- Department of Zoology, Institute of Ecology and Earth Sciences, University of Tartu, Tartu, Estonia
Seasonal polyphenisms are cases in which individuals representing generations occurring in different times of the year systematically differ in their morphological, physiological, and/or behavioral traits. Such differences are often assumed to constitute adaptive responses to seasonally varying environments, but the evidence for this is still scarce. The adaptive character of the response would be corroborated by the pattern in which the decision about choosing a particular seasonal phenotype is made before the onset of respective environmental conditions (anticipatory plasticity). Alternatively, the between-generation differences can be caused by immediate effects of seasonally varying environments (responsive plasticity). Here we reared the larvae of the seasonally polymorphic map butterfly Araschnia levana under two different photoperiodic regimes, which provided different seasonal cues. These two treatments induced direct development and diapause pathways, respectively. Replicating the experiment at different temperatures and levels of host plant quality allowed us to evaluate both the anticipatory and the responsive components of the associated plastic changes in life-history traits. Larvae representing the direct development pathway invariably had higher growth rates and shorter development periods, although the difference between the developmental pathways was smaller at inferior host quality. Body size differences between the developmental pathways turned out to be less consistent, as the natural pattern of higher pupal mass of the directly developing individuals could only be reproduced at lower rearing temperature. Though being considerably modified by immediate environmental effects, the between-generation differences in size, growth rates, and larval are largely based on anticipatory plasticity (= responses to photoperiodic cues) and should be treated as seasonal adaptations in A. levana. In a more general context, we show how investigating the proximate basis of size differences can serve the purpose of identifying the limits of phenotypic plasticity in juvenile growth schedules.
Introduction
Reaction norms for size and time at maturation constitute a core element of the theory of life-history evolution (Stearns, 1992; Roff, 1992, 2002). Evolutionary forces shaping such reaction norms can perhaps be best understood through comparisons among groups such as closely related species (Tammaru et al., 2015; Kivelä et al., 2020), different populations within species (Meister et al., 2017a,b, 2018), and sexes (Teder and Tammaru, 2005). Additionally, the numerous well-known examples of insect polyphenism in general (reviewed by Shapiro, 1976; Simpson et al., 2011; Nylin, 2013) and seasonally polyphenic insects in particular offer an excellent opportunity to compare distinct morphs, which often differ in values of developmental traits (Wiklund et al., 1991; Teder et al., 2010; Morehouse et al., 2013; Kivelä et al., 2015). An obvious advantage of such studies is that the groups to be compared can be considered genetically identical, so that any observed differences can unambiguously be ascribed to phenotypic plasticity.
A particular question to be answered in studies on such reaction norms is that about the proximate basis of the differences in body sizes. The individuals that ultimately attain larger adult sizes may (1) be larger from the beginning, (2) grow for a longer time, or (3) grow faster (Blanckenhorn, 2000; Sõber et al., 2019). As one particular implication, the answer to this question has direct relevance to the problem of evolutionary ecology of growth rate as a separate life-history trait (Arendt, 1997). If the differences in final sizes are due to growth rates, revealing the associated differences in other traits allows us to address the question about costs of fast growth (Dmitriew, 2011). In turn, if the groups being compared do not differ in growth rate, this may indicate that growth is indeed maximized within limits set by physiology, as the classic life-history models often assume (Stearns, 1992; Roff, 1992).
Another proximate level question is how early—in the ontogeny of the individual—the alternative developmental pathways diverge from each other. The answer to this question can contribute to identifying the limits to adaptive evolution of juvenile growth patterns. In particular, in the cases of sexual size dimorphism, the sexes start to diverge already in early phases of larval development (see Stillwell et al., 2014; Vendl et al., 2018; Chelini et al., 2019 for recent studies). This pattern has been interpreted as evidence of some constraint on major sex-related changes late in larval development: differences in adult size cannot be attained in one step but have to be accumulated throughout the development (Tammaru et al., 2010; Meister et al., 2018).
From a different—ultimate rather than proximate—perspective, knowing when and how do size differences appear during immature development can shed light on adaptive nature of the divergent growth patterns (Esperk et al., 2013). Different seasonal generations of insects pass through their immature development in predictably differing environmental conditions, with temperature and food (=host plant, for herbivorous insects) quality being perhaps the most significant seasonally variable parameters. Both temperature and host quality have strong direct effects on larval growth schedules, which may not require any explanation beyond the proximate physiological one (responsive plasticity sensu Whitman and Agrawal, 2009). On the other hand, in seasonal environments, the stages succeeding the larval stage can experience predictably different conditions as well. In such cases, the growing immatures are expected to react to cues of forthcoming environmental changes in a way shaped by natural selection. For example, short photoperiod is frequently used as a cue of the onset of the unfavorable season, which primarily informs developmental decisions related to winter diapause (Tauber et al., 1986; Danks, 1987; Leather et al., 1993; Koštál, 2006; Saunders, 2020). This type of response to environmental factors has been termed anticipatory plasticity and should be considered adaptive (Whitman and Agrawal, 2009).
In the present study, we make use of a comparison of growth patterns between two seasonal generations of a polyphenic butterfly. The two different developmental pathways—direct and diapause development—were induced in the laboratory by manipulating photoperiodic regime. The experiment was replicated under two temperatures and in different times of the year (=different host quality). The larvae were weighed daily to obtain detailed information about their growth curves in different treatments. This allowed us to treat development periods, final masses of instars, and growth rates of individual larvae as separate response variables and to assess plastic responses in all these parameters. The detailed information accumulated facilitated evaluating different adaptive hypotheses, as well as shed light on proximate basis of plastic responses in insect growth schedules.
From the ultimate perspective, the used three-factor design allowed us to explicitly compare effects attributable to responsive vs. anticipatory plasticity. In particular, we proceeded from the assumption that any responses to the photoperiodic cue (especially when consistent across other treatments) would likely have the anticipatory character (Esperk et al., 2013) and used the opportunity to compare the magnitude of such (presumably adaptive) effects to the physiologically based responses to temperature and food quality. The latter are rather universal in insects and should be represented by lower growth rates, longer development times, and lower final body masses as a response to inferior food quality (Teder et al., 2014) and lower growth rates, longer development times, but higher final masses under lower temperatures (Atkinson, 1994, 1996; Atkinson and Sibly, 1997; Angilletta et al., 2004).
Materials and Methods
Study Species
The European map butterfly (Araschnia levana L., Lepidoptera: Nymphalidae) is a temperate Palaearctic insect species that is bivoltine in most of its range (including the study area), although a facultative third generation may occur in the southern parts of its distribution area (Tolman and Lewington, 2008). The species is well known for its striking seasonal polyphenism in wing color and patterning (Friberg and Karlsson, 2010; Ihalainen and Lindstedt, 2012). The between-generation difference in life span (mainly attributable to the different duration of the pupal stage, which is the overwintering stage in this species) is no less impressive, as completing of the life cycle takes approximately 10 weeks in directly developing generation compared to 42 weeks of the diapausing one (Freitak et al., 2019). In addition, the two generations are known to differ in adult abundance (Viidalepp and Remm, 1996), body composition and resource allocation patterns (Friberg and Karlsson, 2010; Morehouse et al., 2013), body design and flight performance (Fric and Konvička, 2002; Friberg and Karlsson, 2010), and immunity (Baudach et al., 2018; Freitak et al., 2019) and body size (about 15% in terms of live mass of the pupae, Freitak et al., 2019; personal observations of the authors). The eggs are laid in string-like clusters, and the larvae aggregate on their host plant (Urtica spp.), whereas the group size declines with larval ontogeny (Ruf, 2002). Like in most temperate insects, seasonal polyphenism in A. levana is controlled primarily by photoperiod: long days during larval stage induce the directly developing form, whereas short-day conditions lead to the diapausing pathway. The decision whether to develop directly or overwinter in the pupal stage is made during the 4th and 5th (i.e., penultimate and final, respectively) larval instars (Friberg et al., 2011).
Experimental Design
To investigate the influence of environmental factors on the life-history traits of the two developmental pathways, map butterfly larvae were reared in controlled conditions under a 2 × 2 × 2 crossed experimental design (Supplementary Table 1). The factors varied were (1) photoperiod—long (18L:6D, inducing direct development) or short day (12L:12D, inducing diapause development), (2) temperature (17 vs. 22°C), and (3) host quality, manipulated by means of rearing the larvae in either early (June) or late season (August).
The progeny of map butterfly females of Estonian origin was transferred to 50-mL plastic vials in groups of 10 newly hatched larvae. The vials were then equally divided between the two rearing chambers set to different photoperiodic regimes, considering equal representation of broods (split-family design) and hatching dates in the treatments. To induce different developmental pathways, one of the chambers was set to the long-day conditions (18L:6D), whereas in the other the short-day conditions (12L:12D) were created. To reduce the potential microclimatic differences of the rearing chambers on larval growth, the photoperiodic treatments (and respective larvae) were rotated between the chambers with a 3-day interval. Leaves of the stinging nettle (Urtica dioica) were provided as food and were changed every other day or more frequently when necessary to avoid food depletion.
The larvae were reared in groups until the end of their 3rd instar being housed individually in the vials of the same type later on. In the cases when 6 or more larvae out of 10 died in the vial before the end of their 3rd instar, the survivors were divided between the vials representing the same brood and the same hatching date. From the beginning of the 4th instar onward, the larvae were weighed daily until pupation.
