- 1Department of Biology, Queen’s University, Kingston, ON, Canada
- 2CEFE UMR 5175, Université de Montpellier, CNRS, EPHE, IRD, Université Paul-Valery Montpellier 3, Montpellier, France
Climate change is forecasted to generate a range of evolutionary changes and plastic responses. One important aspect of avian responses to climate change is how weather conditions may change nestling growth and development. Early life growth is sensitive to environmental effects and can potentially have long-lasting effects on adult phenotypes and fitness. A detailed understanding of both how and when weather conditions affect the entire growth trajectory of a nestling may help predict population changes in phenotypes and demography under climate change. This review covers three main topics on the impacts of weather variation (air temperature, rainfall, wind speed, solar radiation) on nestling growth. Firstly, we highlight why understanding the effects of weather on nestling growth might be important in understanding adaptation to, and population persistence in, environments altered by climate change. Secondly, we review the documented effects of weather variation on nestling growth curves. We investigate both altricial and precocial species, but we find a limited number of studies on precocial species in the wild. Increasing temperatures and rainfall have mixed effects on nestling growth, while increasing windspeeds tend to have negative impacts on the growth rate of open cup nesting species. Thirdly, we discuss how weather variation might affect the evolution of nestling growth traits and suggest that more estimates of the inheritance of and selection acting on growth traits in natural settings are needed to make evolutionary predictions. We suggest that predictions will be improved by considering concurrently changing selection pressures like urbanization. The importance of adaptive plastic or evolutionary changes in growth may depend on where a species or population is located geographically and the species’ life-history. Detailed characterization of the effects of weather on growth patterns will help answer whether variation in avian growth frequently plays a role in adaption to climate change.
Introduction
Avian growth can affect fledgling survival and recruitment, and early life experiences are known to impact adult phenotypes (Haywood and Perrins, 1992; Lindström, 1999; Maness and Anderson, 2013; Merrill and Grindstaff, 2018). Given gradual warming and increasingly stochastic weather conditions under climate change, some growth strategies might result in higher fitness than others. Therefore, predicting future changes in fitness and demography under climate change could depend on understanding the effects of current weather variation on offspring growth. The consequences of weather variation on offspring development will likely be complex and will, to some degree, depend on a species’ life-history and ecological niche.
Growth patterns in birds vary, and differences among populations may be adaptations to their local environments (Ricklefs, 1968; Emlen et al., 1991; Starck and Ricklefs, 1998). Global patterns of variation in avian growth within species can generate hypotheses for how the changing climate will influence growth-related traits. For example, given the observation that individuals tend to be smaller in warmer habitats both within and across species (Bergmann’s Rule; Bergmann, 1847 as cited in Salewski and Watt, 2017) we might predict that for a given species, natural selection will favor nestlings that fledge at a smaller size in a warmer climate (Millien et al., 2006). Introduced House Sparrows Passer domesticus in North America and Australia both exemplify predictions from Bergmann’s rule: birds in the colder regions of both continents are on average larger (Johnston and Selander, 1964, 1973; Andrew et al., 2018). Clines in body size could be driven by natural selection developmental constraints, or both (Fleischer and Johnston, 1984; Andrew et al., 2018). Effects on size during development are important because many bird species approach their final structural size before fledging, meaning there may be little opportunity for compensation later in life (Gill and Prum, 2019).
Plastic adjustment of growth could allow rapid adaptation to changing conditions, but evolution of growth traits might be required if current environmental cues no longer predict future environments. To predict possible phenotypic changes in nestling growth we need to understand how weather variation affects nestling growth, how new weather conditions under climate change are changing the selection on avian growth traits, and the additive genetic and environmental variance of growth traits. Some studies have identified carry-over effects of growth on adult fitness with negative and positive consequences for body size (Gardner et al., 2011; Teplitsky and Millien, 2014; van Gils et al., 2016), but few studies examine whether patterns in growth are adaptive or maladaptive. Examining growth-environment relationships jointly with patterns of genetic change is important because phenotypic plasticity can mask evolutionary change (Merilä et al., 2001; Bonnet et al., 2017; Dobson et al., 2017), and plastic responses can contribute to an adaptive or maladaptive response to changing weather conditions (Ghalambor et al., 2007; Snell-Rood et al., 2018). An essential aspect of studying growth is to quantify it in a way that allows among population, individual and genetic variation to be estimated (Box 1).
BOX 1. Methods for quantifying growth.
Avian growth can be investigated using a broad range of traits and different statistical modeling approaches. Often, measurements of size or shape near fledging are used because these are more comparable across studies and can predict post-fledging survival (Maness and Anderson, 2013). However, single time point measurements can hide variation in growth among individuals if individuals follow different trajectories to the same final size (Figure 1).
Repeated measurements made throughout nestling development can be used to fit a curve to the data as a function of age. For growth data, logistic models are often used, and the model’s asymptote, maximum relative growth rate, and timing of maximum growth may be estimated as biological parameters of interest describing growth (Figures 1, 2A; Tjørve and Tjørve, 2010; Aldredge, 2016). The unified family of growth models proposed by Tjørve and Tjørve (2010, 2017) allows flexible, comparable, and biologically interpretable estimates from various growth model families. Biologically, the asymptote should provide an estimate of the maximum size reached by a nestling. However, the asymptote in many models may not be a good indication of a nestling’s fledging mass, as a nestling could fledge before the maximum mass is reached, or in some avian species nestlings reach an asymptote and then decline in mass before fledging.
Such function-valued trait approaches summarize the growth trajectory and enable researchers to explore whether different environments affect the growth rate, timing, or size of a nestling. Linear and polynomial functions could also be used, with the terms of the polynomial being treated as the parameters of interest. Instead of fitting functions to a growth trajectory, sometimes the average difference between consecutive daily measurements (e.g., Peck et al., 2004; Harter, 2007; Divoky et al., 2015) or the daily deviation from growth in ideal conditions are used as an indication of daily growth (Keller and Noordwijk, 1993).
Character-state models provide a flexible method for modeling growth, where one can estimate age-specific measurements as separate, yet correlated traits (Roff and Wilson, 2014; Figure 2B). Note that character-state and polynomial functions are equivalent in many situations. In a character-state approach covariances among age-specific masses can be fitted across all ages, or be restricted to consecutive ages only, in what is called an “ante-dependence” model (see the supplementary material of Hadfield et al., 2013; Thomson et al., 2017; Hadfield, 2019). Both function-valued trait and character state approaches to modeling growth are identical to models that measure trait plasticity across environments, but replace environmental variation with age (Falconer, 1952; Via and Lande, 1985; Jong, 1990; Gavrilets and Scheiner, 1993; Chevin et al., 2013). Function-valued trait approaches can perform poorly if individuals or genotypes have different curve shapes and can be harder to interpret biologically (Roff and Wilson, 2014). Generally, function-valued trait approaches use fewer parameters. Therefore, when sufficient data are available, comparison of function-valued and character-state approaches might be valuable in determining when during ontogeny function-valued trait approaches are not capturing variation during growth (Morrissey and Liefting, 2016). If a linear model is used, environmental effects on the slope or intercept of the line could be detected, yet a gain or loss of mass at any specific time during growth, which could be biologically important, would be difficult or impossible to detect. A character-state might be better able to determine when during ontogeny the environment affects a trait. Houslay (2017) provides accessible tutorials implemented in the R package MCMCglmm for both character state and function-valued trait approaches that could be used to model avian growth (Hadfield, 2010).
Function-valued trait and character-state approaches can be analyzed in a mixed model framework, so that hierarchical (co)variances are estimated (function-valued trait approach, Aldredge, 2016; character-state approach, Thomson et al., 2017). Further, Bayesian models now allow the fitting of flexible models that can estimate the fixed effects of weather and environmental variation on specific parameters or age-specific traits in a single model (Hadfield, 2010; Bürkner, 2017).
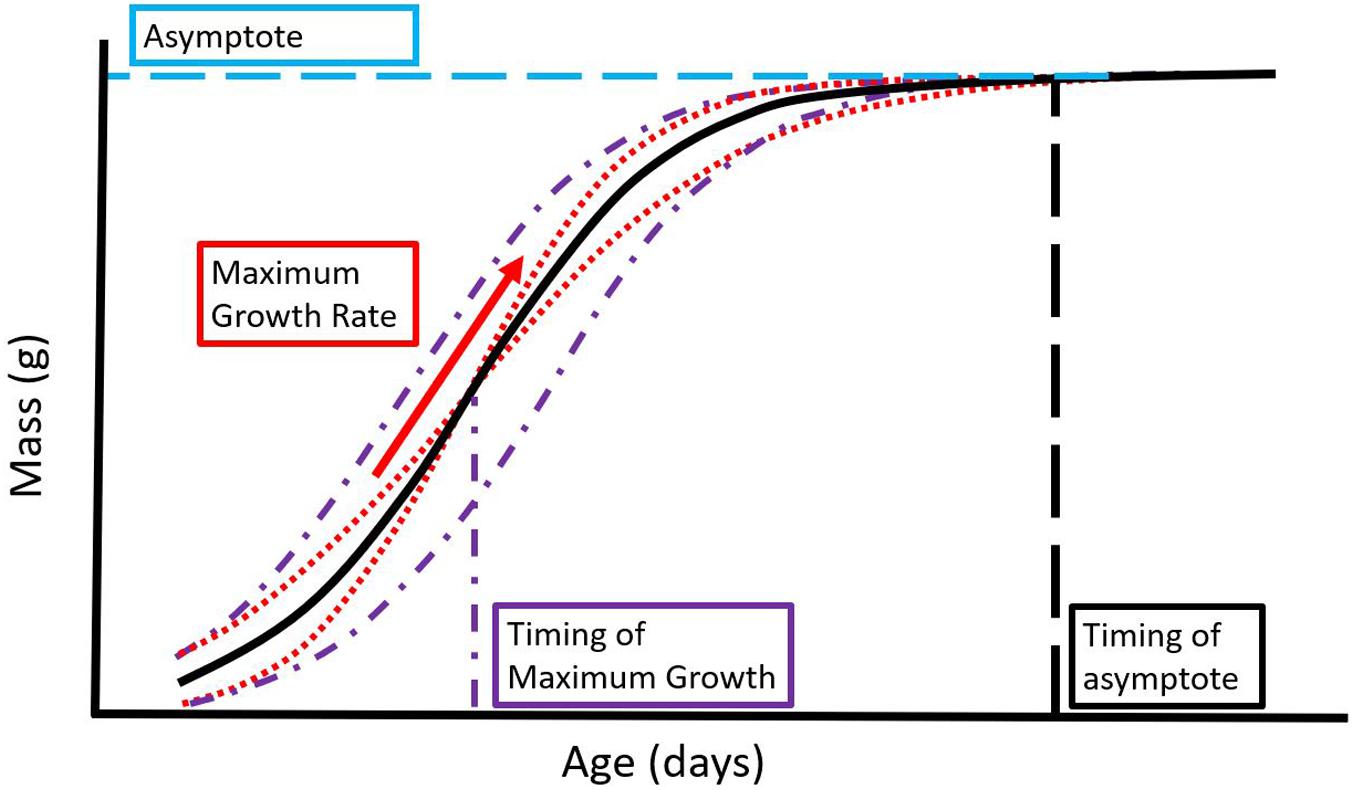
Figure 1. Parameters estimated in a standard logistic curve. The maximum growth rate (red arrow) gives an indication of the speed of growth, the asymptote gives an indication of the nestlings’ maximum size achieved during the period of study (blue horizontal dash), the timing of maximum growth gives an indication of when during ontogeny maximum growth occurs (purple/vertical dash + dotted line), and the timing of the asymptote might indicate developmental timing (black vertical dashed line). Five growth curves are shown, with the same asymptote but varying in the timing of maximum growth (purple dash and dotted lines) or in maximum growth rate (red dotted lines). All curves have the same asymptote as the reference curve (thick black curve) but vary in their trajectory. If a study of nestling growth only measures mass at the asymptote as in this example, variation throughout the trajectory of the growth curve is hidden. Whether this variation impacts fitness is currently unknown.
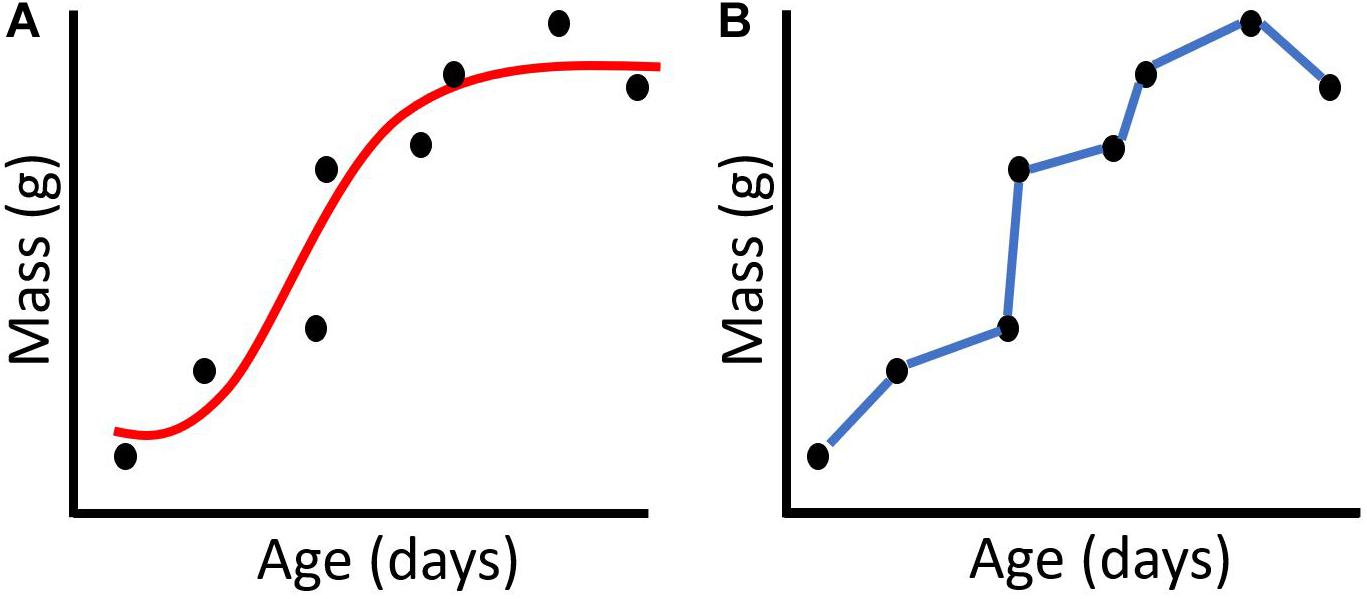
Figure 2. Hypothetical example of a (A) function-valued trait approach and a (B) character-state approach for modeling a chick growth curve.
In this review, we (1) highlight why studying weather-related changes in avian growth might be important to understand avian responses to climate change, (2) review the currently documented effects of weather on avian growth in wild populations, and (3) highlight that understanding the selection operating on growth traits and the inheritance of growth traits is needed to predict future phenotypic responses. We define weather as local within-year variation in temperature (of air and sea surface), solar radiation, precipitation and wind, and climate as the average weather across multiple years for a given region. We explore the effects of minimum, maximum, average, and duration (heatwaves, rainfall) of weather variables.
Importance of Studying Weather-Related Changes in Avian Growth
Understanding weather-related changes in avian growth is important for two reasons: some individuals or genotypes might survive better than others in stressful weather conditions, and weather variation may change a population’s demography through influences on nestling traits.
Plasticity of Avian Growth and Development
Because of genetic, environment, or parental effects individuals will differ in their growth. Understanding the causes of these growth differences is important because some individuals may have higher fitness under changing conditions (Kruuk, 2004; Wilson et al., 2010). In theory, the same individual or genotype could also produce several different phenotypes given different weather conditions (phenotypic plasticity), and the plasticity of an individual’s phenotype could also vary among individuals because of genetic, permanent environment, or parental effects. Plastic changes have gained recognition as common responses to changing environmental conditions (Ghalambor et al., 2007; Charmantier and Gienapp, 2014; Snell-Rood et al., 2018). Central questions in evolutionary ecology are (1) whether plasticity will allow adaptation to new weather conditions, and (2) how plasticity might affect the rate of evolutionary change.
