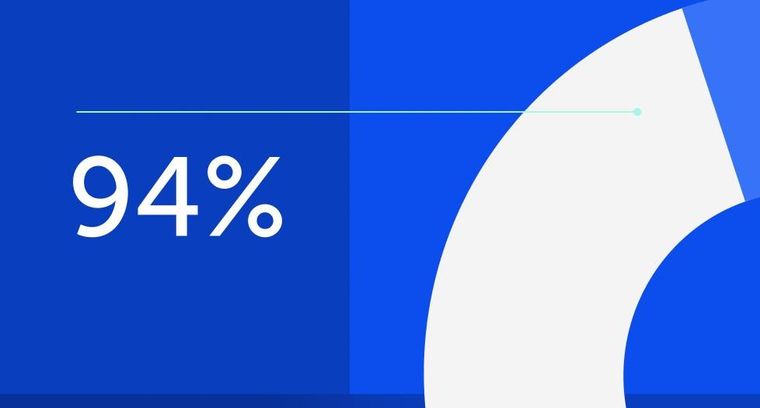
94% of researchers rate our articles as excellent or good
Learn more about the work of our research integrity team to safeguard the quality of each article we publish.
Find out more
ORIGINAL RESEARCH article
Front. Ecol. Evol., 30 April 2021
Sec. Biogeography and Macroecology
Volume 9 - 2021 | https://doi.org/10.3389/fevo.2021.631762
This article is part of the Research TopicInvaders on the Horizon! Scanning the Future of Invasion Science and ManagementView all 16 articles
Canals and other connected waterway systems, including the Chicago Area Waterway System (CAWS), have often facilitated the spread of non-native species. Electric barriers have recently emerged as a method for preventing this spread and protecting uninvaded ecosystems from new invaders. The largest system of electric barriers in the world is in the CAWS and is operated primarily to prevent the spread of invasive Asian carp. It is not known whether these barriers are effective for other species, particularly invasive invertebrates. Here, we provide data regarding the efficacy of an electric field that operates at the same parameters as the electric barrier in the CAWS in affecting behaviors of two invertebrate species, the red swamp crayfish Procambarus clarkii and the amphipod Hyalella azteca. We constructed an electric field within a tank that operates at the same parameters as the existing CAWS barriers and determined the effects of the electric field on our test species. At the electric field parameters of the CAWS barriers, the vast majority of P. clarkii individuals showed altered movement with maintained equilibrium. For H. azteca, behavioral responses were less extreme than for P. clarkii, with a majority of individuals experiencing altered movement. By measuring the orientation of organisms to the electric field, we determined that the test organisms are affected by the electric field, especially at lower field strengths where they exhibited no or little other behavioral response. At lower field strengths, P. clarkii exhibited changes in orientation, but at higher field strengths, individuals were less able to orient themselves. H. azteca exhibited changes in orientation to the electric field at all field strengths. The results of this study suggest that the existing electric barriers may not slow or prevent spread of invasive invertebrates—including amphipods and crayfish—through passive movement attached boats/barges or through downstream drift, but that the barriers may prevent spread by active upstream movement. Overall, our work gives new data regarding the efficacy of electric fields in preventing the spread of invasive invertebrates and can inform management decisions regarding current and future electric barriers in the CAWS.
Invasive freshwater species cause large ecological and economic impacts (Pimentel et al., 2005; Lodge et al., 2016). Preventing the spread of established invaders is a major goal for conservation and management but can be particularly difficult in connected freshwater ecosystems (Strayer, 2010). Whereas many terrestrial invasive organisms can be managed with herbicides, pesticides, and mechanical means, the available technologies for managing freshwater invasive species are more limited because of the difficulty of directly targeting a given species (Manfrin et al., 2019). Recently, there has been the development and limited deployment of novel technologies for controlling invasive species and restricting their spread through freshwater ecosystems. These include bio-bullets (BioBullets1), which target filter-feeding biofouling organisms in industrial settings, electric barriers to deter spread of aquatic organisms (Sparks et al., 2010; Benejam et al., 2015; Kim and Mandrak, 2017), and more recently the suggestion that water saturated with carbon dioxide could be used to inhibit the spread of invasive organisms (Kates et al., 2012; Treanor et al., 2017; Suski, 2020). While these technologies each offer promise, more research is needed to understand how effective they are at deterring spread of a range of non-native species.
The Mississippi River and Laurentian Great Lakes basins have been invaded by many non-native aquatic species, including bighead and silver carp (Hypophthalmichthys nobilis and Hypophthalmichthys molitrix; Kolar et al., 2005), round goby (Neogobius melanostomus; Kornis et al., 2012), rusty crayfish (Faxonius rusticus; Peters et al., 2014), and numerous amphipods (Grigorovich et al., 2005, 2008). These basins were ecologically separated until the 19th century when canals were built to facilitate transport and wastewater disposal. The major connection between them is the Chicago Sanitary and Ship Canal (CSSC), which forms part of the Chicago Area Waterway System (CAWS). The CSSC was opened in 1900 and created the first large permanent waterway connecting the Mississippi and Great Lakes basins. Subsequent canal building has expanded the CAWS, and there are now three harbors in Lake Michigan from which water flows through the CAWS and eventually into the Mississippi River. This connection has allowed several non-native freshwater species to move from the Great Lakes to the Mississippi River and vice versa. Some of these species are presumed to have moved with the aid of boats via hull-fouling (e.g., Dreissenid mussels), while others (e.g., round goby) have presumably been able to transit the system unaided (Holeck et al., 2004; Keller et al., 2011).
Electric barriers are an emerging technology for preventing the spread of invasive aquatic species through waterways and canals (Sparks et al., 2010; Benejam et al., 2015; Kim and Mandrak, 2017). These barriers offer the promise of addressing invasive species threats without affecting the flow of water or the passage of recreational and commercial boats. The largest electric barriers in operation are in the CSSC where they have been developed and parameterized to prevent the upstream spread of Asian carp species from the Mississippi River into the Great Lakes (Holliman et al., 2015; Parker et al., 2015). Although the electric barriers may prevent spread of these large fishes, the CSSC remains a potential route for spread of many other taxa, which pose risks of moving through both upstream and downstream and which may be transported on the hulls of boats. Little information has previously been available about the effect of electric barriers on these other species or modes of transport.
