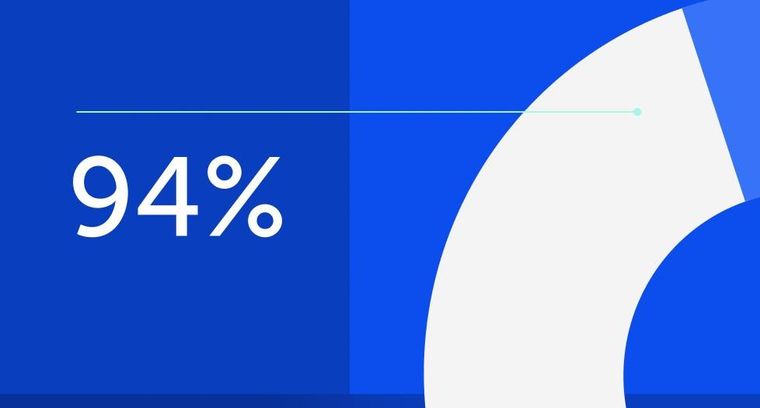
94% of researchers rate our articles as excellent or good
Learn more about the work of our research integrity team to safeguard the quality of each article we publish.
Find out more
ORIGINAL RESEARCH article
Front. Ecol. Evol., 25 June 2021
Sec. Behavioral and Evolutionary Ecology
Volume 9 - 2021 | https://doi.org/10.3389/fevo.2021.658177
This article is part of the Research TopicDeterminants and Consequences of Perceived Predation Risk: From Individual Behavior to Transgenerational EffectsView all 11 articles
A correction has been applied to this article in:
Corrigendum: Predator-Induced Plasticity on Warning Signal and Larval Life-History Traits of the Aposematic Wood Tiger Moth, Arctia plantaginis
Predator-induced plasticity in life-history and antipredator traits during the larval period has been extensively studied in organisms with complex life-histories. However, it is unclear whether different levels of predation could induce warning signals in aposematic organisms. Here, we investigated whether predator-simulated handling affects warning coloration and life-history traits in the aposematic wood tiger moth larva, Arctia plantaginis. As juveniles, a larger orange patch on an otherwise black body signifies a more efficient warning signal against predators but this comes at the costs of conspicuousness and thermoregulation. Given this, one would expect that an increase in predation risk would induce flexible expression of the orange patch. Prior research in this system points to plastic effects being important as a response to environmental changes for life history traits, but we had yet to assess whether this was the case for predation risk, a key driver of this species evolution. Using a full-sib rearing design, in which individuals were reared in the presence and absence of a non-lethal simulated bird attack, we evaluated flexible responses of warning signal size (number of orange segments), growth, molting events, and development time in wood tiger moths. All measured traits except development time showed a significant response to predation. Larvae from the predation treatment developed a more melanized warning signal (smaller orange patch), reached a smaller body size, and molted more often. Our results suggest plasticity is indeed important in aposematic organisms, but in this case may be complicated by the trade-off between costly pigmentation and other life-history traits.
Organisms live in a constantly changing environment, and this variation may have important effects on an individual’s fitness. Evolution (via genetic change) and plasticity (the flexibility of a genotype to change its phenotype in response to environmental cues) are key mechanisms upon which species adapt to environmental variation (Gotthard and Nylin, 1995; Ghalambor et al., 2007). Plasticity can be adaptive, when its response is in the same direction favored by selection (Ghalambor et al., 2007) and can allow a particular genotype to rapidly endure changes in the environment. On the other hand, a non-adaptive phenotype can be far from the adaptive peak and have a reduced fitness under environmental change (Ghalambor et al., 2007).
The role of plasticity in evolution is not straightforward. It is often argued that if plasticity is adaptive, it should deter evolution since it can hide genetic variation on which selection would act, and thus weaken selection (Ghalambor et al., 2007; Gordon et al., 2017). Yet, plasticity is also crucial for population persistence in a changing environment because it can buffer novel variants against purifying selection and may thus, facilitate long-term adaptation by maintaining higher genetic variation (Corl et al., 2018; Perry et al., 2018). Furthermore, induced-responses often involve costs, hindering the adaptive plastic responses by another environmental cue or stress response, thus reducing fitness and generating the pattern of maladaptive response (Morris and Rogers, 2013). Trade-offs in stress responses may limit plastic responses but may also constrain evolution and future adaptation (Koch and Guillaume, 2020).
Predation is one of the most important selective pressures in nature. Hence, predator-induced plasticity is perhaps one of the most relevant plastic responses, and has been shown to stimulate changes in prey morphology, behavior and life history for a variety of taxa (Tollrian, 1995; Tollrian and Dodson, 1999; Roff, 2002; Benard, 2004; Hammill et al., 2008). For example, studies using a variety of predator-simulated attack mechanisms have led to inducible variation in prey responses such as immunosuppression or variation in chemical defenses concentration (toxins) (Watson et al., 2004; Adamo et al., 2017; Bucciarelli et al., 2017; Cinel et al., 2020).
Predator-induced plasticity is common in organisms with complex life cycles, because each developmental phase exposes them to different predators, parasites, and environmental conditions (Benard, 2004; Friman et al., 2009; Mutamiswa et al., 2019). Prey life-history traits that are known to be plastic are growth rate, body size, and development time such as timing to metamorphosis or hatching (Stearns and Koella, 1986; Stearns, 1989; Nylin and Gotthard, 1998; Tollrian and Dodson, 1999). However, the direction and magnitude of plasticity in life-history traits vary widely (reviewed in Benard, 2004) and much is left to still discover and understand. In particular, it is still unknown how short-term selection pressures are linked to long-term fitness (Koch and Guillaume, 2020).
Plastic responses in life history may improve prey success under fluctuating predation risks, but may also involve trade-offs because individual survival might come at a cost to certain traits like reproduction (Tollrian, 1995; Tollrian and Dodson, 1999; Roff, 2002; Benard, 2004; Hammill et al., 2008). For instance, inducible morphological defenses, like neck spines and variation in body morphology in Daphnia pulex increase survival against predation from Chaoborus spp., compared to undefended morphs, but at the cost of a reduction in reproductive success (Hammill et al., 2008). The predatory rotifer Asplanchna brightwellii also induces plastic changes in the ultrastructure of lorica (shell), lorica thickness, lateral spines, and body size of Brachionus herbivores (Brachionus calyciflorus and Brachionus angularis). However, their development is also associated with decreased reproduction or reduced sex investment in herbivores (Yin et al., 2017).
