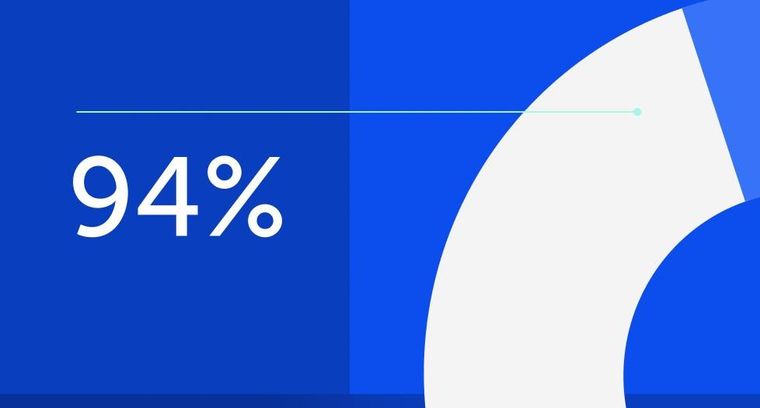
94% of researchers rate our articles as excellent or good
Learn more about the work of our research integrity team to safeguard the quality of each article we publish.
Find out more
ORIGINAL RESEARCH article
Front. Ecol. Evol., 01 June 2021
Sec. Biogeography and Macroecology
Volume 9 - 2021 | https://doi.org/10.3389/fevo.2021.615857
Interactions between cultivated and wild plants with their fungal pathogens have strong ecological, evolutionary and economic implications. Antagonistic interactions, however, have been scantily studied in an applied context by using ecological networks, phylogeny and spatial ecology concurrently. In this study, we describe for the first time, the topological structure of plant-fungi networks involving species of the genus Fusarium and their native and introduced (exotic) cultivated host plants in Mexico. For this, we based our study on a recent database describing the attack on 75 native and introduced plant species, including 35 species of the genus Fusarium. Host plant species varied in their degree of phylogenetical relatedness (Monocots and Dicots) and spatial geographical distribution. Therefore, we also tested whether or not plant-Fusarium networks are phylogenetically structured and highlighted the spatial correlation between pathogens and their host plants across the country. In general, the pathogen-plant network is more specialized and compartmentalized in closely related taxa. Closely related hosts are more likely to share the same pathogenic Fusarium species. Host plants are present in different ecosystems and climates, with regions having more cultivated plant species presenting the highest number of fusaria pathogens. From an economic standpoint, different species of the same taxonomic family may be more susceptible to being attacked by the same species of Fusarium, whereas from an ecological standpoint the movement of pathogens may expose wild and cultivated plants to new diseases. Our study highlights the relevance of interaction intimacy in structuring trophic relationships between plants and fusaria species in native and introduced species. Furthermore, we show that the analytical tools regarding host distribution and phylogeny could permit a rapid assessment of which plant species in a region are most likely to be attacked by a given fusaria.
Fungal pathogens are recognized as the major causal agents of plant diseases and comprise an important threat to wild and cultivated plant species worldwide (Burdon, 1991; Anderson et al., 2004; Benítez-Malvido, 2012). They represent an essential component of biodiversity in natural plant populations and communities and have strong socioeconomic implications in agroecosystems (Burdon, 1991; Desprez-Loustau et al., 2007, 2015). Several fungal species are known for their economic importance as they cause great losses to several agricultural crops and in forestry elsewhere (Anderson et al., 2004; Geiser et al., 2004, 2013). Some of those species belong to the filamentous ascomycete fungi genus Fusarium (Sordariomycetes: Hypocreales: Nectriaceae). The genus is cosmopolitan and includes pathogens that cause diseases in domesticated animals, humans and plants (Leslie and Summerell, 2006). Additionally, a considerable number of fusaria species are toxin-producing plant pathogens of agricultural and medical importance (Summerell et al., 2010). Disease symptoms in plants caused by Fusarium range from foliage blights, branch and stem cankers, floral and vegetative malformation, crown, root and stem rots, as well as vascular wilts (Ploetz, 2006; Ma et al., 2013). There are several fusaria species that cause diseases in staple food crops worldwide (Geiser et al., 2013; Ma et al., 2013), including the following: ear rot of maize (Desjardins et al., 2002), Fusarium head blight (O’Donnell et al., 2000), sudden death syndrome of soybeans (Aoki et al., 2005) and wilt diseases of several others (O’Donnell et al.,1998a,b; Skovgaard et al., 2001). Although pathogenic species of Fusarium have been found globally infecting plants in many horticultural, forest, and ornamental crops, taxa from this genus can be recovered from plants and soil as endophytes and saprobes (Wang et al., 2004; Ploetz, 2006; Summerell et al., 2010; Ma et al., 2013). In fact, recent studies with native plants and soil substrates in natural undisturbed ecosystems indicate that the number of non-pathogenic Fusarium species may be far greater than those causing diseases (Wang et al., 2004; Summerell and Leslie, 2011).
The number of species in the genus has varied from nine to more than 1,000 based on different studies conducted by specialists (Wollenweber and Reinking, 1935; Snyder and Hansen, 1945; Booth, 1971; Gerlach and Nirenberg, 1982; Nelson et al., 1983; Summerell and Leslie, 2011). Recently, Leslie and Summerell (2006) described in detail 70 Fusarium species; however, there are approximately 300 phylogenetically distinct species in 20 monophyletic Fusarium complexes that require further studies for assigning formal species names (Geiser et al., 2013; Ma et al., 2013; O’Donnell et al., 2013). Some fusaria species are native to a particular region and attack native/or local plants, e.g., Fusarium mexicanum, a pathogen of mango that has been found only in Mexico (Otero-Colina et al., 2010), whereas other species are cosmopolitan and attack native and introduced plant species in all regions of the world, e.g., Fusarium verticillioides, a pathogen of maize (Leslie and Summerell, 2006; Summerell et al., 2010).
Because of Fusarium worldwide distribution, the agricultural, economic and health importance of the genus, and of the continuous development of research databases, Fusarium species represent exceptionally rich organisms for studying pathogenic plant-fungal interactions in distinct natural and anthropogenic systems (Geiser et al., 2004, 2013; Park et al., 2010; O’Donnell et al., 2013). Approximately 80% of the economically important plants had at least one disease caused by Fusarium (Leslie and Summerell, 2006). Consequently and due to the high diversity of Fusarium species and of their plant hosts, different fungal and plant species can interact with each other which generates complex ecological networks within a given environment (Pires and Guimarães, 2013; Benítez-Malvido and Dáttilo, 2015).
In the last decades, some studies have found non-random structures in ecological networks (Bascompte et al., 2003; Bascompte and Jordano, 2007; Olesen et al., 2007). For instance, in modular (i.e., compartmentalized) networks there are subgroups of species that interact more frequently among themselves than with species from other subgroups in the network (Olesen et al., 2007). Over the years, studies have shown that antagonistic networks (i.e., one species benefits at the expense of another) tend to be more modular compared to mutualistic networks of free-living species because of a high level of specialization and dependence among species (Prado and Lewinsohn, 2004; Rezende et al., 2007, 2009; Benítez-Malvido and Dáttilo, 2015). Currently, however, the challenge ahead is to understand the proposed mechanisms underlying the modular pattern of these antagonistic networks.
