- 1British Antarctic Survey, Cambridge, United Kingdom
- 2Center for Coastal Physical Oceanography, Old Dominion University, Norfolk, VA, United States
- 3Australian Antarctic Division, Kingston, TAS, Australia
- 4Centre for Marine Socioecology, Institute for Marine and Antarctic Studies, University of Tasmania, Hobart, TAS, Australia
- 5School of GeoSciences, University of Edinburgh, Edinburgh, United Kingdom
- 6CSIRO Oceans and Atmosphere, Hobart, TAS, Australia
- 7Department of Ecology and Evolutionary Biology, Institute of Marine Sciences, University of California, Santa Cruz, Santa Cruz, CA, United States
- 8Institute for Marine and Antarctic Studies, University of Tasmania, Hobart, TAS, Australia
- 9Australian Antarctic Program Partnership, Hobart, TAS, Australia
- 10Department of Marine Science, University of Otago, Dunedin, New Zealand
- 11Escuela de Ciencias del Mar, Pontificia Universidad Católica de Valparaíso, Valparaíso, Chile
- 12Centro de Investigación Dinámica de Ecosistemas Marinos de Altas Latitudes (IDEAL), Valdivia, Chile
- 13Department of Life Sciences, Marine and Environmental Sciences Centre, University of Coimbra, Coimbra, Portugal
Southern Ocean ecosystems are globally important. Processes in the Antarctic atmosphere, cryosphere, and the Southern Ocean directly influence global atmospheric and oceanic systems. Southern Ocean biogeochemistry has also been shown to have global importance. In contrast, ocean ecological processes are often seen as largely separate from the rest of the global system. In this paper, we consider the degree of ecological connectivity at different trophic levels, linking Southern Ocean ecosystems with the global ocean, and their importance not only for the regional ecosystem but also the wider Earth system. We also consider the human system connections, including the role of Southern Ocean ecosystems in supporting society, culture, and economy in many nations, influencing public and political views and hence policy. Rather than Southern Ocean ecosystems being defined by barriers at particular oceanic fronts, ecological changes are gradual due to cross-front exchanges involving oceanographic processes and organism movement. Millions of seabirds and hundreds of thousands of cetaceans move north out of polar waters in the austral autumn interacting in food webs across the Southern Hemisphere, and a few species cross the equator. A number of species migrate into the east and west ocean-basin boundary current and continental shelf regions of the major southern continents. Human travel in and out of the Southern Ocean region includes fisheries, tourism, and scientific vessels in all ocean sectors. These operations arise from many nations, particularly in the Northern Hemisphere, and are important in local communities as well as national economic, scientific, and political activities. As a result of the extensive connectivity, future changes in Southern Ocean ecosystems will have consequences throughout the Earth system, affecting ecosystem services with socio-economic impacts throughout the world. The high level of connectivity also means that changes and policy decisions in marine ecosystems outside the Southern Ocean have consequences for ecosystems south of the Antarctic Polar Front. Knowledge of Southern Ocean ecosystems and their global connectivity is critical for interpreting current change, projecting future change impacts, and identifying integrated strategies for conserving and managing both the Southern Ocean and the broader Earth system.
Introduction
Southern Ocean ecosystems were traditionally considered as largely distinct and separate from ecosystems in the rest of the world’s oceans (Longhurst, 1998; Knox, 2007). This view of a unique isolated ecosystem contrasts with the view of the physical Southern Ocean as the center of the global thermohaline circulation that mediates the exchange of energy and heat between the oceans and influences patterns of circulation and sea level across the planet (Marshall and Speer, 2012; Talley, 2013; Chown et al., 2015). This almost paradoxical view of a highly connected ocean but an isolated ecosystem reflects, in part, the multi-front structure of the upper Southern Ocean. This structure restricts meridional (north-south) exchanges in surface waters, where most life occurs, while there is strong physical connectivity in intermediate and deeper waters through flow along density surfaces. Although the biological isolation of Antarctica has, to some extent, been qualified and its richness highlighted (Clarke and Johnston, 2003; Clarke et al., 2005; Barnes et al., 2006; Chown et al., 2015; Fraser et al., 2018; Chown and Brooks, 2019), the view of a “separate ecosystem” remains pervasive, which constrains approaches to analyses of the structure and function of Southern Ocean ecosystems and their role in the Earth system. This view also influences cultural and socio-economic perceptions, both of which affect the development of conservation and sustainable management measures and assessments of the impacts of change. Climate change is affecting Southern Hemisphere atmosphere and ocean circulation, with associated increases in ocean temperatures and reductions in sea ice, influencing Southern Ocean chemistry (e.g., reductions in pH), and biogeochemistry (e.g., carbon and nutrient budgets) (see Henley et al., 2020; Morley et al., 2020). Simultaneously, some previously exploited whale populations are increasing (Branch et al., 2004; Branch, 2011), and substantial commercial fisheries exist (Cavanagh et al., 2021a, b; Grant et al., 2021). Significant changes are already being observed in Southern Ocean ecosystems, and further perturbations are expected in the coming decades, making the development of an understanding of the impacts of climate change and direct human activities a key research priority (Murphy et al., 2008, 2012; Chown et al., 2012; Murphy and Hofmann, 2012; Constable et al., 2014; Rintoul et al., 2018).
Southern Ocean ecosystems support a range of globally essential ecosystem services, yet the underlying processes involved are poorly understood (Grant et al., 2013; Cavanagh et al., 2021a). The influence of Southern Ocean ecosystems on global ocean biogeochemical processes and carbon budgets is recognized (Sarmiento et al., 2004; Palter et al., 2010; Treguer et al., 2018; Henley et al., 2020), but the scale of influence and the ecological processes involved are less well known (Le Quéré et al., 2016; Treguer et al., 2018). There is evidence of biological exchanges across the Antarctic Polar Front (APF) at all trophic levels (plankton, fish, cephalopods, seabirds, and marine mammals), but the degree of connectivity of Southern Ocean and sub-Antarctic and wider Southern Hemisphere ecosystems has been relatively poorly characterized and quantified (Xavier et al., 1999; Barnes et al., 2006; Bernard et al., 2007; Moon et al., 2017). Views of Southern Ocean ecosystem processes and links with the wider global ocean have generally relied on the zonally (east–west) averaged perspective of Southern Ocean dynamics and north–south exchanges that have dominated analyses of physical dynamics for a number of decades (Turner et al., 2009). Although the importance of local and regional processes in maintaining ecosystem structure and functioning is known, the implications for understanding Southern Ocean scale dynamics and global connectivity (Rintoul, 2018) or implications for conservation and management of living resources (Reid, 2018) has received little consideration. The extent and importance of such ecological connectivity between oceanic ecosystems across the global oceans and the potential for feedback effects has also received little attention in analyses of the impacts of climate change or in the development of Earth system models (IPCC, 2019).
Current national and international bodies involved in fisheries management are generally unable to incorporate ocean scale connections in conservation and management measures. Activities in the Antarctic and the Southern Ocean are regulated under the Antarctic Treaty System (ATS). Development of measures for conservation and management of fisheries within the Southern Ocean is overseen at a circumpolar scale under the ATS by the Commission for Conservation of Antarctic Marine Living Resources (CCAMLR; Press et al., 2019; Reid, 2019). CCAMLR has developed activities with adjacent regional fisheries management bodies, but is yet to engage broadly to influence decisions outside the ATS on matters that affect the Southern Ocean (Anon, 2017). The International Whaling Commission (IWC) and the Agreement on the Conservation of Albatrosses and Petrels (ACAP) also consider Southern Ocean conservation issues within a global remit, while the Convention for the Conservation of Migratory Species (CMS) aims to provide coordinated legal frameworks for conservation of species throughout their migratory range, through the United Nations environmental treaty system. The spread of responsibility for management of living resources in the Southern Ocean and across the Southern Hemisphere in disparate organizations with different remits limits the ability to incorporate ocean scale climate change and human activities as integral inputs to conservation and management.
The rapid changes being observed in Southern Ocean ecosystems and the major changes expected over the next few decades present a critical challenge to the ecosystem-based management approach and make the understanding of the impacts of change a priority (Murphy et al., 2012, 2016; Constable et al., 2014; Reid, 2018). This analysis, a contribution to the Marine Ecosystem Assessment for the Southern Ocean (MEASO), provides an assessment of how the Southern Ocean and associated human activities interact with and have reciprocal dependencies on global ecological and human systems. Here we examine the processes involved in biogeochemical and ecological exchanges between Southern Ocean ecosystems and the wider global ocean along with their importance at regional (circumpolar) and global scales. We note that although the sub-Antarctic Front (SAF) is an important physical structure and the APF an ecological boundary, marking the northern extent of ice-influenced surface waters, there is no fixed definition of the Southern Ocean (Longhurst, 1998; Boltovskoy, 1999; Chapman et al., 2020; Hindell et al., 2020; see Constable et al., this volume, for discussion on the areas being examined in MEASO). We first consider the Southern Ocean’s physical and biogeochemical connectivity with the global ocean, which provides the basic framework for examining ecological connectivity. We then examine ecological connections that depend on the scales of biological movement, from microbes and plankton to highly mobile seabirds and marine mammals, and consider the extent to which these exchanges are important in maintaining populations and local and regional food webs. Lastly, we consider the interactions of human activities and associated impacts in the Southern Ocean, including both regionally-specific and global influences that will be important in conserving and managing the region in the future.
Southern Ocean Ecosystem Connections
Processes Underpinning Connectivity
Two fundamental sets of processes determine the degree of natural ecological connectivity between oceanic ecosystems:
(i) Physical advection processes–operating at different scales, can transport dissolved nutrients and carbon, organic material, and organisms from one ecosystem to another (Mann and Lazier, 2005). This includes transport via ocean currents, eddies, and diffusion or associated with sea ice or in the atmosphere. Atmospheric processes are important in transporting elements into marine ecosystems (e.g., iron or mercury associated with dust deposition, Cossa et al., 2011; Moore et al., 2013) and some microorganisms (Mestre and Höfer, 2021) and in the movement patterns of some seabirds (Weimerskirch et al., 2012).
(ii) Biological processes of movement–operating at different scales, which utilize or can override physical processes over particular scales to allow an organism to achieve spatial displacement from one ecosystem to another or maintain position. Movement processes also transport elements (e.g., macro- and micronutrients), organic material, and organisms between ecosystems (Costa et al., 2012; Shepard et al., 2013; Walther et al., 2015; Hays, 2017).
Physical and Biogeochemical Connections
Physical Connectivity
The general perspective of Southern Ocean connectivity with the global ocean is a two-dimensional zonally-averaged view of meridional exchanges (Figure 1A; Turner et al., 2009). Increased observations and improvements in models over the last decade have enabled the spatial and temporal variability across multiple scales and three-dimensional nature of Southern Ocean connectivity to be better understood (Figure 1 and Supplementary Table 1; Garzoli and Matano, 2011; Marshall and Speer, 2012; Talley, 2013; Frenger et al., 2015; Spence et al., 2017; Tamsitt et al., 2017, 2020; Tanhua et al., 2017; Rintoul, 2018; Foppert et al., 2019; Meijers et al., 2019b; Roach and Speer, 2019; Chapman et al., 2020).
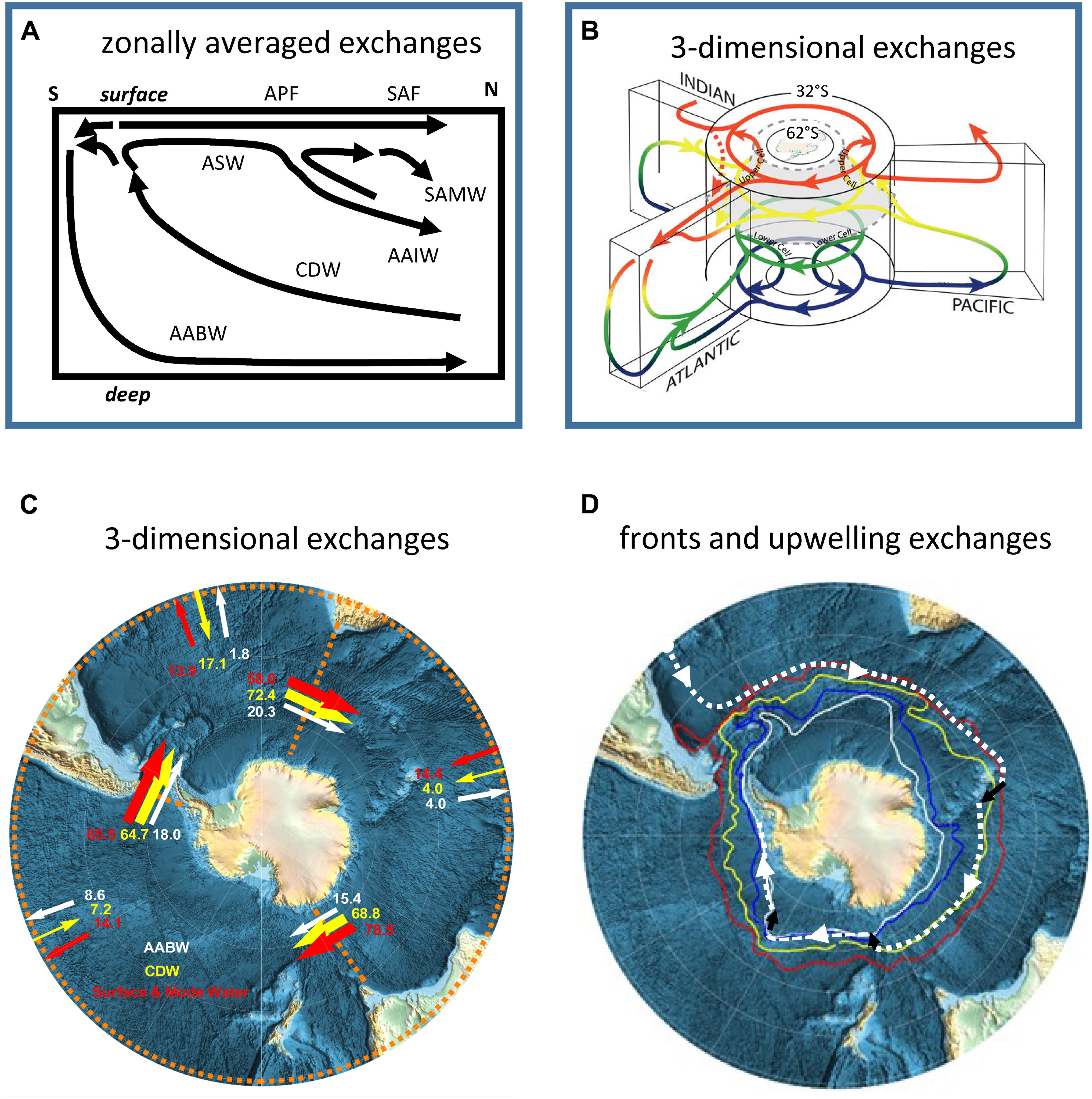
Figure 1. Illustrations of physical connectivity of the Southern Ocean with the wider global ocean. (A) Zonally-averaged vertical view (surface to deep ocean) of the meridional flows of the major water masses into and out of the Southern Ocean (S, south; N, north; APF, Antarctic Polar Front; SAF, Sub-Antarctic Front; AASW, Antarctic Surface Water; CDW, Circumpolar Deep Water; AABW, Antarctic Bottom Water; AAIW, Antarctic Intermediate Water; SAMW, Subantarctic Mode Water). 3-dimensional perspectives of Southern Ocean connectivity: (B) global thermohaline schematic from Lumpkin and Speer (2007). Color indicates approximate density ranges. See their Figure 4 for details;© American Meteorological Society. Used with permission.), (C) model calculations of inter-basin exchanges (depth-integrated residual volume transports, (Sv, where 1 Sv = 106 m3 s– 1) based on Figure 2 of Ferster et al. (2018); orange dotted lines show boundaries over which flows were calculated. Northern boundary = 30°S. (D) general location of the major frontal systems of the Antarctic Circumpolar Current (ACC; SAF = red; APF = yellow; Southern ACC Front, SACCF = blue; Southern Boundary of ACC = SBACC = white), The dotted white line is from the schematic of Tamsitt et al. (2017) showing an idealized particle trajectory illustrating the spiraling pathway of upwelling of southward flowing deep-water in the ACC as it interacts with major topographic features (black arrows; see their Figure 7 for details).
The Southern Ocean is the central connection between the ocean basins in the global overturning circulation (Marshall and Speer, 2012; Talley, 2013), with the extent and intensity of northward or southward exchanges at particular depths being regionally variable around the Southern Ocean (Talley, 2013; Jones et al., 2016; Spence et al., 2017; Rintoul, 2018; Foppert et al., 2019; Meijers et al., 2019a; Figures 1B,C and Supplementary Table 1). There are differential inflows and outputs of Antarctic Bottom Water (AABW), deep water, and intermediate and mode waters in the Pacific, Atlantic and Indian Oceans and between the ocean basins in the Southern Ocean (Ferster et al., 2018; Tamsitt et al., 2020; Yamazaki et al., 2020). The eastern and western boundary regions in the major ocean basins appear to be particularly important in north-south exchange (Jones et al., 2016; Tamsitt et al., 2017). These circulation exchanges also vary inter-annually and seasonally, affecting the volume of water transported as well as heat and salt content (Cerovecki et al., 2019; Meijers et al., 2019a). In contrast to the eastward flowing Antarctic Circumpolar Current (ACC), regions near the continent are characterized by complex flows often dominated by westward flowing currents as part of the Antarctic Coastal Current. The polar gyre systems in the Weddell and Ross Sea regions provide locally complex mixing of water masses, which do not fit a simple two-dimensional zonally averaged view (Rintoul, 2018; MacGilchrist et al., 2019; Yamazaki et al., 2020). Frontal systems comprise a series of dynamic and variable zones (Chapman et al., 2020; Figure 1D). For example, the APF does not have a fixed location and instead varies within a broad zone. Associated with these dynamic frontal zones, there is extensive eddy activity that is most intense in areas of major bathymetric features and moves water across frontal systems in and out of the Southern Ocean (Frenger et al., 2015; Foppert et al., 2017; Tamsitt et al., 2017; Rintoul, 2018; Figure 1D). The formation, direction of rotation, and movement and longevity of the eddies vary in different Southern Ocean regions, while the physical properties and influence also vary with depth and may penetrate to >2,000 m (Frenger et al., 2015). Ekman transport moves water primarily northward (north-eastward) across the ACC and southward in areas closer to the continent, whereas Stokes drift can lead to some southward movement of surface waters and eddy associated transport can move surface waters in both directions (Ito et al., 2010; Holzer and Primeau, 2013; Dufour et al., 2015; Tamsitt et al., 2017; Fraser et al., 2018).
The pathways and time scales by which water and tracers enter and exit the Southern Ocean are a function of the ocean’s full three-dimensional horizontal and vertical structure, thereby providing a very different perspective of connectivity that goes beyond the traditional two-dimensional view of ocean circulation (Figure 1 and Supplementary Table 1). The major physical (oceanic and atmospheric) mechanisms and processes that generate cross-scale exchanges from local to regional, circumpolar and with the global ocean can be represented schematically [Figure 2A; developed from Niiranen et al. (2018), their Figure B1]. In the ocean, these involve spatially (horizontally and vertically) and temporally variable meridional flows associated with major ocean currents and water masses (Figure 2A), while regional and local processes (e.g., eddies, surface flows, and topographic interactions) also result in cross-frontal exchanges (Figures 1, 2A and Supplementary Table 1). Atmospheric processes at different scales further influence exchanges between the Southern Ocean and wider global ocean regions (e.g., local and regional weather patterns, seasonality, and large-scale climatology), affecting the distribution of tracers (e.g., in aerosols and dust) and routes for more efficient and rapid travel by seabirds (Phillips et al., 2007b; Kirby et al., 2008; Egevang et al., 2010; Weimerskirch et al., 2012).
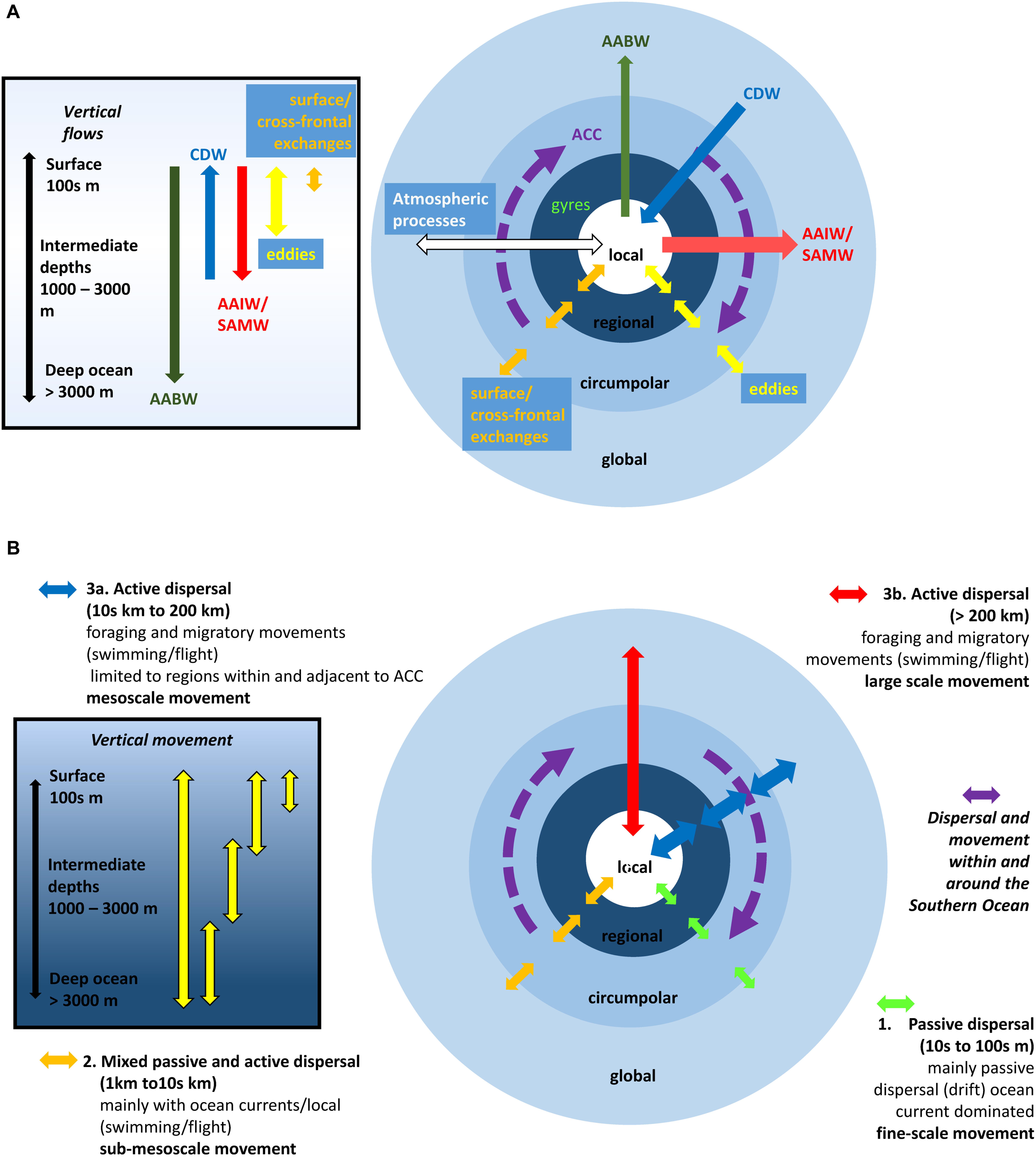
Figure 2. Schematic illustration of (A) the major cross-scale physical exchange processes (see Figure 1 for definitions) and (B) major ecological scales of organism movement and dispersal processes affecting the Southern Ocean. Panels indicate scales of vertical flow and organism movements.
Biogeochemical Connectivity
The central role of the Southern Ocean in the global overturning circulation provides a direct connection with global biogeochemical cycles through the redistribution of biogeochemical properties (nutrients, carbon, and oxygen) amongst the major ocean basins and between the deep ocean, surface ocean, and atmosphere (Henley et al., 2020; see Figure 1B). Arguably, the most important role of the Southern Ocean in the Earth system is its regulation of climate through the exchange of CO2 and other climate-active gases between the ocean and the atmosphere (Sabine et al., 2004; Marinov et al., 2006; Takahashi et al., 2012; Gruber et al., 2019). These exchanges are driven by physical and biological processes and their connectivity (Morrison et al., 2015) with oceanic regions to the north. For example, the Southern Ocean between 30 and 50°S is a major net annual carbon sink and the Southern Ocean as a whole has taken up ∼40% of the total oceanic uptake of anthropogenic CO2 (Orr et al., 2001; Fletcher et al., 2006; DeVries, 2014; Frölicher et al., 2015).
In addition, nutrient export from the Southern Ocean, which is a function of a range of ecosystem processes, exerts an important control on global nutrient distributions, and therefore export production and oxygen and carbon budgets over large oceanic areas north of 30°S (Sarmiento et al., 2004; Marinov et al., 2006; Moore et al., 2018). Surface nutrient concentrations and ratios, particularly in the formation regions of Antarctic Intermediate and Subantarctic Mode Water (AAIW and SAMW), are transported northward, subducted into the ocean interior, and mixed through the thermocline into macronutrient-limited low-latitude surface waters, where they regulate primary production and phytoplankton species composition (Sarmiento et al., 2004; Marinov et al., 2006; Palter et al., 2010; Hauck et al., 2015, 2018). South of the Southern Boundary of the ACC upwelling region (SBACC; Figure 1), water masses move southward and can contribute to AABW formation, thus playing an important role in transferring carbon into the deep ocean (Marinov et al., 2006).
