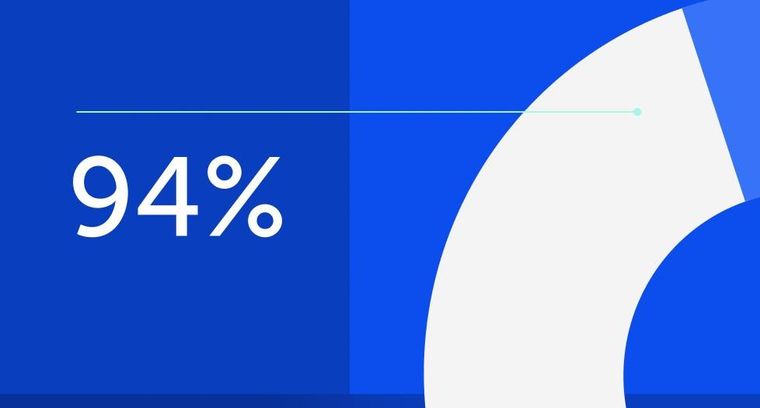
94% of researchers rate our articles as excellent or good
Learn more about the work of our research integrity team to safeguard the quality of each article we publish.
Find out more
ORIGINAL RESEARCH article
Front. Ecol. Evol., 19 March 2021
Sec. Paleontology
Volume 9 - 2021 | https://doi.org/10.3389/fevo.2021.640345
This article is part of the Research TopicTetrapod Water-Land Transition: Reconstructing Soft Tissue Anatomy and FunctionView all 14 articles
The fish-tetrapod transition (which incorporates the related fin-limb and water-land transitions) is celebrated as one of the most important junctions in vertebrate evolution. Sarcopterygian fishes (the “lobe-fins”) are today represented by lungfishes and coelacanths, but during the Paleozoic they were much more diverse. It was some of these sarcopterygians, a lineage of the tetrapodomorph fishes, that gave rise to tetrapods (terrestrial vertebrates with limbs bearing digits). This spectacular leap took place during the Devonian Period. Due to the nature of preservation, it is the hard parts of an animal’s body that are most likely to fossilize, while soft tissues such as muscular and brain tissues, typically fail to do so. Thus, our understanding of the adaptations of the hard skeletal structures of vertebrates is considerably greater than that of the soft tissue systems. Fortunately, the braincases of early vertebrates are often ossified and thereby have the potential to provide detailed morphological information. However, the correspondence between brain and endocast (an internal mold of the cavity) has historically been considered poor in most “lower” vertebrates and consequently neglected in such studies of brain evolution. Despite this, recent work documenting the spatial relationship in extant basal sarcopterygians (coelacanth, lungfish, axolotl, and salamander) has highlighted that this is not uniformly the case. Herein, we quantify and illustrate the brain-endocast relationship in four additional extant basal tetrapod exemplars: neobatrachian anurans (frogs) Breviceps poweri and Ceratophrys ornata; and gymnophionans (caecilians) Gegeneophis ramaswamii and Rhinatrema bivittatum. We show that anurans and caecilians appear to have brains that fill their endocasts to a similar degree to that of lungfishes and salamanders, but not coelacanth. Ceratophrys has considerably lower correspondence between the brain and endocast in the olfactory tract and mesencephalic regions, while Breviceps has low correspondence along its ventral endocranial margin. The brains of caecilians reflect their endocasts most closely (vol. ∼70%). The telencephalon is tightly fitted within the endocast in all four taxa. Our findings highlight the need to adequately assess the brain-endocast relationship in a broad range of vertebrates, in order to inform neural reconstructions of fossil taxa using the Extant Phylogenetic Bracket approach and future studies of brain evolution.
Plants and invertebrates had already ventured onto land in the Silurian Period (419–443 million years ago), well before the transition onto land by the first vertebrates (back-boned animals) during the Devonian Period (359–419 million years ago). Nevertheless, the move from water to land by these animals, known as the “fish-tetrapod transition,” is widely celebrated as a highly significant evolutionary leap which eventually gave rise to roughly half of all today’s vertebrate diversity (including humans).
Due to the nature of fossilization, changes over time in the skeleton are much better documented and understood (e.g., Cloutier et al., 2020) than the related soft tissues such as muscle and brain. In particular, preserved brains are exceedingly rare in the fossil record (although see Pradel et al., 2009), and where they are preserved they unlikely reflect brain morphology during life due to desiccation. As such, the internal part of the skull that houses the brain, or “endocast” has instead been used as a proxy for visualizing the brain, the shape of its component parts and quantitatively assessing their size, in a field of study known as palaeoneurology (Edinger, 1921).
By analyzing cranial endocasts (the internal space within the cranial cavity) of fossil taxa, certain inferences about behavior can be drawn, guided by the principle of proper mass, whereby “the mass of neural tissue controlling a particular function is appropriate (proportionate) to the amount of information processing involved in performing the function” (Jerison, 1973, pg. 8). That is to say, the more important or acute a sense or behavior is to an animal, the more likely that brain region will be relatively larger than would otherwise be expected, and consequently be reflected in the shape of the braincase internally. Applying this principal to endocasts of fossils can be problematic in those taxa which brains incompletely fill their endocasts, and the internal spatial relationship between brain and endocast is not known.
Despite the significance afforded to the fish-tetrapod transition, there remain relatively few known cranial endocasts of tetrapodomorph fishes (stem-tetrapods). The best-known example is that of Eusthenopteron foordi, a tristichopterid fish from the Late Devonian (mid-Frasnian) Escuminac Formation in Canada (Whiteaves, 1883). Using Sollas’ grinding method common in embryology, palaeoichthyologists from the “Stockholm School” and elsewhere, serially ground several Paleozoic 3D-preserved early vertebrates to reconstruct their endocasts, including that of Eusthenopteron foordi (Jarvik, 1980). Romer (1937) followed the same method to reconstruct the endocast of the Permian megalichthyid Ectosteorhachis from the United States.