It is broadly accepted that species are not independent entities within the natural environment and share an evolutionary history among them (Rezende et al., 2007; Bascompte and Jordano, 2013). This indicates that the current architecture of species interactions is not only dependent on recent ecological processes, but also on ancient evolutionary distances (Ives and Godfray, 2006; Bellay et al., 2011; Gilbert et al., 2012). In this sense, we know that both chemical and morphological traits of plants are often phylogenetically related (Farrell, 2001), and therefore, it is possible to expect that the architecture of antagonistic networks involving plants and pathogens can have a phylogenetic signal (Gilbert and Webb, 2007; Vacher et al., 2008). In other words, closely related host plants could more likely share pathogenic species with similar abilities to feed on their host plants (Gilbert and Webb, 2007; Vacher et al., 2008; Gilbert et al., 2012; Benítez-Malvido and Dáttilo, 2015). In addition to the phylogenetic signals, other ecological mechanisms can drive the organizational patterns in interaction networks, such as relative abundance, forbidden links, competition, spatial or temporal segregation, among others (reviewed by Bascompte and Jordano, 2013). Despite recent advances in our understanding of ecological networks, the organizational patterns and mechanisms structuring antagonistic networks involving cultivated and non-cultivated plant species and their pathogens is still poorly understood; see Benítez-Malvido and Dáttilo (2015), however.
Here we integrate the comprehensive information available in the literature on the Fusarium attacking different native and introduced cultivated plant species in Mexico (Fernández-Pavía et al., 2015), to determine characteristics of the community structure and the role of phylogenetic signals in plant-pathogen interactions (Gilbert et al., 2012). In addition, we used spatial ecology tools to determine if the distribution and number of host-Fusarium associations changes across the country (Turner et al., 2001; Wu and Hobbs, 2002). We postulated that if phylogeny imposes restrictions on plant-pathogen interactions, we expect to find subsets of species interacting more with one another within a larger group of species (i.e., modular pattern), leading to network architecture associated with the probability of sharing a pathogen decreases with increasing taxonomic distance between host plants.
Considering the virulence of several Fusarium species and the potential risk of infection of native and introduced crops across the country, the usage of phylogenetic relatedness and spatial ecology concurrently in the analysis of host-Fusarium interactions across Mexico, could improve the effectiveness of disease risk analysis. This approach permits a quick evaluation of which plant species (or crops) in a particular region of the country are most likely to interact with a particular fungal pathogen (see Gilbert et al., 2012). Increasing human impact on natural ecosystems, the accidental movement of pathogens together with their host plants, large-scale intensive agriculture and forestry and climate change have been associated with the emergence of new plant diseases elsewhere (Gilbert, 2002; Anderson et al., 2004; Desprez-Loustau et al., 2007, 2015). In this sense, the documentation of plant-Fusarium interactions and their distribution in Mexico, is essential considering its status as a biologically mega diverse country, which is the center of origin and/or diversification and domestication of several plant species, and considering that contemporary human activities are leading to global climate change and to the accelerated destruction of several natural ecosystems (Espejo-Serna et al., 2004; Espejo-Serna, 2012; Gernandt and Pérez-de la Rosa, 2014; Bufford et al., 2016).
The most current list of Fusarium pathogens in Mexico was used to evaluate the host range of 53 necrotrophic (tissue-killing) and biothrophic (relying on a living host) Fusarium species on a diversity of native and introduced plant species that differed in their degree of taxonomic relatedness, including dicots, monocots and gymnosperms (Fernández-Pavía et al., 2015; Table 1). Currently, a total 198 seed plant species have been recorded of being attacked by the genus Fusarium across the country, including the generic names based on sexual stages linked to Fusarium (e.g., Albonectria, Gibberella, Haematonectria, Nectria, and Neocosmospora (Rossman, 1996; Kvas et al., 2009; Table 1). From this list, only 75 plant species, morpho species and varieties in which the species of fusaria are named, were further considered in our study (Cruz-Miranda, 2011; Gómez-Gómez, 2016; SAGARPA, 2017; Table 1).
Table 1. List of different native and introduced cultivated plant species attacked by species of the genus Fusarium in Mexico.
After a careful depuration of the data set, we firstly mapped the spatial distribution of 75 plant-Fusarium interactions in mainland Mexico (Figure 1). We conducted spatial autocorrelation tests in ArcMap to see if the patterns of the variables pathogen richness and plant richness are clustered or follow a different spatial trend (e.g., random or dispersed). We also conducted a geographically weighted linear regression to look at the dependency of fusaria richness on plant richness. Secondly, because the data set included one taxonomic family of gymnoperms (Pinaceae) with 21 species all of which are infected only by Fusarium circinatum and therefore, to avoid any biases in the phylogenetic and ecological network analyses we focused only on flowering plants. For the construction of plant-Fusarium networks, we considered only the 35 plant species, morphospecies and varieties in which the identity of Fusarium species has been described in detail. In the other 163 plant species, the taxonomic identity of the Fusarium species is not yet known and/or is still in debate. All of those fusarial species included in our study are listed in some of the main fungi world data sets (Leslie and Summerell, 2006; MycoBank http://www.mycobank.org/quicksearch.aspx; Species Fungorum http://www.speciesfungorum.org/Names/Names.asp). For the network and phylogenetic analysis we included 13 native species to the country and 22 introduced species with well described fusaria species. The fusaria infecting the native and introduced flora are diverse, including plant species from all continents and climates which could have lead to novel potential interactions (Table 1).
Figure 1. The spatial distribution and richness of (A) fusaria pathogenic species; and (B) host-plant species present throughout mainland Mexico (see Table 1 and Supplementary Material).
Initially, the Fusarium-plant antagonistic interactions were defined as an adjacency matrix A, in which Aij represents an interaction between the Fusarium species i and the plant species j (Bascompte et al., 2003). We then constructed a bipartite network with the full set of species with the bipartite package (Dormann et al., 2009) in the R software version 3.3.3 (R Development Core Team, 2016). Afterwards, we also constructed one network for native plant species and a second for introduced species interacting with Fusarium. Thus, in these networks, Fusarium and plant species are depicted as nodes, and their interactions are represented by links describing the use of plant species by fusaria species. For each bipartite network we calculted the following descriptors (Dormann et al., 2009; Blüthgen, 2010): (1) network size was defined as the total richness (plant and fusaria species); (2) connectance (C) as the fraction of recorded interactions relative to the total possible interactions; (3) nestedness (NODF) describes the topological pattern related with how interactions are established, it assigns a number between 1 and 0 considering if the majority of the specialist species interact exclusively with more generalist species that also interact with them in return or if there is no such pattern; (4) number of links per species; (5) number of compartments: these are network subgroups that are not connected to another subgroup, related to (6) the modularity (M) that measures the level at which the species tend to be organized in subgroups of species that interact more frequently among themselves than with other members of the network.
In addition, we also calculated the niche overlap (NO) using the Morisita–Horn index (Horn, 1966) defined as follows: where, pij = corresponds to the proportion of species i used by species j and pik = corresponds to the proportion of species i of the total resource pool used by species k. Note that in this case i and j can be either plant or fungi, and therefore, we have the niche overlap (or niche partitioning) for both Fusarium and the hosts. The Morisita-Horn’s index varies from 0, when there is no overlap of resources used, to 1, when there is complete resource overlap.
Similarly, robustness was calculated to evaluate vulnerability to extinction in the Fusarium species in the different networks. This descriptor refers to the area below the extinction curve created by the fraction of species that survive following the removal of a given species, calculated as the exponent a of the function y = 1−xa.
