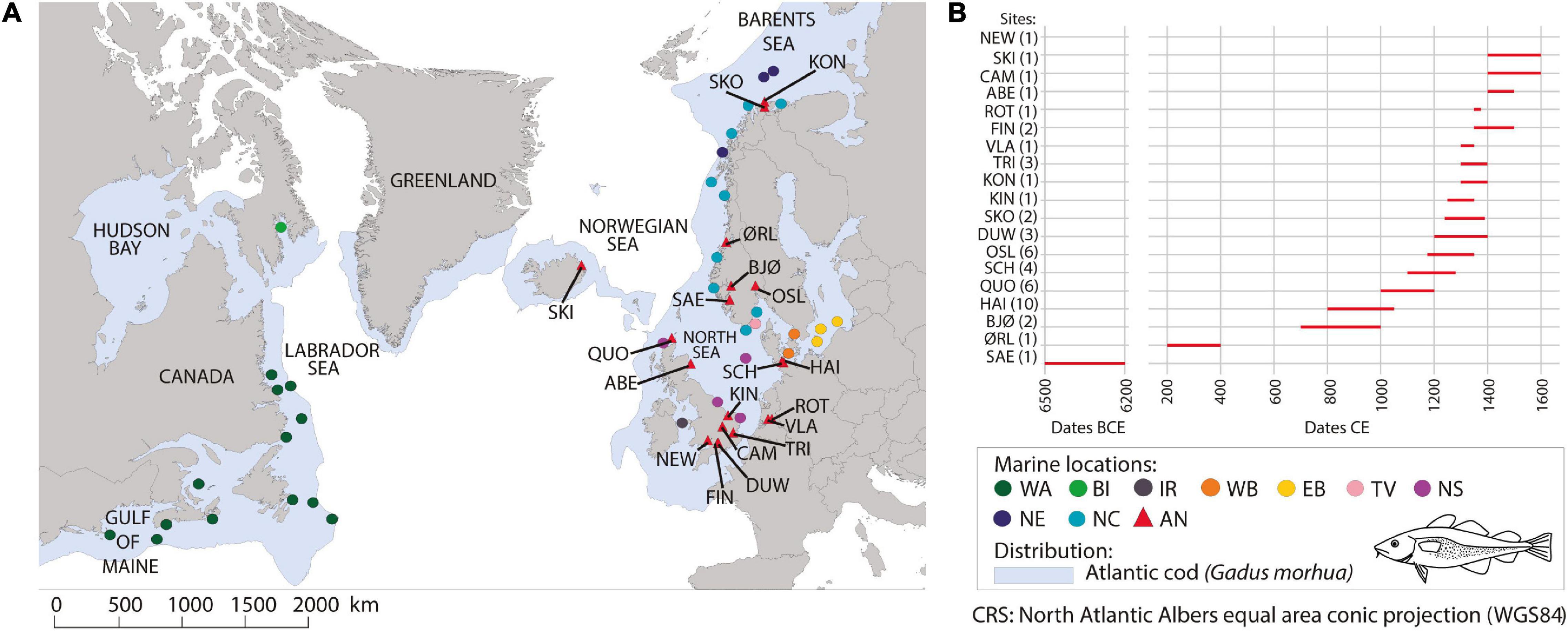
Figure 1. (A) Ancient and modern Atlantic cod sampling locations. The abbreviated name of archeological sites is shown in the map (see also Supplementary Table 1). Modern data were obtained from Jørgensen et al. (2018); Lait et al. (2018) and Barth et al. (2019). Sample sites are grouped according to larger oceanographic location, ecotype and modern or ancient specimens: western Atlantic (WA) = dark green, Baffin Island (BI) = light green, Irish Sea (IS) = gray, North Sea (NS) = purple, western Baltic (WB) = orange, eastern Baltic (EB) = yellow, Tvedestrand fjord (TV) = pink, North East Arctic (NE) = dark blue, Norwegian coast (NC) = blue, and Ancient specimens (AN) = red (i.e., Saevarhelleren = SAE, Ørland Kampflybase = ØRL, Bjørkum = BJØ, Haithabu = HAI, Quoygrew = QUO, Schleswig Schild = SCH, Oslo Mindets Tomt = OSL, Bristol Dundas Wharf = DUW, Skonsvika = SKO, King’s Lynn Raynham House = KIN, Kongshavn = KON, London Trig Lane = TRI, Vlaardingen Gat in de Markt = VLA, Bristol Finzel’s Reach = FIN, Rotterdam, Hoogstraat 13–26 = ROT, Aberdeen = ABE, Cambridge Grand Arcade = CAM, Skriðuklaustur = SKI, Newport Ship = NEW). Modern distribution of Atlantic cod is shown in light blue. Fish illustration drawn by Geir Holm. (B) Cleveland plot showing the age range of ancient samples. Sample age is estimated based on archeological context. The number of individuals per site is represented in brackets after the site name (Supplementary Table 1).
DNA Extraction, Amplification and Sequencing
DNA extraction and library preparation from ancient samples were performed in the aDNA laboratory at the University of Oslo under rigorous measures (Cooper and Poinar, 2000; Gilbert et al., 2005). All ancient samples were processed with the same DNA extraction and library protocols according to Ferrari et al. (2021). In short, bones were UV-treated for 10 min per side and pulverized using a stainless-steel mortar (Gondek et al., 2018). Per specimen, two aliquots containing between 150 and 200 mg of bone powder were used as starting material for DNA extraction. Double-indexed blunt-end sequencing libraries were built from 15 to 16 μl of DNA extract using the Meyer-Kircher protocol (Meyer and Kircher, 2010; Kircher et al., 2012) with the modifications listed in Schroeder et al. (2015) and the single-tube (BEST) protocol (Carøe et al., 2018) with the modifications described in Mak et al. (2017). Sequencing reads were processed using PALEOMIX v1.2.13 (Schubert et al., 2014). Trimming of residual adapter contamination, filtering and collapse of reads was done using AdapterRemoval v.2.1.7 (Lindgreen, 2012). Sequencing reads shorter than 25 bp were discarded. Mapping of remaining reads was performed against the Atlantic cod GadMor3.0 nuclear genome (RefSeq assembly accession GCF_902167405.1; Star et al., 2011; Tørresen et al., 2017) and mitochondrial genome (Johansen and Bakke, 1996) using BWA v.0.7.12 (Li and Durbin, 2009) with the aln algorithm, disabled seeding and minimum quality score of 25. The resulting BAM files were indexed with samtools v.1.9 (Li et al., 2009) and DNA postmortem damage assessed using MapDamage v.2.0.9 (Jónsson et al., 2013). DNA from modern Orkney samples were extracted using a DNeasy Blood & Tissue kit (Qiagen). Libraries were assembled with a TrueSeq DNA PCR-Free Preparation Kit and sequenced on an Illumina HiSeq 2,500. Modern alignment—including Orkney and Barth et al. (2019) samples, and the outgroup Alaska pollock (Gadus chalcogrammus; Malmstrøm et al., 2016) – was carried out using BWA v.0.7.12 with the mem algorithm, and a minimum quality score of 25.
Mitogenomic Analysis
Variant calling was performed using GATK v.4.1.4. (McKenna et al., 2010) simultaneously in all ancient, modern Orkney and Barth et al. (2019) samples, including the outgroup. gVCF files were created for each sample using GATK HaplotypeCaller (with ploidy set to 1). Individual genotypes were combined in one file using GATK CombineGVCFs and GenotypeGVCFs. Filtering was performed using bcftools v.1.9. (Li et al., 2009) and vcftools v.0.1.16. (Danecek et al., 2011) with the following thresholds: FS < 60.0, SOR < 4, MQ > 30.0, QD > 2.0, SnpGap = 10, minGQ = 15, minDP = 3, remove indels = yes, biallelic loci = yes, meanDP < 30 and read depth > 3. Consensus sequences were built using bcftools consensus and aligned using MAFFT v.7.429 (Katoh and Standley, 2013). Available modern mitogenomes obtained from Jørgensen et al. (2018) and Lait et al. (2018) were manually inspected using MEGA v.7 (Kumar et al., 2016) to set as missing the consistent nucleotide differences (between 50 and 100%; Supplementary Table 3) between their Illumina, Sanger and/or Roche 454 technologies with the Illumina sequenced mitogenomes in this study. Control region and half of the tRNA-Pro sequences from all the mitogenomes were excluded from further analyses as these two regions were not fully complete (i.e., 15,696–15,815 positions) after aligning sequences obtained from Jørgensen et al. (2018) and Lait et al. (2018) with the sequences presented in this study and Barth et al. (2019) samples. Thus, all sequences analyzed had 15,695 bp in length. Validated SNPs were annotated as transversion and/or transition using SNP-sites (Page et al., 2016). Checked and modified modern sequences (Jørgensen et al., 2018; Lait et al., 2018) were added and aligned to our multi-fasta alignment using MAFFT v.7.429. Unique sequences were identified with IQTREE v.1.6.12 (Nguyen et al., 2015). File formats required for different software and/or packages were obtained with seqinr and ape (i.e., nexus format; Paradis and Schliep, 2019; Charif et al., 2020), and phylotools (i.e., phylip format; Zhang et al., 2017) packages implemented in R.
Different sample combinations were used to compare the genetic diversity of the ancient samples to those of the modern conspecifics. Given the low spatial structure in the eastern Atlantic region (Árnason and Palsson, 1996; Árnason et al., 1998; Sigurgíslason and Árnason, 2003) and lack of consistent spatial structure amongst specimens (Supplementary Figures 3, 5, 6), all 48 ancient samples were compared as a single group to modern samples grouped into larger marine locations (according to their geographical proximity or ecotype; Figure 1). In addition, a comparison of subsets of multiple specimens from two archeological locations (Quoygrew and Haithabu) for which a more specific temporal pair from the same geographical region could be identified, was performed (Supplementary Table 1). Quoygrew specimens were locally sourced (Harland and Barrett, 2012; Star et al., 2017). Therefore, modern specimens sampled in the same area (i.e., modern Orkney) provide a logical, spatially consistent temporal comparison. However, specimens from Haithabu, were sourced from northern Norway (Star et al., 2017), and belonged to the North East Arctic ecotype. For these traded specimens, the North East Arctic ecotypes provide a spatially relevant temporal comparison, rather than North Sea or western Baltic specimens.
Haplotype (h) and nucleotide diversities (π), number of haplotypes (Nh) and number of polymorphic sites (S) were calculated using DnaSP v.6 (Rozas et al., 2017). To allow direct comparison with earlier CytB results (Árnason, 2004; Olafsdottir et al., 2014; Jørgensen et al., 2018), specific CytB haplotypes based on 250 bp gene fragment as previously reported by Árnason (2004) were identified using MEGA v.7. Demographic histories were determined by Tajima’s D (TD) and Fu’s F (F) neutrality in DnaSP v.6. A different number of specimens were obtained for ancient and modern locations. We corrected for such differences in sample size by randomly downsampling the modern specimens for each of the temporal comparisons (North East Arctic and Orkney) using 1,000 bootstrap replicates. A 95% confidence interval of the genetic parameters; genetic variation (π) and patterns of population demography (TD and F) was calculated from these 1,000 bootstrap replicates that were sampled using a without replacement approach with the sample function implemented in R (R Core Team, 2020) and the fasta.sample function in the FastaUtils package also in R (Salazar, 2020). For the bootstrapping test, π, TD and F from temporally spaced modern locations were re-calculated with the pegas (Paradis, 2010) and PopGenome (Pfeifer et al., 2020) packages implemented in R. Relationships among ancient and modern samples were visualized for whole mitogenome and CytB sequence data, by constructing a mitochondrial haplotype-genealogy graph using Fitchi (Matschiner, 2016) with the ML-based phylogenetic tree obtained with IQTREE v.1.6.12 as input.
