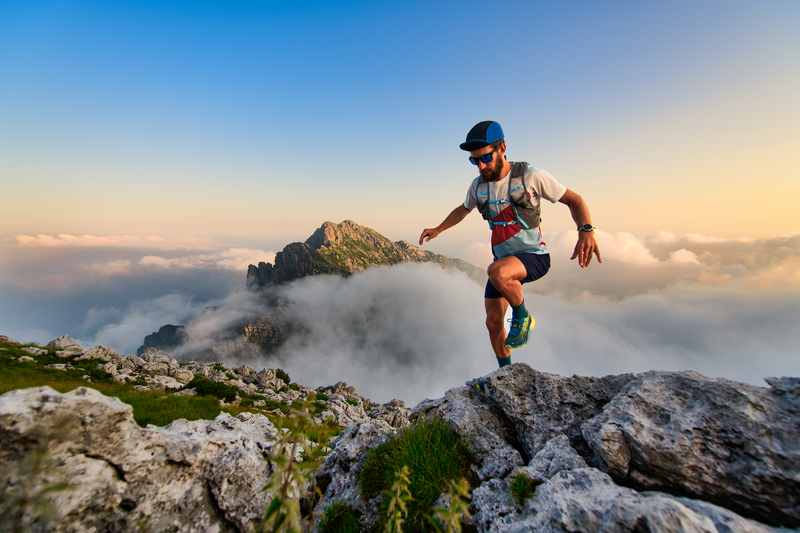
94% of researchers rate our articles as excellent or good
Learn more about the work of our research integrity team to safeguard the quality of each article we publish.
Find out more
ORIGINAL RESEARCH article
Front. Ecol. Evol. , 07 December 2020
Sec. Behavioral and Evolutionary Ecology
Volume 8 - 2020 | https://doi.org/10.3389/fevo.2020.591074
This article is part of the Research Topic Trans-Generational Plasticity View all 11 articles
Transgenerational plasticity, which occurs when the environment experienced by parents changes the phenotype of offspring, is widespread in animal and plant species. Both maternal and paternal environments can underlie transgenerational plasticity, but experimental studies unraveling how their effects interact together and with the personal (both developmental and immediate) environments are still rare. Yet unraveling these interactions is fundamental to understanding how offspring integrate past and present environmental cues to produce adaptive phenotype. Using the hermaphroditic and freshwater snail Physa acuta, we tested how predator cues experienced by offspring, mothers and fathers interact to shape offspring anti-predator behavior. We raised a first generation of snails in the laboratory with or without chemical predator cues and realized full-factorial crosses to disentangle maternal and paternal cues. We then raised the second generation of snails with or without predator cues and assessed, when adults, their escape behavior in two immediate environments (with or without predator cues) and activity in the immediate environment without predator cues. We found that personal, maternal, and paternal predator cues interacted to shape offspring escape behavior and activity. Firstly, for escape behavior, snails integrated the cues from developmental and parental environments only when exposed to predator cues in their immediate environment, suggesting that personal immediate experience must corroborate the risky parental environment to reveal transgenerational plasticity. For activity, this same hypothesis helps explain why no clear pattern of transgenerational plasticity was revealed, as activity was only measured without predator cues in the immediate environment. Secondly, a single maternal exposure to predator cues decreased offspring escape behavior while a single paternal exposure had no effect, surprisingly demonstrating sex-specific transgenerational plasticity for a simultaneous hermaphroditic species. Thirdly, when both mother and father were exposed, paternal cues were integrated by offspring according to their own developmental environment. The paternal exposure then mitigated the reduction in escape behavior due to the maternal exposure only when offspring developed in control condition. Overall, our study highlighted complex patterns of sex-specific transgenerational plasticity resulting from non-additive interactions between parental, developmental and immediate experiences.