Iron is an important limiting micronutrient in the oceanic “high-nutrient-low-chlorophyll (HNLC)” areas of the Southern Ocean, occurring in low concentrations in surface waters and regulating the growth of autotrophic organisms (de Baar et al., 1990; Moore et al., 2013). Iron availability has a particularly strong impact on the uptake of the major nutrients (N, nitrogen; P, phosphorus; Si, Silicon) by diatoms, with iron limitation increasing the Si/N uptake ratio of diatom-dominated communities (Hutchins and Bruland, 1998; Takeda, 1998; Pondaven et al., 2000). This leads to a reduction in relative concentrations of silicon (as silicic acid) south of the SAF by the diatom-dominated primary production, such that surface waters exported northward from the Southern Ocean are Si-deplete and nitrogen- and phosphorus-rich (Treguer et al., 2018; Henley et al., 2020). This promotes non-diatom phytoplankton growth at lower latitudes, with implications for reduced carbon export compared to fast-sinking diatoms (Buesseler, 1998; Ducklow et al., 2001; Armstrong et al., 2009). Similarly, varying N/P uptake ratios of different phytoplankton species influence the N/P ratio of exported waters, with implications for carbon export at lower latitudes (Arrigo et al., 1999; Weber and Deutsch, 2010; Martiny et al., 2013). The spatial and temporal variability of oceanic connections (see section “Physical Connectivity,” Supplementary Table 1) results in structure and variability in the biogeochemical connections. Combined with the physical variability, this generates inter-annual and seasonal variation in the concentration of nutrients, carbon, and oxygen entering the ocean interior.
Ecological Connectivity
Scales of Ocean Processes and Organism Movement
Evolutionary history is a major determinant of the distribution and ecological connectivity of modern Antarctic fauna. The rich contemporary fauna is considered to be largely endemic with in situ adaptation to local conditions occurring during the period of Southern Ocean cooling as Antarctica separated from the last Gondwanan connections 23–40 mya (Clarke and Johnston, 2003; Clarke and Crame, 2010; Fraser et al., 2012). Most Antarctic groups appear to have survived Pleistocene glacial cycles within the Antarctic region, for example, by sheltering in refugia created by polynyas, deeper waters, or geothermal environments (Convey et al., 2009; Allcock and Strugnell, 2012; Fraser et al., 2012, 2014). There are, however, many mobile seabird and marine mammal species that occur and breed both within and north of the APF (Hindell et al., 2020). The Southern Ocean’s intense seasonality is a fundamental driver of life-cycle evolution and hence also a major influence on ecological connectivity (Clarke and Crame, 2010; Murphy et al., 2016; Varpe, 2017).
Passive and active dispersal, movements and migrations are a fundamental aspect of the dynamics of marine species and ecosystems (Nathan et al., 2008; Walther et al., 2015; Schlägel et al., 2020). Passive dispersal of organisms can reduce local accumulation and competition, moving organisms into regions that are more favorable allowing colonization of new habitats. Active movements and migrations allow organisms to shift habitats to cope with changes in environmental conditions, food availability and mortality, which involve trade-offs in terms of overall reproductive success (Clark and Mangel, 2000; Schlägel et al., 2020). A number of seabird and marine mammal species in the polar regions are seasonally migratory in order to access the optimal habitats for their life cycle (see section “Active Dispersal”). For example, some species of whales target colder areas with high densities of prey in the austral summer, and in the austral winter they occupy warm waters for calving, and possibly also skin regeneration (Pitman et al., 2020).
The major migratory movements of seabirds and whales occur over relatively short periods of a few weeks during the austral spring and autumn. This indicates that connectivity between regions will be particularly intense during these periods with concentrated patterns of movement and aggregation in Southern Ocean regions during spring. By moving and migrating the organisms can enhance reproductive success and maintain higher overall abundance. However, it also means that there are marked differences in local and regional abundance spatially and seasonally, with intense aggregation and hence high regional/local abundance in the summer habitats, but very low abundance in winter, while abundance increases in their winter habitats.
The multi-front structure of the Southern Ocean (Figure 1) limits the exchanges of material and organisms in the upper ocean from the most polar southern regions to areas north of the APF and SAF. However, there is much more extensive cross-frontal exchange than was previously understood, and frontal structures do not extend into the deep ocean (Frenger et al., 2015; Foppert et al., 2017; Chapman et al., 2020). Cross-frontal processes and current fows (Figures 1, 2A) provide mechanisms and routes for transferring material and organisms into and out of the Southern Ocean, resulting in broad latitudinal zones of mixing of upper ocean communities. Dispersal of organisms, or particular life cycles stages, in (or above) the oceans results from a combination of physical and biological process interactions at different scales, including passive and active movements. For organisms, as a broad simplification, the relative importance of advective versus active movement processes is a function of their size. Although there are clear exceptions to the general allometric relationship, small organisms tend to be more strongly influenced by physical advective processes, while larger organisms are more mobile and can overcome advective dispersal over smaller scales (Peters, 1983; Andersen et al., 2016; Hays, 2017). Organisms of every size have evolved adaptations that allow them to utilize spatial heterogeneities at different scales (horizontally and vertically) and temporal variability in advective processes to influence retention and dispersal to optimize access to favorable habitats and/or resources (Mann and Lazier, 2005; Costa et al., 2012; Shepard et al., 2013; Walther et al., 2015; Andersen et al., 2016; Hays, 2017). Although many of the exchanges we consider will be two-directional (north–south) and there is valuable information on dispersal and migratory movements of some species, particularly in terms of seasonal migrations of Southern Ocean predators, there has been little focus on the exchanges at most trophic levels.
We consider three scales of ecological connectivity linking the Southern Ocean and global ocean ecosystems based on the movement of Southern Ocean organisms (Supplementary Table 2 and Figure 2B).
Passive Dispersal
The distribution of epipelagic planktonic organisms is primarily driven by passive dispersal, so cross-frontal exchange processes (e.g., eddy activity and surface currents) can greatly affect where they are found. As a result of the variability of these processes (see section “Physical and Biogeochemical Connections”), there are particular areas around the Southern Ocean where exchanges of planktonic organisms across frontal systems most frequently occur (Tamsitt et al., 2017). In areas between the APF and SAF, subduction processes (see section “Physical and Biogeochemical Connections”), move planktonic organisms northward from mode water formation areas (Jones et al., 2016). The success and survival of these expatriate plankton (e.g., Southern Ocean diatoms) depends on local physical and nutrient conditions (e.g., upwelling, cross-thermocline exchanges and silicic acid and iron concentrations).
Studies examining the partitioning of zooplankton communities over regional and circumpolar scales indicate that changes are coincident with the major frontal zones (Froneman et al., 1995; Pakhomov and McQuaid, 1996; Ward et al., 2003; Johnston et al., unpublished data). The SAF and APF can be strong barriers, and the APF is particularly significant as the main distribution limit for many of the true cold water Antarctic species (Deacon, 1982; Boltovskoy, 1999; Griffiths et al., 2009). However, latitudinal movements and variation of frontal positions and associated meandering and eddy shedding, particularly within the Polar Frontal Zone (PFZ), facilitate cross-frontal exchanges (Lutjeharms and Baker, 1980; Bryden, 1983; Heywood et al., 1985; Lutjeharms et al., 1985; Pakhomov et al., 1997; Chiba et al., 2001; Hunt et al., 2001, 2002; Bernard et al., 2007; Foppert et al., 2017). Eddies that drift some distance from their origin provide an effective means of transporting faunas into different water masses (Atkinson et al., 1990; Bernard et al., 2007) and can enhance zooplankton productivity and abundance, particularly at their peripheries (Bernard et al., 2007; Della Penna et al., 2018). Planktonic larvae of benthic species can cross such frontal zones in eddies (Clarke et al., 2005), and also have new and increasing passive opportunities on floating debris such as plastic (Barnes, 2002; Horton and Barnes, 2020). The result of these exchange processes is that there can be wide transition zones between warm- and cold-water communities where they mix to varying extents (Boltovskoy, 1999; Atkinson and Sinclair, 2000). The different plankton communities across the fronts are thus often represented by changes in the balance of taxa or in the abundance of individual species, rather than fundamentally differing faunas (Siegel and Piatkowski, 1990; Tarling et al., 1995; Ward et al., 2012).
Compared to epipelagic species, many mesopelagic species have wider-ranging distributions that are much less constrained by frontal boundaries (Proud et al., 2017; Sutton et al., 2017). Most mesopelagic species also encounter large temperature changes during their seasonal vertical migrations, compared to the comparatively small temperature change across the APF for example, and hence frontal boundaries do not represent faunal boundaries for these taxa (Proud et al., 2017; Sutton et al., 2017). Biogeographic schemes that work well for epipelagic species are therefore not appropriate for deeper dwelling species and many planktonic mesopelagic taxa (e.g., decapods, ostracods, and siphonophores) have distributions that are wider and more likely to cross frontal boundaries compared to their epipelagic counterparts (Angel and Fasham, 1975; Pugh, 1975, 1999; Fasham and Foxton, 1979; Angel, 1999).
Some benthic and intertidal species are now known to cross Southern Ocean fronts and enter Antarctic waters by rafting with buoyant materials at the very surface of the ocean (Fraser et al., 2018). Organisms floating at the surface are affected by Stokes drift, through the horizontal transport of particles with wind-driven surface waves (Fraser et al., 2018). Rafts of buoyant macroalgae, for example, can–through a combination of mesoscale eddies and storm-associated Stokes drift–cross into Antarctic waters from Subantarctic sources north of the APF, some carrying diverse invertebrate and other passengers (Avila et al., 2020).
Mixed Passive and Active Dispersal
A range of Southern Ocean plankton, fish and cephalopods species have cross-frontal distributions while foraging seabirds and marine mammals move across the APF to breed or feed or in shorter seasonal migrations (Catry et al., 2004; Murphy et al., 2007; Ashford et al., 2008; Della Penna et al., 2015; Hunt et al., 2016; Pistorius et al., 2017; Xavier et al., 2018; Saunders et al., 2019; Queirós et al., 2021).
The strong physical transition between warm and cold waters at the APF strongly limits the potential for southward movement of large migratory epipelagic fish species (Hunt et al., 2016). The fish fauna of the Southern Ocean is largely distinct from that north of the APF. The Antarctic fish fauna is primarily dominated by the notothenioid group (Matschiner et al., 2011), which has specific adaptations (Chen et al., 1997), allowing them to exist in the coldest regions of the Southern Ocean. Several other species of fish and cephalopods do have distributions that cross the APF and/or the SAF with evidence of ontogenic shifts in habitats. A key aspect of a number of these species’ life cycles is the importance of advection and physical processes of aggregation and dispersal in maintaining distributions. Mesoscale oceanographic processes, such as fronts, eddies and jets, can have a profound influence on the distribution, abundance, population dynamics and behavior of pelagic fish (Ashford et al., 2005; Petitgas et al., 2013; Hunt et al., 2016; Netburn and Koslow, 2018). Several demersal/semi-demersal fish species with pelagic eggs and larval stages depend on advection to transport offspring from spawning areas to suitable nursery areas, and zonal advection is important in maintaining connectivity amongst populations of such fishes (Ashford et al., 2012; Damerau et al., 2012; Young et al., 2012; Hop and Gjosaeter, 2013; Vestfals et al., 2014; Hunt et al., 2016; Petrik et al., 2016; Huserbraten et al., 2019; Mori et al., 2019). Although there is generally little exchange between fish fauna in the upper ocean across the APF, some species do move across the APF. For example, the commercially exploited Patagonian toothfish (Dissostichus eleginoides) is a species that shows population connectivity at the ocean basin scale and across the APF (Ashford et al., 2005, 2008, 2012). Spatial movements of organisms associated with ocean currents (and sea ice drift in polar waters) influence their distributions and hence susceptibility to transport into or out of the Southern Ocean. Vertical migrations by a range of zooplankton, fish and cephalopod species (see Caccavo et al., 2021; Johnston et al., unpublished data) involve interactions with water masses that may facilitate north-south exchanges across the APF.
Mesopelagic fish are ubiquitous in the world’s ocean and comprise a substantial source of biomass (Irigoien et al., 2014; Saunders et al., 2019). Of the mesopelagic fish, lanternfish (Family Myctophidae; hereafter myctophids) are the most speciose and biomass-dominant group in most oceanic regions (Gjosaeter and Kawaguchi, 1980; Catul et al., 2011), including the Southern Ocean (Hulley, 1981; Lubimova et al., 1987), where they are important in pelagic food webs and biogeochemical cycles (Pakhomov et al., 1996; Murphy et al., 2007; Saunders et al., 2017, 2019; Caccavo et al., 2021). In parts of the Southern Ocean the majority of myctophid species either do not reproduce or their young do not recruit in waters south of the APF (Saunders et al., 2017), with the core populations of most myctophid species centered at predominantly sub-Antarctic, or temperate latitudes (Hulley, 1981; McGinnis, 1982; Loeb et al., 1993; Christiansen et al., 2018). This suggests that the relatively high biomass of myctophid fish in Antarctic waters appears to be sustained by mass immigration of adults from lower latitudes involving oceanographic and behavioral processes and interactions (Saunders et al., 2017). However, the level of connectivity between Antarctic and sub-Antarctic/temperate systems is also unlikely to be uniform for myctophid fish across the Southern Ocean (Koubbi et al., 2011; McMahon et al., 2019). Evidence that migrant individuals do not return from this southward journey and form sink populations in waters south of the APF was presented by Saunders et al. (2017), further highlighting the importance of such regional connectivity pathways in maintaining their distributions.
Some pelagic cephalopod species have distributions that encompass areas north and south of the APF (Xavier et al., 1999), which in some species involves ontogenetic shifts in habitat. Stable isotope analyses of carbon on different parts of the cephalopod beaks show that numerous species move from warmer waters to colder waters during their development (Queirós et al., 2020), indicating similar ontogenetic shifts in habitat to that observed in the mesopelagic fish community (Saunders et al., 2017). More generally, distribution modeling based on relationships with particular oceanographic conditions and analyses of the diets of predators of squid further indicates cross-frontal distributions (Xavier et al., 2016; Pereira et al., 2017). Analyses of the diets of predators of squid, stable isotope ratios, and species distribution models based on habitat preferences have confirmed these cross-frontal distributions around the Southern Ocean (Guerreiro et al., 2015; Xavier et al., 2016; Pereira et al., 2017).
Benthic species show both passive and active transport of organisms between the Southern Ocean and other oceans, e.g., pedunculate barnacles, “hitching a ride” as commensal epifaunal on birds, seals, and whales (Barnes et al., 2004). Shipping has increased passive riding on active transport into and out of the Southern Ocean (Lewis et al., 2003; Lee and Chown, 2009; McCarthy et al., 2019).
Active Dispersal
The migration of large numbers of seabirds and marine mammals into the Southern Ocean during spring to feed, and in many cases to breed, during the short summer period is part of the classical view of the operation of the ecosystem (Knox, 2007). However, major technological advances since the 1990s have greatly increased knowledge of the at-sea activity patterns and movements of a wide range of species (Phillips et al., 2007a; Hindell et al., 2020). During the austral spring/early summer, breeding seabirds and seals aggregate in large colonies on ice-free land in coastal regions around the continent and on islands further north. When young are being fed, foraging distance is restricted and demand for prey is most intense within a few hundred kilometers of the main breeding sites (Croxall et al., 1985; Murphy, 1995). However, during both incubation and chick-rearing, albatrosses and petrels travel long distances during extended foraging trips, including northward across the APF and SAF (Peron et al., 2010). For example, wandering albatross Diomedea exulans that breed south of the APF at South Georgia mainly forage in shelf areas off South America and in oceanic waters around the subtropical convergence (Xavier et al., 2004; Ceia et al., 2015). Some species breeding on sub-Antarctic islands north of the APF do the opposite, flying south to forage in the Southern Ocean (Peron et al., 2010).
At the conclusion of the breeding season, adults, and fledgling seabirds disperse across the Southern Ocean, keeping north of the advancing seasonal pack ice or migrating across the APF (Phillips et al., 2006; Weimerskirch et al., 2015). There is a general movement of animals which bred south of the APF into oceanic waters to its north during autumn and winter (Croxall et al., 2005; Quillfeldt et al., 2013). This is to avoid the lower productivity, harsher weather, and shorter photoperiods during the austral winter, which limit the foraging of visual predators as well as phytoplankton growth: however, dispersal strategies vary greatly among species, breeding sites, and individuals (Delord et al., 2014; Weimerskirch et al., 2015; Phillips et al., 2016). A number of species migrate into coastal waters off South America, southern Africa, and Australasia, which are areas of high productivity and may also be associated with boundary currents or upwelling zones (Phillips et al., 2005, 2006; Quillfeldt et al., 2013). Other species undertake much longer winter migrations northward toward the equator, and a few even move into the Northern Hemisphere (Kopp et al., 2011). The observed patterns and routes of both foraging movements and long-distance migrations of seabirds are often associated with weather patterns as the birds use the wind for efficient travel between areas (Phillips et al., 2007b; Kirby et al., 2008; Egevang et al., 2010; Weimerskirch et al., 2012).
Most seabirds migrating from islands south of the APF in oceanic or continental shelf waters take varied routes across a broad front, but trans-equatorial migrants to or from the Northern Hemisphere often stay relatively close to continental coasts (Shaffer et al., 2006; Egevang et al., 2010; Kopp et al., 2011). The latter are some of the longest migrations of any organisms on the Earth (e.g., Figure 3A). Short-tailed shearwaters Ardenna tenuirostris tracked from Tasmania moved into the Southern Ocean after breeding, before flying north across the western Pacific Ocean to waters near Japan or further north in the Bering Sea (Carey et al., 2014). The classic example of a long-distance seabird migrant is the Arctic tern Sterna paradisaea, which breeds in Europe and the Arctic, then migrates in its non-breeding season to the Antarctic where it feeds in sea ice environments, thereby experiencing an endless summer (Egevang et al., 2010; Redfern and Bevan, 2020).
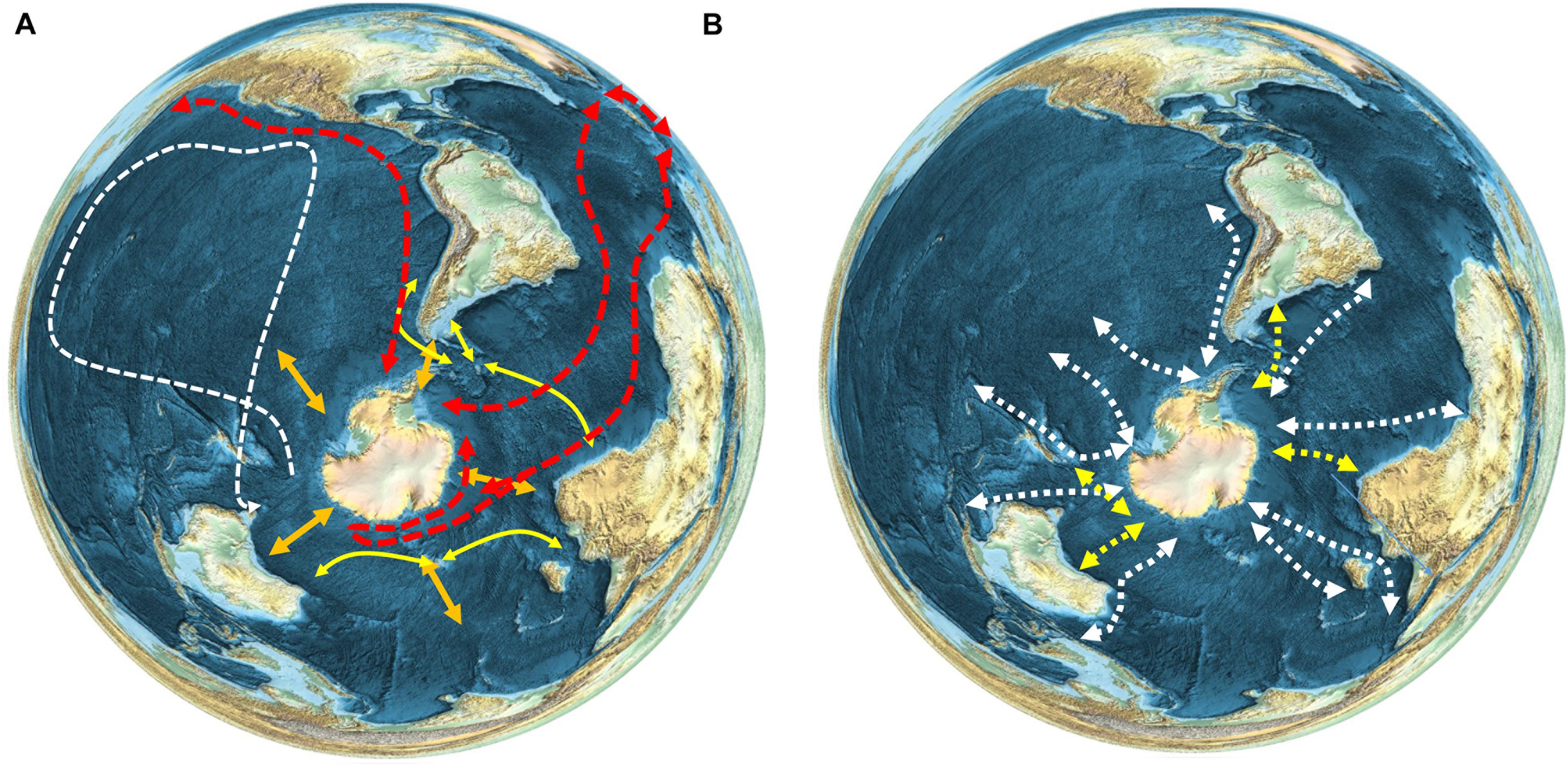
Figure 3. Patterns of movement and migration of seabird and marine mammal species, (A) Seabirds (see text for references). Red–Arctic tern (Sterna paradisaea); White–short-tailed shearwaters (Ardenna tenuirostris); Yellow–black browed albatross (Thalassarche melanophris), wandering albatross (Thalassarche chrysostoma), white-chinned petrel (Procellaria aequinoctialis) and giant petrel (Macronectes giganteus). Orange arrows show the general north-south seasonal migration of seabirds. (B) Whales. White–humpback whale (Megaptera novaeangliae) (Best et al., 1993; Zerbini et al., 2006, 2018; Robbins et al., 2011; Félix and Guzmán, 2014; Fossette et al., 2014; Rosenbaum et al., 2014; Garrigue et al., 2015; Guzman et al., 2015; Riekkola et al., 2018; Bestley et al., 2019; Horton et al., 2020); Yellow – southern right whale (Eubalaena australis) (Best et al., 1993; Zerbini et al., 2018; Mackay et al., 2020; Riekkola et al., 2021).
Cetaceans also migrate south to feed in the Southern Ocean in spring and move northward during autumn to breed at lower latitudes. Due to their size and mobility they can move large distances within the Southern Ocean to target areas of enhanced food availability (Branch et al., 2007; Horton et al., 2017, 2020; Andrews-Goff et al., 2018; Bestley et al., 2019; Figure 3B). It is not known whether their Southern Ocean entry points are diffuse or concentrated, because very few species have been tracked travelling into and out of the polar regions. Humpback whales Megaptera novaeangliae, are the best studied species in this respect; movement studies suggest a preference in some areas for particular migratory routes into the Southern Ocean for this Antarctic krill Euphausia superba feeding species. For example, in the southwest Atlantic region, humpbacks appear to follow a single southbound migratory route, consistent over multiple years and varying environmental conditions (Horton et al., 2020). Humpbacks tracked off east Australia follow multiple distinct migratory routes into the polar regions (Andrews-Goff et al., 2018), while whales migrating from Oceania (South Pacific) have more diffuse southbound movements (Riekkola et al., 2018), suggesting that regional patterns may vary depending on Southern Ocean food predictability. A number of pinniped species, such as Antarctic fur seals Arctocephalus gazella and elephant seals Mirounga leonina, have distributions that cross the PFZ, and a proportion of these populations move further north out of the Southern Ocean during winter (Hindell et al., 2020).
This large number of organisms (fish, cephalopods, seabird, and marine mammals) crossing the APF and PFZ during their life cycle or their seasonal migrations further connects the Southern Ocean to the rest of the globe. These animals constitute a recurrent dispersion vector of microorganisms such as bacteria, archea, fungi, and protists by transporting their attached microbiota in and out of the Southern Ocean [see examples in Mestre and Höfer (2021), and references therein].
Implications of Ecological Connectivity
Seasonal Shifts in Demand for Prey
With better estimates of abundance and much-improved knowledge of seasonal foraging and migration patterns in recent years, it is possible to broadly assess seasonal shifts in demand for prey of key groups of marine predators, including seabirds and cetaceans. We estimated the seasonal shift in and out of the Southern Ocean for these communities and their demand for prey in the Southern Ocean compared with waters north of the APF. Details of the data, methods, and concerns and caveats for calculations of seabird and cetacean demand for prey during and outside of the breeding season are given in the Supplementary Material. Calculation results are presented in Supplementary Tables 3, 4 (seabirds and cetaceans, respectively). We have taken the APF as the major boundary for estimating movements, recognizing that many species will only move into sub-Antarctic regions south of the SAF. The calculations indicate the magnitude of seasonal movement and change in predator abundance, and food requirements in ice influenced polar waters and areas further north. However, these estimates are uncertain, and we regard them as the first set of illustrative estimates of prey demand, which will require further refinement, providing spatially and temporally resolved views of changes in distribution and demand for prey of different predator species.
