- 1Endocrinology Laboratory, German Primate Center, Leibniz Institute for Primate Research, Göttingen, Germany
- 2Max Planck Institute for Evolutionary Anthropology, Leipzig, Germany
- 3Research Group Evolutionary Physiology, Max Planck Institute for Ornithology, Seewiesen, Germany
- 4Behavioral Ecology and Ecophysiology, Department of Biology, University of Antwerp, Antwerp, Belgium
- 5Faculty of Science, School of Biological and Environmental Sciences, Liverpool John Moores University, Liverpool, United Kingdom
- 6Centre for Research and Conservation, Royal Zoological Society of Antwerp, Antwerp, Belgium
- 7Department of Anthropology, Center for the Advanced Study of Human Paleobiology, The George Washington University, Washington, DC, United States
Ecoimmunology conceptualizes the role of immunity in shaping life history in a natural context. Within ecoimmunology, macroimmunology is a framework that explains the effects of habitat and spatial differences on variation in immune phenotypes across populations. Within these frameworks, immune ontogeny—the development of the immune system across an individual life span—has received little attention. Here, we investigated how immune ontogeny from birth until adulthood is affected by age, sex, and developmental environment in a long-lived primate species, the bonobo. We found a progressive, significant decline of urinary neopterin levels, a marker for the cell-mediated immune response, from birth until 5 years of age in both sexes. The overall pattern of age-related neopterin changes was sex-specific, with males having higher urinary neopterin levels than females in the first 3 years of life, and females having higher levels than males between 6 and 8 years. Environmental condition (zoo-housed vs. wild) did not influence neopterin levels, nor did age-related changes in neopterin levels differ between environments. Our data suggest that the post-natal development of cell-mediated immune ontogeny is sex-specific but does not show plasticity in response to environmental conditions in this long-lived primate species. This indicates that cell-mediated immune ontogeny in the bonobo follows a stereotypic and maybe a genetically determined pattern that is not affected by environmental differences in pathogen exposure and energy availability, but that sex is an important, yet often overlooked factor shaping patterns of immune ontogeny. Investigating the causes and consequences of variation in immunity throughout life is critical for our understanding of life-history evolution and strategies, mechanisms of sexual selection, and population dynamics with respect to pathogen susceptibility. A general description of sex-specific immune ontogeny as done here is a crucial step in this direction, particularly when it is considered in the context of a species’ ecology and evolutionary history.
Introduction
Ecoimmunology uses an integrative approach to estimate costs, benefits, and fitness consequences of different immune defense strategies, and relates variation in immune responses to phylogeny, sociality, and ecology (Martin et al., 2006; Demas and Nelson, 2012; Schoenle et al., 2018). Since the emergence of this discipline, research has focused on how immunity—the capacity to resist a particular pathogen—affects life history strategies within species (Brock et al., 2014). Macroimmunology is a framework within ecoimmunology that considers habitat- and spatial-specific differences in immune phenotypes across populations (Becker et al., 2020; Forbes, 2020). While previous studies have focused on variation in immunity of adult individuals, immune ontogeny—the development of the immune system across an individuals’ life span—as a determining factor for variation and plasticity in immune functioning has remained largely unexplored (Goenka and Kollmann, 2015). Preliminary evidence suggests that immunity, but also exposure to immune challenges, can vary across life stages (Beirne et al., 2016; Peters et al., 2019). It is also reasonable to assume that sex-specific differences in development affect immune ontogeny (Love et al., 2008). Additionally, developmental processes are often sensitive to environmental cues, and it is plausible that these parameters also affect immune ontogeny (Martin et al., 2006).
The two branches of the vertebrate immune system, innate immunity (non-specific immune response) and adaptive or acquired immunity (specific immune response), share two response trajectories, the cell-mediated and the humoral response (Murphy and Weaver, 2018). Vertebrates are born with functioning immune systems, but immature and adult individuals differ in immune functionality. These differences in immunity diminish with increasing age (Dowling and Levy, 2014; Simon et al., 2015; Georgountzou and Papadopoulos, 2017). After birth, infants depend mainly on innate immunity because their exposure to antigens in utero is low or absent (PrabhuDas et al., 2011; Dowling and Levy, 2014). During the postnatal phase, the exposure to environmental pathogens stimulates adaptive immune responses and the immune system becomes less tolerant (West, 2002; Goenka and Kollmann, 2015; Simon et al., 2015). There is evidence for ontogenetic changes in immune functioning in humans, and support for this comes from a recent study showing a peak in immune functioning between 5 and 14 years of age (Glynn and Moss, 2020).
Macroimmunology considers variation of environmental parameters such as resource availability, pathogen exposure and diversity on a spatial scale and relates this information to variation in immune system functioning (Becker et al., 2020; Forbes, 2020). The intensity and diversity of pathogen exposure during ontogeny is hypothesized to be an important driver determining adult immunity (McDade, 2003, 2012). Exposure to fewer but probably novel pathogens is expected to favor innate over adaptive immune responses. Correspondingly, populations with high but more familiar pathogen exposure, are predicted to develop adaptive immunity earlier in life (McDade et al., 2016). Contrary to these predictions, innate immunity markers were elevated in human populations with higher pathogen exposure (McDade, 2003; Teran et al., 2011). Environmental differences affect cell-mediated responses in adult chimpanzees, where individuals from a wild population had higher activity of cell-mediated responses than those living in a zoo environment (Behringer et al., 2019). Adult phenotypes that effect health can be the product of differences in developmental processes in response to environmental circumstances (Bateson et al., 2004). To consider the modulatory effect of pathogen exposure on immune ontogeny, comparative data from populations living in different pathogen environments are needed. One way to understand immune ontogeny in relation to ecological pressures and across evolutionary times, is to investigate free-living, genetically diverse, and energetically limited populations of animals using ecoimmunological and macroimmunological approaches (Demas and Nelson, 2012; Forbes, 2020). Comparing wild and captive populations provides an ideal setting to investigate environmental drivers of immune ontogeny because of differences in dietary energy availability and pathogen exposure. Although wild populations are probably exposed to a higher quantity of pathogens, zoo-housed animals are in close contact to humans which may increase the risk of transmission of zoonotic infections and exposure to new pathogens. This is particularly relevant for great apes because of their high susceptibility to human pathogens (Calvignac-Spencer et al., 2012; Dunay et al., 2018). In addition, zoo-housed populations usually receive intense medical care when infected, and this may also affect the strength and quality of their immunity (Courtenay and Santow, 1989). Furthermore, most zoo-housed individuals enjoy a superior and more stable energy supply which permits higher investments into immune system functioning during ontogeny and adulthood without compromising e.g., growth (Urlacher et al., 2018). These differences in intensity and diversity of pathogen exposure and energy supply between wild and zoo environments are therefore ideal to test hypotheses about ontogenetic drivers of adult immunity.
In humans and other mammals, immunity differs between the sexes. Adult females have fewer infections, stronger antibody responses, but also greater vulnerability to autoimmune diseases than males (e.g., Klein and Roberts, 2010; Klein and Flanagan, 2016; Metcalf et al., 2020; but see Kelly et al., 2018). These differences in immunity are seen as sex-specific trade-offs in resource allocation favoring immunity or reproduction (Zuk, 2009). Sex hormones with higher concentrations in females (i.e., estrogens) enhance immune functioning, but those with higher concentrations in males (i.e., androgens) are thought to negatively affect immune responses (Zuk, 2009; Fischer et al., 2015; Kelly et al., 2018) and support behaviors that make adult males more susceptible to infections (Klein, 2000). For example, estradiol increases immune responses associated with pro-inflammatory processes. In contrast, testosterone biases the specific cell-mediated immune response toward anti-inflammatory processes (Fischer et al., 2015; Klein and Flanagan, 2016; Fathi et al., 2021). These interactions between sex steroids and cell-mediated immunity suggest that increases in estradiol and testosterone with the onset of sexual maturation in females and males, respectively, can produce similar sex-specific effects on immune system functioning during development. While sex differences in adult immunity are well described, information about sex-specific immune ontogeny is rare (Fish, 2008; Klein and Flanagan, 2016). For example, inflammatory processes are higher in pre-pubertal boys than girls, but after puberty this relationship is reversed (Yang and Kozloski, 2011; Klein and Flanagan, 2016), and many vaccinations induce a greater antibody response and some a greater cell-mediated response in girls than in boys (Flanagan et al., 2017; Fathi et al., 2021). So far, the few studies available indicate that immune ontogeny in humans is sex-specific and we can therefore expect to find similar differences in the immune ontogeny in non-human primate species.
Like in humans, the life histories of great apes (Hominidae), humans’ closest living relatives, are characterized by a long developmental period. Maturation until adulthood accounts for one third of the total lifespan, the period between the onset of sexual maturation and first reproduction is relatively long, and there is a considerable gap between the time when adult body size is reached and the emergence of reproductive competence (Lancaster and Hamburg, 2008; Robson and Wood, 2008; Walker et al., 2018). On an ultimate level, variation in immune ontogeny is assumed to be affected by a species’ pace-of-life. Apes differ in their innate immune strategy from monkeys with shorter lifespans and a faster pace-of-life. The energetically costly strategy of sterilization over specificity which apes employ is thought to have evolved as a response to higher risk of pathogen exposure during their slow life history or past pathogen exposure (Hawash et al., 2021). So far, studies on the influence of environment and sex on ontogenetic changes in immunity have focused on species with short lifespans and fast pace-of-life. In long-lived species, their long developmental periods provide more time for adaptive adjustments in life history trade-offs in response to environmental factors than in short-lived species. Those adjustments can then lead to a larger variation of functional immune phenotypes (Simon et al., 2015; McDade et al., 2016). In this regard, humans are the best studied long-lived species so far, but many aspects of human immunology have only been studied in the context of pathologies (McDade, 2003; Martin et al., 2006; Tieleman, 2018), and studies documenting the variation in “healthy” immune ontogeny are scarce. The biotopes inhabited by African apes vary in terms of climate, forest cover, faunal composition, and in the frequency and intensity of human contact. Therefore, it is reasonable to assume that pathogen exposure varies accordingly, and that this manifests in species-specific resistance and susceptibility to pathogens. For example, ape species seem to differ in their susceptibility to simian immunodeficiency virus (SIV) (Gao et al., 1999; Li et al., 2012), and differences in pathogen exposure have probably shaped their immune systems (de Groot et al., 2002) and maybe affect their current distribution (Inogwabini and Leader-Williams, 2012). Therefore, great apes are an ideal model taxon to investigate sex-specificities and environmental impacts on the immune ontogeny of slow-paced species that might explain variation in disease susceptibility and range distribution within and between populations.
