- 1British Antarctic Survey, Natural Environment Research Council, Cambridge, United Kingdom
- 2Plymouth Marine Laboratory, Plymouth, United Kingdom
- 3Australian Antarctic Division, Hobart, TAS, Australia
- 4Centre for Marine Socioecology, University of Tasmania, Hobart, TAS, Australia
- 5Sorbonne Université, CNRS, IRD, MHN, Laboratoire d’Océanographie et du Climat: Expérimentations et Approches Numériques (LOCEAN-IPSL), Paris, France
- 6College of Marine Science, University of South Florida, St. Petersburg, FL, United States
- 7Alfred Wegener Institute for Polar and Marine Research, Helmholtz Centre for Polar and Marine Research, Bremerhaven, Germany
- 8Institute for Marine and Antarctic Studies, University of Tasmania, Hobart, TAS, Australia
- 9Department of Earth, Ocean and Atmospheric Sciences, The University of British Columbia, Vancouver, BC, Canada
- 10Escuela de Ciencias del Mar, Pontificia Universidad Católica de Valparaíso, Valparaíso, Chile
- 11Centro de Investigación Dinámica de Ecosistemas Marinos de Altas Latitudes (IDEAL), Valdivia, Chile
- 12Hakai Institute, Heriot Bay, BC, Canada
- 13Institute for the Oceans and Fisheries, The University of British Columbia, Vancouver, BC, Canada
- 14Institute for Extra-Cutting-Edge Science and Technology Avant-Garde Research, Japan Agency for Marine-Earth Science and Technology, Yokosuka City, Japan
- 15Moss Landing Marine Laboratories, California State University, Moss Landing, CA, United States
- 16Institute for Chemistry and Biology of the Marine Environment, University of Oldenburg, Oldenburg, Germany
- 17Helmholtz Institute for Functional Marine Biodiversity (HIFMB) at the University of Oldenburg, Oldenburg, Germany
- 18National Institute of Water and Atmospheric Research Ltd., Wellington, New Zealand
- 19Antarctic Ecosystem Research Division, National Oceanic and Atmospheric Administration Fisheries, South West Fisheries Center, La Jolla, CA, United States
- 20Fishery Resource Analysis and Monitoring Division, Northwest Fisheries Science Center, National Marine Fisheries Service, National Oceanic and Atmospheric Administration, Newport, OR, United States
- 21Virginia Institute of Marine Science, William & Mary, Gloucester Point, VA, United States
- 22School of Oceanography, Shanghai Jiao Tong University, Shanghai, China
- 23Australian Antarctic Program Partnership, Institute for Marine and Antarctic Studies, University of Tasmania, Hobart, TAS, Australia
- 24Institute for Marine Antarctic Studies, University of Tasmania, Hobart, TAS, Australia
- 25Institute of Oceanology, Chinese Academy of Sciences, Qingdao, China
In the Southern Ocean, several zooplankton taxonomic groups, euphausiids, copepods, salps and pteropods, are notable because of their biomass and abundance and their roles in maintaining food webs and ecosystem structure and function, including the provision of globally important ecosystem services. These groups are consumers of microbes, primary and secondary producers, and are prey for fishes, cephalopods, seabirds, and marine mammals. In providing the link between microbes, primary production, and higher trophic levels these taxa influence energy flows, biological production and biomass, biogeochemical cycles, carbon flux and food web interactions thereby modulating the structure and functioning of ecosystems. Additionally, Antarctic krill (Euphausia superba) and various fish species are harvested by international fisheries. Global and local drivers of change are expected to affect the dynamics of key zooplankton species, which may have potentially profound and wide-ranging implications for Southern Ocean ecosystems and the services they provide. Here we assess the current understanding of the dominant metazoan zooplankton within the Southern Ocean, including Antarctic krill and other key euphausiid, copepod, salp and pteropod species. We provide a systematic overview of observed and potential future responses of these taxa to a changing Southern Ocean and the functional relationships by which drivers may impact them. To support future ecosystem assessments and conservation and management strategies, we also identify priorities for Southern Ocean zooplankton research.
Introduction
Metazoan zooplankton (hereafter zooplankton) are globally important. They are ubiquitous and abundant in the world’s oceans (Bar-On et al., 2018; Chiba et al., 2018), playing a critical role in the structure and function (Odum, 1964, 1969) of marine ecosystems, including ‘ecosystem services’ that benefit humans (Millennium Ecosystem Assessment, 2005; Simcock, 2017; Bebianno et al., 2021a,b). Zooplankton are pivotal in the cycling of carbon and nutrients, regulating climate, supporting primary and secondary production, maintaining biodiversity, food web configurations and balance among trophic levels, and sustaining fisheries production, tourism, and human communities (Richardson, 2008; Keister et al., 2012; Pinkerton et al., 2020). Their central trophic position as grazers and prey means they directly affect biomass, structure of food webs, and modulate ecosystem functioning. In some regions, zooplankton are the major grazers and can influence the amount of production that reaches mid and higher trophic level consumers, including fish, squid, marine mammals, and birds. Through the regeneration of nitrogen, zooplankton enhance phytoplankton production (Richardson, 2008). Processes associated with feeding, vertical migration, and mortality also support microbial production and facilitate the transfer of organic matter to the deep ocean: contributing to benthic communities (Ruhl and Smith, 2004; Schnack-Schiel and Isla, 2005), carbon drawdown, storage and sequestration, dimethyl sulfide production (Daly and DiTullio, 1996) and hence a negative (mitigating) feedback on climate change (Jónasdóttir et al., 2015; Steinberg et al., 2017; Barnes et al., 2018).
Like all ecological communities, zooplankton communities are shaped by a combination of intrinsic biotic interactions (e.g., competition, food web interactions) and extrinsic abiotic interactions associated with the influence of environmental stressors (or drivers) on individual species. Several attributes of zooplankton, including poikilothermy, stenothermy, small size (20 μm to 20 mm in length in the case of micro- and mesozooplankton), short generation times and lifecycles (generally <1 year), and complex life histories (often passing through a range of ontogenic stages), render them very sensitive to environmental drivers (Hays et al., 2005; Richardson, 2008). This can be expressed as alterations in, for example, behavior, physiology, phenology, productivity, abundances and shifts in species ranges and distribution, all of which modify zooplankton populations and community dynamics (i.e., changes in population or community structure, dominance, and distribution patterns) and their trophic interactions with other taxa over a range of space and time scales. Through these alterations the structure and function of whole ecosystems can be modified (Doney et al., 2012; Malhi et al., 2020). Zooplankton are therefore not only susceptible to human-induced climate change and concurrent processes (a significant component of global change), but they also propagate climate signals through ecosystems and feedback to biogeochemical cycles, carbon sequestration, and the global climate system. As such they are considered good indicators of water mass types and environmental change (Chiba et al., 2018), and are integral to interpreting the responses of marine ecosystems to climate change, and developing adaptation and mitigation strategies. In addition to observed impacts of natural environmental variability, there are many diverse examples of the impacts of human-induced climate change and other anthropogenic stressors (e.g., pollutants, fisheries) on zooplankton that are having profound and complex ecosystem-level consequences (see Richardson, 2008; Doney et al., 2012; Murphy et al., 2016; Beaugrand and Kirby, 2018).
To enhance understanding and predictions of Earth System functioning and global change under the mounting pressures of the Anthropocene (IPCC, 2018, 2019), it is imperative that we understand the role of zooplankton within the world’s oceans under present conditions, the potential impacts of future change and associated risks (Reid et al., 2003; Atkinson et al., 2012; Constable et al., 2014b; Pecuchet et al., 2020; Kohlbach et al., 2021), and adequately represent zooplankton dynamics in Earth System Models (ESMs) (Le Quéré et al., 2005, 2016; Buitenhuis et al., 2010). This requires specific knowledge of the complex interactions and mechanisms associated with zooplankton dynamics, their sensitivity to drivers of change, and the resultant effects on ecosystems and socioeconomics. Appreciation of these aspects at local, regional and oceanic scales are essential in underpinning decision-making for regulating human activities that impact ecosystems, conserving and managing ecosystems, and planning for the response to future change to promote the resilience and viability of marine ecosystems (Malhi et al., 2020).
Antarctica and the Southern Ocean are inherent to the Earth System. In addition to the perturbations that have already impacted the Southern Ocean, major changes in its habitats and ecosystems are expected over the coming years in response to increased pressures from a range of global (see Morley et al., 2020) and local environmental drivers (see Grant et al., 2021), the majority of which have an anthropogenic component (Murphy et al., 2007b; Rogers et al., 2007; Clarke et al., 2012; Constable et al., 2014b; Gutt et al., 2015; Chown and Brooks, 2019; Kennicutt et al., 2019). Owing to the extensive physical, biogeochemical, and ecological connectivity between Southern Ocean ecosystems and the global ocean, future changes in the structure and functioning of these ecosystems will also have consequences throughout the Earth System (Henley et al., 2020; Murphy et al., 2021). To predict how Southern Ocean ecosystems will respond to global change and the implications for regional and Earth System functioning and decision-making, assessments of the sensitivity of Southern Ocean zooplankton to changes in these drivers are necessary (Constable et al., 2014a,b). Moreover, changes in zooplankton population and community dynamics and their relative importance in Southern Ocean food webs over time will reflect species-specific differences in vulnerability or resilience to these drivers. Together with a mechanistic understanding of the processes involved, this knowledge will enable the development of food web and ecosystem models that are needed for assessing different scenarios of change and projecting how Southern Ocean ecosystem structure and functioning may be impacted. Aside from Antarctic krill (Euphausia superba), which are comparatively well studied, zooplankton are considered to be less well understood but critical to the development of these models (Murphy et al., 2012a,2016, 2018; McCormack et al., 2021).
Southern Ocean zooplankton comprise a diverse range of endemic and non-endemic species that collectively dominate the pelagic biomass. They vary in their body forms and sizes (e.g., from <100 μm copepod nauplii to >1 m jellyfish or salp chains), and in their life history traits and strategies, and occupy a range of habitats and environmental conditions (Atkinson et al., 2012). Many of the endemic species are uniquely adapted to Southern Ocean conditions, including the intense seasonality, sea ice, and low ocean temperatures (Murphy et al., 2016) associated with its cooling >24 mya and formation of the Antarctic Circumpolar Current (ACC) and Antarctic Polar Front (APF) (Clarke and Johnston, 2003; Lagabrielle et al., 2009; Clarke and Crame, 2010). These physical features are indeed fundamental drivers of natural processes, life history evolution, and ecological connectivity across the Southern Ocean (Clarke and Crame, 2010; Clarke et al., 2012; Murphy et al., 2012b,2016; Varpe, 2017), signifying the vulnerability of Southern Ocean biota, including zooplankton, to climate change (Constable et al., 2014b).
A number of consistent and distinct zooplankton communities have been identified in the Southern Ocean, occupying the cold coastal regions and seasonally variable sea ice zone in the south, the open ocean, and the relatively warmer subantarctic areas in the north. Zooplankton abundance is highest in the epipelagic and upper mesopelagic layers (wherein many species undertake diel vertical migrations) though some species may extend to deeper waters during seasonal migrations and intermittent forays. These biogeographic regions are generally defined by sea and pack ice, bathymetry, and the series of oceanographic frontal zones across the Southern Ocean (Hosie et al., 2000; Hunt and Hosie, 2005, 2006; Pinkerton et al., 2010; Ward et al., 2012; Hosie et al., 2014; Steinberg et al., 2015). The associated zooplankton communities are dominated by a small number of metazoan taxa (including euphausiids, copepods, salps, and pteropods) and vary in their structure (i.e., species richness and diversity) and dominance patterns. Interannual variations in community dynamics also occur within and between these regions in relation to seasonal succession and transitions through key life history stages (Atkinson and Sinclair, 2000; Froneman et al., 2000; Pakhomov et al., 2000; Hunt and Hosie, 2006; Ducklow et al., 2012; Murphy et al., 2021). Additional temporal variations in community dynamics may also occur in relation to oceanographic and cryospheric processes. Future shifts in sea ice (Turner et al., 2020), fronts (Chapman et al., 2020) and related bio-physical oceanographic processes (e.g., Pinkerton et al., 2020) in association with human-induced climate change are projected to result in changes in zooplankton distributions (Constable et al., 2014b). There is also concern over the impacts of additional climate-related processes (e.g., atmospheric and oceanographic processes, including ocean acidification) and other direct and indirect anthropogenic drivers (e.g., dynamics of predator and prey populations, the recovery of previously harvested whale species, fisheries, invasive species, and pollution, etc.) on zooplankton and community dynamics and the implications for Southern Ocean ecosystems.
The review by Atkinson et al. (2012) provided an overview of the then-current understanding of the biology of dominant Southern Ocean metazoan zooplankton, focusing primarily on the data available for parameterizing models. In the intervening decade, understanding of the distribution, ecology, and response to change of these taxa has continued to advance concomitant with a greater diversity of research approaches. These advances have been facilitated in part by improved data availability as a result of new surveys and process studies (e.g., Meyer et al., 2017; Wallis, 2018; Wallis et al., 2019; Conroy et al., 2020; Yang et al., 2020), and extensive data rescue and compilation (e.g., Mackey et al., 2012; Atkinson et al., 2017; Tarling et al., 2018; Perry et al., 2019; Pinkerton et al., 2020; Takahashi et al., 2021). At the same time advances have been made in modelling across multiple taxa (e.g., Pinkerton et al., 2020) and of key species such as Antarctic krill (e.g., Constable and Kawaguchi, 2018; Veytia et al., 2020; Sylvester et al., 2021) and Thysanoessa macrura (e.g., Driscoll et al., 2015), and Salpa thompsoni (e.g., Henschke et al., 2018). There has also been progress in the understanding of the role of zooplankton in biogeochemical cycling, including the biological pump via grazing and vertical carbon flux (e.g., Alcaraz et al., 2014; Henschke et al., 2016; Belcher et al., 2019; Cavan et al., 2019; Manno et al., 2020) and their sensitivities to ocean acidification, including the additional synergistic effects of warming, temperature and deoxygenation (e.g., Kawaguchi et al., 2013; Manno et al., 2017, 2018; Ericson et al., 2018; Peck et al., 2018; Bednaršek et al., 2019; Saba et al., 2021). Some of these advances, especially for Antarctic krill, are captured in other reviews, which have generally focused on the ecological effects of climate change (Mackey et al., 2012; Hunt et al., 2016; Chown and Brooks, 2019; Siegert et al., 2019; Rogers et al., 2020) and/or the Antarctic krill fishery (e.g., Meyer et al., 2020; McBride et al., 2021). This contribution adds to this literature with an up-to-date systematic comparison of the major Southern Ocean zooplankton taxa as detailed in the next paragraph. We stress, however, that limitations remain due to the paucity (and/or inaccessibility) of detailed qualitative and quantitative knowledge for many zooplankton species, populations and communities. In particular the underlying processes of their dynamics and causal links to drivers are often poorly understood; furthermore, projections of future changes in Southern Ocean zooplankton that can support policy decisions are limited.
The first Marine Ecosystem Assessment for the Southern Ocean (MEASO) is an international collaborative activity of the Integrating Climate and Ecosystem Dynamics in the Southern Ocean (ICED) programme. The primary aim of MEASO is to assess the status and trends of Southern Ocean habitats, species, and food webs, and the risks to these ecosystems and their services from drivers of change, particularly climate change and related processes. As a core MEASO contribution, this paper synthesizes the current state of knowledge of zooplankton, focusing on the dominant taxa that are understood to have an important role in ecosystem structure and functioning and are susceptible to global change, and for which sufficient data and knowledge are available. We provide an overview of key euphausiids, copepods, salps, and pteropods in terms of their ecology and roles, observed changes, and future prognoses across the Southern Ocean within the MEASO areas [which include protected areas and fishery reporting areas of the Commission for the Conservation of Antarctic Marine Living Resources (CCAMLR), Figure 1]. We synthesize available knowledge on their current circumpolar distributions, observed changes, and the key drivers and mechanisms involved. We also provide an analysis of environmental suitability (modelled abundance) for some of these taxa in the MEASO areas over the past two decades based on samples collected by the international Scientific Committee on Antarctic Research (SCAR) Southern Ocean Continuous Plankton Recorder Survey (SO-CPR). We undertake a qualitative assessment of potential future changes of these key taxa in response to anticipated changes in key physical, chemical, and ecological global and local drivers (Figure 2) that we consider important for these taxa. We also assess their potential resilience (sensu Oliver et al., 2015a) focusing on the mechanisms that may underpin resistance and recovery, which can either be expressed (or examined) at the individual or population level; an aspect that has not been widely explored for these taxa. We conclude by identifying current limitations and important directions for Southern Ocean zooplankton research to enhance marine ecosystem models for the region, develop robust projections of change, and improve understanding of Earth System functioning that will further support policy makers in developing conservation and management strategies.
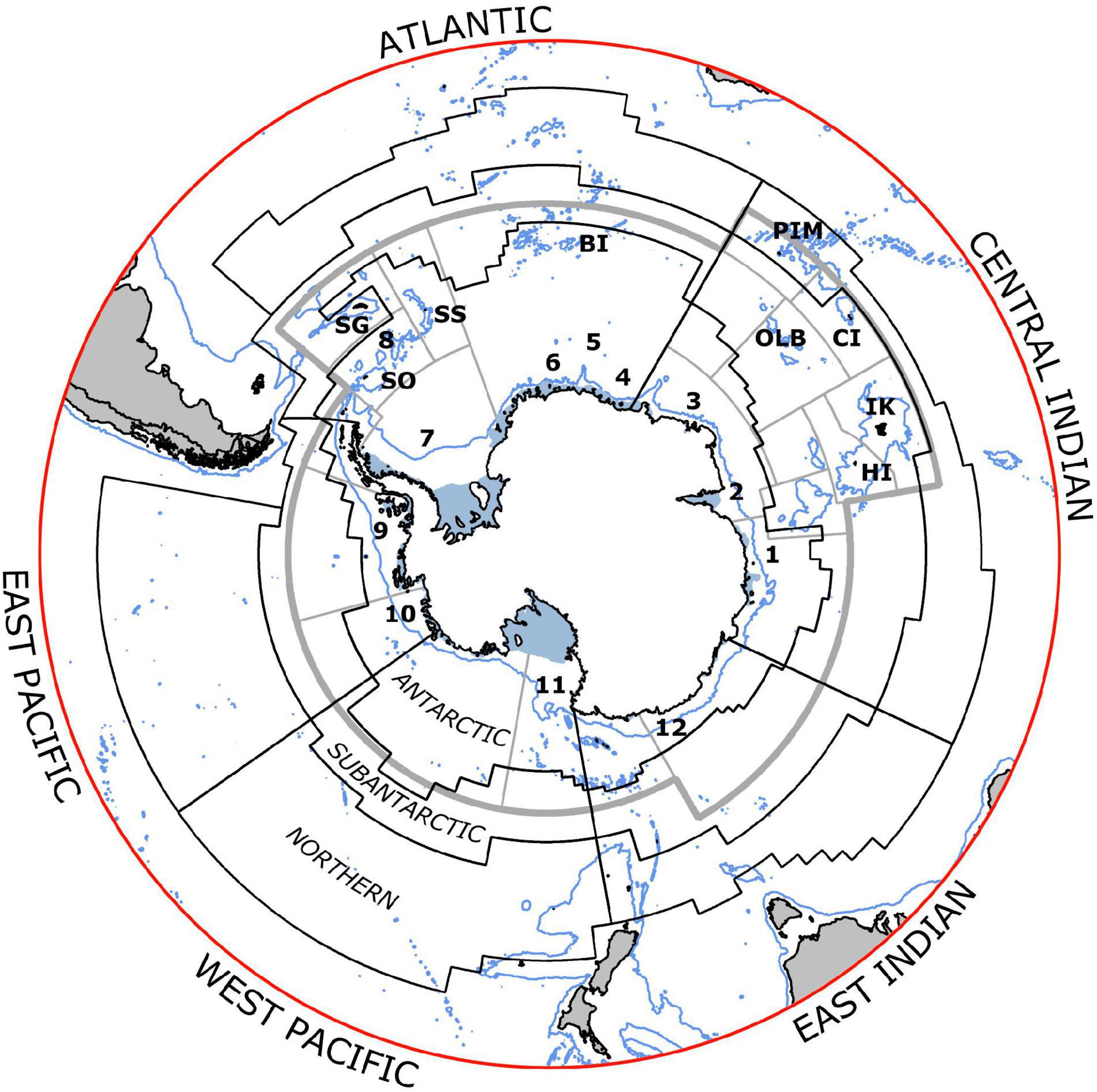
Figure 1. Areas for assessing status and trends of dominant metazoan zooplankton in the Marine Ecosystem Assessment for the Southern Ocean (black lines). Sectors are divided meridionally: corresponding names of sectors are outside the circle. Zones extend from the coast to the Southern Antarctic Circumpolar Current Front (SACCF, Antarctic), to the Subantarctic Front (SAF, Subantarctic) and to the Subtropical Front (STF, Northern). Seas are marked in the Antarctic Zone as (1) Davis, (2) Cooperation, (3) Cosmonaut, (4) Riiser-Larsen, (5) Haakon VII, (6) Lazarev, (7) Weddell, (8) Scotia, (9) Bellingshausen, (10) Amundsen, (11) Ross, and (12) Dumont D’Urville. Islands in the Commission for the Conservation of Antarctic Marine Living Resources (CCAMLR) area include Heard (HI), Isles Kerguelen (IK), Crozet (CI), Prince Edward-Marion (PEM), Bouvet (BI), South Sandwich (SS), South Georgia (SG), South Orkney (SO), Ob and Lena Banks (OLB), and South Shetlands (not initialed but found on the north-western side of Antarctic Peninsula). Gray lines indicate the CCAMLR reporting areas (Subareas and Divisions). NB: The Southern Boundary (not shown) and the STF mark the southern and northern limits of the intense eastward flowing Antarctic Circumpolar Current (ACC, not shown), which reaches up to 2000 km wide and extends from surface waters to 2000–4000 m. The Antarctic Polar Front, APF (not shown) lies between the SACCF and SAF and represents an important ecological boundary. It marks the northern extent of ice-influenced surface waters, and the transition between the cold surface waters to the south and the warmer waters to the north. Within the ACC, the SACCF, APF, and SAF delimit boundaries between different water masses with distinct and relatively homogeneous physical and chemical characteristics. These also coincide with intense narrow currents jets that dominate the ACC (see Orsi et al., 1995; Rintoul, 2018; Park et al., 2019; Chapman et al., 2020).
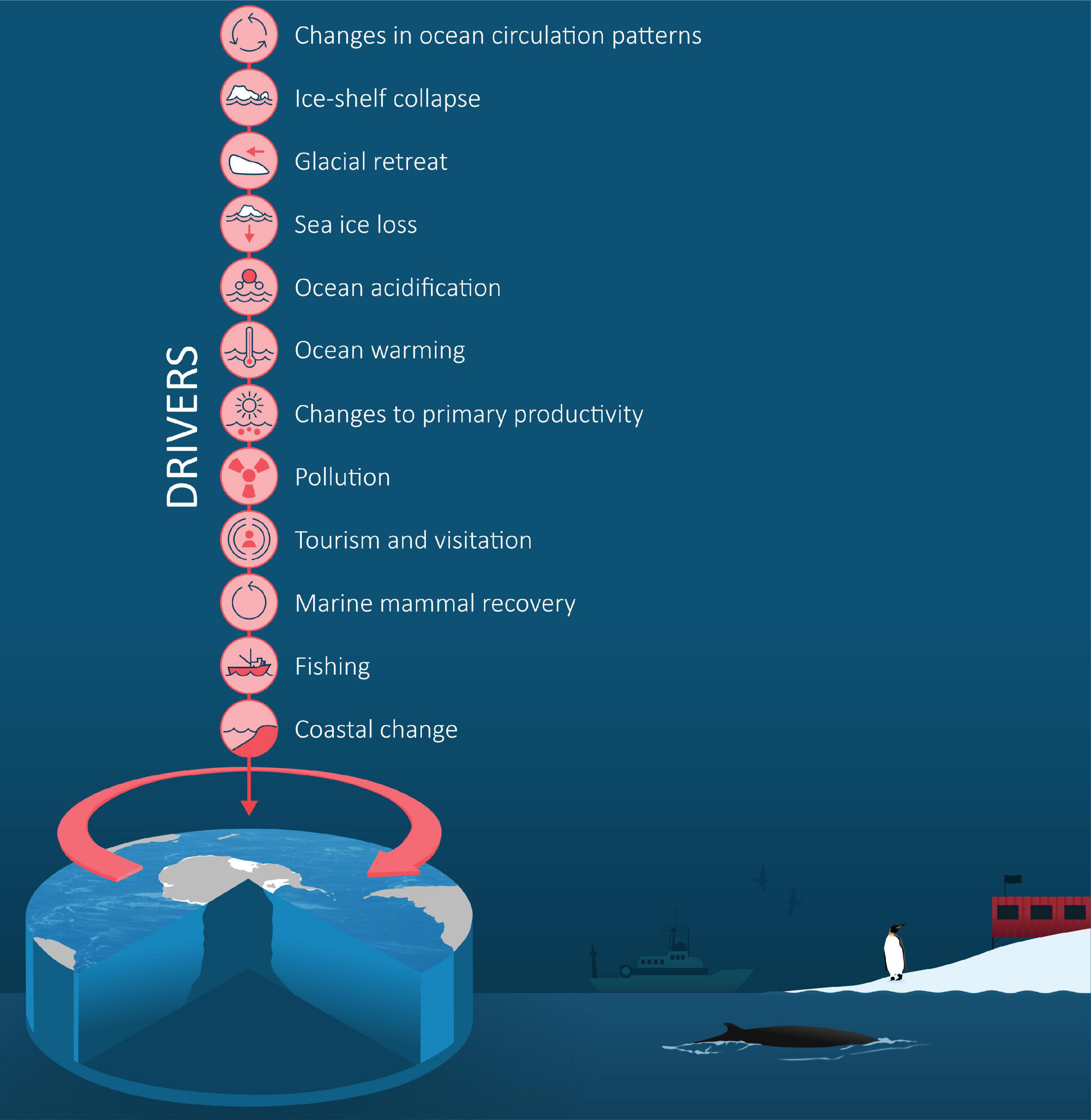
Figure 2. Key drivers of change in metazoan zooplankton taxa within the Southern Ocean. For a complete description of global and local drivers of change in Southern Ocean ecosystems see Morley et al. (2020) and Grant et al. (2021).
Key Taxa in Southern Ocean Zooplankton Communities
Four zooplankton taxa are notable for their biomass and abundance: euphausiids, copepods, salps, and pteropods (Atkinson et al., 2012). There is also increasing awareness and insight into their roles in maintaining Southern Ocean ecosystem structure and function, including locally and globally important ecosystem services (Grant et al., 2013; Rogers et al., 2020; Trebilco et al., 2020; Cavanagh et al., 2021), and their susceptibility to global change. Members of these taxa have evolved distinct and complex life histories, habitat, and environmental preferences which define their spatial and temporal distributions, and form part of the key zooplankton communities described above with varying degrees of dominance and support regional pelagic food webs (Figures 3, 4) across the MEASO areas (see Supplementary Table 1; Bestley et al., 2020; Pinkerton et al., 2020; Brasier et al., 2021; Caccavo et al., 2021; McCormack et al., 2021).
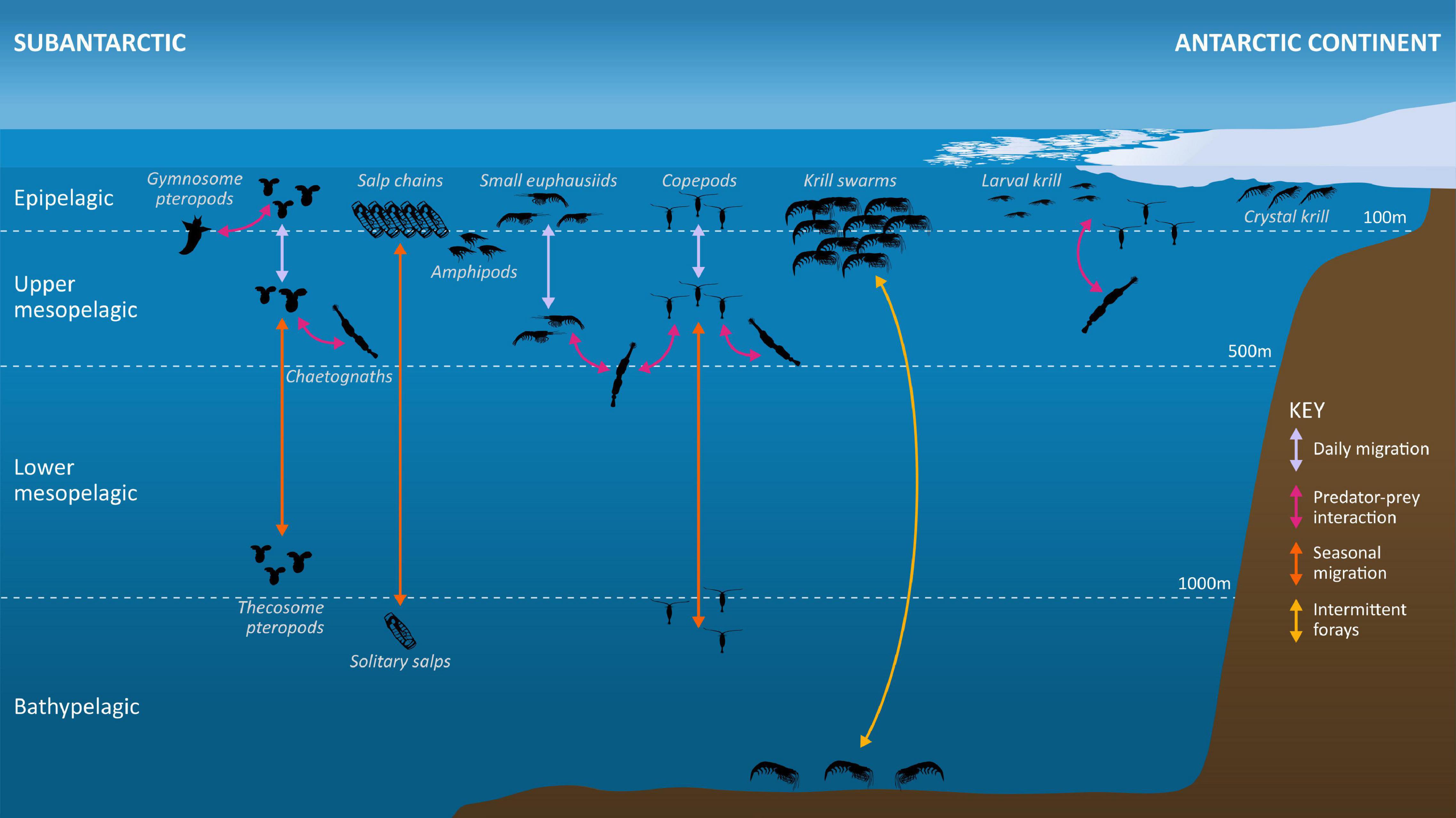
Figure 3. Metazoan zooplankton occupy a range of habitats throughout the Southern Ocean, from the continent to the subantarctic islands, from the surface waters to the deep ocean, and in association with sea ice. They migrate vertically over daily and/or seasonal timescales. Several species of euphausiids, copepods, salps and pteropods are notable for their biomass and importance in maintaining Southern Ocean food webs and ecosystem structure and functioning, including as key consumers and prey items in supporting benthic communities and predator populations, and in biogeochemical cycles and carbon budgets. A number are also important in the diets of fish, marine mammals and seabirds that underpin wildlife tourism and commercially exploited fish species (Antarctic toothfish, Patagonian toothfish, and mackerel icefish), while Antarctic krill (Euphausia superba) is the direct target of harvesting operations. Not illustrated (for clarity) are a variety of other detritivorous or carnivorous groups, for example ostracods, cnidarians, appendicularians, polychaetes, decapods, etc., whose predominance increases with depth. We have illustrated chaetognaths as a major predator of the key taxa illustrated here, although many other taxa such as cnidarians and decapods also perform this function. Intermittent forays: performed by Antarctic krill to the seabed are carried out over a range of timescales from daily to seasonal.
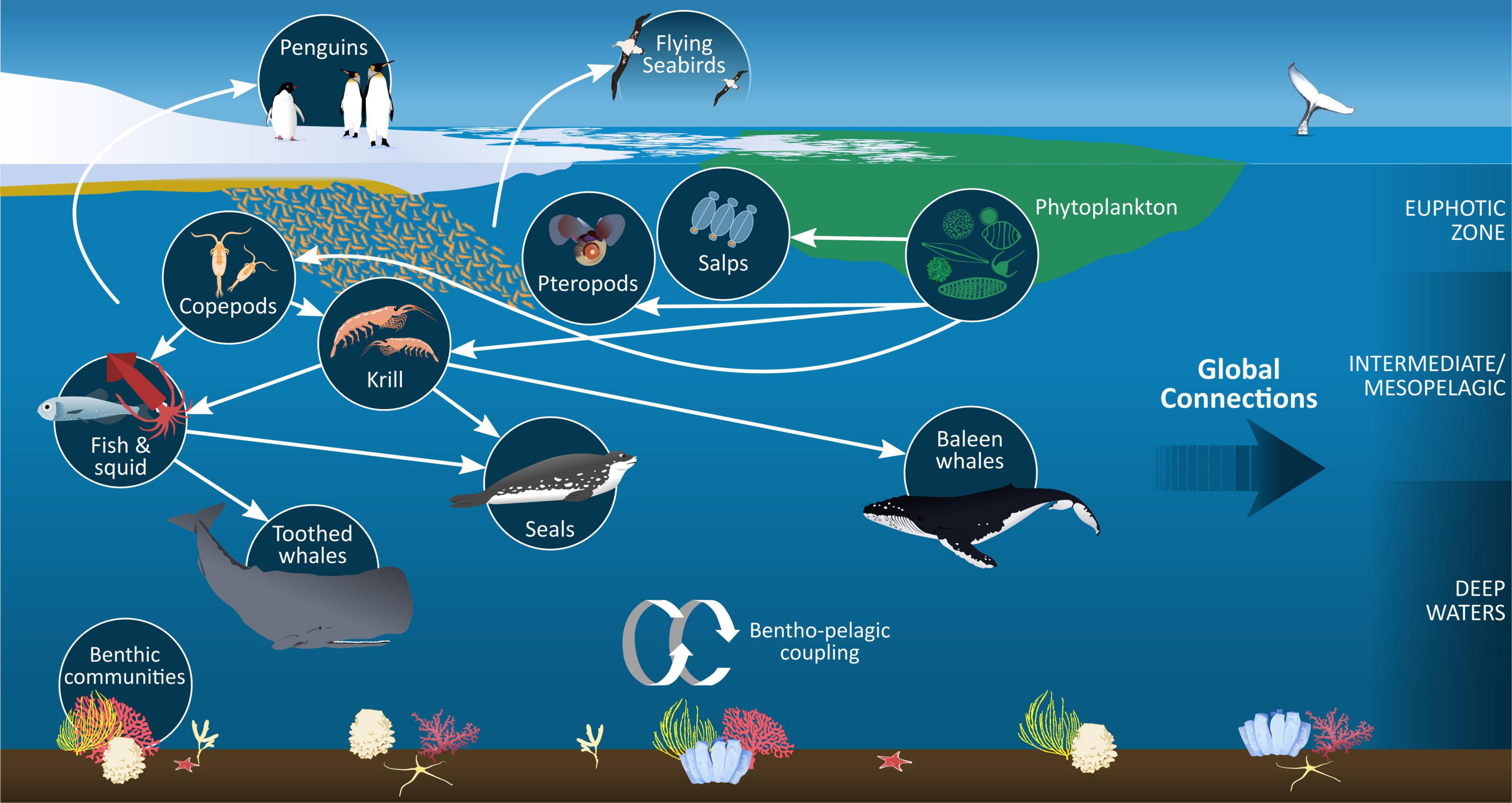
Figure 4. Metazoan zooplankton form integral components of food webs across a range of spatial and temporal scales within the Southern Ocean, providing a link between air breathing predators (including seabirds, seals, and whales), pelagic and benthic communities. Food web connections between these communities are partially determined by feeding strategies and migration patterns/intermittent forays of each zooplankton taxa. Processes associated with zooplankton feeding, vertical migrations and forays, and mortality also contribute to vertical carbon flux, linking productive surface waters with deeper layers and the sediments.