Studying the plasticity of avian growth requires the estimation of how growth patterns can change for a given individual (or a given genotype) across different environments. Such measures are impossible at the individual-level because a bird only grows once. However, in long-lived and philopatric species, associations between growth and weather variation may be likely to result from plastic rather than evolutionary responses because the population genetic background may change little from 1 year to another. In a wild setting, a cross-fostering experiment across a weather gradient (experimental or natural) may reveal if different families have on average different or similar growth patterns across environments (for a discussion on artificial breeding designs, see Roff and Wilson, 2014). To our knowledge, no studies have analyzed cross-fostering in wild birds, with the goal of measuring differences among families in changes in growth patterns across weather gradients.
Because a proportion of the variation in growth traits are determined by parental behavior we might be able to measure the plasticity of nestling growth by considering nestling growth traits as parental traits in species where faithful pairs repeatedly reproduce (Lloyd and Martin, 2004; Wilson et al., 2005). Nestling growth traits that are measured across years for parents can then be considered repeated measures of a parental trait. We can then try to examine the plasticity of the parental care contribution to nestling growth by examining how nestling growth traits vary across environments for a given parent or pair of parents. Importantly, because reproductive investment is a complex function of age and parental condition, such a model will require careful consideration of parental age or breeding experience (McCleery et al., 2008) and the weather conditions parents experience. Consequently, informing such a model will likely require large sample sizes but will provide valuable information on the suite of growth responses individual parents or parental genotypes could generate.
One of the challenges in studying plastic responses in wild populations is determining the environmental variable to which an organism responds (Scheiner, 1993; Gienapp and Brommer, 2014). This problem is further complicated because a trait could be affected by multiple weather variables, interacting weather variables, or even the same weather variables in different ways at different times throughout ontogeny (Figure 3). Recent statistical techniques try to address the difficulty of identifying time windows of weather variation that affect a trait of interest (van de Pol et al., 2016). The van de Pol et al. (2016) method is an exploratory approach that compares multiple windows within a period of time (relative to the biological variable or an absolute time frame) to determine those that best predict (relative to all windows examined) the trait of interest. To understand when during the breeding season weather affects nestling growth, it may be useful to explore weather variation within the prelaying, prenatal, and growth stages (Figure 3). Importantly, we mainly discuss physical growth in this review, but many of the impacts of weather also affect the development (e.g., cognition, thermoregulation, motor function) of nestlings. Understanding how and when during the breeding season development is affected by weather is an additional complexity that will be important to study for a more complete understanding of the impacts of weather.
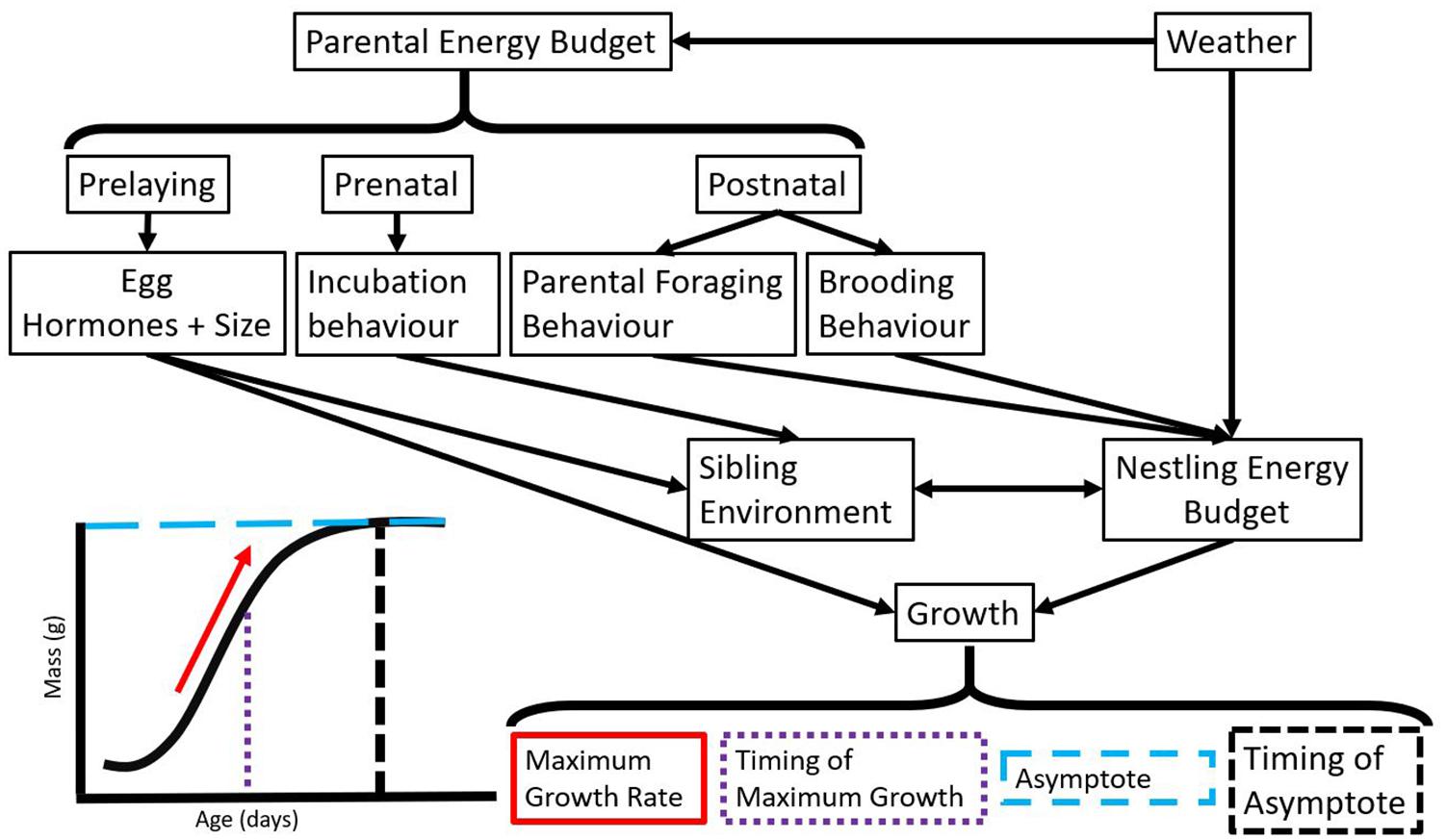
Figure 3. Paths by which weather can impact variation in growth as described by parameters estimated from a general logistic curve. While we display physical growth in this figure, it will be useful to map the development of tissue functions (e.g., cognition, thermoregulation, motor function) onto this growth curve to compare and contrast development and physical growth over the nestling period. Many of the factors that impact growth are also likely to impact development.
In the context of growth and development, identifying environmental correlates may still be challenging because weather variation among prelaying, prenatal, and postnatal periods might be tightly correlated – making it difficult to disentangle during which period weather variation affects a trait. For more easily manipulated weather effects like temperature, experimental work may allow researchers to measure the effects of different time windows by manipulating temperature during specific time periods (Andrew et al., 2017; Andreasson et al., 2018, 2020a; Figure 3). Exploring effects of weather variation outside the breeding season will be valuable if weather patterns are expected to have long-term effects on the resources available or the condition of parents during the breeding season. Understanding the environmental cues that affect the expression of a character is essential because plasticity might allow persistence in the face of changing weather conditions (Vedder et al., 2013), but could also increase the speed of extinction if cues are no longer informative (Reed et al., 2010). Further, in seabirds nestling growth is routinely used as an indicator of environmental conditions (Cairns, 1988). Inferences from such traits require an intimate understanding of the relationship between environmental variation and the measured biological trait (Grémillet and Charmantier, 2010; Brisson-Curadeau et al., 2017).
Nestling Growth and Demography
Weather effects on nestling traits can also affect the demographics of bird populations (Bryant, 1978; Salaberria et al., 2014). In many avian species, growth is likely to affect survival to fledging (nestling survival) and post-fledging survival (juvenile survival). In a review of predictors of juvenile survival, Maness and Anderson (2013) found (in a subset of quality-controlled studies) that nine out of ten studies identify an effect of nestling mass on juvenile survival, but noted that few studies assess whether growth rates affected juvenile survival. Given evidence that growth traits can influence nestling and juvenile survival (Maness and Anderson, 2013), the effects of variation in growth caused by weather patterns might most strongly affect demography in short-lived bird species (Sæther and Bakke, 2000). However, if nestling growth traits determine adult survival or fecundity, weather-induced nestling variation might change the demography of a broad range of avian species. During early life, environmental conditions, like weather, are expected to play a role in shaping adult phenotypes and fitness (Lindström, 1999). A meta-analysis of the effects of early developmental conditions in birds and mammals found that poor early developmental conditions increases the rate of decline in fecundity with age, but developmental conditions do not influence the age-specific decline in survival in adults (Cooper and Kruuk, 2018). However, the latter result relies heavily on mammal studies, since only one avian study in this meta-analysis investigates the effects of the developmental environment on survival senescence (Hammers et al., 2013). Future studies on birds should investigate the effects of early life conditions (including weather) on traits expressed in adults.
Documented Weather Effects on Avian Growth
Understanding current responses to weather enable us to extrapolate responses to predict future changes. Here, we review the effects of weather on growth and discuss the different ontogenic pathways through which weather could impact growth (Figure 3). To cover studies investigating the effect of weather on nestling growth, we searched in the ISI Web of Science on November 2nd, 2020. We used the keywords ‘avian’ OR ‘bird’ + ‘growth’ OR ‘development’ + ‘climate change’ OR ‘global warming’ OR ‘weather’ OR ‘temperature’ OR ‘wind’ OR ‘rainfall’ OR ‘precipitation’ OR ‘sunshine’ OR ‘solar radiation’ OR ‘solar’ OR ‘insolation’ OR ‘experiment’ + ‘ offspring’ OR ‘juvenile’ OR ‘nestling,’ which brought up 989 references. We restricted papers to experimental and observational studies that examined the effect of weather variation on nestling growth, leaving 36 studies. Specifically, we retained studies that examined the effect of weather variables (wind, precipitation, solar radiation, temperature, ice- cover, sea-surface temperature) on nestling phenotypes during the growth period. Weather effects identified from our literature search are included in Tables 1–4.
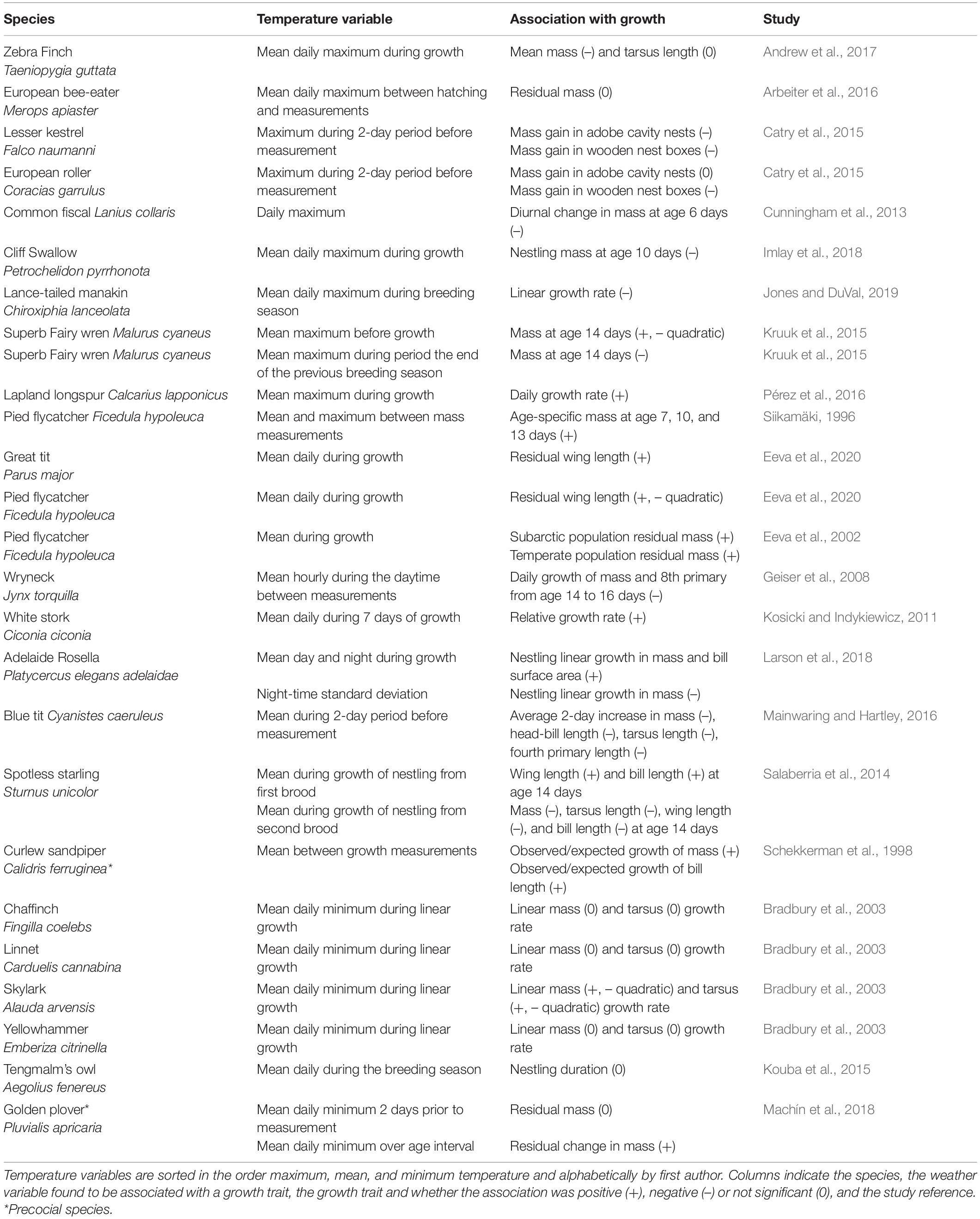
Table 1. Associations between aspects of temperature and nestling growth traits identified from our literature search.
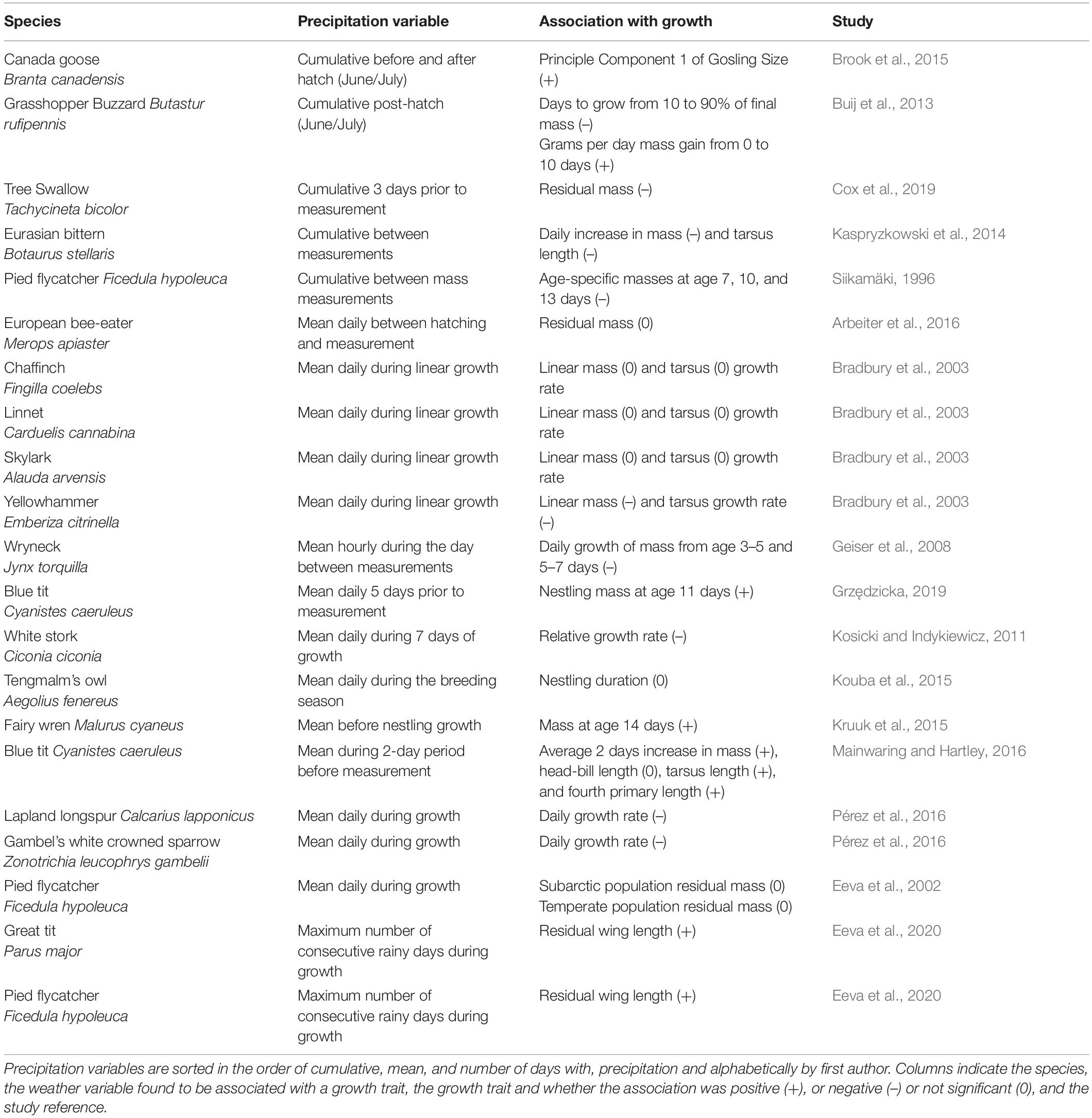
Table 2. Associations between aspects of precipitation and nestling growth traits identified from our literature search.
