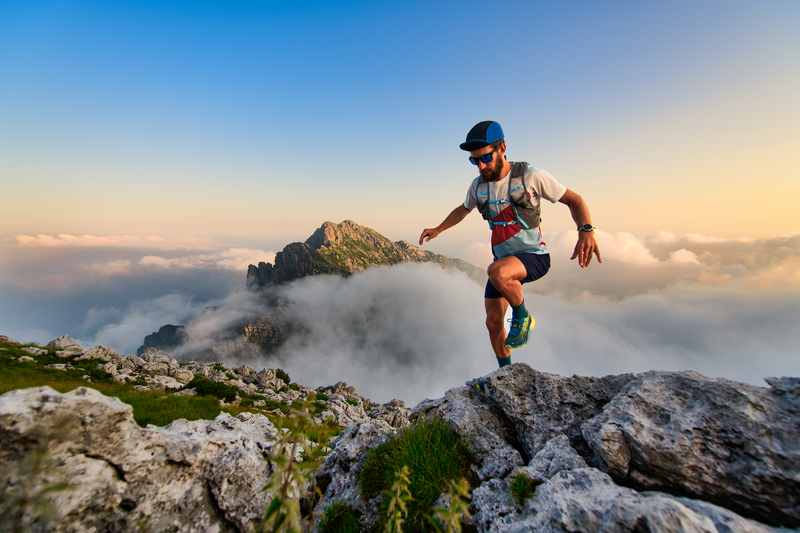
95% of researchers rate our articles as excellent or good
Learn more about the work of our research integrity team to safeguard the quality of each article we publish.
Find out more
ORIGINAL RESEARCH article
Front. Ecol. Evol. , 17 May 2021
Sec. Conservation and Restoration Ecology
Volume 9 - 2021 | https://doi.org/10.3389/fevo.2021.592027
This article is part of the Research Topic Marine Ecosystem Assessment for the Southern Ocean: Meeting the Challenge for Conserving Earth Ecosystems in the Long Term View all 25 articles
Within the framework of the Marine Ecosystem Assessment for the Southern Ocean (MEASO), this paper brings together analyses of recent trends in phytoplankton biomass, primary production and irradiance at the base of the mixed layer in the Southern Ocean and summarises future projections. Satellite observations suggest that phytoplankton biomass in the mixed-layer has increased over the last 20 years in most (but not all) parts of the Southern Ocean, whereas primary production at the base of the mixed-layer has likely decreased over the same period. Different satellite models of primary production (Vertically Generalised versus Carbon Based Production Models) give different patterns and directions of recent change in net primary production (NPP). At present, the satellite record is not long enough to distinguish between trends and climate-related cycles in primary production. Over the next 100 years, Earth system models project increasing NPP in the water column in the MEASO northern and Antarctic zones but decreases in the Subantarctic zone. Low confidence in these projections arises from: (1) the difficulty in mapping supply mechanisms for key nutrients (silicate, iron); and (2) understanding the effects of multiple stressors (including irradiance, nutrients, temperature, pCO2, pH, grazing) on different species of Antarctic phytoplankton. Notwithstanding these uncertainties, there are likely to be changes to the seasonal patterns of production and the microbial community present over the next 50–100 years and these changes will have ecological consequences across Southern Ocean food-webs, especially on key species such as Antarctic krill and silverfish.
Primary production by microalgae communities is the foundation of Southern Ocean food-webs (Deppeler and Davidson, 2017; Boyd et al., 2019), providing the organic matter that ultimately sustains Antarctica’s unique marine ecosystems (Loeb et al., 1997; Atkinson et al., 2004; Hill et al., 2006; Mock et al., 2017; Boyd et al., 2019; McCormack et al., in review). There are also important feedbacks between the microbial communities and Southern Ocean biogeochemistry, carbon sequestration and the global climate system (Siegenthaler and Sarmiento, 1993; Meskhidze and Nenes, 2006; Sarmiento, 2013; McKinley et al., 2017; Henson et al., 2019; Moreau et al., 2020).
Increases of atmospheric carbon dioxide (CO2) from ∼400 μatm today beyond 750 μatm by 2100 will likely lead to multifaceted environmental change in the Southern Ocean, including upper-ocean warming, ocean acidification (OA), changes to incident irradiance, increased vertical mixing in the water column, less sea-ice and changed patterns of nutrient input (including iron) (IPCC, 2019; Henley et al., 2020). These environmental and oceanographic changes will affect microbial community composition, patterns of primary production and ecological pathways in Southern Ocean marine ecosystems (Le Quéré et al., 2016; Schofield et al., 2017; Deppeler and Davidson, 2017; Freeman et al., 2019; Johnston et al., in review).
Despite the importance of projecting future changes to primary production and the microbial community composition of the Southern Ocean, current modelling methods have high uncertainty (Leung et al., 2015; IPCC, 2019). The response of the Southern Ocean microbial community to multiple environmental drivers is complex and poorly understood (Petrou et al., 2016; Deppeler and Davidson, 2017). Unlike most of the world’s oceans, the vast majority of the Southern Ocean is considered to be replete with nitrate and instead, silicate and iron are limiting in some areas and seasons (Banse, 1996; Boyd et al., 2012, 2001; Hiscock et al., 2003; Doblin et al., 2011). Irradiance can also be crucial to primary production (Deppeler and Davidson, 2017; Kim et al., 2018) and microbial community composition (Arrigo et al., 2010; Kropuenske et al., 2010; Van de Poll et al., 2011; Trimborn et al., 2017) in the Southern Ocean, and irradiance, iron and nutrient availability interact in ways not fully understood at present (Strzepek et al., 2012; Luxem et al., 2017; Trimborn et al., 2019). Further complexities arise from co-limitation by iron and other micronutrients (e.g., Mn; Pausch et al., 2019). Microzooplankton grazing, which can reduce NPP by facilitating nutrient loss through the sinking of particulate detritus (e.g., Cadée et al., 1992; Perissinotto and Pakhomov, 1998; Vernet et al., 2011), will be affected by climate change through changes to grazing rates (Sarmento et al., 2010; Caron and Hutchins, 2013; Behrenfeld, 2014; Biermann et al., 2015; Cael and Follows, 2016) and phytoplankton nutrient density (Finkel et al., 2010; Hixson and Arts, 2016).
Environmental changes driving these complex biological responses are, in themselves, complex and not well observed. For example, shoaling of the mixed-layer due to decreased vertical mixing can simultaneously increase mixed-layer irradiance, increase grazing, increase ultraviolet-B damage, decrease nutrient supply, and reduce ‘living space’ (Garibotti et al., 2005; Vernet et al., 2008; Moreau et al., 2010; Boyd et al., 2014; Zhu et al., 2016; Hoppe et al., 2017; Llort et al., 2019; Brown et al., 2019; Höfer et al., 2019). However, vertical mixing is not the only iron supply mechanism operating in the Southern Ocean; iron and nutrients (such as silicate) can be introduced into the upper water column by meltwater (from icebergs, sea ice, and glaciers), atmospheric input of iron aerosols, hydrothermal vents, and microbial recycling – Blain et al. (2007), Cassar et al. (2007), Pollard et al. (2009), Boyd and Ellwood (2010), Tagliabue et al. (2010), Treguer (2014), Lannuzel et al. (2016), Boyd (2019), and Hopwood et al. (2019).
Within the framework of the Marine Ecosystem Assessment for the Southern Ocean (MEASO), we present new analyses of the spatial and seasonal patterns of near-surface chlorophyll-a concentration (chl-a) and satellite-based proxies of primary production in the Southern Ocean from Earth-observing satellites, and relate this to information from ships, shore-stations, and autonomous instruments. We consider both primary production in the surface mixed-layer (from the surface to the depth of the seasonal pycnocline, at ∼50–150 m) and in the ‘deep chlorophyll maximum’ (DCM; Cullen, 2015; Carranza et al., 2018; Uchida et al., 2019). New approaches to track changes in primary production in the DCM based on satellite observations are proposed. Finally, information on future changes to primary producers from global Earth-system models are presented and discussed in the context of our present understanding of the role of multiple-drivers of changes to primary production and our ability to observe, combine and model these drivers.
Both satellite observations of phytoplankton biomass (proxy of chl-a) and satellite-based models were used to describe recent changes to Southern Ocean net primary production (Table 1). There have been many comparisons between in situ and satellite estimates of chl-a in the Southern Ocean. In reprise, some studies conclude that the ‘default’ (i.e., globally tuned) satellite algorithm for chl-a should be adjusted to improve accuracy in the Southern Ocean (Johnson et al., 2013; Jena, 2017), whereas other studies have found that the default global chl-a algorithm was appropriate for the Southern Ocean (Arrigo et al., 2008; Haentjens et al., 2017; Moutier et al., 2019; Del Castillo et al., 2019). The reduction in absolute uncertainty in chl-a from adjusting the algorithm for the Southern Ocean tends to be small (e.g., Johnson et al., 2013) and the effect on trends will likely be even smaller, so we used the global default chl-a algorithm for SeaWiFS and MODIS-Aqua, and blended these as described in Pinkerton (2019).
For the same period, estimates of NPP are available from two widely used models: the Vertically Generalised Production Model, VGPM, Behrenfeld and Falkowski, 1997) and the Carbon Based Production Model (CBPM, Behrenfeld et al., 2005; Westberry et al., 2008). Alternative primary production models specifically developed for the Southern Ocean have been developed (e.g., Arrigo et al., 2008; Moreau et al., 2015) but data are not widely available and validation is scare. The accuracy of VGPM and CBPM data in the Southern Ocean is not known, so a (non-exhaustive) review of the existing NPP literature was carried out for preliminary comparison. A total of 573 measurements of (almost exclusively summer) primary production were sourced from 24 studies and each was assigned to a MEASO zone and sector based on geographical location (Table 2) and compared to satellite-based NPP estimates.
Table 2. Spring-summer integrated net primary production rates (gC m–2 d–1) retrieved from the literature and grouped by MEASO sectors and zones.
To explore changes in the productivity of phytoplankton at the base of the mixed layer in deep chlorophyll maxima (DCM) we propose a novel metric: irradiance at the base of the mixed layer (EDCM, Equation 2).
Both broadband incident irradiance at the sea-surface (par) and diffuse downwelling attenuation (kpar) were obtained from satellite observations, and estimates of mixed layer depth (mld) were provided from a data-assimilating hydrographic model (Table 1). Based on instrumented elephant seals in the Southern Ocean, Carranza et al. (2018) showed that DCM tends to occur close to the base of the mixed layer defined using a potential density threshold criterion of 0.03 kg m–3. Hence, mixed layer depth (zm) was obtained from GLBu0.08 hindcast results (sourced from orca.science.oregonstate.edu) using a potential density difference of 0.03 kg m–3 from the surface (Metzger et al., 2007; Chassignet et al., 2007; Wallcraft et al., 2009). A constant diffuse sea-surface reflectivity (ρs) of 0.07 was assumed (Campbell and Aarup, 1989).
The rationale behind this formulation is that high attenuation in the mixed layer makes it less likely that a DCM is present and vice versa. Essentially, either phytoplankton are distributed relatively evenly through the mixed layer (no DCM, higher mixed-layer attenuation), or are present in a narrow band of elevated concentration at the base of the mixed layer (typically co-located with the nutricline: Cullen, 2015) forming a DCM below a relatively oligotrophic mixed-layer. It is difficult to forecast which of these will occur without good knowledge of the factors involved (inter alia water column structure, nutrient supply/demand, incident irradiance, photoadaptive-capability of phytoplankton species present, loss terms including grazing and sinking; Parslow et al., 2001; Kemp et al., 2006; Cullen, 2015; Carranza et al., 2018; Uchida et al., 2019), but we hypothesize that satellite data may be able to tell us which of these situations has occurred after the event.