Population Dynamics and Demographic Reconstruction
An analysis of molecular variance (AMOVA, 1,000,000 permutations) and population pairwise genetic distances (ΦST) were obtained in Arlequin v.3.5 (Excoffier and Lischer, 2010), to determine the distribution of variation between marine locations and temporally spaced locations. Divergence and coalescent analyses were based on unique sequences only (n = 525 sequences including the outgroup). Substitution model selection for unique sequences was performed using PHYML v.3.1 (Guindon et al., 2010) as implemented in JMODELTEST v.2.1.10 (Guindon and Gascuel, 2003; Darriba et al., 2012). Model selection was determined on the following partitions: 1st, 2nd, and 3rd codons from protein coding regions, rRNAs and tRNAs. Best-fitting models were selected according to the Aikaike Information Criterion (AIC; Supplementary Table 4). Based on these results, phylogenetic estimates were obtained using BEAST v.2.6.3 (Bouckaert et al., 2019).
Bayesian settings for all phylogenetic analyses included two sets of partitions: coding region and non-coding region. Three independent runs to test for chain convergence were run under the Coalescent Constant Population Tree Prior. Tip ages (ancient and modern dates) were included for each set of runs (Supplementary Tables 1, 2). Sample dates for ancient specimens were rounded to a midpoint date—from a given range—where necessary. To achieve high effective sample sizes (ESS = ≥ 200), chain lengths were run 800,000,000 under a substitution rate of 1.14 × 10–8 substitution/site/year as per Lait (2016) assuming a GTR + I (for coding regions) and TIM1 + I (for non-coding regions) models of evolution and a strict clock. Tracer v.1.71 (Rambaut et al., 2018) was used to check for convergence of MCMC and to ensure sufficient sampling. Consensus trees were obtained using TreeAnnotator v.2.6.2—implemented in BEAST v.2.6.3—after 10% burn-in. Final phylogenetic trees were viewed and edited in FigTree v.1.4.4.
Finally, a Coalescent Bayesian Skyline (CBS) analysis was completed to reconstruct the demographic history—including female effective population size (Ne) —of Atlantic cod through time. To assess any confounding effect of past or contemporary population structure (Heller et al., 2013), we analyzed demographic history using 6 different data sets (excluding the outgroup): (I) all 524 sequences, (II) 476 modern sequences (excluding 48 ancient samples), (III) 273 sequences (excluding clades associated with most western Atlantic and Baltic Sea samples), (IV) 368 sequences (excluding the clade associated with most Baltic Sea samples), (V) 429 sequences (excluding clades associated with most western Atlantic samples) and (VI) 48 ancient sequences (excluding all modern samples). The specific clades that were excluded in III, IV and V can be found in Supplementary Figure 4. We used the same MCMC sampling procedure described before with 3 independent runs reaching convergence at high effective sample sizes (ESS = ≥ 200). Chain lengths were run 800,000,000 for data sets I, II and V with a number of bPopSize and bGroupSize of 10; while chain length for data sets III and IV were run 500,000,000 and 50,000 for data set VI with a number of bPopSize and bGroupSize of 5.
Results
Mitogenomic Variation
Sequencing reads from all ancient specimens showed the expected patterns of DNA fragmentation and deamination rates that were consistent with those of authentic aDNA (Supplementary Figure 1). We obtained 48 mitogenomes with at least 3-fold average coverage. We also obtained mitogenomes for 24 modern Orkney specimens (Supplementary Table 2). A total of 2135 SNPs (∼13% of mitogenome positions) were identified among all 545 samples – including the outgroup species Alaska pollock –: 1219 SNPs corresponded to informative sites and 916 SNPs were singletons (Supplementary Table 5).
Nucleotide diversity (π) between modern locations ranged between 0.002 and 0.003 (Table 1) and π of ancient samples did not vary from the values obtained in modern locations. The temporal comparison of specific sites (Quoygrew-Orkney and Haithabu-North East Arctic), showed limited significant differences between genetic statistics of temporally spaced ancient and modern locations (Supplementary Table 6 and Supplementary Figure 2), where Haithabu has significantly lower π and higher F compared to the North East Arctic (Supplementary Figure 2).
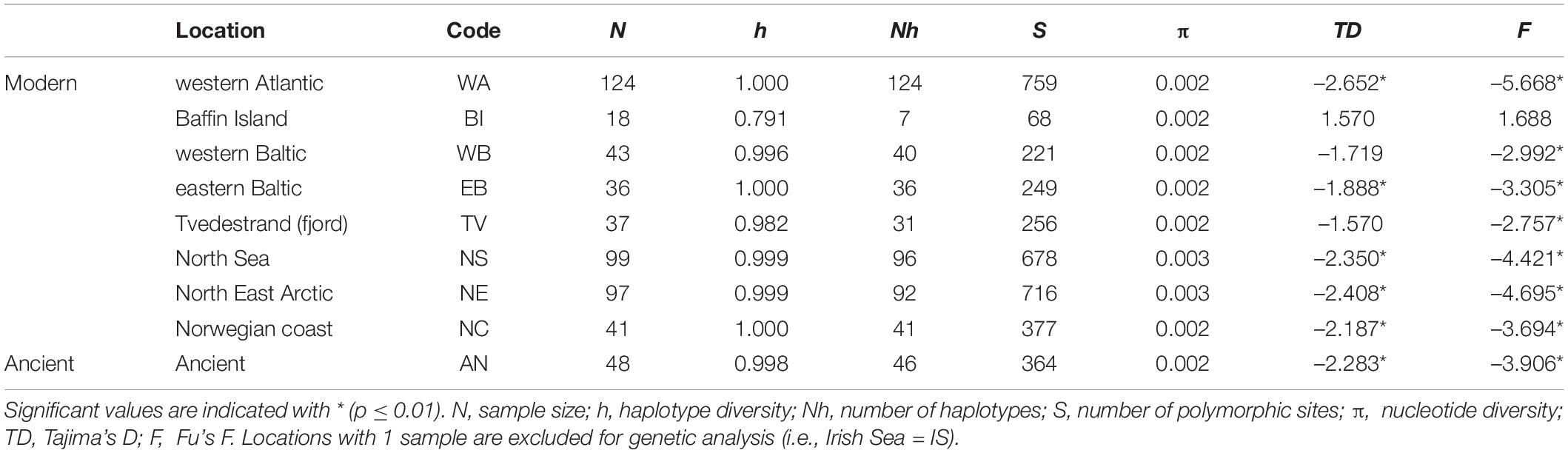
Table 1. Estimates of genetic diversity statistics for Atlantic cod at whole mitogenomes from different marine locations or ecotypes in the North Atlantic (see Supplementary Tables 1, 2, 5).
Neutrality tests showed significant negative values for all Tajima’s D (TD) and F statistics in most locations, except for the western location Baffin Island, and the eastern locations Tvedestrand fjord and western Baltic (Table 1). Overall, there were 486 haplotypes —- including the outgroup—across all 545 samples, of which only 26 were shared between individuals (Figure 2 and Supplementary Table 7). Ancient CytB variation consisted of 7 different haplotypes, including four main haplotypes (A, C, D, and E) previously identified in modern mtDNA studies (Árnason, 2004; Jørgensen et al., 2018). Two novel variations of existing CytB haplotypes were found in western Baltic (haplotype ED) and North Sea (haplotype LI), while another 2 novel variations of existing CytB haplotypes were found among ancient samples (haplotypes LJ and TI). The most prevalent ancient haplotypes were A and E (∼ 40 and 38%, respectively, Supplementary Tables 1, 7), which were also commonly found in modern samples (Supplementary Table 8). The haplotype genealogy for whole mitogenome and CytB sequence data showed an extensive distribution of ancient samples across marine locations (Figure 2 and Supplementary Figure 4). Limited geographic mitogenome structure was observed, except for elevated divergence between western Atlantic and eastern Atlantic locations, and between locations in the western and eastern Baltic Sea and other eastern Atlantic locations (Figures 2B,C and Supplementary Figures 4B,C). A star-like topology is observed for the whole mitogenome and CytB genealogies (Figure 2 and Supplementary Figure 4).
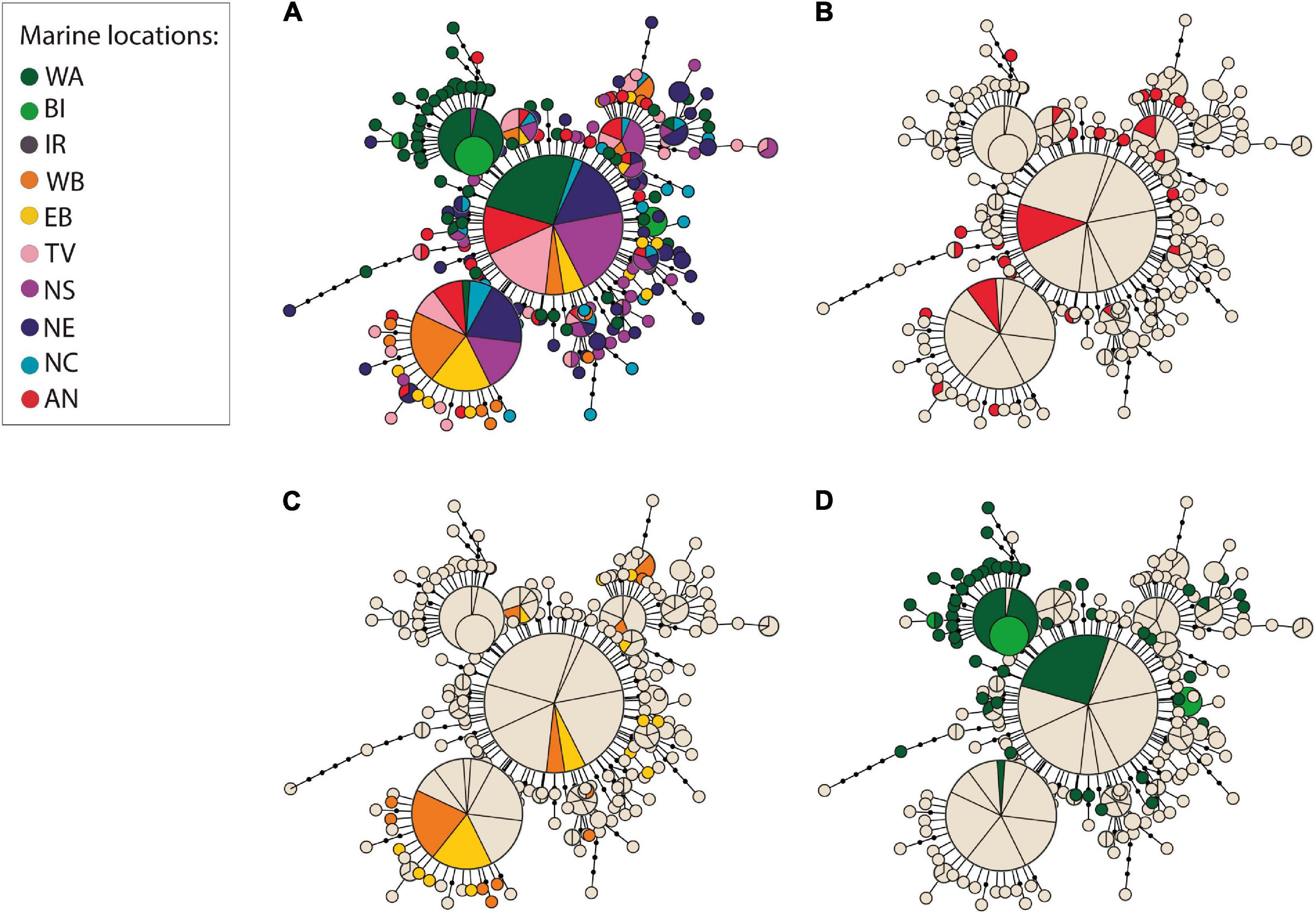
Figure 2. Star-like haplotype genealogy based on complete mitogenomes of 545 samples of Atlantic cod. Only transversions (336) are depicted in this genealogy. (A) Haplotype genealogy colored by location. (B) Haplotype genealogy highlighting ancient specimens as a single group (in red; see Figure 1 and Supplementary Table 1; for specific distribution of sampling sites of ancient samples see Supplementary Figure 3). (C) Haplotype genealogy highlighting samples from the western (orange) and eastern (yellow) Baltic. (D) Haplotype genealogy highlighting western Atlantic (green) and Baffin Island (light green) specimens. Circle size is proportional to haplotype frequency. Black dots indicate intermediate haplotypes not found among the specimens and black lines connecting black circles correspond to mutation steps between haplotypes.
