- Laboratory of Neuroethology, SOKENDAI, The Graduate University for Advanced Studies, Hayama, Japan
We demonstrate that the small white butterfly, Pieris rapae, uses color vision when searching flowers for foraging. We first trained newly emerged butterflies in a series of indoor behavioral experiments to take sucrose solution on paper disks, colored either blue, green, yellow, or red. After confirming that the butterflies were trained to visit a certain colored disk, we presented all disks simultaneously. The butterflies selected the disk of trained color, even among an array of disks with different shades of gray. We performed the training using monochromatic lights and measured the action spectrum of the feeding behavior to determine the targets’ Pieris-subjective brightness. We used the subjective brightness information to evaluate the behavioral results and concluded that Pieris rapae butterflies discriminate visual stimuli based on the chromatic content independent of the intensity: they have true color vision. We also found that Pieris butterflies innately prefer blue and yellow disks, which appears to match with their flower preference in the field, at least in part.
Introduction
Color vision is the ability to discriminate visual stimuli based on the chromatic content irrespective of the brightness. Since the first demonstration of color vision in flower-visiting honeybees (Turner, 1910; Frisch, 1914), it has become a central topic of insect behavioral neuroscience. Foraging honeybee uses a trichromatic system based on the UV, blue, and green-sensitive photoreceptors in their compound eyes (Menzel and Backhaus, 1989; Wakakuwa et al., 2005).
Flowers attract many other insects for pollination, including lepidopterans, dipterans, hemipterans, and coleopterans (Chittka and Thomson, 2001). Their visual systems are not necessarily the same as that of the honeybee. For example, the Japanese yellow swallowtail, Papilio xuthus, has six spectral receptor classes comprising a UV, violet, blue, green, red, and broadband class (Arikawa, 2003). As in the honeybee, Papilio uses color vision for finding flowers (Kelber and Pfaff, 1999; Kinoshita et al., 1999), but it covers a wider spectral range. The wavelength discrimination property of Papilio xuthus exhibits three highly sensitive wavelength regions, indicating that the system is tetrachromatic, with a UV, blue, green, and red channel (Koshitaka et al., 2008).
While Papilio’s color vision has been studied intensively among butterflies, that of the small white, Pieris rapae, another important butterfly species for biology because of its abundance and impact on crops, is poorly understood. The spectral organization of the Pieris compound eye differs from that of Papilio: most notably, Pieris rapae has three classes of red receptors (Blake et al., 2019). Presumably, such a species-specific eye organization creates ecologically essential differences in the color discrimination ability.
The visual characteristics of the cosmopolitan pest species Pieris rapae have been studied particularly well in relationship with its reproductive behavior (Traynier, 1984; Kelber, 2001; Wakakuwa et al., 2004; Blake et al., 2020). Males of the Japanese subspecies Pieris rapae crucivora search for potential mates using the wings’ UV reflection. This is one of the earliest examples of how UV light affects the visual behavior of an insect (Obara and Hidaka, 1968; Obara and Majerus, 2000).
As adults, Pieris butterflies are nectar feeders. They presumably find and discriminate nectar-providing flowers by their colors (Kolb and Scherer, 1982; Scherer and Kolb, 1987; Goulson and Cory, 1993; Kandori and Ohsaki, 1996; Kelber, 2001). However, the previous studies do not conclusively demonstrate color vision per se, because the experiments were not designed to do so. Here we focus on this particular point and establish color vision in foraging Pieris rapae.
Materials and Methods
Materials
We used summer-form virgin females of the small white butterfly Pieris rapae crucivora Boisduval (Lepidoptera, Pieridae) from a laboratory culture derived from eggs laid by females caught around the campus of Sokendai, Hayama, Japan. The hatched larvae were fed on fresh kale leaves at 28°C under a 16 h:8 h light:dark cycle. Pupae were allowed to emerge in a plastic box. We defined the day of emergence as post-emergence day 1.
Experimental Setup
We performed experiments using freely flying butterflies and colored disks in a cage covered with nylon net (W80 × D60 × H45 cm, see Kinoshita et al., 1999). The cage was illuminated with eight 300 W halogen lamps. The reflection spectrum of a MgO-coated surface, placed on the cage floor and measured using a calibrated spectrometer (HSU-100S, Asahi Spectra, Japan), represented the illumination spectrum (Figure 1A, spectrum a). The room temperature was set at 28°C. The cage floor was covered with black cardboard.
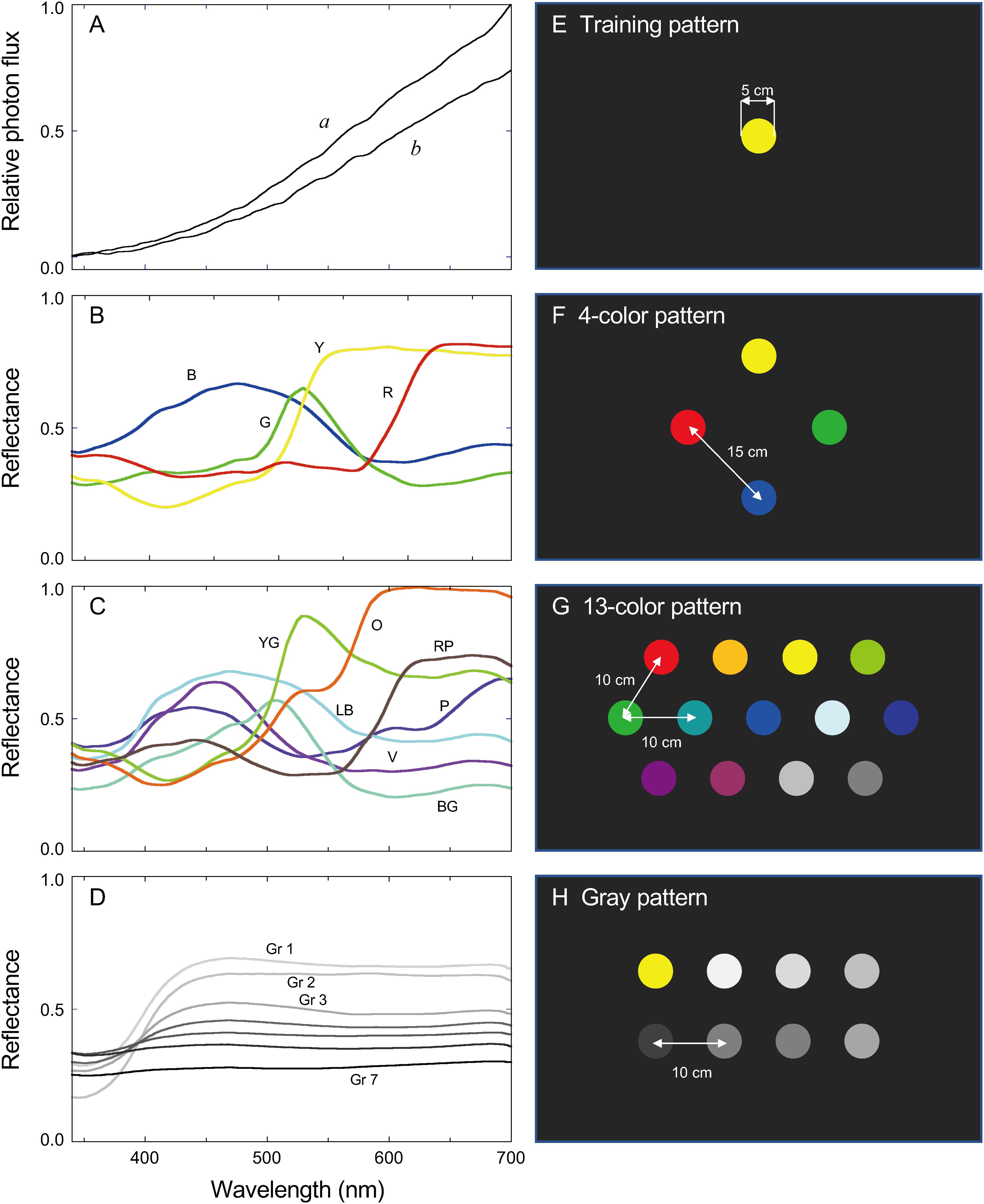
Figure 1. (A) Illumination spectra of the experimental arena: a, eight 300 W halogen lamps for freely flying butterflies; b, four 300 W halogen lamps for tethered butterflies. (B) Reflectance spectra of colored papers used for training. (C) Reflectance spectra of colored papers used in the 13-color pattern (see Figure 1D) together with those shown in (B). (D) Reflectance spectra of papers of seven different shades of gray. (E) Training pattern. (F) 4-color pattern. (G) 13-color pattern. (H) Gray pattern. The subjective brightness of each colored paper, Bi, is listed in Table 1. B, blue; BG, blue-green; G, green; Gr, gray; LB, light-blue; O, orange; P, purple; R, red; RP, red-purple; V, violet; Y, yellow; YG yellow-green.