We recognize that although irradiance at the base of the mixed-layer is likely to be a key factor affecting whether a DCM exists and the level of primary productivity in the DCM (Cullen, 2015), productivity and biomass of phytoplankton at depth will be affected by other factors, such as phytoplankton composition, nutrient concentrations and temperature (Sallée et al., 2015). In addition, mixed layers can contain vertical structure in optical properties (Carranza et al., 2018). To evaluate the utility of EDCM as an indicator of DCM, a comparison was made between EDCM and the amount of phytoplankton biomass in the DCM from three Southern Ocean Carbon and Climate Observations and Modeling (SOCOMM project; Riser et al., 2018; Uchida et al., 2019) profiling drifters between 2013 and 2018. Phytoplankton biomass in the DCM was proxied from these float measurements as the depth-integrated phytoplankton carbon biomass (Cp) minus the surface phytoplankton carbon biomass multiplied by the mixed layer depth, Cp(surf), following Uchida et al. (2019).
Spatial variability in primary productivity was summarised using the MEASO spatial framework (Constable et al., in review; Supplementary Information). Briefly, MEASO defines five longitudinal sectors, three latitudinal zones (Northern between Subtropical and Subantarctic fronts; Subantarctic between Subantarctic and Southern Antarctic Circumpolar Current (ACC) fronts; and Antarctic south of Southern ACC front), and 15 areas from the sector-zone intersects.
Although autonomous profilers are delivering increasingly powerful datasets (e.g., Boyer et al., 2013; Buongiorno Nardelli et al., 2017; Uchida et al., 2019), large-area (Southern Ocean scale) and long-term (decadal) observations of environmental change in the Southern Ocean are predominantly from Earth-observation satellites (e.g., Cavalieri et al., 1990; Reynolds et al., 2002; Haentjens et al., 2017; Del Castillo et al., 2019), sometimes in conjunction with data-assimilating hydrodynamic models (e.g., Metzger et al., 2007; Chassignet et al., 2007; Wallcraft et al., 2009). To describe the oceanographic and environmental setting, this study focused on 4 key environmental data sets for which large-area, spatially resolved and long-term information is available (Table 1): sea-surface temperature (sst); sea ice concentration (ice); mixed layer depth (mld); and photosynthetically active radiation at the sea surface (par). Together, these describe major environmental drivers of change in the Southern but clearly do not capture all factors relevant to primary production, including nutrient supply mechanisms, acidification and grazing.
Linear trends in monthly anomalies (differences from climatological means) for each dataset (chl, sst, ice, mld, par, vgpm) at the pixel level (smallest sampling scale) were determined using the Sen slope (Sen, 1968). This value is the median slope of all pairs of points in the time series. The insensitivity of the Sen slope to outliers means that it is generally the preferred non-parametric method for estimating a linear trend (Hipel and McLeod, 1994). Seasonal trends were calculated using anomalies from three months (spring: September–November; summer: December–February; autumn: March–May; winter: June–August).
The Sen slope was also used to describe trends at the scale of MEASO zones, sectors and areas. Satellite-based estimates of chl-a and NPP fail at low solar elevations, and we have not attempted to “fill in” the winter gaps in satellite data (e.g., as Park et al., 2019). The proportion of missing data increases with latitude and so to avoid any potential bias in area-averages, trends were only calculated when more than half of potential observations for an area were present. The statistical significance of trends was assessed using the non-parametric Mann-Kendall test (Mann, 1945; Kendall, 1975) which does not require a normal distribution assumption. We used the method of Yue and Wang (2004) to adjust the effective number of degrees of freedom for autocorrelation.
The influence of four environmental drivers (sst, ice, mld, par) on chl-a was considered by a multiple linear regression (Equation 1), where the a coefficients minimize the sum of squares of the error (ε). This analysis was not applied to satellite estimates of primary production (vgpm, cbpm or EDCM) to avoid circularity: satellite measurements of chl-a are independent of observations of these environmental drivers but vgpm, cbpm and EDCM are not. Here, is the monthly anomaly in chl (and similarly for other variables).
An approximation to the contribution to overall trend (Sen slope) in chl-a from each candidate driver variable (sst, ice, mld, par) is given as the product of the respective linear coefficient (α) and the trend (Sen slope) of the variable. The analysis was carried out in IDL 8.5 (Research Systems Inc., Boulder, CO, United States).
Although our compilation of in situ measurements of depth-integrated NPP from research vessels and shore-stations was not exhaustive, it nevertheless shows a clear pattern of where primary production has been measured more often to date (Table 2) and highlights the need to improve knowledge of primary productivity in the entire East Indian sector as well as the Subantarctic and Northern zones where data are scarce or non-existent. The paucity of the NPP data meant that no robust conclusions could be drawn as to the relative accuracies of VGPM and CBPM. Both NPP models look to be overestimating NPP in the Northern zone by a similar amount of ∼40% (Figure 1) and underestimating NPP in the Subantarctic and Antarctic zones by 55 ± 15 % (mean ± standard deviation).
Figure 1. Depth-integrated, net primary productivity (NPP) in the Southern Ocean measured in situ from research vessels and estimated from satellite data: (A) Vertically Generalised Production Model (vgpm); and (B) Carbon Based Production Model (cbpm). Data are combined as MEASO areas (blue) and zones (orange, pink, green). Dashed lines indicate 1:1 correspondence.
Satellite observations (Figure 2A) show that chl-a was generally higher in Subantarctic areas, lower south of the Polar Front and that there were some areas of elevated productivity south of the southern limit of the ACC (especially in the Ross Sea and Bellingshausen Sea/western Antarctic Peninsula). Patterns of primary productivity estimated from satellite observations (Figures 2C,E) generally followed those of chl-a (higher values in Subantractic waters and over the Antarctic shelf) but there was a strong positive dependence of NPP on latitude. There were also significant differences in spatial variations of annual-average vgpm compared to cbpm, with vgpm higher to the north of the region, and cbpm higher to the south.
Figure 2. Long-term (1997-2019) mean values of indicators for primary production: (A) chlorophyll-a concentration (chl); (C) net primary production in the water column estimated by the VGPM algorithm (vgpm) and the CBPM algorithm (cbpm) (E). Linear trends (Sen slopes) over the same period are shown for (B) chl; (D) vgpm; (F) cbpm. White indicates missing data. Black lines are nominal positions of (from north): Subtropical Front (STF, solid line), Subantarctic Front (SAF, solid line), average maximum northern extent of seasonal sea ice (dashed line), and the Southern boundary of the Antarctic Circumpolar Current (SB-ACC, solid line).
Increasing trends in chl-a between 1997–2019 were detected by satellites in most Northern and Subantarctic zones (Figure 2B). In contrast, decreasing trends in chl-a were observed in most Antarctic continental shelf-sea waters, especially in the Ross Sea, Weddell Sea and Prydz Bay, except along the western Antarctic Peninsula. Trends in vgpm (Figure 2D) closely followed those in chl-a. Seasonal trend analysis of chl-a and vgpm (see Supplementary Information) shows predominantly positive trends in autumn and negative trends (over the Antarctic shelf) in summer. Trends in cbpm were negative throughout the Southern Ocean (Figure 2F), including in Northern and Subantarctic zones in contrast with vgpm. Seasonal analysis of trends in cbpm suggests that changes in productivity in the summer drive these overall trends.
In terms of environmental drivers of changes in chl-a, we found that only a small amount (mean 5.6%, 5th–95th percentile range 0.9–16.8%) of the monthly anomalies in chl-a over the last 20 years was explained by a linear combination of sst, ice, mld and par (Figure 3). The proportions of variance explained by the individual drivers were less than 2%, with sst explaining most and par the least. The linearized contribution to the trends in chl-a from these individual environmental drivers (Figure 4) showed little spatial structure and were typically small, less than 0.001 mg m–3 y–1, whereas the trends in chl-a were up to ∼0.01 mg m–3 y–1 in some areas of the Southern Ocean.
Figure 3. Proportion of variance explained (R2) in anomalies of chlorophyll-a concentration (chl) by a multiple linear regression of anomalies of environmental drivers: sea-surface temperature (sst), sea ice concentration (sea-ice), mixed layer depth (mld) and incident irradiance at the sea-surface (par). Other information as Figure 2.
Figure 4. Contribution to linear trends in chlorophyll-a concentration (chl) by environmental drivers: (A) sea-surface temperature (sst); (B) sea ice concentration (sea-ice); (C) mixed layer depth (mld); and (D) incident irradiance at the sea-surface (par). Other information as Figure 2.
The regression between irradiance at the base of the mixed layer and the amount of phytoplankton biomass in the DCM from three SOCCOM floats (Figure 5) was highly significant (F95 = 82.7, p < 0.001) with about half of the variance explained (R2 = 0.47).
Figure 5. Comparison in log-space between EDCM (irradiance at the base of the mixed layer as Equation 2) and a measure of phytoplankton carbon biomass in the deep chlorophyll maximum (DCM) from profiling floats (Cp-Cp(surf): see text for details).
Average EDCM values were low through most of the Southern Ocean except in a band close to the southern boundary of the ACC and especially in the Atlantic and Central Indian sectors (Figure 6A). High values of mean EDCM were also found in parts of the Northern zone of the Pacific sector. Trends in EDCM (Figure 6B) were negligible north of the northern limit of seasonal sea ice, and almost exclusively negative south of this. Decreasing trends in EDCM were greatest between longitude 0° and 60° (Atlantic and Central Indian sectors) and occurred almost exclusively during the summer months (December–February: see Supplementary Information).
Figure 6. Irradiance at the base of the mixed layer (EDCM) as a proxy for potential primary production in the deep chlorophyll maximum (DCM). (A) long-term (1997-2019) mean values; (B) linear trends (Sen slopes) over the same period. Other information as Figure 2.
Significant increasing trends in chl-a and vgpm between 1997–2019 were found in all MEASO zones and sectors except for chl-a in the Antarctic zone (Table 3). Increases were highest in the Atlantic and West Pacific sectors. Significant increasing trends were also found for many (chl-a) or most (vgpm) MEASO areas. Positive trends in vgpm were almost exclusively greater in magnitude and more significant than trends in chl-a (with the exception of AON and WPA areas). In contrast, all significant trends in cbpm were negative, and were highest in Subantarctic and Antarctic zones. For the Southern Ocean as a whole, the mean trend and significance in vgpm was 0.8 % y–1 (p < 0.0001) compared to 0.5 % y–1 for chl (p = 0.002), whereas the overall Southern Ocean trend in cbpm was negative (−0.5 % y–1) but not significant (p = 0.17). Significant trends in irradiance at the base of the mixed-layer (EDCM) over the same period were exclusively negative, and substantially larger than trends in chl-a and vgpm especially in the Subantarctic and Antarctic zones and in the Atlantic sector (trends > 5 % y–1 in magnitude). At the scale of the Southern Ocean, the Sen slope in EDCM was highly significant −3.2 % y–1 (p < 0.0001). Plots of time series by zones, sectors and area, and full statistics on trend analysis are given in Supplementary Information.
Table 3. Annual average mean values and long-term linear trends (1997–2019) for: phytoplankton biomass (chl-a concentration); net primary production (NPP) by the vertically-generalised production model (VGPM) and carbon-based production model (CBPM); and irradiance at the base of the mixed-layer (EDCM) proxy of primary production in the deep chlorophyll maximum (DCM).
The present study found that chl-a and NPP were higher in the Atlantic sector than in other sectors of the Southern Ocean, likely as a result of higher iron availability due to land proximity (de Baar et al., 1995; Banse, 1996), and productivity was also elevated around the Kerguelen Plateau and Balleny Islands, consistent with enhanced supply of iron and major nutrients to surface waters (Blain et al., 2007). Annual-average values of chl-a and NPP were also high in some parts of the Antarctic zone, especially Prydz Bay (CIA), Ross Sea (WPA), and Bellingshausen Sea/western Antarctic Peninsula (EPA), likely because of persistent polynyas (Arrigo et al., 2015).
In terms of trends in chl-a, our results agreed with Del Castillo et al. (2019) who found statistically significant increases in chl-a in all sectors of the Southern Ocean, with an especially strong increase in the Northern and Subantarctic zones. Primary production in these zones are seasonally limited by the availability of silicate availability, a constraint which favours phytoplankton communities made up of small flagellates coccolithophores, cyanobacteria, and dinoflagellates (Wright et al., 2010; Balch et al., 2011; Freeman et al., 2019). In these areas, upper-ocean warming in conjunction with higher pCO2 was anticipated to increase phytoplankton primary production (Steinacher et al., 2010; Boyd, 2019) which agrees with positive trends observed in chl-a (Figure 2).