Demographic Patterns and Population Structure
The AMOVA assigned 7.58% of the variation between marine locations (including ancient samples as a single group) while 91.47% of the variation was represented between individuals (ΦCT = 0.076, p ≤ 0.001; ΦST = 0.085, p ≤ 0.000). Pairwise ΦST values (Figure 3 and Supplementary Tables 9, 10) showed significant differentiation levels between all ancient samples and western Atlantic, Baffin Island, western Baltic, eastern Baltic and Tvedestrand fjord. Ancient samples showed higher differentiation when compared to western Atlantic (ΦST = 0.117), and Baffin Island (ΦST = 0.192) in comparison to other eastern Atlantic locations. Among modern samples, western Atlantic, Baffin Island, western Baltic and eastern Baltic showed significant ΦST values when compared to all other locations (Supplementary Tables 9, 10). ΦST values were not significant between North Sea, North East Arctic, Norwegian coast and Ancient samples. Pairwise ΦST values between temporal spaced locations also showed no significant differentiation (Quoygrew and modern Orkney: ΦST = 0.000; p = 0.807; and Haithabu and North East Arctic: ΦST = 0.000; p = 0.456).
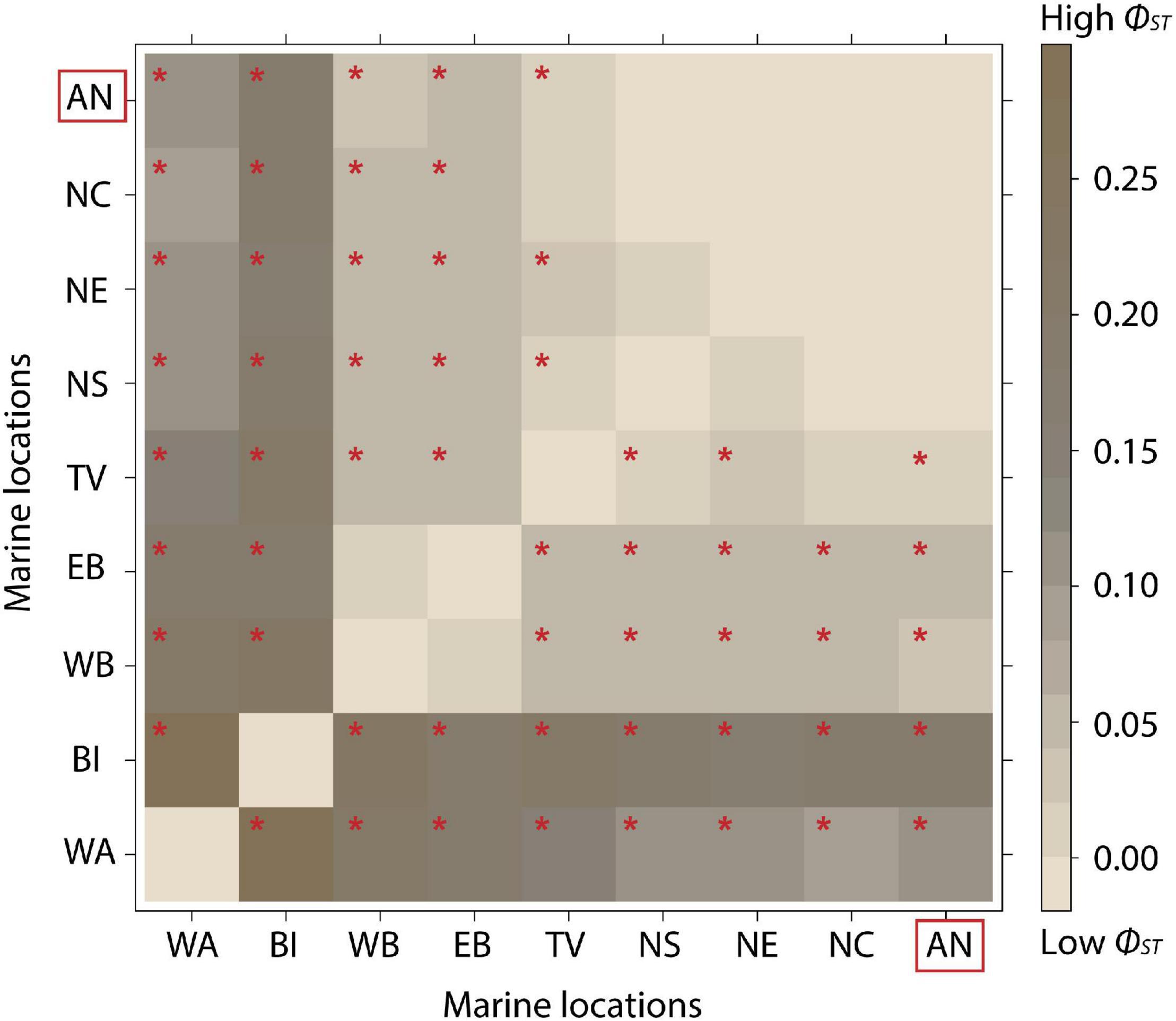
Figure 3. Heatmap of differentiation pairwise (ΦST) between 10 marine locations of Atlantic cod: western Atlantic (WA), Baffin Island (BI), western Baltic (WB), eastern Baltic (EB), Tvedestrand fjord (TV), North Sea (NS), North East Arctic (NE), Norwegian coast (NC), and Ancient specimens (AN). Dark brown colors indicate higher levels of differentiation (ΦST = 0.15–0.30). Light brown corresponds to lower levels of differentiation (ΦST = ≥ 0.10). Significant p-values (p ≤ 0.05) are indicated with a red *p-values are listed in Supplementary Tables 9, 10.
The time-calibrated Bayesian phylogeny for ancient and modern Atlantic cod samples resulted in 2 main clades with an estimated divergence from the most recent common ancestor at 220 kya (95% highest posterior density (HPD) = 194,780—249,980 kya; Figure 4). The first clade, which is not further divided, includes mitogenomes from 6 different widely scattered localities. The second clade was composed by 16 subclades with posterior probability > 0.8, with divergence times of ca. 100 kya. Clades and subclades in the phylogeny were not geographically structured, with the exception of most samples from western Atlantic, and most samples from western and eastern Baltic, which clustered together (Figures 2, 4).
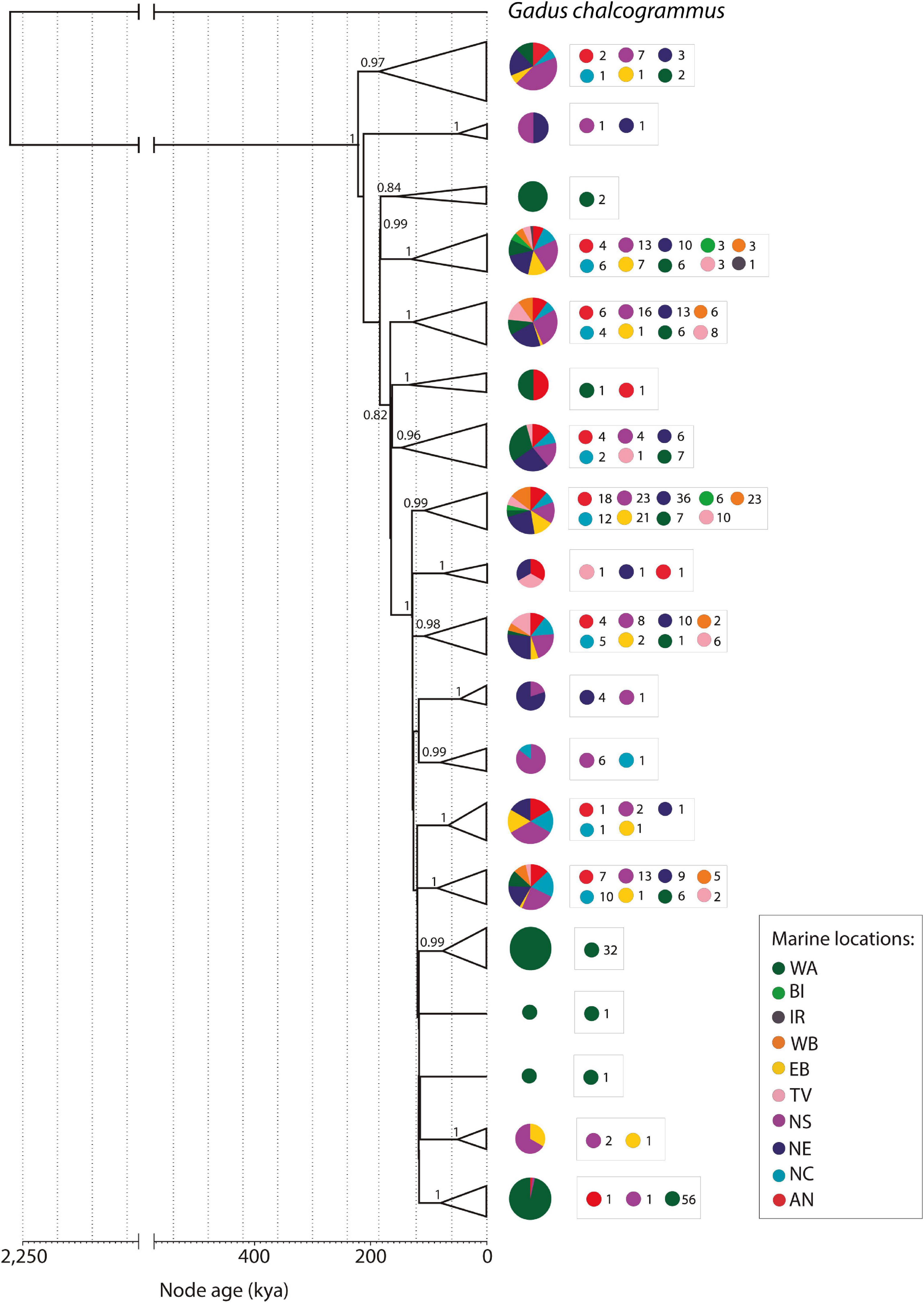
Figure 4. Time calibrated collapsed Bayesian phylogeny of full mitogenomes from 525 Atlantic cod specimens using Alaska pollock (Gadus chalcogrammus) as an outgroup. Pie charts represent the marine locations distributed in each clade. Numbers beside pie charts indicate the number of individuals from each marine location distributed in each clade. Only branches with posterior probability > 0.8 are indicated next to the corresponding clade/subclade. For specific distribution of sampling sites of ancient samples see Supplementary Figure 6.
