- Department of Animal Ecology, Evolution and Biodiversity, Ruhr-University Bochum, Bochum, Germany
Prey species can respond to the presence of predators by inducing phenotypic plastic traits which form morphological, life history or behavioral defenses. These so-called inducible defenses have evolved within a cost-benefit framework. They are only formed when they are needed, and costs associated with defenses are saved when predators are not present. However, a disadvantage compared to permanent defenses are lag phases between predator perception and the full formation of defenses. This may be especially important when the predation risk persists for longer periods, e.g., outlasts one generation and challenges prey offspring. We hypothesized that transgenerational induced phenotypic plasticity reduces lag phases in situations where hazards threaten specimens over several generations. We tested this in three generations of the freshwater crustacean Daphnia lumholtzi using the three-spined stickleback Gasterosteus aculeatus as predator. In the presence of chemical cues from fish D. lumholtzi expresses elongated head and tail spines. In the F0 generation defenses are constraint by a comparatively long lag phase and are not developed prior to the 3rd instar. In the F1, and F2 of induced animals this lag phase is shortened and defenses are developed upon birth. We show that induction of TGP in the mothers takes place already during the juvenile stages and transfers to the offspring generation in forms of shortened time lags and enhanced trait expression. When progeny is additionally exposed to fish cues as embryos, the addition of maternal and embryonic effects further enhances the magnitude of defense expression. Our findings detail a distinguished strategy of transgenerational phenotypic plasticity which allows to shorten lag phases of trait changes in phenotypic plasticity.
Introduction
In environments with fluctuating conditions, dedicated mechanisms that allow fast phenotypic adaptation may be crucial to improve organismal fitness (Auld et al., 2010; Holeski et al., 2012; Shama et al., 2014; Hendry, 2016; Luquet and Tariel, 2016). One such mechanism is phenotypic plasticity, which is defined as the ability of an organism with a given genotype to respond to environmental changes with an adapted phenotype (Bradshaw, 1965; Whitman and Agrawal, 2009). Very often the occurrence of phenotypic plasticity is described within one generation (within generational plasticity WGP; Ezard et al., 2014; English et al., 2015; Auge et al., 2017), but when environmental hazards are long-lasting, offspring performance can be enhanced if they are being prepared by the parents (Agrawal et al., 1999; Uller, 2008). This kind of transgenerational plasticity (TGP) is discussed to be enabled via epigenetic, cytoplasmic, somatic, nutritional and behavioral modifications from parents to offspring (Bonduriansky and Day, 2009; Harris et al., 2012).
Both, the length and the timing of the environmental cue can be decisional for transgenerational responses in the offspring (Donelson et al., 2018). A prolonged exposure to environmental cues can increase the transgenerational effect (Donelson et al., 2018). Timing is crucial as TGP may be limited to early life exposure with critical developmental windows for cue sensitivity (Hanson and Skinner, 2016; Sentis et al., 2018).
TGP has been observed in animals and plants (Mousseau and Fox, 1998; Agrawal et al., 1999) as a response to e.g., biotic stressors (Mousseau and Dingle, 1991; Kumar et al., 2015). One significant biotic stressor is predation, which frequently induces plasticity in forms of inducible defenses in prey (Tollrian and Harvell, 1999). The predation risk is often indicated by chemical cues so-called kairomones, that are unintentionally released by the predator (Dodson and Hanazato, 1995; Weiss et al., 2018). Prey species can perceive these kairomones and thereupon develop defenses ranging from behavioral, via life history, to morphological adaptations (Tollrian and Dodson, 1999; Weiss, 2019). The freshwater crustacean Daphnia is a prime example for developing such defenses. Daphnia pulex, e.g., expresses neckteeth (Krueger and Dodson, 1981; Tollrian, 1993) and D. ambigua expresses helmets (Hebert and Grewe, 1985) in the presence of the phantom midge larva Chaoborus spec. D. atkinsoni develops a crown of thorns (Petrusek et al., 2009) and Daphnia magna grows large and bulky under predation pressure of the tadpole shrimp Triops spec. (Rabus and Laforsch, 2011; Horstmann et al., 2018), and D. longicephala develops huge crests in the head region when exposed to the backswimmer Notonecta spec. (Grant and Bayly, 1981).
Inducible defenses should incur costs, as otherwise they likely would become permanent. These costs can be of different origin, so that they can stem from the organisms’ ability of being plastic per se. In this case, costs, e.g., result from the maintenance of a genetic and physiological architecture to detect and adapt to predation cues. Other costs, like allocation costs, can derive from an increased energy and material demands required for the formation of the defenses. Environmental costs or external costs result from e.g., changes in swimming speed due to aberrant hydrodynamics of the defended morphotype (reviewed in Weiss and Tollrian, 2018a, b).
Besides these costs, the expression of these defenses underlies constraints. There are e.g., time lags that result from the time needed after predator perception until defense formation (Weiss and Tollrian, 2018a, b). In Daphnia such time lags often require at least a complete instar and are dependent on the time needed for signal processing and the change of developmental trajectories to result in adaptive morphotype expression (Weiss, 2019). Furthermore, there are developmental windows in which defenses can be expressed, so that D. pulex only express neckteeth in the early juvenile stages (Imai et al., 2009; Weiss et al., 2016), and D. longicephala express crests only in later developmental stages (Grant and Bayly, 1981; Weiss et al., 2015). Also the degree of defense expression is subject to constraints, as a larger defensive trait requires a longer time until being developed (Miner et al., 2005). One way to minimize constraints may be the shortening of time lags prior to defense expression. In line with the shortening of the time lags, the earlier onset of defense expression also a allows a stronger defense expression within a shorter time frame. Thereby defense expression can be further optimized to the predation risk. Here TGP could serve as a tool which may be especially effective when the parental environment is a proxy of the offspring’s environment. This is for example the case when the predation risk overarches several generation cycles of the prey. Daphnia are often being preyed on by juvenile fish that have a comparatively long juvenile phase. D. lumholtzi is one of the few Daphnia species that develops morphological defenses against fish predation (Engel and Tollrian, 2009; Engel et al., 2014). A transgenerational induction of inducible defenses could further improve the cost-benefit relationship where the benefit of stronger defense expression outweighs the costs. We hypothesized that transgenerational plasticity affects (a) time lags and (b) strength of defense expression. Furthermore, we wanted to elucidate the point in time critical for the induction of TGP.
Materials and Methods
Animal Culture
D. lumholtzi clone TE (Fairfield Reservoir, Texas, United States, kindly provided by R. Sterner) was cultured in artificial Daphnia medium (ADaM; Klüttgen et al., 1994) in 1 L beakers (Weck®, Germany) containing 40–50 age-synchronized individuals under 16:8 h day:night cycle at 20°C ± 1°C. Animals were fed with unlimited food conditions (1.5 g C/L) with the algae Autodesmus obliquus. The beakers were cleaned every 48 h to remove exuviae and excess algae. Half of the medium was exchanged weekly.