Further south, silicate-rich waters in the southern Subantarctic and Antarctic zones tend to favour diatom-dominated communities (Petrou et al., 2016; Balch et al., 2016; Rembauville et al., 2017; Nissen et al., 2018; Trull et al., 2018), and here we found the trends in chl-a to be more mixed, with both increases and decreases over the last few decades. The Ross Sea was the main Antarctic area with negative trends in chl-a, but we are not aware of in situ measurements to confirm this.
To date, the most consistent long-term observations of phytoplankton and factors affecting primary production have been made from coastal research stations, notably along the coastal West Antarctic Peninsula as part of the Long Term Ecological Research Network (LTER; Montes-Hugo et al., 2009; Moreau et al., 2015; Kim et al., 2018; Brown et al., 2019). Over the 20-year LTER record, Kim et al. (2018) found significant positive trends in chl-a at some field stations on the Antarctic Peninsula but decreasing phytoplankton biomass at others. Our study shows increases in chl-a along the West Antarctic Peninsula (Figure 2) but the spatial scale of the satellite data is coarser and unreliable within a few km of the shore. Brown et al. (2019) found that increasing upper ocean stability along the West Antarctic Peninsula between 1993–2017 due to a combination of wind, sea ice and meltwater dynamics, led to enhanced primary production, especially of diatoms. It appears that local-scale forcing (e.g., changes to sea ice, glacier melting, changes to coastal current patterns), and large-scale climate cycles like El Niño Southern Oscillation (ENSO) and SAM both affect long-term change in Antarctic coastal productivity (Montes-Hugo et al., 2009; Schloss et al., 2014; Kim et al., 2018; Brown et al., 2019; Höfer et al., 2019).
It is notable that trends from vgpm were predominantly positive throughout the Southern Ocean whereas trends in cbpm were almost exclusively negative (compare Figures 2D,F). The vgpm data are based on chl-a, so it is expected that trends in chl and vgpm agree, whereas cbpm is based on satellite estimates of the C:Chl ratio and does not use chl-a per se. The paucity of in situ measurements of NPP in the Southern Ocean (see Table 2) means that we cannot empirically compare the accuracy of vgpm versus cbpm, or carry out independent trend analysis, but we note that the assumption of nitrate-limited phytoplankton production implicit in vgpm is unlikely to be valid for the Southern Ocean, so cbpm may simply be more reliable than vgpm. Alternatively, the different patterns of change in chl-a (and vgpm) compared to cbpm may be associated with a change in community composition. Laboratory and shipboard experiments show that the responses of phytoplankton to environmental changes such as these will be species-specific (Hoppe et al., 2013, 2017; Alvain and d’Ovidio, 2014; Trimborn et al., 2017; Andrew et al., 2019; Strzepek et al., 2019). We speculate here that whereas vgpm is essentially showing trends in phytoplankton biomass (via the proxy of chl-a), cbpm could also be responding to changes in the microbial community, at least in terms of size classes. Decreases in cbpm compared to vgpm in the Southern Ocean would be consistent with a shift towards smaller phytoplankton species at high latitudes at the expense of larger species, in line with some predictions (e.g., Rousseaux and Gregg, 2015; Petrou et al., 2016; Deppeler and Davidson, 2017; Trimborn et al., 2017) and observations (e.g., Moline et al., 2004), although changes in size structure vary spatially and with climatic variability such as SAM and ENSO (Montes-Hugo et al., 2008). Higher scattering efficiencies of smaller species would likely lead to lower satellite-estimates of Chl:C ratios and tend to give lower estimates of growth rates (μ) by the cbpm (Behrenfeld et al., 2005). This suggestion is unproven and warrants further research.
It is increasingly clear that the response of phytoplankton to multiple stressors acting concurrently cannot be obtained by superimposing their separate responses (Boyd and Brown, 2015; Zhu et al., 2016; Boyd et al., 2016; Luxem et al., 2017; Andrew et al., 2019; Strzepek et al., 2019; Trimborn et al., 2019; Boyd, 2019). Satellite observations in the Southern Ocean show complex patterns of environmental change; surface warming in much of the Northern zone contrasts with slight cooling trends over the last 40 years further south (Maheshwari et al., 2013; Kostov et al., 2016; Sallée, 2018); average sea ice concentration has decreased in the Amundsen Sea but increased in parts of the Weddell, Bellingshausen and Ross Seas (Vaughan et al., 2013; Zhang et al., 2018; Pinkerton, 2019; IPCC, 2019); irradiance at the sea-surface has increased north of the Subantarctic Front and generally reduced to the south over the last 20 years; over the same period, mixed-layer depths have likely shallowed in the Northern zone, and both deepened and shallowed in different parts of the Subantarctic and Antarctic zones (Leung et al., 2015; Pinkerton, 2019).
Given the multifaceted and non-linear response of phytoplankton to these environmental changes, it is perhaps unsurprising that the linear model with 4 forcing factors (sst, ice, mld and par) explained only a small fraction (5.6%) of the variance in chl-a over the Southern Ocean (Figure 3), and the individual environmental contributions to chl-a trends were negligible (Figure 4). Also, we recognize that satellite observations were not available for important factors including ocean pH, pCO2, and grazing, so these factors were not included as drivers in our empirical analyses of recent changes to chl-a.
Based on long-term sampling from research stations in the Antarctic peninsula region, Kim et al. (2018) showed that variability in chl-a is strongly linked to large-scale climate cycles (ENSO and SAM). Analyses of biogeochemical models are also providing similar insights into these climate-biology relationships acting over large areas (e.g., Hauck et al., 2015). Given the important effects of multi-decadal climate variability such as ENSO and SAM on patterns of primary production, Henson et al. (2010) estimated that ∼40 years of continuous satellite ocean−color data was needed to reliably ascribe any trends in chl-a and production to climate change rather than variability. Del Castillo et al. (2019) repeated the analysis for the Atlantic sector of the Southern Ocean and found that ∼34 years of continuous data were needed. We caution therefore that the satellite record is still not long enough to separate long-term trends from climate variability.
Deep chlorophyll maxima (DCM) can form and persist over several months in the Southern Ocean depending on factors including the state of the seasonal pycnocline and nutricline, rates of nutrient supply, incident irradiance, photoadaptation, grazing and sinking (Parslow et al., 2001; Kemp et al., 2006; Cullen, 2015; Carranza et al., 2018; Uchida et al., 2019). Phytoplankton in the DCM have elevated pigment concentrations, likely indicative of production being light-limited, though iron and silicic acid limitation may also be present (Parslow et al., 2001). The factors affecting when, where and how a deep phytoplankton bloom develops have been extensively studied (review by Cullen, 2015), and although observations generally agree with established hypotheses, the lack of accurate information on key drivers mean that forecasts of DCMs are unreliable (Uchida et al., 2019). For this reason, we presented a new approach to tracking changes in DCM in the Southern Ocean. Based on data from three SOCCOM floats in the Southern Ocean, about half the variability in the amount of phytoplankton biomass in the DCM was explained by our simple metric of irradiance at the base of the mixed layer (EDCM). The comparison shown in Figure 5 provides preliminary support for EDCM being a useful metric for tracking changes in DCMs in the Southern Ocean.
The new EDCM metric revealed a significant decrease in NPP at depth in the Antarctic sector of the Southern Ocean (Figure 6), but there were not co-located hotspots of trends in chl-a, diffuse irradiance attenuation, or mixed layer depth. The interpretation is that relatively small changes over time to attenuation and mixed-layer depth acting together can lead much more significant trends in EDCM. Further analysis of this preliminary result is recommended as variations in DCMs could have important consequences for ecosystems and biogeochemistry because of higher efficiencies of organic matter export from sub-surface primary production (Tilstone et al., 2017; Henley et al., 2020).
Based on Earth-systems models, the ‘Special Report on the Ocean and Cryosphere in a Changing Climate’ (IPCC, 2019; Meredith et al., 2019) included a summary of observed changes in NPP and drivers, and projected future changes. Future projections were based largely on the CMIP5 (Coupled Model Intercomparison Project) which used two Representative Concentration Pathways (RCPs): RCP2.6 (low greenhouse gas emission, high mitigation future) and RCP8.5 (high greenhouse gas emission scenario, ‘business as usual’, in the absence of policies to reduce climate change). Key conclusions from CMIP5 model projections of NPP (Leung et al., 2015; Meredith et al., 2019) were as follows:
• In the Northern MEASO zone, higher mean underwater irradiance (from reduced mixed-layer depths) and higher iron supply were projected. Overall, this projection points to increased primary production in the mixed-layer and increased phytoplankton biomass (Leung et al., 2015; Meredith et al., 2019). These model projections are consistent with recent observations provided in the current study (chl-a, vgpm) and elsewhere (e.g., Le Quéré et al., 2005; Doney, 2006; Del Castillo et al., 2019).
• In the Subantarctic zone, deeper summertime mixed-layer depth together with increased cloud albedo were projected to lead to lower average irradiance in summer, leading to lower chl-a and NPP (Leung et al., 2015). This result agreed with a modelling study by Moore et al. (2018) which found that changes to sea ice and circulation patterns under climate warming scenarios would lead to a global reorganization of nutrient distributions and a steady decline in global-scale marine biological production. However, recent satellite observations show recent increasing rather than decreasing trends in chl-a in this zone (Del Castillo et al., 2019; present study).
• In the Antarctic zone, CMIP5 models generally suggested that less seasonal sea ice and a warming ocean will lead to greater iron supply, higher underwater irradiance and thence to increases in chl-a and NPP (Leung et al., 2015; Rickard and Behrens, 2016). These projected increases agree with recent trends in chl-a and vgpm (present study), except in the Ross Sea where chl-a, vgpm and cbpm all show negative trends.
Confidence in future projections of chl-a and NPP remains low (IPCC, 2019). The strongest drivers of future changes in NPP in CMPI5 models were iron availability and irradiance; acidification and temperature per se were less important (Leung et al., 2015). The balance between different iron-supply mechanisms (i.e., vertical mixing versus aeolian versus ice-mediated supply) are hence crucial to our ability to forecast future changes to the productivity of the Southern Ocean (Boyd et al., 2012; Boyd et al., 2014; Leung et al., 2015; Hutchins and Boyd, 2016; Hopwood et al., 2019). High spatial and seasonal variability in the relative importance of various iron-supply mechanisms, coupled with a lack of long-term observations, the simultaneous change of a number of environmental conditions, and the complexities of phytoplankton response to different environmental drivers hence severely limit our ability to anticipate these changes (Hutchins and Boyd, 2016; Mongwe et al., 2018; Freeman et al., 2019; Smith et al., 2019; Hopwood et al., 2019). In the longer term, longer-time series of satellite observations, increasingly sophisticated laboratory experiments, and more co-ordinated and extensive Antarctic observations such as the Southern Ocean Observing System (SOOS) are expected to help provide the information required to improve these future projections (Newman et al., 2019).
Prognoses of future changes in production in the DCM in the Southern Ocean are not reliable and Earth system models focus instead on depth-integrated estimates of NPP (e.g., Leung et al., 2015; IPCC, 2019). Better satellite-based observation of the occurrence of DCMs in the Southern Ocean using the simple metric described here (EDCM) and better in situ observations using autonomous technology could improve this situation in the future. In particular, the advent of biogeochemical Argo floats (Rembauville et al., 2017; Briggs et al., 2018; Carranza et al., 2018), the SOCOMM project (Riser et al., 2018; Uchida et al., 2019) and Southern Ocean and Climate (SOCLIM) floats1 represent a major step forward.
Although not a focus of the present study, we note that tracking and anticipating changes to primary production in the Southern Ocean should include that within sea ice (Arrigo, 2014; Saenz and Arrigo, 2014; van Leeuwe et al., 2018) and beneath it (Arteaga et al., 2020). Although sea ice algae only contribute ∼1% of total Southern Ocean primary production, and 12–50% of total primary production in the sea ice zone (Kottmeier et al., 1987; Grossi et al., 1987; Saenz and Arrigo, 2014; van Leeuwe et al., 2018), sea ice algae are of disproportionate ecological importance because their production occurs in locations and at times when production in the water column is low (Quetin et al., 1996; Saenz and Arrigo, 2014; McCormack et al., in review). As such, sea ice algae are a crucial bridge between low and high productivity periods for many mid-trophic level species, such as Antarctic krill (Euphausia superba; Daly, 1990; Smetacek et al., 1990; Loeb et al., 1997; Kohlbach et al., 2017; Meyer et al., 2017), and Antarctic silverfish (Pleuragramma antarctica) (Guglielmo et al., 1998; Vacchi et al., 2004).