Seasonal Changes in Prey Demand of Seabirds
We considered just those populations that breed in the Southern Ocean in our consumption estimates, primarily because of uncertainties associated with colony sizes and the proportion of time spent feeding south of the APF by many seabirds that breed north of the APF. However, demand for prey will also be high south of the APF for very abundant species that use these waters during part of their breeding or nonbreeding seasons, for example, short-tailed and sooty shearwaters Ardenna grisea from colonies in Australasia, and Antarctic prions Pachyptila desolata and thin-billed prions P. belcheri from sub-Antarctic islands (Shaffer et al., 2006; Quillfeldt et al., 2013; Carey et al., 2014; Supplementary Material). In total, we estimated that 51 million pairs of seabirds breed annually in the Antarctic region (south of the APF), which translates to an estimated 137 million individuals, including the other life-stages (sabbatical birds, immatures, and juveniles; Figure 4A and Supplementary Table 3). During the breeding season, the at-sea distributions of most species are entirely south of the APF. Of the species which often forage north of the APF during breeding, most use oceanic waters, including grey-headed albatrosses Thalassarche chrysostoma, black-browed albatrosses T. melanophris and cape petrels Daption capense (Phillips et al., 2004). The few exceptions include white-chinned petrel Procellaria aequinoctialis, wandering albatrosses and to a lesser extent the giant petrels Macronectes spp., which frequently travel from South Georgia to the Patagonian Shelf or adjacent shelf-break (Phillips et al., 2006; Jiménez et al., 2016; Granroth-Wilding and Phillips, 2019). By comparison, as most or all individuals of many species are migratory, at-sea distributions during the nonbreeding season are largely or entirely (i.e., >80%) north of the APF. In most cases, this reflects their migration to sub-Antarctic, subtropical, or even tropical oceanic waters (Quillfeldt et al., 2013; Clay et al., 2016, 2018). The exceptions among the most abundant species from South Georgia are white-chinned petrels, which winter on the Patagonian Shelf or the Humboldt Upwelling system off Chile, and black-browed albatrosses, which winter mainly in the Benguela Upwelling system off South Africa and Namibia (Phillips et al., 2005, 2006).
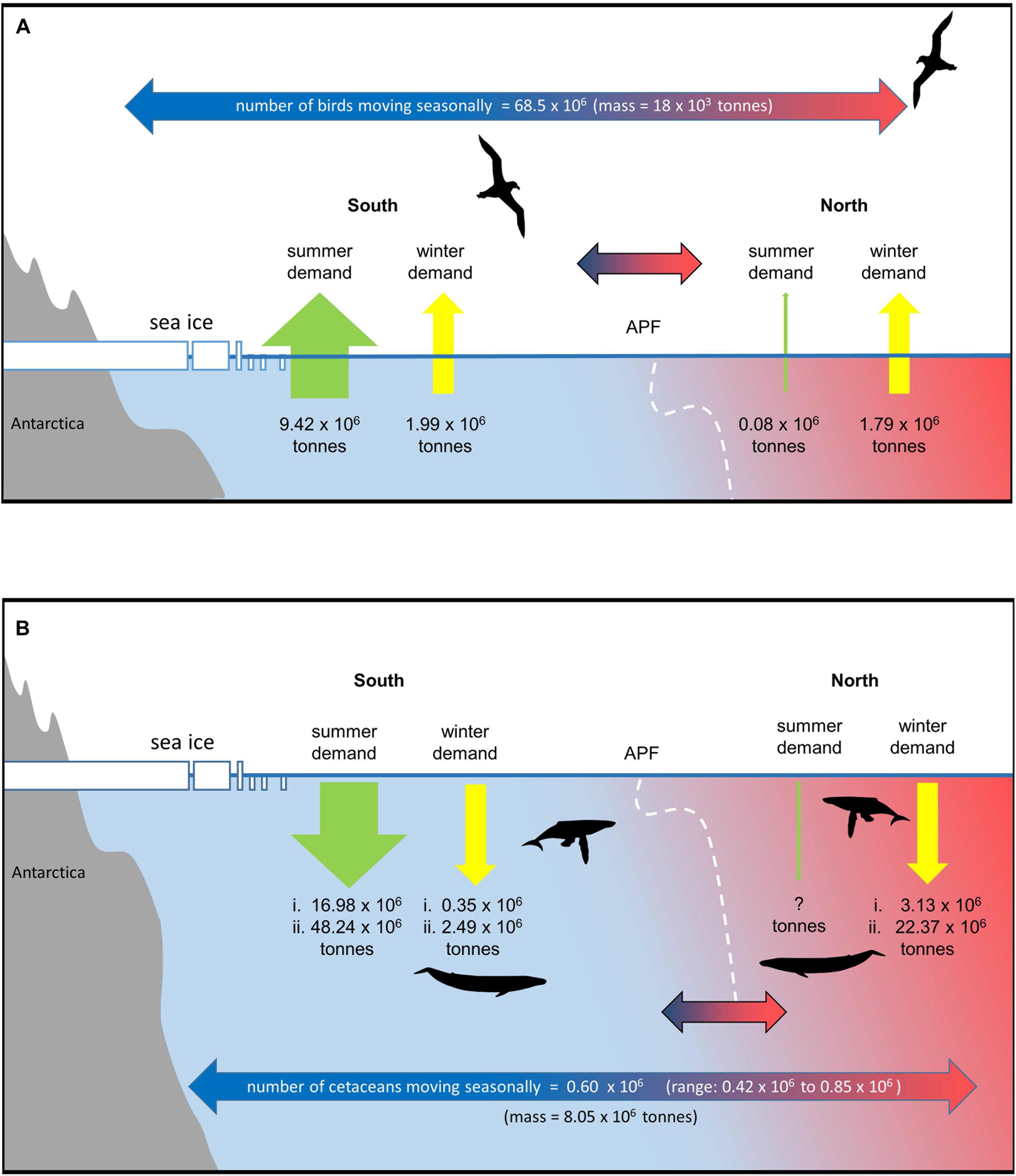
Figure 4. Migration and seasonal changes in prey demand of predators that move seasonally in and out of the Southern Ocean (see text and Supplementary Material for details). The APF is taken as the nominal boundary. Information on population sizes and spatial shifts of many species is very limited and estimates are therefore uncertain and indicative. (A) seabirds, (B) cetaceans (assumes 90% of cetaceans move out of the Southern Ocean in winter (range based on available error estimates). Estimates of summer and winter demand south of the APF and winter demand to the north are based on two estimates of summer consumption rates and two estimates of winter:summer total consumption ratios [(i.) = low summer consumption rate and low winter:summer ratio, (ii.) = high summer consumption rate and high winter:summer ratio]. No estimate is given for summer demand north of the APF.
Taking population sizes, at-sea distributions, and breeding schedules into account 870,000 seabirds from Antarctic colonies feed north of the APF for a total of 159 million bird-days during the breeding season (<0.01% birds in the north). In contrast, around 80 times as many seabirds–68.5 million–from Antarctic colonies move north of the APF for a total of 16,600 million bird-days during the nonbreeding season (51% of birds are in the north in winter; Figure 4A). In terms of biomass, this represents a flux of 18,000 tonnes of seabirds from Antarctic colonies to feed north of the APF in the nonbreeding season, compared with just 1,300 tonnes of seabirds which were feeding there during the breeding season.
The three species contributing to the great majority of this biomass movement in the nonbreeding season are the Antarctic prion, macaroni penguin Eudyptes chrysolophus and white-chinned petrel (8.1, 6.5, and 2.8 tonnes, respectively). Although the total number of seabirds north of the APF during the nonbreeding season is similar to the number that remains to its south (70 vs. 68 million individuals), the latter represents much greater biomass (151 vs. 19 thousand tonnes) of which the top five species (contributing 11–47 thousand tonnes) are all penguins; these are of course much heavier taxa than most of the migrant petrels. Small animals require considerably more energy than large animals for body maintenance per unit mass. Hence, the total energy required from food, and hence the total mass of prey consumed north and south of the APF for the seabirds from Antarctic colonies during the nonbreeding season is similar (1.07 × 1013 kJ and 1.79 million tonnes prey vs. 1.19 × 1013 kJ and 1.99 million tonnes of prey; 47% of the demand is in the north). This is in direct contrast to the breeding season when the relative energy and food intake of Antarctic seabirds south of the APF is 126 times that required to its north (56.5 × 1013 kJ and 9.42 million tonnes of prey vs. 0.4 × 1013 kJ and 74 thousand tonnes of prey; <0.01% in the north; Figure 4A).
Given the locations and sizes of their main colonies, the seabirds from south of the APF which feed to its north during the breeding season are mainly in the Atlantic sector, although smaller numbers of seabirds also feed north of the APF in the Indian Ocean (Phillips et al., 2004, 2006; Wakefield et al., 2011; Granroth-Wilding and Phillips, 2019). The same broad geographical pattern applies during the nonbreeding season, i.e., the largest numbers and biomass of seabirds migrating north of the APF are in the Atlantic sector.
Excepting the south polar skua Stercorarius maccormicki and Wilson’s storm petrel Oceanites oceanicus, the Antarctic seabird populations that move north of the APF remain in the Southern Hemisphere, predominantly in oceanic waters from the sub-Antarctic to the subtropics. In the Atlantic, those in oceanic waters will overlap with the southern portion of at-sea distributions of the huge seabird populations from the South Atlantic Islands. This overlap is greater during the nonbreeding season than the breeding season (Reid et al., 2013; Dias et al., 2017; Ronconi et al., 2018; Jones et al., 2020). Those Antarctic populations that move to a greater or lesser extent close to or onto the Patagonian Shelf or into the Humboldt Current will overlap with huge local breeding populations of seabirds and many long-distance migrants from areas such as New Zealand or the Northern Hemisphere (Spear et al., 2003; Landers et al., 2011; Baylis et al., 2019; Ponchon et al., 2019). In the Indian Ocean, the situation is somewhat different in that the Antarctic seabird populations which feed north of APF overlap very extensively not just during the nonbreeding but also during the breeding season, with the major breeding populations of seabirds on island groups within a few 100 km of the APF (Delord et al., 2014; Reisinger et al., 2015). As such, it is perhaps only the most numerous of the migrant seabirds from the Antarctic region – Antarctic prion and macaroni penguin in oceanic waters, and white-chinned petrel on the Patagonian Shelf–that would have an impact on prey resources north of the APF compared to the demand of local or other migrant seabird species.
Seasonal Changes in Prey Demand of Cetaceans
Given the number, size and consumption rates of Southern Ocean cetaceans, the demand for prey in summer and shifts in winter are potentially very large (Reilly et al., 2004). Estimates of current population numbers and migration patterns of cetaceans are uncertain but there have been significant increases in estimates of abundance over the last 25 years (Jackson et al., 2008, 2016; IWC, 2016). Although uncertain, the available estimates are useful in considering the extent to which the demand for prey shifts seasonally across the APF. We estimate that there are ∼770,000 cetaceans in the Southern Ocean during the summer months, of which ∼667,000 are baleen whales. We assume that 90% of each baleen whale species move northward after summer (Figure 4B and Supplementary Material). Assuming an average body mass equivalent to that of an adult male, this represents a movement of ∼8.05 × 106 tonnes of whale biomass mass each year. As whales live a long time and build up body mass, this means that the carbon may be shifted from areas where it is produced and consumed to often distant regions where it is respired, excreted or lost when whales die (Roman et al., 2014).
For baleen whales we estimate summer consumption of between ∼17 and 48 million tonnes of prey south of the APF (Figure 4B and Supplementary Table 4). This is a higher estimate compared to those in earlier studies, resulting from the larger estimates of population size used in this study (Reilly et al., 2004; Branch, 2007; IWC, 2013, 2016; Trathan and Hill, 2016). Winter demand is highly uncertain. If we assume that 10% of baleen whales stay in the Southern Ocean during winter and continue to feed at summer rates, this results in a prey demand south of the front of between ∼0.35 and 2.5 million tonnes. The proportion of prey consumed outside the summer season is very uncertain; Lockyer (1981) estimated this to be 17%, but reviewing the consumption rate data available for whales, Leaper and Lavigne (2007) suggested that this is likely too low to satisfy the metabolic needs of whales. If the proportion of prey consumed outside of the summer season is 17% or double this value (34%) (Lockyer, 1981; Leaper and Lavigne, 2007; Supplementary Material), estimates of prey demand north of the front range from ∼3 to 22 million tonnes. Although the estimates of winter demand for prey are uncertain, the migration represents a large reduction in prey demand in the south and a very large movement of animal biomass, carbon and nutrients.
Food Web Connectivity
Both passive dispersal processes and active movements of organisms into and out of the Southern Ocean connect food webs in different ecosystems. These processes transfer nutrients, carbon, organic material, and organisms between systems and affect the biogeochemistry, productivity, structure, and functioning of the ecosystems (O’Gorman, 2016; Gounand et al., 2018a, b). This export of production from one ecosystem (source) can provide inputs into another, subsidizing local food webs’ energy budgets. The relative importance of autochthonous (local) production and allochthonous (external) production is a subject often discussed in relation to horizontal transport (between locations) as well as vertical transport (benthic-pelagic coupling) of production within the Southern Ocean (Murphy, 1995; Ansorge et al., 1999; Froneman et al., 1999; Pakhomov et al., 2000; Perissinotto et al., 2000; Murphy et al., 2007, 2012; Hunt et al., 2016; Subalusky and Post, 2019; Treasure et al., 2019; McCormack et al., unpublished data) but there is little understanding of their operation or variability between the Southern Ocean and global ocean ecosystems.
The importance of individual organism and food web processes in biogeochemical cycles in Southern Ocean ecosystems is being elucidated (Murphy and Hofmann, 2012; Cavan et al., 2019; Henley et al., 2020). The passive and active movement of organisms have important impacts on biogeochemical cycles across scales (Henley et al., 2020). Biota cycle and store nutrients (especially iron) and carbon over widely-varying timescales, and hence also act as vectors transporting nutrients vertically, horizontally, and beyond the Southern Ocean. Whist carbon uptake by primary production is similar in magnitude to the direct uptake of CO2 by solubility pump processes across the Southern Ocean, this organic carbon can be transferred into heterotrophic organisms, leading to rapid sequestration and long-term carbon storage in animal biomass, sediments or storage as refractory carbon in the deep ocean (Henley et al., 2020). The processes of vertical carbon export and sequestration associated with the biota (Boyd et al., 2019) and the carbon’s fate depend on the interactions with the three-dimensional flow (Robinson et al., 2014). The movement of large mobile organisms also affects biogeochemical cycles in remote ecosystems through the input of nutrients and carbon (respiration, excretion, and mortality), while also acting as consumers, potentially affecting primary production and biogeochemical cycles (Roman et al., 2014; Doughty et al., 2016).
The migration of large numbers of predators, resulting in the annual northward and southward shifts in the distribution of upper trophic level biomass, is driven by the high-latitude Southern Ocean’s intense seasonality. Dispersal out of the region during winter reduces the local demand for prey and hence the dependence of the remaining highest trophic level productivity on local Southern Ocean production. The mortality rates of zooplankton, fish, and cephalopod populations may, therefore, also be significantly reduced during winter, especially in local shelf areas where summer demand is concentrated (Croxall et al., 1985; Murphy, 1995). Therefore, the effect of the mass movement of organisms and biomass is to reduce and disperse the demand for prey by mid- and upper trophic level species northward and out of the Southern Ocean during winter (Figure 4 and Supplementary Tables 3, 4). However, the total mass of prey required by seabirds may not be that much lower; while adults no longer need to provision chicks, they need to replace plumage, which is energetically demanding and generally occurs during the nonbreeding season (Catry et al., 2013). Although they no longer provision young, their fledged offspring will expend energy feeding for themselves.
This seasonal pattern of movement also represents a major spatial transfer of energy, nutrients, carbon, and biomass across the APF and the SAF. It results in a seasonal pattern of concentration and dispersal of energy demand and increased intensity of food web interactions during the summer (Figure 4 and Supplementary Tables 3, 4). Thus, food webs in the Southern Ocean are highly seasonal and inextricably connected with food webs further north, generating a seasonal interdependence between polar and lower-latitude regional ecosystems. This spatial connectivity is essential in determining the structure and functioning of food webs north and south of the APF. Movement of organisms between ecosystems will affect food web interactions in both areas, generating top-down impacts on lower trophic level and biogeochemical processes and competitive interactions between migrant and resident predators (van Deurs et al., 2016). These processes (advection and movement) coupling ecosystems across scale (metacommunities) are essential in maintaining ecosystems and affects their structure, functioning, biodiversity, and stability and resilience (McCann et al., 2005; Bauer and Hoye, 2014; Walther et al., 2015; Doughty et al., 2016; van Deurs et al., 2016; Bolchoun et al., 2017; Gounand et al., 2018a; Mougi, 2018, 2019; Dunn et al., 2019a).
Within the general pattern of northward movement of seabirds and cetaceans during autumn, a number of species move into eastern and western boundary areas around South America, southern Africa, and Australasia (Figure 3). Some species move along the coastal shelf areas or shelf edges, feeding in local food webs as they transit out of and into the Southern Ocean (Kopp et al., 2011; Andrews-Goff et al., 2018). Processes of food web connectivity are likely to be particularly strong in these areas associated with the ocean basin boundary regions, as these are also often areas of enhanced productivity (Croxall and Wood, 2002; Phillips et al., 2005; Peron et al., 2010). The predators can exploit shifting timings in peak productivity and food availability by migrating, although this brings them into competition with substantial resident populations of predators (see section “Seasonal Changes in Prey Demand of Seabirds”). The extent to which cetaceans impact remote regional ecosystems where they concentrate for breeding during the austral winter is unclear due to reduced feeding in the austral winter, but they still will respire, die, and be predated, and some species continue feeding year-round (Roman et al., 2014; Doughty et al., 2016).
Human Systems Connections
Southern Ocean ecosystems support a range of globally important ecosystem services. Ecosystem services are the benefits that humankind obtains from natural ecosystems and are generally grouped as provisioning (e.g., fishery products), supporting (e.g., nutrient cycling), regulating (e.g., climate regulation), and cultural (e.g., tourism). Assessments of the current and potential future status of Southern Ocean ecosystem services, together with decision-making considerations, are provided in Cavanagh et al. (2021a). The demand for, and importance of these services, for example, for supporting global food security, for recreation (i.e., tourism), and climate regulation, is expected to increase in the future (Rogers et al., 2020; Cavanagh et al., 2021a).
The three major areas of direct human interaction with Southern Ocean ecosystems that involve people’s movement and the associated transfer of material or resources, including contaminants, microorganisms and pollutants (including plastics) are: (i) fisheries, (ii) tourism, and (iii) science activities. There are also transfers associated with national activities and logistics. These interactions are influenced by socio-economic and geopolitical factors, which also set the governance framework.
These operations provide economic activity and employment in countries across the world (Bertram et al., 2007), particularly in the Antarctic gateway ports (Bertram et al., 2007), and support extensive secondary industries, such as operation and maintenance of fishing and commercial tourism (IAATO, 2019) and science vessels. These operations generate carbon emissions through the movement of ships, aeroplanes, people, and associated resources (Amelung and Lamers, 2007; Farreny et al., 2011). Increased ship visits also potentially lead to a higher probability of invasive species introductions into Southern Ocean ecosystems (Lee and Chown, 2009; McCarthy et al., 2019; Morley et al., 2020; Grant et al., 2021). The accessibility of polar regions to human activities (fishing, tourism scientific activities, and shipping) is becoming greater with warming and reduced sea ice distribution and timing induced by climate change (IPCC, 2019; Meredith et al., 2019; Cavanagh et al., 2021a, b).
Fisheries
The Southern Ocean has long played a major role in global production of goods from marine species, with a sequence of exploitation that includes seals, whales, finfish, and krill (Grant et al., 2021). It has also featured heavily in the global rise of illegal, unreported and unregulated (IUU) fishing in the mid-1990s. Global demand led to the near extirpation of Antarctic fur seals in the early 19th century, the great whales during the 20th century, and a number of groundfish (nototheniid species, including Notothenia rossii) in the 1970s and 1980s. The rapid rise of Antarctic krill fishing in the 1970s led to the negotiation of the Convention on the Conservation of Antarctic Marine Living Resources (the “krill” convention) because of fears of overfishing of krill and potential damage to the ecosystem as a whole (Miller, 1991).
Recent assessments of Southern Ocean ecosystem services have emphasized the global significance of Southern Ocean fisheries (Cavanagh et al., 2021a). Over the period of the fishing operations (1970s to present), catches in the two main fisheries [toothfish (Dissostichus spp.) and Antarctic krill] have been taken by vessels registered in states on every continent and in Northern and Southern Hemispheres (Figure 5). The total catch of all species in 2018/19 was 406 thousand tonnes (CCAMLR Statistical Bulletin, 32, see their Table 4.1). The mean annual wholesale value of Southern Ocean toothfish fisheries was US$206.7 million per year for the period from 2011 to 2015 (CCAMLR, 2016), and Antarctic krill (Euphuasia superba) had a first-sale value of between US$100 to 416 million in 2017/2018 (Cavanagh et al., 2021a). Currently, krill catches makeup <0.5% of the global marine fisheries catch (GAMFC), although it has been suggested that potential catch could be equivalent to >10% of GAMFC (Nicol and Foster, 2016).
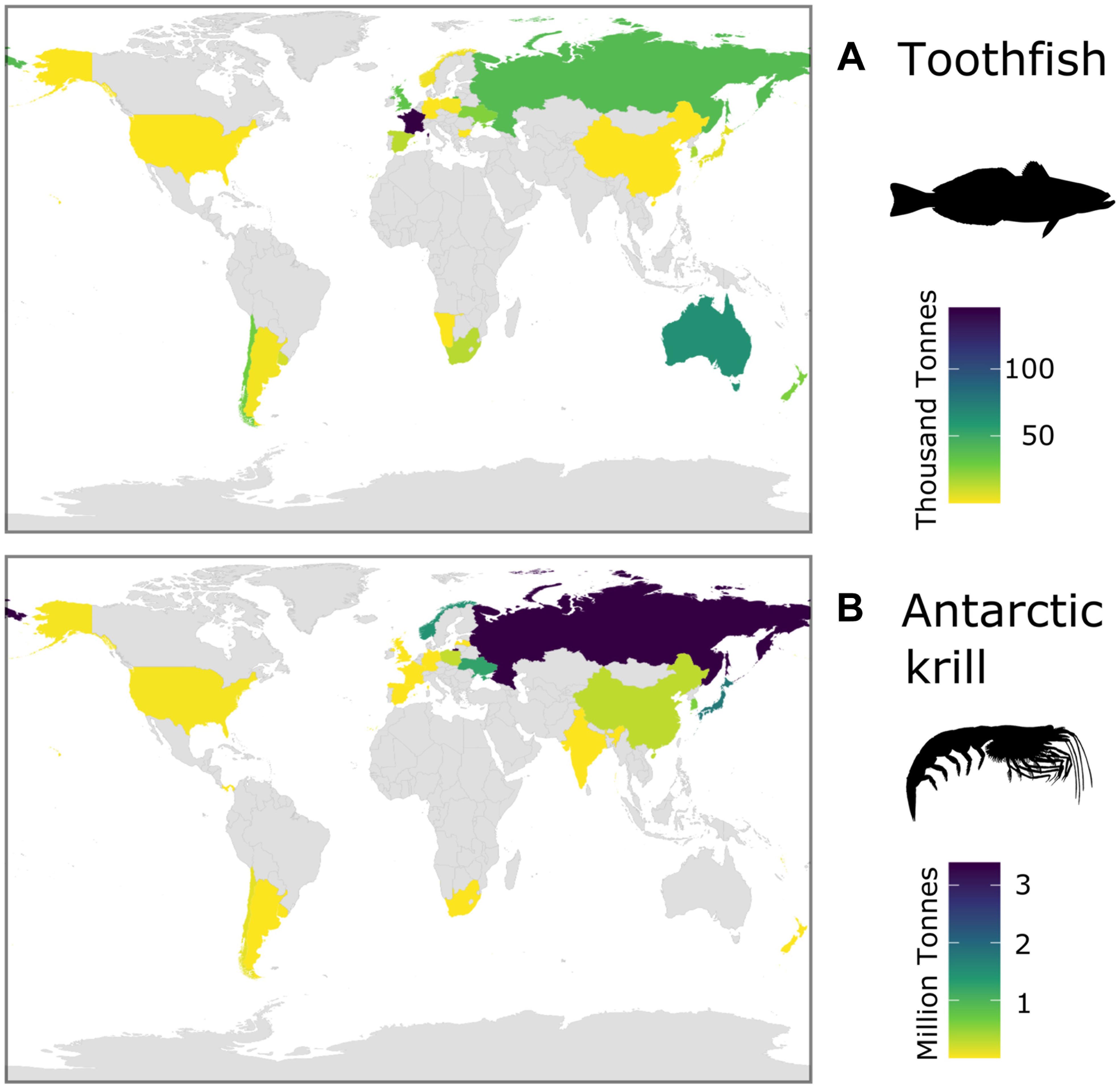
Figure 5. Total catches of the two current major Southern Ocean fisheries over the historical period (1970s to present day) for each Flag State (catch records from all years, CCAMLR): (A) toothfish (Dissostichus spp.) and (B) Antarctic krill (Euphausia superba).