Kairomone Preparation
Fish kairomone was prepared using three-spined sticklebacks (Gasterosteus aculeatus). Fish were not harmed and kept under conditions complying with animal care and welfare. A maximum of 20 fish no larger than 5 cm (body length) were kept in an 80 L glass tank at 15 ± 1°C under constant 12:12 h day:night cycle. Animals were fed ad libitum every 24 h with Chironomus larvae (Amtra, Germany). To produce kairomone enriched medium two fish (size 4–5 cm body length) were transferred into 1 L ADaM for 24 h. Subsequently, fish were removed and the water containing the kairomone was filtered (45 μm GF/C Whatman filter). To prevent bacterial degradation of the kairomone, ampicillin (10 mg/L) (Sigma Aldrich, Germany) was added as reported by Weiss et al. (2012). Kairomone was frozen at −20°C and thawed prior to use. The stock concentration of 2 fish/L was diluted to 0.2 fish/L in the bioassay. The diluted kairomone contained 5 mg/L of ampicillin. Even though ampicillin does not impact Daphnia development (Weiss et al., 2012), the same concentration of 5 mg/L was added to the medium of the control conditions.
Experimental Treatments
In order to determine transgenerational phenotypic plasticity in D. lumholtzi, we performed an experiment in which predator naïve 1st instar juvenile D. lumholtzi were exposed to four treatments (Figure 1). We performed 1.) a control treatment without predator exposure, and 2.) permanent predator exposure. With these treatments we wanted to test if D. lumholtzi performed TGP, and if so in what way. Therefore, we monitored these animals in the first through third juvenile instars in the F0, F1, and F2 generation. With treatment 3.) and 4.), we wanted to disentangle if induction of TGP occurs already in the mother, or if it occurs in the embryos. For that, in treatment 3.) we only exposed the mothers to kairomone. Here, we ensured that embryos did not get in contact with kairomone by removing mothers from kairomone in the 4th juvenile instar (one instar prior to ovulation) and rinsed them 3∗10 min in ADaM to remove residual kairomone transferring them into control conditions until second and third clutch animals were released from the brood pouch. Neonates were then again exposed to kairomones. Consequently, in treatment 4.) we only exposed the embryos developing in naïve mothers to predator cues. For that the females were kept at control conditions until they ovulated. Subsequently, animals were exposed to kairomone until the neonates were released from the mothers’ brood pouch. In treatment 3.) and 4.) we investigated defense expression in the first through third juvenile instars. All specimens of the different treatments were reared individually in 50 mL snap cap vials containing, either ADaM or kairomone enriched medium containing 20 mL medium. ADaM and kairomone enriched medium was refreshed every 48 h to ensure constant experimental conditions. The experiment was started with 5 animals per treatment group. This whole set-up was replicated 10 times.
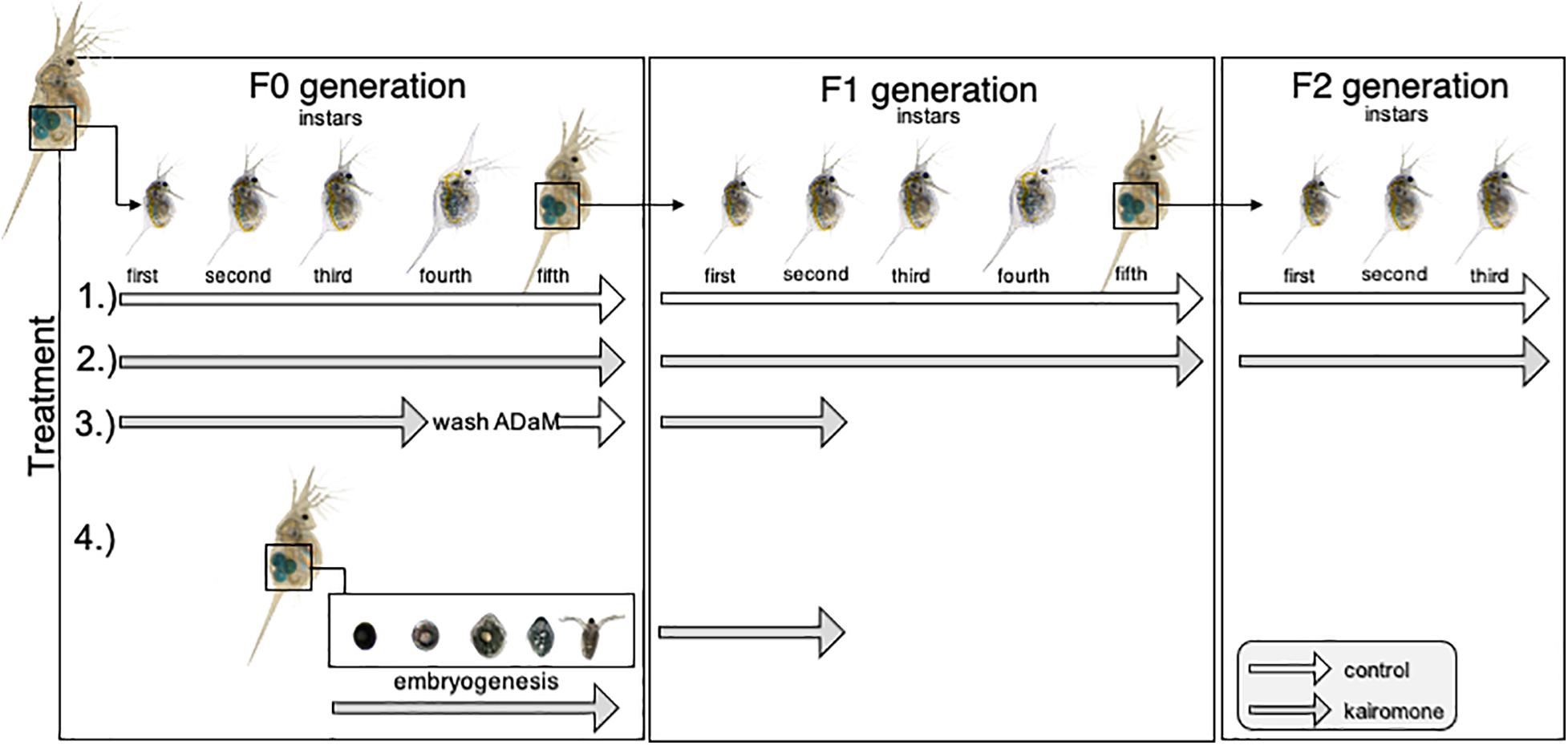
Figure 1. Schematic display of kairomone exposure. 1.) In the control treatment, animals were never exposed to kairomones throughout the whole experiment. 2.) In the permanent kairomone exposure treatment individuals were permanently exposed to kairomones across all three generations. 3.) In the maternal kairomone exposure treatment animals were exposed to kairomone from the 1st until the 4th instar, then washed to remove residual kairomone, and transferred to kairomone-free medium. The 1st clutch was discarded and after being release from the brood pouch the 2nd and 3rd clutch were again exposed to fish kairomone. 4.) In the embryonic kairomone exposure treatment embryos in the mother’s brood pouch were exposed to fish kairomone from ovulation until being released from the brood pouch and continuously exposed until they reached the 2nd juvenile instar. Treatments 3.) and 4.) were raised until the second juvenile instar in the F1 generation.