The euphausiids have similar morphological, reproductive, and behavioral (i.e., swarming, migrating) traits, and trophic niches but they vary substantially in their adult size, life span and adaptations to/associations with ocean temperatures (Cuzin-Roudy et al., 2014; Siegel, 2016). The dominant euphausiids include three endemic species. Antarctic krill is considered a keystone species and is the best studied secondary producer. It is the largest and longest-lived euphausiid in the Southern Ocean and a dominant zooplankton omnivore, capable of forming extensive dense swarms (up to several kilometers in length and tens of meters in depth), and channels substantial primary production directly to higher trophic level predators (Siegel, 2000, 2016). Whilst it has a circumpolar distribution and wide latitudinal range in the cool waters south of the APF, its biomass is concentrated in the iron-enriched waters of the southwest Atlantic sector where phytoplankton blooms are common (a region referred to as high nutrient, high chlorophyll, HNHC). Here Antarctic krill is the target of an international fishery (CCAMLR, 2018; Grant et al., 2021) and supports large populations of fish, marine mammals and seabirds, underpinning wildlife tourism and commercially exploited fish species (Antarctic toothfish, Dissostichus mawsoni, Patagonian toothfish, D. eleginoides, and mackerel icefish, Champsocephalus gunnari). At higher latitudes, the endemic congener E. crystallorophias (ice or crystal krill), becomes more common, being restricted to cold (<0°C) shallow coastal waters associated with sea ice and in polynyas. Thysanoessa macrura is perhaps the most numerically abundant Southern Ocean euphausiid, with a cosmopolitan distribution, contributing to zooplankton communities across a much wider (and more northerly) latitudinal range than E. superba and E. crystallorophias.
In high nutrient, low chlorophyll (HNLC) regions of the Southern Ocean, copepods dominate the zooplankton fauna with a similar biomass but greater total production than Antarctic krill (Atkinson et al., 2012). Across this group, there is considerable diversity in life history traits and strategies, particularly in their morphological, reproductive, and behavioral traits, and dietary preferences, ranging from the small (1 mm) egg brooding, opportunistic omnivorous Oithona similis to the large (∼10 mm) free spawning and primarily herbivorous Rhincalanus gigas. The deep winter diapause (or less active) stage of the endemic Calanoides acutus and some of the larger species, such as R. gigas, integrate energy rich lipid production from phytoplankton across space and time, which fuels upper trophic levels (Schnack-Schiel and Hagen, 1995; Atkinson, 1998; Pasternak and Schnack-Schiel, 2001) and may contribute to a significant, but yet poorly known, proportion of Southern Ocean carbon sequestration via mortality and the “lipid pump”(Shreeve et al., 2005; Jónasdóttir et al., 2015).
Salps (particularly Salpa thompsoni and Ihlea racovitzai), together with euphausiids and copepods, comprise the bulk of the zooplankton both in terms of absolute numbers and biomass (Pakhomov et al., 2002; Pakhomov, 2004; Siegel, 2016). These large gelatinous pelagic tunicates can form seasonally high densities. South of the APF, they are major grazers of smaller phytoplankton, and their importance and spatio-temporal variability in food web linkages, particularly those with higher trophic levels, and vertical carbon flux, is becoming increasingly realized (Kelly et al., 2020; Henschke et al., 2021). Whilst I. racovitzai occurs in cold ice-covered regions close to the Antarctic continent, S. thompsoni occupies a wider latitudinal range, preferring warmer waters of the APF. These temperature preferences generally result in spatial segregation from E. superba, although co-occurrence is also observed. Although the relative role of euphausiids, copepods and salps in carbon and biogeochemical cycling is still poorly resolved despite decades of research, the extensive abundances and biomass of the euphausiids, copepods and salps imply their potential significance in driving biogeochemical cycling and feedback processes across the circumpolar ocean (see Henley et al., 2020).
Pteropods are another important member of the Southern Ocean zooplankton, and some of the highest population densities in the global distribution of this taxa are estimated to occur in this region (Hunt et al., 2008; Roberts et al., 2014). Although they account for a lower biomass and energy flow than euphausiids, copepods, and salps the information on the species typical of the Southern Ocean (representative of the shelled Thecosomata and non-shelled Gymnosomata) has increased in recent years in response to concerns about the impacts of ocean warming and aragonite undersaturation resulting from ocean acidification. This has revealed high ingestion rates and distinct trophic roles of these orders, as well as the potential importance of the thecosomes in carbon flux and their vulnerability to ocean acidification (see Figuerola et al., 2021). Pteropod community structure differs north and south of the APF and their biomass is concentrated between 70 and 80°S and in HNHC regions generally. They are considered consistent and ocassionally dominant components of zooplankton communities, collectively contributing to between 5 and 63% of zooplankton abundance in some locations. There are also important food web dynamics among pteropods communities as gymnosomes are preferential predators on the predominantly herbivorous thecosomes (Weldrick et al., 2019).
Given the apparent importance of euphausiids, copepods, salps, and pteropods it follows that, depending on their response to direct and indirect changes in environmental drivers, they have the potential to modify food webs and the structure and functioning of whole ecosystems across various spatial and temporal scales within the Southern Ocean. A focus on these taxa is therefore integral to understanding, predicting and managing the consequences of the impacts of anthropogenically driven change on Southern Ocean ecosystems. There are of course additional species within these taxa, as well as a range of other taxonomic groups within Southern Ocean zooplankton communities. These include amphipods, chaetognaths, and other detritivorous or carnivorous groups whose predominance increases with depth, such as formanifera, ostracods, cnidarians, appendicularians, polychaetes, and decapods. Unfortunately, lack of knowledge and data on their ecological dynamics and roles in the structure and functioning of Southern Ocean ecosystems precludes them from being considered in the present assessment.
Euphausiids (Family Euphausiidae)
Antarctic krill
Antarctic krill (hereafter krill) is the most studied Southern Ocean zooplankton species (Siegel, 2016). Their high biomass (Siegel and Watkins, 2016), relatively large size (up to >60 mm, Tarling et al., 2016a) and ability to feed on a wide range of prey (e.g., phytoplankton, micro- and mesozooplankton, and detritus) (Pakhomov, 2000; Schmidt et al., 2014, 2018) mean that they have a significant influence on the structure and functioning of Southern Ocean ecosystems. Where krill are abundant, they support large populations of predators (Trathan and Hill, 2016) ranging in size from mesopelagic fish (length c. 0.1 m, including commercially harvested fish species) to baleen whales (length up to c. 25 m). Krill therefore play an important role in regulating the flow of nutrients to mid and higher trophic levels (Atkinson et al., 2001; Whitehouse et al., 2008; Schmidt et al., 2011; Hill et al., 2012). Many krill predators are non-specialists and can adapt to short-term fluctuations in krill abundance by feeding on alternative prey such as copepods, amphipods and fish (Croxall et al., 1999; Waluda et al., 2012). However, the availability of these alternative prey, their own dependence on krill and relative nutrient value, and the flexibility of predator diets will all play a role in determining how changes in the distribution and biomass of krill affects dependent predators and the wider ecosystem (Ducklow et al., 2007; Murphy et al., 2007a,2012a; Staniland et al., 2007; Collins et al., 2008; Shreeve et al., 2009; Hill et al., 2012; Watters et al., 2013; Klein et al., 2018; Saunders et al., 2019). Krill also play a potentially important, but largely unquantified, role in ocean biogeochemical cycles (Cavan et al., 2019) with locally intense feeding, egestion and molting conveying megatons of carbon per year from surface to deep waters. In the marginal ice zone fecal pellet flux has been estimated to be 0.04 G tons C year–1 (Belcher et al., 2019) and in the north Scotia Sea the flux due to molted exoskeletons matches that due to fecal pellets (Manno et al., 2020). Conversely, there is little evidence that krill exert significant top-down control on primary production (Atkinson et al., 2014) although at smaller scales predation impacts may be intense and their nutrient excretion can promote phytoplankton turnover rates. Krill is also the target of the largest Southern Ocean fishery, which currently removes >400 k ton year–1 (CCAMLR, 2020, see also Figure 5).
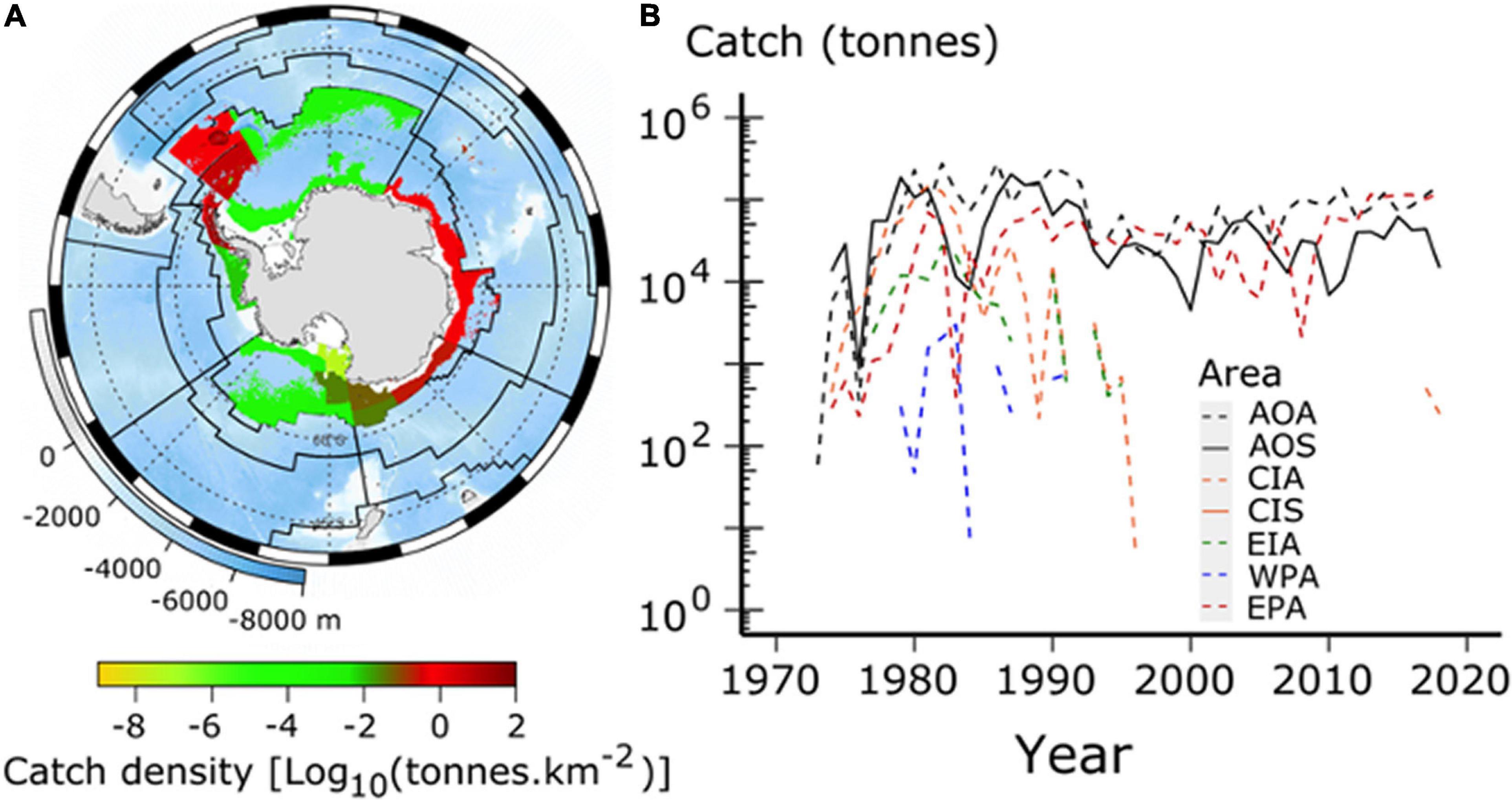
Figure 5. Catches of Antarctic krill (Euphausia superba) from 1970 to 2018, derived from the Commission for the Conservation of Antarctic Marine Living Resources (CCAMLR) Statistical Bulletin 2019. (A) Shows total accumulated catch density over this period as log10 (catch density in tons.km–2) (see colour ramp at bottom). Background is ocean depth in meters (bottom left legend on map). Black lines show boundaries of Marine Ecosystem Assessment for the Southern Ocean (MEASO) areas as depicted in Figure 1. Grey lines show a graticule (see Grant et al., 2021). (B) Shows time series of catches of Antarctic krill in each of the MEASO areas (log10 transformed Y-axis). Legend embedded in plot indicates line types for each area, where acronyms relate to MEASO sectors (first two letters) and zones (last letter). Sectors are Atlantic (AO), Central Indian (CI), East Indian (EI), West Pacific (WP), and East Pacific (EA). Zones are Antarctic (A), Subantarctic (S), and Northern (N).
The importance of krill to the Southern Ocean ecosystem and as a fisheries resource is reflected in a number of dedicated reviews (Everson, 2000; Atkinson et al., 2012; Flores et al., 2012a; Hill et al., 2016; Siegel, 2016; Kawaguchi and Nicol, 2020; Meyer et al., 2020; McBride et al., 2021) and specific sections in more general reviews (Constable et al., 2014b; Hunt et al., 2016; Cavan et al., 2019; Saunders et al., 2019; Rogers et al., 2020; Cavanagh et al., 2021) which consider its population status and response to recent climate change. Despite this attention, current understanding is based on a limited suite of observations primarily from the Antarctic Peninsula and Scotia Sea regions (hereafter the southwest Atlantic), with the majority of sampling targeting the epipelagic zone (<200 m) (Atkinson et al., 2012). Consequently, significant knowledge gaps and uncertainties remain (Meyer et al., 2020).
Current Distribution
Siegel and Watkins (2016) provide a detailed review of the distribution of krill. Although models (Cuzin-Roudy et al., 2014) suggest that most of the 32 million km2 marine area south of the APF constitutes suitable habitat (but see Murphy et al., 2017), the species has only been observed in about 60% of this area (Atkinson et al., 2008) with significant concentrations observed mainly in shelf areas of the southwest Atlantic and the Indian sectors (Mackintosh, 1973; Atkinson et al., 2008; Siegel, 2016). Knowledge about krill distribution is limited in some locales such as the ice-covered western Weddell Sea and eastern Ross Sea, which remain largely inaccessible to sampling. The horizontal distribution of krill is patchy at the regional scale (>1,000,000 km2) and there are between-regional differences in its association with key habitat variables, including bathymetry and sea ice, and in the influence of ocean currents (Nicol et al., 2000a; Hofmann and Murphy, 2004; Atkinson et al., 2008; Jarvis et al., 2010; Silk et al., 2016; Davis et al., 2017). For example, high densities in the Indian sector occur primarily within the seasonal ice zone whereas high densities in the southwest Atlantic can also occur in ice free areas (Murphy et al., 2007a; Atkinson et al., 2008, 2019).
Using data spanning 1926 to 2004, Atkinson et al. (2008) estimated that 70% of the circumpolar krill stock was concentrated in the sector between 0° and 90°W (the southwest Atlantic); however, several independent data sources suggest that the circumpolar krill stock is now more evenly distributed with average densities in the Atlantic sector being about twice those seen in other sectors (Siegel and Watkins, 2016; Yang et al., 2020). At finer spatial scales (<1000 km), krill can be highly aggregated, with the majority of individuals occurring in large swarms (over >300 m2) during summer (Murphy et al., 2004a,b; Thorpe et al., 2007; Tarling et al., 2009; Meyer et al., 2017). The degree of aggregation varies temporally and spatially, possibly associated with food availability and predation risk.
Krill aggregation characteristics are also dependent on demography. This is a long-lived species (potentially surviving >5 years), which spends 1 year in its larval and juvenile stages. Females and males reach maturity at approximately 2 and 3 years, respectively (Reiss et al., 2017). Distributions of the different size, age or maturity categories appear to be dependent on the spatially restricted habitats which support successful spawning and first winter larval survival (Piñones and Fedorov, 2016; Siegel and Watkins, 2016; Meyer et al., 2017; Murphy et al., 2017; Perry et al., 2019; Thorpe et al., 2019). North of the Antarctic Peninsula adults appear to migrate offshore in late spring/early summer to spawn in waters deep enough (1000 m) to allow the developmental decent-ascent cycle, followed by onshore migration for overwintering (Siegel, 1988; Trathan et al., 1993; Reiss et al., 2017; Thorpe et al., 2019; Meyer et al., 2020). Krill are also reported to undergo episodic dispersal in ocean currents which might connect populations over large scales (>1000 km) (Hofmann and Murphy, 2004; Thorpe et al., 2007; Atkinson et al., 2008; Young et al., 2014).
In ice-free conditions krill is more abundant in epipelagic waters (Marr, 1962; Lascara et al., 1999; Jarvis et al., 2010) but the species also occurs in the mesopelagic zone (200 to 1000 m) and has been observed in the bathypelagic zone (>1000 m). It also associates with the seabed in both shallow and deep waters where it feeds on phytodetritus (Schmidt et al., 2011). In ice-covered waters, specialized sampling methods indicate that a large part of the population is in the upper water column, with some proportion of it concentrated at the ice-water interface (Daly and Macaulay, 1988; Marschall, 1988; Daly and Macaulay, 1991; Brierley et al., 2002; Flores et al., 2012a).
Evidence of Past Change
Genetic evidence suggests that krill populations underwent a significant increase over the past 100,000 years, a period that was largely glacial (Goodall-Copestake et al., 2010). More recent changes in population size, demographic structure and distribution have primarily been documented for the southwest Atlantic where regular monitoring was initiated in the early 1990s. However, this monitoring covers only a small proportion (<5%) of the species’ habitat (Hill et al., 2016). There is also a composite dataset, KRILLBASE, which compiles all available circumpolar net sampling data for most years since 1976 as well as some years in the 1920s, 1930s, and 1950s (Atkinson et al., 2017). Regional scale krill biomass in the southwest Atlantic sector has only been surveyed twice, in 2000 and 2019. These two surveys of an area of c. 2 M km2 provided similar biomass estimates (60.3 and 62.6 Mt, respectively) (SC-CCAMLR, 2010; Krafft et al., 2021).
Various indices have been used to examine variability and change in krill population size in the southwest Atlantic over the past several decades. These indices include mesoscale (<125,000 km2) biomass estimates (Fielding et al., 2014; Kinzey et al., 2018), estimates of numerical density (number per unit sea surface area) at various spatial scales (Loeb et al., 1997; Atkinson et al., 2004, 2019; Saba et al., 2014; Loeb and Santora, 2015), and indices derived from time series of predator performance (Watters et al., 2020), predator diets (Forcada and Hoffman, 2014) and isotope signatures (Huang et al., 2011), and anecdotal evidence based on personal field experience (Watters et al., 2013). Some studies indicate a declining trend in krill population size (Loeb et al., 1997; Atkinson et al., 2004, 2019; Huang et al., 2011; Trivelpiece et al., 2011; Forcada and Hoffman, 2014; Loeb and Santora, 2015; Yang et al., 2020) while other studies suggest a more stable trajectory in this sector (Fielding et al., 2014; Saba et al., 2014; Kinzey et al., 2015, 2018; Cox et al., 2018).
Several studies have identified environmental correlates of krill distribution, recruitment, and population size indices (Loeb et al., 1997; Atkinson et al., 2004, 2019; Huang et al., 2011; Trivelpiece et al., 2011; Fielding et al., 2014; Forcada and Hoffman, 2014). These correlates include sea ice in both the southwest Atlantic and Indian Ocean sectors (Loeb et al., 1997; Nicol et al., 2000a,b; Atkinson et al., 2004; Braithwaite et al., 2015) and two broad indicators of ocean circulation and climate variability (El Niño Southern Oscillation, ENSO, and Southern Annular Mode, SAM) in the former (Saba et al., 2014; Loeb and Santora, 2015; Atkinson et al., 2019). Some studies suggest mechanisms by which climate fluctuations propagate through the ecosystem; first affecting regional physics which in turn affect krill recruitment and dispersal, driving population dynamics and hence abundance and biomass (Murphy et al., 1998; Murphy et al., 2007a,b; Thorpe et al., 2007; Saba et al., 2014). These relationships suggest that population changes might be driven, in part, by climate change (Atkinson et al., 2004, 2019; Huang et al., 2011; Trivelpiece et al., 2011; Forcada and Hoffman, 2014).
Limited sampling in the Indian Ocean sectors has not provided any evidence of a trend in that location (Nicol et al., 2000a; Atkinson et al., 2004; Jarvis et al., 2010). Yang et al. (2020) conducted a circumpolar analysis of the available data. They concluded that a detectable decline in krill numerical density (number per m2) was specific to the Atlantic sector, whereas densities in the other sectors changed little over time, resulting in a krill population that is now more evenly distributed around the continent than it was in the past.
Resolving the uncertainty in conclusions about population trajectories, and the influence of spatial scale, time series length and index type (i.e., different indices based on biomass, abundance, population structure of predators) on these conclusions, is a current key research priority (Meyer et al., 2020). It is clear that any reported trend applies only to the time period analyzed and additional evidence is necessary to distinguish it from variability operating over longer timescales (Loeb and Santora, 2015). It is also clear that, even in the southwest Atlantic sector, there are spatial differences in observed patterns of krill variability and change (Atkinson et al., 2004, 2019; Hill et al., 2016; Cox et al., 2018). A recent study suggests some coherence in these patterns, indicating that the krill distribution within this sector has contracted southwards since the 1970s, with a decline in numerical density near their northern limit, and more stability in southern shelf areas (Atkinson et al., 2019).
Response to Drivers
Several important perturbations have affected the Southern Ocean ecosystem over the past two centuries. These include the sequential depletion of krill predators, which began with Antarctic fur seals in the late 18th century and progressed to baleen whales and several finfish stocks in the 20th century (Croxall and Nicol, 2004; Grant et al., 2021). They also include a prolonged period of warming and sea ice loss that occurred in the southwest Atlantic sector in the 20th century (Whitehouse et al., 2008; Stammerjohn et al., 2012; Maksym, 2019; Meredith et al., 2019), albeit with a hiatus over the last two decades (see Morley et al., 2020). Also, commercial harvesting of krill began in the 1970s and gradually became concentrated in the southwest Atlantic sector, moving further south and becoming more winter focused over time (Kawaguchi and Nicol, 2020; Meyer et al., 2020). Of these changes, krill fishing probably had the least impact on krill populations at the circumpolar and regional scales. Catches (circumpolar maximum 528 kt y–1) have always been <1% of available biomass estimates at both of these scales (Hill et al., 2016; Nicol and Foster, 2016; Siegel and Watkins, 2016). Nonetheless, recent studies suggest that fishing can impact the density of krill at small scales (<10 km) and that these effects can propagate through to their predators (Santa Cruz et al., 2018; Krüger et al., 2020; Watters et al., 2020).
The ecosystem effects of predator depletion are potentially complex as recovery occurs at different rates which can alter the balance of predators over long timescales (Murphy, 1995; Croxall and Nicol, 2004). While there is a long-established expectation that increased predation negatively impacts krill populations (Laws, 1977), there is also a hypothesized positive feedback between baleen whale and krill populations. This states that pre-exploitation whale and krill populations may have consumed and recycled larger quantities of iron thereby enhancing overall ocean productivity and the production of krill (Smetacek, 2008; Nicol et al., 2010; Henley et al., 2020). Changes to predator populations as a result of past perturbation are still ongoing (Branch et al., 2007; Branch, 2011; Zerbini et al., 2019) and the full implications of these changes for krill populations remain to be explored.
The most reliable environmental predictor of krill biomass and numerical density discovered to date is sea floor bathymetry (Atkinson et al., 2008; Hill et al., 2009; Silk et al., 2016). Densities are generally highest over island shelves and shelf breaks and decrease offshore, although the lower densities offshore occupy a greater area and constitute most (up to 80%) of the observed biomass (Atkinson et al., 2008; Hill et al., 2009; Silk et al., 2016). However, distribution and abundance are also influenced by a suite of complex associations with other environmental characteristics, including water temperature, ocean acidification (OA), food availability, frontal activity, and the presence of sea ice. Changes in any of these variables have implications for krill populations which are discussed below.
Adult krill, within their optimal thermal range (0–3°C Atkinson et al., 2006; Tarling et al., 2006), are able to grow and reproduce if metabolic demand is met by food intake (Atkinson et al., 2006; Flores et al., 2012a; Hill et al., 2013), but their performance is likely to decrease at temperatures >3°C due to thermal stress (Constable and Kawaguchi, 2018). Larval and juvenile krill, with lower energy reserves compared to adults, are more vulnerable to extended periods of starvation (Hagen et al., 2001; Yoshida et al., 2009). Temperatures >3°C also negatively impact embryo development causing a rapid decline in egg hatching rate and increasing malformation (Perry et al., 2019, 2020).
The reported relationships between krill numerical density and sea ice extent in the southwest Atlantic sector (Atkinson et al., 2004, 2019) and between the circumpolar distributions of both krill and sea ice (Brierley et al., 2002) suggest that population status is closely linked to sea ice conditions. Systematic observations of krill’s association with sea ice began in the late 20th century (e.g., O’Brien, 1987; Daly and Macaulay, 1988; Marschall, 1988; Stretch et al., 1988; Daly and Macaulay, 1991). These studies have revealed a rich variety of contrasting behaviors at different life history stages, in different locations, and at different times of the year, which indicates a high degree of plasticity. In a region of the Lazarev Sea with persistent sea ice cover, the horizontal distribution of krill is related to ice thickness and a large part of the population remains in the upper two meters of the water column throughout the year (Flores et al., 2012a,2014). Elsewhere, in the Scotia-Weddell seas and the Antarctic Peninsula, typically only a proportion of larval and juvenile stages are observed on the under-surface of sea ice, while the rest of the population is distributed throughout the water column (Daly and Macaulay, 1991; Lancraft et al., 1991; Quetin and Ross, 1991; Daly and Zimmerman, 2004; Meyer et al., 2017).
Overwintering larval survival is a critical bottleneck which can affect recruitment and population size (Daly, 1990; Flores et al., 2012b). Cracks and crevices in sea ice provide a refuge from predation, which enhances krill survival (Meyer et al., 2009, 2010, 2017; Schaafsma et al., 2017). Survival is also enhanced by sea ice biota which are a key food source for krill larvae which, unlike adults, lack the lipid reserves to survive winter without feeding (Quetin et al., 1994; Meyer et al., 2002; Meyer, 2012). In the spring melting sea ice promotes phytoplankton production and releases ice algae into the water column, thereby enhancing the food supply available to krill in open water (Schmidt et al., 2018). Ice-conditioned spring phytoplankton blooms fuel the growth and maturation of early life stages, as well as adult nutrition essential for gonadal development and egg production. Consequently, this productivity supports enhanced larval survival and adult reproductive output (Schmidt et al., 2012; Kohlbach et al., 2019). Nonetheless while sea ice is important it is not always essential for first winter survival. Walsh et al. (2020) found that extensive sea ice does not guarantee a strong recruitment and that in the absence of sea ice larval krill can still survive in the water column.
Future Prognoses
Flores et al. (2012b), Murphy et al. (2018), and McBride et al. (2021) provide detailed reviews of potential direct and indirect effects of climate change on krill. These include the negative impacts of OA on the embryonic and post-larval stages (Kawaguchi et al., 2013; Ericson et al., 2018) and of decreased duration and extent of the seasonal sea ice refugia and feeding grounds (Meyer, 2012; Kohlbach et al., 2017; Meyer et al., 2017; Schaafsma et al., 2017). They also include changes to population size and the quality of krill habitats resulting from ocean warming and changes in both their predators and food sources (Wiedenmann et al., 2008; Hill et al., 2013; Saba et al., 2014; Piñones and Fedorov, 2016; Murphy et al., 2017; Klein et al., 2018; Tulloch et al., 2018; Veytia et al., 2020). Such changes might be modified by concurrent changes in predator populations or fishery catch.
The effects of future OA on krill biology are unclear. Embryonic development and hatching are negatively affected by CO2 at near future levels pCO2 >1000 μatm (Kawaguchi et al., 2013). Conversely adult krill are resilient to these levels and can actively maintain the acid-base balance of their body fluids at pCO2 >1000 μatm (Ericson et al., 2018). The only study on combined impacts of acidification and increased temperature to date suggests shorter-term (48 h) effects were primarily pH driven whereas longer-term (21 days) effects on growth and survival were strongly driven by temperature with little to no pH effect (Saba et al., 2021).
Mechanistic understanding of the interaction between the larval and juvenile stages and ice is still limited (Meyer, 2012; Kohlbach et al., 2017; Meyer et al., 2017; Schaafsma et al., 2017), and therefore the implications of ice loss for larval survival, recruitment and krill population size and distribution are not clearly understood (Murphy et al., 2018). Melbourne-Thomas et al. (2016) suggest that increased instability in sea ice will benefit krill by increasing the availability of larval overwintering habitat. Conversely, sea ice decline may impact phytoplankton community structure, reducing the contribution of large lipid-rich diatoms relative to smaller, less easily filtered and less nutritious taxa (e.g., cryptophytes Deppeler and Davidson, 2017; Pinkerton et al., 2020) and potentially reducing krill feeding efficiency, recruitment, and population size (Saba et al., 2014). Groeneveld et al. (2015) suggest that reductions in sea ice extent could lead to a poleward contraction of the krill population.
Several studies have examined the potential direct effects of ocean warming on krill habitat quality, suggesting that habitat contraction is likely as the temperatures at the northern fringes of their range exceed physiological limits (Wiedenmann et al., 2008; Hill et al., 2013; Murphy et al., 2017; Klein et al., 2018; Veytia et al., 2020). Spatial variability in warming and the possibility of localized increases in primary production could enhance habitat quality in some restricted regions, but these enhancements are unlikely to offset degradation elsewhere (Hill et al., 2013; Murphy et al., 2017; Veytia et al., 2020). Climate change impacts are also likely to reduce the availability of reproductive habitat (Piñones and Fedorov, 2016).
The physiological cycle of adult krill is tightly synchronized with photoperiod (Höring et al., 2018; Piccolin et al., 2018) such that reproduction and larval development coincide with the seasonal cycles of food availability (Kawaguchi et al., 2007; Kawaguchi, 2016). Model projections suggest that the timing and duration of such events could shift with future climate change and thus negatively impact krill population size across its current distributional range (Quetin et al., 2007; Veytia et al., 2020).
The populations of several baleen whale species dependent on krill in Southern Ocean feeding grounds are recovering (Branch et al., 2007; Branch, 2011; Zerbini et al., 2019). Model-based projection studies suggest that climate change could delay their recovery due to reduced krill availability (Klein et al., 2018; Tulloch et al., 2019). However, the hypothesized positive feedback loop whereby nutrient recycling by increased whale populations could enhance primary production and support increased krill stocks (Smetacek, 2008; Lavery et al., 2010; Nicol et al., 2010) may counteract this impact.
Thus the prognosis for krill is highly uncertain but the majority of studies suggest that climate change is likely to increase the physiological stress on both early life stages and adult krill. These effects may be compounded by loss and fragmentation of spawning and overwintering habitat. In the worst case, krill may eventually become restricted to a limited number of areas suitable for successful spawning, survival and recruitment (i.e., those with sufficient sea ice cover and reliable seasonal productivity cycles, see Hofmann and Hüsrevoğlu, 2003; Kawaguchi et al., 2007; Kawaguchi, 2016; Thorpe et al., 2019). Reductions in krill biomass and distribution are also likely to impact krill predator populations (Hill et al., 2013; Klein et al., 2018). In projection studies the severity of the impacts on krill and its predators generally increases with the severity of climate change (e.g., Hill et al., 2013; Kawaguchi et al., 2013; Klein et al., 2018).
Resilience
Krill is well known for its behavioral plasticity and variations in life history strategies that have allowed it to flourish in the highly variable Southern Ocean environment and to adapt its pattern of habitat use according to seasonally localized food availability. This plasticity is demonstrated by the high levels of variability in krill’s association with and apparent dependence on sea ice (Meyer et al., 2017) and by rapid acclimation of individuals to the effects of reduced pH (Saba et al., 2021). Adults are able to survive long periods without food using a combination of lipid reserves and reductions in body size (Quetin et al., 1994; Tarling et al., 2016b). Krill have successfully colonized a range of habitats from the ocean surface to abyssal depths, and from continental ice shelves to the APF (Atkinson et al., 2008). This plasticity confers considerable resilience and is likely to ensure the survival of krill as a species. Current understanding of resilience in krill is based primarily on observations of the diversity of krill behaviors and habitats or experiments on individuals. Understanding of the consequences for krill populations is limited. For example, feeding on phytodetritus on the seabed (Schmidt et al., 2011) might allow individuals to avoid adverse conditions closer to the surface. Whether this is sufficient to sustain the current krill population depends on many factors, including the availability and nutritional value of the novel food source and connectivity to other life history critical habitats, which remain to be explored.
Other Euphausiids
Aside from the Antarctic krill, there are twelve other euphausiid species in the Southern Ocean (Cuzin-Roudy et al., 2014). For most of these species there is extremely limited data on their biomass relative to Antarctic krill but their biomass are potentially orders of magnitude lower (however, see Wallis et al., 2020). Two species, E. crystallorophias and Thysanoessa macrura, are the most common taxa among this group, and are abundant and ecologically important as prey for upper trophic levels (Ainley et al., 2004). Others are common members of the pelagic community (E. frigida, E. triacantha, E. vallentini, and T. vicina) and may play important but unknown ecological roles in some regions of Antarctica near or south of the APF (e.g., E. triacantha, Liszka et al., 2021), or the broader sub-Antarctic region (e.g., T. vicina). The patterns of occurrence of these euphausiids are generally defined by the latitudinal and bathymetric features of the Southern Ocean, from the neritic environments of the high Antarctic to the pelagic areas north of the APF (Cuzin-Roudy et al., 2014). Many of these taxa undergo large diel migrations, from deep waters during the day to shallower waters during the night (Taki and Hayashi, 2005; Ono et al., 2011; Liszka et al., 2021). Additionally, these taxa vary in their life history strategy and their degree of omnivory. For example, T. macrura has adaptations including a pair of elongated second legs (thoracic legs) that can be used to catch zooplankton, while E. crystallorophias eat sea ice algae and sea ice microbiota. The variety of feeding strategies suggests that food web interactions amongst these taxa and the broader Antarctic ecosystem will vary greatly with climate change. Owing to their biomass some of these euphausiid species also undoubtedly play an important role in biogeochemical cycling (Guglielmo et al., 2009), however, this has yet to be quantified. Here we focus on the most common and best understood of these other euphausiid taxa, E. crystallorophias and T. macrura.
Euphausia crystallorophias
Crystal krill is smaller than Antarctic krill, reaching a maximum length of ∼40 mm over its 4 to 5-year lifespan (Siegel, 1987). The species typically feeds on small particles in the water column, including phytoplankton, microzooplankton (Kohlbach et al., 2019) and small zooplankton (O’Brien, 1987; Pakhomov and McQuaid, 1996). In addition, juveniles and adults have been observed on the sea floor feeding on phytodetritus (Deibel and Daly, 2007). Crystal krill is also an important prey item for upper trophic levels in the coastal environments of the high Antarctic (Thomas and Green, 1988; Pakhomov, 1997; Ainley et al., 2004).
Current Distribution
Crystal krill is a circumpolar high Antarctic species that replaces Antarctic krill in shallow (<500 m) neritic environments (Thomas and Green, 1988; Pakhomov, 1997; Ainley et al., 2004) covered by sea ice or in open water areas such as polynyas. It has been found as far north as the northern Antarctic Peninsula (Loeb et al., 2009), but has its greatest densities farther south than about 70° (Mauchline, 1969). It is common around the coastal areas of east Antarctica, and the Amundsen Sea (La et al., 2015) and is also abundant on the shelves of the Weddell (Boysen-Ennen and Piatkowski, 1988; Boysen-Ennen et al., 1991) and Ross Seas (Sala et al., 2002; Murase et al., 2006; Davis et al., 2017). Like other euphausiids, E crystallorophias undergoes diel vertical migrations (Conroy et al., 2020) that may deepen in winter, or in the presence of krill predators (Ainley et al., 2015).
Because the oceanography of coastal environments varies greatly around the Antarctic continent, crystal krill populations are largely self-contained, and some modelling studies have shown that population structure may be effectively aided by the currents in the areas of spawning (Piñones et al., 2016) or retained in areas because of the physical processes unique to the coastal ecosystems around the continent. However, genetic studies have shown that the genetic structure is not solely a function of distance between populations, suggesting that small scale processes are also important in the population structure in different parts of the Antarctic (Jarman et al., 2002).
The neritic nature of crystal krill ties reproduction and population dynamics to the seasonal coastal sea ice conditions including the opening of polynyas. Spawning starts under fast ice but peaks during coastal polynya breakout (Pakhomov and Perissinotto, 1996). Crystal krill eggs are neutrally buoyant and concentrate in the surface layers and larvae are associated with sea ice but appear to have a different vertical distribution (either shallower or deeper) than E. superba larvae (Pakhomov and Perissinotto, 1996; Daly and Zimmerman, 2004; Wiebe et al., 2011), which may reduce bottom predation of eggs by shelf benthos and grazing competition between these morphologically similar species, in areas where they overlap. The larvae are also abundant in inshore waters where they develop over the summer and into the following winter, taking about twice as long as E. superba to reach stage furcilia CIV (Kirkwood, 1996) and recruit to juvenile stages in spring (Pakhomov and Perissinotto, 1996; Daly and Zimmerman, 2004).
The biomass of crystal krill can be high in some areas around the Antarctic. For example, La et al. (2015) measured ice krill biomass in the Amundsen Sea polynya and found mean biomasses of 16 g fresh mass m–2, an order of magnitude higher than those found in the Ross Sea (Sala et al., 2002) and of more similar magnitude to those reported for Antarctic krill. Other regions also had high biomass (Boysen-Ennen et al., 1991). In some cases, the regional peculiarities that make some polynyas important for crystal krill consequently make this species more abundant and these areas important for high latitude food webs (Pakhomov and Perissinotto, 1996).
