- 1Institute of Zoology, Slovak Academy of Sciences, Bratislava, Slovakia
- 2Department of Interdisciplinary Life Sciences, Konrad Lorenz Institute of Ethology, University of Veterinary Medicine, Vienna, Austria
Bacteria are known to exert positive and negative influences on animals’ health and fitness. Bacteria, in particular those inhabiting the skin and inner organs of vertebrates, are horizontally or vertically transmitted. Specifically, mothers of bird species can transfer bacterial strains to their offspring when the egg is passing the reproductive tract, as the eggshell rubs against the wall of the uterus. In this context, the female immune system might play an important role in influencing the vertical transmission of bacteria. Here, we investigate the relationship between the major histocompatibility complex (MHC) and cultivable eggshell bacteria originating putatively from the female urogenital tract in a captive population of house sparrows (Passer domesticus). We predict that females with a more variable MHC will transfer fewer bacteria onto the eggshells. Our results show a negative relationship between the number of functional MHC class I alleles and bacteria originating in the urinary tract and growing on a selective medium. This is the first study to find a correlation between female MHC diversity and eggshell bacteria.
Introduction
Animal health is significantly influenced by bacteria (Org et al., 2015). The bacteria an individual harbors can be due to (i) horizontal transmission, which means they are spread from host to host in the same generation; or (ii) vertical transmission, which means they are spread between generations, namely from parents to offspring; or (iii) the host bacteria can originate from the environment.
Bacterial communities can help during digestion, e.g., extracting energy and nutritional compounds (Hooper and Gordon, 2001; Ley et al., 2008), producing vitamins (Hill, 1997), or teaching and training the host immune system (Cebra, 1999; Macpherson and Harris, 2004). However, bacteria can also have a negative effect; for instance, in birds, eggshell bacteria may penetrate the egg and cause problems during embryo development and subsequent survival (Pinowski et al., 1994; D’Alba et al., 2010). Benign or pathogenic bacteria, which are commonly found in the reproductive system, are known to be vertically transmitted to the host’s offspring (Ruiz-de-Castañeda et al., 2011a; Tangkham et al., 2016; You et al., 2019).
In birds, early contact of offspring with bacteria occurs in the ovary and the oviduct of the mother. This happens either before oviposition, through direct contamination of the yolk, albumen, or eggshell membranes (Timoney et al., 1989; Keller et al., 1995; Okamura et al., 2001a,b), or during oviposition, when the egg is squeezed through the gastrointestinal system of the mother (De Reu et al., 2006; Messens et al., 2007). Contact with bacteria also occurs after oviposition, with bacteria from the surrounding environment, e.g., airborne bacteria (Kulkarni and Heeb, 2007; Goodenough and Stallwood, 2012), or bacteria from the nest material or the parent surface (skin and feather bacteria).
Several studies have reported a significant variation in eggshell bacterial composition and abundance between individuals, as well as species (Soler et al., 2011; Peralta-Sánchez et al., 2012, 2018; Schmitt et al., 2017), whereby between-species variation in eggshell bacteria is likely to have been influenced by environmental conditions. In this context, ambient temperature and humidity are well-known parameters influencing breeding conditions and, consequently, the eggshell bacterial community (Park et al., 2015; Leclaire et al., 2019). In contrast, within-species variation in eggshell bacteria is more likely to be related to individual quality, given that environmental conditions should be more similar within than between species. In particular, the immune system of an individual should influence its bacterial composition (Soler et al., 2011; Schmitt et al., 2017). Therefore, we predict a relationship between eggshell bacteria loads and the immunocompetence of a bird mother.
In this context, one important element of the genome responsible for immune system functioning is the major histocompatibility complex (MHC). The MHC is a highly polymorphic gene region, which encodes proteins that present both self and foreign peptides to T cells, which are central for an appropriate adaptive immune response against pathogens (Rock et al., 2016; Goebel et al., 2017). Amino acids—in particular, polymorphic peptide-binding regions (PBR) of the MHC molecules—determine which antigens can be bound and are therefore crucial for the function of each allele (Schwensow et al., 2007). The general assumption is that individuals with a broad antigen-binding repertoire can recognize and eradicate a wider range of pathogens and therefore have fitness advantages over individuals with a narrow MHC repertoire (Penn et al., 2002). A broad antigen-binding repertoire is, however, not always beneficial; in house sparrows (Passer domesticus), a negative effect on hatching success was found (Lukasch et al., 2017a,b).
Major histocompatability complex genes are known to influence the gut microbiota of animals (Toivanen et al., 2001; Lanyon et al., 2007; Bolnick et al., 2014; Lin et al., 2014; Kubinak et al., 2015). Recent evidence suggests that MHC diversity negatively affects the feather microbiota of blue petrels (Halobaena caerulea) (Leclaire et al., 2019) and Leach’s storm petrels (Oceanodroma leucorhoa) (Pearce et al., 2017) and influences bacteria-based skeleton diseases in broiler chicken (Joiner et al., 2005). However, some studies revealed a positive relationship (Hernández-Gómez et al., 2018; Khan et al., 2019), which suggests that the relationship between MHC and microbiota diversity is not yet settled.
In the present study, for a first approach, we used bacterial culture techniques to specifically address urinary tract bacteria (UTI bacteria) and bacteria growing on blood agar, reflecting a more general bacteria composition. Then, we examined whether there is a relationship between MHC diversity and the number of these cultivable eggshell bacteria, partly originating from the urogenital tract of female passerines, in particular, house sparrows (P. domesticus). We predicted that the number of eggshell bacteria originating from the urinary tract would be negatively related to the diversity of functional MHC alleles, whereas a more general bacteria composition, e.g., including bacteria originating from the environment, may not be. Therefore, females with more variable MHC would be expected to transfer fewer bacteria onto the eggshell surface. If this also affects pathogenic bacteria, there could be a transgenerational benefit. In contrast, in house sparrows, a strong immune system could be also negative, e.g., in terms of a reduced fertilization success (Lukasch et al., 2017a,b).