Additionally, we tested for differences in the number of species of fusaria between native and introduced cultivated plants using a t-test. Finally, we used a Spearman correlation to test if the states of Mexico with a higher number of species of cultivated plants corresponded to states with more species of fusaria pathogens. Both t-tests and correlation were performed in the R software version 3.3.3 (R Development Core Team, 2016).
To test if the degree of phylogenetic relatedness among host plant species influences host-pathogen interactions we followed the next steps. Firstly, we built a phylogenetic tree including angiosperms species being attacked by identified fusaria species across Mexico (Figure 2). For the tree construction, we used as a backbone the megaphylogeny generated at the species level by Zanne et al. (2014), which is based on sequences for seven-gene regions available in the GenBank1 and fossil data. This phylogeny includes 98.6 and 51.6% of the families and genera, respectively, of all extant seed plants in the world. We specifically used the updated and expanded version of the Zanne et al. (2014) phylogeny provided by Qian and Jin (2016), which is the largest and most up-to-date time-calibrated species-level phylogeny of seed plants. At the end, we obtained an ultrametric tree (Figure 2) with branch lengths in units of time (millions of years). The used taxonomic designation of species following “The Plant List2.”
Figure 2. Chronogram containing Monocots and Dicots species attacked by fusaria species. Branch lengths represent millions of years. Only host plants attacked by fusaria species with known identity were considered in the analysis.
Secondly, we built two distance matrices, one considering the phylogenetic distance among host species (evolutionary distance matrix), calculated from the phylogenetic tree, and the other one considering the degree of similarity between host species in term of the pathogens that attack them (pathogen similarity matrix). The last matrix was built considering the Jaccard similarity index, calculated using an incidence dataset. Then, we tested for the correlation between the evolutionary distance matrix and the pathogen similarity matrix through a Mantel tests. The significance of the correlation (Spearman’s rank correlation, rS) was computed through 999 permutations, considering an alpha = 0.05 level.
Thirdly, we used the non-Metric Multidimensional Scaling (MDS) ordination for mapping the relationships among host species captured in the pathogen similarity matrix. During this process, we calculated and used the stress value to evaluate how properly the resulting ordination reflects the distance among the host species showed in the pathogen similarity matrix (McCune and Grace, 2002). Then, through the “envfit” function implemented in the vegan R package (Oksanen et al., 2013), we tested for differences between Monocots and Dicots species in terms of the identity of pathogens attacking them. The “envfit” function estimates the centroids corresponding to each group of species in the ordination space and evaluates if the observed distance among centroids is greater than expected by chance –significance testing (P ≤ 0.05) based on 999 permutation.
Host specificity varied among Fusarium species. The number of Fusarium species infecting a particular plant species ranged from one to 15 pathogenic fungi. Most species of plants (59% of all species considered) were attacked by one identified species of Fusarium, whereas only a few were attacked by more than three species concurrently, including maize (Zea mays) with 15 fusaria species, followed by black bean (Phaseolus vulgaris) with ten species, carnation (Dianthus caryophyllus) with six, and mahogany (Swetenia macrophylla) with five fusaria species. The fusaria species with the largest number of plant hosts were Fusarium solani, infecting 15 hosts, and F. verticillioides and F. proliferatus with six hosts each. Furthermore, 52% of fusaria species had only one host plant species which suggests a strong host specificity. No significant differences, however, were detected in the number of species of pathogens infecting native (mean ± SD: 3.14 ± 4.2) and introduced (1.76 ± 1.2) plant species (t = 2.58; df = 33; P = 0.12). Overall, of the 31 fusaria species eleven (34%) infect native and introduced plants concurrently, 13 (41%) were found exclusively in native hosts (F. andiyazi, F. chlamydosporum, F. crookwellense, F. heterosporum, F. lateritium, F. napiforme, F. nygamai, F. poae, F. pseudonygamai, F. sporotrichioides, F. subglutinans, F. temperatum) while eight (25%) only in introduced plant species (F. brachygibbosum, F. fujikuroi, F. globosum, F. meridionale, F. sacchari, F. semitectum. F. thapsinum and F. tricinctum).
In relation to the spatial distribution of antagonistic interactions between fusaria pathogens and their host plants in Mexico (Figure 1), Michoacán was the state with most species of Fusarium (n = 35), followed by the states of Sinaloa (n = 30) and Puebla (n = 28, mean ± SD per state: 10.84 ± 9.23) (Figure 1A). Furthermore, F. oxysporum and F. solani were the pathogen species more widely distributed in the country (n = 28 states, which corresponds to 87% of all states). In addition, Michoacán and Guanajuato were the states with more species of cultivated plants reported with diseases caused by Fusarium: 22 and 16 species, respectively (mean ± SD per state: 6.51 ± 6.06) (Table 1; Figure 1B). Bean (Phaseolus vulgaris) and sugarcane (Saccharum officinarum) were the plant species more widely cultivated in Mexico in terms of locations (bean in 22 states and sugarcane in 12 states, which corresponds to 68 and 37.5% of all states, respectively). The states with more cultivated plant species had a higher number of fusaria species (r = 0.65; P < 0.001). Furthermore, the spatial autocorrelation tests showed that fusaria and plant richness are spatially clustered (fusaria richness Moran’s I = 0.134, z-score = 1.936, P = 0.053; plant richness Moran’s I = 0.125, z-score = 1.89, P = 0.059), and there is less than a 6% likelihood that the observed pattern could be the result of random chance. The geographically weighted linear regression suggests that there is a strong association between fusaria richness and plant richness: R2 = 0.696995, R2Adjusted = 0.671155.
The interaction network of Fusarium and their plants showed a nested arragement. When comparing native and exotic plant-Fusarium networks, Fusarium species established a more nested network with native than with exotic plant species, even when the native and the exotic networks are of similar size (Table 2). In both networks F. solani is the species with the highest number of interactions.
Table 2. Attributes of plant-Fusarium ecological networks in Mexico, including the following: all plant species, native plant species only and introduces plant species only.
In the complete network we identified four modules (groups) of Fusarium species strongly associated with a particular set of plant species, while the native species network has two compartments and the exotic species network seven modules (Figure 3). The size of the modules varied significantly from small modules with very specialized interactions between one plant species and one pathogen species to modules with more than 10 plant species and more than 10 pathogens (Figure 3). Moreover, we found a remarkable difference in the niche overlap between Fusarium species and plant species, considering native and exotic plants. Native plants tended to share more pathogens among themselves (NO = 0.34) than pathogens sharing their host plants (NO = 0.12), while exotic plants share less Fusarium species (NO = 0.08) than the pathogens associated with them (NO = 0.19). In addition, we observed that interactions among Fusarium species and plant hosts were influenced by host taxonomic distance (Mantel r = 0.49; P < 0.001). In other words, taxonomically close host plants tended to share the same pathogenic species. In terms of robustness, the complete network, considering exotic and native plants, showed a similar value than native or exotic-Fusarium networks on their own.
Figure 3. Quantitative bipartite plot of the plant-Fusarium species ecological network for all plant species infected with known fusaria species, including the following: native plant species and introduced plant species to Mexico. The bar size represents the number of interactions each fusaria species (blue) had with each individual native (green) and introduced plant species (orange), and the linkage width represents the proportion of fusaria species involved in such interactions.
