- 1Department of Neurobiology and Behavior, Cornell University, Ithaca, NY, United States
- 2Department of Ecology, Evolution, and Organismal Biology, Brown University, Providence, RI, United States
Understanding how and why behavioral traits diversify during the course of evolution is a longstanding goal of organismal biologists. Historically, this topic is examined from an ecological perspective, where behavioral evolution is thought to occur in response to selection pressures that arise through different social and environmental factors. Yet organismal physiology and biomechanics also play a role in this process by defining the types of behavioral traits that are more or less likely to arise. Our paper explores the interplay between ecological, physiological, and mechanical factors that shape the evolution of an elaborate display in woodpeckers called the drum. Individuals produce this behavior by rapidly hammering their bill on trees in their habitat, and it serves as an aggressive signal during territorial encounters. We describe how different components of the display—namely, speed (bill strikes/beats sec–1), length (total number of beats), and rhythm—differentially evolve likely in response to sexual selection by male-male competition, whereas other components of the display appear more evolutionarily static, possibly due to morphological or physiological constraints. We synthesize research related to principles of avian muscle physiology and ecology to guide inferences about the biomechanical basis of woodpecker drumming. Our aim is to introduce the woodpecker as an ideal study system to study the physiological basis of behavioral evolution and how it relates to selection born through different ecological factors.
Introduction
Understanding how behavioral traits evolve is a longstanding goal of organismal biology. Indeed, most research within the field of behavioral ecology that addresses this objective explores the ecological factors that influence changes to a species’ behavioral program over time (Westneat and Fox, 2010). Such work has resulted in an extremely rich knowledge of environmental factors that create selection pressures, which in turn modify the way that individuals interact with their social and physical surroundings to better survive and reproduce. Yet, at the same time, we must remember that behavior itself is often a manifestation of complex neurobiological and physiological processes. In these cases, complex behaviors occur through concomitant changes to the nervous and/or musculoskeletal systems that determine how individuals express behavior (Bauwens et al., 1995; Clifton et al., 2015; Ding et al., 2016; Fuxjager et al., 2016; Barkan et al., 2018). Our understanding of behavioral evolution from this mechanistic standpoint is murkier than it is from the ecological standpoint—yet, both perspectives are necessary to fully uncover the complex processes by which behavioral changes can (or cannot) occur.
Here, we explore the evolutionary interplay among behavioral evolution and organismal ecology and physiology. We do this by focusing on the evolution of woodpecker “beak behavior,” or the actions of drilling for foraging and nest creation as well as drumming for social signaling. We start by discussing the woodpecker clade and its diversity. We then review how these birds use their bill for important naturally selected and sexually selected behavioral traits. A deeper exploration of the evolution of drumming displays (a territorial signal that is produced by rapidly hitting their bill against a resonant substrate) allows us to assess how selection promotes behavioral diversity, particularly in the face of morphological constraint. We then move the discussion to the physiological and biomechanical basis of woodpecker drilling and drumming. We do this by reviewing the relatively limited literature on the topic and then developing a model for how drumming may be controlled. Our aim is to begin to merge our understanding of the ecological factors associated with the diversification of “beak behavior” and the physiological and mechanical factors that shape this behavior.
Woodpeckers: A Family-Wide Model for Studies of Integrative Evolution
Woodpeckers are an intriguing group of birds. They are contained within the clade Coraciimorphae, which includes a large assemblage of cavity nesting species such as trogons (order: Trogoniformes), hornbills and hoopoes (order: Bucerotiformes), and rollers and kingfishers (order: Coraciiformes) (Jarvis et al., 2014). Woodpeckers themselves are part of the family Picidae, which together with puffbirds (family: Bucconidae), Jacamars (family: Galbulidae), and a variety of toucan and barbets (infraorder: Ramphastides), form the order Piciformes (Jarvis et al., 2014).
Woodpeckers are also highly diverse. For example, they occupy nearly all terrestrial habitats across the globe (except for Australasia and Antarctica). This includes rich temperate and tropical forests, arid plains and savannas, swamps and marshes, and deserts (Bent, 1939; de Kiriline Lawrence, 1967; Short, 1970, 1971). Likewise, woodpecker behavior is equally variable. Some taxa adopt cooperative lifestyles, in which large family groups live together to feed and shelter (Koenig, 1981; Lennartz et al., 1987), whereas other species adopt isolated, nomadic lifestyles (Collins, 2017b; Nickley and Bulluck, 2020). Woodpeckers have also evolved an array of diets and foraging tactics; some species feed generally, while others forage on highly specialized items, such as sap or ants (Spring, 1965; Tate, 1973; Leite et al., 2013). From a morphological perspective, woodpecker body sizes span a wide range. For example, certain piculets are roughly 10 g in mass and no more than 10 cm in body length, whereas Dryocopus woodpeckers weigh around 300 g and are almost 50 cm in body length (Koenig, 1996; Miles et al., 2018). Plumage characteristics of woodpeckers are equally diverse—many species exhibit their own unique color ornaments, which often consist of bright red and yellow on the head (Stradi et al., 1998; Wiebe and Vitousek, 2015; Lammertink et al., 2016; Miller et al., 2019). Some species even have brilliant crests that exaggerate the aesthetic of their head.
Phenotypic differences are not the only reason why woodpeckers are a compelling family for studies of evolution. Similarities among these birds provide an opportunity to explore how different forces of constraint and/or preservation of adaptive traits influence phenotypic evolution in species that have otherwise undergone significant diversification over time. For most woodpeckers, the primary “phenotypic tie” that binds the clade together is their uncanny propensity to use their bill as a hammer or drill on wood in their environment. Here, we refer to this as “beak behavior,” and we roughly categorize it into one of three different functional groups: (i) foraging, (ii) nest building, and (iii) displaying (Figure 1). We hypothesize that this behavior is built from a conserved physiological scaffolding that supports the extensive use of the head and body as a hammer. Presumably, features of this scaffolding arose deep within the Coraciimorphae lineage, where nest excavation first emerged. Yet something must have happened in the woodpecker’s history to modify the mechanisms of pecking, which allows the species to use this behavior more broadly in contexts of feeding, reproduction, and communication (Figure 1). A major goal of our research is to elucidate the physiological scaffolding that underlies “beak behavior,” and assess how it influences the trajectory of phenotypic evolution.
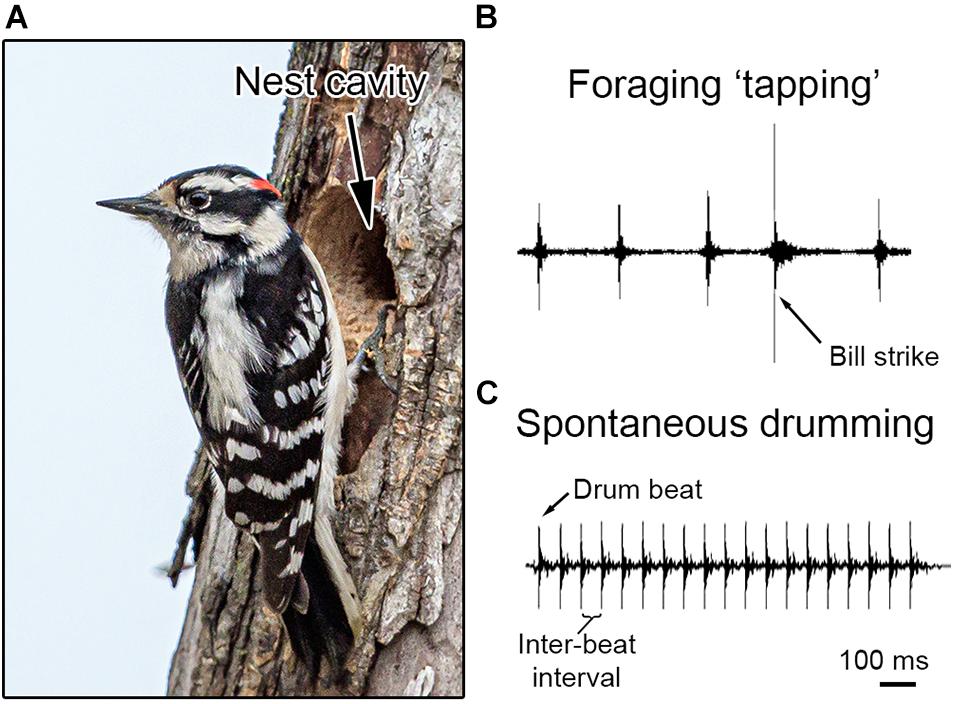
Figure 1. Bill behaviors in the downy woodpecker (Dyrobates pubescens). (A) During the breeding season, downy woodpeckers excavate nest cavities within trees using their bill. These nest cavities serve as a place to raise offspring during the breeding season. Photo credit: Shiva Sheney (CC BY 2.0, no changes or modification were made to the photograph). (B) Throughout the year, woodpeckers will also use their bill to forage on trees for food, including probing for insects (see main text). (C) Most woodpeckers communicate by hammering their bill repeatedly against a tree, which produces an acoustic signal used in courtship and competition. This loud atonal signal is called a “drum.” Each time the bill makes contact with the tree it produces a “drum beat,” with the timing between two beats called the inter-beat interval. Both recordings are at the same temporal scale for comparison, with a scale bar at the bottom right.
Woodpecker “Beak Behavior”: Nest Excavation and Foraging
As discussed above, nest excavation is likely the ancestral form of “beak behavior” in woodpeckers. Woodpeckers and other primary cavity nesting species have the incredible task of excavating a portion of a tree that will serve as a safe place for nesting and egg laying during the breeding season and for roosting year-round (Figure 1A) (Kilham, 1958; Short, 1979; Rudolph et al., 1990). Acquiring and defending territories that have multiple sites suitable for these uses is integral to the bird’s survival and reproductive success. Most of the Picids construct nest cavities each breeding season, with the trees that particular species choose for cavity construction ranging from already-rotting specimens in wetlands to fully mature live trees in densely forested areas (Short, 1979). In either case, nest construction often requires woodpeckers to expend a significant amount of time to use their bill as a drill, chipping away bits of wood to create a relatively large hole in which the bird and its clutch can fit. This process often lasts multiple weeks, but in the end the bird creates a site that provides suitable protection for future offspring (Kilham, 1958; Short, 1979). Although the strenuous physicality of this task requires numerous physiological and morphological adaptations, recent work suggests that nest excavation into multiple layers of dense wood is sometimes facilitated by fungi that soften the wood (Farris et al., 2004; Jusino et al., 2016). Indeed, red-cockaded woodpeckers (Dryobates borealis) transmit fungal spores that hasten the wood-decay of pine trees from their beak into the fresh live wood, as individuals build their nest (Jusino et al., 2016). One might consider this an unusual form of tool use on the part of the woodpecker, which may be adaptive considering that it speeds up the nest excavation processes and likely buffers the sheer physical challenge associated with this part of the reproductive process.
Woodpeckers also forage using different styles of bill-hammering. Some species chip away or excavate tree bark to extract food items from these sites, whereas other birds look for food in holes that are already present in a tree or shrub (Short, 1971; Conner, 1981; Askins, 1983). The latter behavior is probably the more iconic mode of woodpecker feeding, as it is often performed vigorously. In such cases, birds scrape and chip away large pieces of bark to gain access to insect larvae hiding underneath, creating an aesthetic of a jackhammer drilling on a substrate (Askins, 1983). Hairy woodpeckers (Leuconotopicus villosus) and downy woodpeckers (Picoides pubescens), two common North American species, are well known for exhibiting such behavior in parks, greenways, and forests. As downy woodpeckers forage, for example, they often slow pecking motions (Figure 1B) on bark to excavate small insects (Lima, 1983, 1984; Peters and Grubb, 1983). At the same time, other species use their bill to make caches in which they store food items. Acorn woodpeckers (Melanerpes formicivorus) create incredible acorn caches, whereby entire trees are littered with holes that can be used to keep acorns (Koenig et al., 2008).