Materials and Methods
Housing Conditions and Study Setup
The study was conducted in an aviary population of house sparrows at the Konrad-Lorenz Institute of Ethology in Vienna, Austria. In April 2014, 31 breeding pairs with functional MHC allele numbers, known from another study (Lukasch et al., 2017a,b), were set up in 10 aviaries (measuring 2 m × 4 m and 2.6 m high). All aviaries contained three to four pairs, were provided with sufficient nest boxes and nest material, and were equipped with vegetation and perches. Water and commercial food for granivorous passerines were provided ad libitum. These breeding conditions for our aviary population of wild house sparrows were intended to limit the impact of the environment on eggshell bacteria. To further reduce the environmental impact, eggs were collected within 1 h of laying (for details, see below).
To improve comparability, all females were measured prior to the start of laying on the same day (April 15). Tarsus length was determined to the nearest 0.1 mm using calipers, and body mass was estimated with an electronic scale to the nearest 0.1 g (Svensson, 1992). Each bird was fitted with an individual combination of color rings, to later determine the pair composition for each nest box. After the onset of reproduction, fresh commercial food for insectivorous passerines and mealworms was provided four times a day. The nest boxes of each breeding pair were examined daily until the second egg was laid, from which eggshell bacteria were immediately sampled (for methods, see below).
Bacteria Sampling and Cultivation Procedure
We followed a procedure already used for other bird species (Ruiz-de-Castañeda et al., 2011b), in our own previous studies (Brandl et al., 2014; Hoi et al., 2019) and the instructions of the agar manufacturer (Oxoid). After removing the egg from the nest with sterile gloves, we swabbed the egg surface for 20 s using a sterile cotton swab soaked in sterile water. The swab was immediately stored in a transport medium (transport viscose swab with Amies transport medium, Sarstedt). Within 48 h, the swab was transferred to 1.0 ml of phosphate-buffered saline (PBS) in the laboratory. The 15-ml tube, containing 1.0 ml of PBS and the swab, was mixed by vortex agitation for 120 s. One hundred microliter subsamples of the bacterial suspensions were extracted and serially decimal diluted, ranging from no dilution up to 10–6, and applied to separate plates. Usually, about four different concentrations were produced for each sample. After being incubated for 24 h, bacterial colonies originated and developed, each from a single founder bacterium. Thus, each colony-forming unit (CFU) reflected a single bacterium. Based on the number of CFUs developed, the plate with the most suitable number of colonies to separate them as distinct colonies (according to our experience up to 300 colonies/plate) was selected. The number of CFUs was then extrapolated according to the dilution on the respective plate. Finally, to reach the total number for the whole sample of 1.0 ml, this number was multiplied by 10. This ensured that two relative abundance measurements of cultivated bacteria were derived for further analyses (Supplementary Material).
After dilutions were completed, 0.1 ml of all samples and dilutions were spread-plated on non-selective (Columbia agar with 7% sheep blood, Oxoid) and selective (Brilliance UTI, Oxoid) media that indicated total bacterial counts. The plates were then incubated for 24 h at 37°C. After the incubation period, CFU counting, a common method reflecting bacteria density and used in several other studies (Godard et al., 2007; Peralta-Sánchez et al., 2012), was performed for both media separately.
Columbia agar with 7% sheep blood is a highly nutritious, general purpose medium for the isolation and cultivation of non-fastidious and fastidious microorganisms from a variety of clinical and non-clinical materials. The sheep blood supplement allows the culture of most culturable bacteria, regardless of their metabolic requirements1. Columbia blood agar has been used to cultivate bacteria from different animal taxa including birds (Ruiz-de-Castañeda et al., 2011b; Merbach et al., 2019) and mammals (Infante et al., 2008; Di et al., 2020).
Brilliance UTI agar is a chromogenic medium used more specifically for the presumptive identification and differentiation of the main microorganisms that cause UTIs (Wellman-Labadie et al., 2008; Maluta et al., 2014; Barber et al., 2016) including Enterococcus spp., Escherichia coli, Proteus, Morganella, Providencia, Pseudomonas, Staphylococcus, Streptococcus, and coliforms. Therefore, we used two bacterial categories (see above): (i) general bacterial abundance, which included the number of all bacteria growing on the Columbia agar with sheep blood, and (ii) UTI bacterial abundance, which are bacteria growing on the Brilliance UTI agar. These two relative abundance measurements, reflecting the number of CFUs of the two bacterial groups, have been used as a dependent variable.
We analyzed the second egg of 68 clutches in total. Due to missing data in individual subsets, 61 eggs were used for the general bacterial abundance, and 60 eggs were used for UTI bacterial abundance. Of the 31 females used, each female contributed on average 2.2 ± 0.1 (s.e.) eggs.
MHC Genotyping
Characterization of the MHC class I exon 3 encoding parts of the peptide-binding region was carried out using 454 amplicon sequencing (Galan et al., 2010), refined for house sparrows by Karlsson and Westerdahl (2013) at Lund University Sequencing Facility (Faculty of Science), Sweden. For MHC bioinformatics and data processing after 454 sequencing was carried out, the data were extracted and assigned to samples using the program jMHC (Stuglik et al., 2011). MHC diversity was evaluated as the number of different functional alleles per individual.
The MHC genotyping of the female house sparrows was carried out in an earlier study (for details, see Lukasch et al., 2017a,b). The number of functional alleles determined in this subset of females (N = 31) was on average 4.258, ranging from 2 to 8. Based on their chemical properties in the peptide-binding region, these could be translated into 35 unique functional MHC alleles.