We found a significant correlation between the evolutionary distance matrix and the pathogen similarity matrix (rS = 0.219; P = 0.001), pointing out that taxonomically close host species tend to share pathogen species (Figure 2). This result was also consistently obtained (rS = 0.216; P = 0.001) even after removing from the analysis the species (Musa sp., Saccharum officinarum and Citrullus lanatus) that differ more strongly from the rest in terms of their fusaria community, that can be potentially influencing the correlation analysis in a similar way that the outliers.
We also found that the centroids corresponding to the Monocots and Dicots species in the ordination space (Figure 4) were more distant from one another than expected by chance (“envfit” test, r2 = 0.189; P = 0.006), indicating that Monocots and Dicots species tend to be affected by different pathogens. We considered only two axes for the MDS ordination, because additional dimensions did not substantially diminish the resulting stress value (3.558), which according to the Kruskal and Clarke’s rule of thumb corresponds to an ordination with no real risk of drawing false inferences (McCune and Grace, 2002).
Figure 4. Non-Metric Multidimesional Scaling ordination mapping host species dissimilarities in terms of their pathogenic fusaria species. The squares show the centroids corresponding the distribution of Monocots and Dicot species. Species: Agave tequilana (agateq), Allium cepa (allcep), Allium sativum (allsat), Asparagus officinalis (aspoff), Bursera sp. (bursp), Capsicum annuum (capann), Carica papaya (carpap), Cascabela thevetia (casthe), Chrysanthemum grandiflorum (chrgra), Dianthus caryophyllus (diacar), Dioscorea sp. (diosp), Fragaria ananassa (fraana), Gladiolus grandiflorus (glagra), Gladiolus sp. (glasp), Gossypium hirsutum (goshir), Hibiscus sabdariffa (hibsab), Hordeum vulgare (horvul), Mangifera indica (manind), Oryza sativa (orysat), Panicum maximum (panmax), Phaseolus vulgaris (phavul), Senna sp. (sensp), Solanum tuberosum (soltub), Sorghum bicolor (sorbic), Sorghum sp. (sorsp), Swietenia macrophylla (swimac), Tabebuia sp. (tabsp), Theobroma cacao (thecac), Triticum aestivum (triaes), Vicia faba (vicfab), xTriticosecale sp. (xtrsp), Zea may (zeamay). The enlisted plants included species, morpho species of known genera (e.g., Bursera sp., Senna sp., and Tabebuia sp.) and cultivated varieties (Gladiolus sp. and Sorghum sp.). Only fusaria species with known identity were considered in the analysis.
Results from this study indicated various important aspects in the plant-Fusarium ecological networks. Firstly, these networks tended to be specialized and compartmentalized (i.e., modular topology). Secondly, taxonomically close host plants tended to be affected by the same pathogenic fusaria species. Thirdly, fusaria species are spread throughout Mexico’s mainland occupying different ecosystems and climates. Finally, two states located in the central western region of Mexico had the highest number of cultivated plant species and fusaria species. We found that plant-Fusarium interactions are rich in the numer of species for both plants and pathogens. We also showed that in Mexico species of the genus Fusarium are ecologically diverse and affect economically important native and introduced crops. The high number of cultivated exotic plants suggests that a considerable number of fusaria species might have been introduced with their host plants to the country (Bufford et al., 2016). Our findings, however, are incomplete as many other plant species recorded as being infected by Fusarium (118 species) were not included in the analysis because the identity of the pathogen species involved have not yet been described.
The spatial analysis showed that not all plants and fusaria species are distributed everywhere; however, except for Quintana Roo, every other state presented at least one species of Fusarium listed as infecting a plant host (Figure 1). The spatial pattern of plant-fusaria interactions across Mexico could be the consequence of a complex interplay of natural and human driven factors including the following, local climate, land-use practices, agricultural intensity, differences in sampling efforts across the country, among others. Natural environmental conditions and human activities interact to create the spatial patterns in the distribution of plant-fusaria interactions observed in the country.
The spatial distribution of fusaria-plant interactions across Mexico showed a significant positive relationship with the number of introduced plant species. In other words, the greater the number of non-native cultivated plants, the greater the number of fusaria species. The plant and fusaria species in this study have different biogeographical origins, and because of human activities they are spread all across the Mexican mainland. Mexico has been recognized as the centre of origin, diversification and domestication of some taxonomic plant families (e.g., Pinaceae and Agavaceae) and species (e.g., maize, Zea mays; beans, Phaseolus vulgaris; and squash Cucurbita pepo, etc.), considering the broad distribution of fusaria diseases infecting native and introduced plant species and crops, special attention should be given to plant species endemic to Mexico and to those used as staple food crops (Espejo-Serna et al., 2004; Espejo-Serna, 2012; Gernandt and Pérez-de la Rosa, 2014).
Overall, it has been estimated that Mexico holds about 11% of all known plant seed species worldwide (Espejo-Serna et al., 2004). Humans and global climate change have facilitated the introduction, proliferation and severity of plant diseases (Fraser, 2004; Qu et al., 2008; Benítez-Malvido, 2012; Bufford et al., 2016). This is of particular concern for many endemic plant species in Mexico present in ecosystems with high rates of land-use change and transformation as these actions affect the probability of the establishment of introduced pathogens and the distribution and severity of diseases in different tropical and temperate ecosystems (Shaw and Osborne, 2011; Sturrock et al., 2011; Benítez-Malvido, 2012). Several plant species have important cultural and economic connotations including maize and several species of Agavaceae. For instance, around 40% of all Liliopsida that have been described in Mexico are endemic to the country (including Vanilla planifolia and several Agavaceae) (Gernandt and Pérez-de la Rosa, 2014).
An evolutionary ecological perspective provides insights for the understanding of plant-pathogen interactions in a dynamic world and can provide the knowledge for sustainable management of natural, agricultural and forestry systems (Gilbert and Webb, 2007; Gilbert et al., 2012; Desprez-Loustau et al., 2015). The coupling of ecology and evolutionary biology has become an important tool for understanding the origin and proliferation of plant diseases as well as a way to slow disease emergence and severity when possible (Gilbert, 2002; Gilbert and Webb, 2007; Gilbert et al., 2012; Varki, 2012; Vander Wal et al., 2014; Desprez-Loustau et al., 2015). The plant-Fusarium ecological networks in Mexico are shaped by the coevolutionary history of the interacting species (see Desprez-Loustau et al., 2007, 2015; Rezende et al., 2007; Bufford et al., 2016). In some cases, clusters reflected closely related species as in the Fabaceae. The existence of such similarities implies that besides ecological and human factors evolutionary history also explains the patterns of interactions between fusaria species and their plant hosts in Mexico. From commercial, agricultural, forestry and conservation standpoints, this means that different species of the same plant taxonomic Family may be more susceptible to being attacked by the same species of Fusarium in a given area (Gilbert and Webb, 2007; Gilbert et al., 2012; Benítez-Malvido and Dáttilo, 2015). In fact, it has been found that phylogenetic relatedness rather than direct contact, explains many of the emerging associations between native and exotic plant and pathogen species (Bufford et al., 2016).