More recently, advances in computed tomography (CT) scanning technology have enabled several more endocasts to be investigated non-destructively. Gogonasus, from the Late Devonian (Frasnian) Gogo Formation in Australia is known from complete, exceptionally-preserved 3D material (Holland, 2014), while Tungsenia from the Early Devonian (Pragian) of China, and Spodichthys from the Late Devonian (Frasnian) of East Greenland, have had only their ethmosphenoid regions modeled (Snitting, 2008; Lu et al., 2012). Work currently underway (Clement, pers. comm.) will soon add a complete endocast of Cladarosymblema, a megalichthyinid from the Carboniferous of Australia, to the list of those stem-tetrapods where the morphology of the endocast has been examined.
The record of endocasts for basal tetrapods is similarly depauperate, and includes the following: a partial endocast from the well-known Devonian tetrapod Ichthyostega (Clack et al., 2003); a cranial endocast from the early Carboniferous tetrapod Lethiscus (Pardo et al., 2017b); an otic capsule endocast from the Carboniferous temnospondyl, Dendrerpeton (Robinson et al., 2005); two Permian temnospondyls, Eryops and Edops, had their endocasts described in good detail but were made using destructive techniques (Dempster, 1935; Romer and Edinger, 1942); the Permian recumbirostran Brachydectes examined using non-destructive tomographic methods (Pardo and Anderson, 2016); and the Triassic stegocephalians Deinosuchus, Lyrocephalus, and Aphaneramma (Stensiö, 1963). This is, in part, due to many basal crown tetrapods possessing neurocrania that are poorly ossified compared to many tetrapodomorph fishes.
However, despite this pioneering work on these early vertebrates, most comparative neurological or palaeoneurological studies continue to focus on birds and mammals, due to their brains largely filling the cranial vault with a tight correspondence with their braincases (Jerison, 1973). The brains of “lower” (anamniote) vertebrates generally incompletely fills the internal braincase space, and therefore have been considered of limited use for interpreting brain morphology. Jerison (1973, pg. 121) claims that the brain of lungfishes is only 10% as big as the endocast, and that it would thus be “difficult, although not impossible, to understand the brain’s anatomy from an endocranial cast.” Although a large disparity was recorded between the lungfish brain and endocast, the disparity is even greater still in the coelacanth (Latimeria) with a brain just 1% of its endocranial volume (Millot and Anthony, 1965).
Recent work based on CT and magnetic resonance imaging (MRI) scans has interrogated these assertions. Clement et al. (2015) revealed that the brain of a sub-adult Australian lungfish (Neoceratodus) fills the majority of its endocast (∼80%), while the adult coelacanth (Latimeria) was confirmed to indeed have a brain one hundredth of the volume of its capacious cavity (Dutel et al., 2019). While it is well understood that ontogeny can have an effect on relative brain size, adult specimens from both extant lungfish families (Neoceratodontidae, Lepidosirenidae) were later found to have a brain filling upward of 40% of their endocasts, even without correction for ostensible shrinkage (Challands et al., 2020), a proportion that is significantly higher than the reported 10% from Jerison (1973). In fact, more recent data (Yopak et al., 2010; Iwaniuk, 2017; Striedter and Northcutt, 2020) continues to show a more nuanced picture with greater degree of overlap in brain volume to body mass between the “lower” and “higher” vertebrates, and thus we consider that it can be reasonably expected that the relationship between brain and endocast is similarly complicated.
The “heat-map” surface-surface distance measurement method of quantifying the brain-endocast spatial relationship in the Australian lungfish developed by Clement et al. (2015), was recently applied to six basal sarcopterygian taxa as a proxy to elucidate the changes that occurred over the fish-tetrapod transition (Challands et al., 2020). Taxa investigated included the coelacanth (Latimeria chalumnae), Australian lungfish (Neoceratodus forsteri), African lungfish (Protopterus aethiopicus, P. dolloi), the axolotl (Ambystoma mexicanum) and fire newt [Triturus (Cynops) pyrrhogaster].
Challands et al. (2020) confirmed earlier work that showed the coelacanth to be an outlier, with a miniscule brain lying within a cavernous endocast (Dutel et al., 2019), which is in stark contrast to the brain of lungfishes and salamanders that occupy ∼40–50% of the endocast. Those authors found that the Australian and African lungfishes have quite a good fit between brain and endocast internally, although the olfactory tract morphology in Protopterus spp. is not faithfully reflected in its endocast. In the salamanders, Triturus (Cynops) and Ambystoma, there is also quite a tight fit except for the region where the trigeminal nerve complex exits the braincase. Overall, these findings suggest that certain inferences can be made about the size of the brain from its endocast in basal sarcopterygians, in particular with respect to some brain regions, such as the telencephalon (forebrain).
Challands et al. (2020) suggested that the closeness of fit of different regions of the brain to the brain cavity may be influenced by the mandibular musculature and so, to a certain extent, could be predicted from the skull morphology where musculature can be reconstructed. Regions of lowest brain-endocast disparity occurred where bony reinforcement of the neurocranium lies adjacent to mandibular musculature, whereas regions of highest disparity were found in regions with lower reinforcement (e.g., where mandibular masticatory musculature mass was lower or absent). Such regions included where the trigeminal nerve complex exits the braincase and where endolymphatic sacs are present within the braincase.