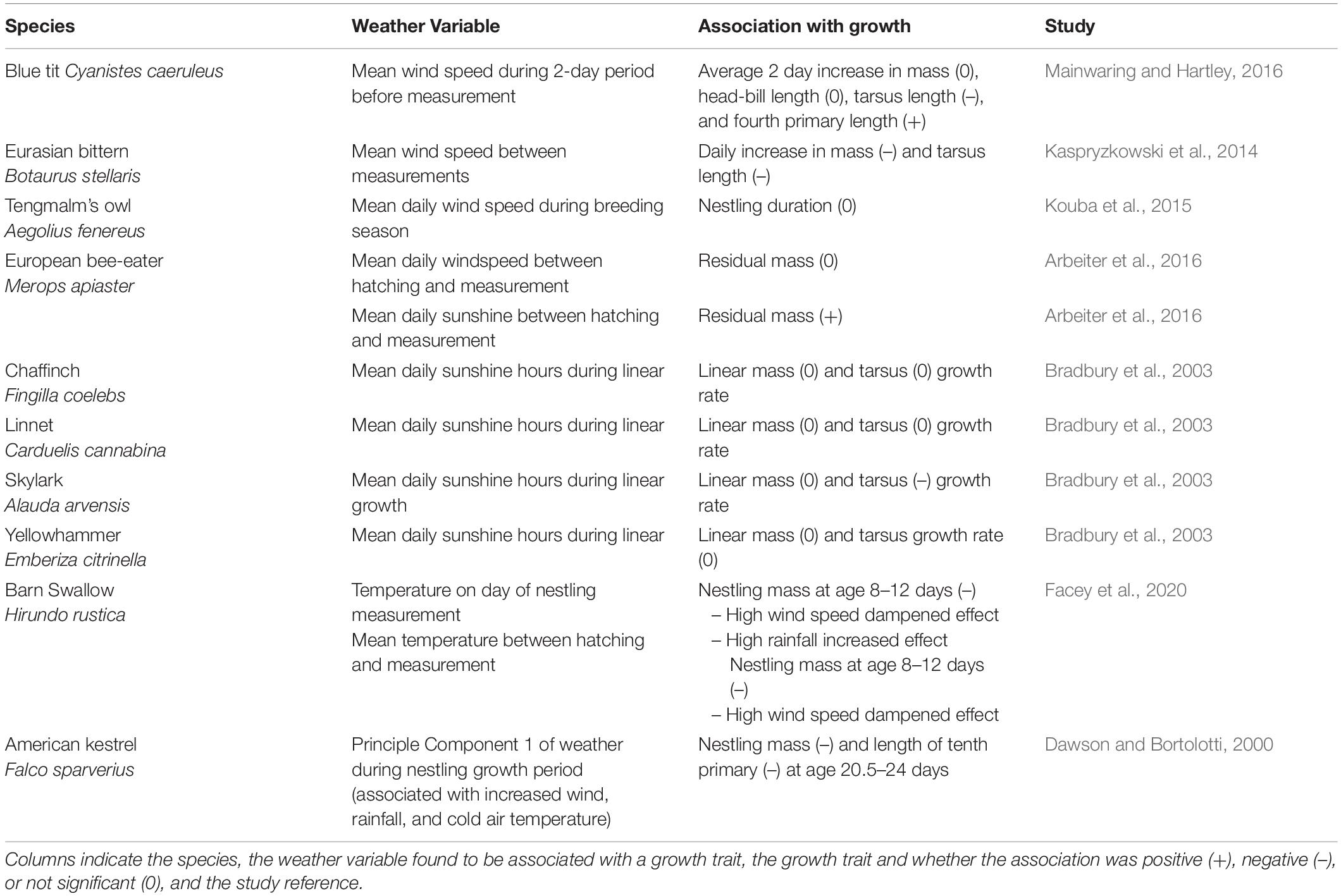
Table 3. Associations between combined weather effects, wind speed, sunshine, and nestling growth traits identified from our literature search.
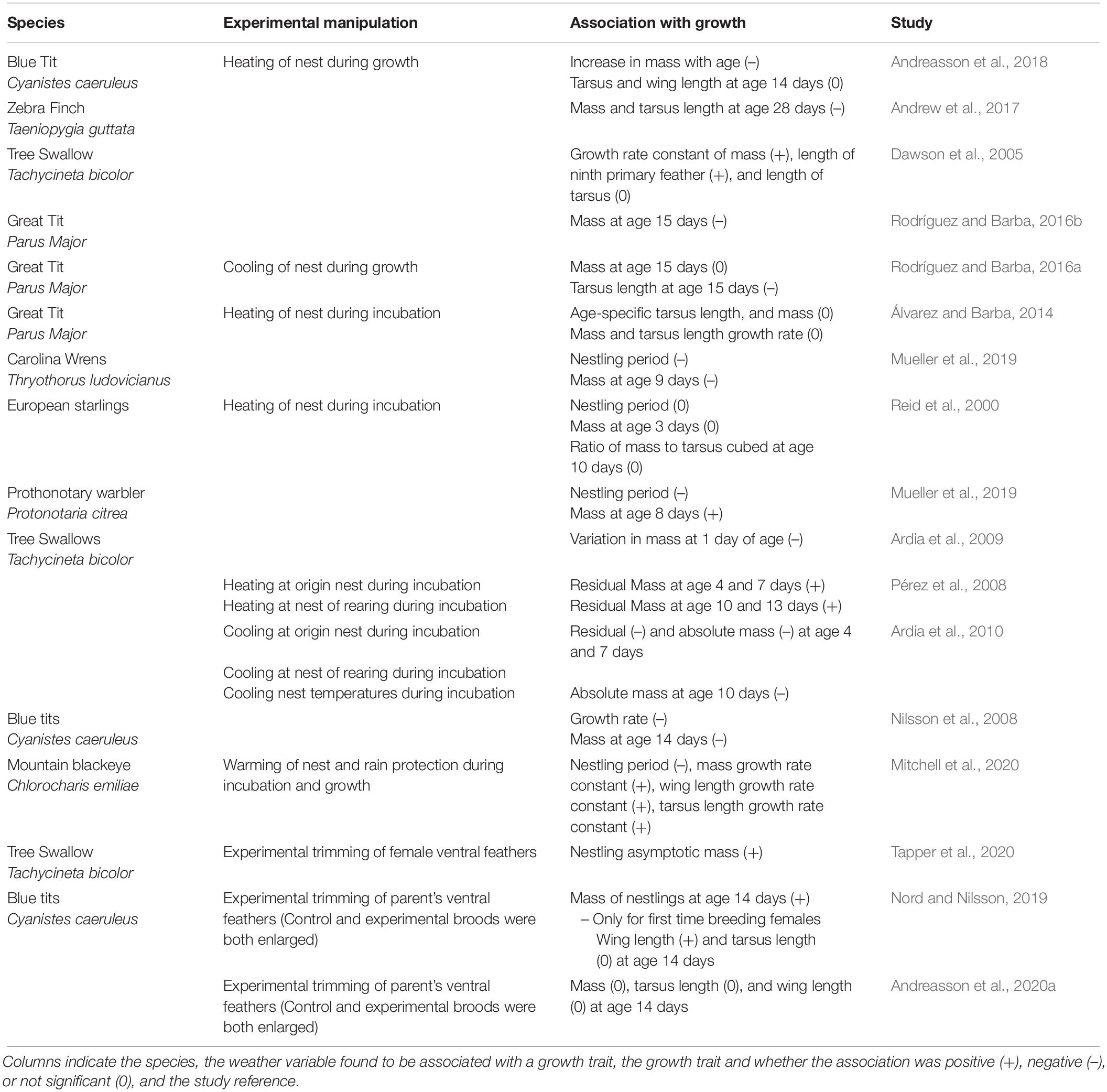
Table 4. Associations between experimental manipulations of environmental conditions and nestling growth traits.
Weather Impact of Growth Through Prenatal Effects
In addition to any effects of weather conditions on nestling growth in birds, conditions during embryonic development could affect post-hatching growth. A mother may influence the size of an egg and the hormones in an egg, potentially in response to environmental cues (Love and Williams, 2008; Bentz et al., 2013). The importance of egg size on nestling growth traits is somewhat uncertain. Effects of short-term weather variation on egg size tend to be limited (Nager and Noordwijk, 1992; Christians, 2002; Thomson and Hadfield, 2017; Griffith et al., 2020), and some studies note that egg size affects hatching traits, but this effect deteriorates through ontogeny (Krist, 2011; Williams, 2012).
Weather variation can, but does not always, influence maternal hormones transported into the egg, and these components can affect nestling growth (Schwabl, 1996; Groothuis et al., 2005; Addison et al., 2008; Groothuis and Schwabl, 2008; Ruuskanen et al., 2016). In particular, weather impacts on food may have a strong effect on maternal hormones deposited in the egg and could aid the matching of brood size to environmental conditions (Verboven et al., 2003; Gasparini et al., 2007; Vergauwen et al., 2012; Benowitz-Fredericks et al., 2013; Müller and Groothuis, 2013; Merkling et al., 2016). Other than hormonal influences, weather could also indirectly impact nestling growth through antioxidants, immunoglobins and antimicrobial agents (Williams, 2012), but these effects might be more indirect, as these compounds are less likely to affect growth directly and instead likely help nestlings survive in the face of environmental challenges (e.g., parasites).
If weather patterns during the prenatal period can predict the environment that a nestling will develop and grow in, a mother may be able to provide cues or influence the phenotype of her offspring to better match its future environment. However, the extent to which mothers provide such signals, and whether these signals are adaptive, is mostly untested in the wild. Whether a species has such cues may depend on the predictability of the environment it evolved in, and whether such cues remain adaptive will depend on how the predictability of the environment changes. Parents may even provide auditory cues to their prenatal offspring to signal warm environments resulting, for instance, in lighter 13-day old nestlings in zebra finches Taeniopygia guttata (Mariette and Buchanan, 2016).
Predicting changes in growth caused by climate change may depend on knowing the effects of an embryo’s thermal environment on the nestling phenotype. Weather can directly affect the thermal environment that avian embryos experience and influence parental incubation behavior (DuRant et al., 2010; Griffith et al., 2016). Embryo thermal tolerance varies among species, with species from colder climates tending to have broader tolerances and lower optimums (Webb, 1987). However, even temperature variation within the range tolerated by an embryo can impact a nestling phenotype (Hepp et al., 2006; DuRant et al., 2010; Nord and Nilsson, 2011).
More experiments warming nest microclimates during incubation on a wide range of species might help clarify the impacts of a warmer incubation environment on nestling growth. Nest microclimate experiments are valuable because they reveal the combined effect of indirect thermal effects on the parents and direct effects on embryos (Table 4). Current results from experiments that warm the nest microclimate are variable and different species from the same study location can have contrasting effects on nestling growth (e.g., Mueller et al., 2019; Table 4). Few studies have experimentally cooled nests, but those that do find that it decreases nestling size and growth (Table 4). A cross-fostering experiment in tree swallows provides an interesting insight because nest warming during incubation increases the early age mass of nestlings incubated in a warm nest (raised by parents in a control nest) and the late-age mass of nestlings incubated in a control nest but raised by parents from a warm nest (Pérez et al., 2008). The cross-fostering experiment suggests carry-over effects of warming on parental care during growth and direct benefits of warming on young nestlings.
Post-hatching Weather Effects on Avian Growth
Variation in weather during the growth period is likely to affect chicks both directly and indirectly. Growth can be influenced by changes in thermal environments (e.g., Cunningham et al., 2013; McKinnon et al., 2013; Tables 1, 4), and changes in food availability and parental care (e.g., Keller and Noordwijk, 1994). Scientists have long been interested in the effects of weather on growth and both historical and recent studies provide a baseline for making qualitative and quantitative predictions for changes in growth under different weather conditions (Lack and Lack, 1951; Hawksley, 1957; Tables 1–4). Predicting how climate change will impact avian growth depends on understanding how nestlings are affected by different weather components and how these weather components are expected to change under climate change.
A Warmer Growing Environment Can Positively or Negatively Impact Nestling Growth
Average global temperatures are expected to continue to increase with climate change, and for many regions, the frequency of heatwaves and variability of thermal environments are expected to increase (Intergovernmental Panel on Climate Change, 2018). Increasing temperatures can result in either challenging or improved growing conditions for nestlings and can operate either directly through thermal changes experienced by nestlings or indirectly by affecting parents’ foraging or brooding behavior (Andreasson et al., 2020b; Table 1 and Figure 3). The effects of warming may vary among species, populations, and environments because some populations may be closer to their thermal limits or may be more susceptible to dehydration. For example, species living in environments below their thermal optimum may benefit from increased natural air temperatures during development (e.g., McKinnon et al., 2013), while increased air temperatures or heatwaves may have strong negative impacts on species living close to their thermal limits (Conradie et al., 2019). The effect of thermal changes depends on the ability of nestlings to thermoregulate. If temperatures are beyond a nestling’s thermal neutral zone (the temperature range that body temperature is maintained with little energy expenditure), nestlings will have inefficient physiological processes unless they allocate energy to thermoregulation. Further, nestlings might use limited energy resources on thermoregulation, instead of growth, to prevent body temperatures outside their thermal maximum (e.g., Andreasson et al., 2018).
In many bird species, warmer temperatures are associated with faster growth, heavier asymptotes, heavier age-specific masses, or have no effects at all (Dyrcz, 1974; Hiraldo et al., 1990; McCarty and Winkler, 1999; see Table 1). But, warming temperatures can also correlate with slower growth and smaller nestlings. In a population of blue tits in Lancashire in the United Kingdom, warmer air temperatures correlate with slower increases of tarsus length, head size, feather length, and body mass (Mainwaring and Hartley, 2016), and in nestling Eastern kingbirds Tyrannus tyrannus high temperatures covary with smaller age-specific masses (Murphy, 1985). Similarly, in arid environments, warmer temperatures correlate with smaller nestlings (Cunningham et al., 2013; Wiley and Ridley, 2016; Andrew et al., 2017; Van de Ven, 2017). In arid environments, warming may frequently approach the thermal maximum of many bird species, resulting in smaller nestlings and increases in nestling mortality either from decreases in parental foraging or direct challenges for nestlings (Cunningham et al., 2013; Wiley and Ridley, 2016; Van de Ven, 2017; Conradie et al., 2019). Studies that experimentally heat passerine nestlings tend to find that warmer conditions slow growth and result in smaller nestlings in warm climates (Rodríguez and Barba, 2016b; Andrew et al., 2017; Table 4), but that warmer conditions have a positive or mixed effect on nestling mass and size in cool temperate environments (Dawson et al., 2005; Andreasson et al., 2018; Table 4). In many instances, it will be important to explore specific aspects of temperature (e.g., maximum, minimum, mean) because they may affect growth differently (Table 1). For example, crimson rosella Platycercus elegans nestlings are heavier when the minimum nest temperature is high, but nestlings are lighter when the mean nest temperature is high (Larson et al., 2015). Finally, birds that produce several broods could face different weather conditions for each brood. For example, because temperatures are warmer when spotless starlings Sturnus unicolor produce their second brood, increasing temperatures result in smaller 14-day old nestlings, while the opposite effect is found for first broods (Salaberria et al., 2014). Different temperature regimes during early and late broods suggest nestlings in late broods might be more strongly impacted by warming conditions unless parents can adjust nesting locations (presumably to cooler locations) for their second broods.