Evolution of pathogen populations can occur in short time scales, especially in ecosystems under strong anthropogenic influence (Palumbi, 2001; Burdon and Thrall, 2008; Qu et al., 2008; Benítez-Malvido, 2012; Jousimo et al., 2014). By comparison, long-lived tree species and other plants are expected to adapt more slowly (Carroll et al., 2014). Some plant species in this study have been introduced and cultivated in Mexico for several decades (e.g., sugar cane, wheat, mango, etc.), and probably their pathogens have already adapted to the environment where they were introduced together with their hosts. This adaptation might explain the lack of significant difference in the number of fusarial species infecting native and exotic plant species despite that the number of fusaria species exclusively infecting introduced plant hosts doubled those infecting native hosts. There is evidence that human activities have intensified the rates of genetic change in both pathogen populations and plant populations, and therefore an evolutionary ecological perspective for disease management needs to be continuously developed (Desprez-Loustau et al., 2007, 2015; Gilbert and Webb, 2007; Soto-Plancarte, 2013; Carroll et al., 2014; Bufford et al., 2016; Santillán-Mendoza et al., 2018). In fact, host species with related pattern of interactions could be more susceptible to the same stochastic events of environmental disturbance (Bascompte and Jordano, 2013).
In general, natural and/or wild plant populations rarely suffer from devastating fungal epidemics contrary to what has been observed in several native and introduced cultivated species (Hansen and Goheen, 2000; Jousimo et al., 2014). The natural regulation of diseases, however, can be hindered by exotic pathogens (Bufford et al., 2016). Pathogens are capable of adapting to new hosts after introductions or becoming more virulent in homogeneous plantations and/or crops (Soto-Plancarte, 2013). This is because frequently individuals of the same species with low genetic variation are grown close together in very large numbers (Vurro et al., 2010). A fungal pathogen may disperse rapidly and effectively under these conditions as postulated by the resource concentration hypothesis (Root, 1973). Therefore, intercropping is recommended over monocultures.
Disease epidemics, in particular those caused by introduced pathogens may cause dramatic, permanent changes in plant populations, forest community structure, species composition and consequently in the ecosystem functioning (Hansen and Goheen, 2000; Rizzo et al., 2002; Weste et al., 2002; Laidlaw and Wilson, 2003; Weste, 2003). For fusaria species host switches may have already been observed from wild to cultivated plants in Mexico (Soto-Plancarte, 2013; Bufford et al., 2016). For instance, two species of Fusarium have been detected and described in mango (Mangifera indica L.) cultivars throughout Mexico (Otero-Colina et al., 2010; Rodríguez-Alvarado et al., 2013; Soto-Plancarte, 2013). One such pathogens is F. mexicanum that seems to be endemic to Mexico since it has not been detected in other mango growing areas outside this country. This pathogen was placed in the American clade according to a biographic clade hypothesis for Fusarium species belonging to the Fusarium fujikuroi species complex proposed by O’Donnell et al. (1998a, b) and based on a phylogenetic analysis using five gene regions (Otero-Colina et al., 2010). A major assumption of this clade hypothesis is that there is a close association between the origin of the fusaria species and the origin of its host plant (O’Donnell et al.,1998a,b); however, some researchers have provided data that support and contradict this hypothesis (Kvas et al., 2009; Summerell et al., 2010). Mango trees were introduced to Mexico from the Philippines by the Spaniards around the end of the 1700s (Iyer and Schnell, 2009). Considering that mango is an introduced species in this country and that F. mexicanum has not been yet detected elsewhere, it is possible that spores (microconidia) of F. mexicanum were disseminated by wind or insect vectors from its original hosts to susceptible mango trees in plantations. That the original host or hosts for F. mexicanum could be native plants is shown by the recent finding of this pathogen in association with herbaceous, native plants of the genus Senna sp. and with trees of Switenia macrophylla (Meliaceae) located near commercial mango orchards and in suburban settings (Soto-Plancarte, 2013; Rodríguez-Alvarado, unpublished data). On the other hand, a native plant species from Mexico, Ipomea batatas, has been found to be infected by F. denticulatum in Africa; however, this plant is not reported to be a host of this fusaria in Mexico (O’Donnell et al.,1998a,b). Overall, these emerging associations between native and exotic plants and pathogens can have a negative impact on managed and natural terrestrial ecosystems (Benítez-Malvido, 2012; Bufford et al., 2016).
Host density but also identity is important for disease development and transmission. The more susceptible the plant hosts, the faster and more widespread will be the proliferation of a given disease (Beldomenico and Begon, 2009). Because the striking differences in the management, usages and commercialization of the plant hosts known to be infected by Fusarium in Mexico, disease control techniques should be implemented accordingly. For instance, the Pinaceae are used for timber, other species are used as ornamental plants, and some other plants are considered as staple foods or have industrial purposes. In addition, some native species from the Agavaceae with economic importance (Agave atrovirens, A. fourcroydes, A. sisalana, A. tequilana) are infected by unidentified fusaria species, which prevented us from including them in our analysis. The same occurred for other important native crops with cultural and economic connotations such as tomato (Solanum lycopersicum), chilli pepper (Capsicum annuum) and trees like the cypress (Taxodium mucronatum).
Fungal pathogens, including Fusarium, represent essential components of biodiversity in natural ecosystems and have strong socio-economic implications in agroecosystems. The ecological costs of disease outbreaks constitute the loss of species and populations as well as ecosystem degradation (Hansen and Goheen, 2000; Rizzo et al., 2002; Weste et al., 2002; Laidlaw and Wilson, 2003; Weste, 2003; Anderson et al., 2004; Vurro et al., 2010). Increasing human impact on forests and other natural systems, including the unintentional movement of pathogens, plant trade, global climate change, and large-scale intensive plantations, has been associated with an unprecedented emergence of new diseases (Carroll et al., 2014). An evolutionary and spatial ecological perspective can help address these significant challenges and provide direction for sustainable management of natural and agricultural systems (Qu et al., 2008; Bufford et al., 2016). It is essential to understand fusaria disease dynamics, the identity of all fusaria pathogens that have been recorded in different plants and in different habitats, the life histories of plant hosts and the effects of the local environmental conditions on them (Benítez-Malvido, 2012). This is the first report of the distribution and diversity of plant-Fusarium interactions throughout Mexico.
The datasets generated for this study are available on request to the corresponding author.
JB-M conceived the idea, spearheaded the writing, and edited and did literature review. GR-A provided the data, spearheaded the writing, and did literature review. MÁ-A, LÁ-C, Ed-V, and AL-N performed the data analysis, contributed to the writing, and created the figures. RG-C provided the data and did literature review. All authors secured the financial contribution. All authors contributed to the article and approved the submitted version.
The authors declare that the research was conducted in the absence of any commercial or financial relationships that could be construed as a potential conflict of interest.
We appreciate the financial and logistic support from Universidad Nacional Autónoma de México (Projects PAPIIT IN-214014, IN-202117, and IN201620 to JB-M). We are grateful to J. M. Lobato-García for technical assistance.