The experiments were performed in 2 years (2010 and 2011) at the University of Tartu, Estonia. To investigate the influence of food quality on larval development, the larvae were reared both in early season (June, the time when directly developing generation is in their larval stage) and in late season (August, the time for the larval stage of diapausing generation). The progeny of wild-collected diapausing individuals was used in all early season experiments, while the offspring of wild-collected directly developing individuals was used in late season experiments. As the quality of nettle leaves has been shown to decline with progressing season (Morehouse et al., 2013), the larvae from the early season rearings were assumed to receive high-quality diet, whereas the diet quality was lower in late season. In 2010, the larvae were reared at 22°C, while the temperature was set to 17°C in 2011.
To examine the proximate effect of light conditions on larval growth rates, the larvae were weighed twice a day during the first 2 days in both early and late season experiment in 2011. Specifically, 12 hours’ weighing interval was targeted (but it varied from 10 to 14 h, for technical reasons). As a result, the “diurnal period” covered only photophase of both photoperiodic treatments, whereas the “nocturnal period” included only scotophase of the short-day treatment and 6 h photophase, followed by 6-h scotophase of the long-day treatment.
Data Analysis
The dependence of development times (durations of the 4th and 5th instar), body masses and growth rates on developmental pathway (direct/diapause), temperature (17/22°C), season (early season/late season), sex (male/female), and their interaction were analyzed by general linear mixed models (PROC MIXED, Littell et al., 2006; data of different experiments pooled). Brood was included as a random factor; denominator degrees of freedom were estimated using the Satterthwaite option. Minimum adequate models were constructed by sequentially removing non-significant interaction terms.
Instar durations were expressed as the time between the two successive molts. Alternatively, active growth phases were calculated based on the time of preceding molt and the time of attaining the peak mass of an instar. Growth rates were calculated as (mass at the end of the period1/3 − mass at the beginning of the period1/3)/(duration of the period) (Esperk and Tammaru, 2004; Tammaru and Esperk, 2007). Two different indices of growth rate were derived. First, instantaneous growth rates were calculated for a 24-h period on the 2nd day of the instar (the “free growth period,” Esperk and Tammaru, 2004; Meister et al., 2017a). Second, integral growth rates were found for the entire positive growth phase of the instar (from the molt until the attainment of peak body mass). In experiments in which the effect of light conditions on larval growth was studied, the observation periods deviating from the targeted 12-h period were corrected according to the actual time span. The larvae that died before the pupation (3–9% mortality during the last two instars in different trials, with no substantial differences between the treatments) and the small proportion of insects (5%, on average) that entered the pupal diapause in the long-day photoperiodic treatments were discarded from further analyses. All analyses were conducted in SAS 9.4 (SAS Institute Inc., Cary, NC, United States).
Results
There was a consistent effect of photoperiod on development time (Figures 1, 2 and Supplementary Table 2). In particular, the time spent in both penultimate and final instar was 10–20% longer in individuals that developed through diapause pathway, compared to directly developing individuals. The magnitude of the difference varied, depending on temperature and season but invariably attained statistical significance (Table 1 and Figures 1, 2). The alternative measure of development time, the period of active growth (from molting until achieving of peak body mass), was also longer in diapausing than in directly developing individuals (Table 2). Individuals reared at 17°C had, on average, 50% longer penultimate and final instar durations and longer active growth phases than those reared at the higher temperature; late-season larvae had 5–10% longer instar durations than those reared in early season (Supplementary Table 2), and instar durations of females were 5–15% longer than in males (all differences statistically significant, Tables 1, 2). The differences in durations of the 4th and 5th instar between the developmental pathways were larger in early than in late season and at lower than at higher rearing temperatures (significant interaction terms, Tables 1, 2 and Supplementary Table 2). However, when analyzed separately by rearing temperature and season, the differences between developmental pathways remained significant in all cases except in the 4th instar active growth phase of larvae reared at low temperature and in late season. In the final instar, the difference between the sexes was higher at lower than at higher rearing temperature and in late than in early season (significant interaction terms, Tables 1, 2).
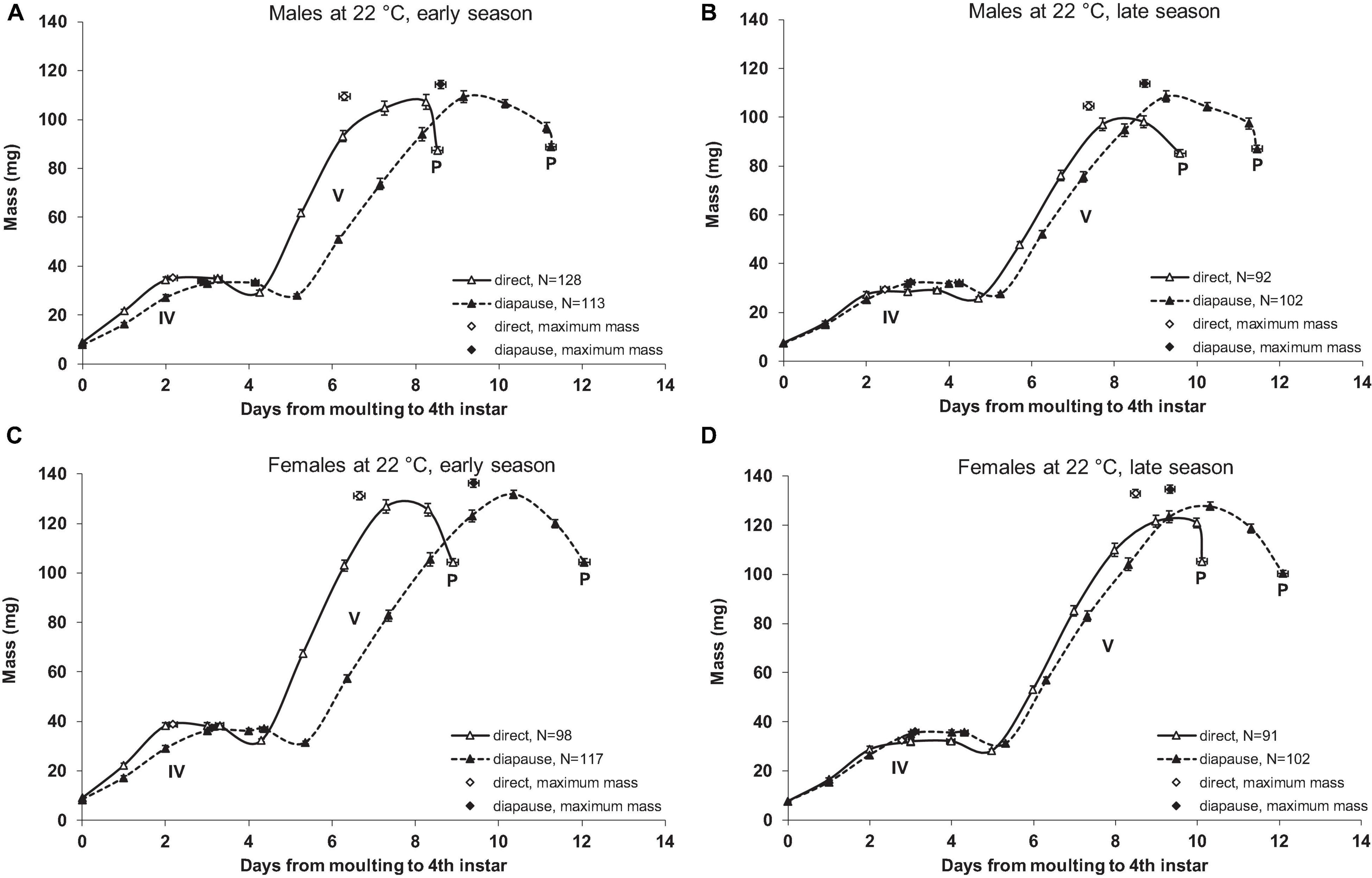
Figure 1. Growth curves of Araschnia levana larvae reared at 22°C in 2010. (A) Male larvae reared in early season (June). (B) Male larvae reared in late season (August). (C) Female larvae reared in early season. (D) Female larvae reared in late season. Direct development [“long day” (18L:6D) photoperiodic treatment] and diapause [“short day” (12L:12D) photoperiodic treatment] pathways were induced. Values are corrected for the effect of brood (using SAS, PROC MIXED, least square means option, Littell et al., 2006). Roman numerals stand for instars, “P” indicates pupation, and “N” marks sample sizes in the beginning of the instars and in the pupal stage. Error bars indicate standard errors; horizontal error bars stand for standard errors for the duration of the 4th and 5th instar and for the time period from molting until attaining peak body mass in respective instar.