Tourism
The Southern Ocean is home to 90% of the world’s penguin species, 50% of the world’s baleen whale species, and includes 80% of the total area of high seas Marine Protected Areas (Boonzaier and Pauly, 2016). The unique biodiversity is a significant driver of tourism, which has increased rapidly over the last 30 years. Over the last decade, the number of commercial tourism voyages almost doubled (223 in 2010–2011, 432 in 2019–2020), while passengers carried on ships has more than doubled (33,438 in 2010–2011, 74,120 in 2019–2020; IAATO, 2019). As a result, the tourism industry directly impacts Southern Ocean ecosystems in terms of ship traffic, landings, and site visits, with current operations, concentrated on the Antarctic Peninsula (Bender et al., 2016; Pertierra et al., 2017; Grant et al., 2021). Tourism has a global footprint in that movement of people to join commercial tourism ships involves air travel to South America (Ushuaia and Punta Arenas), Africa (Cape Town), Australia (Hobart), and New Zealand (Christchurch), or via flights to the South Shetland Islands where visitors may join cruise vessels without having to experience a potentially rough crossing of the Drake Passage (known as fly/cruise tourism; Bertram et al., 2007; IAATO, 2019; Figure 6). The operations of commercial tourism to the Antarctic provide economic activity and employment in countries across the world and particularly the Antarctic gateway ports (Bertram et al., 2007; Figure 6).
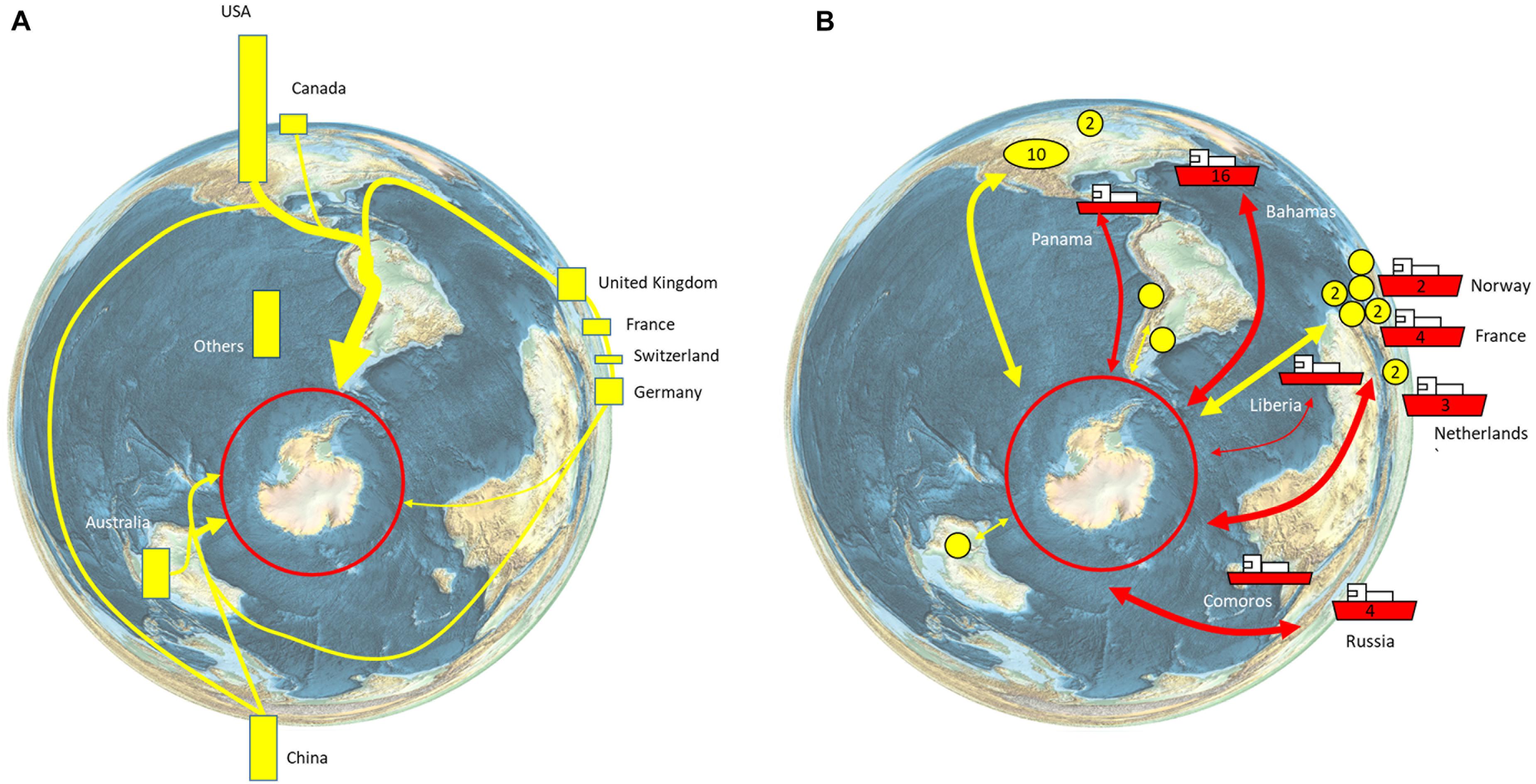
Figure 6. Global connections of the Antarctic tourism industry. (A) Nationalities of tourists. 55,489 sea-borne tourists, of more than 100 different nationalities, visited Antarctica during the 2018–2019 season. The nationalities of the tourists are shown as bars, with bar length indicating the relative percentage of all tourists (IAATO, 2019, their Table 2). The major routes of travel/arrival are indicated schematically. (B) Country of registration of tourism vessels (ships with country of registration label; number of vessels where more than 1 is registered is indicated on the hull). The yellow circles indicate the region of the operator (numbers are given for more than 1 operator in a country (IAATO, 2019, their Appendix 2).
Science
Southern Ocean ecosystems are an increasing focus of international scientific activities as highlighted by the international investment in a new generation of ice-capable research vessels and the construction of new coastal research stations on the continent’s margins (COMNAP, 2017). Furthermore, there has been a rapid increase in the development and deployment of remote and autonomous systems for increasing observations of these ecosystems across multiple spatial and temporal scales (Newman et al., 2019). This increased scientific activity involves extensive inflows into Southern Ocean regions of ships, airplanes, people, and associated resources, while outflows include biological samples, specimens, and data. It also involves national investments that contribute to the support of communities and businesses as well as fundamental scientific endeavors. The Southern Ocean and Antarctic scientists’ global community supports personnel and associated businesses, government and governance structures, non-governmental organizations, and other bodies worldwide.
Society and Culture
People across the world have long had a fascination and concern for Antarctica and the Southern Ocean from the heroic era in the late 19th and early 20th Centuries (Larson, 2011), to the rise of science (Walton, 2005), and the advent of the ATS with its orientation toward the conservation of the region (Hanifah et al., 2012; Hughes et al., 2018). The development and implementation of the Madrid Protocol in 1998 was the result of a global effort to provide long-term protection to the Antarctic environment south of 60°S, instituting a ban on mining in the region in perpetuity, which was in contrast to the negotiations at the time for a convention to regulate mining activity (Scully, 2011). The strength of commitment of Antarctic Treaty nations to these principles was reaffirmed in 2016 in a declaration on the 25th Anniversary of the signing of the Protocol (ATCM, 2016) confirming the central values of peace, science and conservation in the region, as well as the importance of the region to the global system. Antarctica and the Southern Ocean also have cultural links to indigenous cultures, and the expression of ecosystem science through art (Roberts et al., 2021). In recent times, there has been an extensive media and cultural focus on the Southern Ocean as a unique, fragile, and threatened environment. This has and continues to contribute to developing global public awareness and understanding of how the oceans and associated ecosystems will be affected by anthropogenically driven climate and ocean change.
Governance
The various instruments of the ATS regulate all activities in the area south of 60°S (the Antarctic Treaty area), except for whaling, which is managed by the IWC under the Convention on the Regulation of Whaling (1946). The primary instruments in the ATS are the Antarctic Treaty (1959) with its Protocol on Environmental Protection (1991), the Convention on the Conservation of Antarctic Seals (1972; CCAS), which is not now active, and CCAMLR (1980; CAMLR Convention). The area of jurisdiction of CCAMLR was extended to the Antarctic Convergence (APF) in order to encompass the region thought to contain most of the Antarctic marine ecosystem. The instruments of the ATS are open to accession by nations.
The management bodies of the two active components of the ATS are the Antarctic Treaty Consultative Meeting (ATCM) and CCAMLR. Currently, there are 54 signatory Parties to the Antarctic Treaty, of which 29 Parties have demonstrated “substantial research activity” within Antarctica and thereby attained consultative status which includes participation in governance decision-making. The engagement of nations with the Treaty represents approximately two thirds of the global population. The underlying focus of the ATCM and CCAMLR has been conservation of the region (Hanifah et al., 2012; Press et al., 2019).
The importance of the Southern Ocean is recognized in some global governance arrangements. These have included consideration of managing high seas fish stocks through regional fisheries management organizations (RFMOs) and harmonizing the management of migratory species and shared stocks through the UN Fish Stocks Agreement (1995). Although external to the UN system, CCAMLR is recognized as the regional management organization responsible for managing fisheries in the Southern Ocean1. While there are exchanges of information with RFMOs to the north of CCAMLR, there remains to be formal arrangements about shared interests such as fishing for toothfish and the incidental mortality of seabirds (Anon, 2017).
CCAMLR has developed work programs for managing the marine environment, including taking steps to establish marine protected areas, a request from under the Convention on Biological Diversity (SC-CAMLR, 2005), and to conserve Vulnerable Marine Ecosystems arising from the UN Resolution 61/105 in 2006 (SC-CAMLR, 2007), although much work remains in this regard (Anon, 2017; Chown and Brooks, 2019). While CCAMLR and the ATCM remain the regulatory bodies, the recent review of CCAMLR in 2017 recommended that CCAMLR give more attention to demonstrating its preeminent role globally by establishing relationships with external bodies that not only have an interest in Antarctica and the Southern Ocean, a call gaining increasing support (Chown and Brooks, 2019; Dodds, 2019), but also to gain support for global actions that will help CCAMLR achieve the long-term conservation of the region (Anon, 2017). Unlike the ATCM, CCAMLR has yet to establish a program on the effects of climate change, including establishing a relationship with the UN Framework Convention on Climate Change, on the effects of climate change on the conservation of Antarctic biota (Anon, 2017; Cavanagh et al., 2021b).
Why Southern Ocean Ecosystems Matter and Why They Are a Priority for the Future
Southern Ocean ecosystems are inextricably connected in global ocean ecosystems and are important in Earth system processes and human socio-economic systems. Southern Ocean ecosystems connect across multiple trophic levels and temporal and spatial scales that are interconnected by physical, ecological and human exchange processes (Figure 7). Physical processes connect habitats and influence biogeochemistry and productivity (Figures 2, 7), which underpins the flow of energy and carbon through regional food webs. Bio-physical interactions and ecological processes (Figures 2, 7) of dispersal (drift) and active movement (behavior, foraging, and migration) connect ecological systems across scales. Human systems exchange by movement of people, materials, resources, contaminants and pollutants (including plastics). These processes are influenced by a series of proximate (e.g., fisheries and atmospheric greenhouse gas concentrations) and ultimate drivers (e.g., food security and population change). The physical, ecological and human exchange processes are affected by climate change, thereby generating potential feedback effects, with consequences for Southern Ocean and global systems (Figure 7).
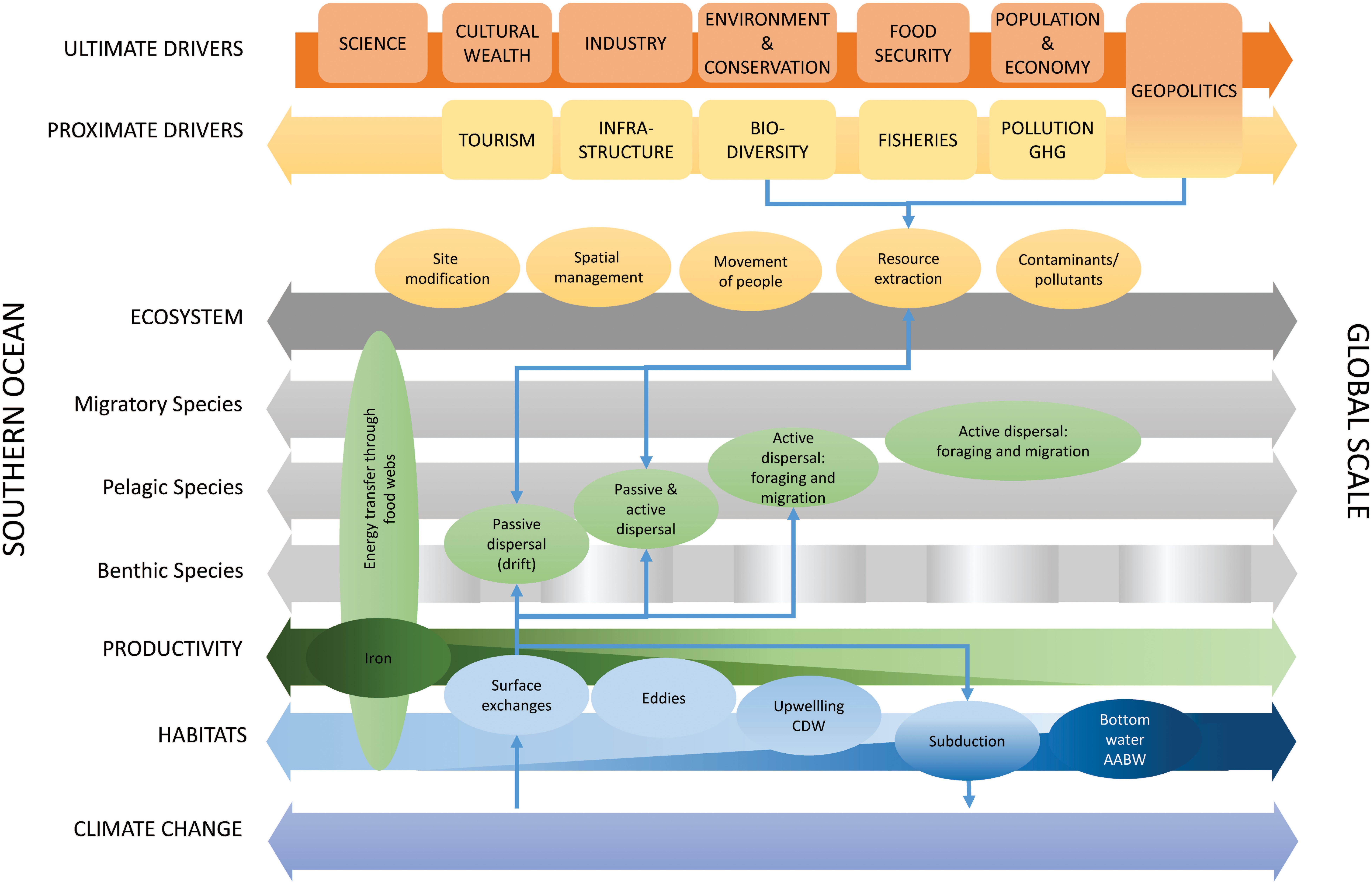
Figure 7. Summary figure of the role of exchange processes (colored ellipses: physical–blue, ecological–green, and human–orange) across interlinked biophysical dimensions (large horizontal arrows) from the Southern Ocean scale to the global scale, the drivers of change in these exchange processes across different dimensions (ultimate and proximate), overlaid with an example of connections and feedbacks across these exchange processes (thin blue arrows). Green shading for iron supply and exchange, and dark blue shading for AABW indicate the decreasing and increasing importance, respectively, of these exchange processes from the Southern Ocean to the global scale. GHG, greenhouse gases. Spatial scale (i.e., from the scale of the Southern Ocean to the global scale) is represented as a continuum rather than a specific demarcation between the Southern Ocean and the rest of the globe, noting that such demarcations are generally geopolitical constructs (with some links to physical ocean features) but that the ecological dimensions and exchange processes represented here occur across many scales. The positions of boxes showing drivers and exchange processes along the horizontal arrows give an indication of whether those are more specific to the Southern Ocean or extend to regional and global scales.
Over the coming decades, changes in Southern Ocean overturning circulation and bottom water formation will happen and will affect global circulation and biogeochemical cycles (IPCC, 2019; Meredith et al., 2019; Morley et al., 2020). These physical changes will undoubtedly impact the supply of nutrients and carbon to Southern Ocean surface waters, and their redistribution horizontally and vertically within the Southern Ocean and to the north (Henley et al., 2020 and references therein). Warming and freshening of the mode and intermediate waters north of the ACC will propagate northward into the interior of the global ocean (IPCC, 2019; Meredith et al., 2019).
Projections based on coupled biogeochemical-ocean circulation models suggest that Southern Ocean primary productivity is likely to increase because of ocean warming and increased iron inputs, but these projections are uncertain and the projected changes vary with latitude (Hauck et al., 2015; Leung et al., 2015; Boyd, 2019; IPCC, 2019; Meredith et al., 2019; Henley et al., 2020; Pinkerton et al., 2021). A more productive Southern Ocean overall has the potential to increase the biological uptake of CO2, with large-scale consequences for biogeochemistry and ocean-climate feedbacks (Del Castillo et al., 2019), although the extent to which this can modulate the effects of carbon emissions later this century is uncertain (Del Castillo et al., 2019; Rogers et al., 2020; Cavanagh et al., 2021a). Importantly, shifts in the location of primary production and the phytoplankton community composition can influence this outcome. Moreover, the dynamics of phytoplankton in the Southern Ocean will impact productivity elsewhere in the world. Increased production in the Southern Ocean could result in reduced quantities of exported macronutrients, and therefore reduced production elsewhere (Holzer and Primeau, 2013; Primeau et al., 2013; Hauck et al., 2015; Bronselaer et al., 2016; Keller et al., 2016; Moore et al., 2018). Further, uncertainty in future changes in Southern Ocean biogeochemistry is compounded by uncertainties in the changes to phytoplankton assemblages in the region (Pinkerton et al., 2021).
As Southern Ocean phytoplankton communities shift southward under global warming, the uptake of nitrogen is expected to be greater relative to phosphorus, leading to a decrease in the N/P ratio of water masses exported to the north (Arrigo et al., 1999; Weber and Deutsch, 2010; Martiny et al., 2013), which would reduce productivity in lower latitudes with potential large-scale implications for carbon export and budgets in those regions (Weber and Deutsch, 2010; Martiny et al., 2013; Hauck et al., 2015, 2018). Conversely, shifts to diatoms in the subantarctic could increase the N/P ratio of exported waters. Similarly, changes in the Si/N ratio of exported waters, driven by phytoplankton species shifts, changes in iron availability and ocean acidification effects on diatom silica production, are also expected to impact upon primary production, phytoplankton species composition and carbon export throughout the low-latitude oceans (Mosseri et al., 2008; Petrou et al., 2019; Henley et al., 2020). The effect of enhanced productivity in the Southern Ocean may lead to reductions in total export of nutrients from the Southern Ocean, resulting in the trapping of nutrients (Moore et al., 2018), with a consequent reduction in productivity across the rest of the global ocean affecting global oxygen and carbon budgets and food webs (Holzer and Primeau, 2013; Primeau et al., 2013; Hauck et al., 2015; Bronselaer et al., 2016; Keller et al., 2016). Nutrient biogeochemistry can also be modified by changes in microbial processes or shifts in community composition, abundance, distribution, behavior, and/or trophic interactions of phytoplankton, zooplankton and higher organisms (Azam et al., 1991; Lavery et al., 2010; Maas et al., 2013; Sailley et al., 2013; Ratnarajah et al., 2014; Schmidt et al., 2016; Cavan et al., 2019; Bestley et al., 2020; Henley et al., 2020; Caccavo et al., 2021; Pinkerton et al., 2021; Johnston et al., unpublished data).
Ocean acidification resulting from increased uptake of CO2 is expected to occur earlier in the Southern Ocean than other ocean regions (Orr et al., 2005; McNeil and Matear, 2008; Feely et al., 2009), and has already been observed across a number of Southern Ocean regions e.g., (Bednarsek et al., 2012; Takahashi et al., 2014). Around 30% of Southern Ocean surface waters are expected to be affected by ocean acidification by 2060 (Hauri et al., 2016). As well as altering biota, food webs and ecosystem functions within the Southern Ocean (Gutt et al., 2015; Henley et al., 2020), the northward export of waters undersaturated with carbonate ions could promote the harmful effects of ocean acidification in the lower-latitude oceans to the north (McNeil and Matear, 2008; Feely et al., 2009).
Food webs in the Southern Ocean and their connectivity to other systems are changing (Murphy et al., 2012; Constable et al., 2014; Meredith et al., 2019) with consequences within (see McCormack et al., unpublished data) and outside the Southern Ocean. The connectivity of ecosystems through transport and movement has the potential to transfer the signals of those changes between ecosystems. Changes in small and large-scale ocean dynamics will affect cross-frontal exchange and the dispersal of plankton. Such changes in the rates of mixing between polar, sub-polar and subtropical waters may also potentially disrupt the life cycles of fish and cephalopod species that cross oceanic fronts during development, thus affecting their role in Southern Ocean food webs (Murphy et al., 2007; McMahon et al., 2019; Saunders et al., 2019; Abreu et al., 2020). The APF is not expected to undergo major changes in location in the coming century (Meijers et al., 2019b), potentially limiting the southward extension of the distributions in the Southern Ocean of pelagic fish species from farther north (Boyce et al., 2008). However, changes in cross-front exchanges due to increases in eddy intensity may allow more frequent opportunistic incursions further south of some species. Modeling suggests that warming coastal and shelf waters around Antarctica (Griffiths et al., 2017) is likely to result in colonization and establishment by diverse non-native species, and have important consequences for Antarctic ecosystems and food webs (but many native species may also benefit from initial warming see Morley et al., 2019).
Southern Ocean waters have the fewest established non indigenous species (NIS) of any large region, which does reflects the general level of geographic and oceanographic exchange (Clarke et al., 2005). Nevertheless vector density for potential NIS travel is increasing, either through shipping (Lewis et al., 2003; McCarthy et al., 2019) or rafting on plastic debris (Barnes et al., 2018). Sustained presence of any given NIS within the Southern Ocean requires not just transport, but transfer, establishment, year-round survival and reproduction. Hughes et al. (2020) considered a list of >100 species thought to pose risk of invasion to Antarctica. They considered that 13 were high risk in terms of likely establishment and severity of impact, if successful. The consequences of NIS spread in the Southern Ocean are still unclear because each is likely to be species identity and local area dependent and may have considerably lag phases. However, Hughes et al. (2020) think the situation is highly concerning (see also Grant et al., 2021), especially as native species are already under pressure from many other interacting stressors (Gutt et al., 2015; Rogers et al., 2020).
The analysis of seabird and whale movement in this study is a first attempt to estimate the magnitude of the summer-winter movement of large numbers of predators into and out of the Southern Ocean. Large uncertainties are associated with these estimates, but the scale of the migration and its ecological importance in connecting ecosystems across the Southern Hemisphere is clear. The demand estimates also highlight that changes affecting predators in the Southern Ocean will be transferred out of the region and impact areas across oceanic and coastal regions on the east and west basin boundaries.
The population sizes of some Southern Ocean species of seasonally migrating predators have undergone major changes over the last few decades, and these are expected to continue in the future. The most profound ecological changes are associated with recovering populations of the great whales that were exploited to very low numbers in the 19th and 20th centuries (Noad et al., 2019). The steady population increases observed over the last 2 decades in several species of great whales (Tulloch et al., 2019) will affect Southern Ocean ecosystems as well as those in shelf regions along the east and west coasts of South America, Africa, and Australasia (Rosenbaum et al., 2014; Noad et al., 2019; Zerbini et al., 2019). As whale populations recover from historical harvesting, the increased demand for prey may increase competition in local food webs, and change the balance of the upper trophic level community. Changes in predator populations will also generate shifts in the absolute and relative numbers of seabirds and marine mammals of different species moving along migration routes.
Threats to Southern Ocean ecosystems from potential future human activities, together with the recovery of historically harvested species and the natural high variability of Southern Ocean ecosystems, will generate major challenges for conservation and management (Chown et al., 2012; Hughes et al., 2018; Chown and Brooks, 2019; Dunn et al., 2019b; McCarthy et al., 2019). The major threat to the structure and functioning of ecosystems in areas south of the APF is from climate change (IPCC, 2019; Meredith et al., 2019), and there are major uncertainties about the directions, magnitude and time scales of impacts. Recent assessments indicate that climate change generally poses a medium to high level of risk for the capacity of the Southern Ocean to support ecosystem services under future climate change (Cavanagh et al., 2021a). Increased pressures may also be exerted on the region by tourism and by increases in global demand for food (FAO, 2018). Pollution is also a potential threat and may increase associated with increased activity through a range of local and global sources, such as oil-spills, marine debris, and ocean and atmospheric transport of microplastics and heavy metals (Grant et al., 2021). Human activities within the region are managed within a mandate to conserve the region (Hughes et al., 2018), but global socio-economic and political processes (Figure 7) will determine the extent to which these threats are realized. In the short-term, the number of visitors may increase although the negative consequences of COVID-19 on tourism and science activities may last for some years (Hughes and Convey, 2020).
Concluding Comments
Ecological processes interact with the full three-dimensional physical structure of the Southern Hemisphere ocean and atmosphere to produce a continuum of connected ecosystems and food webs with intense seasonal dynamics rather than distinct food webs. The two-directional connections between Southern Ocean regional ecosystems and wider Southern Hemisphere and global ocean ecosystems affect the stability and resilience properties and responses to change of these connected ecosystems, forming a “meta-ecosystem” in the global ocean. This ocean-scale ecosystem connectivity and interdependence will influence Earth system-level responses to climate change on time scales of decades to millennia. However, the current generation of Earth system models does not consider the importance of such large scale ecological connectivity and potential feedbacks (Eyring et al., 2016; IPCC, 2019). There is a major need to improve understanding of species ecology, ecosystem structure and functioning and seasonal dynamics throughout the Southern Ocean and how this interacts with the biogeochemistry in the 3-dimensional ocean and the links with global ocean ecosystems (Box 1). Within this there are key questions about how important such north-south ecological exchange processes and energy flows are in Southern Ocean and global ecosystems and carbon budgets and what are the major routes, vectors, magnitudes (and variability) of transfer.
