- 1Department of Earth and Environmental Sciences, Ludwig-Maximilians-Universität, Munich, Germany
- 2SNSB - Bayerische Staatssammlung für Paläontologie und Geologie, Munich, Germany
- 3GeoBio-Center LMU, Ludwig-Maximilians-Universität, Munich, Germany
Octocorallia (class Anthozoa, phylum Cnidaria) is a group of calcifying corals displaying a wide diversity of mineral skeletons. This includes skeletal structures composed of different calcium carbonate polymorphs (aragonite and calcite). This represents a unique feature among anthozoans, as scleractinian corals (subclass Hexacorallia), main reef builders and focus of biomineralization research, are all characterized by an aragonite exoskeleton. From an evolutionary perspective, the presence of aragonitic skeletons in Octocorallia is puzzling as it is observed in very few species and has apparently originated during a Calcite sea (i.e., time interval characterized by calcite-inducing seawater conditions). Despite this, octocorals have been systematically overlooked in biomineralization studies. Here we review what is known about octocoral biomineralization, focusing on the evolutionary and biological processes that underlie calcite and aragonite formation. Although differences in research focus between octocorals and scleractinians are often mentioned, we highlight how strong variability also exists between different octocoral groups. Different main aspects of octocoral biomineralization have been in fact studied in a small set of species, including the (calcitic) gorgonian Leptogorgia virgulata and/or the precious coral Corallium rubrum. These include descriptions of calcifying cells (scleroblasts), calcium transport and chemistry of the calcification fluids. With the exception of few histological observations, no information on these features is available for aragonitic octocorals. Availability of sequencing data is also heterogeneous between groups, with no transcriptome or genome available, for instance, for the clade Calcaxonia. Although calcite represents by far the most common polymorph deposited by octocorals, we argue that studying aragonite-forming could provide insight on octocoral, and more generally anthozoan, biomineralization. First and foremost it would allow to compare calcification processes between octocoral groups, highlighting homologies and differences. Secondly, similarities (exoskeleton) between Heliopora and scleractinian skeletons, would provide further insight on which biomineralization features are driven by skeleton characteristics (shared by scleractinians and aragonitic octocorals) and those driven by taxonomy (shared by octocorals regardless of skeleton polymorph). Including the diversity of anthozoan mineralization strategies into biomineralization studies remains thus essential to comprehensively study how skeletons form and evolved within this ecologically important group of marine animals.
Introduction
Biomineralization refers to the process by which organisms produce minerals. The ability to form biomineral structures is taxonomically widespread and has evolved multiple times during the Earth’s history, including twenty independent origins within Metazoa and four within plants (Knoll, 2003). Biominerals serve a wide variety of biological functions including, among others, body support, defense against predation, navigation (Frankel et al., 1997) and (in plants) the regulation of photosynthesis and ion concentration (He et al., 2014 for review). Many groups of marine invertebrates (e.g., sponges, molluscs, and echinoderms) produce biominerals and among these, anthozoan coral (Cnidaria: Anthozoa) calcium carbonate biomineralization holds an extreme ecological significance, as it forms the 3D-framework of one of the most biodiverse ecosystems on the planet, coral reefs. Within Anthozoa, calcifying corals are found in two distinctive clades: the order Scleractinia (subclass Hexacorallia) and the subclass Octocorallia (Figure 1f). Scleractinian corals currently are the major contributors to reef accretion, and have thus been the focus of biomineralization research over the years (e.g., Tambutté et al., 2011; Falini et al., 2015). On the other hand, calcium carbonate (CaCO3) production in octocorals, commonly referred to as soft corals, is often modest and can be of several orders of magnitude lower compared to scleractinians (Smith and Kinsey, 1976; Herrán et al., 2017; Edinger et al., 2019). Despite this, some octocoral species (primarily the order Helioporacea) can significantly contribute to reef formation (Zann and Bolton, 1985) and can become the main reef builders within different habitats (Shaish et al., 2010; Yasuda et al., 2012). Octocorals can also exhibit higher resilience to environmental conditions, enabling them to outperform and replace scleractinians after events such as coral bleaching, pollution and Acanthaster outbreaks (Nishishira, 1974; Stobart et al., 2005; Ruzicka et al., 2013). Moreover, from a biomineralogical perspective, octocorals are more diverse than scleractinians, as they have evolved a wide range of different biomineralization strategies. These include the production of skeletal structures composed of different calcium carbonate polymorphs (aragonite and calcite) and of organic components (Gorgonin). On the other hand, all modern scleractinian species are characterized by the production of aragonite skeletons only.
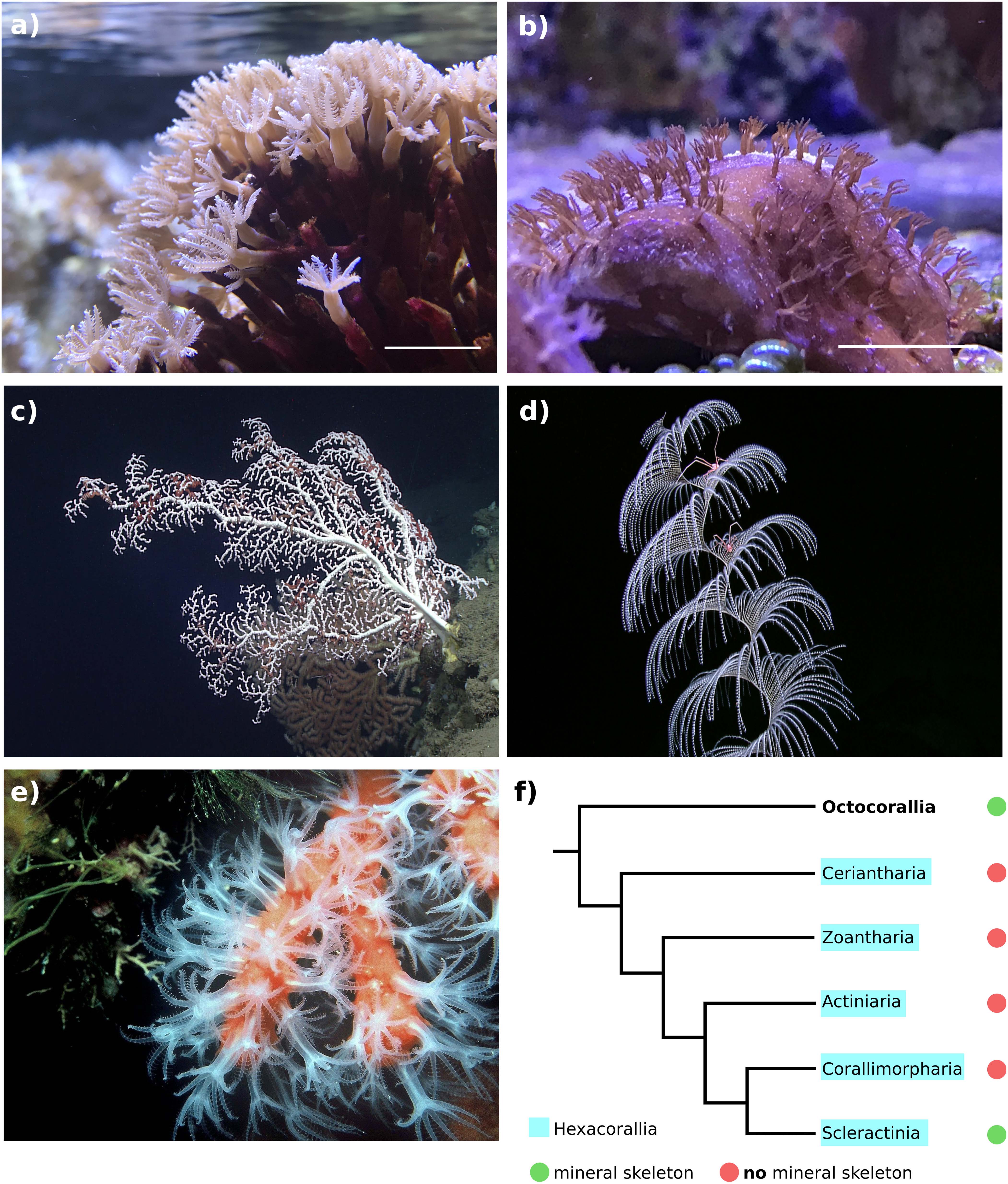
Figure 1. (a) Tubipora musica (order Stolonifera) (scale bar: 1 cm). (b) Heliopora coerulea (order Helioporacea) (scale bar: 1 cm). (c) Paragorgia sp. (order Alcyonacea). (d) Iridogorgia sp. (order Alcyonacea). (e) Corallium rubrum (order Alcyonacea). (f) Phylogenetic tree of class Anthozoa highlighting presence-absence patterns of mineral skeletons. We based the Anthozoa phylogenetic tree on Kayal et al. (2018). Here, Ceriantharia was found as sister taxon to other members of Hexacorallia. This has been recently corroborated in a phylogenomic analysis by McFadden et al. (2021). However, an alternative tree topology puts Ceriantharia as sister to remaining Anthozoa (Stampar et al., 2014). Pictures credit: (a,b) Department of Earth and Environmental Science, Ludwig-Maximilians-Universität München, (c) NOAA Office of Ocean Exploration and Research, Deep Connections 2019, and (d) NOAA Okeanos Explorer Program, Gulf of Mexico 2012 Expedition.
In light of this, octocorals represent an interesting target for biomineralization research. Yet they remain marginally studied. One of the main questions surrounding octocoral calcification is whether the morphological and compositional diversity of their skeletons is related to differences in the cellular and molecular mechanisms employed by them for biomineralization. As in other animal groups, calcification in Octocorallia is a biologically controlled process, in which the precipitation mineral is not a byproduct of metabolic processes (biologically induced mineralization), but is rather under strict physiologic control (Lowenstam, 1981; Mann, 1983). In general, biological control can be broadly subdivided into two main processes, namely, the regulation of ion (e.g., calcium) transport and concentration at the calcification sites (Kingsley and Watabe, 1985; Watabe and Kingsley, 1992; Bertucci et al., 2013), and the secretion of an organic matrix into the mineral fraction of the skeleton (Weiner et al., 1983). The composition of organic matrix in octocorals is of particular interest, as it has been shown—in other calcifying taxa—that it regulates in vitro precipitation of different CaCO3 polymorphs (Goffredo et al., 2011; Laipnik et al., 2019). Despite this, little is known about skeleton formation in octocorals, especially whether these animals have (and how they evolved) the ability to control the formation of different CaCO3 polymorphs. Finally, furthering our knowledge on octocoral calcification would also allow to obtain comparative information on the biomineralization strategies of two different groups of calcifying corals, and better understand how biomineralization evolved within the Anthozoa.
In this review, we examine the research status of calcite and aragonite biomineralization in octocorals. After introducing Octocorallia and highlighting the diversity of their skeletons, we summarize what is known about the evolutionary histories of biomineralization in octocorals, with a focus on polymorph diversity. We then surveyed available information on the cellular and molecular processes responsible for the formation of different skeletons. The aim was to (1) highlight the numerous current knowledge gaps characterizing octocoral biomineralization (especially pertaining to aragonite-forming species), and (2) propose novel potential approaches and/or research avenues to gain insight on the molecular mechanisms underlying such skeleton diversity.
Octocorals: Soft Biomineralizers
The cnidarian subclass Octocorallia represents a group of marine benthic organisms, currently comprising more than 3,400 described valid species (Williams and Cairns, 2015). They inhabit all marine habitats, but do mostly occur in either shallow tropical or deep sea environments. Around 75% of octocoral species have been described from waters below 50 m and the deepest specimen to date was recorded at 6,400 m depth (Roberts et al., 2009; Zapata Guardiola and López González, 2012). They are globally distributed, albeit very few species are cosmopolitan, with the highest diversity occurring in the Indo-Pacific region (Williams and Cairns, 2013; Pérez et al., 2016). Octocorals represent important marine community members and can increase habitats’ spatial complexity (Quattrini et al., 2014). In deep sea environments, for example, octocorals can occur at high densities and sustain biodiverse ecosystems by providing substratum and shelter to other organisms like fishes (Caley and St John, 1996), or nudibranchs, crustaceans, and echinoderms (Krieger and Wing, 2002; Mortensen and Buhl-Mortensen, 2005; De Clippele et al., 2015). As observed in scleractinians, octocoral can also establish symbiotic relationships (obligate or facultative) with photosynthetic microalgae (zooxanthellae) or be non-symbiotic (Fabricius and Alderslade, 2001). This octocoral-algae association is considered mutualistic: the symbiont obtains inorganic nutrients from the coral, while the host receives in turn organic compounds, the products of photosynthesis. Primary productivity is nonetheless usually low, and octocorals often rely also on heterotrophy (e.g., suspension feeding, prey capture) (Sorokin, 1991; Fabricius and Klumpp, 1995; Ribes et al., 1998).
With the sole exception of members of the genus Taiaroa (Bayer and Muzik, 1976), octocorals are colonial, and consist of multiple polyps embedded and interconnected by tissue (coenenchyme). Octocoral colonies can be morphologically very distinct and exhibit a wide range of forms from encrusting to arborescent (Figures 1a–e). Nevertheless, polyps are always characterized by an eightfold symmetry (i.e., they possess eight tentacles and eight internal mesenteries). This represents the synapomorphy of the group and separates Octocorallia from all remaining anthozoan taxa, in which a sixfold symmetry is present. In octocorals, polyp tentacles are also often characterized by lateral branched structures called pinnulae. This does however not constitute an octocoral synapomorphy as species with smooth tentacles have also been described (Alderslade and McFadden, 2007). Biomineralization is also not shared by all octocorals, and species lacking any mineral structure have been described in different groups (Alderslade and Mcfadden, 2011; Benayahu et al., 2017; Lau and Reimer, 2019b).
Taxonomically, Octocorallia is currently divided into three orders: Helioporacea, Pennatulacea, and Alcyonacea (Bayer, 1981b). The first two are grouped based on well-defined characters: the presence of an aragonite skeleton (Helioporacea) and the oozoid, a differentiated polyp that ensures attachment to the substratum (Pennatulacea). Order Alcyonacea is subsequently divided into six sub-ordinal groups based on their skeleton characteristics: Alcyoniina, Holaxonia, Stolonifera, Calcaxonia, Scleraxonia, and Protoalcyonacea (Grasshoff, 1999; Fabricius and Alderslade, 2001). Molecular phylogenetic studies of Octocorallia, however, have repeatedly shown that these groups are artificial and should be abandoned. For example, the analysis McFadden et al. (2006) found the suborders Stolonifera, Alcyoniina, and Scleraxonia polyphyletic. Polyphyly for Scleraxonia was also observed in Sánchez et al. (2003a), while Alcyoniina and Calcaxonia appeared paraphyletic.
Diversity and Composition of Octocoral Skeletons
Compared to modern scleractinians, which all produce massive aragonitic exoskeletons, octocorals have evolved a diverse array of skeletal structures. In the vast majority of species, the skeletal elements consist of multiple micro- or millimetric calcareous structures called sclerites, which occur embedded within the animal tissues. The overall sclerite morphology (e.g., spicule, spindle, rod, plate) (Devictor and Morton, 2010) and the different types of ornamentations can differ sensibly between species (Figure 2) and have been thus used extensively for species delimitation (Bayer, 1981a; Fabricius and Alderslade, 2001; Tentori and van Ofwegen, 2011). Molecular phylogenies have confirmed the taxonomic value of sclerite morphology to some extent, despite also highlighting instances of homoplasy (Sánchez et al., 2003b; Vargas et al., 2010c, 2014; Ament-Velásquez et al., 2016; Poliseno et al., 2017). Morphological plasticity linked to environmental conditions (Prada et al., 2008) and predation (West, 1997) has also been reported. Intraspecific variability is also present and different sclerite types and abundance can be observed within single individuals (Van Alstyne et al., 1992; Vargas et al., 2010a, b). In some species, different sclerite morphologies appear to correlate with their different localization (e.g., polyp, base) within the animal body (Williams, 1986, 1992; Alderslade, 2000; Tentori and van Ofwegen, 2011). Sclerites are found embedded within the mesoglea—a collagen-like substance between the ectodermal and endodermal layers (Barzansky and Lenhoff, 1974)—of the coenenchyme (tissue connecting the polyps) and/or the polyp (Fabricius and Alderslade, 2001).