Stimuli were ø 5 cm disks of colored paper (Training color 120, Nihon Shikisai Co. Ltd., Tokyo Japan) centrally arranged on the cage floor. Figures 1B–D show reflectance spectra of the papers. We presented the disks in four patterns, as shown in Figures 1E–H. The 4-color pattern had disks of four basic colors (blue, green, yellow, and red). The 13-color pattern had the disks of seven other colors (Figure 1C) in addition to the basic four colors (Figure 1B) plus two grays (Gray 2 and 3, Figure 1D). For patterns with more than one disk (Figures 1F–H), we randomized the disks’ relative position during tests. We covered the patterns with anti-reflection glass to protect the patterns from unwanted contamination and suppress specular reflection that might affect the visual perception of the stimuli.
We performed experiments using tethered butterflies and monochromatic lights on a bench setup (Figure 2A), a modified version of what we used previously (Koshitaka et al., 2008). Briefly, the benchtop had a piece of frosted quartz glass as the back-projection screen, which was illuminated from below with monochromatic light of varying wavelength and intensity produced by a 500 W xenon arc, a monochromator, and a set of quartz neutral density filters. We measured the photon flux of the monochromatic lights using a radiometer (Model 470D, Sanso, Tokyo, Japan). The benchtop was illuminated by four 300 W halogen lamps (Figure 1A, spectrum b). The room temperature was set at 28°C.
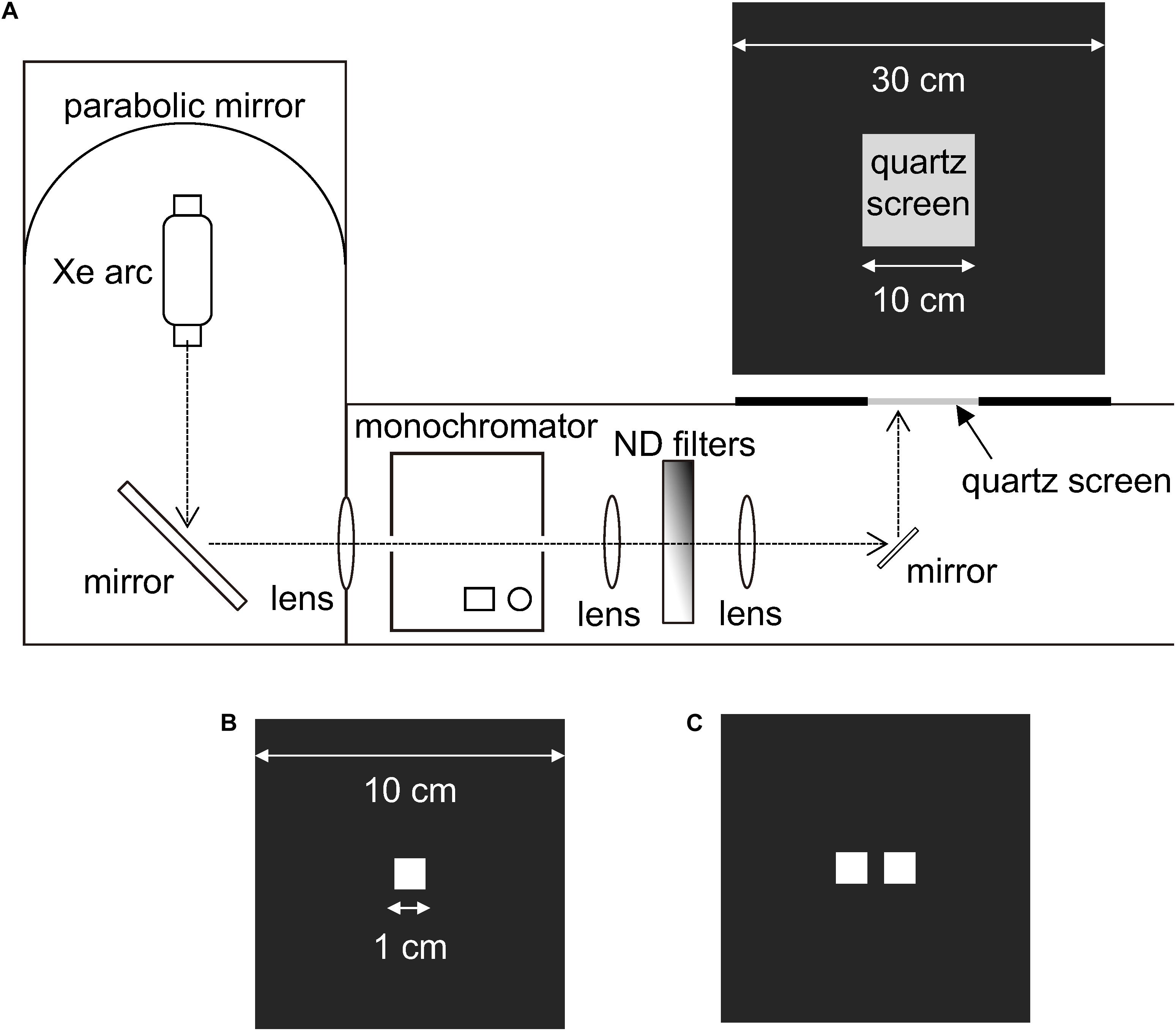
Figure 2. Experimental setup for presenting monochromatic lights. (A) Light from a Xe arc was directed into a monochromator via a mirror. The monochromatic light, whose intensity was attenuated with ND filters, was reflected upwards to a quartz screen. (B) A single-windowed plate used to cover the quartz screen during training sessions. (C) A double-windowed plate used to cover the quartz screen during test sessions.
Training and Tests Using Colored Disks
During the training phase of free-flying butterflies, we released only one butterfly in the cage with a training pattern (Figure 1E), having a few drops of 6% sucrose solution on the disk. We defined a visit as a positive response when the butterfly landed and extended its proboscis toward the colored disk.
To check whether or not the foraging behavior exhibits any bias, we recorded the color of the disk that naïve butterflies visited for the first time. On post-emergence day 4, we released a butterfly, which was never fed after emergence, in the cage with a four-color pattern but no sucrose solution (Figure 1F). We changed the relative position of the four disks for each individual to eliminate any possible effects of disk position.
For testing color vision, we trained butterflies to visit disks of a certain color to forage. On post-emergence day 3, the starved butterflies were individually trained by letting them take 6% sucrose solution on the colored disk of a training pattern (Figure 1E). If they did not visit the disk spontaneously, we manually placed the butterflies on the disk. We repeated the training once a day for 6 days. We quantified the training effect by performing the color learning tests with a four-color pattern (Figure 1F) immediately before the day’s training session. We released a butterfly in the cage and let the butterfly visit the disks five times, and counted the number of visits to each color. If the butterfly visited the disks less than five times within10 min, we finished the test and recorded the performed visits. We randomly changed the array of colors on the pattern after each visit to eliminate a possible positional effect.
We evaluated the final effect of training on post-emergence day 9. We let them visit 10 times on the 13-color pattern (Figure 1G) and five times for the gray-scale pattern (Figure 1H) and then counted the number of visits to each disk. We appropriately changed the disks’ position during the tests.