Coloration is a common morphological defense that is often related to avoiding predator detection and attack (Endler, 1986, 1991a; Caro, 2005). Camouflage is the most common color strategy to avoid predation; it has been studied across a range of animal taxa including lepidopterans, crustaceans, cephalopods, reptiles, amphibians, and fish (Stevens, 2016), and has been shown to also be plastic in some cases. Some examples of background matching plasticity in response to different environmental cues are seen in pygmy grasshoppers, Tetrix subulata and Tetrix ceperoi. Their basic color is altered by the background substrate color, so when in a dark substrate, both species tend to change to a black and dark olive color, whereas in light substrates, the gray color morph dominates. The plasticity in body coloration increases camouflage and is likely to be an adaptation to reduce predation risk (Hochkirch et al., 2008). For organisms that live in complex environments, gaining optimal camouflage is tricky (Merilaita et al., 2017), in particular for mobile animals. Plasticity responses can solve this conundrum. A recent study by Corl et al. (2018) in side-blotched lizards (Uta stansburiana) established the crucial role of plasticity in background matching during the colonization of a new environment. Ancestral plasticity in coloration and divergence in two genes that increase pigmentation (regulators of melanin production), facilitate survival and persistence in novel darker habitats and allows time for subsequent genetic adaptation to fine-tune the plastic response to the new environment.
Aposematism is a complex defense strategy that is based on organisms displaying warning signals (typically conspicuous coloration) to advertise unpalatability or noxiousness (Poulton, 1890; Cott, 1940; Endler, 1991b; Inbar and Lev-Yadun, 2005; Speed and Ruxton, 2005). Although avoidance of conspicuous colors can be innate or inherited (Schuler and Roper, 1992; Mastrota and Mench, 1995; Lindström et al., 1999a), learning typically facilitates the negative association of a warning signal and unprofitability (Mappes et al., 2005). Predators learn to avoid unprofitable prey with conspicuous coloration more quickly compared to cryptic prey (Gittleman and Harvey, 1980; Lindström et al., 1999b). According to classic theory, predators acquire avoidance more quickly when warning signals are invariable (Müller, 1879). Thus, selection by predators should therefore favor the most common morph in the population, leading to positive frequency-dependent selection and monomorphism in aposematic species (Endler and Greenwood, 1988; Joron and Mallet, 1998; Endler and Mappes, 2004; Rowland et al., 2007; Ihalainen et al., 2008; Gordon et al., 2015). Why then, is variation in aposematic systems widespread (Briolat et al., 2019)?
Phenotypic plasticity could potentially provide a mechanism that allows for both monomorphism and variation in warning signals, through physiological plastic changes in color (e.g., changes in melanin or flavonoids production/synthesis triggered by temperature or other environmental stimuli) (Galarza et al., 2019). The desert locust, Schistocerca emarginata, is one of the most famous, but rare, examples of plasticity in aposematic species. Locusts display a shift in coloration as a response to local population density: at low-densities, individuals are solitary and cryptic (green), whereas at high-densities individuals shift to a more gregarious morph with aposematic coloration in the longer wings (yellow and black form) (Sword, 1999). This plasticity is suggested to reduce the cost of conspicuousness when locusts are rare and facilitate predator avoidance learning at high densities. However, this study involves a discrete trait, and there are currently very few studies investigating continuous plastic variation in warning signals.
The larvae of the wood tiger moth Arctia plantaginis [Arctiidae, formerly Parasemia plantaginis (Rönkä et al., 2016)] provide an excellent system to study predator-induced phenotypic plasticity in an aposematic species. Larvae are unpalatable and have a hairy black body with a dorsal continuous orange patch. The length of this patch varies significantly; from an almost entirely black phenotype to almost entirely orange (Ojala et al., 2007; Lindstedt et al., 2009, 2010, 2016; Galarza et al., 2019) and across and within populations (Ojala et al., 2007; Lindstedt et al., 2009, 2016). Previous studies in the species showed that the length of the orange patch is strongly heritable (Lindstedt et al., 2016) with temperature-induced (adaptive) plasticity (Lindstedt et al., 2009; Galarza et al., 2019). However, no study to date has evaluated predation-induced plasticity in the warning signal. Phenotypic plasticity may explain some variation observed in this system, because different signal sizes are beneficial under different conditions. A small signal (smaller orange patch) decreases the risk of predator detection (Lindstedt et al., 2008) but is associated with thermoregulation benefits (Lindstedt et al., 2009). A large orange patch is better at facilitating predator avoidance learning but less efficient at thermoregulation and immune response (Lindstedt, 2008; Lindstedt et al., 2008; Friman et al., 2009; Nielsen and Mappes, 2020). Having a plastic signal may therefore be the most efficient strategy for larvae in variable environments.
In this study we investigate to what extent predator-induced plasticity explains the continuous variation in larval warning coloration, and how predation risk influences larval life-history traits. To do so, we use a split full-sib rearing design, in which individuals are reared in the presence or absence of a non-lethal simulated bird attack. Typical bird attacks toward our hairy caterpillar include multiple pecking, handling, and dropping, which we stimulate. We then examine any changes in the length of the orange patch, as well as larval growth and development in the presence versus absence of this predation risk. If predator-induced plasticity plays a role in the size of the warning signal, we should expect differences in the length of the orange patch between the two predation treatments; this could be represented as a warning signal increase, as a more salient signal facilitates learning avoidance of avian predators, or as a warning signal decreases, as more melanic signals benefits from concealment when at high risk of detection or attack by naïve predators. We predict predation to have a negative effect on larval body size and developmental time (shorter developmental time with fewer instars) as risky environments seem to promote negative life-history shifts in many insects (e.g., Duong and McCauley, 2016).
This experiment uses a laboratory population of wood tiger moth that had been reared and maintained in a greenhouse at the University of Jyväskylä in Central Finland since 2012 from wild Finnish stock. The caterpillars were fed with a mix of lettuce (Lactuca sativa var. crispa) and dandelion leaves (Taraxacum spp.) and kept under greenhouse temperatures that followed the outdoor temperature, between 20 and 30°C during the day (∼20 h) and 15–20°C during the night (∼4 h). In Finland, this species usually has one generation per year and A. plantaginis typically overwinter as a 3rd or 4th instar larva (Lindstedt et al., 2019).