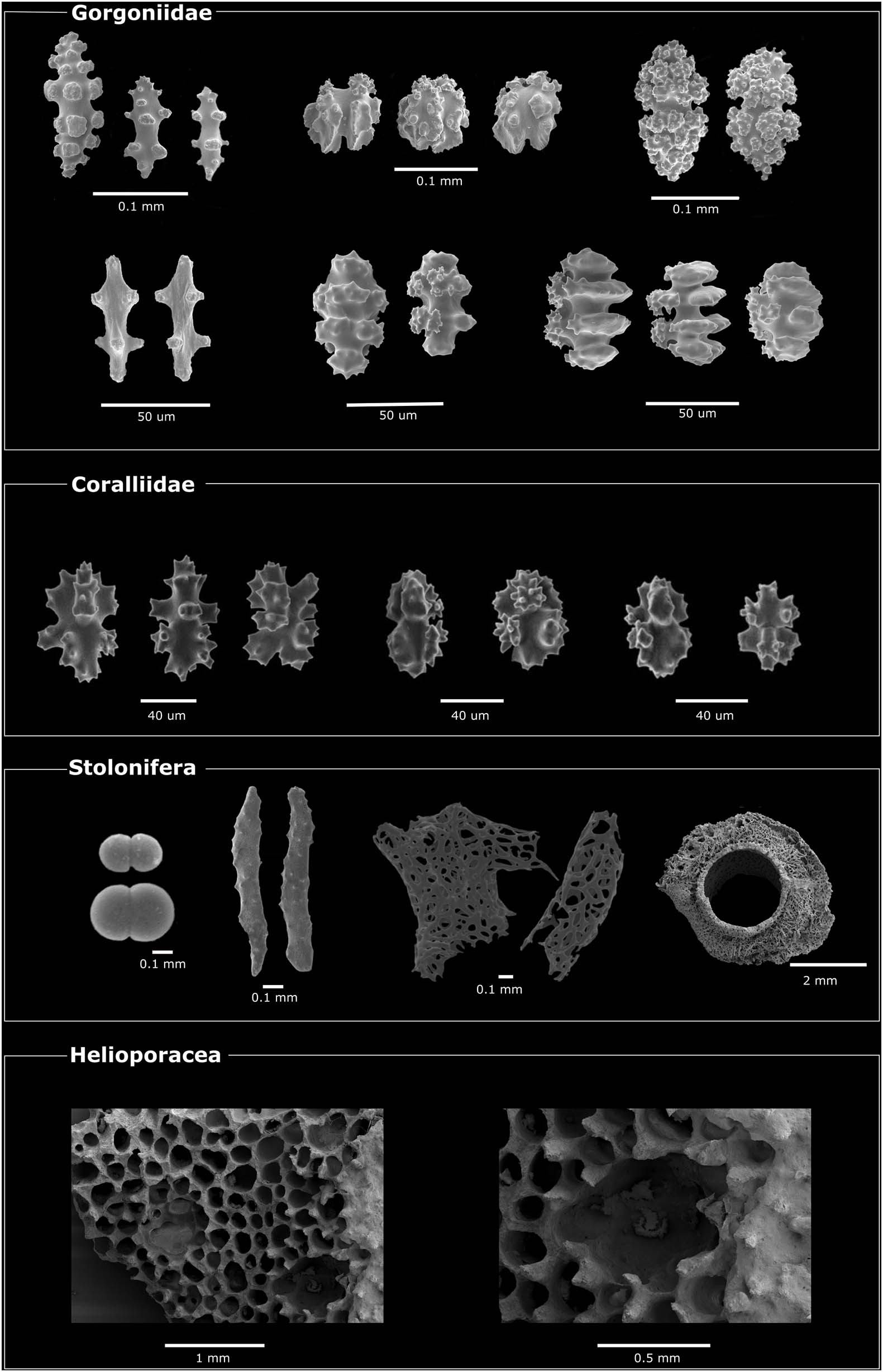
Figure 2. Overview of skeletal structures present in different octocoral clades. Images acquired by scanning electron microscopy (SEM). Images sources: Gorgoniidae from Horvath (2019), Corallidae from Simpson and Watling (2011) (Cambridge Press©, reproduced with permission of copyright holder), Stolonifera from Lau et al. (2019) and personal image, Helioporacea (personal images). Image from Conci (2020).
Mineralogy: Octocoral sclerites are all composed of CaCO3 in the form of high-magnesium calcite. More detailed compositional analyses have been obtained for the sclerites of Mediterranean gorgonians and precious corals. In the former, magnesium concentrations ranged between 1.4 and 2.2% (Weinbauer and Vellmirov, 1995). Slightly higher values (2.1–3.0%) have been found in C. rubrum (Family Corallidae), although variability in magnesium content both within single sclerites, individuals and between colonies was reported (Weinbauer et al., 2000). Strontium is another common component of octocoral sclerites and its concentration values in gogonians can range between 0.1 and 0.2% (Weinbauer and Vellmirov, 1995). Incorporated amounts of magnesium and strontium have been extensively used to reconstruct paleoclimatic conditions. For example, the concentration of magnesium in octocoral sclerites is positively correlated with seawater temperature (Weinbauer and Vellmirov, 1995; Yoshimura et al., 2011), although in C. rubrum this correlation appears not to hold when colonies are exposed to large temperature fluctuations (Vielzeuf et al., 2013). Finally, several trace elements have been detected in the sclerites of C. rubrum including lithium, iron, zinc and barium (Vielzeuf et al., 2013, 2018).
In some octocoral groups a mineral axial skeleton is deposited alongside sclerites. The axis provides support to the colony and can be entirely composed of biogenic calcite or be a combination of scleroproteins (collectively referred to as gorgonin; Bayer, 1981b) and calcite. Axial skeletons can arise from different calcification mechanisms that often entail the fusion of sclerites. In the precious coral Corallium rubrum, for example, sclerites at the tips of colony branches fuse to form the core of the axis, which is secondarily thickened by subsequent deposition of mineral layers (Allemand, 1993; Debreuil et al., 2012). In C. johnsoni however, the axial skeleton appears to be devoid of scleritic material (Lawniczak, 1987). In Octocorallia, different biomineralization strategies could have thus evolved within genera. In the family Ellisellidae, sclerites forming the axial core are often markedly different from those found in the coenenchyme (Devictor and Morton, 2010). Alternatively, sclerites can also assemble into reticulate structures (e.g., Melithaea) (Cuif, 2016). In octocorals producing proteinaceous skeletons, the axis can be reinforced with endogenous/biogenic (high magnesium calcite) or non-endogenous (calcite, aragonite, or amorphous CaCO3) minerals (Lewis et al., 1992). Amounts and origin of the axis mineral fraction can differ between groups. In Calcaxonia, large amounts of non-scleritic or scleritic calcite are present, while members of the suborder Holaxonia are characterized by small quantities of embedded calcium carbonate (Bayer and Macintyre, 2001). In bamboo corals (Family Isididae), the proteinaceous and mineral fractions of the axial skeleton are arranged to form an alternation of flexible joints and rigid mineral internodes (Noé and Dullo, 2006).
Exceptions to the “calcite rule” are members of Order Helioporacea, in which a trabecular skeleton of fibrous aragonite is present (Hill, 1960). In the genus Heliopora and Nanipora, only aragonite is observed, while in the genus Epiphaxum calcite sclerites are formed alongside the aragonitic skeleton (Lozouet and Molodtsova, 2008; Miyazaki and Reimer, 2015). However, compared to the high-magnesium calcite of sclerites, the magnesium content found in Heliopora’s aragonite skeleton is lower (ca. 0.5 mol%) (Velimirov, 1980).
Finally, hydroxyapatite (Ca10(PO4)6(OH)2) has also been detected in some gorgonians and currently represent the only possible instance of likely biologically produced calcium phosphate in cnidarians (Macintyre et al., 2000).
Evolutionary History of Octocoral Biomineralization
The origin of Octocorallia and the evolutionary relationships between different skeletal structures, notably between aragonite skeletons and calcite structures (sclerites and axis), remain largely elusive. This is in part caused by the poor preservation (Cope, 2005) and challenging identification (Deflandre-Rigaud, 1957; Kocurko and Kocurko, 1992) of octocoral skeletal elements in the fossil record, and the current lack of robust phylogenetic analyses for Octocorallia (but see Quattrini et al., 2020).
The earliest putative octocoral fossils currently date back to the Ediacaran (Glaessner, 1985). A putative Cambrian bryozoan fossil (genus Pywackia) (Landing et al., 2010) was later re-classified as an octocoral (Ausich and Babcock, 1998; Taylor et al., 2013), although this claim has been questioned (Landing et al., 2015). Earliest undisputed specimens of the group currently date back to the Ordovician, and belong to the gorgonian genus Petilavenula (Cope, 2005). Paleozoic (Silurian) octocoral fossils also include examples of a spiculite, a type of calcareous rock composed of cemented sclerites (Bengtson, 1981). Other octocoral groups appeared in the fossil record during the Cretaceous period (Figure 3). These include sea pens (order Pennatulacea) (Reich and Kutscher, 2011) and precious corals (family Coralliidae) (Schlagintweit and Gawlick, 2009). The oldest fossil specimens of aragonitic Heliopora and Epiphaxum species also date back to the Creataceous (Eguchi, 1948; Colgan, 1984; Lozouet and Molodtsova, 2008). A recent time-calibrated molecular phylogenetic analysis has corroborated the hypothesis of a Cretaceous origin for helioporaceans, while the proposed origin of pennatulaceans was pushed back to the Carboniferous (Quattrini et al., 2020).
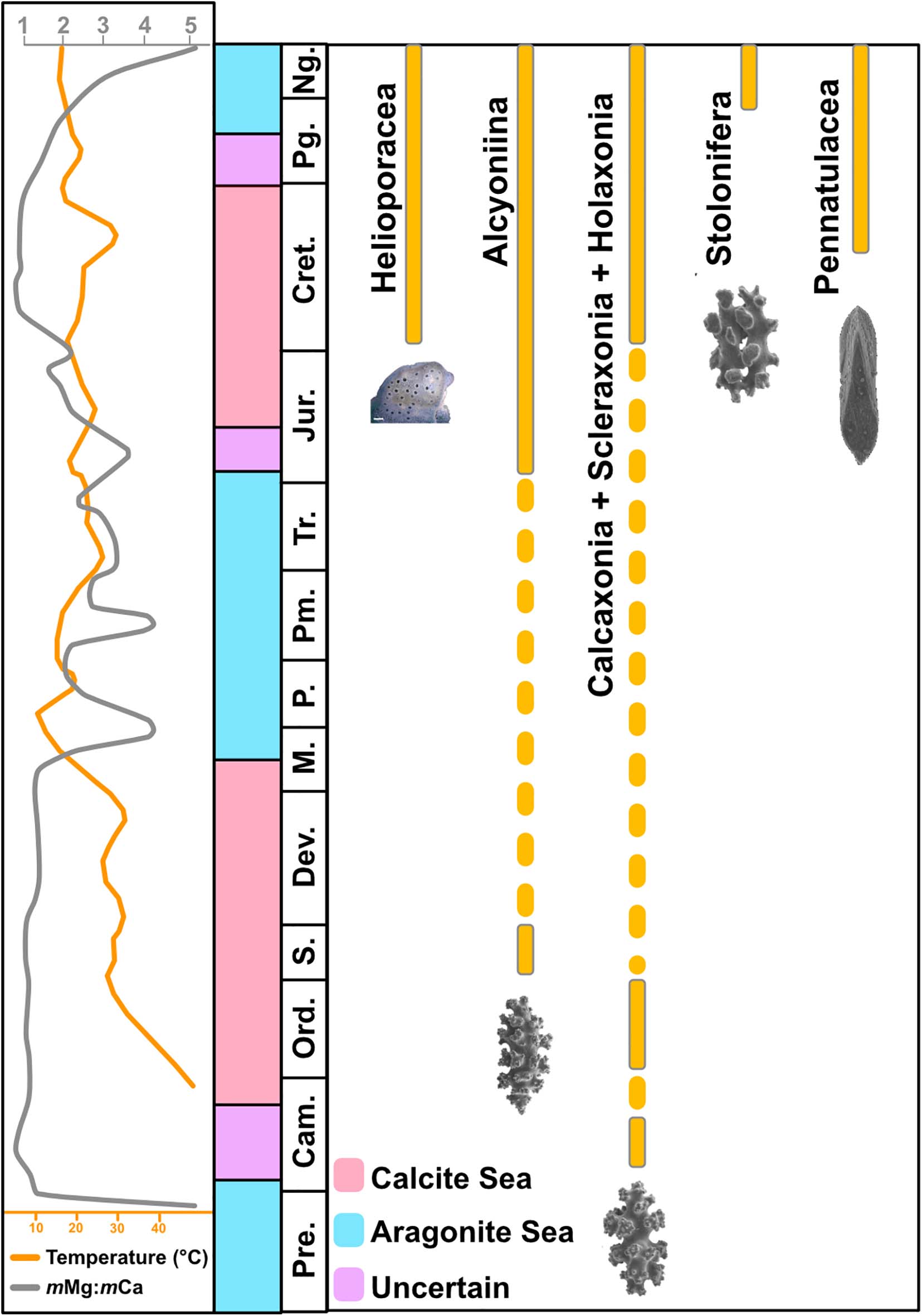
Figure 3. Current fossil record of different octocoral taxa and concurrent environmental conditions (magnesium-calcium molar ratio, mMg:mCa, and sea surface water temperature) during the last 500 Mya. Fossil record based on Reich (2009). Seawater surface temperature and mMg:mCa based on Song et al. (2019) and Hardie (1996), respectively. Skeleton images: Helioporacea (Gert Wörheide), Alcyoniina (McFadden and Ofwegen, 2017) (Magnolia Press© reproduced with permission of copyright holder), Holaxonia (Dautova, 2019), Stolonifera (Lau et al., 2018) and Pennatulacea (Williams, 2015). Pre, Precambrian; Cam, Cambrian; Ord, Ordovician; S, Silurian; Dev, Devonian; M, Mississippian; P, Pennsylvanian; Pm, Permian; Tr, Triassic; Jur, Jurassic; Cret, Cretaceous; Pg, Plaeogene; Ng, Neogene.
The emergence of aragonitic skeletons during the Cretaceous is of evolutionary interest, as it lies in apparent disagreement with the calcite-inducing conditions (i.e., low magnesium-calcium molar ratio, or mMg:mCa) experienced by marine calcifiers during that time (Hardie, 1996; Lowenstein et al., 2001). The mMg:mCa of seawater has been hypothesized to represent one of the main drivers of selective inorganic precipitation of CaCO3 polymorphs, with lower (<2) and higher (>2) values promoting the formation of calcite and aragonite, respectively (Morse et al., 1997; Balthasar and Cusack, 2015). Variations in mMg:mCa during the last 500 Mya have created an alternation between aragonite and calcite-favoring environments (referred to as the so-called “Aragonite-” and “Calcite Seas”), and have been shown to correlate with shifts in the skeleton polymorph produced by major calcificiers (Hardie, 1996; Stanley and Hardie, 1998; Knoll, 2003).
Aragonitic scleractinian corals, for example, represent the main reef framework-builders today (mMg:mCa ca. 5.2) and were responsible for reef formation during the Triassic Aragonite Sea (Stanley, 1981). They were, however, replaced by calcitic rudists (class Bivalvia, phylum Mollusca) during the Cretaceous, when Calcite Sea conditions prevailed (Scott, 1988). In addition to driving shifts in the composition of reef building communities, the seawater mMg:mCa appears to have also influenced the polymorph initially adopted by different organisms (Porter, 2010).