There are three living genera of lungfishes and one genus of coelacanth, which united represent the only extant piscine sarcopterygians, and are therefore of particular relevance to the fish-tetrapod transition. Conversely, on the other side of the extant phylogenetic bracket are lissamphibians, such as frogs, salamanders and caecilians, which can also likely provide equally valuable insights into brain evolution of the first tetrapods. For the purposes outlined in this paper, we have adopted the phylogeny of extant amphibians from Pyron and Wiens (2011) and do not herein attempt to comment of the controversy surrounding the origins of Lissamphibia (Marjanovic and Laurin, 2007; Ruta and Coates, 2007). We accept that the first tetrapods appeared during the Devonian, the tetrapod crown node arose during the Carboniferous, and the extant amphibian orders most likely have their origins dating back throughout the Permo-Triassic (Anderson et al., 2008; Clack, 2012; Pardo et al., 2017a).
Since we cannot assume a priori that salamanders are necessarily the best representatives to interpret the condition of the first tetrapods, we hereby aim to quantify the brain-endocast spatial relationship in four more extant lissamphibian basal tetrapod exemplars: neobatrachian anurans Breviceps poweri, and Ceratophrys ornata; and gymnophionans Gegeneophis ramaswamii and Rhinatrema bivittatum. We hypothesize that these taxa will have brains that fill their endocasts to a similar degree as that of lungfishes and salamanders, and the region of closest fit will be around the forebrain. As more 3D morphological data such as these are created, brain reconstruction models, such as that of Clement et al. (2016), can be further refined by incorporating data from the full extant phylogenetic bracket for any given fossil taxon.
Diffusible iodine-based contrast-enhanced computed tomography (diceCT) scan data of B. poweri (Power’s Rain Frog), Media number: M13270-22958, was obtained under CC BY-NC-SA copyright from MorphoSource1. The pixel size was 21 microns (μm).
The C. ornata (Argentine Horned Frog) diceCT scan data was originally generated by Kleinteich and Gorb (2015), but later downloaded for this study from Dryad Digital Repository2 under a CC0 1.0 Universal Public Domain Dedication license. The pixel size was 27 μm.
The G. ramaswamii (tenmalai blind caecilian) and R. bivittatum (two-lined caecilian) diceCT scan data were downloaded from Duke University (see text footnote 1), with Media numbers: M71659-133946 and M33276-61864, and obtained under -CC BY-NC-SA- attribution. Florida Museum of Natural History, University of Florida provided access to these data, the collection of which was funded by oVert TCN; NSF DBI-1701714. The Gegeneophis dataset was subsequently subsampled so the resultant pixel size was 25 μm, whereas the Rhinatrema data were subsampled to 32 μm.
All data were segmented and rendered, and stereolithographs (STL’s) produced, in MIMICS v.18–19 (Materialise, 1992–2020).
The “closeness of fit” between 3D surface mesh STL’s of the brain and the braincase’s internal “endocast” were analyzed using the “Surface-to-Surface Distance Measurement Script” of Clement et al. (2015) using Python, with script updates from Challands et al. (2020). This custom script is publicly available from3. The resulting images visualize the surface-to-surface distance between STL’s using a “heat map” whereby cooler colors (blue) indicate smaller distances (a closer fit), and warmer colors (red) indicate greater distance.
Comparison of volumes were calculated from 3D model volume values obtained from MIMICS. Figures were prepared and assembled in Adobe Photoshop and Illustrator, GIMP and Inkscape.
The segmented brains (gray) and the brain-endocast overlays (gray and red), and surface-surface distance “heat maps” are shown in Figures 1, 2. Brain volume as a percentage of endocast volume in mm3 is given in Table 1.
Figure 1. 3D models of brains (gray), endocasts (red), and surface-surface distance heatmaps of two anurans in left lateral, ventral and dorsal views. (A–C) Breviceps; (D–F) Ceratophrys. Anterior to the left. cb, cerebellum; hyp, hypophysis; med, medulla oblongata; mes (ot), mesencephalon/optic tectum; tel, telencephalon; ob, olfactory bulb; olf, olfactory tract; n.V, trigeminal nerve complex; n.VIII, auditory nerve. Scale 10 mm.
Figure 2. 3D models of brains (gray), endocasts (red), and surface-surface distance heatmaps of two caecilians in left lateral, ventral and dorsal views. (A–C) Gegeneophis; and (D–F) Rhinatrema. Anterior to the left. cb, cerebellum; med, medulla oblongata; mes (ot), mesencephalon/optic tectum; tel, telencephalon; ob, olfactory bulb. Scale 1 mm.
Table 1. Brain volume as a percentage of endocast volume in mm3 in extant piscine sarcopterygians and select Lissamphibian taxa.
The brain of B. poweri measures 3.0 mm long from the anterior edge of the olfactory bulbs to the spinal cord, 1.3 mm across the widest point of the brain, and 1.4 mm at the highest point of the brain (Figure 1A). The forebrain (consisting of the telencephalon and diencephalon) comprises 48% of the total brain length, the midbrain (mesencephalon) 21%, and the hindbrain (metencephalon and myelencephalon) 31%. The cranial endocast of B. poweri measures 3.4 mm in length, 1.8 mm at its widest point, and 1.6 mm dorsoventrally.
The forebrain of B. poweri is dominated by the large, bulbous, oval-shaped telencephalic (tel) hemispheres which are slightly wider than they are long (Figure 1A). The boundary between the olfactory bulbs and the telencephalon is difficult to discern. There is 0.1 mm of olfactory nerve visible between the olfactory epithelium and the olfactory bulbs. The area around the diencephalon constricts in all axes, and then expands dorsoventrally to accommodate the optic tectum [mes (ot)] in the midbrain. The spinal cord is angled upward dorsally at about 45° from the rest of the brain. The trigeminal (n.V) and auditory (n.VIII) nerves are visible emerging from the hindbrain from the scan data.