Drivers of primary production by sea ice algae include ice-extent and thickness, incident light availability, and nutrient supply (Kottmeier and Sullivan, 1990; Arrigo et al., 1998; Arrigo, 2014; Hobbs et al., 2016; Tedesco and Vichi, 2014), though temperature and salinity can be important (Tedesco and Vichi, 2014; Saenz and Arrigo, 2014). Variations in snow depth will influence both light penetration into sea ice, and nutrient input (Saenz and Arrigo, 2014). Both laboratory manipulations and in situ experiments indicate that sea ice algae are little affected by changes to pH (McMinn, 2017).
Complex models have been developed to simulate the large-scale primary production by sea ice algae (Arrigo et al., 1991, 1997; Arrigo and Sullivan, 1994; Saenz and Arrigo, 2012; Arrigo, 2014; Tedesco and Vichi, 2014) but these have neither been well validated to date (Meiners et al., 2012) nor used to investigate long-term changes in sea ice algae primary production. Furthermore, no future projections of changes to sea ice algae production are available in CMIP5 models (IPCC, 2019).
In the longer term (∼100 years hence) it is likely that sea ice algae primary production will decrease in line with reducing sea ice extent and concentrations throughout the Southern Ocean (IPCC, 2019), but these changes are likely to be spatially heterogenous and non-linear (van Leeuwe et al., 2018). We also note that the ecosystem consequences of a reduction of primary production in sea ice could be significant and far reaching (Atkinson et al., 2004; Meyer et al., 2017; Saenz and Arrigo, 2012; Arrigo, 2014; Tedesco and Vichi, 2014; McCormack et al., in review) so this represents a major gap in forecasting capability.
A summary of recent observed changes and anticipated future changes (Table 4 and Figure 7) shows that primary production in the Southern Ocean will likely increase over the next 100 years, with more primary production in the water column and less in sea ice. The distinctive nature of the microbial community of the Southern Ocean (i.e., the dominant species of phytoplankton, phenology (seasonality) and spatial distribution of primary production) will likely reduce, with shifts towards communities more dominated by small and flagellated species, and with endemic Southern Ocean phytoplankton species losing out to temperate species (Deppeler and Davidson, 2017). Year-to-year variability in primary production in the Southern Ocean will likely increase with increasing prevalence of marine heatwaves (Oliver et al., 2019). Recent increases in sea ice concentration (and potentially associated sea ice algae production) will likely reverse in the next few decades and decrease consistent with less ice in a warming Southern Ocean (IPCC, 2019). The importance of local-scale forcing means that forecasting the effects of climate change on coastal primary production, crucial to the reproductive success of many endemic Antarctic species, is especially challenging (Montes-Hugo et al., 2009; Kim et al., 2018). The effects of future changes to primary production are likely to be greatest for those Southern Ocean species with life-cycles intimately linked to seasonal sea ice growth and retreat, including ‘keystone’ species such as Antarctic krill and silverfish (Smetacek et al., 1990; Quetin et al., 1996; Loeb et al., 1997), and their predators (McCormack et al., in review).
Table 4. Summary of recent observed changes (satellite data) and future projections by MEASO areas of the Southern Ocean.
Figure 7. There are different types of primary production in the Southern Ocean – in sea ice, in the surface mixed-layer of the ocean, and in the deep chlorophyll maximum (DCM). Only some of these types of primary production can be observed by satellite over the last 20–30 years. Earth-system models can project future changes to primary production in the next 50–100 years. Changes vary between the Northern, Subantarctic and Antarctic zones.
Satellite observations show mainly increases in phytoplankton biomass in the Southern Ocean over the last 20 years (1997–2019) (high confidence), but the direction of recent changes to primary production are generally not known except to say that it is likely that primary production has decreased in the Ross Sea over the recent past (medium confidence).
At present the satellite record is not long enough to separate long-term trends from climate variability, so we cannot say whether these observed trends will continue in the future (Henson et al., 2010; Del Castillo et al., 2019) (high confidence).
Over the next 100 years, Earth system models project increasing primary production in the northern and Antarctic MEASO zones but decreases in the Subantarctic zone (Leung et al., 2015; Rickard and Behrens, 2016) (low confidence). Low confidence in these projections arise from the difficulty in mapping supply mechanisms for the key nutrients of iron and silicate in the Southern Ocean and understanding the effects of multiple stressors on different species of Antarctic phytoplankton (Hutchins and Boyd, 2016; Boyd et al., 2016, 2019; Deppeler and Davidson, 2017; Freeman et al., 2019; Meredith et al., 2019; Trimborn et al., 2019).
As well as affecting the total amount of primary production in the Southern Ocean, climate change will likely alter seasonal patterns in production (phenology) and the relative abundances of different types of phytoplankton (Wright et al., 2010; Petrou et al., 2016; Balch et al., 2016; Kaufman et al., 2017; Nissen et al., 2018; Trull et al., 2018) (high confidence). These changes will affect zooplankton, have ecological consequences across Southern Ocean food-webs including on keystone species and top predators (Atkinson et al., 2004; Moline et al., 2004; Pinkerton and Bradford-Grieve, 2014; Johnston et al., in review; McCormack et al., in review) (high confidence).
Primary production by sea ice algae will likely decline in the future as sea ice extent shrinks (medium confidence). Because of the ecological importance of sea ice to a range of key Southern Ocean species, the reduction in Southern Ocean sea ice and its associated primary production could lead to a critical tipping point in Southern Ocean ecosystems (medium confidence).
Better methods of monitoring change to phytoplankton and sea ice algae are needed to improve confidence in future projections of primary production in the Southern Ocean (high confidence). Efforts to maintain long-term coastal observations (e.g., Palmer Long Term Ecological Research Network LTER, e.g., Kim et al., 2018), improve satellite observations, co-ordinate field sampling, and develop and deploy autonomous instrumentation in the Southern Ocean are essential to assess long-term trends (Newman et al., 2019) (high confidence). Long-term, multi-trophic level monitoring is required to understand the effects of changes to primary production on middle and upper level predators such as krill, salps, fish, seabirds and marine mammals (high confidence).
Publicly available datasets were analyzed in this study. This data can be found here: https://oceandata.sci.gsfc.nasa.gov/, http://sites.science.oregonstate.edu/ocean.productivity/, https://nsidc.org/, https://www.ncdc.noaa.gov/isccp.
All authors listed have made a substantial, direct and intellectual contribution to the work, and approved it for publication.
Funding for MP, SD, and AH was provided by New Zealand MBIE Endeavour Fund programme Ross-RAMP (C01X1710) and NZ MBIE Strategic Science Investment Fund: “Coasts and Oceans Programme 4”. JH was supported by ANID (FONDAP-IDEAL 15150003) and FONDECYT (Postdoctorado 3180152). ICED partially supported the assistance of JH to the MEASO workshop. PB was supported by the Australian Department of Industry funded ACE-CRC and AAPP programmes.
The authors declare that the research was conducted in the absence of any commercial or financial relationships that could be construed as a potential conflict of interest.
SeaWiFS and MODIS data were used courtesy of NASA. We acknowledge the HyCOM project for access to mixed-layer depth data. We thank the University of Oregon (United States) for primary production data. Sea ice data were accessed courtesy of the United States National Snow and Ice Data Centre. We also thank Dr. Stacey McCormack (Institute for Marine and Antarctic Studies, University of Tasmania) for the Infographic (Figure 7). We are grateful to two referees and the editors of the Special Issue who provided valuable feedback and suggestions that helped improve this manuscript.
The Supplementary Material for this article can be found online at: https://www.frontiersin.org/articles/10.3389/fevo.2021.592027/full#supplementary-material
Chlorophyll-a / chl-a, The ubiquitous light-harvesting pigment in marine phytoplankton usually expressed as a volumetric concentration (mg m–3); DCM, Deep chlorophyll maximum, a sub-surface phytoplankton bloom; ENSO, El Niño-Southern Oscillation; HNLC, High nitrate low chlorophyll water; IPCC, Intergovernmental Panel on Climate Change; ISCCP, International Satellite Cloud Climatology Project; MODIS, Moderate Resolution Imaging Spectro-radiometer (NASA); NASA, National Aeronautics and Space Administration of the United States; NPP, Net primary production is defined as the depth-integrated rate of carbon incorporation into organic (living) matter after allowing for respiration of algae (gC m–2 d–1); PAR, Photosynthetically active radiation (light between 400 and 700 nm); pCO2, Partial pressure of dissolved carbon dioxide; RCP, Representative Concentration Pathway; SAM, Southern annular mode (a mode of climate variability); SeaWiFS, Sea-viewing Wide Field-of-view Sensor (OrbImage/NASA); VGPM, Vertically-generalised production model.
Alvain, S., and d’Ovidio, F. (2014). “Phytoplankton diversity in the Southern Ocean: a satellite view. Chapter 6.2,” in Biogeographic Atlas of the Southern Ocean, eds C. De Broyer, P. Koubbi, H. J. Griffiths, B. Raymond, C. Udekem d’Acoz, A. P. Van de Putte, et al. (Cambridge: Scientific Committee on Antarctic Research), 260–265.
Andrew, S. M., Morell, H. T., Strzepek, R. F., Boyd, P. W., and Ellwood, M. J. (2019). Iron availability influences the tolerance of Southern Ocean phytoplankton to warming and elevated irradiance. Front. Mar. Sci. 6:681. doi: 10.3389/fmars.2019.00681
Aracena, C., González, H. E., Garcés-Vargas, J., Lange, C. B., Pantoja, S., Muñoz, F., et al. (2018). Primary production in the Southern Ocean, 1997–2006. J. Geophys. Res. Oceans 113:C08004. doi: 10.1029/2007JC004551
Arrigo, K., Sullivan, C., and Kremer, J. (1991). A bio-optical model of Antarctic sea ice. J. Geophys. Res. Oceans 96, 581–610.
Arrigo, K., Worthen, D., Lizotte, M., and Dixon, P. (1997). Primary production in Antarctic sea ice. Science 276, 394–397. doi: 10.1126/science.276.5311.394
Arrigo, K. R., Mills, M. M., Kropuenske, L. R., van Dijken, G. L., Alderkamp, A. −C., and Robinson, D. H. (2010). Photophysiology in two major Southern Ocean phytoplankton taxa: photosynthesis and growth of Phaeocystis antarctica and Fragilariopsus cylindrus under different irradiance levels. Integr. Comp. Biol. 50, 950–966. doi: 10.1093/icb/icq021
Arrigo, K. R., and Sullivan, C. W. (1994). A high-resolution bio-optical model of microalgal growth: Testing using sea-ice algal community time-series data. Limnol. Oceanogr. 39, 609–631. doi: 10.4319/lo.1994.39.3.0609
Arrigo, K. R., van Dijken, G. L., and Bushinsky, S. (2008). Primary production in the Southern Ocean, 1997–2006. J. Geophys. Res. Oceans 113:C08004.