Aragonitic octocorals represent one of the very few exceptions to these patterns, as they appear to have evolved the ability to deposit aragonite skeletons during a Calcite Sea interval (Porter, 2010). On one hand, this could be explained by a currently incomplete fossil record. Aragonite-forming octocorals might have appeared earlier during aragonite-favoring conditions. This would, however, imply an extensive gap in the fossil record. On the other hand, precipitation of aragonite could have been promoted by other environmental variables. During the Cretaceous, several seawater properties differed compared to today’s conditions. These include higher seawater temperatures (above 32°C at low latitudes) in shallow marine habitats (Schouten et al., 2003; Bice et al., 2006), lower pH (Zeebe, 2001) and lower sulfate (SO42–) concentrations (Algeo et al., 2015). Among these, higher seawater temperatures have been shown to promote the co-precipitation of aragonite, alongside calcite, even at mMg:mCa < 1 (Balthasar and Cusack, 2015). The warm surface temperature characterizing the shallow environments inhabited by H. coerulea during the Cretaceous (Zann and Bolton, 1985), might thus have enabled this species to deposit its aragonitic skeleton. A similar scenario has also been proposed to explain the appearance of aragonitic brachiopod shells in the Silurian and Ordovician (Balthasar et al., 2011; Balthasar and Cusack, 2015).
Inferences on the evolution of octocoral biomineralization are furthermore hampered by the current lack of robust phylogenies for Octocorallia. The most comprehensive analysis, published by McFadden et al. (2006), identified two major clades (Holaxonia + Alcyoniina and Pennatulacea + Calcaxonia) plus a third clade consisting of genus Anthomastus and the precious coral Corallium ducale. The blue coral H. coeruela formed a clade with sea pens and calcaxonians. The hypothesis of a close evolutionary relationship between aragonitic octocorals (genera Heliopora and Nanipora) was later corroborated by Miyazaki and Reimer (2015) using different molecular markers. In another phylogeny, based on mitochondrial protein-coding genes, H. coerulea was, however, retrieved as sister to the rest of Octocorallia (Kayal et al., 2013). Nonetheless, the latter study aimed at resolving evolutionary relationships between Cnidaria major clades, and included a smaller taxon sampling of octocorals. In any case, no mitochondrial or nuclear marker have proved effective in resolving deep phylogenetic relationships between octocoral clades to date. This suggests that octocorals might have undergone a rapid radiation. Because phylogenomic analyses with larger taxon sampling to reconstruct the evolutionary history between octocoral groups are currently lacking, there clearly is the need for increased transcriptome and genome sequencing efforts to fill this knowledge gap (Rokas et al., 2005; McFadden et al., 2010). Nonetheless, some sequencing data is already available for some species and octocorals have been included in broader phylogenomic and phylotranscriptomic analyses to assess evolutionary relationships between cnidarian (Zapata et al., 2015; Kayal et al., 2018) or metazoan (Simion et al., 2017) groups. Several octocoral transcriptomes have been sequenced in the last few years and three genomes were released in the last 2 years (Table 1).
The majority of sequencing datasets is derived from octocorals of the suborder Holaxonia, while for other groups the number of species with a sequenced transcriptome/genome is extremely limited. To date, to the best of our knowledge, no data is available for Calcaxonia. Despite the recent increase in availability of octocoral omic resources, dozens of genera remain to be sequenced before phylogenomic (or phylotranscriptomic) analyses, with taxon samplings equivalent to previous studies (e.g., 103 species included in McFadden et al., 2006), can be conducted. In this light, research efforts aimed at resolving the origin and evolution of octocorals should (1) focus on filling the gap in terms of availability of data for calcaxonian octocorals and (2) examine existing phylogenies to prioritize the sequencing of taxa belonging to unresolved clades.
Scleroblasts: The Octocoral Calcifying Cells
Biological control over biomineralization often entails specialized cell types carrying out skeleton formation. For example, cells forming the scleractinian aboral ectoderm (or calicoblastic layer) or the outer epithelium of the molluscan mantle are, respectively, responsible for the deposition of skeletons or shells, respectively (Saleuddin, 1977; Johnston, 1980; Jolly et al., 2004). In octocorals, sclerites are produced by calcifying cells called scleroblasts. These cells likely originate in the ectodermal layer (Hickson, 1895; Woodland, 1905; Bayer and Owre, 1967; Watabe and Kingsley, 1992) and from there migrate into the mesoglea. Scleroblasts can exhibit varying morphological states, which appear to be correlated with different developmental stages of sclerite formation (Watabe and Kingsley, 1992). Based on histological and electron microscopy observations, a two step growth model for sclerite development has been proposed, involving an intracellular and a possible extracellular formation phase (see Watabe and Kingsley, 1992, for a review). Several authors provided a description of scleroblasts associated with different maturation stages in different octocorals, for example Leptogorgia virgulata (Kingsley and Watabe, 1982), Sinularia (Jeng et al., 2011), pennatulaceans (Dunkelberger and Watabe, 1974), and the precious coral C. rubrum (Grillo et al., 1993). An entirely intracellular growth model was initially proposed for the sea pen Renilla reniformis (Dunkelberger and Watabe, 1974), but later observations revealed the presence of an additional extracellular growth phase also in this octocoral species (Watabe, 1981).
Sclerite deposition appears to initially occur within a series of vacuoles present in the cytoplasm of scleroblasts (Watabe and Kingsley, 1992). Secretion of an organic matrix (discussed in the following section) into these vacuoles precedes mineral deposition and continues throughout sclerite formation (Kingsley and Watabe, 1982). The function of these organic matrices apparently is to provide a scaffold for mineral deposition (Lowenstam and Weiner, 1989). During the intracellular phase, scleroblasts are characterized by a prominent nucleus and their cytoplasm is rich in vesicles and mitochondria (Kingsley and Watabe, 1982; Grillo et al., 1993; Figure 4.1a). At this stage, scleroblasts are not solitary in the mesoglea but they instead form cell aggregates (Grillo et al., 1993). As sclerites grow, vacuoles occupy an increasingly larger space within the scleroblast, while the nucleus and cytoplasm are now restricted to the periphery of the cell (Watabe and Kingsley, 1992; Figure 4.1b). These changes are followed by the extrusion of the sclerite into the mesoglea (Figure 4.1c). A detailed description of the transition between the intracellular and extracellular phase has been provided for L. virgulata by Kinglsey and Watanabe (1982). These authors have shown that the extrusion of sclerites involves the fusion of the cell plasma membrane with the vacuole containing the sclerite. The maturation stage at which the sclerites become extracellular appears to vary between species. In L. virgulata, for example, the emergence of sclerites from the scleroblasts occurs at a late growth stage, and sclerites already exhibit their characteristic wart-like branching structures (Kingsley et al., 1987). In the gorgonian Pseudoplexaura flagellosa, on the other hand, crystals are extruded and they eventually aggregate into sclerites extracellularly (Goldberg and Benayahu, 1987). Once extracellular, sclerites can be enveloped by different scleroblasts, which are in contact by means of pseudopod-like extensions (Grillo et al., 1993). In C. rubrum for instance, two scleroblasts joined by septate junctions surround growing sclerites in the mesoglea (Le Goff et al., 2017; Figure 4.2). One or more scleroblasts associated with single sclerites have also been observed in Swiftia exserta (Menzel et al., 2015).
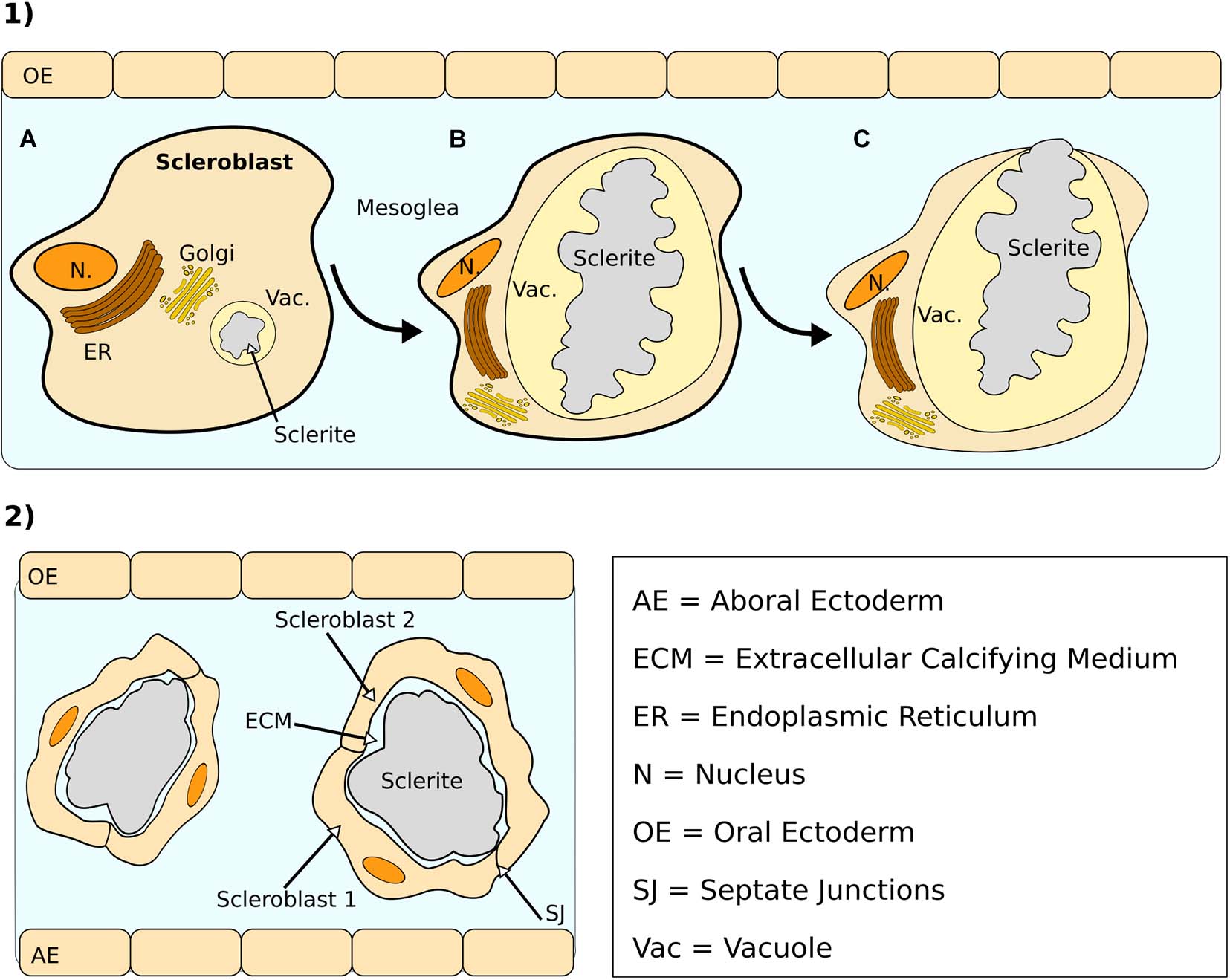
Figure 4. (1) Different sclerite growth phases in Leptogorgia virgulata: (a) early intracellular deposition in a vacuole, (b) latest stage of intracellular deposition, and (c) transition between intracellular and extracellular stage by expulsion from vacuole/cell into the mesogloea. During the latter, vacuole and cell membrane fuse together and the sclerite is extruded from the scleroblasts (based on Watabe and Kingsley, 1992). (2) Extracellular growth of a sclerite in Corallium rubrum. Two scleroblasts—connected by septate junctions (SJ) envelop a single sclerite, surrounded by an extracellular calcifying medium (based on Le Goff et al., 2017).
In Sinularia species, an additional cementation phase takes place at the base of the colony, where mature sclerites are fused together by amorphous calcium carbonate (Jeng et al., 2011). Sclerite fusion is also observed among different stoloniferous octocoral species (Lau and Reimer, 2019a).
Less is known about the cells responsible for the growth of axial skeletons. A description of the epithelial cells responsible for the axis annular growth in C. rubrum has, however, been provided by Grillo et al. (1993). These authors did not report any observable differences between the cells enveloping the axial skeleton and the scleroblasts found in the mesoglea. Based on this, they proposed that these cells constitute actual scleroblasts and that sclerite-forming cells might be the result of calcifying epithelium fragmentation.
Information about calcifying cells in aragonite-forming octocorals is extremely limited but the presence of a calcifying epithelium in H. coerulea was already reported in an early observation (Moseley, 1876). Calcifying cells in H. coerulea were originally defined as “mesodermic” (Moseley, 1876), but were later described as of ectodermal origin (Bourne and Lankester, 1895). The latter authors also reported an increase in size and granule content during the diversification of calcifying (calicoblastic) cells from epithelial cells. The calcifying epithelium of H. coerulea was later further characterized by Le Tissier (1991), who noted the presence of vesicles in the cytoplasm of calicoblastic cells found in areas of active skeleton deposition. However, whether these cells can be classified as “scleroblasts” (homologous to those characterizing calcitic species) is not currently known. In fact, although it appears that both aragonite and calcite skeletons in Octocorallia are deposited by specialized ectodermal calcifying cells, possible differences in cytological characteristics and gene expression patterns between “calcite-forming scleroblasts” and aragonite-forming cells have not been investigated.
Biological Control I: Skeleton Organic Matrix
Octocoral sclerites and skeletons are biocomposite materials formed by a mineral (CaCO3) and an organic fraction. The latter is also referred to as the skeleton organic matrix (SkOM). In scleractinian corals, SkOM quantities can range between 0.3 and 2% of the total dried skeleton weight (Constantz and Weiner, 1988; Allemand et al., 1994; Cuif et al., 2004; Goffredo et al., 2011; Ramos-Silva et al., 2013). Higher amounts have been reported in Octocorallia. In gorgonians, the SkOM represents between 1 and 5% of the skeleton weight (Silberberg et al., 1972; Kingsley and Watabe, 1983). However, a sensibly lower value (ca. 0.04%) was later obtained for the octocoral Sinularia sp. (Rahman et al., 2013). Precisely quantifying organic materials occluded within coral skeletal structures is, however, a challenging task. Following skeleton/sclerites decalcification, SkOM isolation requires a series of washing steps that can contribute to loss of low-molecular weight components from the sample, which in turn can lead to underestimations of SkOM amounts (Puverel et al., 2007; Allemand et al., 2011). Compositionally, the SkOM consists of a diverse mixture of different macromolecules. Major components of both octocoral and scleractinian matrices include proteins (Fukuda et al., 2003; Ramos-Silva et al., 2013), both mono- and polysaccharides (Goldberg, 2001; Naggi et al., 2018; Takeuchi et al., 2018) and lipids (Farre et al., 2010; Reggi et al., 2016). In the gorgonian Eugorgia ampla, lipids can represent ca. 60% of the SkOM and are followed in abundance by proteins (Fox et al., 1969). In Corallium species, for example, proteins constitute ca. 0.01% of the total skeleton weight (Debreuil et al., 2011). Among carbohydrates, glucose, galactose, galactosamine, and mannose were the most represented in the sclerites of Pseudoplexaura (Goldberg, 1988). Additional studies have also focused on the pigments responsible for the coloration of skeletal elements in gorgonians (carotenoids) (Leverette et al., 2008), H. coerulea (biliverdin IXa) (Rüdiger et al., 1968; Hongo et al., 2017) and precious corals (canthaxanthin) (Merlin and Delé-Dubois, 1986; Cvejic et al., 2007; Bracco et al., 2016).
