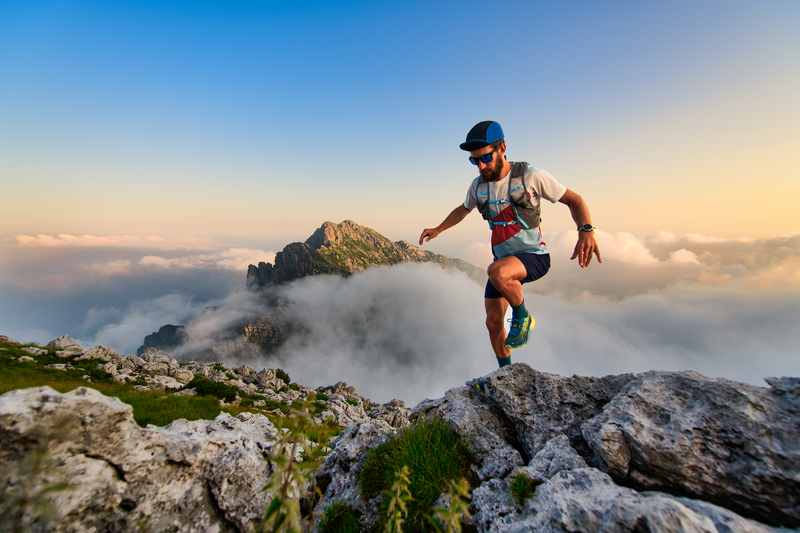
94% of researchers rate our articles as excellent or good
Learn more about the work of our research integrity team to safeguard the quality of each article we publish.
Find out more
MINI REVIEW article
Front. Ecol. Evol. , 19 November 2021
Sec. Population, Community, and Ecosystem Dynamics
Volume 9 - 2021 | https://doi.org/10.3389/fevo.2021.617376
The age, sex, and sexual maturity of individual animals are key parameters in assessing wild populations and informing conservation management strategies. These parameters represent the reproductive potential of a population and can indicate recovery rates or vulnerabilities. Natural populations of wild animals are difficult to study; logistically, economically, and due to the impacts of invasive biomonitoring. Genetic and epigenetic analyses offer a low impact, low cost, and information-rich alternative. As epigenetic mechanisms are intrinsically linked with both biological aging and reproductive processes, DNA methylation can be used as a suitable biomarker for population biology study. This review assesses published research utilizing DNA methylation analysis in relation to three key population parameters: age, sex, and sexual maturity. We review studies on wild vertebrates that investigate epigenetic age relationships, with successful age estimation assays designed for mammals, birds, and fish. For both determination of sex and identification of sexual maturity, very little has been explored regarding DNA methylation-based assays. Related research, however, confirms the links between DNA methylation and these processes. Future development of age estimation assays for underrepresented and key conservation taxa is suggested, as is the experimental development and design of DNA methylation-based assays for both sex and sexual maturity identification, further expanding the genomics toolkit for population biology studies.
We are currently experiencing a worldwide environmental crisis, with animal species increasingly threatened by habitat loss, pollution, climate change, and overexploitation (Thomas et al., 2004; Hooper et al., 2012; Darimont et al., 2015; Maxwell et al., 2016; Hammerschlag and Gallagher, 2017; Nabi et al., 2018; Zabel et al., 2019). Anthropogenic stressors have impacted almost all vertebrate species, with more than one-fifth characterized as “threatened” by the International Union for Conservation of Nature (Hoffmann et al., 2010). Effective conservation policies are therefore necessary to slow global biodiversity losses and maintain viable populations of vertebrates (Reed et al., 2003; Hoffmann et al., 2010). Monitoring the efficacy of conservation efforts and determining current population trends in threatened species requires knowledge of key parameters of a species’ population biology, such as rates of birth, death, and migration.
The size, growth rate, and distribution of an animal population is determined by various life history traits, including age distribution, sex ratio, reproductive interval, and litter size (McNab, 1980; Reed et al., 2003; McRae et al., 2012). Population trend assessments can be made by measuring these characteristics within wild populations. Historically this has been difficult, requiring extensive longitudinal studies incorporating visual surveys or trapping programs (Thompson, 2007; Amano et al., 2011; Camacho et al., 2017; Fox et al., 2018). Furthermore, some research methods for measuring these features, including trapping and other animal handling approaches, can have negative impacts upon study populations such as long-term behavioral changes (Kukalová et al., 2013; Camacho et al., 2017). Post-mortem study is also required for some characteristics, but this is rarely possible for practical or ethical reasons when threatened, endangered, or protected species are involved (Jarman et al., 2015). These considerations highlight the need for low impact alternatives.
Genomic tools provide an alternative, minimally invasive method for measurement of many population biology parameters (Figure 1). Epigenetic analyses, specifically through the analysis of DNA methylation (DNAm), are now allowing for an increased range of animal biological parameters to be studied (Bossdorf et al., 2008; De Paoli-Iseppi et al., 2017a; Herrel et al., 2020; Rey et al., 2020). DNAm analysis primarily involves the determination of percentage rates of methyl group presence at the C5 position of a Cytosine nucleotide adjacent to a Guanine nucleotide, commonly referred to as “CpG” sites (Hannum et al., 2013; Smith et al., 2015; Jin and Liu, 2018). CpG sites are often clustered into “islands” in 5′ regulatory regions of vertebrate genes and are involved in regulating gene expression through hypermethylation (increased methylation, generally decreased transcription) and hypomethylation (decreased methylation, generally increased transcription) within the regulatory region (Hannum et al., 2013). By assessing specific CpG sites, percentage rates of methylation can be determined throughout an animal’s life, and this information can be used in informing on a range of characteristics. DNAm analyses have been applied to the age estimation of wild animals (Polanowski et al., 2014); population differentiation, evolutionary drivers, and phenotypic variation (Liu et al., 2012; Caracappa et al., 2016; Baldanzi et al., 2017); mechanisms of sexual differentiation and developmental processes (Morán and Pérez-figueroa, 2011; Road et al., 2016); and for ecotoxicological purposes (Wang et al., 2009; Nilsen et al., 2016).
Figure 1. Examples of a genetic and epigenetic analyses of vertebrate population biology. Emerging epigenetic analyses hypothesized in this study are denoted with asterisks.
The successful use of DNAm analysis for measuring these population, evolutionary, and biological characteristics raises the possibility of its application to other life history traits (Figure 1). In this review, we identify recent advances within the discipline and important ecologically focused studies utilizing DNAm analysis across three key population biology parameters: age, sex, and attainment of sexual maturity. The potential applications of DNAm analysis are highlighted and key future foci identified for this new tool in the ecologist’s toolbox.