The Supplementary Material for this article can be found online at: https://www.frontiersin.org/articles/10.3389/fevo.2021.615857/full#supplementary-material
Anderson, P. K., Cunningham, A. A., Patel, N. G., Morales, F. J., Epstein, P. R., and Daszak, P. (2004). Emerging infectious diseases of plants: pathogen pollution, climate change and agrotechnology drivers. Trends Ecol. Evol. 19, 535–544. doi: 10.1016/j.tree.2004.07.021
Aoki, T., O’Donnell, K., and Scandiani, M. M. (2005). Sudden death syndrome of soybean in South America is caused by four species of Fusarium: Fusarium brasiliense sp. nov., F. cuneirostrum sp. nov., F. tucumaniae, and F. virguliforme. Mycoscience 46, 162–183. doi: 10.1007/s10267-005-0235-y
Bascompte, J., and Jordano, P. (2007). Plant-animal mutualistic networks: the architecture of biodiversity. Annu. Rev. Ecol. Evol. Syst. 38, 567–593. doi: 10.1146/annurev.ecolsys.38.091206.095818
Bascompte, J., and Jordano, P. (2013). Mutualistic Networks. Princeton, NJ: Princeton University Press, 224.
Bascompte, J., Jordano, P., Melian, C. J., and Olesen, J. (2003). The nested assembly of plant-animal mutualistic networks. Proc. Natl. Acad. Sci. U.S.A. 100, 9383–9387. doi: 10.1073/pnas.1633576100
Beldomenico, P. M., and Begon, M. (2009). Disease spread, susceptibility and infection intensity: vicious circles? Trends Ecol. Evol. 25, 22–27.
Bellay, S., Lima, D. P., Takemoto, R. M., and Luque, J. L. (2011). A host-endoparasite network of Neotropical marine fish: are there organizational patterns? Parasitology 138, 1945–1952. doi: 10.1017/s0031182011001314
Benítez-Malvido, J. (2012). “Fungal diseases Neotropical forests disturbed by humans,” in Conservation Medicine: Applied Cases of Ecological Health, eds A. Aguirre, P. Daszak, and R. S. Ostfeld (Oxford: Oxford University Press), 302–311.
Benítez-Malvido, J., and Dáttilo, W. (2015). Interaction intimacy of pathogens and herbivores with their plant hosts determines the topological structure of ecological networks in different ways. Am. J. Bot. 102, 1–8.
Blüthgen, N. (2010). Why network analysis is often disconnected from community ecology: a critique and an ecologist’s guide. Basic Appl. Ecol. 11:185195.
Bufford, J. L., Hulme, P. E., Sikes, B. A., Cooper, J. A., Johnston, P. R., and Duncan, R. P. (2016). Taxonomic similarity, more than contact opportunity, explains novel plant – pathogen associations between native and alien taxa. New Phytol. 212, 657–667. doi: 10.1111/nph.14077
Burdon, J. J. (1991). Fungal pathogens as selective forces in plant populations and communities. Austr. J. Ecol. 16, 423–432. doi: 10.1111/j.1442-9993.1991.tb01072.x
Burdon, J. J., and Thrall, P. H. (2008). Pathogen evolution across the agroecological interface: implications for disease management. Evol. Appl. 1, 57–65. doi: 10.1111/j.1752-4571.2007.00005.x
Carroll, S. P., Jørgensen, P. S., Kinnison, M. T., Bergstrom, C. T., Denison, R. F., Gluckman, P., et al. (2014). Applying evolutionary biology to address global challenges. Science 80, 1–16. doi: 10.1355/9789812306203-001
Cruz-Miranda, O. L. (2011). Efecto de Extractos Botánicos y Quitosano Sobre el Control de la Pudrición Café en Cormos y Plantas de Gladiolo, Cultivadas en Invernadero. Tesis de Maestría. Mexico: Instituto Politécnico Nacional.
Desjardins, A. E., Munkvold, G. P., Plattner, R. D., and Proctor, R. H. (2002). FUM1: A gene required for fumonisin biosynthesis but not for maize ear rot and ear infection by Gibberella moniliformis in field tests. Mol. Plant Microbe Interac. 15, 1157–1164. doi: 10.1094/mpmi.2002.15.11.1157
Desprez-Loustau, M. R., Aguayo, J., Dutech, C., Hayden, K. J., Husson, C., Jakushkin, B., et al. (2015). An evolutionary ecology perspective to address forest pathology challenges of today and tomorrow. Ann. Forest Sci. 73, 45–67. doi: 10.1007/s13595-015-0487-4
Desprez-Loustau, M. R., Robin, C., Buée, M., Courtecuisse, R., Garbaye, J., Suffert, F., et al. (2007). The fungal dimension of biological invasions. Trends Ecol. Evol. 22, 472–480. doi: 10.1016/j.tree.2007.04.005
Dormann, C. F., Fründ, J., Blüthgen, N., and Gruber, B. (2009). Indices, graphs and null models: analyzing bipartite ecological networks. Open Ecol. 2, 7–24. doi: 10.2174/1874213000902010007
Espejo-Serna, A. (2012). El endemismo en las Liliopsida mexicanas. Acta Botan. Mex. 100, 195–257. doi: 10.21829/abm100.2012.36
Espejo-Serna, A., López-Ferrari, A. R., and Salgado Ugarte, I. (2004). A current estimate of angiosperm diversity in Mexico. Taxon 53, 127–130. doi: 10.2307/4135497
Farrell, B. D. (2001). Evolutionary assembly of the milkweed fauna: cytochrome oxidase i and the age of tetraopes beetles. Mol. Phylogenet. Evol. 18, 467–478. doi: 10.1006/mpev.2000.0888
Fernández-Pavía, S. P., Gregorio-Cipriano, R., Rodríguez-Alvarado, G., Fernández-Pavía, Y. L., Mondragón-Flores, A., Gómez-Dorantes, N., et al. (2015). Enfermedades de Especies Vegetales en Mexico. Morelia: Universidad Michoacana de San Nicolás de Hidalgo, 425.
Fraser, J. (2004). “Climate change impacts on biological systems,” in Proceedings of the Species at risk. Pathways to Recovery Conference, ed. T. D. Hooper (Victoria, BC: Conference Organizing Committee), 1–7.
Geiser, D. M., Aoki, T., Bacon, C. W., Baker, S. E., Bhattacharyya, M. K., Brandt, M. E., et al. (2013). One fungus, one name: defining the genus Fusarium in a scientifically robust way that preserves longstanding use. Phytopathology 103, 400–408. doi: 10.1094/phyto-07-12-0150-le
Geiser, D. M., del Mar Jiménez-Gasco, M., Kang, S., Makalowska, I., Veeraraghavan, N., Ward, T. J., et al. (2004). FUSARIUM-ID v. 1.0: a DNA sequence database for identifying Fusarium. Eur. J. Plant Pathol. 110, 473–479. doi: 10.1007/978-1-4020-2285-2_2
Gerlach, W., and Nirenberg, H. (1982). The Genus Fusarium a Pictorial Atlas. Mitteilungen aus der Biologischen Bundesanstalt fur Land-und Forstwirtschaft. Germany: Berlin-Dahlem.
Gernandt, D. S., and Pérez-de la Rosa, J. A. (2014). Biodiversidad de Pinophyta (coníferas) en México 2. Rev. Mex. Biodiver. 85(Suppl.), S126–S133.
Gilbert, G. S. (2002). Evolutionary ecology of plant diseases in natural ecosystems. Annu. Rev. Phytopathol. 40, 13–43.