Phenotypic plasticity, i.e., change in the phenotype of an organism induced by variation in the environment, can occur within the lifetime of the organism (within-generational plasticity WGP) or across generations (transgenerational plasticity TGP). TGP can occur through its effect on parental condition: favorable or stressful environments affect parental state (e.g., body weight, reproductive performance), which in turn positively or negatively affects offspring state (state-based TGP; Donelan et al., 2020). TGP can also result from natural selection as a mechanism to pass on reliable cues to offspring about their future environment (adaptive TGP; Galloway and Etterson, 2007; Burgess and Marshall, 2014; Yin et al., 2019), allowing them to adjust their phenotype. For example, in the bryozoan Bugula neritina, offspring of copper-exposed mothers were more resistant to copper than those of unexposed mothers (Marshall, 2008), as maternal exposure to copper potentially indicates that the offspring environment will be copper-rich. TGP is therefore a potential mechanism of adaptation for organisms to cope with rapid environmental changes (Agrawal et al., 1999; Donelson et al., 2018). A central question is to understand how information cues and state-based constraints from parental (TGP) and personal (WGP) experiences are integrated by offspring to produce adaptive responses to environment (Stamps and Krishnan, 2014; Leimar and McNamara, 2015; Stein et al., 2018).
Research on TGP has mainly focused on effects of both parents indiscriminately or on effects of maternal environment alone. More recently, some studies have shown that paternal environment can also have a strong effect on offspring phenotype (review in Krawetz, 2005; Crean and Bonduriansky, 2014; Immler, 2018). However, the extent to which offspring attend to cues of their parents can depend on the sex of the parent leading to different maternal and paternal effects on offspring phenotype (sex-specific TGP; e.g., Magiafoglou and Hoffmann, 2003; Ducatez et al., 2012; Bonduriansky et al., 2016; Zuccolo et al., 2016; Emborski and Mikheyev, 2019; Gilad and Scharf, 2019; Burke et al., 2020). Sex-specific TGP may be explained by different mechanisms of cue transmission between sexes (Bell and Hellmann, 2019). Sex-specific TGP may also occur when the sexes are under different selection pressures (e.g., when sexes show sexual dimorphism, differences in reproductive strategies, dispersal or ecology) generating differences in the reliability of maternal and paternal information about the offspring future environment (Kamel et al., 2010; Bell and Hellmann, 2019; Burke et al., 2020). For example, if males and females have different ecologies, only the cue perceived by the same-sex parent can reliably predict offspring environment and offspring should then be more influenced by the same-sex parental cue (Hellmann et al., 2019; Burke et al., 2020).
The effects of maternal and paternal environments can interact with each other. TGP patterns then differ whether one parent or both parents are exposed to environmental variation. This interaction may be even more complicated as offspring also integrate cues from their own personal environments. Personal cues can come from offspring’s past experiences during ontogeny or from their immediate environment. Integrating multiple cues (maternal, paternal and personal) may allow to fine-tune the offspring phenotype according to past and present environmental information. However, it is still not clear how offspring integrate these different sources of information, sometimes consistent or conflicting, and prioritize them. Effects of cues may simply be additive: an increasing number of cues in agreement increases linearly with adaptive phenotype (additive effects; e.g., Akkerman et al., 2016; Zizzari et al., 2016). Effects of cues may also interact and result in non-additive effects (e.g., Galloway, 2001; Hellmann et al., 2019). For example, offspring may respond similarly whether they receive one or several cues in agreement (back-up hypothesis; Bell and Hellmann, 2019). Offspring may also respond only when their personal cues corroborate parental cues or only when both parental cues are consistent (threshold hypothesis; Bell and Hellmann, 2019). Thus, understanding how the effects of personal, maternal and paternal environments interact is necessary to understand variations in TGP patterns and the possible implication of parental sex in its evolution.