Training and Tests Using Monochromatic Lights
During the training phase, we covered the frosted quartz screen with a black plastic plate having a window of 1.0 × 1.0 cm2 in the center (Figure 2B) through which the butterflies saw the light of a particular wavelength. The training started on post-emergence day 3, using starved and tethered butterflies with clipped wings. We brought a tethered butterfly close to the illuminated window and fed it on 6% sucrose solution for a few seconds: the butterflies took sucrose solution using the extended proboscides. We repeated such a brief feeding until the butterfly became satiated. We trained butterflies every day until they showed proboscis extension without reward upon seeing the training monochromatic light.
For measuring the butterflies’ detection threshold for monochromatic lights, we covered the quartz screen with a black plastic plate having two 1.0 × 1.0 cm2 windows separated by a 5 mm gap (Figure 2C). To be strict that the butterflies responded not to windows but the light, we presented two windows, one illuminated with the training wavelength of varying intensity, and the other kept unilluminated. We presumed that the trained butterflies extend the proboscis toward the illuminated window only when the light intensity was above the detection threshold. We changed the position of the illuminated window randomly.
We measured the detection thresholds in the wavelength range from 340 to 680 nm at intervals of 20 or 40 nm using the trained tethered butterflies. Figure 3 shows the protocol of the behavioral experiment. We checked the butterflies’ motivation every time they did not respond to the presented stimulus: the motivation check (MC) was executed by confirming whether the maximum intensity of a given wavelength elicited proboscis extension (Figure 3A). On the first day of the test, we checked whether the butterflies could respond to the training wavelength at 4, 3, 2, or 1 log unit weaker than the training intensity (Figure 3B). On the next day and later, we started the day’s test at the lowest intensity to which the individual responded on the previous day (Figure 3C). We thus determined the threshold intensity at the sampling rate of 0.25 log unit for each individual. We plotted the average number of responding individuals against the photon flux. The photon flux required for eliciting criterion response plotted vs. wavelength yielded the action spectrum of foraging behavior.
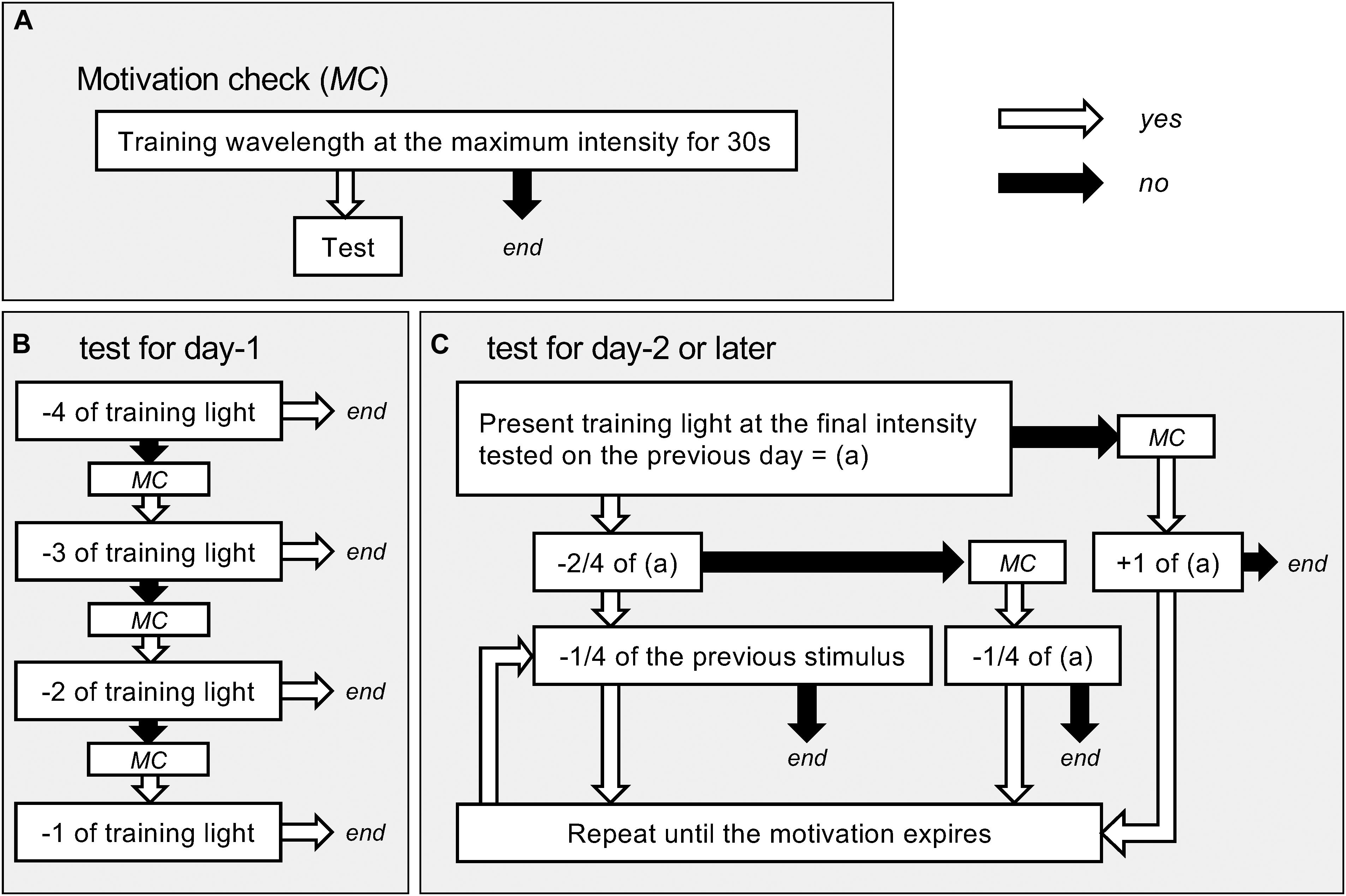
Figure 3. Flow charts of the behavioral experiments. (A) The protocol of checking motivation. The motivation check (MC) was done whenever necessary during the tests. Only when we confirmed the motivation, we continued tests. (B) Test for day-1. (C) Test for day-2 or later. Numbers with plus or minus (e.g., −4, +1/4) mean changes in light intensity in log unit (e.g., 4 log unit darker or 0.25 unit brighter; comparison with the previous test light otherwise specified).
Statistical Analysis
We used Statcel4 add-in for Excel (OMS publishers, Tokyo, Japan) for the analysis and set an alpha value of P < 0.05 as statistically significant. We applied a multinomial test to check the randomness of data distribution. We checked the statistical significance of the innate preference data using the Tukey-Kramer test. To evaluate the learning process of color X, we aggregated data of a particular day (color X vs. the other colors) and performed the Wilcoxon signed-rank test. We applied the same to evaluate the final effect of learning. Supplementary Tables S1–S3 summarize the P-values.
Results
Innate Preference
We tested nine naïve female adults. Five butterflies visited the blue disk, and four visited the yellow disk as the first feeding place in their lives, while none visited the red and green disks (Figure 4 and Supplementary Table S1). The distribution of visits was not random (Multinomial test, P < 0.05).