Arrigo, K. R., van Dijken, G. L., and Strong, A. L. (2015). Environmental controls of marine productivity hot spots around Antarctica. J. Geophys. Res. Oceans 120, 5545–5565. doi: 10.1002/2015JC010888
Arrigo, K. R., Worthen, D. L., Dixon, P., and Lizotte, M. P. (1998). “Primary productivity of near surface communities within Antarctic pack ice,” in Antarctic Sea Ice Biological Processes, Interactions, and Variability: Antarctic Research Series, Vol. 73, eds M. P. Lizotte and K. R. Arrigo (Washington, DC: American Geophysical Union), 23–43. doi: 10.1029/ar073p0023
Arteaga, L. A., Boss, E., Behrenfeld, M. J., Westberry, T. K., and Sarmiento, J. L. (2020). Seasonal modulation of phytoplankton biomass in the Southern Ocean. Nat. Commun. 11:5364. doi: 10.1038/s41467-020-19157-2
Atkinson, A., Siegel, V., Pakhomov, E., and Rothery, P. (2004). Long-term decline in krill stock and increase in salps within the Southern Ocean. Nature 432, 100–103. doi: 10.1038/nature02996
Balch, W. M., Bates, N. R., Lam, P. J., Twining, B. S., Rosengard, S. Z., Bowler, B. C., et al. (2016). Factors regulating the Great Calcite Belt in the Southern Ocean and its biogeochemical significance. Glob. Biogeochem. Cycles 30, 1124–1144. doi: 10.1002/2016gb005414
Balch, W. M., Drapeau, D. T., Bowler, B. C., Lyczskowski, E., Booth, E. S., and Alley, D. (2011). The contribution of coccolithophores to the optical and inorganic carbon budgets during the Southern Ocean Gas Exchange Experiment: new evidence in support of the “Great Calcite Belt” hypothesis. J. Geophys. Res. Oceans 116:C00F06. doi: 10.1029/2011JC006941
Banse, K. (1996). Low seasonality of low concentrations of surface chlorophyll in the Subantarctic water ring: under- water irradiance, iron, or grazing? Prog. Oceanogr. 37, 241–291. doi: 10.1016/s0079-6611(96)00006-7
Behrenfeld, M. J. (2014). Climate-mediated dance of the plankton. Nat. Clim. Change 4, 880–887. doi: 10.1038/nclimate2349
Behrenfeld, M. J., Boss, E., Siegel, D. A., and Shea, D. M. (2005). Carbon-based ocean productivity and phytoplankton physiology from space. Glob. Biogeochem. Cycles 19:GB1006.
Behrenfeld, M. J., and Falkowski, P. G. (1997). Photosynthetic rates derived from satellite-based chlorophyll concentration. Limnol. Oceanogr. 42, 1–20. doi: 10.4319/lo.1997.42.1.0001
Biermann, A., Lewandowska, A., Engel, A., and Riebesell, U. (2015). Organic matter partitioning and stoichiometry in response to rising water temperature and copepod grazing. Mar. Ecol. Prog. Ser. 522, 49–65. doi: 10.3354/meps11148
Blain, S., Quéguiner, B., Armand, L., Belviso, S., Bombled, B., Bopp, L., et al. (2007). Effect of natural iron fertilization on carbon sequestration in the Southern Ocean. Nature 446, 1070–1074.
Boyd, P., and Ellwood, M. (2010). The biogeochemical cycle of iron in the ocean. Nat. Geosci. 3, 675–682. doi: 10.1038/ngeo964
Boyd, P. W. (2019). Physiology and iron modulate diverse responses of diatoms to a warming Southern Ocean. Nat. Clim. Change 9, 148–152. doi: 10.1038/s41558-018-0389-1
Boyd, P. W., Arrigo, K. R., Strzepek, R., and van Dijken, G. L. (2012). Mapping phytoplankton iron utilization: Insights into Southern Ocean supply mechanisms. J. Geophys. Res. Oceans 117:C06009. doi: 10.1029/2011JC007726
Boyd, P. W., and Brown, C. J. (2015). Modes of interaction between environmental drives and marine biota. Front. Mar. Sci. 2:9. doi: 10.3389/fmars.2015.00009
Boyd, P. W., Crossley, A. C., DiTullio, G. R., Griffiths, F. B., Hutchins, D. A., Queguiner, B., et al. (2001). Control of phytoplankton growth by iron supply and irradiance in the subantarctic Southern Ocean: experimental results from the SAZ Project. J. Geophys. Res. Oceans 106, 31573–31583. doi: 10.1029/2000jc000348
Boyd, P. W., Dillingham, P. W., McGraw, C. M., Armstrong, E. A., Cornwall, C. E., Feng, Y.-Y., et al. (2016). Physiological responses of a Southern Ocean diatom to complex future ocean conditions. Nat. Clim. Change 6, 207–213. doi: 10.1038/nclimate2811
Boyd, P. W., Kawaguchi, S., Strzepek, R., Meiners, K., Hallegraeff, G., McMinn, A., et al. (2019). Report Card: Potential Tipping Points for Life in the Southern Ocean. Hobart, TAS: Antarctic Climate and Ecosystems Cooperative Research Centre, doi: 10.25959/100.00031768
Boyd, P. W., Lennartz, S. T., Glover, D. M., and Doney, S. C. (2014). Biological ramifications of climate-change mediated oceanic multi-stressors. Nat. Clim. Change 5, 71–79. doi: 10.1038/NCLIMATE2441
Boyer, T. P., Antonov, J. I., Baranova, O. K., Coleman, C., Garcia, H. E., Grodsky, A., et al. (2013). “World Ocean database 2013,” in NOAA Atlas NESDIS, Vol. 72, ed. S. Levitus (Silver Spring, MD: NOAA), 209.
Bracher, A. U., Kroon, B. M. A., and Lucas, M. I. (1999). Primary production, physiological state and composition of phytoplankton in the Atlantic sector of the Southern Ocean. Mar. Ecol. Prog. Ser. 190, 1–16. doi: 10.3354/meps190001
Briggs, E. M., Martz, T. R., Talley, L. D., Mazloff, M. R., and Johnson, K. S. (2018). Physical and biological drivers of biogeochemical tracers within the seasonal sea ice zone of the Southern Ocean from profiling floats. J. Geophys. Res. Oceans 123, 746–758. doi: 10.1002/2017jc012846
Brown, M. S., Munro, D. R., Feehan, C. J., Sweeney, C., Ducklow, H. W., and Schofield, O. M. (2019). Enhanced oceanic CO2 uptake along the rapidly changing West Antarctic Peninsula. Nat. Clim. Change 9, 678–683. doi: 10.1038/s41558-41019-40552-41553
Buongiorno Nardelli, B., Guinehut, S., Verbrugge, N., Cotroneo, Y., Zambianchi, E., and Iudicone, D. (2017). Southern Ocean mixed-layer seasonal and interannual variations from combined satellite and in situ data. J. Geophys. Res. Oceans 122, 10042–10060. doi: 10.1002/2017JC013314
Cadée, G., González, H., and Schnack-Schiel, S. (1992). Krill diet affects faecal string settling. Polar Biol. 12, 75–80. doi: 10.1007/978-3-642-77595-6
Cael, B. B., and Follows, M. J. (2016). On the temperature dependence of oceanic export efficiency. Geophys. Res. Lett. 43, 5170–5175. doi: 10.1002/2016GL068877
Campbell, J. W., and Aarup, T. (1989). Photosynthetically available radiation at high latitudes. Limnol. Oceanogr. 34, 1490–1499. doi: 10.4319/lo.1989.34.8.1490
Caron, D. A., and Hutchins, D. A. (2013). The effects of changing climate on microzooplankton grazing and community structure: drivers, predictions and knowledge gaps. J. Plank. Res. 35, 235–252. doi: 10.1093/plankt/fbs091
Carranza, M. M., Gille, S. T., Franks, P. J., Johnson, K. S., Pinkel, R., and Girton, J. B. (2018). When mixed layers are not mixed. Storm-driven mixing and bio-optical vertical gradients in mixed layers of the Southern Ocean. J. Geophys. Res. Oceans 123, 7264–7289. doi: 10.1029/2018jc014416
Cassar, N., Bender, M. L., Barnett, B. A., Fan, S., Moxim, W. J., Levy, H., et al. (2007). The Southern Ocean biological response to Aeolian iron deposition. Science 317, 1067–1070. doi: 10.1126/science.1144602
Cavalieri, D., Gloerson, P., and Zwally, J. (1990). “DMSP SSM/I daily polar gridded sea-ice concentrations, 1997 to 2006,” in Digital media, eds J. Maslanik and J. Stroeve (Boulder, CO: National Snow and Ice Data Center).
Chassignet, E. P., Hurlburt, H. E., Smedstad, O. M., Halliwell, G. R., Hogan, P. J., Wallcraft, A. J., et al. (2007). The HYCOM (HYbrid Coordinate Ocean Model) data assimilative system. J. Mar. Syst. 65, 60–83.
Clark, D. K. (1997). MODIS Algorithm Theoretical Basis Document – Bio-optical algorithms – Case I waters. Washington, DC: NASA.
Cullen, J. J. (2015). Subsurface chlorophyll maximum layers: Enduring enigma or mystery solved? Ann. Rev. Mar. Sci. 7, 207–239. doi: 10.1146/annurev-marine-010213-135111
Daly, K. (1990). Overwintering development, growth, and feeding of larval Euphausia superba in the Antarctic marginal ice zone. Limnol. Oceanogr. 35, 1564–1576. doi: 10.4319/lo.1990.35.7.1564
de Baar, H. J. W., de Jong, J. T. M., Bakker, D. C. E., Löscher, B. M., Veth, C., Bathmann, U., et al. (1995). Importance of iron for plankton blooms and carbon dioxide drawdown in the Southern Ocean. Nature 373, 412–415. doi: 10.1038/373412a0
Del Castillo, C. E., Signorini, S. R., Karaköylü, E. M., and Rivero−Calle, S. (2019). Is the Southern Ocean getting greener? Geophys. Res. Lett. 46, 6034–6040. doi: 10.1029/2019gl083163
Deppeler, S. L., and Davidson, A. T. (2017). Southern Ocean phytoplankton in a changing climate. Front. Mar. Sci. 4:40. doi: 10.3389/fmars.2017.00040
Dierssen, H. M., Vernet, M., and Smith, R. C. (2000). Optimizing models for remotely estimating primary production in Antarctic coastal waters. Antarct. Sci. 12, 20–32. doi: 10.1017/S0954102000000043
Doblin, M. A., Petrou, K. L., Shelly, K., Westwood, K., van den Enden, R., Wright, S., et al. (2011). Diel variation of chlorophyll-a fluorescence, phytoplankton pigments and productivity in the Sub-Antarctic and Polar Front Zones south of Tasmania, Australia. Deep-Sea Res. II 58, 2189–2199. doi: 10.1016/j.dsr2.2011.05.021
Doney, S. C. (2006). Oceanography – Plankton in a warmer world. Nature 444, 695–696. doi: 10.1038/444695a
El-Sayed, S. Z. (1970). “On the productivity of the Southern Ocean,” in Antarctic Ecology, Vol. I, ed. M. W. Holdgate (New York: Academic Press), 119–135.