The CAWS remains a major risk for spread of damaging invasive species, and much effort has been expended to understand how species move through it, which species pose a large risk for future spread, and how that spread can be prevented (Veraldi et al., 2011). After round goby became established in the Great Lakes, there was sufficient concern about its potential to spread into the Mississippi River Basin that the construction of electric barriers in the CSSC was proposed and approved (Sparks et al., 2010). Unfortunately, by the time the first barrier (known as the Demonstration Barrier) was operational in 2002, round goby was already established in the Mississippi River Basin (Kornis et al., 2012). Subsequent barriers (Barrier IIA and IIB) were constructed in 2009, and all three barriers now operate to deter the spread of bighead and silver carp (these species are often collectively referred to as Asian carp) from the Mississippi River Basin to the Great Lakes (Veraldi et al., 2011; Parker et al., 2015). The barriers consist of steel cable electrodes that create direct current (DC) pulses in the CSSC at an electric field strength of 2.3 V/in, a frequency of 34 Hz, and a pulse length of 2.3 ms (USACE, 2011). These parameters were established based on tests with bighead and silver carp (Holliman et al., 2015). A further barrier (Permanent Barrier I) is planned to be operational by 2021 and will be the first barrier with flexibility to adjust settings for electric field strength, frequency, and pulse duration (Charles Shea, USACE, personal communication). Testing is currently being conducted by the United States Army Corps of Engineers to determine the parameters at which Permanent Barrier I will be operated. Although the electric barriers in the CAWS are operated to prevent the spread of invasive silver and bighead carp, many other non-native species present risks of moving through this system, and it is unknown whether the barriers could effectively prevent their spread. Previous studies have found that electric fields can alter the behavior of crustaceans including fright or anxiety-like responses, attempted escape from the field, and involuntary restless movement, but the effect of electric fields on mobility and mortality in invertebrates is largely unknown (Biswas, 1971, 2008; Vannini and Insom, 1976; Fossat et al., 2014; Perrot-Minnot et al., 2017). If the barriers are not effective, then greater resources and actions may be necessary to prevent the spread of these species. In this study, we have tested the effect of different electric field strengths on two aquatic invertebrate species: Hyalella azteca, a small invertebrate amphipod that is native to the region, and Procambarus clarkii (red swamp crayfish), a large invasive crayfish that is currently of great concern due to its recent arrival and spread in the Great Lakes region.
These species were chosen for two reasons. First, electric fields are known to affect different sized organisms in different ways, and the two species used in our experiment measured are of quite different sizes (H. azteca average size of 4.6 mm; P. clarkii average size of 20.2 mm). Second, these species either are already of invasion concern in the region or are taxonomically and morphologically similar to species of concern. P. clarkii is an invasion concern as a crayfish with recently detected established populations in inland and Great Lakes-adjacent waters in Illinois, Ohio, Michigan, and Wisconsin (Ellison, 2015; Bunk and Van Egeren, 2016; Jacobs and Keller, 2017; Donato et al., 2018; Smith et al., 2018; Egly et al., 2019; Oficialdegui et al., 2019). Native to the south-central United States and northern Mexico, P. clarkii is a generalist species that can survive in a wide range of freshwater habitats and is already globally widely established (USGS; Cruz and Rebelo, 2007; Larson and Olden, 2012). Invasive populations of P. clarkii can reduce biomass and species richness of macrophytes, leading to stable state shifts, reduced abundance of macroinvertebrates, and displaced native crayfishes through mechanisms such as competition for food and shelter (Rodríguez et al., 2003; Paglianti and Gherardi, 2004; Gherardi and Acquistapace, 2007; Matsuzaki et al., 2009; Twardochleb et al., 2013). We used juvenile P. clarkii since the effect of the electric field on an organism is directly proportional to organism size (Sternin et al., 1976; Miranda, 2009), so juveniles are likely to be less affected by the electric field than adults and thus more likely to pass through the barrier unhindered.
We chose H. azteca as a proxy for the amphipod Apocorophium lacustre (scud), a filter-feeding amphipod native to estuaries on the Atlantic coasts of North America and Europe (Shoemaker, 1934; Bousefield, 1973; Faasse and van Moorsel, 2003). A. lacustre has not previously been reared in any lab and is difficult to maintain. H. azteca is similar in size and has similar habitat preferences to A. lacustre, is readily available from biological supplies houses, and has well-established protocols for care (Grigorovich et al., 2008). A. lacustre was first recorded as established in the lower Mississippi River in 1987 (Payne et al., 1989) and has since spread extensively within the Mississippi River basin to occupy the Ohio River, upper Mississippi River, and the Illinois River. Its current known distribution extends to within 100 river kilometers of Lake Michigan (Heard, 1982; Payne et al., 1989; Grigorovich et al., 2008; Keller et al., 2017).
Our work investigates the effects of electric fields on these two invertebrates. We created an electric field contained within a tank which operates at the same parameters as the existing electric barriers in the CAWS. We are among the first to develop a relatively straightforward system using inexpensive materials for testing the effects of electric fields on smaller organisms. After constructing our electric field, we conducted experiments to determine the effects of the electric field on our test species and classified organism behavior and used order parameter analysis to quantify amphipod and crayfish response to the electric field. Although our study focuses on static electric fields rather than the experience of an organism approaching and moving through an electric field, the behavior and orientation of organisms within an electric field are critical to understanding potential effects of electric barriers on organisms. Based on our initial results, we conducted experiments with higher electric field strengths than are produced in the CSSC to determine whether realistic increases could produce a viable barrier to the spread of invertebrate invaders.
Juvenile P. clarkii were reared in lab from adult females that were caught in the North Branch of the Chicago River in October 2018 and October 2019. Juvenile P. clarkii were kept in aerated tanks with dechlorinated water and fed every 3–5 days. Hyalella azteca specimens were purchased from an online vendor and maintained in the lab in aerated tanks with dechlorinated water and fed every 3–5 days.
We constructed an electric field within a rectangular glass tank (122 cm long, 32 cm wide, and 34 cm deep) and calibrated it to produce the same electric field strength and waveform as the electric barriers in the CAWS. Our equipment consisted of three elements: a modified backpack electrofishing unit (ETS PK-C2); a power supply (Volteq HY3010EX), which supplies a DC to the electrofishing unit and replaces its battery; and two Type 316 stainless steel plate electrodes (38 cm × 27 cm) placed at opposite ends of the tank (Figure 1). The electrofishing unit was rewired so that the anode and cathode lines were attached with jumper cables to the electrodes. These were placed at each end of the tank and produced a uniform electric field throughout the tank.