Statistical Analyses
Generalized linear mixed models with a negative binomial error distribution were fit to test the effect of the female number of functional MHC alleles for general bacterial abundance and UTI bacterial abundance from the eggshell surface. Female identity and aviary identity were used as random effects. Other potential factors, namely the start of egg laying and the female body condition, which is known to be related to the immune system (Dannemiller et al., 2017), were also included in the model. For the start of egg laying, a continuous series of numbers was used, taking January 1 as the first day. For the female body condition, we used the residuals from a linear regression between body mass not explained by size (tarsus length). A model with negative binomial distribution was used because this method performs better than log-transformation of count data.
Both models were fit using R version 3.6.0 (R Core Team, 2019), package lme4 (Bates et al., 2015). To calculate pseudo-R2 values for non-linear regression models, we used the R package MuMIn. To ensure that the results were not biased by a correlation between variables, we calculated the variance inflation factors (VIF) of the models (Zuur and Ieno, 2016). None of the variables exceeded two, which is the recommended threshold (Zuur et al., 2010). Model assumptions such as normality of residuals, overdispersion, and zero inflation were tested by the package DHARMa (Hartig, 2020). Normality of residuals was visually tested using a Q–Q plot and the Kolmogorov–Smirnov test (general bacterial abundance model, p = 0.32; UTI bacteria abundance model, p = 0.70). Models for count data following a negative binomial or Poisson distribution do not assume homogeneity of variance. However, they have other additional assumptions that we tested for, such as overdispersion (general bacterial abundance model, p = 0.35; UTI bacteria abundance model, p = 0.23) and zero inflation (general bacterial abundance model, p = 0.97; UTI bacteria abundance model, p = 1). All of these assumptions were met in both models.
Results
When examining MHC diversity in terms of the number of functional alleles (fMHCsum) in relation to the general bacterial numbers, we found no significant effect (Table 1a and Figure 1A, pseudo-R2 = 0.19). The parameter estimates predicted a 27.3% decrease in bacterial numbers, with an increase in the number of functional alleles. However, this effect was only near significance (p = 0.077, see Table 1a). The female body condition (residual body mass not explained by tarsus length) did not influence bacterial numbers at all; however, there was a significant seasonal effect, namely that bacterial numbers increase with time during the season (Table 1).
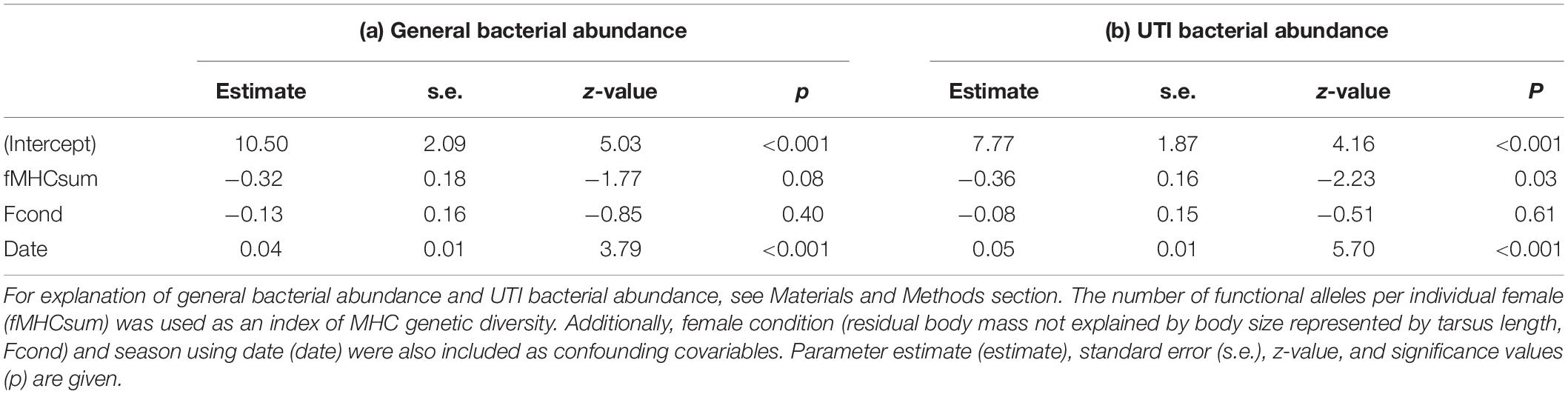
Table 1. Relationship between the MHC diversity on general bacterial (a) and UTI bacterial abundance (b).