Tropical woodpeckers differ slightly in their foraging behavior than temperate ones. For example, feeding via excavation is rarer, and tropic woodpeckers are even less likely to cache food for later (Askins, 1983). Instead, woodpeckers of the tropics often raid arboreal ant nests and termite mounds, pecking through the dense structures these invertebrates have constructed to shield themselves from predators (Askins, 1983). Other species, like the Kaempfer’s Woodpecker (Celeus obrieni), drill through the internodes of bamboo stems and feed on the ants that shelter inside (Leite et al., 2013). Although these strategies are quite different from their temperate relatives, it is abundantly clear that tropic woodpeckers still leverage bill-hammering to get access to food.
Woodpecker Drumming
Woodpeckers also use “beak behavior” for social communication. The most common example is the drum, or the loud staccato sound that penetrates the environment when an individual rapidly hammers its bill against a tree (Figure 1C). Ornithologists have long known that drumming is a territorial signal produced by resident birds as they settle and defend their territory during the breeding season. Early documentation of drumming comes from researchers like Brewster (1876), who described the drums of yellow-bellied sapsuckers (Sphyrapicus varius) and noted that they were often produced when an individual’s breeding territory was being invaded by another (Brewster, 1876). This phenomenon-increased drumming behavior during territorial interactions—has been noted many times since, often in North American woodpeckers (Bent, 1939; Kilham, 1959, 1960, 1969, 1974, 1977; de Kiriline Lawrence, 1967; Ligon, 1970; Winkler and Short, 1978).
One of the interesting aspects of drumming as a social signal is that it may function over both short and long spatial scales. During agonistic encounters, for example, individuals will perform drums when opponents invade their territories. At the same time, residents often broadcast drums at specific times in the day (e.g., dawn) (Kilham, 1958, 1974; de Kiriline Lawrence, 1967), much like a resident songbird sings at the morning’s first light to broadly advertise to neighbors that they still occupy the area (Burt and Vehrencamp, 2005).
Several studies used field experiments to better understand the function of drumming behavior. This work often employs simulated territorial intrusions, or STIs, in populations of free-living birds. The idea is to test how residents respond to encounters in which they hear a drum display on their territory, as though it is being invaded by an interloper. STI methodology is compellingly simple: an experimenter broadcasts a putative aggressive signal (in this case a drum) in a resident’s territory and then observes the resident’s behavioral response (Searcy et al., 2006). Studies across multiple woodpecker species demonstrate that playback of drums reliably elicits aggressive behavior from resident individuals, including both males and females (Winkler and Short, 1978; Dodenhoff et al., 2001; Schuppe et al., 2016; Figarski, 2017; Schuppe and Fuxjager, 2018). For example, when downy woodpeckers are presented with drumming playback, both sexes engage in a wide range of behaviors to defend their territories, including calls and attack flights, as well as drums (Schuppe et al., 2016; Schuppe and Fuxjager, 2018). Additional work in red-bellied woodpeckers demonstrates a significant level of dynamism in these responses (Miles and Fuxjager, 2019). For instance, males that encounter an unfamiliar intruder (via STI) on their territory for the first time begin their agonistic defensive routines largely through flight displays, and not drums. However, if resident males experience additional territorial intrusions on subsequent days, they flip the order in which they produce these behaviors (they start encounters off with drums, and then segue into flights). This latter context also results in the production of significantly more drumming across the board. Interestingly, these territorial strategies change if a resident male’s female partner (who does not partake in territorial defense) is present during the STI—in such cases, males dramatically reduce the number of drums they produce, no matter how many STIs they have accrued. Instead, they rely mostly on aggressive calling behavior and attack flights.
Altogether, these observational and experimental studies imply that drumming is an aggressive signal. In fact, drumming meets well-established criteria that distinguish agonistic display behavior: (i) drum production increases in aggressive contexts, (ii) receivers respond to drums by also producing this behavior, and (iii) drum signals predict robust aggressive responses from territorial residents (Searcy and Beecher, 2009). It is also important to recognize, however, that drumming may have other functions related to social signaling. For instance, some authors propose that drumming mediates elements of mate choice (Kilham, 1974, 1979). Tests of this idea, or any other that explores the functional significance of drumming outside the context of territoriality, are rarely (if ever) addressed in a rigorous experimental fashion.
Drum Displays: Speed, Length, and Rhythm
Establishing a connection between drumming and territoriality is only the first step toward understanding how this display works. Like many studies in animal communication, one can attempt to “decode” drumming by figuring out the way that the signal’s components underlie its functionality. Drumming is ideal for such work, because the display’s acoustics are relatively simple—each beat is an atonal burst of broadband sound, much like a handclap (Figures 1C, 2). As such, there are only a handful of ways to regulate this display. Individuals, for example, might modify the drum’s speed (number of beats produced over time) or length (total number of beats) (Figure 2). Likewise, individuals can adjust elements of the signal’s rhythm by altering elements of cadence (description of how speed changes over time) or acceleration (description of the direction of speed change over time) (see Figure 2; Miles et al., 2018, 2020; Schuppe and Fuxjager, 2018). Of course, individuals may also modify amplitude (volume) or dominant frequencies within the broadband spectra that define beat acoustics.
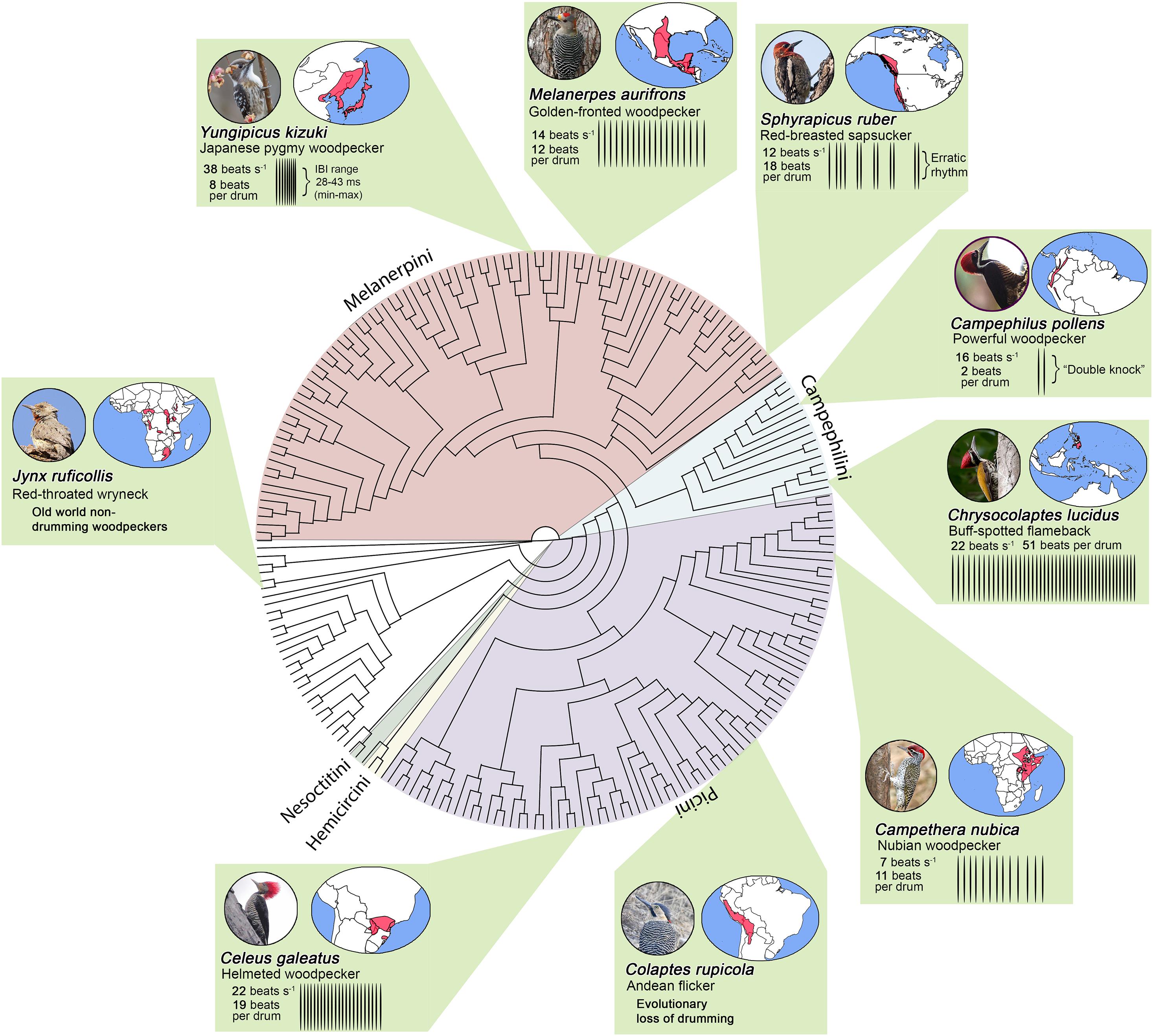
Figure 2. Cladogram of the woodpeckers (Picidae) from Shakya et al. (2017). Colors within the phylogenetic tree illustrate the five main woodpecker tribes and non-drumming old world woodpeckers (e.g., wrynecks). Green boxes illustrate how drums vary across the woodpecker phylogeny. Woodpecker drums differ in terms of speed (beats/sec), length (total number of beats), and rhythm (Miles et al., 2018, 2020). The Japanese pygmy woodpecker (Yungipicus kizuki) exhibits the fastest drums. This species is able to strike its bill at rates that can exceed 38 beats s–1 (or a strike every 28–43 ms). The buff-spotted woodpecker (Chrysocolaptes lucidus), a species found throughout eastern Asia, exhibits one of the longest drums (∼51 beats per drum). Some birds also exhibit drums with a-typical patterns. For instance, sapsuckers exhibit erratic drum rhythms, and the Powerful woodpecker produces “double-knocks” rather than the longer drums seen in most woodpeckers. Boxes also illustrate that woodpeckers occupy diverse habitats throughout the world. Photo credits: Japanese pygmy woodpecker (Ik T, CC BY 2.0); golden-fronted woodpecker (Roberto González, CC BY 2.0); red-breasted sapsucker (Beck Matsubara, CC BY 2.0); powerful woodpecker (Alan Harper, CC BY 2.0); buff-spotted flameback (Tareq Ahmed, CC BY 2.0); nubian woodpecker (Brad Schram, CC BY 2.0); Andean flicker (Vil Sandi, CC BY 2.0); helmeted woodpecker (Hector Bottai, CC BY 3.0); and red-throated wryneck (Derek Keats, CC BY 2.0). No photographs have been altered.
Recent experimental work focuses on the effects of drum speed, length, and rhythm during territorial contests. Hints that these variables are functionally important arise through research showing notable variation in parameters of speed and length within populations and among species (Stark et al., 1998; Schuppe et al., 2016; Miles et al., 2018; Schuppe and Fuxjager, 2018). For example, studies in red-bellied woodpeckers show that individuals actively engaging in territorial interactions exhibit drums that are longer than drums produced spontaneously in the morning (Wilkins and Ritchison, 1999). This finding provides further support for the notion that individuals adjust how they drum to regulate the effectiveness or potency of the signal in a context-dependent manner.
Other research looks specifically at the role of drum speed in territorial competition. In downy woodpeckers, for example, the average drum speed is about 16 beats s–1, with 23 beats s–1 as the absolutely fastest drum we have ever recorded (Schuppe and Fuxjager, 2018). If resident birds are presented (via STI) with engineered drums in which the time interval between subsequent beats is decreased by 8 ms, they become significantly more aggressive and produce more agonistic behavior in response (see Figure 3A; Schuppe and Fuxjager, 2018). This high performance “rapid drum” falls within the natural distribution of downy woodpecker drum speeds, which means that resident birds are responding to a display that they might normally encounter. It is therefore thought that residents increase their aggressive response to this display because they perceive it as a more potent threat from an intruder. Consistent with this notion is additional work showing that resident birds presented with the “rapid drum” stimulus attempt to match its speed by producing drums with inter-beat intervals that are roughly 4 ms faster than residents presented with control drums (slower, low performance drums; Figure 3B). If this finding did in fact reflect a resident’s attempt to match the social threat with that of an intruder, it is notable that the resident falls short of fully doing so (Figure 3C). This is likely because drum speed may be bound by an upper physiological limit (more on this topic below). Regardless, these data support the idea that speed is a critical component of drum effectiveness during aggressive disputes.