One property of the octocoral SOM, shared by many different calcifying organisms (Puverel et al., 2005; Marin and Luquet, 2007; Mann et al., 2010), is the abundance of proteins highly enriched in acidic (isoelectric point < 4.5) amino acids, primarily aspartic acid (Watabe and Kingsley, 1992; Rahman and Oomori, 2009). One possible biological reason being the proposed interaction of carboxylic groups, found in the side chain of aspartic acid, interacting with calcium ions (Weiner and Hood, 1975). The mechanisms underlying SkOM regulation over coral calcification remain, however, elusive, and no functional information is currently available. The first octocoral skeletal protein to be characterized was extracted from the sclerites of C. rubrum, and named scleritin (Debreuil et al., 2012). This occurred almost a decade after the isolation of the first skeletal protein (galaxin) from a scleractinian skeleton (Fukuda et al., 2003). Scleritin does not possess any known protein domain and appears solely expressed by scleroblasts found in the mesoglea, but not by the cells in calcifying epithelium forming the axial skeleton (Debreuil et al., 2012). As previously mentioned, the latter have been classified as composed of scleroblasts, due to the absence of morphological differences with the mesogleal sclerite-producing cells (Grillo et al., 1993). However, the expression pattern of scleritin suggests that cells involved in these two calcification processes (i.e., sclerite formation and axial skeleton deposition) employ different proteins and are thus characterized by different gene expression profiles. Another extracellular protein (ECMP-67), with the ability to regulate CaCO3 polymorphism in vitro, was also isolated from the sclerites of Lobophytum crassum (Rahman et al., 2011).
Over the years, different coral SkOM components - including proteins, polysaccharides and lipids—have been in fact shown to promote the formation of different calcium carbonate polymorphs in vitro (Rahman and Oomori, 2009; Reggi et al., 2016; Naggi et al., 2018; Laipnik et al., 2019). However, whether these molecules possess the same properties in vivo remains to be determined. More recently, the advent of mass spectroscopy coupled with transcriptomics has enabled researchers to shift from the analysis of single proteins to the characterization of whole skeletal proteomes. This allowed to describe the “skeletogenic proteins” (Jackson et al., 2007), “skeletome” (Ramos-Silva et al., 2013) or “biomineralization toolkits” (Drake et al., 2013) in different calcifying organisms. This approach was recently applied to obtain the first proteomes found in octocoral skeletons and sclerites and provide a comparative analysis of octocorals producing aragonite (H. coerulea) and calcite (Tubipora musica and S. cf. cruciata) (Conci et al., 2020). An extremely low overlap was reported between aragonite and calcite-producing species, pointing to different protein repertoires being used by species forming different polymorphs. The only instance of a shared protein was a carbonic anhydrase homolog to CruCA-4. This protein was originally described, alongside other five carbonic anhydrases, in C. rubrum and found overexpressed in tissues enriched in calcifying cells (Le Goff et al., 2016). Carbonic anhydrases (CAs) are a superfamily of enzymes responsible for catalyzing the interconversion between carbon dioxide (CO2) and bicarbonate (HCO3–) (Supuran, 2008), and are commonly found in different animal skeletons, including scleractinian corals (Miyamoto et al., 1996; Mann et al., 2008; Ramos-Silva et al., 2013; Mann, 2015). In anthozoan corals, CAs can regulate the concentration of HCO3– in calcification spaces (see Bertucci et al., 2013 for a review). Scleritin was identified in the organic matrix of both T. musica and S. cf. cruciata, but not in the aragonitic H. coerulea (Conci et al., 2020), consistent with the hypothesis of its involvement in sclerite formation. Nonetheless, despite not being secreted into the skeleton, scleritin homologs are actively being expressed in H. coerulea (Conci et al., 2019). This could point on one hand to H. coerulea scleritin playing a different role (not requiring secretion into the skeleton) in calcification. Alternatively, the protein could hold an unrelated function and could have been recruited for biomineralization by octocorals producing calcite sclerites. In this light, comparison of the characteristics and function of calcification-related proteins in both aragonite and calcite-forming octocorals could highlight differences between groups and detect co-option events of proteins for biomineralization.
Biological Control II: Ion Transport and Regulation
For CaCO3 precipitation to occur, different physio-chemical conditions have to be reached within the calcification spaces. One critical parameter is the saturation state (Ω) of the solution with respect to a certain CaCO3 polymorph (ΩAragonite or ΩCalcite). This value is calculated as the ratio of Ca2+ and CO32– ion activity products to the stoichiometric solubility product of aragonite or calcite (Morse and Mackenzie, 1990). At Ω = 1 solid mineral and seawater are in equilibrium, while at Ω> 1 and Ω< 1 precipitation and dissolution, respectively, occur. The saturation state is also intimately linked to the pH of the solution as this can influence the availability of CO32– ions. It has been suggested that corals might be able to modulate the composition of their calcifying fluids, with respect to seawater, to promote CaCO3 precipitation and enhance calcification. In scleractinian corals for example, ΩAr of calcifying fluids can be on average around 12 (DeCarlo et al., 2017) and display diurnal cyclic variability (DeCarlo et al., 2019). This value is sensibly higher compared to seawater in reef environments (ΩAr ca. 3) (Mongin et al., 2016).
Several chemical parameters of the calcifying fluids have been studied in scleractinian corals (Cai et al., 2016; Comeau et al., 2017; Sevilgen et al., 2019), while information on octocorals is very limited. This is partly due to the presence of additional tissue layers preventing direct access to the calcification space (Le Goff et al., 2017). Information on the pH of calcifying fluids (pHcf) in both coral groups is however available. In scleractinian corals, pHcf is higher compared to the pHsw, but increases in seawater acidity appear to cause declines in the pH of calcifying fluids (Venn et al., 2013; Holcomb et al., 2014). Contrarily, early analyses in C. rubrum (based on boron isotopic analysis) did not detect the presence of significant pH upregulation between seawater and the pHcf of the axial skeleton (McCulloch et al., 2012). This was later corroborated by pH-sensitive dye-based measurements showing that sclerites and axis growth occur at a pH of 7.97 ± 0.15 and 7.89 ± 0.09, respectively (Le Goff et al., 2017). In the same study however, pHcf was found more alkaline compared to the cytoplasm of calcifying cells, which implies—as in scleractinians (Zoccola et al., 2004)—the active proton removal from calcification spaces, possibly operated by a Ca2+-ATPase (Le Goff et al., 2017). Measurements for sclerites were obtained during the extracellular phase (i.e., sclerites enveloped by two scleroblasts), while no information is currently available for pH of the calcifying medium within scleroblasts vacuoles (i.e., intracellular stage).
Analysis was then conducted at normal pHsw values. Whether C. rubrum is able to maintain pH homeostasis under seawater acidification conditions has not been determined. Differences in pH regulation between scleractinian skeletons and octocoral sclerites could be related to the different localization or composition (aragonite vs. calcite) of the skeletal structures. For example, the cells and/or mesoglea surrounding octocoral sclerites could buffer the effects of seawater chemistry. Surrounding tissues have, for instance, been proposed to act as a protective barrier against the effects of ocean acidification (OA) in octocorals (discussed in the next section; Gabay et al., 2014). However, the multi-step (intracellular and extracellular) growth model of octocoral sclerites previously described, opens to the possibility that sclerites could experience different environments—in terms of calcifying fluids chemistry and their interaction with seawater—during their formation.
Ocean acidification also appears to impact CaCO3 polymorphs differently, with calcite being apparently less affected (although depending on magnesium content) (Ries et al., 2009). A possible approach to further elucidate pHcf regulation mechanisms in octocorals could be the characterization of calcifying fluids pH in H. coerulea, for which no information is currently available. Such analysis could determine, on one hand, whether the absence of pHcf regulation is indeed a common trait among Octocorallia, independently of the characteristics of the mineral structures (calcite sclerites/axis vs. aragonite skeleton). Alternatively, aragonitic octocorals could exhibit pHcf increases as observed in scleractinian, pointing to pH upregulation representing a required feature for aragonite deposition in both coral groups.
Within the calcification space, calcium concentration in scleractinians is not necessarily higher compared to seawater, but some species can modulate calcium levels in response to decreases in seawater pH (DeCarlo et al., 2018). For octocorals, to the best of our knowledge, no information has been obtained. Calcium transport to calcification sites has been studied in Leptogorgia virgulata and a general model of calcium transfer has nonetheless been provided by Watabe and Kingsley (1992). Transport of Ca2+ during the intracellular growth of sclerites in octocorals represents a multi-step process (Figure 5). To reach the calcification space, calcium first diffuses from seawater into the outer epithelial cells and is actively extruded into the mesoglea. Studies involving specific transporters inhibitors have highlighted the action of Ca2+-ATPases during this step, while Na+-K+ exchangers don’t appear to be involved (Kingsley and Watabe, 1984). Once in the mesoglea, the majority of calcium ions reach the axis epithelium and are incorporated into the axial skeleton, while others diffuse into the scleroblasts. Inside calcifying cells, calcium is transported inside electron-dense bodies (Kingsley and Watabe, 1985) to the calcifying vacuoles where sclerites form (Kingsley and Watabe, 1982). Both the membrane of scleroblasts and calcium-transporting vesicles exhibit signs of Ca2+-ATPases activity (Kingsley and Watabe, 1984).
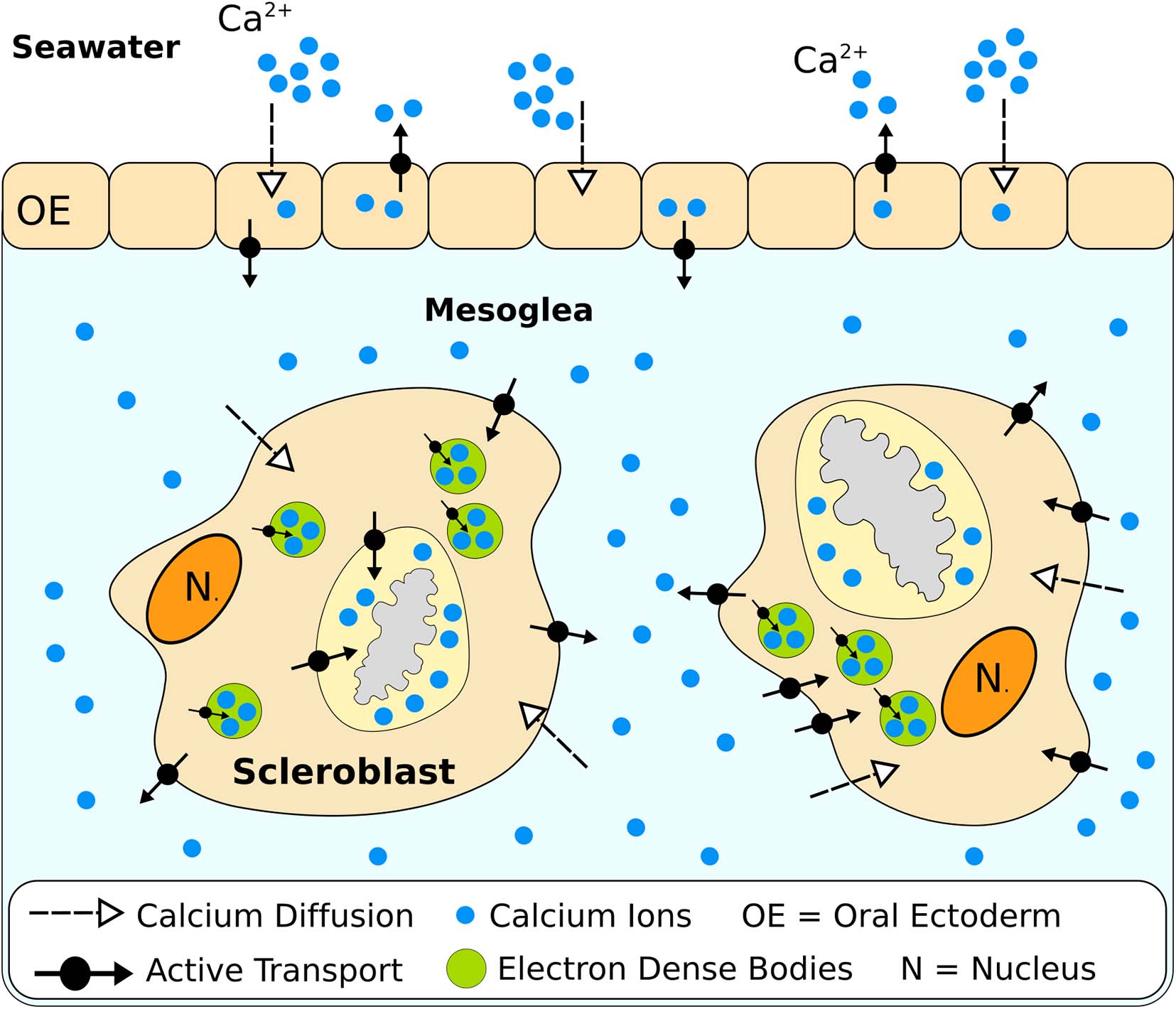
Figure 5. Proposed model of calcium transport from seawater to growing sclerites in Leptogorgia virgulata (based on Watabe and Kingsley, 1992).
The origin and transport of carbon (CO2 and HCO3–) has also been investigated in L. virgulata (Lucas and Knapp, 1997). In this species, carbonic anhydrase represented the major carbon source followed by bicarbonate. Inhibition of carbonic anhydrases caused significant declines in incorporation rates of both dissolved and metabolically produced CO2, pointing to an important role of these enzymes in the regulation of carbon availability. Carbonic anhydrases, for example, have been proposed to operate on the membrane of calcifying vacuoles and electron-dense bodies (Kingsley and Watabe, 1987; Lucas and Knapp, 1996) and the aforementioned presence of CruCA-4 homologs inside octocoral skeletons confirms the presence of these enzymes within the calcifying medium (Conci et al., 2020).
Anthropogenic Threats to Octocoral Biomineralization
The effects of anthropic stressors on the ability of marine organisms to calcify have been the topic of a number of studies. In corals, for example, changes in calcification rates and growth have been studied in relation to different stressors, such as seawater temperature (Howe and Marshall, 2002; Marshall and Clode, 2004), pollution (Spencer Davies, 1990; Biscéré et al., 2015) and turbidity (Kendall et al., 1985; Browne, 2012).
However, particular focus has been dedicated to study the effects of the so-called “ocean acidification” (OA), i.e., the decrease in sea surface water pH caused by the increase in the atmospheric carbon dioxide (CO2) concentration (Hoegh-Guldberg et al., 2007; Solomon et al., 2007). Ocean acidification linked detrimental effects on biomineralization have been reported for multiple marine groups including calcifying algae (Kuffner et al., 2008), scleractinian corals (Marubini et al., 2003; Hoegh-Guldberg et al., 2007; Mollica et al., 2018) and molluscs (Comeau et al., 2009; Gazeau et al., 2013). Research has however also highlighted several instances of resilience to ocean acidification, highlighting species-specific responses to low pH (Cross et al., 2015a, b; González-Pech et al., 2017; Lenz and Edmunds, 2017).
Octocorals appear to exhibit varying responses to ocean acidification. An inverse correlation between calcification rates and pCO2 have been reported for Eunicea flexuosa when exposed to a pH range of 8.1 and 7.1 (Gómez et al., 2015). Contrarily, in another study no changes in branch extension and sclerite structure could be observed in the same species at pH 7.75 compared to control values (pH 8.1) (Enochs et al., 2016). Lower pH (7.6 and 7.3) had no effects on polyp weight and protein content in different octocorals nor in chlorophyll abundance ordensity of its symbiotic algae (Gabay et al., 2013). One possible explanation behind octocoral resilience could be the location inside the mesoglea of their sclerites with the surrounding tissues acting as a protective barrier (Gabay et al., 2014). A similar scenario has also been proposed for the organic layers protecting mollusc shells against dissolution (Rodolfo-Metalpa et al., 2011). From an ecological perspective, different tolerance to changing environmental conditions is of importance as it can determine which species will thrive or decline under future conditions, and cause profound changes in marine communities composition (Fabricius et al., 2011). And shifts from stony (more vulnerable) to soft coral (more resistant)-dominated environments have already been observed (Inoue et al., 2013).