Data Acquisition
All animals were monitored, and digital images were acquired to measure defense expression.
We used a stereomicroscope (SZX 16 Olympus; Germany) equipped with a digital camera (ColorView III, SIS Imaging Solutions Olympus; Germany) in combination with the analysis software Cell^D (SIS Soft Imaging System Solutions, Olympus Germany). We determined the Daphnias’ head spine length from the tip of the head spine to the upper margin of the compound eye).
Head Spine Growth
We determined the absolute head spine growth per generation by calculating the differences of head spine length between the 3rd and 1st juvenile instar per individual animal in each generation.
Statistics
Based on a Shapiro-Wilk test, all data followed a normal distribution so that we tested for differences between treatments using a factorial ANOVA, followed by a Bonferroni post hoc test. We compared head spine lengths of animals obtained from the 2nd and 3rd clutch from the permanent predator exposure across the three generations in the 1st juvenile instar. Animals of the 2nd and 3rd clutch were pooled into one treatment as we did not detect clutch specific differences (again using an ANOVA). Additionally, we compared them with head spine lengths of the control treatment within each generation throughout the first three juvenile instars. Head spine lengths of maternal and embryonic predator exposure were compared to each other and the F1 control. Furthermore, we compared the increase in head spine length from the 1st to the 3rd juvenile instar across generations in the permanent predator exposure. All statistical analyses were performed with Statistica 14 (Statsoft Inc.).
Results
In the F0 generation the continuous exposure to fish kairomones, significantly induced expression of morphological defenses in forms of elongated head spines in the 3rd juvenile instar. Defended D. lumholtzi have a median head spine length of 222.17 μm while undefended specimens have a median head spine length of 162.25 μm. In the earlier juvenile instars defenses are not expressed and predator exposed D. lumholtzi show a median head spine length of 160.63 μm in the first and 167.87 μm in the 2nd juvenile instar (Figure 2A and Supplementary Tables 1, 2). In the 1st juvenile instar of the F1 and F2 generation medium head spine length reaches 190.75 and 201.64 μm in predator exposed D. lumholtzi. This is significantly larger than the head spine length of control D. lumholtzi of the equivalent generations (median 166.25 and 163.85 μm (Figures 2B,C and Supplementary Tables 3, 4).
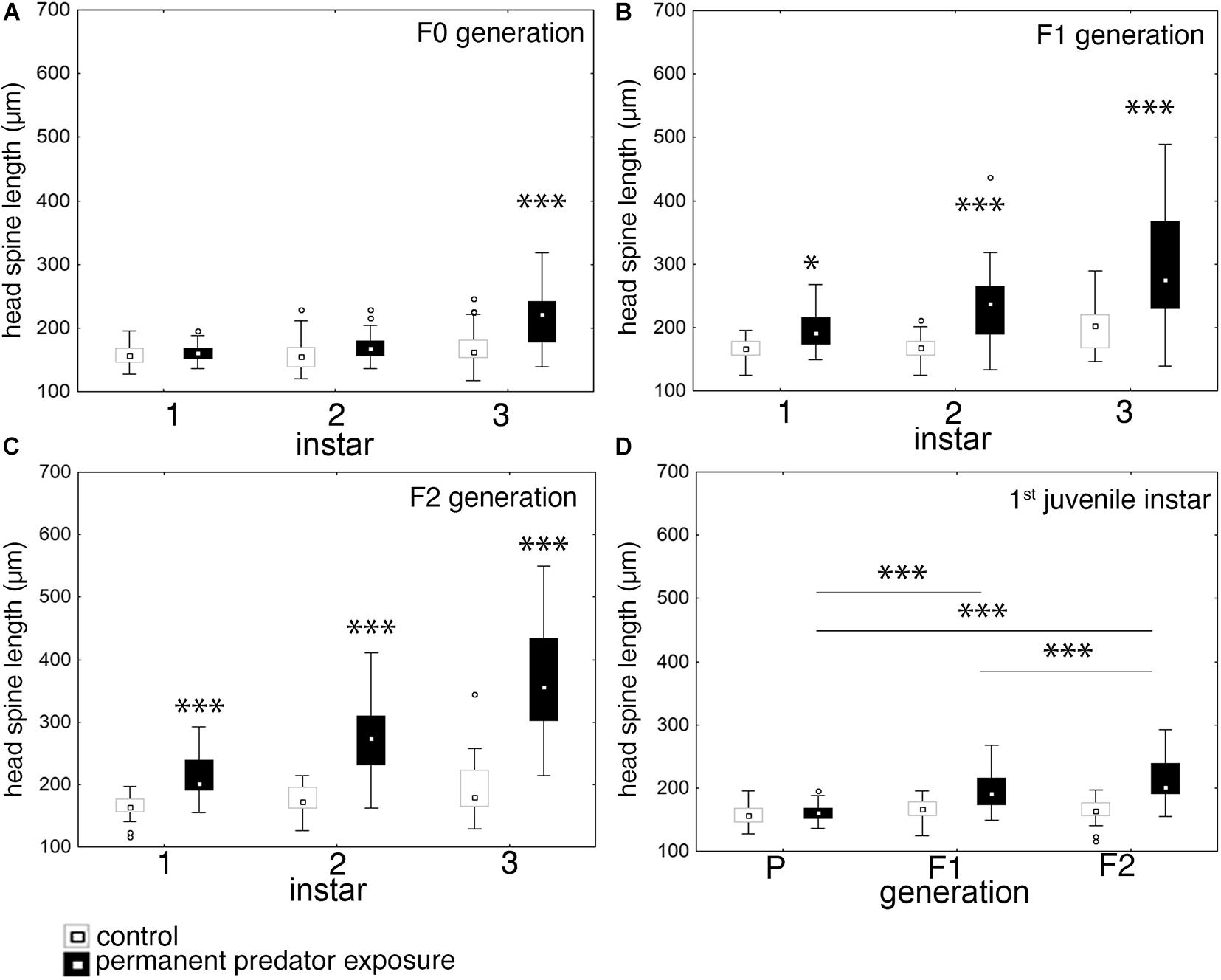
Figure 2. Effect of permanent predator exposure on head spine length in D. lumholtzi. (A) F0 generation: head spine length in the first three juvenile instars in control and permanently fish exposed D. lumholtzi; ANOVA: F(2, 203) = 11.466, P ≤ 0.001. (B) F1 generation: head spine length in the first three juvenile instars in control and permanently fish exposed D. lumholtzi; ANOVA: F(2, 237) = 10.064, P ≤ 0.001. (C) F2 generation: head spine length in the first three juvenile instars in control and permanently fish exposed D. lumholtzi; ANOVA: F(2, 199) = 25.83, P ≤ 0.001. (D) Head spine length in the first juvenile instar in control and permanently fish exposed D. lumholtzi across the P, F1, and F2 generation. ANOVA: F(2, 249) = 17.77, P ≤ 0.001. Displayed are medians (square), midspread 50% (box) and the 25th percentile (lower whisker); 75th percentile (upper whisker), with outliers (circles). ∗P ≤ 0.05; ∗∗∗P ≤ 0.001. Bonferroni post hoc analysis in Supplementary Tables 1–6.