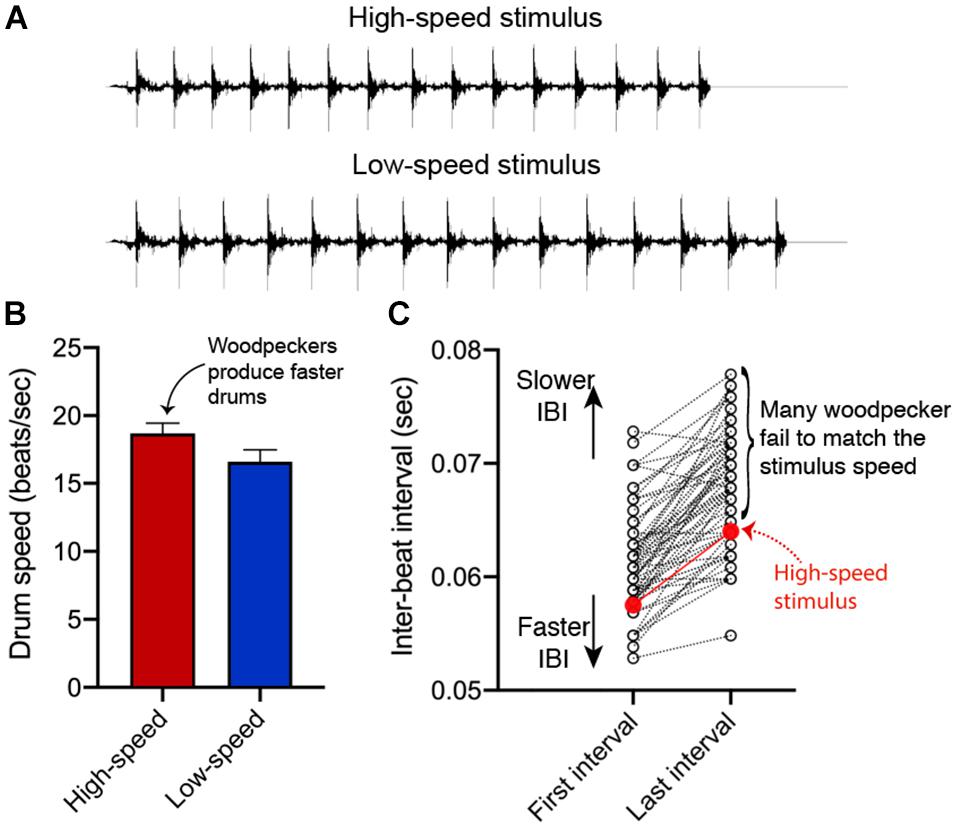
Figure 3. Residents modulate the speed of their own drum displays to resemble that of the high-speed stimulus. (A) Representative waveform diagrams of high-speed and low-speed drum stimuli that were used during stimulated territorial intrusions (STIs). (B) In response to STIs with the high-speed stimulus, territorial residents produce significantly faster drums compared to those that heard the low-speed drum. Bars represent mean ± standard error (C). Yet, many residents were not able to produce drums that were as fast as the high-speed drum stimulus. Residents on average produced drums that were approximately 4 ms slower than the actual high-speed stimulus. All data are reanalyzed from Schuppe and Fuxjager (2018).
Similar types of studies have also looked at drum length. For example, downy woodpeckers produce drums that include roughly 16 beats. When residents are subjected to STIs that broadcast longer drums with 19 beats, they again become more aggressive and produce more agonistic behavior (Schuppe et al., 2016). This study did not assess whether residents attempt to match drum length with that of the intruder; however, this work showed that resident males and females appear to coordinate their aggressive response to intruders when they hear engineered “long drums.” Again, these findings suggest that residents perceive longer drums as more threatening agonistic signals, and thus adjust their territorial response accordingly.
We suspect that sexual selection by male-male competition drives the evolutionary elaboration of drum speed and length, at least in downy woodpeckers. This idea is based on recent studies that suggest that elaborate displays produced through body and/or appendage movement reflect honest information regarding the health or condition of the signaler (Nowicki et al., 2002; Müller et al., 2010). The physiological link between these two variables—motor control of gesture and condition—are still being worked out, but there is a growing body of literature that suggests even the performance of routine locomotory tasks quickly becomes more challenging when they are performed at higher speeds (Wynn et al., 2015; Amir Abdul Nasir et al., 2017). In this way, competitive drumming may push woodpeckers to their performance limit, such that low quality individuals are incapable of drumming as fast or as long. Research in other taxa is fully consistent with this view, as signal length appears to influence the outcome of social interactions across a broad range of animals. In territorial disputes, for example, individuals capable of producing longer signals (e.g., more repetitive elements) are often perceived as superior competitors (Behr et al., 2006; Rivera-Gutierrez et al., 2010; Mager et al., 2012). Future work will be needed to test these ideas using multiple approaches. One starting point is to determine whether individuals with faster drums exhibit better health or body condition. Another more direct way includes determining whether individuals with faster and longer drums exhibit greater reproductive success.
Several studies also look at the functional effect of drum rhythm. Downy and red-bellied woodpeckers, for example, live in sympatry across much of eastern North America, and the two species frequently share overlapping territories. Although both taxa produce drums that are similar in average speed and length, rhythm is the one key difference between their drums—downy woodpeckers produce a drum with a cadence that slows down at a linear rate, whereas red-bellied woodpeckers produce a drum that speeds up at an exponential rate (Schuppe and Fuxjager, 2018). If a typical downy woodpecker drum is engineered so that its rhythm resembles that of a red-bellied woodpecker, then downy woodpeckers stop responding to it during an STI. Likewise, if a red-bellied woodpecker drum is engineered to resemble the rhythm of a downy woodpecker, then red-bellied woodpeckers stop responding to it (Schuppe and Fuxjager, 2018). Among other North American species, resident woodpeckers also exhibit subdued behavioral responses to STIs of either sympatric or allopatric species that exhibit markedly different drum speeds, cadences, or acceleration patterns (Dodenhoff et al., 2001). However, when species encounter (via STI) heterospecific intruders that produce drums of similar length and speed then their response is comparable to that of a conspecific intruder (Dodenhoff et al., 2001). These results are consistent with the idea that rhythm encodes species identity, a concept that extends across much of the woodpecker family. Large-scale comparative analyses indicate that sympatric sister taxa are more likely to have different cadence patterns to their drum (and to a lesser extent different acceleration patterns), compared to allopatric sister pairs.
Evolution of Drumming Behavior
Because drumming behavior is shared among most of the woodpecker lineage, we can also begin to study this signal at a macroevolutionary level. This approach can highlight potential principles that guide the evolutionary “construction” of drum displays. Such work is challenging when applied to many other types of signals. Birdsong, for example, is so complex and variable among species that it becomes difficult to track how homologous elements of the display might change through time, without reducing these elements into variables (e.g., principle components) that poorly track the display’s complexity (Goodale and Podos, 2010; Weir and Price, 2019). But drumming behavior differs because of the limited number of ways by which it varies from species to species; thus, we can more easily track how specific components of the signal likely change over time.
Ancestral state reconstruction of drumming behavior reveals that the signal likely first arose at the base of Picinae. This observation suggests that the signal was then retained through time, such that variation in the signal that deviates from this ancestral state evolved by way of either selection or neutral processes. Machinery that underlies a bird’s ability to drum therefore presumably evolved early in the species’ history and was similarly likely retained as the taxa within this clade diversified. Only a few woodpeckers (≈5) have completely lost drumming behavior, and many of these birds inhabit environments without trees (Miles et al., 2018). For example, ground woodpeckers (Geocolaptes olivaceus) live in holes in the ground throughout the treeless grasslands of southern Africa; individuals of this species have evolved wing displays in lieu of drumming (Short, 1971). Andean flickers (Colaptes rupicola) have also lost drumming from their behavioral repertoire, as they occupy the grasslands high up in the Andean mountains (Short, 1970, 1971). Interestingly, there are published anecdotal observations of Andean flickers producing drum-like displays in populations that have re-colonized habitats containing trees (Fjeldsa, 1991). Such findings speak to the notion that woodpeckers have a largely conserved neurobiological program for drumming, even in species that have lost the behavior altogether.
How exactly do drum displays differ across the woodpecker family? The answer centers on the three main components of the drum described above: speed, length, and rhythm (Figures 2, 3). For example, Japanese pygmy woodpeckers (Yungipicus kizuki) from the deciduous forests of northeastern Asia drum at ≈40 beats s–1, whereas Nubian woodpeckers (Campethera nubica) from Central and Eastern Africa drum at ≈8 beats s–1. Length variation is similarly extreme, with many Campephilus woodpeckers producing short “double knock” (2 beat) drums, while greater flamebacks (Chrysocolaptes lucidus) of the Indian subcontinent produce ≈50 beats per drum. Similarly, species like the South American helmeted woodpecker (Celeus galeatus) show a striking linear deceleration in speed, whereas other species like the North American golden-fronted woodpecker (Melanerpes aurifrons) show non-linear change in speed characterized by acceleration and then deceleration. Outside of these patterns, species within the Sphyrapicus genus exhibit unusual drum rhythms that are characterized by erratic changes in speed.
Our past work tests whether phenomenological signatures of strong sexual selection mark species differences in any of these display parameters. Specifically, we use sexual size dimorphism (SSD), which is one of the best predictors of the strength of sexual selection in birds [males are generally larger than females in taxa that evolve in response to relatively strong intrasexual selection; SSD (Dale et al., 2007; Székely et al., 2007)]. Analyses indicate that SSD positively predicts variation in drum length, but not drum speed (Miles et al., 2018). This finding suggests that these two components of the display are modular, in that they can change over time somewhat independently of each other. Morphological constraint provides insight into why length may be more modular and become elaborated by sexual selection. Although we found no evidence of a relationship between body size and drum length, this morphological variable is associated with speed (Miles et al., 2018). These two variables form a triangular distribution, in which the hypotenuse reflects a statistically significant negative relationship between body size and species’ drum speed. In short, larger woodpeckers exhibit a notable tradeoff between size and speed, but length is unconstrained in this manner. Thus, these findings suggest that sexual selection drives the elaboration of drum elements that are less constrained. Another insight from our work that is consistent with this idea is that drum length shows a greater evolutionary rate than drum speed.
In a separate study, we were interested in how a component of this signal, rhythm, that is in part used for species recognition is also influenced by sexual selection. We find that greater SSD values positively predict whether complex cadences and acceleration patterns are present in a particular species (Miles et al., 2020). Woodpecker species with larger males are therefore more likely to produce a drum that either increases or decreases in speed (or both) at a linear (e.g., consistent slowdown in inter-beat interval) or non-linear cadence (e.g., exponential increase in inter-beat interval speed). This suggests that sexual selection by male-male competition potentially influences how drum rhythm changes over time, alongside effects of selection through conspecific recognition (see above). Importantly, we also find that body size does not predict facets of rhythm, suggesting that morphology itself does not constrain innovation in this feature of the drum like it does for speed.
The evolutionary interplay among speed, length, and rhythm is also likely complex, as these components of the drum can influence how the others evolve. Rhythm, for example, can have potent effects on the way that drum speed and length change over time (Miles et al., 2020). When a drum takes on a complex cadence or acceleration pattern (anything not constant), then evolutionary rates of both speed and length are depressed. Likewise, this also means that drums with a constant rhythm—no change in speed over the course of a single drum—potentiate the evolution of display speed and length parameters. Either way, these findings provide clear evidence that one component of the display can dramatically alter how other components evolve, which of course influences the phenotypic “options” for sexual selection.
However, our studies also suggest that rhythm unequally constrains length and speed evolution. Rates of length evolution, for instance, are lower in species that drum with linear cadences, compared to those that drum with non-linear cadences (Miles et al., 2020). This difference is not observed with respect to rates of speed evolution, whereby constraint severity is likely similar for non-linear cadences and linear cadences alike. Thus, these data again point to drum length as a less constrained element of the drum signal, creating a potential “path of least resistance” for length display elaboration to occur.