Within the African great apes, bonobos (Pan paniscus) and chimpanzees (P. troglodytes) are closely related to each other, and to humans. Given the importance of developmental changes for the reconstruction of phylogenetic trends, there is increasing interest in the postnatal development of bonobos and chimpanzees (Hare and Yamamoto, 2015; Lee et al., 2020; Behringer et al., 2020). Yet, information on immune ontogeny in these species is rare. Bonobos are particularly interesting for studying immunity and its ontogeny because recent studies revealed that their major histocompatibility complex (MHC) class I profile has undergone selection reducing its variability compared to humans and chimpanzees, presumably due to selection for malaria protecting MHC allotypes (e.g., Wroblewski et al., 2017; de Groot et al., 2018; Maibach and Vigilant, 2019). It is assumed that such a reduction in genetic diversity of the MHC influences the functioning of immunity in this species (Sommer, 2005; de Groot et al., 2017). Additionally, the onset of sexual maturation in female bonobos occurs about three years earlier than in males (Behringer et al., 2014). Therefore, sex-specific differences in immune ontogeny are predicted to occur at different ages.
In our study, we focus on cell-mediated immune ontogeny. Cell-mediated immunity targets intracellular pathogens such as all viruses (e.g., Human alphaherpesvirus 3), certain bacteria (e.g., Mycobacterium leprae and M. tuberculosis), and certain protozoa (e.g., Plasmodium falciparum and Leishmania spp.) (Thakur et al., 2019). The activation of cell-mediated immunity can be assessed by measuring neopterin, a biomarker mainly produced by monocyte-derived macrophages and dendritic cells. Neopterin can be readily measured in blood and urine (Fuchs et al., 1992; Hoffmann et al., 2003; Winkler et al., 2003a), the latter enabling studies on cell-mediated immunity to be conducted non-invasively in natural settings with exposure to a natural set of pathogens.
In this study, we investigated the effects of age, environment (wild vs. zoo-housed), and sex on cell-mediated immune ontogeny in bonobos. We measured urinary neopterin as a marker of cell-mediated immunity in individuals ranging in age between birth until 18 years of age. Using human studies as a conceptual benchmark, we considered the impact of age, environment and sex on immune ontogeny and tested the following predictions: (1) Age: If the immune system shifts from predominantly cell-mediated to more humoral responses during the first years of life, neopterin levels will be elevated in infant bonobos and decline with age. (2) Environment: If the pattern of immune ontogeny is plastic in response to pathogen exposure and energy availability, we expect higher neopterin levels and a later decline in individuals from wild populations compared to those from captive populations. (3) Sex: If sex-specific developmental processes such as the increase of sex steroid hormones with the beginning of sexual maturation affect immune ontogeny, we expect neopterin levels to decline about three years earlier in females than in males.
Materials and Methods
Study Sites and Subjects
Between June 2008 and October 2019, 597 urine samples (female: 375; male: 222) were collected from two wild bonobo communities (Bompusa East and Bompusa West community) at the LuiKotale field site, Democratic Republic of the Congo (Hohmann and Fruth, 2003). All subjects were habituated to human presence before the start of the study period and all were individually known. Samples represent 34 individual females (average: 11 samples per individual) and 21 individual males (average: 11 samples per individual). For wild subjects, the month and year of birth were known for 13 individuals, and for these we set the date of birth to the 15th of the respective month. For 12 individuals only the year of birth was known, and we set the birth date to June 15th of the respective year. For the remaining 30 individuals exact birthdates are known. Zoo-housed bonobos were sampled between January 2012 and September 2018, and 237 urine samples (female: 137; male: 100) were collected in nine different zoos. These samples represent 49 individual females (average: three samples per individual) and 26 individual males (average: four samples per individual). All zoo-housed bonobos were of known age and were housed in mixed-sex groups of different sizes. Age range was 0–18 years of age for both populations (zoo average: eight years; wild average: five years). All samples were collected at a time when individuals did not show symptoms of infection or injury (e.g., running noses, coughing or sneezing, visible wounds).
Urine Collection
Urine samples were collected opportunistically throughout the day between 6:00 and 20:00 h. Samples were collected on plastic sheets or the floor for zoo-housed bonobos, and from vegetation for wild animals. Samples were protected from direct sunlight to avoid neopterin degradation (Fuchs et al., 1992; Behringer et al., 2017) and were excluded when contamination with feces was detected. In the field, urine samples were frozen in liquid nitrogen upon arrival at camp on the same day. Zoo samples were frozen immediately after collection. All urine samples were transported frozen to the Max Planck Institute for Evolutionary Anthropology in Leipzig, Germany, and later to the German Primate Center, Göttingen, Germany for analysis.
Sample Preparation and Neopterin Measurement
Prior to analysis, urine samples were thawed, vortex-mixed for 10 seconds, and subsequently centrifuged for 5 minutes. We measured neopterin levels with a commercial neopterin ELISA kit (Neopterin ELISA, Ref. RE59321, IBL International GmbH, Hamburg, Germany), previously validated for use with bonobo urine (Behringer et al., 2017). Initially, we determined specific gravity (SG) in all samples using a digital handheld refractometer (TEC, Ober-Ramstadt, Germany). SG population average was 1.005 for zoo-housed individuals, and 1.011 for wild bonobos. Highly diluted samples with a SG of <1.003 were excluded from the data set (N = 28). Prior to neopterin measurement, urine samples were diluted (1:10–1:200 depending on SG) with the provided assay buffer, and samples were measured in duplicate according to the supplier’s instructions (for details see Behringer et al., 2017). Inter-assay variation for high- and low-value quality controls was 5.8 and 6.1% (N = 28 assays), respectively. Intra-assay variation was 6.4%. All neopterin concentrations were adjusted for variation in urine volume and concentration using the samples’ specific gravity (SG) value (Miller et al., 2004). Final neopterin concentrations are expressed in ng/ml corrected for SG.
Statistics
Expecting age-related but non-linear patterns in urinary neopterin levels, we fitted a generalized additive mixed model (GAM) (Wood, 2011) with gaussian-identity link function using R (R Development Core Team, 2008) to our data. The GAM is composed of a sum of smooth functions of covariates and provides a structure for generalizing a general linear model by allowing additivity of non-linear functions of the variables (Wood, 2004, 2017; Ravindra et al., 2019). We used the R package “mgcv” version 1.8–27 (Wood, 2017) to investigate age-related changes in urinary neopterin from birth through early adulthood (18 years of age). Urinary neopterin levels were log-transformed. Sex and environmental condition (zoo-housed, wild) were included as ordered factors, and daytime of sample collection as a control predictor. To model changes in neopterin levels with age, we included age as a smooth term with a penalized cubic regression. We included interaction-like terms of age with sex, and age with environmental condition by using the “by” argument to the smooth term (Wood, 2017). To control for time between sample collection until measurement, we included sample storage time as a smooth term. Sample storage time ranged between 6 months and nearly 12 years. Because sex and environmental condition are ordered factors, an indicator vector is generated for each level, but not for the first level of the ordered factor. Individual identity and zoo/community were included as random effects to account for unequal and repeated sampling of individuals across ages and environments, and both were included in random slopes with age. The basis dimension, k was set to 5. The choice of basis dimensions determines the maximum possible degrees of freedom allowed for each model term (Wood, 2017). To control for the potential influence of time of sample collection, this parameter was included as a fixed effect in the model as a control predictor. To investigate sex differences, we ran a difference plot using the package “itsadug” (van Rij et al., 2020) implemented in the R package “mgcv.” Differences between the sexes were estimated from the GAM model, in which the smooth term for the difference between males and females is calculated, including confidence intervals (CIs). We compared the full with the null model using “CompareML.” The null model included only the control and random factors, as well as random slopes. Diagnostics were done using “gam.check.” Model assumptions were assessed by visual inspections of a histogram, a q–q plot of the residuals, and by plotting residuals against fitted values and basis dimension. The lowest k-index <1 was 0.43 and all other model assumptions were met. Concurvity, the situation where a smooth term can be approximated by some combination of the others, was not an issue.
Results
The full—null model comparison revealed significance (Df = 10; p < 0.001). From birth until the age of five, urinary neopterin levels declined significantly and progressively with age and remained mostly stable at older ages (Figures 1, 2). Environmental conditions did not explain differences in neopterin levels in our data set (Table 1 and Figure 1, Penvironment = 0.924) or changes in urinary neopterin levels with age (Table 1 smooth terms and Figure 1). Sex did not predict neopterin levels in our dataset (Table 1, Psex = 0.865), but the smooth function for the interaction-like term for age with sex was significant. This indicates that age-related changes of urinary neopterin levels differ between the sexes (Table 1 smooth terms and Figure 2). Independent of the environmental context, urinary neopterin levels were significantly higher in males than in females during the first three years of life (Figure 3). This pattern reversed at around six years when neopterin levels of females increased successively until they became significantly higher than the levels of males (Figure 3).