Both adults and larvae are aposematic. Larvae are hairy and unprofitable (Lindstedt et al., 2008). Within the first two instars they are cryptically colored, but around their third instar larvae develop a warning signal that grows with age (Ojala et al., 2007) that can cover from 3 to 4 segments (30% of the body length) up to 7 segments (equivalent to 80% of the total body; Figure 1; Lindstedt et al., 2008). This species is known to be a capital feeder, so emergence mass is set by resource allocation in the immature stages (Ojala et al., 2007). Individuals remain at the larval stage for most of their life cycle, on average 60 days. During this stage, larvae undergo four to seven instars. Following the larval stage, they pupate and emerge as adults 7–10 days later (Ojala et al., 2007).
Figure 1. Variation in larval warning signal. Large orange patch (left) and small orange patch (right).
We evaluated predator-induced plasticity in a full-sib rearing design, in which we selected larvae from 20 families. From each family, we randomly selected 24 individuals of the same age (same hatching day), and these were evenly split and reared in two environments: presence (treatment; N = 240) and absence of a simulated bird predator attack (control; N = 240).
We started the experiment when the larvae were 10 days old (around instar 2), and reared them individually in petri dishes. At this point, all larvae still had cryptic coloration. We applied a non-lethal simulated bird attack stimulus in the predator treatment. We used a simulated predator handling to assume a predator induce response, as it has been shown in other studies that a physical non-lethal simulated predator attack induce prey responses to the perceived risk (Watson et al., 2004; Adamo et al., 2017; Bucciarelli et al., 2017; Cinel et al., 2020). Typical bird attack toward a hairy caterpillar include multiple pecking, dropping, and billing, which we tried to stimulate by taking the larva and gently handling it (squeezing) with soft tweezers (to avoid damaging the skin) 20 times. Most larvae returned to regular behavior not long after receiving the stimulus and no regurgitation and defecation were observed during simulated attack (Authors Pers. Obs.) Although the stimulated predator attack cannot exactly imitate a true bird attack with actual mortality, based on observing both real and simulated attacks we are rather convinced this manipulation was very close to a non-lethal predator attack. The treatment was applied every second day and continued until the larvae reached pupation, excluding the days when they were obviously molting. In the control environment, we reared the larvae under normal conditions without applying the bird attack stimulus. Furthermore, all individuals, from both treatments, were checked and fed daily, the petri dishes were clean (old food was removed and feces), and kept under greenhouse conditions. To ensure that the plastic responses were not a product of the handling stress, but an actual predator-induced response, the simulated predation handling was applied during the cleaning and feeding time so that both treatment groups were disturbed around similar times. We recorded the dates, when the stimulus was applied and the molting events, as well as the signal size after every molt in each petri dish. The experiment was conducted during the summer season (May to July 2015) at the University of Jyväskylä.
Traits were measured as follows: (A) warning signal size by counting the number of orange segments every time the larva molted (Lindstedt et al., 2008). Since the segments are distinguishable it was not necessary to disturb the larva to take the measurement. (B) molts by recording the dates and times the larva molted. This was done by checking daily the presence of dried skin from a molted larva. (C) growth rate: the difference between the final body size and the initial body size (mm) over the total days until the last measurement (before pupation or death). Body length in millimeters was measured using a digital caliper (Mitutoyo 500-181-21). All larvae were measured at the beginning of the experiment (when the treatment had not yet been implemented), and then approximately every sixth day until they reached pupation or died. Larvae were not manipulated during the measurements. If they were curled, they were left undisturbed until they had returned to seemingly normal behavior and were thus possible to measure. (D) development time by recording the days from hatching until pupation and (E) pupal weight was recorded in milligrams using a Mettler Xs204 digital scale.
We used linear mixed-effects models to estimate the effects of the non-lethal predator stimulus on each of the following response variables separately: warning signal size, molting events, growth rate, developmental time, and pupal weight. The predator treatment was included as the only fixed factor for all the models. In the case of the orange signal size and molting, for which the data distribution were not clear, we selected the best model fit by comparing the AIC values of models with different distributions (Supplementary Table 1). To estimate the variation of the response due to the family, and to estimate the presence of family-by-treatment interaction, we compared models with the following random effect structures: (a) family and (b) family-by-treatment. The family-by-treatment interaction was retained in analyses if it improved the fit of the model, as judged by Likelihood Ratio tests (model comparison estimates reported in Supplementary Table 2 and full models with random effect structures in Table 1). The analyses were done with all individuals except in the molting events and developmental time analyses, when only considered those that reached pupation.
Table 1. Full summary statistics for the influence of fixed effect, predation, random effect [family (all traits) and its interaction with predation (family-by- treatment)] effects on the warning signal and larval-history traits of A. plantaginis.
We ran a survival analysis, to examine whether our light non-lethal predation stimulus induced high mortality between our treatments. Results importantly showed no significant differences between the control and the treatment [Cox proportional hazards model (P = 0.15), Supplementary Table 3].
The level of significance in all analyses was set at p < 0.05. The Satterthwaite approximation for degrees of freedom was applied in the linear mixed-effects models. All the statistical analyses were performed in R (v4.0.2; R Core Team 2020).
Larvae reared in the simulated predator handling environment displayed an orange band significantly smaller than individuals reared in the control environment (F1,427.87 = 4.634, p = 0.031; Figure 2). The phenotypic variation due to the family effect was about 15% (Table 1). In the predator treatment group, the orange band was 0.3 segments smaller than in the control (average signal 5 orange segments). Predation had a significant effect on most of the larval history traits. The larvae molted in the control group around three times after the experiment started, and 0.4 significantly more times in the predator environment (F1,312.34 = 14.776, p ≤ 0.001; Figure 3), they grew 0.08 mm less per day (F1,427.3 = 19.753, p ≤ 0.001) compare to the control ones (average 0.4 mm per day; Figure 4), the treatment did not, however, significantly affect larval developmental time (F1,311.57 = 2.33, p = 0.127; Figure 5) with 48 days in average until reach pupation. Finally, the average pupal weight in normal conditions was 201.635 mg, while in individuals from the predation treatment weighted 35.22 mg less (F1,313.39 = 78.573, p ≤ 0.001; Figure 6 and Table 1).
Figure 2. Larval orange signal size based on raw data (i.e., each black and gray line represents a family). Boxplots show the mean values on control and predator treatment. * means significance.
Figure 3. Number of molting events based on raw data (i.e., each black and gray line represents a family). Boxplots show the mean values on control and predator treatment. *** means high significance.
Figure 5. Larval development time based on raw data (i.e., each black and gray line represents a family). Boxplots show the mean values on control and predator treatment. + means outlier.
Figure 6. Pupal weight based on raw data (i.e., each black and gray line represents a family). Boxplots show the mean values on control and predator treatment. *** means high significance; + means outlier.