Sensitivity to Thermal Variation Could Vary Throughout Nestling Ontogeny
To understand the impacts of temperature variation, we also need to investigate the potentially varying impacts of a thermal challenge during different time points in ontogeny. To predict warming effects on growth patterns it may be important to identify periods of weather within a season that might impact growth and determine how these time periods are predicted to change.
Development of thermoregulation varies among species, but endothermy develops after hatching in birds and earlier during ontogeny in precocial species than in altricial species (Dunn, 1975; Whittow and Tazawa, 1991; Nichelmann and Tzschentke, 2002; Price and Dzialowski, 2018). Because of better thermoregulatory abilities, young precocial nestlings may be better able to survive temperature challenges than young altricial species (Hohtola and Visser, 1998). Within a species, mass is an important determinant of thermoregulatory ability (Visser, 1998), but most studies investigate relationships between size and thermoregulation among species and it is unknown how this relates to variation among nestlings within a species. In the context of a warming environment, smaller birds could be better able to cope with heat stress through faster evaporative cooling (McKechnie and Wolf, 2010), but evaporative cooling causes water loss, leaving small birds vulnerable to dehydration (Whitfield et al., 2015). Consequently, small nestlings may be more susceptible to high temperatures in water-limited environments than large nestlings because of potential dehydration (Whitfield et al., 2015). However, many small birds that live in hot and arid environments will have adaptive strategies, like facultative hyperthermia, that allow tolerance of challenging high temperatures (Gerson et al., 2019; Freeman et al., 2020).
To predict the effects of a warming environment on avian growth, it will be important to know whether hot temperatures during early life can improve tolerance of warmer environments later in life. Evidence from poultry suggests temperature conditioning during incubation and early ages can improve growth performance in warm and cold environments (Nichelmann and Tzschentke, 2002; Shinder et al., 2002; Loyau et al., 2015; Oke et al., 2020; reviewed in Nord and Giroud, 2020). No study has investigated the effects of short-term prenatal exposure to hot or cold temperatures in wild birds, but continuous exposure to low incubation temperatures generally reduces a nestling’s thermal regulatory ability (reviewed in Nord and Giroud, 2020).
How Does Adult Thermoregulation Relate to Offspring Thermoregulation?
According to the ‘climate variability hypothesis,’ high latitude birds may be better able to tolerate temperature variation (Stevens, 1989). This hypothesis suggests that organisms that experience more variable climates should have wider thermal tolerances, and climate variation tends to increase with latitude (Stevens, 1989). A meta-analysis of non-migratory birds supports the climate variability hypothesis – adults of bird species living in variable environments can tolerate a broader range of temperatures (Khaliq et al., 2014). Importantly, migratory bird species do not demonstrate a trend toward increased or decreased tolerance to environmental conditions with latitude. However, when trying to predict the impacts of weather changes on bird species, the variability of the environments where the species evolved may be important. Given this empirical result on adults, we might predict that the offspring of species adapted to more variable environments can tolerate more warming. Note, however, that the relevance of tolerance in adults depends on how tolerance of offspring is related to adult tolerance. If offspring have broader or narrower thermal niches than adults, they may have better or worse resilient than adults to direct effects of temperature challenges. No studies of wild birds have measured thermal tolerance development and compared it to adult birds’ thermal tolerance (Nord and Giroud, 2020).
Weather Impacts Growth Through Changes in Food Availability
Weather variation affects the timing of food abundance, which, depending on when reproduction occurs, can affect the food available for nestling growth (Naef-Daenzer and Keller, 1999; Both et al., 2006; Daunt et al., 2006; Visser et al., 2006). Major components of climate change are shifts in the mean and variability of weather conditions. A consequence of shifting weather conditions is that cues used by birds to time their breeding may become unreliable predictors of food abundance (Bonamour et al., 2019). The mismatch hypothesis suggests that species at lower trophic levels are better able to track shifts in climatic variation than are those at upper trophic levels, resulting in top consumers being less able to time their reproductive events to match food abundance (Stenseth et al., 2002; Thackeray et al., 2016). Because of changing or variable climatic conditions, many birds may have fewer available food resources while offspring develop. For example, both rainfall changes and an inability to temporarily track shifts in prey species can decrease food availability during nestling growth (e.g., black kites Milvus migrans Hiraldo et al., 1990; Lapland longspurs and white crowned sparrows Pérez et al., 2016). In Baird’s sandpiper Calidris bairdii, increases in asynchrony of hatching date with peak biomass of crane flies (Tipulidae) correlates with a slower maximum growth rate (McKinnon et al., 2012). In thick-billed murres Uria lomvia, earlier ice break-up covaries with decreases in Arctic cod Boreogadus saida and increases in capelin Mallotus villosus in the murre diet. Further, hatching later relative to the ice breakup is associated with smaller 14-day old nestlings (Gaston and Elliott, 2014).
Some avian nestlings demonstrate a remarkable ability to persist under periods of scarce food. Laboratory research and avian husbandry suggest that, in some bird species, nestling growth is incredibly flexible in response to food restriction. Chickens Gallus gallus domesticus, Japanese quail Coturnix japonica, and mallards Anas platyrhynchos raised on restricted diets pause their growth (sometimes for months) then resume typical growth trajectories when food restriction stops, with no evident effect on adult morphology (Jordan, 1953; Singsen et al., 1964; reviewed in Schew and Ricklefs, 1998). Similarly, some seabird species can slow chick growth and delay fledging in years with low food abundance or poor weather conditions (Barrett and Rikardsen, 1992; Weimerskirch et al., 2001; Chiaradia and Nisbet, 2006; Kuepper et al., 2018), and white-fronted bee-eaters Merops bullockoides can pause growth during periods of low food availability (Emlen et al., 1991). Some species can maintain growth early in the nestling stage because of spare yolk (Romanoff, 1944), but nestlings may be more vulnerable to food shortages when the yolk is depleted. For many species extending growth will not be possible because the time window for breeding is restricted (e.g., many Arctic breeding birds). If the timing of the breeding season is constrained, it may be optimal to mature at a smaller size rather than arrest growth until suitable food sources become available.
Life-History Strategy May Determine How Parental Care Changes Under More Difficult Weather Conditions
Weather can also have indirect effects on growth by influencing the foraging or brooding behavior of parents (Taylor, 1983; Machmer and Ydenberg, 1990). The degree to which birds can adjust their parental effort is likely to depend on their life-history, possibly resulting in differing magnitudes of impacts of weather variation in species with high versus low adult survival (Ghalambor and Martin, 2001). For instance, under unpredictable or stressful conditions, long-lived species may invest less in reproductive effort, including parental care, with a stronger impact on nestling growth. All species face trade-offs in their allocation of resources into reproduction and survival (Stearns, 1989). These trade-offs may be particularly apparent in altricial species and in species with nestlings dependent on parents for food or thermoregulation. Parents can cope with fewer resources by increasing foraging effort at the expense of their body condition – helping chicks to survive challenging weather conditions. For example, in tree swallows, increases in rainfall correlate with increases in parental provisioning at the expense of declines in adult body condition (Cox et al., 2019). Alternatively, parents may invest less in reproduction during stressful situations to preserve or maintain their body condition. Yellow-nosed albatrosses Diomedea chlororhynchos appear to increase provisioning in response to poor nestling condition only if food is plentiful, limiting the costs of reproduction for themselves in unfavorable years (Weimerskirch et al., 2001). Future work could determine which strategy is adaptive for different life-histories under unpredictable or extreme food conditions.
Other Components of Weather Can Also Affect Nestling Growth
Climate change is increasing the frequency of heavy precipitation events (Intergovernmental Panel on Climate Change, 2018), and rainfall can have variable effects on avian growth (Table 2). The effects of rainfall on nestling growth may depend on when rainfall occurs relative to a species’ breeding season (Kruuk et al., 2015). During nestling growth, rainfall can decrease growth rates and age-specific morphometrics (Siikamäki, 1996; Kosicki and Indykiewicz, 2011; Kaspryzkowski et al., 2014; Pérez et al., 2016; Cox et al., 2019, but see Mainwaring and Hartley, 2016; Grzędzicka, 2019; Table 2). Decreases in growth can result from nestlings having difficulty thermoregulating because of wet downy feathers or difficult foraging conditions for parents (Nye, 1964; Keller and Noordwijk, 1994). In great tits, days with some rainfall greater than 1 mm result in decreases in daily mass gain by nestlings. The negative association between rainfall and daily mass gain in great tit nestlings is suspected to be caused by decreases in foraging of parents because experimental trapping of adults has negative effects on growth comparable to daily rainfall (Keller and Noordwijk, 1994). In hot and dry regions, prey abundance may increase in rainy conditions and will improve foraging conditions for parents and nestling growth rates (Sicurella et al., 2014).
In some studies rainfall has mixed effects on growth or only impacts nestlings at specific ages. Robinson et al. (2017) categorized 3 years of their study on Arctic peregrine falcons Falco peregrinus tundrius as cool and wet or as warm and dry. In cool and wet years, nestlings grew the fastest, grew to a lower-than-average asymptote, and attain their maximum growth rate at a younger age (Robinson et al., 2017). In little auks Alle alle, rainfall during the nestling period correlates with decreases in the masses of young nestlings, while in red-capped larks Calandrella cinerea monthly rainfall has a positive effect on the mass of older nestlings, but no effect on young nestlings (Konarzewski and Taylor, 1989; Ndithia et al., 2017).
In contrast to rainfall during nestling growth, rainfall outside the breeding season might increase avian growth rates and masses because of the generally beneficial effects of rainfall on vegetation and insect abundance. In a population of superb fairy wrens Malurus cyaneus, increases in rainfall prior to nestling growth result in heavier nestling masses (Kruuk et al., 2015). Higher rainfall during the nestling stage is associated with increases in gosling size in the Canada goose Branta canadensis, but the authors suggest that high rainfall during growth is likely associated with high rainfall prior to the nestling stage (Brook et al., 2015). The authors suggest that disentangling whether rainfall during growth had a negative effect on Canada goose nestling growth might be hard because the observed effect was a combination of earlier rainfall effects on vegetation and direct effects of rainfall on nestling size.
In many regions, global climate change is expected to increase ocean wind speeds but decrease land wind speeds (Torralba et al., 2017). Increases in wind speeds often correlate with lower age-specific nestling masses in seabirds. Common terns Sterna hirundo, for example, display reductions in growth when wind speeds are high (Langham, 1968), maybe because of a reduction in the ability of parents to capture prey (Taylor, 1983). Interestingly, high wind speeds do not appear to affect nestling growth of a relative of common terns, sandwich terns Thalasseus sandvicensis, perhaps because of differences between these two species in foraging behavior or morphology (Langham, 1968; Taylor, 1983). In the little auk, wind speed and decreases in visibility correlate with lower masses in nestlings older than 5 days (Konarzewski and Taylor, 1989). Environmental differences at breeding locations might determine whether a weather variable impacts growth in black-legged kittiwakes Rissa tridactyla, wind speeds do not affect nestlings on a colony in the Gulf of Alaska, but high wind speeds do reduce the growth of nestlings on a colony in the Norwegian Sea (Elliott et al., 2014; Christensen-Dalsgaard et al., 2018). Marine birds may need to change or increase their foraging behavior with changing weather conditions, or offspring will need to adjust to lower or variable food provisioning.
Components of foraging behavior in birds can vary among individuals, and individuals may change their behavior differently in response to weather conditions (Woo et al., 2008; Patrick et al., 2014). Differences among individuals in foraging and how foraging behavior develops may be essential to determine which strategies are adaptive in an altered environment. Beyond effects on foraging, wind may stress (e.g., via noise) nestlings directly, resulting in altered growth phenotypes (Crino et al., 2020). Evidence from blue tits suggests that increases in wind speeds negatively affect the growth of fourth primary feathers, mass and tarsus, so a decline in average wind speeds on land may result in more favorable growing conditions for some land birds (Table 3; but see Sicurella et al., 2014).
Variation in sea-surface temperature is also likely to impact food availability for many seabirds. Average sea-surface temperatures are expected to increase globally, with some regions warming more rapidly than others (Intergovernmental Panel on Climate Change, 2013). Sea-surface temperature changes are likely to affect marine bird species by changing their prey species’ distribution, abundance, and phenology, generally resulting in lower food availability and, in extreme cases, in mass seabird die-offs (Piatt et al., 2020). Associations between warm sea-surface temperatures and slower daily mass gain are found in numerous seabird species (Bertram et al., 1991; Hedd et al., 2002; Gjerdrum et al., 2003; Smithers et al., 2003; Peck et al., 2004; Ancona et al., 2011; but see Pinaud et al., 2005). Additionally, sea-ice coverage, age, and distribution will be important for provisioning in ice-associated seabirds (Gaston and Elliott, 2014; Divoky et al., 2015). Generally, increases in sea-surface temperatures correlate with decreases in nestling masses in seabirds, likely because of low prey availability during breeding.
Lastly, the interaction of different weather effects may change our predictions of future change because interactions among weather variables or other changing variables could enhance or ameliorate negative effects. For example, in a study of barn swallows Hirundo rustica, nestling mass between age 8–12 days is negatively associated with increasing temperatures, and this effect is stronger during heavier rainfall, but weaker during high wind speeds (Facey et al., 2020).
Variation in Weather Conditions May More Strongly Impact Later-Hatching Nestlings
The hatching order of a nestling within a brood might play an important role in how weather interacts with growth. In species with asynchronous hatching, older nestlings can outcompete younger nestlings for food, and younger nestlings will frequently grow more slowly, fledge at a smaller size, or die from starvation or aggression from older nestlings (Mock and Parker, 1998). Siblicide can be facultative or obligate. When siblicide is facultative, aggression and siblicide within a brood may be less frequent when food availability and weather conditions are favorable (e.g., Bortolotti et al., 1991; Reynolds, 1996). Egg-hormones, parental incubation, and parental feeding behavior might all modulate competition among nestlings (Mock and Parker, 1997; Müller and Groothuis, 2013). Weather conditions could act as cues for changes in physiology or behavior that promote or impede competition among nestlings. If climate change generally results in weather conditions that are unfavorable for growth (less food, increased thermal stress), the strongest impacts of changing weather conditions will likely be on later hatching nestlings.
Weather and the Evolution of Avian Growth
Because weather can influence avian growth in many ways, a change in weather will likely result in altered selection on nestling growth traits and influence the evolution of nestling growth traits that are heritable. To predict the evolution of avian growth traits, we need to understand the evolvability of these traits as well as natural selection acting on them. Both these components of evolution, i.e., growth evolvability and natural selection on growth, can change according to environmental variations.
Estimation of the Evolutionary Potential of Growth Is Challenging
Little is known about the heritability of nestling growth traits under different weather conditions, making it difficult to predict how and whether nestling traits will evolve in response to changing climate. Similarly, little is known about how variation in weather affects natural selection on growth traits.
Fewer studies have investigated the heritability of growth parameters such as the asymptote, growth rate, and timing of maximum growth (Figure 1) in the wild. Tarsus length at 13–15 days of age in European starlings is heritable when analyzed using either offspring-midparent regressions (h2 = 0.43 [SE = 0.12]) or full-sibling analyses (h2 = 0.30 [SE = 0.22]) in a partial cross fostering experiment (Smith and Wettermark, 1995). Such high heritability for tarsus length is similar to findings of many other avian quantitative genetic studies (Postma and Brommer, 2014). However, when Smith and Wettermark (1995) used an analysis of variance to estimate the heritability of logistic growth curve components fit to the starling’s masses, they found the asymptote to be heritable (h2 = 0.25 [SE = 0.21]) but not the inflection point or growth constant. Age-specific size and mass measurements also have been found to be heritable in medium ground finches, and great tits (h2 of fledging mass = 0.24 [SE = 0.02]; Garant et al., 2005).