As an organism ages, many biological characteristics change, including size, behavior, and sexual maturity. These changes impact an individual’s ability to survive and reproduce effectively. This in turn affects the population as a whole, and population age structures can indicate past events and future growth potential (Jarman et al., 2015; Riekkola et al., 2018). Population age profiles can therefore act as an indicator of population decline or recovery (Lebreton et al., 1992; Riekkola et al., 2018). Historically, most age estimation methods required either post-mortem study, costly long-term visual surveys, or invasive capture and release strategies (Kukalová et al., 2013; Chevallier et al., 2017; Carroll et al., 2018). Molecular methods had been developed for human age estimation but were found to have limited accuracy or required certain sample types (Zubakov et al., 2010; De Paoli-Iseppi et al., 2017a). The ability to determine age using DNAm analysis of samples obtained from live wild animals offers a minimally invasive and accurate alternative.
Age estimation by DNAm analysis is a recent development, with assays first developed for humans (Bocklandt et al., 2011; Horvath, 2013). DNAm was initially shown to correlate with age in humans at promoters of three gene regions (Bocklandt et al., 2011). Subsequently, assays were developed to age a range of human and mouse tissues through methylation profiles of a set of CpG sites (Bocklandt et al., 2011; Koch et al., 2011; Horvath, 2013; Horvath and Raj, 2018). DNAm age estimators for wild animals were adapted and produced soon after, with the first being for the humpback whale (Megaptera novaeangliae; Polanowski et al., 2014). The humpback epigenetic age assay achieved a mean difference between predicted and actual age of 3.8 years (when assessing whales of known age), an estimation more accurate than alternative methods at the time. This model was then successfully applied to wild whale populations of indeterminate age (Polanowski et al., 2014; Riekkola et al., 2018). This highlighted the potential of DNAm analysis for accurate age estimation of wild species, and importantly for species where age estimation through other methodologies was practically or ethically difficult (Jarman et al., 2015).
Epigenetic age assays have several constraints that are not common in most genetic analyses. Due to DNAm variation between tissue types, a result of gene expression variation between tissues, only a single tissue type can generally be used for age estimators (Anastasiadi et al., 2018; Jung et al., 2019). Also, age associated CpG sites may vary between species, both in their location and strength of correlation (De Paoli-Iseppi et al., 2017b). While assays developed for one species have been applied to another, including a study on minke whales (Balaenoptera bonaerensis) utilizing the humpback assay, different CpG sites correlated with age than those identified for humpback whales (Tanabe et al., 2020). This indicates the potential need for complete re-identification of age associated CpG sites in each new species. Additionally, some tissue types, especially those easily and ethically collected such as feathers, may add additional complications or not be suitable for age estimation or other epigenetic analyses (De Paoli-Iseppi et al., 2017b). The initial development effort appears to be generally justified, however, with a variety of successful age estimation models developed for captive and model organisms (Anastasiadi and Piferrer, 2020; Lowe et al., 2020). It is now being rapidly adopted by the ecological field, with DNAm-based age associated studies in wild animals increasing steadily since the first in 2014 (Table 1).
While many successful DNAm age estimation assays have been developed for mammals, there has been limited research involving non-mammalian species. Only two groups have investigated DNAm and age relationships in wild birds. A study focused on the short-tailed shearwater (a seabird, Ardenna tenuirostris) found age-associated CpG sites identified in mammals were not conserved in birds, and an accurate age assay could not be generated using the selected markers (De Paoli-Iseppi et al., 2017b). Follow-up research, however, was able to successfully identify age-related genomic regions and develop the first epigenetic age estimation assay for birds (De Paoli-Iseppi et al., 2019). The other bird-focused study found DNAm relationships between age and heterozygosity in black grouse (Lyrurus tetrix), but their goal was not to develop an assay for age determination (Soulsbury et al., 2018). In reptiles, age negatively correlated with global DNAm in wild alligators (Alligator mississippiensis) in two studies, a trend related to the process of epigenetic drift, but age estimation was not the objective of either study (Parrott et al., 2014; Jones et al., 2015; Nilsen et al., 2016). Similarly, a recent study on lizards also identified relationships between increased age and global demethylation, but age determination once again was not attempted (Sargsyan et al., 2019). No DNAm-based age estimation studies on wild amphibians or fish have been completed to date. However, the first piscine epigenetic clock has been developed using captive seabass (Dicentrarchus labrax), with age estimation possible to within 2.15 years (Anastasiadi and Piferrer, 2020). These studies all highlight the efficacy of epigenetic age estimation across taxa groups, and the expansion of research into critical conservation species is recommended, especially for those taxa where traditional age estimation techniques are not appropriate.
Sex is one of the most significant traits affecting an individual animal’s phenotype. Population sex ratios are important in determining population growth rate potential and often reflect the effects of environmental pressures (Donald, 2007). However, sex is difficult to determine visually in many species (Shaw et al., 2003). This is exemplified by the many bird species that do not have external sexual characteristics (Griffiths and Tiwari, 1993). Genetic methods for determining bird sex by PCR testing for sex chromosomes have been developed to overcome this problem (Griffiths and Tiwari, 1993; Huynen et al., 2002). Similar tests for mammals have also been developed (Shaw et al., 2003; Kurose et al., 2005; Lindsay and Belant, 2008; Strah and Kunej, 2019). Many vertebrates, however, do not have chromosomally determined sex (Trukhina et al., 2013). Turtles undergo temperature-dependent sex determination, and several fish species can change sex throughout their lives (Allsop and West, 2003; Radhakrishnan et al., 2018; Bista and Valenzuela, 2020). This environmental sex determination involves large-scale epigenetic regulation of gene expression (Tachibana, 2016).
DNAm levels have been linked to sexual differentiation, maintenance, and age of sexual maturation (Morán and Pérez-figueroa, 2011; Wen et al., 2014; Domingos et al., 2018; Radhakrishnan et al., 2018). As sexual differentiation is facilitated by epigenetic mechanisms, DNAm analyses should theoretically be able to identify sex using biomarkers for sex specific DNAm variation. However, to date, no such assay has been developed, but research into epigenetic mechanisms of sex determination have demonstrated that it is possible (Matsumoto et al., 2013; Kuroki and Tachibana, 2018; Metzger and Schulte, 2018). For example, DNAm levels regulating the aromatase gene in turtles and fish are known to be altered by temperature, leading to either male or female gonadogenesis (Navarro-Martín et al., 2011; Matsumoto et al., 2013). By assessing methylation patterns within these genes, related sex-specific genes, and their promoter regions, markers for sex identification could be developed.