Organisms with complex life cycles that express plasticity in their defense and life-history traits may have a selective advantage because flexibility in strategies may allow a relatively rapid response to a range of threats and thus, increased survival. However, environmental change is often multivariate, and organisms are exposed simultaneously to multiple stressors that may have opposing effects. Furthermore, organisms can rarely can optimally respond to different stimulus since such responses may be costly and trade-off with other important traits leading to less optimal or even maladaptive responses.
We examined plastic responses to predation in the aposematic wood tiger moth larvae in warning signal efficacy and life-history traits. Aposematism is an interesting strategy to investigate potential plasticity for warning signal, since its efficacy is strongly dependent on both environments (light, background) and receivers’ vision and cognition. Thus, it is unlikely that there is only one optimal strategy for warning signal conspicuousness and potential for the plastic responses could greatly improve prey survival. Interestingly, the only example of adaptive plasticity of warning signal we are aware of is the famous desert locust, Schistocerca emarginata (Sword, 1999) that change its coloration in response to population density. Our study species, wood tiger moth larvae show continuous variation in the size of their orange warning signal, which suppresses predators willingness to attack (Lindstedt et al., 2008; Nielsen and Mappes, 2020) but at the same time, when large, increases the prey conspicuousness which in turn increases the attack risk by naïve predators (Mappes et al., 2014). We investigated whether larvae could perceive predation risk and respond on it by rearing larvae in two different environments: with and without non-lethal predator stimulus. As we expected, we found that overall predation induced plasticity in defense and life-history traits. The predator stimulus reduced orange signal size, as predicted, increased molting events, decreased growth rate, body size, and pupal mass. In contrast, the treatment did not affect developmental time. We also found a clear family effect showing that there is additive genetic variation for all measured life history traits, but no interactions between the predator treatment. This means that families overall responded to the treatment similarly although they might have expressed differences in the mean plastic responses.
We expected predation to induce a positive (increase of orange patch length) or negative (decrease of orange patch length) plastic response in larval warning signals, because larger orange patches in A. plantaginis have been proven to enhance avoidance learning of avian predators (Lindstedt et al., 2008). On the other hand, a small signal decreases the risk of predator detection (Lindstedt et al., 2008) and it is also associated with thermoregulation benefits (Lindstedt et al., 2009).
Our results show that larvae from the predator environment expressed a smaller final orange signal, and thus a more melanized body. First, this might be explained by varying costs and benefits of warning signals under different predation environments. For example, a large signal can enhance predator avoidance learning (Lindstedt et al., 2008), but it may be costly against naïve predators because it increases conspicuousness and the risk of detection (Lindstedt et al., 2008). Smaller warning signals might therefore be favored by selection when the risk of detection by naïve predators is high (Endler and Mappes, 2004; Lindstedt et al., 2008).
Second, the contrast between the two colors (orange and black) might be more important in conspicuousness indicating prey unprofitability (Aronsson and Gamberale-Stille, 2012) and its detectability. Indeed, studies done by Tullberg et al. (2005); Bohlin et al. (2008), Caro et al. (2013); Barnett and Cuthill (2014), Barnett et al. (2016); Nielsen and Mappes (2020) suggest that contrasting color patterns are more efficient warning signals against predators than a homogeneous warningly colored signal. Furthermore, they proposed that contrasting color patterns might hold a double purpose: low detectability (camouflage) at a distance, but more conspicuous when close. Suggesting that intermedium levels of internal contrast seem to be a more efficient warning signal, because it keeps the balance between cryptic and aposematic function. In our case, reduction of the orange warning signal itself may have been a response of better highlighting this color contrast and an adaptive response to decrease conspicuousness.
Third, the plasticity in the signal might also indicate a non-adaptive response to the stress suggesting that our high predation risk simulation was perhaps too strong (squeezing the larvae 20 times every time stimulus was applied) or lasted too long (every second day until pupation or death). Under novel conditions, it is expected that many of the initial plastic responses are non-adaptive, because selection has not had the opportunity to shape the plastic response (Ghalambor et al., 2007, 2015). However, it should be noted that we did not find significant mortality differences between the treatments and all larvae returned to normal behavior soon after the stimulus was applied.
Fourth, increased larva cuticular melanin deposition is a common stress-response in insects to conditions such as exposure to UV radiation; probably due to the photoprotective properties (Debecker et al., 2015). It can also be a stress response to low temperatures (Goulson, 1994; Galarza et al., 2019), potentially a thermoregulation benefit to adjust to new conditions (Galarza et al., 2019), and predator risk. Under predation risk increased melanin can be an anticipatory response to the potential risk of cuticular wounding (Duong and McCauley, 2016), because of the important role of melanin in immune response and wound healing (Barnes and Siva-Jothy, 2000; Elliot et al., 2003; Friman et al., 2009; Beckage, 2011; Parle et al., 2016). Our stimulus, however, non-lethal caused larvae to suffer some hair loss during the attack simulation (Abondano Pers Obs). This loss could have stimulated an anticipatory risk of cuticular wounding in our larvae (Duong and McCauley, 2016) thus activating the melanization cascade, a physiological mechanism involved in immune responses and cuticle plugging and healing (De Gregorio et al., 2002; Beckage, 2011).
The plasticity responses observed in life-history traits under the treatment can be also considered non-adaptive response, according to life-history theory which should predict faster life histories under predation risk (Stearns and Koella, 1986; Abrams and Rowe, 1996; Arendt, 1997; Nylin and Gotthard, 1998; Roff, 2002; Benard, 2004). Instead, larvae in our predation treatment showed a lower growth rate, a smaller final body size, and a lighter pupal weight but similar developmental times compared to the control. A slow growth rate could be compensated with longer development times, but at the cost of increased risk of predation. On the other hand, a fast growth could mean avoiding predation risks but at the cost of reaching metamorphosis at an optimal size.
The lower growth rate and smaller final body size could be potentially explained by an indirect effect of the increase in melanization through the costly melanin hypothesis. Some studies suggest darker-induced phenotypes might be costly (as resources have to be allocated for melanin synthesis) in terms of lower growth rates and smaller body sizes (Goulson, 1994; Debecker et al., 2015; Galarza et al., 2019; Lindstedt et al., 2019). The results could also be explained by environmental stress during development. Here, constant manipulation or stress could alter overall physiological functioning, challenging the organism to keep metabolic functions normal (Chevin and Hoffmann, 2017; Taborsky et al., 2020). As a result of the cumulative stress, larvae in the predator treatment could have been limited to reach a minimal viable weight (MVW), defined by Davidowitz et al. (2003) as the minimal amount of resources necessary for a developing larva to successfully pupate. Lastly, our results could reflect altered foraging activity. Larvae could reduce their feeding activity as a behavioral defense to reduce predation risk, which results in less energy allocated in growth (Lima and Dill, 1990; Stamp and Bowers, 1993; Cressler et al., 2010). Because A. plantaginis is a capital breeder (Ojala et al., 2007), a lower growth rate, together with a limited developmental time, could constrain the resources allocated for pupation, leading to a lighter pupal weight. This could be considered as a stress-response based on the finding by Galarza et al. (2019), in which larvae of A. plantaginis reared in low temperatures, showed a lower growth rate, but a longer developmental time leading to similar pupal weights compared to larvae reared in high temperatures.