Advances in statistical techniques used in quantitative genetics now allow a more accurate measurement of the evolutionary parameters of nestling growth in wild populations. Mixed models called ‘animal models’ that use between-individual relatedness within a population and can handle uneven sampling designs offer an improvement over offspring-midparent regressions (Kruuk, 2004; Wilson et al., 2010). Use of an animal model in house sparrows shows the nestling age-specific masses and tarsus lengths to be heritable (h2 for mass at 5 days = 0.57 [SE = 0.19], mass at 10 days = 0.86 [SE = 0.13]; tarsus at 5 days = 0.81 [SE = 0.22], tarsus at 10 days = 0.63 [SE = 0.11]; Bonneaud et al., 2009). In a more recent large cross-fostering experiment of blue tits, age-specific masses throughout a nestling’s growth period are found to have low heritabilities using an animal model (h2 ranged from 0.07 [SE = 0.04] at 0 days to 0.09 (SE = 0.03] at 6 days (Hadfield et al., 2013). The authors attributed the low additive genetic variances and heritabilities of nestling age-specific masses to their multivariate statistics and experimental design, where they controlled for the effects of viability selection when estimating (co)variances among nestlings (Hadfield et al., 2013). Analyses accounting for missing data from individuals that do not survive until nestling mass measurement can help avoid biases in estimates of selection and additive genetic variances for nestling traits (see Hadfield, 2008 for a thorough discussion)
In poultry, the heritability of growth parameters (asymptote, the timing of maximum growth, growth rate, age-specific mass/size) can be moderately heritable (e.g., h2 range = 0.15 – 0.66; Grossman and Bohren, 1985; Mignon-Grasteau, 1999; N’Dri et al., 2006; Dana et al., 2011; Haunshi et al., 2012) and selection on domestic species provides evidence that growth traits can evolve under these controlled settings (Marks, 1990 as cited in Steigner et al., 1992; Noordwijk and Marks, 1998; Zuidhof et al., 2014). Heritability of avian growth might be higher in husbandry settings compared to wild populations because of controlled rearing conditions, which reduce the amount of environmental variation. Quantitative genetic studies on avian growth in wild conditions are presently too scarce to make more robust conclusions on the expected levels of heritability, so we encourage more studies to estimate the additive genetic variance of nestling growth parameters in wild avian populations.
Heritability Is Environmentally Dependent
An important note relevant to weather conditions is that heritability measures are only applicable to the population and the environment where they are measured. The denominator in the calculation of heritability, phenotypic variance, is equal to the sum of genetic variance and environmental variance. Hence, an increase in the environmental variance of a trait results in a decrease in the heritability estimate. Additionally, environmental variation can impact the level of additive genetic variation estimated for a given trait (Gebhardt-Henrich and Noordwijk, 1994; Charmantier and Garant, 2005; Wood and Brodie, 2016). Early papers measuring the heritability of growth traits noted that poor environmental conditions during growth might restrict the expression of additive genetic variance, resulting in lower heritability of the fledgling or adult phenotypic traits in unfavorable environments (Gebhardt-Henrich and Noordwijk, 1991, 1994; Gebhardt-Henrich, 1992). The original hypothesis of environmental influence on the heritability of growth by Noordwijk (1982 as cited in Noordwijk and Marks, 1998) suggests that under unfavorable conditions, a nestling might not reach its genetically determined size, but instead would be constrained because of maturation at a set age. However, under favorable conditions a nestling will reach a final size before age-induced maturation. Therefore, the hypothesis suggests that variation in the asymptote under unfavorable conditions might be more likely to reflect environmental differences among nestlings and the variation under favorable conditions might reflect genetic differences among individuals (Noordwijk and Marks, 1998). A change in the genes that underlie the phenotypic expression of a trait could also cause a change in additive genetic variation between environments (Wood and Brodie, 2016). For example, in a hot environment, genes that play a role in heat tolerance might largely determine the additive genetic variance in a growth trait, while in a thermoneutral environment, genes that play a role in metabolism and growth might explain most of the differences among individual growth trajectories. Therefore, weather variation could affect rates of evolution through an increase or decrease in heritability by increasing or decreasing the environmental and/or the additive genetic contribution to variation in nestling growth traits. Lastly, because heritability is dependent on environmental variance, directly reporting the additive genetic variance of a trait scaled by the mean will allow accurate comparisons of evolvability across traits and species (Houle, 1992; Hansen et al., 2011). It is important for researchers to report both metrics when trying to evaluate the general evolvability of any trait.
While we might not know how heritability will change with different weather patterns, we do know that annual changes in the heritability of nestling size traits in response to changes in environmental conditions have been observed. Following a brood size manipulation experiment in great tits, Gebhardt-Henrich and Noordwijk (1991) found that heritability of mass at 15 days of age (near asymptotic mass) might be lower in large broods (average h2 = 0.40 [SE = 0.54]) than in small broods (average h2 = 0.75 [SE = 0.36]), but only in years when environmental conditions are unfavorable. Notably, standard errors are large, and results are not statistically significant, but this study is one of the earliest to postulate an effect of environmental conditions on evolutionary parameters in nestling traits. In a comparison of age-specific size measurements following a brood size manipulation in blue tits, Kunz and Ekman (2000) detected high heritability estimates for age-specific tarsus (age 8 and 10 days), wing (age 6 days), and mass (age 6 and 8 days) measurements in smaller compared to larger broods. While not focused on weather conditions, other more recent studies have noted higher heritability in more favorable environments (Charmantier et al., 2004; Garant et al., 2005).
Experimental studies on poultry provide insight into how weather impacts might influence the evolutionary response of chick growth traits. Marks (1996) examined the response to artificial selection for increased 4-week body mass in restricted versus full nutritional diet lines of Japanese quail (Coturnix japonica) and found that the restricted diet line had much lower phenotypic increases (no changes in phenotype for many generations) in 4-week body mass. The quail chick’s growth conditions are artificial but could indicate that evolutionary changes in nestling size will be slower in environments where weather conditions reduce food availability, as is expected for climate change in many ecosystems.
Generally, these studies indicate that the heritability of fledging mass increases under favorable conditions, but we should cautiously extrapolate from the current literature because the taxonomic range of current results is restricted, and many studies do not investigate the effects of weather. Further, no studies to our knowledge evaluated the changing heritability of growth rates or the timing of maximum growth in wild populations.
Perspectives on Adding Complexity and Interactive Effects
Beyond the genetic variance of a trait, the evolution of a trait also depends on its genetic covariance with other traits (Willham, 1972; Arnold et al., 2008; Walsh and Lynch, 2018). The evolution of growth traits may be constrained because of genetic correlations among traits. For example, genes that increase asymptotic mass may decrease the maximum growth rate. Therefore, weather variation could affect the evolution of growth traits by influencing the genetic covariance among traits. To date, no study has investigated how weather variation shapes genetic covariances among chick growth traits. Hence it is difficult to determine if genetic constraints or the effect of weather on genetic constraints will have an important role in the evolutionary response of avian growth traits to climate change.
Finally, in addition to a given growth trait’s evolutionary trajectory being dependent on genetic correlations, selection on growth traits induced by climate change can interact with other selective forces. For instance, comparative studies have shown that adult and nestling birds are repeatedly smaller in cities than in surrounding rural areas (Bailly et al., 2016; Caizergues et al., 2018). However, as with weather effects, such trends have not yet been attributed to either plastic or evolutionary processes. Several factors that directly influence growth and are affected by climate change (e.g., food availability) are also affected by growing urbanization. Predictive models will hence need at some point to include the complexity of these different and interacting selective forces. Some authors (e.g., Grimm et al., 2008) have hypothesized that since urban areas are ‘heat islands,’ cities provide an interesting opportunity to study how global warming will impact specific traits (Rivkin et al., 2019). Describing growth curves in urban birds could, hence, be informative in understanding how warmer temperatures and changes in resources influence avian growth. Gene flow from populations adapted to urban habitats into populations in more natural habitats could also provide individuals pre-adapted to warmer conditions (e.g., adaptation with gene flow; Tigano and Friesen, 2016).
Conclusion
Human-induced warming has resulted in average global temperatures increasing by 1°C since the preindustrial period, and temperatures are likely to increase by 1.5°C or more in the next 2–3 decades (Intergovernmental Panel on Climate Change, 2018). If the impacts of weather on a species are known, qualitative or quantitative predictions for growth trait changes from weather-growth models can be used to predict future growth trait changes. If the additive genetic variance and fitness associated with differences in growth traits can be estimated, these models could be improved with evolutionary and demographic information (Jenouvrier and Visser, 2011; Vedder et al., 2013).
We have outlined multiple weather components that affect growth traits of avian species. The future of this research will involve trying to predict adaptive responses to these changes. We think the main questions to address next are: (1) what growth trajectories are adaptive in an environment altered by climate change? (2) what is the potential for a given population of birds to adapt to climate change through either plastic or evolutionary adjustments in growth and development? (3) how will environmental changes alter parental effects on growth? and (4) is the potential for adaptation of growth or development altered by the predictability of weather or environmental conditions? To answer these questions, we need more information on the relationship between individual fitness and growth curves and the genetic (co)variance of chick growth traits.
Author Contributions
DS conducted the literature review. DS, VLF, and AC conceived the project idea and wrote the manuscript. All the authors contributed to the article and approved the submitted version.
Funding
We acknowledge grants from the Natural Sciences and Engineering Council of Canada (Grant # 493789-16), a TD Fellowship in Arctic Environmental Issues, and Queen’s University Graduate Awards for funding to conduct this research.
Conflict of Interest
The authors declare that the research was conducted in the absence of any commercial or financial relationships that could be construed as a potential conflict of interest.
Acknowledgments
We thank Arnaud Grégoire, Céline Teplitsky, the Friesen lab at Queen’s University and the Ecologie Evolutive Empirique, Communication & Coopération team at the CEFE University for helpful discussion. We are also thankful for comments from the two reviewers and editor that helped improve this manuscript.
References
Addison, B., Benowitz-Fredericks, Z. M., Hipfner, J. M., and Kitaysky, A. S. (2008). Are yolk androgens adjusted to environmental conditions? A test in two seabirds that lay single-egg clutches. Gen. Comp. Endocrinol. 158, 5–9.
Aldredge, R. A. (2016). Using non-linear mixed effects models to identify patterns of chick growth in House Sparrows Passer domesticus. Ibis 158, 16–27.
Álvarez, E., and Barba, E. (2014). Behavioural responses of great tits to experimental manipulation of nest temperature during incubation. Ornis Fenn. 91, 220–230.
Ancona, S., Sánchez-Colón, S., Rodríguez, C., and Drummond, H. (2011). El Niño in the Warm Tropics: local sea temperature predicts breeding parameters and growth of blue-footed boobies. J. Anim. Ecol. 80, 799–808. doi: 10.1111/j.1365-2656.2011.01821.x
Andreasson, F., Hegemann, A., Nord, A., and Nilsson, J. -Å (2020a). Experimental facilitation of heat loss affects work rate and innate immune function in a breeding passerine bird. J. Exp. Biol. 223, jeb219790. doi: 10.1242/jeb219790
Andreasson, F., Nilsson, J. -Å, and Nord, A. (2020b). Avian reproduction in a warming world. Front. Ecol. Evol. 8:576331. doi: 10.3389/fevo.2020.576331
Andreasson, F., Nord, A., and Nilsson, J. -Å (2018). Experimentally increased nest temperature affects body temperature, growth and apparent survival in blue tit nestlings. J. Avian Biol. 49, 1–14. doi: 10.1111/jav.01620
Andrew, S. C., Awasthy, M., Griffith, A. D., Nakagawa, S., and Griffith, S. C. (2018). Clinal variation in avian body size is better explained by summer maximum temperatures during development than by cold winter temperatures. Auk 135, 206–217. doi: 10.1642/AUK-17-129.1
Andrew, S. C., Hurley, L. L., Mariette, M. M., and Griffith, S. C. (2017). Higher temperatures during development reduce body size in the Zebra Finch in the laboratory and in the wild. J. Evol. Biol. 30, 2156–2164. doi: 10.1111/jeb.13181
Arbeiter, S., Schulze, M., Tamm, P., and Hahn, S. (2016). Strong cascading effect of weather conditions on prey availability and annual breeding performance in European bee-eaters Merops apiaster. J. Ornithol. 157, 155–163. doi: 10.1007/s10336-015-1262-x
Ardia, D. R., Pérez, J. H., Chad, E. K., Voss, M. A., and Clotfelter, E. D. (2009). Temperature and life history: experimental heating leads female tree swallows to modulate egg temperature and incubation behaviour. J. Anim. Ecol. 78, 4–13.
Ardia, D. R., Pérez, J. H., and Clotfelter, E. D. (2010). Experimental cooling during incubation leads to reduced innate immunity and body condition in nestling tree swallows. Proc. R. Soc. B Biol. Sci. 277, 1881–1888. doi: 10.1098/rspb.2009.2138
Arnold, S. J., Bürger, R., Hohenlohe, P. A., Ajie, B. C., and Jones, A. G. (2008). Understanding the evolution and stability of the g-matrix. Evolution 62, 2451–2461. doi: 10.1111/j.1558-5646.2008.00472.x
Bailly, J., Scheifler, R., Berthe, S., Clément-Demange, V.-A., Leblond, M., Pasteur, B., et al. (2016). From eggs to fledging: negative impact of urban habitat on reproduction in two tit species. J. Ornithol. 157, 377–392. doi: 10.1007/s10336-015-1293-3
Barrett, R. T., and Rikardsen, F. (1992). Chick growth, fledging periods and adult mass loss of Atlantic Puffins Fratercula arctica during Years of Prolonged Food Stress. Colon. Waterbirds 15, 24–32. doi: 10.2307/1521351
Benowitz-Fredericks, Z. M., Kitaysky, A. S., Welcker, J., and Hatch, S. A. (2013). Effects of food availability on yolk androgen deposition in the black-legged kittiwake (Rissa tridactyla), a seabird with facultative brood reduction. PLoS One 8:e0062949. doi: 10.1371/journal.pone.0062949
Bentz, A. B., Navara, K. J., and Siefferman, L. (2013). Phenotypic plasticity in response to breeding density in tree swallows: an adaptive maternal effect? Horm. Behav. 64, 729–736. doi: 10.1016/j.yhbeh.2013.08.009
Bergmann, C. (1847). Ueber die verhältnisse der wärmeökonomie der thiere zu ihrer grösse. Göttinger Studien 1, 595–708.
Bertram, D. F., Kaiser, G. W., and Ydenberg, R. C. (1991). Patterns in the provisioning and growth of nestling Rhinoceros Auklets. Auk 108, 842–852. doi: 10.1093/auk/108.4.842
Bonamour, S., Chevin, L.-M., Charmantier, A., and Teplitsky, C. (2019). Phenotypic plasticity in response to climate change: the importance of cue variation. Philos. Trans. R. Soc. B Biol. Sci. 374, 20180178. doi: 10.1098/rstb.2018.0178
Bonneaud, C., Sinsheimer, J. S., Richard, M., Chastel, O., and Sorci, G. (2009). Mhc polymorphisms fail to explain the heritability of phytohaemagglutinin-induced skin swelling in a wild passerine. Biol. Lett. 5, 784–787. doi: 10.1098/rsbl.2009.0435
Bonnet, T., Wandeler, P., Camenisch, G., and Postma, E. (2017). Bigger is fitter? Quantitative genetic decomposition of selection reveals an adaptive evolutionary decline of body mass in a wild rodent population. PLoS Biol. 15:e1002592. doi: 10.1371/journal.pbio.1002592
Bortolotti, G. R., Wiebe, K. L., and Iko, W. M. (1991). Cannibalism of nestling American kestrals by their parents and siblings. Can. J. Zool. 69, 1447–1453.