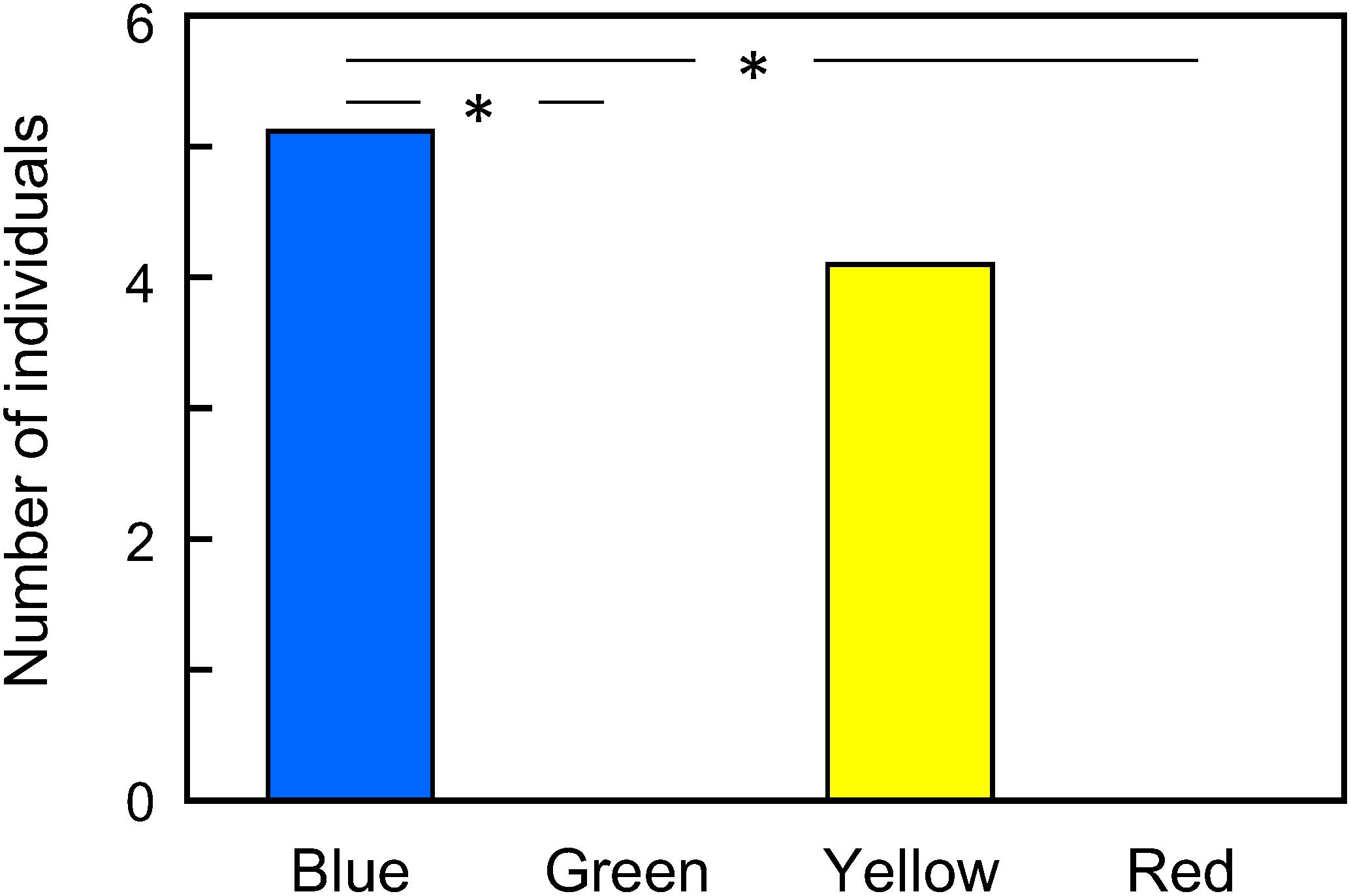
Figure 4. First color choice of naïve Pieris rapae on a 4-color pattern. The data distribution was non-random (Multinomial test, P < 0.05). ∗P < 0.05 (Tukey-Kramer test, Supplementary Table S1).
Learning Process
We collected data of butterflies that visited any disk more than five times a day for 5 days: 24 individuals met the criterion. The butterflies trained to the blue disk visited the blue disk exclusively after a single training (Figure 5A). The butterflies trained to the yellow disk exhibited a similar pattern. On the other hand, those trained to the red or green disk preferred the yellow and blue disks after the first training. Preference to the disk of trained color significantly increased day by day, reaching almost maximum on post-emergence day 7 for green-trained butterflies and day 6 for red-trained ones (P < 0.05, Wilcoxon signed-rank test, Supplementary Table S2).
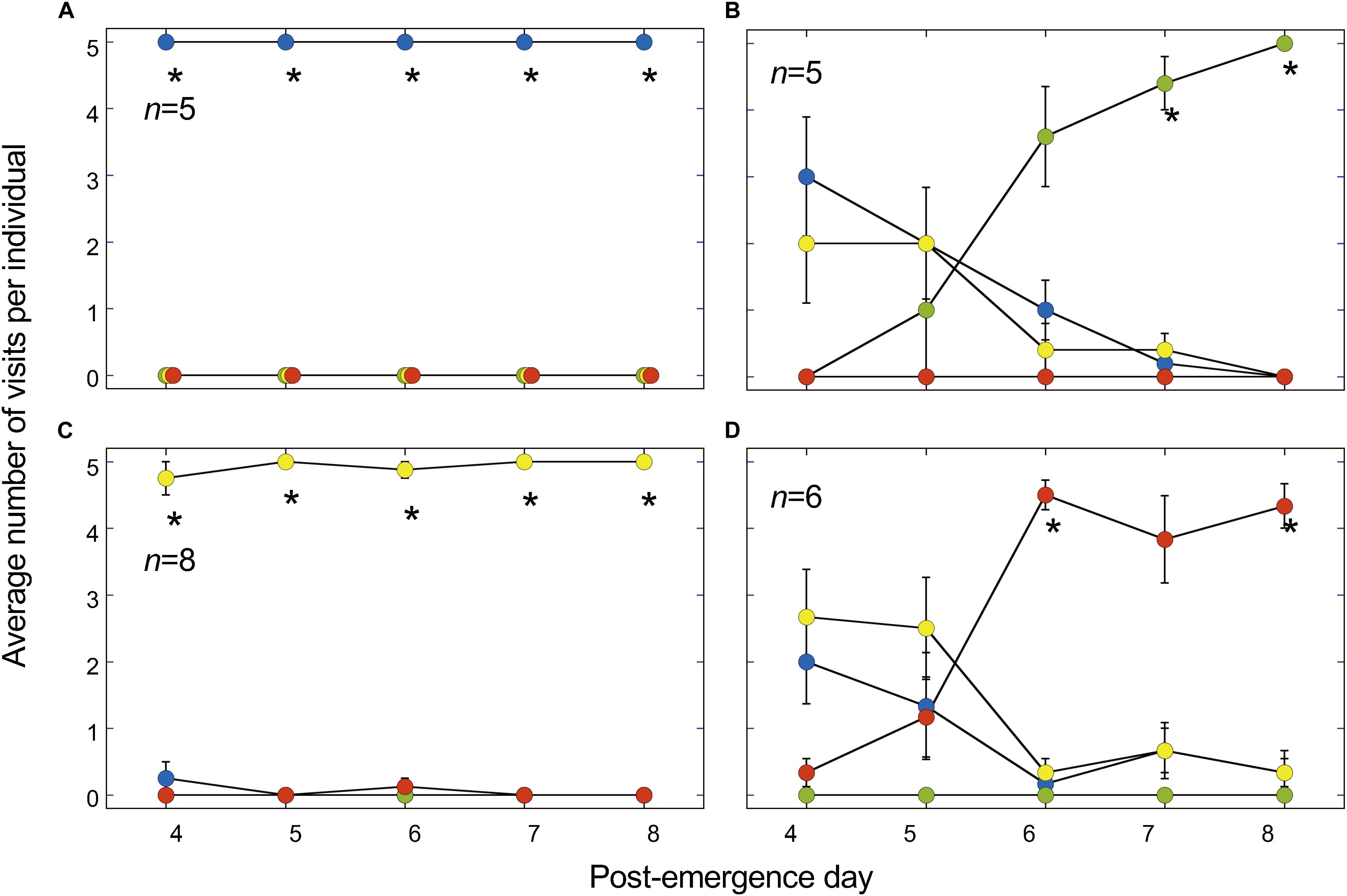
Figure 5. Visit numbers after a training period lasting 5 days on a disk of a particular color. (A) Blue-training. (B) Green-training. (C) Yellow-training. (D) Red-training. Asterisks (*) indicate that the training color was selected significantly more often than the sum of other colors. P < 0.05 (Wilcoxon signed-rank test, Supplementary Table S2). n, number of tested individuals. Values are means ± S.E.M.