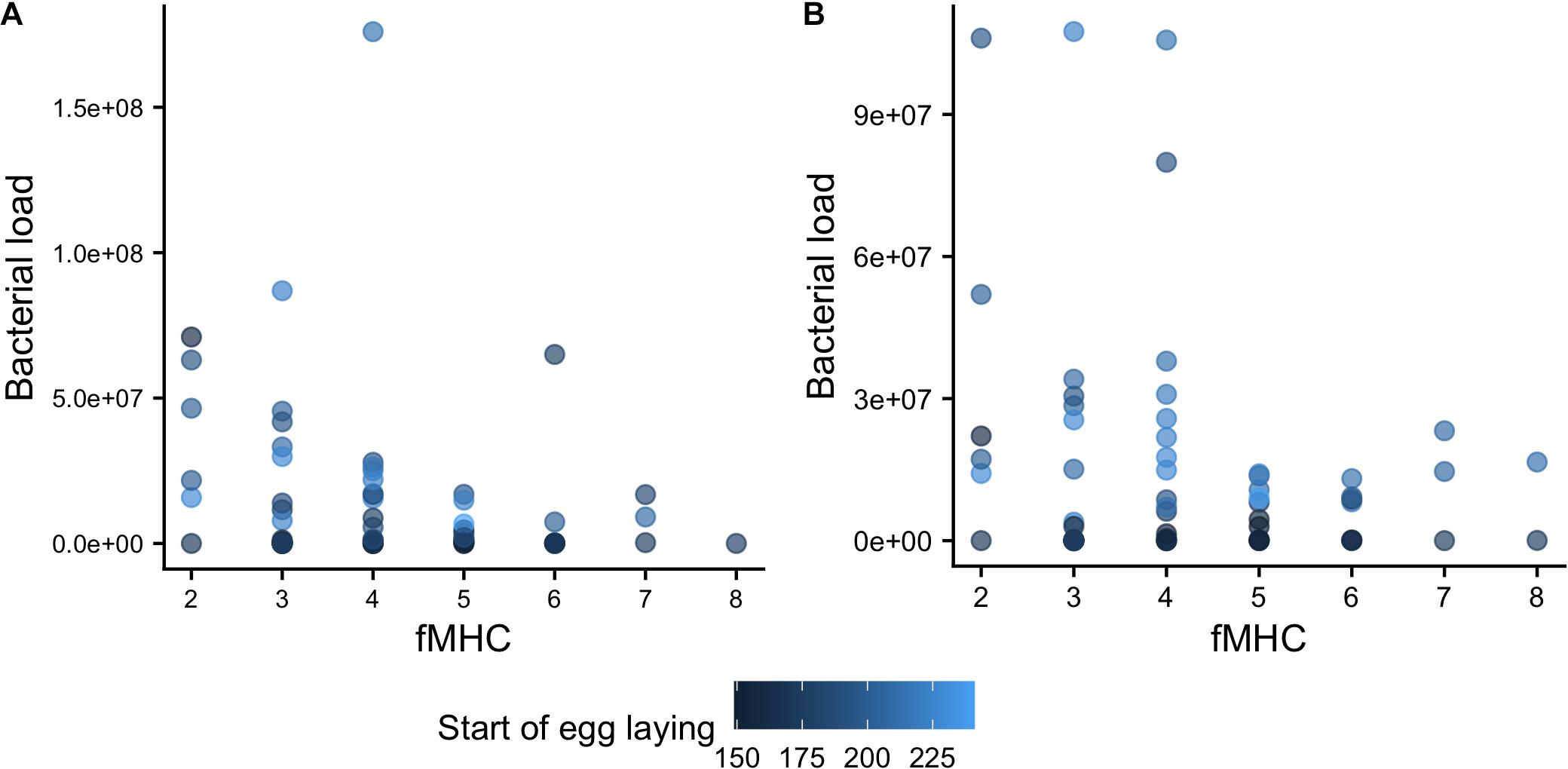
Figure 1. Relationship of general bacterial (A) and UTI bacterial abundance (B) with the number of functional alleles per individual female (fMHCsum) which is an index of MHC genetic diversity. The color gradient represents the start of egg laying (a day from the beginning of the calendar year) of a particular female ranging from gray at the beginning of the breeding season to light blue at the end.
In contrast, a significant negative effect was found when examining MHC diversity in terms of the number of functional alleles (fMHCsum) in relation to UTI bacterial abundance (Table 1b and Figure 1B, pseudo-R2 = 0.31). The abundance of UTI bacteria found on the eggshell of a female decreased by 30.2% with every extra functional allele. Again, the season was revealed to be important and the female body condition was not. Similar to the general bacterial numbers, the positive parameter estimate suggested an increase in UTI bacteria over the season (Table 1).
Discussion
Our results support the prediction that eggshell bacterial numbers, cultivated on different media, are negatively related to the number of fMHC alleles and that this effect of female fMHC seems stronger with regard to UTI bacteria likely originating from the female urogenital tract. There seems to be a similar trend in general bacterial numbers, although a significant effect could only be found in the case of UTI bacteria. This is the first study that has revealed evidence of a relationship between female MHC diversity and eggshell bacteria. These findings suggest that maternal MHC plays a role in hatching success and offspring survival (Lukasch et al., 2017a). However, further research is needed to determine which eggshell bacteria are particularly important in this context.
The non-significant relationship between general bacterial numbers and female functional MHC diversity may be due to the fact that general bacterial numbers also include bacteria originating from the environment outside the female body. We did not expect a strong relationship between general bacteria in relation to the female immune system. The weaker impact of bacteria growing on blood agar is probably also due to bacterial samples being taken immediately after laying, where eggs were only briefly exposed to the environment. Consequently, it is likely that eggshell bacteria originating from the environment have less time to settle and their occurrence is more stochastic. The type of nest boxes, nest box position, and nest material provided are also the same. Hence, no striking differences in environmental conditions are to be expected between nest boxes, whereas variation in bacterial numbers between nest boxes is huge (see Supplementary Material and Figure 1). Thus, environmentally transmitted eggshell bacteria should mainly originate from the body surface of the mother (Soler et al., 2012; Jacob et al., 2014). It has been shown that bacteria transmitted to the eggshell, originating from the parental skin or feathers, can be affected by uropygial gland wax composition, which in turn is influenced by the female immune system (Soler et al., 2012; Jacob et al., 2014) and nest material.
In this context, it was found that bacteria originating from the environment, such as the feather microbial community in blue petrels (H. caerulea), can be affected by the fMHC diversity of the parents (Leclaire et al., 2019). A likely underlying mechanism is that MHC variants alter preen wax composition and, consequently, the bacterial composition, shown for seabirds (Leclaire et al., 2019) and songbirds (Slade et al., 2016).
The female body condition had no effect on eggshell bacterial abundance, probably because resource investment could be balanced between the immune system and other activities (Westneat and Birkhead, 1998).
We also discovered a seasonal effect on eggshell bacterial abundance, namely an increase in abundance of both bacterial groups when sampled later in the season. This increase was not due to a temperature increase on the egg surface, provoked by incubation, because bacterial samples were always taken immediately after laying and prior to the start of incubation (Ruiz-de-Castañeda et al., 2011a). Similarly, a positive relationship between eggshell bacterial load and the laying date in active magpie (Pica pica) nests was found, due to parental activities (Soler et al., 2015). However, since the egg was again taken immediately after laying, this explanation might be less important in our results.