BOX 1. Knowledge gaps and future research priorities.
Global Connectivity. There is an urgent need to develop quantitative understanding of the global connectivity of Southern Ocean ecosystems. With changes already occurring in ecosystems in the Southern Ocean and across the Southern Hemisphere the impacts of change are being transferred into and out of the Southern Ocean with variability in that transfer both spatially and temporally. With Earth system models poorly representing Southern Ocean systems, and signals within the Southern Ocean being confounded by the potential for ecological connectivity, new approaches are required to understand the impacts of change in Southern Ocean ecosystems and the consequent effects in the wider Earth system and to manage and adapt to the global consequences of that change. Developing such approaches to consider two-way ecosystem connectivity and impacts of change should be an important focus for international research communities, including the science programs of the Scientific Committee on Antarctic Research (SCAR), the Integrating Climate and Ecosystem Dynamics in the Southern Ocean programme (ICED) and the global Integrated Marine Biosphere Research programme (IMBeR).
Three-Dimensional View. Detailed information is available to highlight the three-dimensional nature of Southern Ocean circulation, and understanding of how it is likely to change over the next half century is sufficient to investigate and quantify the biogeochemical and ecological implications for ecological connectivity and ecosystem services from the Southern Ocean (see section “Southern Ocean Connections” and Supplementary Table 1). More information is particularly required on the pathways of exchange and especially the subregional nature of the connections from the Southern Ocean to remote areas of the global ocean. The priority is to identify and focus research in these subregions where connectivity is most likely to influence the internal dynamics of Southern Ocean ecosystems and has greatest influence in the global ocean. We also need to improve our understanding of how individual species and food webs operate in the full three-dimensional Southern Ocean, in the sea ice and full depth ocean, and how these processes influence biogeochemical cycles, particularly in the APF and SAF frontal zones. Quantifying fluxes is a priority and more direct measurements and full-depth analyses are required of the nutrient, carbon and biological properties of waters flowing across the regions of the APF and SAF, how these are produced, modified and impact ecosystems in remote regions. The development of time-series observations is important, as the exchange processes will show strong seasonal and interannual variability and we need to start developing base-lines against which to assess future changes.
Cross-Frontal Exchange. There is little information on the magnitude of cross-frontal exchanges in maintaining or influencing the dynamics of most Southern Ocean biota, and how these exchanges influence trophic structure, particularly in ecosystems outside of the Southern Ocean. The importance of these movements of large numbers of seabirds, marine mammals and possibly larger fish in connecting Southern Ocean and wider Southern Hemisphere and global ocean ecosystems has generally not been considered. The calculations in this study of seasonal movement of seabirds and cetaceans and associated shifts in the demand for prey into and out of the Southern Ocean (see section “Implications of Ecological Connectivity”) show that these are important in both Southern Ocean and remote regional ecosystems. However, these preliminary calculations also highlight the uncertainty associated with such calculations and the need to improve assessments of seasonal changes in abundance, distribution and energy and prey requirements. Understanding winter-time habitat use by predators is a crucial, understudied, element of this. This requires focused studies of subregional ecosystems of the Southern Ocean, examining the spatial operation of food webs and how these vary seasonally, and how extra-Southern Ocean processes impact on these. Ensuring the Southern Ocean Observing System (SOOS) program has observations that can be used to specifically assess exchange processes would be a valuable step. However, new approaches aimed at quantifying energy and carbon flows and the connectedness of ocean ecosystems are also required. Developing observation networks in regions where ecological exchanges are most intense may be valuable, for example, the major boundary and coastal current regions appear to be particularly important in some of the major biogeochemical and ecological exchange processes (see sections “Physical and Biogeochemical Connections” and “Ecological Connectivity”). Such regions would be areas where enhanced observation networks of biogeochemical and ecosystem processes could be deployed, including, for example, networks of moorings or autonomous vehicles and seabird and marine mammal tracking systems.
Modeling Ecological Connectivity. Ultimately, the only way to examine the role of ecological connections in food webs across the wide range of spatial and temporal scales required is through the development of system-level ecological models. Such models are needed to quantify the relative importance to ecosystem services of local and remote change, and for projecting future ecosystem states based on projected changes to the Earth system. There are few models available that can be used to assess ecological connectivity across multiple trophic levels within food webs. This requires the development of dynamic models of food webs across scales, which link physical, biogeochemical and ecological processes. A key aspect of this will be the development of high-resolution models of local and regional whole ecosystem processes operating within larger scale models (e.g., circumpolar or global) to allow seasonal and spatial (horizontal and vertical) connectivity to be examined. Moreover, these models are needed to assess the probabilities of invasive marine species becoming problematic in the Southern Ocean and for better understanding the infiltration and impact of plastics in the system. Current Earth system models do not take account the connectedness of oceanic ecosystems, its potential role in transferring impacts of change and the influence of cross-scale ecological exchanges on local and regional ecosystem stability and resilience. Earth system models are required that explicitly include cross-scale ecological interactions and whole ecosystem processes to allow analyses of their direct and indirect influence on climate processes and impacts of change.
Human Pressures. Southern Ocean ecosystems are likely to be affected by global pressures from future changes within human systems. Current human activities that involve transfers of people and material in and out of the Southern Ocean have a global footprint. Improved understanding is required of the national and international drivers of these processes in order to develop projections of how such activities may develop in the future, particularly as a result of climate change and population increases. Such understanding will be crucial for generating scenarios and shared socio-economic pathways of future change that can be used in conjunction with climate projections to inform future decision making for the Southern Ocean and throughout the global ocean.
Governance. At present insufficient attention is given to cross-system connections by regulatory bodies within and surrounding the Southern Ocean. There is an urgent need for the further development of conservation and management measures that encompass multiple regional systems and conservation and management bodies. Given the connectivity between Southern Ocean and global ocean ecosystems and the two-way effects, developing activities within the Antarctic Treaty System will be important. Taking account of the importance of Southern Ocean ecosystems and their connectivity in the wider Earth system will also be important for regulatory bodies that consider ecosystems across the Southern Hemisphere and throughout the global ocean.
The ecological connectivity between Southern Ocean and global ocean ecosystems underpin the processes that maintain essential ocean ecosystem functions and services and are important in maintaining human societal and economic processes within the Earth system (Steffen et al., 2020; Cavanagh et al., 2021a). The effects of change and decision making across the global ocean will affect Southern Ocean ecosystems, and in turn, decisions made for the Southern Ocean will affect ecosystems and human systems across the planet. Geopolitics will affect the ability of CCAMLR and other international bodies (Dodds, 2019) to make decisions that will be required as climate-driven changes become clearer, and the pressures from increased direct human impacts increase driven by demand and increased accessibility.
There is an urgent need to develop conservation and management consortia between CCAMLR and adjacent bodies that operate at Southern Hemisphere scales (Anon, 2017). The ecological connectivity of Southern Ocean and global ocean ecosystems has important implications for decision making for conservation and management in the Southern Ocean and across the Southern Hemisphere. Managing the Southern Ocean in isolation does not consider the potential effects of that management on people and regions outside of the Southern Ocean, nor does it recognize the effects that actions external to the region have on the dynamics of Southern Ocean systems. Maintaining connectivity of populations, food webs, and the flow of genes across scales will also help to support ecological resilience to climate change. Prediction and management of the impacts of human activities and climate change on Southern Ocean ecosystems requires a view that recognizes and incorporates the connectivity and linkages of these ecosystems to global ocean ecosystems.
Such large-scale connections between ecosystems will be important throughout the world’s oceans in developing conservation and management approaches and understanding the impacts of change (Dubois et al., 2016; Gounand et al., 2018b; Young et al., 2018; Dunn et al., 2019a; Kenchington et al., 2019; Xu et al., 2019; Zuercher and Galloway, 2019; Guihou et al., 2020). However, oceanic ecosystems’ ecological connectivity may be particularly important in the high-latitude and seasonal polar ocean ecosystems (Niiranen et al., 2018). It is clearly a major aspect of the structure and functioning of Southern Ocean ecosystems, which are already undergoing major change. Those changes will impact areas north of the APF and SAF and across the Southern Hemisphere and have global consequences. Developing a quantitative understanding of global ocean ecosystems’ connectedness and role in determining responses to change, including impacts on climate processes, is an urgent requirement for improving the representation of ecological processes in Earth system models. Understanding the impacts of change in the Southern Ocean and managing and adapting to the global consequences of that change requires new approaches to studying ocean ecosystems that consider the connectedness of global ocean ecosystems (Box 1). Developing such a global perspective requires the integration of research communities and ocean management activities, linking Southern Ocean and global research communities. That is a priority of the Integrating Climate and Ecosystem Dynamics in the Southern Ocean programme (ICED), which is part of the global Integrated Marine Biosphere Research programme (IMBeR), and co-sponored by the Scientific Committee on Ocean Research (SCOR), Future Earth and the Scientific Committee on Antarctic Research (SCAR). This is important not only from a Southern Ocean perspective, but is also required for the development of Earth System analyses and management across the global ocean, which needs to take account of Southern Ocean ecosystem processes to improve understanding, future projections and ocean governance.
The Southern Ocean has not been remote for a long time, and its regional ecosystems have never been separate. The pathways of connection and feedback are important in the Earth system and in human systems. We now have an advanced understanding of local ecosystem processes and the importance of interactions across scales, and how this affects their structure and functioning, including food web interactions and processes. Yet current analyses and models of ocean ecosystems and their responses to change often assume that such systems are only local and affected by the bottom-up physical impacts of changing environmental conditions.
This analysis shows that Southern Ocean ecosystem processes are inextricably linked to global ocean ecosystems across many scales. This conceptual shift in understanding has global implications for assessing, analysing and modeling ocean ecosystems (Bauer and Hoye, 2014; Walther et al., 2015; van Deurs et al., 2016; Gounand et al., 2018a; Dunn et al., 2019a). Given the scale of the exchanges, the amount of nutrients, carbon and energy being transferred and the number and biomass of organisms moving over large areas of the ocean, these processes are likely to be important in both the direct climate-related processes (i.e., biogeochemical cycles and carbon budgets) and in determining resilience properties of ecosystems across scales and hence responses to change, with potentially indirect impacts on climate processes (Bauer and Hoye, 2014; Doughty et al., 2016). Analyses and models are required that implicitly consider the scale of ecological process connections across multiple trophic levels and how these affect ecosystem properties of stability and resilience and their responses to variability and change at local, regional, ocean basin, and global scales.
Data Availability Statement
The data used in the study are available in the cited literature. Further details of methods and data and additional references are included in the Supplementary Material. The original contributions presented in the study are included in the article/Supplementary Material, further inquiries can be directed to the corresponding author.
Ethics Statement
This work involved synthesising information from previously published studies.
Author Contributions
EM initiated and coordinated the project and led the writing of the manuscript to which all authors contributed. NJ, EH, AC, and SH contributed substantially to the development, coordination, and writing of the manuscript. EM, RP, and JJ developed the prey demand calculations. EH, AC, JM-T, RT, and ST contributed to development of the figures. RP, JJ, JM-T, RT, RC, GT, RS, DB, DC, SC, CF, JH, KH, CS, ST, PT, and JX contributed significantly to the development of ideas, writing of the manuscript, and completion of the figures. All authors have approved the version for submission.
Funding
This work was supported by the UK RI- Natural Environment Research Council (NERC) through the British Antarctic Survey (BAS) ALI-Science Southern Ocean ecosystems project of the BAS Ecosystems Programme. EH was supported by the United States National Science Foundation Grant OPP-1643652. SH was supported by the United Kingdom Natural Environment Research Council through Grant NE/K010034/1. CF was also supported by a Rutherford Discovery Fellowship from the Royal Society of New Zealand (RDF-UOO1803) and by the New Zealand Antarctic Science Platform (Project 3). SC was also supported by the Australian Fisheries Research Development Corporation through Grant FRDC2018/133. JH was supported by ANID (FONDAP-IDEAL 15150003) and FONDECYT (Regular 1211338). ICED also co-supported JH attendance at the MEASO workshop. JX was supported by the strategic program of MARE (Marine and Environmental Sciences Centre), financed by the Foundation for Science and Technology, Portugal (UIDB/04292/2020).
Conflict of Interest
The authors declare that the research was conducted in the absence of any commercial or financial relationships that could be construed as a potential conflict of interest.
The handling editor is currently organizing a Research Topic with two of the authors JM-T and AC, and confirms the absence of any other collaboration.
Publisher’s Note
All claims expressed in this article are solely those of the authors and do not necessarily represent those of their affiliated organizations, or those of the publisher, the editors and the reviewers. Any product that may be evaluated in this article, or claim that may be made by its manufacturer, is not guaranteed or endorsed by the publisher.
Acknowledgments
This manuscript developed from a series of international activities coordinated by the Integrating Climate and Ecosystem Dynamics in the Southern Ocean Programme (ICED) and is a contribution to ICED and the international Integrated Marine Biosphere Research (IMBeR) programme and the MEASO assessment. The MEASO assessment is an activity coordinated as part of the ICED programme. This paper also contributes to the “Integrated Science to Inform Antarctic and Southern Ocean Conservation” (Ant-ICON) research programme of the Scientific Committee on Antarctic Research (SCAR). We thank colleagues in the BAS Ecosystems and ICED programme for discussions of Southern Ocean ecosystem processes. We also thank the reviewers for their comments, which helped improve the manuscript.
Supplementary Material
The Supplementary Material for this article can be found online at: https://www.frontiersin.org/articles/10.3389/fevo.2021.624451/full#supplementary-material
Supplementary Data Sheet 1 | Supplementary details of methods used to calculate seasonal changes in seabird and cetacean demand for prey with additional references. Supplementary tables presenting information on ocean exchange processes, scales of movement of Southern Ocean organisms and seabird and cetacean demand calculations and associated additional references.
Footnotes
References
Abreu, J., Phillips, R. A., Ceia, F. R., Ireland, L., Paiva, V. H., and Xavier, J. C. (2020). Long-term changes in habitat and trophic level of Southern Ocean squid in relation to environmental conditions. Sci. Rep. 10:15215. doi: 10.1038/s41598-020-72103-72106
Allcock, A. L., and Strugnell, J. M. (2012). Southern ocean diversity: new paradigms from molecular ecology. Trends Ecol. Evol. 27, 520–528. doi: 10.1016/j.tree.2012.05.009
Amelung, B., and Lamers, M. (2007). Estimating the greenhouse gas emissions from antarctic tourism tourism in mar. Environment 4, 121–133. doi: 10.3727/154427307784772020
Andersen, K. H., Berge, T., Gonçalves, R. J., Hartvig, M., Heuschele, J., Hylander, S., et al. (2016). Characteristic sizes of life in the oceans, from bacteria to whales. Ann. Rev. Mar. Sci. 8, 217–241. doi: 10.1146/annurev-marine-122414-134144
Andrews-Goff, V., Bestley, S., Gales, N. J., Laverick, S. M., Paton, D., Polanowski, A. M., et al. (2018). Humpback whale migrations to Antarctic summer foraging grounds through the southwest Pacific Ocean. Sci. Rep. 8:12333. doi: 10.1038/s41598-018-30748-30744
Angel, M., and Fasham, M. (1975). Analysis of the vertical and geographic distribution of the abundant species of planktonic ostracods in the North-east Atlantic. J. Mar. Biol. Assoc. 55, 709–737. doi: 10.1017/s0025315400017379
Angel, M. V. (1999). “Ostracoda,” in South Atlantic Zooplankton, ed. D. Boltovskoy (Leiden: Bakhuys Publishers), 815–868.
Anon (2017). “Second Performance Review of CCAMLR – Final Report of the Panel,” in Report submitted to the Commission for the Conservation of Antarctic Marine Living Resources. Hobart, TAS: CCAMLR. Available online at: https://www.ccamlr.org/en/organisation/second-ccamlr-performance-review (accessed July 01, 2021).
Ansorge, I. J., Froneman, P. W., Pakhomov, E. A., Lutjeharms, J. R. E., Perissinotto, R., and van Ballegooyen, R. C. (1999). Physical-biological coupling in the waters surrounding the Prince Edward Islands (Southern Ocean). Polar Biol. Biol. 21, 135–145. doi: 10.1007/s003000050344
Armstrong, R. A., Peterson, M. L., Lee, C., and Wakeham, S. G. (2009). Settling velocity spectra and the ballast ratio hypothesis. Deep Sea Res. Part II 56, 1470–1478. doi: 10.1016/j.dsr2.2008.11.032
Arrigo, K. R., Robinson, D. H., Worthen, D. L., Dunbar, R. B., DiTullio, G. R., VanWoert, M., et al. (1999). Phytoplankton community structure and the drawdown of nutrients and CO2 in the Southern Ocean. Science 283, 365–367. doi: 10.1126/science.283.5400.365
Ashford, J. R., Fach, B. A., Arkhipkin, A. I., and Jones, C. M. (2012). Testing early life connectivity supplying a marine fishery around the Falkland Islands. Fish. Res. 121, 144–152. doi: 10.1016/j.fishres.2012.01.023
Ashford, J. R., Jones, C. M., Hofmann, E., Everson, I., Moreno, C., Duhamel, G., et al. (2005). Can otolith elemental signatures record the capture site of Patagonian toothfish (Dissostichus eleginoides), a fully marine fish in the Southern Ocean? Can. J. Fish. Aquat. Sci. 62, 2832–2840. doi: 10.1139/f05-191
Ashford, J. R., Jones, C. M., Hofmann, E. E., Everson, I., Moreno, C. A., Duhamel, G., et al. (2008). Otolith chemistry indicates population structuring by the Antarctic Circumpolar Current. Can. J. Fish. Aquat. Sci. 65, 135–146. doi: 10.1139/f07-158
ATCM (2016). “Santiago declaration on the twenty fifth anniversary of the signing of the protocol on environmental protection to the antarctic treaty,” in Proceedings of the Declaration on 30th May 2016 at the XXXIX Antarctic Treaty Consultative Meeting, (Santiago).
Atkinson, A., and Sinclair, J. D. (2000). Zonal distribution and seasonal vertical migration of copepod assemblages in the Scotia Sea. Polar Biol. Biol. 23, 46–58.
Atkinson, A., Ward, P., Peck, J. M., and Murray, A. W. (1990). Mesoscale distribution of zooplankton around South Georgia. Deep Sea Res Part A. 37, 1213–1227.
Avila, C., Angulo-Preckler, C., Martín-Martín, R. P., Figuerola, B., Griffiths, H. J., and Waller, C. L. (2020). Invasive marine species discovered on non–native kelp rafts in the warmest Antarctic island. Sci. Rep. 10:1639. doi: 10.1038/s41598-020-58561-y
Azam, F., Smith, D. C., and Hollibaugh, J. T. (1991). The role of the microbial loop in antarctic pelagic ecosystems. Polar Res. 10, 239–243. doi: 10.1111/j.1751-8369.1991.tb00649.x
Barnes, D. K. A. (2002). Biodiversity - Invasions by marine life on plastic debris. Nature 416, 808–809. doi: 10.1038/416808a
Barnes, D. K. A., Hodgson, D. A., Convey, P., Allen, C. S., and Clarke, A. (2006). Incursion and excursion of Antarctic biota: past, present and future. Glob. Ecol. Biogeogr. 15, 121–142. doi: 10.1111/j.1466-822x.2006.00216.x
Barnes, D. K. A., Morley, S. A., Bell, J., Brewin, P., Brigden, K., Collins, M., et al. (2018). Marine plastics threaten giant Atlantic Marine Protected Areas. Curr. Biol. 28, R1137–R1138. doi: 10.1016/j.cub.2018.08.064
Barnes, D. K. A., Warren, N. L., Webb, K., Phalan, B., and Reid, K. (2004). Polar pedunculate barnacles piggy-back on pycnogona, penguins, pinniped seals and plastics. Mar. Ecol. Prog. Ser. 284, 305–310. doi: 10.3354/meps284305
Bauer, S., and Hoye, B. J. (2014). Migratory animals couple biodiversity and ecosystem functioning worldwide. Science 344:1242552. doi: 10.1126/science.1242552
Baylis, A. M., Tierney, M., Orben, R. A., Warwick-Evans, V., Wakefield, E., Grecian, W. J., et al. (2019). Important at-sea areas of colonial breeding marine predators on the southern patagonian shelf. Sci. Rep. 9:17452. doi: 10.1038/s41598-019-44695-44691
Bednarsek, N., Tarling, G. A., Bakker, D. C. E., Fielding, S., Jones, E. M., Venables, H. J., et al. (2012). Extensive dissolution of live pteropods in the Southern Ocean. Nat. Geosci. 5, 881–885. doi: 10.1038/ngeo1635
Bender, N. A., Crosbie, K., and Lynch, H. J. (2016). Patterns of tourism in the Antarctic Peninsula region: a 20-year analysis. Antarct. Sci. 28, 194–203. doi: 10.1017/s0954102016000031
Bernard, A. T. F., Ansorge, I. J., Froneman, P. W., Lutjeharms, J. R. E., Bernard, K. S., and Swart, N. C. (2007). Entrainment of antarctic euphausiids across the Antarctic polar front by a cold eddy. Deep-Sea Res. Part I 54, 1841–1851. doi: 10.1016/j.dsr.2007.06.007
Bertram, E., Muir, S., and Stonehouse, B. (2007). “Gateway ports in the development of Antarctic tourism,” in Prospects for Polar Tourism, eds J. Snyder and B. Stonehouse (Cambridge, MA: CABI Publications).
Best, P. B., Payne, R., Rowntree, V. J., Palazzo, J. T., and Both, M. D. (1993). Long-range movements of South Atlantic right whales (Eubalaena australis). Mar. Mamm. Sci. 9, 227–234. doi: 10.1111/j.1748-7692.1993.tb00451.x
Bestley, S., Andrews-Goff, V., van Wijk, E., Rintoul, S. R., Double, M. C., and How, J. (2019). New insights into prime Southern Ocean forage grounds for thriving Western Australian humpback whales. Sci. Rep. 9:13988. doi: 10.1038/s41598-019-50497-50492
Bestley, S., Ropert-Coudert, Y., Bengtson Nash, S., Brooks, C. M., Cotté, C., Dewar, M., et al. (2020). Marine ecosystem assessment for the southern ocean: birds and marine mammals in a changing climate. Front. Ecol. Evol. 8:566936. doi: 10.3389/fevo.2020.566936
Bolchoun, L., Drossel, B., and Allhoff, K. T. (2017). Spatial topologies affect local food web structure and diversity in evolutionary metacommunities. Sci. Rep. 7:1818. doi: 10.1038/s41598-017-01921-y
Boonzaier, L., and Pauly, D. (2016). Marine protection targets: an updated assessment of global progress. Oryx 50, 27–35. doi: 10.1017/s0030605315000848
Boyce, D. G., Tittensor, D. P., and Worm, B. (2008). Effects of temperature on global patterns of tuna and billfish richness. Mar. Ecol. Prog. Ser. 355, 267–276. doi: 10.3354/meps07237
Boyd, P. W. (2019). Physiology and iron modulate diverse responses of diatoms to a warming Southern Ocean. Nat. Clim. Change 9, 148–152. doi: 10.1038/s41558-018-0389-381
Boyd, P. W., Claustre, H., Levy, M., Siegel, D. A., and Weber, T. (2019). Multi-faceted particle pumps drive carbon sequestration in the ocean. Nature 568, 327–335. doi: 10.1038/s41586-019-1098-1092
Branch, T. A. (2007). Abundance of Antarctic blue whales south of 60°S from three complete circumpolar sets of surveys. J. Cetacean Res. Manag. 9, 253–262.
Branch, T. A. (2011). Humpback abundance south of 60°S from three complete circumpolar sets of surveys. J. Cetacean Res. Manag. 3, 53–70. doi: 10.47536/jcrm.vi.305
Branch, T. A., Matsuoka, K., and Miyashita, T. (2004). Evidence for increases in Antarctic blue whales based on Bayesian modelling. Mar. Mamm. Sci. 20, 726–754. doi: 10.1111/j.1748-7692.2004.tb01190.x
Branch, T. A., Stafford, K. M., Palacios, D. M., Allison, C., Bannister, J. L., Burton, C. L. K., et al. (2007). Past and present distribution, densities and movements of blue whales Balaenoptera musculus in the Southern Hemisphere and northern Indian Ocean. Mamm. Rev. 37, 116–175. doi: 10.1111/j.1365-2907.2007.00106.x
Bronselaer, B., Zanna, L., Munday, D. R., and Lowe, J. (2016). The influence of Southern Ocean winds on the North Atlantic carbon sink. Glob. Biogeochem. Cycles 30, 844–858. doi: 10.1002/2015gb005364
Bryden, H. (1983). “The Southern Ocean,” in Eddies in Marine Science, ed. A. Robinson (Berlin: Springer), 265–277.