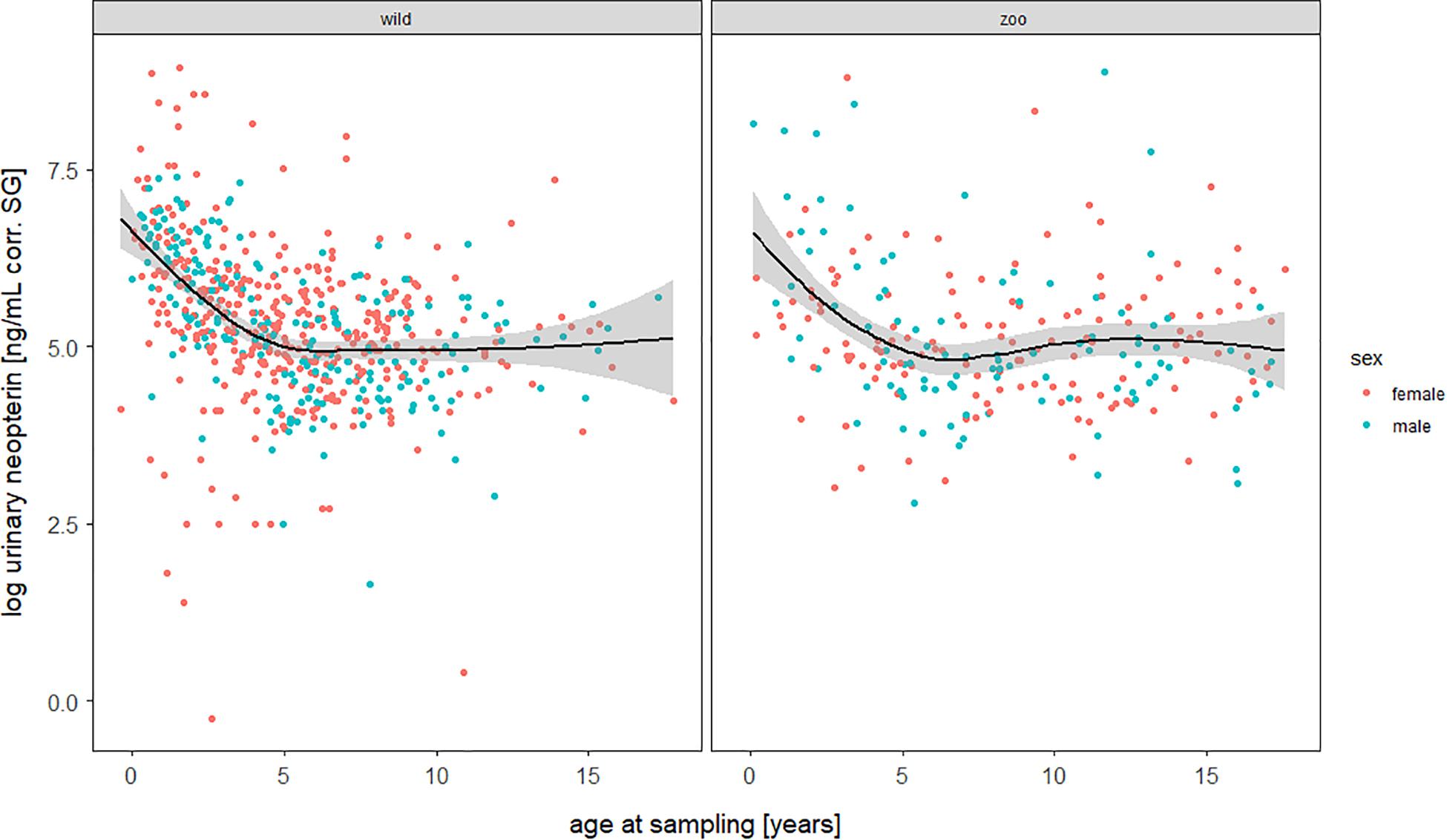
Figure 1. Log-transformed urinary neopterin levels corrected for specific gravity (corr. SG) obtained from wild (left) and zoo-housed (right) bonobos in relation to chronological age at sampling. Each filled circle represents a sample: females in red, males in blue. Lines represent the fitted sigmoidal model for the data set. Shaded areas represent bootstrapped 95% confidence intervals for expected urinary neopterin levels. Total N = 806 urine samples, Nwild = 578 samples, Nzoo–housed = 228 samples.
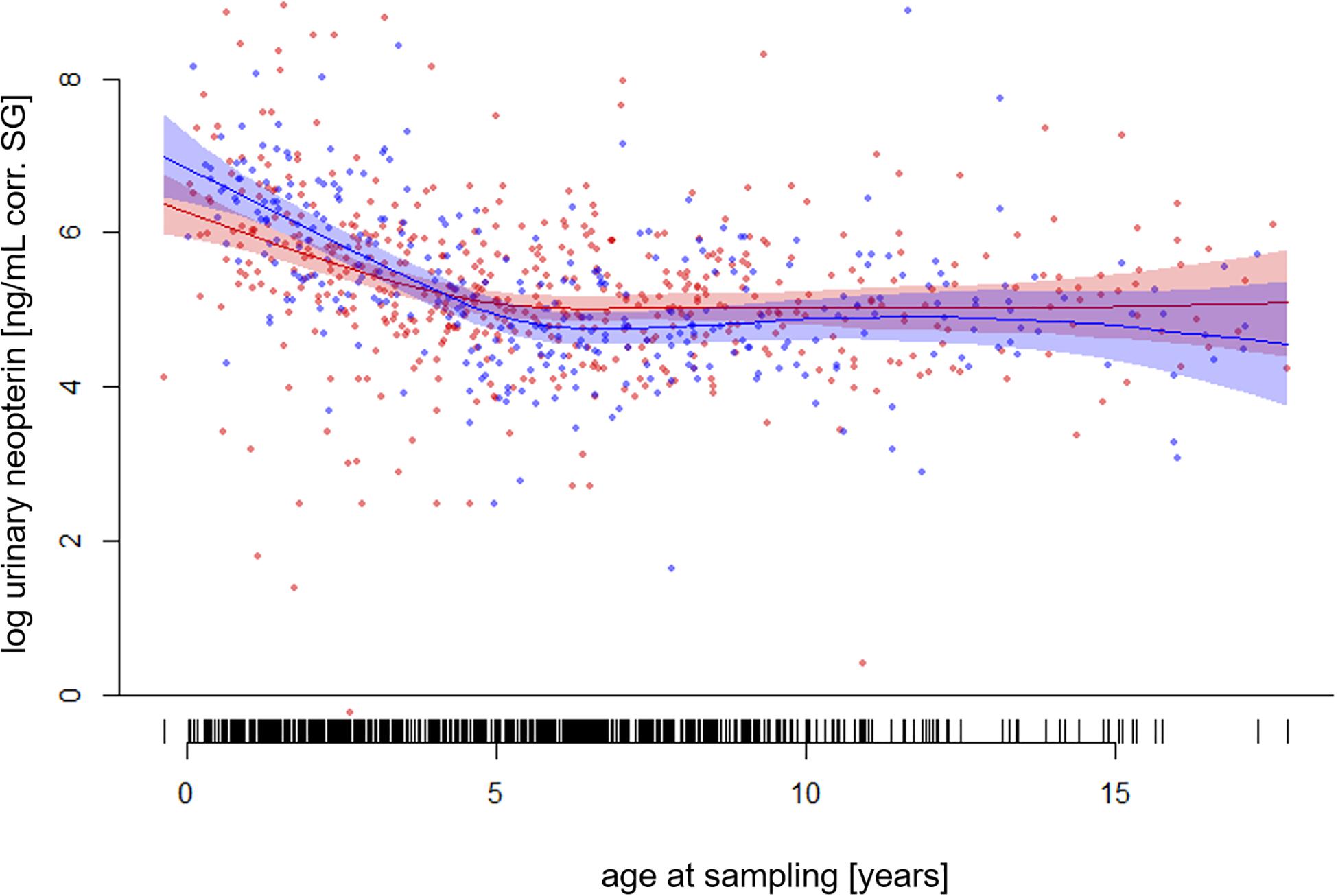
Figure 2. Log-transformed urinary neopterin levels corrected for specific gravity (corr. SG) obtained from wild and zoo-housed female (red) and male (blue) bonobos in relation to chronological age at sampling. Each filled circle and vertical black line at the buttom represents a sample. Lines represent the fitted model for the data set. Shaded areas represent bootstrapped 95% confidence intervals for expected urinary neopterin levels. Total N = 806 urine samples, Nfemale = 495 samples, Nmale = 311 samples.
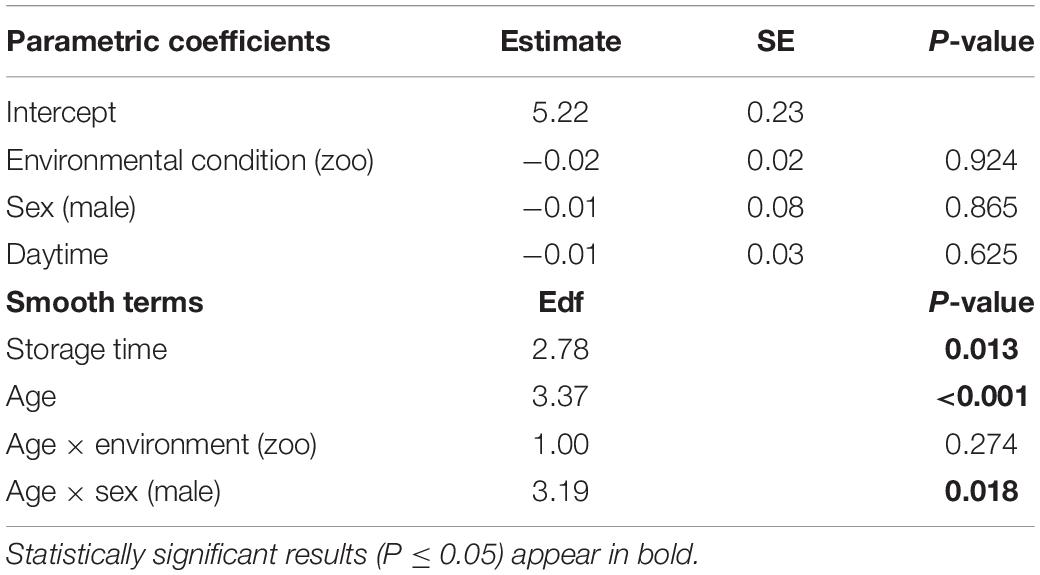
Table 1. Estimates of parametric coefficients and effective degrees of freedom of smooth terms for urinary neopterin levels in bonobos between birth and 18 years of age (generalized additive model, R2 = 0.22, deviance explained = 24.4%).