Both, C., Bouwhuis, S., Lessells, C. M., and Visser, M. E. (2006). Climate change and population declines in a long-distance migratory bird. Nature 441, 81–83. doi: 10.1038/nature04539
Bradbury, R. B., Wilson, J. D., Moorcroft, D., Morris, A. J., and Perkins, A. J. (2003). Habitat and weather are weak correlates of nestling condition and growth rates of four UK farmland passerines. Ibis 145, 295–306. doi: 10.1046/j.1474-919X.2003.00142.x
Brisson-Curadeau, E., Patterson, A., Whelan, S., Lazarus, T., and Elliott, K. H. (2017). Tracking Cairns: biologging improves the use of seabirds as sentinels of the sea. Front. Mar. Sci. 4:357. doi: 10.3389/fmars.2017.00357
Brook, R. W., Leafloor, J. O., Abraham, K. F., and Douglas, D. C. (2015). Density dependence and phenological mismatch: consequences for growth and survival of sub-arctic nesting Canada geese. Avian Conserv. Ecol. 10, 1. doi: 10.5751/ACE-00708-100101
Bryant, D. M. (1978). Environmental influences on growth and survival of nestling House Martins Delichon Urbica. Ibis 120, 271–283. doi: 10.1111/j.1474-919X.1978.tb06788.x
Buij, R., Folkertsma, I., Kortekaas, K., De Iongh, H. H., and Komdeur, J. (2013). Effects of land-use change and rainfall in Sudano-Sahelian West Africa on the diet and nestling growth rates of an avian predator. Ibis 155, 89–101. doi: 10.1111/ibi.12006
Bürkner, P.-C. (2017). brms: an R package for bayesian multilevel models using stan. J. Stat. Softw. 80, 1–28. doi: 10.18637/jss.v080.i01
Cairns, D. K. (1988). Seabirds as indicators of marine food supplies. Biol. Oceanogr. 5, 261–271. doi: 10.1080/01965581.1987.10749517
Caizergues, A. E., Grégoire, A., and Charmantier, A. (2018). Urban versus forest ecotypes are not explained by divergent reproductive selection. Proc. R. Soc. B Biol. Sci. 285, 20180261. doi: 10.1098/rspb.2018.0261
Catry, I., Catry, T., Patto, P., Franco, A. M. A., and Moreira, F. (2015). Differential heat tolerance in nestlings suggests sympatric species may face different climate change risks. Clim. Res. 66, 13–25. doi: 10.3354/cr01329
Charmantier, A., and Garant, D. (2005). Environmental quality and evolutionary potential: lessons from wild populations. Proc. R. Soc. B Biol. Sci. 272, 1415–1425. doi: 10.1098/rspb.2005.3117
Charmantier, A., and Gienapp, P. (2014). Climate change and timing of avian breeding and migration: evolutionary versus plastic changes. Evol. Appl. 7, 15–28. doi: 10.1111/eva.12126
Charmantier, A., Kruuk, L. E. B., and Lambrechts, M. M. (2004). Parasitism reduces the potential for evolution in a wild bird population. Evolution 58, 203–206. doi: 10.1554/03-350
Chevin, L. M., Collins, S., and Lefèvre, F. (2013). Phenotypic plasticity and evolutionary demographic responses to climate change: taking theory out to the field. Funct. Ecol. 27, 967–979. doi: 10.1111/j.1365-2435.2012.02043.x
Chiaradia, A., and Nisbet, I. C. T. (2006). Plasticity in parental provisioning and chick growth in Little Penguins Eudyptula minor in years of high and low breeding success. Ardea 94, 254–270.
Christensen-Dalsgaard, S., May, R. F., Barrett, R. T., Langset, M., Sandercock, B. K., and Lorentsen, S. (2018). Prevailing weather conditions and diet composition affect chick growth and survival in the black-leggedd kittiwake. Mar. Ecol. Progr. Ser. 604, 237–249. doi: 10.3354/meps12744
Christians, J. K. (2002). Avian egg size: variation within species and inflexibility within individuals. Biol. Rev. Camb. Philos. Soc. 77, 1–26. doi: 10.1017/s1464793101005784
Conradie, S. R., Woodborne, S. M., Cunningham, S. J., and McKechnie, A. E. (2019). Chronic, sublethal effects of high temperatures will cause severe declines in southern African arid-zone birds during the 21st century. Proc. Natl. Acad. Sci. U.S.A. 116, 14065–14070. doi: 10.1073/pnas.1821312116
Cooper, E. B., and Kruuk, L. E. B. (2018). Ageing with a silver-spoon: a meta-analysis of the effect of developmental environment on senescence. Evol. Lett. 2, 460–471. doi: 10.1002/evl3.79
Cox, A. R., Robertson, R. J., Lendvai, A. Z., Everitt, K., and Bonier, F. (2019). Rainy springs linked to poor nestling growth in a declining avian aerial insectivore (Tachycineta bicolor). Proc. R. Soc. B Biol. Sci. 286, 20190018. doi: 10.1098/rspb.2019.0018
Crino, O. L., Driscoll, S. C., Brandl, H. B., Buchanan, K. L., and Griffith, S. C. (2020). Under the weather: corticosterone levels in wild nestlings are associated with ambient temperature and wind. Gen. Comp. Endocrinol. 285, 113247. doi: 10.1016/j.ygcen.2019.113247
Cunningham, S. J., Martin, R. O., Hojem, C. L., and Hockey, P. A. R. (2013). Temperatures in excess of critical thresholds threaten nestling growth and survival in a rapidly-warming arid savanna: a study of common fiscals. PLoS One 8:e74613. doi: 10.1371/journal.pone.0074613
Dana, N., Waaij, E. H., vander, Arendonk, J. A. M., and van. (2011). Genetic and phenotypic parameter estimates for body weights and egg production in Horro chicken of Ethiopia. Trop. Anim. Health Prod. 43, 21–28. doi: 10.1007/s11250-010-9649-4
Daunt, F., Afanasyev, V., Silk, J. R. D., and Wanless, S. (2006). Extrinsic and intrinsic determinants of winter foraging and breeding phenology in a temperate seabird. Behav. Ecol. Sociobiol. 59, 381–388. doi: 10.1007/s00265-005-0061-4
Dawson, R. D., and Bortolotti, G. R. (2000). Reproductive success of American kestrels: the role of prey abundance and weather. Condor 102, 814–822. doi: 10.1093/condor/102.4.814
Dawson, R. D., Lawrie, C. C., and O’Brien, E. L. (2005). The importance of microclimate variation in determining size, growth and survival of avian offspring: experimental evidence from a cavity nesting passerine. Oecologia 144, 499–507. doi: 10.1007/s00442-005-0075-7
Divoky, G. J., Lukacs, P. M., and Druckenmiller, M. L. (2015). Effects of recent decreases in arctic sea ice on an ice-associated marine bird. Prog. Oceanogr. 136, 151–161. doi: 10.1016/j.pocean.2015.05.010
Dobson, F. S., Becker, P. H., Arnaud, C. M., Bouwhuis, S., and Charmantier, A. (2017). Plasticity results in delayed breeding in a long-distant migrant seabird. Ecol. Evol. 7, 3100–3109. doi: 10.1002/ece3.2777
Dunn, E. H. (1975). The timing of endothermy in the development of altrical birds. Condor 77, 288–293. doi: 10.2307/1366224
DuRant, S. E., Hepp, G. R., Moore, I. T., Hopkins, B. C., and Hopkins, W. A. (2010). Slight differences in incubation temperature affect early growth and stress endocrinology of wood duck (Aix sponsa) ducklings. J. Exp. Biol. 213, 45–51. doi: 10.1242/jeb.034488
Dyrcz, A. (1974). Factors affecting the growth rate of nestling great reed warblers and reed warblers at Milicz, Poland. Ibis 116, 330–339. doi: 10.1111/j.1474-919X.1974.tb00128.x
Eeva, T., Espín, S., Sánchez-Virosta, P., and Rainio, M. (2020). Weather effects on breeding parameters of two insectivorous passerines in a polluted area. Sci. Total Environ. 729, 138913. doi: 10.1016/j.scitotenv.2020.138913
Eeva, T., Lehikoinen, E., Rönkä, M., Lummaa, V., and Currie, D. (2002). Different responses to cold weather in two pied flycatcher populations. Ecography 25, 705–713. doi: 10.1034/j.1600-0587.2002.250606.x
Elliott, K. H., Chivers, L. S., Bessey, L., Gaston, A. J., Hatch, S. A., Kato, A., et al. (2014). Windscapes shape seabird instantaneous energy costs but adult behavior buffers impact on offspring. Mov. Ecol. 2, 17. doi: 10.1186/s40462-014-0017-2
Emlen, S. T., Wrege, P. H., Demong, N. J., and Hegner, R. E. (1991). Flexible growth rates in nestling White-Fronted Bee-Eaters: a possible adaptation to short-term food shortage. Condor 93, 591–597. doi: 10.2307/1368191
Facey, R. J., Vafidis, J. O., Smith, J. A., Vaughan, I. P., and Thomas, R. J. (2020). Contrasting sensitivity of nestling and fledgling Barn Swallow Hirundo rustica body mass to local weather conditions. Ibis 162, 1163–1174. doi: 10.1111/ibi.12824
Fleischer, R. C., and Johnston, R. F. (1984). The relationship between winter climate and selection on body size of house sparrows. Can. J. Zool. 62, 405–410. doi: 10.1139/z84-063
Freeman, M. T., Czenze, Z. J., Schoeman, K., and McKechnie, A. E. (2020). Extreme hyperthermia tolerance in the world’s most abundant wild bird. Sci. Rep. 10, 13098. doi: 10.1038/s41598-020-69997-7
Garant, D., Kruuk, L. E. B., Wilkin, T. A., McCleery, R. H., and Sheldon, B. C. (2005). Evolution driven by differential dispersal within a wild bird population. Nature 433, 60–65. doi: 10.1038/nature03051
Gardner, J. L., Peters, A., Kearney, M. R., Joseph, L., and Heinsohn, R. (2011). Declining body size: a third universal response to warming? Trends Ecol. Evol. 26, 285–291. doi: 10.1016/j.tree.2011.03.005
Gasparini, J., Boulinier, T., Gill, V. A., Gil, D., Hatch, S. A., and Roulin, A. (2007). Food availability affects the maternal transfer of androgens and antibodies into eggs of a colonial seabird. J. Evol. Biol. 20, 874–880. doi: 10.1111/j.1420-9101.2007.01315.x
Gaston, A. J., and Elliott, K. H. (2014). Seabird diet changes in northern Hudson Bay, 1981-2013, reflect the availability of schooling prey. Mar. Ecol. Prog. Ser. 513, 211–223. doi: 10.3354/meps10945
Gavrilets, S., and Scheiner, S. M. (1993). The genetics of phenotypic plasticity. V. Evolution of reaction norm shape. J. Evol. Biol. 6, 31–48. doi: 10.1046/j.1420-9101.1993.6010031.x
Gebhardt-Henrich, S. G. (1992). Heritability of growth curve parameters and heritability of final size: a simulation study. Growth Dev. Aging 56, 23–33.
Gebhardt-Henrich, S. G., and Noordwijk, A. J. V. (1994). The genetical ecology of nestling growth in the Great Tit. Environmental influences on the expression of genetic variances during growth. Funct. Ecol. 8, 469–476. doi: 10.2307/2390071
Gebhardt-Henrich, S. G., and Noordwijk, A. J. V. (1991). Nestling growth in the Great Tit I. Heritability estimates under different environmental conditions. J. Evol. Biol. 4, 341–362. doi: 10.1046/j.1420-9101.1991.4030341.x
Geiser, S., Arlettaz, R., and Schaub, M. (2008). Impact of weather variation on feeding behaviour, nestling growth and brood survival in wrynecks Jynx torquilla. J. Ornithol. 149, 597–606. doi: 10.1007/s10336-008-0305-y
Gerson, A. R., McKechnie, A. E., Smit, B., Whitfield, M. C., Smith, E. K., Talbot, W. A., et al. (2019). The functional significance of facultative hyperthermia varies with body size and phylogeny in birds. Funct. Ecol. 33, 597–607. doi: 10.1111/1365-2435.13274
Ghalambor, C. K., and Martin, T. E. (2001). Fecundity-survival trade-offs and parental risk-taking in birds. Science 292, 494–497. doi: 10.1126/science.1059379
Ghalambor, C. K., McKay, J. K., Carroll, S. P., and Reznick, D. N. (2007). Adaptive versus non-adaptive phenotypic plasticity and the potential for contemporary adaptation in new environments. Funct. Ecol. 21, 394–407. doi: 10.1111/j.1365-2435.2007.01283.x
Gienapp, P., and Brommer, J. E. (2014). “Evolutionary dynamics in response to climate change,” in Quantitative Genetics in the Wild, eds A. Charmantier, D. Garant, and L. E. B. Kruuk (New York, NY: Oxford University Press), 254–273.
Gill, F. B., and Prum, R. O. (2019). Ornithology, Fourth Edition. New York, NY: W.H. Freeman & Co., 1022–1025.
Gjerdrum, C., Vallée, A. M. J., St. Clair, C. C., Bertram, D. F., Ryder, J. L., and Blackburn, G. S. (2003). Tufted puffin reproduction reveals ocean climate variability. Proc. Natl. Acad. Sci. USA 100, 9377–9382. doi: 10.1073/pnas.1133383100
Grémillet, D., and Charmantier, A. (2010). Shifts in phenotypic plasticity constrain the value of seabirds as ecological indicators of marine ecosystems. Ecol. Appl. Publ. Ecol. Soc. Am. 20, 1498–1503. doi: 10.1890/09-1586.1
Griffith, S. C., Andrew, S. C., McCowan, L. S. C., Hurley, L. L., Englert Duursma, D., Buchanan, K. L., et al. (2020). Egg size is unrelated to ambient temperature in the opportunistically breeding zebra finch. J. Avian Biol. 51, 1–14. doi: 10.1111/jav.02356
Griffith, S. C., Mainwaring, M. C., Sorato, E., and Beckmann, C. (2016). High atmospheric temperatures and “ambient incubation” drive embryonic development and lead to earlier hatching in a passerine bird. R. Soc. Open Sci. 3, 150371. doi: 10.1098/rsos.150371
Grimm, N. B., Faeth, S. H., Golubiewski, N. E., Redman, C. L., Wu, J., Bai, X., et al. (2008). Global change and the ecology of cities. Science 319, 756–760. doi: 10.1126/science.1150195
Groothuis, T. G. G., Müller, W., von Engelhardt, N., Carere, C., and Eising, C. (2005). Maternal hormones as a tool to adjust offspring phenotype in avian species. Neurosci. Biobehav. Rev. 29, 329–352. doi: 10.1016/j.neubiorev.2004.12.002
Groothuis, T. G. G., and Schwabl, H. (2008). Hormone-mediated maternal effects in birds: mechanisms matter but what do we know of them? Philos. Trans. R. Soc. Lond. B. Biol. Sci. 363, 1647–1661. doi: 10.1098/rstb.2007.0007
Grossman, M., and Bohren, B. B. (1985). Logistic growth curve of chickens: heritability of parameters. J. Hered. 76, 459–462. doi: 10.1093/oxfordjournals.jhered.a110145
Grzędzicka, E. (2019). Impact of rainfall on the offspring PHA-response and body mass in the Eurasian blue tit. Ecol. Res. 34, 85–93. doi: 10.1111/1440-1703.1005
Hadfield, J. (2019). MCMCglmm: MCMC Generalised Linear Mixed Models. Available online at: https://CRAN.R-project.org/package=MCMCglmm (accessed February 20, 2020).
Hadfield, J. D. (2008). Estimating evolutionary parameters when viability selection is operating. Proc. R. Soc. B Biol. Sci. 275, 723–734. doi: 10.1098/rspb.2007.1013
Hadfield, J. D. (2010). MCMC methods for multi-response generalized linear mixed models: the MCMCglmm R package. J. Stat. Softw. 33, 1–22. doi: 10.1002/ana.22635
Hadfield, J. D., Heap, E. A., Bayer, F., Mittell, E. A., and Crouch, N. M. A. (2013). Disentangling genetic and prenatal sources of familial resemblance across ontogeny in a wild passerine. Evolution 67, 2701–2713. doi: 10.1111/evo.12144
Hammers, M., Richardson, D. S., Burke, T., and Komdeur, J. (2013). The impact of reproductive investment and early-life environmental conditions on senescence: support for the disposable soma hypothesis. J. Evol. Biol. 26, 1999–2007. doi: 10.1111/jeb.12204
Hansen, T. F., Pélabon, C., and Houle, D. (2011). Heritability is not evolvability. Evol. Biol. 38, 258–277. doi: 10.1007/s11692-011-9127-6
Harter, B. B. (2007). Black Guillemots as Indicators of Change in the Near-Shore Arctic Marine Ecosystem. Ph. D. Thesis, University of Manitoba, Winnipeg, CA.
Haunshi, S., Shanmugam, M., Padhi, M. K., Niranjan, M., Rajkumar, U., Reddy, M. R., et al. (2012). Evaluation of two Indian native chicken breeds for reproduction traits and heritability of juvenile growth traits. Trop. Anim. Health Prod. 44, 969–973. doi: 10.1007/s11250-011-9994-y
Hawksley, O. (1957). Ecology of a breeding population of arctic terns. Bird Band 28, 57–92. doi: 10.2307/4510623
Haywood, S., and Perrins, C. M. (1992). Is clutch size in birds affected by environmental conditions during growth? Proc. R. Soc. Lond. B Biol. Sci. 249, 195–197. doi: 10.1098/rspb.1992.0103
Hedd, A., Ryder, J. L., Cowen, L. L., and Bertram, D. F. (2002). Inter-annual variation in the diet, provisioning and growth of Cassin’s auklet at Triangle Island, British Columbia: responses to variation in ocean climate. Mar. Ecol. Prog. Ser. 229, 221–232. doi: 10.3354/meps229221
Hepp, G. R., Kennamer, R. A., and Johnson, M. H. (2006). Maternal effects in Wood Ducks: incubation temperature influences incubation period and neonate phenotype. Funct. Ecol. 20, 308–314. doi: 10.1111/j.1365-2435.2006.01108.x
Hiraldo, F., Veiga, J. P., and Máñez, M. (1990). Growth of nestling Black Kites Milvus migrans: effects of hatching order, weather and season. J. Zool. 222, 197–214. doi: 10.1111/j.1469-7998.1990.tb05672.x
Hohtola, E., and Visser, H. (1998). “Development of locomotion and endothermy in altricial and precocial birds,” in Avian Growth and Development: Evolution within the Altricial-Precocial Spectrum, eds J. M. Starck and R. E. Ricklefs (New York, NY: Oxford University Press), 157–170.