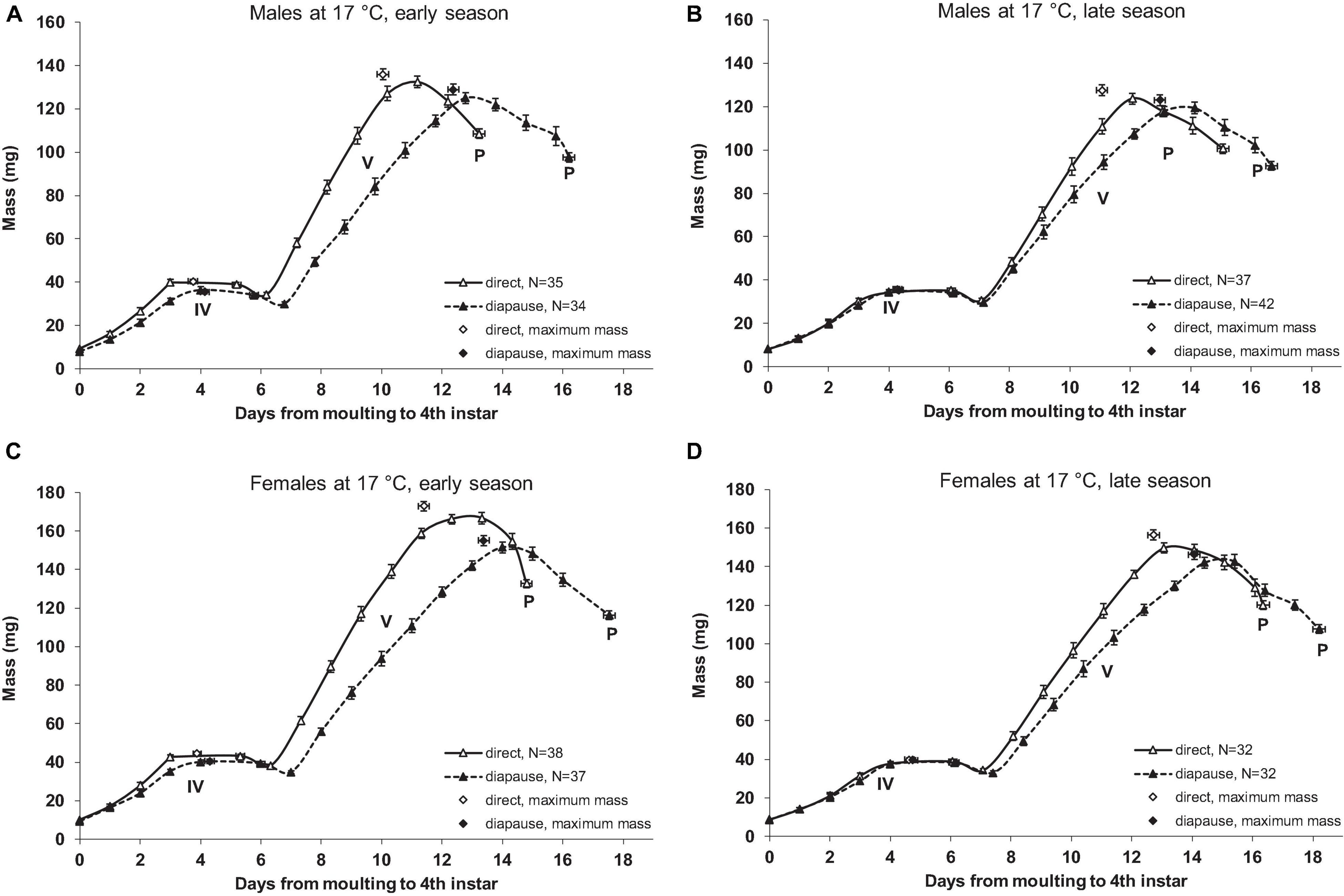
Figure 2. Growth curves of Araschnia levana larvae reared at 17°C. Direct development [“long day” (18L:6D) photoperiodic treatment] and diapause [“short day” (12L:12D) photoperiodic treatment] pathways were induced in 2011. (A) Male larvae reared in early season. (B) Male larvae reared in late season. (C) Female larvae reared in early season. (D) Female larvae reared in late season. Direct development and diapause pathways were induced. See Figure 1 for details.
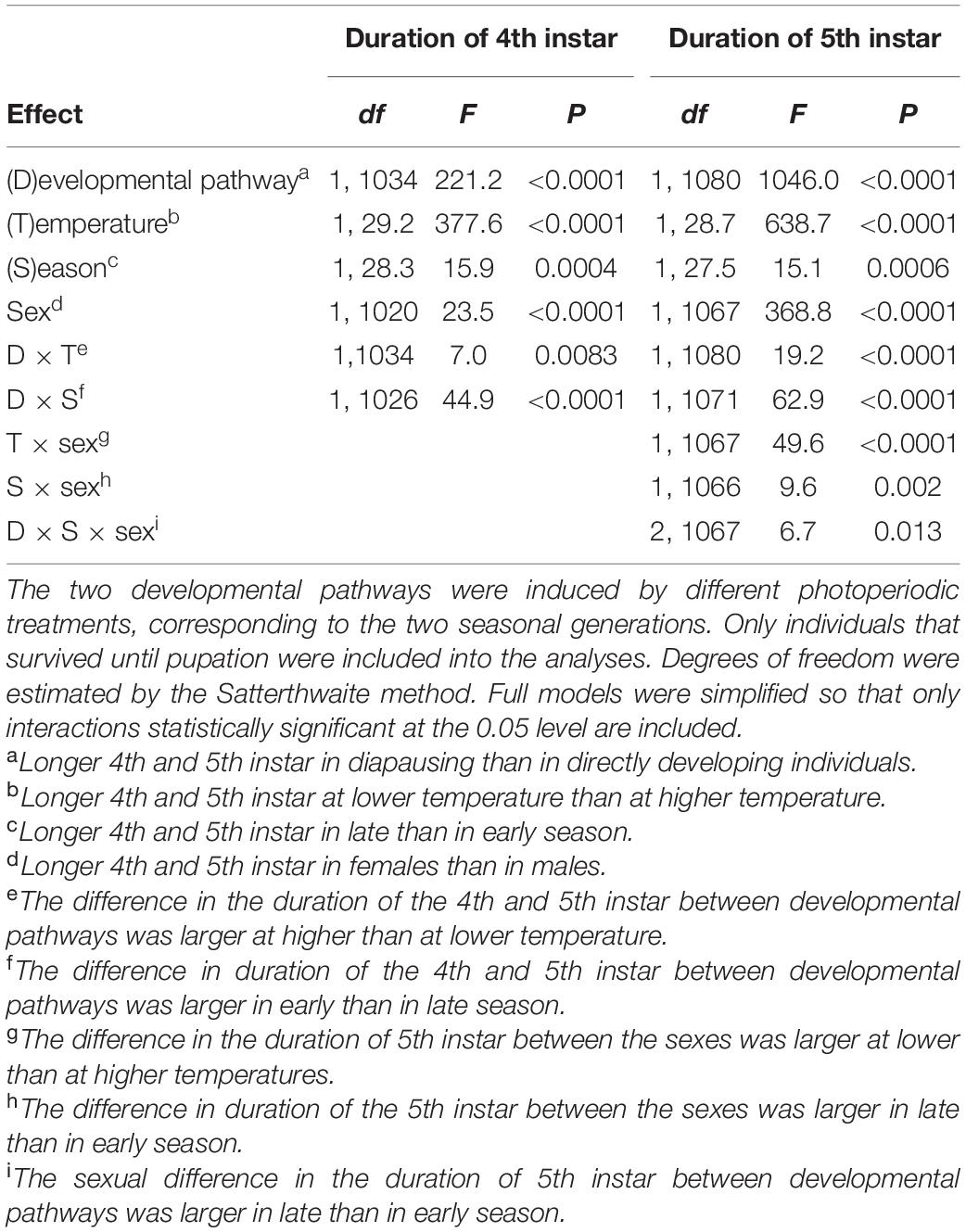
Table 1. Duration of the 4th and 5th instar of Araschnia levana larvae as explained by developmental pathway (direct vs. diapause development), temperature (17 vs. 20°C), season (June vs. August), and sex; linear mixed models (SAS, Proc MIXED, type 3 sums of squares) with brood (progeny of one female) as a random effect.