El-Sayed, S. Z., Holm-Hansen, O., and Biggs, D. C. (1983). Phytoplankton standing crop, primary productivity, and near-surface nitrogenous nutrient fields in the Ross Sea, Antarctica. Deep Sea Res. I 30, 871–886. doi: 10.1016/0198-0149(83)90005-5
El-Sayed, S. Z., and Mandelli, E. F. (1965). “Primary production and standing crop of phytoplankton in the Weddell Sea and Drake Passage,” in Biology of the Antarctic Sea II, Vol. 5, ed. G. A. Llano (Washington, DC: American Geophysical Union), 87–106. doi: 10.1029/ar005p0087
El-Sayed, S. Z., and Taguchi, S. (1981). Primary production and standing crop of phytoplankton along the ice-edge in theWeddell Sea. Deep Sea Res. I 28, 1017–1032. doi: 10.1016/0198-0149(81)90015-7
Figueiras, F. G., Estrada, M., Lopez, O., and Arbones, B. (1998). Photosynthetic parameters and primary production in the Bransfield Strait: relationships with mesoscale hydrographic structures. J. Mar. Syst. 17, 129–141. doi: 10.1016/s0924-7963(98)00034-7
Finkel, Z. V., Beardall, J., Flynn, K. J., Quigg, A., Rees, T. A. V., and Raven, J. A. (2010). Phytoplankton in a changing world: cell size and elemental stoichiometry. J. Plank. Res. 32, 119–137. doi: 10.1093/plankt/fbp098
Freeman, N. M., Munro, D. R., Sprintall, J., Mazloff, M. R., Purkey, S. G., Rosso, I., et al. (2019). The observed seasonal cycle of macronutrients in Drake Passage: relationship to fronts and utility as a model metric. J. Geophys. Res. Oceans 124, 4763–4783. doi: 10.1029/2019jc015052
Frouin, R., McPherson, J., Ueyoshi, K., and Franz, B. A. (2012). A time series of photosynthetically available radiation at the ocean surface from SeaWiFS and MODIS data. Rem. Sens. Mar. Environ. II:852519. doi: 10.1117/12.981264
Garibotti, I., Vernet, M., Smith, R. C., and Ferrario, M. E. (2005). Interannual variability in the distribution of the phytoplankton standing stock across the seasonal sea-ice zone west of the Antarctic Peninsula. J. Plankton Res. 27, 825–843. doi: 10.1093/plankt/fbi056
Grossi, S. M., Kottmeier, S. T., Moe, R. L., Taylor, G. T., and Sullivan, C. W. (1987). Sea ice microbial communities. VI. Growth and. primary production in bottom ice under graded snow cover. Mar. Ecol. Prog. Ser. 35, 153–164. doi: 10.3354/meps035153
Guglielmo, L., Granata, A., and Greco, S. (1998). Distribution and abundance of postlarval and juvenile Pleuragramma antarcticum (Pisces, Nototheniidae) off Terra Nova Bay (Ross Seas Antarctica). Polar Biol. 19, 37–51. doi: 10.1007/s003000050214
Haentjens, N., Boss, E., and Talley, L. D. (2017). Revisiting Ocean Color algorithms for chlorophyll a and particulate organic carbon in the Southern Ocean using biogeochemical floats. J. Geophys. Res. Oceans 122, 6583–6593. doi: 10.1002/2017JC012844
Hauck, J., Völker, C., Wolf-Gladrow, D. A., Laufkötter, C., Vogt, M., Aumont, O., et al. (2015). On the Southern Ocean CO2 uptake and the role of the biological carbon pump in the 21st century. Global Biogeochem. Cycles 29, 1451–1470. doi: 10.1002/2015GB005140
Helbling, E. W., Villafañe, V. E., and Holm-Hansen, O. (1995). Variability of phytoplankton distribution and primary production around Elephant Island, Antarctica, during 1990–1993. Polar Biol. 15, 233–246.
Henley, S., Cavan, E. L., Fawcett, S. E., Kerr, R., Monteiro, T., Sherrell, R. M., et al. (2020). Changing biogeochemistry of the Southern Ocean and its ecosystem implications. Front. Mar. Sci. 7:581. doi: 10.3389/fmars.2020.00581
Henson, S., Le Moigne, F., and Giering, S. (2019). Drivers of carbon export efficiency in the global ocean. Glob. Biogeochem. Cycles 33, 891–903. doi: 10.1029/2018GB006158
Henson, S. E., Sarmiento, J. L., Dunne, J. P., Bopp, L., Lima, L., Doney, S. C., et al. (2010). Detection of anthropogenic climate change in satellite records of ocean chlorophyll and productivity. Biogeoscience 7, 621–640. doi: 10.5194/bg-7-621-2010
Hill, S. L., Murphy, E. J., Reid, K., Trathan, P. N., and Constable, A. J. (2006). Modelling Southern Ocean ecosystems: krill, the food-web, and the impacts of harvesting. Biol. Rev. 81, 581–608. doi: 10.1017/S1464793106007123
Hipel, K. W., and McLeod, A. I. (1994). Time Series Modelling of Water Resources and Environmental Systems. Amsterdam: Elsevier.
Hiscock, M. R., Marra, J., Smith, W. O. J., Goericke, R., Measures, C., Vink, S., et al. (2003). Primary productivity and its regulation in the Pacific Sector of the Southern Ocean. Deep Sea Res. II 50, 533–558. doi: 10.1016/S0967-0645(02)00583-0
Hixson, S. M., and Arts, M. T. (2016). Climate warming is predicted to reduce omega-3, long-chain, polyunsaturated fatty acid production in phytoplankton. Glob. Change Biol. 22, 2744–2755. doi: 10.1111/gcb.13295
Hobbs, W. R., Massom, R., Stammerjohn, S., Reid, P., Williams, G., and Meier, W. (2016). A review of recent changes in Southern Ocean sea ice, their drivers and forcings. Glob. Planet Change 143, 228–250. doi: 10.1016/j.gloplacha.2016.06.008
Höfer, J., Giesecke, R., Hopwood, M. J., Carrera, V., Alarcón, E., and González, H. E. (2019). The role of water column stability and wind mixing in the production/export dynamics of two bays in the Western Antarctic Peninsula. Prog. Oceanogr. 174, 105–116. doi: 10.1016/j.pocean.2019.01.005
Holm-Hansen, O., and Mitchell, B. G. (1991). Spatial and temporal distribution of phytoplankton and primary production in the western Bransfield Strait region. Deep Sea Res. 38, 961–980. doi: 10.1016/0198-0149(91)90092-t
Hoppe, C. J. M., Hassler, C. S., Payne, C. D., Tortell, P. D., Rost, B., and Trimborn, S. (2013). Iron limitation modulates Ocean Acidification effects on Southern Ocean phytoplankton communities. PLoS One 8:e79890. doi: 10.1371/journal.pone.0079890
Hoppe, C. J. M., Klaas, C., Ossebaar, S., Soppa, M. A., Cheah, W., Laglera, L. M., et al. (2017). Controls of primary production in two phytoplankton blooms in the Antarctic Circumpolar Current. Deep Sea Res. Part II Top. Stud. Oceanogr. 138, 63–73. doi: 10.1016/j.dsr2.2015.10.005
Hopwood, M. J., Carroll, D., Höfer, J., Achterberg, E. P., Meire, L., Le Moigne, F. A. C., et al. (2019). Highly variable iron content modulates iceberg-ocean fertilisation and potential carbon export. Nat. Commun. 10:5261. doi: 10.1038/s41467-019-13231-0
Horne, A. J., Fogg, G. E., and Eagle, D. J. (1969). Studies in situ of the primary production of an area of inshore Antarctic Sea. J. Mar. Biol. Ass. UK. 49, 393–405. doi: 10.1017/s0025315400035980
Hutchins, D. A., and Boyd, P. W. (2016). Marine phytoplankton and the changing ocean iron cycle. Nat. Clim. Change 6, 1072–1079. doi: 10.1038/nclimate3147
IPCC (2019). “Summary for Policymakers,” in IPCC Special Report on the Ocean and Cryosphere in a Changing Climate, eds H.-O. Pörtner, D. C. Roberts, V. Masson-Delmotte, P. Zhai, M. Tignor, E. Poloczanska, et al. (Geneva: IPCC).
Jena, B. (2017). The effect of phytoplankton pigment composition and packaging on the retrieval of chlorophyll-a concentration from satellite observations in the Southern Ocean. Int. J. Rem. Sens. 38, 3763–3784. doi: 10.1080/01431161.2017.1308034
Johnson, R., Strutton, P. G., Wright, S. W., McMinn, A., and Meiners, K. M. (2013). Three improved Satellite Chlorophyll algorithms for the Southern Ocean. J. Geophys. Res. Oceans 118, 3694–3703. doi: 10.1002/jgrc.20270
Karl, D. M., Tilbrook, B. D., and Tien, G. (1991). Seasonal coupling of organic matter production and particle flux in the western Bransfield Strait, Antartica. Deep Sea Res. I 38, 1097–1126. doi: 10.1016/0198-0149(91)90098-z
Kaufman, D. E., Friedrichs, M. A. M., Smith, W. O. Jr., Hofmann, E. E., Dinniman, M. S., and Hemmings, J. C. P. (2017). Climate change impacts on southern Ross Sea phytoplankton composition, productivity, and export. J. Geophys. Res. Oceans 122, 2339–2359. doi: 10.1002/2016jc012514
Kemp, A. E. S., Pearce, R. B., Grigorov, I., Rance, J., Lange, C. B., Quilty, P., et al. (2006). Production of giant marine diatoms and their export at oceanic frontal zones: implications for Si and C flux from stratified oceans. Glob. Biogeochem. Cycles 20, 1–13. doi: 10.1029/2006GB002698
Kim, H., Ducklow, H. W., Abele, D., Ruiz Barlett, E. M., Buma, A. G. J., Meredith, M. P., et al. (2018). Inter-decadal variability of phytoplankton biomass along the coastal West Antarctic Peninsula. Philos. T. R. Soc. A 376:20170179. doi: 10.1098/rsta.2017.0179
Kohlbach, D., Lange, B. A., Schaafsma, F. L., David, C., Vortkamp, M., Graeve, M., et al. (2017). Ice algae-produced carbon is critical for overwintering of Antarctic Krill Euphausia superba. Front. Mar. Sci. 4:310. doi: 10.3389/fmars.2017.00310
Kostov, Y., Marshall, J., Hausmann, U., Armour, K. C., Ferreira, D., Holland, M., et al. (2016). Fast and slow responses of Southern Ocean sea surface temperature to SAM in coupled climate models. Clim. Dyn. 48, 1595–1609. doi: 10.1007/s00382-016-3162-z
Kottmeier, S. T., Grossi, S. M., and Sullivan, C. W. (1987). Sea ice microbial communities. VIII. Bacterial production in annual sea ice of McMurdo Sound, Antarctica. Mar. Ecol. Prog. Ser. 35, 175–186. doi: 10.3354/meps035175
Kottmeier, S. T., and Sullivan, C. W. (1990). Bacterial biomass and production in pack ice of Antarctic marginal ice edge zones. Deep Sea Res. I 37, 1311–1330. doi: 10.1016/0198-0149(90)90045-w
Kropuenske, L. R., Mills, M. M., Van Dijken, G. L., Alderkamp, A. −C., Berg, G. M., Robinson, D. H., et al. (2010). Strategies and rates of photoacclimation in two major Southern Ocean phytoplankton taxa: Phaeocystis antarctica (Haptophyta) and Fragilariopsis cylindrus (Bacillariophyceae). J. Phycol. 50, 950–966. doi: 10.1093/icb/icq021
Lannuzel, D., Vancoppenolle, M., van der Merwe, P., de Jong, J., Meiners, K. M., Grotti, M., et al. (2016). Iron in sea ice: review and new insights. Elementa 4:000130.
Le Quéré, C., Buitenhuis, E. T., Moriarty, R., Alvain, S., Aumont, O., Bopp, L., et al. (2016). Role of zooplankton dynamics for Southern Ocean phytoplankton biomass and global biogeochemical cycles. Biogeosciences 13, 4111–4133. doi: 10.5194/bg-13-4111-2016
Le Quéré, C., Harrison, S. P., Prentice, I. C., Buitenhuis, E. T., Aumont, O., Bopp, L., et al. (2005). Ecosystem dynamics based on plankton functional types for global ocean biogeochemistry models. Glob. Change Biol. 11, 2016–2040.
Leung, S., Cabré, A., and Marinov, I. (2015). A latitudinally banded phytoplankton response to 21st century climate change in the Southern Ocean across the CMIP5 model suite. Biogeosciences 12, 5715–5734. doi: 10.5194/bg-12-5715-2015
Llort, J., Lévy, M., Sallée, J. B., and Tagliabue, A. (2019). Nonmonotonic response of primary production and export to changes in mixed−layer depth in the Southern Ocean. Geophys. Res. Lett. 46, 3368–3377. doi: 10.1029/2018GL081788
Loeb, V., Siegel, V., Holm-Hansen, O., Hewitt, R., Fraser, W., Trivelpiece, W., et al. (1997). Effects of sea-ice extent and krill or salp dominance on the Antarctic food web. Nature 387, 897–900. doi: 10.1038/43174
Luxem, K. E., Ellwood, M. J., and Strzepek, R. F. (2017). Intraspecific variability in Phaeocystis antarctica’s response to iron and light stress. PLoS One 12:e0179751. doi: 10.1371/journal.pone.0179751
Maheshwari, M., Singh, R. K., Oza, S. R., and Kumar, R. (2013). An investigation of the Southern Ocean surface temperature variability using long-term optimum interpolation SST data. ISRN Oceanogr. 2013:392632.
Mandelli, E. F., and Burkholder, P. R. (1965). Primary productivity in the Gerlache and Bransfield Straits of Antactica. J. Mar. Res. 24, 15–27.
Mann, H. B. (1945). Nonparametric tests against trend. Econometrica 13, 245–259. doi: 10.2307/1907187
McKinley, G. A., Fay, A. R., Lovenduski, N. S., and Pilcher, D. J. (2017). Natural variability and anthropogenic trends in the ocean carbon sink. Ann. Rev. Mar. Sci. 9, 125–150.