Evidence of Past Change
From an evolutionary perspective E. crystallorophias is highly adapted to the high Antarctic ecosystem and may be constrained in a warming future owing to some genetic adaptations. Between 40 and 400 kya, E. crystallorophias underwent a population crash or directional selection to cold adaptation over time owing to periods of glaciation and deglaciation that resulted in the loss of the genetic diversity in its heat shock proteins. The diversity of heat shock proteins is thought to provide phenotypic plasticity to species to tolerate and adapt to changing environments (Cascella et al., 2015; Papot et al., 2016).
There are few long-term studies of crystal krill with which to draw conclusions about past changes. Along the northern Antarctic Peninsula, where the U.S. Antarctic Marine Living Resources (AMLR) Program has sampled since the early 1990s, crystal krill are sporadically found around the South Shetland Islands and also on the west side of the peninsula but the data are not sufficient to build a time series to examine changes in their temporal abundance (U.S. AMLR unpublished data). Further west along the Antarctic Peninsula, there is some evidence for variability in crystal krill populations, in conjunction with variability in other ice-dependent taxa like silverfish (Ross et al., 2014). A 30 year time-series, from 1990 through to 2019, shows an increase in crystal krill abundance in the southern part of that study area, and is attributed to an increase in primary production or more favorable timing of spring sea ice retreat for larvae (Steinberg et al., 2015). Along other coastal regions of the Southern Ocean, patterns of distribution are similar over time, reflecting the neritic, polynya, and ice-covered waters that have shaped the life history of this important species. In the Prydz Bay region of the East Antarctic (Pakhomov and Perissinotto, 1996; Yang et al., 2011) and in the Ross Sea, surveys of crystal krill distribution conducted over the last several decades are not sufficient to document changes in abundance or biomass but do show considerable variability.
Response to Drivers
The high Antarctic, neritic nature of crystal krill suggests that its productivity and response to environmental drivers is tied to ecological adaptations associated with the oscillating regime of shelf environments and may be strongly influenced by the dynamics of coastal polynyas (Pakhomov and Perissinotto, 1996).
Future Prognoses
There have been few assessments of the likely trajectory for E. crystallorophias under different climate change scenarios. However, because crystal krill is a high Antarctic species, and has a narrow thermal habitat constrained to waters ≤2°C (Guglielmo et al., 2009), this makes crystal krill stocks vulnerable to climate change. Given their lower diversity of heat shock protein systems, E. crystallorophias may be physiologically less resilient to thermal shocks, so in geographic areas where ocean surface and subsurface areas are warming it could be more vulnerable to climatic change compared to Antarctic krill (Cascella et al., 2015; Papot et al., 2016).
Changes in sea ice extent and duration, especially at the northern limits of its range, are likely to negatively impact its preferred habitat greatly. Declines in the extent and duration of sea ice (Stammerjohn et al., 2008, 2012) or the timing of closing of polynyas will impact both larval and juveniles stages that rely on seasonal primary production in their first year of life, and will impact post-larvae perhaps by limiting their ability to accumulate lipids necessary for reproduction.
Simulations of future changes in sea ice concentrations suggest that the extreme loss of spawning habitat might greatly diminish the reproductive success of crystal krill (Smith et al., 2014). In some areas, simulations of future temperature, ice and vertical mixing depths on the Ross Sea continental shelf suggested that stratification will increase and favor diatoms, which likely would allow for enhanced ingestion by crystal krill, but due to the loss of ice (and spawning habitat) in the next 100 years, populations would decrease significantly (Smith et al., 2014). Further simulations suggested that the seasonal structure of phytoplankton production may change, with a shift toward Phaeocystis antarctica, a colonial haptophyte, but that this production would be largely unavailable to crystal krill (Kaufman et al., 2017). Both future scenarios suggest a mechanism for the decline in crystal krill success.
Changes in sea ice dynamics along the coast and warming of waters would, in general, create additional stresses on this thermally constrained taxon directly by affecting its habitat but also indirectly as increased water temperatures could make some environments more hospitable to other species like Antarctic krill or T. macrura. Interactions with these and other species (e.g., silverfish) are unknown. Continued study of the biology and ecology of crystal krill is warranted, and the establishment of monitoring programme in selected high Antarctic environments could provide data to better constrain projections about the future status and trends of this species and the animals that are dependent on it.
Resilience
The limited (thermal and geographical) range of crystal krill (Guglielmo et al., 2009) and the genetic adaptations that made it successful during periods of increased glaciation are likely to negatively impact this species in the future (Cascella et al., 2015; Papot et al., 2016). The capacity to move to other areas is undoubtedly low, and its neritic nature means that this taxon is not likely to be resilient to directional climate change as the quality of physical habitat at lower latitudes it occupies declines over the foreseeable future (Mackey et al., 2012; Steinberg et al., 2015). Areas in the high Southern Ocean (Pakhomov and Perissinotto, 1996), such as in the Ross Sea, the Amundsen Sea (La et al., 2015) or the southern most parts of the Weddell Sea, where reproductive polynyas may open earlier, may provide refugia for some populations of crystal krill, but lower latitude areas at the limits of its range may result in local extinction of some populations.
Thysanoessa macrura
T. macrura is likely the most numerically abundant euphausiid species in the Southern Ocean, although its circumpolar biomass is unknown (Nordhausen, 1992). It is smaller than Antarctic krill and has a maximum size similar to crystal krill (∼42 mm) but with a shorter lifespan (<4 years) (Siegel, 2000; Haraldsson and Siegel, 2014; Driscoll et al., 2015). T. macrura is omnivorous but more predatory than E. superba (Hagen and Kattner, 1998), feeding on phytoplankton, microzooplankton, and copepods, as well as Antarctic krill larvae (Hopkins, 1985; Hopkins and Torres, 1989; Driscoll, 2019). Through its omnivorous diet, it can receive significant parts of its carbon budget from ice algae (Kohlbach et al., 2019). T. macrura is also an important prey item for upper trophic levels, including both midwater and demersal fish, marine mammals and seabirds. T. macrura’s position as a grazer, predator and prey means that the species’ trophic position contributes significantly to various energetic pathways.
Current Distribution
In contrast to the thermally constrained and high Antarctic life history of crystal krill, T. macrura is a substantially more cosmopolitan taxon (Cuzin-Roudy et al., 2014; Pinkerton et al., 2020). T. macrura is by far the most-identified euphausiid species in Southern Ocean Continuous Plankton Recorder (SO-CPR) survey (Pinkerton et al., 2020). It is found in pelagic and coastal waters across a wide thermal gradient, from near freezing water (−1.8°C) to warm waters (10°C) in the northern latitudes where it overlaps with its congener T. vicina (Hempel and Marschoff, 1980). T. macrura is ubiquitous and relatively abundant throughout its range, without the concentration in the Atlantic sector that is evident in E. superba (McLeod et al., 2010; Yang et al., 2020). In some areas its average abundance can be higher than that of Antarctic krill (Loeb and Santora, 2015; Steinberg et al., 2015). Few zooplankton surveys conduct net tows below 200 m, however, those that have found that T. macrura has a deeper median depth distribution than Antarctic krill (Lancraft et al., 1989; Taki et al., 2008; Marrari et al., 2011) suggesting that most estimates of T. macrura abundance, especially near surface methods such as CPR, are generally biased low. T. macrura also tends to be form less dense and more spatially distributed aggregations during winter, rather than forming dense aggregations and concentrating inshore like E. superba (Nordhausen, 1994; Loeb and Santora, 2015; Driscoll, 2019).
Evidence of Past Change
Like most other zooplankton taxa that are not directly targeted for long-term studies, the temporal patterns in T. macrura abundance are less well defined than the spatial patterns of its distribution. In the Prydz Bay Region (Yang et al., 2011) and along the Antarctic Peninsula temporal patterns in abundance showed considerable variability over the past three decades (1992–2013) (Loeb and Santora, 2015; Steinberg et al., 2015; Driscoll, 2019) but do not show any trends indicative of changing population size. There is some indication that their abundance along the Peninsula may be related to changing climate drivers because growth has been correlated with temperature; however, the drivers of variability in the population are unknown. Pinkerton et al. (2020) found evidence of small (∼0.2%/year) increasing trends of habitat suitability for T. macrura between 1997 and 2018 (see also Section “Past Changes in Zooplankton: A Modelled Example Using Continuous Plankton Recorder Data”).
Response to Drivers
T. macrura’s wide latitudinal distribution suggests it may be more adaptable to changes in its environment, particularly increasing ocean temperature, than other Southern Ocean euphausiids. T. macrura has shown temperature dependent growth, with slower growth in colder Weddell Sea influenced waters compared to warmer Antarctic Circumpolar Current (ACC) waters (Driscoll et al., 2015). In contrast to E. superba’s dependence on summer primary production for reproductive success, T. macrura spawn in winter and early spring using wax ester lipids to support egg development (Hagen and Kattner, 1998; Wallis et al., 2017). This life history strategy decouples production from the spring bloom which is required for gonad development in E. superba. T. macrura also have a faster larval development rate than the Euphausia species (Nordhausen, 1992; Haraldsson and Siegel, 2014; Wallis, 2018) where later stage larvae are found during summer (Makarov, 1979) and recruitment to juvenile stages has been observed by autumn, suggesting changes in abundance are likely to be driven by direct forcing on the populations (Marrari et al., 2011). Loeb and Santora (2015) also found that postlarval T. macrura abundances near the north Antarctic Peninsula were correlated with lagged ENSO conditions.
Future Prognoses
Temperature dependent growth models for T. macrura indicate increased growth under future climate change scenarios (Driscoll et al., 2015; Richerson et al., 2015). These models also indicate that the biomass per recruit may increase in the future and surpass that of E. superba in some areas (Richerson et al., 2015). Habitat suitability modelling for euphausiids sampled by the CPR, in which catches are dominated by T. macrura (Pinkerton et al., 2020) suggested that increasing environmental suitability between the APF and the northern annual limit of sea ice were related to surface warming and to deepening of the mixed layer depth, whereas predicted decreases in the Pacific sectors were related to shallowing mixed layer depths. Further north, increases in euphausiid environmental suitability were primarily correlated to increased primary production.
Resilience
Thysanoessa macrura may be relatively resilient to the climatic changes and future environments in the Southern Ocean over the next 50 to 100 years (Richerson et al., 2018). This resilience may be driven by several factors: its wide thermal tolerance (Driscoll et al., 2015), its omnivorous diet (Phleger et al., 2002), its highly flexible life cycle, and its independence from the spring bloom and sea ice cycle for reproductive success (Hagen and Kattner, 1998; Haraldsson and Siegel, 2014; Wallis, 2018). However, future climate-influenced changes in prey communities, predators, competitors, and other biotic conditions still may have the potential to impact this species in ways that are difficult to predict. Because of its relatively cosmopolitan distribution, flexible life history and high abundance, future studies should focus on T. macrura’s role in shunting energy around the short and generic phytoplankton-krill-predator food chain model, especially in open ocean environments in the northern extent of its range.
Copepods (Subclass Copepoda)
At the Southern Ocean scale, copepods have a biomass at least equivalent to that of Antarctic krill, with a total production far exceeding that of krill (Conover and Huntley, 1991; Voronina, 1998; Shreeve et al., 2005). Their biomass is dominated by large species of the genera Calanoides, Calanus, Rhincalanus, and Metridia, which perform varying degrees of seasonal vertical migration between summer feeding grounds in the epipelagic zone and overwintering depths below 200 m. The numerically dominant smaller species have a diversity of life history strategies, including the small, egg-brooding, epipelagic cyclopoid Oithona similis (Cornils et al., 2017), seasonal migrants such as Ctenocalanus citer (Schnack-Schiel and Mizdalski, 1994) and sea ice dependent genera such as Drescheriella, Paralabidocera, and Stephos (Schnack-Schiel et al., 1995; Tanimura et al., 1996; Swadling et al., 2004). As a group, copepods provide food for a diversity of both invertebrate and vertebrate predators. Invertebrates are thought to be particularly important copepod predators (Hill et al., 2012), including chaetognaths, amphipods, euphausiids, and cnidarians. Among the vertebrate predators, smaller myctophid fish are particularly important predators of the larger copepods (Collins et al., 2008; Shreeve et al., 2009; Saunders et al., 2019), but also among the air-breathing predators, small petrels include an important component of copepods in their diet. Commensurate with their high circumpolar biomass and production relative to krill and salps, their grazing impacts are also substantial (Hill et al., 2012). In generalizing about the trophic roles of whole taxonomic groups such as copepods, it is important to emphasize the large diversity in size and feeding modes. The major size increase of each species through its ontogeny will expand this overall size range even further. Thus, large predatory groups including species such as Paraeuchaeta antarctica, feed on other copepods, and intraguild predation among the copepods is likely to be important (Hopkins, 1985; Hopkins and Torres, 1989). In addition to the raptorial feeding displayed by P. antarctica, copepod species use suspension and ambush feeding modes, with variations in the contribution of motile cells, non-motile cells, and detrital particles in the diet.
Current Distribution
Copepods typically have circumpolar distributions, similar to other Antarctic zooplankton groups. Latitudinally, a series of circumpolar fronts mark water mass discontinuities and help to define their thermal envelopes. The circumpolar distributions of the dominant copepods were first charted by the Discovery Expeditions of the 1920s and 1930s (Ommanney, 1936; Andrews, 1966) and then by the extensive Soviet expeditions (e.g., Voronina, 1972) with large scale coverage recently provided by the SCAR SO-CPR survey that began in 1991 (Hosie et al., 2003; McLeod et al., 2010; Takahashi et al., 2011; Pinkerton et al., 2020). These distributions have been further resolved by national survey programmes (e.g., Pakhomov and Froneman, 2000; Ward et al., 2006, 2012; Swadling et al., 2010). However, despite the high degree of collaboration over many areas of the Southern Ocean (e.g., the Pacific sectors), many remain under-studied due to their inaccessibility or lack of regular voyages (McLeod et al., 2010). Based on SO-CPR measurements, habitat suitability modelling has been used to “fill in” these spatial sampling gaps and provide insights into seasonal and long-term variability of copepods in the Southern Ocean (Pinkerton et al., 2010, 2020) (see also Section “Past Changes in Zooplankton: A Modelled Example Using Continuous Plankton Recorder Data”).
Evidence of Past Change
There are few copepod time series of sufficient length in the Southern Ocean from which we can document and understand climate change effects over multiple decades. One of the longest, the U.S. AMLR time series, focused on the tip of the Antarctic Peninsula, shows ENSO influences on copepod abundance, acting through the translation of frontal systems and associated high and low phytoplankton concentrations across their study area (Loeb et al., 2010; Zhang et al., 2020).
However, longer timescales of observation are provided by a series of comparisons of distributions between the Discovery and the modern eras, with these comparisons needing to adjust for sampling differences (Vuorinen et al., 1997; Ward et al., 2008). Having standardized sampling methods, Ward et al. (2018) found that the biomass-dominant species Calanoides acutus, Rhincalanus gigas, and Calanus simillimus have increased in abundance in the last 70 years by 20–55%. Further, Tarling et al. (2018) found that the main copepods in the southwest Atlantic sector of the Southern Ocean had broadly conserved their spatial distributions despite a mean ∼0.74°C surface warming. In other words, they are nowadays inhabiting warmer surface temperatures than they were 80 years ago. This is an important result as it is the exception to the general expectations of range shifts (generally polewards to cooler waters) under warming scenarios (Beaugrand et al., 2009). Indeed, Antarctic range shifts have been found in the Atlantic sector, both for E. superba and salps (Pakhomov et al., 2002; Atkinson et al., 2004, 2019), in keeping with those found for aquatic and terrestrial ectotherms more generally (Parmesan and Yohe, 2003). Positive trends for 1997–2018 in modelled environmental suitability for copepods by Pinkerton et al. (2020) were found between the northern extent of seasonal sea ice and the southern limit of the ACC, with linear trends predicted to be highest in the Atlantic and Indian sectors and to exceed 6%/year in some places (see also Section “Past Changes in Zooplankton: A Modelled Example Using Continuous Plankton Recorder Data”).
Response to Drivers
Copepods comprise numerous species with differing vertical and horizontal distributions and life histories (Atkinson, 1998). This means that responses to drivers may differ over time and space. Likewise, drivers can be direct, for example warmer temperatures increasing physiological rates, or indirect, for example climate affecting food sources (Moline et al., 2004; Montes-Hugo et al., 2009) which in turn affect the copepods.
Three “universal” responses to rapid warming have been described for ecototherms. Copepods may shift (1) in geographical range (i.e., polewards range shifts Beaugrand et al., 2009), (2) in phenology (i.e., earlier when warmer Mackas et al., 2012), and (3) in body size (i.e., smaller species’, life stages or adult sizes in warmer environments Daufresne et al., 2009; Horne et al., 2016). The relative degree of expression of these responses is poorly known for zooplankton generally; it is certainly not known for Antarctic copepods. Due to the lack of seasonal coverage over multiple years we have particularly poor understanding of their phenological responses, for example to the rapid changes in sea ice duration along the Western West Antarctic Peninsula (WAP) (Stammerjohn et al., 2012; Henley et al., 2019).
While polar ectotherms are known generally for their stenothermy, the physiology of Southern Ocean copepods is still not well understood. Acclimation has a key role in governing responses to stress, so short term experiments measuring acute stress responses (for example to temperature or OA) are hard to interpret in the context of long-term climate change. For this reason, growth and egg production rate experiments on freshly collected copepods are, arguably, the best indicator of how the natural present-day variation in both food and temperature dictate copepod performance. This allows at least a glimpse of how they may fare in a changing world. Using this method on the ubiquitous, albeit, colder water cyclopoid, Oithona similis, the balance between fecundity and mortality was found to be sensitive to low temperatures, suggesting that increasing temperatures in the Scotia Sea may result in increased abundances (Ward and Hirst, 2007). Likewise, Ward and Shreeve (1998) demonstrated extension of large calanoid egg hatching times with colder temperatures. Shreeve et al. (2002) found changes in egg production rates, carbon mass and abundance of copepod populations at South Georgia were all strongly dependent on food concentrations, saturating only at high (>3μg Chl a L–1) values. This suggests the importance of food limitation in most Southern Ocean environments. This result is consistent with habitat modelling of copepods in the Southern Ocean (Pinkerton et al., 2020) which found that although copepod abundances were related to sea surface temperature (SST) at the large scale, changes to their abundance at a given location were most strongly related to changes in phytoplankton biomass.
Effects from the gradual increase in OA are hard to determine experimentally, but in an acute response microcosm experiment, Tarling et al. (2016b) found that copepods show a stronger preference for dinoflagellates when in elevated pCO2 conditions, demonstrating that changes in food quality and altered grazing selectivity may be a major consequence of OA (Tarling et al., 2016b).
Future Prognoses
An increase in small copepod species has been suggested as a possible future scenario in a warmer Southern Ocean (Murphy et al., 2016), and an Ecopath model experiment of krill or copepod dominance showed that copepod dominance would have major implications for the higher trophic levels that can be supported (Hill et al., 2012). Likewise, commonly made projections include general poleward range shifts of biota and increases in open water blooms over high latitude shelves formerly covered with ice (Constable et al., 2014b; McBride et al., 2014).
While an increasing number of studies are making large scale projections, this review has highlighted the paucity of basic knowledge needed to predict how copepods will respond to future changes in temperature, sea ice, food quantity and quality, and OA. The data obtained so far suggest that we should be very cautious in applying climatic envelope modelling of the type applied successfully to Calanus in the northern hemisphere (Helaouët and Beaugrand, 2007). In fact, north Atlantic Calanus may be an exception rather than the rule, since copepods in that region have showed widely divergent shifts over the last 60 years, with no evidence that their distributions tracked the shifting isotherms (Chivers et al., 2017). In addition to a suite of model-specific issues (Brun et al., 2016), the species may show resilience in maintaining a fixed geographical distribution (Tarling et al., 2018).
Some knowledge of how distributions have changed over past decades is, in our view, essential to generate informed projections for the future of copepods (and all zooplankton taxa examined here). For copepods there is much scope for progress in this area, given the wealth of data on this group that have been collected in Antarctica since the Discovery Expeditions, from a wide variety of nations working across the Southern Ocean. Efforts to retrieve and compile Antarctic krill and salp data have already shown their worth (Atkinson et al., 2017) and national historical data sets on copepods have already been compiled (O’Brien et al., 2017; Cornils et al., 2018). The SO-CPR will yield increasingly valuable insights into both phenology and long-term change (McLeod et al., 2010; Takahashi et al., 2011; Mackey et al., 2012), and these various data sources need to be combined to provide the spatio-temporal coverage needed to examine climate change responses.
Resilience
The traditional view is that future warming rates will be too fast to allow genetic-level adaptation to respond in time (reviewed in Ji et al., 2010; Beaugrand and Kirby, 2018). However, this view has been challenged (Dam, 2013; Peijnenburg and Goetze, 2013), and there is debate over the extent to which species can adapt genetically in situ, adjust phenotypically (e.g., changes in body size, behavior) or otherwise compensate by changes in their phenology, or spatial distribution (Chivers et al., 2017). The importance of adaptation has been suggested (Dam, 2013) and in this context the observations of cryptic speciation in two major copepod species Oithona similis in the Southern Ocean is noteworthy (Cornils et al., 2017).
Efforts have been made to define the thermal niches of Antarctic copepods (McGinty et al., 2018) and then to project their distributions in a warmer environment based on the assumption of a fixed thermal niche (Mackey et al., 2012). However, Tarling et al. (2018) found that spatial distributions of copepods within the southwest Atlantic sector did not change, despite over 70 years of warming. This result therefore questions the common assumptions underlying range shift projections. Several candidate explanations for this resilience to distribution shifts need to be investigated. For example, one possibility is that the distributions of species are partially “anchored” by suitable food concentrations. These are related to fixed areas of elevated topography that promote iron fertilization, leading to locally elevated phytoplankton and thus copepod densities. Likewise, the marginal ice zone and sea ice is known to promote feeding conditions in both winter (Kohlbach et al., 2018) and by pre-conditioning effects after ice retreat (Schmidt et al., 2018). Again, this could help to weaken the direct link from temperature to copepod abundance. Another mechanism may be adaptation at a genetic level, or phenotypically, for instance with a reduction in body size (see above).
To understand how climatic drivers affect copepods, we need to study their effects in tandem, for example how food and temperature interact to driving copepod performance. This is achievable through egg production or somatic growth experiments on freshly collected copepods (Shreeve et al., 2002, 2005). Longer term incubations (involving realistic OA doses and acclimated copepods) have now shown that, in general, copepods appear to be fairly resilient to end of century projections of OA, but the degree of resilience to more local, but potentially acute effects such as increased glacial flour from glacial melt is poorly known (Garcia et al., 2019). Notwithstanding these uncertainties, the degree of resilience of copepods to climate change is central to understanding how food webs work and the higher predator species that may benefit, for example from a krill or a copepod-dominated food web.
Salps (Order Salpida)
Salps are gelatinous pelagic tunicates with individuals usually ranging between 2 and 4 cm long but reaching 16 cm in length in the Southern Ocean. Seven species are recorded south of the Subtropical Convergence, although only two (Salpa thompsoni and Ihlea racovitzai) are documented south of the APF (Foxton, 1961). Salps are major contributors to the total wet mass of three major metazoans (salps, copepods, euphausiids) and rank third in terms of dry or carbon mass after copepods and krill (Voronina, 1998). Salps are non-selective feeders, with a highly effective filter capable of capturing a wide range of prey sizes, although they are most suited to digesting small flagellates and, to some degree, diatoms (von Harbou et al., 2011; Pakhomov et al., 2019). As major grazers, salps are important mediators of vertical carbon flux (Pakhomov et al., 2002; Pakhomov, 2004; Bernard et al., 2012; Alcaraz et al., 2014). Despite their gelatinous nature, the perception that salps are a “trophic cul-de-sac” is changing, with increasing evidence of the importance of salps in the diets of various groups of Antarctic animals (Pakhomov et al., 2002; Gili et al., 2006; Jarman et al., 2013). Here we concentrate on Salpa thompsoni and Ihlea racovitzai.
Current Distribution
Salpa thompsoni is by far the most numerous and widely distributed Southern Ocean salp species, with the highest densities observed in the warmer mid-latitude waters of the Southern Ocean within the Antarctic Polar Frontal Zone (Foxton, 1966; Pakhomov et al., 2002). S. thompsoni is a flexible species able to tolerate a range of habitats, with potentially significant implications for the food web. While S. thompsoni can be sampled over a broad thermal range (−1.5 to 9°C), it primarily occurs in warmer waters of 2 to 5°C, possibly because cooler temperatures may reduce their reproductive fitness through failed embryo development (Henschke and Pakhomov, 2019). Ihlea racovitzai is a smaller, cold-water, much less studied counterpart of S. thompsoni only found in low numbers in the ice-covered regions closer to the Antarctic continent (Foxton, 1966).
Evidence of Past Change
Observations from 1926 to 2003 suggest that salps have undergone a general increase in densities and a circum-Antarctic southward expansion in their distribution (Pakhomov et al., 2002; Atkinson et al., 2004). Despite extreme patchiness in their distribution and large inter-annual variability in abundance, during recent decades, local salp populations have shifted significantly southward, intruding into areas generally dominated by Antarctic krill (Pakhomov et al., 2011; Steinberg et al., 2015; Henschke and Pakhomov, 2019). This was demonstrated by empirical observations in the Prydz Bay Region during the 1990s (Pakhomov, 2000). Similar trends were observed over four decades from the 1970s in the Scotia Sea and around the Antarctic Peninsula (Loeb et al., 1997; Steinberg et al., 2015). The historic increase in salps was also captured in the ecosystem models of Hoover et al. (2012) around Antarctic Peninsula.
Response to Drivers
Salps have a unique life cycle, with alternating sexual and asexual reproduction (Foxton, 1966). The 1-year conceptual model of the S. thompsoni life cycle described by Foxton (1966) has recently been challenged. Empirical observations of growth rates and modelling currently support a notion that S. thompsoni asexual forms could live for as long as 2 years (Loeb and Santora, 2012), although this species may complete its entire life cycle, i.e., from egg to egg, passing through both the sexual and asexual stages, in as little as 3 months (Pakhomov and Hunt, 2017; Henschke et al., 2018; Groeneveld et al., 2020). This allows salps under certain water temperatures and food concentrations to sustain localized rapid population increases (blooms), mostly through asexual reproduction (Pakhomov et al., 2002). Currently, salp responses to temperature, food composition and concentrations as well as other environmental parameters are not well understood (Pakhomov et al., 2002; von Harbou et al., 2011; Pakhomov and Hunt, 2017). The most recent modelling studies indicate that salp development is sensitive to food concentrations (Henschke et al., 2018; Groeneveld et al., 2020) and salps have a preference for picoplankton (Pakhomov et al., 2019). At the same time, S. thompsoni distribution strongly correlates negatively with the ice cover and positively with the warm water intrusions into high Antarctic regions (Pakhomov, 2000; Pakhomov et al., 2002, 2011; Rogers et al., 2020). Furthermore, following a reanalysis of multi-year data sets on salp biology, Henschke and Pakhomov (2019) postulated that temperature strongly drives S. thompsoni population dynamics.
Future Prognoses
Model-based projections (based on proposed changes in primary production regimes) indicate the potential for replacement of Antarctic krill by pelagic tunicates in the regions around the Antarctic Peninsula (Suprenand and Ainsworth, 2017). Recent observations (1970s to present) suggest a general increase in salp densities and a southward expansion in their distribution (Pakhomov et al., 2002; Atkinson et al., 2004). Antarctic krill and S. thompsoni habitats seem to show little overlap on thousands of km and meters to km scales, while both species often co-occur at mesoscales (10s to 100s km) (Loeb et al., 1997; Nicol et al., 2000b). The suggested interspecies interactions between krill and salps range from avoidance through to competition to predation, but these are not well understood (Le Fèvre et al., 1998; Pakhomov and Froneman, 2004). It seems that S. thompsoni cannot establish persistent populations in the high Antarctic and its occurrence at high latitudes is likely sustained by advection of warm waters (Pakhomov et al., 2011). This may change in a warmer Southern Ocean, although the consequences of higher salp numbers for the ecology of the Southern Ocean are hard to predict. An enhancement in the interactions between krill and salps, for instance, will have major effects on both the planktonic food web and the contributions of krill and salps to the carbon sink (Atkinson et al., 2012).
Salps are important mediators of the vertical carbon flux (Pakhomov et al., 2002; Pakhomov and Froneman, 2004; Alcaraz et al., 2014). Tunicates produce large, fast sinking (>1000 m day–1) and carbon-rich (up to 40% dry weight) fecal pellets, increasing the speed and efficiency of transfer of nutrients and carbon from surface waters (Bruland and Silver, 1981; Perissinotto and Pakhomov, 1998b). Even in areas where salps do not dominate plankton biomass (e.g., the Scotia Sea), their pellets contribute significantly to downward carbon flux (Manno et al., 2015). Furthermore, they perform migrations between the surface and 300–500 m during summer and reside at depths of 500–1000 m during winter, actively enhancing carbon flux into deep water (Manno et al., 2015). Therefore, the downward flux of carbon could significantly increase in the future due to an increase in salp biomass and range expansion (Ducklow et al., 2012; Rogers et al., 2020). However, the rate of fragmentation of salp fecal pellets through coprophagy may be as high as 80% in the upper water layer (Iversen et al., 2017), increasing uncertainty in the above predictions.
The importance of salps in Southern Ocean food webs and biogeochemical processes is now widely recognized and reflected in recent modelling studies. For the Antarctic Peninsula, the model of Hoover et al. (2012) recreated the historic decline in krill and increase in salps, while that of Suprenand and Ainsworth (2017) suggested a further decline in krill biomass, and an increase in salp biomass by 2050. With the current and expected future increase in salp biomass, their inclusion in biogeochemical models is becoming increasingly recognized as important to realistically parameterize the Southern Ocean carbon cycle (Henschke et al., 2016). Their contribution to iron recycling within the Southern Ocean has also been highlighted (Maldonado et al., 2016; Cabanes et al., 2017). A key limitation to further advancement of biogeochemical models is the paucity of data on physiological requirements and limitations, feeding preferences, and of biomass observations for model parameterization and validation (Le Quéré et al., 2016).
The response of salps to future climate change will have a variety of implications for predators, fisheries and biogeochemical cycling. To understand the impacts of change in these ecosystems there is a need to improve assessments of the distribution and abundance of salps in the Southern Ocean, salp interactions with other major metazoan grazers (particularly krill), and the factors (both abiotic and biotic) that drive their population dynamics
Resilience
Salp genetic studies are in their infancy but it appears that salps possess fast-evolving genes, potentially pointing to a faster turnover rate of the population and an enhanced selection for new life cycle traits (Jue et al., 2016; Batta-Lona et al., 2017; Goodall-Copestake, 2017, 2018). It may be hypothesized that salps have a higher capacity to adapt to abrupt shifts in the environment (Bucklin et al., 2018; Bitter et al., 2019). A combination of fast population turnover rates (Henschke et al., 2018; Groeneveld et al., 2020) and an ability to repeatedly produce chains of hundreds of genetically identical individuals during asexual reproduction would enhance chances of finding an environmental match, thus maintaining salp genetic diversity and resilience in the changing environment (Bucklin et al., 2018).
Pteropods (Order Pteropoda)
Pteropods are pelagic gastropod molluscs with a global distribution, represented by six species in the Southern Ocean. They can be divided into two orders: the shelled thecosomes (Thecosomata), comprising Limacina helicina antarctica, L. retroversa australis, Clio pyramidata, and C. piatkowskii, and the shell-less gymnosomes (Gymnosomata), comprising Spongiobranchaea australis and Clione limacina antarctica. Limacina spp. are mesozooplankton typically in the 1–5 mm size range, while Clio spp. and gymnosomes are macrozooplankton typically in the 10–20 mm size range. Time series show a mean pteropod contribution to zooplankton abundance of ∼ 11% in the vicinity of the Prince Edward Islands and South Georgia, 14% in the WAP, and <5% in the East Antarctic; however, their contribution can periodically exceed 85% (Hunt et al., 2008; Steinberg et al., 2015). Thecosomes can be highly abundant and are important trophically as grazers and prey. A detailed review on the ecological role of pteropods in the Southern Ocean can be found in Hunt et al. (2008). From a biogeochemical point of view, pteropods play an important role in the direct export of carbon and sequestration to the deep ocean through the sinking of dead individuals and fecal pellets (Manno et al., 2010, 2018). Their calcification also contributes to the carbonate counter pump which releases CO2 back into surface waters (Manno et al., 2018). Thecosome pteropods produce shells from aragonite, a metastable and relatively soluble form of biogenic calcium carbonate (CaCO3) (Mucci, 1983) and are regarded as sensitive indicators of OA (Fabry et al., 2008; Bednaršek et al., 2017a; Manno et al., 2017). This is particularly relevant in the polar regions where ocean uptake of anthropogenic CO2 and the undersaturation of carbonate ions resulting from OA is progressing most rapidly (Orr et al., 2005).
Current Distribution
Pteropods occur throughout Antarctic and sub-Antarctic waters but species composition differs north and south of the APF reflecting distinct temperature tolerances (van der Spoel et al., 1999; Hunt et al., 2008; McLeod et al., 2010). Globally, the highest average pteropod biomass is estimated to occur within the 70–80°S latitudinal band of the Southern Ocean (39.71 ± 93 mg C m–3 Bednaršek et al., 2012a). Hot spots of pteropod occurrence in the Southern Ocean include in the vicinity of islands and the Ross Sea. Densities exceeding 1000 ind.m–3 have been recorded from around South Georgia, the APF, and in the Ross Sea (Pane et al., 2004; Hunt et al., 2008). All Southern Ocean pteropod species are predominantly epipelagic (Hunt et al., 2008; Akiha et al., 2017) with the exception of C. piatkowskii, which is mesopelagic (van der Spoel et al., 1999). Both diel and seasonal migration occurs, with most species occurring deeper in the water column (to 1000 m) during winter (Hunt et al., 2008; Flores et al., 2014).
Evidence of Past Change
The longest published pteropod time series is from the Palmer Long Term Ecological Research (PLTER) area off the WAP (Steinberg et al., 2015). Summer surveys have been conducted continuously since 1993, in the form of a north-south grid that spans the coast, shelf and continental slope. Over the time series, pteropod abundance has shown large interannual variability and while gymnosome abundance increased up to 2017, thecosomes have shown no long-term trend throughout the WAP (Thibodeau et al., 2019). Sub-regionally, L. h. antarctica abundance has increased in the slope region, while C. pyramidata abundance has increased in the south. The overall pattern is dominated by an alternation between high and low abundance that is negatively correlated with the Multivariate ENSO Index, and positively correlated with SST, sea ice, and primary production (Steinberg et al., 2015). Gymnosome abundance tracks thecosome abundance, reflecting their trophic dependency. Similar trends in pteropod abundance were observed by the U.S. AMLR Program time series in the northern Antarctic Peninsula region (Loeb and Santora, 2013). The other significant pteropod time series comes from the SO-CPR surveys (1997 to present), which has mainly focused on the Pacific sectors thus far (McLeod et al., 2010). Although a detailed analysis of the time series has yet to be conducted, the combined data have been used to model the abundance of major groups with respect to chlorophyll-a concentration, net primary productivity, SST, mixed layer depth, sea ice and the spatial gradient of SST (Pinkerton et al., 2020). Based on the relationship between pteropod abundance and these environmental parameters, that study suggested a significant increase in environmental suitability for pteropods in many areas south of the APF in the last 20 years, but a significant decrease over the Ross Sea Shelf (see also Section “Past Changes in Zooplankton: A Modelled Example Using Continuous Plankton Recorder Data”). A caveat with these model results is that they do not include a representation of OA or oxygenation state.