Physiology and Biomechanics of Drumming and Drilling
Like any motor activity, beak behavior in woodpeckers must operate within the constraints of physical principles, as well as physiological “rules” that are common to muscle-driven systems. Below we review how physical and physiological demands may shape the evolution of beak behavior. Because there are few empirical studies of mechanics in drumming or drilling, we use inference from other forms of movement or communication signals to explore how mechanical demands may shape the evolution of these behaviors.
Drumming Is a Fast Activity
An obvious feature of drumming is that it is a high-speed activity. By plotting average drumming rates for different species, organized by genus, one can easily see that (i) drumming speeds are variable both within and across genera; and (ii) many species drum at rates exceeding 20 beats per second (Figure 4). Speed matters, because fast motions place demands on the neuromuscular system, and thus the evolution of fast drumming may have been driven by its usefulness as an honest signal of condition. Below we present benchmarks to put drumming in context of other movements, and we speculate how fast speed may translate to physiological challenges.
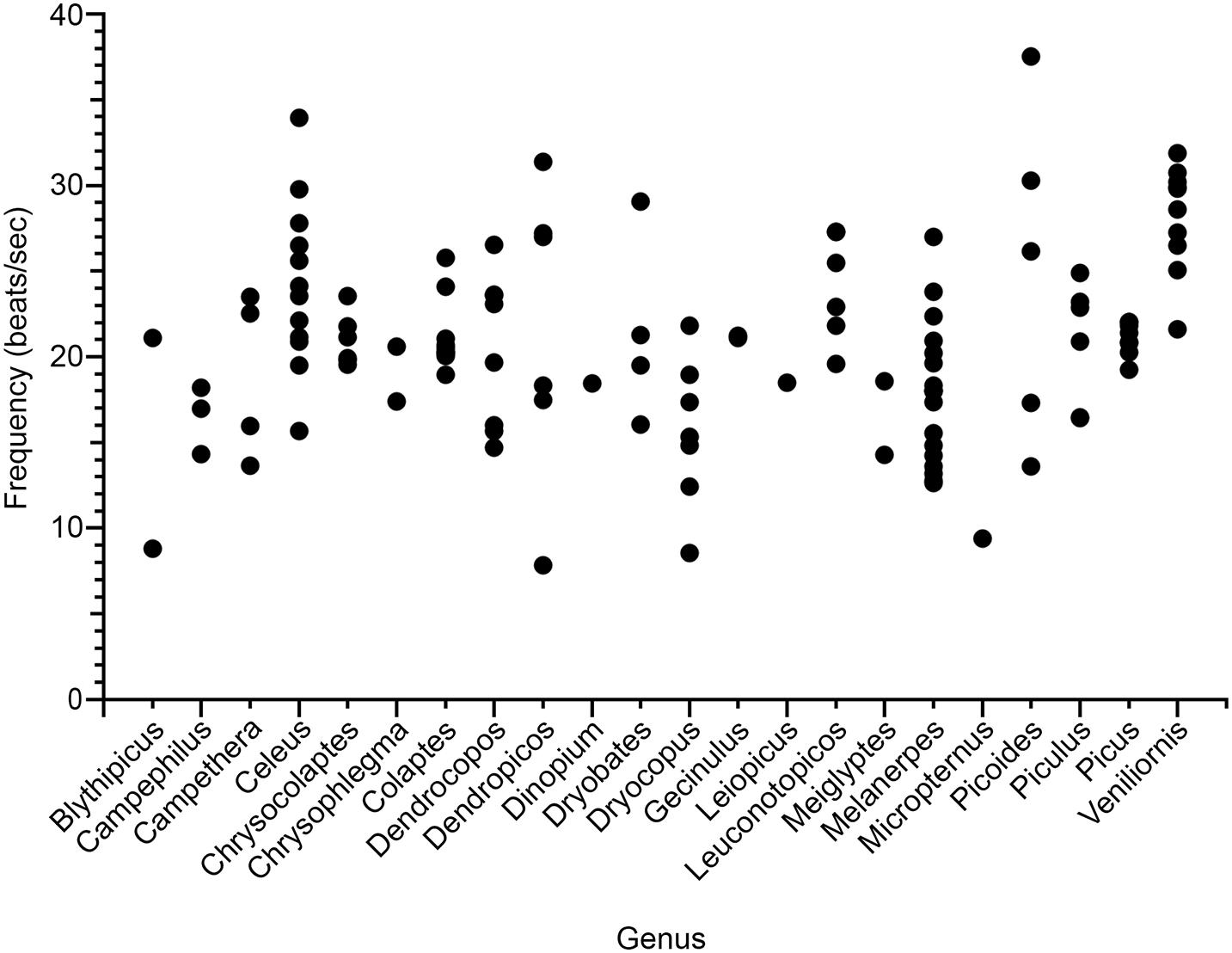
Figure 4. Variability in drumming frequency (beats s–1) within and between 22 woodpecker genera. Each point represents the mean (n ≥ 2) frequency of a single species extracted from audio recordings accessed through the Macaulay Library at the Cornell Lab of Ornithology and Xeno-canto (data from Miles et al., 2018).
Is drumming unusually fast? Figure 5 compares cyclic motion frequency across a range of vertebrate activities, from locomotory movements to motions involved in communication signals and some non-locomotor movements (e.g., cat paw-shake). The goal in compiling these data is not to produce an exhaustive summary, but rather to visualize broadly how motion frequencies compare for different kinds of activities. Perhaps the most obvious insight from Figure 5 is that communication signals can occur at much higher frequencies than locomotor movements. This is arguably consistent with the idea that sexual selection pushes performance to physiological limits, while also undoubtedly related to the differing mechanical demands of communication vs. locomotion. Drilling also involves rapid motions, but drilling frequencies are generally lower than drumming frequencies (Miles et al., 2018), and so selection for the fastest speeds has most likely been associated with drumming.
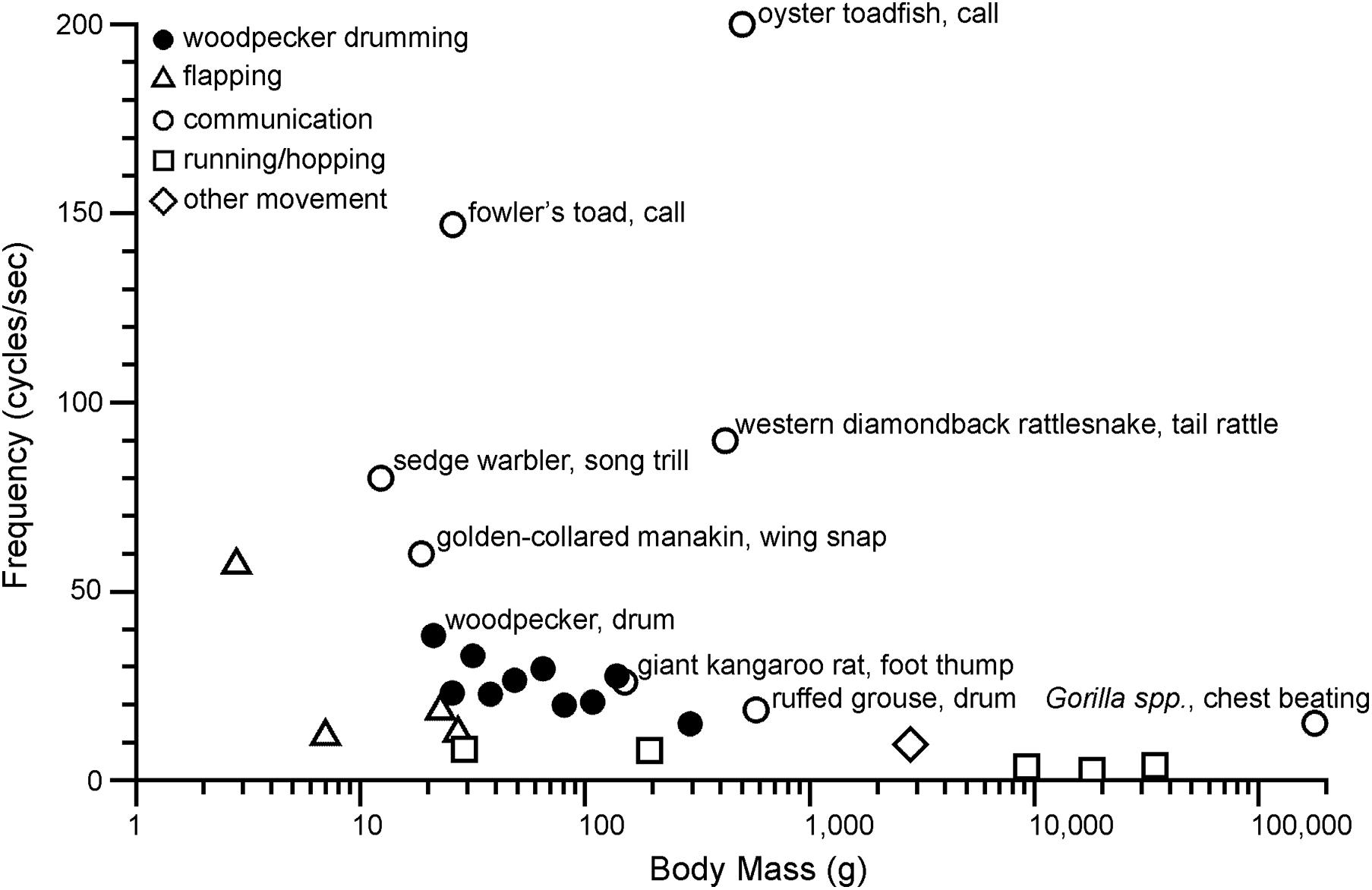
Figure 5. Woodpecker drumming relative to other fast frequency (cycles s–1) movements plotted by body mass (g) on a logarithmic scale. Woodpecker drumming (solid circles) includes from left to right: Japanese pygmy woodpecker, Downy woodpecker, Ladder-backed woodpecker, Nuttall’s woodpecker, Red-cockaded woodpecker, Hairy woodpecker, Acorn woodpecker, Lewis’s woodpecker, Northern flicker, and Pileated woodpecker. To limit woodpeckers to a subset of species that drum, the species with the fastest known drum (Japanese pygmy woodpecker) and North American species were selected. Communication (open circles) organisms are labeled on the graph. Flapping (open triangles) includes from left to right: Ruby-throated hummingbird, little broad-nosed bat, common chaffinch, and downy woodpecker. Running/hopping (open squares) includes from left to right: white mouse, ground squirrel, domestic dog, red kangaroo, and cheetah. Other movement (open diamond) includes domestic cat paw shake. Data sourced from: Zweifel (1968), Dawson and Taylor (1973), Walton and Anderson (1988), Heglund and Taylor (1988), Griffiths (1991), Rome et al. (1996), Schaeffer et al. (1996), Tobalske (1996), Goller and Suthers (1996), Randall (1997), Langefors et al. (1998), Pennycuick (2001), Bullen and McKenzie (2002), Stein and Uy (2006), Fine et al. (2009), Zihlman et al. (2011), Garcia et al. (2012), Hudson et al. (2012), Mahalingam and Welch (2013), Fusani et al. (2014), Miles et al. (2018), Schuppe and Fuxjager (2018), Déaux et al. (2020). See electronic Supplementary Material for more detail.
Given the uncertainties of our broad analysis of motion frequency (discussed below), conclusions drawn from this comparison must be made with caution. However, we feel that a roughly quantitative assessment leads to a few insights. The observation that the frequency of woodpecker drumming falls above the frequency of locomotor movements for similarly sized vertebrates supports the idea that drumming is a fast activity that presents a physiological challenge. At the same time, woodpecker drumming frequencies fall below the very fast communication signals of a diverse range of vertebrates. For some of the fastest motions in Figure 5, “superfast” muscles have been identified as a key specialization for generating high frequencies (Rome et al., 1996; Schaeffer et al., 1996; Rome and Lindstedt, 1998; Elemans et al., 2004; Fuxjager et al., 2016; Zweifel, 2017). These muscles allow for very fast activation and deactivation, but at the expense of maximum force production (peak tetanic isometric force). Woodpecker drumming frequencies fall below the frequencies of movements in which superfast muscles have been identified, but contractile property measurements will be required to evaluate whether such specializations occur in woodpeckers.