In this laboratory study, we investigated how effects of maternal, paternal and personal (both developmental and immediate) exposures to cues of predator presence interact to shape anti-predator behaviors. Many prey engage in anti-predatory behaviors when they or their parents detect predator cues (predator-induced WGP: Lima and Dill, 1990; predator-induced TGP: review in Tariel et al., 2020). We used the freshwater snail Physa acuta as our model system. Physa species are known for their anti-predator behavior: they escape by crawling-out the water or seek refuge after detecting crayfish or fish odors (Alexander and Covich, 1991; DeWitt et al., 1999; Turner et al., 1999). One study has also shown a reduction of activity after detection of crayfish odors (Sih and McCarthy, 2002). We have already shown predator-induced WGP and TGP on escape behavior of P. acuta (Luquet and Tariel, 2016; Tariel et al., 2020), while another study detected only WGP and no TGP (Beaty et al., 2016). However, none of these studies have yet disentangled the potential interactions between immediate, developmental, maternal and paternal environments. We raised a parental generation of snails with or without chemical predator cues (non-lethal predators) and realized full-factorial crosses. We then raised the offspring generation with or without predator cues. At the adult stage, we measured two anti-predator behaviors: (1) escape behavior in two immediate environments (with or without predator cues) and (2) activity without predator cues in the immediate environment. P. acuta is a simultaneous hermaphrodite with internal fertilization and does not benefit from any parental care. These reproductive characteristics imply that the mother (egg donor) and father (sperm donor) have the same ecology, gamete dispersal and no sexual dimorphism: predator cues perceived by the mother and father should carry the same information about future predator presence and trigger the same response on offspring anti-predator behavior; hence, we do not expect sex-specific TGP (Bell and Hellmann, 2019; Burke et al., 2020). We can then more easily study how personal (immediate and developmental), maternal and paternal cues interact to influence offspring anti-predator behaviors.
Wild adult Physa acuta snails were collected in February 2017 in a lentic backwater of the Rhône River in Lyon, France (N 45°48′06″ E 04°55′33″). P. acuta is a globally invasive freshwater snail from North America (Lydeard et al., 2016). These snails constituted the F0 generation (see Figure 1 for number of individuals; see Appendix 1 for the schematic experimental design). In the laboratory, F0 snails were pooled in a 10 L plastic box filled with dechlorinated tap water (control water hereafter) and interbreed overnight. Then, they were isolated in 80 mL plastic boxes (these rearing boxes were used until the end of the experiment; 4.5 × 6 cm) filled with control water to ensure that a box contained the progeny of only one F0 snail (i.e., one F1 family per box). They laid eggs during 24 h, which was enough for most snails to lay an egg mass, and then were removed. F1 eggs developed until hatching at 25°C (ca. 7 days). After hatching and until the end of snail development, they were reared in a room at 25°C with a 12 h/12 h photoperiod. Snails were fed ad libitum with boiled and mixed lettuce. The water and food were renewed twice a week. Siblings of each F1 family developed together for 10 days in control water without being manipulated (P. acuta newborns are very small and easily damaged). Then, siblings of each F1 family were split into two developmental environments: six siblings remained in control (C) water and six siblings moved to predator-cue (P) water (Figure 1). F1 families with less than 12 siblings were discarded (see Figure 1 for number of families). After 7 days, F1 snails were isolated in the same environment (control or predator-cue water) and developed individually until 31 days old to reach a size large enough to be sexually mature (see Figure 1 for number of individuals). The predator-cue water was obtained by (1) mixing the rearing water of six Orconectes limosus crayfish (reared individually in 4 L box of dechlorinated tap water) and (2) “infusing” several smashed P. acuta adult snails (one snail for every 4 L) for 1 h before using the predator-cue water. These smashed snails were then used as crayfish food.
Figure 1. Full factorial design to disentangle the effects of maternal, paternal, developmental and immediate exposures to predator cues on anti-predator behaviors. F1 (maternal and paternal) generation were reared in two developmental environments (“C” stands for control and “P” for predator-cue environment). Then four combinations of maternal x paternal environments (CxC, CxP, PxC, and PxP) were realized. Finally, F2 (offspring) generation were reared in the same two developmental environments. Number of families (N) and number of individuals (n) are indicated, including number of individuals used for behavioral assessments.