Response to Drivers
Pteropod abundance is strongly positively correlated with primary production (chlorphyll-a biomass) (Seibel and Dierssen, 2003; Hunt et al., 2008; Steinberg et al., 2015; Pinkerton et al., 2020), and environmental forcing of primary production is therefore expected to be an important driver of pteropod change. Global time series indicate that, to date, temperature and productivity have been the dominant drivers of change in pteropod species composition and abundance (Mackas and Galbraith, 2012; Beaugrand et al., 2013). The vulnerability of thecosome pteropods to OA, specifically aragonite saturation state (Ωar, for which 1 is generally considered the vulnerability threshold), makes this another potentially important driver of change (Comeau et al., 2010; Bednaršek et al., 2012a; Lischka and Riebesell, 2012; Bednaršek et al., 2014; Thabet et al., 2015; Manno et al., 2017; Mekkes et al., 2021). The magnitude of their sub-lethal and lethal responses to OA depends on life-stage, with larvae and juveniles being substantially more affected by Ωar than adults (Bednaršek et al., 2019). Maternal and embryonic OA stress was demonstrated to reduce the percentage of L. h. antarctica eggs successfully reaching the organogenesis stage by 80% (Manno et al., 2016). Numerous studies have demonstrated synergistic effects, leading to outcomes that are nonlinear and sometimes severe (Bednaršek et al., 2016). Experiments combining OA with warming, deoxygenation, freshening or increased stratification have demonstrated synergistic effects among drivers of change leading to increased mortality (Lischka et al., 2011), reduced calcification (Comeau et al., 2010), shell degradation and malformation (Lischka and Riebesell, 2012; Gardner et al., 2018), altered metabolic rates (Maas et al., 2011, 2016) and changes to swimming performance and behavior (Manno et al., 2012; Bednaršek and Ohman, 2015). Further, in situ observations along the California Current System, have demonstrated the negative impact of OA in combination with warming and deoxygenation on pteropod population (Bednaršek et al., 2018). The majority of these studies were not carried out on Southern Ocean species, and it has been shown that responses in the same, or closely related, species can vary between environments (Maas et al., 2016). Nevertheless, some Antarctic studies have been performed. Gardner et al. (2018) found that early life stages of L. h. antarctica in the Scotia Sea exhibited shell malformation and dissolution when exposed to warming and OA, with mortality reaching up to 39%. Meanwhile, Hoshijima et al. (2017) found that metabolic rates increased at low pH and that this increase was amplified at higher temperatures in the same species in the Ross Sea.
Future Prognoses
Southern Ocean warming is expected to result in a change in pteropod species distribution, specifically a southward shift in sub-Antarctic taxa (Gardner et al., 2018). Evidence also suggests that ongoing warming, sea ice loss, and increased productivity will favor increased pteropod abundance (Seibel and Dierssen, 2003; Steinberg et al., 2015). Projections for future Southern Ocean productivity are spatially variable, with productivity forecast to decrease in the sub-Antarctic and increase toward the Antarctic continental shelf (Deppeler and Davidson, 2017; Pinkerton et al., 2021). While productivity may favor pteropods in southern regions, they may be increasingly negatively impacted by OA (Manno et al., 2007; Bednaršek et al., 2012a). Southern Ocean surface waters are expected to start becoming undersaturated with respect to aragonite as early as 2030 (McNeil and Matear, 2008), affecting ∼ 30% of surface waters by 2060 and >70% by 2100 (Hauri et al., 2016). Aragonite undersaturation will affect southern waters first, progressively moving northwards. Furthermore, both shell dissolution and survival in aragonite undersaturated conditions may be exacerbated by concurrent warming (Bednaršek et al., 2019). The development of predictive models of pteropods responses to environmental change requires further research into the effect of stressors, particularly the synergistic effects of multiple drivers. Future research will benefit from the application of new molecular tools, e.g., transcriptomics, in measuring the physiological response of pteropods to stressors (Johnson and Hofmann, 2016; Johnson et al., 2019).
Resilience
While a number of studies have documented the effect of OA on pteropod physiology and shell condition, the importance of carbonate chemistry as a control on pteropod populations in the Southern Ocean has yet to be demonstrated. No relationship was found between Ωar and pteropod abundance in the WAP time series (Thibodeau et al., 2019). The fact that Ωar has yet to become undersaturated across most Southern Ocean surface waters may explain the lack of any population relationship with carbonate chemistry. However, it should be noted that Southern Ocean pteropods do already experience Ωar undersaturated conditions on a seasonal basis through winter occupancy of Ωar depleted waters (Hunt et al., 2008; Jiang et al., 2015; Manno et al., 2016). Arctic L. helicina experience greater exposure to aragonite undersaturated conditions, with Ωar <1 occurring in surface waters of the Beaufort Sea (Yamamoto-Kawai et al., 2009) and Amundson Gulf (Niemi et al., 2021). In situ studies of the Arctic L. helicina showed that the periostracum of this species was an effective barrier to exposure to Ωar undersaturated waters (over 4 days of incubation), and that dissolution to the inner shell only occurred when this was breached by previous physical damage (Peck et al., 2016a,b). However, other studies (Bednaršek et al., 2016, 2021; León et al., 2019; Niemi et al., 2021) do not agree that dissolution only occurred at the time of the periostracum breach. Furthermore, Peck et al. (2018) revealed that, despite losing the entire thickness of the original shell in localized areas, L. helicina can maintain shell integrity through thickening the inner shell wall. Nevertheless, this resilience is likely to come at a metabolic cost, which such organisms are unlikely to maintain over the longer term. In fact Niemi et al. (2021) found limited evidence for shell repair in the Amundson Gulf even when food was abundant, while Bednaršek et al. (2021) found reduced shell thickness as evidence of exposure.
Past Changes in Zooplankton: A Modelled Example Using Continuous Plankton Recorder Data
The SO-CPR survey was established in 1991 to map the spatial and temporal patterns of zooplankton through the Southern Ocean (Hosie et al., 2003). The CPR is towed behind a ship and collects samples of zooplankton from ∼10 m depth, which are preserved in formalin and subsequently morphologically identified in the laboratory. SO-CPR surveys continue to be carried out (Takahashi et al., 2021). At various stages this dataset has been analyzed to help understand spatial distributions and variation/change in zooplankton over time (e.g., Hunt and Hosie, 2005, 2006; McLeod et al., 2010; Pinkerton et al., 2010, 2020; Takahashi et al., 2011, 2021; Meilland et al., 2016). Pinkerton et al. (2020) combined SO-CPR data and oceanographic conditions (namely: chlorophyll-a concentration, net primary productivity, SST, mixed layer depth, sea ice concentration, and the spatial gradient of SST as an indicator of ocean fronts) to understand long-term trends in the environmental suitability for broad groups of zooplankton in the Southern Ocean. Using the relationship between zooplankton and environmental data collected by the CPR tows, the authors used statistical models to extrapolate information on the environmental suitability of zooplankton to unsampled areas. “Environmental suitability” was defined as the expected abundance of a species or group of zooplankton at a given location and time based on environmental properties and used as a measure of ecological niche.
Six key taxonomic groups of zooplankton were considered by Pinkerton et al. (2020)1 : Copepoda (Calanoida), Euphausiidae (numerically dominated in SO-CPR data by Thysanoessa macrura, 64%; E. superba comprised only 9%), Oithona similis, and pteropods, Foraminifera and Fritillaria spp. Salps were rarely identified in the CPR samples. Analysis using Boosted Regression Tree (BRT) models provided insights into the patterns of and changes in environmental suitabilities for these broad zooplankton groups over the period 1997–2018. Analysis was also carried out for all these groups combined (total abundance). See Pinkerton et al. (2020) for a spatial plot of CPR samples, which are predominantly from East Antarctica and the Ross Sea region.
The modelling of Pinkerton et al. (2020) suggested higher environmental suitability for all zooplankton between the SAF and the Southern limit of the ACC and lower suitability to the north and south. Lower abundances of euphausiids were predicted south of the APF and higher abundances of copepods were predicted between the APF and the SAF. Modelled environmental suitability for pteropods was highest over the Ross Sea shelf.
For the key groups presented in this study (i.e., Euphausiidae, Copepoda (Calanoida and Oithona similis), and pteropods) we replotted the absolute-values of mean and long-term linear trends in environmental suitability from Pinkerton et al. (2020) (Supplementary Figures 1,2). Based on data from Pinkerton et al. (2020), time series of change in environmental suitability for total abundance over the past two decades were calculated for MEASO Sectors, Zones and Areas [Figures 6 and 7 (Sectors, Zones) and Supplementary Figure 3 (Areas)]. Based on Pinkerton et al. (2020), the highest increase in modelled environmental suitability for zooplankton (combined) across all Zones and Sectors was in the MEASO sea ice zone (+2.1% per year on average). Our analysis also showed that changes environmental suitability for zooplankton total abundance were higher in the Atlantic Sector (1.1% per year) and Central Indian Sector (0.8% per year) than in the other MEASO Sectors, and statistically significant (p <0.05). For comparison, at the scale of the Southern Ocean, Pinkerton et al. (2020) reported that the average annual trend in environmental suitability for total zooplankton abundance over the same period was just 0.6%. Pinkerton et al. (2020) showed that changes in environmental suitability for zooplankton total abundance were predominantly driven by improving conditions for copepods (cyclopoid and calanoid trends of 0.6% per year on average), foraminifera (0.85% per year) and Fritillaria (0.8% per year); for euphausiids, the average annual trend was only 0.2%, and for pteropods there was no significant trend in modelled environmental suitability at the scale of the Southern Ocean.
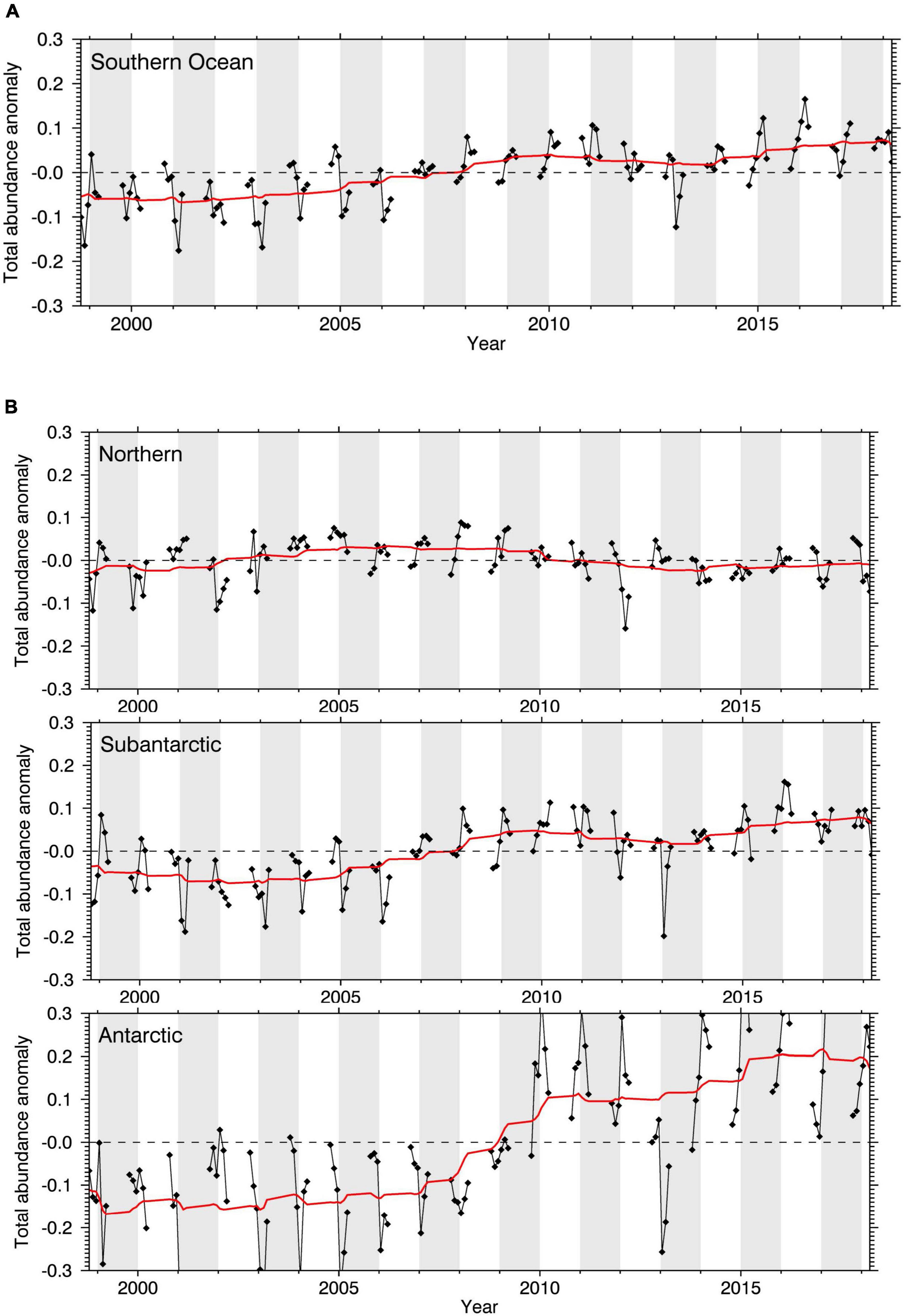
Figure 6. Time series of change in environmental suitability for total zooplankton abundance, i.e., Euphausiidae, Copepoda (Calanoida and Oithona similis), and pteropods, from the combined Boosted Regression Tree model of Pinkerton et al. (2020) for the Southern Ocean Marine Ecosystem Assessment for the Southern Ocean (MEASO) (A) study area, (B) Sectors. Time Series change for each individual MEASO Zones and Area are given in Figure 7 and Supplementary Figure 3, respectively. The environmental suitability anomaly (difference from the monthly climatological value) is shown for each month over period 1998–2018. Gray shading indicates calendar years. Black points and lines are monthly anomalies (differences from long-term monthly means). Red lines are smoothed changes (4-year running median).
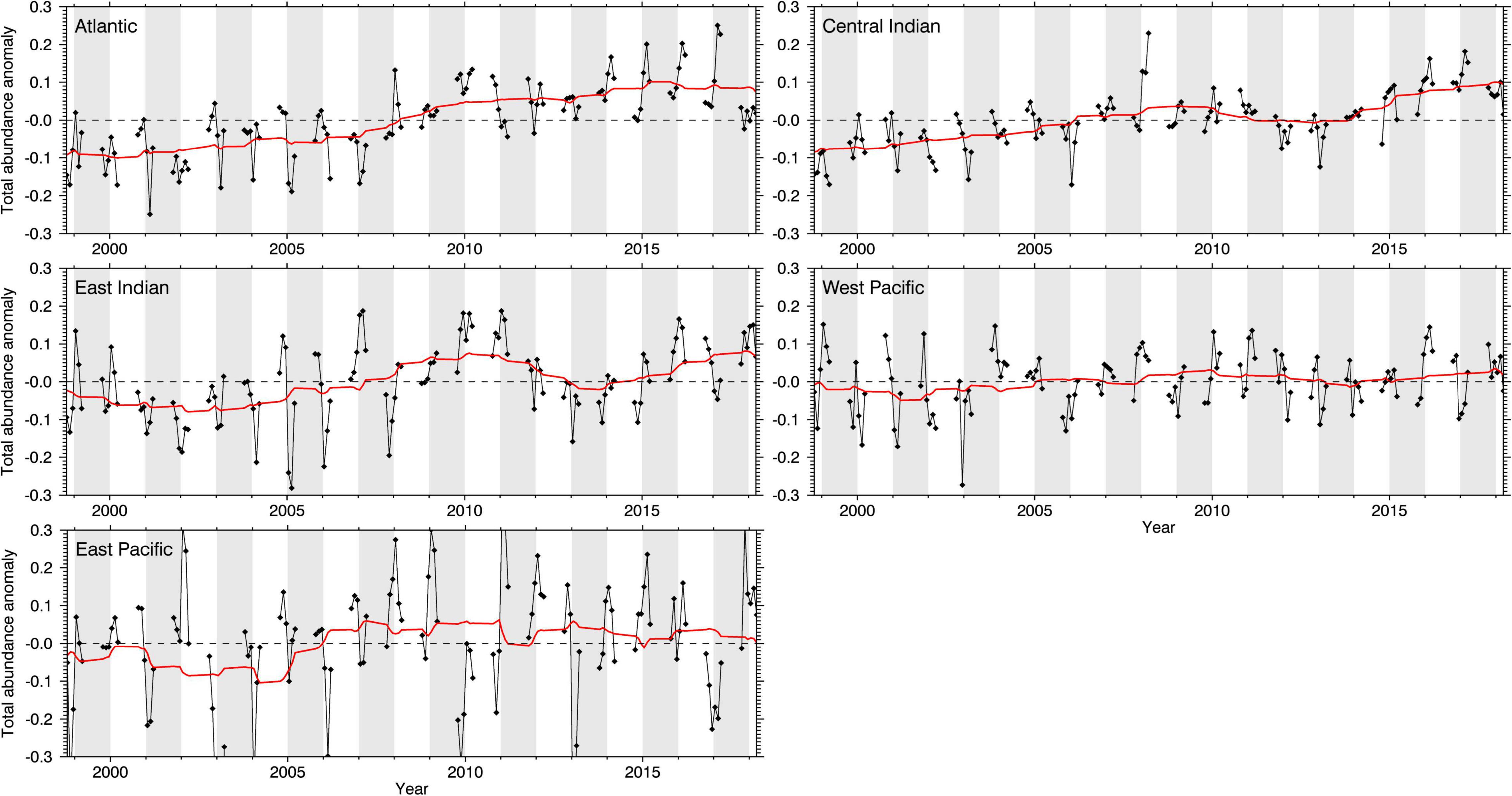
Figure 7. Time series of change in environmental suitability for total zooplankton abundance, i.e., Euphausiidae, Copepoda (Calanoida and Oithona similis), and pteropods, from the combined Boosted Regression Tree model of Pinkerton et al. (2020) for Southern Ocean MEASO Zones. Time Series change for each individual MEASO Area are given in Supplementary Figure 3. The environmental suitability anomaly (difference from the monthly climatological value) is shown for each month over period 1998–2018. Gray shading indicates calendar years. Black points and lines are monthly anomalies (differences from long-term monthly means). Red lines are smoothed changes (4-year running median).
Although environmental suitability modelling such as Pinkerton et al. (2020) can provide information on changes to amenable conditions for zooplankton, the analysis should be considered hypothetical in that it is not certain that zooplankton abundances actually change in response to these oceanographic drivers. In other words, ecological niches may expand, but populations size may not respond proportionately. Some evidence for this resilience of distribution was provided in the Atlantic sector, where Tarling et al. (2018) found that distribution centers of copepods had not shifted south over the last century to keep track with the warming thermal regime. Another caveat is that environmental conditions used to define the zooplankton habitat niche are necessarily limited to those for which information are available, and these are predominantly physical in nature. Unobserved ecological feedbacks can thus be missed. This, and small-scale issues, are reasons why only approximately half (at best) of observed variability in zooplankton abundances can be described by environmental-statistical models. Better understanding of the life histories and environmental and ecological dependencies of Southern Ocean zooplankton are as crucial as ever.
Additionally, broad groupings of zooplankton were used in Pinkerton et al. (2020) to avoid issues arising from difficulties in identifying some developmental stages of some species in CPR data. In particular, euphausiids are fragile with respect to the CPR collection process making their identification challenging. Genetic and image-analysis methods are under development for CPR data to address these identification issues (Pinkerton, unpublished data).
Summaries and Assessments
A summary of the current role of these zooplankton taxa in the Southern Ocean, based on the information detailed in Sections Euphausiids (Family Euphausiidae), Copepods (Subclass Copepoda), Salps (Order Salpida), and Pteropods (Order Pteropoda) is presented in Supplementary Table 1. A summary of environmental tolerances of these taxa (based on recent empirical studies) are also presented in Supplementary Table 2.
An assessment of the influence of anticipated changes in key physical, chemical, and ecological drivers on the distribution and abundance of these taxa is summarized in Tables 1, 2. This assessment represents the authors’ (unweighted) expert consensus based on current understanding and information detailed in Sections Euphausiids (Family Euphausiidae), Copepods (Subclass Copepoda), Salps (Order Salpida), Pteropods (Order Pteropoda) and Past Changes in Zooplankton: A modelled example using Continuous Plankton Recorder Data (including references cited therein). A qualitative assessment of the potential future changes in zooplankton in response to these drivers and their potential capacity for resilience is presented in Supplementary Table 3 as a series of statements. This also represents the authors’ (unweighted) expert consensus based on current understanding and information detailed in the Sections listed above (including references cited therein).
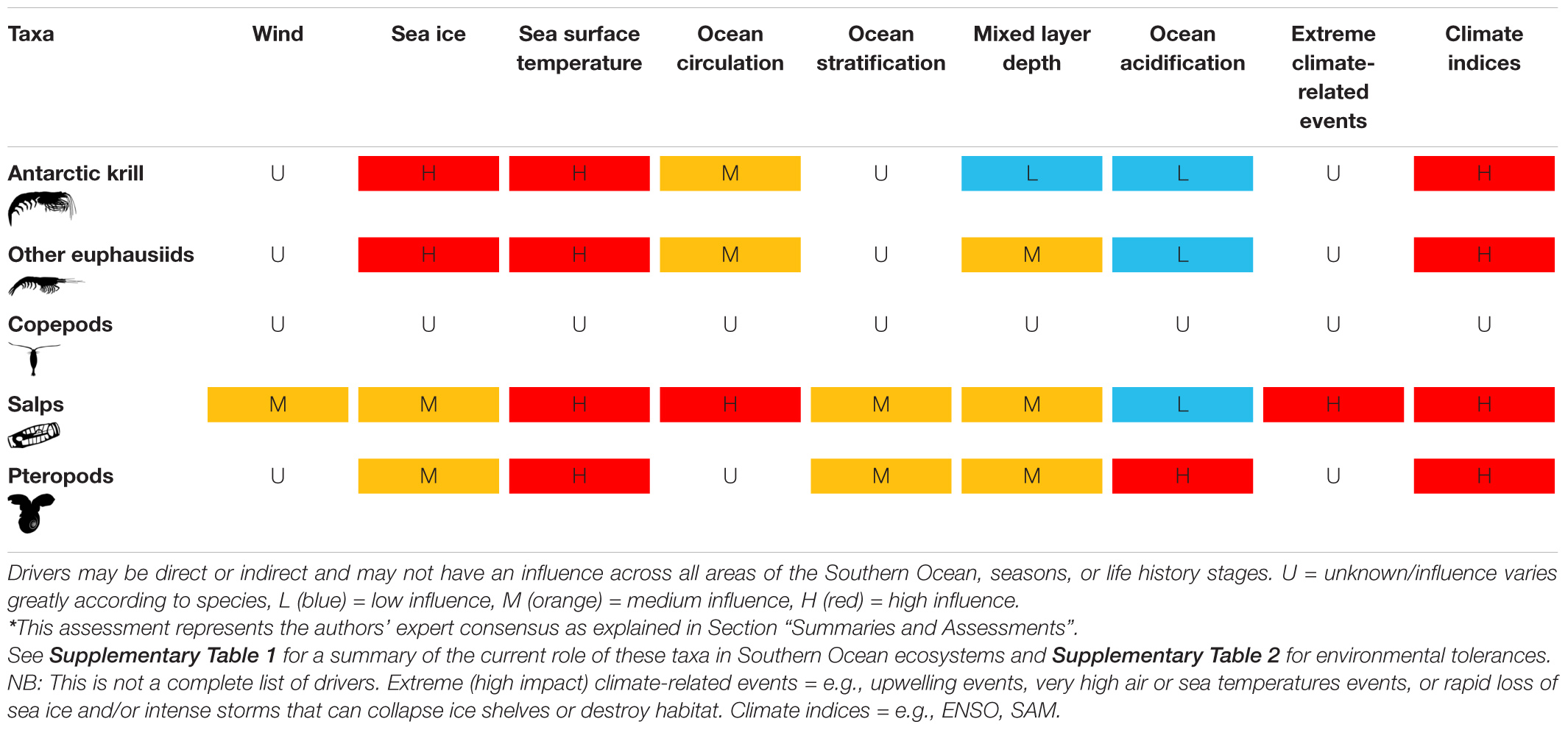
Table 1. Assessment* of the potential impacts of key physical and chemical drivers of change on the distribution and abundance of key Southern Ocean zooplankton taxa (based on information provided in Sections “Euphausiids (Family Euphausiidae), Copepods (Subclass Copepoda), Salps (Order Salpida), Pteropods (Order Pteropoda), and Past Changes in Zooplankton: A Modelled Example Using Continuous Plankton Recorder Data”, including references cited therein). See Supplementary Table 3 for a more detailed assessment of the potential future changes in these taxa (in response to some of these drivers) and their potential capacity for resilience.
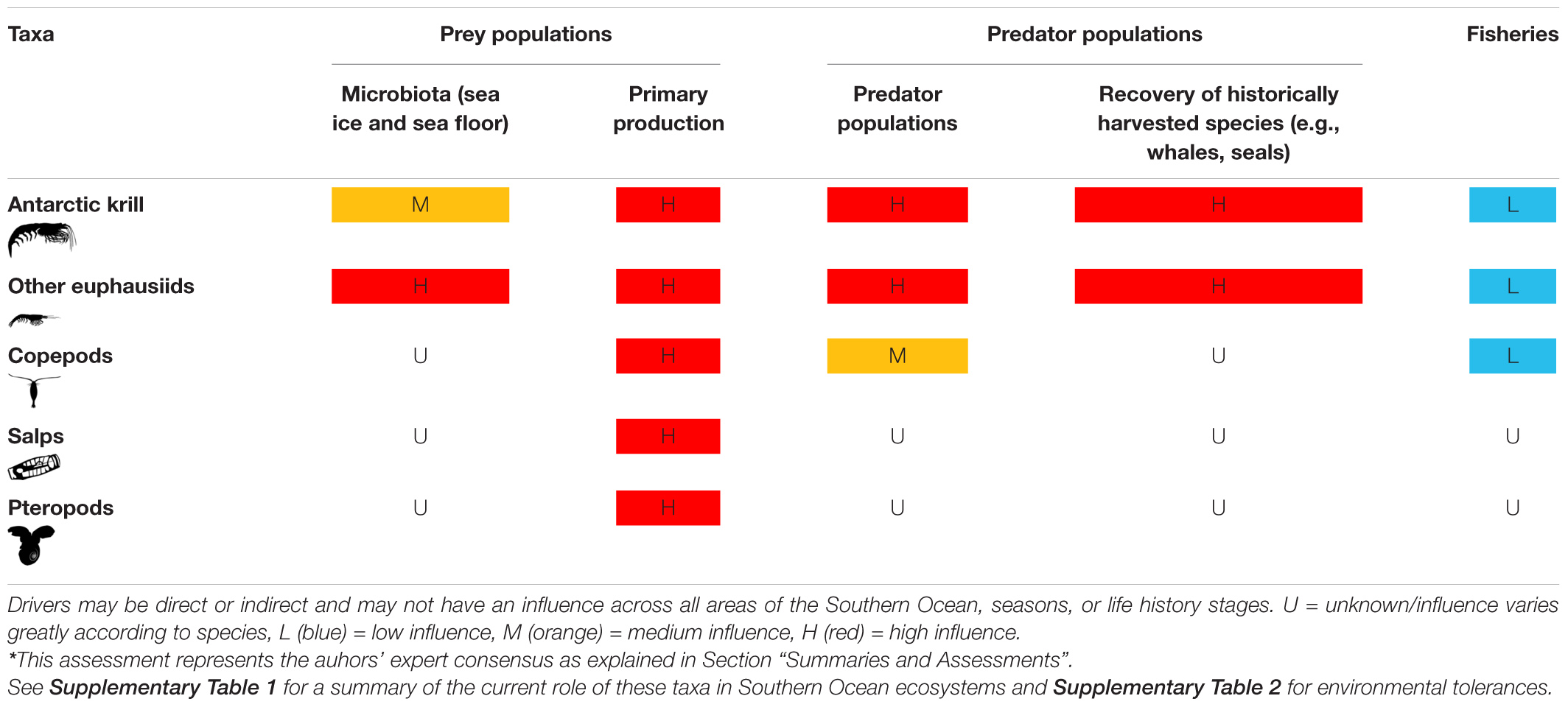
Table 2. Assessment* of the potential impacts of key ecological drivers of change on the distribution and abundance of key Southern Ocean zooplankton taxa (based in information in Sections “Euphausiids (Family Euphausiidae), Copepods (Subclass Copepoda), Salps (Order Salpida), Pteropods (Order Pteropoda), and Past Changes in Zooplankton: A Modelled Example Using Continuous Plankton Recorder Data”, including references cited therein). See Supplementary Table 3 for a more detailed assessment of the potential future changes in these taxa (in response to some of these drivers) and their potential capacity for resilience.
Concluding Comments
Understanding the processes by which key global and local drivers of change impact the habitat features, biodiversity, structure, functioning and variability of marine ecosystems across a range of spatial, temporal, and organizational scales is crucial to developing conservation and sustainable management strategies that can anticipate and respond to global change impacts. Zooplankton form an integral part of Southern Ocean marine ecosystems which are extensively connected to the global ocean (Murphy et al., 2021). Their inclusion in regional and circumpolar assessments is therefore vital to understanding present and future change in this globally important ocean and the ramifications for Earth System functioning (Murphy et al., 2008; Hofmann, 2016). Although a number of studies have recently assessed the potential future responses of these marine ecosystems to a changing Southern Ocean, few have provided detailed syntheses on the expected changes in zooplankton (Mackey et al., 2012; Hunt et al., 2016; Chown and Brooks, 2019; Siegert et al., 2019; Rogers et al., 2020).
This overview provides a synthesis of the current understanding of four key metazoan zooplankton taxa, euphausiids, copepods, salps and pteropods, which are important members of zooplankton communities and food webs across the Southern Ocean. It illustrates the range of life history strategies, habitat, and environmental associations and observed and modelled changes in their distribution and abundance across the circumpolar ocean. It has also highlighted their varied responses to physical, chemical, and ecological drivers and some of the mechanisms involved. Moreover, it has emphasized that despite the extensive research on Southern Ocean zooplankton (dating back to the 1872-6 HMS Challenger expedition Hosie et al., 2000), and growing awareness of the role of these taxa in modulating ecosystem structure and function, there are major gaps in our current understanding of their physiology, life history strategies and processes, population dynamics, distribution and abundance, food web and competitive interactions across a range of spatial and temporal scales. There are also gaps in our understanding of their roles in biogeochemical cycling and roles underpinning fisheries and wildlife tourism. Many of the limitations and uncertainties in this understanding (across a range of spatial, temporal, and organizational scales) can be attributed to the status of field, process and modelling studies of these taxa, including insights into their capacity for biological resilience. These reflect the difficulties in studying these taxa under laboratory conditions and sampling and observing them in situ across a range of spatio-temporal scales, particularly over large parts of the open ocean, within the sea ice zone, at depth, and during seasonal transitions and the winter months. There is also a paucity of multidecadal biological time series of sufficient duration to quantify the variability and rates of changes of relevant oceanic variables and zooplankton species or be definitive about the causal mechanisms (O’Brien et al., 2017; Cornils et al., 2018). Furthermore, the Southern Ocean is characterized by high natural spatial and temporal variability and has experienced ecosystem-level changes through the historical exploitation of marine living resources (Clarke et al., 2012). Observed changes in zooplankton are therefore currently difficult to attribute to specific climate or ecological processes.
Future Prognoses
Many current scenarios of future changes in key physical, chemical, and ecological drivers that are relevant to zooplankton distribution and abundance are highly uncertain. This can be attributed in part to the current suite of projections of physical and chemical drivers (based on current global climate models – i.e., CMIP5, available at the time of writing) that are poorly constrained and highly uncertain. These models are either not at the appropriate spatial or temporal scales or do not resolve key processes (e.g., primary production, sea ice and oceanic processes- except for the position of the APF, see Morley et al., 2020) to enable the interpretation of biological change in the Southern Ocean (Murphy et al., 2018). In addition, scenarios of other key local drivers (e.g., fisheries, predator impacts, whale recovery, tourism, pollution, and invasive species) also remain uncertain (see also Grant et al., 2021). The limitations and uncertainties surrounding the fundamental understanding of these taxa (as discussed above) together with the status of these scenarios, means that there is insufficient data to provide comprehensive prognoses for future changes in zooplankton with definitive levels of confidence (sensu Intergovernmental Panel on Climate Change, IPCC, e.g., Mastrandrea et al., 2010) either within each of the MEASO areas or at specific time and space scales relevant to the needs of conservation and management (see Box 1). Future prognoses with attached levels of confidence have, however, been made for Southern Ocean zooplankton within the IPCC’s Working Group 2 reports (e.g., IPCC, 2014) and Special Report on the Ocean and Cryosphere in a Changing Climate (IPCC, 2019). McBride et al. (2021) have considered the impacts of climate-driven environmental change on Antarctic krill in the context of CCAMLR fisheries management. The potential impacts of change on Antarctic krill within CCAMLR statistical Area 48 have also been considered by Murphy et al. (2018) and serve as a model for other species and regions (see also future work below). The future prognoses presented here represent the authors’ consensus view based on expert knowledge and the best available published evidence. Indications are that the response of these zooplankton to future change will be complex and vary across both life history stages and scales of space, time, and ecological organization, against a backdrop of natural variability. Their response to multiple drivers (including their variability over space and time and their additive, synergistic, or antagonistic effects) and their potential for biological resilience (Murphy et al., 2016; Gruber et al., 2021) will further add to this complexity but remain largely unexplored.
BOX 1. Research gaps and priorities: Current limitations to projections of future zooplankton change.
To develop robust future projections of changes in the distribution and abundance of zooplankton communities within the MEASO assessment areas requires a number of elements. These include (1) scenarios of key physical, chemical and ecological drivers within a given assessment area (based on Representative Concentration Pathway emissions scenarios where relevant), (2) mechanistic understanding of the impacts of key drivers on zooplankton within a given assessment area, and (3) development of zooplankton (and ecosystem) models that can be coupled with scenarios of key drivers to project qualitative or quantitative changes in zooplankton populations or communities within a given area. Development of these elements, together with clarification of the uncertainties and caveats involved, will facilitate conservation and management options for Southern Ocean ecosystems. Key limitations currently include:
Fundamental understanding of zooplankton ecology over a range of spatio-temporal and organizational scales (from local to circumpolar, seasonal to multi-decadal, and species to ecosystems) and the mechanistic processes involved. These limitations reflect the difficulties in studying zooplankton in the laboratory and the field, and the lack of time series data essential for detecting long-term trends in key areas, e.g.,:
•Physiological (energy and nutrient demands, tolerance to environmental stressors, such as temperature, starvation, oxygen, and pH− see also drivers below), morphological and behavioral traits.
•Growth, development and reproduction, feeding and dietary preferences, food quality.
•Life histories, traits, and strategies (including behaviors during life history events such as foraging and reproductive strategies, migrations, seasonal development and overwintering, competitive/anti-predator behaviors) and phenology.
•Phenotypic plasticity.
•Role in food webs (trophic ecology including modes of feeding, strength and direction of predator-prey interactions, ingestion and egestion rates, energy transfer, top-down and bottom-up controls, competition), particularly during winter when phytoplankton production in water column and sea ice is limited.
•Role in biogeochemical cycling (i.e., particle export and sequestration of organic material and the chemical cycling of nutrients).
•Distribution (horizontal and vertical), abundance/biomass and production estimates, spatial population connectivity, demography and community composition.
•Hotspot locations for key ecological processes (e.g., spawning, larval development and recruitment, overwintering, migrations, feeding, population concentrations, connectivity and advection routes, biogeochemical cycling).
•Natural variability in zooplankton dynamics (across seasonal to multi-decadal timescales).
•Changes in zooplankton dynamics that represent deviations from assumed natural variability.
•Key natural environmental and anthropogenic drivers of zooplankton dynamics, variability and change. Mechanistic understanding of their impacts, including additive/synergistic/antagonistic effects of multiple drivers, e.g.,:
- Sea ice dynamics, ocean warming, stratification, circulation, acidification, and climate oscillations.
- Prey (e.g., phytoplankton biomass, species assemblages) and predator dynamics (e.g., predator biomass, species assemblages, recovery of cetaceans).
- Current fisheries (including their impacts on different life cycle stages).
- Other possible drivers (e.g., pollution, tourism, invasive species).
•Resilience of zooplankton to multiple drivers of change (including additive, synergistic, or antagonistic effects).
Ecosystem models that can enhance understanding of zooplankton dynamics, variability and change, ecosystem structure and function, and inform regional and global assessments, conservation, and management decisions. There is a paucity of models underpinned by (a) to conceptualize and quantify ecological processes relevant to zooplankton over a range of spatio-temporal and organizational scales, e.g.,:
•Individual species models (e.g., life history models representing life history traits and rates, life history strategies, physiological, morphological, and behavioral traits, biogeochemical cycling and simulating the effects of drivers on key life history stages and processes, i.e., life history models embedded in full 3D ocean physics models that include sea ice).
•Food web models (e.g., representing regional and temporal variations in zooplankton community composition, trophic links and alternative energy pathways to higher trophic levels).
•Biogeochemical models (e.g., representing the role of different zooplankton species).
•End-to-end ecosystem models that integrate physical, biogeochemical and biological processes across multiple species, trophic levels, and spatio-temporal scales.
Comprehensive standardized future scenarios of key physical, chemical, biological, and ecological drivers including:
•Scenarios for sea ice, ocean warming, stratification, circulation and acidification and phytoplankton change. The current suite of global climate model projections available [i.e., CMIP(5) at the time of writing] to develop scenarios of zooplankton drivers under different emission pathways are poorly constrained and highly uncertain. They are too low in spatial resolution (>100 km) to resolve many key processes relevant to these taxa (e.g., related to sea ice, ice shelves, upwelling, cross-shelf exchange, connectivity, and retention), which often occur over smaller spatial scales. Differentiating between patterns of natural internal variability versus global anthropogenic change in these drivers is also complex. Local modes of atmospheric variability (e.g., Southern Annular Mode, Amundsen Sea Low, El Niño events) and potential extreme climate-related events may further influence ocean, biogeochemical and sea ice processes.