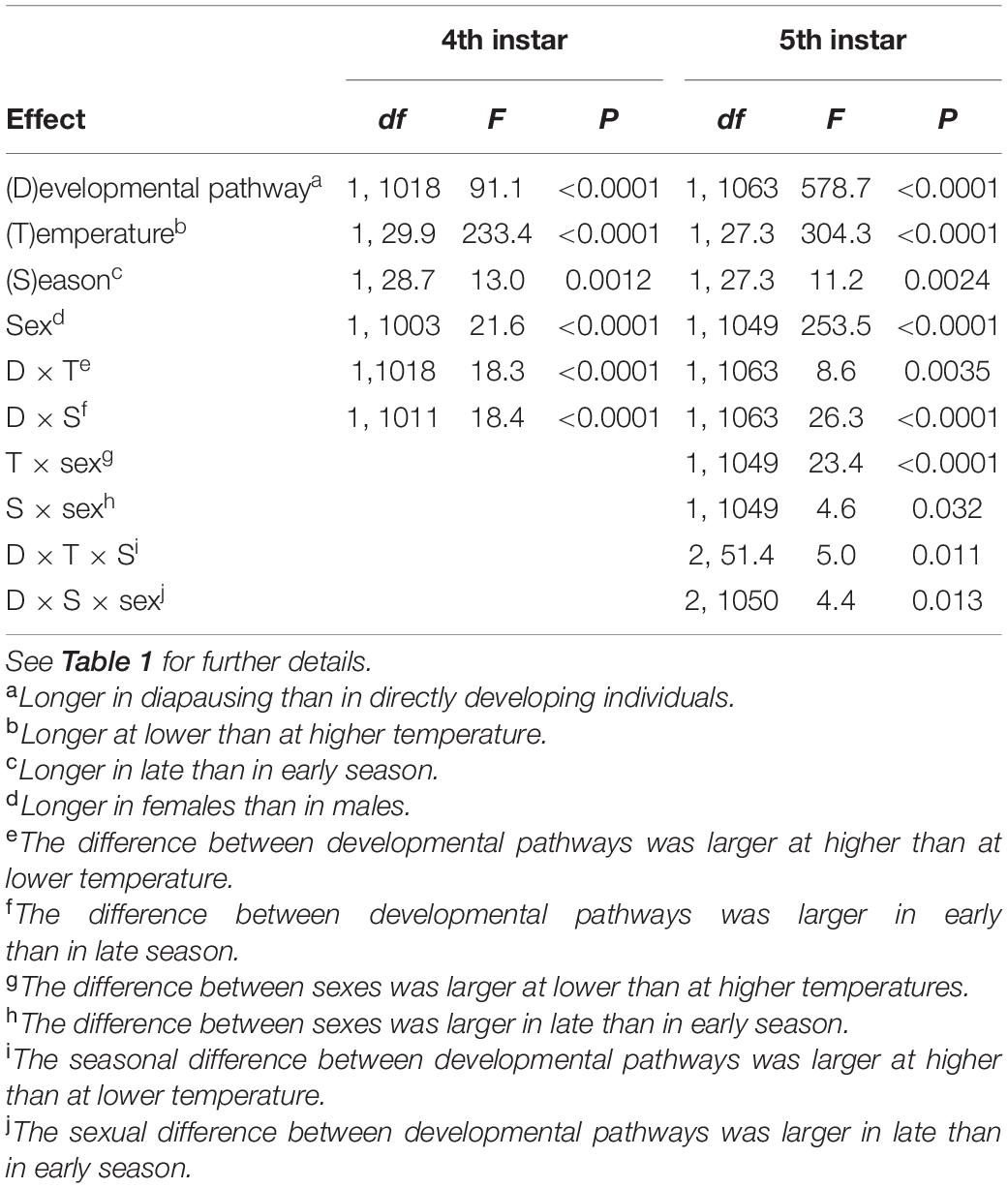
Table 2. Duration of active growth phase (from molt until attainment of peak body mass) in the 4th and 5th instar Araschnia levana larvae as explained by developmental pathway, temperature, season, and sex; linear mixed models (SAS, Proc MIXED, type 3 sums of squares) with brood (progeny of one female) as a random effect.
There was a consistent effect of photoperiod on larval growth rates (Figures 1, 2 and Supplementary Table 2). Instantaneous and integral growth rates, both in the 4th and 5th instar, were higher in directly developing than in diapausing individuals and in individuals reared at the higher than at the lower temperature (Tables 3, 4 and Supplementary Table 2). In both instars, integral growth rates were higher in early than in late season (Table 4); however, there were no seasonal differences in instantaneous growth rates (Table 3). There was no significant sexual difference in growth rates in the 4th instar, but in the 5th instar, females had higher instantaneous growth rates and lower integral growth rates than males (Tables 3, 4). There was a larger difference in integral growth rates between developmental pathways at higher than at lower temperature and in early than in late season (significant interaction terms, Table 4). When analyzed separately by rearing temperature and season, the differences in growth rates between the developmental pathways remained significant in all cases with one exception (4th instar integral growth rate at low temperature in late season).
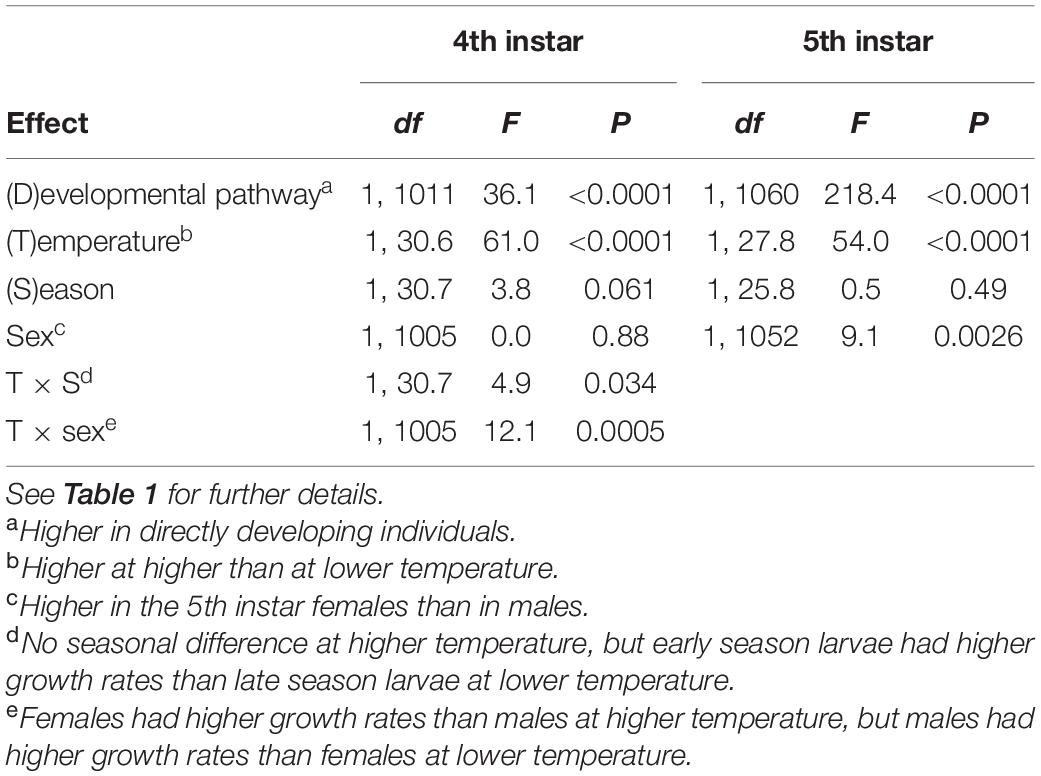
Table 3. Instantaneous growth rates (in second day of the instar) in the 4th and 5th instar Araschnia levana larvae as explained by developmental pathway, temperature, season, and sex; linear mixed models (SAS, Proc MIXED, type 3 sums of squares) with brood (progeny of one female) as a random effect.
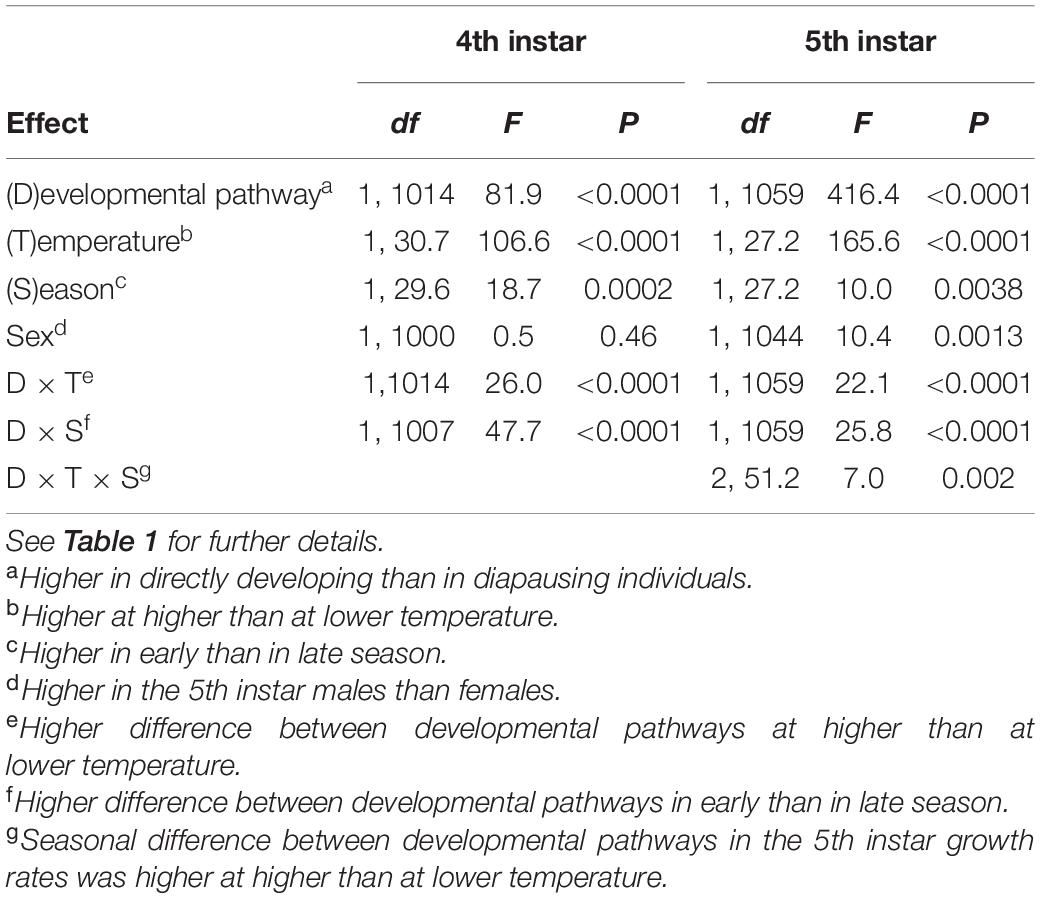
Table 4. Integral growth rates (calculated as peak mass of instar1/3− mass at the beginning of the instar1/3)/(duration of the period from molt to attainment of peak body mass] in the 4th and 5th instar Araschnia levana larvae as explained by developmental pathway, temperature, season, and sex; linear mixed models (SAS, Proc MIXED, type 3 sums of squares) with brood (progeny of one female) as a random effect.