Alternatively, the seasonal change in bacterial abundances before the start of incubation may be due to the accumulation of bacteria in the female tract (cloaca) over time. It is well known that bacteria can be sexually transmitted to a great extent (Lombardo et al., 1996). Intra- as well as extrapair copulation activity may lead to an accumulating effect and an increase in bacterial abundance. However, earlier studies in our aviary population did not reveal an obvious change in copulation behavior during that period, and genetic analyses revealed no evidence for extrapair paternity (unpublished results). An explanation could be that male paternity guarding is more efficient under aviary conditions. However, the lack of extrapair paternity does not necessarily imply a lack of extrapair copulations. Thus, bacterial accumulation in the female cloaca is a possibility, but the cloaca is regularly emptied and bacteria transferred by males should therefore be removed to a great extent. If bacteria were to accumulate in the female, one would also expect older females to harbor more bacteria. However, there is no indication for this, and in our study, all females originated from the same age cohort, which prevents further conclusions. It is most likely that ambient conditions, e.g., temperature for bacteria development, improve and lead to a growing contamination of food and water, which may influence gut bacterial compositions. The lack of clear difference in the seasonal effect between general and UTI bacteria, at least when looking at the parameter estimate (Table 1), suggests an indirect environmental effect, e.g., bacteria transferred through the gut, rather than a direct ambient effect.
There is evidence that female house sparrows with higher MHC diversity are more effective in reducing a higher proportion of male-transmitted bacteria (Lukasch et al., 2017a). However, experiments would be necessary to demonstrate causal relationships between female MHC and bacterial composition. An experimental approach would also be necessary to determine bacterial effects on chick fitness. One possibility would be to compare control incubated eggs with sterilized and incubated eggs. Finally, it would be interesting to determine the importance of different bacterial strains for offspring fitness.
Conclusion
Female house sparrows, which harbor a more diverse MHC, lay eggs with less cultivable eggshell bacteria. This is the first evidence of a possible alternative route of maternal effects. In particular, we found evidence that the transgenerational transmission of bacteria can depend on the immune genotype of the mother. However, given that the effect of bacteria can be negative or positive, it is questionable whether a good maternal immune system is beneficial for the offspring. It may depend very much on the bacterial strains involved. Further research is needed to investigate the impact of bacteria on embryo development and whether mothers are able to selectively act against specific bacterial strains.
Data Availability Statement
The original contributions presented in the study are included in the article/Supplementary Material, further inquiries can be directed to the corresponding author/s.
Ethics Statement
The animal study was reviewed and approved by the Ethical Commission of the Austrian Ministry of Sciences (ZI.09/0997/2011).
Author Contributions
HH contributed to the study concept and preparation of the manuscript. MP contributed to the observation in the aviaries, bacterial sampling from the eggshells, and statistical analysis. AD and JK contributed to the bacterial sampling and bacterial cultivation. BL contributed to the MHC determination. All authors were involved in the interpretation of the results and approved the revised manuscript before its submission.
Funding
This work was supported by the VEGA Scientific Grant Agency of the Ministry of Education, Science, Research and Sport of the Slovak Republic and the Slovak Academy of Sciences under Grant 02/0034/15 (AD and JK).
Conflict of Interest
The authors declare that the research was conducted in the absence of any commercial or financial relationships that could be construed as a potential conflict of interest.
Acknowledgments
We would like to thank Renate Hengsberger for her help throughout the whole writing and submission process and two referees for their very fruitful and thorough suggestions to significantly improve the manuscript.
Supplementary Material
The Supplementary Material for this article can be found online at: https://www.frontiersin.org/articles/10.3389/fevo.2021.615667/full#supplementary-material
Footnotes
References
Barber, A. E., Norton, J. P., Wiles, T. J., and Mulvey, M. A. (2016). Strengths and limitations of model systems for the study of urinary tract infections and related pathologies. Microbiol. Mol. Biol. Rev. 80, 351–367. doi: 10.1128/mmbr.00067-15
Bates, D., Mächler, M., Bolker, B., and Walker, S. (2015). Fitting linear mixed-effects models using lme4. J. Stat. Softw. 67, 1–48. doi: 10.18637/jss.v067.i01
Bolnick, D. I., Snowberg, L. K., Caporaso, J. G., Lauber, C., Knight, R., and Stutz, W. E. (2014). Major histocompatibility complex class IIb polymorphism influences gut microbiota composition and diversity. Mol. Ecol. 23, 4831–4845. doi: 10.1111/mec.12846
Brandl, H. B., van Dongen, W. F. D., Darolová, A., Krištofík, J., Majtan, J., and Hoi, H. (2014). Composition of bacterial assemblages in different components of reed warbler nests and a possible role of egg incubation in pathogen regulation. PLoS One 9:e114861. doi: 10.1371/journal.pone.0114861
Cebra, J. J. (1999). Influences of microbiota on intestinal immune system development. Am. J Clin. Nutr. 69, 1046S–1051S. doi: 10.1093/ajcn/69.5.1046s
D’Alba, L., Oborn, A., and Shawkey, M. D. (2010). Experimental evidence that keeping eggs dry is a mechanism for the antimicrobial effects of avian incubation. Naturwissenschaften 97, 1089–1095. doi: 10.1007/s00114-010-0735-2
Dannemiller, N. G., Webb, C. T., Wilson, K. R., Bentler, K. T., Mooers, N. L., Ellis, J. W., et al. (2017). Impact of body condition on influenza A virus infection dynamics in mallards following a secondary exposure. PLoS One 12:e0175757. doi: 10.1371/journal.pone.0175757
De Reu, K., Grijspeerdt, K., Messens, W., Heyndrickx, M., Uyttendaele, M., Debevere, J., et al. (2006). Eggshell factors influencing eggshell penetration and whole egg contamination by different bacteria, including Salmonella enteritidis. Int. J Food Microbiol. 112, 253–260. doi: 10.1016/j.ijfoodmicro.2006.04.011
Di, J., Zhang, J., Cao, L., Huang, T.-T., Zhang, J.-X., Mi, Y.-N., et al. (2020). Hydrogen peroxide-mediated oxygen enrichment eradicates Helicobacter pylori in vitro and in vivo. Antimicrob. Agents Chemother. 64:e02192-19. doi: 10.1128/aac.02192-19
Galan, M., Guivier, E., Caraux, G., Charbonnel, N., and Cosson, J.-F. (2010). A 454 multiplex sequencing method for rapid and reliable genotyping of highly polymorphic genes in large-scale studies. BMC Genom. 11:296. doi: 10.1186/1471-2164-11-296
Godard, R. D., Wilson, C. M., Frick, J. W., Siegel, P. B., and Bowers, B. B. (2007). The effects of exposure and microbes on hatchability of eggs in open-cup and cavity nests. J. Avian Biol. 38, 709–716.