Learning Results
Figure 6 shows the cumulative results of the trained butterflies tested on the 13-color pattern. The stacked bars are arranged with the training colors on the leftmost. The yellow-trained butterflies visited yellow, the training color, most frequently as expected (P < 0.05, Wilcoxon signed-rank test). However, those trained to green and blue, respectively, chose preferentially blue-green and violet, followed by the training colors. The red-trained butterflies visited orange most frequently, followed by purple and then by red. The 13-color pattern had two gray disks, gray 2 and gray 3 (Figures 1D,G), but no butterflies visited them. We also tested the trained butterflies on a gray-scale pattern together with a colored disk of respective training (Figure 1H). We found all butterflies visited the colored disk: none visited any of the gray disks.
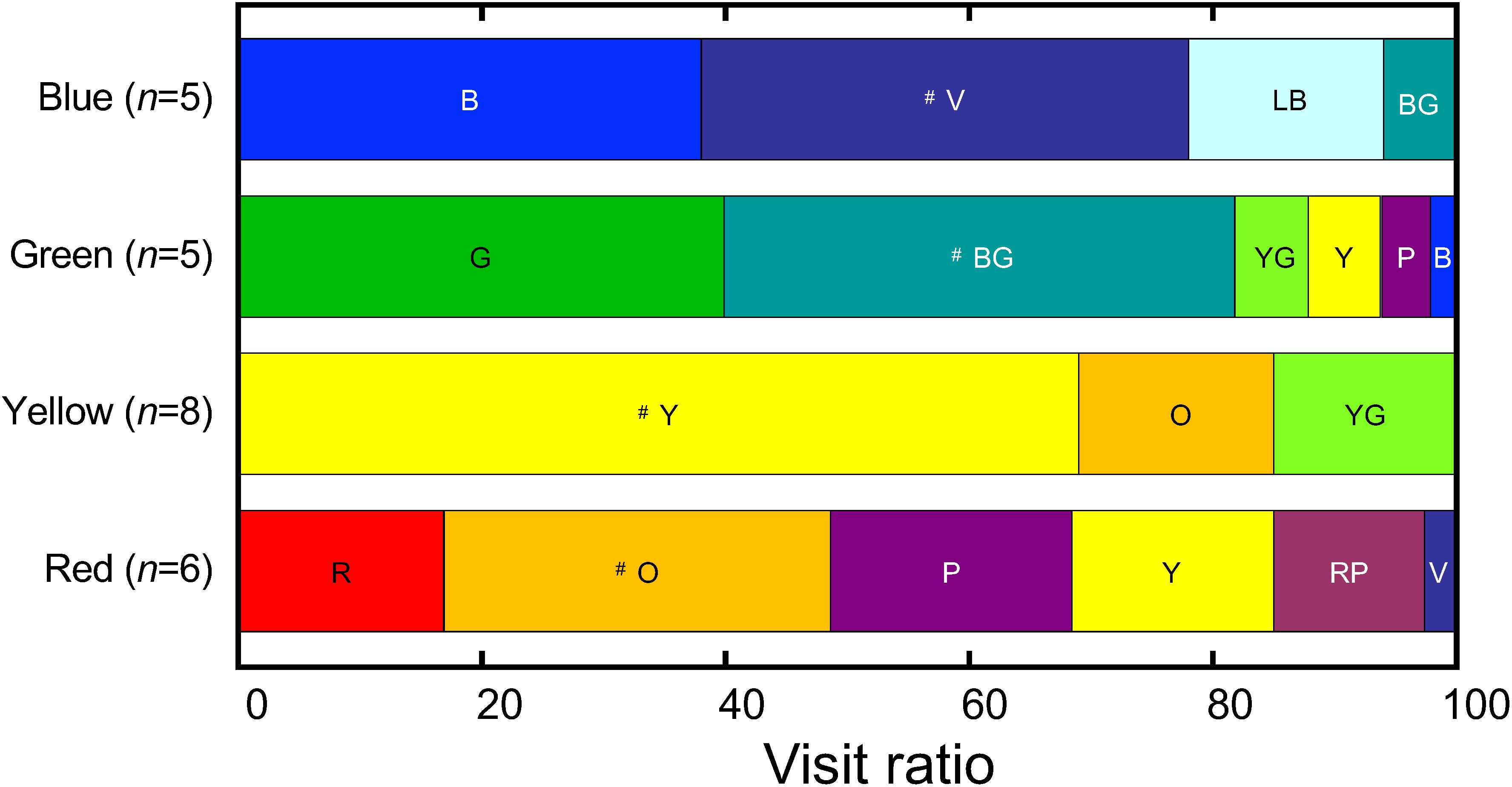
Figure 6. The visit ratio after the 5-day training, measured on day 6 using the 13-color pattern. For the reflection spectrum, the subjective brightness, and the abbreviation of each color, see Figure 1 and Table 1. The symbol # indicates the most frequently visited color in each group. No butterflies selected Gray 2 and 3. n, the number of tested individuals. For the results of statistical analysis, see Supplementary Table S3.
Action Spectrum of Proboscis Extension
We could successfully train 3–6 butterflies to 1 of the 14 test wavelengths ranging from 340 to 680 nm. Figure 7A shows the response-log intensity functions for the proboscis extension response. The solid curves are the best fits of sigmoidal function for each wavelength. Reciprocals of the photon number eliciting a criterion response give an action spectrum. As the curves’ slopes vary, we took the criteria at 20, 50, and 80% response, yielding three similar spectra, with only slight variations in the long-wavelength region (Figure 7B).
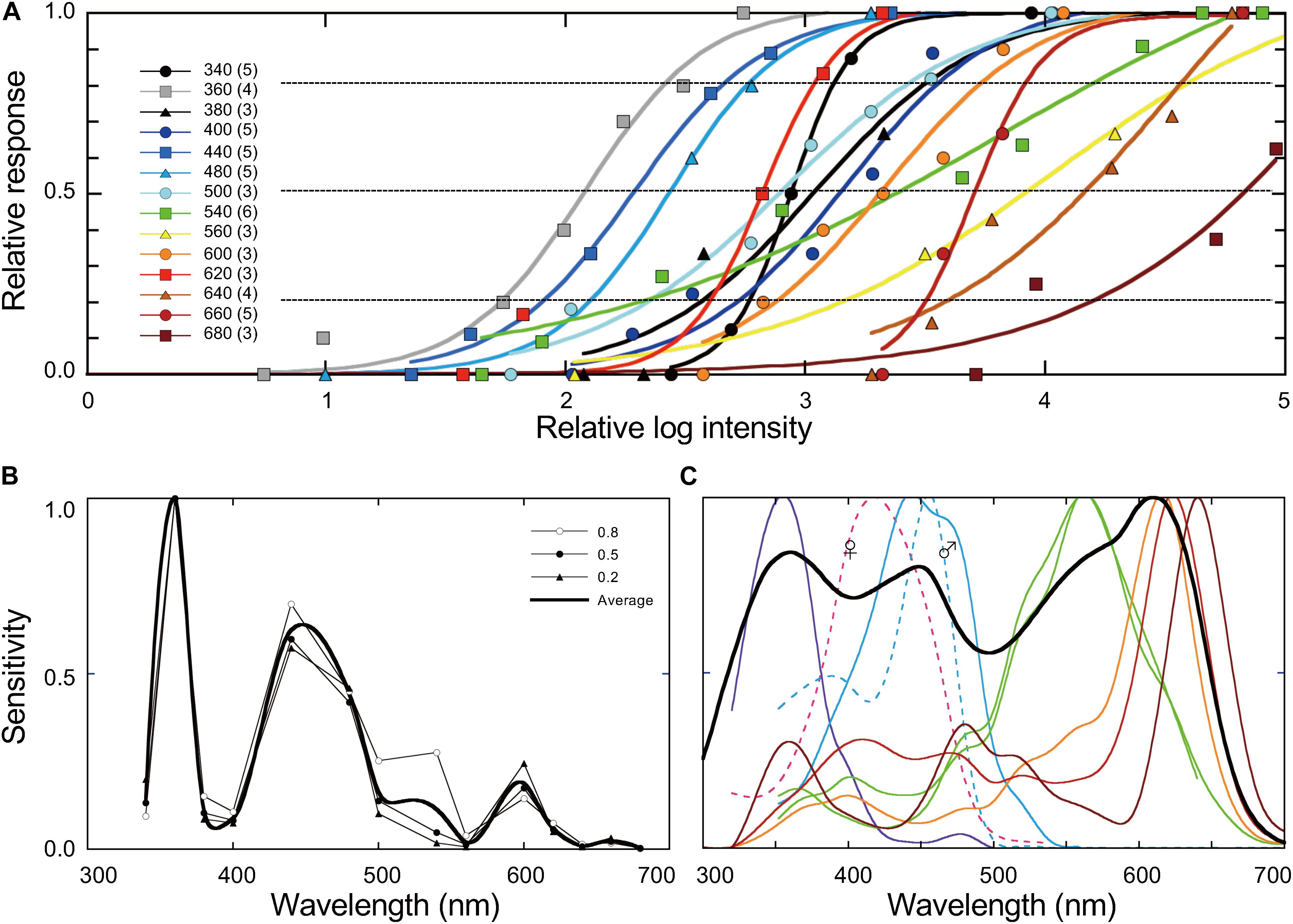
Figure 7. (A) Dependency of proboscis extension response on the intensity of monochromatic light. The inset shows the tested wavelengths with the number of individuals in parentheses. Response values of 1.0 indicate where all individuals extended their proboscis toward the stimulus light. Solid curves are the best fits of a sigmoidal function. (B) Action spectra based on A at 80, 50, and 20% as the criteria. The bold curve is the average of three spectra. (C) The photoreceptor spectral sensitivities (colored curves, spline-interpolated) and the estimated spectral sensitivity of the entire compound eye of female Pieris rapae (bold curve, the sum of the ommatidial absorptance spectra in Stavenga and Arikawa (2011), weighted by the ommatidial occupation ratio). The dashed curves show the sex-specific receptors.