Most studies into epigenetic control of vertebrate sexual mechanisms and development are performed under controlled laboratory conditions (Wen et al., 2014; Laing et al., 2018; Ortega-Recalde et al., 2019). Recently, however, research has begun to also focus upon wild animal populations (Domingos et al., 2018; Martín-del-campo et al., 2018; Soulsbury et al., 2018). The first such study assessed Atlantic salmon (Salmo salar) and DNAm levels during maturation, finding high DNAm variation between two of three analyzed tissue types (gonads and brain, though not in liver) between maturation states (Morán and Pérez-figueroa, 2011). This suggested that early maturation was mostly mediated by epigenetic processes and not genetic variation. Contaminant impact on gonadal growth was assessed in the European eel (Anguilla anguilla), finding a potential role of DNAm in transcriptional regulation of a gene linked to gonadal development in female eels (Pierron et al., 2014). Similar correlations were found in the Japanese flounder (Paralichthys olivaceus) between DNAm levels and the sex-related genes dmrt1 and cyp19a, which are responsible for testes development and enzymes involved in estrogen synthesis (Wen et al., 2014). These links were supported by previous studies under experimental conditions (Navarro-Martín et al., 2011). A recent study also found that DNAm is a likely mechanism for natural sex maintenance, with DNAm suggested to be largely responsible for regulating expression of cyp19a in Chinese sea perch (Lateolabrax maculatus; Chen et al., 2018). Correlations have also been found between dmrt1 and cyp19a expression and DNAm, as well as sex-specific alternative splicing, within wild barramundi (Lates calcarifer) (Domingos et al., 2018). A whole genome approach in stickleback fish (Gasterosteus aculeatus) demonstrated hypermethylation of the X chromosome in females, suggesting DNAm was playing a role in suppressing X and Y chromosome recombination and possible sex-specific gene expression levels (Metzger and Schulte, 2018). Further research on zebrafish (Danio rerio) linked DNAm with both gonad transformation and identified that demethylation of female-linked genetic regions was critical in sex determination for that species (Ortega-Recalde et al., 2019). These studies all support the relationship between sex and DNAm variation, highlighting both the importance of epigenetic processes in sex determination and the future potential of DNAm biomarkers for sex identification. These biomarkers would be most beneficial for ecological assessments of animals that lack both phenotypic or genomic sex markers, such as turtle hatchlings and other reptile species, or as a value-adding tool where DNA methylation analyses are already being undertaken (Tezak et al., 2020).
Sexual maturity is reached when an organism can first successfully reproduce. The age that sexual maturity is reached varies greatly in vertebrates, both within and between species, and can be heavily influenced by a range of environmental factors, such as diet (Angelini and Ghiara, 1984; Ricklefs, 2010; Soliman et al., 2014). The age of first reproduction (AFR) is significant in determining the reproductive potential of a population (Angelini and Ghiara, 1984; Mourocq et al., 2016). The AFR can be used to determine the total fecundity of an individual by deducting the AFR from the total lifespan, or reproductive senescence in some mammals (Mourocq et al., 2016; Ellis et al., 2018). Determining the AFR is therefore key in understanding a species’ reproductive capability and thus an important consideration in conservation management strategies.
In humans, and many other vertebrates, the onset of puberty commonly involves the release of gonadotropin-releasing hormone (GnRH) and the activation of the hypothalamic-pituitary-gonadal axis, a set of endocrine glands which are critical to the functioning of the reproductive system. This leads to the production of sexual hormones and the transition into sexual maturity (Ojeda et al., 2010; Lomniczi et al., 2013; Rzeczkowska et al., 2014). Epigenetic controls have recently been identified as a key mechanism within this process (Lomniczi et al., 2013; Aylwin et al., 2019).
Sexual maturation in humans has been found to be mediated by epigenetic controls, with hypermethylation of key puberty-linked genes Eed and Cbx7 preceding puberty (Lomniczi et al., 2013). Inhibiting DNA methylation at these sites caused pubertal failure in rats. In rhesus monkeys (Macaca mulatta), increased methylation of the GnRH gene and increased levels of GnRH mRNA (messenger RNA) as puberty progressed has been demonstrated (Kurian and Terasawa, 2013). Similarly, dosing zebrafish with dexamethasone to imitate early life stress altered methylation in a GnRH gene promoter region, resulting in decreased spermatogenesis and reduced reproductive functions (Khor et al., 2016). More recently, conserved patterns of epigenetic controls in rat and goat sexual maturation have been indicated (Yang et al., 2018). Two genes linked with pubertal pathways, Edn3 and PTPRN2, showed similar increases in expression but significant variation in methylation patterns between species, indicating their potential role in the timing of species-specific puberty onset. In bovines, DNAm within sperm cells change between early pubertal and late pubertal states (Lambert et al., 2018). Bulls aged 10 months (early pubertal) were shown to have altered methylation profiles compared to sexually mature bulls. This potentially resulted in the value of early pubertal bull’s spermatozoa being compromised and lead to negative phenotypic traits due to altered epigenetic landscapes. This is a phenomenon initially identified in human studies (Kobayashi et al., 2009). Recent research has also identified varied DNAm patterns across the pubertal boundary, with clear differences in DNAm levels between pubertal states reported in pigs (Yuan et al., 2019). This indicates the potential presence of differentially methylated CpGs for the development of DNAm assays to assess sexual maturity.
Genetic tools are well established in conservation and ecological applications. This is due to their ability to generate large amounts of data from small tissue samples harvested from wild animals with minimal impact (Angeloni et al., 2012). Their widespread uptake has been further facilitated by the continued development of high throughput DNA sequencing platforms and related reductions in sequencing costs during the last decade (Schwarze et al., 2020). The importance of epigenetic processes in an ecological context is now also becoming clear (Parrott and Bertucci, 2019; Herrel et al., 2020). This is attributable to both the bridging role between environments and the individual that epigenetics fills, and its intrinsic links with many developmental mechanisms (Rey et al., 2020). The information contained within the methylome can help researchers understand many of these processes, as well as be utilized as a valuable biomarker for informing on individual characteristics. Epigenetic research has, to date, been largely restricted to human and model organism study. These analyses are now being extended to non-model and wild animal species. They can now be utilized to assess key population parameters which have previously been prohibitively difficult or impossible to determine.