While at first glance woodpecker drumming may appear to occur at a relatively low frequency when compared with the calls of toads and toadfish, it is important to acknowledge that frequency is only one component of what makes a movement mechanically demanding. All of the very high frequency motions included on Figure 5 involve muscles driving the motion of relatively light loads. The muscles that drive a warbler’s trill, for example, are moving only air and relatively light structures of the syrinx, as well as possibly the mass of some respiratory muscles (Hartley, 1990; Wild et al., 1998; Suthers et al., 1999). Woodpecker drumming involves motions of the head and there appear to be specializations of neck muscles for this motion (Jenni, 1981; Schuppe et al., 2018), but many models of drumming suggest that motion of the body is important, driven by muscles of the hindlimbs (Vincent et al., 2007). This is significant, because the load determines the force and power that muscles must produce, thus the combination of relatively high frequency and high load of woodpecker drumming may be quite demanding.
Muscular Demands of Drumming and Drilling
Studies of woodpecker drumming mechanics are quite limited, but several lines of evidence support the idea that drumming places significant demands on the mechanical performance of skeletal muscles. When presented with a high frequency drum in an STI, woodpeckers appear to have a limited ability to increase their own drum speed—they can only boost their speed a few milliseconds faster than their typical, “unchallenged” speed. As mentioned above, this finding supports the idea that drum speeds occur at or near an individual’s physiological limit (Schuppe et al., 2018; Figure 3). Such high frequency motions can challenge muscles in several ways. Foremost, to cycle at high frequencies, muscles must turn on (activate) and off (deactivate) rapidly. Activation of muscles is governed by voltage-gated Ca2+ channels in the sarcoplasmic reticulum. This action is passive (does not require energy) and can be quite rapid. Muscle deactivation requires the use of ATP for active pumping of Ca2+ from the myoplasm to the sarcoplasmic reticulum, against a concentration gradient, and thus muscle relaxation is generally slower than activation. Muscles that cycle rapidly, as measured typically by a short duration of twitch force, require specializations such as a high density of activation machinery (e.g., sarcoplasmic reticulum, t-tubules) and a high mitochondrial density to fuel the high metabolic demand of Ca2+ pumps (Rome and Lindstedt, 1998; Rome, 2006). This takes space that might otherwise be occupied by contractile machinery, and the energy demands of calcium pumps incur a metabolic cost in every muscle contraction. In muscles specialized for very fastest cycling, superfast muscles, a well-developed calcium buffering mechanism involving parvalbumin also appears to be essential (Rome et al., 1996; Rome and Lindstedt, 1998; Nelson et al., 2018).
Anatomical and molecular specializations provide some clues regarding potential modifications for rapid calcium cycling in woodpeckers. The longus colli ventralis muscle of the neck is enlarged and studies have identified physiological adaptations associated with quick relaxation in this muscle (Jenni, 1981; Schuppe et al., 2018). Elevated expression of two protein encoding genes [parvalbumin and sarcoplasmic reticulum Ca2+ ATPase 1 (SERCA1)] that promote rapid Ca2+ transients was observed in the longus colli ventralis muscle in both downy and red-bellied woodpeckers. The protein products of these genes assist in moving and retaining myoplasmic Ca2+ back into the sarcoplasmic reticulum, leading to muscle relaxation. This increase was not seen in a woodpecker muscle with no role in drumming or in a non-woodpecker species that exhibits slower drum-like movements during foraging (Schuppe et al., 2018). Given this evidence, the fivefold increase in gene expression of parvalbumin and SERCA1 in a drumming muscle appears to be a specialization that supports the drumming behavior.
In addition to the challenges of muscle activation/deactivation, high-frequency movement can involve a high speed of muscle shortening, and this presents challenges that are different and somewhat independent from those of turning muscle on and off. The simplest measure of intrinsic muscle speed of shortening is Vmax, the theoretical unloaded maximal speed of shortening, which can be measured via a series of contractions at different speeds in an isolated, maximally activated muscle (Hill, 1938). Muscles with a high Vmax are metabolically costly, as faster shortening speeds involve higher activities of the ATPase involved in cross-bridge cycling (Bárány, 1967). Further, the force-velocity relationship of muscle dictates a trade-off between speed and force that can impact fast motions, because fast motions often require high forces.
The mechanical power required for drumming is likely also considerable. Power is the product of force and velocity. It has been established that drumming involves high speeds of movement, and the accelerations required with each reversal of direction of body motion will also involve high forces. Liu et al. (2017) used a piezoelectric film mounted under a wood block to measure impact forces during beak behavior (likely drilling) in a great spotted woodpecker (Dendrocopos major) and found values as high as 19.6 Newtons, a force corresponding to 200–300X body weight for this species. High-speed film estimates of acorn woodpeckers measured decelerations on impact that were 500–1500 g (May et al., 1979). Further, whether drums are produced for territorial defense or for attracting mates, drum volume (acoustic energy) is likely important. The production of acoustic energy requires muscular work, and, while the total acoustic energy produced in a drum is unknown, it is clear from other examples of sound production in nature that acoustic signals can be mechanically demanding.
While drilling motions are generally slower than drumming motions, they are likely to be mechanically demanding for reasons beyond speed. The drilling motions associated with excavation require mechanical work to break down the substrate. The relative proportion of drilling power that is associated with motions of the body vs. substrate breakdown is unknown and likely to be variable depending on substrate qualities and behavior (e.g., nest excavating vs. foraging).
Sources of Power for Drumming and Drilling
It seems reasonable to assume that the evolution of rapid drumming involved selection for morphological and physiological features that allow for sustained power production to maintain the drumming motion and produce sound. There are few empirical studies of drumming or drilling kinematics or kinetics, and so our understanding of where and how power is produced is quite limited. Theoretical models of the drumming motion are in many cases based on minimal empirical data, and assumptions vary. Some models place power production within the neck (Liu et al., 2015), whereas others assume hindlimb muscles play a role (Vincent et al., 2007).
A theoretical, schematic analysis of the flow of power during drumming highlights the many possible energy sources and sinks, as well as a number of possible sites for elastic mechanisms that might recycle energy (Figure 6). Ultimately, muscle actuation must drive the motion, and muscles in the limbs, neck, and even potentially the base of the tail may act as a source of energy for movement. The back-and-forth motion of drumming involves as much deceleration as acceleration, and so the muscles that power, for example, the acceleration of the head towards the substrate might also sink (dissipate) energy as they reverse the motion of the head backwards in preparation for the next strike. Elastic mechanisms can store and recover energy, for example, kinetic energy lost as the body decelerates might be stored in springy tendons, and the recoil of these tendons could reaccelerate the body in the other direction.
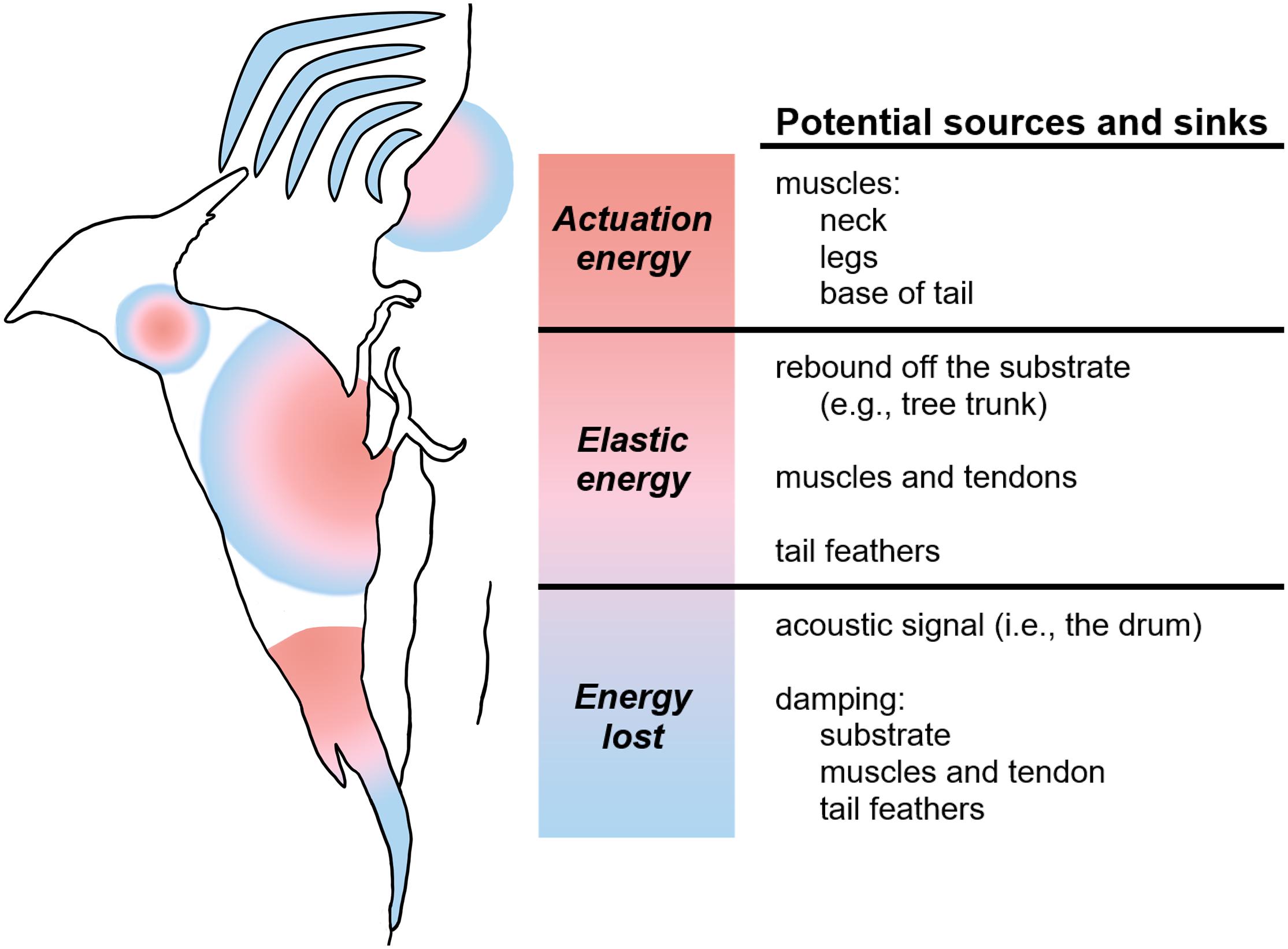
Figure 6. Schematic demonstrating potential energy sources and sinks in the woodpecker drumming system. Red represents the actuation energy to begin and maintain drumming; pink, the elastic energy that aids in the continuation of the behavior after actuation; and blue, the energy lost from the system.
One known sink of energy is the acoustic energy of the drumming sound. Mechanical work done by muscles is necessary to produce this energy. Does the acoustic energy in the drum signal represent a significant energy sink? We are not aware of existing measures of acoustic power of drumming. Measurements of sound power in other animal signals tell us that acoustic energy content is generally low. For example, Brackenbury (1979) measured acoustic power output for 17 species of songbirds and found values ranging from 10 to 870 mW kg–1 body weight. Even the loudest bird in the study, the song thrush (Turdus philomelos), produced a call with a sound energy of 0.87 W kg–1, which, for reference, is nearly two orders of magnitude lower than the 40–60 W kg–1 of mechanical power measured for steady flight in similarly sized budgerigars (Melopsittacus undulatus). It is difficult, however, to dismiss the mechanical demands of acoustic energy production as negligible because the efficiency of conversion of mechanical work to acoustic energy is generally quite low (Fletcher, 2007).