The Bayesian skyline analysis using different subsets of the data revealed a consistent pattern of step-wise population expansions followed by periods of constant population size. Expansions around 150, 50, and 10 kya are present in most subsets (Figure 5). A population expansion of Atlantic cod was identified ca. 50 kya in all subsets. The most recent expansion identified (around 10 kya), is only present in data sets that include clades with most Baltic Sea specimens (Figures 5A,B,E). Despite such differences, all analyses agree with a high and increasing female effective population size (Ne) of Atlantic cod (Ne = ca. 1,000,000—10,000,000) during the last ca. 100 kya, with highest estimates of Ne during the last few millennia (Figure 5).
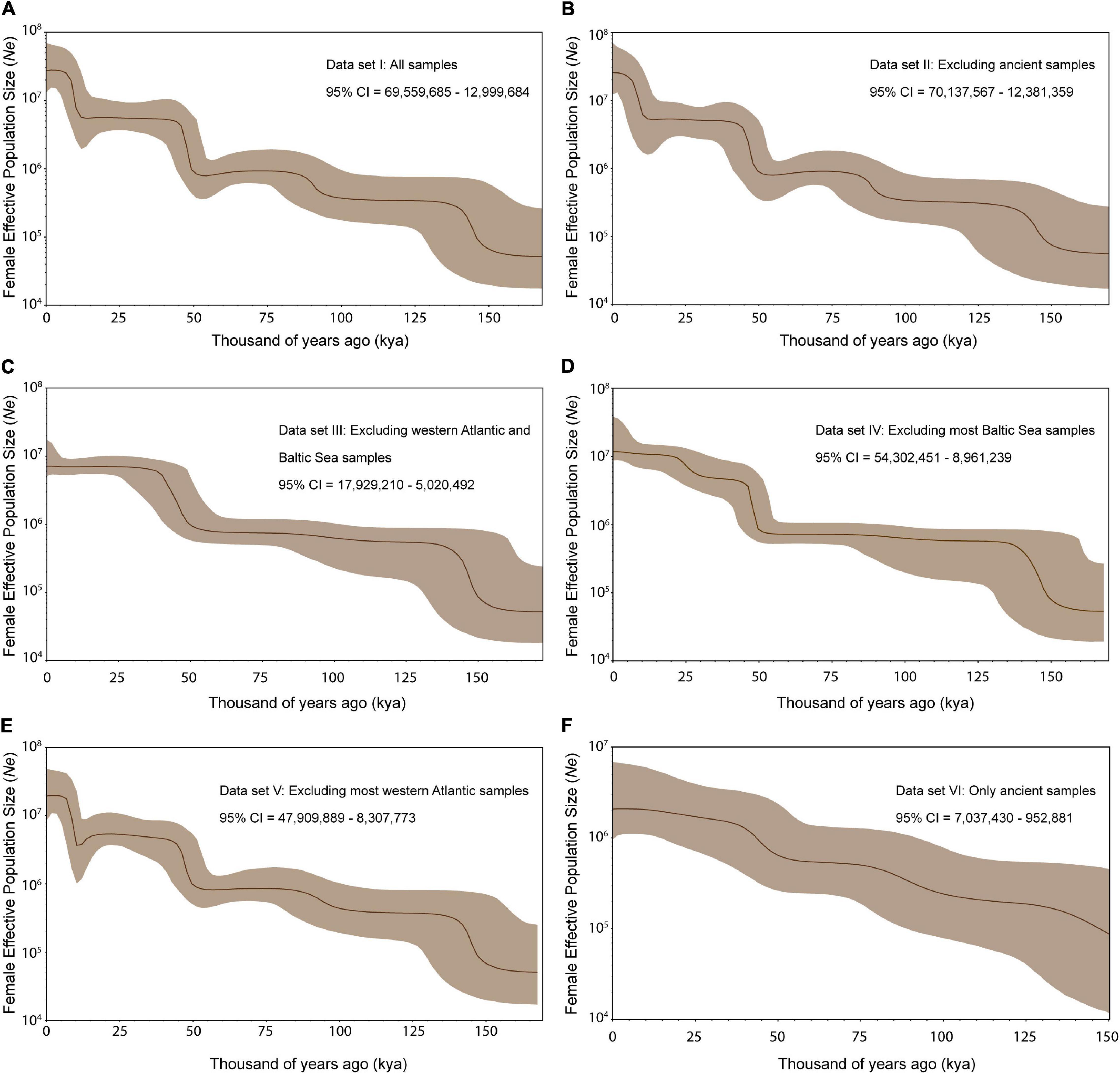
Figure 5. Atlantic cod demographic history representing the fluctuations of effective female population size (Ne; in dark brown line) based on (A) data set I: all 524 sequences, (B) data set II: 476 modern sequences (excluding 48 ancient samples), (C) data set III: 273 sequences (excluding clades associated with most western Atlantic and Baltic Sea samples), (D) data set IV: 368 sequences (excluding the clade associated with most Baltic Sea samples), (E) data set V: 429 sequences (excluding clades associated with most western Atlantic samples) and (F) data set VI: 48 ancient sequences (excluding all modern samples). The 95% CI corresponds to the light brown area. The most recent 95% CI at time 0 is shown in each respective panel. Description of the clades excluded in data sets III, IV, and V can be found in Supplementary Figure 7.
Discussion
Here, we compared modern and ancient mtDNA diversity in Atlantic cod to investigate whether observed historical and contemporaneous census population declines (Hutchinson et al., 2003; Hylen et al., 2008; Limburg et al., 2008; Bartolino et al., 2012; Jonsson et al., 2016; Brattey et al., 2018) have had mitogenomic consequences. The temporal comparison of 48 ancient specimens to 496 modern conspecifics did not reveal consistent significant mitogenomic changes or measurable effective genetic population declines through time. Below, we discuss reasons why such genomic impacts may not be observed.
First, mitogenomic variation is high in modern Atlantic cod and is characterized by limited genetic differentiation between populations and incomplete lineage sorting over large spatial scales across its range in the North Atlantic (Jørgensen et al., 2018; Lait et al., 2018). Low observed genetic differentiation (ΦST) between Tvedestrand fjord and other Norwegian coastal locations, as well as between the North Sea, the North East Arctic and the Norwegian coast confirm this lack of geographic structuring over large parts of the eastern Atlantic (Figure 3). Indeed, the non-significant differentiation of all ancient samples with modern North Sea, North East Arctic and Norwegian coast is fully consistent with their presumed geographical origin and highlights the long-term lack of mtDNA structure in this region. Non-significant ΦST values between the Norwegian coastal locations and Tvedestrand fjord indicate possible recent migration of fish between such coastal communities and more restricted fjord populations (Knutsen et al., 2011). Compared to many terrestrial ecosystems, where populations can often be isolated by physical barriers—which restrain interbreeding and dispersal—(Hauser and Carvalho, 2008; Exadactylos et al., 2019), in marine ecosystems the absence of physical barriers promotes larger panmictic populations and Atlantic cod is no exception (Berg et al., 2016, 2017; Sodeland et al., 2016; Barth et al., 2017). Thus, a combination of low spatial resolution of mtDNA data as a result of continuous gene flow and connectivity may mask any local temporal erosion of mitogenomic diversity (Welch et al., 2012) in Atlantic cod.
Second, we determined high long-term estimates of effective population size (Ne = ca. 1,000,000–10,000,000; Figure 5), which is in agreement with earlier observations in Atlantic cod (Hardie et al., 2006; Therkildsen et al., 2010; Pinsky et al., 2021). Estimates of Ne can remain high in economically important fish species, even if their populations have experienced a large biomass decline (Hauser and Carvalho, 2008) since it takes hundreds of generations (i.e., depending on the generation time of the species; Amos and Balmford, 2001; Frankham et al., 2002) for the actual population numbers and breeding populations to be reflected in Ne (Hauser and Carvalho, 2008). In fact, simulations have shown that a population with theoretical Ne of 100 (which is several orders of magnitude lower than observed in Atlantic cod) would retain 75% of heterozygosity after 57 generations (Frankham et al., 2002; Welch et al., 2012). Given that such population declines take a very long time to lead to measurable genomic consequences, mtDNA—as a single locus—will have limited power to record such changes in populations of high Ne (Allentoft et al., 2014; Johnson et al., 2018; Thomas et al., 2019; Spencer, 2020). The absence of significant genetic changes in this study is consistent with the absence of such changes in genome-wide data using historical samples of Atlantic cod from the western and eastern Atlantic (Pinsky et al., 2021) and with the absence of such changes in mitogenomic data from other taxa that have similarly high estimates of Ne as Atlantic cod, such as the Pacific herring (Speller et al., 2012; Moss et al., 2016), the Hawaiian petrel (Welch et al., 2012) and even extinct species such as the New Zealand moa (Allentoft et al., 2014), the passenger pigeon (Murray et al., 2017) and the great auk (Thomas et al., 2019).
In contrast, temporal losses of mitogenomic diversity and/or declines in Ne have been reported in species that have suffered population fragmentation (e.g., resulting in small effective population sizes) or that have experienced limited connectivity, such as the steppe bison (Shapiro et al., 2004), the Scandinavian arctic fox (Nyström et al., 2006), cave bears (Stiller et al., 2010), the Iberian lynx (Casas-Marce et al., 2017), the Iberian salmon (Consuegra et al., 2002) and the common bream (Ciesielski and Makowiecki, 2005). Interestingly, a loss of haplotypic variation has been identified—using CytB sequence data—for a single period (i.e., 15th to 16th centuries, out of 6 temporal periods investigated) in an Icelandic population of Atlantic cod (Olafsdottir et al., 2014). There are two potential explanations for this discrepancy. First, nearly all substitutions that comprise the CytB haplotypes can be affected by post-mortem deamination (i.e., they consist of C > T and G > A substitutions). Most of the ancient sequences (90%) investigated in Olafsdottir et al. (2014) were obtained in a single round of PCR without evaluation of such post-mortem deamination. Therefore, such bias due to post-mortem damage cannot be excluded. Second, our sampling does not include many specimens from Iceland (Figure 1 and Supplementary Table 1), and it remains possible that—with 156 samples—a local effect has been observed in Olafsdottir et al. (2014), which we do not detect in our data.