•Scenarios for predator and prey dynamics, recovery of cetacean populations, and fisheries. Aside from those relevant to Antarctic krill within the Scotia Sea ecosystem, there are few available scenarios of changes in these ecological drivers.
Coupled physical, chemical, and biological models that can be forced by future scenarios of key drivers to generate qualitative and quantitative projections of future changes in zooplankton species, communities, and ecosystems across a range of spatio-temporal scales.
Population Level Changes
Based on our current understanding of the key zooplankton taxa, observed changes and the mechanisms involved, anticipated changes in sea ice, ocean warming, ocean circulation, mixed layer depth, primary production, and predators were considered here as important direct and indirect drivers of future change in these biotas. Whilst (the anticipated direction of) many of these drivers are expected to have an overall negative effect (e.g., decreased egg production rates, growth, food availability/suitability, feeding efficiency, availability of suitable habitat for spawning, reproduction and overwintering, population size and abundance, range contraction, and competition) on some species (e.g., E. superba, E. crystallorophias), other species may experience an overall positive effect. For example, increased growth rates, feeding efficiency, habitat availability, population size and abundance, and range expansion of T. macrura, salps, and pteropods. A potential positive feedback loop, generated by the recovery of whale populations in the short term (see McBride et al., 2021), on phytoplankton productivity may also result in increased abundance of E. superba, E. crystallorophias, and T. macrura (Schmidt et al., 2011; Ratnarajah et al., 2014). For some species and localities, however, the positive effects of some drivers may be offset by the negative effects of other drivers – for example, enhanced feeding efficiency of E. crystallorophias in response to increased diatoms in phytoplankton communities may not be sufficient to counteract the impacts of sea ice loss on spawning habitat and reproductive success. Ocean acidification is expected to have negative consequences for the thecosome pteropods, starting in the higher latitudes first. It must be stressed however, that these drivers may not have an influence across all life stages of the taxa considered, or across all areas of their distribution. Competitive interactions brought about by driver impacts will also be important, but the nature of many of these are unknown. We also only considered the potential impacts of each driver in isolation and recognize that the additive, synergistic, or antagonistic impacts of multiple drivers on these taxa will be important and warrants further attention. Although we did not assess the impacts of some other global drivers, such as ocean deoxygenation, increased ultraviolet radiation, or recovery of the ozone hole over Antarctica and the Southern Ocean, or local drivers, such as pollution (e.g., microplastics), invasive species, parasites, or pathogens (see Grant et al., 2021), existing and emerging research suggests that these may also elicit future changes in zooplankton (Flores et al., 2012a; Rowlands et al., 2021). All of these will have important implications for the dynamics of zooplankton population dynamics and their spatial connectivity.
Community Level Changes
Although not considered explicitly in this assessment, predicting changes in zooplankton community dynamics will be key to predicting the resultant effects on regional food webs and ecosystem structure and functioning. As we have noted, the range of zooplankton communities that occupy different regions of the Southern Ocean are often represented by changes in the balance of taxa or in the abundance of species as opposed to fundamentally different faunas (see Murphy et al., 2021). Collectively, these communities support an array of biodiversity and energy pathways that enable the maintenance of ecosystem structure and functioning (Murphy et al., 2012a,2016; Barnes and Pálinkó, 2017; Trebilco et al., 2020; McCormack et al., 2021). These include the classic short, efficient energy pathway from phytoplankton through to baleen whales via Antarctic krill. This was long viewed as the dominant food chain in the Antarctic marine ecosystem and in some areas, such as the southwest Atlantic sector, sustains large populations of higher trophic level predators (Clarke, 1985; Hill et al., 2006; Murphy et al., 2007a,2012a; Siegel, 2016; Saunders et al., 2019). There are, however, alternative pathways that transfer energy to higher trophic levels and sustain other predators less dependent on krill including a range of seals, sea birds, icefish, toothfish and toothed whales. These pathways may be dominated for example, by copepods (Murphy et al., 2012a) or salps (Atkinson et al., 2004). Although understanding of the regional and temporal variations in these alternative pathways is emerging (Murphy et al., 2013; Hill et al., 2021) and such pathways may provide different links between pelagic-benthic and pelagic-mesopelagic communities, unless primary production changes they cannot support the same degree of predator demand as the phytoplankton-krill-predator pathway (Murphy et al., 2007a). This is because their increased complexity and concomitant trophic transfers are associated with greater energy loss (Murphy et al., 2012a,2016). Changes in structure and dominance characteristics of zooplankton communities, the causal mechanisms, and the effects on spatio-temporal variations in these energy pathways (and interactions between them) will therefore be important in interpreting future changes in Southern Ocean ecosystems.
Of greatest concern south of the APF is a shift from Antarctic krill dominated communities in the HNHC waters of the Southwest Atlantic Sector, to communities dominated by salps and/or copepods in response to ocean warming and sea ice loss. Such a shift would have important consequences for food webs here, including species that rely on Antarctic krill as a food source, particularly higher trophic level predators (see Bestley et al., 2020). Disruption of the short, energetically efficient food chain afforded by Antarctic krill may not be sufficient to support current levels of predator populations in the region. Changes in grazing pressure under the dominance of salps and/or copepods (which favor smaller phytoplankton species than ingested by Antarctic krill) may also have a top down influence on primary producers and their community dynamics and dominance patterns (see Pinkerton et al., 2021). Crucially this shift would also affect the operation and success of the Antarctic krill fishery (see Trebilco et al., 2020), the mackerel icefish, Antarctic and Patagonian toothfish fisheries, and wildlife tourism (see Grant et al., 2021). Consequences for regional biogeochemical cycling and contributions to climate regulation would also ensue (see Henley et al., 2020). Similarly, a shift from E. crystallorophias dominated communities in the neritic regions and sea ice zone of the high Antarctic to ones dominated by Antarctic krill, Thysanoessa macrura, and/or salps in response to ocean warming and sea ice loss will have several consequences for food webs, including for predator populations that rely on E. crystallorophias, and for primary producers and their community dynamics. There will also be effects on wildlife tourism, regional biogeochemical cycling, and climate regulation associated with such shifts in dominance. Ocean warming may also generate a shift in open ocean communities which are currently dominated by copepods and indirectly support the Antarctic krill fishery, wildlife tourism and potentially contribute significantly to biogeochemical cycling and carbon sequestration. Although overall increases in salps in open ocean (and other zooplankton) communities may have negative consequences for ecosystems services, they could enhance the potential for carbon export and support benthic communities (Trebilco et al., 2020). The implications for the loss of pteropods from the various zooplankton communities discussed here and the food webs they are part of, in response to increased ocean acidification, are less clear (Hunt et al., 2008). These potential shifts highlight the urgent need for improved research into zooplankton species other than Antarctic krill2.
The characterization of zooplankton communities in space and time across the Southern Ocean is challenging, especially given the vast scale and oceanographic complexity (Wiebe et al., 2017; Pinkerton et al., 2020), and relatively few studies have examined community dynamics and interactions. Nevertheless, an understanding of shifts in dominance at the community level will reveal important competitive and trophic interactions that may be missed if focusing solely on population level responses to change. A shift from autecological to synecological studies that examine life history traits and strategies, population and community dynamics is therefore required to facilitate more robust predictions of the cascading effects of global change through Southern Ocean food webs, and the associated top down and bottom up forcings.
Resilience
Just as some species may be vulnerable to drivers of future change, they may also be resilient (Keister et al., 2012; Murphy et al., 2016; Tarling et al., 2018). Resilience (sensu Oliver et al., 2015a) has gained increasing attention in interpreting change impacts on zooplankton and other marine species and for its application to ecosystem based management (Richardson, 2008; Watters et al., 2013; Constable et al., 2014a). There is also recognition that community dynamics may have potentially profound consequences for the resilience of ecosystem functions, including those upon which humans depend (Oliver et al., 2015b). Despite this, current projections of species and ecosystem change do not account for the capacity for resilience, for example the ability of communities to retain locations and distributional ranges (Angilletta, 2009; Tarling et al., 2018) and maintain processes that contribute to ecosystem function. Nor do they account for the negative impacts on biological resilence, particularly in response to multiple drivers of change.
Although we considered the potential resilience of zooplankton taxa to the impacts of key drivers, they are uncertain. Some of the taxa assessed here, including E. superba, Thysanoessa macrura, some copepods and salps, exhibit varying degrees of phenotypic plasticity and high variabilities in their life history strategies which confer their potential resilience to some drivers, at least up to a certain level. E. crystallorophias, however, has a limited thermal range and their genetic adaptation to past periods of increased glaciation imply that it is unlikely to be resilient to future climate change. The seasonal Ωar undersaturated conditions that thecosome pteropods already experience, together with an ability to maintain shell integrity may be indicative of a level of resilience. This, however, may come at a metabolic cost that is unlikely to be maintained over the long-term. A concerted effort to parameterize potential resilience and reduce associated uncertainties will enhance predictions of the distributional range of zooplankton species and communities (Tarling et al., 2018). Given that Southern Ocean zooplankton will be under the complex spatially and temporally variable influence of multiple drivers of change, further research into the individual as well as the additive, synergistic, or antagonistic effects of these on resilience is urgently required (Murphy et al., 2016; Gruber et al., 2021). These zooplankton taxa dominate the mid trophic level of many Southern Ocean food webs, transferring energy from lower to higher trophic levels. The low functional redundancy (i.e., a small number of species performing the majority of core ecological functions) of Southern Ocean food webs at this key trophic level renders these ecosystems particularly sensitive to change (Atkinson et al., 2014; Murphy et al., 2016). Understanding the influence of community dynamics on the manifestation of alternative energy pathways (Ducklow et al., 2007; Ainley et al., 2015) and the resilience of ecosystem functions is therefore also integral to predicting change.
Consequences for Ecosystem Structure and Functioning
Given the current understanding of the role of dominant zooplankton taxa assessed here in Southern Ocean ecosystems, it is clear that future changes in their population and community dynamics will have potentially wide ranging and complex consequences for biodiversity and ecosystem structure and functioning, including ecosystem services. Future changes in these zooplankton communities may alter food web connections within regional ecosystems (see McCormack et al., 2021), with implications for biogeochemical cycling, carbon flux and sequestration (see Henley et al., 2020), population and community dynamics, and energy flows to benthic communities (see Brasier et al., 2021) and between primary producers (see Pinkerton et al., 2021) through to mid- (see Caccavo et al., 2021) and higher trophic level predators (see Bestley et al., 2020), fisheries production, and wildlife tourism (see Rogers et al., 2020; Trebilco et al., 2020; Cavanagh et al., 2021; Grant et al., 2021). These may have ramifications for the resilience of population-, community- and ecosystem-level structure and functioning, and the emergent properties of regional ecosystems across the Southern Ocean. In addition, these zooplankton may also be involved in feedback process that modify the physical and chemical conditions in the Southern Ocean and regional climate processes (see Henley et al., 2020). Before we can predict the resultant effects on whole ecosystems and socioeconomics with any level of confidence, however, we must address key knowledge gaps on the complex interactions and mechanisms associated with zooplankton dynamics, their functional roles, and their sensitivities to drivers of change.
Future Work: Moving Toward Robust Quantitative Projections
The current trajectory of global greenhouse gas emission pathways (IPCC, 2018, 2019, 2021) means that we may not be on track to limit global warming to 1.5°C above pre-industrial levels and so we must accept that future climate change will be rapid, large ecological changes will occur, and future research must hence be centered on providing options for conservation and management. Robust qualitative and quantitative projections of future changes in Southern Ocean zooplankton are therefore needed to support global and regional assessments, and conservation and ecosystem-based management strategies that can anticipate or adapt to natural and anthropogenically driven change. As such, they must be focused on the appropriate species (and life history stages), processes, space, and timescales. Projections of the distributions and abundances of zooplankton populations and communities can be used to assess how these taxa may directly or indirectly influence the structure and functioning of Southern Ocean ecosystems, such as biodiversity, food webs, biogeochemical cycling and climate regulation, energy transfer to higher trophic level predators, and provisioning of fisheries and wildlife tourism. This requires three key areas of development. Firstly, a comprehensive understanding of zooplankton ecology, the internal and external drivers of zooplankton dynamics (e.g., ontogenic and species-specific physiology, morphology, behavior, dietary preferences and feeding strategies, life history strategies, populations, and community structure, distributions and dominance patterns, spatial connectivity and food web interactions, etc.) and seasonal development, past and present variability and change in zooplankton dynamics, attribution of the causes of zooplankton change to natural versus anthropogenic drivers, zooplankton resilience, and the mechanisms involved. Secondly, a suite of ecological models, including coupled physical, biogeochemical, and biological models, that can conceptualize and quantify these and relevant ecological processes. Thirdly, by driving these models with regionally appropriate scenarios of changes in key natural and anthropogenic drivers, robust projections of future changes in zooplankton can be made across a range of spatial, temporal, and organizational scales. Unfortunately, detailed knowledge (both qualitative and quantitative) for many zooplankton species, life history stages, populations and communities is limited to a few areas, and primarily during the austral spring/summer, and the underlying processes and causal mechanisms of their dynamics are poorly understood. Added to this scarcity, zooplankton data are not well centralized – they are dispersed across many storage platforms, in different formats, and in some cases are inaccessible. Whilst progress has been made in data synthesis and model development for some species (e.g., Antarctic krill) and scenarios and projections based on these, projections for zooplankton across the MEASO Areas and other key areas that can support policy decisions are restricted. Current key limitations are outlined in Box 1.
Given the mounting pressures of anthropogenic change, research resources need to be focused on the timely delivery of tailored information to policymakers that can support conservation and management options in the near-term. A coherent strategy that prioritizes Southern Ocean zooplankton research over the coming years to achieve this is therefore urgently required. Such a strategic approach (e.g., see Box 2) has already begun for Antarctic krill in the context of fisheries management through initiatives such as ICED and the SCAR Krill Action Group (SKAG) (Murphy et al., 2018; Meyer et al., 2020). An all-encompassing set of priorities for zooplankton is yet to be fully explored and consolidated, and will be a future collaborative activity of ICED.
BOX 2. Research gaps and priorities: Improving projections of future zooplankton change.
Robust qualitative and quantitative projections of zooplankton change are urgently needed to undertake assessments of current and future risks and inform conservation and management options for Southern Ocean ecosystems over the coming decades. A strategy for zooplankton research that prioritizes aspects in Box 1 to deliver these projections is therefore warranted. This must address knowledge gaps in fundamental (qualitative and quantitative) ecological understanding and facilitate model development by focusing on:
Key species and communities that are of importance to conservation and management and/or assumed or known to have important roles in ecosystem structure and functioning (including ecosystem services) either now or in the future.
Key regions and habitats that are of importance to the development of conservation and management strategies (this includes not only for, e.g., existing/planned Marine Protected Areas and the Commission for the Conservation of Antarctic Marine Living Resources (CCAMLR) Statistical Areas, but other ecologically important areas or hotspots that influence zooplankton dynamics). This should also include regions and habitats (and seasons) that are poorly sampled.
Projections of zooplankton change over short timescales (and within key regions) that are relevant to conservation and management (e.g., the next 3, 5 to 10 years in addition to the next few decades) and consider, incorporate, and communicate the uncertainties underpinning them (e.g., in understanding of ecological processes and scenarios of key drivers, and hence model parameterization).
Examples of near-term (2–5 years) and longer-term (>5 years) research priorities (in addition to information on habitats) include:
Near-term research priorities
Synthesis of existing data on distribution and abundance: Mine, collate and synthesize existing circumpolar-scale information and data on the spatio-temporal distributions of key taxa across key regions and habitats (particularly sea ice environments).
Life history models: Develop life history models for key species (that incorporate phenotypic plasticity) can be used to estimate population dynamics across space and time scales. In combination with observational data, they can be used to explore the biological and environmental factors that impact critical life history processes/events and drive population dynamics, thus giving an indication of the vulnerabilities of species to changing conditions. They can also be used to explore species influence on ecosystem functioning including energy flows, nutrient cycling, climate regulation, and the provisioning of fisheries and wildlife tourism under changing conditions.
Biogeochemical roles: Improve understanding of the role of zooplankton in biogeochemical cycles, particularly in carbon budgets and particulate organic matter export and remineralization.
Communication of uncertainties and risks: Evaluate and communicate all sources of uncertainty (e.g., in ecological, physical, and chemical processes, observations, model formulations, parameter estimates, model evaluation, etc.) and their incorporation into projections of change. Explore ways of dealing with current uncertainties. E.g., approaches that evaluate risks (based on the magnitude and probability of change) and incorporate uncertainty rather than specific trajectories of zooplankton change under future scenarios.
Resolution of conflicting views: Resolve conflicting views in zooplankton ecology, e.g., of trends in distribution and abundances in Antarctic krill, poleward shifts, and resilience of zooplankton in response to multiple drivers of environmental change.
Longer-term priorities
Mechanistic understanding: Identifying the underlying mechanisms that control observed patterns of zooplankton dynamics is crucial to predicting how they will respond to future change. A focus on generating specifically defined rationales explaining observed patterns of zooplankton communities and environmental variables, particularly sea ice, ocean temperatures and ocean acidification is needed. These will be important for informing the development of ecological models, projections of change, and testing conservation and management approaches.
Life histories, traits, and strategies: These are fundamental determinants of zooplankton population dynamics and their responses to change. They also underpin conservation and ecosystem-based management decisions and stock assessments. A more complete understanding and quantification of these aspects can reveal many of the factors that constrain the success of different species across spatial and temporal scales. This forms the basis of predicting zooplankton responses to future change and improving conservation and management decisions.
Life history models: Refine life history models based on improved mechanistic understanding.
Community dynamics and interactions: Shift from autecological to synecological studies to improve understanding of community dynamics (i.e., changes in community structure, dominance, and distribution patterns), trophic interactions with other taxa, and the seasonal development of these processes.
Biogeochemical roles: Develop a more complete understanding of the role of zooplankton in biogeochemical cycling and feedbacks.
Uncertainties and risks: Continue to evaluate, refine, and communicate all sources of uncertainty and communicate associated risks.
Promote data availability and application: Continue to mine, collate, and synthesize data relevant to zooplankton habitats, distribution and abundance and promote open access.
Such a strategy must be developed by a broad range of relevant stakeholders including ecologists, physicists, biogeochemists, climate and conservation scientists, social scientists, environmental economists, fisheries managers, industry representatives, and relevant programmes and initiatives such as ICED, MEASO, SCAR (and relevant research programmes therein including SKAG, SCAR Plastic in polar environments Action Group, PLASTIC-AG, and the Southern Ocean Observing System, SOOS), the Antarctic Treaty System (including CCAMLR and the Committee for Environmental Protection, CEP), and international treaties and organizations (e.g., the International Whaling Commission, IWC, the Agreement on the Conservation of Albatrosses and Petrels, ACAP, the IPCC, and the Intergovernmental Science-Policy Platform on Biodiversity and Ecosystem Services, IPBES). This will also foster a shared understanding of the challenges involved and the nature of existing and new multi- and interdisciplinary research approaches needed to address them.
Whilst extensive future circumpolar field efforts can improve data coverage, offering much needed spatially and temporally comprehensive insight into population and community dynamics and ecological processes, they cannot yield near-term support for conservation and management. Likewise, many of the large international observational programmes that are underway or being developed to detect change in physical, chemical, and biological aspects of the Southern Ocean will take some years to fully develop. This means that our ability to be definitive about the attribution of zooplankton and wider ecological changes to natural and/or anthropogenic change in this highly variable ocean is limited. In the interim, focusing on research areas that can support model parameterization and development, particularly better mechanistic understanding, will be important. This will allow us to test and explore ecological processes based on the best available knowledge, while communicating assumptions and uncertainties. It will also allow us to constrain model projections of future zooplankton change, enabling assessments of the potential range of impacts of change together with the associated uncertainties and risks they entail.
A strategy for zooplankton research must therefore address knowledge gaps in the fundamental (qualitative and quantitative) ecological understanding and the model development required to generate robust projections of ecological change and undertake assessments of current and future risks. We still need very basic, circumpolar-scale information on the spatio-temporal distributions of key taxa across a range of habitats (particularly sea ice environments) throughout the Southern Ocean. The mining, collation and synthesis of the large volumes of existing data provides a tractable approach to address this primary priority issue (e.g., Cornils et al., 2018). However, knowledge of distribution, abundance, life history traits and strategies and the seasonal development of population dynamics, biogeochemical roles and food web interactions are also a priority. These aspects in turn underpin models and projections of responses to future change, which also need to incorporate detailed assessments of the underpinning model assumptions and various other sources of model uncertainty. Such an endeavor requires an international collaborative effort and will benefit from the support of national Antarctic research programmes and international research programmes and initiatives, such as the Southern Ocean focused ICED, SCAR, and the global Integrated Marine Biosphere Research project (IMBeR).
Data Availability Statement
The data used in this study are available in the cited literature. Further details of methods and data and additional references are included in the Supplementary Material. The original contributions presented in this study are included in the article/Supplementary Material, further inquiries can be directed to the corresponding author.
Ethics Statement
Ethical review and approval was not required for the animal study because this article does not involve new or lab fieldwork.
Author Contributions
NMJ, EJM, and AJC conceived the project. NMJ coordinated the project, leading and significantly contributing to the development, and writing and editing of the manuscript. EJM and AA contributed significantly to the development and writing of the manuscript. SLH, SK, CSR, RD, AA, KMS, EAP, BPVH, and GAT contributed significantly to writing the manuscript (particularly Sections 1–5). MHP, GAT, and AA contributed to figure production. MHP provided analyses of SO-CPR data. All authors contributed to the writing of the manuscript, expert consenus assessments, and approved the final manuscript.
Funding
NMJ and EJM were supported by the UKRI Natural Environment Research Council (NERC) through the British Antarctic Survey (BAS) ALI-Science Southern Ocean ecosystems project of the BAS Ecosystems Team. NMJ was also supported by the European Union Horizon 2020 Research and Innovation Programme project PolarRES (under Grant agreement no. 101003590). JH was funded by ANID (FONDAP-IDEAL 15150003) and FONDECYT (Postdoctorado 3180152) and his attendance at the MEASO Workshop in Woking, United Kingdom was supported by ICED. MHP was funded by NIWA SSIF Programme 4, MBIE (Ross-RAMP, C01X1710; ASP, ANTA1801), and Fisheries New Zealand project ZBD2018-01. AA and SLH’s contributions were funded by WWF.
Conflict of Interest
AJC is a Topic Editor of this MEASO Special Issue. NMJ, EJM, AJC, and JH are members of MEASO Steering Committee. MHP was employed by National Institute of Water and Atmospheric Research Ltd.
The remaining authors declare that the research was conducted in the absence of any commercial or financial relationships that could be construed as a potential conflict of interest.
Publisher’s Note
All claims expressed in this article are solely those of the authors and do not necessarily represent those of their affiliated organizations, or those of the publisher, the editors and the reviewers. Any product that may be evaluated in this article, or claim that may be made by its manufacturer, is not guaranteed or endorsed by the publisher.
Acknowledgments
This manuscript builds on discussions during the Integrating Climate and Ecosystem Dynamics in the Southern Ocean (ICED) programme’s Marine Ecosystem Assessment of the Southern Ocean (MEASO) 2018 Conference (held in Hobart, TAS, Australia, 2018), the MEASO Workshop 1 (hosted by the WWW-UK, Woking, United Kingdom, 2019), and the MEASO Workshop 2 (held at the IMBeR Open Science Conference, Future Oceans2, Brest, France, 2019). We thank all of the workshop participants. We acknowledge the SCAR SO-CPR project and the Marine Biological Association CPR Survey (formerly SAHFOS, Plymouth, United Kingdom).
Supplementary Material
The Supplementary Material for this article can be found online at: https://www.frontiersin.org/articles/10.3389/fevo.2021.624692/full#supplementary-material
Supplementary Figure 1 | Modelled environmental suitability for (A) total zooplankton, i.e., Euphausiidae, Copepoda (Calanoida and Oithona similis), and pteropods, (B) Euphausiidae, (C) Copepoda (Calanoida), (D) Oithona similis, and (E) pteropod abundance from the combined Boosted Regression Tree model of Pinkerton et al. (2020) showing average modelled daily abundance (counts per 5 nautical mile CPR segment) over the 6 months (October to March) and years 1998–2018. No analysis was possible for areas shown in white, for example because environmental conditions were outside the observations. Black lines are nominal positions of (from north): Subantarctic Front, Antarctic Polar Front, average maximum northern extent of seasonal sea ice (dashed), and the Southern Boundary of the Antarctic Circumpolar Current.
Supplementary Figure 2 | Modelled environmental suitability for (A) total zooplankton, i.e., Euphausiidae, Copepoda (Calanoida and Oithona similis), and pteropods, (B) Euphausiidae, (C) Copepoda (Calanoida), (D) Oithona similis, and (E) pteropod abundance from the combined Boosted Regression Tree model of Pinkerton et al. (2020) showing long-term, linear trends in abundance (trend units are 1000 counts per 5 nautical miles of CPR segment per year). Sen slope values showing the changes in modelled abundance at each point. Colored vales are significant (p < 0.05). Warm colors indicate a positive (increasing) trend in the environmental suitability, and cold colors a negative (decreasing) trend. White areas have no significant trend. Areas shown gray have no data. Black lines are nominal positions of (from north): Subantarctic Front, Antarctic Polar Front, average maximum northern extent of seasonal sea ice (dashed), and the Southern Boundary of the Antarctic Circumpolar Current.
Supplementary Figure 3 | Time series of change in environmental suitability for total zooplankton abundance, i.e., Euphausiidae, Copepoda (Calanoida and Oithona similis), and pteropods, from the combined Boosted Regression Tree model of Pinkerton et al. (2020) for individual Marine Ecosystem Assessment for the Southern Ocean (MEASO) Areas. Imbedded three letter codes in figure relate to MEASO sectors (first two letters) and zones (last letter). Sectors are Atlantic (AO), Central Indian (CI), East Indian (EI), West Pacific (WP), and East Pacific (EA). Zones are Antarctic (A), Subantarctic (S), Northern (N). See also Figure 1 in main paper. The environmental suitability anomaly (difference from the monthly climatological value) is shown for each month over period 1998–2018. Gray shading indicates calendar years. Black points and lines are monthly anomalies (differences from long-term monthly means). Red lines are smoothed changes (4-year running median).
Supplementary Table 1 | Current role of zooplankton in Southern Ocean ecosystems (based on information provided in taxonomic Sections Euphausiids (Family Euphausiidae), Copepods (Subclass Copepoda), Salps (Order Salpida), and Pteropods (Order Pteropoda)). Roles may vary across areas of the Southern Ocean, seasons, or with life history stages. See Figure 1 for locations and key to MEASO areas. See Supplementary Table 2 for environmental tolerances.
Supplementary Table 2 | Environmental tolerances of zooplankton in Southern Ocean ecosystems (based on empirical studies). n/a = data not available.
Supplementary Table 3 | Future prognoses* for Southern Ocean zooplankton in response to changes in key physical, chemical, and ecological drivers (based on information provided in Sections Euphausiids (Family Euphausiidae), Copepods (Subclass Copepoda), Salps (Order Salpida), Pteropods (Order Pteropoda), and Past Changes in Zooplankton: A modelled example using Continuous Plankton Recorder Data (including references cited therein)) and Supplementary Tables 1, 2. Prognoses may vary across areas of the Southern Ocean, seasons, or life history stages. See Figure 1 for locations and key to MEASO areas.
Footnotes
- ^ See Pinkerton et al. (2020) for assumptions, limitations, and caveats of the analyses.
- ^ A Web of Science search (using appropriate terms to capture Southern Ocean research on the four taxa and key species examined in this study) reveals a prevalence of publications on krill and Antarctic krill over the past 10 years, compared with copepod, salps and pteropods.
References
Aarset, A. V., and Torres, J. J. (1989). Cold resistance and metabolic responses to salinity variations in the amphipod Eusirus antarcticus and the krill Euphausia superba. Polar Biol. 9, 491–497. doi: 10.1007/BF00261032
Ainley, D. G., Ballard, G., Jones, R. M., Jongsomjit, D., Pierce, S. D., Smith, W. O. J., et al. (2015). Trophic cascades in the western Ross Sea, Antarctica: revisited. Mar. Ecol. Prog. Ser. 534, 1–16. doi: 10.3354/meps11394
Ainley, D. G., Ribic, C. A., Ballard, G., Heath, S., Gaffney, I., Karl, B. J., et al. (2004). Geographic structure of Adélie penguin populations: overlap in colony-specific foraging areas. Ecol. Monogr. 74, 159–178. doi: 10.1890/02-4073
Akiha, F., Hashida, G., Makabe, R., Hattori, H., and Sasaki, H. (2017). Distribution in the abundance and biomass of shelled pteropods in surface waters of the Indian sector of the Antarctic Ocean in mid-summer. Polar Sci. 12, 12–18. doi: 10.1016/j.polar.2017.02.003
Alcaraz, M., Almeda, R., Duarte, C. M., Horstkotte, B., Lasternas, S., and AgustÃ, S. (2014). Changes in the C, N, and P cycles by the predicted salps-krill shift in the Southern Ocean. Front. Mar. Sci. 1:45. doi: 10.3389/fmars.2014.00045
Andrews, K. J. (1966). The distribution and life-history of Calanoides acutus (Giesbrecht). Discov. Rep. 34, 117–162.
Angilletta, M. J. Jr. (2009). Thermal Adaptation: A Theoretical and Empirical Synthesis. New York, NY: Oxford University Press. doi: 10.1093/acprof:oso/9780198570875.001.1
Atkinson, A. (1998). Life cycle strategies of epipelagic copepods in the Southern Ocean. J. Mar. Syst. 15, 289–311. doi: 10.1016/S0924-7963(97)00081-X
Atkinson, A., Hill, S. L., Barange, M., Pakhomov, E. A., Raubenheimer, D., Schmidt, K., et al. (2014). Sardine cycles, krill declines, and locust plagues: revisiting ‘wasp-waist’ food webs. Trends Ecol. Evol. 29, 309–316. doi: 10.1016/j.tree.2014.03.011
Atkinson, A., Hill, S. L., Pakhomov, E. A., Anadon, R., Chiba, S., Daly, K. L., et al. (2017). KRILLBASE: a circumpolar database of Antarctic krill and salp numerical densities, 1926–2016. Earth Syst. Sci. Data 9, 193–210. doi: 10.5194/essd-9-193-2017
Atkinson, A., Hill, S. L., Pakhomov, E. A., Siegel, V., Reiss, C. S., Loeb, V. J., et al. (2019). Krill (Euphausia superba) distribution contracts southward during rapid regional warming. Nat. Clim. Change 9:142. doi: 10.1038/s41558-018-0370-z
Atkinson, A., Shreeve, R. S., Hirst, A. G., Rothery, P., Tarling, G. A., Pond, D. W., et al. (2006). Natural growth rates in Antarctic krill (Euphausia superba): II. Predictive models based on food, temperature, body length, sex, and maturity stage. Limnol. Oceanogr. 51, 973–987. doi: 10.4319/lo.2006.51.2.0973
Atkinson, A., Siegel, V., Pakhomov, E., and Rothery, P. (2004). Long-term decline in krill stock and increase in salps within the Southern Ocean. Nature 432:100. doi: 10.1038/nature02996
Atkinson, A., Siegel, V., Pakhomov, E. A., Rothery, P., Loeb, V., Ross, R. M., et al. (2008). Oceanic circumpolar habitats of Antarctic krill. Mar. Ecol. Progr. Ser. 362, 1–23. doi: 10.3354/meps07498
Atkinson, A., and Sinclair, J. D. (2000). Zonal distribution and seasonal vertical migration of copepod assemblages in the Scotia Sea. Polar Biol. 23, 46–58. doi: 10.1007/s003000050007
Atkinson, A., Ward, P., Hunt, B., Pakhomov, E., and Hosie, G. (2012). An overview of Southern Ocean zooplankton data: abundance, biomass, feeding and functional relationships. CCAMLR Sci. 19, 171–218.
Atkinson, A., Whitehouse, M. J., Priddle, J., Cripps, G. C., Ward, P., and Brandon, M. A. (2001). South Georgia, Antarctica: a productive, cold water, pelagic ecosystem. Mar. Ecol. Prog. Ser. 216, 279–308. doi: 10.3354/meps216279
Barnes, A. J., and Pálinkó, I. (2017). Preface. J. Mol. Struct. 1:1140. doi: 10.1016/j.molstruc.2016.12.027
Barnes, D. K. A., Fleming, A., Sands, C. J., Quartino, M. L., and Deregibus, D. (2018). Icebergs, sea ice, blue carbon and Antarctic climate feedbacks. Philos. Trans. R. Soc. A Math. Phys. Eng. Sci. 376:20170176. doi: 10.1098/rsta.2017.0176
Bar-On, Y. M., Phillips, R., and Milo, R. (2018). The biomass distribution on Earth. Proc. Natl. Acad. Sci. U.S.A. 115, 6506–6511. doi: 10.1073/pnas.1711842115
Batta-Lona, P., Maas, A., O’Neill, R., Wiebe, P., and Bucklin, A. (2017). Transcriptomic profiles of spring and summer populations of the Southern Ocean salp, Salpa thompsoni, in the Western Antarctic Peninsula region. Polar Biol. 40, 1261–1276. doi: 10.1007/s00300-016-2051-6
Beaugrand, G., and Kirby, R. R. (2018). How do marine pelagic species respond to climate change? Theories and observations. Annu. Rev. Mar. Sci. 10, 169–197. doi: 10.1146/annurev-marine-121916-063304
Beaugrand, G., Luczak, C., and Edwards, M. (2009). Rapid biogeographical plankton shifts in the North Atlantic Ocean. Glob. Change Biol. 15, 1790–1803. doi: 10.1111/j.1365-2486.2009.01848.x
Beaugrand, G., McQuatters-Gollop, A., Edwards, M., and Goberville, E. (2013). Long-term responses of North Atlantic calcifying plankton to climate change. Nat. Clim. Change 3, 263–267. doi: 10.1038/nclimate1753
Bebianno, M., Calumpong, H., Chiba, S., Evans, K., Garcia-Soto, C., Kamara, O., et al. (2021a). “Chapter 01, Overall summary,” in World Ocean Assessment II, Vol. I, (New York, NY: United Nations), 3–35. doi: 10.18356/9789216040062c004
Bebianno, M., Calumpong, H., Chiba, S., Evans, K., Garcia-Soto, C., Kamara, O., et al. (2021b). “Chapter 02, Approach to the assessment,” in World Ocean Assessment II, Vol. I, (New York, NY: United Nations), 39–45. doi: 10.18356/9789216040062c005
Bednaršek, N., Feely, R. A., Beck, M. W., Glippa, O., Kanerva, M., and Engström-Öst, J. (2018). El niño-related thermal stress coupled with upwelling-related ocean acidification negatively impacts cellular to population-level responses in pteropods along the california current system with implications for increased bioenergetic costs. Front. Mar. Sci. 5:486. doi: 10.3389/fmars.2018.00486
Bednaršek, N., Feely, R. A., Howes, E. L., Hunt, B. P. V., Kessouri, F., León, P., et al. (2019). Systematic review and meta-analysis toward synthesis of thresholds of ocean acidification impacts on calcifying pteropods and interactions with warming. Front. Mar. Sci. 6:227. doi: 10.3389/fmars.2019.00227
Bednaršek, N., Feely, R. A., Tolimieri, N., Hermann, A. J., Siedlecki, S. A., Waldbusser, G. G., et al. (2017a). Exposure history determines pteropod vulnerability to ocean acidification along the US West Coast. Sci. Rep. 7:4526. doi: 10.1038/s41598-017-03934-z
Bednaršek, N., Klinger, T., Harvey, C. J., Weisberg, S., McCabe, R. M., Feely, R. A., et al. (2017b). New ocean, new needs: application of pteropod shell dissolution as a biological indicator for marine resource management. Ecol. Indic. 76, 240–244. doi: 10.1016/j.ecolind.2017.01.025
Bednaršek, N., Harvey, C. J., Kaplan, I. C., Feely, R. A., and Možina, J. (2016). Pteropods on the edge: cumulative effects of ocean acidification, warming, and deoxygenation. Prog. Oceanogr. 145, 1–24. doi: 10.1016/j.pocean.2016.04.002
Bednaršek, N., Možina, J., Vogt, M., and Brien, C. (2012a). The global distribution of pteropods and their contribution to carbonate and carbon biomass in the modern ocean. Earth Syst. Sci. Data 4, 167–186. doi: 10.5194/essd-4-167-2012
Bednaršek, N., Naish, K.-A., Feely, R. A., Hauri, C., Kimoto, K., Hermann, A. J., et al. (2021). Integrated assessment of ocean acidification risks to pteropods in the northern high latitudes: regional comparison of exposure, sensitivity and adaptive capacity. Front. Mar. Sci. 8:1282. doi: 10.3389/fmars.2021.671497
Bednaršek, N., and Ohman, M. D. (2015). Changes in pteropod distributions and shell dissolution across a frontal system in the California current system. Mar. Ecol. Prog. Ser. 523, 93–103. doi: 10.3354/meps11199
Bednaršek, N., Tarling, G. A., Bakker, D. C. E., Fielding, S., and Feely, R. A. (2014). Dissolution dominating calcification process in polar pteropods close to the point of aragonite undersaturation. PLoS One 9:e109183. doi: 10.1371/journal.pone.0109183
Bednarsek, N., Tarling, G. A., Bakker, D. C. E., Fielding, S., Jones, E. M., Venables, H. J., et al. (2012b). Extensive dissolution of live pteropods in the Southern Ocean. Nat. Geosci. 5, 881–885. doi: 10.1038/ngeo1635
Belcher, A., Henson, S. A., Manno, C., Hill, S. L., Atkinson, A., Thorpe, S. E., et al. (2019). Krill faecal pellets drive hidden pulses of particulate organic carbon in the marginal ice zone. Nat. Commun. 10, 1–8. doi: 10.1038/s41467-019-08847-1
Bernard, K. S., Steinberg, D. K., and Schofield, O. M. E. (2012). Summertime grazing impact of the dominant macrozooplankton off the Western Antarctic Peninsula. Deep Sea Res. Part I Oceanogr. Res. Pap. 62, 111–122. doi: 10.1016/j.dsr.2011.12.015
Bestley, S., Ropert-Coudert, Y., Bengtson Nash, S., Brooks, C. M., Cotté, C., Dewar, M., et al. (2020). Marine ecosystem assessment for the Southern Ocean: birds and marine mammals in a changing climate. Front. Ecol. Evol. 8:338. doi: 10.3389/fevo.2020.566936
Bitter, M. C., Kapsenberg, L., Gattuso, J. P., and Pfister, C. A. (2019). Standing genetic variation fuels rapid adaptation to ocean acidification. Nat. Commun. 10:5821. doi: 10.1038/s41467-019-13767-1
Boysen-Ennen, E., Hagen, W., Hubold, G., and Piatkowski, U. (1991). Zooplankton biomass in the ice-covered Weddell Sea, Antarctica. Mar. Biol. 111, 227–235. doi: 10.1007/bf01319704
Boysen-Ennen, E., and Piatkowski, U. (1988). Meso- and macrozooplankton communities in the Weddell Sea, Antarctica. Polar Biol. 9, 17–35. doi: 10.1007/bf00441761
Braithwaite, J. E., Meeuwig, J. J., Letessier, T. B., Jenner, K. C. S., and Brierley, A. S. (2015). From sea ice to blubber: linking whale condition to krill abundance using historical whaling records. Polar Biol. 38, 1195–1202. doi: 10.1007/s00300-015-1685-0
Branch, T. A. (2011). Humpback whale abundance south of 60°S from three complete circumpolar sets of surveys. J. Cetacean Res. Manag. 3, 53–69. doi: 10.47536/jcrm.vi.305
Branch, T. A., Stafford, K. M., Palacios, D. M., Allison, C., Bannister, J. L., Burton, C. L. K., et al. (2007). Past and present distribution, densities and movements of blue whales Balaenoptera musculus in the Southern Hemisphere and northern Indian Ocean. Mamm. Rev. 37, 116–175. doi: 10.1111/j.1365-2907.2007.00106.x
Brasier, M. J., Barnes, D., Bax, N., Brandt, A., Christianson, A. B., Constable, A. J., et al. (2021). Responses of Southern Ocean seafloor habitats and communities to global and local drivers of change. Front. Mar. Sci. 8:109. doi: 10.3389/fmars.2021.622721
Brierley, A. S., Fernandes, P. G., Brandon, M. A., Armstrong, F., Millard, N. W., McPhail, S. D., et al. (2002). Antarctic krill under sea ice: elevated abundance in a narrow band just south of ice edge. Science 295, 1890–1892. doi: 10.1126/science.1068574
Bruland, K. W., and Silver, M. W. (1981). Sinking rates of fecal pellets from gelatinous zooplankton (salps, pteropods, doliolids). Mar. Biol. 63, 295–300. doi: 10.1007/bf00395999
Brun, P., Kiørboe, T., Licandro, P., and Payne, M. R. (2016). The predictive skill of species distribution models for plankton in a changing climate. Glob. Change Biol. 22, 3170–3181. doi: 10.1111/gcb.13274
Bucklin, A., Divito, K., Batta-Lona, P., Wiebe, P. H., Questel, J. M., and O’Neill, R. J. (2018). “Genomic basis of adaptation of the Southern Ocean salp, Salpa thompsoni. Where the poles come together,” in Proceedings of the SCAR & IASC Open Science Conference, Davos.