Larvae in the predator treatment underwent a higher number of molting events than the control ones. Individuals may increase their molting under conditions that constrain body size to compensate for this reduced growth rate, because body size increases at each larval molt. This stress response to unfavorable conditions is commonly observed in most insect species (Esperk et al., 2007), and may work as a compensatory mechanism for larvae to reach the threshold size and survive in adverse conditions (Nijhout, 1998). It could also help larvae reach pupation at the optimal size for hibernation under stressful conditions (Esperk et al., 2007; Barraclough et al., 2014). However, larvae did not differ in the age at pupation and the final body size was smaller in the predation treatment, suggesting the increase in molts did not work as a compensatory mechanism to increase body size or pupal mass. Perhaps the increment in molts could be explained as a response to restore the hair lost during the attack simulation in the predation treatment. Although hairiness does not decrease predator attacks or enhance avoidance learning of avian predators (Lindstedt et al., 2008), it can play an important role in protecting prey against another type of predators [e.g., insects; (Dyer, 1995)]. A higher number of molting events (increase in hairiness), together with increased melanization (decrease in orange patch size) may therefore be an investment in antipredator defenses, and a trade-off with investment in growth (larval growth rate and pupal weight).
Understanding how organisms and populations can adapt to changing environmental conditions is a crucial topic in evolutionary biology, considering the rapid environmental changes caused by human activity. Here, we have demonstrated that phenotypic plasticity is a potential mechanism for aposematic species to respond to novel stressful environments. We found that high predation risk, a novel condition for the larvae, induced plasticity in the warning signal by increasing melanin deposition. Furthermore, the predation treatment induced stress responses in life-history traits, indicating a potential trade-off in resource allocation for signal and life-history traits. However, to what extent phenotypic plasticity is beneficial, creating novel opportunities for selection, or harmful, constraining adaptive potential in a challenging environment, is yet unclear (Sgrò et al., 2016; Oostra et al., 2018). Future empirical studies are needed to investigate long-term fitness consequences of phenotypic plasticity, and whether plastic responses in warning signals are linked to changes in antipredator behavior. For example, larvae with a more conspicuous signal could be expected to spend more time in exposed locations compared to larvae with a more cryptic signal (Nielsen and Mappes, 2020). Finally, our study investigated phenotypic plasticity in laboratory-reared individuals with a homogenous population history. However, the evolutionary history of the population might have important effects on its plasticity, and future studies should therefore investigate phenotypic plasticity in wild populations with different evolutionary histories to better understand whether plastic responses are adaptive and how insect populations will perform under different scenarios.
The original contributions presented in the study are included in the article/Supplementary Material, further inquiries can be directed to the corresponding author.
SG designed the study. DA conducted the experiments and led the writing of the manuscript. DA and SG analyzed the data. All authors contributed critically to the drafts, edited the manuscript, and approved the final version.
This study was funded by the Academy of Finland; project nos. 319124 and 2100000256 to JM and postdoctoral project no. 2100002744 to SG.
The authors declare that the research was conducted in the absence of any commercial or financial relationships that could be construed as a potential conflict of interest.
We would like to thank Kaisa Suisto for help with larva rearing and Andrés López-Sepulcre for helping in the statistical analyses. Liisa Hämäläinen and Lee Dietterich provided valuable feedback on the manuscript. We would also like to thank two reviewers for comments that improved an earlier version of the manuscript.
The Supplementary Material for this article can be found online at: https://www.frontiersin.org/articles/10.3389/fevo.2021.658177/full#supplementary-material
Adamo, S. A., Easy, R. H., Kovalko, I., MacDonald, J., McKeen, A., Swanburg, T., et al. (2017). Predator exposure-induced immunosuppression: trade-off, immune redistribution or immune reconfiguration?. J. Exp. Biol. 220, 868–875. doi: 10.1242/jeb.153320
Arendt, J. D. (1997). Adaptive Intrinsic Growth Rates: an Integration Across Taxa. Q. Rev. Biol. 72, 149–177. doi: 10.1086/419764
Aronsson, M., and Gamberale-Stille, G. (2012). Evidence of signaling benefits to contrasting internal color boundaries in warning coloration. Behav. Ecol. 24, 349–354. doi: 10.1093/beheco/ars170
Barnes, A. I., and Siva-Jothy, M. T. (2000). Density-dependent prophylaxis in the mealworm beetle Tenebrio molitor L. (Coleoptera: Tenebrionidae): cuticular melanization is an indicator of investment in immunity. Proc. R. Soc. B Biol. Sci. 267, 177–182. doi: 10.1098/rspb.2000.0984
Barnett, J. B., and Cuthill, I. C. (2014). Distance-dependent defensive coloration. Curr. Biol. 24, R1157–R1158. doi: 10.1016/j.cub.2014.11.015
Barnett, J. B., Scott-Samuel, N. E., and Cuthill, I. C. (2016). Aposematism: balancing salience and camouflage. Biol. Lett. 12, 2–5. doi: 10.1098/rsbl.2016.0335
Barraclough, E. I., Burgess, E. P. J., Kean, A. M., and Malone, L. A. (2014). Growth and development in a lepidopteran with variable instar number, Pseudocoremia suavis (Geometridae), under standard rearing conditions and when parasitised by Meteorus pulchricornis (Hymenoptera: Braconidae). Eur. J. Entomol. 111, 501–511. doi: 10.14411/eje.2014.062