Photoperiod had an influence on body sizes, but interactive effects were substantial (Figures 1, 2 and Supplementary Table 2). In 2011 experiments in which the proximate effect of light conditions on growth was studied, directly developing individuals had higher instantaneous growth rates during the 4th instar 2nd-day diurnal period (30% higher growth rates; F1,257 = 46.0, P < 0.0001) and the 5th instar 2nd-day diurnal period (30% higher growth rates; F1,269 = 51.9, P < 0.0001) than diapause-destined individuals. However, the growth rates did not differ significantly between the developmental pathways during the 2nd night (i.e., nocturnal phase of the second day) in either 4th instar (F1,265 = 2.2, P = 0.14) or 5th instar (F1,261 = 3.1, P = 0.077).
Pupal masses were 5% higher in diapausing than in directly developing individuals, 15% higher at lower than at higher rearing temperature, 5% higher in early than in late season (Supplementary Table 2), and 20% higher in females than in males (all differences statistically significant, Table 5 and Figures 1, 2). However, when analyzed separately by rearing temperature (justified by significant interaction term, Table 5), directly developing individuals achieved significantly (10%) higher pupal masses only at 17°C, whereas there were no significant differences in pupal mass between developmental pathways at 22°C (Supplementary Table 2).
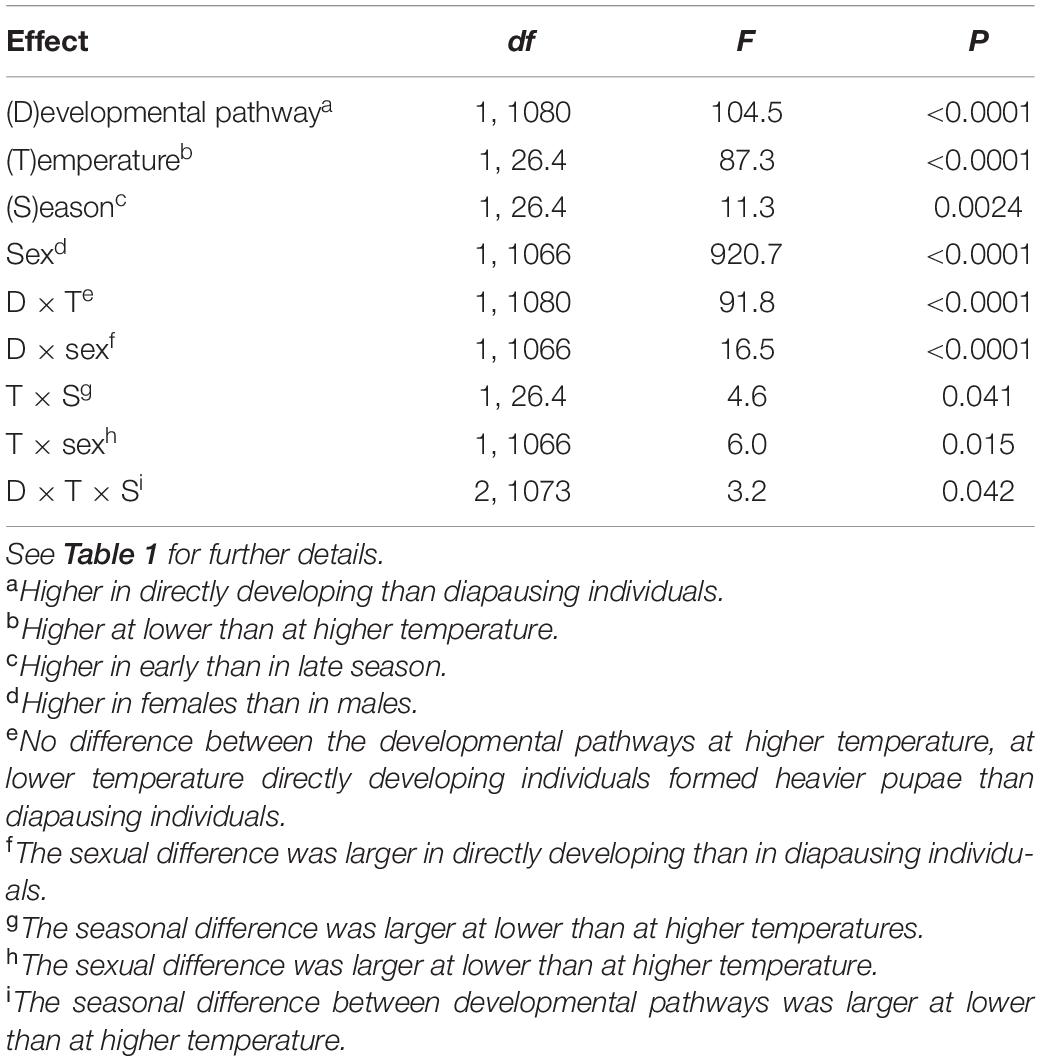
Table 5. Pupal mass of Araschnia levana as explained by developmental pathway, temperature, season, and sex; linear mixed models (SAS, Proc MIXED, type 3 sums of squares) with brood (progeny of one female) as a random effect.
Premolt masses and peak masses in the 4th and 5th instar were significantly higher in in early than in late season and, with the exception of the 4th instar premolt mass, at lower than at higher rearing temperatures (Tables 6, 7, Supplementary Table 2, and Figures 1, 2). However, the difference in both penultimate and last instar masses between the developmental pathways was strongly influenced by rearing conditions (indicated by significant interaction terms between developmental pathway and rearing temperature and between developmental pathway and season, Tables 6, 7 and Supplementary Table 2). In particular, in the 4th instar and in the beginning of the 5th instar, directly developing individuals were heavier than diapausing individuals only in the early season, whereas diapausing individuals achieved higher masses in late season (Tables 6, 7 and Figures 1, 2). Consistently, body mass in the 5th instar and peak mass in the 4th instar was higher in directly developing than in diapausing individuals only at 17°C, whereas diapausing individuals were heavier than directly developing ones at 22°C.
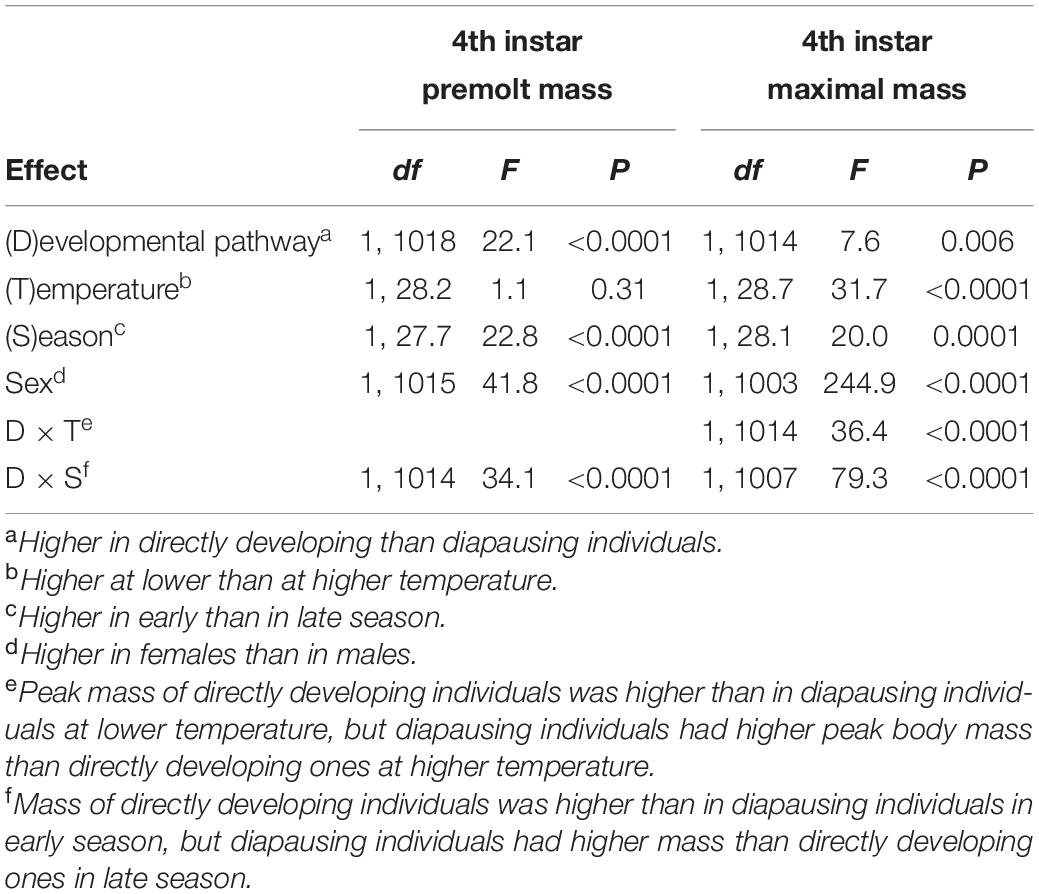
Table 6. Fourth instar premolt mass (mass before the molt to the 4th instar) and 4th instar peak mass of Araschnia levana larvae as explained by developmental pathway, temperature, season, and sex; linear mixed models (SAS, Proc MIXED, type 3 sums of squares) with brood (progeny of one female) as a random effect. See Table 1 for further details.