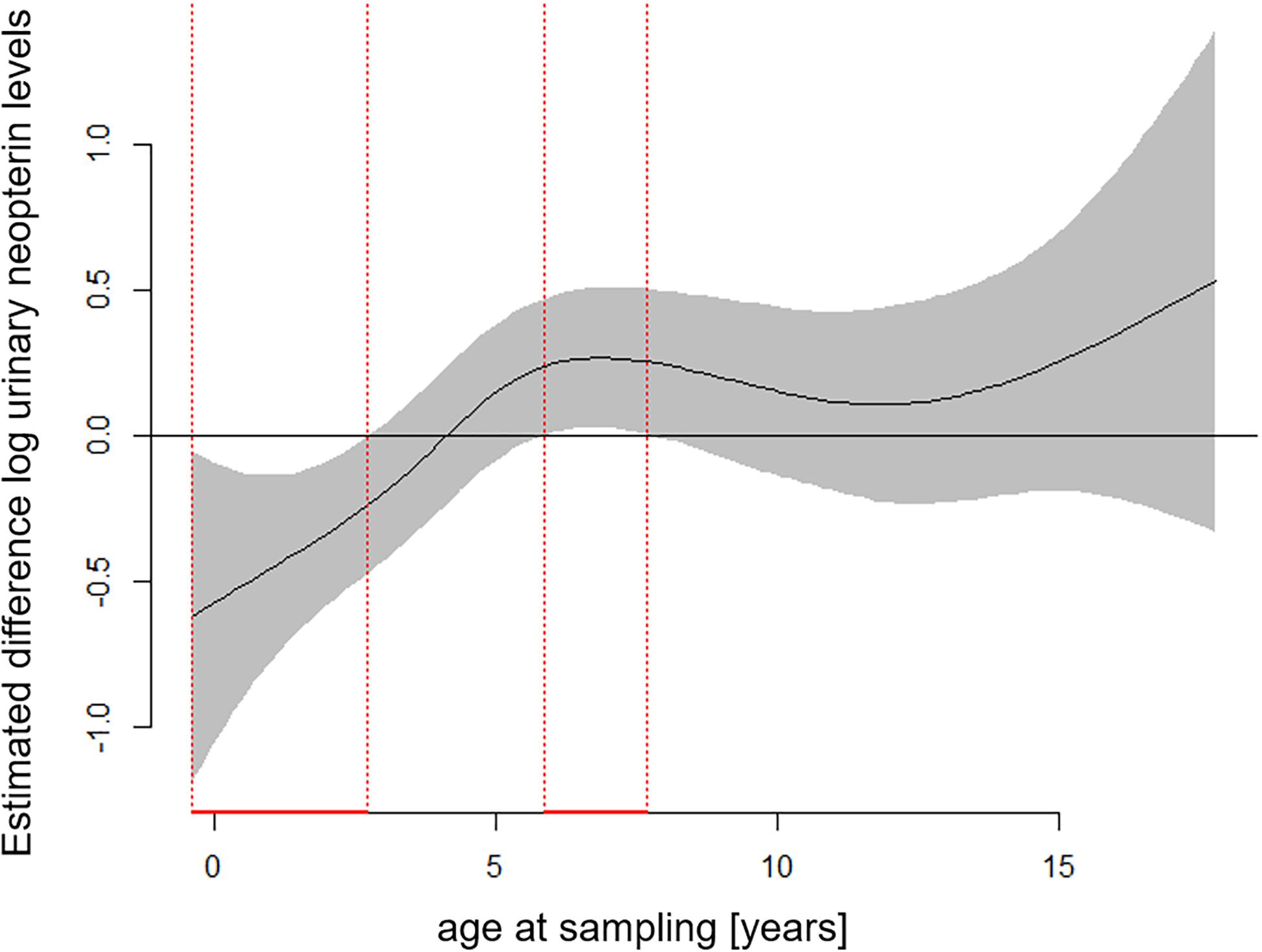
Figure 3. Estimated differences in age-related urinary neopterin levels for male and female bonobos. Negative values represent higher neopterin levels in males and positive values represent higher neopterin levels in females. Age periods with significantly different neopterin levels between the sexes are indicated in red: Males have significantly higher neopterin levels between 0.4 and 2.7 years of age, while females have significantly higher levels between 5.9 and 7.7 years of age. Total N = 806 urine samples.
Discussion
In this study we used urinary neopterin as a biomarker to investigate the effects of age, environment, and sex on cell-mediated immune ontogeny across the first 18 years of life in the bonobo, a long-lived primate species with a slow life-history. As predicted, urinary neopterin values declined progressively from birth until the age of approximately five years, after which they remained mostly stable at low levels. In contrast to our expectations, urinary neopterin levels were not influenced by environmental condition nor were changes with age environment-specific. However, sex influenced the pattern of age-related neopterin levels, with males having higher neopterin levels than females in the first three years of life and lower levels between six and eight years of age.
Neopterin Levels Decline With Age
In wild and zoo-housed bonobos, urinary neopterin levels declined after birth and stabilized between four and five years of age. These age-related changes in urinary neopterin levels of immature bonobos are in line with data from humans (Fuchs et al., 1992; Winkler et al., 2003a; Girgin et al., 2012). The decline in neopterin levels may represent the shift from predominantly cell-mediated toward more humoral immune responses during immune ontogeny. This could be verified by assessing humoral immunity markers, but this is challenging in non-invasive samples like urine.
Neopterin is produced by activated macrophages and monocytes, when these immune cells are stimulated by the cytokine interferon gamma (Fuchs et al., 1993; Murr et al., 2002) and other stimuli such as lipopolysaccharide (Troppmair et al., 1988; Sghiri et al., 2005; Mosser and Edwards, 2008). Importantly, interferon gamma is produced by adaptive immune cells (specifically T helper cells type 1) in response to intracellular infections (Murr et al., 2002) and by innate immune cells such as natural killer cells (Yu et al., 2006). Therefore, neopterin secretion represents both innate and adaptive immune responses. Both types of immune responses will be challenged frequently during early development when the organism is confronted with pathogens for the first time (Winkler et al., 2003a, b). Consequently, changes in neopterin levels during ontogeny reflect the activation of cell-mediated immunity but cannot be used to distinguish between the activation of innate and adaptive immune responses. The higher urinary neopterin levels in the first five years of life in bonobos therefore indicate that infant bonobos rely predominantly on cell-mediated immunity during infancy and that humoral immune responses become more important with time. A more differentiated view of immune ontogeny requires additional markers of specific immune responses, in particular, humoral immune responses, but many of these markers can so far only be measured in blood samples. Our results on bonobo immune ontogeny are not only interesting in the context of ecoimmunology, but they also have practical implications for conservation efforts of this endangered species, given that the transmission of diseases, particularly respiratory diseases, threatens wild and zoo-housed ape populations (e.g., King et al., 2005; Leendertz et al., 2006; Scully et al., 2018). As immature apes are important vectors of disease transmission within social groups (Kuehl et al., 2008), identification of sensitive windows during immune ontogeny can support management and conservation efforts.
Neopterin Levels Are Independent of Environmental Context
Contrary to our predictions, cell-mediated immune ontogeny in bonobos was not affected by environmental conditions. Urinary neopterin levels in wild and zoo-housed bonobos showed comparable changes with age, suggesting that these aspects of immune ontogeny during early life are stereotypic and more determined by genetic factors than modulated by environmental factors. This result corresponds with findings in humans. Only small differences in immune functioning (blood cultures and cytokine assays) were found between children growing up in urban vs. rural environments in the Tropics (Teran et al., 2011), which are also expected to differ in pathogen exposure. In the first three months of life, early immune ontogeny was found to follow a stereotypical pattern, and within this time, pre-term and term children converged on a shared trajectory (Olin et al., 2018). Other environmental factors, such as early-life adversity and/or early-life stress, are known to affect immune ontogeny and have long-term consequences on immune responses (Garner et al., 2015; Danese and Baldwin, 2017; Brenhouse et al., 2018). For example, humans that were subject to early-life adversity during childhood show greater cell-mediated inflammatory reactions (Altemus et al., 2003), and a higher pro-inflammatory state in adulthood (Baumeister et al., 2016). Considering variation in the social environment will contribute to interpret individual differences in immune ontogeny in apes.
The finding that urinary neopterin levels in wild and zoo-housed bonobos are similar was unexpected because neopterin levels of adult wild chimpanzees were found to be significantly higher than those of zoo-housed individuals, and this finding was assumed to reflect environmental differences in pathogen exposure (Behringer et al., 2019). Interestingly, urinary neopterin levels of immature bonobos (both zoo-housed and wild around 360 ng/mL corr. SG) in our study were on average nearly half of those of immature wild chimpanzees [average 759 (ng/mL corr. SG)] (Löhrich et al., 2018). This difference could indicate species-specific differences in immune functioning, different past and current environmental conditions, or a combination of both. While some wild chimpanzee populations live in fragmented habitats and in close vicinity to human settlements (e.g., Hockings et al., 2009; McCarthy et al., 2017; Garriga et al., 2019), bonobos inhabit large forests tracts where human population densities are very low and human encroachment is of modest intensity (IUCN and ICCN, 2012). Accordingly, direct contact between bonobos and humans and hence exposure to human-transmitted pathogens may be much lower than for most populations of chimpanzees and gorillas (Devaux et al., 2019). High neopterin levels in wild chimpanzees compared to bonobos might therefore reflect human-associated or other habitat-specific conditions in virus prevalence. Additionally, low neopterin levels in bonobos compared to chimpanzees could also indicate past selection for elevated immune resistance toward specific pathogens in bonobos. African apes differ in their susceptibility to Plasmodium parasite species which cause malaria. In humans, malaria infections increase neopterin levels when cell-mediated immune responses are mounted against these intracellular parasites (Brown et al., 1990, 1991; Fuchs et al., 1992; Biemba et al., 2000; te Witt et al., 2010). Wild chimpanzees also suffer from malaria infections, caused by a diversity of Plasmodium species (De Nys et al., 2014; Otto et al., 2014; Herbert et al., 2015) and likely have the same neopterin response to this infection as humans.
Although, bonobos live in an area of high malaria prevalence, malaria infections are almost absent in bonobos (Krief et al., 2010; Liu et al., 2017), presumably due to past selection for particular malaria protecting MHC allotypes (Sanchez-Mazas et al., 2017; de Groot et al., 2018). The low neopterin levels of bonobos compared to chimpanzees reported in this study might therefore be a result of malaria resistance and/or reflect more profound differences in immune functioning between the species. Other viruses known to increase neopterin levels also differ in prevalence between humans, chimpanzees and bonobos. Human immunodeficiency virus infections markedly increase neopterin levels in humans (Fuchs et al., 1992), and a similar immune response is reported for macaques infected with the related simian immunodeficiency virus (SIV) (Higham et al., 2015). SIV infections are common and widespread in wild chimpanzees (Gao et al., 1999; Li et al., 2012), but no bonobo sample from the wild or sanctuary has tested positive for SIV (Li et al., 2012; Medkour et al., 2021). Therefore, low neopterin levels in wild bonobos could also reflect differences in SIV prevalence between these species.