When comparing the strength of defense expression across generations, we find that defenses increase from the F0 to the F2 generation. Head spine lengths in the first juvenile instar are significantly larger in the F1 (190.74 μm) and F2 (201.64 μm) in comparison to the F0 (160.38 μm) generation (Figure 2D and Supplementary Tables 5, 6). Also, between the F1 and F2 generation head spines are significantly different (Figure 2D and Supplementary Tables 5, 6). This also holds true in the second and third juvenile instar.
Head Spine Growth Rate
We determined the head spine growth, by calculating the absolute increase in head spine length between the 3rd and the 1st juvenile instar. We observed an increase in absolute head spine length across all generations. In the F1 generation, head spine growth is significantly larger than in the F0 generations. In the F2 generation head spine growth significantly exceeds this increase (Figure 3 and Supplementary Tables 7, 8).
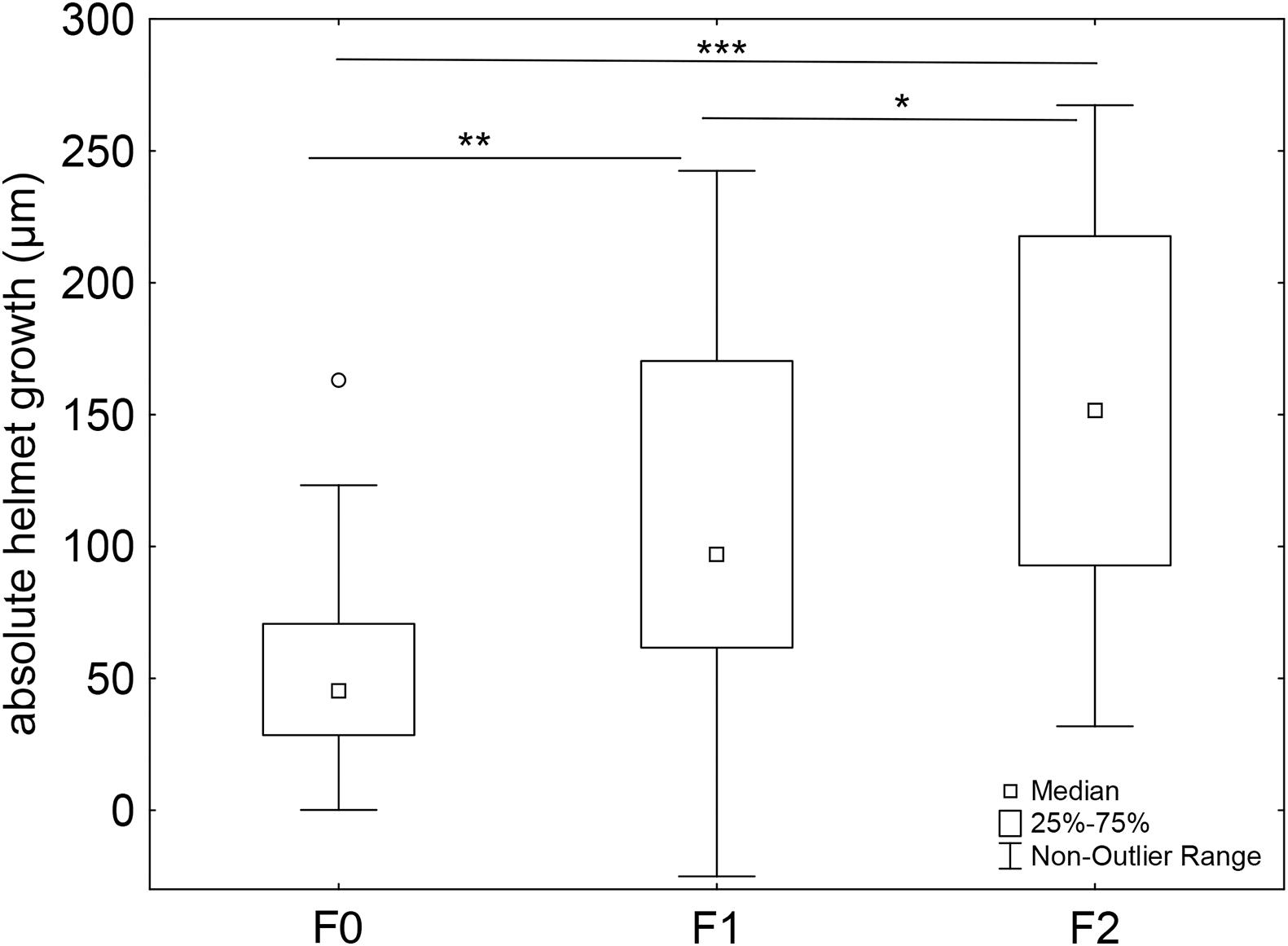
Figure 3. Absolute head spine growth across generations. The proportional increase in head spine length from the 1st to the 3rd juvenile instar increases across generations when D. lumholtzi is permanently exposed to kairomones. F0 generation: n = 29, F1 generation: n = 37, F2 generation: n = 35. F(2, 98) = 11.008, P ≤ 0.001. Bonferroni post hoc comparison. Displayed are medians (square), midspread 50% (box) and the 25th percentile (lower whisker); 75th percentile (upper whisker), with outliers (circles). *P ≤ 0.05; **P ≤ 0.01; ***P ≤ 0.001. Bonferroni post hoc analysis in Supplementary Tables 7, 8.