Discussion
Octocorals (Cnidaria: Anthozoa) represent a group of benthic marine organisms that have evolved a wide variety of biomineralization strategies. The composite biomaterials produced by these organisms include structures composed of different calcium carbonate (CaCO3) polymorphs (aragonite and calcite). From an evolutionary perspective, the presence of aragonite skeletons in Octocorallia is puzzling as it is observed in an extremely low number of species in a single clade, the Helioporacea. Apparently, this occurrence represents one of the very few instances of aragonite formation originating during a Calcite Sea interval (Porter, 2010). Despite the diversity of their skeletal elements, octocorals have only been marginally studied for biomineralization research. This has been partially caused by their low contribution to CaCO3 production and reef formation (Smith and Kinsey, 1976; Herrán et al., 2017; Edinger et al., 2019) compared to scleractinian corals, the other group of calcifying anthozoans and main reef builders in today’s oceans. Additionally, octocoral species often inhabit deeper marine habitats compared to scleractinians (Roberts et al., 2009), which can hinder field observation and the collection of specimens for follow-up studies. With the aim to identify current knowledge gaps and propose possible future research approaches, here we reviewed the research status of octocoral biomineralization. We focused and compared what is known about the evolutionary, cellular and molecular processes underlying different octocoral skeletons, with a focus on CaCO3 polymorph (i.e., aragonite and calcite) diversity.
Although differences in research output between scleractinian and octocoral calcification are often mentioned, we highlighted how differences—both in terms of data availability and scientific knowledge—exist with respect to the process of biomineralization between different octocoral groups. Several mechanisms and features of octocoral biomineralization, such as the characteristics of the calcifying cells (e.g., scleroblasts) or Ca2+ transport, have mostly been investigated in species depositing calcite. Although calcite represents by far the most commonly deposited polymorph in octocorals, studying aragonite formation provides the opportunity to further our understanding on multiple aspects of the origin and evolution of octocoral and scleractinian biomineralization. Firstly, differences between aragonite and calcite formation are not necessarily limited to skeleton polymorphism. In fact, calcite and aragonite-forming species also differ in terms of other calcification-related properties, such as the composition of the organic matrix proteome (Conci et al., 2020) and possibly the type and characteristics of calcifying cells and epithelia. With the exception of early descriptions from the late nineteenth century (e.g., Bourne and Lankester (1895), no study has in fact focused on the calcifying cells of aragonitic octocorals. In addition, the different localization of calcitic and aragonitic structures (i.e., surrounded by tissue or not) could also entail the different regulation of the chemistry and pH of the calcification fluids, as observed between scleractinians (Venn et al., 2013; Holcomb et al., 2014) and Corallium rubrum (Le Goff et al., 2017). In fact, the scleractinian-like characteristics of helioporacean skeletons (i.e., aragonite exoskeleton), provide the unique opportunity to compare aragonite formation between Octocorallia and Scleractinia, and in turn determine which biomineralization features are driven by phylogeny (i.e., properties shared by octocorals producing different polymorphs) and which are instead related to the characteristics of the skeleton (i.e., properties shared by aragonitic octocorals and scleractinians). So far, this research avenue has not been fully explored, but at present the data at hand suggest that the aragonitic Heliopora coerulea employs a different biomineralization strategy compared to both calcitic octocorals and scleractinians. The only aspect for which information is currently available for all three groups concerns the composition of the skeleton proteomes. Their analyses have shown that very few proteins found in the skeleton of Heliopora coerulea are also found in other calcifying corals, irrespective of whether those are of calcite or aragonite (Ramos-Silva et al., 2013; Conci et al., 2020).
Difference in research focus and information availability are not limited to calcite vs. aragonite species, but can also be observed within the former. The majority of information for calcite-forming octocorals has been obtained from a small set of species, including the gorgonian Leptogorgia virgulata and the precious coral Corallium rubrum. This includes (1) the current knowledge on ion sources and transport needed for calcification, (2) detailed description of scleroblasts, and (3) both mechanisms of intracellular and extracellular formation of sclerites. Although extremely informative, a research approach focusing on very few “model species” may lead to an underestimation of the diversity of biomineralization strategies exhibited by different octocoral groups. The possible presence of substantial differences in sclerites and/or skeleton formation that can also occur even within a single genus (e.g., Corallium) (Lawniczak, 1987), requires extensive comparative analyses instead.
In the light of this, a possible step could be to taxonomically widen the generation of—omic resources, optimized to encompass a broad diversity of octocoral skeletal structures. The simultaneous presence, within Octocorallia, of calcite, aragonite and aragonite + calcite skeletons represents an ideal scenario to compare gene repertories across species producing different polymorphs, and compare those with scleractinian corals. Moreover, in addition to the aforementioned need for DNA sequencing data from the Calcaxonia, sequencing projects for skeleton-lacking octocorals (e.g., genus Phenganax) (Alderslade and Mcfadden, 2011) would also be beneficial, as they would allow to detect differences in the molecular mechanisms of biomineralization between calcifying and non-calcifying octocorals. The increase in the availability of octocoral genomes and transcriptomes would then in turn allow to progressively conduct more taxonomically comprehensive phylogenetic analysis, and resolve deep evolutionary relationships between clades, and ultimately how biomineralization in Octocorallia evolved.
Author Contributions
NC wrote the manuscript. SV and GW edited the manuscript. All authors contributed to the discussion.
Conflict of Interest
The authors declare that the research was conducted in the absence of any commercial or financial relationships that could be construed as a potential conflict of interest.
References
Alderslade, P. (2000). Four new genera of soft corals (Coelenterata: octocorallia), with notes on the classification of some established taxa. Zool. Meded. 74, 237–249.
Alderslade, P., and McFadden, C. S. (2007). Pinnule-less polyps: a new genus and new species of Indo-Pacific Clavulariidae and validation of the soft coral genus Acrossota and the family Acrossotidae (Coelenterata: octocorallia). Zootaxa 1400, 27–44. doi: 10.11646/zootaxa.1400.2
Alderslade, P., and Mcfadden, C. S. (2011). A new sclerite-free genus and species of Clavulariidae (Coelenterata: octocorallia). Zootaxa 3104, 64–68. doi: 10.11646/zootaxa.3104.1.6
Algeo, T. J., Luo, G. M., Song, H. Y., Lyons, T. W., and Canfield, D. E. (2015). Reconstruction of secular variation in seawater sulfate concentrations. Biogeosciences 12, 2131–2151. doi: 10.5194/bg-12-2131-2015
Allemand, D. (1993). The biology and skeletogenesis of the Mediterranean Red Coral: a review. Precious Corals Octocorals Res. 2, 19–39.
Allemand, D., Cuif, J. P., Watabe, N., Oishi, M., and Kawaguchi, T. (1994). The organic matrix of skeletal structures of the Mediterranean red coral, Corallium rubrum. Bull. Inst. Océanogr. 14, 129–139.
Allemand, D., Tambutté, É, Zoccola, D., and Tambutté, S. (2011). “Coral calcification, cells to reefs,” in Coral Reefs: An Ecosystem in Transition, eds Z. Dubinksy and N. Stambler (Dordrecht: Springer), 119–150. doi: 10.1007/978-94-007-0114-4_9
Ament-Velásquez, S. L., Breedy, O., Cortés, J., Guzman, H. M., Wörheide, G., and Vargas, S. (2016). Homoplasious colony morphology and mito-nuclear phylogenetic discordance among Eastern Pacific octocorals. Mol. Phylogenet. Evol. 98, 373–381. doi: 10.1016/j.ympev.2016.02.023
Ausich, W. I., and Babcock, L. E. (1998). The phylogenetic position of Echmatocrinus brachiatus, a probable octocoral from the Burgess Shale. Palaeontology 41, 193–202.
Balthasar, U., and Cusack, M. (2015). Aragonite-calcite seas—Quantifying the gray area. Geology 43, 99–102. doi: 10.1130/g36293.1
Balthasar, U., Cusack, M., Faryma, L., Chung, P., Holmer, L. E., Jin, J., et al. (2011). Relic aragonite from ordovician–silurian brachiopods: implications for the evolution of calcification. Geology 39, 967–970. doi: 10.1130/g32269.1
Barzansky, B., and Lenhoff, H. M. (1974). On the chemical composition and developmental role of the mesoglea of Hydra. Integr. Comp. Biol. 14, 575–581. doi: 10.1093/icb/14.2.575
Bayer, F. M. (1981a). Bibliography of Octocorallia 1469-1977. Seminarios de Biologia Marinha. Rio de Janeiro: Academia Brasileira de Ciências, 29–102.
Bayer, F. M. (1981b). Key to the genera of Octocorallia exclusive of Pennatulacea (Coelenterata: anthozoa), with diagnosis of new taxa. Proc. Biol. Soc. Washington 94, 902–947.
Bayer, F. M., and Macintyre, I. G. (2001). The mineral component of the axis and holdfast of some gorgonacean octocorals (Coelenteratea: anthozoa), with specieal reference to the family Gorgoniidae. Proc. Biol. Soc. Washington 114, 309–345.
Bayer, F. M., and Muzik, K. M. (1976). A new solitary octocoral, Taiaroa tauhou gen. et sp. nov. (Coelenterata: protoalcyonaria) from New Zealand. J. R. Soc. N. Z. 6, 499–515. doi: 10.1080/03036758.1976.10421488
Bayer, F. M., and Owre, H. B. (1967). The Free Living Lower Invertebrates. New York, NY: The Mcmillan CO.
Benayahu, Y., McFadden, C. S., and Shoham, E. (2017). Search for mesophotic octocorals (Cnidaria, Anthozoa) and their phylogeny: I. A new sclerite-free genus from Eilat, northern Red Sea. ZooKeys 680, 1–11. doi: 10.3897/zookeys.680.12727
Bertucci, A., Moya, A., Tambutté, S., Allemand, D., Supuran, C. T., and Zoccola, D. (2013). Carbonic anhydrases in anthozoan corals—A review. Bioorg. Med. Chem. 21, 1437–1450. doi: 10.1016/j.bmc.2012.10.024
Bice, K. L., Birgel, D., Meyers, P. A., Dahl, K. A., Hinrichs, K.-U., and Norris, R. D. (2006). A multiple proxy and model study of Cretaceous upper ocean temperatures and atmospheric CO2 concentrations : cretaceous multiple proxy study. Paleoceanography 21:A2002.
Biscéré, T., Rodolfo-Metalpa, R., Lorrain, A., Chauvaud, L., Thébault, J., Clavier, J., et al. (2015). Responses of two scleractinian corals to cobalt pollution and ocean acidification. PLoS One 10:e0122898. doi: 10.1371/journal.pone.0122898
Bourne, G. C., and Lankester, E. R. (1895). IX. On the structure and affinities of <Heliopora coerulea, Pallas. With some observations on the structure of Xenia and Heteroxenia. Philos. Trans. R. Soc. Lond. (B) 186, 455–483. doi: 10.1098/rstb.1895.0009
Bracco, S., Fumagalli, P., Fusi, P., Santambrogio, C., Rolandi, V., and Brajkovic, A. (2016). Identification of the chromophores in Corallium rubrum gem quality corals by HPLC/UV, ESI-MS and 1H NMR spectroscopy. Period. Mineral. 85, 83–93.
Browne, N. K. (2012). Spatial and temporal variations in coral growth on an inshore turbid reef subjected to multiple disturbances. Mar. Environ. Res. 77, 71–83. doi: 10.1016/j.marenvres.2012.02.005
Cai, W.-J., Ma, Y., Hopkinson, B. M., Grottoli, A. G., Warner, M. E., Ding, Q., et al. (2016). Microelectrode characterization of coral daytime interior pH and carbonate chemistry. Nat. Commun. 7:11144.
Caley, M. J., and St John, J. (1996). Refuge availability structures assemblages of tropical reef fishes. J. Anim. Ecol. 65, 414–428. doi: 10.2307/5777
Colgan, M. W. (1984). “The cretaceous coral Heliopora (Octocorallia, Coenothecalia)—a common indo-pacific reef builder,” in Living Fossils, eds N. Eldredge and S. M. Stanley (New York, NY: Springer New York), 266–271. doi: 10.1007/978-1-4613-8271-3_33
Comeau, S., Gorsky, G., Jeffree, R., Teyssié, J.-L., and Gattuso, J.-P. (2009). Impact of ocean acidification on a key Arctic pelagic mollusc (Limacina helicina). Biogeosciences 6, 1877–1882. doi: 10.5194/bg-6-1877-2009
Comeau, S., Tambutté, E., Carpenter, R. C., Edmunds, P. J., Evensen, N. R., Allemand, D., et al. (2017). Coral calcifying fluid pH is modulated by seawater carbonate chemistry not solely seawater pH. Proc. Biol. Sci. 284:20161669. doi: 10.1098/rspb.2016.1669
Conci, N. (2020). Molecular Biomineralization of Octocoral Skeletons: Calcite Versus Aragonite. Dissertation, LMU München, Munich.
Conci, N., Lehmann, M., Vargas, S., and Wörheide, G. (2020). Comparative proteomics of octocoral and scleractinian skeletomes and the evolution of coral calcification. Genome Biol. Evol. 12, 1623–1635. doi: 10.1093/gbe/evaa162
Conci, N., Wörheide, G., and Vargas, S. (2019). New non-bilaterian transcriptomes provide novel insights into the evolution of coral skeletomes. Genome Biol. Evol. 11, 3068–3081. doi: 10.1093/gbe/evz199
Constantz, B., and Weiner, S. (1988). Acidic macromolecules associated with the mineral phase of scleractinian coral skeletons. J. Exp. Zool. 248, 253–258. doi: 10.1002/jez.1402480302
Cope, J. C. W. (2005). OCTOCORALLIAN AND HYDROID FOSSILS FROM THE LOWER ORDOVICIAN OF WALES. Palaeontology 48, 433–445. doi: 10.1111/j.1475-4983.2005.00455.x
Cross, E. L., Peck, L. S., and Harper, E. M. (2015a). Ocean acidification does not impact shell growth or repair of the Antarctic brachiopod Liothyrella uva (Broderip, 1833). J. Exp. Mar. Bio. Ecol. 462, 29–35. doi: 10.1016/j.jembe.2014.10.013
Cross, E. L., Peck, L. S., Lamare, M. D., and Harper, E. M. (2015b). No ocean acidification effects on shell growth and repair in the New Zealand brachiopod Calloria inconspicua (Sowerby, 1846). ICES J. Mar. Sci. 73, 920–926. doi: 10.1093/icesjms/fsv031
Cuif, J.-P. (2016). “Calcification in the cnidaria through time: an overview of their skeletal patterns from individual to evolutionary viewpoints,” in The Cnidaria, Past, Present and Future: The world of Medusa and Her Sisters, eds S. Goffredo and Z. Dubinsky (Cham: Springer International Publishing), 163–179. doi: 10.1007/978-3-319-31305-4_11
Cuif, J.-P., Dauphin, Y., Berthet, P., and Jegoudez, J. (2004). Associated water and organic compounds in coral skeletons: quantitative thermogravimetry coupled to infrared absorption spectrometry. Geochem. Geophys. Geosyst. 5:Q11011.
Cvejic, J., Tambutté, S., Lotto, S., Mikov, M., Slacanin, I., and Allemand, D. (2007). Determination of canthaxanthin in the red coral (Corallium rubrum) from Marseille by HPLC combined with UV and MS detection. Mar. Biol. 152, 855–862. doi: 10.1007/s00227-007-0738-5
Dautova, T. N. (2019). New species of deep-water Calcigorgia gorgonians (Anthozoa: octocorallia) from the Sea of Okhotsk, with a re-diagnosis and a taxonomic review of the genus. Eur. J. Taxon. 549, 1–27. doi: 10.5852/ejt.2019.549
De Clippele, L. H., Buhl-Mortensen, P., and Buhl-Mortensen, L. (2015). Fauna associated with cold water gorgonians and sea pens. Cont. Shelf Res. 105, 67–78. doi: 10.1016/j.csr.2015.06.007
Debreuil, J., Tambutté, E., Zoccola, D., Deleury, E., Guigonis, J.-M., Samson, M., et al. (2012). Molecular cloning and characterization of first organic matrix protein from sclerites of red coral, Corallium rubrum. J. Biol. Chem. 287, 19367–19376. doi: 10.1074/jbc.m112.352005
Debreuil, J., Tambutté, S., Zoccola, D., Segonds, N., Techer, N., Marschal, C., et al. (2011). Specific organic matrix characteristics in skeletons of Corallium species. Mar. Biol. 158, 2765–2774. doi: 10.1007/s00227-011-1775-7
DeCarlo, T. M., Comeau, S., Cornwall, C. E., and McCulloch, M. T. (2018). Coral resistance to ocean acidification linked to increased calcium at the site of calcification. Proc. Biol. Sci. 285:20180564. doi: 10.1098/rspb.2018.0564
DeCarlo, T. M., D’Olivo, J. P., Foster, T., Holcomb, M., Becker, T., and McCulloch, M. T. (2017). Coral calcifying fluid aragonite saturation states derived from Raman spectroscopy. Biogeosciences 14, 5253–5269. doi: 10.5194/bg-14-5253-2017
DeCarlo, T. M., Ross, C. L., and McCulloch, M. T. (2019). Diurnal cycles of coral calcifying fluid aragonite saturation state. Mar. Biol. 166:28.