Buitenhuis, E. T., Rivkin, R. B., Sailley, S., and Le Quéré, C. (2010). Biogeochemical fluxes through microzooplankton. Glob. Biogeochem. Cycles 24:4. doi: 10.1029/2009GB003601
Cabanes, D. J. E., Norman, L., Santos-Echeandía, J., Iversen, M. H., Trimborn, S., Laglera, L. M., et al. (2017). First evaluation of the role of salp fecal pellets on iron biogeochemistry. Front. Mar. Sci. 3:289. doi: 10.3389/fmars.2016.00289
Caccavo, J. A., Christiansen, H., Constable, A. J., Ghigliotti, L., Trebilco, R., Brooks, C. M., et al. (2021). Productivity and change in fish and squid in the Southern Ocean. Front. Ecol. Evol. 9:351. doi: 10.3389/fevo.2021.624918
Cascella, K., Jollivet, D., Papot, C., Leger, N., Corre, E., and Ravaux, J. (2015). Diversification, evolution and subfunctionalization of 70 kDa heat-shock proteins in two species of Antarctic krill: differences in thermal habitats, responses and implications under climate change. PLoS One 10:e0121642. doi: 10.1371/journal.pone.0121642
Cavan, E. L., Belcher, A., Atkinson, A., Hill, S. L., Kawaguchi, S., McCormack, S., et al. (2019). The importance of Antarctic krill in biogeochemical cycles. Nat. Commun. 10:4742. doi: 10.1038/s41467-019-12668-7
Cavanagh, R. D., Melbourne-Thomas, J., Grant, S. M., Barnes, D. K. A., Hughes, K. A., Halfter, S., et al. (2021). Future risk for Southern Ocean ecosystem services under climate change. Front. Mar. Sci. 7:1224. doi: 10.3389/fmars.2020.615214
CCAMLR (2020). Report of the Thirty-Ninth Meeting of the Commission. CCAMLR-39. Hobart, TAS: CCAMLR.
Chapman, C. C., Lea, M.-A., Meyer, A., Sallée, J.-B., and Hindell, M. (2020). Defining Southern Ocean fronts and their influence on biological and physical processes in a changing climate. Nat. Clim. Change 10, 209–219. doi: 10.1038/s41558-020-0705-4
Chiba, S., Batten, S., Martin, C. S., Ivory, S., Miloslavich, P., and Weatherdon, L. V. (2018). Zooplankton monitoring to contribute towards addressing global biodiversity conservation challenges. J. Plankton Res. 40, 509–518. doi: 10.1093/plankt/fby030
Chivers, W. J., Walne, A. W., and Hays, G. C. (2017). Mismatch between marine plankton range movements and the velocity of climate change. Nat. Commun. 8, 1–8. doi: 10.1038/ncomms14434
Chown, S. L., and Brooks, C. M. (2019). The state and future of Antarctic environments in a global context. Annu. Rev. Environ. Resour. 44, 1–30. doi: 10.1146/annurev-environ-101718-033236
Clarke, A. (1985). “Energy flow in the Southern Ocean food web,” in Antarctic Nutrient Cycles and Food Webs, eds W. R. Siegfried, P. R. Condy, and R. M. Laws (Berlin: Springer), 573–580. doi: 10.1007/978-3-642-82275-9_78
Clarke, A., and Crame, J. A. (2010). Evolutionary dynamics at high latitudes: speciation and extinction in polar marine faunas. Philos. Trans. R. Soc. Lond. B Biol. Sci. 365, 3655–3666. doi: 10.1098/rstb.2010.0270
Clarke, A., and Johnston, N. M. (2003). Antarctic marine benthic diversity. Oceanogr. Mar. Biol. 41, 47–114.
Clarke, A., Johnston, N. M., Murphy, E. J., and Rogers, A. D. (2012). “Introduction: Antarctic ecology in a changing world,” in Antarctic Ecosystems, eds A. D. Rogers, N. M. Johnston, E. J. Murphy, and A. Clarke (Chichester: Wiley-Blackwell), 1–9. doi: 10.1002/9781444347241.ch
Collins, M. A., Xavier, J. C., Johnston, N. M., North, A. W., Enderlein, P., Tarling, G. A., et al. (2008). Patterns in the distribution of myctophid fish in the northern Scotia Sea ecosystem. Polar Biol. 31, 837–851. doi: 10.1007/s00300-008-0423-2
Comeau, S., Gorsky, G., Alliouane, S., and Gattuso, J. P. (2010). Larvae of the pteropod Cavolinia inflexa; exposed to aragonite undersaturation are viable but shell-less. Mar. Biol. 157, 2341–2345. doi: 10.1007/s00227-010-1493-6
Conover, R. J., and Huntley, M. (1991). Copepods in ice-covered seas—distribution, adaptations to seasonally limited food, metabolism, growth patterns and life cycle strategies in polar seas. J. Mar. Syst. 2, 1–41. doi: 10.1016/0924-7963(91)90011-I
Conroy, J., Steinberg, D., Thibodeau, P., and Schofield, O. (2020). Zooplankton diel vertical migration during Antarctic summer. Deep Sea Res. 162:103324. doi: 10.1016/j.dsr.2020.103324
Constable, A. J., Costa, D., Murphy, E. J., Hoffman, J. I., Schofield, O., Press, A., et al. (2014a). “Assessing status and change in Southern Ocean ecosystems,” in Biogeographic Atlas of the Southern Ocean, eds C. De Broyer, P. Koubbi, H. J. Griffiths, B. Raymond, A. Van de Putte, B. Danis, et al. (Cambridge: SCAR), 404–407.
Constable, A. J., Melbourne-Thomas, J., Corney, S. P., Arrigo, K. R., Barbraud, C., Barnes, D. K., et al. (2014b). Climate change and Southern Ocean ecosystems I: how changes in physical habitats directly affect marine biota. Glob. Change Biol. 20, 3004–3025. doi: 10.1111/gcb.12623
Constable, A. J., and Kawaguchi, S. (2018). Modelling growth and reproduction of Antarctic krill, Euphausia superba, based on temperature, food and resource allocation amongst life history functions. ICES J. Mar. Sci. 75, 738–750. doi: 10.1093/icesjms/fsx190
Cornils, A., Sieger, R., Mizdalski, E., Schumacher, S., Grobe, H., and Schnack-Schiel, S. B. (2018). Copepod species abundance from the Southern Ocean and other regions (1980–2005) – a legacy. Earth Syst. Sci. Data 10, 1457–1471. doi: 10.5194/essd-10-1457-2018
Cornils, A., Wend-Heckmann, B., and Held, C. (2017). Global phylogeography of Oithona similis s.l. (Crustacea, Copepoda, Oithonidae) – a cosmopolitan plankton species or a complex of cryptic lineages? Mol. Phylogenet. Evol. 107, 473–485. doi: 10.1016/j.ympev.2016.12.019
Cox, M. J., Candy, S., de la Mare, W. K., Nicol, S., Kawaguchi, S., and Gales, N. (2018). No evidence for a decline in the density of Antarctic krill Euphausia superba Dana, 1850, in the Southwest Atlantic sector between 1976 and 2016. J. Crustac. Biol. 38, 656–661. doi: 10.1093/jcbiol/ruy072
Croxall, J. P., and Nicol, S. (2004). Management of Southern Ocean fisheries: global forces and future sustainability. Antarct. Sci. 16:569. doi: 10.1017/S0954102004002330
Croxall, J. P., Reid, K., and Prince, P. A. (1999). Diet, provisioning and productivity responses of marine predators to differences in availability of Antarctic krill. Mar. Ecol. Prog. Ser. 177, 115–131. doi: 10.3354/meps177115
Cuzin-Roudy, J., Irisson, J. O., Penot, F., Kawaguchi, S., and Vallet, C. (2014). “Southern Ocean Euphausiids,” in Biogeographic Atlas of the Southern Ocean, eds C. De Broyer, P. Koubbi, H. J. Griffiths, B. Raymond, B. Danis, S. Grant, et al. (Cambridge: SCAR), 309–320.
Daly, K. L. (1990). Overwintering development, growth, and feeding of larval Euphausia superba in the Antarctic marginal ice zone. Limnol. Oceanogr. 35, 1564–1576. doi: 10.4319/lo.1990.35.7.1564
Daly, K. L., and DiTullio, G. R. (1996). “Particulate dimethylsulfoniopropionate removal and dimethylsulfide production by zooplankton in the Southern Ocean,” in Biological and Environmental Chemistry of DMSP and Related Sulfonium Compounds, eds R. P. Kiene, P. T. Visscher, M. D. Keller, and G. O. Kirst (Boston, MA: Springer), 223–238. doi: 10.1007/978-1-4613-0377-0_20
Daly, K. L., and Macaulay, M. C. (1988). Abundance and distribution of krill in the ice edge zone of the Weddell Sea, austral spring 1983. Deep Sea Res. 35, 21–41. doi: 10.1016/0198-0149(88)90055-6
Daly, K. L., and Macaulay, M. C. (1991). The influence of physical and biological mesoscale dynamics on the seasonal distribution and behavior of Euphausia superba in the antarctic marginal ice zone. Mar. Ecol. Prog. Ser. 79, 37–66. doi: 10.3354/meps079037
Daly, K. L., and Zimmerman, J. J. (2004). Comparisons of morphology and neritic distributions of Euphausia crystallorophias and Euphausia superba furcilia during autumn and winter west of the Antarctic Peninsula. Polar Biol. 28, 72–81. doi: 10.1007/s00300-004-0660-y
Dam, H. G. (2013). Evolutionary adaptation of marine zooplankton to global change. Annu. Rev. Mar. Sci. 5, 349–370. doi: 10.1146/annurev-marine-121211-172229
Daufresne, M., Lengfellner, K., and Sommer, U. (2009). Global warming benefits the small in aquatic ecosystems. Proc. Natl. Acad. Sci. U.S.A. 106, 12788–12793. doi: 10.1073/pnas.0902080106
Davis, L. B., Hofmann, E. E., Klinck, J. M., Piñones, A., and Dinniman, M. S. (2017). Distributions of krill and Antarctic silverfish and correlations with environmental variables in the western Ross Sea, Antarctica. Mar. Ecol. Prog. Ser. 584, 45–65. doi: 10.3354/meps12347
Deibel, D., and Daly, K. L. (2007). Zooplankton processes in Arctic and Antarctic polynyas. Elsev. Oceanogr. Ser. 74, 271–322. doi: 10.1016/S0422-9894(06)74009-0
Deppeler, S. L., and Davidson, A. T. (2017). Southern Ocean phytoplankton in a changing climate. Front. Mar. Sci. 4:40. doi: 10.3389/fmars.2017.00040
Doney, S. C., Ruckelshaus, M., Duffy, J. E., Barry, J. P., Chan, F., English, C. A., et al. (2012). Climate change impacts on marine ecosystems. Annu. Rev. Mar. Sci. 4, 11–37. doi: 10.1146/annurev-marine-041911-111611
Driscoll, R. M. (2019). Adapted to Environmental Change: Life History, Diet, and Habitat Choice of Krill in Winter. Doctoral thesis. Santa Cruz, CA: University of California.
Driscoll, R. M., Reiss, C. S., and Hentschel, B. T. (2015). Temperature-dependent growth of Thysanoessa macrura: inter-annual and spatial variability around Elephant Island, Antarctica. Mar. Ecol. Prog. Ser. 529, 49–61. doi: 10.3354/meps11291
Ducklow, H. W., Baker, K., Martinson, D. G., Quetin, L. B., Ross, R. M., Smith, R. C., et al. (2007). Marine pelagic ecosystems: the west Antarctic Peninsula. Philos. Trans. R. Soc. B Biol. Sci. 362, 67–94. doi: 10.1098/rstb.2006.1955
Ducklow, H. W., Clarke, A., Dickhut, R., Doney, S. C., Geisz, H., Huang, K., et al. (2012). “The marine ecosystem of the west Antarctic Peninsula,” in Antarctic Ecosystems in a Changing World, eds A. D. Rogers, N. M. Johnston, E. J. Murphy, and A. Clarke (Korolev: John Wiley and Sons), 121–159. doi: 10.1002/9781444347241.ch5
Ericson, J. A., Hellessey, N., Kawaguchi, S., Nicol, S., Nichols, P. D., Hoem, N., et al. (2018). Adult Antarctic krill proves resilient in a simulated high CO2 ocean. Commun. Biol. 1:190. doi: 10.1038/s42003-018-0195-3
Everson, I. (2000). Krill: Biology, Ecology and Fisheries. Hoboken, NJ: John Wiley & Sons. doi: 10.1002/9780470999493
Fabry, V. J., Seibel, B. A., Feely, R. A., and Orr, J. C. (2008). Impacts of ocean acidification on marine fauna and ecosystem processes. ICES J. Mar. Sci. 65, 414–432. doi: 10.1093/icesjms/fsn048
Fielding, S., Watkins, J. L., Trathan, P. N., Enderlein, P., Waluda, C. M., Stowasser, G., et al. (2014). Interannual variability in Antarctic krill (Euphausia superba) density at South Georgia, Southern Ocean: 1997–2013. ICES J. Mar. Sci. 71, 2578–2588. doi: 10.1093/icesjms/fsu104
Figuerola, B., Hancock, A. M., Bax, N., Cummings, V. J., Downey, R., Griffiths, H. J., et al. (2021). A review and meta-analysis of potential impacts of ocean acidification on marine calcifiers from the Southern Ocean. Front. Mar. Sci. 8:24. doi: 10.3389/fmars.2021.584445
Flores, H., Atkinson, A., Kawaguchi, S., Krafft, B. A., Milinevsky, G., Nicol, S., et al. (2012a). Impact of climate change on Antarctic krill. Mar. Ecol. Prog. Ser. 458, 1–19. doi: /10.3354/meps09831
Flores, H., Van Franeker, J. A., Siegel, V., Haraldsson, M., Strass, V., Meesters, E. H., et al. (2012b). The association of Antarctic krill Euphausia superba with the under-ice habitat. PLoS One 7:e31775. doi: 10.1371/journal.pone.0031775
Flores, H., Hunt, B. P. V., Kruse, S., Pakhomov, E. A., Siegel, V., van Franeker, J. A., et al. (2014). Seasonal changes in the vertical distribution and community structure of Antarctic macrozooplankton and micronekton. Deep Sea Res. Part I Oceanogr. Res. Pap. 84:127. doi: 10.1016/j.dsr.2013.11.001
Forcada, J., and Hoffman, J. I. (2014). Climate change selects for heterozygosity in a declining fur seal population. Nature 511, 462–465. doi: 10.1038/nature13542
Foxton, P. (1966). The distribution and life-history of Salpa thompsoni Foxton with observations on a related species Salpa gerlachei. Discov. Rep. 34, 1–116.
Froneman, P. W., Pakhomov, E. A., Perissinotto, R., and Meaton, V. (2000). Zooplankton structure and grazing in the Atlantic sector of the Southern Ocean in late austral summer 1993 Part 2. Biochemical zonation. Deep Sea Res. Part I Oceanogr. Res. Pap. 47, 1687–1702. doi: 10.1016/S0967-0637(99)00121-1
Garcia, M. D., Severini, M. D. F., Spetter, C., Abbate, M. C. L., Tartara, M. N., Nahuelhual, E. G., et al. (2019). Effects of glacier melting on the planktonic communities of two Antarctic coastal areas (Potter Cove and Hope Bay) in summer. Reg. Stud. Mar. Sci. 30:100731. doi: 10.1016/j.rsma.2019.100731
Gardner, J., Manno, C., Bakker, D. C. E., Peck, V. L., and Tarling, G. A. (2018). Southern Ocean pteropods at risk from ocean warming and acidification. Mar. Biol. 165, 8–8. doi: 10.1007/s00227-017-3261-3
Gili, J.-M., Rossi, S., Pagès, F., Orejas, C., Teixidó, N., López-González, P. J., et al. (2006). A new trophic link between the pelagic and benthic systems on the Antarctic shelf. Mar. Ecol. Prog. Ser. 322, 43–49. doi: 10.3354/meps322043
Goodall-Copestake, W., Perez-Espona, S., Clark, M., Murphy, E., Seear, P., and Tarling, G. (2010). Swarms of diversity at the gene cox1 in Antarctic krill. Heredity 104:513. doi: 10.1038/hdy.2009.188
Goodall-Copestake, W. P. (2017). One tunic but more than one barcode: evolutionary insights from dynamic mitochondrial DNA in Salpa thompsoni (Tunicata: Salpida). Biol. J. Linn. Soc. 120, 637–648. doi: 10.1111/bij.12915
Goodall-Copestake, W. P. (2018). nrDNA:mtDNA copy number ratios as a comparative metric for evolutionary and conservation genetics. Heredity (Edinb) 121, 105–111. doi: 10.1038/s41437-018-0088-8
Gradinger, R., and Schnack-Schiel, S. (1998). Potential effect of ice formation on Antarctic pelagic copepods: salinity induced mortality of Calanus propinquus and Metridia gerlachei in comparison to sympagic acoel turbellarians. Polar Biol. 20, 139–142. doi: 10.1007/s003000050288
Grant, S. M., Hill, S. L., Trathan, P. N., and Murphy, E. J. (2013). Ecosystem services of the Southern Ocean: trade-offs in decision-making. Antarct. Sci. 25, 603–617. doi: 10.1017/S0954102013000308
Grant, S. M., Waller, C. L., Morley, S. A., Barnes, D. K. A., Brasier, M. J., Double, M. C., et al. (2021). Local drivers of change in Southern Ocean ecosystems: human activities and policy implications. Front. Ecol. Evol. 9:365. doi: 10.3389/fevo.2021.624518
Groeneveld, J., Berger, U., Henschke, N., Pakhomov, E. A., Reiss, C. S., and Meyer, B. (2020). Blooms of a key grazer in the Southern Ocean–an individual-based model of Salpa thompsoni. Prog. Oceanogr. 185:102339. doi: 10.1016/j.pocean.2020.102339
Groeneveld, J., Johst, K., Kawaguchi, S., Meyer, B., Teschke, M., and Grimm, V. (2015). How biological clocks and changing environmental conditions determine local population growth and species distribution in Antarctic krill (Euphausia superba): a conceptual model. Ecol. Modell. 303, 78–86. doi: 10.1016/j.ecolmodel.2015.02.009
Gruber, N., Boyd, P. W., Frölicher, T. L., and Vogt, M. (2021). Biogeochemical extremes and compound events in the ocean. Nature 600, 395–407. doi: 10.1038/s41586-021-03981-7
Guglielmo, L., Donato, P., Zagami, G., and Granata, A. (2009). Spatio-temporal distribution and abundance of Euphausia crystallorophias in Terra Nova Bay (Ross Sea, Antarctica) during austral summer. Polar Biol. 32, 347–367. doi: 10.1007/s00300-008-0546-5
Gutt, J., Bertler, N., Bracegirdle, T. J., Buschmann, A., Comiso, J., Hosie, G., et al. (2015). The Southern Ocean ecosystem under multiple climate change stresses–an integrated circumpolar assessment. Glob. Change Biol. 21, 1434–1453. doi: 10.1111/gcb.12794
Hagen, W., and Kattner, G. (1998). Lipid metabolism of the Antarctic euphausiid Thysanoessa macrura and its ecological implications. Limnol. Oceanogr. 43, 1894–1901. doi: 10.4319/lo.1998.43.8.1894
Hagen, W., Kattner, G., Terbrüggen, A., and Van Vleet, E. (2001). Lipid metabolism of the Antarctic krill Euphausia superba and its ecological implications. Mar. Biol. 139, 95–104. doi: 10.1007/s002270000527
Haraldsson, M., and Siegel, V. (2014). Seasonal distribution and life history of Thysanoessa macrura (Euphausiacea, Crustacea) in high latitude waters of the Lazarev Sea, Antarctica. Mar. Ecol. Prog. Ser. 495, 105–118. doi: 10.3354/meps10553
Hauri, C., Friedrich, T., and Timmermann, A. (2016). Abrupt onset and prolongation of aragonite undersaturation events in the Southern Ocean. Nat. Clim. Change 6, 172–176. doi: 10.1038/nclimate2844
Hays, G. C., Richardson, A. J., and Robinson, C. (2005). Climate change and marine plankton. Trends Ecol. Evol. 20, 337–344. doi: 10.1016/j.tree.2005.03.004
Helaouët, P., and Beaugrand, G. (2007). Macroecology of Calanus finmarchicus and C. helgolandicus in the North Atlantic Ocean and adjacent seas. Mar. Ecol. Prog. Ser. 345, 147–165. doi: 10.3354/meps06775
Hempel, I., and Marschoff, E. (1980). Euphausiid larvae in the Atlantic sector of the Southern Ocean. Meeresforschung 28, 32–47.
Henley, S. F., Cavan, E. L., Fawcett, S. E., Kerr, R., Monteiro, T., Sherrell, R. M., et al. (2020). Changing biogeochemistry of the Southern Ocean and its ecosystem implications. Front. Mar. Sci. 7:581. doi: 10.3389/fmars.2020.00581
Henley, S. F., Schofield, O. M., Hendry, K. R., Schloss, I. R., Steinberg, D. K., Moffat, C., et al. (2019). Variability and change in the west Antarctic Peninsula marine system: research priorities and opportunities. Prog. Oceanogr. 173, 208–237. doi: 10.1016/j.pocean.2019.03.003
Henschke, N., Blain, S., Cherel, Y., Cotte, C., Espinasse, B., Hunt, B. P. V., et al. (2021). Population demographics and growth rate of Salpa thompsoni on the Kerguelen Plateau. J. Mar. Syst. 214:103489. doi: 10.1016/j.jmarsys.2020.103489
Henschke, N., Everett, J. D., Richardson, A. J., and Suthers, I. M. (2016). Rethinking the role of salps in the ocean. Trends Ecol. Evol. 31, 720–733. doi: 10.1016/j.tree.2016.06.007
Henschke, N., and Pakhomov, E. A. (2019). Latitudinal variations in Salpa thompsoni reproductive fitness. Limnol. Oceanogr. 64, 575–584. doi: 10.1002/lno.11061
Henschke, N., Pakhomov, E. A., Groeneveld, J., and Meyer, B. (2018). Modelling the life cycle of Salpa thompsoni. Ecol. Modell. 387, 17–26. doi: 10.1016/j.ecolmodel.2018.08.017
Hill, S. L., Atkinson, A., Darby, C., and Fielding, S. (2016). Is current management of the Antarctic krill fishery in the Atlantic sector of the Southern Ocean precautionary? CCAMLR Sci. 23, 17–30.
Hill, S. L., Keeble, K., Atkinson, A., and Murphy, E. J. (2012). A foodweb model to explore uncertainties in the South Georgia shelf pelagic ecosystem. Deep Sea Res. Part II Top. Stud. Oceanogr. 59, 237–252. doi: 10.1016/j.dsr2.2011.09.001
Hill, S. L., Murphy, E. J., Reid, K., Trathan, P. N., and Constable, A. J. (2006). Modelling Southern Ocean ecosystems: krill, the food-web, and the impacts of harvesting. Biol. Rev. 81, 581–608. doi: 10.1017/S1464793106007123
Hill, S. L., Phillips, T., and Atkinson, A. (2013). Potential climate change effects on the habitat of Antarctic krill in the Weddell quadrant of the Southern Ocean. PLoS One 8:e72246. doi: 10.1371/journal.pone.0072246
Hill, S. L., Pinkerton, M. H., Ballerini, T., Cavan, E. L., Gurney, L. J., Martins, I., et al. (2021). Robust model-based indicators of regional differences in food-web structure in the Southern Ocean. J. Mar. Syst. 220:103556. doi: 10.1016/j.jmarsys.2021.103556
Hill, S. L., Trathan, P. N., and Agnew, D. J. (2009). The risk to fishery performance associated with spatially resolved management of Antarctic krill (Euphausia superba) harvesting. ICES J. Mar. Sci. 66, 2148–2154. doi: 10.1093/icesjms/fsp172
Hofmann, E. (2016). IMBeR 2016- 2025: Science Plan and Implementation Strategy. Bergen: IMBeR International Project Office.
Hofmann, E. E., and Hüsrevoğlu, Y. S. (2003). A circumpolar modeling study of habitat control of Antarctic krill (Euphausia superba) reproductive success. Deep Sea Res. Part II Top. Stud. Oceanogr. 50, 3121–3142. doi: 10.1016/j.dsr2.2003.07.012
Hofmann, E. E., and Murphy, E. J. (2004). Advection, krill, and Antarctic marine ecosystems. Antarct. Sci. 16, 487–499. doi: 10.1017/s0954102004002275
Hoover, C., Pitcher, T., and Pakhomov, E. (2012). The Antarctic Peninsula marine ecosystem model and simulations 1978-present. Fish. Center Res. Rep. 20, 108–188.
Hopkins, T. (1985). Food web of an Antarctic midwater ecosystem. Mar. Biol. 89, 197–212. doi: 10.1007/BF00392890
Hopkins, T. L., and Torres, J. J. (1989). Midwater food web in the vicinity of a marginal ice zone in the western Weddell Sea. Deep Sea Res. Part A Oceanogr. Res. Pap. 36, 543–560. doi: 10.1016/0198-0149(89)90005-8
Höring, F., Teschke, M., Suberg, L., Kawaguchi, S., and Meyer, B. (2018). Light regime affects the seasonal cycle of Antarctic krill (Euphausia superba): impacts on growth, feeding, lipid metabolism, and maturity. Can. J. Zool. 96, 1203–1214. doi: 10.1139/cjz-2017-0353
Horne, C. R., Hirst, A. G., Atkinson, D., Neves, A., and Kiørboe, T. (2016). A global synthesis of seasonal temperature–size responses in copepods. Glob. Ecol. Biogeogr. 25, 988–999. doi: 10.1111/geb.12460
Hoshijima, U., Wong, J. M., and Hofmann, G. E. (2017). Additive effects of pCO2 and temperature on respiration rates of the Antarctic pteropod Limacina helicina antarctica. Conserv. Physiol. 5, 1–14. doi: 10.1093/conphys/cox064
Hosie, G., Fukuchi, M., and Kawaguchi, S. (2003). Development of the Southern Ocean continuous plankton recorder survey. Prog. Oceanogr. 58, 263–283. doi: 10.1016/j.pocean.2003.08.007
Hosie, G., Mormede, S., Kitchener, J., Takahashi, K., and Raymond, B. (2014). “Near-surface zooplankton communities,” in Biogeographic Atlas of the Southern Ocean, eds C. De Broyer, P. Koubbi, H. J. Griffiths, B. Raymond, A. Van de Putte, B. Danis, et al. (Cambridge: SCAR), 422–430.
Hosie, G. W., Schultz, M. B., Kitchener, J. A., Cochran, T. G., and Richards, K. (2000). Macrozooplankton community structure off East Antarctica (80–150°E) during the Austral summer of 1995/1996. Deep Sea Res. Part II Top. Stud. Oceanogr. 47, 2437–2463. doi: 10.1016/S0967-0645(00)00031-X
Huang, T., Sun, L., Stark, J., Wang, Y., Cheng, Z., Yang, Q., et al. (2011). Relative changes in krill abundance inferred from Antarctic fur seal. PLoS One 6:e27331. doi: 10.1371/journal.pone.0027331
Hunt, B. P., and Hosie, G. W. (2005). Zonal structure of zooplankton communities in the Southern Ocean South of Australia: results from a 2150 km continuous plankton recorder transect. Deep Sea Res. Part I Top. Stud. Oceanogr. 52, 1241–1271. doi: 10.1016/j.dsr.2004.11.019
Hunt, B. P., and Hosie, G. W. (2006). The seasonal succession of zooplankton in the Southern Ocean south of Australia, part I: the seasonal ice zone. Deep Sea Res. Part I Top. Stud. Oceanogr. 53, 1182–1202. doi: 10.1016/j.dsr.2006.05.001
Hunt, B. P. V., Pakhomov, E. A., Hosie, G. W., Siegel, V., Ward, P., and Bernard, K. S. (2008). Pteropods in Southern Ocean ecosystems. Prog. Oceanogr. 78, 193–221. doi: 10.1016/j.pocean.2008.06.001
Hunt, G. L. J., Drinkwater, K. F., Arrigo, K., Berge, J., Daly, K. L., Danielson, S., et al. (2016). Advection in polar and sub-polar environments: impacts on high latitude marine ecosystems. Prog. Oceanogr. 149, 40–81. doi: 10.1016/j.pocean.2016.10.004
Huntley, M. E., Sykes, P. F., and Marin, V. (1989). Biometry and trophodynamics of Salpa thompsoni foxton (Tunicata: Thaliacea) near the Antarctic Peninsula in austral summer, 1983-1984. Polar Biol. 10, 59–70. doi: 10.1007/BF00238291
IPCC (2014). “Climate change 2014: impacts, adaptation, and vulnerability. Part B: regional aspects,” in Contribution of Working Group II to the Fifth Assessment Report of the Intergovernmental Panel on Climate Change, eds V. R. Barros, C. B. Field, D. J. Dokken, M. D. Mastrandrea, K. J. Mach, T. E. Bilir, et al. (Cambridge: Cambridge University Press).
IPCC (2018). “Summary for Policymakers,” in Global Warming of 1.5°C. An IPCC Special Report on the Impacts of Global Warming of 1.5°C Above Pre-Industrial Levels and Related Global Greenhouse gas Emission Pathways, in the Context of Strengthening the Global Response to the Threat of Climate Change, Sustainable Development, and Efforts to Eradicate poverty, eds V. Masson-Delmotte, P. Zhai, D. Roberts, J. Skea, P. R. Shukla, A. Pirani, et al. (Geneva: IPCC).
IPCC (2019). “Summary for policymakers,” in IPCC Special Report on the Ocean and Cryosphere in a Changing Climate, eds H.-O. Pörtner, D. C. Roberts, V. Masson-Delmotte, P. Zhai, M. Tignor, E. Poloczanska, et al. (Geneva: IPCC).
IPCC (2021). “Summary for Policymakers,” in: Climate Change 2021: The Physical Science Basis. Contribution of Working Group I to the Sixth Assessment Report of the Intergovernmental Panel on Climate Change eds V. Masson-Delmotte, P. Zhai, A. Pirani, S. L. Connors, C. Péan, S. Berger, et al. (in press).