Maternal vs. Embryonic Predator Exposure
We aimed to determine if this earlier onset and enhancement of defense expression is due to effects of fish kairomones in the maternal environment, the embryonic environment or both. Naïve offspring and animals that were exposed to predator cues during embryogenesis do not show the expression of defenses in the first two juvenile instars. When mothers were exposed to kairomones during the first four juvenile instars, their offspring develop defenses already in the 2nd juvenile instar, when exposed to kairomones upon birth. This effect is intensified, when both the maternal and embryonic environment experienced kairomones (Figure 4 and Supplementary Tables 9, 10).
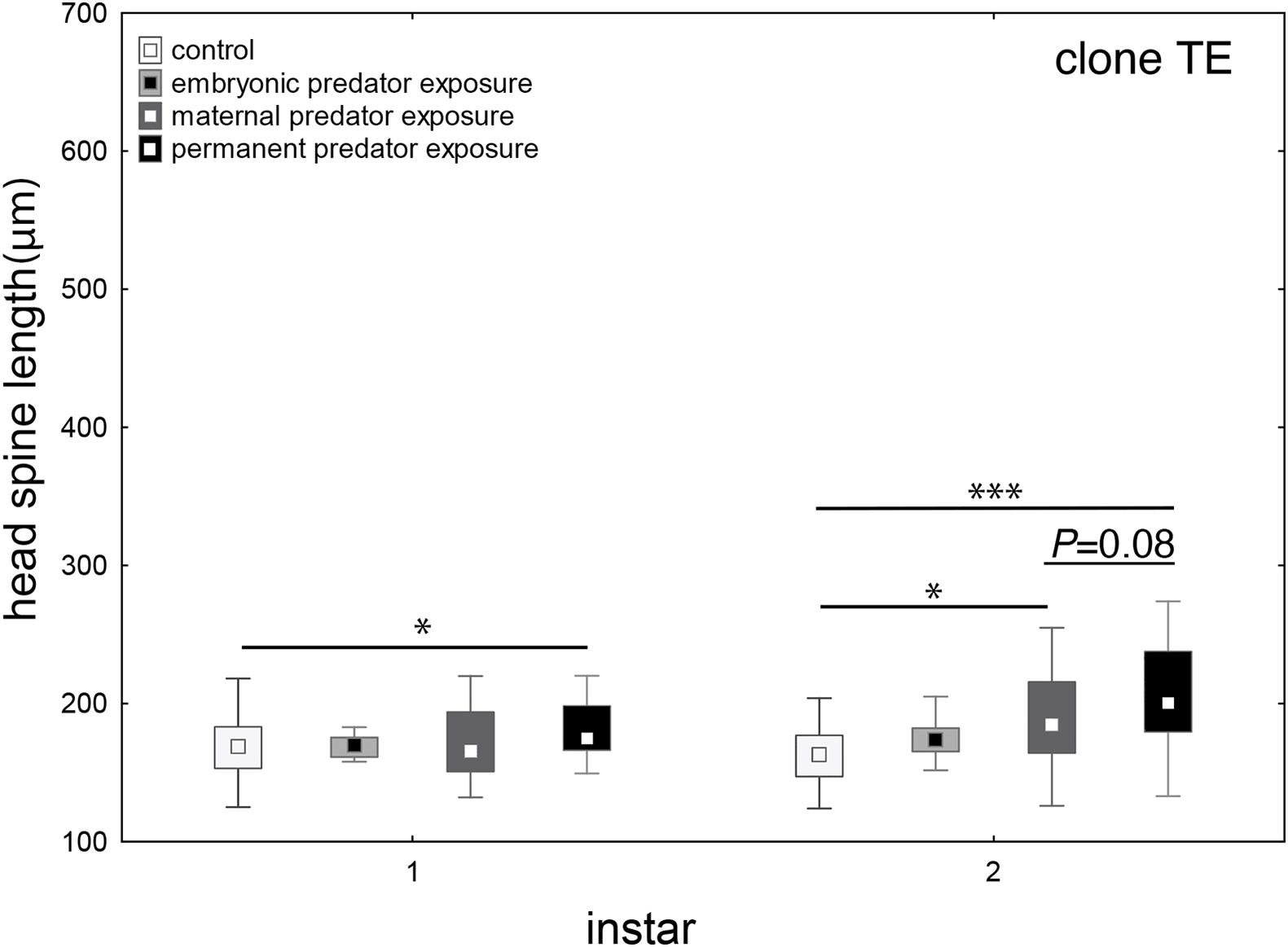
Figure 4. Maternal vs. embryonic predator exposure. When D. lumholtzi embryos are exposed to fish kairomones, defenses are not developed in the first two juvenile instars. When the mothers are exposed to kairomones, head spines in the offspring are significantly larger in the 2nd juvenile instar. When D. lumholtzi is permanently exposed to kairomone, i.e., the mothers and the embryos, head spines of the offspring is significantly increased already in the 1st juvenile instar. Displayed are medians (square), midspread 50% (box) and the 25th percentile (lower whisker); 75th percentile (upper whisker), with outliers (circles). ∗P ≤ 0.05; ∗∗∗P ≤ 0.001. ANOVA and Bonferroni post hoc analysis in Supplementary Tables 9, 10.
Discussion
Within- and transgenerational phenotypic plasticity are relevant mechanisms controlling adaptive responses to environmental changes (Donelson et al., 2018). WGP is selected when spatially and/or temporally heterogeneous cues indicate a predation risk, and costs are associated (Tollrian and Harvell, 1999; Walsh et al., 2016). If the environment experienced by the parents, serves as a proxy of the environment of the offspring, then TGP is expected to evolve (Luquet and Tariel, 2016). This has e.g., been reported in fish exposed D. ambigua (Walsh et al., 2016). Here, local adaptations to distinctive fluctuations in the density of the fish population were observed. If the fish population is consistently large, then TGP is selected, while WGP is favored when the fish population is changing more frequently (Walsh et al., 2016). WGP and TGP has also been reported in Chaoborus-exposed D. cucullata (Agrawal et al., 1999). D. cucullata express WGP in form of helmets, when exposed to predator cues short term. When the predator exposure covers generations, the offspring of Chaoborus-exposed mothers express significantly larger defenses than the offspring of non-exposed mothers (Agrawal et al., 1999).
Similarly, D. lumholtzi that are exposed to predator cues from birth display WGP in form of head spines from the 3rd juvenile instar onward. We observed TGP when D. lumholtzi is permanently exposed to fish kairomones. Then the daughter generations expressed significantly larger head spines than the F0 generation. Already juveniles in the first instar of the F1 and F2 show significantly larger head spines than their parents and grandparents. This increased growth is enhanced in the subsequent instars, so that also the 2nd and the 3rd juvenile instar show significantly larger head spines than the F0 generation. In the F0 generation, fish exposed D. lumholtzi (from fish naïve mothers) expressed increased head spines not before the 3rd juvenile instar. This means that without TGP there is a comparatively long lag phase, i.e., a reaction delay associated with defense expression if parents are in contact with kairomones. The offspring of these animals, however, express their defenses already upon birth. Consequently, the lag phase that initially comprised two molting cycles in the F0 generation is eliminated in the F1 and F2. Shortening the lag phase, could be a way to reduce the trade-offs associated with the defense, as during the lag phase without defenses the animals are still vulnerable to their predators and fitness levels are not yet elevated (Weiss and Tollrian, 2018a, b). Immediate defense expression may serve advantageous against predators like juvenile fish (shortly after their larval stage, i.e., 0+) that prefer smaller prey items such as juvenile D. lumholtzi (Kolar and Wahl, 1998).