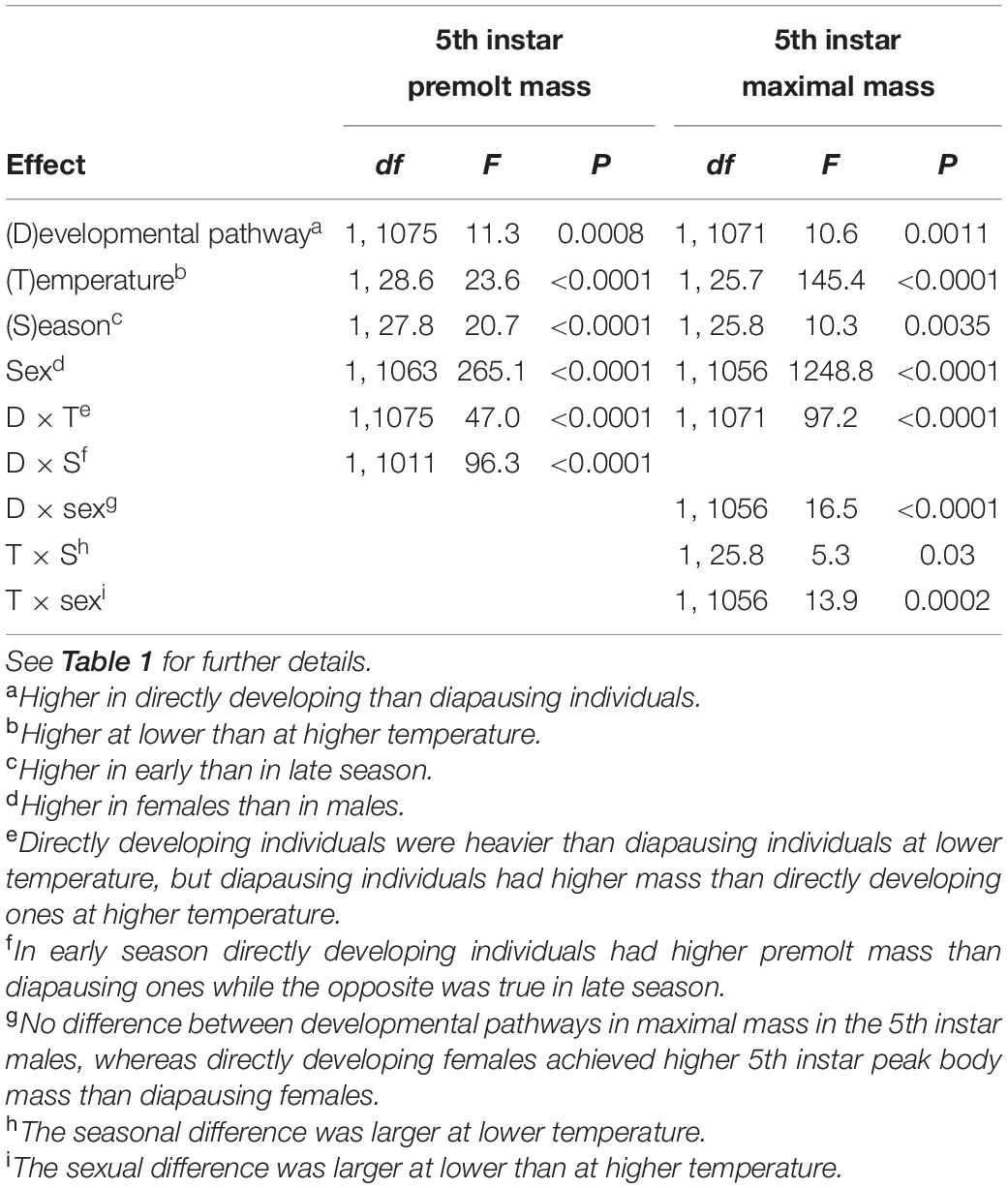
Table 7. Fifth instar premolt mass (mass before the molt to the 5th instar) and 5th instar peak mass of Araschnia levana larvae as explained by developmental pathway, temperature, season, and sex; linear mixed models (SAS, Proc MIXED, type 3 sums of squares) with brood (progeny of one female) as a random effect.
Discussion
Our results demonstrate that the two seasonal generations of the European map butterfly differ in various parameters of larval growth schedules. The seasonal generations (directly developing vs. diapause-destined individuals) were induced experimentally by photoperiodic treatments. Most clearly and consistently, there were considerable among-generation differences in instar-specific development times—the larvae that were to produce diapausing pupae spent more time in both final and penultimate larval instars. At low (but not high) temperatures, the diapause development larvae also attained lower pupal masses. Directly developing larvae had higher growth rates than those heading toward diapause, consistently so across different rearing conditions, sexes, and larval instars, irrespectively of the way how the growth rates were expressed (instantaneous or integral). However, in more detailed comparisons, instantaneous growth rates of the developmental pathways significantly differed only during the diurnal but not in the nocturnal phase.
Our results show that the between-generation differences in developmental schedules contain a considerable element of anticipatory plasticity and can therefore be considered adaptive. This conclusion is based on the observation that some of such differences, induced here by photoperiodic treatments, consistently appeared in all combinations of the two other environmental factors manipulated in the experiment. The between-generation differences cannot thus solely be based on proximate effects of temperature and host quality during larval development. Nevertheless, both temperature and host quality still had an influence on parameters of larval growth schedules, as well as showed numerous interactive effects with the developmental pathway. In part, the lower body size of the spring-flying generation of the map butterfly can thus still be based on responsive (non-adaptive) effects of inferior food quality the larvae encounter at the end of summer, with some additional impact of temperatures experienced during larval development. Overall, in our experiments, the effects of host quality (season) tended to be somewhat weaker (for development time and growth rates) or similar (for body mass) to those of developmental pathway (Figures 1, 2 and Supplementary Table 2). In contrast, the effect of temperature was larger than the difference between developmental pathways. Nevertheless, we have to consider that the difference between the temperature treatments (5°C) considerably exceeded the differences expected between natural developmental periods of the two seasonal generations.
Of the interactive effects, we find it notable that the differences between developmental pathways were clearly more pronounced on high-quality spring food compared to the lower-quality nettle leaves offered in August. We see here an interesting parallel to the observation that, in sexually size dimorphic species, also the sexual size dimorphism (SSD) is more pronounced in favorable environmental conditions (Teder and Tammaru, 2005; Stillwell and Davidowitz, 2010).
Photoperiod is the factor that is most commonly used as a cue for forthcoming seasons by temperate insects (Beck, 1980; Saunders, 2020). Responses to photoperiod are therefore commonly considered to have an anticipatory character but they may nevertheless include elements of responsive plasticity. Indeed, in several insects, feeding behavior and growth patterns have been shown to differ between the day and the night, typically in the way that larvae feed more actively and have higher growth rates at night (Berger and Gotthard, 2008; Berger et al., 2011). Surprisingly, however, in our experiments, directly developing larvae had higher growth rates than diapause-destined larvae during the diurnal, but not during the nocturnal phase of the active growth period in both penultimate and final instar. As conditions during the diurnal phase were exactly the same for both developmental pathways, these differences can only be ascribed to anticipatory and not to responsive plasticity. Thus, the response to the photoperiod very likely has an adaptive character in A. levana. On the other hand, it cannot be excluded that responses of larval growth to temperature and food quality can also contain anticipatory/adaptive elements, rather than being entirely based on some universal physiological relationships. In our opinion, such hypotheses should best be approached through cross-species comparison revealing phylogenetically conserved—physiologically based—elements of reaction norms and the effects that range beyond those (Tammaru et al., 2015; Kivelä et al., 2020).
Adaptive value of the seasonal differences in developmental schedules of A. levana is not obvious (see Morehouse et al., 2013, for discussion). On average, in the field, the individuals of the diapausing generation are clearly smaller (Reinhardt, 1984; personal observations of the authors from the study area) than their directly developing offspring, which have their adult period in summer. As the adaptive explanation, it has been proposed that summer generation adults of A. levana (like several other butterflies) are selected for higher mobility (Fric and Konvička, 2002; Fric et al., 2006). Additionally, seasonal differences in larval mortality rates have a strong potential to affect generation-specific optima in body sizes: under high predation risk, attempting to grow large should be selected against because both of increased cost of spending more time in the vulnerable larval stage and the higher cost of being large (and therefore more apparent) per se (Remmel and Tammaru, 2009; Teder et al., 2010; Remmel et al., 2011). This explanation is, however, hardly applicable to explain seasonal differences in body size in A. levana as the larvae attain larger sizes when growing in June, which is the time when overall larval mortality rates are expected to peak in boreal forest landscapes (Remmel et al., 2009). The mortality hypothesis could, nevertheless, be used to explain the longer developmental periods of the diapausing individuals: it is most likely safer to spend a few extra days as a larva in late August compared to June. The benefits of having a longer development period in the diapausing individuals can be associated with acquiring immunological advantage (as shown for A. levana in particular: Vilcinskas and Vogel, 2016; Baudach et al., 2018; Freitak et al., 2019) or facilitation of other physiological processes needed to prepare the insect for an 8-month-long diapause (Hahn and Denlinger, 2007, 2011; Wang et al., 2007; Lehmann et al., 2016).