Based on our findings, we hypothesize that the low levels of urinary neopterin in immature wild bonobos could be explained by a lower prevalence of intracellular pathogens in their environment. If the bonobo immune system is adapted to familiar pathogens prevalent in their environment (i.e., malaria), then it is possible that infections with these pathogens do not elicit a strong immune response (old friends hypothesis, see Rook et al., 2003), and that certain aspects of their immune system function differently because of these past selection events (Sommer, 2005; de Groot et al., 2017).
However, the low urinary neopterin levels reported in our study could also reflect prevalence, frequency, and type of pathogens specific to our study population that are not representative of bonobos as a species, or are specific to younger individuals. Additional studies are therefore needed to determine if this species difference in neopterin levels persists in adult bonobos, and if acute neopterin responses to specific pathogen infections differ between wild bonobos and chimpanzees. Examining broad differences in pathogen susceptibility and prevalence between the two species and how they shape species-specific age-related immune patterns will require integrating neopterin measurements with local disease ecology of wild bonobos and chimpanzees living in diverse habitats.
The bonobo-chimpanzee-human comparison can serve as a model to investigate consequences of past selection pressures on present immune systems, highlighting extant species-specific differences in immune ontogeny and functioning. Immune system differences between the species might reflect consequences of past environmental changes and isolation of certain populations. For example, the selection for malaria resistance in bonobos might have resulted in a greater susceptibility to viruses and/or other pathogens, therefore limiting species distribution to specific habitats (Inogwabini and Leader-Williams, 2012). These differences may have had far reaching consequences for the adaptive potential to new environments during the evolution of these species. Today, these immunological differences might also impact current risks of extinction because of changing disease landscapes with climate change and globalization.
Changes in Neopterin Levels During Ontogeny Are Sex-Specific
We found support for our prediction that differences in the age of onset of reproductive maturation should influence sex-specific patterns of cell-mediated immune ontogeny. Compared to females, males had higher neopterin levels during the first three years of life, and lower levels between the ages 6–8 years. There are various, not mutually exclusive, explanations for the sex differences in neopterin levels, including effects of steroid sex hormones, sex-biased maternal investment, genetic disposition, and differences in maturation pace. Testing them remains an interesting but challenging topic for future work. Sex-biased maternal investment in offspring until weaning age is a widespread phenomenon in animals e.g., apes (Boesch, 1997) monkeys (Hinde, 2009), voles (Koskela et al., 2004), and ungulates (Hewison and Gaillard, 1999). Apart from maternal effects caused by caregiving behavior (Bercovitch, 2002; Koskela et al., 2004), maternal milk composition may vary in relation to offspring sex (Hinde, 2009; Galante et al., 2018). Of particular relevance to immune ontogeny is the finding that human mothers provide less secretory immunoglobulin to sons than to daughters with their milk (Fujita et al., 2019). Intriguingly, the sex-specific differences in neopterin levels found in our study diminish around weaning age in apes (de Lathouwers and Van Elsacker, 2006; Fahy et al., 2014; Bãdescu et al., 2016). Thus, it is tempting to speculate that the early life sex differences in bonobo neopterin production reflect lower activation of cell-mediated immunity in breastfeeding female offspring caused by the higher availability of immunoglobulins through breastmilk.
Between six and eight years of age female bonobos showed significantly higher neopterin levels than males. This timing corresponds to the onset of sexual maturation in female bonobos, which occurs on average three years earlier than in male bonobos. In humans, the onset of sexual maturation affects immune responses and the corresponding increases in sex hormone levels seem to be potent immune system modulators (Fischer et al., 2015; Klein and Flanagan, 2016). The onset of maturation in female bonobos, indicated by rising testosterone levels, happens around 5–6 years of age (Behringer et al., 2014). Rising estrogen levels during puberty are suspected to increase inflammatory immune responses in girls (Flanagan et al., 2017) and this could possibly be associated with elevated neopterin levels at this age. The same mechanism could be responsible for the elevated neopterin levels in maturing bonobo females. Another possible explanation is that the change of neopterin in adolescent females is stimulated by exposure to novel pathogen environments. At the age of 5–6 years, females start to emigrate from their natal group by visiting neighboring groups until they become an established resident in one of them (Lee et al., 2020; Sakamaki et al., 2015). In the process, they are probably in contact with a large number of unfamiliar individuals and this may expose them to new pathogens (Ryu et al., 2020). But as the effect was also seen in zoo-housed female bonobos, increased pathogen exposure may not be the proximate cause for increased neopterin levels at this age. To conclude, our findings indicate that hormonal changes with the onset of sexual maturation potentially influence the timing and magnitude of shifts in cell-mediated immune system responses during development in bonobos.
Conclusion
We investigated the effects of environment and sex on cell-mediated immune ontogeny from birth to adulthood in a long-lived primate using urinary neopterin. Our results suggest that cell-mediated immune ontogeny follows a stereotypical pattern in the first years of life that is unaffected by environmental context but differs between the sexes. The findings contrast hypotheses predicting that differences in pathogen exposure and energy availability during ontogeny affect immune ontogeny and cause differences in adult immune functioning. Our results also indicate that sex can be an important, yet often overlooked factor shaping patterns of immune ontogeny. We propose that sex biases in maternal investment and changes in androgenic and estrogenic hormone levels associated with the onset of sexual maturation are drivers for sex-specific differences in cell-mediated immune ontogeny. In comparison to wild immature chimpanzees, wild immature bonobos had low neopterin levels. These macroimmunological differences between the species can be caused by adaptive genetic differences in immunity, environmental pathogen exposure, and interactions between these factors. In conjunction these findings highlight that a species’ ecology and evolutionary history should be considered when interpreting species differences in immune functioning.
Our findings are relevant for ecoimmunology and macroimmunology because current hypotheses emphasize environmental factors during ontogeny in shaping adult immune functioning. The results of our study indicate that genetic and sex-specific processes are also important and future studies should take them into account. This can be achieved by integrating hormone measurements, behavioral observations, and assessment of specific pathogen exposure with genetic and immunological data.
By comparing bonobos, chimpanzees, and humans, we can investigate the extent of species-specific differences in immune ontogeny and functioning among closely related hominids. Underlying differences may have resulted from past selection pressures on immune systems and may have had far reaching consequences for the adaptive potential during the evolution of these species. These evolutionary signals might also make certain species more vulnerable to extinction as climate change, globalization, and increasing human contact are currently rapidly transforming disease landscapes.
We are aware that our study has looked at only one aspect of immunity with a functional biomarker that indicates activity of only a part of the cell-mediated immune response. Therefore, our conclusions are limited to this aspect of immune ontogeny, and do not necessarily translate directly to other aspects of immunity. In the future, studies should try to measure a complementary set of biomarkers that provide specific information about certain aspects of immune functioning. This means that current methods have to be adapted and extended for their use in non-invasive samples.
Investigating the causes and consequences of variation in immunity throughout life is critical for our understanding of life-history, sexual selection and population dynamics. Insights into immune ontogeny are a crucial step in this direction, but they need to be investigated in the context of a species’ ecology and evolutionary history. The frameworks of ecoimmunology and macroimmunology offer crucial guidance for these endeavors, and clinical research about the developmental origins of health and disease can benefit by integrating these different viewpoints.
Data Availability Statement
The datasets presented in this study can be found in online repositories. The names of the repository/repositories and accession number(s) can be found below: Online repository: GRO.publications: https://doi.org/10.25625/ALKB7H.
Ethics Statement
Ethical review and approval was not required for the animal study because sample collection was non-invasive. The protocol for urine sample collection in zoo-housed individuals was approved by the authorities of each zoo and supported by the coordinators of the bonobo studbook. Permission to conduct the research at the LuiKotale field site was granted by the Institut Congolais pour la Conservation de la Nature (ICCN).
Author Contributions
VB, CD, GH, and BF: conception and design. JS, MK, SL, GH, and BF: sample acquisition. VB and MH: sample analysis. VB: statistical analysis. VB, CD, GH, BF, and MH: drafting of the manuscript. All authors were involved in interpretation of the data, revised, reviewed, and approved the final version of the manuscript.
Funding
Long-term data collection at LuiKotale and the zoos was funded by the Max-Planck-Society and the Royal Zoological Society of Antwerp. Additional support ensuring the collection of long-term data came from Bonobo Alive, The Federal Ministry of Education and Research (Germany), the Leakey Foundation, the Wenner-Gren Foundation, and The George Washington University. Funding for laboratory analyses was provided by the German Primate Center. Further, the study was financially supported by the Deutsche Forschungsgesellschaft (BE 5511/4-1).
Conflict of Interest
The authors declare that the research was conducted in the absence of any commercial or financial relationships that could be construed as a potential conflict of interest.
The reviewer NT declared a past co-authorship with one of the authors MH to the handling editor.
Acknowledgments
We thank the Institut Congolais pour la Conservation de la Nature (ICCN), and the people of Lompole for granting permission to conduct fieldwork on bonobos in their forest in the buffer zone of Salonga National Park. We are extremely grateful to all assistants of the LuiKotale Bonobo Project. The project would not have been possible without the help of the care takers, curators, veterinarians and directors of the zoos which were hosting the research project. We would like to express our sincere thanks to the staff of Columbus Zoo and Aquarium, La Vallée des Singes, Milwaukee County Zoo, Planckendael Wild Animal Park, Wilhelma Stuttgart, Zoo Berlin, Zoo Cologne, Zoo Frankfurt, and Zoo Leipzig. We are grateful for statistical advice to Andreas Berghänel. We are grateful to the editor and the two reviewers for their helpful comments. We are also grateful to Puma Murmelmeister, Schlimme Mimi, and Pueppi von Connewitz for their unfailing moral support.