Iversen, M. H., Pakhomov, E. A., Hunt, B. P. V., van der Jagt, H., Wolf-Gladrow, D., and Klaas, C. (2017). Sinkers or floaters? Contribution from salp pellets to the export flux during a large bloom event in the Southern Ocean. Deep Sea Res. Part II Top. Stud. Oceanogr. 138, 116–125. doi: 10.1016/j.dsr2.2016.12.004
Jarman, S. N., Elliott, N. G., Nicol, S., and McMinn, A. (2002). Genetic differentiation in the Antarctic coastal krill Euphausia crystallorophias. Heredity 88, 280–287. doi: 10.1038/sj.hdy.6800041
Jarman, S. N., McInnes, J. C., Faux, C., Polanowski, A. M., Marthick, J., Deagle, B. E., et al. (2013). Adelie penguin population diet monitoring by analysis of food DNA in scats. PLoS One 8:e82227. doi: 10.1371/journal.pone.0082227
Jarvis, T., Kelly, N., Kawaguchi, S., van Wijk, E., and Nicol, S. (2010). Acoustic characterisation of the broad-scale distribution and abundance of Antarctic krill (Euphausia superba) off East Antarctica (30-80 E) in January-March 2006. Deep Sea Res. Part II Top. Stud. Oceanogr. 57, 916–933. doi: 10.1016/j.dsr2.2008.06.013
Ji, R., Edwards, M., Mackas, D. L., Runge, J. A., and Thomas, A. C. (2010). Marine plankton phenology and life history in a changing climate: current research and future directions. J. Plankton Res. 32, 1355–1368. doi: 10.1093/plankt/fbq062
Jiang, L.-Q., Feely, R., Carter, B., Greeley, D., Gledhill, D., and Arzayus, K. (2015). Climatological distribution of aragonite saturation state in the global oceans. Glob. Biogeochem. Cycles 29, 1656–1673. doi: 10.1002/2015GB005198
Johnson, K. M., and Hofmann, G. E. (2016). A transcriptome resource for the Antarctic pteropod Limacina helicina antarctica. Mar. Genomics 28, 25–28. doi: 10.1016/j.margen.2016.04.002
Johnson, K. M., Wong, J. M., Hoshijima, U., Sugano, C. S., and Hofmann, G. E. (2019). Seasonal transcriptomes of the Antarctic pteropod, Limacina helicina antarctica. Mar. Environ. Res. 143, 49–59. doi: 10.1016/j.marenvres.2018.10.006
Jónasdóttir, S. H., Visser, A. W., Richardson, K., and Heath, M. R. (2015). Seasonal copepod lipid pump promotes carbon sequestration in the deep North Atlantic. Proc. Natl. Acad. Sci.U.S.A. 112, 12122–12126. doi: 10.1073/pnas.1512110112
Jue, N. K., Batta-Lona, P. G., Trusiak, S., Obergfell, C., Bucklin, A., O’Neill, M. J., et al. (2016). Rapid evolutionary rates and unique genomic signatures discovered in the first reference genome for the Southern Ocean Salp, Salpa thompsoni (Urochordata, Thaliacea). Genome Biol. Evol. 8, 3171–3186. doi: 10.1093/gbe/evw215
Kaufman, D. E., Friedrichs, M. A. M., Smith, W. O. Jr., Hofmann, E. E., Dinniman, M. S., and Hemmings, J. C. P. (2017). Climate change impacts on southern Ross Sea phytoplankton composition, productivity, and export. J. Geophys. Res. Oceans 122, 2339–2359. doi: 10.1002/2016jc012514
Kawaguchi, S. (2016). “Reproduction and larval development in Antarctic krill (Euphausia superba),” in Biology and Ecology of Antarctic Krill Advances in Polar Ecology, ed. V. Siegel (cham: Springer), 225–246. doi: 10.1007/978-3-319-29279-3_6
Kawaguchi, S., Ishida, A., King, R., Raymond, B., Waller, N., Constable, A., et al. (2013). Risk maps for Antarctic krill under projected Southern Ocean acidification. Nat. Clim. Change 3:843. doi: 10.1038/nclimate1937
Kawaguchi, S., Kurihara, H., King, R., Hale, L., Berli, T., Robinson, J. P., et al. (2011). Will krill fare well under Southern Ocean acidification? Biol. Lett. 7, 288–291. doi: 10.1098/rsbl.2010.0777
Kawaguchi, S., and Nicol, S. (2020). Krill fishery. Fish. Aquac. 9:137. doi: 10.1093/oso/9780190865627.003.0006
Kawaguchi, S., Yoshida, T., Finley, L., Cramp, P., and Nicol, S. (2007). The krill maturity cycle: a conceptual model of the seasonal cycle in Antarctic krill. Polar Biol. 30, 689–698. doi: 10.1007/s00300-006-0226-2
Keister, J. E., Bonnet, D., Chiba, S., Johnson, C. L., Mackas, D. L., and Escribano, R. (2012). Zooplankton population connections, community dynamics, and climate variability. ICES J. Mar. Sci. 69, 347–350. doi: 10.1093/icesjms/fss034
Kelly, P., Corney, S. P., Melbourne-Thomas, J., Kawaguchi, S., Bestley, S., Fraser, A., et al. (2020). Salpa thompsoni in the Indian Sector of the Southern Ocean: environmental drivers and life history parameters. Deep Sea Res. Part II Top. Stud. Oceanogr. 174:104789. doi: 10.1016/j.dsr2.2020.104789
Kennicutt, M. C., Bromwich, D., Liggett, D., Njåstad, B., Peck, L., Rintoul, S. R., et al. (2019). Sustained Antarctic research: a 21st century imperative. One Earth 1, 95–113. doi: 10.1016/j.oneear.2019.08.014
Kinzey, D., Watters, G. M., and Reiss, C. S. (2015). Selectivity and two biomass measures in an age-based assessment of Antarctic krill (Euphausia superba). Fish. Res. 168, 72–84. doi: 10.1016/j.fishres.2015.03.023
Kinzey, D., Watters, G. M., and Reiss, C. S. (2018). Parameter estimation using randomized phases in an integrated assessment model for Antarctic krill. PLoS One 13:e0202545. doi: 10.1371/journal.pone.0202545
Kirkwood, J. M. (1996). The developmental rate of Euphausia crystallorophias larvae in Ellis Fjord, Vestfold Hills, Antarctica. Polar Biol. 16, 527–530. doi: 10.1007/BF02329073
Klein, E. S., Hill, S. L., Hinke, J. T., Phillips, T., and Watters, G. M. (2018). Impacts of rising sea temperature on krill increase risks for predators in the Scotia Sea. PLoS One 13:e0191011. doi: 10.1371/journal.pone.0191011
Kohlbach, D., Graeve, M., Lange, B. A., David, C., Schaafsma, F. L., van Franeker, J. A., et al. (2018). Dependency of Antarctic zooplankton species on ice algae-produced carbon suggests a sea ice-driven pelagic ecosystem during winter. Glob. Change Biol. 24, 4667–4681. doi: 10.1111/gcb.14392
Kohlbach, D., Lange, B. A., Graeve, M., Vortkamp, M., and Flores, H. (2019). Varying dependency of Antarctic euphausiids on ice algae-and phytoplankton-derived carbon sources during summer. Mar. Biol. 166:79. doi: 10.1007/s00227-019-3527-z
Kohlbach, D., Lange, B. A., Schaafsma, F. L., David, C., Vortkamp, M., Graeve, M., et al. (2017). Ice algae-produced carbon is critical for overwintering of Antarctic krill Euphausia superba. Front. Mar. Sci. 4:310. doi: 10.3389/fmars.2017.00310
Kohlbach, D., Schmidt, K., Hop, H., Wold, A., Al-Habahbeh, A. K., Belt, S. T., et al. (2021). Winter carnivory and diapause counteract the reliance on ice algae by Barents Sea Zooplankton. Front. Mar. Sci. 8:266. doi: 10.3389/fmars.2021.640050
Krafft, B. A., Macaulay, G. J., Skaret, G., Knutsen, T., Bergstad, O. A., Lowther, A., et al. (2021). Standing stock of Antarctic krill (Euphausia superba Dana, 1850) (Euphausiacea) in the Southwest Atlantic sector of the Southern Ocean, 2018–19. J. Crustac. Biol. 41:3. doi: 10.1093/jcbiol/ruab046
Krüger, L., Huerta, M. F., Santa Cruz, F., and Cárdenas, C. A. (2020). Antarctic krill fishery effects over penguin populations under adverse climate conditions: implications for the management of fishing practices. Ambio 50, 560–571. doi: 10.1007/s13280-020-01386-w
La, H. S., Lee, H., Fielding, S., Kang, D., Ha, H. K., Atkinson, A., et al. (2015). High density of ice krill (Euphausia crystallorophias) in the Amundsen sea coastal polynya, Antarctica. Deep Sea Res. Part I Oceanogr. Res. Pap. 95, 75–84. doi: 10.1016/j.dsr.2014.09.002
Lagabrielle, Y., Goddéris, Y., Donnadieu, Y., Malavieille, J., and Suarez, M. (2009). The tectonic history of Drake Passage and its possible impacts on global climate. Earth Planet. Sci. Lett. 279, 197–211. doi: 10.1016/j.epsl.2008.12.037
Lancraft, T. M., Hopkins, T. L., Torres, J. J., and Donnelly, J. (1991). Oceanic micronektonic/macrozooplanktonic community structure and feeding in ice covered Antarctic waters during the winter (Ameriez 1988). Polar Biol. 11, 157–167. doi: 10.1007/BF00240204
Lancraft, T. M., Torres, J. J., and Hopkins, T. L. (1989). Micronekton and macrozooplankton in the open waters near Antarctic ice edge zones (Ameriez 1983 and 1986). Polar Biol. 9, 225–233. doi: 10.1007/BF00263770
Lascara, C. M., Hofmann, E. E., Ross, R. M., and Quetin, L. B. (1999). Seasonal variability in the distribution of Antarctic krill, Euphausia superba, west of the Antarctic Peninsula. Deep Sea Res. Part I Oceanogr. Res. Pap. 46, 951–984. doi: 10.1016/S0967-0637(98)00099-5
Lavery, T. J., Roudnew, B., Gill, P., Seymour, J., Seuront, L., Johnson, G., et al. (2010). Iron defecation by sperm whales stimulates carbon export in the Southern Ocean. Proc. R. Soc. B Biol. Sci. 277, 3527–3531. doi: 10.1098/rspb.2010.0863
Laws, R. M. (1977). Seals and whales of the Southern Ocean. Philos. Trans. R. Soc. Lond. B Biol. Sci. 279, 81–96. doi: 10.1098/rstb.1977.0073
Le Fèvre, J., Legendre, L., and Rivkin, R. B. (1998). Fluxes of biogenic carbon in the Southern Ocean: roles of large microphagous zooplankton. J. Mar. Syst. 17, 325–345. doi: 10.1016/S0924-7963(98)00047-5
Le Quéré, C., Buitenhuis, E. T., Moriarty, R., Alvain, S., Aumont, O., Bopp, L., et al. (2016). Role of zooplankton dynamics for Southern Ocean phytoplankton biomass and global biogeochemical cycles. Biogeosciences 13, 4111–4133. doi: 10.5194/bg-13-4111-2016
Le Quéré, C., Harrison, S. P., Colin Prentice, I., Buitenhuis, E. T., Aumont, O., Bopp, L., et al. (2005). Ecosystem dynamics based on plankton functional types for global ocean biogeochemistry models. Glob. Change Biol. 11, 2016–2040. doi: 10.1111/j.1365-2486.2005.1004.x
León, P., Bednaršek, N., Walsham, P., Cook, K., Hartman, S. E., Wall-Palmer, D., et al. (2019). Relationship between shell integrity of pelagic gastropods and carbonate chemistry parameters at a Scottish Coastal Observatory monitoring site. ICES J. Mar. Sci. 77, 436–450. doi: 10.1093/icesjms/fsz178
Lischka, S., Büdenbender, J., Boxhammer, T., and Riebesell, U. (2011). Impact of ocean acidification and elevated temperatures on early juveniles of the polar shelled pteropod Limacina helicina: mortality, shell degradation, and shell growth. Biogeosciences 8, 919–932. doi: 10.5194/bg-8-919-2011
Lischka, S., and Riebesell, U. (2012). Synergistic effects of ocean acidification and warming on overwintering pteropods in the Arctic. Glob. Change Biol. 18, 3517–3528. doi: 10.1111/gcb.12020
Liszka, C. M., Robinson, C., Manno, C., Stowasser, G., and Tarling, G. A. (2021). Diel vertical migration of a Southern Ocean euphausiid, Euphausia triacantha, and its metabolic response to consequent short-term temperature changes. Mar. Ecol. Prog. Ser. 660, 37–52. doi: 10.3354/meps13618
Loeb, V., Hofmann, E. E., Klinck, J. M., and Holm-Hansen, O. (2010). Hydrographic control of the marine ecosystem in the South Shetland-Elephant Island and Bransfield Strait region. Deep Sea Res. Part II Top. Stud. Oceanogr. 57, 519–542. doi: 10.1016/j.dsr2.2009.10.004
Loeb, V., Siegel, V., Holm-Hansen, O., Hewitt, R., Fraser, W., Trivelpiece, W., et al. (1997). Effects of sea-ice extent and krill or salp dominance on the Antarctic food web. Nature 387:897. doi: 10.1038/43174
Loeb, V. J., Hofmann, E. E., Klinck, J. M., Holm-Hansen, O., and White, W. B. (2009). ENSO and variability of the Antarctic Peninsula pelagic marine ecosystem. Antarct. Sci. 21, 135–148. doi: 10.1017/S0954102008001636
Loeb, V. J., and Santora, J. A. (2012). Population dynamics of Salpa thompsoni near the Antarctic Peninsula: growth rates and interannual variations in reproductive activity (1993–2009). Prog. Oceanogr. 96, 93–107. doi: 10.1016/j.pocean.2011.11.001
Loeb, V. J., and Santora, J. A. (2013). Pteropods and climate off the Antarctic Peninsula. Prog. Oceanogr. 116, 31–48. doi: 10.1016/j.pocean.2013.05.030
Loeb, V. J., and Santora, J. A. (2015). Climate variability and spatiotemporal dynamics of five Southern Ocean krill species. Prog. Oceanogr. 134, 93–122. doi: 10.1016/j.pocean.2015.01.002
Maas, A. E., Elder, L. E., Dierssen, H. M., and Seibel, B. A. (2011). Metabolic response of Antarctic pteropods (Mollusca: Gastropoda) to food deprivation and regional productivity. Mar. Ecol. Prog. Ser. 441, 129–139. doi: 10.3354/meps09358
Maas, A. E., Lawson, G. L., and Wang, Z. A. J. B. (2016). The metabolic response of thecosome pteropods from the North Atlantic and North Pacific Oceans to high CO2 and low O2. Biogeosciences 13:6191. doi: 10.5194/bg-13-6191-2016
Mackas, D., Greve, W., Edwards, M., Chiba, S., Tadokoro, K., Eloire, D., et al. (2012). Changing zooplankton seasonality in a changing ocean: comparing time series of zooplankton phenology. Prog. Oceanogr. 97, 31–62. doi: 10.1016/j.pocean.2011.11.005
Mackas, D. L., and Galbraith, M. D. (2012). Pteropod time-series from the NE Pacific. ICES J. Mar. Sci. J. Cons. 69, 448–459. doi: 10.1093/icesjms/fsr163
Mackey, A., Atkinson, A., Hill, S., Ward, P., Cunningham, N., Johnston, N., et al. (2012). Antarctic macrozooplankton of the southwest Atlantic sector and Bellingshausen Sea: baseline historical distributions (Discovery Investigations, 1928–1935) related to temperature and food, with projections for subsequent ocean warming. Deep Sea Res. Part II Top. Stud. Oceanogr. 59, 130–146. doi: 10.1016/j.dsr2.2011.08.011
Mackintosh, N. A. (1973). Distribution of post-larval krill in the Antarctic. Discov. Rep. 36, 95–156.
Makarov, R. (1979). Larval distribution and reproductive ecology of Thysanoessa macrura (Crustacea: Euphausiacea) in the Scotia Sea. Mar. Biol. 52, 377–386. doi: 10.1007/BF00389079
Maksym, T. (2019). Arctic and Antarctic Sea Ice Change: contrasts, commonalities, and causes. Ann. Rev. Mar. Sci. 11, 187–213. doi: 10.1146/annurev-marine-010816-060610
Maldonado, M. T., Surma, S., and Pakhomov, E. A. (2016). Southern Ocean biological iron cycling in the pre-whaling and present ecosystems. Philos. Trans. A Math. Phys. Eng. Sci. 374:2081. doi: 10.1098/rsta.2015.0292
Malhi, Y., Franklin, J., Seddon, N., Solan, M., Turner, M. G., Field, C. B., et al. (2020). Climate change and ecosystems: threats, opportunities and solutions. Philos. Trans. R. Soc. B Biol. Sci. 375:20190104. doi: 10.1098/rstb.2019.0104
Manno, C., Bednaršek, N., Tarling, G. A., Peck, V. L., Comeau, S., Adhikari, D., et al. (2017). Shelled pteropods in peril: assessing vulnerability in a high CO2 ocean. Earth Sci. Rev. 169, 132–145. doi: 10.1016/j.earscirev.2017.04.005
Manno, C., Fielding, S., Stowasser, G., Murphy, E., Thorpe, S., and Tarling, G. (2020). Continuous moulting by Antarctic krill drives major pulses of carbon export in the north Scotia Sea, Southern Ocean. Nat. Commun. 11:6051. doi: 10.1038/s41467-020-19956-7
Manno, C., Giglio, F., Stowasser, G., Fielding, S., Enderlein, P., and Tarling, G. A. (2018). Threatened species drive the strength of the carbonate pump in the northern Scotia Sea. Nat. Commun. 9:4592. doi: 10.1038/s41467-018-07088-y
Manno, C., Morata, N., and Primicerio, R. (2012). Limacina retroversa’s response to combined effects of ocean acidification and sea water freshening. Estuar. Coast. Shelf Sci. 113, 163–171. doi: 10.1016/j.ecss.2012.07.019
Manno, C., Peck, V. L., and Tarling, G. A. (2016). Pteropod eggs released at high pCO2 lack resilience to ocean acidification. Sci. Rep. 6:25752. doi: 10.1038/srep25752
Manno, C., Sandrini, S., Tositti, L., and Accornero, A. (2007). First stages of degradation of Limacina helicina shells observed above the aragonite chemical lysocline in Terra Nova Bay (Antarctica). J. Mar. Syst. 68, 91–102. doi: 10.1016/j.jmarsys.2006.11.002
Manno, C., Stowasser, G., Enderlein, P., Fielding, S., and Tarling, G. A. (2015). The contribution of zooplankton faecal pellets to deep-carbon transport in the Scotia Sea (Southern Ocean). Biogeosciences 12, 1955–1965. doi: 10.5194/bg-12-1955-2015
Manno, C., Tirelli, V., Accornero, A., and Fonda Umani, S. (2010). Importance of the contribution of Limacina helicina faecal pellets to the carbon pump in Terra Nova Bay (Antarctica). J. Plankton Res. 32, 145–152. doi: 10.1093/plankt/fbp108
Marr, J. W. S. (1962). The natural history and geography of the Antarctic krill (Euphausia superba Dana). Discov. Rep. 32, 33–464.
Marrari, M., Daly, K. L., Timonin, A., and Semonina, T. (2011). The zooplankton of Marguerite Bay, western Antarctic Peninsula-Part II: vertical distributions and habitat partitioning. Deep Sea Res. Part II Top. Stud. Oceanogr. 13, 1614–1629. doi: 10.1016/j.dsr2.2010.12.006
Marschall, H. P. (1988). The overwintering strategy of Antarctic krill under the pack-ice of the Weddell Sea. Polar Biol. 9, 129–135. doi: 10.1007/BF00442041
Mastrandrea, M. D., Field, C. B., Stocker, T. F., Edenhofer, O., Ebi, K. L., Frame, D. J., et al. (2010). Guidance Note for Lead Authors of the IPCC Fifth Assessment Report on Consistent Treatment of Uncertainties. Geneva: IPCC.
McBride, M. M., Dalpadado, P., Drinkwater, K. F., Godø, O. R., Hobday, A. J., Hollowed, A. B., et al. (2014). Krill, climate, and contrasting future scenarios for Arctic and Antarctic fisheries. ICES J. Mar. Sci. 71, 1934–1955. doi: 10.1093/icesjms/fsu002
McBride, M. M., Schram Stokke, O., Renner, A. H. H., Krafft, B. A., Bergstad, O. A., Biuw, M., et al. (2021). Antarctic krill Euphausia superba: spatial distribution, abundance, and management of fisheries in a changing climate. Mar. Ecol. Prog. Ser. 668, 185–214. doi: 10.3354/meps13705
McCormack, S. A., Melbourne-Thomas, J., Trebilco, R., Griffith, G., Hill, S. L., Hoover, C., et al. (2021). Southern Ocean food web modelling: progress, prognoses, and future priorities for research and policy makers. Front. Ecol. Evol. 9:626. doi: 10.3389/fevo.2021.624763
McGinty, N., Barton, A. D., Record, N. R., Finkel, Z. V., and Irwin, A. J. (2018). Traits structure copepod niches in the North Atlantic and Southern Ocean. Mar. Ecol. Prog. Ser. 601, 109–126. doi: 10.3354/meps12660
McLeod, D. J., Hosie, G. W., Kitchener, J. A., Takahashi, K. T., and Hunt, B. P. V. (2010). Zooplankton Atlas of the Southern Ocean: the SCAR SO-CPR survey (1991–2008). Polar Sci. 4, 353–385. doi: 10.1016/j.polar.2010.03.004
McNeil, B. I., and Matear, R. J. (2008). Southern Ocean acidification: a tipping point at 450-ppm atmospheric CO2. Proc. Natl. Acad. Sci. 105, 18860–18864. doi: 10.1073/pnas.0806318105
Meilland, J., Fabri-Ruiz, S., Koubbi, P., Monaco, C. L., Cotte, C., Hosie, G. W., et al. (2016). Planktonic foraminiferal biogeography in the Indian sector of the Southern Ocean: contribution from CPR data. Deep Sea Res. Part I Oceanogr. Res. Pap. 110, 75–89. doi: 10.1016/j.dsr.2015.12.014
Mekkes, L., Sepúlveda-Rodríguez, G., Bielkinaitë, G., Wall-Palmer, D., Brummer, G.-J. A., Dämmer, L. K., et al. (2021). Effects of ocean acidification on calcification of the Sub-Antarctic Pteropod Limacina retroversa. Front. Mar. Sci. 8:157. doi: 10.3389/fmars.2021.581432
Melbourne-Thomas, J., Corney, S. P., Trebilco, R., Meiners, K. M., Stevens, R. P., Kawaguchi, S., et al. (2016). Under ice habitats for Antarctic krill larvae: could less mean more under climate warming? Geophys. Res. Lett. 43:322. doi: 10.1002/2016gl070846
Meredith, M., Sommerkorn, M., Cassotta, S., Derksen, C., Ekaykin, A., Hollowed, A., et al. (2019). “Special report on the ocean and cryosphere,” in A Changing Climate, eds D. C. Roberts, V. Masson-Delmotte, P. Zhai, M. Tignor, E. Poloczanska, K. Mintenbeck, et al. (Geneva: IPCC).
Meyer, B. (2012). The overwintering of Antarctic krill, Euphausia superba, from an ecophysiological perspective. Polar Biol. 35, 15–37. doi: 10.1007/s00300-011-1120-0
Meyer, B., Atkinson, A., Bernard, K., Brierley, A. S., Driscoll, R. M., Hill, S. L., et al. (2020). Successful ecosystem-based management of Antarctic krill should address uncertainties in krill recruitment, behaviour and ecological adaptation. Commun. Earth Environ. 1:28. doi: 10.1038/s43247-020-00026-1
Meyer, B., Atkinson, A., Stöbing, D., Oettl, B., Hagen, W., and Bathmann, U. (2002). Feeding and energy budgets of Antarctic krill Euphausia superba at the onset of winter—I. Furcilia III larvae. Limnol. Oceanogr. 47, 943–952. doi: 10.4319/lo.2002.47.4.0943
Meyer, B., Auerswald, L., Siegel, V., Spahić, S., Pape, C., Fach, B. A., et al. (2010). Seasonal variation in body composition, metabolic activity, feeding, and growth of adult krill Euphausia superba in the Lazarev Sea. Mar. Ecol. Prog. Ser. 398, 1–18. doi: 10.3354/meps08371
Meyer, B., Freier, U., Grimm, V., Groeneveld, J., Hunt, B. P., Kerwath, S., et al. (2017). The winter pack-ice zone provides a sheltered but food-poor habitat for larval Antarctic krill. Nat. Ecol. Evol. 1:1853. doi: 10.1038/s41559-017-0368-3
Meyer, B., Fuentes, V., Guerra, C., Schmidt, K., Atkinson, A., Spahic, S., et al. (2009). Physiology, growth, and development of larval krill Euphausia superba in autumn and winter in the Lazarev Sea, Antarctica. Limnol. Oceanogr. 54, 1595–1614. doi: 10.4319/lo.2009.54.5.1595
Millennium Ecosystem Assessment (2005). Ecosystems and Human Well-Being: Synthesis. Washington, DC: Island Press.
Moline, M. A., Claustre, H., Frazer, T. K., Schofield, O., and Vernet, M. (2004). Alteration of the food web along the Antarctic Peninsula in response to a regional warming trend. Glob. Change Biol. 10, 1973–1980. doi: 10.1111/j.1365-2486.2004.00825.x
Montes-Hugo, M., Doney, S. C., Ducklow, H. W., Fraser, W., Martinson, D., Stammerjohn, S. E., et al. (2009). Recent changes in phytoplankton communities associated with rapid regional climate change along the western Antarctic Peninsula. Science 323, 1470–1473. doi: 10.1126/science.1164533
Morley, S. A., Abele, D., Barnes, D. K. A., Cárdenas, C. A., Cotté, C., Gutt, J., et al. (2020). Global drivers on Southern Ocean ecosystems: changing physical environments and anthropogenic pressures in an earth system. Front. Mar. Sci. 7:1097. doi: 10.3389/fmars.2020.547188
Mucci, A. (1983). The solubility of calcite and aragonite in seawater at various salinities, temperatures, and one atmosphere total pressure. Am. J. Sci. 283, 780–799. doi: 10.2475/ajs.283.7.780
Murase, H., Tamura, T., Matsuoka, K., Nishiwaki, S., Yasuma, H., Matsukura, R., et al. (2006). Distribution Patterns and Biomasses of Antarctic Krill (Euphausia superba) and Ice Krill (E. crystallorophias) with Referece to Antarctic Minke Whales in the Ross Sea in 2004/05 using Kaiyo Maru-JARPA Joint Survey Data. SC/D06/J24. Cambridge: International Whaling Commission.
Murphy, E., Johnston, N., Corney, S., and Reid, K. (2018). Integrating Climate and Ecosystem Dynamics in the Southern Ocean (ICED) Programme: Report of the ICED-CCAMLR Projections Workshop, 5-7 Apr 2018. SC-CAMLR-XXXVII/BG/16, in: SC-CAMLR-XXXVII. Hobart, TAS: CCAMLR.
Murphy, E., Thorpe, S., Watkins, J., and Hewitt, R. (2004a). Modeling the krill transport pathways in the Scotia Sea: spatial and environmental connections generating the seasonal distribution of krill. Deep Sea Res. Part II Top. Stud. Oceanogr. 51, 1435–1456. doi: 10.1016/S0967-0645(04)00090-6
Murphy, E., Watkins, J., Meredith, M., Ward, P., Trathan, P., and Thorpe, S. (2004b). Southern Antarctic circumpolar current front to the northeast of South Georgia: horizontal advection of krill and its role in the ecosystem. J. Geophys. Res. Oceans 109:C01029. doi: 10.1029/2002JC001522
Murphy, E., Watkins, J., Trathan, P., Reid, K., Meredith, M., Thorpe, S., et al. (2007a). Spatial and temporal operation of the Scotia Sea ecosystem: a review of large-scale links in a krill centred food web. Philos. Trans. R. Soc. Lond. Ser. B Biol. Sci. 362, 113–148. doi: 10.1098/rstb.2006.1957
Murphy, E. J., Trathan, P. N., Watkins, J. L., Reid, K., Meredith, M. P., Forcada, J., et al. (2007b). Climatically driven fluctuations in Southern Ocean ecosystems. Proc. R. Soc. B Biol. Sci. 274, 3057–3067. doi: 10.1098/rspb.2007.1180
Murphy, E. J. (1995). Spatial structure of the Southern Ocean ecosystem: predator-prey linkages in Southern Ocean food webs. J. Anim. Ecol. 63, 333–347. doi: 10.2307/5895
Murphy, E. J., Cavanagh, R. D., Drinkwater, K. F., Grant, S. M., Heymans, J. J., Hofmann, E. E., et al. (2016). Understanding the structure and functioning of polar pelagic ecosystems to predict the impacts of change. Proc. Biol. Sci. 283:1844. doi: 10.1098/rspb.2016.1646
Murphy, E. J., Cavanagh, R. D., Hofmann, E. E., Hill, S. L., Constable, A. J., Costa, D. P., et al. (2012a). Developing integrated models of Southern Ocean food webs: including ecological complexity, accounting for uncertainty and the importance of scale. Prog. Oceanogr. 102, 74–92. doi: 10.1016/j.pocean.2012.03.006
Murphy, E. J., Watkins, J. L., Reid, K., Trathan, P. N., Everson, I., Croxall, J. P., et al. (1998). Interannual variability of the South Georgia marine ecosystem: biological and physical sources of variation in the abundance of krill. Fish. Oceanogr. 7, 381–390. doi: 10.1046/j.1365-2419.1998.00081.x
Murphy, E. J., Watkins, J. L., Trathan, P. N., Reid, K., Meredith, M. P., Hill, S. L., et al. (2012b). “Spatial and temporal operation of the Scotia Sea ecosystem,” in Antarctic Ecosystems, eds A. D. Rogers, N. M. Johnston, E. J. Murphy, and A. Clarke (Chichester: Wiley-Blackwell), 160–212. doi: 10.1002/9781444347241.ch6
Murphy, E. J., Cavanagh, R. D., Johnston, N. M., Reid, K., and Hofmann, E. E. (2008). “Integrating climate and ecosytem dynamics in the Southen Ocean (ICED): a circumpolar ecosystem programme,” in Global Ocean Ecosystem Dynamics GLOBEC Report No. 26. Integrated Marine Biogeochemistry and Ecosystem Research IMBER Report No.2, ed. M. Barange, Globec International Project Office.
Murphy, E. J., Hofmann, E. E., Watkins, J. L., Johnston, N. M., Piñones, A., Ballerini, T., et al. (2013). Comparison of the structure and function of Southern Ocean regional ecosystems: the Antarctic Peninsula and South Georgia. J. Mar. Syst. 109–110, 22–42. doi: 10.1016/j.jmarsys.2012.03.011
Murphy, E. J., Johnston, N. M., Hofmann, E. E., Phillips, R. A., Jackson, J., Constable, A. J., et al. (2021). Global connectivity of Southern Ocean ecosystems. Front. Ecol. Evol. 9:624451. doi: 10.3389/fevo.2021.624451
Murphy, E. J., Thorpe, S. E., Tarling, G. A., Watkins, J. L., Fielding, S., and Underwood, P. (2017). Restricted regions of enhanced growth of Antarctic krill in the circumpolar Southern Ocean. Sci. Rep. 7:6963. doi: 10.1038/s41598-017-07205-9
Nicol, S., Bowie, A., Jarman, S., Lannuzel, D., Meiners, K. M., and van der Merwe, P. (2010). Southern Ocean iron fertilization by baleen whales and Antarctic krill. Fish Fish. 11, 203–209. doi: 10.1111/j.1467-2979.2010.00356.x
Nicol, S., and Foster, J. (2016). “The fishery for Antarctic krill: Its current status and management regime,” in Biology and Ecology of Antarctic krill Advances in Polar Ecology, ed V. Siegel (Switzerland: Springer, International Publishing), 387–421. doi: 10.1007/978-3-319-29279-3_11
Nicol, S., Kitchener, J., King, R., Hosie, G., and William, K. (2000a). Population structure and condition of Antarctic krill (Euphausia superba) off East Antarctica (80–150 E) during the Austral summer of 1995/1996. Deep Sea Res. Part II Top. Stud. Oceanogr. 47, 2489–2517. doi: 10.1016/S0967-0645(00)00033-3
Nicol, S., Pauly, T., Bindoff, N. L., Wright, S., Thiele, D., Hosie, G. W., et al. (2000b). Ocean circulation off east Antarctica affects ecosystem structure and sea-ice extent. Nature 406, 504–507. doi: 10.1038/35020053
Niemi, A., Bednaršek, N., Michel, C., Feely, R. A., Williams, W., Azetsu-Scott, K., et al. (2021). Biological impact of ocean acidification in the Canadian Arctic: widespread severe Pteropod shell dissolution in Amundsen Gulf. Front. Mar. Sci. 8:222. doi: 10.3389/fmars.2021.600184
Nordhausen, W. (1992). Distribution and growth of larval and adult Thysanoessa macrura (Euphausiacea) in the Bransfield Strait Region, Antarctica. Mar. Ecol. Prog. Ser. Oldendorf 83, 185–196. doi: 10.3354/meps083185
Nordhausen, W. (1994). Winter abundance and distribution of Euphausia superba, E. crystallorophias, and Thysanoessa macrura in Gerlache Strait and Crystal Sound, Antarctica. Mar. Ecol. Prog. Ser. 109, 131–131. doi: 10.3354/meps109131
O’Brien, D. (1987). Direct observations of the behavior of Euphausia superba and Euphausia crystallorophias (Crustacea: Euphausiacea) under pack ice during the Antarctic spring of 1985. J. Crustac. Biol. 7, 437–448. doi: 10.2307/1548293
O’Brien, T. D., Lorenzoni, L., Isensee, K., and Valdés, L. (2017). “What are marine ecological time series telling us about the ocean? A status report,” in IOC Technical Series, eds T. D. O’Brien, L. Lorenzoni, K. Isensee, and L. Valdés (Paris: IOC-UNESCO).
Odum, E. P. (1969). The strategy of ecosystem development. Science 164, 262–270. doi: 10.1126/science.164.3877.262
Oliver, T. H., Heard, M. S., Isaac, N. J. B., Roy, D. B., Procter, D., Eigenbrod, F., et al. (2015a). Biodiversity and resilience of ecosystem functions. Trends Ecol. Evol. 30, 673–683. doi: 10.1016/j.tree.2015.08.009
Oliver, T. H., Isaac, N. J. B., August, T. A., Woodcock, B. A., Roy, D. B., and Bullock, J. M. (2015b). Declining resilience of ecosystem functions under biodiversity loss. Nat. Commun. 6:10122. doi: 10.1038/ncomms10122
Ommanney, F. D. (1936). Rhincalanus gigas (Brady) a Copepod of the Southern Macroplankton. Cambridge: University Press.
Ono, A., Moteki, M., Amakasu, K., Toda, R., Horimoto, N., Hirano, D., et al. (2011). Euphausiid community structure and population structure of Euphausia superba off Adélie Land in the Southern Ocean during austral summer 2003, 2005 and 2008. Polar Sci. 5, 146–165. doi: 10.1016/j.polar.2011.04.006
Orr, J. C., Fabry, V. J., Aumont, O., Bopp, L., Doney, S. C., Feely, R. A., et al. (2005). Anthropogenic ocean acidification over the twenty-first century and its impact on calcifying organisms. Nature 437, 681–686. doi: 10.1038/nature04095
Orsi, A. H., Whitworth, T., and Nowlin, W. D. (1995). On the meridional extent and fronts of the Antarctic Circumpolar Current. Deep Sea Res. Part I Oceanogr. Res. Pap. 42, 641–673. doi: 10.1016/0967-0637(95)00021-W
Pakhomov, E., and Froneman, P. (2000). Composition and spatial variability of macroplankton and micronekton within the Antarctic Polar Frontal Zone of the Indian Ocean during Austral autumn 1997. Polar Biol. 23, 410–419. doi: 10.1007/s003000050462
Pakhomov, E., and Froneman, P. (2004). Zooplankton dynamics in the eastern Atlantic sector of the Southern Ocean during the Austral summer 1997/1998—Part 1: community structure. Deep Sea Res. Part II Top. Stud. Oceanogr. 51, 2599–2616. doi: 10.1016/j.dsr2.2000.11.001
Pakhomov, E., and Perissinotto, R. (1996). Antarctic neritic krill Euphausia crystallorophias: spatio-temporal distribution, growth and grazing rates. Deep Sea Res. Part I Oceanogr. Res. Pap. 43, 59–87. doi: 10.1016/0967-0637(95)00094-1
Pakhomov, E., Perissinotto, R., McQuaid, C., and Froneman, P. (2000). Zooplankton structure and grazing in the Atlantic sector of the Southern Ocean in late austral summer 1993: part 1. Ecological zonation. Deep Sea Res. Part I Oceanogr. Res. Pap. 47, 1663–1686. doi: 10.1016/S0967-0637(99)00122-3
Pakhomov, E. A. (1997). Feeding and exploitation of the food supply by demersal fishes in the Antarctic part of the Indian Ocean. J. Icthyol. 37, 360–380.
Pakhomov, E. A. (2000). Demography and life cycle of Antarctic krill, Euphausia superba, in the Indian sector of the Southern Ocean: long-term comparison between coastal and open-ocean regions. Can. J. Fish. Aquat. Sci. 57, 68–90. doi: 10.1139/f00-175
Pakhomov, E. A. (2004). Salp/krill interactions in the eastern Atlantic sector of the Southern Ocean. Deep Sea Res. Part II Top. Stud. Oceanogr. 51, 2645–2660. doi: 10.1016/j.dsr2.2001.03.001
Pakhomov, E. A., Dubischar, C. D., Hunt, B. P. V., Strass, V., Cisewski, B., Siegel, V., et al. (2011). Biology and life cycles of pelagic tunicates in the Lazarev Sea, Southern Ocean. Deep Sea Res. Part II Top. Stud. Oceanogr. 58, 1677–1689. doi: 10.1016/j.dsr2.2010.11.014
Pakhomov, E. A., Froneman, P. W., and Perissinotto, R. (2002). Salp/krill interactions in the Southern Ocean: spatial segregation and implications for the carbon flux. Deep Sea Res. Part II Top. Stud. Oceanogr. 49, 1881–1907. doi: 10.1016/S0967-0645(02)00017-6
Pakhomov, E. A., Henschke, N., Hunt, B. P. V., Stowasser, G., and Cherel, Y. (2019). Utility of salps as a baseline proxy for food web studies. J. Plankton Res. 41, 3–11. doi: 10.1093/plankt/fby051
Pakhomov, E. A., and Hunt, B. P. V. (2017). Trans-Atlantic variability in ecology of the pelagic tunicate Salpa thompsoni near the Antarctic Polar Front. Deep Sea Res. Part II Top. Stud. Oceanogr. 138, 126–140. doi: 10.1016/j.dsr2.2017.03.001
Pakhomov, E. A., and McQuaid, C. D. (1996). Distribution of surface zooplankton and seabirds across the Southern Ocean. Polar Biol. 16, 271–286. doi: 10.1007/s003000050054
Pane, L., Feletti, M., Francomacaro, B., and Mariottini, G. L. (2004). Summer coastal zooplankton biomass and copepod community structure near the Italian Terra Nova Base (Terra Nova Bay, Ross Sea, Antarctica). J. Plankton Res. 26, 1479–1488. doi: 10.1093/plankt/fbh135
Papot, C., Cascella, K., Toullec, J.-Y., and Jollivet, D. (2016). Divergent ecological histories of two sister Antarctic krill species led to contrasted patterns of genetic diversity in their heat-shock protein (hsp70) arsenal. Ecol. Evol. 6, 1555–1575. doi: 10.1002/ece3.1989
Park, Y.-H., Park, T., Kim, T.-W., Lee, S.-H., Hong, C.-S., Lee, J.-H., et al. (2019). Observations of the Antarctic Circumpolar Current over the Udintsev fracture zone, the narrowest choke point in the Southern Ocean. J. Geophys. Res. Oceans 124, 4511–4528. doi: 10.1029/2019JC015024
Parmesan, C., and Yohe, G. (2003). A globally coherent fingerprint of climate change impacts across natural systems. Nature 421:37. doi: 10.1038/nature01286
Pasternak, A. F., and Schnack-Schiel, S. B. (2001). Seasonal feeding patterns of the dominant Antarctic copepods Calanus propinquus and Calanoides acutus in the Weddell Sea. Polar Biol. 24, 771–784. doi: 10.1007/s003000100283
Peck, V. L., Oakes, R. L., Harper, E. M., Manno, C., and Tarling, G. A. (2018). Pteropods counter mechanical damage and dissolution through extensive shell repair. Nat. Commun. 9:264. doi: 10.1038/s41467-017-02692-w
Peck, V. L., Tarling, G. A., Manno, C., and Harper, E. M. (2016a). Reply to comment by Bednarsek, et al. Deep Sea Res. Part II Top. Stud. Oceanogr. 127, 57–59. doi: 10.1016/j.dsr2.2016.03.007
Peck, V. L., Tarling, G. A., Manno, C., Harper, E. M., and Tynan, E. (2016b). Outer organic layer and internal repair mechanism protects pteropod Limacina helicina from ocean acidification. Deep Sea Res. Part II Top. Stud. Oceanogr. 127, 41–52. doi: 10.1016/j.dsr2.2015.12.005
Pecuchet, L., Blanchet, M.-A., Frainer, A., Husson, B., Jørgensen, L. L., Kortsch, S., et al. (2020). Novel feeding interactions amplify the impact of species redistribution on an Arctic food web. Glob. Change Biol. 26, 4894–4906. doi: 10.1111/gcb.15196
Peijnenburg, K. T., and Goetze, E. (2013). High evolutionary potential of marine zooplankton. Ecol. Evol. 3, 2765–2781.