Discussion
Color Vision
For a convincing demonstration of color vision in an animal, one has to demonstrate that the animal can visually discriminate “colored” stimuli, either objects or light sources, based on their spectral contents irrespective of the intensity. Here, the intensity is crucial because we need to know the intensity for the animal. The animal-subjective brightness can be obtained as the product of the physical intensity of the stimuli and the animal’s sensitivity to spectral lights.
The spectral sensitivity of compound eyes can be fairly easily measured by electroretinography (Belušič, 2011). Such a spectral sensitivity may be acceptable as the first approximation for this purpose, but in reality, the sensitivity often differs depending on the behavioral context. For example, the UV-violet, blue, and green-yellow region of the spectrum, respectively, elicits the escape, feeding, and oviposition behavior in Pieris butterflies (Scherer and Kolb, 1987; Kelber, 2001). With this in mind, we measured the intensity dependency of the proboscis extension for foraging toward monochromatic lights using trained butterflies (Figure 7A). The resulting action spectrum of proboscis extension, A(λ), exhibits higher sensitivity in the short wavelength region with the maximum at 360 nm. We used the average of three behaviorally determined action spectra (Figure 7B, bold curve) to evaluate the subjective brightness of the stimuli for Pieris rapae.
We calculated the Pieris-subjective brightness of colored paper i, Bi, under the present experimental condition by:
where I(λ) is the irradiance spectrum of illumination (Figure 1A, spectrum a), Ri (λ) is the reflectance spectrum of colored paper i (Figures 1B–D), and λ is the wavelength. Table 1 shows the relative Bi values of all colored papers normalized to the brightest gray’s value. For the colors used in the 4-color pattern, the Bi values are 0.80 (blue), 0.79 (yellow), 0.70 (red), and 0.57 (green).
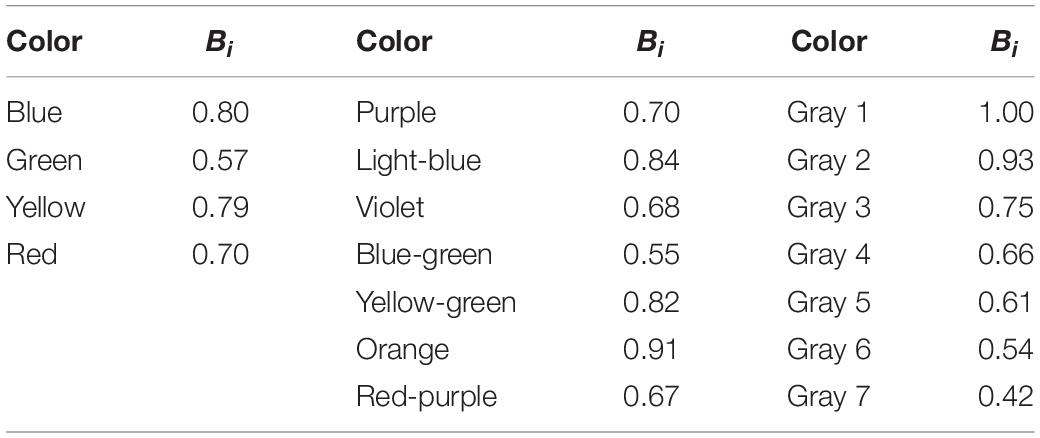
Table 1. Pieris-subjective intensity, Bi, of each colored paper under the illumination of the spectrum a in Figure 1A.
Naïve butterflies selected a blue or yellow disk as the feeding site for the first time in their lives. Butterflies tend to select brighter stimuli (Kinoshita et al., 2011), which may be related to the positive phototaxis often observed in insects (Nouvian and Galizia, 2020). For Pieris, the blue and yellow disks are equally bright, so the butterflies may have selected these disks because they appear brighter than the red and green disks and not necessarily because of an innate color preference.
The innate preference for “blue” is often reported in lepidopteran species (Kelber, 1997; Yoshida et al., 2015; Satoh et al., 2016). However, the terminology of “innate color preference” needs to be carefully re-addressed. The innate preference can be easily modified by further training. Figure 5 shows how the training on the 4-color pattern proceeded in time in the present study. Clearly, there are two distinct patterns. The selection pattern of the butterflies trained to the blue or yellow disk persisted throughout the test (Figures 5A,C): the training with the innately preferred disks was extremely effective. On the other hand, it was necessary to have two and three training sessions to learn green and red as the feeding site (Figures 5B,C): after the first training, the butterflies selected the yellow or blue disk, presumably depending on their innate preference.
Did butterflies make selections solely based on the colors in the 4-color pattern? Indeed, Papilio xuthus could learn to forage on a darker disk while they innately prefer brighter stimuli. If Pieris butterflies use brightness as the cue, they will confuse a gray disk sharing the brightness with the training disk. We considered that the confusion could occur when we presented the training disk with all grays, particularly between the following combinations: blue (subjective brightness = 0.80)/yellow (0.79) vs. gray 3 (0.75), green (0.57) vs. gray 6 (0.54), and red (0.70) vs. gray 4 (0.66) (see Table 1). However, any confusion never happened when tested on the gray pattern (Figure 1G). We thus conclude that Pieris rapae has true color vision and uses it when searching for food.
Implications of the Action Spectrum
The colored solid curves in Figure 7C are photoreceptor spectral sensitivities recorded in the compound eyes of female Pieris rapae: they are of the UV (λmax = 350 nm), violet (420 nm), blue (450 nm), green (560 nm), dual-peaked green (560 nm), orange (610 nm), red (620 nm), and deep-red (640 nm) classes (Blake et al., 2019). The dashed curves represent the spectral sensitivities of sex-specific receptors (Arikawa et al., 2005). The photoreceptors are embedded in ommatidia, each containing nine photoreceptor cells in three different combinations, making the eye a mesh of three spectrally distinct types of ommatidia. For example, a type I ommatidium has one UV, one blue, two green, and five orange receptors, each bearing a rhodopsin-containing rhabdomere to form a photoreceptive structure along the ommatidial axis.
Using the thorough anatomical and optical information about the compound eye of Pieris rapae, we previously calculated the absorptance spectrum for each ommatidial type (Stavenga and Arikawa, 2011). We also know that the ratio of type I, II, and III ommatidia is approximately 2:1:1 (Qiu et al., 2002). Here we calculated the sum of the ommatidial absorptance spectra, weighted by the ommatidial occupation ratio, as an estimation of the entire eye’s spectral sensitivity (Figure 7C).