The cranial endocast of B. poweri, when viewed laterally, steadily increases in both dorsoventral and lateral diameter from the olfactory bulbs through to the hindbrain and then rapidly constricts near the spinal cord (Figures 1B,C). When viewing the cranial endocast dorsally, the shape of forebrain is almost circular, with an abrupt widening from the olfactory bulbs and then constricting behind the optic tecta. The endocast is close to the surface of the brain (<0.1 mm) on the ventral side of the olfactory tracts, the dorsal side of the olfactory bulbs, and the dorsal and lateral sides of the optic tecta in the midbrain. The greatest distance between the brain and cranial endocast is on the ventral side of the brain, where distances are >0.17 mm over the majority of the area (Figure 1C).
The brain of C. ornata (Figure 1D) measures 4.2 mm from the anterior edge of the olfactory bulbs to the spinal cord and is 1.1 mm at its widest point and 1.2 mm at the highest point of the brain. The forebrain comprises 43% of the total brain length, the midbrain 31%, and the hindbrain 26%. The cranial endocast of C. ornata measures 5.2 mm long, 2.1 mm at the widest point, and 1.7 mm in height.
The olfactory tracts (olf) extend a relatively long way into the cranial endocast (1.2 mm, 23% of the length of the endocast). The olfactory tracts run parallel to each other and lie 0.3 mm apart. The olfactory bulbs (ob) are recognizable as a separate bulge distinct from the telencephalon (tel) anteriorly, which extends anteroventrally from the olfactory tracts as triangular-shaped structures. The telencephalic hemispheres are small, paired, oval prominences. The brain narrows considerably in the diencephalic region and the mid- and hindbrain angles ventrally downward some 45° from the preceding forebrain. The hypophyseal region (hyp) is short and angled posteroventrally. The brain expands only slightly in the midbrain (mes) to accommodate the optic tecta. The brain then narrows toward the hindbrain (med) and the anterior edge of the spinal cord. The trigeminal (n.V), facial (n.VII), auditory (n.VIII), glossopharyngeal (n.IX) and vagus nerves (n.X) are visible from the scan data in the hindbrain region (Figure 1D).
In contrast to the bulbous brain, the cranial endocast of C. ornata is smooth and lacking obvious protuberances (Figure 1E). It slowly widens from the olfactory epithelia to the midbrain. Following the course of the brain, the mid- and hindbrain endocast regions also angles down ventrally to the anterior end of the spinal cord. Dorsally, the widest region accommodates the midbrain and the nerves emerging from the hindbrain (n.V-X), and then tapers toward the hindbrain ventral edge. The surface-surface distances between the cranial endocast and brain of C. ornata are <0.1 mm on the ventral surfaces of the diencephalon and hindbrain, and on the dorsal surfaces of the telencephalon and optic tecta. There are larger distances of >0.2 mm between the brain and the endocast on the dorsal surface, where the olfactory nerves extend into the endocast, and on the lateral surfaces near the trigeminal nerve’s entry to the brain (Figure 1F).
The brain of G. ramaswamii measures 6.0 mm from the anterior edge of the olfactory bulbs to the spinal cord, is 3.4 mm wide across the broadest point of the brain, and is 1.2 mm in height (Figure 2A). The forebrain contributes 61% of the total brain length, while the midbrain and hindbrain contribute 17 and 22%, respectively. The cranial endocast measures 6.2 mm in length, 3.7 mm in width, and 1.2 mm in height.
The scans of the brain and cranial endocast of G. ramaswamii have resulted in some compression on the right lateral edge of the specimen. However, this is the only distortion visible on the scans and anatomical features are still recognizable and measurements have been approximated along the compressed edge.
The forebrain of G. ramaswamii consists of large telencephalic hemispheres (tel) that are much longer than they are wide (Figure 2A). When viewed laterally, the height of the brain slowly increases from the anterior edge of the olfactory bulbs (ob) to the midpoint of the telencephalon, and then slightly decreases in height toward the posterior edge of the hindbrain. The brain narrows behind the midbrain (mesencephalon) before it expands as a conical structure in the hindbrain toward the spinal cord. The optic tectum (mes) appears as a single protuberance rather than a paired structure. From the lateral view, the brain appears approximately linear.
The cranial endocast of G. ramaswamii fits very tightly around the forebrain (Figures 2B,C), with <0.2 mm gap between the brain and the endocast. The brain and endocast constrict to slightly different degrees in the mid- and hindbrain region, leaving distances of >0.3 mm in these regions.
The brain of R. bivittatum measures 5.5 mm from the anterior edge of the olfactory bulbs to the spinal cord, is 2.1 mm wide across the broadest point at the midbrain, and is 1.7 mm in height at the highest part of the brain (Figure 2D). The forebrain contributes 63% of the total brain length, with the midbrain and hindbrain contributing 24 and 13%, respectively. The cranial endocast measures 5.7 mm in length, 2.3 mm in width and 2.0 mm in height.
When observing the brain of R. bivittatum dorsally, the forebrain (tel) maintains a similar width along the majority of the telencephalon, which appears as an elongated pair of rectangular structures without any expansion postero-laterally (Figure 2D). The olfactory bulbs (ob) are recognizable anteriorly, where the brain narrows slightly and the two hemispheres are separated by a small groove. The midbrain increases in diameter along all axes around the optic tecta, and the hindbrain tapers toward the spinal cord. From the lateral view, the brain appears approximately linear except for some dorsal expansion of the mesencephalic region (mes).
The cranial endocast of R. bivittatum closely mirrors the shape of the forebrain, with <0.3 mm of distance between the brain and endocast (Figures 2E,F). The endocast widens where the midbrain expands, and maintains the short distance of <0.3 mm between the brain and braincase. The endocast then slightly narrows around the posterior edge of the midbrain and the hindbrain, but not to the same extent that the midbrain contracts, leaving distances >0.3 mm between the majority of these areas (Figures 2E,F).