In the lower temperature treatment, we were able to reproduce the natural pattern of higher pupal masses of the directly developing generation. These data are therefore usable for the analysis of the proximate basis of attaining a size difference. We see that the larger final size of directly developing insects should be attributed to their higher growth rates but not to longer growing periods. This pattern differs from the emerging general picture according to which, when “needed,” larger sizes of insects are attained through prolonging growth periods and not through increasing instantaneous growth rates. This applies to comparisons among sexes (Tammaru et al., 2010; Teder et al., 2014; Sõber et al., 2019), populations within species (Vellau et al., 2013; Meister et al., 2018), and also seasonal generations (Esperk et al., 2013). Our comparison of seasonal generations in A. levana broadens the view indicating that exceptions to this rule can exist. We propose that the situation in A. levana can be reconciled with the general picture assuming that here natural selection has targeted developmental periods specifically rather than body sizes. This view is corroborated by the much clearer and consistent among-generation differences in developmental periods than larval sizes as revealed by the present study. The longer development periods of the diapause development larvae are paralleled by similar observations in some other butterflies (Wiklund et al., 1991; Aalberg Haugen et al., 2012; Kivelä et al., 2019) and are predicted by some models (Kivelä et al., 2013). As the perhaps most intuitive explanation, such long developmental periods may be selected for to facilitate physiological preparation for overwintering, whereas warning appearance and group living habits in A. levana—likely reducing larval mortality rates—may be seen as permissive for such a strategy to evolve. As an aside, the conclusion about flexibility of larval growth rates in A. levana is supported also by sex-related difference in this variable, detected in this study (c.f., Tammaru et al., 2010; Stillwell et al., 2014; Sõber et al., 2019).
It is notable that changes specific to developmental pathway were not limited to the final larval instar. Directly developing and overwintering insects differed in instar-specific growth periods, growth rates, and body sizes also in the penultimate instar. This result may be seen as corroborating the view that developmental constraints preclude considerable changes in larval growth parameters at the level of one larval instar (Tammaru, 1998; Kivelä et al., 2020), and any major differences have to be accumulated through modifying development during a number larval instars. This is, however, in some contrast with the observation that changes specific to developmental pathway are limited to the last larval instar in some other butterflies (Friberg et al., 2012; Kivelä et al., 2019).
In conclusion, the present study shows that controlled laboratory experiments comparing the growth schedules of different seasonal generations of insects can yield data usable for the analysis of seasonal adaptations, which largely provide the framework for life-history evolution in seasonal environments. Moreover, in a more general context, such comparisons yield information about what can and what cannot be plastically modified in the growth curve of an insect (Tammaru et al., 2015; Kivelä et al., 2020).
Data Availability Statement
The data is now published and could be found here: https://doi.org/10.5061/dryad.xksn02vfh.
Author Contributions
TE and TT contributed roughly equally to all stages of manuscript preparation. Both authors contributed to the article and approved the submitted version.
Funding
This study was supported by the Estonian Research Council grant PRG741.
Conflict of Interest
The authors declare that the research was conducted in the absence of any commercial or financial relationships that could be construed as a potential conflict of interest.
Acknowledgments
We thank Karmen Süld, Madle Timm, Killu Timm, Kristiina Ehapalu, Katre Hirv, Taavet Kukk, Getter Kala, and Daniel Valdma for the assistance in the laboratory.
Supplementary Material
The Supplementary Material for this article can be found online at: https://www.frontiersin.org/articles/10.3389/fevo.2021.612330/full#supplementary-material
References
Aalberg Haugen, I. M., Berger, D., and Gotthard, K. (2012). The evolution of alternative developmental pathways: footprints of selection on life-history traits in a butterfly. J. Evol. Biol. 25, 1377–1388. doi: 10.1111/j.1420-9101.2012.02525.x
Angilletta, M. J. Jr., Steury, T. D., and Sears, M. W. (2004). Temperature, growth rate, and body size in ectotherms: fitting pieces of a life-history puzzle. Integr. Compar. Biol. 44, 498–509. doi: 10.1093/icb/44.6.498
Arendt, J. D. (1997). Adaptive intrinsic growth rates: an integration across taxa. Q. Rev. Biol. 72, 149–177. doi: 10.1086/419764
Atkinson, D. (1994). Temperature and organism size a biological law for ectotherms. Adv. Ecol. Res. 25, 1–58. doi: 10.1016/S0065-2504(08)60212-3
Atkinson, D. (1996). “Ectotherm life-history responses to developmental temperature,” in Animals and Temperature: Phenotypic and Evolutionary Adaptation, Society for Experimental Biology Seminar Series, eds I. Johnston and A. Bennett (Cambridge: Cambridge University Press), 183–204. doi: 10.1017/CBO9780511721854.009
Atkinson, D., and Sibly, R. M. (1997). Why are organisms usually bigger in colder environments? Making sense of a life history puzzle. Trends Ecol. Evol. 12, 235–239. doi: 10.1016/S0169-5347(97)01058-6
Baudach, A., Lee, K.-Z., Vogel, H., and Vilcinskas, A. (2018). Immunological larval polyphenism in the map butterfly Araschnia levana reveals the photoperiodic modulation of immunity. Evol. Ecol. 8, 4891–4898. doi: 10.1002/ece3.4047
Berger, D., Friberg, M., and Gotthard, K. (2011). Divergence and ontogenetic coupling of larval behaviour and thermal reaction norms in three closely related butterflies. Proc. R. Soc. Lond. Ser. B Biol. Sci. 278, 313–320. doi: 10.1098/rspb.2010.1398
Berger, D., and Gotthard, K. (2008). Time stress, predation risk and diurnal-nocturnal foraging trade-offs in larval prey. Behav. Ecol. Sociobiol. 62, 1655–1663. doi: 10.1007/s00265-008-0594-4
Blanckenhorn, W. U. (2000). The evolution of body size: what keeps organisms small? Q. Rev. Biol. 75, 385–407. doi: 10.1086/393620
Chelini, M.-C., Delong, J. P., and Hebets, E. A. (2019). Ecophysiological determinants of sexual size dimorphism: integrating growth trajectories, environmental conditions, and metabolic rates. Oecologia 191, 61–71. doi: 10.1007/s00442-019-04488-9
Danks, H. V. (1987). Insect Dormancy: an Ecological Perspective. Ottawa: Biological Survey of Canada.
Dmitriew, C. M. (2011). The evolution of growth trajectories: what limits growth rate? Biol. Rev. 86, 97–116. doi: 10.1111/j.1469-185X.2010.00136.x
Esperk, T., Stefanescu, C., Teder, T., Wiklund, C., Kaasik, A., and Tammaru, T. (2013). Distinguishing between anticipatory and responsive plasticity in a seasonally polyphenic butterfly. Evol. Ecol. 27, 315–332. doi: 10.1007/s10682-012-9598-7
Esperk, T., and Tammaru, T. (2004). Does the ‘investment principle’ model explain moulting strategies in lepidopteran larvae? Physiol. Entomol. 29, 56–66. doi: 10.1111/j.1365-3032.2004.0365.x
Freitak, D., Tammaru, T., Sandre, S.-L., Meister, H., and Esperk, T. (2019). Longer life span is associated with elevated immune activity in a seasonally polyphenic butterfly. J. Evol. Biol. 32, 653–665. doi: 10.1111/jeb.13445
Friberg, M., Aalberg Haugen, I. M., Dahlerus, J., Gotthard, K., and Wiklund, C. (2011). Asymmetric life-history decision-making in butterfly larvae. Oecologia 165, 301–310. doi: 10.1007/s00442-010-1804-0
Friberg, M., Dahlerus, J., and Wiklund, C. (2012). Strategic larval decision-making in a bivoltine butterfly. Oecologia 169, 623–635. doi: 10.1007/s00442-011-2238-z
Friberg, M., and Karlsson, B. (2010). Life-history polyphenism in the map butterfly (Araschnia levana): developmental constraints versus season-specific adaptations. Evol. Ecol. Res. 12, 603–615.
Fric, Z., Klimova, M., and Konvička, M. (2006). Mechanical design indicates differences in mobility among butterfly generations. Evol. Ecol. Res. 8, 1511–1522.
Fric, Z., and Konvička, M. (2002). Generations of the polyphenic butterfly Araschnia levana differ in body design. Evol. Ecol. Res. 4, 1017–1032.
Hahn, D. A., and Denlinger, D. L. (2007). Meeting the energetic demands of insect diapause: nutrient storage and utilization. J. Insect Physiol. 53, 760–773. doi: 10.1016/j.jinsphys.2007.03.018
Hahn, D. A., and Denlinger, D. L. (2011). Energetics of insect diapause. Annu. Rev. Entomol. 56, 103–121. doi: 10.1146/annurev-ento-112408-085436
Ihalainen, E., and Lindstedt, C. (2012). Do avian predators select for seasonal polyphenism in the European map butterfly Araschnia levana (Lepidoptera: Nymphalidae)? Biol. J. Linnean Soc. 106, 737–748. doi: 10.1111/j.1095-8312.2012.01922.x
Kivelä, S. M., Davis, R. B., Esperk, T., Gotthard, K., Mutanen, M., Valdma, D., et al. (2020). Comparative analysis of larval growth in Lepidoptera reveals instar-level constraints. Funct. Ecol. 34, 1391–1403. doi: 10.1111/1365-2435.13556
Kivelä, S. M., Gotthard, K., and Lehmann, P. (2019). Developmental plasticity in metabolism but not in energy reserve accumulation in a seasonally polyphenic butterfly. J. Exper. Biol. 222:jeb202150. doi: 10.1242/jeb.202150
Kivelä, S. M., Svensson, B., Tiwe, A., and Gotthard, K. (2015). Thermal plasticity of growth and development varies adaptively among alternative developmental pathways. Evolution 69, 2399–2413. doi: 10.1111/evo.12734
Kivelä, S. M., Välimäki, P., and Gotthard, K. (2013). Seasonality maintains alternative life-history phenotypes. Evolution 67, 3145–3160. doi: 10.1111/evo.12181
Koštál, V. (2006). Eco-physiological phases of insect diapause. J. Insect Physiol. 52, 113–127. doi: 10.1016/j.jinsphys.2005.09.008
Leather, S. R., Walters, K. F. A., and Bale, J. S. (1993). The Ecology of Insect Overwintering. Cambridge: Cambridge University Press.