Figure 1. Diagram of experimental electric barrier setup, including power supply, electrofishing unit, tank, steel plate electrodes, and cathode and anode lines.
To confirm that our system produced the desired electric field, we compared the true output of the electric field with the expected output based on the backpack readings (Supplementary Material 3). In particular, we measured the voltage, current, frequency, duty cycle, and waveform integrity in the tank using a Fluke 87V Industrial Multimeter, Fluke 124B Industrial Scopemeter, and Fluke 80i-119s AC/DC Current Clamp (US Fish and Wildlife Service, 2017). We used both the multimeter and the scopemeter to test voltage. The average difference of the true output voltage compared with the expected output was 1.2% as measured by the multimeter and 3.3% as measured by the scopemeter. The multimeter measured current between 0.04 and 0.10 A, higher than the expected output when measuring a range of currents from 0 to 1.1 A. We also used the scopemeter to measure frequency and duty cycle. The scopemeter confirmed that the waveform of the output was as expected. The average difference was 2.1% for frequency and 6.4% for duty cycle. These differences are minor considering the range over which we ran the electric field (see below) and confirm that the electric field produced was similar to that of the barrier in the CAWS.
All experiments were conducted using lab water at both ambient water temperature (mean ± standard deviation = 20.3 ± 1.4°C) and ambient specific conductivity (mean ± standard deviation = 321.6 ± 56.8 μS/cm). To recreate the parameters of the electric barrier in the CAWS, the initial settings on the electrofishing unit were 106 V, 34 Hz, pulse length = 2.3 ms, and duty cycle = 7.2%, creating an electric field of 2.3 V/in (USACE, 2011).
Each trial began with five individual organisms (haphazardly selected from a tank containing the available pool of individuals) placed in a non-conductive nylon mesh container in the center of the tank. Trials consisted of three consecutive phases of 5 min: pre-stimulus, stimulus, and post-stimulus (following Kim and Mandrak, 2017). The electric field was off for the pre-stimulus phase, on for the stimulus phase, and off again for the post-stimulus phase. Trials were recorded with a video recorder (GoPro, HERO4) placed overhead at the center of the tank. During each trial, we recorded the behavior at the end of each minute and response to a physical prodding with non-conductive rod every 2.5 min. All organisms were kept for 24 h after each trial to check for delayed mortality. It was not possible to record the behavior of each individual organism over time. Instead, we recorded the number of organisms in each behavioral state at each time. All personnel who recorded behaviors were trained by the lead author and frequently coordinated with each other in an effort to limit observer bias (e.g., two observers viewing the same organism but describing the behavior differently). Our study included distinct behavior categories, which made it difficult to make a mistake in classification, and we often had multiple observers recording results independently, which when compared always displayed >90% agreement between observers. We were not able to make the observers “blind” to whether the barrier was on or off due to safety issues that could arise (Holman et al., 2015). We acknowledge that having different observers and not knowing whether the barrier was on/off may have led to some bias, but for the reasons described, we believe this would have been minimal.
Using the video recordings and SolidWorks software (BIOVIA, 2018; Supplementary Material 4), we later determined the orientation of each individual to the electric field every minute during the pre- and post-stimulus phases and approximately every 30 s during the stimulus phase. Initial trials were conducted at the field strength currently produced in the CSSC. Based on results in these trials, we tested the effects on crayfish at 25%, 50%, 200%, 300%, and 400% of the electric barrier’s field strength by changing voltage to 26, 53, 212, 318, and 424 V, respectively, while leaving all other parameters constant. We did the same for H. azteca except that we did not test at 25 and 50% because of the minimal response observed during the initial (100%) trials for this species.
Three trials were conducted for each combination of field strength and species, and each trial included five individuals. For juvenile crayfish, 18 trials were conducted using a total of 90 individuals (mean carapace length ± standard deviation = 20.2 ± 7.2 mm, n = 90; Supplementary Table 1). There were inevitable differences in sizes of individuals available for the different trials. The only trial where this may have been important is the 25% trial, which had somewhat smaller organisms than the other trials (see section “Results”). For amphipods, 12 trials were conducted using a total of 60 individuals (mean length ± standard deviation = 4.6 ± 1.0 mm, n = 60; Supplementary Table 2). Individual organisms were not reused in any trials.
Observations and video were used to score behaviors during each trial. During the 5-min stimulus phase, organism behavior was recorded and classified every minute for each individual as one of five categories: no change in behavior, individual exhibits normal behavior; altered movement, individual exhibits difficulty in moving or swimming; rigid and maintaining equilibrium, body is rigid but stays upright and organism maintains equilibrium; rigid and lost equilibrium, body is rigid with no motor functions and organism is not maintaining itself in upright position; and mortality, a loss of equilibrium and motor functions with no recovery, death.
We recorded the influence of the electric field on organism orientation by determining the order parameters and director angles of organisms when the barrier was off (pre- and post-stimulus phases) and on (stimulus phase). For a system of rods or rod-like objects, the order parameter and director angle can be used to describe how well the objects align and in what direction, respectively. Aquatic species such as fish will often orient themselves in a preferred direction relative to a pulsed electric field. In some cases, the fish may swim toward the anode with their bodies oriented parallel to the electric field (Halsband, 1967; Lamarque, 1967). In others, fish will orient themselves perpendicular to the electric field to minimize the electric potential across their bodies, which in turn minimizes the discomfort experienced (Burger et al., 2015). If the long axis of an organism is oriented parallel to the electric field (in our case, this would be an organism oriented with its posterior end pointed at one electrode and the anterior end pointed at the other), the angle would be measured as 0°. If the long axis is perpendicular, it would be measured as 90°.