A range of wild species have now had age estimation assays based upon DNAm markers successfully designed and applied (Polanowski et al., 2014; Wright et al., 2018; De Paoli-Iseppi et al., 2019). This has allowed for the determination of age structures within populations and as a tool for assessing population recovery (Riekkola et al., 2018). The development of DNAm-based age estimation assays for wild reptiles, amphibians, and fish, as well as further increasing those for mammals and birds, would further facilitate the assessment of this important biological trait in wild populations. This is especially true for threatened, endangered, or protected species where traditional methods are not viable. Age estimation assay development for key threatened species should therefore be a future focus, as should the attempted development of multi-species assays for more cost-effective applications of this tool.
While the utility of DNAm-based age estimators is evident, they have some limitations that should be considered when designing a research program. Firstly, there can be variation between chronological age and biological age of an individual (Horvath and Raj, 2018). This issue affects the questions that can be answered with a DNAm age assay, as in some cases biological age may be of more interest than chronological age (Horvath and Raj, 2018; Bell et al., 2019). Secondly, epigenetic relationships with animal age vary between tissue type (Illingworth et al., 2008; Jung et al., 2019). The second issue determines the sampling strategies that can be used, as many species cannot have all tissues sampled easily or ethically.
Epigenetic processes involved with both sexual maturity and sex determination in vertebrates have now been identified, opening the doors to a range of research prospects (Lomniczi et al., 2013; Rzeczkowska et al., 2014). The identification of sex specific DNAm patterns could be utilized to determine animal sex quickly and accurately. This is especially important in species where sex is not chromosomally determined and impossible to test for with current genomic tools or visually assess and should be a research priority. Additionally, continued research into DNAm processes involved with sexual maturity could allow for the development of assays able to determine the pubertal stage of animals. DNAm biomarkers as an indicator for species-specific sexual maturity would facilitate a greater understanding of this key parameter for population reproductive capability (Casale et al., 2011). Future research should focus upon the analysis of DNAm differentiation between prepubertal and sexually mature vertebrate taxa, with subsequent assays for maturity developed.
The next important steps are to continue to develop and assess these epigenetically focused assays for a greater range of vertebrate species and applications. A combined epigenetic toolkit would be able to rapidly identify three important parameters of population biology from one tissue sample. The age, sex, and pubertal stage could all be assessed. These could potentially be multiplexed into a singular assay and be complemented by conventional population genetic or kinship analyses (Figure 1). This would allow for a rapid, cost-effective, and in-depth assessment of a wild population with minimal sampling requirements. This toolkit would facilitate effective and informed management decisions, allowing for expanded biomonitoring options in a rapidly shifting and heavily impacted global biodiversity environment.
This review was conceptualized and designed by MH and SJ with MH, SJ, BS, and MB contributing to the writing and editing of this manuscript. All authors contributed to the article and approved the submitted version.
The authors declare that the research was conducted in the absence of any commercial or financial relationships that could be construed as a potential conflict of interest.
All claims expressed in this article are solely those of the authors and do not necessarily represent those of their affiliated organizations, or those of the publisher, the editors and the reviewers. Any product that may be evaluated in this article, or claim that may be made by its manufacturer, is not guaranteed or endorsed by the publisher.
We would like to acknowledge Curtin University and the Trace and Environmental DNA Laboratory for their support and assistance in accessing information relevant to this review. We give thanks as well to the BioRender team; Figure 1 was created with Biorender.com. We would also like to thank the members of the TrEnD Laboratory for their insights and constant support.
Allsop, D. J., and West, S. A. (2003). Constant relative age and size at sex change for sequentially hermaphroditic fish. J. Evol. Biol. 16, 921–929. doi: 10.1046/j.1420-9101.2003.00590.x
Amano, M., Yamada, T. K., Brownell, R. L., and Uni, Y. (2011). Age determination and reproductive traits of killer whales entrapped in ice off Aidomari, Hokkaido, Japan. J. Mammal. 92, 275–282. doi: 10.1644/10-MAMM-A-276.1
Anastasiadi, D., and Piferrer, F. (2020). A clockwork fish: age prediction using DNA methylation-based biomarkers in the European seabass. Mol. Ecol. Resour. 20, 387–397. doi: 10.1111/1755-0998.13111
Anastasiadi, D., Esteve-Codina, A., and Piferrer, F. (2018). Consistent inverse correlation between DNA methylation of the first intron and gene expression across tissues and species. Epigenetics Chromatin 11:37. doi: 10.1186/s13072-018-0205-1
Angelini, F., and Ghiara, G. (1984). Reproductive modes and strategies in vertebrate evolution. Boll. Zool. 51, 121–203. doi: 10.1080/11250008409439459