Measures of metabolic efficiency of sound production (metabolic power/sound power) in several insects and anurans provide at least an upper limit of the direct cost of producing sound energy. Efficiency values of biological sound production range from 0.2 to 6.4% (Prestwich, 1994). The metabolic cost of birdsong has received considerable attention. Results vary but generally find a relatively low cost of singing, with an elevation in energy consumption during song that is generally below 2-fold, and in some cases negligible (Oberweger and Goller, 2001; Ward et al., 2003; Zollinger and Brumm, 2015). Though birdsong is metabolically inexpensive, other acoustic signals, such as echolocation in bats, can be costly, with rates as high as 9.5 RMR in stationary bats (Speakman and Racey, 1991). The mechanical efficiency of sound production depends on a number of physical characteristics, including the size of the radiator, the wavelength of the sound, and the relative impedance of the radiator and sound-conducting medium (Prestwich, 1994). Given the distinctive nature of woodpecker drumming, it is difficult to draw conclusions about whether the acoustic energy content of a drum represents a significant portion of the mechanical energy budget of the drumming motion. Measurements of the energy content of the drum sound would improve our understanding.
Given the potentially high cost of rapid cyclic motions of the body and head during drumming, we hypothesize that the evolution of drumming as a signal hinges on mechanistic innovations that increase the efficiency of the drumming motion. The storage and recovery of energy by elastic mechanisms has the potential to significantly decrease the work that must be done by muscle contraction. Energy stored as elastic strain energy can be subsequently released, and work recovered from elastic sources is work muscles do not have to perform. For example, when the head decelerates from its backward motion, kinetic energy can be converted to elastic strain energy, and the release of this energy can power the acceleration of the head toward the substrate. Figure 6 identifies several hypothesized sites of elastic energy storage and recovery. Muscles and tendons of the limbs and neck may store and recover energy cyclically. Such mechanisms have been assumed in some mathematical models of drumming mechanics (Vincent et al., 2007; Liu et al., 2015), and woodpecker drumming has been modeled as a forced harmonic oscillator (Collins, 2017a). Tail feathers often brace the body during drumming. They can be observed to bend with the drumming motion, and may serve a spring-like function, as has sometimes been assumed (Vincent et al., 2007). Unknown is the extent to which elastic rebound on impact with the substrate contributes to motion. Spring-like behavior of wood has been considered in some mathematical models of drumming (Vincent et al., 2007), but whether such rebound is a significant or insignificant contributor remains to be determined. The material properties of the drumming substrate will be an important determinant of the elastic behavior. Woodpeckers choose a variety of drumming substrates, typically dead wood sites but also flexible metal substrates (e.g., gutters, chimney flashing). Studies of whether birds choose drumming sites with favorable elastic properties are underway.
Playback experiments suggest that woodpeckers operate near a physiological limit when drumming, but it is not clear what mechanical and/or neurological tasks may set this performance limit. At the muscle level, rapid drumming requires both rapid processes of activation/deactivation (Schuppe et al., 2018), as well as possibly high speeds of muscle shortening. Peak power output of muscle is a potential constraint, and elastic mechanisms may also set limits that are difficult to escape. And, physiologically demanding movement tasks are also demanding of motor control mechanisms (Barske et al., 2011; Clifton et al., 2015; Fuxjager et al., 2016), thus fidelity of motor control is also a possible limiting factor for performance.
The potential sources of power for drilling are the same as those for drumming. The need to break down substrate during drilling is likely to mean more energy is lost in each cycle, thus drilling may be associated with higher demands for net positive muscle power. We speculate that for this reason elastic mechanisms may be less important during drilling than during drumming, but this hypothesis remains to be tested.
Impact Risk of Beak Behavior
Many, possibly most, studies of the mechanics of drumming focus on the question of how woodpeckers can repeatedly strike their heads against a relatively stiff substrate without suffering brain injury. These studies are often motivated by a desire to reduce the chance of human brain injury, for example by improving helmet design through bio-inspiration (May et al., 1979; Mao et al., 2014; Liu et al., 2017). The production of sound with a high speed impact is unusual as an acoustic signal, and casual observation suggests that when impacts of similar speed and stiffness occur elsewhere in nature, the goal is often to cause or at least threaten damage [e.g., the impact of high-speed mantis shrimp claw “clubs” with mollusk shells or the horn collisions of big-horn sheep (Kitchener, 1988; Crane et al., 2018)]. Drumming itself is assumed to be derived from the drilling behavior that is meant to be destructive to wood as birds forage and excavate nests. Thus, it seems reasonable to hypothesize that anatomical or physiological specializations may have been required to reduce the chance of injury in the high-impact behavior of drumming.
Studies of mechanisms that might reduce the chance of injury during the impact phase of drilling or drumming have used mathematical models, anatomical observations, and some materials testing to probe for possible adaptations. Early analysis acknowledged that small size provides some protective effect (May et al., 1979). For a given acceleration the force on the brain will be proportional to mass while the cross-sectional area will scale with the 2/3 power of mass, thus stress (force/area) should decline with decreasing size for a given acceleration. Gibson (2006) observed further that differences in the orientation of the braincase between birds and humans increase the relative cross-sectional area of the brain in the direction of acceleration. Using measures of head deceleration taken for acorn woodpeckers (May et al., 1979) and a concussion tolerance curve for humans (Ono et al., 1980; Gibson, 2006) concluded that the accelerations woodpeckers experience on impact (≈600 to 1500 g) are well below that expected to cause injury (≈4,600 to 6,000 g). This analysis requires a number of assumptions, including that injury leading to concussion occurs at the same stress in human and woodpecker brains. It remains unclear if such a calculation, which puts woodpecker drumming and drilling impacts under a threshold for concussion in a single blow, means that mechanisms are not needed to reduce possible damage from repeated high impact accelerations.
Several anatomical features have been proposed to act as protective mechanisms against brain injury in woodpeckers. An idea central to many studies is that anatomical structures act as a damper, dissipating the energy of impact and thus reducing the energy left to accelerate the brain, much like a crumple zone in a car protects passengers. Features that have been proposed as dampers include the microanatomy of skull spongy bone (Wang et al., 2011), the micro and nano-structure of the ramphotheca of the beak (Lee et al., 2014; Liu et al., 2017), and the hyoid and associated muscles (Wang et al., 2011; Jung et al., 2016). Implicit in these functional interpretations is the idea that selection has favored these energy dissipating mechanisms. A challenge to this reasoning, acknowledged in some cases (Shaw, 2002; Liu et al., 2017), is that energy dissipated by anatomical structures is energy lost to its intended purpose, that is, the breakdown of wood in the case of drilling or the production of acoustic energy in the case of drumming. Other protective mechanisms that have been proposed include a tight packing of the brain within the brain case, which reduces the “sloshing” of the brain that may be associated with injury (Shaw, 2002) and a minimization of rotational accelerations that may also increase the risk of injury (May et al., 1979; Shaw, 2002). The putative mechanical risks associated with drumming need further investigation, as they may help explain why this signal evolved in the first place.
Summary
Here, we review the relatively small body of literature that explores woodpeckers drumming. We emphasis how ecological and mechanical factors likely interact to shape display design, painting an integrative picture of behavioral evolution. We highlight many avenues for future work that further expand our understanding of this process. In this way, research on woodpecker drumming serves as an example of how classic organismal biology can elucidate broader principles that underlie life and its diversity. Our manuscript is therefore as much of a starting point for additional research as it is a snapshot of completed work.
Author Contributions
ES and AR contributed to the writing of the manuscript and the synthesis of its ideas. TR and MF contributed to the writing of the manuscript, establishing the manuscript main points and ideas, and provided funding to support the research and effort. All authors contributed to the article and approved the submitted version.
Funding
This work was supported by the National Science Foundation (NSF) Graduate Research Fellowship under grant no. 2040433 (to AR), Bushnell Research and Education Fund (to AR), NSF grant IOS-1947472 (to MF), NSF grant OISE-1952542 (to MF), National Institutes of Health (NIH) grant AR055295 (to TR), and NSF grant EFMA-1832795 (to TR). Any opinion, findings, and conclusions or recommendations expressed in this material are those of the authors and do not necessarily reflect the views of the NSF or NIH.
Conflict of Interest
The authors declare that the research was conducted in the absence of any commercial or financial relationships that could be construed as a potential conflict of interest.
Publisher’s Note
All claims expressed in this article are solely those of the authors and do not necessarily represent those of their affiliated organizations, or those of the publisher, the editors and the reviewers. Any product that may be evaluated in this article, or claim that may be made by its manufacturer, is not guaranteed or endorsed by the publisher.
Acknowledgments
We thank Ghislaine Cardenas Posada, M.C. Miles, Daniel Tobiansky, Rich Marsh, Jarrod Petersen, and Mary Kate O’Donnell for thoughtful input, conservations, and/or perspectives on this topic over the years.
Supplementary Material
The Supplementary Material for this article can be found online at: https://www.frontiersin.org/articles/10.3389/fevo.2021.649146/full#supplementary-material
References
Amir Abdul Nasir, A. F., Clemente, C. J., Wynn, M. L., and Wilson, R. S. (2017). Optimal running speeds when there is a trade-off between speed and the probability of mistakes. Funct. Ecol. 2017:12902. doi: 10.1111/1365-2435.12902
Askins, R. A. (1983). Foraging ecology of temperate-zone and tropical woodpeckers. Ecology 1983:1937215. doi: 10.2307/1937215
Bárány, M. (1967). ATPase activity of myosin correlated with speed of muscle shortening. J. Gen. Physiol. 50:197. doi: 10.1085/jgp.50.6.197
Barkan, C. L., Kelley, D. B., and Zornik, E. (2018). Premotor neuron divergence reflects vocal evolution. J. Neurosci. 2018:0089. doi: 10.1523/JNEUROSCI.0089-18.2018
Barske, J., Schlinger, B. A., Wikelski, M., and Fusani, L. (2011). Female choice for male motor skills. Proc. R. Soc. B Biol. Sci. 278, 3523–3528. doi: 10.1098/rspb.2011.0382
Bauwens, D., Garland Jnr, T., Castilla, A. M., and Van Damme, R. (1995). Evolution of sprint speed in lacertid lizards: Morphological, physiological, and behavioral covariation. Evolution 1995:2321. doi: 10.1111/j.1558-5646.1995.tb02321.x
Behr, O., Von Helversen, O., Heckel, G., Nagy, M., Voigt, C. C., and Mayer, F. (2006). Territorial songs indicate male quality in the sac-winged bat Saccopteryx bilineata (Chiroptera, Emballonuridae). Behav. Ecol. 2006:13. doi: 10.1093/beheco/arl013
Bent, A. C. (1939). Life histories of North American wookpeckers. Order Piciformes. Bull. United States Natl. Museum. 1939, 1–334.
Brackenbury, J. H. (1979). Power Capabilities of the Avian Sound-Producing System. J. Exp. Biol. 78, 163–166.
Brewster, W. (1876). The Yellow-bellied Woodpecker (Sphyrapicus varius). Bull. Nuttall Ornithol. Club 1, 63–70.
Bullen, R. D., and McKenzie, N. L. (2002). Scaling bat wingbeat frequency and amplitude. J. Exp. Biol. 205, 2615–2626.
Burt, J. M., and Vehrencamp, S. L. (2005). Dawn chorus as an interactive communication network 320. Anim. Comm. Networks 2005:19. doi: 10.1017/CBO9780511610363.019
Clifton, G. T., Hedrick, T. L., and Biewener, A. A. (2015). Western and Clark’s grebes use novel strategies for running on water. J. Exp. Biol. 2015:118745. doi: 10.1242/jeb.118745
Collins, M. D. (2017a). Periodic and transient motions of large woodpeckers. Sci. Rep. 2017:13035. doi: 10.1038/s41598-017-13035-6
Collins, M. D. (2017b). Video evidence and other information relevant to the conservation of the Ivory-billed Woodpecker (Campephilus principalis). Heliyon 2017:230. doi: 10.1016/j.heliyon.2017.e00230
Conner, R. (1981). Seasonal Changes in Woodpecker Foraging Patterns. Auk Ornithol. Adv. 1981:562. doi: 10.1093/auk/98.3.562
Crane, R. L., Cox, S. M., Kisare, S. A., and Patek, S. N. (2018). Smashing mantis shrimp strategically impact shells. J. Exp. Biol. 221:176099. doi: 10.1242/jeb.176099
Dale, J., Dunn, P. O., Figuerola, J., Lislevand, T., Székely, T., and Whittingham, L. A. (2007). Sexual selection explains Rensch’s rule of allometry for sexual size dimorphism. Proc. R. Soc. B Biol. Sci. 2007:1043. doi: 10.1098/rspb.2007.1043
Dawson, T. J., and Taylor, C. R. (1973). Energetic cost of locomotion in kangaroos. Nature 246, 313–314. doi: 10.1038/246313a0
de Kiriline Lawrence, L. (1967). A comparative life-history study of four species of woodpeckers. Ornithol. Monogr. 1967, 1–156.