Benard, M. F. (2004). Predator-induced phenotypic plasticty in organisms with complex life histories. Annu. Rev. Ecol. Evol. Syst. 35, 651–673. doi: 10.2307/annurev.ecolsys.35.021004.30000024
Bohlin, T., Tullberg, B. S., and Merilaita, S. (2008). The effect of signal appearance and distance on detection risk in an aposematic butterfly larva (Parnassius apollo). Anim. Behav. 76, 577–584. doi: 10.1016/j.anbehav.2008.02.012
Briolat, E. S., Burdfield-Steel, E. R., Paul, S. C., Rönkä, K. H., Seymoure, B. M., Stankowich, T., et al. (2019). Diversity in warning coloration: selective paradox or the norm?. Biolo. Rev. 94, 388–414. doi: 10.1111/brv.12460
Bucciarelli, G. M., Shaffer, H. B., Green, D. B., and Kats, L. B. (2017). An amphibian chemical defense phenotype is inducible across life history stages. Sci. Rep. 7:8185. doi: 10.1038/s41598-017-08154-z
Caro, T., Stankowich, T., Kiffner, C., and Hunter, J. (2013). Are spotted skunks conspicuous or cryptic?. Ethol. Ecol. Evol. 25, 144–160. doi: 10.1080/03949370.2012.744359
Chevin, L. M., and Hoffmann, A. A. (2017). Evolution of phenotypic plasticity in extreme environments. Philo. Trans. R. Soc. B Biol. Sci. 372:20160138. doi: 10.1098/rstb.2016.0138
Cinel, S. D., Hahn, D. A., and Kawahara, A. Y. (2020). Predator-induced stress responses in insects: a review. J. Insect Physiol. 122:104039. doi: 10.1016/j.jinsphys.2020.104039
Corl, A., Bi, K., Luke, C., Challa, A. S., Stern, A. J., Sinervo, B., et al. (2018). The Genetic Basis of Adaptation following Plastic Changes in Coloration in a Novel Environment. Curr. Biol. 28, 2970–2977. doi: 10.1016/j.cub.2018.06.075
Cressler, C. E., King, A. A., and Werner, E. E. (2010). Interactions between behavioral and life-history trade-offs in the evolution of integrated predator-defense plasticity. Am. Nat. 176, 276–288. doi: 10.1086/655425
Davidowitz, G., D’Amico, L. J., and Nijhout, H. F. (2003). Critical weight in the development of insect body size. Evol. Dev. 5, 188–197. doi: 10.1046/j.1525-142X.2003.03026.x
De Gregorio, E., Han, S. J., Lee, W. J., Baek, M. J., Osaki, T., Kawabata, S. I., et al. (2002). An immune-responsive Serpin regulates the melanization cascade in Drosophila. Dev. Cell 3, 581–592. doi: 10.1016/S1534-5807(02)00267-8
Debecker, S., Sommaruga, R., Maes, T., and Stoks, R. (2015). Larval UV exposure impairs adult immune function through a trade-off with larval investment in cuticular melanin. Funct. Ecol. 29, 1292–1299. doi: 10.1111/1365-2435.12435
Duong, T., and McCauley, S. (2016). Predation risk increases immune response in a larval dragonfly (Leucorrhinia intacta). Ecology 97, 1605–1610. doi: 10.1890/15-1964.1
Dyer, L. A. (1995). Tasty Generalists and Nasty Specialists? Antipredator mechanisms in Tropical Lepidopteran Larvae. Ecology 76, 1483–1496. doi: 10.2307/1938150
Elliot, S. L., Blanford, S., Horton, C. M., and Thomas, M. B. (2003). Fever and phenotype: transgenerational effect of disease on desert locust phase state. Ecol. Lett. 6, 830–836. doi: 10.1046/j.1461-0248.2003.00487.x
Endler, J. A. (1986). “Defense Against Predators,” in Predator-Prey Relationships, Perspectives And Approaches From The Study Of Lower Vertebrates, eds M. E. Feder and G. E. Lauder (Chicago: University of Chicago Press), 109–134.
Endler, J. A. (1991a). “Interactions between predators and prey,” in Behavioural Ecology: An Evolutionary Approach, 3rd edn, eds J.R. Krebs and N.B. Davies (Oxford: Blackwell), 169–196.
Endler, J. A. (1991b). Variation in the appearance of guppy color patterns to guppies and their predators under different visual conditions. Vision Res. 31, 587–608. doi: 10.1016/0042-6989(91)90109-I
Endler, J. A., and Greenwood, J. J. D. (1988). Frequency-Dependent Predation, Crypsis and Aposematic Coloration [and Discussion]. Philos. Trans. R. Soc. Lond. B Biol. Sci. 319, 505–523. doi: 10.1098/rstb.1988.0062
Endler, J. A., and Mappes, J. (2004). Predator mixes and the conspicuousness of aposematic signals. Am. Nat. 163, 532–547. doi: 10.1086/382662
Esperk, T., Tammaru, T., and Nylin, S. (2007). Intraspecific variability in number of larval instars in insects. J. Econ. Entomol. 100, 627–645. doi: 10.1093/jee/100.3.627
Friman, V.-P., Lindstedt, C., Hiltunen, T., Laakso, J., and Mappes, J. (2009). Predation on multiple trophic levels shapes the evolution of pathogen virulence. PLoS One 4:e6761. doi: 10.1371/journal.pone.0006761
Galarza, J. A., Dhaygude, K., Ghaedi, B., Suisto, K., Valkonen, J., and Mappes, J. (2019). Evaluating responses to temperature during pre-metamorphosis and carry-over effects at post-metamorphosis in the wood tiger moth (Arctia plantaginis). Philos. Trans. R. Soc. B Biol. Sci. 374:20190295. doi: 10.1098/rstb.2019.0295
Ghalambor, C. K., Hoke, K. L., Ruell, E. W., Fischer, E. K., Reznick, D. N., and Hughes, K. A. (2015). Non-adaptive plasticity potentiates rapid adaptive evolution of gene expression in nature. Nature 525, 372–375. doi: 10.1038/nature15256
Ghalambor, C. K., McKay, J. K., Carroll, S. P., and Reznick, D. N. (2007). Adaptive versus non-adaptive phenotypic plasticity and the potential for contemporary adaptation in new environments. Funct. Ecol. 21, 394–407. doi: 10.1111/j.1365-2435.2007.01283.x
Gittleman, J. L., and Harvey, P. H. (1980). Why are distasteful prey not cryptic?. Nature 286, 149–150. doi: 10.1038/286149a0
Gordon, S. P., Hendry, A. P., and Reznick, D. N. (2017). Predator-induced Contemporary Evolution, Phenotypic Plasticity, and the Evolution of Reaction Norms in Guppies. Copeia 105, 514–522. doi: 10.1643/CE-16-522
Gordon, S. P., Reznick, D., Arendt, J. D., Roughton, A., Ontiveros Hernandez, M. N., Bentzen, P., et al. (2015). Selection analysis on the rapid evolution of a secondary sexual trait. Proc. R. Soc. B Biol. Sci. 282:20151244. doi: 10.1098/rspb.2015.1244
Gotthard, K., and Nylin, S. (1995). Adaptive Plasticity and Plasticity as an Adaptation: a Selective Review of Plasticity in Animal Morphology and Life History. Oikos 74, 3–17. doi: 10.2307/3545669