The Extant Phylogenetic Bracket method (EPB), whereby two or more extant outgroups are used to provide rigorous limits of biological inference to a fossil taxon in question (Witmer, 1995), is a valued approach to aid in the reconstruction of soft tissues in extinct taxa. Although extant tetrapods are separated by over 360 million years from the first terrestrial vertebrates, the anatomy and morphology of their closest piscine relatives (sarcopterygian fishes) and basal tetrapods (lissamphibians) can provide invaluable insight into the condition of their long extinct kin.
The brain-endocast relationships of piscine sarcopterygians and salamanders have been considered elsewhere (Clement et al., 2015; Dutel et al., 2019; Challands et al., 2020) but are complemented by the additions in this study investigating frogs and caecilians to fulfill the EPB for Palaeozoic stem tetrapods (Figure 3). By quantifying the spatial relationship between the brain and internal cavity of the braincase (the endocast) across sarcopterygians ranging from the coelacanth, both lungfish families, and all three orders of extant amphibians, we have provided further information that can be used to identify a range of brain volumes for early stem tetrapods (assuming the EPB method is robust), and thus have established likely minimum volumes for the brains of these animals.
Figure 3. Brain-braincase relationship in extant piscine sarcopterygians and select Lissamphibian taxa. Endocasts (light brown) shown in transparent overlay on brains (pink) in left lateral view. Latimeria, Neoceratodus, Protopterus, Ambystoma, and Cynops from Challands et al. (2020), phylogeny adapted from Amemiya et al. (2013) and Pyron and Wiens (2011). Images not to scale. Gegeneophis silhouette drawn by AMC, all other animal silhouettes from http://phylopic.org, see Acknowledgments for individual image credits.
Caecilians are enigmatic and generally poorly understood animals although recent work has elucidated data on their crania relating to its musculature (Kleinteich and Haas, 2007), shape (Müller et al., 2009; Sherratt et al., 2014), and modularity (Marshall et al., 2019). Work on braincase morphology (Maddin et al., 2012a, b) and the labyrinth system (Maddin and Anderson, 2012; Maddin and Sherratt, 2014) has provided a breadth of new information, but to the best of our knowledge, we are herein the first to produce cranial endocasts for the lineage.
As the sister-group to all other caecilians (Pyron and Wiens, 2011), the Rhinatrematidae retain features lacking in other taxa (e.g., still possessing a tail), and as one of the most basal caecilians it may thus reasonably be expected to retain a more plesiomorphic condition of the brain and braincase. Indeed, the telencephalic hemispheres of Rhinatrema are elongate, remaining narrow along their whole length without the expansion posterolaterally as in other more derived caecilians such as Ichthyophis (Nieuwenhuys et al., 1998) and Typhlonectes (Schmidt et al., 1996; Striedter and Northcutt, 2020). In this aspect the telencephalon actually resembles those of several other sarcopterygians more so than other caecilians (Challands et al., 2020) and perhaps reflects the plesiomorphic condition. The braincase of a stem caecilian from the Jurassic Period, Eocaecilia, suggests that earliest members of this lineage retained a more robust skull than is found in more recent, derived taxa (Maddin et al., 2012a).
In contrast, as a member of the Caeciliidae, Gegeneophis is a more derived caecilian than Rhinatrema (Pyron and Wiens, 2011), and its brain shape, with posterolaterally-expanded telencephalic hemispheres, is more typical for the group. Being a blind animal, one might expect the optic tectum to be reduced in Gegeneophis but it appears to remain as prominent as in other taxa (Figure 1). Nevertheless, the entire morphology of caecilians is strongly modified in association with their ecology, and the close correspondence internally between their brain and braincase likely reflects morphological constraints related to their fossorial lifestyle.
Compared to caecilians, anurans are a much more diverse and well-studied group. The brains of frogs and toads have been considered relatively similar across taxa, although there is evidence of variation in brain morphology correlated with seasonality (Luo et al., 2017), habitat and locomotion (Taylor et al., 1995; Manzano et al., 2017). The brains of both Breviceps and Ceratophrys are both a similar size in proportion to their overall body size (brain length 20–22% of snout/vent length), but that of Breviceps fills its endocast to a greater degree than Ceratophrys, by almost 15%. While the olfactory canals are short in Breviceps, those in Ceratophrys extend far anteriorly before exiting the braincase, similar to the condition in some lungfishes (Protopterus spp., see Challands et al., 2020, Figure 6). Breviceps is a burrowing frog (Hofrichter, 2000), thus its close relationship between the brain and braincase may be related to its highly fossorial lifestyle, as we have hypothesized for caecilians also. However, of the six frog brain morphotypes shown in Manzano et al. (2017), we note that the brain of Breviceps is most similar in overall morphology to that of the “hopper-walker” Rhinella fernandezae, the Bella Vista Toad. On the other hand, Ceratophrys has a brain more similar to the waxy monkey tree frog, Phyllomedusa sauvagii, a “climber-walker,” or largely arboreal frog (Manzano et al., 2017). It is clear that further work is required to elucidate brain ecomorphology in the hyper diverse and specialized anurans.
While employing the full Extant Phylogenetic Bracket to reconstruct soft tissue in fossils is considered a relatively rigorous approach, the inclusion of particularly specialized or derived groups must only be done so with caution. One may consider that the lower limit of brain volume in the first tetrapods may be similar to the condition found in Latimeria, although we do not believe this to be the case. Extant coelacanths live in the deep ocean and retain an intracranial joint, while lungfish and tetrapods lost this feature early in their history. The enlargement of the notochord in Latimeria (Dutel et al., 2019) and the persistence of the intracranial joint both plausibly contribute to the large disparity between the brain and endocast in this taxon, where its expansion is most likely limited by both ecological and biomechanical constraints. Furthermore, it is known that fish living in the deep-sea and less complex environments tend to have smaller brains compared to their shallow water counterparts (Kotrschal, 1998).