Buesseler, K. O. (1998). The decoupling of production and particulate export in the surface ocean. Glob. Biogeochem. Cycles 12, 297–310. doi: 10.1029/97gb03366
Caccavo, J. A., Christiansen, H., Constable, A. J., Ghigliotti, L., Trebilco, R., Brooks, C. M., et al. (2021). Productivity and change in fish and squid in the Southern Ocean. Front. Ecol. Evol. 9:624918. doi: 10.3389/fevo.2021.624918
Carey, M. J., Phillips, R. A., Silk, J. R. D., and Shaffer, S. A. (2014). Trans-equatorial migration of short-tailed shearwaters revealed by geolocators. Emu 114, 352–359. doi: 10.1071/mu13115
Catry, P., Phillips, R. A., Phalan, B., Silk, J. R. D., and Croxall, J. P. (2004). Foraging strategies of grey-headed albatrosses Thalassarche chrysostoma: integration of movements, activity and feeding events. Mar. Ecol. Prog. Ser. 280, 261–273. doi: 10.3354/meps280261
Catry, P., Poisbleau, M., Lecoq, M., and Phillips, R. A. (2013). Differences in the timing and extent of annual moult of black-browed albatrosses Thalassarche melanophris living in contrasting environments. Polar Biol. Biol. 36, 837–842. doi: 10.1007/s00300-013-1309-1305
Catul, V., Gauns, M., and Karuppasamy, P. K. (2011). A review on mesopelagic fishes belonging to family Myctophidae. Rev. Fish Biol. Fish. 21, 339–354. doi: 10.1007/s11160-010-9176-9174
Cavan, E. L., Belcher, A., Atkinson, A., Hill, S. L., Kawaguchi, S., McCormack, S., et al. (2019). The importance of Antarctic krill in biogeochemical cycles. Nat. Commun. 10:4742. doi: 10.1038/s41467-019-12668-12667
Cavanagh, R. D., Melbourne-Thomas, J., Grant, S. M., Barnes, D. K. A., Hughes, K. A., Halfter, S., et al. (2021a). Future risk for southern ocean ecosystem services under climate change. Front. Mar. Sci. 7:592027. doi: 10.3389/fevo.2021.592027
Cavanagh, R. D., Trathan, P. N., Hill, S. L., Melbourne-Thomas, J., Meredith, M. P., Hollyman, P., et al. (2021b). Utilising IPCC assessments to support the ecosystem approach to fisheries management within a warming Southern Ocean. Mar. Pol. 131:104589. doi: 10.1016/j.marpol.2021.104589
CCAMLR (2016). “The value of marine resources harvested in the CCAMLR Convention Area – an assessment of GVP,” in Commission for the Conservation of Antarctic Marine Living Resources, (Hobart, TAS).
Ceia, F. R., Ramos, J. A., Phillips, R. A., Cherel, Y., Jones, D. C., Vieira, R. P., et al. (2015). Analysis of stable isotope ratios in blood of tracked wandering albatrosses fails to distinguish a C-13 gradient within their winter foraging areas in the southwest Atlantic Ocean. Rapid Commun. Mass Spectrom. 29, 2328–2336. doi: 10.1002/rcm.7401
Cerovecki, I., Meijers, A. J. S., Mazloff, M. R., Gille, S. T., Tamsitt, V. M., and Holland, P. R. (2019). The effects of enhanced sea ice export from the ross sea on recent cooling and freshening of the Southeast pacific. J. Clim. 32, 2013–2035. doi: 10.1175/jcli-d-18-0205.1
Chapman, C. C., Lea, M.-A., Meyer, A., Sallée, J.-B., and Hindell, M. (2020). Defining Southern Ocean fronts and their influence on biological and physical processes in a changing climate. Nat. Clim. Change 10, 209–219. doi: 10.1038/s41558-020-0705-704
Chen, L. B., DeVries, A. L., and Cheng, C. H. C. (1997). Convergent evolution of antifreeze glycoproteins in Antarctic notothenioid fish and Arctic cod. Proc. Natl. Acad Sci. U S A. 94, 3817–3822. doi: 10.1073/pnas.94.8.3817
Chiba, S., Ishimaru, T., Hosie, G. W., and Fukuchi, M. (2001). Spatio-temporal variability of zooplankton community structure off east Antarctica (90 to 160 degrees E). Mar. Ecol. Prog. Ser. 216, 95–108. doi: 10.3354/meps216095
Chown, S. L., and Brooks, C. M. (2019). The state and future of Antarctic environments in a global context. Annu. Rev. Environ. Resour. 44, 1–30. doi: 10.1146/annurev-environ-101718-133236
Chown, S. L., Clarke, A., Fraser, C. I., Cary, S. C., Moon, K. L., and McGeoch, M. A. (2015). The changing form of Antarctic biodiversity. Nature 522, 431–438. doi: 10.1038/nature14505
Chown, S. L., Lee, J. E., Hughes, K. A., Barnes, J., Barrett, P. J., Bergstrom, D. M., et al. (2012). Challenges to the future conservation of the Antarctic. Science 337, 158–159. doi: 10.1126/science.1222821
Christiansen, H., Dettai, A., Heindler, F. M., Collins, M. A., Duhamel, G., Hautecoeur, M., et al. (2018). Diversity of mesopelagic fishes in the Southern Ocean - a phylogeographic perspective using DNA barcoding. Front. Ecol. Evol. 6:120. doi: 10.3389/fevo.2018.00120
Clark, C. W., and Mangel, M. (2000). Dynamic State Variable Models in Ecology: Methods and Applications. New York: NY: Oxford University Press.
Clarke, A., Barnes, D. K. A., and Hodgson, D. A. (2005). How isolated is Antarctica? Trends Ecol. Evol. 20, 1–3. doi: 10.1016/j.tree.2004.10.004
Clarke, A., and Crame, J. A. (2010). Evolutionary dynamics at high latitudes: speciation and extinction in polar marine faunas. Phil. Trans. Roy. Soc. B 365, 3655–3666. doi: 10.1098/rstb.2010.0270
Clarke, A., and Johnston, N. M. (2003). Antarctic marine benthic diversity. Oceanogr. Mar. Biol. 41, 47–114.
Clay, T. A., Manica, A., Ryan, P. G., Silk, J. R., Croxall, J. P., Ireland, L., et al. (2016). Proximate drivers of spatial segregation in non-breeding albatrosses. Sci. Rep. 6:29932. doi: 10.1038/srep29932
Clay, T. A., Pearmain, E. J., McGill, R. A., Manica, A., and Phillips, R. A. (2018). Age-related variation in non-breeding foraging behaviour and carry-over effects on fitness in an extremely long-lived bird. Func. Ecol. 32, 1832–1846. doi: 10.1111/1365-2435.13120
Constable, A. J., Melbourne-Thomas, J., Corney, S. P., Arrigo, K. R., Barbraud, C., Barnes, D. K. A., et al. (2014). Climate change and Southern Ocean ecosystems I: how changes in physical habitats directly affect marine biota. Glob. Change Biol. 20, 3004–3025. doi: 10.1111/gcb.12623
Convey, P., Stevens, M. I., Hodgson, D. A., Smellie, J. L., Hillenbrand, C.-D., Barnes, D. K. A., et al. (2009). Exploring biological constraints on the glacial history of Antarctica. Q. Sci. Rev. 28, 3035–3048. doi: 10.1016/j.quascirev.2009.08.015
Cossa, D., Heimburger, L. E., Lannuzel, D., Rintoul, S. R., Butler, E. C. V., Bowie, A. R., et al. (2011). Mercury in the Southern Ocean. Geochim. Cosmochim. Acta 75, 4037–4052. doi: 10.1016/j.gca.2011.05.001
Costa, D. P., Breed, G. A., and Robinson, P. W. (2012). New insights into pelagic migrations: implications for ecology and conservation. Ann. Rev. Ecol., Evol. Syst. 43, 73–96. doi: 10.1146/annurev-ecolsys-102710-145045
Croxall, J. P., Prince, P. A., and Ricketts, C. (1985). “Relationships between prey life-cycles and the extent, nature and timing of seal and seabird predation in the Scotia Sea,” in Antarctic Nutrient Cycles and Food Webs, eds W. R. Siegfried, P. R. Condy, and R. M. Laws (Berlin: Springer-Verlag).
Croxall, J. P., Silk, J. R. D., Phillips, R. A., Afanasyev, V., and Briggs, D. R. (2005). Global circumnavigations: tracking year-round ranges of nonbreeding albatrosses. Science 307, 249–250. doi: 10.1126/science.1106042
Croxall, J. P., and Wood, A. G. (2002). The importance of the Patagonian shelf for top predator species breeding at South Georgia. Aquat. Cons. Mar. Freshw. Ecosyst. 12, 101–118. doi: 10.1002/aqc.480
Damerau, M., Matschiner, M., Salzburger, W., and Hanel, R. (2012). Comparative population genetics of seven notothenioid fish species reveals high levels of gene flow along ocean currents in the southern Scotia Arc. Antarctica. Polar Biol. 35, 1073–1086. doi: 10.1007/s00300-012-1155-x
de Baar, H. J. W., Buma, A. G. J., Nolting, R. F., Cadée, G. C., Jacques, G., and Tréguer, P. J. (1990). On iron limitation of the Southern Ocean: experimental observations in the Weddell and Scotia Seas. Mar. Ecol. Prog. Ser. 65, 105–122. doi: 10.3354/meps065105
Deacon, G. E. R. (1982). Physical and biological zonation in the Southern Ocean. Deep Sea Res. Part A. 29, 1–15. doi: 10.1016/0198-0149(82)90058-90059
Del Castillo, C. E., Signorini, S. R., Karaköylü, E. M., and Rivero-Calle, S. (2019). Is the Southern Ocean getting greener? Geophys. Res. Lett. 46, 6034–6040. doi: 10.1029/2019GL083163
Della Penna, A., De Monte, S., Kestenare, E., Guinet, C., and d’Ovidio, F. (2015). Quasi-planktonic behavior of foraging top marine predators. Sci. Rep. 5:18063. doi: 10.1038/srep18063
Della Penna, A., Trull, T. W., Wotherspoon, S., De Monte, S., Johnson, C. R., and d’Ovidio, F. (2018). Mesoscale variability of conditions favoring an iron-induced diatom bloom downstream of the kerguelen plateau. J. Geophys. Res. Oceans 123, 3355–3367. doi: 10.1029/2018jc013884
Delord, K., Barbraud, C., Bost, C.-A., Deceuninck, B., Lefebvre, T., Lutz, R., et al. (2014). Areas of importance for seabirds tracked from French southern territories, and recommendations for conservation. Mar. Pol. 48, 1–13. doi: 10.1016/j.marpol.2014.02.019
DeVries, T. (2014). The oceanic anthropogenic CO2 sink: storage, air-sea fluxes, and transports over the industrial era. Glob. Biogeochem. Cycles 28, 631–647. doi: 10.1002/2013gb004739
Dias, M. P., Oppel, S., Bond, A. L., Carneiro, A. P. B., Cuthbert, R. J., Gonzalez-Solis, J., et al. (2017). Using globally threatened pelagic birds to identify priority sites for marine conservation in the South Atlantic Ocean. Biol. Conserv. 211, 76–84. doi: 10.1016/j.biocon.2017.05.009
Dodds, K. (2019). Reflecting on the 60th anniversary of the Antarctic Treaty. Polar Rec. 55, 311–316. doi: 10.1017/S0032247419000536
Doughty, C. E., Roman, J., Faurby, S., Wolf, A., Haque, A., Bakker, E. S., et al. (2016). Global nutrient transport in a world of giants. Proc. Natl. Acad. Sci. U S A. 113, 868–873. doi: 10.1073/pnas.1502549112
Dubois, M., Rossi, V., Ser-Giacomi, E., Arnaud-Haond, S., Lopez, C., and Hernandez-Garcia, E. (2016). Linking basin-scale connectivity, oceanography and population dynamics for the conservation and management of marine ecosystems. Glob. Ecol. Biogeogr. 25, 503–515. doi: 10.1111/geb.12431
Ducklow, H. W., Steinber, D. K., and Buesseler, K. O. (2001). Upper Ocean carbon export and the biological pump. Oceanography 14, 51–58. doi: 10.5670/oceanog.2001.06
Dufour, C. O., Griffies, S. M., de Souza, G. F., Frenger, I., Morrison, A. K., Palter, J. B., et al. (2015). Role of mesoscale eddies in cross-frontal transport of heat and biogeochemical tracers in the Southern Ocean. J. Phys. Oceanogr. 45, 3057–3081. doi: 10.1175/jpo-d-14-0240.1
Dunn, D. C., Harrison, A. L., Curtice, C., DeLand, S., Donnelly, B., Fujioka, E., et al. (2019a). The importance of migratory connectivity for global ocean policy. Proc. Roy Soc. B 286:20191472. doi: 10.1098/rspb.2019.1472
Dunn, M. J., Forcada, J., Jackson, J. A., Waluda, C. M., Nichol, C., and Trathan, P. N. (2019b). A long-term study of gentoo penguin (Pygoscelis papua) population trends at a major Antarctic tourist site, Goudier Island, Port Lockroy. Biodivers. Conserv. 28, 37–53. doi: 10.1007/s10531-018-1635-1636
Egevang, C., Stenhouse, I. J., Phillips, R. A., Petersen, A., Fox, J. W., and Silk, J. R. D. (2010). Tracking of Arctic terns Sterna paradisaea reveals longest animal migration. Proc. Nati. Acad Sci. U S A. 107, 2078–2081. doi: 10.1073/pnas.0909493107
Eyring, V., Bony, S., Meehl, G. A., Senior, C. A., Stevens, B., Stouffer, R. J., et al. (2016). Overview of the coupled model intercomparison project phase 6 (CMIP6) experimental design and organization. Geosci. Model Dev. 9, 1937–1958. doi: 10.5194/gmd-9-1937-2016
FAO (2018). The Future of Food and Agriculture – Alternative Pathways to 2050. Summary Version. Rome: FAO.
Farreny, R., Oliver-Sola, J., Lamers, M., Amelung, B., Gabarrell, X., Rieradevall, J., et al. (2011). Carbon dioxide emissions of Antarctic tourism. Ant. Sci. 23, 556–566. doi: 10.1017/s0954102011000435
Fasham, M., and Foxton, P. (1979). Zonal distribution of pelagic Decapoda (Crustacea) in the eastern North Atlantic and its relation to the physical oceanography. J. Exp.Mar. Biol. Ecol. 37, 225–253. doi: 10.1016/0022-0981(79)90062-90065
Feely, R. A., Doney, S. C., and Cooley, S. R. (2009). Ocean acidification: present conditions and future changes in a High-CO2 World. Oceanography 22, 36–47. doi: 10.5670/oceanog.2009.95
Félix, F., and Guzmán, H. M. (2014). Satellite tracking and sighting data analyses of Southeast Pacific humpback whales (Megaptera novaeangliae): is the migratory route coastal or oceanic? Aquat. Mamm. 40, 329–340. doi: 10.1578/am.40.4.2014.329
Ferster, B. S., Subrahmanyam, B., Fukumori, I., and Nyadjro, E. S. (2018). Variability of Southern Ocean Transports. J. Phys. Oceanogr. 48, 2667–2688. doi: 10.1175/jpo-d-18-0055.1
Fletcher, S. E. M., Gruber, N., Jacobson, A. R., Doney, S. C., Dutkiewicz, S., Gerber, M., et al. (2006). Inverse estimates of anthropogenic CO2 uptake, transport, and storage by the ocean. Glob. Biogeochem. Cycles 20:GB2002. doi: 10.1029/2005gb002530
Foppert, A., Donohue, K. A., Watts, D. R., and Tracey, K. L. (2017). Eddy heat flux across the Antarctic Circumpolar current estimated from sea surface height standard deviation. J. Geophys. Res. Oceans 122, 6947–6964. doi: 10.1002/2017jc012837
Foppert, A., Rintoul, S. R., and England, M. H. (2019). Along-Slope variability of cross-slope eddy transport in East Antarctica. Geophys. Res. Lett. 46, 8224–8233. doi: 10.1029/2019gl082999
Fossette, S., Heide-Jorgensen, M. P., Jensen, M. V., Kiszka, J., Bernbe, M., Bertrand, N., et al. (2014). Humpback whale (Megaptera novaeangliae) post breeding dispersal and southward migration in the western Indian Ocean. J. Exp.Mar. Biol. Ecol. 450, 6–14. doi: 10.1016/j.jembe.2013.10.014
Fraser, C. I., Morrison, A. K., Hogg, A. M., Macaya, E. C., van Sebille, E., Ryan, P. G., et al. (2018). Antarctica’s ecological isolation will be broken by storm-driven dispersal and warming. Nat. Clim. Change 8, 704–708. doi: 10.1038/s41558-018-0209-207
Fraser, C. I., Nikula, R., Ruzzante, D. E., and Waters, J. M. (2012). Poleward bound: biological impacts of Southern Hemisphere glaciation. Trends Ecol. Evol. 27, 462–471. doi: 10.1016/j.tree.2012.04.011
Fraser, C. I., Terauds, A., Smellie, J., Convey, P., and Chown, S. L. (2014). Geothermal activity helps life survive glacial cycles. Proc. Nati. Acad Sci. U S A. 111, 5634–5639. doi: 10.1073/pnas.1321437111
Frenger, I., Münnich, M., Gruber, N., and Knutti, R. (2015). Southern Ocean eddy phenomenology. J. Geophys. Res. Oceans 120, 7413–7449. doi: 10.1002/2015JC011047
Frölicher, T. L., Sarmiento, J. L., Paynter, D. J., Dunne, J. P., Krasting, J. P., and Winton, M. (2015). Dominance of the Southern Ocean in anthropogenic carbon and heat uptake in CMIP5 models. J. Clim. 28, 862–886. doi: 10.1175/jcli-d-14-00117.1
Froneman, P., McQuaid, C., and Perissinotto, R. (1995). Biogeographic structure of the microphytoplankton assemblages of the south Atlantic and Southern Ocean during austral summer. J. Plank. Res. 17, 1791–1802. doi: 10.1093/plankt/17.9.1791
Froneman, P. W., Ansorge, I. J., Pakhomovo, E. A., and Lutjeharms, J. R. E. (1999). Plankton community structure in the physical environment surrounding the Prince Edward Islands (Southern Ocean). Polar Biol. 22, 145–155. doi: 10.1007/s003000050404
Garrigue, C., Clapham, P. J., Geyer, Y., Kennedy, A. S., and Zerbini, A. N. (2015). Satellite tracking reveals novel migratory patterns and the importance of seamounts for endangered South Pacific humpback whales. R. Soc. Open Sci. 2:150489. doi: 10.1098/rsos.150489
Garzoli, S. L., and Matano, R. (2011). The South Atlantic and the Atlantic meridional overturning circulation. Deep Sea Res. Part II 58, 1837–1847. doi: 10.1016/j.dsr2.2010.10.063
Gjosaeter, H., and Kawaguchi, K. (1980). “A review of the world resources of mesopelagic fish,” in Food and Agriculture Organization of the United Nations, Fisheries Technical Paper FAO, (Rome: FAO).
Gounand, I., Harvey, E., Little, C. J., and Altermatt, F. (2018a). Meta-Ecosystems 2.0: rooting the theory into the field. Trends Ecol. Evol. 33, 36–46. doi: 10.1016/j.tree.2017.10.006
Gounand, I., Little, C. J., Harvey, E., and Altermatt, F. (2018b). Cross-ecosystem carbon flows connecting ecosystems worldwide. Nat. Commun. 9:94825. doi: 10.1038/s41467-018-07238-7232
Granroth-Wilding, H. M., and Phillips, R. A. (2019). Segregation in space and time explains the coexistence of two sympatric sub-Antarctic petrels. IBIS 161, 101–116. doi: 10.1111/ibi.12584
Grant, S., Waller, C. L., Morley, S. A., Barnes, D. K. A., Brasier, M. J., and Double, M. C. (2021). Local drivers of change in Southern Ocean ecosystems: human activities and policy implications. Front. Ecol. Evol. 26:624518. doi: 10.3389/fevo.2021.624518
Grant, S. M., Hill, S. L., Trathan, P. N., and Murphy, E. J. (2013). Ecosystem services of the Southern Ocean: trade-offs in decision-making. Ant. Sci. 25, 603–617. doi: 10.1017/s0954102013000308
Griffiths, H. J., Barnes, D. K., and Linse, K. (2009). Towards a generalized biogeography of the Southern Ocean benthos. J. Biogeogr. 36, 162–177. doi: 10.1111/j.1365-2699.2008.01979.x
Griffiths, H. J., Meijers, A. J. S., and Bracegirdle, T. J. (2017). More losers than winners in a century of future Southern Ocean seafloor warming. Nat. Clim. Change 7, 749–754. doi: 10.1038/nclimate3377
Gruber, N., Landschützer, P., and Lovenduski, N. S. (2019). The variable southern ocean carbon sink. Ann. Rev. Mar. Sci. 11, 159–186. doi: 10.1146/annurev-marine-121916-163407
Guerreiro, M., Phillips, R. A., Cherel, Y., Ceia, F. R., Alvito, P., Rosa, R., et al. (2015). Habitat and trophic ecology of Southern Ocean cephalopods from stable isotope analyses. Mar. Ecol. Prog. Ser. 530, 119–134. doi: 10.3354/meps11266
Guihou, K., Piola, A., Palma, E. D., and Chidichimo, M. P. (2020). Dynamical connections between large marine ecosystems of austral South America based on numerical simulations. Ocean Sci. 16, 271–290. doi: 10.5194/os-16-271-2020
Gutt, J., Bertler, N., Bracegirdle, T. J., Buschmann, A., Comiso, J., Hosie, G., et al. (2015). The Southern Ocean ecosystem under multiple climate change stresses - an integrated circumpolar assessment. Glob. Change Biol. 21, 1434–1453. doi: 10.1111/gcb.12794
Guzman, H. M., Condit, R., Pérez-Ortega, B., Capella, J. J., and Stevick, P. T. (2015). Population size and migratory connectivity of humpback whales wintering in Las Perlas Archipelago, Panama. Mar. Mamm. Sci. 31, 90–105. doi: 10.1111/mms.12136
Hanifah, N. A., Shah, R. M., and Hashim, R. (2012). “Impact of human activities in antarctica: dissecting the concepts of ecologically sustainable development and natural reserve for peace and science,” in Proceedings of the International Conference on Environmental Science and Development, eds Y. Dan and H. Kong 177–181. (Amsterdam: Elsevier). doi: 10.1016/j.apcbee.2012.03.028
Hauck, J., Lenton, A., Langlais, C., and Matear, R. (2018). The fate of carbon and nutrients exported out of the Southern Ocean. Glob. Biogeochem. Cycles 32, 1556–1573. doi: 10.1029/2018gb005977
Hauck, J., Volker, C., Wolf-Gladrow, D. A., Laufkotter, C., Vogt, M., Aumont, O., et al. (2015). On the Southern Ocean CO2 uptake and the role of the biological carbon pump in the 21st century. Glob. Biogeochem. Cycles 29, 1451–1470. doi: 10.1002/2015gb005140
Hauri, C., Friedrich, T., and Timmermann, A. (2016). Abrupt onset and prolongation of aragonite undersaturation events in the Southern Ocean. Nat. Clim. Change 6, 172–176. doi: 10.1038/nclimate2844
Hays, G. C. (2017). Ocean currents and marine life. Curr. Biol. 27, R470–R473. doi: 10.1016/j.cub.2017.01.044
Henley, S. F., Cavan, E. L., Fawcett, S. E., Kerr, R., Monteiro, T., Sherrell, R. M., et al. (2020). Changing biogeochemistry of the Southern Ocean and its ecosystem implications. Front. Mar. Sci. 7:581. doi: 10.3389/fmars.2020.00581
Heywood, R., Everson, I., and Priddle, J. (1985). The absence of krill from the South Georgia zone, winter 1983. Deep Sea Res. Part A. 32, 369–378. doi: 10.1016/0198-0149(85)90085-90088
Hindell, M. A., Reisinger, R. R., Ropert-Coudert, Y., Hückstädt, L. A., Trathan, P. N., Bornemann, H., et al. (2020). Tracking of marine predators to protect Southern Ocean ecosystems. Nature 580, 87–92. doi: 10.1038/s41586-020-2126-y
Holzer, M., and Primeau, F. W. (2013). Global teleconnections in the oceanic phosphorus cycle: patterns, paths, and timescales. J. Geophys. Res. Oceans 118, 1775–1796. doi: 10.1002/jgrc.20072
Hop, H., and Gjosaeter, H. (2013). Polar cod (Boreogadus saida) and capelin (Mallotus villosus) as key species in marine food webs of the Arctic and the Barents Sea. Mar. Biol. Res. 9, 878–894. doi: 10.1080/17451000.2013.775458
Horton, A. A., and Barnes, D. K. A. (2020). Microplastic pollution in a rapidly changing world: implications for remote and vulnerable marine ecosystems. Sci. Total Environ. 738:140349. doi: 10.1016/j.scitotenv.2020.140349
Horton, T. W., Hauser, N., Zerbini, A. N., Francis, M. P., Domeier, M. L., Andriolo, A., et al. (2017). Route fidelity during marine megafauna migration. Front. Mar. Sci. 4:422. doi: 10.3389/fmars.2017.00422
Horton, T. W., Zerbini, A. N., Andriolo, A., Danilewicz, D., and Sucunza, F. (2020). Multi-Decadal humpback whale migratory route fidelity despite oceanographic and geomagnetic change. Front. Mar. Sci. 7:414. doi: 10.3389/fmars.2020.00414
Hughes, K. A., Constable, A., Frenot, Y., Lopez-Martinez, J., McIvor, E., Njastad, B., et al. (2018). Antarctic environmental protection: strengthening the links between science and governance. Environ. Sci. Policy 83, 86–95. doi: 10.1016/j.envsci.2018.02.006
Hughes, K. A., and Convey, P. (2020). Implications of the COVID-19 pandemic for Antarctica. Ant. Sci. 32, 426–439. doi: 10.1017/S095410202000053X
Hughes, K. A., Pescott, O. L., Peyton, J., Adriaens, T., Cottier-Cook, E. J., Key, G., et al. (2020). Invasive non-native species likely to threaten biodiversity and ecosystems in the Antarctic Peninsula region. Glob. Change Biol. 26, 2702–2716. doi: 10.1111/gcb.14938
Hulley, P. A. (1981). Results of the research cruises of FRV walther herwig to South America. Arch. Fischereiw. 31, 1–300.