Houle, D. (1992). Comparing evolvability and variability of quantitative traits. Genetics 130, 195–204.
Houslay, T. M. (2017). Tutorials. Available online at: https://tomhouslay.com/tutorials/ (accessed February 20, 2020).
Imlay, T. L., Nickerson, D., and Horn, A. G. (2018). Temperature and breeding success for cliff swallows (Petrochelidon pyrrhonota) nesting on man-made structures: ecological traps? Can. J. Zool. 97, 429–435. doi: 10.1139/cjz-2018-0224
Intergovernmental Panel on Climate Change (2013). Climate change 2013: The Physical Science Basis. Geneva: IPCC.
Jenouvrier, S., and Visser, M. E. (2011). Climate change, phenological shifts, eco-evolutionary responses and population viability: towards a unifying predictive approach. Int. J. Biometeorol. 55, 905–919.
Johnston, R. F., and Selander, R. K. (1964). House sparrows: rapid evolution of races in North America. Science 144, 548–550.
Johnston, R. F., and Selander, R. K. (1973). Evolution in the House Sparrow. II. Adaptive differentiation in North American populations. Evolution 25, 1–28. doi: 10.2307/2406496
Jones, B. C., and DuVal, E. H. (2019). Direct and indirect effects of the El Niño Southern Oscillation on development and survival of young of a tropical passerine. Oecologia 190, 485–496. doi: 10.1007/s00442-019-04418-9
Jong, G. D. (1990). Quantitative Genetics of reaction norms. J. Evol. Biol. 3, 447–468. doi: 10.1046/j.1420-9101.1990.3050447.x
Jordan, J. S. (1953). Effects of starvation on wild mallards. J. Wildl. Manag. 17, 304–311. doi: 10.2307/3797112
Kaspryzkowski, Z., Polak, M., and Chylarecki, P. (2014). Effects of weather conditions, time of breeding, brood size, and hatching order on Eurasian Bittern nestling growth in a food-rich fishpond habitat. Ann. Zool. Fenn. 51, 477–487. doi: 10.5735/086.051.0602
Keller, L. F., and Noordwijk, A. J. V. (1993). A method to isolate environmental effects on nestling growth, illustrated with examples from the great tit (Parus major). Funct. Ecol. 7, 493–502. doi: 10.2307/2390037
Keller, L. F., and Noordwijk, A. J. V. (1994). Effects of local environmental conditions on nestling growth. Ardea 82, 349–362. doi: 10.5167/uzh-53659
Khaliq, I., Hof, C., Prinzinger, R., Böhning-Gaese, K., and Pfenninger, M. (2014). Global variation in thermal tolerances and vulnerability of endotherms to climate change. Proc. R. Soc. B Biol. Sci. 281, 20141097. doi: 10.1098/rspb.2014.1097
Konarzewski, M., and Taylor, J. R. E. (1989). The influence of weather conditions on growth of Little Auk Alle alle chicks. Ornis Scand. 20, 112–116. doi: 10.2307/3676877
Kosicki, J. Z., and Indykiewicz, P. (2011). Effects of breeding date and weather on nestling development in White Storks Ciconia ciconia. Bird Study 58, 178–185. doi: 10.1080/00063657.2011.554531
Kouba, M., Bartoš, L., Korpimäki, E., and Zárybnická, M. (2015). Factors affecting the duration of nestling period and fledging order in Tengmalm’s Owl (Aegolius funereus): effect of wing length and hatching sequence. PloS ONE 10:e0121641. doi: 10.1371/journal.pone.0121641
Krist, M. (2011). Egg size and offspring quality: a meta-analysis in birds. Biol. Rev. 86, 692–716. doi: 10.1111/j.1469-185X.2010.00166.x
Kruuk, L. E. B. (2004). Estimating genetic parameters in natural populations using the “animal model.”. Philos. Trans. R. Soc. B Biol. Sci. 359, 873–890. doi: 10.1098/rstb.2003.1437
Kruuk, L. E. B., Osmond, H. L., and Cockburn, A. (2015). Contrasting effects of climate on juvenile body size in a Southern Hemisphere passerine bird. Glob. Change Biol. 21, 2929–2941. doi: 10.1111/gcb.12926
Kuepper, N. D., Marek, C., Coria, N., Libertelli, M. M., and Quillfeldt, P. (2018). Facultative hypothermia as a survival strategy during snowstorm induced food shortages in Antarctic storm-petrel chicks. Comp. Biochem. Phys. A 224, 74–83. doi: 10.1016/j.cbpa.2018.06.018
Kunz, and Ekman (2000). Genetic and environmental components of growth in nestling blue tits (Parus caeruleus). J. Evol. Biol. 13, 199–212. doi: 10.1046/j.1420-9101.2000.00158.x
Lack, E., and Lack, D. (1951). The breeding biology of the Swift Apus Apus. Ibis 93, 501–546. doi: 10.1111/j.1474-919X.1951.tb05457.x
Langham, N. P. E. (1968). The Comparative Biology of Terns, Sterna spp. Doctoral dissertation, Durham University, Durham.
Larson, E. R., Eastwood, J. R., Buchanan, K. L., Bennett, A. T. D., and Berg, M. L. (2015). How does nest-box temperature affect nestling growth rate and breeding success in a parrot? Emu 115, 247–255. doi: 10.1071/MU14081
Larson, E. R., Eastwood, J. R., Micallef, S., Wehbe, J., Bennett, A. T. D., and Berg, M. L. (2018). Nest microclimate predicts bill growth in the Adelaide rosella (Aves: Psittaculidae). Biol. J. Linn. Soc. 124, 339–349. doi: 10.1093/biolinnean/bly058
Lindström, J. (1999). Early development and fitness in birds and mammals. Trends Ecol. Evol. 14, 343–348. doi: 10.1016/S0169-5347(99)01639-0
Lloyd, J. D., and Martin, T. E. (2004). Nest-site preference and maternal effects on offspring growth. Behav. Ecol. 15, 816–823. doi: 10.1093/beheco/arh085
Love, O. P., and Williams, T. D. (2008). Plasticity in the adrenocortical response of a free-living vertebrate: the role of pre- and post-natal developmental stress. Horm. Behav. 54, 496–505. doi: 10.1016/j.yhbeh.2008.01.006
Loyau, T., Bedrani, L., Berri, C., Métayer-Coustard, S., Praud, C., Coustham, V., et al. (2015). Cyclic variations in incubation conditions induce adaptive responses to later heat exposure in chickens: a review. Animal 9, 76–85. doi: 10.1017/S1751731114001931
Machín, P., Fernándex-Elipe, J., and Klaassen, R. H. G. (2018). The relative importance of food abundance and weather on the growth of a sub-arctic shorebird chick. Behav. Ecol. Sociobiol. 72, 42. doi: 10.1007/s00265-018-2457-y
Machmer, M. M., and Ydenberg, R. C. (1990). Weather and Osprey foraging energetics. Can. J. Zool. 68, 40–43. doi: 10.1139/z90-007
Mainwaring, M. C., and Hartley, I. R. (2016). Local weather conditions have complex effects on the growth of blue tit nestlings. J. Therm. Biol. 60, 12–19. doi: 10.1016/j.jtherbio.2016.05.005
Maness, T. J., and Anderson, D. J. (2013). Predictors of juvenile survival in birds. Ornithol. Monogr. 78, 1–55. doi: 10.1525/om.2013.78.1.1
Mariette, M. M., and Buchanan, K. L. (2016). Prenatal acoustic communication programs offspring for high posthatching temperatures in a songbird. Science 353, 812–814. doi: 10.1126/science.aaf7049
Marks, H. L. (1990). “Genetics of growth and meat production in other Galliforms (Japenese Quail, Guinea fowl, and ring-necked pheasants),” in Poultry Breeding and Genetics, ed. R. D. Crawford (Amsterdam: Elsevier Science Publishers), 677–690.
Marks, H. L. (1996). Long-term selection for body weight in japanese quail under different environments. Poult. Sci. 75, 1198–1203. doi: 10.3382/ps.0751198
McCarty, J. P., and Winkler, D. W. (1999). Relative importance off environmental variables in determining the growth off nestling Tree Swallows Tachycineta bicolor. Ibis 141, 286–296. doi: 10.1111/j.1474-919X.1999.tb07551.x
McCleery, R. H., Perrins, C. M., Sheldon, B. C., and Charmantier, A. (2008). Age-specific reproduction in a long-lived species: the combined effects of senescence and individual quality. Proc. R. Soc. B Biol. Sci. 275, 963–970. doi: 10.1098/rspb.2007.1418
McKechnie, A. E., and Wolf, B. O. (2010). Climate change increases the likelihood of catastrophic avian mortality events during extreme heat waves. Biol. Lett. 6, 253–256. doi: 10.1098/rsbl.2009.0702
McKinnon, L., Nol, E., and Juillet, C. (2013). Arctic-nesting birds find physiological relief in the face of trophic constraints. Sci. Rep. 3, 1816. doi: 10.1038/srep01816
McKinnon, L., Picotin, M., Bolduc, E., Juillet, C., and Bêty, J. (2012). Timing of breeding, peak food availability, and effects of mismatch on chick growth in birds nesting in the High Arctic. Can. J. Zool. 90, 961–971. doi: 10.1139/z2012-064
Merilä, J., Kruuk, L. E. B., and Sheldon, B. C. (2001). Cryptic evolution in a wild bird population. Nature 412, 76–79. doi: 10.1038/35083580
Merkling, T., Perrot, C., Helfenstein, F., Ferdy, J.-B., Gaillard, L., Lefol, E., et al. (2016). Maternal effects as drivers of sibling competition in a parent–offspring conflict context? An experimental test. Ecol. Evol. 6, 3699–3710. doi: 10.1002/ece3.1777
Merrill, L., and Grindstaff, J. L. (2018). Early life stress strengthens trait covariance: a plastic response that results in reduced flexibility. Am. Nat. 192, 593–604. doi: 10.1086/699839
Mignon-Grasteau, S. (1999). Genetic parameters of growth curve parameters in male and female chickens. Br. Poult. Sci. 40, 44–51. doi: 10.1080/00071669987827
Millien, V., Kathleen Lyons, S., Olson, L., Smith, F. A., Wilson, A. B., and Yom-Tov, Y. (2006). Ecotypic variation in the context of global climate change: revisiting the rules. Ecol. Lett. 9, 853–869. doi: 10.1111/j.1461-0248.2006.00928.x
Mitchell, A. E., Boersma, J., Anthony, A., Kitayama, K., and Martin, T. E. (2020). Experimental amelioration of harsh weather speeds growth and development in a tropical montane songbird. Am. Nat. 196, E110–E118. doi: 10.1086/710151
Mock, D. W., and Parker, G. A. (1997). The Evolution of Sibling Rivalry. Oxford: Oxford University Press.
Mock, D. W., and Parker, G. A. (1998). Siblicide, family conflict, and the evolutionary limits of selfishness. Anim. Behav. 56, 1–10.
Morrissey, M. B., and Liefting, M. (2016). Variation in reaction norms: statistical considerations and biological interpretation. Evolution. 70, 1944–1959. doi: 10.1111/evo.13003
Mueller, A. J., Miller, K. D., and Bowers, E. K. (2019). Nest microclimate during incubation affects posthatching development and parental care in wild birds. Sci. Rep. 9, 5161. doi: 10.1038/s41598-019-41690-4
Müller, M., and Groothuis, T. G. (2013). Within-clutch variation in yolk testosterone as an adaptive maternal effect to modulate avian sibling competition; evidence from a comparative study. Am. Nat. 181, 125–136.
Murphy, M. T. (1985). Nestling eastern kingbird growth: effects of initial size and ambient temperature. Ecology 66, 162–170. doi: 10.2307/1941316
Naef-Daenzer, B., and Keller, L. F. (1999). The foraging performance of great and blue tits (Parus major and P. caeruleus) in relation to caterpillar development, and its consequences for nestling growth and fledging weight. J. Anim. Ecol. 68, 708–718. doi: 10.1046/j.1365-2656.1999.00318.x
Nager, R. G., and Noordwijk, A. J. V. (1992). Energetic limitation in the egg-laying period of great tits. Proc. R. Soc. B Biol. Sci. 249, 259–263. doi: 10.1098/rspb.1992.0112
Ndithia, H. K., Bakari, S. N., Matson, K. D., Muchai, M., and Tieleman, B. I. (2017). Georgraphical and temporal variation in environmental conditions affects nestling growth but not immune function in a year-round breeding equatorial lark. Front. Zool. 14:28. doi: 10.1186/s12983-017-0213
N’Dri, A. L., Mignon-Grasteau, S., Sellier, N., Tixier-Boichard, M., and Beaumont, C. (2006). Genetic relationships between feed conversion ratio, growth curve and body composition in slow-growing chickens. Br. Poult. Sci. 47, 273–280. doi: 10.1080/00071660600753664
Nichelmann, M., and Tzschentke, B. (2002). Ontogeny of thermoregulation in precocial birds. Comp. Biochem. Physiol. A. Mol. Integr. Physiol. 131, 751–763. doi: 10.1016/S1095-6433(02)00013-2
Nilsson, J. F., Stjernman, M., and Nilsson, J. -Å (2008). Experimental reduction of incubation temperature affects both nestling and adult blue tits Cyanistes caeruleus. J. Avian Biol. 39, 553–559. doi: 10.1111/j.0908-8857.2008.04199.x
Noordwijk, A. J. V. (1982). Variation in body weight of the great tit, heritability and condition. Verh. Kon. Ned. Akad. Wetensch. Afd. Natuurk. Tweede Reeks 79, 9–12.
Noordwijk, A. J. V., and Marks, H. L. (1998). “Genetic aspects of growth,” in Avian Growth and Development: Evolution within the Altricial-Precocial Spectrum, eds J. M. Starck and R. E. Ricklefs (New York: Oxford University Press), 305–319.
Nord, A., and Giroud, S. (2020). Lifelong effects of thermal challenges during development in birds and mammals. Front. Physiol 11:419. doi: 10.3389/fphys.2020.00419
Nord, A., and Nilsson, J. -Å (2011). Incubation temperature affects growth and energy metabolism in blue tit nestlings. Am. Nat. 178, 639–651. doi: 10.1086/662172
Nord, A., and Nilsson, J. -Å (2019). Heat dissipation rate constrains reproductive investment in a wild bird. Funct. Ecol. 33, 250–259.
Nye, P. (1964). Heat loss in wet ducklings and chicks. Ibis 106, 189–197. doi: 10.1111/j.1474-919X.1964.tb03695.x
Oke, O. E., Alo, E. T., Oke, F. O., Oyebamiji, Y. A., Ijaiya, M. A., Odefemi, M. A., et al. (2020). Early age thermal manipulation on the performance and physiological response of broiler chickens under hot humid tropical climate. J. Therm. Biol. 88, 102517. doi: 10.1016/j.jtherbio.2020.102517
Patrick, S. C., Bearhop, S., Grémillet, D., Lescroël, A., Grecian, W. J., Bodey, T. W., et al. (2014). Individual differences in searching behaviour and spatial foraging consistency in a central place marine predator. Oikos 123, 33–40. doi: 10.1111/j.1600-0706.2013.00406.x
Peck, D. R., Smithers, B. V., Krockenberger, A. K., and Congdon, B. C. (2004). Sea surface temperature constrains wedge-tailed shearwater foraging success within breeding seasons. Mar. Ecol. Prog. Ser. 281, 259–266. doi: 10.3354/meps281259
Pérez, J. H., Ardia, D. R., Chad, E. K., and Clotfelter, E. D. (2008). Experimental heating reveals nest temperature affects nestling condition in tree swallows Tachycineta bicolor. Biol. Lett. 4, 468–471. doi: 10.1098/rsbl.2008.0266
Pérez, J. H., Krause, J. S., Chmura, H. E., Bowman, S., McGuigan, M., Asmus, A. L., et al. (2016). Nestling growth rates in relation to food abundance and weather in the Arctic. Auk 133, 261–272. doi: 10.1642/AUK-15-111.1
Piatt, J. F., Parrish, J. K., Renner, H. M., Schoen, S. K., Jones, T. T., Arimitsu, M. L., et al. (2020). Extreme mortality and reproductive failure of common murres resulting from the northeast Pacific marine heatwave of 2014-2016. PLoS One 15:e0226087. doi: 10.1371/journal.pone.0226087
Pinaud, D., Cherel, Y., and Weimerskirch, H. (2005). Effect of environmental variability on habitat selection, diet, provisioning behaviour and chick growth in yellow-nosed albatrosses. Mar. Ecol. Prog. Ser. 298, 295–304. doi: 10.3354/meps298295
Postma, E., and Brommer, J. E. (2014). “Four decades of estimating heritabilities in wild vertebrate populations,” in Quantitative Genetics in the Wild, eds A. Charmantier, D. Garant, and L. E. B. Kruuk (New York, NY: Oxford University Press), 16–33.