In addition to the earlier and stronger initial expression of head spines also their growth rate is increased. When continuously exposed to predator cues, D. lumholtzi head spines grow faster with every subsequent generation that we tested. Such a rapid growth of the defensive structure further enhances survival chances as the defensive effect of the head spines is thereby shifted to earlier instars. The length and rigidity of spines is discussed to prevent ingestion and leads to avoidance by the predator (Engel et al., 2014). I.e., when the head spines outgrow the fishes’ gape, predator handling becomes more complicated, and consumption is impaired. These head spines get stuck in fish’s buccal cavity (Kolar and Wahl, 1998). The fish then shake their heads and flare their opercula attempting to dislodge their prey. The fish gain experience and then reject defended prey with higher frequency and this reduces D. lumholtzi mortality rates (Kolar and Wahl, 1998). Concludingly, progeny of permanently exposed D. lumholtzi are not only prepared by being born with a larger defense but also their defense subsequently grows faster.
Transgenerational phenotypic plasticity has been reported to dependent on a dedicated developmental window in which TGP is induced, so not only the exposure length but also the timing is decisional (Duncan et al., 2014). Very often not only the embryonic but also the parental environment was shown to impact the offsprings’ phenotypes (Shea et al., 2011; Luquet and Tariel, 2016; Heckwolf et al., 2018). We therefore wanted to determine if this earlier onset and boost of defense expression is due to effects of fish kairomones in the maternal environment, the embryonic environment or both. We therefore tested two distinctive phases, i.e., prior to and during embryo development. Daphnia spec. have a dedicated reproductive strategy, i.e., mothers give rise to genetically identical offspring (Smirnov, 2013). During this kind of parthenogenesis, mothers deposit subitaneous embryos in the brood pouch, that directly develop into juvenile offspring (embryogenesis) (Smirnov, 2013). When exposing naïve D. lumholtzi TE to kairomones during embryogenesis, this does not induce TGP; i.e., the lag phase is neither eliminated nor shortened and head spines are not boosted. This means that D. lumholtzi is not able to perceive and/or react to kairomones during prenatal development. Also, Laforsch and Tollrian did not detect prenatal growth of defensive structures during D. lumholtzi embryogenesis but found that spines are folded along the body which allows for embryonic growth of the traits (Laforsch and Tollrian, 2004). When we expose D. lumholtzi mothers to kairomones and remove them prior to conception, this results in TGP as lag times are shortened and defenses are boosted in the 2nd juvenile instar. Therefore, TGP must be inducible before embryogenesis. It is well possible that the environmental information is imprinted on the primordial germ cells that develop in the mother and thereby the offspring is prepared for future environmental conditions (Hemberger et al., 2009).
In comparison to offspring from mothers’ that were exposed to kairomones until the 4th instar, permanently exposed D. lumholtzi have larger defenses. Similarly, the lag phase which is shortened by one instar in the offspring of not continuously exposed mothers, is shortened by two instars in the permanently exposed animals. Together with the fact, that the daphnia themselves are not sensitive to kairomones during embryogenesis this clearly shows that the shortening of the lag phase is a result of TGP. TGP initiates early defense expression so that WGP can start earlier and on a higher level. This TGP effect is intensified when the mothers are permanently exposed to kairomone, and could be an additive effect from the prolonged kairomone exposure.
Mechanistically TGP of inducible defenses is discussed to be associated with epigenetic inheritance where gene activity and/or accessibility is altered through differential methylation patterns, micro RNAs, or histone modifications (Vandegehuchte et al., 2010; Walsh et al., 2016; Fallet et al., 2020). These changes can be passed on to the next generation enhancing the offspring’s performance (Feil, 2008; Sentis et al., 2018). Vandegehuchte et al. showed that D. magna, e.g., is able to pass on differential methylation patterns to the next two generations (Vandegehuchte et al., 2010). Furthermore, it was shown that methylation patterns from kairomone exposed Daphnia ambigua were passed on to following generations (Schield et al., 2016). The mechanisms that underly the formation of TGP in D. lumholtzi will be of interest in future experiments. Likewise, it remains to be investigated how long TGP persists in D. lumholtzi when predator cues are absent. In D. cucullata the TGP effect disappeared, after the disappearance of the predator cue (Agrawal et al., 1999). This scenario is well-imaginable for D. lumholtzi; when the predator disappears, the defenses will most likely stop growing and the animals will save the costs associated with defense expression. The following generation will most likely be undefended upon birth. It will be interesting to test if the offspring of predator exposed grandmothers and unexposed mothers, will react more sensitive to predator cues in comparison to offspring from naïve grandparents.
Taken together, D. lumholtzi shows a distinctive form of transgenerational phenotypic plasticity, where the defenses are developed faster and larger which enhances the defensive effect.
Data Availability Statement
All data is provided in the Supplementary Material provided alongside of the manuscript.
Ethics Statement
The animal study was reviewed and approved by the Ruhr University Bochum Animal Welfare Office.
Author Contributions
LW and RT designed the study. AG, MJ, and MV performed the experiments. AG analyzed the data. AG, RT, and LW wrote the manuscript. All authors contributed to the final version of the manuscript.
Conflict of Interest
The authors declare that the research was conducted in the absence of any commercial or financial relationships that could be construed as a potential conflict of interest.
Acknowledgments
We thank Elli Buschtöns for valuable help with the Daphnia and algae culture.