The orientation of each individual to the electric field was measured five times each during the pre- and post-stimulus phases (every 1 min) and 10 times during the stimulus phase (every 30 s) for a total of 100 measurements per trial. These values were used to determine the average orientation of the organisms to the electric field, called the director angle, and how well the organisms line up with one other, called the order parameter (Andrienko, 2018). When examining the director angle, we are looking to see if the organisms are aligning themselves either parallel (0°) or perpendicular (90°) to the electric field or are oriented in some other direction. If the director angle is close to 0° or 90°, then organisms are likely responding the electric field. The order parameter S, is given by the following equation,
where θ is the angle of orientation measured relative to the average orientation and the angle brackets indicate that we are averaging over all measurements. S ranges in value from 1, which indicates that all objects in a system are perfectly aligned, to 0, which indicates that objects in a system are randomly oriented. An order parameter of 0.5 would indicate that the organisms are somewhat aligned but have a typical deviation from the director angle of 30°. In this work, we used an increase in order parameter to indicate that the organisms were reacting to the electric field and attempting to align in a preferred direction.
At 100% of the existing electric barrier field strength, most P. clarkii individuals experienced altered movement (46%) or rigidity with maintained equilibrium (36%; Figure 2A). Fewer P. clarkii experienced rigidity and lost equilibrium (10%), and none died either during the experiment or the 24 h following. For H. azteca, behavioral responses at 100% of the existing barrier strength were similar (Figure 2B), with a majority of individuals (57%) remaining responsive but experiencing altered movement. H. azteca individuals also displayed rigidity with maintained equilibrium (31%) and rigidity with lost equilibrium (12%). No H. azteca died during the experiment at 100% of existing barrier field strength or during the 24 h following. Results in Figure 2 are aggregated across time to show overall behavior during the stimulus phase at different electric field strengths because reactions across the five organisms in each trial did not change consistently throughout the 5-min stimulus phase for either species (see Supplementary Figures 1, 2 for full time series data).
Figure 2. Aggregated behavior across time during 5 min of stimulus phase for (A) juvenile Procambarus clarkii (n = 15 for each barrier strength) for 25%, 50%, 100%, 200%, 300%, and 400% of existing barrier strength; and (B) Hyalella azteca (n = 15 for each barrier strength) for 100%, 200%, 300%, and 400% of existing barrier strength.
At electric field strengths ≥200% of the existing electric barrier field strength, the number of strong behavioral responses increased for P. clarkii, with the majority of individuals experiencing rigidity and lost equilibrium (64% at 200% barrier strength, 77% at 300% barrier strength, 91% at 400% barrier strength; Figure 2A). Again, none were killed during the experiments, and all recovered within 24 h after the experiments. At electric barrier strengths lower than the existing barrier (25 and 50%), behavioral effects on P. clarkii were less extreme. At 25% of the existing barrier’s electric field strength, P. clarkii did not exhibit any behavioral response; but at 50% of the existing barrier’s electric field strength, a majority of individuals displayed altered movement (72%). We note that juvenile crayfish used in the trials for 25% of the existing barrier’s strength were slightly smaller (average size = 12.7 mm vs. average size for all juveniles tested of 20.2 mm). This was due to the availability of individuals and may have affected the behavioral response seen in the 25% trial.
For the amphipod H. azteca, an increasing number of individuals experienced rigidity and lost equilibrium at electric field strengths ≥200% of the existing barrier, with 51% of individuals experiencing rigidity and lost equilibrium at 400% (Figure 2B). None were killed during the experiments, and all except three individuals survived for 24 h after the experiment. Two H. azteca from the 200% trial died, and one from the 400% trial.
For every trial, we calculated director angle and order parameter when the electric field was on (stimulus phase) and off (pre- and post-stimulus phases). At 25% of the existing electric barrier’s electric field strength, P. clarkii did not exhibit a behavioral response recorded on our five-point scale (see above) but did exhibit changes in orientation. These changes in orientation are characterized by an increase in the order parameter (Figure 3A) and a director angle close to 90° (i.e., perpendicular alignment to the electric field) when the electric field was on (Figure 4). At 50% and 100% of the existing barrier’s field strength, we observed a larger increase in the order parameter when the electric field was turned on and a director angle close to 90°. However, at electric field strengths ≥200% of the existing electric barrier, the increases in order parameter were smaller, and the director angle when the electric field was turned on was not close to 0° or 90°.
Figure 3. Average order parameter values while barrier was turned off (pre- and post-stimulus phases) and while barrier was turned on (stimulus phase) for different barrier strengths for (A) Procambarus clarkii and (B) Hyalella azteca.
Figure 4. Average and range of director angle values for Procambarus clarkii and Hyalella azteca during stimulus phase for different barrier strengths. Juvenile P. clarkii is displayed in blue, and H. azteca is displayed in red.
Hyalella azteca exhibited changes in orientation to the electric field at all electric field strengths, characterized by an increase in the order parameter and a director angle close to 90° (perpendicular alignment) when the electric field was turned on (Figure 4). At 200% of the existing barrier’s electric field strength, we observed a larger increase in the order parameter when the electric field was turned on than other voltages (Figure 3B). At 400% of the existing barrier’s electric field strength, order parameter values and director angles indicate that organisms were still capable of responding to the electric field. At all electric field strengths, individuals were able to maintain equilibrium, and order parameter values and director angles can be inferred to be a response to the discomfort of the electric field.
It is unknown whether the existing barrier in the CSSC would deter the passage of invertebrates such as our study species. Based on our results, it appears that the electric field created by the existing barriers would affect behavior of organisms similar to those used in our study when they were within the barriers. Our results indicate that invertebrates similar in size to our two study species may be less affected by electric fields than Asian carp (Holliman et al., 2015; Parker et al., 2015). When exposed to an electric field identical to that in the CSSC, many P. clarkii and H. azteca were able to maintain equilibrium, many remained mobile, and none were killed. This indicates that individuals of either species could survive transit through the barrier at its existing strength. Transit could occur attached to the hull of a boat or in downstream drift (i.e., from the Great Lakes to the Mississippi basin). Our work did not directly address whether either of these species would be deterred from independent movement upstream through the barrier at its current strength.
Our constructed electric field is flexible in its parameters, and this allowed us to test the effects of lower electric field strengths on P. clarkii and higher electric field strengths on both P. clarkii and H. azteca. For P. clarkii, at ≥50% of the existing barrier’s electric field strength, some individuals exhibited altered movement and rigidity, but there was only a consistent loss of equilibrium at ≥200% of the existing barrier’s electric field strength. Similarly, at ≥100% of the existing barrier’s electric field strength, H. azteca individuals exhibited altered movement and rigidity but did not consistently experience loss of equilibrium even at 400% of the existing barrier’s electric field strength. Few individuals of either species died during the experiments, and this was only at very high electric field strengths.