Goebel, J., Promerová, M., Bonadonna, F., McCoy, K. D., Serbielle, C., Strandh, M., et al. (2017). 100 million years of multigene family evolution: origin and evolution of the avian MHC class IIB. BMC Genom. 18:460. doi: 10.1186/s12864-017-3839-7
Goodenough, A. E., and Stallwood, B. (2012). Differences in culturable microbial communities in bird nestboxes according to orientation and influences on offspring quality in great tits (Parus major). Microb. Ecol. 63, 986–995. doi: 10.1007/s00248-011-9992-7
Hartig, F. (2020). DHARMa: Residual Diagnostics for Hierarchical (Multi-Level/Mixed) Regression Models. R Package Version 0.2.7 [Online].
Hernández-Gómez, O., Briggler, J. T., and Williams, R. N. (2018). Influence of immunogenetics, sex and body condition on the cutaneous microbial communities of two giant salamanders. Mol. Ecol. 27, 1915–1929. doi: 10.1111/mec.14500
Hill, M. J. (1997). Intestinal flora and endogenous vitamin synthesis. Eur. J Cancer Prev. 6, S43–S45. doi: 10.1097/00008469-199703001-00009
Hoi, H., Darolová, A., Krištofík, J., Poláček, M., Majtán, J., Zeman, M., et al. (2019). Covariation between eggshell colouration and eggshell bacteria abundance and egg characteristics in blackbirds. Ethol. Ecol. Evol. 31, 249–265. doi: 10.1080/03949370.2019.1575910
Hooper, L. V., and Gordon, J. I. (2001). Commensal host-bacterial relationships in the gut. Science 292, 1115–1118. doi: 10.1126/science.1058709
Infante, B., Villar, S., Palma, S., Merello, J., Valencia, R., Torres, L., et al. (2008). BALB/c Mice resist infection with Bartonella bacilliformis. BMC Res. Notes 1:103. doi: 10.1186/1756-0500-1-103
Jacob, S., Immer, A., Leclaire, S., Parthuisot, N., Ducamp, C., Espinasse, G., et al. (2014). Uropygial gland size and composition varies according to experimentally modified microbiome in Great tits. BMC Evol. Biol. 14:134. doi: 10.1186/1471-2148-14-134
Joiner, K. S., Hoerr, F. J., van Santen, E., and Ewald, S. J. (2005). The avian major histocompatibility complex influences bacterial skeletal disease in broiler breeder chickens. Vet. Pathol. 42, 275–281. doi: 10.1354/vp.42-3-275
Karlsson, M., and Westerdahl, H. (2013). Characteristics of MHC class I genes in house sparrows Passer domesticus as revealed by long cDNA transcripts and amplicon sequencing. J. Mol. Evol. 77, 8–21. doi: 10.1007/s00239-013-9575-y
Keller, L. H., Benson, C. E., Krotec, K., and Eckroade, R. J. (1995). Salmonella enteritidis colonization of the reproductive tract and forming and freshly laid eggs of chickens. Infect. Immun. 63, 2443–2449. doi: 10.1128/Iai.63.7.2443-2449.1995
Khan, M. A. W., Stephens, W. Z., Mohammed, A. D., Round, J. L., and Kubinak, J. L. (2019). Does MHC heterozygosity influence microbiota form and function? PLoS One 14:e0215946. doi: 10.1371/journal.pone.0215946
Kubinak, J. L., Stephens, W. Z., Soto, R., Petersen, C., Chiaro, T., Gogokhia, L., et al. (2015). MHC variation sculpts individualized microbial communities that control susceptibility to enteric infection. Nat. Commun. 6:8642. doi: 10.1038/ncomms9642
Kulkarni, S., and Heeb, P. (2007). Social and sexual behaviours aid transmission of bacteria in birds. Behav. Proc. 74, 88–92. doi: 10.1016/j.beproc.2006.10.005
Lanyon, C. V., Rushton, S. P., O’Donnell, A. G., Goodfellow, M., Ward, A. C., Petrie, M., et al. (2007). Murine scent mark microbial communities are genetically determined. FEMS Microbiol. Ecol. 59, 576–583. doi: 10.1111/j.1574-6941.2006.00252.x
Leclaire, S., Strandh, M., Dell’Ariccia, G., Gabirot, M., Westerdahl, H., and Bonadonna, F. (2019). Plumage microbiota covaries with the major histocompatibility complex in blue petrels. Mol. Ecol. 28, 833–846. doi: 10.1111/mec.14993
Ley, R. E., Lozupone, C. A., Hamady, M., Knight, R., and Gordon, J. I. (2008). Worlds within worlds: evolution of the vertebrate gut microbiota. Nat. Rev. Microbiol. 6, 776–788. doi: 10.1038/nrmicro1978
Lin, P., Bach, M., Asquith, M., Lee, A. Y., Akileswaran, L., Stauffer, P., et al. (2014). HLA-B27 and human β2-microglobulin affect the gut microbiota of transgenic rats. PLoS One 9:e105684. doi: 10.1371/journal.pone.0105684