Supplementary Material
The Supplementary Material for this article can be found online at: https://www.frontiersin.org/articles/10.3389/fevo.2021.637421/full#supplementary-material
References
Agrawal, A., Laforsch, C., and Tollrian, R. (1999). Transgenerational induction of defences in animals and plants. Nature 401, 60–63. doi: 10.1038/43425
Auge, G. A., Leverett, L. D., Edwards, B. R., and Donohue, K. (2017). Adjusting phenotypes via within- and across-generational plasticity. New Phytol. 216, 343–349. doi: 10.1111/nph.14495
Auld, J. R., Agrawal, A. A., and Relyea, R. A. (2010). Re-evaluating the costs and limits of adaptive phenotypic plasticity. Proc. Biol. Sci. 277, 503–511. doi: 10.1098/rspb.2009.1355
Bonduriansky, R., and Day, T. (2009). Nongenetic inheritance and its evolutionary implications. Annu. Rev. Ecol. Evol. Syst. 40, 103–125. doi: 10.1146/annurev.ecolsys.39.110707.173441
Bradshaw, A. D. (1965). Evolutionary significance of phenotypic plasticity in plants. Adv. Genet. 13, 115–155. doi: 10.1016/S0065-2660(08)60048-6
Dodson, S. I., and Hanazato, T. (1995). Commentary on effects of anthropogenic and natural organic chemicals on development, swimming behavior, and reproduction of Daphnia, a key member of aquatic ecosystems. Environ. Health Perspect. 103, 7–11. doi: 10.1289/ehp.95103s47
Donelson, J. M., Salinas, S., Munday, P. L., and Shama, L. N. S. (2018). Transgenerational plasticity and climate change experiments: where do we go from here? Glob. Chang. Biol. 24, 13–34. doi: 10.1111/gcb.13903
Duncan, E. J., Gluckman, P. D., and Dearden, P. K. (2014). Epigenetics, plasticity, and evolution: how do we link epigenetic change to phenotype? J. Exp. Zool. Part B Mol. Dev. Evol. 322, 208–220. doi: 10.1002/jez.b.22571
Engel, K., Schreder, T., and Tollrian, R. (2014). Morphological defences of invasive Daphnia lumholtzi protect against vertebrate and invertebrate predators. J. Plankton Res. 36, 1140–1145. doi: 10.1093/plankt/fbu023
Engel, K., and Tollrian, R. (2009). Inducible defences as key adaptations for the successful invasion of Daphnia lumholtzi in North America? Proc. Biol. Sci. 276, 1865–1873. doi: 10.1098/rspb.2008.1861
English, S., Pen, I., Shea, N., and Uller, T. (2015). The information value of non-genetic inheritance in plants and animals. PLoS One 10:e0116996. doi: 10.1371/journal.pone.0116996
Ezard, T. H. G., Prizak, R., and Hoyle, R. B. (2014). The fitness costs of adaptation via phenotypic plasticity and maternal effects. Funct. Ecol. 28, 693–701. doi: 10.1111/1365-2435.12207
Fallet, M., Luquet, E., David, P., and Cosseau, C. (2020). Epigenetic inheritance and intergenerational effects in mollusks. Gene. 729:144166. doi: 10.1016/j.gene.2019.144166
Feil, R. (2008). Epigenetics, an emerging discipline with broad implications. C. R. Biol. 331, 837–843. doi: 10.1016/j.crvi.2008.07.027
Grant, J. W. G., and Bayly, I. A. E. (1981). Predator induction of crests in morphs of the Daphnia carinata king complex. Limnol. Oceanogr. 26, 201–218. doi: 10.4319/lo.1981.26.2.0201
Hanson, M. A., and Skinner, M. K. (2016). Developmental origins of epigenetic transgenerational inheritance. Environ. Epigenet. 2:dvw002. doi: 10.1093/eep/dvw002
Harris, K. D. M., Bartlett, N. J., and Lloyd, V. K. (2012). Daphnia as an emerging epigenetic eodel erganism. Genet. Res. Int. 2012:147892. doi: 10.1155/2012/147892
Hebert, P. D. N., and Grewe, P. M. (1985). Chaoborus-induced shifts in the morphology of Daphnia ambigua. Limnol. Oceanogr. 30, 1291–1297.
Heckwolf, M. J., Meyer, B. S., Döring, T., Eizaguirre, C., and Reusch, T. B. H. (2018). Transgenerational plasticity and selection shape the adaptive potential of sticklebacks to salinity change. Evol. Appl. 11, 1873–1885. doi: 10.1111/eva.12688
Hemberger, M., Dean, W., and Reik, W. (2009). Epigenetic dynamics of stem cells and cell lineage commitment: digging Waddington’s canal. Nat. Rev. Mol. Cell Biol. 10, 526–537. doi: 10.1038/nrm2727
Hendry, A. P. (2016). Key questions on the role of phenotypic plasticity in eco-evolutionary dynamics. J. Hered. 107, 25–41. doi: 10.1093/jhered/esv060
Holeski, L. M., Jander, G., and Agrawal, A. A. (2012). Transgenerational defense induction and epigenetic inheritance in plants. Trends Ecol. Evol. 27, 618–626. doi: 10.1016/j.tree.2012.07.011
Horstmann, M., Topham, A. T., Stamm, P., Kruppert, S., Colbourne, J. K., Tollrian, R., et al. (2018). Scan, extract, wrap, compute—a 3D method to analyse morphological shape differences. PeerJ 6:e4861. doi: 10.7717/peerj.4861
Imai, M., Naraki, Y., Tochinai, S., and Miura, T. (2009). Elaborate regulations of the predator-induced polyphenism in the water flea Daphnia pulex: kairomone-sensitive periods and life-history tradeoffs. J. Exp. Zool. A Ecol. Genet. Physiol. 311, 788–795. doi: 10.1002/jez.565
Klüttgen, B., Dülmer, U., Engels, M., and Ratte, H. T. (1994). ADaM, an artificial freshwater for the culture of zooplankton. Water Res. 28, 743–746. doi: 10.1016/0043-1354(94)90157-0
Kolar, C. S., and Wahl, D. H. (1998). Daphnid morphology deters fish predators. Oecologia 116, 556–564. doi: 10.1007/s004420050621
Krueger, D. A., and Dodson, S. I. (1981). Embryological induction and predation ecology in Daphnia pulex. Limnol. Oceanogr. 26, 219–223. doi: 10.4319/lo.1981.26.2.0219
Kumar, S., Kumari, R., and Sharma, V. (2015). Transgenerational inheritance in plants of acquired defence against biotic and abiotic stresses: implications and applications. Agric. Res. 4, 109–120. doi: 10.1007/s40003-015-0170-x
Laforsch, C., and Tollrian, R. (2004). Embryological aspects of inducible morphological defenses in Daphnia. J. Morphol. 262, 701–707. doi: 10.1002/jmor.10270
Luquet, E., and Tariel, J. (2016). Offspring reaction norms shaped by parental environment: interaction between within- and trans-generational plasticity of inducible defenses. BMC Evol. Biol. 16:209. doi: 10.1186/s12862-016-0795-9
Miner, B. G., Sultan, S. E., Morgan, S. G., Padilla, D. K., and Relyea, R. A. (2005). Ecological consequences of phenotypic plasticity. Trends Ecol. Evol. 20, 685–692. doi: 10.1016/j.tree.2005.08.002
Mousseau, T. A., and Dingle, H. (1991). “Maternal effects in insects: examples, constraints and geographic variation,” in Proceedings of the 4th International Congress of Systematic and Evolutionary, Vol. 2, ed. E. Dudley (College Park, MD: University of Maryland), 745–761.