Angeloni, F., Wagemaker, N., Vergeer, P., and Ouborg, J. (2012). Genomic toolboxes for conservation biologists. Evol. Appl. 5, 130–143. doi: 10.1111/j.1752-4571.2011.00217.x
Aylwin, C. F., Toro, C. A., Shirtcliff, E., and Lomniczi, A. (2019). Emerging genetic and epigenetic mechanisms underlying pubertal maturation in adolescence. J. Res. Adolesc. 29, 54–79. doi: 10.1111/jora.12385
Baldanzi, S., Watson, R., McQuaid, C. D., Gouws, G., and Porri, F. (2017). Epigenetic variation among natural populations of the South African sandhopper Talorchestia capensis. Evol. Ecol. 31, 77–91. doi: 10.1007/s10682-016-9877-9
Beal, A. P., Kiszka, J. J., Wells, R. S., and Eirin-Lopez, J. M. (2019). The bottlenose dolphin epigenetic aging tool (BEAT): a molecular age estimation tool for small cetaceans. Front. Mar. Sci. 6:561. doi: 10.3389/fmars.2019.00561
Bell, C. G., Lowe, R., Adams, P. D., Baccarelli, A. A., Beck, S., Bell, J. T., et al. (2019). DNA methylation aging clocks: challenges and recommendations. Genome Biol. 20:249. doi: 10.1186/s13059-019-1824-y
Bista, B., and Valenzuela, N. (2020). Turtle insights into the evolution of the reptilian karyotype and the genomic architecture of sex determination. Genes 11, 1–16. doi: 10.3390/genes11040416
Bocklandt, S., Lin, W., Sehl, M. E., Sánchez, F. J., Sinsheimer, J. S., Horvath, S., et al. (2011). Epigenetic predictor of age. PLoS One 6:e14821. doi: 10.1371/journal.pone.0014821
Bors, E. K., Baker, C. S., Wade, P. R., O’Neill, K. B., Shelden, K. E., Thompson, M. J., et al. (2021). An epigenetic clock to estimate the age of living beluga whales. Evol. Appl. 14, 1263–1273. doi: 10.1111/eva.13195
Bossdorf, O., Richards, C. L., and Pigliucci, M. (2008). Epigenetics for ecologists. Ecol. Lett. 11, 106–115. doi: 10.1111/j.1461-0248.2007.01130.x
Camacho, C., Canal, D., and Potti, J. (2017). Lifelong effects of trapping experience lead to age-biased sampling: lessons from a wild bird population. Anim. Behav. 130, 133–139. doi: 10.1016/j.anbehav.2017.06.018
Caracappa, S., Pisciotta, A., Persichetti, M. F., Caracappa, G., Alduina, R., and Arculeo, M. (2016). Nonmodal scutes patterns in the Loggerhead Sea Turtle (Caretta caretta): a possible epigenetic effect? Can. J. Zool. 94, 379–383. doi: 10.1139/cjz-2015-0248
Carroll, E. L., Bruford, M. W., DeWoody, J. A., Leroy, G., Strand, A., Waits, L., et al. (2018). Genetic and genomic monitoring with minimally invasive sampling methods. Evol. Appl. 11, 1094–1119. doi: 10.1111/eva.12600
Casale, P., Mazaris, A., and Freggi, D. (2011). Estimation of age at maturity of loggerhead sea turtles Caretta caretta in the Mediterranean using length-frequency data. Endanger. Species Res. 13, 123–129. doi: 10.3354/esr00319
Chen, X., He, Y., Wang, Z., and Li, J. (2018). Expression and DNA methylation analysis of cyp19a1a in Chinese sea perch Lateolabrax maculatus. Comp. Biochem. Physiol. Part B Biochem. Mol. Biol. 226, 85–90. doi: 10.1016/j.cbpb.2018.07.008
Chevallier, C., Gauthier, G., and Berteaux, D. (2017). Age estimation of live arctic foxes Vulpes lagopus based on teeth condition. Wildlife Biol. 4:wlb.00304. doi: 10.2981/wlb.00304
Darimont, C. T., Fox, C. H., Bryan, H. M., and Reimchen, T. E. (2015). The unique ecology of human predators. Science 349, 858–860. doi: 10.1126/science.aac4249
De Paoli-Iseppi, R., Deagle, B. E., McMahon, C. R., Hindell, M. A., Dickinson, J. L., and Jarman, S. N. (2017a). Measuring animal age with dna methylation: from humans to wild animals. Front. Genet. 8:106. doi: 10.3389/fgene.2017.00106
De Paoli-Iseppi, R., Polanowski, A. M., McMahon, C., Deagle, B. E., Dickinson, J. L., Hindell, M. A., et al. (2017b). DNA methylation levels in candidate genes associated with chronological age in mammals are not conserved in a long-lived seabird. PLoS One 12:e0189181. doi: 10.1371/journal.pone.0189181
De Paoli-Iseppi, R., Deagle, B. E., Polanowski, A. M., McMahon, C. R., Dickinson, J. L., Hindell, M. A., et al. (2019). Age estimation in a long-lived seabird (Ardenna tenuirostris) using DNA methylation-based biomarkers. Mol. Ecol. Resour. 19, 411–425. doi: 10.1111/1755-0998.12981
Domingos, J. A., Budd, A. M., Banh, Q. Q., Goldsbury, J. A., Zenger, K. R., and Jerry, D. R. (2018). Sex-specific dmrt1 and cyp19a1 methylation and alternative splicing in gonads of the protandrous hermaphrodite barramundi. PLoS One 13:e0204182. doi: 10.1371/journal.pone.0204182
Donald, P. F. (2007). Adult sex ratios in wild bird populations. Ibis 149, 671–692. doi: 10.1111/j.1474-919X.2007.00724.x
Ellis, S., Franks, D. W., Nattrass, S., Cant, M. A., Bradley, D. L., Giles, D., et al. (2018). Postreproductive lifespans are rare in mammals. Ecol. Evol. 8, 2482–2494. doi: 10.1002/ece3.3856
Fox, C. J., Benjamins, S., Masden, E. A., and Miller, R. (2018). Challenges and opportunities in monitoring the impacts of tidal-stream energy devices on marine vertebrates. Renew. Sustain. Energy Rev. 81, 1926–1938. doi: 10.1016/j.rser.2017.06.004
Griffiths, R., and Tiwari, B. (1993). Primers for the differential amplification of the sex-determining region Y gene in a range of mammal species. Mol. Ecol. 2, 405–406. doi: 10.1111/j.1365-294X.1993.tb00034.x
Hammerschlag, N., and Gallagher, A. J. (2017). Extinction risk and conservation of the Earth’s national animal symbols. Bioscience 67, 744–749. doi: 10.1093/biosci/bix054
Hannum, G., Guinney, J., Zhao, L., Zhang, L., Hughes, G., Sadda, S., et al. (2013). Genome-wide methylation profiles reveal quantitative views of human aging rates. Mol. Cell 49, 359–367. doi: 10.1016/j.molcel.2012.10.016
Herrel, A., Joly, D., and Danchin, E. (2020). Epigenetics in ecology and evolution. Funct. Ecol. 34, 381–384. doi: 10.1111/1365-2435.13494