The order parameter and director angle analysis offer complementary metrics for investigating the effects of electric fields on invertebrates. At 25% of the existing barriers’ field strength, there was no apparent change in P. clarkii behavior, but this species was clearly orienting itself to reduce exposure to the electric field. As described in the section “Materials and Methods”, this may have been influenced by the smaller size of the organisms used in this trial, which we would expect to make them less susceptible to the effects of the electric field (see section “Results”). This effect was also apparent at 50% and 100% of the barrier strength, but at 200% and above, P. clarkii individuals were less able to orient themselves. This is consistent with most individuals being rigid at and above this field strength and presumably unable to orient themselves in relation to the electric field. This effect was not observed for H. azteca, which were still able to orient themselves at 90° to the electric field at the maximum field strength of 400%. These results show that invertebrates are sensitive to electric fields even at low strengths relative to the existing CSSC barrier, and it is plausible that this sensitivity may provide a deterrent to active upstream movement.
Previous results from the CSSC show that the electric field weakens near the hulls of metal boats (Dettmers et al., 2005). Dettmers et al. (2005) confined fish to non-conductive cages attached to both non-conductive and steel-hulled boats traveling through the electric barrier to determine whether steel-hulled barges reduce the electric field immediately around their hulls, potentially allowing fish to move through the electric barriers. They found that fish swimming alongside steel-hulled boats took about three times longer to become immobilized than those swimming alongside non-conductive boats. Although measurements have not been taken at the interface of the boat and water, this suggests that fouling organisms would experience a far lower strength of electric field than a barrier puts into the water column. Fouling organisms, which are similar in size to H. azteca—such as the invasive Apocorophium lacustre—may have a similar response. For these organisms, our behavioral results suggest that even at full strength, the existing barriers are unlikely to cause dislodgement of fouling organisms from boats. While further studies investigating how metal barges affect the electric field would be helpful, the available evidence suggests that the existing barriers are unlikely to prevent the spread of organisms that move attached to hulls. Additionally, crayfish species such as P. clarkii have the ability to spread to new waterbodies through overland dispersal (Ramalho and Anastácio, 2014). Although our study does not address this method of spread, the existing electric barriers in the CAWS would be ineffective in preventing overland dispersal of P. clarkii.
The additional electric barrier being designed for the CAWS will be capable of flexible operating parameters (Charles Shea, USACE, personal communication). Our results show that very large increases in field strength would be required to appreciably change the effect on invertebrates. It is possible, however, that changes to waveform, pulse duration, and/or pulse length may make the barrier more effective for addressing the risks from invasive invertebrates. We did not experiment across changes in these parameters, but we urge that more research on this be conducted because it is possible that parameters exist, which better balance prevention of movement for fishes and invertebrates without simply increasing the strength of the electric field.
The two invertebrate species that we tested measured an average of 4.6 mm for H. azteca and 20.2 mm for juvenile P. clarkii. Previous studies have shown that organism size is an important determinant of the effects of electric fields, with larger organisms generally being more affected (Sternin et al., 1976; Miranda, 2009). Although we tested two invertebrates of varying average size, there are taxonomic and morphological differences among invertebrates that likely also affect organism response. For example, mollusks have shells and are most likely to transit the barriers attached to boats. We thus consider it unlikely that they would be strongly affected by the barriers, although this should be tested. Zooplankton, on the other hand, is most likely to drift through the barrier in the water column. Based on our results, we would not expect such organisms to be killed, and downstream drift is presumably a feasible mode of spread through the barrier. Further data on the electric barrier’s effects on a larger taxonomic range would be useful in determining parameters for future electric barriers.
A species of particular and immediate concern for spread through the CSSC and into Lake Michigan is the amphipod A. lacustre. This species is morphologically similar to H. azteca and has a distribution in the CAWS up to the Dresden Island Pool, 40 river kilometers from the existing electric barrier (Grigorovich et al., 2008; Keller et al., 2017; Egly et al., 2021) and within 100 river kilometers of Lake Michigan. Due to its proximity to the Great Lakes, its ability to withstand a wide range of environmental conditions (Szöcs et al., 2014), and the high abundances it reaches in its invasive range, which may alter food webs by displacing native amphipods (Heard, 1982; Grigorovich et al., 2005, 2008), A. lacustre is listed in the United States Army Corps of Engineers’ Great Lakes and Mississippi River Interbasin Study as a High Risk Aquatic Invasive Species (Veraldi et al., 2011). This species is known to be transported as a fouling organism on boats (Power et al., 2006). Our results indicate that the existing electric barriers are unlikely to prevent its spread, and thus that additional management will be necessary if the species is to be prevented from entering the Great Lakes.
Previous studies of electric barriers have largely focused on fishes. These have found that electric barriers can restrict movement of sea lamprey (Swink, 1999), common carp (Verrill and Berry, 1995), and Asian carps (Sparks et al., 2010; Parker et al., 2015), but there are large gaps in information regarding effectiveness of electric barriers, particularly concerning operating parameters, effects on non-target species, and how effects depend on organism size. Our study is the first of which we are aware that begins to fill gaps in the research by providing methods for construction of an electric field contained in a tank using inexpensive materials and providing data on the response of invertebrates to electric fields. Results suggest that electric barriers may deter the spread of invasive invertebrates similar to our study organisms but only if those organisms pose a risk of spreading upstream by their own locomotion. Further research into electric barriers operating with different parameters may find parameters that have stronger effects.
The raw data supporting the conclusions of this article will be made available by the authors, without undue reservation.
RE, RP, ZC, HM, and JS constructed the experimental electric barrier. RE and RK designed the experiments. RE, RP, ZC, HM, and JS conducted the experiments and analyzed the data. RE and RK wrote the manuscript with assistance from all co-authors.
Sampling was conducted under Illinois Department of Natural Resources Permit Number A18.6222. This research was financially supported by the Illinois Department of Natural Resources Grant Number RC20FW144B and Illinois Indiana Sea Grant Number F0008309702040.
The authors declare that the research was conducted in the absence of any commercial or financial relationships that could be construed as a potential conflict of interest.
Jonathan Cirone, Liz Chaparro, and Andrew Fischer aided with construction of the barrier and experiments. Colette Copic aided with field sampling. Kannon Larison, Justin Hameline, and Sarah Sukanen aided with editing the manuscript. Julia Egly and Jonathan Cirone created diagram of the barrier setup.