Mousseau, T. A., and Fox, C. W. (1998). The adaptive significance of maternal effects. Trends Ecol. Evol. 13, 403–406. doi: 10.1016/S0169-5347(98)01472-4
Petrusek, A., Tollrian, R., Schwenk, K., Haas, A., and Laforsch, C. (2009). A “crown of thorns” is an inducible defense that protects Daphnia against an ancient predator. Proc. Natl. Acad. Sci. U.S.A. 106, 2248–2252. doi: 10.1073/pnas.0808075106
Rabus, M., and Laforsch, C. (2011). Growing large and bulky in the presence of the enemy: Daphnia magna gradually switches the mode of inducible morphological defences. Funct. Ecol. 25, 1137–1143.
Schield, D. R., Walsh, M. R., Card, D. C., Andrew, A. L., Adams, R. H., and Castoe, T. A. (2016). EpiRADseq: scalable analysis of genomewide patterns of methylation using next-generation sequencing. Methods Ecol. Evol. 7, 60–69. doi: 10.1111/2041-210X.12435
Sentis, A., Bertram, R., Dardenne, N., Ramon-Portugal, F., Espinasse, G., Louit, I., et al. (2018). Evolution without standing genetic variation: change in transgenerational plastic response under persistent predation pressure. Heredity 121, 266–281. doi: 10.1038/s41437-018-0108-8
Shama, L. N. S., Strobel, A., Mark, F. C., and Wegner, K. M. (2014). Transgenerational plasticity in marine sticklebacks: maternal effects mediate impacts of a warming ocean. Funct. Ecol. 28, 1482–1493. doi: 10.1111/1365-2435.12280
Shea, N., Pen, I., and Uller, T. (2011). Three epigenetic information channels and their different roles in evolution. J. Evol. Biol. 24, 1178–1187. doi: 10.1111/j.1420-9101.2011.02235.x
Tollrian, R. (1993). Neckteeth formation in Daphnia pulex as an example of continuous phenotypic plasticity morphological effects of Chaoborus kairomone concentration and their quantification. J. Plankton Res. 15, 1309–1318.
Tollrian, R., and Dodson, S. I. (1999). “Inducible defenses in cladocera: constraints, costs and multipredator environments,” in The Ecology and Evolution of Inducible Defenses, eds R. Tollrian and C. D. Harvell (Princeton, NJ: Princeton University Press), 177–202.
Tollrian, R., and Harvell, C. D. (1999). The ecology and evolution of induced defenses. Q. Rev. Biol. 65, 323–340. doi: 10.1086/416841
Uller, T. (2008). Developmental plasticity and the evolution of parental effects. Trends Ecol. Evol. 23, 432–438. doi: 10.1016/j.tree.2008.04.005
Vandegehuchte, M. B., Lemière, F., Vanhaecke, L., Vanden Berghe, W., and Janssen, C. R. (2010). Direct and transgenerational impact on Daphnia magna of chemicals with a known effect on DNA methylation. Comp. Biochem. Physiol. C Toxicol. Pharmacol. 151, 278–285. doi: 10.1016/j.cbpc.2009.11.007
Walsh, M. M. R. M., Castoe, T., Holmes, J., Packer, M., Biles, K., Walsh, M. M. R. M., et al. (2016). Local adaptation in transgenerational responses to predators. Proc. R. Soc. B Biol. Sci. 283:20152271. doi: 10.1098/rspb.2015.2271
Weiss, L. C. (2019). Sensory ecology of predator-induced phenotypic plasticity. Front. Behav. Neurosci. 12:330. doi: 10.3389/fnbeh.2018.00330
Weiss, L. C., Albada, B., Becker, S. M., Meckelmann, S. W., Klein, J., Meyer, M., et al. (2018). Identification of Chaoborus kairomone chemicals that induce defences in Daphnia. Nat. Chem. Biol. 14, 1133–1139. doi: 10.1038/s41589-018-0164-7
Weiss, L. C., Heilgenberg, E., Deussen, L., Becker, S. M. S. M., Kruppert, S., and Tollrian, R. (2016). Onset of kairomone sensitivity and the development of inducible morphological defenses in Daphnia pulex. Hydrobiologia 779, 135–145. doi: 10.1007/s10750-016-2809-4
Weiss, L. C., Kruppert, S., Laforsch, C., and Tollrian, R. (2012). Chaoborus and Gasterosteus anti-predator responses in Daphnia pulex are mediated by independent cholinergic and gabaergic neuronal signals. PLoS One 7:e36879. doi: 10.1371/journal.pone.0036879
Weiss, L. C., Leimann, J., and Tollrian, R. (2015). Predator-induced defences in Daphnia longicephala: location of kairomone receptors and timeline of sensitive phases to trait formation. J. Exp. Biol. 218, 2918–2926. doi: 10.1242/jeb.124552
Weiss, L. C., and Tollrian, R. (2018a). “Inducible defenses in crustacea,” in The Natural History of the Crustacea, Life Histories, Vol. 5, eds G. Welborn and M. Thiel (New York: Oxford University Press).
Weiss, L. C., and Tollrian, R. (2018b). “Predator induced defenses in crustacea,” in The Natural History of Crustacea: Life Histories, Vol. 5, eds G. Welborn and M. Thiel (New York: Oxford University Press), 303–321.
Keywords: transgenerational phenotypic plasticity, within generational phenotypic plasticity, head spines, kairomone, predator, Daphnia, inducible defenses
Citation: Graeve A, Janßen M, Villalba de la Pena M, Tollrian R and Weiss LC (2021) Higher, Faster, Better: Maternal Effects Shorten Time Lags and Increase Morphological Defenses in Daphnia lumholtzi Offspring Generations. Front. Ecol. Evol. 9:637421. doi: 10.3389/fevo.2021.637421
Received: 03 December 2020; Accepted: 30 April 2021;
Published: 21 May 2021.
Edited by:
Chiara Morosinotto, Novia University of Applied Sciences, FinlandReviewed by:
Andreas Walzer, University of Natural Resources and Life Sciences Vienna, AustriaLaura Stein, University of Oklahoma, United States
Copyright © 2021 Graeve, Janßen, Villalba de la Pena, Tollrian and Weiss. This is an open-access article distributed under the terms of the Creative Commons Attribution License (CC BY). The use, distribution or reproduction in other forums is permitted, provided the original author(s) and the copyright owner(s) are credited and that the original publication in this journal is cited, in accordance with accepted academic practice. No use, distribution or reproduction is permitted which does not comply with these terms.
*Correspondence: Linda C. Weiss, TGluZGEuV2Vpc3NAcnViLmRl