Moreover, both caecilians and anurans are considered to be highly adapted to their respective modes of life as fossorial animals or specialized jumpers and vocalizers. Consideration of the ecology and habitat of the first tetrapods (Clack, 2012) lends support to the use of lungfishes and salamanders as the best extant representatives for interpreting the brain morphology in the earliest terrestrial vertebrates. The combination of data from the coelacanth, lungfish and now all three orders of extant amphibians herein demonstrates the importance of considering ecology and habit when using the EPB.
If the coelacanth, caecilians and frogs are used to estimate and constrain the brain volume of extinct stem and/or early tetrapods then we might expect a range of brain volumes of between 1–78% of the internal brain cavity/endocast. Such a range is hardly informative and using the EPB may perhaps more useful in constraining character states in extinct taxa. For example, we may infer the presence of elongate parallel telencephalic hemispheres in extinct early tetrapod taxa seeing as this condition is seen in the extant, yet basal, caecilian Rhinatrema.
Eliminating those taxa that possess characters not seen in early tetrapods (e.g., Latimeria due to the presence of an intracranial joint) and those taxa that are ecologically highly specialized (Gymnophiona and Anura) limits brain volume estimates to be restricted to those seen in extant Dipnoi and Caudata i.e., between 38–47%. That said, many early tetrapods were themselves highly specialized in terms of ecology and habit, for example the limbless Lethiscus (Pardo et al., 2017b) and the robust, possibly burrowing recumbirostran Brachydectes (Pardo and Anderson, 2016), and so it may be expected that in some taxa, the brain volume in these extreme cases may be more similar to different extant, but ecologically similar taxa. The emphasis here is on selecting a suitable EPB that reflects both phylogeny and ecology so as to obtain informed estimates of features in extinct taxa rather than using a wholesale approach.
In conclusion, we analyzed the correspondence between the brain and endocast in two anurans, B. poweri and C. ornata, and two caecilians, R. bivittatum and G. ramaswamii, using diceCT data. We found that these taxa had brains that filled their endocasts to a greater extent than that of extant salamanders and lungfishes, and infer that such disparity may be a product of their highly specialized ecology and habit. The telencephalon (forebrain) was the region of closest fit across all taxa. Ceratophrys differed from the other taxa in that it had considerable surface-surface distance surrounding the olfactory tracts and mesencephalon. Our findings help to constrain the minimum and maximum expected brain volumes for extinct tetrapods employing an Extant Phylogenetic Bracket approach. However, we emphasize the need to make an informed choice for the EPB and not to assume inferences can be made from the phylogenetic position of the EPB taxa alone, but also to consider ecology, habit and lifestyle. Lastly, we have created the first virtual cranial endocasts for frogs and caecilians and envisage that these will be useful for future analyses of endocast shape in amphibians.
The original contributions presented in the study are included in the article/Supplementary Materials, further inquiries can be directed to the corresponding author/s.
Ethical review and approval was not required for the animal study because data already existed freely available online (we did not deal with the animals scanned ourselves, the data was from previous studies).
AC conceived and designed the study. AC and CM performed the 3D segmentation and wrote the first draft of the manuscript. TC analyzed the data. AC and TC created figures and Supplementary Data. SC, TC, and JL inputted to the writing of the manuscript. JL provided materials and software. All authors contributed to the interpretation of results, editing of the manuscript, and have approved this final version for re-submission.
AC, SC, CM, and JL are supported by funding from Australian Research Council grant DP 200103398. TC was supported by Callidus Services Ltd., United Kingdom.
The authors declare that the research was conducted in the absence of any commercial or financial relationships that could be construed as a potential conflict of interest.
We would like to thank Thomas Kleinteich for use of the Ceratophrys dataset and Hugo Dutel for supplying STL’s of Latimeria for use in Figure 3. We would also like to thank Catherine Early and David Blackburn for bringing my attention to caecilian scan data available on MorphoSource (https://www.morphosource.org/). Animal silhouettes displayed in figures are copyright-free images courtesy of PhyloPic (http://www.phylopic.org/): Latimeria, Rhinatrema, Yan Wong; Neoceratodus, Roberto Díaz Sibaja; Protopterus, Ceratophrys, Steven Traver; Ambystoma, Jake Warner; Cynops, Neil Kelley; and Breviceps, under CC BY 3.0 from Nobu Tamura at http://phylopic.org/image/a3b917bc-142d-4314-aa82-2215f5487daf/. We would also like to thank two reviewers and Guest Associate Editor Ingmar Werneburg for their criticisms that improved an earlier draft of this manuscript.
The Supplementary Material for this article can be found online at: https://www.frontiersin.org/articles/10.3389/fevo.2021.640345/full#supplementary-material
Amemiya, C. T., Alfoldi, J., Lee, A. P., Fan, S. H., Philippe, H., MacCallum, I., et al. (2013). The African coelacanth genome provides insights into tetrapod evolution. Nature 496, 311–316. doi: 10.1038/Nature12027
Anderson, J. S., Reisz, R. R., Scott, D., Frobisch, N. B., and Sumida, S. S. (2008). A stem batrachian from the Early Permian of Texas and the origin of frogs and salamanders. Nature 453, 515–518. doi: 10.1038/nature06865
Challands, T. J., Pardo, J. D., and Clement, A. M. (2020). Mandibular musculature constrains brain-endocast disparity between sarcopterygians. R. Soc. Open Sci. 7, 1–20.
Clack, J. A. (2012). Gaining Ground: The Origin and Evolution of Tetrapods. Bloomington, IN: Indiana University Press.