Third, we do not observe major novel mtDNA lineages in the ancient data, nor observe a significant loss of such lineages over time. Instead, the majority of Atlantic cod mtDNA lineages observed in ancient and modern samples today have originated ca. 100–150 kya (Figure 4), during a period of population expansion (Figure 5). Therefore, the gain of such lineages—and associated population expansions—in Atlantic cod is more likely caused by changes in abundance driven by major historical climatic events such as eustatic oscillations in sea level, and the interglacial and warming periods experienced during the last glacial maximum ca. 23,000 kya (Bigg et al., 2008) and the Wisconsinan (ca. 110–120 kya) and Illinoian (ca. 200–130 kya) glaciations (Gibbard and Van Kolfschoten, 2005) as described by Lait et al. (2018). For instance, we only observe the most recent population expansion ca. 10 kya (Figures 5A,B,E) when including those mtDNA clades which are strongly associated with the Baltic Sea. The timing of this expansion is in agreement with the development of the Baltic Sea (ca. 7,000–8,000 years; Ojaveer et al., 2010; Wenne et al., 2020) which has led to genetically distinct Atlantic cod populations that have adapted to local environmental conditions (i.e., salinity and temperature; Johannesson and Andre, 2006; Berg et al., 2015; Wenne et al., 2020). Therefore, the observed changes in Ne reflect past population demography rather than recent and contemporary demographic changes (Lombal et al., 2020).
It is clear from zooarcheological evidence that Atlantic cod has periodically experienced intense exploitation in the distant past, particularly around the North Sea and the Baltic Sea (Barrett et al., 1999; Enghoff, 1999; Olson and Walther, 2007; Orton et al., 2011). This fishing pressure became even greater in the 19th and 20th centuries (e.g., Thurstan et al., 2010). Landings of Atlantic cod exceeded 4,000,000 tons during 1960–1990s in the North Atlantic Ocean (Shelton and Morgan, 2014). In particular, landings surpassed 600,000 tons in Iceland by ca. 1930s (Drinkwater, 2006), 354,000 tons in the North Sea during ca. 1970s (Bannister, 2004), 200–400,000 tons in the eastern Baltic during 1960–1990s (MacKenzie et al., 2002), 650,000 tons in North East Arctic between 1937 and 1938 up to 800–1,200,000 tons in ca. 1950s (Sætersdal and Hylen, 1964; Hylen, 2002). Such high levels of exploitation led to major reductions in present abundances of most Atlantic cod populations (i.e., Food and Agriculture Organization [Fao], 2020-2021a,b). Nonetheless, for the reasons discussed above, our results indicate that such population declines of Atlantic cod did not lead to a detectable impact on the mtDNA genome on the time scale we investigated here.
Taken together, our results highlight that historical and contemporaneous anthropogenic pressures such as commercial fisheries have had little impact on the ancient mitogenomic diversity of a wide-spread marine species with high gene flow such as Atlantic cod. Future ancient DNA studies should consider the inclusion of nuclear genomic data and extensive sampling on a local scale—considering a temporal comparison of specimens from the same geographical region—to assess the effects of climate and human exploitation with greater statistical power. Finally, our observations do not contradict evidence that overfishing has had negative consequences for the abundance of Atlantic cod and they do not oppose information about the important implications of genetic variation in evolutionary biology, ecology and conservation biology. Instead, our observations suggest that conservation management measures aimed toward the demographic recovery of Atlantic cod in the eastern Atlantic, if achievable by conservation management measures, will not be constrained by recent loss of historical mitogenomic variation.
Data Availability Statement
The datasets presented in this study can be found in online repositories. The names of the repository/repositories and accession number(s) can be found below: https://www.ebi.ac.uk/ena, PRJEB42959.
Author Contributions
BS and JB: conceptualization, project design, and supervision. GF and LM-G: laboratory work and data curation. LM-G with input from GF, TO, LA, BS, and JB: formal analysis. HB and MS: analytical advice. JB, IY, IJ, JH, RN, DO, SH-D, US, AH, and RB: ancient Atlantic cod specimens. FB: modern Orkney specimens. JB, IY, IJ, JH, RN, DO, SH-D, US, AH, and RB: archeological context information. LM-G and BS: data visualization. JB, BS, KJ, SJ, and DO: funding acquisition. LM-G and BS with input from JB: writing—original draft. All authors writing—review and editing.
Funding
This work was supported by Research Council of Norway projects “Catching the Past” (262777) and “The Aqua Genome Project” (221734), Leverhulme Trust Project MRF-2013-065 and the European Union’s Horizon 2020 Research and Innovation Programme under the Marie Skłodowska-Curie grant agreement No. 813383.
Conflict of Interest
The authors declare that the research was conducted in the absence of any commercial or financial relationships that could be construed as a potential conflict of interest.
Acknowledgments
We thank Agata T. Gondek-Wyrozemska for help with processing the ancient specimens and Francis Neat for providing modern Orkney specimens. We also thank Oliver Kersten and Michael Matschiner for advice during analyses. Finally, we thank M. Skage, S. Kollias and A. Tooming-Klunderud at the Norwegian Sequencing Centre for sequencing and processing of samples. Analyses were performed on the SAGA Cluster using the resources and assistance from the SIGMA2 Metacenter, the Norwegian National Infrastructure for High Performance Computing and Data Storage.
Supplementary Material
The Supplementary Material for this article can be found online at: https://www.frontiersin.org/articles/10.3389/fevo.2021.671281/full#supplementary-material
References
Allentoft, M. E., Heller, R., Oskam, C. L., Lorenzen, E. D., Hale, M. L., Gilbert, M. T. P., et al. (2014). Extinct New Zealand megafauna were not in decline before human colonization. Proc. Natl. Acad. Sci.U. S. A. 111, 4922–4927. doi: 10.1073/pnas.1314972111
Amos, W., and Balmford, A. (2001). When does conservation genetics matter? Heredity 87, 257–265. doi: 10.1046/j.1365-2540.2001.00940.x
Árnason, E. (2004). Mitochondrial cytochrome b DNA variation in the high-fecundity Atlantic cod: trans-Atlantic clines and shallow gene genealogy. Genetics 166, 1871–1885. doi: 10.1093/genetics/166.4.1871
Árnason, E., and Palsson, S. (1996). Mitochondrial cytochrome b DNA sequence variation of Atlantic cod Gadus morhua, from Norway. Mol. Ecol. 5, 715–724. doi: 10.1111/j.1365-294x.1996.tb00368.x
Árnason, E., Petersen, P. H., Kristinsson, K., Sigurgíslason, H., and Pálsson, S. (2000). Mitochondrial cytochrome b DNA sequence variation of Atlantic cod from Iceland and Greenland. J. Fish Biol. 56, 409–430. doi: 10.1006/jfbi.1999.1167
Árnason, E., Petersen, P. H., and Pálsson, S. (1998). Mitochondrial cytochrome b DNA sequence variation of Atlantic cod, Gadus morhua, from the Baltic and the White Seas. Hereditas 129, 37–43. doi: 10.1111/j.1601-5223.1998.00037.x
Bannister, R. C. A. (2004). “The rise and fall of cod (Gadus morhua, L.) in the North Sea,” in Management of Shared Fish Stocks, eds A. I. L. Payne, C. M. O’Brien, and S. I. Rogers (Oxford: Blackwell Publishing), 316–338. doi: 10.1002/9780470999936.ch19
Barrett, J. H. (2019). An environmental (pre) history of European fishing: past and future archaeological contributions to sustainable fisheries. J. Fish Biol. 94, 1033–1044. doi: 10.1111/jfb.13929
Barrett, J. H., Boessenkool, S., Kneale, C. J., O’Connell, T. C., and Star, B. (2020). Ecological globalisation, serial depletion and the medieval trade of walrus rostra. Quat. Sci. Rev. 229:106122. doi: 10.1016/j.quascirev.2019.106122
Barrett, J. H., Locker, A. M., and Roberts, C. M. (2004). ‘Dark Age Economics’ revisited: the English fish bone evidence AD 600-1600. Antiquity 78, 618–636. doi: 10.1017/s0003598x00113262
Barrett, J. H., Nicholson, R. A., and Cerón-Carrasco, R. (1999). Archaeo-ichthyological Evidence for Long-term Socioeconomic Trends in Northern Scotland: 3500 BC to AD 1500. J. Archaeol. Sci. 26, 353–388. doi: 10.1006/jasc.1998.0336
Barrett, J. H., Orton, D., Johnstone, C., Harland, J., Van Neer, W., Ervynck, A., et al. (2011). Interpreting the expansion of sea fishing in medieval Europe using stable isotope analysis of archaeological cod bones. J. Archaeol. Sci. 38, 1516–1524. doi: 10.1016/j.jas.2011.02.017
Barth, J. M., Berg, P. R., Jonsson, P. R., Bonanomi, S., Corell, H., Hemmer−Hansen, J., et al. (2017). Genome architecture enables local adaptation of Atlantic cod despite high connectivity. Mol. Ecol. 26, 4452–4466. doi: 10.1111/mec.14207
Barth, J. M. I., Villegas-Ríos, D., Freitas, C., Moland, E., Star, B., André, C., et al. (2019). Disentangling structural genomic and behavioural barriers in a sea of connectivity. Mol. Ecol. 28, 1394–1411. doi: 10.1111/mec.15010
Bartolino, V., Cardinale, M., Svedäng, H., Linderholm, Hans, W., Casini, M., et al. (2012). Historical spatiotemporal dynamics of eastern North Sea cod. Can. J. Fish. Aquat. Sci. 69, 833–841. doi: 10.1139/f2012-028
Berg, P. R., Jentoft, S., Star, B., Ring, K. H., Knutsen, H., Lien, S., et al. (2015). Adaptation to Low Salinity Promotes Genomic Divergence in Atlantic Cod (Gadus morhua L.). Genome Biol. Evol. 7, 1644–1663. doi: 10.1093/gbe/evv093
Berg, P. R., Star, B., Pampoulie, C., Bradbury, I. R., Bentzen, P., Hutchings, J. A., et al. (2017). Trans-oceanic genomic divergence of Atlantic cod ecotypes is associated with large inversions. Heredity 119, 418–428. doi: 10.1038/hdy.2017.54
Berg, P. R., Star, B., Pampoulie, C., Sodeland, M., Barth, J. M. I., Knutsen, H., et al. (2016). Three chromosomal rearrangements promote genomic divergence between migratory and stationary ecotypes of Atlantic cod. Sci. Rep. 6:23246. doi: 10.1038/srep23246
Bigg, G. R., Cunningham, C. W., Ottersen, G., Pogson, G. H., Wadley, M. R., and Williamson, P. (2008). Ice-age survival of Atlantic cod: agreement between palaeoecology models and genetics. Proc. R. Soc. B Biol. Sci. 275, 163–173. doi: 10.1098/rspb.2007.1153
Bouckaert, R., Vaughan, T. G., Barido-Sottani, J., Duchêne, S., Fourment, M., Gavryushkina, A., et al. (2019). BEAST 2.5: an advanced software platform for Bayesian evolutionary analysis. PLoS Comput. Biol. 15:e1006650. doi: 10.1371/journal.pcbi.1006650
Brattey, J., Cadigan, N., Dwyer, K. S., Healey, B. P., Ings, D. W., Lee, E. M., et al. (2018). Assessment of the Northern Cod (Gadus morhua) stock in NAFO Divisions 2J3KL in 2016. DFO Can. Sci. Advis. Sec. (CSAS) Res. Doc. 2018/018 107, 20–24.