Hoffmann, M., Hilton-Taylor, C., Angulo, A., Böhm, M., Brooks, T. M., Butchart, S. H. M., et al. (2010). The impact of conservation on the status of the world’s vertebrates. Science 330, 1503–1509. doi: 10.1126/science.1194442
Hooper, D. U., Adair, E. C., Cardinale, B. J., Byrnes, J. E. K., Hungate, B. A., Matulich, K. L., et al. (2012). A global synthesis reveals biodiversity loss as a major driver of ecosystem change. Nature 486, 105–108. doi: 10.1038/nature11118
Horvath, S. (2013). DNA methylation age of human tissues and cell types. Genome Biol. 14:R115. doi: 10.1186/gb-2013-14-10-r115
Horvath, S., and Raj, K. (2018). DNA methylation-based biomarkers and the epigenetic clock theory of ageing. Nat. Rev. Genet. 19, 371–384. doi: 10.1038/s41576-018-0004-3
Huynen, L., Millar, C. D., and Lambert, D. M. (2002). A DNA test to sex ratite birds. Mol. Ecol. 11, 851–856. doi: 10.1046/j.1365-294x.2002.01483.x
Illingworth, R., Kerr, A., Desousa, D., Jørgensen, H., Ellis, P., Stalker, J., et al. (2008). A novel CpG island set identifies tissue-specific methylation at developmental gene loci. PLoS Biol. 6:e22. doi: 10.1371/journal.pbio.0060022
Ito, H., Udono, T., Hirata, S., and Inoue-Murayama, M. (2018). Estimation of chimpanzee age based on DNA methylation. Sci. Rep. 8:9998. doi: 10.1038/s41598-018-28318-9
Jarman, S. N., Polanowski, A. M., Faux, C. E., Robbins, J., De Paoli-Iseppi, R., Bravington, M., et al. (2015). Molecular biomarkers for chronological age in animal ecology. Mol. Ecol. 24, 4826–4847. doi: 10.1111/mec.13357
Jin, Z., and Liu, Y. (2018). DNA methylation in human diseases. Genes Dis. 5, 1–8. doi: 10.1016/j.gendis.2018.01.002
Jones, M. J., Goodman, S. J., and Kobor, M. S. (2015). DNA methylation and healthy human aging. Aging Cell 14, 924–932. doi: 10.1111/acel.12349
Jung, S. E., Lim, S. M., Hong, S. R., Lee, E. H., Shin, K. J., and Lee, H. Y. (2019). DNA methylation of the ELOVL2, FHL2, KLF14, C1orf132/MIR29B2C, and TRIM59 genes for age prediction from blood, saliva, and buccal swab samples. Forensic Sci. Int. Genet. 38, 1–8. doi: 10.1016/j.fsigen.2018.09.010
Khor, Y. M., Soga, T., and Parhar, I. S. (2016). Early-life stress changes expression of GnRH and kisspeptin genes and DNA methylation of GnRH3 promoter in the adult zebrafish brain. Gen. Comp. Endocrinol. 227, 84–93. doi: 10.1016/j.ygcen.2015.12.004
Kobayashi, H., Hiura, H., John, R. M., Sato, A., Otsu, E., Kobayashi, N., et al. (2009). DNA methylation errors at imprinted loci after assisted conception originate in the parental sperm. Eur. J. Hum. Genet. 17, 1582–1591. doi: 10.1038/ejhg.2009.68
Koch, C. M., Suschek, C. V., Lin, Q., Bork, S., Goergens, M., Joussen, S., et al. (2011). Specific age-associated DNA methylation changes in human dermal fibroblasts. PLoS One 6:e16679. doi: 10.1371/journal.pone.0016679
Kukalová, M., Gazárková, A., and Adamík, P. (2013). Should i stay or should i go? The influence of handling by researchers on den use in an arboreal nocturnal rodent. Ethology 119, 848–859.
Kurian, J. R., and Terasawa, E. (2013). Epigenetic control of gonadotropin releasing hormone neurons. Front. Endocrinol. 4:61. doi: 10.3389/fendo.2013.00061
Kuroki, S., and Tachibana, M. (2018). Epigenetic regulation of mammalian sex determination. Mol. Cell. Endocrinol. 468, 31–38. doi: 10.1016/j.mce.2017.12.006
Kurose, N., Masuda, R., and Tatara, M. (2005). Fecal DNA analysis for identifying species and sex of sympatric carnivores: a noninvasive method for conservation on the Tsushima Islands, Japan. J. Hered. 96, 688–697. doi: 10.1093/jhered/esi124
Laing, L. V., Viana, J., Dempster, E. L., Uren Webster, T. M., van Aerle, R., Mill, J., et al. (2018). Sex-specific transcription and DNA methylation profiles of reproductive and epigenetic associated genes in the gonads and livers of breeding zebrafish. Comp. Biochem. Physiol. 222, 16–25. doi: 10.1016/j.cbpa.2018.04.004
Lambert, S., Blondin, P., Vigneault, C., Labrecque, R., Dufort, I., and Sirard, M. A. (2018). Spermatozoa DNA methylation patterns differ due to peripubertal age in bulls. Theriogenology 106, 21–29. doi: 10.1016/j.theriogenology.2017.10.006
Lebreton, J. D., Burnham, K. P., Clobert, J., and Anderson, D. R. (1992). Modeling survival and testing biological hypotheses using marked animals: a unified approach with case studies. Ecol. Monogr. 62, 67–118. doi: 10.2307/2937171
Lindsay, A. R., and Belant, J. L. (2008). A simple and improved PCR-based technique for white-tailed deer (Odocoileus virginianus) sex identification. Conserv. Genet. 9, 443–447. doi: 10.1007/s10592-007-9326-y
Liu, S., Sun, K., Jiang, T., Ho, J. P., Liu, B., and Feng, J. (2012). Natural Epigenetic variation in the female great roundleaf bat (Hipposideros armiger) populations. Mol. Genet. Genomics 287, 643–650. doi: 10.1007/s00438-012-0704-x
Lomniczi, A., Loche, A., Castellano, J. M., Ronnekleiv, O. K., Bosch, M., Kaidar, G., et al. (2013). Epigenetic control of female puberty. Nat. Neurosci. 16, 281–289. doi: 10.1038/nn.3319
Lowe, R., Danson, A. F., Rakyan, V. K., Yildizoglu, S., Saldmann, F., Viltard, M., et al. (2020). DNA methylation clocks as a predictor for ageing and age estimation in naked mole-rats, Heterocephalus glaber. Aging 12, 4394–4406. doi: 10.18632/aging.102892
Martín-del-campo, R., Bárcenas-ibarra, A., Sifuentes-romero, I., Llera-Herrera, R., and García-Gasca, A. (2018). Methylation status of the putative Pax6 promoter in olive ridley sea turtle embryos with eye defects: an initial approach. Mech. Dev. 154, 287–295. doi: 10.1016/j.mod.2018.08.005