The Supplementary Material for this article can be found online at: https://www.frontiersin.org/articles/10.3389/fevo.2021.631762/full#supplementary-material
Supplementary Figure 1 | Behavior of juvenile Procambarus clarkii during stimulus (barrier on) and post-stimulus (barrier off) phases for (A) 25%, (B) 50%, (C) 100%, (D) 200%, (E) 300%, and (F) 400% of existing barrier strengths.
Supplementary Figure 2 | Behavior of Hyalella azteca during stimulus (barrier on) and post-stimulus (barrier off) phases for (A) 100%, (B) 200%, (C) 300%, and (D) 400% of existing barrier strengths.
Supplementary Table 1 | Size of Procambarus clarkii individuals used in experiments for different electric field strengths.
Supplementary Table 2 | Size of Hyallela azteca individuals used in experiments for different electric field strengths.
Supplementary Material 3 | Electric barrier calibration report.
Supplementary Material 4 | Order Parameter Data Collection/SolidWorks.
Andrienko, D. (2018). Introduction to liquid crystals. J. Mol. Liq. 267, 520–541. doi: 10.1016/j.molliq.2018.01.175
Benejam, L., Saura-Mas, S., Montserrat, J., Torres, F., and Macies, M. (2015). Could electric fish barriers help to manage native populations of European crayfish threatened by crayfish plague (Aphanomyces astaci)? Manag. Biol. Invasions 6, 307–310. doi: 10.3391/mbi.2015.6.3.10
BIOVIA (2018). SolidWorks. Available online at: https://www.solidworks.com/ (accessed May 28, 2019).
Biswas, K. P. (1971). On the behaviour of marine crustaceans in an electrical field of alternating current. Fish. Technol. 8, 89–97.
Biswas, K. P. (2008). Electrosensitivity and response of mud crab Scylla serrata in homogeneous and heterogeneous electric field. Zool. Res. Hum. Welf. 8, 323–332.
Bousefield, E. L. (1973). Shallow-Water Gammaridean Amphipoda of New England. Ithaca, NY: Comstock Publishing, 312.
Bunk, H., and Van Egeren, S. (2016). “Attempted eradication of ambitious architects: Procambarus clarkii, the red swamp crayfish in three SE Wisconsin Ponds – successes and failures,” in Upper Midwest Invasive Species Conference, (La Crosse, WI), 12–13.
Burger, C. V., Parkin, J. W., O’Farrell, M., and Murphy, A. (2015). Improving technology designed for deterrence offers hydroelectric facility managers options to both control and protect fish as they move through and around hydro projects. Hydro Rev. 34, Available online at: https://www.hydroreview.com/2015/06/29/barrier-technology-helps-deter-fish-at-hydro-facilities/#gref (accessed August 24, 2020).
Cruz, M. J., and Rebelo, R. (2007). Colonization of freshwater habitats by an introduced crayfish, Procambarus clarkii, in Southwest Iberian Peninsula. Hydrobiologia 575, 191–201. doi: 10.1007/s10750-006-0376-9
Dettmers, J. M., Boisvert, B., Barkley, T., and Sparks, R. E. (2005). Potential Impact of Steel-Hulled Barges on Movement of Fish Across an Electric Barrier to Prevent the Entry of Invasive Carp Into Lake Michigan. Zion, IL: Illinois State Natural History Survey.
Donato, R., Rollandin, M., Favaro, L., Ferrarese, A., Pessani, D., and Ghia, D. (2018). Habitat use and population structure of the invasive red swamp crayfish Procambarus clarkii (Girard, 1852) in a protected area in northern Italy. Knowl. Manag. Aquat. Ecosyst. 2018:10. doi: 10.1051/kmae/2018002
Egly, R. M., Annis, G. M., Lindsay Chadderton, W., Peters, J. A., and Larson, E. R. (2019). Predicting the potential distribution of the non-native Red Swamp Crayfish Procambarus clarkii in the Laurentian Great Lakes. J. Great Lakes Res. 45, 150–159. doi: 10.1016/j.jglr.2018.11.007
Egly, R. M., O’Shaughnessey, E. M., and Keller, R. P. (2021). Updated occurrence data and species distribution modeling of the invasive amphipod Apocorophium lacustre in North America. Freshw. Sci. 40, 162–174. doi: 10.1086/713071
Ellison, G. (2015). Invasive Crayfish Discovery Sparks DNR Trapping Effort in Lake Macatawa | MLive.com. Mlive Media Gr. Available online at: http://www.mlive.com/news/grand-rapids/index.ssf/2015/07/red_swamp_crayfish_michigan.html (accessed November 2, 2017).
Faasse, M., and van Moorsel, G. (2003). The north-american amphipods, Melita nitida (Smith, 1873) and Incisocalliope aestuarius (Watling and Maurer, 1973) (Crustacea: Amphipoda: Gammaridea), introduced to the Western Scheldt estuary (The Netherlands). Aquat. Ecol. 37, 13–22. doi: 10.1023/A:1022120729031
Fossat, P., Bacqué-Cazenave, J., De Deurwaerdère, P., Delbecque, J. P., and Cattaert, D. (2014). Anxiety-like behavior in crayfish is controlled by serotonin. Science 344, 1293–1297. doi: 10.1126/science.1248811
Gherardi, F., and Acquistapace, P. (2007). Invasive crayfish in Europe: the impact of Procambarus clarkii on the littoral community of a Mediterranean lake. Freshw. Biol. 54, 1249–1259. doi: 10.1111/j.1365-2427.2007.01760.x
Grigorovich, I. A., Angradi, T. R., Emery, E. B., and Wooten, M. S. (2008). Invasion of the Upper Mississippi River system by saltwater amphipods. Fundam. Appl. Limnol. 173, 67–77. doi: 10.1127/1863-9135/2008/0173-0067
Grigorovich, I. A., Kang, M., and Ciborowski, J. J. H. (2005). Colonization of the laurentian great lakes by the amphipod Gammarus tigrinus, a native of the North American Atlantic coast. J. Great Lakes Res. 31, 333–342. doi: 10.1016/S0380-1330(05)70264-1
Halsband, E. (1967). “Basic principles of electric fishing,” in Fishing with Electricity: Its Application to Biology and Management, ed. R. Vibert (Rome: Food and Agriculture Organization of the United Nations), 57–64.