Perissinotto, R., and Pakhomov, E. A. (1997). Feeding association of the copepod Rhincalanus gigas with the tunicate salp Salpa thompsoni in the Southern Ocean. Mar. Biol. 127, 479–483.
Perissinotto, R., and Pakhomov, A. (1998a). The trophic role of the tunicate Salpa thompsoni in the Antarctic marine ecosystem. J. Mar. Syst. 17, 361–374. doi: 10.1016/S0924-7963(98)00049-9
Perissinotto, R., and Pakhomov, E. A. (1998b). Contribution of salps to carbon flux of marginal ice zone of the Lazarev Sea, Southern Ocean. Mar. Biol. 131, 25–32.
Perry, F. A., Atkinson, A., Sailley, S. F., Tarling, G. A., Hill, S. L., Lucas, C. H., et al. (2019). Habitat partitioning in Antarctic krill: spawning hotspots and nursery areas. PLoS One 14:e0219325. doi: 10.1371/journal.pone.0219325
Perry, F. A., Kawaguchi, S., Atkinson, A., Sailley, S. F., Tarling, G. A., Mayor, D. J., et al. (2020). Temperature–induced hatch failure and Nauplii malformation in Antarctic Krill. Front. Mar. Sci. 7:501. doi: 10.3389/fmars.2020.00501
Phleger, C. F., Nelson, M. M., Mooney, B. D., and Nichols, P. D. (2002). Interannual and between species comparison of the lipids, fatty acids and sterols of Antarctic krill from the US AMLR Elephant Island survey area. Comp. Biochem. Physiol. B Biochem. Mol. Biol. 131, 733–747. doi: 10.1016/s1096-4959(02)00021-0
Piccolin, F., Suberg, L., King, R., Kawaguchi, S., Meyer, B., and Teschke, M. (2018). The seasonal metabolic activity cycle of Antarctic Krill (Euphausia superba): evidence for a role of photoperiod in the regulation of endogenous rhythmicity. Front. Physiol. 9:1715. doi: 10.3389/fphys.2018.01715
Pinkerton, M. H., Boyd, P. W., Deppeler, S., Hayward, A., Höfer, J., and Moreau, S. (2021). Evidence for the impact of climate change on primary producers in the Southern Ocean. Front. Ecol. Evol. 9:134. doi: 10.3389/fevo.2021.592027
Pinkerton, M. H., Décima, M., Kitchener, J. A., Takahashi, K. T., Robinson, K. V., Stewart, R., et al. (2020). Zooplankton in the Southern Ocean from the continuous plankton recorder: distributions and long-term change. Deep Sea Res. Part I Oceanogr. Res. Pap. 162:103303. doi: 10.1016/j.dsr.2020.103303
Pinkerton, M. H., Smith, A. N., Raymond, B., Hosie, G. W., Sharp, B., Leathwick, J. R., et al. (2010). Spatial and seasonal distribution of adult Oithona similis in the Southern Ocean: predictions using boosted regression trees. Deep Sea Res. Part I Oceanogr. Res. Pap. 57, 469–485.
Piñones, A., and Fedorov, A. V. (2016). Projected changes of Antarctic krill habitat by the end of the 21st century. Geophys. Res. Lett. 43, 8580–8589.
Piñones, A., Hofmann, E. E., Dinniman, M. S., and Davis, L. B. (2016). Modeling the transport and fate of euphausiids in the Ross Sea. Polar Biol. 39, 177–187.
Quetin, L., Ross, R., and Clarke, A. (1994). “Krill energetics: seasonal and environmental aspects of the physiology of Euphausia superba,” in Southern Ocean ecology: the BIOMASS Perspective, ed. S. El-Sayed (Cambridge: Cambridge University Press).
Quetin, L. B., and Ross, R. M. (1991). Behavioral and physiological characteristics of the Antarctic krill, Euphausia superba. Am. Zool. 31, 49–63.
Quetin, L. B., Ross, R. M., Fritsen, C. H., and Vernet, M. (2007). Ecological responses of Antarctic krill to environmental variability: can we predict the future? Antarct. Sci. 19, 253–266. doi: 10.1017/s0954102007000363
Ratnarajah, L., Bowie, A. R., Lannuzel, D., Meiners, K. M., and Nicol, S. (2014). The biogeochemical role of baleen whales and krill in Southern Ocean nutrient cycling. PLoS One 9:e114067. doi: 10.1371/journal.pone.0114067
Reid, P. C., Colebrook, J. M., Matthews, J. B. L., and Aiken, J. (2003). The continuous plankton recorder: concepts and history, from plankton indicator to undulating recorders. Prog. Oceanogr. 58, 117–173. doi: 10.1016/j.pocean.2003.08.002
Reinke, M. (1987). On the feeding and locomotory physiology of Salpa thompsoni and Salpa fusiformis. Ber. Polarforsch. 36, 1–89.
Reiss, C. S., Cossio, A., Santora, J. A., Dietrich, K. S., Murray, A., Mitchell, B. G., et al. (2017). Overwinter habitat selection by Antarctic krill under varying sea-ice conditions: implications for top predators and fishery management. Mar. Ecol. Prog. Ser. 568, 1–16.
Richardson, A. J. (2008). In hot water: zooplankton and climate change. ICES J. Mar. Sci. 65, 279–295. doi: 10.1093/icesjms/fsn028
Richerson, K., Driscoll, R., and Mangel, M. (2018). Increasing temperature may shift availability of euphausiid prey in the Southern Ocean. Mar. Ecol. Prog. Ser. 588, 59–70.
Richerson, K., Watters, G. M., Santora, J. A., Schroeder, I. D., and Mangel, M. (2015). More than passive drifters: a stochastic dynamic model for the movement of Antarctic krill. Mar. Ecol. Prog. Ser. 529, 35–48.
Rintoul, S. R. (2018). The global influence of localized dynamics in the Southern Ocean. Nature 558, 209–218. doi: 10.1038/s41586-018-0182-3
Roberts, D., Hopcroft, R. R., and Hosie, G. W. (2014). “Southern Ocean pteropods,” in Biogeographic Atlas of the Southern Ocean, eds C. De Broyer, P. Koubbi, H. J. Griffiths, B. Raymond, A. Van de Putte, B. Danis, et al. (Cambridge: SCAR), 276–283.
Rogers, A. D., Frinault, B. A. V., Barnes, D. K. A., Bindoff, N. L., Downie, R., Ducklow, H. W., et al. (2020). Antarctic futures: an assessment of climate-driven changes in ecosystem structure, function, and service provisioning in the Southern Ocean. Ann. Rev. Mar. Sci. 12, 87–120. doi: 10.1146/annurev-marine-010419-011028
Rogers, A. D., Murphy, E. J., Johnston, N. M., and Clarke, A. (2007). Introduction. Antarctic ecology: from genes to ecosystems. Part 2. Evolution, diversity and functional ecology. Philos. Trans. R. Soc. B Biol. Sci. 362, 2187–2189. doi: 10.1098/rstb.2007.2135
Ross, R., Quetin, L., Baker, K., Vernet, M., and Smith, R. (2000). Growth limitation in young Euphusia superba under field conditions. Limnol. Oceanogr. 45, 31–43. doi: 10.4319/lo.2000.45.1.0031
Ross, R. M., and Quetin, L. B. (1989). Energetic cost to develop to the first feeding stage of Euphausia superba Dana and the effect of delays in food availability. J. Exp. Mar. Biol. Ecol. 133, 103–127.
Ross, R. M., Quetin, L. B., Newberger, T., Shaw, C. T., Jones, J. L., Oakes, S. A., et al. (2014). Trends, cycles, interannual variability for three pelagic species west of the Antarctic Peninsula 1993-2008. Mar. Ecol. Prog. Ser. 515, 11–32.
Rowlands, E., Galloway, T., Cole, M., Lewis, C., Peck, V., Thorpe, S., et al. (2021). The effects of combined ocean aidification and nanoplastic exposures on the embryonic development of Antarctic krill. Front. Mar. Sci. 8:1080. doi: 10.3389/fmars.2021.709763
Ruhl, H. A., and Smith, K. L. (2004). Shifts in deep-sea community structure linked to climate and food supply. Science 305, 513–515. doi: 10.1126/science.1099759
Saba, G., Bockus, A., Shaw, C., and Seibel, B. (2021). Combined effects of ocean acidification and elevated temperature on feeding, growth, and physiological processes of Antarctic krill (Euphausia superba). Mar. Ecol. Prog. Ser. 665, 1–18. doi: 10.3354/meps13715
Saba, G. K., Fraser, W. R., Saba, V. S., Iannuzzi, R. A., Coleman, K. E., Doney, S. C., et al. (2014). Winter and spring controls on the summer food web of the coastal West Antarctic Peninsula. Nat. Commun. 5:4318.
Saba, G. K., Schofield, O., Torres, J. J., Ombres, E. H., and Steinberg, D. K. (2012). Increased feeding and nutrient excretion of adult Antarctic krill, Euphausia superba, exposed to enhanced carbon dioxide (CO2). PLoS One 7:e52224. doi: 10.1371/journal.pone.0052224
Saenz, B. T., Ainley, D. G., Daly, K. L., Ballard, G., Conlisk, E., Elrod, M. L., et al. (2020). Drivers of concentrated predation in an Antarctic marginal-ice-zone food web. Sci. Rep. 10:7282. doi: 10.1038/s41598-020-63875-y
Sala, A., Azzali, M., and Russo, A. (2002). Krill of the Ross Sea: distribution, abundance and demography of Euphausia superba and Euphausia crystallorophias during the Italian Antarctic Expedition (January-February 2000). Sci. Mar. 66, 123–133.
Santa Cruz, F., Ernst, B., Arata, J. A., and Parada, C. (2018). Spatial and temporal dynamics of the Antarctic krill fishery in fishing hotspots in the Bransfield Strait and South Shetland Islands. Fish. Res. 208, 157–166.
Saunders, R. A., Tarling, G. A., Hill, S., and Murphy, E. J. (2019). Myctophid fish (family Myctophidae) are central consumers in the food web of the Scotia Sea (Southern Ocean). Front. Mar. Sci. 6:530. doi: 10.3389/fmars.2019.00530
Schaafsma, F. L., Kohlbach, D., David, C., Lange, B. A., Graeve, M., Flores, H., et al. (2017). Spatio-temporal variability in the winter diet of larval and juvenile Antarctic krill, Euphausia superba, in ice-covered waters. Mar. Ecol. Progr. Ser. 580, 101–115.
Schmidt, K., Atkinson, A., Pond, D. W., and Ireland, L. C. (2014). Feeding and overwintering of Antarctic krill across its major habitats: the role of sea ice cover, water depth, and phytoplankton abundance. Limnol. Oceanogr. 59, 17–36.
Schmidt, K., Atkinson, A., Steigenberger, S., Fielding, S., Lindsay, M. C., Pond, D. W., et al. (2011). Seabed foraging by Antarctic krill: implications for stock assessment, bentho-pelagic coupling, and the vertical transfer of iron. Limnol. Oceanogr. 56, 1411–1428.
Schmidt, K., Atkinson, A., Venables, H. J., and Pond, D. W. (2012). Early spawning of Antarctic krill in the Scotia Sea is fuelled by “superfluous” feeding on non-ice associated phytoplankton blooms. Deep Sea Res. Part II Top. Stud. Oceanogr. 59, 159–172.
Schmidt, K., Brown, T. A., Belt, S. T., Ireland, L. C., Taylor, K. W., Thorpe, S. E., et al. (2018). Do pelagic grazers benefit from sea ice? Insights from the Antarctic sea ice proxy IPSO25. Biogeosciences 15, 1987–2006.
Schnack-Schiel, S., and Mizdalski, E. (1994). Seasonal variations in distribution and population structure of Microcalanus pygmaeus and Ctenocalanus citer (Copepoda: Calanoida) in the eastern Weddell Sea, Antarctica. Mar. Biol. 119, 357–366.
Schnack-Schiel, S. B., and Hagen, W. (1995). Life-cycle strategies of Calanoides acutus, Calanus propinquus, and Metridia gerlachei (Copepoda: Calanoida) in the eastern Weddell Sea, Antarctica. ICES J. Mar. Sci. 52, 541–548. doi: 10.1016/1054-3139(95)80068-9
Schnack-Schiel, S. B., and Isla, E. (2005). The role of zooplankton in the pelagic-benthic coupling of the Southern Ocean. Sci. Mar. 69, 39–55. doi: 10.3989/scimar.2005.69s239
Schnack-Schiel, S. B., Thomas, D., Dieckmann, G. S., Eicken, H., Gradinger, R., Spindler, M., et al. (1995). Life cycle strategy of the Antarctic calanoid copepod Stephos longipes. Prog. Oceanogr. 36, 45–75.
Seibel, B. A., and Dierssen, H. M. (2003). Cascading trophic impacts of reduced biomass in the Ross Sea, Antarctica: just the tip of the iceberg? Biol. Bull. 205, 93–97.
Shreeve, R. S., Collins, M. A., Tarling, G. A., Main, C. E., Ward, P., and Johnston, N. M. (2009). Feeding ecology of myctophid fishes in the northern Scotia Sea. Mar. Ecol. Prog. Ser. 386, 221–236. doi: 10.3354/meps08064
Shreeve, R. S., Tarling, G. A., Atkinson, A., Ward, P., Goss, C., and Watkins, J. (2005). Relative production of Calanoides acutus (Copepoda: Calanoida) and Euphausia superba (Antarctic krill) at South Georgia, and its implications at wider scales. Mar. Ecol. Prog. Ser. 298, 229–239.
Shreeve, R. S., Ward, P., and Whitehouse, M. J. (2002). Copepod growth and development around South Georgia: relationships with temperature, food and krill. Mar. Ecol. Prog. Ser. 233, 169–183.
Siegel, V. (1987). Age and growth of Antarctic Euphausiacea (Crustacea) under natural conditions. Mar. Biol. 96, 483–495.
Siegel, V. (1988). “A concept of seasonal variation of krill (Euphausia superba) distribution and abundance West of the Antarctic Peninsula,” in Antarctic Ocean and Resources Variability, ed. D. Sahrhage (Berlin, Heidelberg: Springer-Verlag), 219–230.
Siegel, V. (2000). Krill (Euphausiacea) life history and aspects of population dynamics. Can. J. Fish. Aquat. Sci. 57, 130–150.
Siegel, V., and Watkins, J. L. (2016). “Distribution, biomass and demography of Antarctic krill, Euphausia superba,” in Biology and Ecology of Antarctic Krill Advances in Polar Ecology, ed. V. Siegel (Switzerland: Springer), 21–100.
Siegert, M., Atkinson, A., Banwell, A., Brandon, M., Convey, P., Davies, B., et al. (2019). The Antarctic Peninsula under a 1.5°C global warming scenario. Front. Environ. Sci. 7:102. doi: 10.3389/fenvs.2019.00102
Silk, J. R., Thorpe, S. E., Fielding, S., Murphy, E. J., Trathan, P. N., Watkins, J. L., et al. (2016). Environmental correlates of Antarctic krill distribution in the Scotia Sea and southern Drake Passage. ICES J. Mar. Sci. 73, 2288–2301.
Smetacek, V. (2008). “Are declining krill stocks a result of global warming or of the decimation of the whales,” in Impacts of Global Warming on Polar Systems, ed. C. M. Duarte (Bilbao: Fundación BBVA), 47–83.
Smith, W. O. Jr., Ainley, D. G., Arrigo, K. R., and Dinniman, M. S. (2014). The oceanography and ecology of the Ross Sea. Annu. Rev. Mar. Sci. 6, 469–487.
Stammerjohn, S., Massom, R., Rind, D., and Martinson, D. (2012). Regions of rapid sea ice change: an inter-hemispheric seasonal comparison. Geophys. Res. Lett. 39:6.
Stammerjohn, S. E., Martinson, D., Smith, R., Yuan, X., and Rind, D. (2008). Trends in Antarctic annual sea ice retreat and advance and their relation to El Niño–Southern Oscillation and Southern Annular Mode variability. J. Geophys. Res. Oceans 113:1029.
Staniland, I. J., Boyd, I. L., and Reid, K. (2007). An energy–distance trade-off in a central-place forager, the Antarctic fur seal (Arctocephalus gazella). Mar. Biol. 152, 233–241. doi: 10.1007/s00227-007-0698-9
Steinberg, D. K., Landry, M. R., and Annual, R. (2017). Zooplankton and the ocean carbon cycle. Annu. Rev. Mar. Sci. 9, 413–444.
Steinberg, D. K., Ruck, K. E., Gleiber, M. R., Garzio, L. M., Cope, J. S., Bernard, K. S., et al. (2015). Long-term (1993–2013) changes in macrozooplankton off the Western Antarctic Peninsula. Deep Sea Res. Part I Oceanogr. Res. Pap. 101, 54–70. doi: 10.1016/j.dsr.2015.02.009
Stretch, J. J., Hamner, P. P., Hamner, W. M., Michel, W. C., Cook, J., and Sullivan, C. W. (1988). Foraging behavior of Antarctic krill Euphausia superba on sea ice microalgae. Mar. Ecol. Prog. Ser. 44, 131–139.
Suprenand, P., and Ainsworth, C. (2017). Trophodynamic effects of climate change-induced alterations to primary production along the Western Antarctic Peninsula. Mar. Ecol. Prog. Ser. 569, 37–54. doi: 10.3354/meps12100
Swadling, K., McKinnon, A., De’ath, G., and Gibson, J. (2004). Life cycle plasticity and differential growth and development in marine and lacustrine populations of an Antarctic copepod. Limnol. Oceanogr. 49, 644–655.
Swadling, K. M., Kawaguchi, S., and Hosie, G. W. (2010). Antarctic mesozooplankton community structure during BROKE-West (30 E–80 E), January–February 2006. Deep Sea Res. Part II Top. Stud. Oceanogr. 57, 887–904.
Sylvester, Z. T., Long, M. C., and Brooks, C. M. (2021). Detecting climate signals in Southern Ocean krill growth habitat. Front. Mar. Sci. 8:708. doi: 10.3389/fmars.2021.669508
Takahashi, K., Hosie, G., Cowen, T., Hunt, B., Kitchener, J., McLeod, D., et al. (2021). Report on the Status and Trends of Southern Ocean Zooplankton based on the SCAR Southern Ocean Continuous Plankton Recorder (SO-CPR) Survey. Cambridge: Scientific Committee on Antarctic Research (SCAR).
Takahashi, K. T., Hosie, G. W., McLeod, D. J., and Kitchener, J. A. (2011). Surface zooplankton distribution patterns during austral summer in the Indian sector of the Southern Ocean, south of Australia. Polar Sci. 5, 134–145. doi: 10.1016/j.polar.2011.04.003
Taki, K., and Hayashi, T. (2005). Characteristics of seasonal variation in diurnal vertical migration and aggregation of Antarctic krill (Euphausia superba) in the Scotia Sea, using Japanese fishery data. CCAMlR Sci. 12, 163–172.
Taki, K., Yabuki, T., Noiri, Y., Hayashi, T., and Naganobu, M. (2008). Horizontal and vertical distribution and demography of euphausiids in the Ross Sea and its adjacent waters in 2004/2005. Polar Biol. 31, 1343–1356.
Tanimura, A., Hoshiai, T., and Fukuchi, M. (1996). The life cycle strategy of the ice-associated copepod, Paralabidocera antarctica (Calanoida, Copepoda), at Syowa Station, Antarctica. Antarct. Sci. 8, 257–266.
Tarling, G. A. (2020). Routine metabolism of Antarctic krill (Euphausia superba) in South Georgia waters: absence of metabolic compensation at its range edge. Mar. Biol. 167:108. doi: 10.1007/s00227-020-03714-w
Tarling, G. A., Hill, S. L., Peat, H., Fielding, S., Reiss, C. S., and Atkinson, A. (2016a). Growth and shrinkage in Antarctic krill Euphausia superba is sex-dependent. Mar. Ecol. Prog. Ser. 547, 61–78.
Tarling, G. A., Peck, V., Ward, P., Ensor, N., Achterberg, E., Tynan, E., et al. (2016b). Effects of acute ocean acidification on spatially-diverse polar pelagic foodwebs: insights from on-deck microcosms. Deep Sea Res. Part II Top. Stud. Oceanogr. 127, 75–92.
Tarling, G. A., Klevjer, T., Fielding, S., Watkins, J., Atkinson, A., Murphy, E., et al. (2009). Variability and predictability of Antarctic krill swarm structure. Deep Sea Res. Part I Oceanogr. Res. Pap. 56, 1994–2012. doi: 10.1016/j.dsr.2009.07.004
Tarling, G. A., Shreeve, R. S., Hirst, A. G., Atkinson, A., Pond, D. W., Murphy, E. J., et al. (2006). Natural growth rates in Antarctic krill (Euphausia superba): I. Improving methodology and predicting intermolt period. Limnol. Oceanogr. 51, 959–972.
Tarling, G. A., Ward, P., and Thorpe, S. E. (2018). Spatial distributions of Southern Ocean mesozooplankton communities have been resilient to long-term surface warming. Glob. Chang. Biol. 24, 132–142. doi: 10.1111/gcb.13834
Thabet, A. A., Maas, A. E., Lawson, G. L., and Tarrant, A. M. (2015). Life cycle and early development of the thecosomatous pteropod Limacina retroversa in the Gulf of Maine, including the effect of elevated CO2 levels. Mar. Biol. 162, 2235–2249. doi: 10.1007/s00227-015-2754-1
Thibodeau, P. S., Steinberg, D. K., Stammerjohn, S. E., and Hauri, C. (2019). Environmental controls on pteropod biogeography along the Western Antarctic Peninsula. Limnol. Oceanogr. 64, S240–S256. doi: 10.1002/lno.11041
Thomas, P., and Green, K. (1988). Distribution of Euphausia crystallorophias within Prydz Bay and its importance to the inshore marine ecosystem. Polar Biol. 8, 327–331.
Thorpe, S., Murphy, E., and Watkins, J. (2007). Circumpolar connections between Antarctic krill (Euphausia superba Dana) populations: investigating the roles of ocean and sea ice transport. Deep Sea Res. Part I Oceanogr. Res. Pap. 54, 792–810.
Thorpe, S. E., Tarling, G. A., and Murphy, E. J. (2019). Circumpolar patterns in Antarctic krill larval recruitment: an environmentally driven model. Mar. Ecol. Prog. Ser. 613, 77–96. doi: 10.3354/meps12887
Trathan, P., Priddle, J., Watkins, J., Miller, D., and Murray, A. (1993). Spatial variability of Antarctic krill in relation to mesoscale hydrography. Mar. Ecol. Prog. Ser. 98, 61–71.
Trathan, P. N., and Hill, S. L. (2016). “The importance of krill predation in the Southern Ocean,” in Biology and Ecology of Antarctic krill Advances in Polar Ecology, ed. V. Siegel (cham: Springer), 321–350. doi: 10.1007/978-3-319-29279-3_9
Trebilco, R., Melbourne-Thomas, J., and Constable, A. J. (2020). The policy relevance of Southern Ocean food web structure: Implications of food web change for fisheries, conservation and carbon sequestration. Mar. Policy 115:103832. doi: 10.1016/j.marpol.2020.103832
Trivelpiece, W. Z., Hinke, J. T., Miller, A. K., Reiss, C. S., Trivelpiece, S. G., and Watters, G. M. (2011). Variability in krill biomass links harvesting and climate warming to penguin population changes in Antarctica. Proc. Natl. Acad. Sci. U.S.A. 108, 7625–7628.
Tulloch, V. J. D., Plagányi, ÉE., Brown, C., Richardson, A. J., and Matear, R. J. (2019). Future recovery of baleen whales is imperiled by climate change. Glob.Change Biol. 25, 1263–1281.
Tulloch, V. J. D., Plagányi, ÉE., Matear, R., Brown, C. J., and Richardson, A. J. (2018). Ecosystem modelling to quantify the impact of historical whaling on Southern Hemisphere baleen whales. Fish Fish. 19, 117–137. doi: 10.1111/faf.12241
Turner, J., Guarino, M. V., Arnatt, J., Jena, B., Marshall, G. J., Phillips, T., et al. (2020). Recent decrease of summer sea ice in the Weddell Sea, Antarctica. Geophys. Res. Lett. 47:e2020GL087127. doi: 10.1029/2020GL087127
van der Spoel, S., Dadon, J. R., and Boltovskoy, D. (1999). South Atlantic Zooplankton. Leiden: Backhuys Publishers.
Varpe, Ø (2017). Life history adaptations to seasonality. Integr. Comp. Biol. 57, 943–960. doi: 10.1093/icb/icx123
Veytia, D., Corney, S., Meiners, K. M., Kawaguchi, S., Murphy, E. J., and Bestley, S. (2020). Circumpolar projections of Antarctic krill growth potential. Nat. Clim. Change 10, 568–575. doi: 10.1038/s41558-020-0758-4
von Harbou, L., Dubischar, C. D., Pakhomov, E. A., Hunt, B. P. V., Hagen, W., and Bathmann, U. V. (2011). Salps in the Lazarev Sea, Southern Ocean: I. Feeding dynamics. Mar. Biol. 158, 2009–2026. doi: 10.1007/s00227-011-1709-4
Voronina, N. (1972). The spatial structure of interzonal copepod populations in the Southern Ocean. Mar. Biol. 15, 336–343.
Voronina, N. M. (1998). Comparative abundance and distribution of major filter-feeders in the Antarctic pelagic zone. J. Mar. Syst. 17, 375–390. doi: 10.1016/S0924-7963(98)00050-5
Vuorinen, I., Hänninen, J., Bonsdorff, E., Boormann, B., and Angel, M. V. (1997). Temporal and spatial variation of dominant pelagic Copepoda (Crustacea) in the Weddell Sea (Southern Ocean) 1929 to 1993. Polar Biol. 18, 280–291.
Wallis, J. (2018). A description of the post-naupliar development of Southern Ocean krill (Thysanoessa macrura). Polar Biol. 41, 2399–2407.
Wallis, J. R., Kawaguchi, S., and Swadling, K. M. (2017). Sexual differentiation, gonad maturation, and reproduction of the Southern Ocean euphausiid Thysanoessa macrura (Sars, 1883) (Crustacea: Euphausiacea). J. Crustac.Biol. 38, 107–118. doi: 10.1093/jcbiol/rux091
Wallis, J. R., Maschette, D., Wotherspoon, S., Kawaguchi, S., and Swadling, K. M. (2020). Thysanoessa macrura in the southern Kerguelen region: population dynamics and biomass. Deep Sea Res. Part II Top. Stud. Oceanogr. 174:104719. doi: 10.1016/j.dsr2.2019.104719
Wallis, J. R., Melvin, J. E., King, R., and Kawaguchi, S. (2019). In situ growth rate estimates of Southern Ocean krill, Thysanoessa macrura. Antarct. Sci. 31, 116–122. doi: 10.1017/S0954102019000063
Walsh, J., Reiss, C. S., and Watters, G. M. (2020). Flexibility in Antarctic krill Euphausia superba decouples diet and recruitment from overwinter sea-ice conditions in the northern Antarctic Peninsula. Mar. Ecol. Prog. Ser. 642, 1–19.
Waluda, C. M., Hill, S. L., Peat, H. J., and Trathan, P. N. (2012). Diet variability and reproductive performance of macaroni penguins Eudyptes chrysolophus at Bird Island, South Georgia. Mar. Ecol. Prog. Ser. 466, 261–274.
Ward, P., Atkinson, A., and Tarling, G. (2012). Mesozooplankton community structure and variability in the Scotia Sea: a seasonal comparison. Deep Sea Res. Part II Top. Stud. Oceanogr. 59, 78–92.
Ward, P., and Hirst, A. (2007). Oithona similis in a high latitude ecosystem: abundance, distribution and temperature limitation of fecundity rates in a sac spawning copepod. Mar. Biol. 151, 1099–1110.
Ward, P., Meredith, M. P., Whitehouse, M. J., and Rothery, P. (2008). The summertime plankton community at South Georgia (Southern Ocean): comparing the historical (1926/1927) and modern (post 1995) records. Prog. Oceanogr. 78, 241–256.
Ward, P., and Shreeve, R. (1998). Egg hatching times of Antarctic copepods. Polar Biol. 19, 142–144.
Ward, P., Shreeve, R., Atkinson, A., Korb, B., Whitehouse, M., Thorpe, S., et al. (2006). Plankton community structure and variability in the Scotia Sea: Austral summer 2003. Mar. Ecol. Prog. Ser. 309, 75–91.
Ward, P., Tarling, G. A., and Thorpe, S. E. (2018). Temporal changes in abundances of large calanoid copepods in the Scotia Sea: comparing the 1930s with contemporary times. Polar Biol. 41, 2297–2310.
Watters, G. M., Hill, S., Hinke, J., Matthews, J., and Reid, K. (2013). Decision-making for ecosystem-based management: evaluating options for a krill fishery with an ecosystem dynamics model. Ecol. Appl. 23, 710–725.
Watters, G. M., Hinke, J. T., and Reiss, C. S. (2020). Long-term observations from Antarctica demonstrate that mismatched scales of fisheries management and predator-prey interaction lead to erroneous conclusions about precaution. Sci. Rep. 10, 1–9.
Weldrick, C. K., Trebilco, R., Davies, D. M., and Swadling, K. M. (2019). Trophodynamics of Southern Ocean pteropods on the southern Kerguelen Plateau. Ecol. Evol. 9, 8119–8132. doi: 10.1002/ece3.5380
Wessels, W., Auerswald, L., Pakhomov, E. A., Pakhomova, L. G., Meyer, B., and Michael, L. (2018). “The performance of krill vs. salps to withstand in a warming Southern Ocean,” in Reports on Polar and Marine Research ed. H. Bornemann (Bremerhavn: Alfred Wegener Institute for Polar and Marine Research).
Whitehouse, M., Meredith, M., Rothery, P., Atkinson, A., Ward, P., and Korb, R. (2008). Rapid warming of the ocean around South Georgia, Southern Ocean, during the 20th century: forcings, characteristics and implications for lower trophic levels. Deep Sea Res. Part I Oceanogr. Res. Pap. 55, 1218–1228.
Wiebe, P. H., Ashjian, C. J., Lawson, G. L., Piñones, A., and Copley, N. J. (2011). Horizontal and vertical distribution of euphausiid species on the Western Antarctic Peninsula US GLOBEC Southern Ocean study site. Deep Sea Res. Part II Top. Stud. Oceanogr. 58, 1630–1651.
Wiebe, P. H., Atkinson, A. A., O’Brien, T. D., Thompson, P. A., Hosie, G., Lorenzoni, L., et al. (2017). “Southern Ocean,” in What are Marine Ecological Time Series Telling us About the Ocean? A Status Report, IOC-UNESCO, IOC Technical Series, eds T. D. O’Brien, L. Lorenzoni, K. Isensee, and L. Valdés (Paris: UNESCO).
Wiedenmann, J., Cresswell, K., and Mangel, M. (2008). Temperature-dependent growth of Antarctic krill: predictions for a changing climate from a cohort model. Mar. Ecol. Prog. Ser. 358, 191–202.
Yamamoto-Kawai, M., McLaughlin, F. A., Carmack, E. C., Nishino, S., and Shimada, K. (2009). Aragonite undersaturation in the Arctic Ocean: effects of ocean acidification and sea ice melt. Science 326, 1098–1100. doi: 10.1126/science.1174190
Yang, G., Atkinson, A., Hill, S. L., Guglielmo, L., Granata, A., and Li, C. (2020). Changing circumpolar distributions and isoscapes of Antarctic krill: Indo-Pacific habitat refuges counter long-term degradation of the Atlantic sector. Limnol. Oceanogr. 66, 272–287.
Yang, G., Li, C., and Sun, S. (2011). Inter-annual variation in summer zooplankton community structure in Prydz Bay, Antarctica, from 1999 to 2006. Polar Biol. 34, 921–932.
Yoshida, T., Kawaguchi, S., Meyer, B., Virtue, P., Penschow, J., and Nash, G. (2009). Structural changes in the digestive glands of larval Antarctic krill (Euphausia superba) during starvation. Polar Biol. 32, 503–507. doi: 10.1007/s00300-008-0569-y
Yoshida, T., Toda, T., Hirano, Y., Matsuda, T., and Kawaguchi, S. (2004). Effect of temperature on embryo development time and hatching success of the Antarctic krill Euphausia superba Dana in the laboratory. Mar. Freshw. Behav. Physiol. 37, 137–145. doi: 10.1080/10236240410001705789
Young, E. F., Thorpe, S. E., Banglawala, N., and Murphy, E. J. (2014). Variability in transport pathways on and around the South Georgia shelf, Southern Ocean: implications for recruitment and retention. J. Geophys. Res. Oceans 119, 241–252.
Zerbini, A. N., Adams, G., Best, J., Clapham, P. J., Jackson, J. A., and Punt, A. E. (2019). Assessing the recovery of an Antarctic predator from historical exploitation. R. Soc. Open Sci. 6:190368.
Keywords: zooplankton, ecosystems, Southern Ocean, global change, projections, ecosystem services, management, conservation
Citation: Johnston NM, Murphy EJ, Atkinson A, Constable AJ, Cotté C, Cox M, Daly KL, Driscoll R, Flores H, Halfter S, Henschke N, Hill SL, Höfer J, Hunt BPV, Kawaguchi S, Lindsay D, Liszka C, Loeb V, Manno C, Meyer B, Pakhomov EA, Pinkerton MH, Reiss CS, Richerson K, Smith WO Jr, Steinberg DK, Swadling KM, Tarling GA, Thorpe SE, Veytia D, Ward P, Weldrick CK and Yang G (2022) Status, Change, and Futures of Zooplankton in the Southern Ocean. Front. Ecol. Evol. 9:624692. doi: 10.3389/fevo.2021.624692
Received: 17 November 2020; Accepted: 30 December 2021;
Published: 17 June 2022.
Edited by:
Jess Melbourne-Thomas, Oceans and Atmosphere (CSIRO), AustraliaReviewed by:
Humberto E. González, Universidad Austral de Chile, ChileNina Bednarsek, Southern California Coastal Water Research Project, United States
Copyright © 2022 Johnston, Murphy, Atkinson, Constable, Cotté, Cox, Daly, Driscoll, Flores, Halfter, Henschke, Hill, Höfer, Hunt, Kawaguchi, Lindsay, Liszka, Loeb, Manno, Meyer, Pakhomov, Pinkerton, Reiss, Richerson, Smith, Steinberg, Swadling, Tarling, Thorpe, Veytia, Ward, Weldrick and Yang. This is an open-access article distributed under the terms of the Creative Commons Attribution License (CC BY). The use, distribution or reproduction in other forums is permitted, provided the original author(s) and the copyright owner(s) are credited and that the original publication in this journal is cited, in accordance with accepted academic practice. No use, distribution or reproduction is permitted which does not comply with these terms.
*Correspondence: Nadine M. Johnston, bm1qQGJhcy5hYy51aw==