Deflandre-Rigaud, M. (1957). A classification of fossil alcyonarian sclerites. Micropaleontology 3, 357–366. doi: 10.2307/1484441
Devictor, S. T., and Morton, S. L. (2010). Identification guide to the shallow water (0–200 m) octocorals of the South Atlantic Bight. Zootaxa 2599, 1–62. doi: 10.11646/zootaxa.2599.1.1
Drake, J. L., Mass, T., Haramaty, L., Zelzion, E., Bhattacharya, D., and Falkowski, P. G. (2013). Proteomic analysis of skeletal organic matrix from the stony coral Stylophora pistillata. Proc. Natl. Acad. Sci. U.S.A. 110, 3788–3793. doi: 10.1073/pnas.1301419110
Dunkelberger, D. G., and Watabe, N. (1974). An ultrastructural study on spicule formation in the pennatulid colony Renilla reniformis. Tissue Cell 6, 573–586. doi: 10.1016/0040-8166(74)90001-9
Edinger, E., Baker, K., Bélanger, D., Greeley, K., de Moura Neves, B., Sherwood, O., et al. (2019). Estimating calcium carbonate production by Northwest Atlantic octocorals. Geophys. Res. Abstr. 21.
Eguchi, M. (1948). Fossil helioporidae from Japan and the South Sea Islands. J. Paleontol. 22, 362–364.
Enochs, I. C., Manzello, D. P., Wirshing, H. H., Carlton, R., and Serafy, J. (2016). Micro-CT analysis of the Caribbean octocoral Eunicea flexuosa subjected to elevated pCO2. ICES J. Mar. Sci. 73, 910–919. doi: 10.1093/icesjms/fsv159
Fabricius, K., and Alderslade, P. (2001). Soft Corals and Sea Fans: A Comprehensive Guide to the Tropical Shallow Water Genera of the Central-West Pacific, the Indian Ocean and the Red Sea. Cape Cleveland, QLD: Australian Institute of Marine Science.
Fabricius, K. E., and Klumpp, D. W. (1995). Widespread mixotrophy in reef-inhabiting soft corals:the influence of depth, and colony expansion and contraction on photosynthesis. Mar. Ecol. Prog. Ser. 125, 195–204. doi: 10.3354/meps125195
Fabricius, K. E., Langdon, C., Uthicke, S., Humphrey, C., Noonan, S., De’ath, G., et al. (2011). Losers and winners in coral reefs acclimatized to elevated carbon dioxide concentrations. Nat. Clim. Chang. 1, 165–169. doi: 10.1038/nclimate1122
Falini, G., Fermani, S., and Goffredo, S. (2015). Coral biomineralization: a focus on intra-skeletal organic matrix and calcification. Semin. Cell Dev. Biol. 46, 17–26. doi: 10.1016/j.semcdb.2015.09.005
Farre, B., Cuif, J.-P., and Dauphin, Y. (2010). Occurrence and diversity of lipids in modern coral skeletons. Zoology 113, 250–257. doi: 10.1016/j.zool.2009.11.004
Fox, D. L., Smith, V. E., Grigg, R. W., and MacLeod, W. D. (1969). Some structural and chemical studies of the microspicules in the fan-coral Eugorgia ampla verrill. Comp. Biochem. Physiol. 28, 1103–1114. doi: 10.1016/0010-406x(69)90550-7
Frankel, R. B., Bazylinski, D. A., Johnson, M. S., and Taylor, B. L. (1997). Magneto-aerotaxis in marine coccoid bacteria. Biophys. J. 73, 994–1000. doi: 10.1016/s0006-3495(97)78132-3
Fukuda, I., Ooki, S., Fujita, T., Murayama, E., Nagasawa, H., Isa, Y., et al. (2003). Molecular cloning of a cDNA encoding a soluble protein in the coral exoskeleton. Biochem. Biophys. Res. Commun. 304, 11–17. doi: 10.1016/s0006-291x(03)00527-8
Gabay, Y., Benayahu, Y., and Fine, M. (2013). Does elevatedpCO2affect reef octocorals? Ecol. Evol. 3, 465–473. doi: 10.1002/ece3.351
Gabay, Y., Fine, M., Barkay, Z., and Benayahu, Y. (2014). Octocoral tissue provides protection from declining oceanic pH. PLoS One 9:e91553. doi: 10.1371/journal.pone.0091553
Gazeau, F., Parker, L. M., Comeau, S., Gattuso, J.-P., O’Connor, W. A., Martin, S., et al. (2013). Impacts of ocean acidification on marine shelled molluscs. Mar. Biol. 160, 2207–2245. doi: 10.1007/s00227-013-2219-3
Goffredo, S., Vergni, P., Reggi, M., Caroselli, E., Sparla, F., Levy, O., et al. (2011). The skeletal organic matrix from Mediterranean coral Balanophyllia europaea influences calcium carbonate precipitation. PLoS One 6:e22338. doi: 10.1371/journal.pone.0022338
Goldberg, W. M. (1988). Chemistry, histochemistry and microscopy of the organic matrix of spicules from a gorgonian coral. Histochemistry 89, 163–170. doi: 10.1007/bf00489919
Goldberg, W. M. (2001). Acid polysaccharides in the skeletal matrix and calicoblastic epithelium of the stony coral Mycetophyllia reesi. Tissue Cell 33, 376–387. doi: 10.1054/tice.2001.0191
Goldberg, W. M., and Benayahu, Y. (1987). Spicule formation in the gorgonian coral Pseudoplexaura flagellosa. 1: demonstration of intracellular and extracellular growth and the effect of ruthenium red during decalcification. Bull. Mar. Sci. 40, 287–303.
Gómez, C. E., Paul, V. J., Ritson-Williams, R., Muehllehner, N., Langdon, C., and Sánchez, J. A. (2015). Responses of the tropical gorgonian coral Eunicea fusca to ocean acidification conditions. Coral Reefs 34, 451–460. doi: 10.1007/s00338-014-1241-3
González-Pech, R. A., Vargas, S., Francis, W. R., and Wörheide, G. (2017). Transcriptomic resilience of the montipora digitata holobiont to low pH. Front. Mar. Sci. 4:403. doi: 10.3389/fmars.2017.00403
Grasshoff, M. (1999). The Shallow Water Gorgonians of New Caledonia and Adjacent Islands:(Coelenterata: Octocorallia). Frankfurt: Senckenbergische Naturforschende Gesellschaft.
Grillo, M.-C., Goldberg, W. M., and Allemand, D. (1993). Skeleton and sclerite formation in the precious red coral Corallium rubrum. Mar. Biol. 117, 119–128. doi: 10.1007/bf00346433
Hardie, L. A. (1996). Secular variation in seawater chemistry: an explanation for the coupled secular variation in the mineralogies of marine limestones and potash evaporites over the past 600 m.y. Geology 24, 279–283. doi: 10.1130/0091-7613(1996)024<0279:svisca>2.3.co;2
He, H., Veneklaas, E. J., Kuo, J., and Lambers, H. (2014). Physiological and ecological significance of biomineralization in plants. Trends Plant Sci. 19, 166–174. doi: 10.1016/j.tplants.2013.11.002
Herrán, N., Narayan, G. R., Reymond, C. E., and Westphal, H. (2017). Calcium carbonate production, coral cover and diversity along a distance gradient from stone town: a case study from Zanzibar, Tanzania. Front. Mar. Sci. 4:412. doi: 10.3389/fmars.2017.00412
Hill, D. (1960). Possible intermediates between Alcyonaria and Tabulata, Tabulata and Rugosa and Rugosa and Hexacoralla. 21st. Rept. Intern. Geol. Congr. 22, 51–58.
Hoegh-Guldberg, O., Mumby, P. J., Hooten, A. J., Steneck, R. S., Greenfield, P., Gomez, E., et al. (2007). Coral reefs under rapid climate change and ocean acidification. Science 318, 1737– 1742.
Holcomb, M., Venn, A. A., Tambutté, E., Tambutté, S., Allemand, D., Trotter, J., et al. (2014). Coral calcifying fluid pH dictates response to ocean acidification. Sci. Rep. 4:5207.
Hongo, Y., Yasuda, N., and NagaI, S. (2017). Identification of genes for synthesis of the blue pigment, biliverdin IXα, in the blue coral Heliopora coerulea. Biol. Bull. 232, 71–81. doi: 10.1086/692661
Horvath, E. A. (2019). A review of gorgonian coral species (Cnidaria, Octocorallia, Alcyonacea) held in the santa barbara museum of natural history research collection: focus on species from Scleraxonia, Holaxonia, Calcaxonia - Part III: suborder holaxonia continued, and suborder Calcaxonia. Zookeys 860, 183–306.
Howe, S. A., and Marshall, A. T. (2002). Temperature effects on calcification rate and skeletal deposition in the temperate coral, Plesiastrea versipora (Lamarck). J. Exp. Mar. Bio. Ecol. 275, 63–81. doi: 10.1016/s0022-0981(02)00213-7
Inoue, S., Kayanne, H., Yamamoto, S., and Kurihara, H. (2013). Spatial community shift from hard to soft corals in acidified water. Nat. Clim. Chang. 3, 683–687. doi: 10.1038/nclimate1855
Jackson, D. J., Macis, L., Reitner, J., Degnan, B. M., and Wörheide, G. (2007). Sponge paleogenomics reveals an ancient role for carbonic anhydrase in skeletogenesis. Science 316, 1893–1895. doi: 10.1126/science.1141560
Jeng, M.-S., Huang, H.-D., Dai, C.-F., Hsiao, Y.-C., and Benayahu, Y. (2011). Sclerite calcification and reef-building in the fleshy octocoral genus Sinularia (Octocorallia: alcyonacea). Coral Reefs 30, 925–933. doi: 10.1007/s00338-011-0765-z
Johnston, I. S. (1980). The ultrastructure of skeletogenesis in hermatypic corals. Int. Rev. Cytol. 67, 171–214. doi: 10.1016/s0074-7696(08)62429-8
Jolly, C., Berland, S., Milet, C., Borzeix, S., Lopez, E., and Doumenc, D. (2004). Zona localization of shell matrix proteins in mantle of Haliotis tuberculata (Mollusca, Gastropoda). Mar. Biotechnol. 6, 541–551. doi: 10.1007/s10126-004-3129-7
Karako-Lampert, S., Zoccola, D., Salmon-Divon, M., Katzenellenbogen, M., Tambutté, S., Bertucci, A., et al. (2014). Transcriptome analysis of the scleractinian coral Stylophora pistillata. PLoS One 9:e88615. doi: 10.1371/journal.pone.0088615
Kayal, E., Bentlage, B., Sabrina Pankey, M., Ohdera, A. H., Medina, M., Plachetzki, D. C., et al. (2018). Phylogenomics provides a robust topology of the major cnidarian lineages and insights on the origins of key organismal traits. BMC Evol. Biol. 18:68. doi: 10.1186/s12862-018-1142-0
Kayal, E., Roure, B., Philippe, H., Collins, A. G., and Lavrov, D. V. (2013). Cnidarian phylogenetic relationships as revealed by mitogenomics. BMC Evol. Biol. 13:5. doi: 10.1186/1471-2148-13-5
Kendall, J. J., Powell, E. N., Connor, S. J., Bright, T. J., and Zastrow, C. E. (1985). Effects of turbidity on calcification rate, protein concentration and the free amino acid pool of the coral Acropora cervicornis. Mar. Biol. 87, 33–46. doi: 10.1007/bf00397003
Kingsley, R. J., Bernhardt, A. M., Wilbur, K. M., and Watabe, N. (1987). Scleroblast cultures from the gorgonian Leptogorgia virgulata (Lamarck) (Coelenterata: gorgonacea). In Vitro Cell. Dev. Biol. 23, 297–302. doi: 10.1007/bf02623713
Kingsley, R. J., and Watabe, N. (1982). Ultrastructural investigation of spicule formation in the gorgonian Leptogorgia virgulata (Lamarck) (Coelenterata: gorgonacea). Cell Tissue Res. 223, 325–334. doi: 10.1007/bf01258493
Kingsley, R. J., and Watabe, N. (1983). Analysis of proteinaceous components of the organic matrices of spicules from the gorgonian Leptogorgia virgulata. Comp. Biochem. Physiol. Part B Comp. Biochem. 76, 443–447. doi: 10.1016/0305-0491(83)90273-0
Kingsley, R. J., and Watabe, N. (1984). Calcium uptake in the gorgonian Leptogorgia virgulata. The effects of atpase inhibitors. Comp. Biochem. Physiol. A Physiol. 79, 487–491. doi: 10.1016/0300-9629(84)90551-6
Kingsley, R. J., and Watabe, N. (1985). An autoradiographic study of calcium transport in spicule formation in the gorgonian Leptogorgia virgulata (Lamarck) (Coelenterata: gorgonacea). Cell Tissue Res. 239, 305–310.
Kingsley, R. J., and Watabe, N. (1987). Role of carbonic anhydrase in calcification in the gorgonian Leptogorgia virgulata. J. Exp. Zool. 241, 171–180. doi: 10.1002/jez.1402410203
Knoll, A. H. (2003). Biomineralization and evolutionary history. Rev. Mineral. Geochem. 54, 329–356. doi: 10.1515/9781501509346-016
Kocurko, M. J., and Kocurko, D. J. (1992). Fossil octocorallia of the red bluff formation. Lower Oligocene Mississippi. J. Paleontol. 66, 594–602. doi: 10.1017/s0022336000024458
Krieger, K. J., and Wing, B. L. (2002). Megafauna associations with deepwater corals (Primnoa spp.) in the Gulf of Alaska. Hydrobiologia 471, 83–90.
Kuffner, I. B., Andersson, A. J., Jokiel, P. L., Rodgers, K. S., and Mackenzie, F. T. (2008). Decreased abundance of crustose coralline algae due to ocean acidification. Nat. Geosci. 1, 114–117. doi: 10.1038/ngeo100
Laipnik, R., Bissi, V., Sun, C.-Y., Falini, G., Gilbert, P. U. P. A., and Mass, T. (2019). Coral acid rich protein selects vaterite polymorph in vitro. J. Struct. Biol. 209:107431. doi: 10.1016/j.jsb.2019.107431
Landing, E., Antcliffe, J. B., Brasier, M. D., and English, A. B. (2015). Distinguishing Earth’s oldest known bryozoan (Pywackia, late Cambrian) from pennatulacean octocorals (Mesozoic–Recent). J. Paleontol. 89, 292–317. doi: 10.1017/jpa.2014.26
Landing, E., English, A., and Keppie, J. D. (2010). Cambrian origin of all skeletalized metazoan phyla—Discovery of Earth’s oldest bryozoans (Upper Cambrian, southern Mexico). Geology 38, 547–550. doi: 10.1130/g30870.1
Lau, Y. W., and Reimer, J. D. (2019a). A first phylogenetic study on stoloniferous octocorals off the coast of Kota Kinabalu, Sabah, Malaysia, with the description of two new genera and five new species. Zookeys 872, 127–158. doi: 10.3897/zookeys.872.36288
Lau, Y. W., and Reimer, J. D. (2019b). Zooxanthellate, sclerite-free, and pseudopinnuled octocoral Hadaka nudidomus gen. nov. et sp. nov. (Anthozoa, octocorallia) from mesophotic reefs of the Southern Ryukyus Islands. Diversity 11:176. doi: 10.3390/d11100176
Lau, Y. W., Stokvis, F. R., Imahara, Y., and Reimer, J. D. (2019). The stoloniferous octocoral, Hanabira yukibana, gen. nov., sp. nov., of the southern Ryukyus has morphological and symbiont variation. Contrib. Zool. 88, 54–77. doi: 10.1163/18759866-20191355
Lau, Y. W., Stokvis, F. R., van Ofwegen, L. P., and Reimer, J. D. (2018). Stolonifera from shallow waters in the north-western Pacific: a description of a new genus and two new species within the Arulidae (Anthozoa, Octocorallia). Zookeys 790, 1–19.