References
Altemus, M., Cloitre, M., and Dhabhar, F. S. (2003). Enhanced cellular immune response in women with ptsd related to childhood abuse. Am. J. Psychiatry 160, 1705–1707. doi: 10.1176/appi.ajp.160.9.1705
Bãdescu, I., Katzenberg, M. A., Watts, D. P., and Sellen, D. W. (2016). A novel fecal stable isotope approach to determine the timing of age-related feeding transitions in wild infant chimpanzees. Am. Phys. Anthropol. 162, 285–299. doi: 10.1002/ajpa.23116
Bateson, P., Barker, D., Clutton-Brock, T., Deb, D., D’Udine, B., Foley, R. A., et al. (2004). Developmental plasticity and human health. Nature 430, 419–421. doi: 10.1038/nature02725
Baumeister, D., Akhtar, R., Ciufolini, S., Pariante, C. M., and Mondelli, V. (2016). Childhood trauma and adulthood inflammation: a meta-analysis of peripheral C-reactive protein, interleukin-6 and tumour necrosis factor-α. Mol. Psychiatry 21, 642–649. doi: 10.1038/mp.2015.67
Becker, D. J., Albery, G. F., Kessler, M. K., Lunn, T. J., Falvo, C. A., and Czirják, G. Á, et al. (2020). Macroimmunology: the drivers and consequences of spatial patterns in wildlife immune defence. J. Anim. Ecol. 89, 972–995. doi: 10.1111/1365-2656.13166
Behringer, V., Deschner, T., Deimel, C., Stevens, J. M. G., and Hohmann, G. (2014). Age-related changes in urinary testosterone levels suggest differences in puberty onset and divergent life history strategies in bonobos and chimpanzees. Horm. Behav. 66, 525–533. doi: 10.1016/j.yhbeh.2014.07.011
Behringer, V., Stevens, J. M. G., Deschner, T., and Hohmann, G. (2020). “Growing up: comparing ontogeny of bonobos and chimpanzees,” in Chimpanzees in Context: A Comparative Perspective on Chimpanzee Behavior, Cognition, Conservation, and Welfare, eds L. M. Hopper and S. R. Ross (Chicago, IL: University of Chicago Press).
Behringer, V., Stevens, J. M. G., Leendertz, F. H., Hohmann, G., and Deschner, T. (2017). Validation of a method for the assessment of urinary neopterin levels to monitor health status in non-human-primate species. Front. Physiol. 8:51. doi: 10.3389/fphys.2017.00051
Behringer, V., Stevens, J. M. G., Wittig, R. M., Crockford, C., Zuberbühler, K., Leendertz, F. H., et al. (2019). Elevated neopterin levels in wild, healthy chimpanzees indicate constant investment in unspecific immune system. BMC Zool. 4:2. doi: 10.1186/s40850-019-0041-1
Beirne, C., Waring, L., McDonald, R. A., Delahay, R., and Young, A. (2016). Age-related declines in immune response in a wild mammal are unrelated to immune cell telomere length. Proc. R. Soc. B Biol. Sci. 283:20152949. doi: 10.1098/rspb.2015.2949
Bercovitch, F. B. (2002). Sex-biased parental investment in primates. Intern. J. Primatol. 23, 905–921. doi: 10.1023/A:1015585117114
Biemba, G., Gordeuk, V. R., Thuma, P., and Weiss, G. (2000). Markers of inflammation in children with severe malarial anaemia. Trop. Med. Intern. Health 5, 256–262. doi: 10.1046/j.1365-3156.2000.00545.x
Boesch, C. (1997). Evidence for dominant wild female chimpanzees investing more in sons. Anim. Behav. 54, 811–815. doi: 10.1006/anbe.1996.0510
Brenhouse, H. C., Danese, A., and Grassi-Oliveira, R. (2018). ““Neuroimmune impacts of early-life stress on development and psychopathology,” in Neuroendocrine Regulation of Behavior Current Topics in Behavioral Neurosciences, eds L. M. Coolen and D. R. Grattan (Cham: Springer International Publishing), 423–447. doi: 10.1007/7854_2018_53
Brock, P. M., Murdock, C. C., and Martin, L. B. (2014). The history of ecoimmunology and its integration with disease ecology. Integr. Compar. Biol. 54, 353–362. doi: 10.1093/icb/icu046
Brown, A. E., Teja-Isavadharm, P., and Webster, H. K. (1991). Macrophage activation in vivax malaria: fever is associated with increased levels of neopterin and interferon-gamma. Parasite Immunol. 13, 673–679. doi: 10.1111/j.1365-3024.1991.tb00562.x
Brown, A. E., Webster, H. K., Teja-Isavadharm, P., and Keeratithakul, D. (1990). Macrophage activation in falciparum malaria as measured by neopterin and interferon-gamma. Clin. Exper. Immunol. 82, 97–101. doi: 10.1111/j.1365-2249.1990.tb05410.x
Calvignac-Spencer, S., Leendertz, S. A. J., Gillespie, T. R., and Leendertz, F. H. (2012). Wild great apes as sentinels and sources of infectious disease. Clin. Microbiol. Infect. 18, 521–527. doi: 10.1111/j.1469-0691.2012.03816.x
Courtenay, J., and Santow, G. (1989). Mortality of wild and captive chimpanzees. Folia Primatol. 52, 167–177. doi: 10.1159/000156395
Danese, A., and Baldwin, J. R. (2017). Hidden wounds? inflammatory links between childhood trauma and psychopathology. Annu. Rev. Psychol. 68, 517–544. doi: 10.1146/annurev-psych-010416-044208
de Groot, N. G., Heijmans, C. M. C., Helsen, P., Otting, N., Pereboom, Z., Stevens, J. M. G., et al. (2017). Limited MHC class I intron 2 repertoire variation in bonobos. Immunogenetics 69, 677–688. doi: 10.1007/s00251-017-1010-x
de Groot, N. G., Otting, N., Doxiadis, G. G. M., Balla-Jhagjhoorsingh, S. S., Heeney, J. L., van Rood, J. J., et al. (2002). Evidence for an ancient selective sweep in the MHC class I gene repertoire of chimpanzees. Proc. Natl. Acad. Sci. U.S.A. 99, 11748–11753. doi: 10.1073/pnas.182420799
de Groot, N. G., Stevens, J. M. G., and Bontrop, R. E. (2018). Does the MHC confer protection against malaria in bonobos? Trends Immunol. 39, 768–771. doi: 10.1016/j.it.2018.07.004
de Lathouwers, M., and Van Elsacker, L. (2006). Comparing infant and juvenile behavior in bonobos (Pan paniscus) and chimpanzees (Pan troglodytes): a preliminary study. Primates 47, 287–293. doi: 10.1007/s10329-006-0179-7
De Nys, H. M., Calvignac-Spencer, S., Boesch, C., Dorny, P., Wittig, R. M., Mundry, R., et al. (2014). Malaria parasite detection increases during pregnancy in wild chimpanzees. Mal. J. 13:143. doi: 10.1186/1475-2875-13-413
Devaux, C. A., Mediannikov, O., Medkour, H., and Raoult, D. (2019). Infectious disease risk across the growing human-non human primate interface: a review of the evidence. Front. Public Health 7:305. doi: 10.3389/fpubh.2019.00305
Dowling, D. J., and Levy, O. (2014). Ontogeny of early life immunity. Trends Immunol. 35, 299–310. doi: 10.1016/j.it.2014.04.007
Dunay, E., Apakupakul, K., Leard, S., Palmer, J. L., and Deem, S. L. (2018). Pathogen transmission from humans to great apes is a growing threat to primate conservation. Ecohealth 15, 148–162. doi: 10.1007/s10393-017-1306-1
Fahy, G. E., Richards, M. P., Fuller, B. T., Deschner, T., Hublin, J.-J., and Boesch, C. (2014). Stable nitrogen isotope analysis of dentine serial sections elucidate sex differences in weaning patterns of wild chimpanzees (Pan troglodytes): weaning in Chimpanzees. Am. J. Phys. Anthropol. 153, 635–642. doi: 10.1002/ajpa.22464
Fathi, A., Addo, M. M., and Dahlke, C. (2021). Sex differences in immunity: implications for the development of novel vaccines against emerging pathogens. Front. Immunol. 11:601170. doi: 10.3389/fimmu.2020.601170
Fischer, J., Jung, N., Robinson, N., and Lehmann, C. (2015). Sex differences in immune responses to infectious diseases. Infection 43, 399–403. doi: 10.1007/s15010-015-0791-9
Fish, E. N. (2008). The X-files in immunity: sex-based differences predispose immune responses. Nat. Rev. Immunol. 8, 737–744. doi: 10.1038/nri2394
Flanagan, K. L., Fink, A. L., Plebanski, M., and Klein, S. L. (2017). Sex and gender differences in the outcomes of vaccination over the life course. Annu. Rev. Cell Dev. Biol. 33, 577–599. doi: 10.1146/annurev-cellbio-100616-060718
Forbes, K. M. (2020). Ecoimmunology at spatial scales. J. Anim. Ecol. 89, 2210–2213. doi: 10.1111/1365-2656.13296
Fuchs, D., Weiss, G., Reibnegger, G., and Wachter, H. (1992). The role of neopterin as a monitor of cellular immune activation in transplantation, inflammatory, infectious, and malignant diseases. Crit. Rev. Clin. Lab. Sci. 29, 307–344. doi: 10.3109/10408369209114604
Fuchs, D., Weiss, G., and Wachter, H. (1993). Neopterin, biochemistry and clinical use as a marker for cellular immune reactions. Intern. Archiv. Aller. Immunol. 101, 1–6. doi: 10.1159/000236491
Fujita, M., Wander, K., Paredes Ruvalcaba, N., and Brindle, E. (2019). Human milk sIgA antibody in relation to maternal nutrition and infant vulnerability in northern Kenya. Evol. Med. Public Health 2019, 201–211. doi: 10.1093/emph/eoz030
Galante, L., Milan, A., Reynolds, C., Cameron-Smith, D., Vickers, M., and Pundir, S. (2018). Sex-specific human milk composition: the role of infant sex in determining early life nutrition. Nutrients 10:1194. doi: 10.3390/nu10091194
Gao, F., Bailes, E., Robertson, D. L., Chen, Y., Rodenburg, C. M., Michael, S. F., et al. (1999). Origin of HIV-1 in the chimpanzee Pan troglodytes troglodytes. Nature 397, 436–441. doi: 10.1038/17130
Garner, A. S., Forkey, H., and Szilagyi, M. (2015). Translating developmental science to address childhood adversity. Acad. Pediatr. 15, 493–502. doi: 10.1016/j.acap.2015.05.010
Garriga, R. M., Marco, I., Casas-Díaz, E., Acevedo, P., Amarasekaran, B., Cuadrado, L., et al. (2019). Factors influencing wild chimpanzee (Pan troglodytes verus) relative abundance in an agriculture-swamp matrix outside protected areas. PLoS One 14:e0215545. doi: 10.1371/journal.pone.0215545