Carøe, C., Gopalakrishnan, S., Vinner, L., Mak, S. S., Sinding, M. H. S., Samaniego, J. A., et al. (2018). Single−tube library preparation for degraded DNA. Methods Ecol. Evol. 9, 410–419. doi: 10.1111/2041-210x.12871
Carr, S., Snellen, A., Howse, K., and Wroblewski, J. (1995). Mitochondrial DNA sequence variation and genetic stock structure of Atlantic cod (Gadus morhua) from bay and offshore locations on the Newfoundland continental shelf. Mol. Ecol. 4, 79–88. doi: 10.1111/j.1365-294x.1995.tb00194.x
Casas-Marce, M., Marmesat, E., Soriano, L., Martínez-Cruz, B., Lucena-Perez, M., Nocete, F., et al. (2017). Spatiotemporal dynamics of genetic variation in the Iberian lynx along its path to extinction reconstructed with ancient DNA. Mol. Biol. Evol. 34, 2893–2907. doi: 10.1093/molbev/msx222
Castañeda, R. A., Burliuk, C. M. M., Casselman, J. M., Cooke, S. J., Dunmall, K. M., Forbes, L. S., et al. (2020). A Brief History of Fisheries in Canada. Fisheries 45, 303–318. doi: 10.1002/fsh.10449
Charif, D., Lobry, J. R., Necsulea, A., Palmeira, L., Penel, S., Perriere, G., et al. (2020). Package ‘seqinr. URL: http://seqinr.r-forge.r-project.org/
Ciesielski, S., and Makowiecki, D. (2005). Ancient and modern mitochondrial haplotypes of common bream (Abramis brama L.) in Poland. Ecol. Freshw. Fish 14, 278–282. doi: 10.1111/j.1600-0633.2005.00097.x
Consuegra, S., García, de Leániz, C., Serdio, A., González Morales, M., Straus, L., et al. (2002). Mitochondrial DNA variation in Pleistocene and modern Atlantic salmon from the Iberian glacial refugium. Mol. Ecol. 11, 2037–2048. doi: 10.1046/j.1365-294x.2002.01592.x
Cooper, A., and Poinar, H. N. (2000). Ancient DNA: do it right or not at all. Science 289, 1139–1139.
R Core Team. (2020). R: A language and environment for statistical computing. Vienna, Austria: R Foundation for Statistical Computing.
Danecek, P., Auton, A., Abecasis, G., Albers, C. A., Banks, E., DePristo, M. A., et al. (2011). The variant call format and VCFtools. Bioinformatics 27, 2156–2158. doi: 10.1093/bioinformatics/btr330
Darriba, D., Taboada, G. L., Doallo, R., and Posada, D. (2012). jModelTest 2: more models, new heuristics and parallel computing. Nat. Methods 9, 772–772. doi: 10.1038/nmeth.2109
Drinkwater, K. F. (2006). The regime shift of the 1920s and 1930s in the North Atlantic. Prog. Oceanogr. 68, 134–151. doi: 10.1016/j.pocean.2006.02.011
Edvardsson, R., Patterson, W. P., Bárðarson, H., Timsic, S., and Ólafsdóttir, G. Á (2019). Change in Atlantic cod migrations and adaptability of early land-based fishers to severe climate variation in the North Atlantic. Quat. Res. 2019, 1–11. doi: 10.1017/qua.2018.147
Enghoff, I. B. (1999). Fishing in the Baltic Region from the 5th century BC to the 16th century AD: evidence from Fish Bones. Archaeofauna 8, 41–85.
Exadactylos, A., Rigby, M. J., Geffen, A. J., and Thorpe, J. P. (2007). Conservation aspects of natural populations and captive-bred stocks of turbot (Scophthalmus maximus) and Dover sole (Solea solea) using estimates of genetic diversity. ICES J. Mar. Sci. 64, 1173–1181. doi: 10.1093/icesjms/fsm086
Exadactylos, A., Vafidis, D., Tsigenopoulos, C. S., and Gkafas, G. A. (2019). High Connectivity of the White Seabream (Diplodus sargus, L. 1758) in the Aegean Sea, Eastern Mediterranean Basin. Animals 9:979. doi: 10.3390/ani9110979
Excoffier, L., and Lischer, H. E. (2010). Arlequin suite ver 3.5: a new series of programs to perform population genetics analyses under Linux and Windows. Mol. Ecol. Resour. 10, 564–567. doi: 10.1111/j.1755-0998.2010.02847.x
Ferrari, G., Cuevas, A., Gondek-Wyrozemska, A. T., Ballantyne, R., Kersten, O., Pálsdóttir, A. H., et al. (2021). The preservation of ancient DNA in archaeological fish bone. J. Archaeol. Sci. 126:105317. doi: 10.1016/j.jas.2020.105317
Food and Agriculture Organization [Fao]. (2020-2021a). International Council for the Exploration of the Sea (ICES). ICES Advice 2019. Cod - Western English Channel, Bristol Channel, Celtic Sea and Southwest of Ireland. FIRMS Reports. In: Fisheries and Resources Monitoring System (FIRMS) [online]. Rome: Food and Agriculture Organization doi: 10.1016/j.jas.2020.105317
Food and Agriculture Organization [Fao]. (2020-2021b). International Council for the Exploration of the Sea (ICES). ICES Advice 2020. Cod - Baltic Sea (Eastern part). FIRMS Reports. In: Fisheries and Resources Monitoring System (FIRMS) [online]. Rome: Food and Agriculture Organization.
Fortes, G. G., and Paijmans, J. L. (2015). “Analysis of whole mitogenomes from ancient samples,” in Whole genome amplification, (Heidelberg: Springer), 179–195. doi: 10.1007/978-1-4939-2990-0_13
Frankham, R., Ballou, J. D., and Briscoe, D. A. (2002). “Chapter 10: Loss of genetic diversity in small populations,” in Introduction to conservation genetics, ed. K. H. McInness (Cambridge, UK: Cambridge University Press), 227–253. doi: 10.1017/cbo9780511808999.013
Gibbard, P., and Van Kolfschoten, T. (2005). “The Pleistocene and Holocene Epochs,” in A Geologic Time Scale 2004, eds A. G. Smith, F. M. Gradstein, and J. G. Ogg (Cambridge: Cambridge University Press), 441–452. doi: 10.1017/cbo9780511536045.023
Gilbert, M. T. P., Bandelt, H.-J., Hofreiter, M., and Barnes, I. (2005). Assessing ancient DNA studies. Trends Ecol. Evol. 20, 541–544. doi: 10.1016/j.tree.2005.07.005
Gondek, A. T., Boessenkool, S., and Star, B. (2018). A stainless-steel mortar, pestle and sleeve design for the efficient fragmentation of ancient bone. BioTechniques 64, 266–269. doi: 10.2144/btn-2018-0008
Guindon, S., Dufayard, J.-F., Lefort, V., Anisimova, M., Hordijk, W., and Gascuel, O. (2010). New Algorithms and Methods to Estimate Maximum-Likelihood Phylogenies: assessing the Performance of PhyML 3.0. Syst. Biol. 59, 307–321. doi: 10.1093/sysbio/syq010
Guindon, S., and Gascuel, O. (2003). A simple, fast, and accurate algorithm to estimate large phylogenies by maximum likelihood. Syst. Biol. 52, 696–704. doi: 10.1080/10635150390235520
Hardie, D. C., Gillett, R. M., and Hutchings, J. A. (2006). The effects of isolation and colonization history on the genetic structure of marine-relict populations of Atlantic cod (Gadus morhua) in the Canadian Arctic. Can. J. Fish. Aquat. Sci. 63, 1830–1839. doi: 10.1139/f06-085
Harland, J., and Barrett, J. H. (2012). “Chapter 7: The Maritime Economy, Fish Bone,” in Being an Islander: Production and identity at Quoygrew, Orkney, AD 900-1600, ed. J. H. Barrett (Cambridge: McDonald Institute for Archaeological Research), 115–138.
Hauser, L., and Carvalho, G. R. (2008). Paradigm shifts in marine fisheries genetics: ugly hypotheses slain by beautiful facts. Fish Fish. 9, 333–362. doi: 10.1111/j.1467-2979.2008.00299.x
Heller, R., Chikhi, L., and Siegismund, H. R. (2013). The Confounding Effect of Population Structure on Bayesian Skyline Plot Inferences of Demographic History. PLoS One 8:e62992. doi: 10.1371/journal.pone.0062992
Hofman, C. A., Rick, T. C., Fleischer, R. C., and Maldonado, J. E. (2015). Conservation archaeogenomics: ancient DNA and biodiversity in the Anthropocene. Trends Ecol. Evol. 30, 540–549. doi: 10.1016/j.tree.2015.06.008
Hutchinson, W. F., Oosterhout, C. V., Rogers, S. I., and Carvalho, G. R. (2003). Temporal analysis of archived samples indicates marked genetic changes in declining North Sea cod (Gadus morhua). Proc. R. Soc. Lon. B Biol. Sci. 270, 2125–2132. doi: 10.1098/rspb.2003.2493
Hylen, A. (2002). Fluctuations in abundance of Northeast Arctic cod during the 20th century. ICES Mar. Sci. Symp. 215, 543–550.
Hylen, A., Nakken, O., and Nedreaas, K. (2008). Northeast Arctic cod: fisheries, life history, stock fluctuations and management. Norwegian spring-spawning herring and Northeast Arctic cod. Trondheim: Tapir Academic Press, 83–118.
Johannesson, K., and Andre, C. (2006). INVITED REVIEW: life on the margin: genetic isolation and diversity loss in a peripheral marine ecosystem, the Baltic Sea. Mol. Ecol. 15, 2013–2029. doi: 10.1111/j.1365-294x.2006.02919.x
Johansen, S., and Bakke, I. (1996). The complete mitochondrial DNA sequence of Atlantic cod (Gadus morhua): relevance to taxonomic studies among codfishes. Mol. Mar. Biol. Biotechnol. 5, 203–214.