The estimated eye sensitivity is broad with the maximum in the red wavelength range (Figure 7C), while the behavioral action spectrum exhibits some prominent peaks with the maximum at 360 nm (Figure 7B). The difference in these spectra indicates that not all photoreceptors equally contribute to the present behavioral context, i.e., the floral foraging. The contribution of the UV, violet, and blue receptors appears to be much stronger than that of the green and red receptors. Green receptors, which exist in all ommatidia (Blake et al., 2019), make a complete hexagonal lattice, presumably serving motion and shape vision. In fact, a set of green receptors feeds information to the channel for motion vision in the Papilio visual system (Stewart et al., 2015). However, it is difficult to assume that green and red receptors do not contribute to color vision at all in Pieris rapae. The receptors may contribute more to color vision in other behaviors such as searching for mates, egg-laying, or even escaping: color information processing may be context-dependent.
Animals often exhibit spontaneous reactions to the light of specific wavelengths, known as the wavelength-specific or hard-wired behaviors, which do not require learning (Menzel, 1979). The function for feeding reaction of Pieris brassicae exhibits a major sensitivity band peaking at 450 nm and another lower peak at 600 nm (see Figure 8 of Scherer and Kolb, 1987). These peaks perfectly match those of our action spectrum except for the largest peak in the UV (Figure 7B). If not a species difference, the difference must be attributed to the experimental design: while Scherer and Kolb (1987) recorded hard-wired reactions of non-trained individuals, we used trained butterflies with monochromatic lights. The trained butterflies presumably learned the UV light as a certain color connected to the food because foraging butterflies use color vision, as discussed in this study. In other words, the action spectrum demonstrates the subjective brightness as well as the detection threshold of monochromatic lights as colors. Both spectra are informative and valuable, but it is important to be aware of the difference between them when evaluating and/or using them.
Comparative Aspects
Honeybee compound eyes contain UV, B, and G receptors, which serve as the basis of their trichromatic color vision (Menzel and Backhaus, 1989). This spectral organization is shared by many bee species (Peitsch et al., 1992).
Recent studies on butterfly eyes have revealed much more diversity in this respect (van der Kooi et al., 2021). The Japanese yellow swallowtail, Papilio xuthus, has six classes (UV, violet, blue, green, red, broadband) of receptors (Arikawa, 2003), four of which serve in the UV-B-G-R tetrachromatic vision (Koshitaka et al., 2008). Pieris rapae has eight receptor classes, including three varieties of red-sensitive photoreceptors (Figure 7C). Such species-specific eye organization may create a difference in the color discrimination ability among butterfly species, which will be ecologically significant.
In the well-trained butterflies, we evaluated the color discrimination ability on the 13-color pattern. The butterflies visited the training colors and some other colors: most likely, the visited colors appeared similar to the butterflies. The confusion was most prominent in red-trained butterflies, where red was less preferred than orange and purple (Figure 6): none of the visited disks by the red-trained butterflies attracted significantly more individuals than others (Supplementary Table S3). However, we did not see such confusion in red in Papilio xuthus where the red training resulted in the most robust effect in the test using a 13-color pattern (Kinoshita et al., 1999).
These results suggest that the discrimination ability in the red range is better in Papilio xuthus than in Pieris rapae. This somehow matches with a field study, where Tanaka (1991) analyzed the color of flowers visited by 61 diurnal insect species (23 Hymenoptera, 16 Lepidoptera, 12 Coleoptera, 10 Diptera) for foraging. The field study demonstrates that the flower-visiting behavior of three species belonging to the genus Papilio, i.e., P. xuthus, P. machaon, and P. bianor, is strongly biased to red flowers. On the other hand, no other species, including Pieris rapae, exhibited such a strong red preference: Pieris rapae visited yellow flowers most frequently (Tanaka, 1991). The less frequent visits to red flowers of Pieris rapae is rather enigmatic because it has a wide variety of red receptors, with distinct spectral sensitivities, and the overall eye sensitivity is high in the red wavelength range (Figure 7C). We have assumed that the multiple red receptors of Pieris rapae may contribute to enhance contrast sensitivity and/or color discrimination in the long-wavelength spectral region (Qiu and Arikawa, 2003). However, the present study on virgin females contradicts this assumption. The results may be different in males and/or even in mated females. Multiple red receptors also exist in another pierid species, Colias erate, which does not exhibit a red preference either (Tanaka, 1991). In the case of Colias erate, the peak wavelengths of the red receptors’ spectral sensitivity are well-separated only in females (Ogawa et al., 2013). The female-specific multiple red receptors of Colias erate may therefore be adaptive for assessing the quality of clover leaves for oviposition.
Sexual dimorphism in photoreceptor spectral sensitivities appears common among butterflies (Sison-Mangus et al., 2006; McCulloch et al., 2016), including Pieris rapae. In Pieris rapae, the “violet” receptors exist in fluorescing ommatidia in males. The fluorescing material absorbs the violet light and converts the violet receptors into double-peaked blue (dB) receptors (Figure 7C). When we discovered the dimorphism in Pieris rapae crucivora, we assumed that the male-specific dB receptors would help males to locate the UV-reflecting females efficiently upon mating (Arikawa et al., 2005). However, we recently found the same sexual dimorphism in the eyes of Pieris rapae rapae, the European subspecies, where the female-specific UV reflection is absent (Blake et al., 2019). It thus raises a question of whether or not the violet receptors in females and the dB receptors in males affect the color discrimination upon floral foraging. The biological significance of sexual dimorphism in vision requires further attention in future studies.
Data Availability Statement
The raw data supporting the conclusions of this article will be made available by the authors, without undue reservation.
Author Contributions
KA, YN, and MK designed the research and wrote the manuscript. YN and HK performed the behavioral experiments and optical measurements. YN, HK, and KA performed the data analysis. All authors read, commented, and approved the final version of the manuscript.
Funding
This work was supported by the JSPS KAKENHI (18H05273 to KA and 17K07485 to MK) and the SOKENDAI Advanced Sciences Synergy Program (SASSP) to MK.
Conflict of Interest
The authors declare that the research was conducted in the absence of any commercial or financial relationships that could be construed as a potential conflict of interest.
Acknowledgments
We thank Doekele Stavenga for the critical reading of the manuscript.
Supplementary Material
The Supplementary Material for this article can be found online at: https://www.frontiersin.org/articles/10.3389/fevo.2021.650069/full#supplementary-material
References
Arikawa, K. (2003). Spectral organization of the eye of a butterfly. Papilio. J. Comp. Physiol. A 189, 791–800. doi: 10.1007/s00359-003-0454-7
Arikawa, K., Wakakuwa, M., Qiu, X., Kurasawa, M., and Stavenga, D. G. (2005). Sexual dimorphism of short-wavelength photoreceptors in the small white butterfly, Pieris rapae crucivora. J. Neurosci. 25, 5935–5942. doi: 10.1523/JNEUROSCI.1364-05.2005
Blake, A. J., Hahn, G. S., Grey, H., Kwok, S. A., McIntosh, D., and Gries, G. (2020). Polarized light sensitivity in Pieris rapae is dependent on both color and intensity. J. Exp. Biol. 223(Pt 13):jeb220350. doi: 10.1242/jeb.220350
Blake, A. J., Pirih, P., Qiu, X., Arikawa, K., and Gries, G. (2019). Compound eyes of the small white butterfly Pieris rapae have three distinct classes of red photoreceptors. J. Comp. Physiol. A 205, 553–565. doi: 10.1007/s00359-019-01330-8
Chittka, L., and Thomson, J. D. (2001). Cognitive ecology of Pollination: Animal Behavior and Floral Evolution. Cambridge: Cambridge University Press.
Goulson, D., and Cory, J. S. (1993). Flower constancy and learning in foraging preferences of the green-veined white butterfly Pieris napi. Ecol. Entomol. 18, 315–320. doi: 10.1111/j.1365-2311.1993.tb01107.x
Kandori, I., and Ohsaki, N. (1996). The learning abilities of the white cabbage butterfly, Pieris rapae, foraging for flowers. Res. Popul. Ecol. 38, 111–117. doi: 10.1007/bf02514977
Kelber, A. (1997). Innate preferences for flower features in the hawkmoth Macroglossum stellatarum. J. Exp. Biol. 200, 827–836.