Hunt, B., Pakhomov, E., and McQuaid, C. (2001). Short-term variation and long-term changes in the oceanographic environment and zooplankton community in the vicinity of a sub-Antarctic archipelago. Mar. Biol. 138, 369–381. doi: 10.1007/s002270000467
Hunt, B. P. V., Pakhomov, E. A., and McQuaid, C. D. (2002). Community structure of mesozooplankton in the Antarctic polar frontal zone in the vicinity of the Prince Edward Islands (Southern Ocean): small-scale distribution patterns in relation to physical parameters. Deep Sea Res. Part II 49, 3307–3325. doi: 10.1016/s0967-0645(02)00085-81
Hunt, G. L., Drinkwater, K. F., Arrigo, K., Berge, J., Daly, K. L., Danielson, S., et al. (2016). Advection in polar and sub-polar environments: impacts on high latitude marine ecosystems. Prog. Oceanogr. 149, 40–81. doi: 10.1016/j.pocean.2016.10.004
Huserbraten, M. B. O., Eriksen, E., Gjosaeter, H., and Vikebo, F. (2019). Polar cod in jeopardy under the retreating Arctic sea ice. Commun. Biol. 2:407. doi: 10.1038/s42003-019-0649-642
Hutchins, D. A., and Bruland, K. W. (1998). Iron-limited diatom growth and Si : N uptake ratios in a coastal upwelling regime. Nature 393, 561–564. doi: 10.1038/31203
IAATO (2019). “IAATO overview of Antarctic Tourism: 2018-19 season and preliminary estimates for 2019-20 season,” in Proceedings of the Antarctic Treaty Consultative Meeting XLII, 1 -11 July, 2019, (Prague).
IPCC (2019). “IPCC special report on the ocean and cryosphere,” in A Changing Climate, eds H.-O. Poörtner, D. C. Roberts, V. Masson-Delmotte, P. Zhai, M. Tignor, E. Poloczanska, et al. (Geneva: IPCC).
Irigoien, X., Klevjer, T. A., Rostad, A., Martinez, U., Boyra, G., Acuna, J. L., et al. (2014). Large mesopelagic fishes biomass and trophic efficiency in the open ocean. Nat. Commun. 5:3271. doi: 10.1038/ncomms4271
Ito, T., Woloszyn, M., and Mazloff, M. (2010). Anthropogenic carbon dioxide transport in the Southern Ocean driven by Ekman flow. Nature 463, 80–83. doi: 10.1038/nature08687
IWC (2013). Annex G: report of the sub-committee on in-depth assessments. J. Cetacean Res. Manag. 14, 195–213.
IWC (2016). Annex H: report of the sub-committee on other Southern hemisphere whale stocks. J. Cetacean Res. Manag. 17, 250–282.
Jackson, J. A., Carroll, E. L., Smith, T. D., Zerbini, A. N., Patenaude, N. J., and Scott Baker, C. (2016). An integrated approach to historical population assessment of the great whales: case of the New Zealand southern right whale. R. Soc. Open Sci. 3, 150669–150669. doi: 10.1098/rsos.150669
Jackson, J. A., Patenaude, N. J., Carroll, E. L., and Baker, C. S. (2008). How many whales were there after whaling? inference from contemporary mtDNA diversity. Mol. Ecol. 17, 236–251. doi: 10.1111/j.1365-294X.2007.03497.x
Jiménez, S., Domingo, A., Brazeiro, A., Defeo, O., Wood, A. G., Froy, H., et al. (2016). Sex-related variation in the vulnerability of wandering albatrosses to pelagic longline fleets. Anim.Conserv. 19, 281–295. doi: 10.1111/acv.12245
Jones, C. W., Phillips, R. A., Grecian, W. J., and Ryan, P. G. (2020). Ecological segregation of two superabundant, morphologically similar, sister seabird taxa breeding in sympatry. Mar. Biol. 167:45. doi: 10.1007/s00227-020-3645-3647
Jones, D. C., Meijers, A. J. S., Shuckburgh, E., Sallee, J. B., Haynes, P., McAufield, E. K., et al. (2016). How does subantarctic mode water ventilate the southern hemisphere subtropics? J. Geophys. Res. Oceans 121, 6558–6582. doi: 10.1002/2016jc011680
Keller, D. P., Kriest, I., Koeve, W., and Oschlies, A. (2016). Southern Ocean biological impacts on global ocean oxygen. Geophys. Res. Lett. 43, 6469–6477. doi: 10.1002/2016gl069630
Kenchington, E., Wang, Z. L., Lirette, C., Murillo, F. J., Guijarro, J., Yashayaev, I., et al. (2019). Connectivity modelling of areas closed to protect vulnerable marine ecosystems in the northwest Atlantic. Deep-Sea Res. Part I 143, 85–103. doi: 10.1016/j.dsr.2018.11.007
Kirby, J. S., Stattersfield, A. J., Butchart, S. H. M., Evans, M. I., Grimmett, R. F. A., Jones, V. R., et al. (2008). Key conservation issues for migratory land- and waterbird species on the world’s major flyways. Bird Conserv. Int. 18, S49–S73. doi: 10.1017/s0959270908000439
Kopp, M., Peter, H.-U., Mustafa, O., Lisovski, S., Ritz, M. S., Phillips, R. A., et al. (2011). South polar skuas from a single breeding population overwinter in different oceans though show similar migration patterns. Mar. Ecol. Prog. Ser. 435, 263–267. doi: 10.3354/meps09229
Koubbi, P., Moteki, M., Duhamel, G., Goarant, A., Hulley, P. A., O’Driscoll, R., et al. (2011). Ecoregionalization of myctophid fish in the Indian sector of the Southern Ocean: results from generalized dissimilarity models. Deep-Sea Res. Part II 58, 170–180. doi: 10.1016/j.dsr2.2010.09.007
Landers, T. J., Rayner, M. J., Phillips, R. A., and Hauber, M. E. (2011). Dynamics of seasonal movements by a trans-Pacific migrant, the Westland petrel Procellaria westlandica. Condor 113, 71–79. doi: 10.1525/cond.2011.100064
Larson, E. J. (2011). An Empire of Ice: Scott, Shackleton, and the Heroic Age of Antarctic Science. New Haven, CT: Yale University Press.
Lavery, T. J., Roudnew, B., Gill, P., Seymour, J., Seuront, L., Johnson, G., et al. (2010). Iron defecation by sperm whales stimulates carbon export in the Southern Ocean. Proc. of the Roy. Soc. B. 277, 3527–3531. doi: 10.1098/rspb.2010.0863
Le Quéré, C., Buitenhuis, E. T., Moriarty, R., Alvain, S., Aumont, O., Bopp, L., et al. (2016). Role of zooplankton dynamics for Southern Ocean phytoplankton biomass and global biogeochemical cycles. Biogeosciences 13, 4111–4133. doi: 10.5194/bg-13-4111-2016
Leaper, R., and Lavigne, D. (2007). How much do large whales eat? J. Cetacean Res. Manag. 9, 179–188.
Lee, J. E., and Chown, S. L. (2009). Temporal development of hull-fouling assemblages associated with an Antarctic supply vessel. Mar. Ecol. Prog. Ser. 386, 97–105. doi: 10.3354/meps08074
Leung, S., Cabre, A., and Marinov, I. (2015). A latitudinally banded phytoplankton response to 21st century climate change in the Southern Ocean across the CMIP5 model suite. Biogeosciences 12, 5715–5734. doi: 10.5194/bg-12-5715-2015
Lewis, P. N., Hewitt, C. L., Riddle, M., and McMinn, A. (2003). Marine introductions in the Southern Ocean: an unrecognised hazard to biodiversity. Mar. Pollut. Bull. 46, 213–223. doi: 10.1016/s0025-326x(02)00364-368
Lockyer, C. (1981). Growth and Energy Budgets of Large Baleen Whales from the Southern Hemisphere. Rome: FAO, 379–487.
Loeb, V. J., Kellermann, A. K., Koubbi, P., North, A. W., and White, M. G. (1993). Antarctic larval fish assemblages - a review. Bull. Mar. Sci. 53, 416–449.
Lubimova, T., Shust, K., and Popkov, V. (1987). Specific features in the ecology of Southern Ocean mesopelagic fish of the family Myctophidae. Moscow: Nauka Press.
Lumpkin, R., and Speer, K. (2007). Global ocean meridional overturning. J. Phys. Oceanogr. 37, 2550–2562. doi: 10.1175/jpo3130.1
Lutjeharms, J., Walters, N., and Allanson, B. (1985). “Oceanic frontal systems and biological enhancement,” in Antarctic Nutrient Cycles and Food Webs, eds W. R. Siegfried, P. R. Condy, and R. M. Laws (Berlin: Springer), 11–21. doi: 10.1007/978-3-642-82275-9_3
Lutjeharms, J. R. E., and Baker, D. J. Jr. (1980). A statistical analysis of the meso-scale dynamics of the Southern Ocean. Deep Sea Res. Part A. 27, 145–159. doi: 10.1016/0198-0149(80)90093-x
Maas, E. W., Law, C. S., Hall, J. A., Pickmere, S., Currie, K. I., Chang, F. H., et al. (2013). Effect of ocean acidification on bacterial abundance, activity and diversity in the Ross Sea, Antarctica. Aquat. Microb. Ecol. 70, 1–15. doi: 10.3354/ame01633
MacGilchrist, G., Garabato, A. C. N., Brown, P. J., Jullion, L., Bacon, S., Bakker, D. C. E., et al. (2019). Reframing the carbon cycle of the subpolar Southern Ocean. Sci. Adv. 5:eaav6410. doi: 10.1126/sciadv.aav6410
Mackay, A. I., Bailleul, F., Carroll, E. L., Andrews-Goff, V., Baker, C. S., Bannister, J., et al. (2020). Satellite derived offshore migratory movements of southern right whales (Eubalaena australis) from Australian and New Zealand wintering grounds. PLoS One 15:e0231577. doi: 10.1371/journal.pone.0231577
Mann, K. H., and Lazier, J. R. N. (2005). Marine Ecology Comes of Age, in Dynamics of Marine Ecosystems. Hoboken, NJ: Wiley, doi: 10.1002/9781118687901.ch1
Marinov, I., Gnanadesikan, A., Toggweiler, J. R., and Sarmiento, J. L. (2006). The Southern Ocean biogeochemical divide. Nature 441, 964–967. doi: 10.1038/nature04883
Marshall, J., and Speer, K. (2012). Closure of the meridional overturning circulation through Southern Ocean upwelling. Nat. Geosci. 5, 171–180. doi: 10.1038/ngeo1391
Martiny, A. C., Pham, C. T. A., Primeau, F. W., Vrugt, J. A., Moore, J. K., Levin, S. A., et al. (2013). Strong latitudinal patterns in the elemental ratios of marine plankton and organic matter. Nat. Geosci. 6, 279–283. doi: 10.1038/ngeo1757
Matschiner, M., Hanel, R., and Salzburger, W. (2011). On the origin and trigger of the notothenioid adaptive radiation. PLoS One 6:e0018911. doi: 10.1371/journal.pone.0018911
McCann, K. S., Rasmussen, J. B., and Umbanhowar, J. (2005). The dynamics of spatially coupled food webs. Ecol. Lett. 8, 513–523. doi: 10.1111/j.1461-0248.2005.00742.x
McCarthy, A. H., Peck, L. S., Hughes, K. A., and Aldridge, D. C. (2019). Antarctica: the final frontier for marine biological invasions. Glob. Chang. Biol. 25, 2221–2241. doi: 10.1111/gcb.14600
McGinnis, R. F. (1982). Biogeography of Lanternfishes (Myctophidae) South of 30 S. Washington, D.C: American Geophysical Union. doi: 10.1017/pab.2021.2
McMahon, C. R., Hindell, M. A., Charrassin, J. B., Corney, S., Guinet, C., Harcourt, R., et al. (2019). Finding mesopelagic prey in a changing Southern Ocean. Sci. Rep. 9:19013. doi: 10.1038/s41598-019-55152-55154
McNeil, B. I., and Matear, R. J. (2008). Southern Ocean acidification: a tipping point at 450-ppm atmospheric CO2. Proc. Natl. Acad. Sci. U S A. 105, 18860–18864. doi: 10.1073/pnas.0806318105
Meijers, A. J. S., Cerovecki, I., King, B. A., and Tamsitt, V. (2019a). A see-saw in Pacific subantarctic mode water formation driven by atmospheric modes. Geophys. Res. Lett. 46, 13152–13160. doi: 10.1029/2019gl085280
Meijers, A. J. S., Meredith, M. P., Murphy, E. J., Chambers, D. P., Belchier, M., and Young, E. F. (2019b). The role of ocean dynamics in king penguin range estimation. Nat. Clim. Change 9, 120–121. doi: 10.1038/s41558-018-0388-382
Meredith, M., Sommerkorn, M., Cassotta, S., Derksen, C., Ekaykin, A., Hollowed, A., et al. (2019). “Polar regions,” in IPCC Special Report on the Ocean and Cryosphere in a Changing Climate, eds H.-O. Poörtner, D. C. Roberts, V. Masson-Delmotte, P. Zhai, M. Tignor, E. Poloczanska, K. (Geneva: IPCC).
Mestre, M., and Höfer, J. (2021). The microbial conveyor belt: connecting the globe through dispersion and dormancy. Trends Microbiol. 29, 482–492. doi: 10.1016/j.tim.2020.10.007
Miller, D. G. M. (1991). Exploitation of Antarctic marine living resources: a brief history and a possible approach to managing the krill fishery. South Afr. J. Mar. Sci. 10, 321–339. doi: 10.2989/02577619109504642
Moon, K. L., Chown, S. L., and Fraser, C. I. (2017). Reconsidering connectivity in the sub-Antarctic. Biol. Rev. 92, 2164–2181. doi: 10.1111/brv.12327
Moore, C. M., Mills, M. M., Arrigo, K. R., Berman-Frank, I., Bopp, L., Boyd, P. W., et al. (2013). Processes and patterns of oceanic nutrient limitation. Nat. Geosci. 6, 701–710. doi: 10.1038/ngeo1765
Moore, J. K., Fu, W. W., Primeau, F., Britten, G. L., Lindsay, K., Long, M., et al. (2018). Sustained climate warming drives declining marine biological productivity. Science 359, 1139–1142. doi: 10.1126/science.aao6379
Mori, M., Corney, S. P., Melbourne-Thomas, J., Klocker, A., Kawaguchi, S., Constable, A., et al. (2019). Modelling dispersal of juvenile krill released from the Antarctic ice edge: ecosystem implications of ocean movement. J. Mar. Syst. 189, 50–61. doi: 10.1016/j.jmarsys.2018.09.005
Morley, S. A., Abele, D., Barnes, D. K. A., Cárdenas, C. A., Cotté, C., Gutt, J., et al. (2020). Global drivers on southern ocean ecosystems: changing physical environments and anthropogenic pressures in an earth system. Front. Mar. Sci. 7:547188. doi: 10.3389/fmars.2020.547188
Morley, S. A., Barnes, D. K. A., and Dunn, M. J. (2019). Predicting which species succeed in climate-forced polar seas. Front. Mar. Sci. 5:507. doi: 10.3389/fmars.2018.00507
Morrison, A. K., Frölicher, T. L., and Sarmiento, J. L. (2015). Upwelling in the Southern Ocean. Phys. Today 68, 27–32. doi: 10.1063/pt.3.2654
Mosseri, J., Queguiner, B., Armand, L., and Cornet-Barthaux, V. (2008). Impact of iron on silicon utilization by diatoms in the Southern Ocean: a case study of Si/N cycle decoupling in a naturally iron-enriched area. Deep Sea Res. Part II 55, 801–819. doi: 10.1016/j.dsr2.2007.12.003
Mougi, A. (2018). Spatial compartmentation and food web stability. Sci. Rep. 8:16237. doi: 10.1038/s41598-018-34716-w
Mougi, A. (2019). Adaptive migration promotes food web persistence. Sci. Rep. 9:12632. doi: 10.1038/s41598-019-49143-49148
Murphy, E. J. (1995). Spatial structure of the Southern Ocean ecosystem – predator-prey linkages in Southern Ocean food webs. J. Anim. Ecol. 64, 333–347. doi: 10.2307/5895
Murphy, E. J., Cavanagh, R. D., Drinkwater, K. F., Grant, S. M., Heymans, J. J., Hofmann, E. E., et al. (2016). Understanding the structure and functioning of polar pelagic ecosystems to predict the impacts of change. Proc. Roy Soc. B 283, 1–10. doi: 10.1098/rspb.2016.1646
Murphy, E. J., Cavanagh, R. D., Hofmann, E. E., Hill, S. L., Constable, A. J., Costa, D. P., et al. (2012). Developing integrated models of Southern Ocean food webs: including ecological complexity, accounting for uncertainty and the importance of scale. Prog. Oceanogr. 102, 74–92. doi: 10.1016/j.pocean.2012.03.006
Murphy, E. J., Cavanagh, R. D., Johnston, N. M., Reid, K., and Hofmann, E. E. (2008). Integrating Climate and Ecosystem Dynamics in the Southern Ocean (ICED): A Circumpolar Ecosystem Programme. Plymouth: GLOBEC & IMBER, IGBP &SCOR.
Murphy, E. J., and Hofmann, E. E. (2012). End-to-end in Southern Ocean ecosystems. Curr. Opin. Environ. Sustainab. 4, 264–271. doi: 10.1016/j.cosust.2012.05.005
Murphy, E. J., Watkins, J. L., Trathan, P. N., Reid, K., Meredith, M. P., Thorpe, S. E., et al. (2007). Spatial and temporal operation of the Scotia Sea ecosystem: a review of large-scale links in a krill centred food web. Phil. Trans. Roy. Soc. B 362, 113–148. doi: 10.1098/rstb.2006.1957
Nathan, R., Getz, W. M., Revilla, E., Holyoak, M., Kadmon, R., Saltz, D., et al. (2008). A movement ecology paradigm for unifying organismal movement research. Proc. Natl. Acad. Sci. U S A. 105, 19052–19059. doi: 10.1073/pnas.0800375105
Netburn, A. N., and Koslow, J. A. (2018). Mesopelagic fish assemblages across oceanic fronts: a comparison of three frontal systems in the southern California current ecosystem. Deep Sea Res. Part I 134, 80–91. doi: 10.1016/j.dsr.2018.03.005
Newman, L., Heil, P., Trebilco, R., Katsumata, K., Constable, A., van Wijk, E., et al. (2019). Delivering sustained, coordinated, and integrated observations of the southern Ocean for global impact. Front. Mar. Sci. 6:433. doi: 10.3389/fmars.2019.00433
Nicol, S., and Foster, J. (2016). “The fishery for Antarctic krill: its current status and management regime,” in Biology and Ecology of Antarctic Krill, ed. V. Siegel (Berlin: Springer), 387–421. doi: 10.1007/978-3-319-29279-3_11
Niiranen, S., Richter, A., Blenckner, T., Stige, L. C., Valmarn, M., and Eikeset, A. M. (2018). Global connectivity and cross-scale interactions create uncertainty for Blue Growth of Arctic fisheries. Mar. Pol. 87, 321–330. doi: 10.1016/j.marpol.2017.10.024
Noad, M., Kniest, E., and Dunlop, R. A. (2019). Boom to bust? Implications for the continued rapid growth of the eastern Australian humpback whale population despite recovery. Popu. Ecol. 61, 198–209. doi: 10.1002/1438-390x.1014
O’Gorman, E. J. (2016). It’s only a matter of time: the altered role of subsidies in a warming world. J. Anim. Ecol. 85, 1133–1135. doi: 10.1111/1365-2656.12560
Orr, J. C., Fabry, V. J., Aumont, O., Bopp, L., Doney, S. C., Feely, R. A., et al. (2005). Anthropogenic ocean acidification over the twenty-first century and its impact on calcifying organisms. Nature 437, 681–686. doi: 10.1038/nature04095
Orr, J. C., Maier-Reimer, E., Mikolajewicz, U., Monfray, P., Sarmiento, J. L., Toggweiler, J. R., et al. (2001). Estimates of anthropogenic carbon uptake from four three-dimensional global ocean models. Glob. Biogeochem. Cycles 15, 43–60. doi: 10.1029/2000gb001273
Pakhomov, E. A., Froneman, P. W., Ansorge, I. J., and Lutjeharms, J. R. E. (2000). Temporal variability in the physico-biological environment of the Prince Edward Islands (Southern Ocean). J. Mar. Syst. 26, 75–95. doi: 10.1016/s0924-7963(00)00041-45
Pakhomov, E. A., and McQuaid, C. D. (1996). Distribution of surface zooplankton and seabirds across the Southern Ocean. Polar Biol. 16, 271–286. doi: 10.1007/s003000050054
Pakhomov, E. A., Perissinotto, R., and McQuaid, C. D. (1996). Prey composition and daily rations of myctophid fishes in the Southern Ocean. Mar. Ecol. Prog. Ser. 134, 1–14. doi: 10.3354/meps134001
Pakhomov, E. A., Verheye, H. M., Atkinson, A., Laubscher, R. K., and TauntonClark, J. (1997). Structure and grazing impact of the mesozooplankton community during late summer 1994 near South Georgia. Antarctica. Polar Biol. 18, 180–192. doi: 10.1007/s003000050175
Palter, J. B., Sarmiento, J. L., Gnanadesikan, A., Simeon, J., and Slater, R. D. (2010). Fueling export production: nutrient return pathways from the deep ocean and their dependence on the meridional overturning circulation. Biogeosciences 7, 3549–3568. doi: 10.5194/bg-7-3549-2010
Pereira, J. M., Paiva, V. H., and Xavier, J. C. (2017). Using seabirds to map the distribution of elusive pelagic cephalopod species. Mar. Ecol. Prog. Ser. 567, 257–262. doi: 10.3354/meps12020
Perissinotto, R., Lutjeharms, J. R. E., and van Ballegooyen, R. C. (2000). Biological-physical interactions and pelagic productivity at the Prince Edward Islands, Southern Ocean. J. Mar. Syst. 24, 327–341. doi: 10.1016/s0924-7963(99)00093-97
Peron, C., Delord, K., Phillips, R. A., Charbonnier, Y., Marteau, C., Louzao, M., et al. (2010). Seasonal variation in oceanographic habitat and behaviour of white-chinned petrels Procellaria aequinoctialis from Kerguelen Island. Mar. Ecol. Prog. Ser. 416, 267–284. doi: 10.3354/meps08785
Pertierra, L. R., Hughes, K. A., Vega, G. C., and Olalla-Tarraga, M. A. (2017). High resolution spatial mapping of human footprint across antarctica and its implications for the strategic conservation of avifauna. PLoS One 12:e0173649. doi: 10.1371/journal.pone.0173649
Peters, R. H. (1983). The Ecological Implications of Body Size. Cambridge: Cambridge University Press.