McMinn, A. (2017). Reviews and syntheses: ice acidification, the effects of ocean acidification on sea ice microbial communities. Biogeosciences 14, 3927–3935. doi: 10.5194/bg-14-3927-2017
Meiners, K. M., Vancoppenolle, M., Thanassekos, S., Dieckmann, G. S., Thomas, D. N., Tison, J. L., et al. (2012). Chlorophyll a in Antarctic sea ice from historical ice core data. Geophys. Res. Lett. 39:L21602. doi: 10.1029/2012GL053478
Meredith, M., Sommerkorn, M., Cassotta, S., Mackintosh, A., Ottersen, G., Derksen, C., et al. (2019). “Polar regions,” in IPCC Special Report on the Ocean and Cryosphere in a Changing Climate, eds H.-O. Pörtner, D. C. Roberts, V. Masson-Delmotte, P. Zhai, M. Tignor, E. Poloczanska, et al. (Geneva: IPCC).
Meskhidze, N., and Nenes, A. (2006). Phytoplankton and cloudiness in the Southern Ocean. Science 314, 1419–1423. doi: 10.1126/science.1131779
Metzger, J., Hurlburt, H., Wallcraft, A., Smedstad, O. M., Kara, B., Shriver, J., et al. (2007). “1/12° Global HYCOM Evaluation and Validation,” in Proceedings of the 11th HYCOM Consortium Meeting 24-26 April 2007, (Hancock County, MS: Stennis Space Center).
Meyer, B., Freier, U., Grimm, V., Groeneveld, J., Hunt, B. P. V., Kerwath, S., et al. (2017). The winter pack-ice zone provides a sheltered but food-poor habitat for larval Antarctic krill. Nat. Ecol. Evol. 1, 1853–1861. doi: 10.1038/s41559-017-0368-3
Mock, T., Otillar, R., Strauss, J., McMullan, M., Paajanen, P., Schmutz, J., et al. (2017). Evolutionary genomics of the cold-adapted diatom Fragilariopsis cylindrus. Nature 541, 536–540. doi: 10.1038/nature20803
Moline, M. A., Claustre, H., Frazer, T. K., Schofield, O., and Vernet, M. (2004). Alteration of the food web along the Antarctic Peninsula in response to a regional warming trend. Glob. Change Biol. 10, 1973–1980. doi: 10.1111/j.1365-2486.2004.00825.x
Moline, M. A., and Prézelin, B. B. (1997). High-resolution time-series data for primary production and related parameters at a Palmer LTER coastal site: implications for modeling carbon fixation in the southern ocean. Polar Biol. 17, 39–53. doi: 10.1007/s003000050103
Mongwe, N. P., Vichi, M., and Monteiro, P. M. S. (2018). The seasonal cycle of pCO2 and CO2 fluxes in the Southern Ocean: Diagnosing anomalies in CMIP5 Earth system models. Biogeosciences 155, 2851–2872. doi: 10.5194/bg-15-2851-2018
Montes-Hugo, M., Doney, S. C., Ducklow, H. W., Fraser, W., Martinson, D., Stammerjohn, S. E., et al. (2009). Recent changes in phytoplankton communities associated with rapid regional climate change along the western Antarctic Peninsula. Science 323, 1470–1473. doi: 10.1126/science.1164533
Montes-Hugo, M. A., Vernet, M., Martinson, D., Smith, R., and Iannuzzi, R. (2008). Variability on phytoplankton size structure in the western Antarctic Peninsula (1997–2006). Deep Sea Res II 55, 2106–2117. doi: 10.1016/j.dsr2.2008.04.036
Moore, J. K., Fu, W., Primeau, F., Britten, G. L., Lindsay, K., Long, M., et al. (2018). Sustained climate warming drives declining marine biological productivity. Science 359, 1139–1143. doi: 10.1126/science.aao
Moreau, S., Boyd, P. W., and Strutton, P. G. (2020). Remote assessment of the fate of phytoplankton in the Southern Ocean sea-ice zone. Nat. Commun. 11:3108. doi: 10.1038/s41467-020-16931-0
Moreau, S., Ferreyra, G. A., Mercier, B., Lemarchand, K., Lionard, M., Roy, S., et al. (2010). Variability of the microbial community in the Western Antarctic Peninsula from late fall to spring during a low-ice cover year. Polar Biol. 33, 1599–1614. doi: 10.1007/s00300-010-0806-z
Moreau, S., Mostajir, B., Bélanger, S., Schloss, I. R., Vancoppenolle, M., Demers, S., et al. (2015). Climate change enhances primary production in the western Antarctic Peninsula. Glob. Change Biol. 21, 2191–2205. doi: 10.1111/gcb.12878
Morel, A., Huot, Y., Gentili, B., Werdell, P. J., Hooker, S. B., and Franz, B. A. (2007). Examining the consistency of products derived from various ocean color sensors in open ocean (Case 1) waters in the perspective of a multi-sensor approach. Rem. Sens. Environ. 111, 69–88. doi: 10.1016/j.rse.2007.03.012
Moutier, W., Thomalla, S. J., Bernard, S., Wind, G., Ryan-Keogh, T. J., and Smith, M. E. (2019). Evaluation of Chlorophyll-a and POC MODIS aqua products in the Southern Ocean. Rem. Sens. 11:1793. doi: 10.3390/rs11151793
NASA Goddard Space Flight Center, Ocean Ecology Laboratory, and Ocean Biology Processing Group. (2018a). SeaWiFS Ocean Color Reprocessing 2018.0. Available online at: https://oceancolor.gsfc.nasa.gov/reprocessing/r2018/seawifs/ (accessed June, 2020).
NASA Goddard Space Flight Center, Ocean Ecology Laboratory, and Ocean Biology Processing Group. (2018b). MODIS/Aqua Ocean Color Reprocessing 2018.0. Available online at: https://oceancolor.gsfc.nasa.gov/reprocessing/r2018/aqua/ (accessed June, 2020).
Newman, L., Heil, P., Trebilco, R., Katsumata, K., Constable, A., van Wijk, E., et al. (2019). Delivering sustained, coordinated, and integrated observations of the Southern Ocean for global impact. Front. Mar. Sci. 6:433. doi: 10.3389/fmars.2019.00433
Nissen, C., Vogt, M., Münnich, M., Gruber, N., and Haumann, F. A. (2018). Factors controlling coccolithophore biogeography in the Southern Ocean. Biogeosciences 15, 6997–7024. doi: 10.5194/bg-15-6997-2018
Oliver, E. C. J., Burrows, M. T., Donat, M. G., Gupta, A. S., Alexander, L. V., Perkins-Kirkpatrick, S. E., et al. (2019). Projected Marine Heatwaves in the 21st century and the potential for ecological impact. Front. Mar. Sci. 6:734. doi: 10.3389/fmars.2019.00734
O’Reilly, J. E., and Sherman, K. (2016). “Chapter 5.1: primary productivity and trends,” in IOC-UNESCO and UNEP (2016). Large Marine Ecosystems: Status and Trends, (Nairobi: United Nations Environment Programme), 91–99.
Park, J., Kim, J.-H., Kim, H., Kim, B.-K., Bae, D., Jo, Y.-H., et al. (2019). Reconstruction of ocean color data using machine learning techniques in Polar regions: focusing on Cape Hallett, Ross Sea. Rem. Sens. 11:1366. doi: 10.3390/rs11111366
Parslow, J. S., Boyd, P. W., Rintoul, S. R., and Griffiths, F. B. (2001). A persistent subsurface chlorophyll maximum in the Interpolar Frontal Zone south of Australia: seasonal progression and implications for phytoplankton-light-nutrient interactions. J. Geophys. Res. Oceans 106, 31543–31557. doi: 10.1029/2000JC000322
Pausch, F., Bischof, K., Trimborn, S., and Jesus, B. (2019). Iron and manganese co-limit growth of the Southern Ocean diatom Chaetoceros debilis. PLoS One 14:e0221959. doi: 10.1371/journal.pone.0221959
Perissinotto, R., and Pakhomov, E. A. (1998). Contribution of salps to carbon flux of marginal ice zone of the Lazarev Sea, Southern Ocean. Mar. Biol. 131, 25–32. doi: 10.1007/s002270050292
Petrou, K., Kranz, S. A., Trimborn, S., Hassler, C. S., Ameijeiras, S. B., Sackett, O., et al. (2016). Southern Ocean phytoplankton physiology in a changing climate. J. Plant Physiol. 203, 135–150. doi: 10.1016/j.jplph.2016.05.004
Pinkerton, M. H. (2019). Change in Environmental Conditions of the Southern Ocean Observed by Satellites and Data-assimilating Models to 2019. CCAMLR Document WG-EMM-19/39. Concarneau: CCAMLR, 22.
Pinkerton, M. H., and Bradford-Grieve, J. M. (2014). Characterizing foodweb structure to identify potential ecosystem effects of fishing in the Ross Sea, Antarctica. ICES J. Mar. Sci. 71, 1542–1553. doi: 10.1093/icesjms/fst230
Pollard, R. T., Salter, I., Sanders, R. J., Lucas, M. I., Moore, C. M., Mills, R. A., et al. (2009). Southern Ocean deep-water carbon export enhanced by natural iron fertilization. Nature 457, 577–580. doi: 10.1038/nature07716
Quetin, L. B., Ross, R. M., Frazer, T. K., and Haberman, K. L. (1996). “Factors affecting distribution and abundance of zooplankton, with an emphasis on Antarctic krill, Euphausia superba,” in Foundations for Ecological Research West of the Antarctic Peninsula: Antarctic Research Series, Vol. 70, eds R. M. Ross, E. E. Hofmann, and L. B. Quetin (Washington, DC: American Geophysical Union), 357–371. doi: 10.1029/ar070p0357
Rembauville, M., Briggs, N., Ardyna, M., Uitz, J., Catala, P., Penkerc’ch, C., et al. (2017). Plankton assemblage estimated with BGC-Argo floats in the Southern Ocean: implications for seasonal successions and particle export in the mesopelagic. J. Geophys. Res. Oceans 122, 8278–8292. doi: 10.1002/2017JC013067
Reynolds, R. W., Rayner, N. A., Smith, T. M., Stokes, D. C., and Wang, W. (2002). An improved in situ and satellite SST analysis for climate. J. Clim. 15, 1609–1625. doi: 10.1175/1520-0442(2002)015<1609:aiisas>2.0.co;2
Rickard, G., and Behrens, E. (2016). CMIP5 earth system models with biogeochemistry: a ross sea assessment. Antarct. Sci. 28, 327–346. doi: 10.1017/s0954102016000122
Riser, S. C., Swift, D., and Drucker, R. (2018). Profiling floats in SOCCOM: technical capabilities for studying the Southern Ocean. J. Geophys. Res. Oceans 123, 4055–4073. doi: 10.1002/2017jc013419
Rousseaux, C. S., and Gregg, W. W. (2015). Recent decadal trends in global phytoplankton composition. Glob. Biogeochem. Cycles 29, 1674–1688. doi: 10.1002/2015GB005139
Saenz, B. T., and Arrigo, K. R. (2012). Simulation of a sea ice ecosystem using a hybrid model for slush layer desalination. J. Geophys. Res. Oceans 117:C05007. doi: 10.1029/2011JC007544
Saenz, B. T., and Arrigo, K. R. (2014). Annual primary production in Antarctic sea ice during 2005–2006 from a sea ice state estimate. J. Geophys. Res. Oceans 119, 3645–3678. doi: 10.1002/2013jc009677
Saggiomo, V., Carrada, G. C., Mangoni, O., Ribera d’Alcalà, M., and Russo, A. (1998). Spatial and temporal variability of size-fractionated biomass and primary production in the Ross Sea (Antarctica) during austral spring and summer. J. Mar. Syst. 17, 115–127. doi: 10.1016/s0924-7963(98)00033-5
Saijo, Y., and Kawashima, T. (1964). Primary production in the Antarctic Ocean. J. Oceanogr. Soc. Japan 19, 190–196. doi: 10.5928/kaiyou1942.19.190
Sallée, J.-B., Llort, J., Tagliabue, A., and Lévy, M. (2015). Characterization of distinct bloom phenology regimes in the Southern Ocean. ICES J. Mar. Sci. 72, 1985–1998. doi: 10.1093/icesjms/fsv069
Sarmento, H., Montoya, J. M., Vazquez-Dominguez, E., Vaque, D., and Gasol, J. M. (2010). Warming effects on marine microbial food web processes: how far can we go when it comes to predictions? Philos. Trans. R. Soc. B Biol. Sci. 365, 2137–2149. doi: 10.1098/rstb.2010.0045
Savidge, G., Harbour, D., Gilpin, L. C., and Boyd, P. W. (1995). Phytoplankton distributions and production in the Bellingshausen Sea, Austral spring 1992. Deep Sea Res. II 42, 1201–1224. doi: 10.1016/0967-0645(95)00062-u
Schloss, I. R., Wasilowska, A., Dumont, D., Almandoz, G. O., Hernando, M. P., Michaud-Tremblay, C. A., et al. (2014). On the phytoplankton bloom in coastal waters of southern King George Island (Antarctica) in January 2010: An exceptional feature? Limnol. Oceanogr. 59, 195–210. doi: 10.4319/lo.2014.59.1.0195
Schofield, O., Brown, M., Kohut, J., Nardelli, S., Saba, G., Waite, N., et al. (2017). Changes in the upper ocean mixed layer and phytoplankton productivity along the West Antarctic Peninsula. Philos. T. R. Soc. A 376:20170173. doi: 10.1098/rsta.2017.0173
Sen, P. K. (1968). Estimates of the regression coefficient based on Kendall’s tau. J. Am. Stat. Assess. 63, 1379–1389. doi: 10.1080/01621459.1968.10480934
Siegenthaler, U., and Sarmiento, J. (1993). Atmospheric carbon dioxide and the ocean. Nature 365:119.