Price, E. R., and Dzialowski, E. M. (2018). Development of endothermy in birds: patterns and mechanisms. J. Comp. Physiol. 188, 373–391. doi: 10.1007/s00360-017-1135-0
Reed, T. E., Waples, R. S., Schindler, D. E., Hard, J. J., and Kinnison, M. T. (2010). Phenotypic plasticity and population viability: the importance of environmental predictability. Proc. R. Soc. B Biol. Sci. 277, 3391–3400. doi: 10.1098/rspb.2010.0771
Reid, J. M., Monaghan, P., and Ruxton, G. D. (2000). Resource allocation between reproductive phases: the importance of thermal conditions in determining the cost of incubation. Proc. R. Soc. B Biol. Sci. 267, 37–41. doi: 10.1098/rspb.2000.0963
Reynolds, P. S. (1996). Brood reduction and siblicide in Black-billed magpies (Pica pica). Auk 113, 189–199.
Ricklefs, R. E. (1968). Patterns of Growth in Birds. Ibis 110, 419–451. doi: 10.1111/j.1474-919X.1968.tb00058.x
Rivkin, L. R., Santangelo, J. S., Alberti, M., Aronson, M. F. J., de Keyzer, C. W., Diamond, S. E., et al. (2019). A roadmap for urban evolutionary ecology. Evol. Appl. 12, 384–398. doi: 10.1111/eva.12734
Robinson, B. G., Franke, A., and Derocher, A. E. (2017). Weather-mediated decline in prey delivery rates causes food-limitation in a top avian predator. J. Avian Biol. 48, 748–758. doi: 10.1111/jav.01130
Rodríguez, S., and Barba, E. (2016a). Effects of cool nest microclimates on nestling development: an experimental study with Mediterranean great tits Parus major. Ardeola. 63, 251–260. doi: 10.13157/arla.63.2.2016.ra2
Rodríguez, S., and Barba, E. (2016b). Nestling growth is impaired by heat stress: an experimental study in a Mediterranean great tit population. Zool. Stud. 55, e40. doi: 10.6620/zs.2016.55-40
Roff, D. A., and Wilson, A. J. (2014). “Quantifying genotype-by-environment interactions in laboratory systems,” in Genotype–Environment Interactions and Sexual Selection, eds J. Hunt and D. J. Hosken (Oxford: John Wiley & Sons), 101–136.
Romanoff, A. L. (1944). Avian spare yolk and its assimilation. Auk 61, 235–241. doi: 10.2307/4079367
Ruuskanen, S., Groothuis, T. G. G., Schaper, S. V., Darras, V. M., de Vries, B., and Visser, M. E. (2016). Temperature-induced variation in yolk androgen and thyroid hormone levels in avian eggs. Gen. Comp. Endocrinol. 235, 29–37. doi: 10.1016/j.ygcen.2016.05.026
Salaberria, C., Celis, P., López-Rull, I., and Gil, D. (2014). Effects of temperature and nest heat exposure on nestling growth, dehydration and survival in a Mediterranean hole-nesting passerine. Ibis 156, 265–275. doi: 10.1111/ibi.12121
Salewski, V., and Watt, C. (2017). Bergmann’s rule: a biophysiological rule examined in birds. Oikos 126, 161–172. doi: 10.1111/oik.03698
Sæther, B.-E., and Bakke, Ø (2000). Avian life history variation and contribution of demographic traits to the population growth rate. Ecology 81, 642–653.
Scheiner, S. M. (1993). Genetics and evolution of phenotypic plasticity. Annu. Rev. Ecol. Syst. 24, 35–68. doi: 10.1146/annurev.es.24.110193.000343
Schekkerman, H., Roomen, M. W. J., and Underhill, L. G. (1998). Growth, behaviour of broods and weather-related variation in breeding productivity of Curlew Sandpipers Calidris Ferruginea. Ardea 86, 153–168.
Schew, W. A., and Ricklefs, R. E. (1998). “Developmental plasticity,” in Avian Growth and Development: Evolution within the Altricial-Precocial Spectrum, eds J. M. Starck and R. E. Ricklefs (New York, NY: Oxford University Press), 288–300.
Schwabl, H. (1996). Maternal testosterone in the avian egg enhances postnatal growth. Comp. Biochem. Physiol. A Physiol. 114, 271–276. doi: 10.1016/0300-9629(96)00009-6
Shinder, D., Luger, D., Rusal, M., Rzepakovsky, V., Bresler, V., and Yahav, S. (2002). Early age cold conditioning in broiler chickens (Gallus domesticus) : thermotolerance and growth responses. J. Therm. Biol. 27, 517–523. doi: 10.1016/S0306-4565(02)00025-6
Sicurella, B., Caffi, M., Caprioli, M., Rubolini, D., Saino, N., and Ambrosini, R. (2014). Weather conditions, brood size and hatching order affect Common Swift Apus apus nestlings’ survival and growth. Bird Study 62, 64–77. doi: 10.1080/00063657.2014.989193
Siikamäki, P. (1996). Nestling growth and mortality of Pied Flycatchers Ficedula hypoleuca in relation to weather and breeding effort. Ibis 138, 471–478. doi: 10.1111/j.1474-919X.1996.tb08067.x
Singsen, E. P., Nagel, J., Patrick, S. G., and Matterson, L. D. (1964). The effect of a lysine deficiency on body weight and age at sexual maturity of meat-type pullets. Poult. Sci. 43, 786–787. doi: 10.3382/ps.0430786
Smith, H. G., and Wettermark, K. J. (1995). Heritability of nestling growth in cross-fostered European Starlings Sturnus vulgaris. Genetics 141, 657–665.
Smithers, B. V., Peck, D. R., Krockenberger, A. K., and Congdon, B. C. (2003). Elevated sea-surface temperature, reduced provisioning and reproductive failure of wedge-tailed shearwaters (Puffinus pacificus) in the southern Great Barrier Reef, Australia. Mar. Freshw. Res. 54, 973–977. doi: 10.1071/mf02137
Snell-Rood, E. C., Kobiela, M. E., Sikkink, K. L., and Shephard, A. M. (2018). Mechanisms of plastic rescue in novel environments. Annu. Rev. Ecol. Evol. Syst. 49, 331–354. doi: 10.1146/annurev-ecolsys-110617-062622
Starck, J. M., and Ricklefs, R. E. (1998). Avian Growth and Development: Evolution within the Altricial-Precocial Spectrum. Oxford: Oxford University Press on Demand.
Stearns, S. C. (1989). Trade-offs in life-history evolution. Funct. Ecol. 3, 259–268. doi: 10.2307/2389364
Steigner, J. W., Nestor, K. E., and Lilburn, M. S. (1992). Growth and development of lines of Japanese quail, Coturnix coturnix japonica, divergently selected for body weight at four weeks of age. Comp. Biochem. Physiol. 102, 389–393. doi: 10.1016/0300-9629(92)90152-g
Stenseth, N. C., Mysterud, A., Ottersen, G., Hurrell, J. W., Chan, K. S., and Lima, M. (2002). Ecological effects of climate fluctuations. Science 297, 1292–1296. doi: 10.1126/science.1071281
Stevens, G. C. (1989). The latitudinal gradient in geographical range: how so many species coexist in the tropics. Am. Nat. 133, 240–256.
Tapper, S., Nocera, J. J., and Burness, G. (2020). Heat dissipation capacity influences reproductive performance in an aerial insectivore. J. Exp. Biol. 223, jeb222232. doi: 10.1242/jeb.222232
Taylor, I. R. (1983). Effect of wind on the foraging behaviour of Common and Sandwich Terns. Ornis Scand. 14, 90–96. doi: 10.2307/3676011
Teplitsky, C., and Millien, V. (2014). Climate warming and Bergmann’s rule through time: is there any evidence? Evol. Appl. 7, 156–168. doi: 10.1111/eva.12129
Thackeray, S. J., Henrys, P. A., Hemming, D., Bell, J. R., Botham, M. S., Burthe, S., et al. (2016). Phenological sensitivity to climate across taxa and trophic levels. Nature 535, 241–245. doi: 10.1038/nature18608
Thomson, C. E., Bayer, F., Crouch, N., Farrell, S., Heap, E., Mittell, E., et al. (2017). Selection on parental performance opposes selection for larger body mass in a wild population of blue tits. Evolution 71, 716–732. doi: 10.1111/evo.13169
Thomson, C. E., and Hadfield, J. D. (2017). Prenatal maternal effects appear to be insensitive to experimental or natural environmental variation. Funct. Ecol. 31, 2008–2020. doi: 10.1111/1365-2435.12896
Tigano, A., and Friesen, V. L. (2016). Genomics of local adaptation with gene flow. Mol. Ecol. 25, 2144–2164. doi: 10.1111/mec.13606
Tjørve, E., and Tjørve, K. M. C. (2010). A unified approach to the Richards-model family for use in growth analyses: why we need only two model forms. J. Theor. Biol. 267, 417–425. doi: 10.1016/j.jtbi.2010.09.008
Tjørve, K. M. C., and Tjørve, E. (2017). Modelling avian growth with the Unified-Richards: as exemplified by wader-chick growth. J. Avian Biol. 48, 770–784. doi: 10.1111/jav.00992
Torralba, V., Doblas-Reyes, F. J., and Gonzalez-Reviriego, N. (2017). Uncertainty in recent near-surface wind speed trends: a global reanalysis intercomparison. Environ. Res. Lett. 12, 114019. doi: 10.1088/1748-9326/aa8a58
van de Pol, M., Bailey, L. D., McLean, N., Rijsdijk, L., Lawson, C. R., and Brouwer, L. (2016). Identifying the best climatic predictors in ecology and evolution. Methods Ecol. Evol. 7, 1246–1257. doi: 10.1111/2041-210X.12590
Van de Ven, T. M. F. N. (2017). Implications of Climate Change on the Reproductive Success of the Southern Yellow-billed Hornbill, Tockus leucomelas. Doctoral dissertation, University of Cape Town, Cape Town.
van Gils, J. A., Lisovski, S., Lok, T., Meissner, W., Ozarowska, A., de Fouw, J., et al. (2016). Body shrinkage due to Arctic warming reduces red knot fitness in tropical wintering range. Sci. Rep. 352, 819–822. doi: 10.1126/science.aad6351
Vedder, O., Bouwhuis, S., and Sheldon, B. C. (2013). Quantitative assessment of the importance of phenotypic plasticity in adaptation to climate change in wild bird populations. PLoS Biol. 11:e1001605. doi: 10.1371/journal.pbio.1001605
Verboven, N., Monaghan, P., Evans, D. M., Schwabl, H., Evans, N., Whitelaw, C., et al. (2003). Maternal condition, yolk androgens and offspring performance: a supplemental feeding experiment in the lesser black-backed gull (Larus fuscus). Proc. Biol. Sci. 270, 2223–2232. doi: 10.1098/rspb.2003.2496
Vergauwen, J., Goerlich, V., Groothuis, T. G. G., Eens, M., and Muller, W. (2012). Food conditions affect yolk testosterone deposition but not incubation attendance. Gen. Comp. Endocrinol. 176, 112–119.
Via, S., and Lande, R. (1985). Genotype-environment interaction and the evolution of phenotypic plasticity. Evolution 39, 505–522. doi: 10.1111/j.1558-5646.1985.tb00391.x
Visser, G. H. (1998). “Development of temperature regulation,” in Avian Growth and Development: Evolution within the Altricial-Precocial Spectrum, eds J. M. Starck and R. E. Ricklefs (New York: Oxford University Press), 117–150.
Visser, M. E., Holleman, L. J. M., and Gienapp, P. (2006). Shifts in caterpillar biomass phenology due to climate change and its impact on the breeding biology of an insectivorous bird. Oecologia 147, 164–172. doi: 10.1007/s00442-005-0299-6
Walsh, B., and Lynch, M. (2018). Evolution and Selection of Quantitative Traits. Oxford, New York: Oxford University Press.
Webb, D. R. (1987). Thermal tolerance of avian embryos: a review. Condor 89, 874–888. doi: 10.2307/1368537
Weimerskirch, H., Zimmermann, L., and Prince, P. A. (2001). Influence of environmental variability on breeding effort in a long-lived seabird, the Yellow-nosed Albatross. Behav. Ecol. 12, 22–30. doi: 10.1093/oxfordjournals.beheco.a000374
Whitfield, M. C., Smit, B., McKechnie, A. E., and Wolf, B. O. (2015). Avian thermoregulation in the heat: scaling of heat tolerance and evaporative cooling capacity in three southern African arid-zone passerines. J. Exp. Biol. 218, 1705–1714. doi: 10.1242/jeb.121749
Whittow, G. C., and Tazawa, H. (1991). The early development of thermoregulation in birds. Physiol. Zool. 64, 1371–1390.
Wiley, E. M., and Ridley, A. R. (2016). The effects of temperature on offspring provisioning in a cooperative breeder. Anim. Behav. 117, 187–195. doi: 10.1016/j.anbehav.2016.05.009
Willham, R. L. (1972). The role of maternal effects in animal breeding. 3. Biometrical aspects of maternal effects in animals. J. Anim. Sci. 35, 1288–1293.
Williams, T. (2012). Physiological Adaptations for Breeding in Birds. Woodstock: Princeton University Press.
Wilson, A. J., Pilkington, J. G., Pemberton, J. M., Coltman, D. W., Overall, A. D. J., Byrne, K. A., et al. (2005). Selection on mothers and offspring: whose phenotype is it and does it matter? Evolution 59, 451–463. doi: 10.1111/j.0014-3820.2005.tb01003.x
Wilson, A. J., Réale, D., Clements, M. N., Morrissey, M. M., Postma, E., Walling, C. A., et al. (2010). An ecologist’s guide to the animal model. J. Anim. Ecol. 79, 13–26. doi: 10.1111/j.1365-2656.2009.01639.x
Woo, K. J., Elliott, K. H., Davidson, M., Gaston, A. J., and Davoren, G. K. (2008). Individual specialization in diet by a generalist marine predator reflects specialization in foraging behaviour. J. Anim. Ecol. 77, 1082–1091. doi: 10.1111/j.1365-2656.2008.01429.x
Wood, C. W., and Brodie, E. D. (2016). Evolutionary response when selection and genetic variation covary across environments. Ecol. Lett. 19, 1189–1200. doi: 10.1111/ele.12662
Keywords: environmental change, development, heritability, life-history, maternal effects, natural selection, plasticity
Citation: Sauve D, Friesen VL and Charmantier A (2021) The Effects of Weather on Avian Growth and Implications for Adaptation to Climate Change. Front. Ecol. Evol. 9:569741. doi: 10.3389/fevo.2021.569741
Received: 05 June 2020; Accepted: 04 January 2021;
Published: 22 January 2021.
Edited by:
Andreas Nord, Lund University, SwedenReviewed by:
Simon Charles Griffith, Macquarie University, AustraliaKate Buchanan, Deakin University, Australia
Copyright © 2021 Sauve, Friesen and Charmantier. This is an open-access article distributed under the terms of the Creative Commons Attribution License (CC BY). The use, distribution or reproduction in other forums is permitted, provided the original author(s) and the copyright owner(s) are credited and that the original publication in this journal is cited, in accordance with accepted academic practice. No use, distribution or reproduction is permitted which does not comply with these terms.
*Correspondence: Drew Sauve, MGFzNjlAcXVlZW5zdS5jYQ==; c2F1dmUuZHJld0BnbWFpbC5jb20=