Goulson, D. (1994). Determination of larval melanization in the moth, Mamestra brassicae, and the role of melanin in thermoregulation. Heredity 73, 471–479. doi: 10.1038/hdy.1994.145
Hammill, E., Rogers, A., and Beckerman, A. P. (2008). Costs, benefits and the evolution of inducible defences: a case study with Daphnia pulex. J. Evol. Biol. 21, 705–715. doi: 10.1111/j.1420-9101.2008.01520.x
Hochkirch, A., Deppermann, J., and Gröning, J. (2008). Phenotypic plasticity in insects: the effects of substrate color on the coloration of two ground-hopper species. Evol. Dev. 10, 350–359. doi: 10.1111/j.1525-142X.2008.00243.x
Ihalainen, E., Lindström, L., Mappes, J., and Puolakkainen, S. (2008). Can experienced birds select for Müllerian mimicry?. Behav. Ecol. 19, 362–368. doi: 10.1093/beheco/arm151
Inbar, M., and Lev-Yadun, S. (2005). Conspicuous and aposematic spines in the animal kingdom. Naturwissenschaften 92, 170–172. doi: 10.1007/s00114-005-0608-2
Joron, M., and Mallet, J. L. B. (1998). Diversity in mimicry: paradox or paradigm?. Trends Ecol. Evol. 13, 461–466. doi: 10.1016/S0169-5347(98)01483-9
Koch, E. L., and Guillaume, F. (2020). Additive and mostly adaptive plastic responses of gene expression to multiple stress in Tribolium castaneum. PLoS Genet. 16:e1008768. doi: 10.1371/journal.pgen.1008768
Lima, S. L., and Dill, L. M. (1990). Behavioral decisions made under the risk of predation: a review and prospectus. Can. J. Zool 68, 619–640. doi: 10.1139/z90-092
Lindstedt, C. (2008). Maintenance of Variation in Warning Signals under Opposing Selection Pressures. Finland: University of Jyväskylä.
Lindstedt, C., Lindström, L., and Mappes, J. (2008). Hairiness and warning colours as components of antipredator defence: additive or interactive benefits?. Anim. Behav. 75, 1703–1713. doi: 10.1016/j.anbehav.2007.10.024
Lindstedt, C., Lindström, L., and Mappes, J. (2009). Thermoregulation constrains effective warning signal expression. Evolution 63, 469–478. doi: 10.1111/j.1558-5646.2008.00561.x
Lindstedt, C., Morehouse, N., Pakkanen, H., Casas, J., Christides, J. P., Kemppainen, K., et al. (2010). Characterizing the pigment composition of a variable warning signal of Parasemia plantaginis larvae. Funct. Ecol. 24, 759–766. doi: 10.1111/j.1365-2435.2010.01686.x
Lindstedt, C., Schroderus, E., Lindström, L., Mappes, T., and Mappes, J. (2016). Evolutionary constraints of warning signals: a genetic trade-off between the efficacy of larval and adult warning coloration can maintain variation in signal expression. Evolution 70, 2562–2572. doi: 10.1111/evo.13066
Lindstedt, C., Suisto, K., and Mappes, J. (2019). Appearance before performance? Nutritional constraints on life-history traits, but not warning signal expression in aposematic moths. J. Anim. Ecol. 89, 494–505. doi: 10.1111/1365-2656.13103
Lindström, L., Alatalo, R. V., and Mappes, J. (1999a). Reactions of hand-reared and wild-caught predators toward warningly colored, gregarious, and conspicuous prey. Behav. Ecol. 10, 317–322. doi: 10.1093/beheco/10.3.317
Lindström, L., Alatalo, R. V., Mappes, J., Riipi, M., and Vertainen, L. (1999b). Can aposematic signals evolve by gradual change?. Nature 397, 249–251. doi: 10.1038/16692
Mappes, J., Kokko, H., Ojala, K., and Lindström, L. (2014). Seasonal changes in predator community switch the direction of selection for prey defences. Nat. Commun. 5:5016. doi: 10.1038/ncomms6016
Mappes, J., Marples, N., and Endler, J. A. (2005). The complex business of survival by aposematism. Trends Ecol. Evol. 20, 598–603. doi: 10.1016/j.tree.2005.07.011
Mastrota, N. F., and Mench, J. A. (1995). Colour avoidance in northern bobwhites: effects of age, sex and previous experience. Anim. Behav. 50, 519–526. doi: 10.1006/anbe.1995.0266
Merilaita, S., Scott-Samuel, N. E., and Cuthill, I. C. (2017). How camouflage works. Philos. Trans. R. Soc. B Biol. Sci. 372:20160341. doi: 10.1098/rstb.2016.0341
Morris, M. R. J., and Rogers, S. M. (2013). Overcoming maladaptive plasticity through plastic compensation. Curr. Zool. 59, 526–536. doi: 10.1093/czoolo/59.4.526
Müller, F. (1879). Ituna and Thyridia; a remarkable case of mimicry in butterflies. Trans. Entomol. Soc. Lond. 1879:100.
Mutamiswa, R., Machekano, H., Chidawanyika, F., and Nyamukondiwa, C. (2019). Life-stage related responses to combined effects of acclimation temperature and humidity on the thermal tolerance of Chilo partellus (Swinhoe) (Lepidoptera: Crambidae). J. Therm. Biol. 79, 85–94. doi: 10.1016/j.jtherbio.2018.12.002
Nielsen, M. E., and Mappes, J. (2020). Out in the open: behavior’s effect on predation risk and thermoregulation by aposematic caterpillars. Behav. Ecol. 31, 1031–1039. doi: 10.1093/beheco/araa048
Nylin, S., and Gotthard, K. (1998). Plasticity in life-history traits. Annu. Rev. Entomol. 43, 63–83. doi: 10.1146/annurev.ento.43.1.63
Ojala, K., Lindström, L., and Mappes, J. (2007). Life-history constraints and warning signal expression in an arctiid moth. Funct. Ecol. 21, 1162–1167. doi: 10.1111/j.1365-2435.2007.01322.x
Oostra, V., Saastamoinen, M., Zwaan, B. J., and Wheat, C. W. (2018). Strong phenotypic plasticity limits potential for evolutionary responses to climate change. Nat. Commun. 9:1005. doi: 10.1038/s41467-018-03384-9
Parle, E., Dirks, J., and Taylor, D. (2016). Damage, repair and regeneration in insect cuticle: the story so far, and possibilities for the future. Arthropod Struct. Dev. 46, 49–55. doi: 10.1016/j.asd.2016.11.008
Perry, B. W., Schield, D. R., and Castoe, T. A. (2018). Evolution: plasticity versus Selection, or Plasticity and Selection?. Curr. Biol. 28, R1104–R1106. doi: 10.1016/j.cub.2018.07.050
Abrams, P. A., and Rowe, L. (1996). The Effects of Predation on the Age and Size of Maturity of Prey. Evolution 50, 1052–1061. doi: 10.1111/j.1558-5646.1996.tb02346.x
Poulton, E. (1890). The Colours of Animals: Their Meaning and Use, Especially Considered in the Case of Insects. New York: D. Appleton and Company.