Lawniczak, A. (1987). Les modalités de croissance de l’axe calcaire chez Corallium johnsoni. Senckenb. Marit. 19, 149–161.
Le Goff, C., Ganot, P., Zoccola, D., Caminiti-Segonds, N., Allemand, D., and Tambutté, S. (2016). Carbonic anhydrases in cnidarians: novel perspectives from the octocorallian Corallium rubrum. PLoS One 11:e0160368. doi: 10.1371/journal.pone.0160368
Le Goff, C., Tambutté, E., Venn, A. A., Techer, N., Allemand, D., and Tambutté, S. (2017). In vivo pH measurement at the site of calcification in an octocoral. Sci. Rep. 7:11210.
Le Tissier, M. D. (1991). The nature of the skeleton and skeletogenic tissues in the Cnidaria. Hydrobiologia 216, 397–402. doi: 10.1007/978-94-011-3240-4_57
Lenz, E. A., and Edmunds, P. J. (2017). Branches and plates of the morphologically plastic coral Porites rus are insensitive to ocean acidification and warming. J. Exp. Mar. Bio. Ecol. 486, 188–194. doi: 10.1016/j.jembe.2016.10.002
Leverette, C. L., Warren, M., Smith, M.-A., and Smith, G. W. (2008). Determination of carotenoid as the purple pigment in Gorgonia ventalina sclerites using Raman microscopy. Spectrochim. Acta A Mol. Biomol. Spectrosc. 69, 1058–1061. doi: 10.1016/j.saa.2007.07.018
Lewis, J. C., Barnowski, T. F., and Telesnicki, G. J. (1992). Characteristics of carbonates of gorgonian axes (coelenterata, octocorallia). Biol. Bull. 183, 278–296. doi: 10.2307/1542215
Lowenstam, H. A. (1981). Minerals formed by organisms. Science 211, 1126–1131. doi: 10.1126/science.7008198
Lowenstam, H. A., and Weiner, S. (1989). On Biomineralization, New York, NY: Oxford University Press, 144–167.
Lowenstein, T. K., Timofeeff, M. N., Brennan, S. T., Hardie, L. A., and Demicco, R. V. (2001). Oscillations in Phanerozoic seawater chemistry: evidence from fluid inclusions. Science 294, 1086–1088. doi: 10.1126/science.1064280
Lozouet, P., and Molodtsova, T. (2008). Filling a gap: the first occurrences of Epiphaxum (Cnidaria: helioporacea: lithotelestidae) in the eocene, oligocene and miocene. Palaeontology 51, 241–250. doi: 10.1111/j.1475-4983.2007.00744.x
Lucas, J. M., and Knapp, L. W. (1996). Biochemical characterization of purified carbonic anhydrase from the octocoral Leptogorgia virgulata. Mar. Biol. 126, 471–477. doi: 10.1007/bf00354629
Lucas, J. M., and Knapp, L. W. (1997). A physiological evaluation of carbon sources for calcification in the octocoral Leptogorgia virgulata (Lamarck). J. Exp. Biol. 200, 2653–2662.
Macintyre, I. G., Bayer, F. M., Logan, M. A. V., and Skinner, H. C. W. (2000). Possible vestige of early phosphatic biomineralization in gorgonian octocorals (Coelenterata). Geology 28, 455–458. doi: 10.1130/0091-7613(2000)028<0455:pvoepb>2.3.co;2
Mann, K. (2015). The calcified eggshell matrix proteome of a songbird, the zebra finch (Taeniopygia guttata). Proteome Sci. 13:29.
Mann, K., Poustka, A. J., and Mann, M. (2008). The sea urchin (Strongylocentrotus purpuratus) test and spine proteomes. Proteome Sci. 6:22. doi: 10.1186/1477-5956-6-22
Mann, K., Wilt, F. H., and Poustka, A. J. (2010). Proteomic analysis of sea urchin (Strongylocentrotus purpuratus) spicule matrix. Proteome Sci. 8:33. doi: 10.1186/1477-5956-8-33
Mann, S. (1983). “Mineralization in biological systems,” in Inorganic Elements in Biochemistry Structure and Bonding, eds P. H. Connett, H. Folłmann, M. Lammers, S. Mann, J. D. Odom, and K. E. Wetterhahn (Berlin: Springer Berlin Heidelberg), 125–174. doi: 10.1007/bfb0111320
Marin, F., and Luquet, G. (2007). “Unusually acidic proteins in biomineralization,” in Handbook of Biomineralization, ed. E. Buerlein (Weinheim: Wiley-VCH Verlag GmbH), 273–290. doi: 10.1002/9783527619443.ch16
Marshall, A. T., and Clode, P. (2004). Calcification rate and the effect of temperature in a zooxanthellate and an azooxanthellate scleractinian reef coral. Coral Reefs 23, 218–224. doi: 10.1007/s00338-004-0369-y
Marubini, F., Ferrier-Pages, C., and Cuif, J.-P. (2003). Suppression of skeletal growth in scleractinian corals by decreasing ambient carbonate-ion concentration: a cross-family comparison. Proc. Biol. Sci. 270, 179–184. doi: 10.1098/rspb.2002.2212
McCulloch, M., Trotter, J., Montagna, P., Falter, J., Dunbar, R., Freiwald, A., et al. (2012). Resilience of cold-water scleractinian corals to ocean acidification: boron isotopic systematics of pH and saturation state up-regulation. Geochim. Cosmochim. Acta 87, 21–34. doi: 10.1016/j.gca.2012.03.027
McFadden, C. S., France, S. C., Sánchez, J. A., and Alderslade, P. (2006). A molecular phylogenetic analysis of the Octocorallia (Cnidaria: anthozoa) based on mitochondrial protein-coding sequences. Mol. Phylogenet. Evol. 41, 513–527. doi: 10.1016/j.ympev.2006.06.010
McFadden, C. S., and Ofwegen, L. P. V. (2017). Revisionary systematics of the endemic soft coral fauna (Octocorallia: alcyonacea: alcyoniina) of the agulhas bioregion, South Africa. Zootaxa 4363, 451–488. doi: 10.11646/zootaxa.4363.4.1
McFadden, C. S., Quattrini, A. M., Brugler, M. R., Cowman, P. F., Dueñas, L. F., Kitahara, M. V., et al. (2021). Phylogenomics, origin and diversification of anthozoans (Phylum Cnidaria). Syst. Biol. syaa103. doi: 10.1093/sysbio/syaa103
McFadden, C. S., Sánchez, J. A., and France, S. C. (2010). Molecular phylogenetic insights into the evolution of Octocorallia: a review. Integr. Comp. Biol. 50, 389–410. doi: 10.1093/icb/icq056
Menzel, L. P., Tondo, C., Stein, B., and Bigger, C. H. (2015). Histology and ultrastructure of the coenenchyme of the octocoral Swiftia exserta, a model organism for innate immunity/graft rejection. Zoology 118, 115–124. doi: 10.1016/j.zool.2014.09.002
Merlin, J. C., and Delé-Dubois, M. L. (1986). Resonance Raman characterization of polyacetylenic pigments in the calcareous skeleton. Comp. Biochem. Physiol. Part B Comp. Biochem. 84, 97–103. doi: 10.1016/0305-0491(86)90277-4
Miyamoto, H., Miyashita, T., Okushima, M., Nakano, S., Morita, T., and Matsushiro, A. (1996). A carbonic anhydrase from the nacreous layer in oyster pearls. Proc. Natl. Acad. Sci. U.S.A. 93, 9657–9660. doi: 10.1073/pnas.93.18.9657
Miyazaki, Y., and Reimer, J. D. (2015). A new genus and species of octocoral with aragonite calcium-carbonate skeleton (Octocorallia, helioporacea) from Okinawa, Japan. Zookeys 511, 1–23.
Mollica, N. R., Guo, W., Cohen, A. L., Huang, K.-F., Foster, G. L., Donald, H. K., et al. (2018). Ocean acidification affects coral growth by reducing skeletal density. Proc. Natl. Acad. Sci. U.S.A. 115, 1754–1759. doi: 10.1073/pnas.1712806115
Mongin, M., Baird, M. E., Tilbrook, B., Matear, R. J., Lenton, A., Herzfeld, M., et al. (2016). The exposure of the great barrier reef to ocean acidification. Nat. Commun. 7:10732.
Morse, J. W., and Mackenzie, F. T. (1990). Geochemistry of Sedimentary Carbonates. Amsterdam: Elsevier.
Morse, J. W., Wang, Q., and Tsio, M. Y. (1997). Influences of temperature and Mg:Ca ratio on CaCO3 precipitates from seawater. Geology 25, 85–87. doi: 10.1130/0091-7613(1997)025<0085:iotamc>2.3.co;2
Mortensen, P. B., and Buhl-Mortensen, L. (2005). Morphology and growth of the deep-water gorgonians Primnoa resedaeformis and Paragorgia arborea. Mar. Biol. 147, 775–788. doi: 10.1007/s00227-005-1604-y
Moseley, H. N. (1876). On the structure and relations of the alcyonarian Heliopora caerulea with some account of the anatomy of a species of Sarcophyton, notes on the structure of species of the genera Millepora, Pocillopora, and Stylaster, and remarks on the affinities of certain palaeozoic corals. Philos. Trans. R. Soc. Lond. 166, 91–129. doi: 10.1098/rstl.1876.0004
Naggi, A., Torri, G., Iacomini, M., Colombo Castelli, G., Reggi, M., Fermani, S., et al. (2018). Structure and function of stony coral intraskeletal polysaccharides. ACS Omega 3, 2895–2901. doi: 10.1021/acsomega.7b02053
Nishishira, M. (1974). Human interference with the coral community and Acanthaster infestation of Okinawa. Proc. 2nd Int. Coral Reef Symp. 1974, 577–590.
Noé, S. U., and Dullo, W.-C. (2006). Skeletal morphogenesis and growth mode of modern and fossil deep-water isidid gorgonians (Octocorallia) in the West Pacific (New Zealand and Sea of Okhotsk). Coral Reefs 25, 303–320. doi: 10.1007/s00338-006-0095-8
Pérez, C. D., de Moura Neves, B., Cordeiro, R. T., Williams, G. C., and Cairns, S. D. (2016). “Diversity and distribution of octocorallia,” in The Cnidaria, Past, Present and Future: The world of Medusa and her sisters, eds S. Goffredo and Z. Dubinsky (Cham: Springer International Publishing), 109–123. doi: 10.1007/978-3-319-31305-4_8
Poliseno, A., Feregrino, C., Sartoretto, S., Aurelle, D., Wörheide, G., McFadden, C. S., et al. (2017). Comparative mitogenomics, phylogeny and evolutionary history of Leptogorgia (Gorgoniidae). Mol. Phylogenet. Evol. 115, 181–189. doi: 10.1016/j.ympev.2017.08.001
Porter, S. M. (2010). Calcite and aragonite seas and the de novo acquisition of carbonate skeletons. Geobiology 8, 256–277. doi: 10.1111/j.1472-4669.2010.00246.x
Prada, C., Schizas, N. V., and Yoshioka, P. M. (2008). Phenotypic plasticity or speciation? A case from a clonal marine organism. BMC Evol. Biol. 8:47. doi: 10.1186/1471-2148-8-47
Puverel, S., Houlbrèque, F., Tambutté, E., Zoccola, D., Payan, P., Caminiti, N., et al. (2007). Evidence of low molecular weight components in the organic matrix of the reef building coral, Stylophora pistillata. Comp. Biochem. Physiol. A Mol. Integr. Physiol. 147, 850–856. doi: 10.1016/j.cbpa.2006.10.045
Puverel, S., Tambutté, E., Pereira-Mouriès, L., Zoccola, D., Allemand, D., and Tambutté, S. (2005). Soluble organic matrix of two Scleractinian corals: partial and comparative analysis. Comp. Biochem. Physiol. B Biochem. Mol. Biol. 141, 480–487. doi: 10.1016/j.cbpc.2005.05.013
Quattrini, A. M., Etnoyer, P. J., Doughty, C., English, L., Falco, R., Remon, N., et al. (2014). A phylogenetic approach to octocoral community structure in the deep Gulf of Mexico. Deep Sea Res. Part 2 Top. Stud. Oceanogr. 99, 92–102. doi: 10.1016/j.dsr2.2013.05.027
Quattrini, A. M., Rodríguez, E., Faircloth, B. C., Cowman, P. F., Brugler, M. R., Farfan, G. A., et al. (2020). Palaeoclimate ocean conditions shaped the evolution of corals and their skeletons through deep time. Nat Ecol Evol. 4, 1531–1538. doi: 10.1038/s41559-020-01291-1
Rahman, M. A., and Oomori, T. (2009). In vitro regulation of CaCO3 crystal growth by the highly acidic proteins of calcitic sclerites in soft coral, Sinularia polydactyla. Connect. Tissue Res. 50, 285–293. doi: 10.3109/03008200802714933
Rahman, M. A., Oomori, T., and Wörheide, G. (2011). Calcite formation in soft coral sclerites is determined by a single reactive extracellular protein. J. Biol. Chem. 286, 31638–31649. doi: 10.1074/jbc.m109.070185
Rahman, M. A., Shinjo, R., Oomori, T., and Wörheide, G. (2013). Analysis of the proteinaceous components of the organic matrix of calcitic sclerites from the soft coral Sinularia sp. PLoS One 8:e58781. doi: 10.1371/journal.pone.0058781
Ramos-Silva, P., Kaandorp, J., Huisman, L., Marie, B., Zanella-Cléon, I., Guichard, N., et al. (2013). The skeletal proteome of the coral Acropora millepora: the evolution of calcification by co-option and domain shuffling. Mol. Biol. Evol. 30, 2099–2112. doi: 10.1093/molbev/mst109
Reggi, M., Fermani, S., Samorì, C., Gizzi, F., Prada, F., Dubinsky, Z., et al. (2016). Influence of intra-skeletal coral lipids on calcium carbonate precipitation. CrystEngComm 18, 8829–8833. doi: 10.1039/c6ce01939k
Reich, M. (2009). “A critical review of octocorallian fossil record (Cnidaria: anthozoa),” in Proceedings of the International Conference on the Cambrian Explosion–Walcott, eds M. R. Smith, L. J. O’Brien, and J. B. Caron (Toronto: Burgess Shale Consortium).
Reich, M., and Kutscher, M. (2011). Sea pens (Octocorallia: pennatulacea) from the late cretaceous of northern Germany. J. Paleontol. 85, 1042–1051. doi: 10.1666/10-109.1
Ribes, M., Coma, R., and Gili, J.-M. (1998). Heterotrophic feeding by gorgonian corals with symbiotic zooxanthella. Limnol. Oceanogr. 43, 1170–1179. doi: 10.4319/lo.1998.43.6.1170
Ries, J. B., Cohen, A. L., and McCorkle, D. C. (2009). Marine calcifiers exhibit mixed responses to CO2-induced ocean acidification. Geology 37, 1131–1134. doi: 10.1130/g30210a.1
Roberts, J. M., Murray Roberts, J., Wheeler, A., Freiwald, A., and Cairns, S. (2009). Cold Water Corals: The Biology and Geology of Deep-Sea Coral Habitats. Cambridge: Cambridge University Press.