Johnson, B. M., Kemp, B. M., and Thorgaard, G. H. (2018). Increased mitochondrial DNA diversity in ancient Columbia River basin Chinook salmon Oncorhynchus tshawytscha. PLoS One 13:e0190059. doi: 10.1371/journal.pone.0190059
Jónsson, H., Ginolhac, A., Schubert, M., Johnson, P. L., and Orlando, L. (2013). mapDamage2. 0: fast approximate Bayesian estimates of ancient DNA damage parameters. Bioinformatics 29, 1682–1684. doi: 10.1093/bioinformatics/btt193
Jonsson, P. R., Corell, H., André, C., Svedäng, H., and Moksnes, P. O. (2016). Recent decline in cod stocks in the North Sea–Skagerrak–Kattegat shifts the sources of larval supply. Fish. Oceanogr. 25, 210–228. doi: 10.1111/fog.12146
Jørgensen, T. E., Karlsen, B. O., Emblem, Å, Breines, R., Andreassen, M., Rounge, T. B., et al. (2018). Mitochondrial genome variation of Atlantic cod. BMC Res. Notes 11:397. doi: 10.1186/s13104-018-3506-3
Katoh, K., and Standley, D. M. (2013). MAFFT multiple sequence alignment software version 7: improvements in performance and usability. Mol. Biol. Evol. 30, 772–780. doi: 10.1093/molbev/mst010
Keighley, X., Pálsson, S., Einarsson, B. F., Petersen, A., Fernández-Coll, M., Jordan, P., et al. (2019). Disappearance of Icelandic walruses coincided with Norse settlement. Mol. Biol. Evol. 36, 2656–2667. doi: 10.1093/molbev/msz196
Kircher, M., Sawyer, S., and Meyer, M. (2012). Double indexing overcomes inaccuracies in multiplex sequencing on the Illumina platform. Nucleic Acids Res. 40:e3. doi: 10.1093/nar/gkr771
Knutsen, H., Olsen, E. M., Jorde, P. E., Espeland, S. H., André, C., and Stenseth, N. C. (2011). Are low but statistically significant levels of genetic differentiation in marine fishes ’biologically meaningful’? A case study of coastal Atlantic cod. Mol Ecol. 20, 768–783. doi: 10.1111/j.1365-294X.2010.04979.x
Kumar, S., Stecher, G., and Tamura, K. (2016). MEGA7: molecular evolutionary genetics analysis version 7.0 for bigger datasets. Mol. Biol. Evol. 33, 1870–1874. doi: 10.1093/molbev/msw054
Lait, L. A. (2016). A mitogenomic study of four at-risk maring fish species: Atlantic wolffish, spotted wolffish, northern wolffish, and Atlantic cod, with special emphasis on the waters off Newfoundland and Labrador. Ph.D. thesis, Canada: Memorial University of Newfoundland.
Lait, L. A., Marshall, H. D., and Carr, S. M. (2018). Phylogeographic mitogenomics of Atlantic cod Gadus morhua: variation in and among trans−Atlantic, trans−Laurentian, Northern cod, and landlocked fjord populations. Ecol. Evol. 8, 6420–6437. doi: 10.1002/ece3.3873
Li, H., and Durbin, R. (2009). Fast and accurate short read alignment with Burrows–Wheeler transform. Bioinformatics 25, 1754–1760. doi: 10.1093/bioinformatics/btp324
Li, H., Handsaker, B., Wysoker, A., Fennell, T., Ruan, J., Homer, N., et al. (2009). The sequence alignment/map format and SAMtools. Bioinformatics 25, 2078–2079. doi: 10.1093/bioinformatics/btp352
Limburg, K. E., Walther, Y., Hong, B., Olson, C., and Storå, J. (2008). Prehistoric versus modern Baltic Sea cod fisheries: selectivity across the millennia. Proc. R. Soc. B Biol. Sci. 275, 2659–2665. doi: 10.1098/rspb.2008.0711
Lindgreen, S. (2012). AdapterRemoval: easy cleaning of next-generation sequencing reads. BMC Res. Notes 5:337. doi: 10.1186/1756-0500-5-337
Lombal, A. J., O’dwyer, J. E., Friesen, V., Woehler, E. J., and Burridge, C. P. (2020). Identifying mechanisms of genetic differentiation among populations in vagile species: historical factors dominate genetic differentiation in seabirds. Biol. Rev. 95, 625–651. doi: 10.1111/brv.12580
Louis, M., Skovrind, M., Samaniego Castruita, J. A., Garilao, C., Kaschner, K., Gopalakrishnan, S., et al. (2020). Influence of past climate change on phylogeography and demographic history of narwhals. Monodon monoceros. Proc. R. Soc. B 287:20192964. doi: 10.1098/rspb.2019.2964
MacKenzie, B. R., Alheit, J., Conley, D. J., Holm, P., and Kinze, C. C. (2002). Ecological hypotheses for a historical reconstruction of upper trophic level biomass in the Baltic Sea and Skagerrak. Can. J. Fish. Aqua. Sci. 59, 173–190. doi: 10.1139/f01-201
Mak, S. S. T., Gopalakrishnan, S., Carøe, C., Geng, C., Liu, S., Sinding, M. S., et al. (2017). Comparative performance of the BGISEQ-500 vs Illumina HiSeq2500 sequencing platforms for palaeogenomic sequencing. Gigascience 6, 1–13. doi: 10.1093/gigascience/gix049
Malmstrøm, M., Matschiner, M., Tørresen, O. K., Star, B., Snipen, L. G., Hansen, T. F., et al. (2016). Evolution of the immune system influences speciation rates in teleost fishes. Nat. Genet. 48, 1204–1210. doi: 10.1038/ng.3645
Matschiner, M. (2016). Fitchi: haplotype genealogy graphs based on the Fitch algorithm. Bioinformatics 32, 1250–1252. doi: 10.1093/bioinformatics/btv717
McKenna, A., Hanna, M., Banks, E., Sivachenko, A., Cibulskis, K., Kernytsky, A., et al. (2010). The Genome Analysis Toolkit: a MapReduce framework for analyzing next-generation DNA sequencing data. Genome Res. 20, 1297–1303. doi: 10.1101/gr.107524.110
Meyer, M., and Kircher, M. (2010). Illumina sequencing library preparation for highly multiplexed target capture and sequencing. Cold Spring Harb. Protoc. 2010:db.rot5448.
Mieszkowska, N., Genner, M. J., Hawkins, S. J., and Sims, D. W. (2009). “Chapter 3 Effects of Climate Change and Commercial Fishing on Atlantic Cod Gadus morhua,” in Advances in Marine Biology, ed. D. W. Sims (Cambridge: Academic Press), 213–273. doi: 10.1016/s0065-2881(09)56003-8
Mitchell, K. J., and Rawlence, N. J. (2021). Examining Natural History through the Lens of Palaeogenomics. Trends Ecol. Evol. 36, 258–267. doi: 10.1016/j.tree.2020.10.005
Moss, M. L., Rodrigues, A. T., Speller, C. F., and Yang, D. Y. (2016). The historical ecology of Pacific herring: tracing Alaska Native use of a forage fish. J. Archaeol. Sci. Rep. 8, 504–512. doi: 10.1016/j.jasrep.2015.10.005
Murray, G. G., Soares, A. E., Novak, B. J., Schaefer, N. K., Cahill, J. A., Baker, A. J., et al. (2017). Natural selection shaped the rise and fall of passenger pigeon genomic diversity. Science 358, 951–954. doi: 10.1126/science.aao0960
Nguyen, L.-T., Schmidt, H. A., Von Haeseler, A., and Minh, B. Q. (2015). IQ-TREE: a fast and effective stochastic algorithm for estimating maximum-likelihood phylogenies. Mol. Biol. Evol. 32, 268–274. doi: 10.1093/molbev/msu300
Nicholls, J., Allaire, B., and Holm, P. (2021). The Capacity Trend Method: a new approach for enumerating the Newfoundland cod fisheries (1675–1790). Histori. Methods J. Quan. Interdiscip. History 2021, 1–14. doi: 10.1080/01615440.2020.1853643
Nikulina, E. A., and Schmölcke, U. (2016). Reconstruction of the historical distribution of sturgeons (Acipenseridae) in the eastern North Atlantic based on ancient DNA and bone morphology of archaeological remains: implications for conservation and restoration programmes. Divers. Distrib. 22, 1036–1044. doi: 10.1111/ddi.12461
Nyström, V., Angerbjörn, A., and Dalén, L. (2006). Genetic consequences of a demographic bottleneck in the Scandinavian arctic fox. Oikos 114, 84–94. doi: 10.1111/j.2006.0030-1299.14701.x
Ojaveer, H., Jaanus, A., MacKenzie, B. R., Martin, G., Olenin, S., Radziejewska, T., et al. (2010). Status of Biodiversity in the Baltic Sea. PLoS One 5:e12467. doi: 10.1371/journal.pone.0012467
Olafsdottir, G. A., Westfall, K. M., Edvardsson, R., and Palsson, S. (2014). Historical DNA reveals the demographic history of Atlantic cod (Gadus morhua) in medieval and early modern Iceland. Proc. Biol. Sci. 281:20132976. doi: 10.1098/rspb.2013.2976
Olson, C., and Walther, Y. (2007). Neolithic cod (Gadus morhua) and herring (Clupea harengus) fisheries in the Baltic Sea, in the light of fine-mesh sieving: a comparative study of subfossil fishbone from the late Stone Age sites at Ajvide, Gotland, Sweden and Jettböle, Åland, Finland. Environ. Archaeol. 12, 175–185. doi: 10.1179/174963107x226435
Oosting, T., Star, B., Barrett, J. H., Wellenreuther, M., Ritchie, P. A., and Rawlence, N. J. (2019). Unlocking the potential of ancient fish DNA in the genomic era. Evol. Appl. 12, 1513–1522. doi: 10.1111/eva.12811
Orton, D. C., Makowiecki, D., de Roo, T., Johnstone, C., Harland, J., Jonsson, L., et al. (2011). Stable Isotope Evidence for Late Medieval (14th–15th C) Origins of the Eastern Baltic Cod (Gadus morhua) Fishery. PLoS One 6:e27568. doi: 10.1371/journal.pone.0027568
Orton, D. C., Morris, J., Locker, A., and Barrett, J. H. (2014). Fish for the city: meta-analysis of archaeological cod remains and the growth of London’s northern trade. Antiquity 88, 516–530. doi: 10.1017/S0003598X00101152
Page, A. J., Taylor, B., Delaney, A. J., Soares, J., Seemann, T., Keane, J. A., et al. (2016). SNP-sites: rapid efficient extraction of SNPs from multi-FASTA alignments. Microb. Genom. 2:e000056. doi: 10.1099/mgen.0.000056
Paijmans, J. L., Gilbert, M. T. P., and Hofreiter, M. (2013). Mitogenomic analyses from ancient DNA. Mol. Phylogenet. Evol. 69, 404–416. doi: 10.1016/j.ympev.2012.06.002
Paradis, E. (2010). pegas: an R package for population genetics with an integrated–modular approach. Bioinformatics 26, 419–420. doi: 10.1093/bioinformatics/btp696