Déaux, E. C., O’Neil, N. P., Jensen, A. M., Charrier, I., and Iwaniuk, A. N. (2020). Courtship display speed varies daily and with body size in the Ruffed Grouse (Bonasa umbellus). Ethology 126, 528–539. doi: 10.1111/eth.13004
Ding, Y., Berrocal, A., Morita, T., Longden, K. D., and Stern, D. L. (2016). Natural courtship song variation caused by an intronic retroelement in an ion channel gene. Nature 2016:19093. doi: 10.1038/nature19093
Dodenhoff, D. J., Stark, R. D., and Johnson, E. V. (2001). Do woodpecker drums encode information for species recognition? Condor 2001:103. doi: 10.1650/0010-54222001103[0143:DWDEIF]2.0.CO;2
Elemans, C. P. H., Spierts, I. L. Y., Müller, U. K., Van Leeuwen, J. L., and Goller, F. (2004). Superfast muscles control dove’s trill. Nature 431:146. doi: 10.1038/431146a
Farris, K. L., Huss, M. J., and Zack, S. (2004). The role of foraging woodpeckers in the decomposition of ponderosa pine snags. Condor 2004:7484. doi: 10.1650/7484
Figarski, T. (2017). Contrasting seasonal reactions of two sibling woodpeckers to playback stimulation in urban areas - Implications for inventory and monitoring of the Syrian woodpecker. Behaviour 2017:3452. doi: 10.1163/1568539X-00003452
Fine, M. L., King, C. B., and Cameron, T. M. (2009). Acoustical properties of the swimbladder in the oyster toadfish opsanus tau. J. Exp. Biol. 212, 3542–3552. doi: 10.1242/jeb.033423
Fjeldsa, J. (1991). Andean flicker, Colaptes rupicola, nesting in trees and drumming. Le Gerfaut. 81:57.
Fletcher, N. H. (2007). “Animal bioacoustics”, in Springer Handbook of Acoustics, ed. Thomas D. Rossing, New York, NY, 785–802.
Fusani, L., Barske, J., Day, L. D., Fuxjager, M. J., and Schlinger, B. A. (2014). Physiological control of elaborate male courtship: Female choice for neuromuscular systems. Neurosci. Biobehav. Rev. 46, 534–546. doi: 10.1016/j.neubiorev.2014.07.017
Fuxjager, M. J., Goller, F., Dirkse, A., Sanin, G. D., and Garcia, S. (2016). Select forelimb muscles have evolved superfast contractile speed to support acrobatic social displays. Elife 5, 1–13. doi: 10.7554/eLife.13544
Garcia, M., Charrier, I., Rendall, D., and Iwaniuk, A. N. (2012). Temporal and Spectral Analyses Reveal Individual Variation in a Non-Vocal Acoustic Display: The Drumming Display of the Ruffed Grouse (Bonasa umbellus, L.). Ethology 118, 292–301. doi: 10.1111/j.1439-0310.2011.02011.x
Gibson, L. J. (2006). Woodpecker pecking: How woodpeckers avoid brain injury. J. Zool. 270, 462–465. doi: 10.1111/j.1469-7998.2006.00166.x
Goller, F., and Suthers, R. A. (1996). Role of syringeal muscles in gating airflow and sound production in singing brown thrashers. J. Neurophysiol. 75, 867–876. doi: 10.1152/jn.1996.75.2.867
Goodale, E., and Podos, J. (2010). Persistence of song types in Darwin’s finches, Geospiza fortis, over four decades. Biol. Lett. 2020:165. doi: 10.1098/rsbl.2010.0165
Griffiths, B. Y. R. I. (1991). Shortening of muscle fibres during stretch of the active cat medial gastrocnemius muscle: the role of tendon compliance. J. Physiol. 436, 219–236.
Hartley, R. S. (1990). Expiratory muscle activity during song production in the canary. Respir. Physiol. 81, 177–187. doi: 10.1016/0034-5687(90)90044-Y
Heglund, N. C., and Taylor, C. R. (1988). Speed, Stride Frqequency and Energy Cost Per Stride: How Do They Change With Body Size and Gait? J. Exp. Biol. 138, 301–318.
Hill, A. V. (1938). The heat of shortening and the dynamic constants of muscle. Proc. R. Soc. London. Ser. B - Biol. Sci. 126, 136–195. doi: 10.1098/rspb.1938.0050
Hudson, P. E., Corr, S. A., and Wilson, A. M. (2012). High speed galloping in the cheetah (Acinonyx jubatus) and the racing greyhound (Canis familiaris): Spatio-Temporal and kinetic characteristics. J. Exp. Biol. 215, 2425–2434. doi: 10.1242/jeb.066720
Jarvis, E. D., Mirarab, S., Aberer, A. J., Li, B., Houde, P., Li, C., et al. (2014). Whole-genome analyses resolve early branches in the tree of life of modern birds. Science 2014:1253451. doi: 10.1126/science.1253451
Jenni, V. L. (1981). Das Skelettmuskelsystem des Halses von Buntspecht und Mittelspecht Dendrocopos major and medius. J. Für Ornithol. 122, 37–63.
Jung, J. Y., Naleway, S. E., Yaraghi, N. A., Herrera, S., Sherman, V. R., Bushong, E. A., et al. (2016). Structural analysis of the tongue and hyoid apparatus in a woodpecker. Acta Biomater. 37, 1–13. doi: 10.1016/j.actbio.2016.03.030
Jusino, M. A., Lindner, D. L., Banik, M. T., Rose, K. R., and Walters, J. R. (2016). Experimental evidence of a symbiosis between red-cockaded woodpeckers and fungi. Proc. R. Soc. B Biol. Sci. 2016:106. doi: 10.1098/rspb.2016.0106
Kilham, L. (1958). Pair Formation, Mutual Tapping and Nest Hole Selection of Red-Bellied Woodpeckers. Auk 1958:401977. doi: 10.2307/4081977
Kilham, L. (1959). Behavior and Methods of Communication of Pileated Woodpeckers. Condor 1959:1365307. doi: 10.2307/1365307
Kilham, L. (1960). Courtship and Territorial Behavior of Hairy Woodpeckers. Auk 1960:4082482. doi: 10.2307/4082482
Kilham, L. (1969). Reproductive behavior of Hairy Woodpeckers. III. Agonistic behavior in relation to courtship and territory. Wilson Bull 81, 169–183.
Kilham, L. (1977). Early Breeding Season Behavior of Red-Headed Woodpeckers. Auk 1977:231. doi: 10.1093/auk/94.2.231
Kilham, L. (1979). Courtship and the Pair-Bond of Pileated Woodpeckers. Auk Ornithol. Adv 1979:587. doi: 10.1093/auk/96.3.587
Kitchener, A. (1988). An analysis of the forces of fighting of the blackbuck (Antilope cervicapra) and the bighorn sheep (Ovis canadensis) and the mechanical design of the horn of bovids. J. Zool. 214, 1–20. doi: 10.1111/j.1469-7998.1988.tb04983.x
Koenig, W. D. (1981). Reproductive Success, Group Size, and the Evolution of Cooperative Breeding in the Acorn Woodpecker. Am. Nat. 1981:283726. doi: 10.1086/283726
Koenig, W. D. (1996). Woodpeckers: An Identification Guide to the Woodpeckers of the World Hans Winkler David A. Christie David Nurney. Auk 1996:4089007. doi: 10.2307/4089007
Koenig, W. D., Schaefer, D. J., Mambelli, S., and Dawson, T. E. (2008). Acorns, insects, and the diet of adult versus nestling Acorn Woodpeckers. J. Field Ornithol. doi: 10.1111/j.1557-9263.2008.00174.x
Lammertink, M., Kopuchian, C., Brandl, H. B., Tubaro, P. L., and Winkler, H. (2016). A striking case of deceptive woodpecker colouration: The threatened Helmeted Woodpecker Dryocopus galeatus belongs in the genus Celeus. J. Ornithol. 2016, 1254. doi: 10.1007/s10336-015-1254-x
Langefors, A., Hasselquist, D., and von Schantz, T. (1998). Extra-Pair Fertilizations in the Sedge Warbler. J. Avian Biol. 29:134. doi: 10.2307/3677191
Lee, N., Horstemeyer, M. F., Rhee, H., Nabors, B., Liao, J., and Williams, L. N. (2014). Hierarchical multiscale structure - Property relationships of the red-bellied woodpecker (Melanerpes carolinus) beak. J. R. Soc. Interface 11:274. doi: 10.1098/rsif.2014.0274
Leite, G. A., Pinheiro, R. T., Marcelino, D. G., Figueira, J. E. C., and Delabie, J. H. C. (2013). Foraging behavior of kaempfer’s woodpecker (celeus obrieni), a bamboo specialist. Condor 2013:120062. doi: 10.1525/cond.2013.120062
Lennartz, M. R., Hooper, R. G., and Harlow, R. F. (1987). Sociality and cooperative breeding of red-cockaded woodpeckers, Picoides borealis. Behav. Ecol. Sociobiol. 1987:572629. doi: 10.1007/BF00572629
Ligon, J. D. (1970). Behavior and Breeding Biology of the Red-Cockaded Woodpecker. Auk 1970:4083919. doi: 10.2307/4083919
Lima, S. L. (1983). Downy woodpecker foraging behavior: foraging by expectation and energy intake rate. Oecologia 1983:399223. doi: 10.1007/BF00399223
Lima, S. L. (1984). Downy woodpecker foraging behaviour: efficient sampling in simple stochastic environments. Ecology 1984:1939468. doi: 10.2307/1939468
Liu, Y. Z., Qiu, X. M., Ma, H. L., Fu, W. W., and Yu, T. X. (2017). A study of woodpecker’s pecking process and the impact response of its brain. Int. J. Impact Eng. 108, 263–271. doi: 10.1016/j.ijimpeng.2017.05.016
Liu, Y., Qiu, X., Yu, T., Tao, J., and Cheng, Z. (2015). How does a woodpecker work? An impact dynamics approach. Acta Mech. Sin. Xuebao 31, 181–190. doi: 10.1007/s10409-015-0399-4
Mager, J. N., Walcott, C., and Piper, W. H. (2012). Male common loons signal greater aggressive motivation by lengthening territorial yodels. Wilson J. Ornithol. 2012:24. doi: 10.1676/11-024.1
Mahalingam, S., and Welch, K. C. (2013). Neuromuscular control of hovering wingbeat kinematics in response to distinct flight challenges in the ruby-throated hummingbird, Archilochus colubris. J. Exp. Biol. 216, 4161–4171. doi: 10.1242/jeb.089383
Mao, H., Huang, Q., Wang, J., and Zhu, M. (2014). An analysis of shock isolation characteristics of a head of a woodpecker and its application to a bionic helmet. J. Vibroengi. 16, 1821–1830.