Rodolfo-Metalpa, R., Houlbrèque, F., Tambutté, É, Boisson, F., Baggini, C., Patti, F. P., et al. (2011). Coral and mollusc resistance to ocean acidification adversely affected by warming. Nat. Clim. Chang. 1, 308–312. doi: 10.1038/nclimate1200
Rokas, A., Krüger, D., and Carroll, S. B. (2005). Animal evolution and the molecular signature of radiations compressed in time. Science 310, 1933–1938. doi: 10.1126/science.1116759
Rüdiger, W., Klose, W., Vuillaume, M., and Barbier, M. (1968). On the structure of pterobilin, the blue pigment ofPieris brassicae. Experientia 24, 1000–1000. doi: 10.1007/bf02138705
Ruzicka, R. R., Colella, M. A., Porter, J. W., Morrison, J. M., Kidney, J. A., Brinkhuis, V., et al. (2013). Temporal changes in benthic assemblages on Florida Keys reefs 11 years after the 1997/1998 El Niño. Mar. Ecol. Prog. Ser. 489, 125–141.
Saleuddin, A. S. M. (1977). Ultrastructural studies on the formation of the periostracum inHelix aspersa (Mollusca). Calcif. Tissue Res. 22, 49–65. doi: 10.1007/bf02010346
Sánchez, J. A., Lasker, H. R., and Taylor, D. J. (2003a). Phylogenetic analyses among octocorals (Cnidaria): mitochondrial and nuclear DNA sequences (lsu-rRNA, 16S and ssu-rRNA, 18S) support two convergent clades of branching gorgonians. Mol. Phylogenet. Evol. 29, 31–42. doi: 10.1016/s1055-7903(03)00090-3
Sánchez, J. A., McFadden, C. S., France, S. C., and Lasker, H. R. (2003b). Molecular phylogenetic analyses of shallow-water Caribbean octocorals. Mar. Biol. 142, 975–987. doi: 10.1007/s00227-003-1018-7
Schlagintweit, F., and Gawlick, H.-J. (2009). The incertae sedis Carpathoporella Dragastan, 1995, from the lower cretaceous of albania: skeletal elements (sclerites, internodes/branches, holdfasts) of colonial octocorals. Facies 55, 553–573. doi: 10.1007/s10347-009-0185-5
Schouten, S., Hopmans, E. C., Forster, A., van Breugel, Y., Kuypers, M. M. M., and Sinninghe Damsteì, J. S. (2003). Extremely high sea-surface temperatures at low latitudes during the middle Cretaceous as revealed by archaeal membrane lipids. Geology 31, 1069–1072. doi: 10.1130/g19876.1
Scott, R. W. (1988). Evolution of late Jurassic and early Cretaceous reef biotas. Palaios 3, 184–193. doi: 10.2307/3514529
Sevilgen, D. S., Venn, A. A., Hu, M. Y., Tambutté, E., de Beer, D., Planas-Bielsa, V., et al. (2019). Full in vivo characterization of carbonate chemistry at the site of calcification in corals. Sci Adv 5:eaau7447. doi: 10.1126/sciadv.aau7447
Shaish, L., Levy, G., Katzir, G., and Rinkevich, B. (2010). Coral reef restoration (bolinao, philippines) in the face of frequent natural catastrophes. Restor. Ecol. 18, 285–299. doi: 10.1111/j.1526-100x.2009.00647.x
Silberberg, M. S., Ciereszko, L. S., Jacobson, R. A., and Smith, E. C. (1972). Evidence for a collagen-like protein within spicules of coelenterates. Comp. Biochem. Physiol. Part B Comp. Biochem. 43, 323–332. doi: 10.1016/0305-0491(72)90291-x
Simion, P., Philippe, H., Baurain, D., Jager, M., Richter, D. J., Di Franco, A., et al. (2017). A large and consistent phylogenomic dataset supports sponges as the sister group to all other animals. Curr. Biol. 27, 958–967. doi: 10.1016/j.cub.2017.02.031
Simpson, A., and Watling, L. (2011). Precious corals (Coralliidae) from north-western Atlantic Seamounts. J. Mar. Biol. Assoc. U. K. 91, 369–382. doi: 10.1017/s002531541000086x
Smith, S. V., and Kinsey, D. W. (1976). Calcium carbonate production, coral reef growth, and sea level change. Science 194, 937–939. doi: 10.1126/science.194.4268.937
Solomon, S., Manning, M., Marquis, M., Qin, D., Chen, Z., Avery, K. B., et al. (2007). Climate Change 2007: The Physical Science Basis: Working Group I Contribution to the Fourth Assessment Report of the IPCC. Cambridge: Cambridge Univ. Press.
Song, H., Wignall, P. B., Song, H., Dai, X., and Chu, D. (2019). Seawater temperature and dissolved oxygen over the past 500 million years. J. Earth Sci. 30, 236–243. doi: 10.1007/s12583-018-1002-2
Sorokin, Y. I. (1991). Biomass, metabolic rates and feeding of some common reef zoantharians and octocorals. Mar. Freshw. Res. 42, 729–741. doi: 10.1071/mf9910729
Spencer Davies, P. (1990). A rapid method for assessing growth rates of corals in relation to water pollution. Mar. Pollut. Bull. 21, 346–348. doi: 10.1016/0025-326x(90)90797-c
Stampar, S. N., Maronna, M. M., Kitahara, M. V., Reimer, J. D., and Morandini, A. C. (2014). Fast-evolving mitochondrial DNA in Ceriantharia: a reflection of hexacorallia paraphyly? PLoS One 9:e86612. doi: 10.1371/journal.pone.0086612
Stanley, G. D. Jr. (1981). Early history of scleractinian corals and its geological consequences. Geology 9, 507–511. doi: 10.1130/0091-7613(1981)9<507:ehosca>2.0.co;2
Stanley, S. M., and Hardie, L. A. (1998). Secular oscillations in the carbonate mineralogy of reef-building and sediment-producing organisms driven by tectonically forced shifts in seawater chemistry. Palaeogeogr. Palaeoclimatol. Palaeoecol. 144, 3–19. doi: 10.1016/s0031-0182(98)00109-6
Stobart, B., Teleki, K., Buckley, R., Downing, N., and Callow, M. (2005). Coral recovery at aldabra atoll, seychelles: five years after the 1998 bleaching event. Philos. Trans. A Math. Phys. Eng. Sci. 363, 251–255. doi: 10.1098/rsta.2004.1490
Takeuchi, T., Plasseraud, L., Ziegler-Devin, I., Brosse, N., Shinzato, C., Satoh, N., et al. (2018). Biochemical characterization of the skeletal matrix of the massive coral, Porites australiensis–The saccharide moieties and their localization. J. Struct. Biol. 203, 219–229. doi: 10.1016/j.jsb.2018.05.011
Tambutté, S., Holcomb, M., Ferrier-Pagès, C., Reynaud, S., Tambutté, É, Zoccola, D., et al. (2011). Coral biomineralization: from the gene to the environment. J. Exp. Mar. Bio. Ecol. 408, 58–78. doi: 10.1016/j.jembe.2011.07.026
Taylor, P. D., Berning, B., and Wilson, M. A. (2013). Reinterpretation of the cambrian “bryozoan” Pywackia as an octocoral. J. Paleontol. 87, 984–990. doi: 10.1666/13-029
Tentori, E., and van Ofwegen, L. P. (2011). Patterns of distribution of calcite crystals in soft corals sclerites. J. Morphol. 272, 614–628. doi: 10.1002/jmor.10942
Van Alstyne, K. L., Wylie, C. R., Paul, V. J., and Meyer, K. (1992). Antipredator defenses in tropical pacific soft corals (coelenterata: alcyonacea). I. sclerites as defenses against generalist carnivorous fishes. The Biological Bulletin 182, 231–240. doi: 10.2307/1542116
Vargas, S., Breedy, O., and Guzman, H. M. (2010a). The phylogeny of Pacifigorgia (coelenterata, octocorallia, gorgoniidae): a case study of the use of continuous characters in the systematics of the octocorallia. Zoosystema 32, 5–18. doi: 10.5252/z2010n1a1
Vargas, S., Breedy, O., Siles, F., and Guzman, H. M. (2010b). How many kinds of sclerite? Towards a morphometric classification of gorgoniid microskeletal components. Micron 41, 158–164. doi: 10.1016/j.micron.2009.08.009
Vargas, S., Eitel, M., Breedy, O., and Schierwater, B. (2010c). Molecules match morphology: mitochondrial DNA supports bayer’s lytreia – bebryce – heterogorgia (alcyonacea : octocorallia) clade hypothesis. Invertebr. Syst. 24:23. doi: 10.1071/is09033
Vargas, S., Guzman, H. M., Breedy, O., and Wörheide, G. (2014). Molecular phylogeny and DNA barcoding of tropical eastern Pacific shallow-water gorgonian octocorals. Mar. Biol. 161, 1027–1038. doi: 10.1007/s00227-014-2396-8
Velimirov, B. (1980). growth aspects and magnesium carbonate concentrations of the reef building octocoral Heliopora coerulea (pallas) after transplantation. Mar. Ecol. 1, 155–168. doi: 10.1111/j.1439-0485.1980.tb00217.x
Venn, A. A., Tambutté, E., Holcomb, M., Laurent, J., Allemand, D., and Tambutté, S. (2013). Impact of seawater acidification on pH at the tissue–skeleton interface and calcification in reef corals. Proc. Natl. Acad. Sci. U.S.A. 110, 1634–1639. doi: 10.1073/pnas.1216153110
Vielzeuf, D., Gagnon, A. C., Ricolleau, A., Devidal, J.-L., Balme-Heuze, C., Yahiaoui, N., et al. (2018). Growth kinetics and distribution of trace elements in precious corals. Front. Earth Sci. Chin. 6:167. doi: 10.3389/feart.2018.00167
Vielzeuf, D., Garrabou, J., Gagnon, A., Ricolleau, A., Adkins, J., Günther, D., et al. (2013). Distribution of sulphur and magnesium in the red coral. Chem. Geol. 355, 13–27. doi: 10.1016/j.chemgeo.2013.07.008
Watabe, N. (1981). Crystal growth of calcium carbonate in the invertebrates. Prog. Cryst. Growth Charact. Mater. 4, 99–147. doi: 10.1016/0146-3535(81)90050-2
Watabe, N., and Kingsley, R. J. (1992). “Calcification in octocorals,” in Hard Tissue Mineralization and Demineralization, eds S. Suga and N. Watabe (Tokyo: Springer Japan), 127–147. doi: 10.1007/978-4-431-68183-0_9
Weinbauer, M. G., Brandstätter, F., and Velimirov, B. (2000). On the potential use of magnesium and strontium concentrations as ecological indicators in the calcite skeleton of the red coral (Corallium rubrum). Mar. Biol. 137, 801–809. doi: 10.1007/s002270000432
Weinbauer, M. G., and Vellmirov, B. (1995). Calcium, magnesium and strontium concentrations in the calcite sclerites of Mediterranean gorgonians (coelenterata: octocorallia). Estuar. Coast. Shelf Sci. 40, 87–104. doi: 10.1016/0272-7714(95)90015-2
Weiner, S., and Hood, L. (1975). Soluble protein of the organic matrix of mollusk shells: a potential template for shell formation. Science 190, 987–989. doi: 10.1126/science.1188379
Weiner, S., Traub, W., and Lowenstam, H. A. (1983). “Organic matrix in calcified exoskeletons,” in Biomineralization and Biological Metal Accumulation, eds P. Westbroek and E. W. de Jong (Dordrecht: Springer), 205–224. doi: 10.1007/978-94-009-7944-4_18
West, J. M. (1997). Plasticity in the sclerites of a gorgonian coral: tests of water motion. Light Level Damage Cues. Biol. Bull. 192, 279–289. doi: 10.2307/1542721
Williams, G. C. (1986). Morphology, Systematics, and Variability of the Southern African Soft Coral Alcyonium Variabile (J. Stuart Thomson, 1921)(Octocorallia, Alcyoniidae). Gardens: South African Museum.
Williams, G. C. (1992). The alcyonacea of southern Africa. stoloniferous octocorals and soft corals. Ann. S. Afr. Mus. 100, 249–358.
Williams, G. C. (2015). A new genus and species of pennatulacean octocoral from equatorial West Africa (Cnidaria, Anthozoa, Virgulariidae). Zookeys 546, 39–50. doi: 10.3897/zookeys.546.6344
Williams, G. C., and Cairns, S. D. (2013). Biodiversity Myth Busters, Octocoral Research Center. Available online at: http://researcharchive.calacademy.org/research/izg/Biodiversity%20Myth%20Busters%202.html (accessed March 9, 2015).
Williams, G. C., and Cairns, S. D. (2015). Systematic List of Valid Octocoral Genera, Octocoral Research Center. Available online at: http://researcharchive.calacademy.org/research/izg/OCTOCLASS.htm (accessed March 9, 2015).
Woodland, W. (1905). Memoirs: studies in spicule formation: I.–spicule formation in Alcyonium digitatum; with remarks on the histology. J. Cell Sci. 2, 283–304.
Yasuda, N., Abe, M., Takino, T., Kimura, M., Lian, C., Nagai, S., et al. (2012). Large-scale mono-clonal structure in the north peripheral population of blue coral, Heliopora coerulea. Mar. Genomics 7, 33–35. doi: 10.1016/j.margen.2012.02.001
Yoshimura, T., Tanimizu, M., Inoue, M., Suzuki, A., Iwasaki, N., and Kawahata, H. (2011). Mg isotope fractionation in biogenic carbonates of deep-sea coral, benthic foraminifera, and hermatypic coral. Anal. Bioanal. Chem. 401, 2755–2769. doi: 10.1007/s00216-011-5264-0
Zann, L. P., and Bolton, L. (1985). The distribution, abundance and ecology of the blue coral Heliopora coerulea (Pallas) in the Pacific. Coral Reefs 4, 125–134. doi: 10.1007/bf00300871
Zapata, F., Goetz, F. E., Smith, S. A., Howison, M., Siebert, S., Church, S. H., et al. (2015). Phylogenomic analyses support traditional relationships within cnidaria. PLoS One 10:e0139068. doi: 10.1371/journal.pone.0139068
Zapata Guardiola, R., and López González, P. J. (2012). Revision and redescription of the species previously included in the genus Amphilaphis studer and wright in studer, 1887 (Octocorallia: primnoidae). Sci. Mar. 76, 357–380. doi: 10.3989/scimar.03278.18b
Zeebe, R. E. (2001). Seawater pH and isotopic paleotemperatures of cretaceous oceans. Palaeogeogr. Palaeoclimatol. Palaeoecol. 170, 49–57. doi: 10.1016/s0031-0182(01)00226-7
Keywords: octocorallia, biomineralization, aragonite, evolutionary biology, calcite
Citation: Conci N, Vargas S and Wörheide G (2021) The Biology and Evolution of Calcite and Aragonite Mineralization in Octocorallia. Front. Ecol. Evol. 9:623774. doi: 10.3389/fevo.2021.623774
Received: 30 October 2020; Accepted: 01 February 2021;
Published: 19 February 2021.
Edited by:
Frederic Marin, Délégation Centre-Est (CNRS), FranceReviewed by:
Philippe Ganot, Centre Scientifique de Monaco, MonacoFelipe Aguilera, University of Concepcion, Chile
Copyright © 2021 Conci, Vargas and Wörheide. This is an open-access article distributed under the terms of the Creative Commons Attribution License (CC BY). The use, distribution or reproduction in other forums is permitted, provided the original author(s) and the copyright owner(s) are credited and that the original publication in this journal is cited, in accordance with accepted academic practice. No use, distribution or reproduction is permitted which does not comply with these terms.
*Correspondence: Gert Wörheide, d29lcmhlaWRlQGxtdS5kZQ==