Paradis, E., and Schliep, K. (2019). ape 5.0: an environment for modern phylogenetics and evolutionary analyses in R. Bioinformatics 35, 526–528. doi: 10.1093/bioinformatics/bty633
Pfeifer, B., Wittelsbuerger, U., Li, H., Handsaker, B., and Pfeifer, M. B. (2020). Package ‘PopGenome’.URL: https://CRAN.R-project.org/package=PopGenome
Pinnegar, J. K., and Engelhard, G. H. (2008). The ‘shifting baseline’phenomenon: a global perspective. Rev. Fish Biol. Fish. 18, 1–16. doi: 10.1007/s11160-007-9058-6
Pinsky, M. L., Eikeset, A. M., Helmerson, C., Bradbury, I. R., Bentzen, P., Morris, C., et al. (2021). Genomic stability through time despite decades of exploitation in cod on both sides of the Atlantic. Proc. Natl. Acad. Sci. U. S. A. 118:e2025453118. doi: 10.1073/pnas.2025453118
Rambaut, A., Drummond, A. J., Xie, D., Baele, G., and Suchard, M. A. (2018). Posterior Summarization in Bayesian Phylogenetics Using Tracer 1.7. Syst. Biol. 67, 901–904. doi: 10.1093/sysbio/syy032
Rodrigues, A. S., Monsarrat, S., Charpentier, A., Brooks, T. M., Hoffmann, M., Reeves, R., et al. (2019). Unshifting the baseline: a framework for documenting historical population changes and assessing long-term anthropogenic impacts. Philos. Trans. R. Soc. B 374:20190220. doi: 10.1098/rstb.2019.0220
Rozas, J., Ferrer-Mata, A., Sánchez-DelBarrio, J. C., Guirao-Rico, S., Librado, P., Ramos-Onsins, S. E., et al. (2017). DnaSP 6: DNA sequence polymorphism analysis of large data sets. Mol. Biol. Evol. 34, 3299–3302. doi: 10.1093/molbev/msx248
Salazar, G. (2020). FastaUtils: Utilities for DNA/RNA sequence processing. URL: https://github.com/GuillemSalazar/FastaUtils.git
Schroeder, H., Ávila-Arcos, M. C., Malaspinas, A.-S., Poznik, G. D., Sandoval-Velasco, M., Carpenter, M. L., et al. (2015). Genome-wide ancestry of 17th-century enslaved Africans from the Caribbean. Proc. Natl. Acad. Sci. U. S. A. 112, 3669–3673. doi: 10.1073/pnas.1421784112
Schubert, M., Ermini, L., Der Sarkissian, C., Jónsson, H., Ginolhac, A., Schaefer, R., et al. (2014). Characterization of ancient and modern genomes by SNP detection and phylogenomic and metagenomic analysis using PALEOMIX. Nat. Protoc. 9:1056. doi: 10.1038/nprot.2014.063
Seersholm, F. V., Cole, T. L., Grealy, A., Rawlence, N. J., Greig, K., Knapp, M., et al. (2018). Subsistence practices, past biodiversity, and anthropogenic impacts revealed by New Zealand-wide ancient DNA survey. Proc. Natl. Acad. Sci. U. S. A. 115:7771. doi: 10.1073/pnas.1803573115
Selim, S. A., Blanchard, J. L., Bedford, J., and Webb, T. J. (2016). Direct and indirect effects of climate and fishing on changes in coastal ecosystem services: a historical perspective from the North Sea. Reg. Environ. Change 16, 341–351. doi: 10.1007/s10113-014-0635-7
Shapiro, B., Drummond, A. J., Rambaut, A., Wilson, M. C., Matheus, P. E., Sher, A. V., et al. (2004). Rise and fall of the Beringian steppe bison. Science 306, 1561–1565. doi: 10.1126/science.1101074
Shelton, P. A., and Morgan, M. J. (2014). Impact of maximum sustainable yield-based fisheries management frameworks on rebuilding North Atlantic cod stocks. J. Northwest Atlant. Fish. Sci. 46, 15–25. doi: 10.2960/j.v46.m697
Sigurgíslason, H., and Árnason, E. (2003). Extent of mitochondrial DNA sequence variation in Atlantic cod from the Faroe Islands: a resolution of gene genealogy. Heredity 91, 557–564. doi: 10.1038/sj.hdy.6800361
Sodeland, M., Jorde, P. E., Lien, S., Jentoft, S., Berg, P. R., Grove, H., et al. (2016). “Islands of Divergence” in the Atlantic Cod Genome Represent Polymorphic Chromosomal Rearrangements. Genome Biol. Evol. 8, 1012–1022. doi: 10.1093/gbe/evw057
Speller, C. F., Hauser, L., Lepofsky, D., Moore, J., Rodrigues, A. T., Moss, M. L., et al. (2012). High Potential for Using DNA from Ancient Herring Bones to Inform Modern Fisheries Management and Conservation. PLoS One 7:e51122. doi: 10.1371/journal.pone.0051122
Spencer, H. G. (2020). Beyond Equilibria: the Neglected Role of History in Ecology and Evolution. Quart. Rev. Biol. 95, 311–321. doi: 10.1086/711803
Star, B., Barrett, J. H., Gondek, A. T., and Boessenkool, S. (2018). Ancient DNA reveals the chronology of walrus ivory trade from Norse Greenland. Proc. R. Soc. B Biol. Sci. 285:20180978. doi: 10.1098/rspb.2018.0978
Star, B., Boessenkool, S., Gondek, A. T., Nikulina, E. A., Hufthammer, A. K., Pampoulie, C., et al. (2017). Ancient DNA reveals the Arctic origin of Viking Age cod from Haithabu. Germany. Proc. Natl. Acad. Sci. U. S. A. 114, 9152–9157. doi: 10.1073/pnas.1710186114
Star, B., Nederbragt, A. J., Jentoft, S., Grimholt, U., Malmstrøm, M., Gregers, T. F., et al. (2011). The genome sequence of Atlantic cod reveals a unique immune system. Nature 477, 207–210.
Stiller, M., Baryshnikov, G., Bocherens, H., Grandal, d’Anglade, A., Hilpert, B., et al. (2010). Withering Away—25,000 Years of Genetic Decline Preceded Cave Bear Extinction. Mol. Biol. Evol. 27, 975–978. doi: 10.1093/molbev/msq083
Sætersdal, G., and Hylen, A. (1964). The decline of the skrei fisheries: a review of the landing statistics 1866-1957 and an evaluation of the effects of the postwarincrease in the total exploitation of the arctic cod. Fiskeridirektoratets havforskningsinstitutt 13, 56–69.
Therkildsen, N. O., Nielsen, E. E., Swain, D. P., and Pedersen, J. S. (2010). Large effective population size and temporal genetic stability in Atlantic cod (Gadus morhua) in the southern Gulf of St. Lawrence. Can. J. Fish. Aquat. Sci. 67, 1585–1595. doi: 10.1139/f10-084
Thomas, J. E., Carvalho, G. R., Haile, J., Rawlence, N. J., Martin, M. D., Ho, S. Y. W., et al. (2019). Demographic reconstruction from ancient DNA supports rapid extinction of the great auk. Elife 8:e47509. doi: 10.7554/eLife.47509
Thurstan, R. H., Brockington, S., and Roberts, C. M. (2010). The effects of 118 years of industrial fishing on UK bottom trawl fisheries. Nat. Commun. 1:15. doi: 10.1038/ncomms1013
Tørresen, O. K., Star, B., Jentoft, S., Reinar, W. B., Grove, H., Miller, J. R., et al. (2017). An improved genome assembly uncovers prolific tandem repeats in Atlantic cod. BMC Genomics 18:95. doi: 10.1186/s12864-016-3448-x
Venter, O., Sanderson, E. W., Magrach, A., Allan, J. R., Beher, J., Jones, K. R., et al. (2016). Sixteen years of change in the global terrestrial human footprint and implications for biodiversity conservation. Nat. Commun. 7:12558. doi: 10.1038/ncomms12558
Welch, A. J., Wiley, A. E., James, H. F., Ostrom, P. H., Stafford, Jr., and Fleischer, R. C. (2012). Ancient DNA reveals genetic stability despite demographic decline: 3,000 years of population history in the endemic Hawaiian petrel. Mol. Biol. Evol. 29, 3729–3740. doi: 10.1093/molbev/mss185
Wenne, R., Bernaś, R., Kijewska, A., Poćwierz-Kotus, A., Strand, J., Petereit, C., et al. (2020). SNP genotyping reveals substructuring in weakly differentiated populations of Atlantic cod (Gadus morhua) from diverse environments in the Baltic Sea. Sci. Rep. 10, 1–15.
Zhang, J., Pei, N., Mi, X., and Zhang, M. J. (2017). Package ‘phylotools’. dimension 12. URL: https://github.com/helixcn/phylotools
Keywords: population structure, fisheries, human exploitation, phylogenomics, population expansion, demographic history
Citation: Martínez-García L, Ferrari G, Oosting T, Ballantyne R, van der Jagt I, Ystgaard I, Harland J, Nicholson R, Hamilton-Dyer S, Baalsrud HT, Brieuc MSO, Atmore LM, Burns F, Schmölcke U, Jakobsen KS, Jentoft S, Orton D, Hufthammer AK, Barrett JH and Star B (2021) Historical Demographic Processes Dominate Genetic Variation in Ancient Atlantic Cod Mitogenomes. Front. Ecol. Evol. 9:671281. doi: 10.3389/fevo.2021.671281
Received: 26 February 2021; Accepted: 06 May 2021;
Published: 04 June 2021.
Edited by:
Melissa T. R. Hawkins, Smithsonian Institution, United StatesReviewed by:
Martin Taylor, University of East Anglia, United KingdomAthanasios Exadactylos, University of Thessaly, Greece
Copyright © 2021 Martínez-García, Ferrari, Oosting, Ballantyne, van der Jagt, Ystgaard, Harland, Nicholson, Hamilton-Dyer, Baalsrud, Brieuc, Atmore, Burns, Schmölcke, Jakobsen, Jentoft, Orton, Hufthammer, Barrett and Star. This is an open-access article distributed under the terms of the Creative Commons Attribution License (CC BY). The use, distribution or reproduction in other forums is permitted, provided the original author(s) and the copyright owner(s) are credited and that the original publication in this journal is cited, in accordance with accepted academic practice. No use, distribution or reproduction is permitted which does not comply with these terms.
*Correspondence: Lourdes Martínez-García, bC5tLmdhcmNpYUBpYnYudWlvLm5v; Bastiaan Star, YmFzdGlhYW4uc3RhckBpYnYudWlvLm5v
†ORCID: Lourdes Martínez-García, orcid.org/0000-0002-1582-3611; Giada Ferrari, orcid.org/0000-0002-0850-1518; Tom Oosting, orcid.org/0000-0002-7031-0747; Rachel Ballantyne, orcid.org/0000-0002-6506-3163; Helle Tessand Baalsrud, orcid.org/0000-0002-4161-3247; Marine Servane Ono Brieuc, orcid.org/0000-0001-8601-2122; Lane M. Atmore, orcid.org/0000-0002-8903-8149; Kjetill S. Jakobsen, orcid.org/0000-0002-8861-5397; Sissel Jentoft, orcid.org/0000-0001-8707-531X; David Orton, orcid.org/0000-0003-4069-8004; James H. Barrett, orcid.org/0000-0002-6683-9891; Bastiaan Star, orcid.org/0000-0003-0235-9810