Petitgas, P., Rijnsdorp, A. D., Dickey-Collas, M., Engelhard, G. H., Peck, M. A., Pinnegar, J. K., et al. (2013). Impacts of climate change on the complex life cycles of fish. Fish. Oceanogr. 22, 121–139. doi: 10.1111/fog.12010
Petrik, C. M., Duffy-Anderson, J. T., Castruccio, F., Curchitser, E. N., Danielson, S. L., Hedstrom, K., et al. (2016). Modelled connectivity between walleye pollock (Gadus chalcogrammus) spawning and age-0 nursery areas in warm and cold years with implications for juvenile survival. ICES J. Mar. Sci. 73, 1890–1900. doi: 10.1093/icesjms/fsw004
Petrou, K., Baker, K. G., Nielsen, D. A., Hancock, A. M., Schulz, K. G., and Davidson, A. T. (2019). Acidification diminishes diatom silica production in the Southern Ocean. Nat. Clim. Change 9, 781–786. doi: 10.1038/s41558-019-0557-y
Phillips, R. A., Catry, P., Silk, J. R. D., Bearhop, S., McGill, R., Afanasyev, V., et al. (2007a). Movements, winter distribution and activity patterns of Falkland and brown skuas: insights from loggers and isotopes. Mar. Ecol. Prog. Ser. 345, 281–291. doi: 10.3354/meps06991
Phillips, R. A., Croxall, J. P., Silk, J. R. D., and Briggs, D. R. (2007b). Foraging ecology of albatrosses and petrels from South Georgia: two decades of insights from tracking technologies. Aquat. Cons. Mar. Freshw. Ecosyst. 17, S6–S21. doi: 10.1002/aqc.906
Phillips, R. A., Gales, R., Baker, G. B., Double, M. C., Favero, M., Quintana, F., et al. (2016). The conservation status and priorities for albatrosses and large petrels. Biol. Conserv. 201, 169–183. doi: 10.1016/j.biocon.2016.06.017
Phillips, R. A., Silk, J. R. D., Croxall, J. P., and Afanasyev, V. (2006). Year-round distribution of white-chinned petrels from South Georgia: relationships with oceanography and fisheries. Biol. Conserv. 129, 336–347. doi: 10.1016/j.biocon.2005.10.046
Phillips, R. A., Silk, J. R. D., Croxall, J. P., Afanasyev, V., and Bennett, V. J. (2005). Summer distribution and migration of nonbreeding albatrosses: individual consistencies and implications for conservation. Ecology 86, 2386–2396. doi: 10.1890/04-1885
Phillips, R. A., Silk, J. R. D., Phalan, B., Catry, P., and Croxall, J. P. (2004). Seasonal sexual segregation in two Thalassarche albatross species: competitive exclusion, reproductive role specialization or foraging niche divergence? Proc. Roy Soc. B 271, 1283–1291. doi: 10.1098/rspb.2004.2718
Pinkerton, M. H., Boyd, P. W., Deppeler, S., Hayward, A., Höfer, J., and Moreau, S. (2021). Evidence for the impact of climate change on primary producers in the Southern Ocean. Front. Ecol. Evol. 9:592027. doi: 10.3389/fevo.2021.592027
Pistorius, P., Hindell, M., Crawford, R., Makhado, A., Dyer, B., and Reisinger, R. (2017). At-sea distribution and habitat use in king penguins at sub-Antarctic Marion Island. Ecol. Evol. 7, 3894–3903. doi: 10.1002/ece3.2833
Pitman, R. L., Durban, J. W., Joyce, T., Fearnbach, H., Panigada, S., and Lauriano, G. (2020). Skin in the game: epidermal molt as a driver of long-distance migration in whales. Mar. Mamm. Sci. 36, 565–594. doi: 10.1111/mms.12661
Ponchon, A., Cornulier, T., Hedd, A., Granadeiro, J. P., and Catry, P. (2019). Effect of breeding performance on the distribution and activity budgets of a predominantly resident population of black-browed albatrosses. Ecol. Evol. 9, 8702–8713. doi: 10.1002/ece3.5416
Pondaven, P., Ragueneau, O., Treguer, P., Hauvespre, A., Dezileau, L., and Reyss, J. L. (2000). Resolving the ‘opal paradox’ in the Southern Ocean. Nature 405, 168–172. doi: 10.1038/35012046
Press, A., Hodgson-Johnston, I., and Constable, A. (2019). “The principles of the convention on the conservation of antarctic marine living resources: why its commission is not a regional fisheries management organisation,” in Governing Marine Living Resources in the Polar Regions, eds L. Nengye, B. M. Cassandra, and Q. Tianbao (Cheltenham: Edward Elgar Publishing), 9–29. doi: 10.4337/9781788977432.00010
Primeau, F. W., Holzer, M., and DeVries, T. (2013). Southern Ocean nutrient trapping and the efficiency of the biological pump. J. Geophys. Res. Oceans 118, 2547–2564. doi: 10.1002/jgrc.20181
Proud, R., Cox, M. J., and Brierley, A. S. (2017). Biogeography of the global ocean’s mesopelagic zone. Curr. Biol. 27, 113–119. doi: 10.1016/j.cub.2016.11.003
Pugh, P. (1975). The distribution of siphonophores in a transect across the North Atlantic Ocean at 32 N. J. Exp. Mar. Biol. Ecol. 20, 77–97. doi: 10.1016/0022-0981(75)90103-3
Pugh, P. R. (1999). “Siphonophores,” in South Atlantic Zooplankon, ed. D. Boltovskoy (Leiden: Backhuys Publishers), 467–512.
Queirós, J. P., Fenwick, M., Stevens, D. W., Cherel, Y., Ramos, J. A., and Xavier, J. C. (2020). Ontogenetic changes in habitat and trophic ecology of the giant Antarctic octopus Megaleledone setebos inferred from stable isotope analyses in beaks. Mar. Biol. 167:56. doi: 10.1007/s00227-020-3666-3662
Queirós, J. P., Hilário, A., Thompson, D., Ceia, F. R., Elliott, G., Walker, K., et al. (2021). From hot to cold waters: new insights in the habitat and trophic ecology of Southern Ocean squids during their life cycle. Mar. Ecol. Prog. Ser. 659, 113–126. doi: 10.3354/meps13551
Quillfeldt, P., Masello, J. F., Navarro, J., and Phillips, R. A. (2013). Year-round distribution suggests spatial segregation of two small petrel species in the South Atlantic. J. Biogeogr. 40, 430–441. doi: 10.1111/jbi.12008
Ratnarajah, L., Bowie, A. R., Lannuzel, D., Meiners, K. M., and Nicol, S. (2014). The biogeochemical role of baleen whales and krill in southern ocean nutrient cycling. PLoS One 9:e0114067. doi: 10.1371/journal.pone.0114067
Redfern, C. P. F., and Bevan, R. M. (2020). Use of sea ice by arctic terns Sterna paradisaea in Antarctica and impacts of climate change. J. Avian Biol. 51, 1–8. doi: 10.1111/jav.02318
Reid, K. (2018). “Climate change impacts, vulnerabilities and adaptations: Southern Ocean marine fisheries,” in Impacts of Climate Change on Fisheries and Aquaculture: Synthesis of Current Knowledge, Adaptation and Mitigation Options, eds M. Barange, T. Bahri, M. C. M. Beveridge, K. L. Cochrane, S. Funge-Smith, and F. Poulain (Rome: FAO Fisheries and Aquaculture).
Reid, K. (2019). “Commission for the Conservation of Antarctic Marine Living Resources (CCAMLR): implementation of conservation of Southern Ocean marine living resources,” in Governing Marine Living Resources in the Polar Regions, eds N. Liu, C. M. Brooks, and T. Qin (Cheltenham: Edward Elgar Publishing), 30–42. doi: 10.4337/9781788977432.00011
Reid, T. A., Wanless, R. M., Hilton, G. M., Phillips, R. A., and Ryan, P. G. (2013). Foraging range and habitat associations of non-breeding Tristan albatrosses: overlap with fisheries and implications for conservation. Endanger. Species Res. 22, 39–49. doi: 10.3354/esr00528
Reilly, S., Hedley, S., Borberg, J., Hewitt, R., Thiele, D., Watkins, J., et al. (2004). Biomass and energy transfer to baleen whales in the South Atlantic sector of the Southern Ocean. Deep Sea Res. Part II 51, 1397–1409. doi: 10.1016/j/dsr2.2004.06.008
Reisinger, R. R., Keith, M., Andrews, R. D., and de Bruyn, P. J. N. (2015). Movement and diving of killer whales (Orcinus orca) at a Southern Ocean archipelago. J. Exp.Mar. Biol. Ecol. 473, 90–102. doi: 10.1016/j.jembe.2015.08.008
Riekkola, L., Childerhouse, S., Zerbini, A. N., Andrews-Goff, V., Constantine, R., Cole, R., et al. (2021). “An unexpected journey: tracking southern right whales from the New Zealand sub-Antarctic wintering grounds,” in Paper SC/68c/SH02 presented to the IWC Scientific Committee, (Schaffhausen).
Riekkola, L., Zerbini, A. N., Andrews, O., Andrews-Goff, V., Baker, C. S., Chandler, D., et al. (2018). Application of a multi-disciplinary approach to reveal population structure and Southern Ocean feeding grounds of humpback whales. Ecol. Ind. 89, 455–465. doi: 10.1016/j.ecolind.2018.02.030
Rintoul, S. R. (2018). The global influence of localized dynamics in the Southern Ocean. Nature 558, 209–218. doi: 10.1038/s41586-018-0182-183
Rintoul, S. R., Chown, S. L., DeConto, R. M., England, M. H., Fricker, H. A., Masson-Delmotte, V., et al. (2018). Choosing the future of Antarctica. Nature 562:E5. doi: 10.1038/s41586-018-0369-367
Roach, C. J., and Speer, K. (2019). Exchange of water between the ross gyre and ACC assessed by lagrangian particle tracking. J. Geophys. Res. Oceans 124, 4631–4643. doi: 10.1029/2018jc014845
Robbins, J., Dalla Rosa, L., Allen, J. M., Mattila, D. K., Secchi, E. R., Friedlaender, A. S., et al. (2011). Return movement of a humpback whale between the Antarctic Peninsula and American Samoa: a seasonal migration record. Endanger. Species Res. 13, 117–121. doi: 10.3354/esr00328
Roberts, L., Kutay, C., Melbourne-Thomas, J., Petrou, K., Benson, T. M., Fiore, D., et al. (2021). Enabling enduring evidence-based policy for the southern ocean through cultural arts practices. Front. Ecol. Evol. 9:616089. doi: 10.3389/fevo.2021.616089
Robinson, J., Popova, E. E., Yool, A., Srokosz, M., Lampitt, R. S., and Blundell, J. R. (2014). How deep is deep enough? Ocean iron fertilization and carbon sequestration in the Southern Ocean. Geophys. Res. Lett. 41, 2489–2495. doi: 10.1002/2013gl058799
Rogers, A. D., Frinault, B. A. V., Barnes, D. K. A., Bindoff, N. L., Downie, R., Ducklow, H. W., et al. (2020). Antarctic futures: an assessment of climate-driven changes in ecosystem structure, function, and service provisioning in the Southern Ocean. Annu. Rev. Mar. Sci. 12, 87–120. doi: 10.1146/annurevmarine-010419-011028
Roman, J., Estes, J. A., Morissette, L., Smith, C., Costa, D., McCarthy, J., et al. (2014). Whales as marine ecosystem engineers. Front. Ecol. Env. 12:377–385. doi: 10.1890/130220
Ronconi, R. A., Schoombie, S., Westgate, A. J., Wong, S. N., Koopman, H. N., and Ryan, P. G. (2018). Effects of age, sex, colony and breeding phase on marine space use by great shearwaters Ardenna gravis in the South Atlantic. Mar. Biol. 165:58. doi: 10.1007/s00227-018-3299-x
Rosenbaum, H. C., Maxwell, S. M., Kershaw, F., and Mate, B. (2014). Long-range movement of humpback whales and their overlap with anthropogenic activity in the South Atlantic Ocean. Conserv. Biol. 28, 604–615. doi: 10.1111/cobi.12225
Sabine, C. L., Feely, R. A., Gruber, N., Key, R. M., Lee, K., Bullister, J. L., et al. (2004). The oceanic sink for anthropogenic CO2. Science 305, 367–371. doi: 10.1126/science.1097403
Sailley, S. F., Ducklow, H. W., Moeller, H. V., Fraser, W. R., Schofield, O. M., Steinberg, D. K., et al. (2013). Carbon fluxes and pelagic ecosystem dynamics near two western Antarctic Peninsula Adelie penguin colonies: an inverse model approach. Mar. Ecol. Prog. Ser. 492, 253–272. doi: 10.3354/Meps10534
Sarmiento, J. L., Gruber, N., Brzezinski, M. A., and Dunne, J. P. (2004). High-latitude controls of thermocline nutrients and low latitude biological productivity. Nature 427, 56–60. doi: 10.1038/nature02127
Saunders, R. A., Collins, M. A., Stowasser, G., and Tarling, G. A. (2017). Southern Ocean mesopelagic fish communities in the Scotia Sea are sustained by mass immigration. Mar. Ecol. Prog. Ser. 569, 173–185. doi: 10.3354/meps12093
Saunders, R. A., Hill, S. L., Tailing, G. A., and Murphy, E. J. (2019). Myctophid Fish (Family Myctophidae) are central consumers in the food web of the Scotia Sea (Southern Ocean). Front. Mar. Sci. 6:530. doi: 10.3389/fmars.2019.00530
SC-CAMLR (2005). “Report of the twenty-fourth meeting of the Scientific Committee of CCAMLR,” in Commission for the Conservation of Antarctic Marine Living Resources, (Hobart, TAS).
SC-CAMLR (2007). “Report of the twenty-sixth meeting of the Scientific Committee of CCAMLR,” in Commission for the Conservation of Antarctic Marine Living Resources, (Hobart, TAS).
Schlägel, U. E., Grimm, V., Blaum, N., Colangeli, P., Dammhahn, M., Eccard, J. A., et al. (2020). Movement-mediated community assembly and coexistence. Biol. Rev. 95, 1073–1096. doi: 10.1111/brv.12600
Schmidt, K., Schlosser, C., Atkinson, A., Fielding, S., Venables, H. J., Waluda, C. M., et al. (2016). Zooplankton gut passage mobilizes lithogenic iron for ocean productivity. Curr. Biol. 26, 2667–2673. doi: 10.1016/j.cub.2016.07.058
Scully, T. (2011). “The development of the antarctic treaty system,” in Science Diplomacy: Antarctica, Science, and the Governance of International Spaces, eds P. Berkman, M. Lang, D. Walton, and O. Young (Washington DC: Smithsonian Contributions to Knowledge), 29–38.
Shaffer, S. A., Tremblay, Y., Weimerskirch, H., Scott, D., Thompson, D. R., Sagar, P. M., et al. (2006). Migratory shearwaters integrate oceanic resources across the Pacific Ocean in an endless summer. Proc. Nati. Acad Sci. U S A. 103, 12799–12802. doi: 10.1073/pnas.0603715103
Shepard, E. L. C., Wilson, R. P., Rees, W. G., Grundy, E., Lambertucci, S. A., and Vosper, S. B. (2013). Energy landscapes shape animal movement ecology. Am. Nat. 182, 298–312. doi: 10.1086/671257
Siegel, V., and Piatkowski, U. (1990). Variability in the macrozooplankton community off the Antarctic Peninsula. Polar Biol. 10, 373–386. doi: 10.1007/BF00237825
Spear, L. B., Ainley, D. G., and Webb, S. W. (2003). Distribution, abundance and behaviour of Buller’s, Chatham Island and Salvin’s albatrosses off Chile and Peru. IBIS 145, 253–269. doi: 10.1046/j.1474-919x.2003.00151.x
Spence, P., Holmes, R. M., Hogg, A. M., Griffies, S. M., Stewart, K. D., and England, M. H. (2017). Localized rapid warming of West Antarctic subsurface waters by remote winds. Nat. Clim. Change 7, 595–603. doi: 10.1038/nclimate3335
Steffen, W., Richardson, K., Rockström, J., Schellnhuber, H. J., Dube, O. P., Dutreuil, S., et al. (2020). The emergence and evolution of earth system science. Nat. Rev. Earth Environ. 1, 54–63. doi: 10.1038/s43017-019-0005-6
Subalusky, A. L., and Post, D. M. (2019). Context dependency of animal resource subsidies. Biol. Rev. 94, 517–538. doi: 10.1111/brv.12465
Sutton, T. T., Clark, M. R., Dunn, D. C., Halpin, P. N., Rogers, A. D., Guinotte, J., et al. (2017). A global biogeographic classification of the mesopelagic zone. Deep Sea Research Part I 126, 85–102. doi: 10.1016/j.dsr.2017.05.006
Takahashi, T., Sutherland, S. C., Chipman, D. W., Goddard, J. G., Ho, C., Newberger, T., et al. (2014). Climatological distributions of pH, pCO2, total CO2, alkalinity, and CaCO3 saturation in the global surface ocean, and temporal changes at selected locations. Mar. Chem. 164, 95–125. doi: 10.1016/j.marchem.2014.06.004
Takahashi, T., Sweeney, C., Hales, B., Chipman, D. W., Newberger, T., Goddard, J. G., et al. (2012). The changing carbon cycle in the Southern Ocean. Oceanography 25, 26–37. doi: 10.5670/oceanog.2012.71
Takeda, S. (1998). Influence of iron availability on nutrient consumption ratio of diatoms in oceanic waters. Nature 393, 774–777. doi: 10.1038/31674
Talley, L. D. (2013). Closure of the global overturning circulation through the Indian, Pacific, and Southern Oceans: schematics and transports. Oceanography 26, 80–97. doi: 10.5670/oceanog.2013.07
Tamsitt, V., Cerovecki, I., Josey, S. A., Gille, S. T., and Schulz, E. (2020). Mooring observations of air-sea heat fluxes in two subantarctic mode water formation regions. J. Clim. 33, 2757–2777. doi: 10.1175/jcli-d-19-0653.1
Tamsitt, V., Drake, H. F., Morrison, A. K., Talley, L. D., Dufour, C. O., Gray, A. R., et al. (2017). Spiraling pathways of global deep waters to the surface of the Southern Ocean. Nat. Commun. 8:172. doi: 10.1038/s41467-017-00197-190
Tanhua, T., Hoppema, M., Jones, E. M., Stoven, T., Hauck, J., Davila, M. G., et al. (2017). Temporal changes in ventilation and the carbonate system in the Atlantic sector of the Southern Ocean. Deep Sea Res. Part II 138, 26–38. doi: 10.1016/j.dsr2.2016.10.004
Tarling, G. A., Ward, P., Sheader, M., Williams, J. A., and Symon, C. (1995). Distribution patterns of macrozooplankton assemblages in the southwest Atlantic. Mar. Ecol. Prog. Ser. 120, 29–40. doi: 10.3354/meps120029
Trathan, P., and Hill, S. L. (2016). “The importance of krill predation in the Southern Ocean,” in Biology and Ecology of Antarctic Krill, ed. V. Siegel (Switzerland: Springer), 321–350. doi: 10.1007/978-3-319-29279-3_9
Treasure, A. M., Ruzicka, J. J., Pakhomov, E. A., and Ansorge, I. J. (2019). Physical transport mechanisms driving sub-antarctic Island marine ecosystems. Ecosystems 22, 1069–1087. doi: 10.1007/s10021-018-0326-321
Treguer, P., Bowler, C., Moriceau, B., Dutkiewicz, S., Gehlen, M., Aumont, O., et al. (2018). Influence of diatom diversity on the ocean biological carbon pump. Nat. Geosci. 11, 27–37. doi: 10.1038/s41561-017-0028-x
Tulloch, V. J. D., Plaganyi, E. E., Brown, C., Richardson, A. J., and Matear, R. (2019). Future recovery of baleen whales is imperiled by climate change. Glob. Change Biol. 25, 1263–1281. doi: 10.1111/gcb.14573
Turner, J., Bindschadler, R. A., Convey, P., Di Prisco, G., Fahrbach, E., Gutt, J., et al. (2009). Antarctic Climate Change and the Environment. Cambridge: SCAR.
van Deurs, M., Persson, A., Lindegren, M., Jacobsen, C., Neuenfeldt, S., Jorgensen, C., et al. (2016). Marine ecosystem connectivity mediated by migrant-resident interactions and the concomitant cross-system flux of lipids. Ecol. Evo. 6, 4076–4087. doi: 10.1002/ece3.2167
Varpe, O. (2017). Life history adaptations to seasonality. Integr. Comp. Biol. 57, 943–960. doi: 10.1093/icb/icx123
Vestfals, C. D., Ciannelli, L., Duffy-Anderson, J. T., and Ladd, C. (2014). Effects of seasonal and interannual variability in along-shelf and cross-shelf transport on groundfish recruitment in the eastern Bering Sea. Deep Sea Research Part II 109, 190–203. doi: 10.1016/j.dsr2.2013.09.026
Wakefield, E. D., Phillips, R. A., Trathan, P. N., Arata, J., Gales, R., Huin, N., et al. (2011). Habitat preference, accessibility and competition limit the global distribution of breeding black-browed albatrosses. Ecol. Monogr. 81, 141–167. doi: 10.1890/09-0763.1
Walther, B. D., Munguia, P., and Fuiman, L. A. (2015). Frontiers in marine movement ecology: mechanisms and consequences of migration and dispersal in marine habitats. Biol. Lett. 11:20150146. doi: 10.1098/rsbl.2015.0146
Walton, D. W. H. (2005). Antarctica and the global jigsaw – a centennial perspective. Arch. Nat. History 32, 394–401. doi: 10.3366/anh.2005.32.2.394
Ward, P., Atkinson, A., Venables, H. J., Tarling, G. A., Whitehouse, M. J., Fielding, S., et al. (2012). Food web structure and bioregions in the Scotia Sea: a seasonal synthesis. Deep Sea Res. Part II 59, 253–266. doi: 10.1016/j.dsr2.2011.08.005
Ward, P., Whitehouse, M., Brandon, M., Shreeve, R., and Wooddwalker, R. (2003). Mesozooplankton community structure across the antarctic circumpolar current to the north of South Georgia: Southern Ocean. Mar. Biol. 143, 121–130. doi: 10.1007/s00227-003-1019-1016
Weber, T. S., and Deutsch, C. (2010). Ocean nutrient ratios governed by plankton biogeography. Nature 467, 550–554. doi: 10.1038/nature09403
Weimerskirch, H., Delord, K., Guitteaud, A., Phillips, R. A., and Pinet, P. (2015). Extreme variation in migration strategies between and within wandering albatross populations during their sabbatical year, and their fitness consequences. Sci. Rep. 5:8853. doi: 10.1038/srep08853
Weimerskirch, H., Louzao, M., de Grissac, S., and Delord, K. (2012). Changes in wind pattern alter albatross distribution and life-history traits. Science 335, 211–214. doi: 10.1126/science.1210270
Xavier, J. C., Cherel, Y., Allcock, L., Rosa, R., Sabirov, R. M., Blicher, M. E., et al. (2018). A review on the biodiversity, distribution and trophic role of cephalopods in the Arctic and Antarctic marine ecosystems under a changing ocean. Mar. Biol. 165:93. doi: 10.1007/s00227-018-3352-3359
Xavier, J. C., Raymond, B., Jones, D. C., and Griffiths, H. (2016). Biogeography of cephalopods in the southern ocean using habitat suitability prediction models. Ecosystems 19, 220–247. doi: 10.1007/s10021-015-9926-9921
Xavier, J. C., Rodhouse, P. G., Trathan, P. N., and Wood, A. G. (1999). A Geographical Information System (GIS) atlas of cephalopod distribution in the southern ocean. Ant. Sci. 11, 61–62. doi: 10.1017/s0954102099000097
Xavier, J. C., Trathan, P. N., Croxall, J. P., Wood, A. G., Podesta, G., and Rodhouse, P. G. (2004). Foraging ecology and interactions with fisheries of wandering albatrosses (Diomedea exulans) breeding at South Georgia. Fisher. Oceanogr. 13, 324–344. doi: 10.1111/j.1365-2419.2004.00298.x
Xu, Y. J., Si, Y. L., Wang, Y., Zhang, Y., Prins, H. H. T., Cao, L., et al. (2019). Loss of functional connectivity in migration networks induces population decline in migratory birds. Ecol. Appl. 29:e01960. doi: 10.1002/eap.1960
Yamazaki, K., Aoki, S., Shimada, K., Kobayashi, T., and Kitade, Y. (2020). Structure of the Subpolar Gyre in the Australian-Antarctic Basin Derived From Argo Floats. J. Geophys. Res. Oceans 125:e2019JC015406. doi: 10.1029/2019jc015406
Young, E. F., Rock, J., Meredith, M. P., Belchier, M., Murphy, E. J., and Carvalho, G. R. (2012). Physical and behavioural influences on larval fish retention: contrasting patterns in two Antarctic fishes. Mar. Ecol. Prog. Ser. 465, 201–215. doi: 10.3354/meps09908
Young, E. F., Tysklind, N., Meredith, M. P., de Bruyn, M., Belchier, M., Murphy, E. J., et al. (2018). Stepping stones to isolation: impacts of a changing climate on the connectivity of fragmented fish populations. Evol. Appl. 11, 978–994. doi: 10.1111/eva.12613
Zerbini, A. N., Adams, G., Best, J., Clapham, P. J., Jackson, J. A., and Punt, A. E. (2019). Assessing the recovery of an Antarctic krill predator from historical exploitation. Roy. Soc. Open Sci. 6:190368. doi: 10.1098/rsos.190368
Zerbini, A. N., Ajó, A. F., Andriolo, A., Clapham, P. J., Crespo, E. A., González, R., et al. (2018). “Satellite tracking of southern right whales (Eubalaena australis) from Golfo San Matías, Rio Negro Province, Argentina,” in Paper SC/67b/CMP17 presented to the IWC Scientific Committee, (Schaffhausen).
Zerbini, A. N., Andriolo, A., Heide-Jorgensen, M. P., Pizzorno, J. L., Maia, Y. G., VanBlaricom, G. R., et al. (2006). Satellite-monitored movements of humpback whales Megaptera novaeangliae in the southwest Atlantic Ocean. Mar. Ecol. Prog. Ser. 313, 295–304. doi: 10.3354/meps313295
Keywords: Southern Ocean, ecological connections, food webs, socio-economic, climate change, fisheries, global connectivity, scale
Citation: Murphy EJ, Johnston NM, Hofmann EE, Phillips RA, Jackson JA, Constable AJ, Henley SF, Melbourne-Thomas J, Trebilco R, Cavanagh RD, Tarling GA, Saunders RA, Barnes DKA, Costa DP, Corney SP, Fraser CI, Höfer J, Hughes KA, Sands CJ, Thorpe SE, Trathan PN and Xavier JC (2021) Global Connectivity of Southern Ocean Ecosystems. Front. Ecol. Evol. 9:624451. doi: 10.3389/fevo.2021.624451
Received: 31 October 2020; Accepted: 22 June 2021;
Published: 04 August 2021.
Edited by:
Anne Babcock Hollowed, National Oceanic and Atmospheric Administration (NOAA), United StatesReviewed by:
Mohammad Imam Hasan Reza, Independent Researcher, Chittagong, BangladeshEdward Urban, Scientific Committee on Oceanic Research, United States
Copyright © 2021 Murphy, Johnston, Hofmann, Phillips, Jackson, Constable, Henley, Melbourne-Thomas, Trebilco, Cavanagh, Tarling, Saunders, Barnes, Costa, Corney, Fraser, Höfer, Hughes, Sands, Thorpe, Trathan and Xavier. This is an open-access article distributed under the terms of the Creative Commons Attribution License (CC BY). The use, distribution or reproduction in other forums is permitted, provided the original author(s) and the copyright owner(s) are credited and that the original publication in this journal is cited, in accordance with accepted academic practice. No use, distribution or reproduction is permitted which does not comply with these terms.
*Correspondence: Eugene J. Murphy, ZS5tdXJwaHlAYmFzLmFjLnVr