Clack, J. A., Ahlberg, P. E., Finney, S. M., Dominguez Alonso, P., Robinson, J., and Ketcham, R. A. (2003). A uniquely specialized ear in a very early tetrapod. Nature 425, 65–69. doi: 10.1038/nature01904
Clement, A. M., Nysjö, J., Strand, R., and Ahlberg, P. E. (2015). Brain – endocast relationship in the Australian lungfish, Neoceratodus forsteri, elucidated from tomographic data (Sarcopterygii: Dipnoi). PLoS One 10:e0141277. doi: 10.1371/journal.pone.0141277
Clement, A. M., Strand, R., Nysjö, J., Long, J. A., and Ahlberg, P. E. (2016). A new method for reconstructing brain morphology: applying the brain-neurocranial spatial relationship in an extant lungfish to a fossil endocast. R. Soc. Open Sci. 3:160307. doi: 10.1098/rsos.160307
Cloutier, R., Clement, A. M., Lee, M. S. Y., Noël, R., Béchard, I., Roy, V., et al. (2020). Elpistostege and the origin of the vertebrate hand. Nature 579, 549–554. doi: 10.1038/s41586-020-2100-8
Dempster, W. T. (1935). The brain case and endocranial cast of Eryops megacephalus (Cope). J. Comp. Neurol. 62, 171–196. doi: 10.1002/cne.900620108
Dutel, H., Galland, M., Tafforeau, P., Long, J. A., Fagan, M. J., Janvier, P., et al. (2019). Neurocranial development of the coelacanth and the evolution of the sarcopterygian head. Nature doi: 10.1038/s41586-019-1117-3
Holland, T. (2014). The endocranial anatomy of Gogonasus andrewsae Long, 1985 revealed through micro CT-scanning. Earth Environ. Sci. Trans. R. Soc. Edinb. 105, 9–34. doi: 10.1017/s1755691014000164
Iwaniuk, A. N. (2017). “The evolution of cognitive brains in non-mammals,” in Evolution of the Brain, Cognition, and Emotion in Vertebrates, eds S. Watanabe, M. A. Hofman, and T. Shimizu (Berlin: Springer), 101–124. doi: 10.1007/978-4-431-56559-8_5
Kleinteich, T., and Gorb, S. N. (2015). Frog tongue acts as muscle-powered adhesive tape. R. Soc. Open Sci. 2:150333. doi: 10.1098/rsos.150333
Kleinteich, T., and Haas, A. (2007). Cranial musculature in the larva of the caecilian, Ichthyophis kohtaoensis (Lissamphibia: Gymnophiona). J. Morphol. 268, 74–88. doi: 10.1002/jmor.10503
Kotrschal, K. (1998). Fish brains: evolution and environmental relationships. Rev. Fish Biol. Fish. 8, 373–408.
Lu, J., Zhu, M., Long, J. A., Zhao, W., Senden, T. J., Jia, L. T., et al. (2012). The earliest known stem-tetrapod from the Lower Devonian of China. Nat. Commun. 3:1160. doi: 10.1038/ncomms2170
Luo, Y., Zhong, M. J., Huang, Y., Li, F., Liao, W. B., and Kotrschal, A. (2017). Seasonality and brain size are negatively associated in frogs: evidence for the expensive brain framework. Sci. Rep. 7:16629.
Maddin, H. C., and Anderson, J. S. (2012). Evolution of the amphibian ear with implications for lissamphibian phylogeny: insight gained from the caecilian inner ear. Fieldiana Life Earth Sci. 76, 59–77. doi: 10.3158/2158-5520-5.1.59
Maddin, H. C., Jenkins, F. A. J., and Anderson, J. S. (2012a). The braincase of Eocaecilia micropodia (Lissamphibia, Gymnophiona) and the origin of caecilians. PLoS One 7:e50743. doi: 10.1371/journal.pone.0050743
Maddin, H. C., Russell, A. P., and Anderson, J. S. (2012b). Phylogenetic implications of the morphology of the braincase of caecilian amphibians (Gymnophiona). Zool. J. Linn. Soc. 166, 160–201.
Maddin, H. C., and Sherratt, E. (2014). Influence of fossoriality on inner ear morphology: insights from caecilian amphibians. J. Anat. 225, 83–93. doi: 10.1111/joa.12190
Manzano, A. S., Herrel, A., Fabre, A. C., and Abdala, V. (2017). Variation in brain anatomy in frogs and its possible bearing on their locomotor ecology. J. Anat. 231, 38–58. doi: 10.1111/joa.12613
Marjanovic, D., and Laurin, M. (2007). Fossils, molecules, divergence times, and the origin of lissamphibians. Syst. Biol. 56, 369–388. doi: 10.1080/10635150701397635
Marshall, A. F., Bardua, C., Gower, D. J., Wilkinson, M., Sherratt, E., and Goswami, A. (2019). High-density three-dimensional morphometric analyses support conserved static (intraspecific) modularity in caecilian (Amphibia: Gymnophiona) crania. Biol. J. Linn. Soc. 126, 721–742. doi: 10.1093/biolinnean/blz001
Millot, J., and Anthony, J. (1965). Anatomie de Latimeria Chalumnae: Système Nerveux et Organes des sens. Centre National de la Recherche Scientifique. Paris: Centre National de la Recherche Scientifique.
Müller, H., Wilkinson, M., Loader, S. P., Wirkner, C. S., and Gower, D. J. (2009). Morphology and function of the head in foetal and juvenile Scolecomorphus kirkii (Amphibia: Gymnophiona: Scolecomorphidae). Biol. J. Linn. Soc. 96, 491–504. doi: 10.1111/j.1095-8312.2008.01152.x
Nieuwenhuys, R., Donkelaar, H. J., and Nicholson, C. (1998). The Central Nervous System of Vertebrates. Berlin: Springer.
Pardo, J. D., and Anderson, J. S. (2016). Cranial morphology of the Carboniferous-Permian tetrapod Brachydectes newberryi (Lepospondyli, Lysorophia): new data from μCT. PLoS One 11:1–34.