Lehmann, P., Pruisscher, P., Posledovich, D., Carlsson, M., Käkelä, R., Tang, P., et al. (2016). Energy and lipid metabolism during direct and diapause development in a pierid butterfly. J. Exper. Biol. 219, 3049–3060. doi: 10.1242/jeb.142687
Littell, R. C., Milliken, G. A., Stroup, W. W., Wolfinger, R. D., and Schabenberger, O. (2006). SAS for Mixed Models, 2nd Edn, Cary: SAS Institute.
Meister, H., Hämäläinen, R., Valdma, D., Martverk, M., and Tammaru, T. (2018). How to become larger: ontogenetic basis of among-population size differences in a moth. Entomol. Exper. Appl. 166, 4–16. doi: 10.1111/eea.12634
Meister, H., Esperk, T., Välimäki, P., and Tammaru, T. (2017a). Evaluating the role and measures of juvenile growth rate: latitudinal variation in insect life histories. Oikos 126, 1726–1737. doi: 10.1111/oik.04233
Meister, H., Tammaru, T., Sandre, S.-L., and Freitak, D. (2017b). Sources of variance in immunological traits: evidence of congruent latitudinal trends across species. J. Exper. Biol. 220, 2606–2615. doi: 10.1242/jeb.154310
Morehouse, N. I., Mandon, N., Christides, J. P., Body, M., Bimbard, G., and Casas, J. (2013). Seasonal selection and resource dynamics in a seasonally polyphenic butterfly. J. Evol. Biol. 26, 175–185. doi: 10.1111/jeb.12051
Nylin, S. (2013). Induction of diapause and seasonal morphs in butterflies and other insects: knowns, unknowns and the challenge of integration. Physiol. Entomol. 38, 96–104.
Remmel, T., Davison, J., and Tammaru, T. (2011). Quantifying predation on folivorous insect larvae: the perspective of life-history evolution. Biol. J. Linnean Soc. 104, 1–18. doi: 10.1111/j.1095-8312.2011.01721.x
Remmel, T., and Tammaru, T. (2009). Size-dependent predation risk in tree-feeding insects with different colouration strategies: a field experiment. J. Anim. Ecol. 78, 973–980. doi: 10.1111/j.1365-2656.2009.01566.x
Remmel, T., Tammaru, T., and Mägi, M. (2009). Seasonal mortality trends in tree-feeding insects: a field experiment. Ecol. Entomol. 34, 98–106. doi: 10.1111/j.1365-2311.2008.01044.x
Roff, D. A. (1992). The Evolution of Life Histories. Theory and Analysis. New York, NY: Chapman and Hall.
Ruf, C. (2002). Social Life-Styles in Caterpillars: Behavioral Mechanisms and Ecological Consequences. Bayreuth: Universität Bayreuth.
Saunders, D. S. (2020). Dormancy, diapause, and the role of the circadian system in insect photoperiodism. Annu. Rev. Entomol. 65, 373–389. doi: 10.1146/annurev-ento-011019-025116
Simpson, S. J., Sword, G. A., and Lo, N. (2011). Polyphenism in insects. Curr. Biol. 21, R738–R749. doi: 10.1016/j.cub.2011.06.006
Sõber, V., Sandre, S.-L., Esperk, T., Teder, T., and Tammaru, T. (2019). Ontogeny of sexual size dimorphism revisited: females grow for a longer time and also faster. PLoS One 14:e0215317. doi: 10.1371/journal.pone.0215317
Stillwell, R. C., and Davidowitz, G. (2010). A developmental perspective on the evolution of sexual size dimorphism of a moth. Proc. R. Soc. Lond. Ser. B Biol. Sci. 277, 2069–2074. doi: 10.1098/rspb.2009.2277
Stillwell, R. C., Daws, A., and Davidowitz, G. (2014). The ontogeny of sexual size dimorphism of a moth: when do males and females grow apart? PLoS One 9:e106548. doi: 10.1371/journal.pone.0106548
Tammaru, T. (1998). Determination of adult size in a folivorous moth: constraints at instar level? Ecol. Entomol. 23, 80–89. doi: 10.1046/j.1365-2311.1998.00106.x
Tammaru, T., and Esperk, T. (2007). Growth allometry of immature insects: larvae do not grow exponentially. Funct. Ecol. 21, 1099–1105. doi: 10.1111/j.1365-2435.2007.01319.x
Tammaru, T., Esperk, T., Ivanov, V., and Teder, T. (2010). Proximate sources of sexual size dimorphism in insects: locating constraints on larval growth schedules. Evol. Ecol. 24, 161–175. doi: 10.1007/s10682-009-9297-1
Tammaru, T., Vellau, H., Esperk, T., and Teder, T. (2015). Searching for constraints by cross-species comparison: reaction norms for age and size at maturity in insects. Biol. J. Linnean Soc. 114, 296–307. doi: 10.1111/bij.12417
Tauber, M. J., Tauber, C. A., and Masaki, S. (1986). Seasonal Adaptations of Insects. Oxford: Oxford University Press.
Teder, T., Esperk, T., Remmel, T., Sang, A., and Tammaru, T. (2010). Counterintuitive size patterns in bivoltine moths: late-season larvae grow larger despite lower food quality. Oecologia 162, 117–125. doi: 10.1007/s00442-009-1439-1
Teder, T., and Tammaru, T. (2005). Sexual size dimorphism within species increases with body size in insects. Oikos 108, 321–328. doi: 10.1111/j.0030-1299.2005.13609.x
Teder, T., Vellau, H., and Tammaru, T. (2014). Age and size at maturity: a quantitative review of diet-induced reaction norms in insects. Evolution 68, 3217–3228. doi: 10.1111/evo.12518
Tolman, T., and Lewington, R. (2008). Collins Butterfly Guide. The Most Complete Guide to the Butterflies of Britain and Europe. London: HarperCollins.
Vellau, H., Leppik, E., Frerot, B., and Tammaru, T. (2013). Detecting a difference in reaction norms for size and time at maturation: pheromone strains of the European corn borer (Ostrinia nubilalis: Lepidoptera: Crambidae). Evol. Ecol. Res. 15, 589–599.
Vendl, T., Šípek, P., Kouklík, O., and Kratochvíl, L. (2018). Hidden complexity in the ontogeny of sexual size dimorphism in male-larger beetles. Nat. Sci. Rep. 8:5871. doi: 10.1038/s41598-018-24047-1
Vilcinskas, A., and Vogel, H. (2016). Seasonal phenotype-specific transcriptional reprogramming during metamorphosis in the European map butterfly Araschnia levana. Evol. Ecol. 6, 3476–3485. doi: 10.1002/ece3.2120
Wang, X.-P., Yang, Q., Zhou, X.-M., Zhao, F., and Lei, C. (2007). Effect of photoperiod associated with diapause induction on the accumulation of metabolites in Sericinus montelus (Lepidoptera: Papilionidae). Appl. Entomol. Zool. 42, 419–424. doi: 10.1303/aez.2007.419
Whitman, D. W., and Agrawal, A. A. (2009). “What is phenotypic plasticity and why is it important?,” in Phenotypic Plasticity in Insects. Mechanisms and Consequences, eds D. W. Whitman and T. N. Antakrishnan (Einfeld: Science Publishers), 1–63. doi: 10.1201/b10201
Keywords: reaction norms, age and size at maturity, photoperiodism, overwintering, Nymphalidae, insects, sexual size dimorphism
Citation: Esperk T and Tammaru T (2021) Ontogenetic Basis of Among-Generation Differences in Size-Related Traits in a Polyphenic Butterfly. Front. Ecol. Evol. 9:612330. doi: 10.3389/fevo.2021.612330
Received: 30 September 2020; Accepted: 25 January 2021;
Published: 01 March 2021.
Edited by:
Olivia Roth, GEOMAR Helmholtz Centre for Ocean Research Kiel, GermanyReviewed by:
Jofre Carnicer, University of Barcelona, SpainCarita Lindstedt, University of Jyväskylä, Finland
Copyright © 2021 Esperk and Tammaru. This is an open-access article distributed under the terms of the Creative Commons Attribution License (CC BY). The use, distribution or reproduction in other forums is permitted, provided the original author(s) and the copyright owner(s) are credited and that the original publication in this journal is cited, in accordance with accepted academic practice. No use, distribution or reproduction is permitted which does not comply with these terms.
*Correspondence: Toomas Esperk, dG9tZUB1dC5lZQ==