Lombardo, M. P., Thorpe, P. A., Cichewicz, R., Henshaw, M., Millard, C., Steen, C., et al. (1996). Communities of cloacal bacteria in tree swallow families. Condor 98, 167–172. doi: 10.2307/1369521
Lukasch, B., Westerdahl, H., Strandh, M., Knauer, F., Winkler, H., Moodley, Y., et al. (2017a). Major histocompatibility complex genes partly explain early survival in house sparrows. Sci. Rep. 7:6571. doi: 10.1038/s41598-017-06631-z
Lukasch, B., Westerdahl, H., Strandh, M., Winkler, H., Moodley, Y., Knauer, F., et al. (2017b). Genes of the major histocompatibility complex highlight interactions of the innate and adaptive immune system. PeerJ 5:e3679. doi: 10.7717/peerj.3679
Macpherson, A. J., and Harris, N. L. (2004). Interactions between commensal intestinal bacteria and the immune system. Nat. Rev. Immunol. 4, 478–485. doi: 10.1038/nri1373
Maluta, R. P., Logue, C. M., Casas, M. R. T., Meng, T., Guastalli, E. A. L., Rojas, T. C. G., et al. (2014). Overlapped sequence types (STs) and serogroups of avian pathogenic (APEC) and human extra-intestinal pathogenic (ExPEC) Escherichia coli Isolated in Brazil. PLoS One 9:e105016. doi: 10.1371/journal.pone.0105016
Merbach, S., Peters, M., Kilwinski, J., and Reckling, D. (2019). Suttonella ornithocola-associated mortality in tits in germany. Berliner Münchener Tierärztliche Wochenschrift 132, 459–463. doi: 10.2376/0005-9366-18065
Messens, W., Grijspeerdt, K., De Reu, K., De Ketelaere, B., Mertens, K., Bamelis, F., et al. (2007). Eggshell Penetration of Various Types of Hens’ Eggs by Salmonella enterica Serovar Enteritidis. J. Food Prot. 70, 623–628. doi: 10.4315/0362-028x-70.3.623
Okamura, M., Kamijima, Y., Miyamoto, T., Tani, H., Sasai, K., and Baba, E. (2001a). Differences among six Salmonella serovars in abilities to colonize reproductive organs and to contaminate eggs in laying hens. Avian. Dis. 45, 61–69. doi: 10.2307/1593012
Okamura, M., Miyamoto, T., Kamijima, Y., Tani, H., Sasai, K., and Baba, E. (2001b). Differences in abilities to colonize reproductive organs and to contaminate eggs in intravaginally inoculated hens and in vitro adherences to vaginal explants between Salmonella enteritidis and other Salmonella Serovars. Avian. Dis. 45, 962–971. doi: 10.2307/1592875
Org, E., Parks, B. W., Joo, J. W. J., Emert, B., Schwartzman, W., Kang, E. Y., et al. (2015). Genetic and environmental control of host-gut microbiota interactions. Genom. Res. 25, 1558–1569. doi: 10.1101/gr.194118.115
Park, S., Choi, S., Kim, H., Kim, Y., Kim, B.-S., Beuchat, L. R., et al. (2015). Fate of mesophilic aerobic bacteria and Salmonella enterica on the surface of eggs as affected by chicken feces, storage temperature, and relative humidity. Food Microbiol. 48, 200–205. doi: 10.1016/j.fm.2015.01.003
Pearce, D. S., Hoover, B. A., Jennings, S., Nevitt, G. A., and Docherty, K. M. (2017). Morphological and genetic factors shape the microbiome of a seabird species (Oceanodroma leucorhoa) more than environmental and social factors. Microbiome 5:146. doi: 10.1186/s40168-017-0365-4
Penn, D. J., Damjanovich, K., and Potts, W. K. (2002). MHC heterozygosity confers a selective advantage against multiple-strain infections. Proc. Natl. Acad. Sci. U.S.A. 99, 11260–11264. doi: 10.1073/pnas.162006499
Peralta-Sánchez, J., Martín-Platero, A. M., Wegener-Parfrey, L., Martínez-Bueno, M., Rodríguez-Ruano, S., Navas-Molina, J. A., et al. (2018). Bacterial density rather than diversity correlates with hatching success across different avian species. FEMS Microbiol. Ecol. 94:fiy022. doi: 10.1093/femsec/fiy022
Peralta-Sánchez, J. M., Martín-Vivaldi, M., Martín-Platero, A. M., Martínez-Bueno, M., Oñate, M., Ruiz-Rodríguez, M., et al. (2012). Avian life history traits influence eggshell bacterial loads: a comparative analysis. Ibis 154, 725–737. doi: 10.1111/j.1474-919X.2012.01256.x
Pinowski, J., Barkowska, M., Kruszewicz, A. H., and Gruszewicz, A. G. (1994). The causes of the mortality of eggs and nestlings of Passer spp. J. Biosci. 19, 441–451. doi: 10.1007/Bf02703180
R Core Team (2019). R: A Language and Environment for Statistical Computing [Online]. Vienna: R Foundation for Statistical Computing.