Matsumoto, Y., Buemio, A., Chu, R., Vafaee, M., and Crews, D. (2013). Epigenetic control of gonadal aromatase (cyp19a1) in temperature-dependent sex determination of red-eared slider turtles. PLoS One 8:e63599. doi: 10.1371/journal.pone.0063599
Maxwell, S. L., Fuller, R. A., Brooks, T. M., and Watson, J. E. M. (2016). Biodiversity: the ravages of guns, nets and bulldozers. Nature 536, 143–145. doi: 10.1038/536143a
McNab, B. K. (1980). Food habits, energetics, and the population biology of mammals. Am. Nat. 116, 106–124. doi: 10.1086/283614
McRae, L., Böhm, M., Deinet, S., Gill, M., and Collen, B. (2012). The Arctic species trend index: using vertebrate population trends to monitor the health of a rapidly changing ecosystem. Biodiversity 13, 144–156. doi: 10.1080/14888386.2012.705085
Metzger, D. C. H., and Schulte, P. M. (2018). The DNA methylation landscape of stickleback reveals patterns of sex chromosome evolution and effects of environmental salinity. Genome Biol. Evol. 10, 775–785. doi: 10.1093/gbe/evy034
Morán, P., and Pérez-figueroa, A. (2011). Methylation changes associated with early maturation stages in the Atlantic salmon. BMC Genet. 12:86. doi: 10.1186/1471-2156-12-86
Mourocq, E., Bize, P., Bouwhuis, S., Bradley, R., Charmantier, A., de la Cruz, C., et al. (2016). Life span and reproductive cost explain interspecific variation in the optimal onset of reproduction. Evolution 70, 296–313. doi: 10.1111/evo.12853
Nabi, G., McLaughlin, R. W., Hao, Y., Wang, K., Zeng, X., Khan, S., et al. (2018). The possible effects of anthropogenic acoustic pollution on marine mammals’ reproduction: an emerging threat to animal extinction. Environ. Sci. Pollut. Res. 25, 19338–19345. doi: 10.1007/s11356-018-2208-7
Navarro-Martín, L., Viñas, J., Ribas, L., Díaz, N., Gutiérrez, A., Di Croce, L., et al. (2011). DNA methylation of the gonadal aromatase (cyp19a) promoter is involved in temperature-dependent sex ratio shifts in the European sea bass. PLoS Genet. 7:e1002447. doi: 10.1371/journal.pgen.1002447
Nilsen, F. M., Parrott, B. B., Bowden, J. A., Kassim, B. L., Somerville, S. E., Bryan, T. A., et al. (2016). Global DNA methylation loss associated with mercury contamination and aging in the American alligator (Alligator mississippiensis). Sci. Total Environ. 545–546, 389–397. doi: 10.1016/j.scitotenv.2015.12.059
Ojeda, S. R., Lomniczi, A., Loche, A., Matagne, V., Kaidar, G., Sandau, U. S., et al. (2010). The transcriptional control of female puberty. Brain Res. 1364, 164–174. doi: 10.1016/j.brainres.2010.09.039
Ortega-Recalde, O., Day, R. C., Gemmell, N. J., and Hore, T. A. (2019). Zebrafish preserve global germline DNA methylation while sex-linked rDNA is amplified and demethylated during feminisation. Nat. Commun. 10:3053. doi: 10.1038/s41467-019-10894-7
Parrott, B. B., and Bertucci, E. M. (2019). Epigenetic aging clocks in ecology and evolution. Trends Ecol. Evol. 34, 767–770. doi: 10.1016/j.tree.2019.06.008
Parrott, B. B., Bowden, J. A., Kohno, S., Cloy-McCoy, J. A., Hale, M. D., Bangma, J. T., et al. (2014). Influence of tissue, age, and environmental quality on DNA methylation in Alligator mississippiensis. Reproduction 147, 503–513. doi: 10.1530/rep-13-0498
Pierron, F., Bureau du Colombier, S., Moffett, A., Caron, A., Peluhet, L., Daffe, G., et al. (2014). Abnormal ovarian DNA methylation programming during gonad maturation in wild contaminated fish. Environ. Sci. Technol. 48, 11688–11695. doi: 10.1021/es503712c
Polanowski, A. M., Robbins, J., Chandler, D., and Jarman, S. N. (2014). Epigenetic estimation of age in humpback whales. Mol. Ecol. Resour. 14, 976–987. doi: 10.1111/1755-0998.12247
Radhakrishnan, S., Literman, R., Neuwald, J. L., and Valenzuela, N. (2018). Thermal response of epigenetic genes informs turtle sex determination with and without sex chromosomes. Sex. Dev. 12, 308–319. doi: 10.1159/000492188
Reed, D. H., O’Grady, J. J., Brook, B. W., Ballou, J. D., and Frankham, R. (2003). Estimates of minimum viable population sizes for vertebrates and factors influencing those estimates. Biol. Conserv. 113, 23–34. doi: 10.1016/S0006-3207(02)00346-4
Rey, O., Eizaguirre, C., Angers, B., Baltazar-Soares, M., Sagonas, K., Prunier, J. G., et al. (2020). Linking epigenetics and biological conservation: towards a conservation epigenetics perspective. Funct. Ecol. 34, 414–427. doi: 10.1111/1365-2435.13429
Ricklefs, R. E. (2010). Life-history connections to rates of aging in terrestrial vertebrates. Proc. Natl. Acad. Sci. U.S.A. 107, 10314–10319. doi: 10.1073/pnas.1005862107
Riekkola, L., Zerbini, A. N., Andrews, O., Andrews-Goff, V., Baker, C. S., Chandler, D., et al. (2018). Application of a multi-disciplinary approach to reveal population structure and Southern Ocean feeding grounds of humpback whales. Ecol. Indic. 89, 455–465. doi: 10.1016/j.ecolind.2018.02.030
Road, U., Nagabhushana, A., and Mishra, R. K. (2016). Finding clues to the riddle of sex determination in zebrafish. J. Biosci. 41, 145–155. doi: 10.1007/s12038-016-9593-1
Rzeczkowska, P. A., Hou, H., Wilson, M. D., and Palmert, M. R. (2014). Epigenetics: a new player in the regulation of mammalian puberty. Neuroendocrinology 99, 139–155. doi: 10.1159/000362559
Sargsyan, A., Simonyan, A., Hovhannisyan, G., Arakelyan, M., and Aroutiounian, R. (2019). Application of the comet assay, micronucleus test and global DNA methylation analysis in Darevskia lizards as a sentinel organism for genotoxic monitoring of soil pollution. Mutat. Res. Genet. Toxicol. Environ. Mutagen. 842, 117–124. doi: 10.1016/j.mrgentox.2018.10.005