To generate the F2 generation, we performed three types of pairs with F1 snails (each partner in a pair generated a progeny as P. acuta is a hermaphrodite; Figure 1):
(1) the two partners coming from control environment, to generate combination of maternal C × paternal C environments;
(2) the two partners coming from predator-cue environment, to generate combination of maternal P × paternal P environments;
(3) the two partners coming from different environments, to generate combinations of maternal C × paternal P environments and maternal P × paternal C environments.
We paired partners from different families, and we were careful to associate the same families in the three types of pairs. To keep the partner identity during copulation, we painted shells (Henry and Jarne, 2007). We put the two partners into a rearing box for 24 h to copulate (P. acuta prefers outcross; Tsitrone et al., 2003). We then submitted snails of the F2 generation to the two treatments (control or predator-cue) following the same protocol as for the F1 generation (see Figure 1 for number of individuals). As F2 snails developed slower, they developed until 61 days old to reach a size large enough for their weight to be accurately measured.
Finally, our full factorial design resulted in four combinations of maternal and paternal environments (CxC, PxC, CxP, PxP) and in two F2 offspring developmental environments (C, P) (Figure 1).
We assessed two anti-predator behaviors: escape behavior by the time taken to crawl-out the water (sec) and activity by the total covered distance (cm). These two behaviors were assessed on two different subsamples of F2 snails (Figure 1). The sub-samples were composed of as many different F2 families as possible.
For each trial of escape behavior, the snail first acclimated in a chamber at the center of a behavioral arena (4.5 × 6 cm box with 2.3 cm water and 0.7 cm polystyrene at the bottom). A minute later, we opened the acclimation chamber and recorded time taken by the snail to crawl to the water surface with JWatcher (Blumstein and Daniel, 2007). After 5 min, we put back the snail in its rearing box for at least 1 h before the next trial and we changed arena water. For each snail, the escape behavior trial was repeated two times in each immediate environment (two times in control water first and two times in predator-cue water after).
For each trial of activity, the snail first acclimated 1 min in the entire behavioral arena (11.5 × 17.5 cm box with 0.6 cm water). We then recorded the snail position every 30 s on a grid (0.5 cm tiles) drawn at the bottom of the arena. After 5 min, we put back the snail in its rearing box for at least 1 h before the next trial and we changed arena water. The activity trial was repeated two times only in control water. As snails escape (crawl-out the water) in predator-cue water, the activity could not be assessed in this immediate environment.
After assessing behavior, we gently dried snails with a paper towel and measured total wet mass (body and shell) with an electronic scale at the nearest 0.0001 g.
We tested with two separate linear mixed models (LMMs) whether maternal, paternal and developmental environments influenced F2 snails’ escape behavior and activity. For the escape behavior, a LMM was used instead of a survival model as just a few trials were censored (censoring means that the snail did not crawl-out the water within the five min trial; 32 trials out of 640 trials). The time to crawl-out was transformed with a log10 transformation to achieve normality. We also multiplied by −1 the time to crawl-out to better reflect escape behavior, as a short time to crawl-out is associated with high escape behavior. The fixed effects of the two LMMs included maternal (E1m), paternal (E1p) and developmental (E2) environments and all interactions. For the LMM of escape behavior, we added the immediate (I) environment and all interactions. For all LMMs, we also added the scaled snail mass to control for size. We reduced the number of fixed effects using the model selection explained in Zuur et al. (2009). The random effect of the two LMMs included individual identity to control for intrinsic differences in behavior between individuals. We fitted LMMs with restricted maximum likelihood estimation and Kenward and Roger’s approximation for degrees of freedom using the lmer() function (Bates et al., 2015). Type 2 F-tests were used for significance of fixed effects with the package lmerTest (Kuznetsova et al., 2017). Post hoc comparisons were realized with the package emmeans (Lenth, 2019) to compare the “control” treatment (both parents not exposed: CxC) with the other treatments (only exposed mothers: PxC; only exposed fathers: CxP; and both parents exposed: PxP). These post hoc comparisons are reported in Appendix 2. All statistical analyses were performed with R 3.6.0 (R Development Core Team, 2019).