Rock, K. L., Reits, E., and Neefjes, J. (2016). Present yourself! By MHC class I and MHC class II molecules. Trends Immunol. 37, 724–737. doi: 10.1016/j.it.2016.08.010
Ruiz-de-Castañeda, R., Vela, A. I., González-Braojos, S., Briones, V., and Moreno, J. (2011a). Drying eggs to inhibit bacteria: incubation during laying in a cavity nesting passerine. Behav. Proc. 88, 142–148. doi: 10.1016/j.beproc.2011.08.012
Ruiz-de-Castañeda, R., Vela, A. I., Lobato, E., Briones, V., and Moreno, J. (2011b). Prevalence of potentially pathogenic culturable bacteria on eggshells and in cloacae of female Pied Flycatchers in a temperate habitat in central Spain. J. Field Ornithol. 82, 215–224. doi: 10.1111/j.1557-9263.2011.00324.x
Schmitt, C., Garant, D., Bélisle, M., and Pelletier, F. (2017). Linking innate immunogenetic variation with phenotypic traits in a wild population of tree swallows, Tachycineta bicolor. Biol. J Linn. Soc. 121, 685–697. doi: 10.1093/biolinnean/blx022
Schwensow, N., Fietz, J., Dausmann, K. H., and Sommer, S. (2007). Neutral versus adaptive genetic variation in parasite resistance: importance of major histocompatibility complex supertypes in a free-ranging primate. Heredity 99, 265–277. doi: 10.1038/sj.hdy.6800993
Slade, J. W. G., Watson, M. J., Kelly, T. R., Gloor, G. B., Bernards, M. A., and MacDougall-Shackleton, E. A. (2016). Chemical composition of preen wax reflects major histocompatibility complex similarity in songbirds. Proc. R. Soc. B 283:20161966. doi: 10.1098/rspb.2016.1966
Soler, J. J., Peralta-Sánchez, J. M., Flensted-Jensen, E., Martín-Platero, A. M., and Møller, A. P. (2011). Innate humoural immunity is related to eggshell bacterial load of European birds: a comparative analysis. Naturwissenschaften 98, 807–813. doi: 10.1007/s00114-011-0830-z
Soler, J. J., Peralta-Sánchez, J. M., Martín-Platero, A. M., Martín-Vivaldi, M., Martínez-Bueno, M., and Møller, A. P. (2012). The evolution of size of the uropygial gland: mutualistic feather mites and uropygial secretion reduce bacterial loads of eggshells and hatching failures of European birds. J. Evol. Biol. 25, 1779–1791. doi: 10.1111/j.1420-9101.2012.02561.x
Soler, J. J., Ruiz-Rodríguez, M., Martín-Vivaldi, M., Peralta-Sánchez, J. M., Ruiz-Castellano, C., and Tomás, G. (2015). Laying date, incubation and egg breakage as determinants of bacterial load on bird eggshells: experimental evidence. Oecologia 179, 63–74. doi: 10.1007/s00442-015-3322-6
Stuglik, M. T., Radwan, J., and Babik, W. (2011). jMHC: software assistant for multilocus genotyping of gene families using next-generation amplicon sequencing. Mol. Ecol. Res. 11, 739–742. doi: 10.1111/j.1755-0998.2011.02997.x
Svensson, L. (1992). Identification Guide to European Passerines. Thetford: British Trust for Ornithology.
Tangkham, W., Janes, M., and LeMieux, F. (2016). Prevalence and distribution of campylobacter jejuni in small-scale broiler operations. J. Food Prot. 79, 75–81. doi: 10.4315/0362-028X.JFP-15-331
Timoney, J. F., Shivaprasad, H. L., Baker, R. C., and Rowe, B. (1989). Egg transmission after infection of hens with Salmonella enteritidis phage type 4. Vet. Rec. 125, 600–601. doi: 10.1136/vr.125.24.600
Toivanen, P., Vaahtovuo, J., and Eerola, E. (2001). Influence of major histocompatibility complex on bacterial composition of fecal flora. Infect. Immun. 69, 2372–2377. doi: 10.1128/IAI.69.4.2372-2377.2001
Wellman-Labadie, O., Lakshminarayanan, R., and Hincke, M. T. (2008). Antimicrobial properties of avian eggshell-specific C-type lectin-like proteins. FEBS Lett. 582, 699–704. doi: 10.1016/j.febslet.2008.01.043
Westneat, D. F., and Birkhead, T. R. (1998). Alternative hypotheses linking the immune system and mate choice for good genes. Proc. R. Soc. B 265, 1065–1073.
You, J., Wu, Y., Zhang, X., Wang, X., Gong, J., Zhao, Z., et al. (2019). Efficient fecal-oral and possible vertical, but not respiratory, transmission of emerging Chlamydia gallinacea in broilers. Vet. Microbiol. 230, 90–94. doi: 10.1016/j.vetmic.2019.01.018
Zuur, A. F., and Ieno, E. N. (2016). A protocol for conducting and presenting results of regression-type analyses. Methods Ecol. Evol. 7, 636–645. doi: 10.1111/2041-210X.12577
Keywords: eggshell bacteria, MHC diversity, bacterial abundance, bacterial culture, Passer domesticus
Citation: Darolová A, Poláček M, Krištofík J, Lukasch B and Hoi H (2021) First Evidence of a Relationship Between Female Major Histocompatibility Complex Diversity and Eggshell Bacteria in House Sparrows (Passer domesticus). Front. Ecol. Evol. 9:615667. doi: 10.3389/fevo.2021.615667
Received: 09 October 2020; Accepted: 26 January 2021;
Published: 24 February 2021.
Edited by:
Claus Wedekind, University of Lausanne, SwitzerlandReviewed by:
Jamie C. Winternitz, Bielefeld University, GermanyLaetitia Georgina Elisabeth Wilkins, University of California, Davis, United States
Copyright © 2021 Darolová, Poláček, Krištofík, Lukasch and Hoi. This is an open-access article distributed under the terms of the Creative Commons Attribution License (CC BY). The use, distribution or reproduction in other forums is permitted, provided the original author(s) and the copyright owner(s) are credited and that the original publication in this journal is cited, in accordance with accepted academic practice. No use, distribution or reproduction is permitted which does not comply with these terms.
*Correspondence: Herbert Hoi, aGVyYmVydC5ob2lAdmV0bWVkdW5pLmFjLmF0; orcid.org/0000-0002-3038-9673
†Present address: Miroslav Poláček, Department of Forest Ecology, Faculty of Forestry and Wood Sciences, Czech University of Life Sciences, Prague, Czechia