May, P. R. A., Fuster, J. M., Haber, J., and Hirschman, A. (1979). Woodpecker Drilling Behavior: An Endorsement of the Rotational Theory of Impact Brain Injury. Arch. Neurol. 36, 370–373. doi: 10.1001/archneur.1979.00500420080011
Miles, M. C., and Fuxjager, M. J. (2019). Social context modulates how the winner effect restructures territorial behaviour in free-living woodpeckers. Anim. Behav. 2019:11. doi: 10.1016/j.anbehav.2019.02.011
Miles, M. C., Schuppe, E. R., and Fuxjager, M. J. (2020). Selection for rhythm as a trigger for recursive evolution in the elaborate display system of woodpeckers. Am. Nat. 2020:707748. doi: 10.1086/707748
Miles, M. C., Schuppe, E. R., Ligon, R. M., and Fuxjager, M. J. (2018). Macroevolutionary patterning of woodpecker drums reveals how sexual selection elaborates signals under constraint. Proc. R. Soc. B Biol. Sci. 2018:2628. doi: 10.1098/rspb.2017.2628
Miller, E. T., Leighton, G. M., Freeman, B. G., Lees, A. C., and Ligon, R. A. (2019). Ecological and geographical overlap drive plumage evolution and mimicry in woodpeckers. Nat. Commun. 2019:9721. doi: 10.1038/s41467-019-09721-w
Müller, W., Vergauwen, J., and Eens, M. (2010). Testing the developmental stress hypothesis in canaries: Consequences of nutritional stress on adult song phenotype and mate attractiveness. Behav. Ecol. Sociobiol. 2010:989. doi: 10.1007/s00265-010-0989-x
Nelson, F. E., Hollingworth, S., Marx, J. O., Baylor, S. M., and Rome, L. C. (2018). Small Ca2+ releases enable hour-long high-frequency contractions in midshipman swimbladder muscle. J. Gen. Physiol. 150, 127–143. doi: 10.1085/jgp.201711760
Nickley, B., and Bulluck, L. P. (2020). Red-headed Woodpecker (Melanerpes erythrocephalus) winter roost-site selection in a burned forest stand. Wilson J. Ornithol. 2020:774. doi: 10.1676/1559-4491-131.4.774
Nowicki, S., Searcy, W. A., and Peters, S. (2002). Brain development, song learning and mate choice in birds: A review and experimental test of the “nutritional stress hypothesis.”. J. Compar. Physiol. A 2002:3. doi: 10.1007/s00359-002-0361-3
Oberweger, K., and Goller, F. (2001). The metabolic cost of birdsong production. J. Exp. Biol. 204, 3379–3388.
Ono, K., Kikuchi, A., Nakamura, M., Kobayashi, H., and Nakamura, N. (1980). Human head tolerance to sagittal impact reliable estimation deduced from experimental head injury using subhuman primates and human cadaver skulls. SAE Tech. Pap. 89, 101–160. doi: 10.4271/801303
Pennycuick, C. J. (2001). Speeds and wingbeat frequencies of migrating birds compared with calculated benchmarks. J. Exp. Biol. 204, 3283–3294.
Peters, W. D., and Grubb, T. C. (1983). An experimental analysis of sex-specific foraging in the downy woodpecker, Picoides pubescens. Ecology 1983:1937498. doi: 10.2307/1937498
Prestwich, K. N. (1994). The energetics of acoustic signaling in anurans and insects. Integr. Comp. Biol. 1994:625. doi: 10.1093/icb/34.6.625
Randall, J. A. (1997). Species-specific footdrumming in kangaroo rats: Dipodomys ingens, D. deserti, D. spectabilis. Anim. Behav. 54, 1167–1175. doi: 10.1006/anbe.1997.0560
Rivera-Gutierrez, H. F., Pinxten, R., and Eens, M. (2010). Multiple signals for multiple messages: Great tit, Parus major, song signals age and survival. Anim. Behav. 2010:002. doi: 10.1016/j.anbehav.2010.06.002
Rome, L. C. (2006). Design and function of superfast muscles: New insights into the physiology of skeletal muscle. Annu. Rev. Physiol. 68, 193–221. doi: 10.1146/annurev.physiol.68.040104.105418
Rome, L. C., and Lindstedt, S. L. (1998). The quest for speed: muscles built for high-frequency contractions. News Physiol. Sci. 13, 261–268. doi: 10.1152/physiologyonline.1998.13.6.261
Rome, L. C., Syme, D. A., Hollingworth, S., Lindstedt, S. L., and Baylor, S. M. (1996). The whistle and the rattle: The design of sound producing muscles. Proc. Natl. Acad. Sci. U. S. A. 93, 8095–8100. doi: 10.1073/pnas.93.15.8095
Rudolph, D., Conner, R., and Turner, J. (1990). Competition for red-cockaded woodpecker roost and nest cavities: effects of resin age and entrance diameter. Wilson Bull 102, 23–36.
Schaeffer, P. J., Conley, K. E., and Lindstedt, S. L. (1996). Structural correlates of speed and endurance in skeletal muscle: The rattlesnake tailshaker muscle. J. Exp. Biol. 199, 351–358.
Schuppe, E. R., and Fuxjager, M. J. (2018). High-speed displays encoding motor skill trigger elevated territorial aggression in downy woodpeckers. Funct. Ecol. 2018:13010. doi: 10.1111/1365-2435.13010
Schuppe, E. R., Petersen, J. O., and Fuxjager, M. J. (2018). Woodpecker drumming behavior is linked to the elevated expression of genes that encode calcium handling proteins in the neck musculature. J. Exp. Biol. 221, 1–5. doi: 10.1242/jeb.180190
Schuppe, E. R., Sanin, G. D., and Fuxjager, M. J. (2016). The social context of a territorial dispute differentially influences the way individuals in breeding pairs coordinate their aggressive tactics. Behav. Ecol. Sociobiol. 2016:2088. doi: 10.1007/s00265-016-2088-0
Searcy, W. A., and Beecher, M. D. (2009). Song as an aggressive signal in songbirds. Anim. Behav. 2009:11. doi: 10.1016/j.anbehav.2009.08.011
Searcy, W. A., Anderson, R. C., and Nowicki, S. (2006). Bird song as a signal of aggressive intent. Behav. Ecol. Sociobiol. 2006:161. doi: 10.1007/s00265-006-0161-9
Shakya, S. B., Fuchs, J., Pons, J. M., and Sheldon, F. H. (2017). Tapping the woodpecker tree for evolutionary insight. Mol. Phylogenet. Evol. 2017:005. doi: 10.1016/j.ympev.2017.09.005
Shaw, N. A. (2002). The neurophysiology of concussion. Prog. Neurobiol. 67, 281–344. doi: 10.1016/S0301-0082(02)00018-7
Short, L. L. J. (1970). Notes on the Habits of Some Argentine a Peruvian Woodpeckers (Aves, Picidae). Am. Museum Novit. 1970:2413.
Speakman, J. R., and Racey, P. A. (1991). No cost of echolocation for bats in flight. Nature 350, 421–423. doi: 10.1038/350421a0
Spring, L. W. (1965). Climbing and Pecking Adaptations in Some North American Woodpeckers. Condor 1965:1365612. doi: 10.2307/1365612
Stark, R. D., Dodenhoff, D. J., and Johnson, E. V. (1998). A quantitative analysis of Woodpecker drumming. Condor 1998:1370276. doi: 10.2307/1370276
Stein, A. C., and Uy, J. A. C. (2006). Plumage brightness predicts male mating success in the lekking golden-collared manakin. Manacus Vitellinus. Behav. Ecol. 17, 41–47. doi: 10.1093/beheco/ari095
Stradi, R., Hudon, J., Celentano, G., and Pini, E. (1998). Carotenoids in bird plumage: the complement of yellow and red pigments in true woodpeckers (Picinae). Comp. Biochem. Physiol. - B Biochem. Mol. Biol. 1998:10033. doi: 10.1016/S0305-0491(98)10033-0
Suthers, R. A., Goller, F., and Pytte, C. (1999). The neuromuscular control birdsong. Philos. Trans. R. Soc. Lond. B. Biol. Sci. 354, 927–939. doi: 10.1098/rstb.1999.0444
Székely, T., Lislevand, T., and Figuerola, J. (2007). Sexual size dimorphism in birds in Sex, Size and Gender Roles: Evolutionary Studies of Sexual Size Dimorphism. BioScience 58, 460–461. doi: 10.1093/acprof:oso/9780199208784.003.0004
Tate, J. (1973). Methods and Annual Sequence of Foraging by the Sapsucker. Auk 1973:4084364. doi: 10.2307/4084364
Tobalske, B. W. (1996). Scaling of muscle composition, wing morphology, and intermittent flight behavior in woodpeckers. Auk 113, 151–177. doi: 10.2307/4088943
Vincent, J. F. V., Sahinkaya, M. N., and O’Shea, W. (2007). A woodpecker hammer. Proc. Inst. Mech. Eng. Part C J. Mech. Eng. Sci. 221, 1141–1147. doi: 10.1243/09544062JMES574
Walton, M., and Anderson, B. D. (1988). The aerobic cost of saltatory locomotion in the fowler’s toad (Bufo woodhousei fowleri). J. Exp. Biol. 136, 273–288.
Wang, L., CheungJason, J. T. M., Pu, F., Li, D., Zhang, M., and Fan, Y. (2011). Why do woodpeckers resist head impact injury: A biomechanical investigation. PLoS One 6, 1–8. doi: 10.1371/journal.pone.0026490
Ward, S., Speakman, J. R., and Slater, P. J. B. (2003). The energy cost of song in the canary, Serinus canaria. Anim. Behav. 2003:2250. doi: 10.1006/anbe.2003.2250
Weir, J. T., and Price, T. D. (2019). Song playbacks demonstrate slower evolution of song discrimination in birds from Amazonia than from temperate North America. PLoS Biol. 2019:300478. doi: 10.1371/journal.pbio.3000478
Wiebe, K. L., and Vitousek, M. N. (2015). Melanin plumage ornaments in both sexes of Northern Flicker are associated with body condition and predict reproductive output independent of age. Auk 2015:1. doi: 10.1642/AUK-14-281.1
Wild, J. M., Goller, F., and Suthers, R. A. (1998). Inspiratory muscle activity during bird song. J. Neurobiol. 36, 441–453. doi: 10.1002/(SICI)1097-4695(19980905)36:3<441::AID-NEU11<3.0.CO;2-E
Wilkins, H. D., and Ritchison, G. (1999). Drumming and tapping by Red-bellied Woodpeckers: Description and possible causation. J. F. Ornithol. 70, 578–586.
Winkler, H., and Short, L. (1978). A comparative analysis of acoustical signals in pied woodpeckers (Aves, Picoides). Bull. Am. Museum Nat. Hist. 1978:160.
Wynn, M. L., Clemente, C., Nasir, A. F. A. A., and Wilson, R. S. (2015). Running faster causes disaster: Trade-offs between speed, manoeuvrability and motor control when running around corners in northern quolls (Dasyurus hallucatus). J. Exp. Biol. 2015:111682. doi: 10.1242/jeb.111682
Zihlman, A. L., McFarland, R. K., and Underwood, C. E. (2011). Functional anatomy and adaptation of male gorillas (Gorilla gorilla gorilla) With Comparison to Male Orangutans (Pongo pygmaeus). Anat. Rec. 294, 1842–1855. doi: 10.1002/ar.21449
Zollinger, S. A., and Brumm, H. (2015). Why birds sing loud songs and why they sometimes don’t. Anim. Behav. 2015:30. doi: 10.1016/j.anbehav.2015.03.030
Zweifel, R. G. (2017). Effects of Temperature, Body Size, and Hybridization on Mating Calls of Toads, Bufo a. americanus and Bufo woodhousii fowleri Author (s): Richard G. Zweifel Published by: American Society of Ichthyologists and Herpetologists (ASIH) Stable URL. Am. Soc. Ichtyologists Herpetol. 1968, 269–285.
Keywords: display behavior, muscle physiology, sexual selection, spring mass system, behavioral evolution
Citation: Schuppe ER, Rutter AR, Roberts TJ and Fuxjager MJ (2021) Evolutionary and Biomechanical Basis of Drumming Behavior in Woodpeckers. Front. Ecol. Evol. 9:649146. doi: 10.3389/fevo.2021.649146
Received: 04 January 2021; Accepted: 29 June 2021;
Published: 26 July 2021.
Edited by:
Damian Octavio Elias, University of California, Berkeley, United StatesReviewed by:
Michael Collins, U.S. Naval Research Laboratory, United StatesCarl Soulsbury, University of Lincoln, United Kingdom
Copyright © 2021 Schuppe, Rutter, Roberts and Fuxjager. This is an open-access article distributed under the terms of the Creative Commons Attribution License (CC BY). The use, distribution or reproduction in other forums is permitted, provided the original author(s) and the copyright owner(s) are credited and that the original publication in this journal is cited, in accordance with accepted academic practice. No use, distribution or reproduction is permitted which does not comply with these terms.
*Correspondence: Matthew J. Fuxjager, bWF0dGhld19mdXhqYWdlckBicm93bi5lZHU=
†These authors have contributed equally to this work