Smetacek, V., Scharek, R., and Nothig, E.-M. (1990). “Seasonal and regional variation in the pelagial and its relationship to the life cycle of krill,” in Antarctic Ecosystems, Ecological Change and Conservation, eds K. R. Kerry and G. Hempel (Berlin: Springer), 103–114. doi: 10.1007/978-3-642-84074-6_10
Smith, G. C., Allard, R., Babin, M., Bertino, L., Chevallier, M., Corlett, G., et al. (2019). Polar ocean observations: a critical gap in the observing system and its effect on environmental prediction. Front. Mar. Sci. 6:429. doi: 10.3389/fmars.2019.0042
Smith, W. O. Jr., and Nelson, D. M. (1990). Phytoplankton growth and new production in the Weddell Sea MIZ in the austral spring and autumn. Limnol. Oceanogr. 35, 809–821. doi: 10.4319/lo.1990.35.4.0809
Steinacher, M., Joos, F., Frölicher, T. L., Bopp, L., Cadule, P., Cocco, V., et al. (2010). Projected 21st century decrease in marine productivity: a multi-model analysis. Biogeosciences 7, 979–1005. doi: 10.5194/bg-7-979-2010
Strzepek, R. F., Boyd, P. W., and Sunda, W. G. (2019). Photosynthetic adaptation to low iron, light, and temperature in Southern Ocean phytoplankton. Proc. Natl Acad. Sci. U.S.A. 116, 4388–4393. doi: 10.1073/pnas.1810886116
Strzepek, R. F., Hunter, K. A., Frew, R. D., Harrison, P. J., and Boyd, P. W. (2012). Iron-light interactions differ in Southern Ocean phytoplankton. Limnol. Oceanogr. 57, 1182–1200. doi: 10.4319/lo.2012.57.4.1182
Tagliabue, A., Bopp, L., Dutay, J., Bowie, A. R., Chever, F., Jean-Baptiste, P., et al. (2010). Hydrothermal contribution to the oceanic dissolved iron inventory. Nat. Geosci 3, 252–256. doi: 10.1038/ngeo818
Tedesco, L., and Vichi, M. (2014). Sea ice biogeochemistry: a guide for modellers. PLoS One 9:e89217. doi: 10.1371/journal.pone.0089217
Tilstone, G. H., Lange, P. K., Misra, A., Brewin, R. J. W., and Cain, T. (2017). Microphytoplankton photosynthesis, primary production and potential export production in the Atlantic Ocean. Prog. Oceanogr. 158, 109–129. doi: 10.1016/j.pocean.2017.01.006
Treguer, P. J. (2014). The Southern Ocean silica cycle. C. R. Geoscience 346, 279–286. doi: 10.1016/j.crte.2014.07.003
Trimborn, S., Thoms, S., Bischof, K., and Beszteri, S. (2019). Susceptibility of two Southern Ocean phytoplankton key species to iron limitation and high light. Front. Mar. Sci. 6:167. doi: 10.3389/fmars.2019.00167
Trimborn, S., Thoms, S., Brenneis, T., Heiden, J., Beszteri, S., and Bischof, K. (2017). Two Southern Ocean diatoms are more sensitive to ocean acidification and changes in irradiance than the prymnesiophyte Phaeocystis antarctica. Physiol. Plant. 160, 155–170. doi: 10.1111/ppl.12539
Trull, T. W., Passmore, A., Davies, D. M., Smit, T., Berry, K., and Tilbrook, B. (2018). Distribution of planktonic biogenic carbonate organisms in the Southern Ocean south of Australia: a baseline for ocean acidification impact assessment. Biogeosciences 15, 31–49. doi: 10.5194/bg-15-31-2018
Uchida, T., Balwada, D., Abernathey, R., Prend, C. J., Boss, E., and Gille, S. T. (2019). Southern Ocean phytoplankton blooms observed by biogeochemical floats. J. Geophys. Res. Oceans 124, 7328–7343. doi: 10.1029/2019JC015355
Uitz, J., Claustre, H., Griffiths, F. B., Ras, J., Garcia, N., and Sandroni, V. (2009). A phytoplankton class− specific primary production model applied to the Kerguelen islands region (Southern Ocean). Deep Sea Res. I 56, 541–560. doi: 10.1016/j.dsr.2008.11.006
Vacchi, M., La Mesa, M., Dalù, M., and Macdonald, J. (2004). Early life stages in the life cycle of Antarctic silverfish, Pleuragramma antarcticum in Terra Nova Bay, Ross Sea. Antarc. Sci. 16, 299–305. doi: 10.1017/s0954102004002135
Van de Poll, W., Lagunas, M., de Vries, T., Visser, R. J. W., and Buma, A. G. J. (2011). Non−photochemical quenching of chlorophyll fluorescence and xanthophyll cycle responses after excess PAR and UVR in Chaetoceros brevis, Phaeocystis antarctica and coastal Antarctic phytoplankton. Mar. Ecol. Prog. Ser. 426, 119–131. doi: 10.3354/meps09000
van Leeuwe, M. A., Tedesco, L., Arrigo, K. R., Assmy, P., Campbell, K., Meiners, K. M., et al. (2018). Microalgal community structure and primary production in Arctic and Antarctic sea ice: a synthesis. Elem. Sci. Anth. 6:4. doi: 10.1525/elementa.267
Varela, M., Fernandez, E., and Serret, P. (2002). Size-fractionated phytoplankton biomass and primary production in the Gerlache and south Bransfield Straits (Antarctic Peninsula) in Austral summer 1995–1996. Deep Sea Res. II 49, 749–768. doi: 10.1016/s0967-0645(01)00122-9
Vaughan, D. G., Comiso, J. C., Allison, I., Carrasco, J., Kaser, G., Kwok, R., et al. (2013). “Observations: cryosphere,” in Climate Change 2013: The Physical Science Basis, Contribution of Working Group I to the Fifth Assessment Report of the IPCC, eds T. F. Stocker, G.-K. Qin, M. Plattner, M. M. B. Tignor, S. K. Allen, J. Boschung, et al. (Cambridge: Cambridge University Press), 317–382.
Verlencar, X. N., Somasunder, K., and Qasim, S. Z. (1990). Regeneration of nutrients and biological productivity in Antarctic Waters. Mar. Ecol. Prog. Ser. 61, 41–59. doi: 10.3354/meps061041
Vernet, M., Martinson, D., Iannuzzi, R., Stammerjohn, S., Kozlowski, W., Sines, K., et al. (2008). Primary production within the sea-ice zone west of the Antarctic Peninsula: I-sea ice, summer mixed layer, and irradiance. Deep Res. Part II 55, 2068–2085. doi: 10.1016/j.dsr2.2008.05.021
Vernet, M., Sines, K., Chakos, D., Cefarelli, A., and Ekern, L. (2011). Impacts on phytoplankton dynamics by free-drifting icebergs in the NW Weddell Sea. Deep Sea Res. Part II Top. Stud. Oceanogr. 58, 1422–1435. doi: 10.1016/j.dsr2.2010.11.022
von Bodungen, B., Smetacek, V. S., Tilzer, M. M., and Zeitzschel, B. (1986). Primary production and sedimentation during spring in the Antarctic Peninsula region. Deep Sea Res. I 33, 177–194. doi: 10.1016/0198-0149(86)90117-2
Wallcraft, A. J., Metzger, E. J., and Carroll, S. N. (2009). Software Design Description for the HYbrid Coordinate Ocean Model (HYCOM), Version 2.2. US Naval Research Laboratory Report, NRL/MR/7320-09-9166. Available online at: https://www.hycom.org/hycom/documentation/63-hycom-users-manual-and-guide (accessed June, 2020).
Westberry, T., Behrenfeld, M. J., Siegel, D. A., and Boss, E. (2008). Carbon-based primary productivity modeling with vertically resolved photoacclimation. Glob. Biogeochem. Cycles 22:GB2024. doi: 10.1029/2007GB003078
Wright, S. W., van den Enden, R. L., Pearce, I., Davidson, A. T., Scott, F. J., and Westwood, K. J. (2010). Phytoplankton community structure and stocks in the Southern Ocean (30–80E) determined by CHEMTAX analysis of HPLC pigment signatures. Deep Sea Res. Part II 57, 758–778. doi: 10.1016/j.dsr2.2009.06.015
Yue, S., and Wang, C. Y. (2004). The Mann-Kendall test modified by effective sample size to detect trend in serially correlated hydrological series. Water Res. Manag. 18, 201–218. doi: 10.1023/B:WARM.0000043140.61082.60
Zhang, L., Delworth, T. L., Cooke, W., and Yang, X. (2018). Natural variability of Southern Ocean convection as driver of observed climate trends. Nat. Clim. Change 9, 59–65, doi: 10.1038/s41558-018-0350-3
Keywords: phytoplankton, climate, Antarctica, biogeochemistry, deep chlorophyll maximum, ocean colour, MODIS, SeaWiFS
Citation: Pinkerton MH, Boyd PW, Deppeler S, Hayward A, Höfer J and Moreau S (2021) Evidence for the Impact of Climate Change on Primary Producers in the Southern Ocean. Front. Ecol. Evol. 9:592027. doi: 10.3389/fevo.2021.592027
Received: 06 August 2020; Accepted: 19 February 2021;
Published: 17 May 2021.
Edited by:
Andrew John Constable, Independent Researcher, Hobart, AustraliaReviewed by:
Clara Jule Marie Hoppe, Alfred Wegener Institute Helmholtz Centre for Polar and Marine Research (AWI), GermanyCopyright © 2021 Pinkerton, Boyd, Deppeler, Hayward, Höfer and Moreau. This is an open-access article distributed under the terms of the Creative Commons Attribution License (CC BY). The use, distribution or reproduction in other forums is permitted, provided the original author(s) and the copyright owner(s) are credited and that the original publication in this journal is cited, in accordance with accepted academic practice. No use, distribution or reproduction is permitted which does not comply with these terms.
*Correspondence: Matthew H. Pinkerton, bS5waW5rZXJ0b25Abml3YS5jby5ueg==
Disclaimer: All claims expressed in this article are solely those of the authors and do not necessarily represent those of their affiliated organizations, or those of the publisher, the editors and the reviewers. Any product that may be evaluated in this article or claim that may be made by its manufacturer is not guaranteed or endorsed by the publisher.
Research integrity at Frontiers
Learn more about the work of our research integrity team to safeguard the quality of each article we publish.