Georgountzou, A., and Papadopoulos, N. G. (2017). Postnatal innate immune development: from birth to adulthood. Front. Immunol. 8:957. doi: 10.3389/fimmu.2017.00957
Girgin, G., Baydar, T., Fuchs, D., Sahin, G., Özmert, E., and Yurdakök, K. (2012). Evaluation of serum and urinary levels of some pteridine pathway components in healthy turkish children. Pteridines 23, 90–95. doi: 10.1515/pteridines.2012.23.1.90
Glynn, J. R., and Moss, P. A. H. (2020). Systematic analysis of infectious disease outcomes by age shows lowest severity in school-age children. Sci. Data 7:329. doi: 10.1038/s41597-020-00668-y
Goenka, A., and Kollmann, T. R. (2015). Development of immunity in early life. J. Infect. 71, S112–S120. doi: 10.1016/j.jinf.2015.04.027
Hawash, M. B. F., Sanz-Remón, J., Grenier, J.-C., Kohn, J., Yotova, V., Johnson, Z., et al. (2021). Primate innate immune responses to bacterial and viral pathogens reveals an evolutionary trade-off between strength and specificity. Proc. Natl. Acad. Sci. U.S.A. 118:e2015855118. doi: 10.1073/pnas.2015855118
Herbert, A., Boundenga, L., Meyer, A., Moukodoum, D. N., Okouga, A. P., Arnathau, C., et al. (2015). Malaria-like symptoms associated with a natural Plasmodium reichenowi infection in a chimpanzee. Mal. J. 14:220. doi: 10.1186/s12936-015-0743-y
Hewison, A. J. M., and Gaillard, J.-M. (1999). Successful sons or advantaged daughters? The Trivers-Willard model and sex-biased maternal investment in ungulates. Trends Ecol. Evol. 14, 229–234. doi: 10.1016/S0169-5347(99)01592-X
Higham, J. P., Kraus, C., Stahl-Hennig, C., Engelhardt, A., Fuchs, D., and Heistermann, M. (2015). Evaluating noninvasive markers of nonhuman primate immune activation and inflammation. Am. J. Phys. Anthropol. 158, 673–684. doi: 10.1002/ajpa.22821
Hinde, K. (2009). Richer milk for sons but more milk for daughters: sex-biased investment during lactation varies with maternal life history in rhesus macaques. Am. J. Hum. Biol. 21, 512–519. doi: 10.1002/ajhb.20917
Hockings, K. J., Anderson, J. R., and Matsuzawa, T. (2009). Use of wild and cultivated foods by chimpanzees at Bossou, republic of guinea: feeding dynamics in a human-influenced environment. Am. J. Primatol. 71, 636–646. doi: 10.1002/ajp.20698
Hoffmann, G., Wirleitner, B., and Fuchs, D. (2003). Potential role of immune system activation-associated production of neopterin derivatives in humans. Inflamm. Res. 52, 313–321. doi: 10.1007/s00011-003-1181-9
Hohmann, G., and Fruth, B. (2003). Lui Kotal - A new site for field research on bonobos in the Salonga National Park. Pan Afr. News 10, 25–27. doi: 10.5134/143430
Inogwabini, B.-I., and Leader-Williams, N. (2012). Effects of epidemic diseases on the distribution of bonobos. PLoS One 7:e51112. doi: 10.1371/journal.pone.0051112
Kelly, C. D., Stoehr, A. M., Nunn, C., Smyth, K. N., and Prokop, Z. M. (2018). Sexual dimorphism in immunity across animals: a meta-analysis. Ecol. Lett. 21, 1885–1894. doi: 10.1111/ele.13164
King, T., Chamberlan, C., and Courage, A. (2005). Rehabilitation of orphan gorillas and bonobos in the Congo. Intern. Zoo News 52, 198–209.
Klein, S. L. (2000). The effects of hormones on sex differences in infection: from genes to behavior. Neurosci. Biobehav. Rev. 24, 627–638. doi: 10.1016/S0149-7634(00)00027-0
Klein, S. L., and Flanagan, K. L. (2016). Sex differences in immune responses. Nat. Rev. Immunol. 16, 626–638. doi: 10.1038/nri.2016.90
Klein, S. L., and Roberts, C. (eds) (2010). Sex Hormones and Immunity to Infection. Berlin: Springer, doi: 10.1007/978-3-642-02155-8
Koskela, E., Huitu, O., Koivula, M., Korpimäki, E., and Mappes, T. (2004). Sex-biased maternal investment in voles: importance of environmental conditions. Proc. R. Soc. Lond. Ser. B Biol. Sci. 271, 1385–1391. doi: 10.1098/rspb.2004.2711
Krief, S., Escalante, A. A., Pacheco, M. A., Mugisha, L., André, C., Halbwax, M., et al. (2010). On the diversity of malaria parasites in African Apes and the origin of Plasmodium falciparum from bonobos. PLoS Pathog. 6:e1000765. doi: 10.1371/journal.ppat.1000765
Kuehl, H. S., Elzner, C., Moebius, Y., Boesch, C., and Walsh, P. D. (2008). The price of play: self-organized infant mortality cycles in chimpanzees. PLoS One 3:e2440. doi: 10.1371/journal.pone.0002440
Lancaster, J. B., and Hamburg, B. A. (2008). School-Age Pregnancy and Parenthood: Bisocial Dimensions. New Brunswick, NJ: AldineTransaction.
Lee, S. M., Murray, C. M., Lonsdorf, E. V., Fruth, B., Stanton, M. A., Nichols, J., et al. (2020). Wild bonobo and chimpanzee females exhibit broadly similar patterns of behavioral maturation but some evidence for divergence. Am. J. Phys. Anthropol. 171, 100–109. doi: 10.1002/ajpa.23935
Leendertz, F. H., Pauli, G., Maetz-Rensing, K., Boardman, W., Nunn, C., Ellerbrok, H., et al. (2006). Pathogens as drivers of population declines: the importance of systematic monitoring in great apes and other threatened mammals. Biol. Conserv. 131, 325–337. doi: 10.1016/j.biocon.2006.05.002
Li, Y., Ndjango, J.-B., Learn, G. H., Ramirez, M. A., Keele, B. F., Bibollet-Ruche, F., et al. (2012). Eastern chimpanzees, but not bonobos, represent a simian immunodeficiency virus reservoir. J. Virol. 86, 10776–10791. doi: 10.1128/JVI.01498-12
Liu, W., Sherrill-Mix, S., Learn, G. H., Scully, E. J., Li, Y., Avitto, A. N., et al. (2017). Wild bonobos host geographically restricted malaria parasites including a putative new Laverania species. Nat. Commun. 8:1635. doi: 10.1038/s41467-017-01798-5
Löhrich, T., Behringer, V., Wittig, R. M., Deschner, T., and Leendertz, F. H. (2018). The use of neopterin as a noninvasive marker in monitoring diseases in wild chimpanzees. Ecohealth 15, 792–803. doi: 10.1007/s10393-018-1357-y
Love, O. P., Salvante, K. G., Dale, J., and Williams, T. D. (2008). Sex-specific variability in the immune system across life-history stages. Am. Natur. 172, E99–E112. doi: 10.1086/589521
Maibach, V., and Vigilant, L. (2019). Reduced bonobo MHC class I diversity predicts a reduced viral peptide binding ability compared to chimpanzees. BMC Evol. Biol. 19:14. doi: 10.1186/s12862-019-1352-0
Martin, L. B., Weil, Z. M., and Nelson, R. J. (2006). Refining approaches and diversifying directions in ecoimmunology. Integrat. Compar. Biol. 46, 1030–1039. doi: 10.1093/icb/icl039
McCarthy, M. S., Lester, J. D., and Stanford, C. B. (2017). Chimpanzees (Pan troglodytes) flexibly use introduced species for nesting and bark feeding in a human-dominated habitat. Int. J. Primatol. 38, 321–337. doi: 10.1007/s10764-016-9916-y
McDade, T. W. (2003). Life history theory and the immune system: steps toward a human ecological immunology. Am. J. Phys. Anthropol. 122, 100–125. doi: 10.1002/ajpa.10398
McDade, T. W. (2012). Early environments and the ecology of inflammation. Proc. Natl. Acad. Sci. U.S.A. 109, 17281–17288. doi: 10.1073/pnas.1202244109
McDade, T. W., Georgiev, A. V., and Kuzawa, C. W. (2016). Trade-offs between acquired and innate immune defenses in humans. Evol. Med. Public Health 2016, 1–16. doi: 10.1093/emph/eov033
Medkour, H., Castaneda, S., Amona, I., Fenollar, F., André, C., Belais, R., et al. (2021). Potential zoonotic pathogens hosted by endangered bonobos. Sci. Rep. 11:6331. doi: 10.1038/s41598-021-85849-4
Metcalf, C. J. E., Roth, O., and Graham, A. L. (2020). Why leveraging sex differences in immune trade-offs may illuminate the evolution of senescence. Funct. Ecol. 34, 129–140. doi: 10.1111/1365-2435.13458
Miller, R. C., Brindle, E., Holman, D. J., Shofer, J., Klein, N. A., Soules, M. R., et al. (2004). Comparison of specific gravity and creatinine for normalizing urinary reproductive hormone concentrations. Clin. Chem. 50, 924–932. doi: 10.1373/clinchem.2004.032292
Mosser, D. M., and Edwards, J. P. (2008). Exploring the full spectrum of macrophage activation. Nat. Rev. Immunol. 8, 958–969. doi: 10.1038/nri2448
Murphy, K., and Weaver, C. (2018). Janeway Immunologie. Berlin: Springer, doi: 10.1007/978-3-662-56004-4
Murr, C., Widner, B., Wirleitner, B., and Fuchs, D. (2002). Neopterin as a marker for immune system activation. Curr. Drug Metab. 3, 175–187. doi: 10.2174/1389200024605082
Olin, A., Henckel, E., Chen, Y., Lakshmikanth, T., Pou, C., Mikes, J., et al. (2018). Stereotypic immune system development in newborn children. Cell 174, 1277–1292.e14. doi: 10.1016/j.cell.2018.06.045
Otto, T. D., Rayner, J. C., Böhme, U., Pain, A., Spottiswoode, N., Sanders, M., et al. (2014). Genome sequencing of chimpanzee malaria parasites reveals possible pathways of adaptation to human hosts. Nat. Commun. 5:4754. doi: 10.1038/ncomms5754
Peters, A., Delhey, K., Nakagawa, S., Aulsebrook, A., and Verhulst, S. (2019). Immunosenescence in wild animals: meta-analysis and outlook. Ecol. Lett. 22, 1709–1722. doi: 10.1111/ele.13343
PrabhuDas, M., Adkins, B., Gans, H., King, C., Levy, O., Ramilo, O., et al. (2011). Challenges in infant immunity: implications for responses to infection and vaccines. Nat. Immunol. 12, 189–194. doi: 10.1038/ni0311-189
R Development Core Team (2008). R: A Language and Environment for Statistical Computing. Vienna: R foundation for statistical computing.