Kelber, A. (2001). Receptor based models for spontaneous colour choices in flies and butterflies. Entomol. Exp. Appl. 99, 231–244. doi: 10.1046/j.1570-7458.2001.00822.x
Kelber, A., and Pfaff, M. (1999). True colour vision in the orchard butterfly, Papilio aegeus. Naturwissenschaften 86, 221–224. doi: 10.1007/s001140050601
Kinoshita, M., Shimada, N., and Arikawa, K. (1999). Colour vision of the foraging swallowtail butterfly Papilio xuthus. J. Exp. Biol. 202, 95–102.
Kinoshita, M., Yamazato, K., and Arikawa, K. (2011). Polarization-based brightness discrimination in the foraging butterfly, Papilio xuthus. Phil. Trans. R. Soc. B 366, 688–696. doi: 10.1098/rstb.2010.0200
Kolb, G., and Scherer, C. (1982). Experiments on wavelength specific behavior of Pieris brassicae L. during drumming and egg-laying. J. Comp. Physiol. A 149, 325–332. doi: 10.1007/bf00619148
Koshitaka, H., Kinoshita, M., Vorobyev, M., and Arikawa, K. (2008). Tetrachromacy in a butterfly that has eight varieties of spectral receptors. Proc. Biol. Sci. 275, 947–954. doi: 10.1098/rspb.2007.1614
McCulloch, K. J., Osorio, D., and Briscoe, A. D. (2016). Sexual dimorphism in the compound eye of Heliconius erato: a nymphalid butterfly with at least five spectral classes of photoreceptor. J. Exp. Biol. 219, 2377–2387. doi: 10.1242/jeb.136523
Menzel, R. (1979). “Spectral sensitivity and color vision in invertebrates,” in Invertebrate Photoreceptors, ed. H. Autrum (Berlin: Springer-Verlag), 503–580. doi: 10.1007/978-3-642-66999-6_9
Menzel, R., and Backhaus, W. (1989). “Color vision in honey bees: phenomena and physiological mechanisms,” in Facets of Vision, eds D. G. Stavenga and R. C. Hardie (Berlin: Springer-Verlag), 281–297. doi: 10.1007/978-3-642-74082-4_14
Nouvian, M., and Galizia, C. G. (2020). Complexity and plasticity in honey bee phototactic behaviour. Sci. Rep. 10:7872. doi: 10.1038/s41598-020-64782-y
Obara, Y., and Hidaka, T. (1968). Recognition of the female by the male, on the basis of ultra-violet reflection, in the white cabbage butterfly, Pieris rapae crucivora Boisduval. Proc. Jap. Acad. 44, 829–832. doi: 10.2183/pjab1945.44.829
Obara, Y., and Majerus, M. E. N. (2000). Initial mate recognition in the British cabbage butterfly, Pieris rapae rapae. Zool. Sci. 17, 725–730. doi: 10.2108/zsj.17.725
Ogawa, Y., Kinoshita, M., Stavenga, D. G., and Arikawa, K. (2013). Sex-specific retinal pigmentation results in sexually dimorphic long-wavelength-sensitive photoreceptors in the Eastern Pale Clouded Yellow butterfly, Colias erate. J. Exp. Biol. 216, 1916–1923. doi: 10.1242/jeb.083485
Peitsch, D., Fietz, A., Hertel, H., Desouza, J., Ventura, D. F., and Menzel, R. (1992). The spectral input systems of hymenopteran insects and their receptor-based colour vision. J. Comp. Physiol. A 170, 23–40. doi: 10.1007/bf00190398
Qiu, X., and Arikawa, K. (2003). Polymorphism of red receptors: Sensitivity spectra of proximal photoreceptors in the small white butterfly, Pieris rapae crucivora. J. Exp. Biol. 206, 2787–2793. doi: 10.1242/jeb.00493
Qiu, X., Vanhoutte, K. A., Stavenga, D. G., and Arikawa, K. (2002). Ommatidial heterogeneity in the compound eye of the male small white butterfly, Pieris rapae crucivora. Cell Tissue Res. 307, 371–379. doi: 10.1007/s00441-002-0517-z
Satoh, A., Kinoshita, M., and Arikawa, K. (2016). Innate preference and learning of colour in the male cotton bollworm Helicoverpa armigera. J. Exp. Biol. 219, 3857–3860. doi: 10.1242/jeb.148064
Scherer, C., and Kolb, G. (1987). Behavioral experiments on the visual processing of color stimuli in Pieris brassicae L. (Lepidoptera). J. Comp. Physiol. A 160, 645–656. doi: 10.1007/bf00611937
Sison-Mangus, M. P., Bernard, G. D., Lampel, J., and Briscoe, A. D. (2006). Beauty in the eye of the beholder: the two blue opsins of lycaenid butterflies and the opsin gene-driven evolution of sexually dimorphic eyes. J. Exp. Biol. 209, 3079–3090. doi: 10.1242/jeb.02360
Stavenga, D. G., and Arikawa, K. (2011). Photoreceptor spectral sensitivities of the Small White butterfly Pieris rapae crucivora interpreted with optical modeling. J. Comp. Physiol. A 197, 373–385. doi: 10.1007/s00359-010-0622-5
Stewart, F. J., Kinoshita, M., and Arikawa, K. (2015). The butterfly Papilio xuthus detects visual motion using chromatic contrast. Biol. Lett. 11:20150687. doi: 10.1098/rsbl.2015.0687
Traynier, R. M. M. (1984). Associative learning in the ovipositional behaviour of the cabbage butterfly, Pieris rapae. Physiol. Entomol. 9, 465–472. doi: 10.1111/j.1365-3032.1984.tb00789.x
Turner, C. H. (1910). Experiments on color-vision of the honey bee. Biol. Bull. 19, 257–279. doi: 10.2307/1536088
van der Kooi, C. J., Stavenga, D. G., Arikawa, K., Belušič, G., and Kelber, A. (2021). Evolution of insect color vision: from spectral sensitivity to visual ecology. Ann. Rev. Entomol. 66, 435–461. doi: 10.1146/annurev-ento-061720-071644
Wakakuwa, M., Kurasawa, M., Giurfa, M., and Arikawa, K. (2005). Spectral heterogeneity of honeybee ommatidia. Naturwissenschaften 92, 464–467. doi: 10.1007/s00114-005-0018-5
Wakakuwa, M., Stavenga, D. G., Kurasawa, M., and Arikawa, K. (2004). A unique visual pigment expressed in green, red and deep-red receptors in the eye of the small white butterfly, Pieris rapae crucivora. J. Exp. Biol. 207, 2803–2810. doi: 10.1242/jeb.01078
Keywords: insect, photoreceptor, spectral sensitivity, action spectrum, chromaticity, subjective brightness
Citation: Arikawa K, Nakatani Y, Koshitaka H and Kinoshita M (2021) Foraging Small White Butterflies, Pieris rapae, Search Flowers Using Color Vision. Front. Ecol. Evol. 9:650069. doi: 10.3389/fevo.2021.650069
Received: 06 January 2021; Accepted: 04 March 2021;
Published: 22 March 2021.
Edited by:
Johannes Spaethe, Julius Maximilian University of Würzburg, GermanyReviewed by:
Adam James Blake, Simon Fraser University, CanadaBalamurali G. S., Indian Institute of Science Education and Research, Thiruvananthapuram, India
Copyright © 2021 Arikawa, Nakatani, Koshitaka and Kinoshita. This is an open-access article distributed under the terms of the Creative Commons Attribution License (CC BY). The use, distribution or reproduction in other forums is permitted, provided the original author(s) and the copyright owner(s) are credited and that the original publication in this journal is cited, in accordance with accepted academic practice. No use, distribution or reproduction is permitted which does not comply with these terms.
*Correspondence: Kentaro Arikawa, YXJpa2F3YUBzb2tlbi5hYy5qcA==