Roff, D. (2002). “Life History Evolution,” in Encyclopedia of Biodiversity, ed. S. A. Levin (Sunderland: Sinauer Associates), 631–641.
Rönkä, K., Mappes, J., Kaila, L., and Wahlberg, N. (2016). Putting Parasemia in its phylogenetic place: a molecular analysis of the subtribe Arctiina (Lepidoptera). Syst. Entomol. 41, 844–853. doi: 10.1111/syen.12194
Rowland, H. M., Ihalainen, E., Lindström, L., Mappes, J., and Speed, M. P. (2007). Co-mimics have a mutualistic relationship despite unequal defences. Nature 448, 64–67. doi: 10.1038/nature05899
Schuler, W., and Roper, T. (1992). “Responses to warning coloration in avian predators,” in Advances in the Study of Behavior, eds P. J. B. Slater, J. S. Rosenblatt, C. Beer, and M. Milinski (Cambridge: Academic Press), 111–146. doi: 10.1016/s0065-3454(08)60143-6
Sgrò, C. M., Terblanche, J. S., and Hoffmann, A. A. (2016). What Can Plasticity Contribute to Insect Responses to Climate Change?. Annu. Rev. Entomol. 61, 433–451. doi: 10.1146/annurev-ento-010715-023859
Speed, M. P., and Ruxton, G. D. (2005). Warning Displays in Spiny Animals: one (More) Evolutionary Route To Aposematism. Evolution 59, 2499–2508. doi: 10.1554/05-267.1
Stamp, N. E., and Bowers, M. D. (1993). Presence of predatory wasps and stinkbugs alters foraging behavior of cryptic and non-cryptic caterpillars on plantain (Plantago lanceolata). Oecologia 95, 376–384. doi: 10.1007/BF00320992
Stearns, S. C. (1989). The evolutionary significance of phenotypic plasticity. BioScience 39, 436–445. doi: 10.2307/1311135
Stearns, S. C., and Koella, J. C. (1986). The Evolution of Phenotypic Plasticity in Life-History Traits: predictions of Reaction Norms for Age and Size at Maturity. Evolution 40, 893–913. doi: 10.2307/2408752
Stevens, M. (2016). Color change, phenotypic plasticity, and camouflage. Front. Ecol. Evol. 4:1–10. doi: 10.3389/fevo.2016.00051
Taborsky, B., English, S., Fawcett, T. W., Kuijper, B., Leimar, O., McNamara, J. M., et al. (2020). Towards an Evolutionary Theory of Stress Responses. Trends Ecol. Evol. 36, 39–48. doi: 10.1016/j.tree.2020.09.003
Tollrian, R. (1995). Predator-Induced Morphological Defenses: costs, Life History Shifts, and Maternal Effects in Daphnia Pulex. Ecology 76, 1691–1705. doi: 10.2307/1940703
Tollrian, R., and Dodson, S. I. (1999). “Inducible defenses in Cladocera: constraints, costs and multipredator environments,” in The Ecology and Evolution of Inducible Defenses, eds E. R. Tollrian and C. D. Harvell (Princeton: Princeton University Press), 177–202. doi: 10.2307/j.ctv1ddd1cn.15
Tullberg, B. S., Merilaita, S., and Wiklund, C. (2005). Aposematism and crypsis combined as a result of distance dependence: functional versatility of the colour pattern in the swallowtail butterfly larva. Proc. R. Soc. B Biol. Sci. 272, 1315–1321. doi: 10.1098/rspb.2005.3079
Watson, R. T., Mathis, A., and Thompson, R. (2004). Influence of physical stress, distress cues, and predator kairomones on the foraging behavior of Ozark zigzag salamanders, Plethodon angusticlavius. Behav. Processes 65, 201–209. doi: 10.1016/j.beproc.2003.09.007
Keywords: predation, Plastic response, Aposematism, Life-history, antipredator, Larva, Costs, maladaptation
Citation: Almeida DA, Mappes J and Gordon S (2021) Predator-Induced Plasticity on Warning Signal and Larval Life-History Traits of the Aposematic Wood Tiger Moth, Arctia plantaginis. Front. Ecol. Evol. 9:658177. doi: 10.3389/fevo.2021.658177
Received: 25 January 2021; Accepted: 02 June 2021;
Published: 25 June 2021.
Edited by:
Chiara Morosinotto, Novia University of Applied Sciences, FinlandReviewed by:
Matthew R. E. Symonds, Deakin University, AustraliaCopyright © 2021 Almeida, Mappes and Gordon. This is an open-access article distributed under the terms of the Creative Commons Attribution License (CC BY). The use, distribution or reproduction in other forums is permitted, provided the original author(s) and the copyright owner(s) are credited and that the original publication in this journal is cited, in accordance with accepted academic practice. No use, distribution or reproduction is permitted which does not comply with these terms.
*Correspondence: Diana Abondano Almeida, QWJvbmRhbm9BbG1laWRhQGJpby51bmktZnJhbmtmdXJ0LmRl
†Present address: Diana Abondano Almeida, Department of Wildlife-/Zoo-Animal-Biology and Systematics, Faculty of Biological Sciences, Goethe-Universität Frankfurt am Main, Frankfurt, Germany; Swanne Gordon, Department of Biology, Washington University in St. Louis, St. Louis, MO, United States
Disclaimer: All claims expressed in this article are solely those of the authors and do not necessarily represent those of their affiliated organizations, or those of the publisher, the editors and the reviewers. Any product that may be evaluated in this article or claim that may be made by its manufacturer is not guaranteed or endorsed by the publisher.
Research integrity at Frontiers
Learn more about the work of our research integrity team to safeguard the quality of each article we publish.