Gilbert, G. S., and Webb, C. O. (2007). Phylogenetic signal in plant pathogen–host range. Proc. Natl. Acad. Sci. U.S.A. 104, 4979–4983. doi: 10.1073/pnas.0607968104
Gilbert, G. S., Magarey, R., Suiter, K., and Webb, C. O. (2012). Evolutionary tools for phytosanitary risk analysis: phylogenetic signal as a predictor of host range of plant pests and pathogens. Evolut. Appl. 5, 869–878. doi: 10.1111/j.1752-4571.2012.00265.x
Gómez-Gómez, A. A. (2016). “Producción y comercio internacional de la Gladiola mexicana. Producción y comercio internacional de la Gladiola mexicana,” in Producción, Comercialización y Medio Ambiente, eds F. Rérez, E. Figueroa, and L. Godínez (Texcoco: © ECORFAN), 152–164.
Hansen, E. M., and Goheen, E. M. (2000). Phellinus weirii and other native root pathogens as determinants of forest structure and process in western North America. Annu. Rev. Phytopathol. 38, 515–539. doi: 10.1146/annurev.phyto.38.1.515
Horn, H. S. (1966). Measurement of “overlap” in comparative ecological studies. Am. Natural. 100, 419–424. doi: 10.1086/282436
Ives, A. R., and Godfray, H. C. J. (2006). Phylogenetic analysis of trophic associations. Am. Natur. 168, E1–E14.
Iyer, C. P. A., and Schnell, R. J. (2009). “Breeding and genetics,” in The Mango: Botany, Production and Uses, 2 Edn, ed. R. E. Litz (Wallingford: CAB International).
Jousimo, J., Tack, A. J. M., Ovaskainen, O., Mononen, T., Susi, H., Tollenaere, C., et al. (2014). Ecological and evolutionary effects of fragmentation on infectious disease dynamics. Science 344, 1289–1293. doi: 10.1126/science.1253621
Kvas, M., Marasas, W. F. O., Wingfield, B. D., Wingfield, M. J., and Steenkamp, E. T. (2009). Diversity and evolution of Fusarium species in the Gibberella fujikuroi complex. Fungal Divers. 1–21. Available online at: http://hdl.handle.net/2263/13444
Laidlaw, W. S., and Wilson, B. A. (2003). Floristic and structural characteristics of a coastal health and exhibiting symptoms of Phytophtora cinamomi infestation in the eastern Otway Ranges, Victoria. Austr. J. Bot. 51, 283–293. doi: 10.1071/bt02100
Leslie, J. F., and Summerell, B. A. (2006). The Fusarium Laboratory Manual. Iowa: Blackwell Publishing, 388.
Ma, L. J., Geiser, D. M., Proctor, R. H., Rooney, A. P., O’Donnell, K., Trail, F., et al. (2013). Fusarium pathogenomics. Annu. Rev. Microbiol. 67, 399–416.
Nelson, P. E., Toussoun, T. A., and Marasas, W. F. O. (1983). Fusarium Species: An Illustrated Manual for Identification. University Park, PA: Pennsylvania State University Press.
O’Donnell, K., Cigelnik, E., and Nirenberg, H. I. (1998a). Molecular systematics and phylogeograpgy of the Gibberella fujikuroi species complex. Mycologia 90, 465–493. doi: 10.2307/3761407
O’Donnell, K., Deanna, A., Sutton, M. G., Rinaldi, B. A. J., Sarver, S., Arunmozhi, B., et al. (2010). Internet-accessible DNA sequence database for identifying fusaria from human and animal infections. J. Clin. Microbiol. 48, 3708–3718. doi: 10.1128/jcm.00989-10
O’Donnell, K., Kistler, H. C., Cigelnik, E., and Ploetz, R. C. (1998b). Multiple evolutionary origins of the fungus causing Panama disease of banana: concordant evidence from nuclear and mitochondrial gene genealogies. Proc. Natl. Acad. Sci. U.S.A. 95, 2044–2049. doi: 10.1073/pnas.95.5.2044
O’Donnell, K., Kistler, H. C., Tacke, B. K., and Casper, H. H. (2000). Gene genealogies reveal global phylogeographic structure and reproductive isolation among lineages of Fusarium graminearum, the fungus causing wheat scab. Proc. Natl. Acad. Sci. U.S.A. 97, 7905–7910. doi: 10.1073/pnas.130193297
O’Donnell, K., Rooney, A. P., Proctor, R. H., Brown, D. W., McCormick, S. P., Ward, T. J., et al. (2013). Phylogeneticanalyses of RPB1 and RPB2 support a middle Cretaceous origin for a clade comprising all agriculturally and medically important fusaria. Fungal Genet. Biol. 52, 20–31. doi: 10.1016/j.fgb.2012.12.004
Oksanen, J., Blanchet, F. G., Kindt, R., Legendre, P., Minchi, P. R., O’Hara, R. B., et al. (2013). vegan: Community Ecology Package. R package Version 2.0-6.
Olesen, J. M., Bascompte, J., Dupont, Y. L., and Jordano, P. (2007). The modularity of pollination networks. Proc. Natl. Acad. Sci. U.S.A. 104, 19891–19896. doi: 10.1073/pnas.0706375104
Otero-Colina, G., Rodríguez-Alvarado, G., Fernández-Pavía, S. P., Maymon, M., Ploetz, R. C., Aoki, T., et al. (2010). Identification and characterization of a novel etiological agent of mango malformation disease in Mexico, Fusarium mexicanum sp. nov. Phytopathology 11, 1176–1184. doi: 10.1094/phyto-01-10-0029
Palumbi, S. R. (2001). Humans as the world’s greatest evolutionary force. Science 293, 1786–1790. doi: 10.1126/science.293.5536.1786
Park, B., Park, J., Cheong, K.-C., Choi, J., Jung, K., Kim, D., et al. (2010). Cyber infrastructure for Fusarium: three integrated platforms supporting strain identification, phylogenetics, comparative genomics, and knowledge sharing. Nucleotid Acids Res. 39, D640–D646.
Pires, M. M., and Guimarães, P. R. (2013). Interaction intimacy organizes networks of antagonistic interactions in different ways. J. R. Soc. Interface 10:20120649. doi: 10.1098/rsif.2012.0649
Ploetz, R. C. (2006). Fusarium-induced diseases of tropical, perennial crops. Phytopathology 96, 648–652. doi: 10.1094/phyto-96-0648
Prado, P. I., and Lewinsohn, T. M. (2004). Compartments in insect-plant associations and their consequences for community structure. J. Anim. Ecol. 73, 1168–1178. doi: 10.1111/j.0021-8790.2004.00891.x
Qian, H., and Jin, Y. (2016). An updated megaphylogeny of plants, a tool for generating plant phylogenies and an analysis of phylogenetic community structure. J. Plant Ecol. 9, 233–239. doi: 10.1093/jpe/rtv047
Qu, B., Lia, H. P., Zhanga, J. B., Xua, Y. B., Huanga, T., Wua, A. B., et al. (2008). Geographic distribution and genetic diversity of Fusarium graminearum and F. asiaticum on wheat spikes throughout China. Plant Pathol. 57, 15–24.
R Development Core Team (2016). R: A Language and Environment for Statistical Computing. Vienna: R Foundation for Statistical Computing.
Rezende, E. L., Albert, E., Fortuna, M. A., and Bascompte, J. (2009). Compartments in a marine food web associated with phylogeny, body mass, and habitat structure. Ecol. Lett. 12, 779–788. doi: 10.1111/j.1461-0248.2009.01327.x
Rezende, E., Lavabre, J. E., Guimaraes, P. R., Jordano, P., and Bascompte, J. (2007). Non-random coextinctions in phylogenetically structured mutualistic networks. Nature 448, 925–928. doi: 10.1038/nature05956
Rizzo, D. M., Garbelotto, M., Davidson, J. M., Salaughter, G. W., and Koike, S. T. (2002). Phytophtora ramorum as the cause of extensive mortality of Querqus ssp. and Lothocarpus densiflorus in California. Plant Dis. 86, 205–214.