Heard, R. W. (1982). Guide to Common Tidal Marsh Invertebrates of the Northeastern Gulf of Mexico. Biloxi, MS: Mississippi-Alabama Sea Grant Consortium, 82.
Holeck, K. T., Mills, E. L., Margaret, H. J. M., Robert, R. D., Colautti, I., and Ricciardi, A. (2004). Bridging troubled waters: biological invasions, transoceanic shipping, and the Laurentian Great Lakes. Bioscience 54, 919–929.
Holliman, F. M., Killgore, K. J., and Shea, C. (2015). Development of Operational Protocols for Electric Barrier Systems on the Chicago Sanitary and Ship Canal: Induction of passage-preventing behaviors in small sizes of Silver Carp. Vicksburg, MA: U.S. Army Engineer Research and Development Center.
Holman, L., Head, M. L., Lanfear, R., and Jennions, M. D. (2015). Evidence of experimental bias in the life sciences: why we need blind data recording. PLoS Biol. 13:e1002190. doi: 10.1371/journal.pbio.1002190
Jacobs, A. I., and Keller, R. P. (2017). Straddling the divide: invasive aquatic species in Illinois and movement between the Great Lakes and Mississippi basins. Biol. Invasions 19, 635–646. doi: 10.1007/s10530-016-1321-0
Kates, D., Dennis, C., Noatch, M. R., and Suski, C. D. (2012). Responses of native and invasive fishes to carbon dioxide: potential for a nonphysical barrier to fish dispersal. Can. J. Fish. Aquat. Sci. 69, 1748–1759. doi: 10.1139/f2012-102
Keller, R. P., Drake, J. M., Drew, M. B., and Lodge, D. M. (2011). Linking environmental conditions and ship movements to estimate invasive species transport across the global shipping network. Divers. Distrib. 17, 93–102. doi: 10.1111/j.1472-4642.2010.00696.x
Keller, R. P., Habeeb, G., Henry, T., and Brenner, J. (2017). Non-native amphipod, Apocorophium lacustre (Vanhoffen, 1911), in the Illinois River and Chicago Area Waterway System. Manag. Biol. Invasions 8, 377–382. doi: 10.3391/mbi.2017.8.3.11
Kim, J., and Mandrak, N. E. (2017). Effects of vertical electric barrier on the behaviour of common carp. Manag. Biol. Invasions 8, 497–505. doi: 10.3391/mbi.2017.8.4.04
Kolar, C. S., Chapman, D. C., Courtenay, W. R. J., Housel, C. M., Williams, J. D., and Jennings, D. P. (2005). Asian Carps of the Genus Hypophthalmichthys (Pisces, Cyprinidae) - A Biological Synopsis and Environmental Risk Assessment. Available online at: https://digitalcommons.unl.edu/ (accessed March 11, 2020).
Kornis, M. S., Mercado-Silva, N., and Vander Zanden, M. J. (2012). Twenty years of invasion: a review of round goby Neogobius melanostomus biology, spread and ecological implications. J. Fish Biol. 80, 235–285. doi: 10.1111/j.1095-8649.2011.03157.x
Lamarque, P. (1967). “Electrophysiology of fish subject to the action of an electric field,” in Fishing with Electricity: Its Application to Biology and Management, ed. R. Vibert (London: Food and Agriculture Organization of the United Nations), 63–92.
Larson, E. R., and Olden, J. D. (2012). Crayfish occupancy and abundance in lakes of the Pacific Northwest. USA. Freshw. Sci. 32, 94–107. doi: 10.1899/12-051.1
Lodge, D. M., Simonin, P. W., Burgiel, S. W., Keller, R. P., Bossenbroek, J. M., Jerde, C. L., et al. (2016). Risk analysis and bioeconomics of invasive species to inform policy and management. Annu. Rev. Environ. Resour. 41, 453–488. doi: 10.1146/annurev-environ-110615-085532
Manfrin, C., Souty-Grosset, C., Anastácio, P., Reynolds, J., and Giulianini, P. (2019). Detection and control of invasive freshwater crayfish: from traditional to innovative methods. Diversity 11:5. doi: 10.3390/d11010005
Matsuzaki, S. S., Usio, N., Takamura, N., and Washitani, I. (2009). Contrasting impacts of invasive engineers on freshwater ecosystems: an experiment and meta-analysis. Oecologia 158, 673–686. doi: 10.1007/s00442-008-1180-1
Miranda, L. E. (2009). “Standardizing electrofishing power for boat electrofishing: chapter 14,” in Standard Methods for Sampling North American Freshwater Fishes, eds S. A. Bonar, W. A. Hubert, and D. W. Willis (Bethesda, MD: American Fisheries Society), 223–230.
Oficialdegui, F. J., Clavero, M., Sánchez, M. I., Green, A. J., Boyero, L., Michot, T. C., et al. (2019). Unravelling the global invasion routes of a worldwide invader, the red swamp crayfish (Procambarus clarkii). Freshw. Biol. 64, 1382–1400. doi: 10.1111/fwb.13312
Paglianti, A., and Gherardi, F. (2004). Combined effects of temperature and diet on growth and survival of young-of-year crayfish: a comparison between indigenous and invasive species. J. Crustac. Biol. 24, 140–148. doi: 10.1651/C-2374
Parker, A. D., Glover, D. C., Finney, S. T., Rogers, P. B., Stewart, J. G., and Simmonds, R. L. (2015). Direct observations of fish incapacitation rates at a large electrical fish barrier in the Chicago Sanitary and Ship Canal. J. Great Lakes Res. 41, 396–404. doi: 10.1016/j.jglr.2015.03.004
Payne, B. S., Bingham, C. R., and Miller, A. C. (1989). Life History and Production of Dominant Larval Insects on Stone Dikes in the Lower Mississippi River. Vicksburg, MS: U.S. Army Engineer Waterways Experiment Station.