Schwarze, K., Buchanan, J., Fermont, J. M., Dreau, H., Tilley, M. W., Taylor, J. M., et al. (2020). The complete costs of genome sequencing: a microcosting study in cancer and rare diseases from a single center in the United Kingdom. Genet. Med. 22, 85–94. doi: 10.1038/s41436-019-0618-7
Shaw, C. N., Wilson, P. J., and White, B. N. (2003). A reliable molecular method of gender determination for mammals. J. Mammal. 84, 123–128. doi: 10.1644/1545-15422003084<0123:ARMMOG<2.0.CO;2
Smith, G., Smith, C., Kenny, J. G., Chaudhuri, R. R., and Ritchie, M. G. (2015). Genome-wide DNA methylation patterns in wild samples of two morphotypes of threespine stickleback (Gasterosteus aculeatus). Mol. Biol. Evol. 32, 888–895. doi: 10.1093/molbev/msu344
Soliman, A., De Sanctis, V., and Elalaily, R. (2014). Nutrition and pubertal development. Indian J. Endocrinol. Metab. 18(Suppl. 1), 39–47. doi: 10.4103/2230-8210.145073
Soulsbury, C. D., Lipponen, A., Wood, K., Mein, C. A., Hoffman, J. I., and Lebigre, C. (2018). Age- and quality-dependent DNA methylation correlate with melanin-based coloration in a wild bird. Ecol. Evol. 8, 6547–6557. doi: 10.1002/ece3.4132
Strah, R., and Kunej, T. (2019). Molecular sexing assays in 114 mammalian species: In silico sequence reanalysis and a unified graphical visualization of diagnostic tests. Ecol. Evol. 9, 5018–5028. doi: 10.1002/ece3.5093
Tachibana, M. (2016). Epigenetics of sex determination in mammals. Reprod. Med. Biol. 15, 59–67. doi: 10.1007/s12522-015-0223-7
Tanabe, A., Shimizu, R., Osawa, Y., Suzuki, M., Ito, S., Goto, M., et al. (2020). Age estimation by DNA methylation in the Antarctic minke whale. Fish. Sci. 86, 35–41. doi: 10.1007/s12562-019-01371-7
Tezak, B., Sifuentes-Romero, I., Milton, S., and Wyneken, J. (2020). Identifying sex of neonate turtles with temperature-dependent sex determination via small blood samples. Sci. Rep. 10:5012. doi: 10.1038/s41598-020-61984-2
Thomas, C. D., Cameron, A., Green, R. E., Bakkenes, M., Beaumont, L. J., Collingham, Y. C., et al. (2004). Extinction risk from climate change. Nature 427, 145–148. doi: 10.1038/nature02121
Thompson, G. G. (2007). Terrestrial vertebrate fauna surveys for the preparation of environmental impact assessments; how can we do it better? A Western Australian example. Environ. Impact Assess. Rev. 27, 41–61. doi: 10.1016/j.eiar.2006.08.001
Thompson, M. J., VonHoldt, B., Horvath, S., and Pellegrini, M. (2017). An epigenetic aging clock for dogs and wolves. Aging 9, 1055–1068. doi: 10.18632/aging.101211
Trukhina, A. V., Lukina, N. A., Wackerow-Kouzova, N. D., and Smirnov, A. F. (2013). The variety of vertebrate mechanisms of sex determination. Biomed Res. Int. 2013:587460. doi: 10.1155/2013/587460
Wang, Y., Wang, C., Zhang, J., Chen, Y., and Zuo, Z. (2009). DNA hypomethylation induced by tributyltin, triphenyltin, and a mixture of these in Sebastiscus marmoratus liver. Aquat. Toxicol. 95, 93–98. doi: 10.1016/j.aquatox.2009.06.008
Wen, A. Y., You, F., Sun, P., Li, J., Xu, D. D., Wu, Z. H., et al. (2014). CpG methylation of dmrt1 and cyp19a promoters in relation to their sexual dimorphic expression in the Japanese flounder Paralichthys olivaceus. J. Fish Biol. 84, 193–205. doi: 10.1111/jfb.12277
Wright, P. G. R., Mathews, F., Schofield, H., Morris, C., Burrage, J., Smith, A., et al. (2018). Application of a novel molecular method to age free-living wild Bechstein’s bats. Mol. Ecol. Resour. 18, 1374–1380. doi: 10.1111/1755-0998.12925
Yang, C., Ye, J., Liu, Y., Ding, J., Liu, H., Gao, X., et al. (2018). Methylation pattern variation between goats and rats during the onset of puberty. Reprod. Domest. Anim. 53, 793–800. doi: 10.1111/rda.13172
Yuan, X., Ye, S., Chen, Z., Pan, X., Huang, S., Li, Z., et al. (2019). Dynamic DNA methylation of ovaries during pubertal transition in gilts. BMC Genomics 20:510. doi: 10.1186/s12864-019-5884-x
Zabel, F., Delzeit, R., Schneider, J. M., Seppelt, R., Mauser, M., and Václavík, T. (2019). Global impacts of future cropland expansion and intensification on agricultural markets and biodiversity. Nat. Commun. 10:2844.
Keywords: population biology, sexual maturity, sex determination, epigenetics, DNA methylation, age estimation, conservation genetics, epigenetic assays
Citation: Heydenrych MJ, Saunders BJ, Bunce M and Jarman SN (2021) Epigenetic Measurement of Key Vertebrate Population Biology Parameters. Front. Ecol. Evol. 9:617376. doi: 10.3389/fevo.2021.617376
Received: 14 October 2020; Accepted: 29 October 2021;
Published: 19 November 2021.
Edited by:
Olivier Rey, Université de Perpignan Via Domitia, FranceReviewed by:
Jérôme Prunier, UMR 5321 Station d’Ecologie Théorique et Expérimentale (SETE), FranceCopyright © 2021 Heydenrych, Saunders, Bunce and Jarman. This is an open-access article distributed under the terms of the Creative Commons Attribution License (CC BY). The use, distribution or reproduction in other forums is permitted, provided the original author(s) and the copyright owner(s) are credited and that the original publication in this journal is cited, in accordance with accepted academic practice. No use, distribution or reproduction is permitted which does not comply with these terms.
*Correspondence: Matthew J. Heydenrych, bWF0dGhld2poZXlkZW5yeWNoQGdtYWlsLmNvbQ==
Disclaimer: All claims expressed in this article are solely those of the authors and do not necessarily represent those of their affiliated organizations, or those of the publisher, the editors and the reviewers. Any product that may be evaluated in this article or claim that may be made by its manufacturer is not guaranteed or endorsed by the publisher.
Research integrity at Frontiers
Learn more about the work of our research integrity team to safeguard the quality of each article we publish.