Ravindra, K., Rattan, P., Mor, S., and Aggarwal, A. N. (2019). Generalized additive models: building evidence of air pollution, climate change and human health. Environ. Intern. 132:104987. doi: 10.1016/j.envint.2019.104987
Robson, S. L., and Wood, B. (2008). Hominin life history: reconstruction and evolution. J. Anat. 212, 394–425. doi: 10.1111/j.1469-7580.2008.00867.x
Rook, G. A. W., Martinelli, R., and Brunet, L. R. (2003). Innate immune responses to mycobacteria and the downregulation of atopic responses. Curr. Opin. Aller. Clin. Immunol. 3, 337–342. doi: 10.1097/00130832-200310000-00003
Ryu, H., Hill, D. A., Sakamaki, T., Garai, C., Tokuyama, N., and Furuichi, T. (2020). Occurrence and transmission of flu-like illness among neighboring bonobo groups at Wamba. Primates 61, 775–784. doi: 10.1007/s10329-020-00832-3
Sakamaki, T., Behncke, I., Laporte, M., Mulavwa, M., Ryu, H., and Takemoto, H. (2015). “Intergroup transfer of females and social relationships between immigrants and residents in bonobo (Pan paniscus) societies,” in Dispersing Primate Females eds T. Furuichi, J. Yamagiwa, and F. Aureli (Tokyo: Springer Japan), 127–164. doi: 10.1007/978-4-431-55480-6_6
Sanchez-Mazas, A., Černý, V., Di, D., Buhler, S., Podgorná, E., Chevallier, E., et al. (2017). The HLA-B landscape of Africa: signatures of pathogen-driven selection and molecular identification of candidate alleles to malaria protection. Mol. Ecol. 26, 6238–6252. doi: 10.1111/mec.14366
Schoenle, L. A., Downs, C. J., and Martin, L. B. (2018). “An introduction to ecoimmunology,” in Advances in Comparative Immunology, ed. E. L. Cooper (Cham: Springer), 901–932. doi: 10.1007/978-3-319-76768-0_26
Scully, E. J., Basnet, S., Wrangham, R. W., Muller, M. N., Otali, E., Hyeroba, D., et al. (2018). Lethal respiratory disease associated with human Rhinovirus C in wild chimpanzees, Uganda, 2013. Emerg. Infect. Dis. 24, 267–274. doi: 10.3201/eid2402.170778
Sghiri, R., Feinberg, J., Thabet, F., Dellagi, K., Boukadida, J., Ben Abdelaziz, A., et al. (2005). Gamma interferon is dispensable for neopterin production in vivo. CVI 12, 1437–1441. doi: 10.1128/CDLI.12.12.1437-1441.2005
Simon, A. K., Hollander, G. A., and McMichael, A. (2015). Evolution of the immune system in humans from infancy to old age. Proc. R. Soc. B Biol. Sci. 282:20143085. doi: 10.1098/rspb.2014.3085
Sommer, S. (2005). The importance of immune gene variability (MHC) in evolutionary ecology and conservation. Front. Zool. 2:16. doi: 10.1186/1742-9994-2-16
te Witt, R., van Wolfswinkel, M. E., Petit, P. L., van Hellemond, J. J., Koelewijn, R., van Belkum, A., et al. (2010). Neopterin and procalcitonin are suitable biomarkers for exclusion of severe Plasmodium falciparum disease at the initial clinical assessment of travellers with imported malaria. Mal. J. 9:255. doi: 10.1186/1475-2875-9-255
Teran, R., Mitre, E., Vaca, M., Erazo, S., Oviedo, G., Hübner, M. P., et al. (2011). Immune system development during early childhood in tropical Latin America: evidence for the age-dependent down regulation of the innate immune response. Clin. Immunol. 138, 299–310. doi: 10.1016/j.clim.2010.12.011
Thakur, A., Mikkelsen, H., and Jungersen, G. (2019). Intracellular pathogens: host immunity and microbial persistence strategies. J. Immunol. Res. 2019, 1–24. doi: 10.1155/2019/1356540
Tieleman, B. I. (2018). Understanding immune function as a pace of life trait requires environmental context. Behav. Ecol. Sociobiol. 72:55. doi: 10.1007/s00265-018-2464-z
Troppmair, J., Nachbaur, K., Herold, M., Aulitzky, W., Tilg, H., Gastl, G., et al. (1988). In-vitro and in-vivo studies on the induction of neopterin biosynthesis by cytokines, alloantigens and lipopolysaccharide (LPS). Clin. Exp. Immunol. 74, 392–397.
Urlacher, S. S., Ellison, P. T., Sugiyama, L. S., Pontzer, H., Eick, G., Liebert, M. A., et al. (2018). Tradeoffs between immune function and childhood growth among Amazonian forager-horticulturalists. Proc. Natl. Acad. Sci. U.S.A. 115, E3914–E3921. doi: 10.1073/pnas.1717522115
van Rij, J., Wieling, M., Baayen, R., and van Rijn, H. (2020). itsadug: Interpreting Time Series and Autocorrelated Data Using GAMMs. R Package.
Walker, K. K., Walker, C. S., Goodall, J., and Pusey, A. E. (2018). Maturation is prolonged and variable in female chimpanzees. J. Hum. Evol. 114, 131–140. doi: 10.1016/j.jhevol.2017.10.010
West, L. J. (2002). Defining critical windows in the development of the human immune system. Hum. Exper. Toxicol. 21, 499–505. doi: 10.1191/0960327102ht288oa
Winkler, C., Frick, B., Schroecksnadel, K., Wirleitner, B., and Fuchs, D. (2003a). Follow-up of urinary neopterin concentrations in two healthy children until adolescence. Pteridines 14, 102–107. doi: 10.1515/pteridines.2003.14.3.102
Winkler, C., Wirleitner, B., Werner, E. R., and Fuchs, D. (2003b). Urinary neopterin concentrations in healthy individuals with household contact. Pteridines 14, 34–38. doi: 10.1515/pteridines.2003.14.1.34
Wood, S. N. (2004). Stable and efficient multiple smoothing parameter estimation for generalized additive models. J. Am. Statist. Assoc. 99, 673–686. doi: 10.1198/016214504000000980
Wood, S. N. (2011). Fast stable restricted maximum likelihood and marginal likelihood estimation of semiparametric generalized linear models: estimation of Semiparametric Generalized Linear Models. J. R. Statist. Soc. Ser. B 73, 3–36. doi: 10.1111/j.1467-9868.2010.00749.x
Wood, S. N. (2017). Generalized Additive Models: An Introduction with R, 2nd Edn, Boca Raton: Taylor & Francis Group.
Wroblewski, E. E., Guethlein, L. A., Norman, P. J., Li, Y., Shaw, C. M., Han, A. S., et al. (2017). Bonobos maintain immune system diversity with three functional types of MHC-B. J. Immunol. 198, 3480–3493. doi: 10.4049/jimmunol.1601955
Yang, Y., and Kozloski, M. (2011). Sex differences in age trajectories of physiological dysregulation: inflammation, metabolic syndrome, and allostatic load. J. Gerontol. Ser. A 66A, 493–500. doi: 10.1093/gerona/glr003
Yu, J., Wei, M., Becknell, B., Trotta, R., Liu, S., Boyd, Z., et al. (2006). Pro- and antiinflammatory cytokine signaling: reciprocal antagonism regulates interferon-gamma production by human natural killer cells. Immunity 24, 575–590. doi: 10.1016/j.immuni.2006.03.016
Keywords: ecoimmunology, macroimmunology, immune ontogeny, bonobo, Pan paniscus, development of immunocompetence, neopterin
Citation: Behringer V, Deimel C, Stevens JMG, Kreyer M, Lee SM, Hohmann G, Fruth B and Heistermann M (2021) Cell-Mediated Immune Ontogeny Is Affected by Sex but Not Environmental Context in a Long-Lived Primate Species. Front. Ecol. Evol. 9:629094. doi: 10.3389/fevo.2021.629094
Received: 16 December 2020; Accepted: 16 April 2021;
Published: 19 May 2021.
Edited by:
Elise Huchard, UMR 5554 Institut des Sciences de l’Evolution de Montpellier (ISEM), FranceReviewed by:
Nicole A. Thompson Gonzalez, University of New Mexico, United StatesNoah Snyder-Mackler, Arizona State University, United States
Copyright © 2021 Behringer, Deimel, Stevens, Kreyer, Lee, Hohmann, Fruth and Heistermann. This is an open-access article distributed under the terms of the Creative Commons Attribution License (CC BY). The use, distribution or reproduction in other forums is permitted, provided the original author(s) and the copyright owner(s) are credited and that the original publication in this journal is cited, in accordance with accepted academic practice. No use, distribution or reproduction is permitted which does not comply with these terms.
*Correspondence: Verena Behringer, VkJlaHJpbmdlckBkcHouZXU=
†These authors have contributed equally to this work
‡These authors share senior authorship