Perrot-Minnot, M.-J., Banchetry, L., and Cézilly, F. (2017). Anxiety-like behaviour increases safety from fish predation in an amphipod crustacea. R. Soc. Open Sci. 4, 171558. doi: 10.1098/rsos.171558
Peters, J. A., Cooper, M. J., Creque, S. M., Kornis, M. S., Maxted, J. T., Perry, W. L., et al. (2014). Historical changes and current status of crayfish diversity and distribution in the Laurentian Great Lakes. J. Great Lakes Res. 40, 35–46. doi: 10.1016/j.jglr.2014.01.003
Pimentel, D., Zuniga, R., and Morrison, D. (2005). Update on the environmental and economic costs associated with alien-invasive species in the United States. Ecol. Econ. 52, 273–288. doi: 10.1016/j.ecolecon.2004.10.002
Power, A. M., Mitchell, M., Walker, R., Posey, M., Alphin, T., and Belcher, C. (2006). Baseline Port Surveys for Introduced Marine Molluscan, Crustacean and Polychaete Species in the South Atlantic Bight. Washington, DC: NOAA.
Ramalho, R. O., and Anastácio, P. M. (2014). Factors inducing overland movement of invasive crayfish (Procambarus clarkii) in a ricefield habitat. Hydrobiologia 746, 135–146. doi: 10.1007/s10750-014-2052-9
Rodríguez, C. F., Bécares, E., and Fernández-Aláez, M. (2003). Shift from clear to turbid phase in Lake Chozas (NW Spain) due to the introduction of American red swamp crayfish (Procambarus clarkii). Hydrobiologia 506–509, 421–426. doi: 10.1023/B:HYDR.0000008626.07042.87
Shoemaker, C. R. (1934). The amphipod genus Corophium on the east coast of America. Proc. Biol. Soc. Washingt. 47, 23–32.
Smith, K., Roth, B. M., Herbst, S. J., Thoma, R. F., Popoff, N., Hayes, D. B., et al. (2018). Assessment of invasion risks for red swamp crayfish (Procambarus clarkii) in Michigan, USA. Manag. Biol. Invasions 9, 405–415. doi: 10.3391/mbi.2018.9.4.04
Sparks, R. E., Barkley, T. L., Creque, S. M., Dettmers, J. M., and Stainbrook, K. M. (2010). Evaluation of an electric fish dispersal barrier in the Chicago Sanitary and Ship Canal. Am. Fish. Soc. Symp. 74, 139–161.
Sternin, V. G., Nikonorov, I. V., Bumeister, Y. K., Sternin, V. G., Nikonorov, I. V., and Bumeister, Y. K. (1976). Electrical Fishing: Theory and Practice. Jerusalem. Available online at: https://ui.adsabs.harvard.edu/abs/1976STIN.7623480S/abstract (accessed October 16, 2020).
Strayer, D. L. (2010). Alien species in fresh waters: ecological effects, interactions with other stressors, and prospects for the future. Freshw. Biol. 55, 152–174. doi: 10.1111/j.1365-2427.2009.02380.x
Suski, C. D. (2020). Development of carbon dioxide barriers to deter invasive fishes: Insights and lessons learned from Bigheaded Carp. Fishes 5:25. doi: 10.3390/fishes5030025
Swink, W. D. (1999). Effectiveness of an electrical barrier in blocking a sea lamprey spawning migration on the Jordan River, Michigan. North Am. J. Fish. Manag. 19, 397–405. doi: 10.1577/1548-86751999019<0397:eoaebi<2.0.co;2
Szöcs, E., Coring, E., Bäthe, J., and Schäfer, R. B. (2014). Effects of anthropogenic salinization on biological traits and community composition of stream macroinvertebrates. Sci. Total Environ. 468–469, 943–949. doi: 10.1016/j.scitotenv.2013.08.058
Treanor, H. B., Ray, A. M., Layhee, M., Watten, B. J., Gross, J. A., Gresswell, R. E., et al. (2017). Using carbon dioxide in fisheries and aquatic invasive species management. Fisheries 42, 621–628. doi: 10.1080/03632415.2017.1383903
Twardochleb, L. A., Olden, J. D., and Larson, E. R. (2013). A global meta-analysis of the ecological impacts of nonnative crayfish. Freshw. Sci. 32, 1367–1382. doi: 10.1899/12-203.1
United State Fish and Wildlife Service (2017). Electrofishing: Backpack Calibration. Washington, DC: US Fish and Wildlife Service.
USACE (2011). Dispersal Barrier Efficacy Study – Interim Report IIA: USGS Red Swamp Crayfish (Procambarus clarkii) Factsheet. Chicago, IL: USACE.
Vannini, M., and Insom, E. (1976). Notes on the behaviour of crustacean decapods in an electric field. Ital. J. Zool. 10, 265–269. doi: 10.1080/00269786.1976.10736271
Veraldi, F. M., Baerwaldt, K., Herman, B., Herleth-King, S., Shanks, M., Kring, L., et al. (2011). Non-Native Species of Concern and Dispersal Risk for the Great Lakes and Mississippi River Interbasin Study. Chicago, IL: US Army Corps of Engineers, 35.
Keywords: invasive species, crayfish, Procambarus clarkii, Apocorophium lacustre, Chicago Sanitary and Ship Canal, amphipod
Citation: Egly RM, Polak RD, Cook ZA, Moy HD, Staunton JT and Keller RP (2021) Development and First Tests of a Lab-Scale Electric Field for Investigating Potential Effects of Electric Barriers on Aquatic Invasive Invertebrates. Front. Ecol. Evol. 9:631762. doi: 10.3389/fevo.2021.631762
Received: 20 November 2020; Accepted: 22 March 2021;
Published: 30 April 2021.
Edited by:
Ana Sofia Vaz, University of Granada, SpainReviewed by:
Alexandre Mestre, University of Valencia, SpainCopyright © 2021 Egly, Polak, Cook, Moy, Staunton and Keller. This is an open-access article distributed under the terms of the Creative Commons Attribution License (CC BY). The use, distribution or reproduction in other forums is permitted, provided the original author(s) and the copyright owner(s) are credited and that the original publication in this journal is cited, in accordance with accepted academic practice. No use, distribution or reproduction is permitted which does not comply with these terms.
*Correspondence: Rachel M. Egly, cmVnbHlAbHVjLmVkdQ==
Disclaimer: All claims expressed in this article are solely those of the authors and do not necessarily represent those of their affiliated organizations, or those of the publisher, the editors and the reviewers. Any product that may be evaluated in this article or claim that may be made by its manufacturer is not guaranteed or endorsed by the publisher.
Research integrity at Frontiers
Learn more about the work of our research integrity team to safeguard the quality of each article we publish.