Pardo, J. D., Small, B. J., and Huttenlocker, A. K. (2017a). Stem caecilian from from the Triassic of Colorado sheds light on the origins of Lissamphibia. Proc. Natl. Acad. Sci. U. S. A. 114, E5389–E5395. doi: 10.1073/pnas.1706752114
Pardo, J. D., Szostakiwskyj, M., Ahlberg, P. E., and Anderson, J. S. (2017b). Hidden morphological diversity among early tetrapods. Nature 546, 642–645. doi: 10.1038/nature22966
Pradel, A., Langer, M., Maisey, J. G., Geffard-Kuriyama, D., Cloetens, P., Janvier, P., et al. (2009). Skull and brain of a 300-million-year-old chimaeroid fish revealed by synchrotron holotomography. Proc. Natl. Acad. Sci. U. S. A. 106, 5224–5228. doi: 10.1073/pnas.0807047106
Pyron, R. A., and Wiens, J. J. (2011). A large-scale phylogeny of Amphibia including over 2800 species, and a revised classification of extant frogs, salamanders, and caecilians. Mol. Phylogenet. Evol. 61, 543–583. doi: 10.1016/j.ympev.2011.06.012
Robinson, J., Ahlberg, P. E., and Koentges, G. (2005). The braincase and middle ear region of Dendrerpeton acadianum (Tetrapoda: Temnospondyli). Zool. J. Linn. Soc. 143, 577–597. doi: 10.1111/j.1096-3642.2005.00156.x
Romer, A. S. (1937). The braincase of the Carboniferous Crossopterygian Megalichthys nitidus. Bull. Mus. Comp. Zool. 132, 1–73. doi: 10.1007/978-3-319-23534-9_1
Romer, A. S., and Edinger, T. (1942). Endocranial casts and brains of living and fossil amphibia. J. Comp. Neurol. 77, 355–389. doi: 10.1002/cne.900770203
Ruta, M., and Coates, M. I. (2007). Dates, nodes and character conflict: addressing the lissamphibian origin problem. J. Syst. Palaeontol. 5, 69–122. doi: 10.1017/s1477201906002008
Schmidt, A., Wake, D. B., and Wake, M. H. (1996). Motor nuclei of nerves innervating the tongue and hypoglossal musculature in a caecilian (Amphibia: Gymnophiona), as revealed by HRP transport. J. Comp. Neurol. 370, 342–349. doi: 10.1002/(sici)1096-9861(19960701)370:3<342::aid-cne5>3.0.co;2-4
Sherratt, E., Gower, D. J., Klingenberg, C. P., and Wilkinson, M. (2014). Evolution of cranial shape in Caecilians (Amphibia: Gymnophiona). Evol. Biol. 41, 528–545. doi: 10.1007/s11692-014-9287-2
Snitting, D. (2008). A redescription of the anatomy of the Late Devonian Spodichthys buetleri Jarvik, 1985 (Sarcopterygii, Tetrapodomorpha) from East Greenland. J. Vertebr. Paleontol. 28, 637–655. doi: 10.1671/0272-4634(2008)28[637:arotao]2.0.co;2
Stensiö, E. (1963). The Brain and the Cranial Nerves in Fossil, Lower Craniate Vertebrates. Oslo: Skrifter utgitt av Det Norske Videnskaps-Akademi, 1–120.
Striedter, G. F., and Northcutt, R. G. (2020). Brains Through Time: A Natural History of Vertebrates. New York, NY: Oxford University Press.
Taylor, G. M., Nol, E., and Boire, D. (1995). Brain regions and encephalization in anurans: adaptation or stability? Brain Behav. Evol. 45, 96–109. doi: 10.1159/000113543
Whiteaves, J. F. (1883). Recent discoveries of fossil fishes in the Devonian rocks of Canada. Am. Nat. 17, 158–164. doi: 10.1086/273271
Witmer, L. M. (1995). “The extant phylogenetic bracket and the importance of reconstructing soft tissues in fossils,” in Functional Morphology in Vertebrate Palaeontology, ed. J. Thomason (Cambridge: Cambridge University Press), 19–33.
Keywords: Sarcopterygii, Anura, Gymnophiona, neurobiology, brain, endocast, diceCT
Citation: Clement AM, Mensforth CL, Challands TJ, Collin SP and Long JA (2021) Brain Reconstruction Across the Fish-Tetrapod Transition; Insights From Modern Amphibians. Front. Ecol. Evol. 9:640345. doi: 10.3389/fevo.2021.640345
Received: 11 December 2020; Accepted: 25 February 2021;
Published: 19 March 2021.
Edited by:
Ingmar Werneburg, University of Tübingen, GermanyReviewed by:
Min Zhu, Institute of Vertebrate Paleontology and Paleoanthropology, Chinese Academy of Sciences, ChinaCopyright © 2021 Clement, Mensforth, Challands, Collin and Long. This is an open-access article distributed under the terms of the Creative Commons Attribution License (CC BY). The use, distribution or reproduction in other forums is permitted, provided the original author(s) and the copyright owner(s) are credited and that the original publication in this journal is cited, in accordance with accepted academic practice. No use, distribution or reproduction is permitted which does not comply with these terms.
*Correspondence: Alice M. Clement, YWxpY2UuY2xlbWVudEBmbGluZGVycy5lZHUuYXU=
Disclaimer: All claims expressed in this article are solely those of the authors and do not necessarily represent those of their affiliated organizations, or those of the publisher, the editors and the reviewers. Any product that may be evaluated in this article or claim that may be made by its manufacturer is not guaranteed or endorsed by the publisher.
Research integrity at Frontiers
Learn more about the work of our research integrity team to safeguard the quality of each article we publish.