Rodríguez-Alvarado, G., Fernández-Pavía, S., Otero-Colina, G., Ploetz, R. C., Aoki, T., O’Donnell, K., et al. (2013). Identification and characterization of Fusarium mexicanum causing mango malformation disease in México. Acta Horticult. 992, 337–384.
Root, R. B. (1973). Organization of a plant-arthropod association in simple and diverse habitats: the fauna of collards (Brassica oleracea). Ecol. Monogr. 43, 95–124. doi: 10.2307/1942161
Rossman, A. Y. (1996). Morphological and molecular perspectives on systematics of the hypocreales. Mycologia 88, 1–19. doi: 10.2307/3760780
SAGARPA (2017). Planeación Agrícola Nacional 2017-2030. Sorgo: Grano Mexicana. Secretaría de Agricultura, Ganadería, Desarrollo Rural, Pesca y Alimentación. Mexico: SAGARPA.
Santillán-Mendoza, R., Fernández-Pavía, S. P., O’Donnell, K., Ploetz, R. C., Ortega-Arreola, R., Vázquez-Marrufo, G., et al. (2018). A novel disease of big-leaf mahogany caused by two Fusarium species in Mexico. Plant Dis. 102, 1965–1972. doi: 10.1094/pdis-01-18-0060-re
Shaw, M. W., and Osborne, T. M. (2011). Geographic distribution of plant pathogens in response to climate change. Plant Pathol. 60, 31–43. doi: 10.1111/j.1365-3059.2010.02407.x
Skovgaard, K., Nirenberg, H. I., O’Donnell, K., and Rosendahl, S. (2001). Evolution of Fusarium oxysporum f. sp. vasinfectum races inferred from multigene genealogies. Phytopathology 91, 1231–1237. doi: 10.1094/phyto.2001.91.12.1231
Snyder, W. C., and Hansen, H. N. (1945). The species concept in Fusarium with reference to Discolor and other sections. Am. J. Bot. 32, 657–666. doi: 10.1002/j.1537-2197.1945.tb05172.x
Soto-Plancarte, A. (2013). Fuentes de Inóculo de Especies de Fusarium Causantes de la Malformación del Mango (Mangifera indica L.). Tesis de Maestría. Morelia: Universidad Michoacana de San Nicolás de Hidalgo.
Sturrock, R. N., Frankel, S. J., Brown, A. V., Hennon, P. E., Kliejunas, J. T., Lewis, K. J., et al. (2011). Climate change and forest diseases. Plant Pathol. 60, 133–149.
Summerell, B. A., and Leslie, J. F. (2011). Fifty years of Fusarium: how could nine species have ever been enough? Fung. Diver. 50, 135–144. doi: 10.1007/s13225-011-0132-y
Summerell, B. A., Laurence, M. H., Liew, E. C., and Leslie, J. F. (2010). Biogeography and phylogeography of Fusarium: a review. Fung. Diver. 44, 3–13. doi: 10.1007/s13225-010-0060-2
Turner, M. G., Gardner, R. H., and O’Neill, R. V. (2001). Landscape Ecology in Theory and Practice: Pattern and Process. New York, NY: Springer-Verlag.
Vacher, C., Piou, D., and Desprez-Loustau, M. L. (2008). Architecture of an antagonistic tree/fungus network: the asymmetric influence of past evolutionary history. PLoS One 3:e1740. doi: 10.1371/journal.pone.0001740
Vander Wal, E., Garant, D., Calmé, S., Chapman, C., Festa-Bianchet, M., Millien, V., et al. (2014). Applying evolutionary concepts to wildlife disease ecology and management. Evol. Appl. 7, 856–868. doi: 10.1111/eva.12168
Varki, A. (2012). Nothing in medicine makes sense, except in the light of evolution. J. Mol. Med. 90, 481–494. doi: 10.1007/s00109-012-0900-5
Vurro, M., Bonciani, B., and Vannacci, G. (2010). Emerging infectious diseases of crop plants in developing countries: impact on agriculture and socio-economic consequences. Food Secur. 2, 113–132. doi: 10.1007/s12571-010-0062-7
Wang, B., Brubaker, C. L., and Burdon, J. J. (2004). Fusarium species and Fusarium wilt pathogens associated with native Gossypium populations in Australia. Mycol. Res. 108, 35–44. doi: 10.1017/s0953756203008803
Weste, G. (2003). Th e dieback cycle in Victorian forests: a 30-year study of changes caused by Phytophtora cinnamoni in Victorian open forests, woodlands and heathlands. Austr. Plant Pathol. 32, 247–256. doi: 10.1071/ap03013
Weste, G., Brown, K., Kennedy, J., and Walshe, T. (2002). Phytophtora cinnamoni infestation - a 24-year study of vegetation change in forests and woodlands of the Grampians, Western Victoria. Austr. J. Bot. 50, 247–274. doi: 10.1071/bt01073
Wollenweber, H. W., and Reinking, O. A. (1935). Die Fusarien, ihre Beschrei- Bung, Schadwirkung, und Bekampfung. Berlin: Verlag Paul Parey.
Wu, J., and Hobbs, R. (2002). Key issues and research priorities in landscape ecology: an idiosyncratic synthesis. Lands. Ecol. 17, 355–365.
Keywords: ecological networks, exotic species, Fusarium, plant-pathogen interactions, phylogenetically structured networks, spatial distribution
Citation: Benítez-Malvido J, Rodríguez-Alvarado G, Álvarez-Añorve M, Ávila-Cabadilla LD, del-Val E, Lira-Noriega A and Gregorio-Cipriano R (2021) Antagonistic Interactions Between Fusaria Species and Their Host Plants Are Influenced by Host Taxonomic Distance: A Case Study From Mexico. Front. Ecol. Evol. 9:615857. doi: 10.3389/fevo.2021.615857
Received: 21 December 2020; Accepted: 19 April 2021;
Published: 01 June 2021.
Edited by:
Shan Huang, Senckenberg Biodiversity and Climate Research Centre, GermanyReviewed by:
Dominik Merges, Centre for Translational Biodiversity Genomics (LOEWE-TBG), GermanyCopyright © 2021 Benítez-Malvido, Rodríguez-Alvarado, Álvarez-Añorve, Ávila-Cabadilla, del-Val, Lira-Noriega and Gregorio-Cipriano. This is an open-access article distributed under the terms of the Creative Commons Attribution License (CC BY). The use, distribution or reproduction in other forums is permitted, provided the original author(s) and the copyright owner(s) are credited and that the original publication in this journal is cited, in accordance with accepted academic practice. No use, distribution or reproduction is permitted which does not comply with these terms.
*Correspondence: Julieta Benítez-Malvido, amJlbml0ZXpAY2llY28udW5hbS5teA==
Disclaimer: All claims expressed in this article are solely those of the authors and do not necessarily represent those of their affiliated organizations, or those of the publisher, the editors and the reviewers. Any product that may be evaluated in this article or claim that may be made by its manufacturer is not guaranteed or endorsed by the publisher.
Research integrity at Frontiers
Learn more about the work of our research integrity team to safeguard the quality of each article we publish.