Maternal (E1m), paternal (E1p), developmental (E2) and immediate (I) environments all influenced escape behavior and their effects interacted with each other (significant E1m × E1p × E2 interaction and E1m × E1p × I interaction in Table 1 and Figure 2). In the immediate environment without predator cues, neither maternal nor paternal environments influenced escape behavior (Figure 2A and Appendix 2). In the immediate environment with predator cues, both maternal and paternal environments influenced escape behavior. The single maternal exposure to predator cues (PxC) resulted in a 49 s (61%) slower escape than unexposed parents (CxC; black solid arrows on Figure 2B) while the single paternal exposure (CxP) did not influence escape behavior (Figure 2B and Appendix 2). The combination of maternal and paternal exposures (PxP) resulted in a similar time to escape than unexposed parents (CxC) when offspring developed without predator cues (Figure 2Bi and Appendix 2). Conversely, the combination of maternal and paternal exposures (PxP) resulted in a 28 s (31%) slower escape than unexposed parents (CxC) when offspring developed with predator cues (Figure 2Bii and Appendix 2).
Figure 2. How maternal, paternal, and developmental exposures to predator cues influenced escape behavior (time to crawl-out the water in seconds) in the (A) control and (B) predator-cue immediate environments. “C” stands for control environment and “P” stands for predator-cue environment. On each graph, the “C” developmental environment is shown on the left panel (Ai, Bi) and the “P” development environment is shown on the right panel (Aii, Bii). The x-axis represents the four combinations of maternal x paternal environments (CxC, PxC, CxP, and PxP). The horizontal dotted line is located at the mean of CxC to facilitate the comparisons with PxC, CxP, and PxP (see Appendix 2 for post hoc comparisons). Dots are for snails from non-exposed mothers while squares are for snails from exposed mothers. White shapes are for snails from non-exposed fathers while gray shapes are for snails from exposed fathers. To see the effect of single maternal exposure, single paternal exposure or combined parental exposures, follow the legend on the top right corner. Black arrows indicate a significant effect (Appendix 2). Points are mean ± SE.
Differences in escape behavior among snails were consistent across the different behavioral trials (significant individual random effect in Table 1).
Maternal (E1m), paternal (E1p) and developmental (E2) environments all influenced activity and their effects interacted with each other (significant E1m × E1p × E2 interaction in Table 2 and Figure 3). However, post hoc comparisons did not reveal significant differences between the activity of offspring from unexposed parents (CxC) to the activity of offspring from exposed mothers, exposed fathers or both exposed parents (PxC, CxP, or PxP).
Figure 3. How maternal, paternal and developmental exposures to predator cues influenced activity (covered distance in centimeters) in control immediate environment. “C” stands for control environment and “P” for predator-cue environment. The “C” developmental environment is shown on the left panel (i) and the “P” developmental environment is shown on the right panel (ii). The x-axis represents the four combinations of maternal x paternal environments (CxC, PxC, CxP, and PxP). The horizontal dotted line is located at the mean of CxC to facilitate the comparisons with PxC, CxP, and PxP (see Appendix 2 for post hoc comparisons). Dots are for snails from non-exposed mothers while squares are for snails from exposed mothers. White shapes are for snails from non-exposed fathers while gray shapes are for snails from exposed fathers. To see the effect of single maternal exposure, single paternal exposure or combined parental exposures, follow the legend on the top right corner. Points are mean ± SE.
Differences in activity among snails were consistent across the different behavioral trials (significant individual random effect in Table 2).
We know little about how offspring integrate cues from the maternal, paternal, developmental and immediate environments (Stein et al., 2018). Recent theory showed that the relative weight given to a cue depends on its accuracy as a predictor of selective conditions in the future (Leimar and McNamara, 2015). In the context of maternal and paternal cue integration, both additive (Akkerman et al., 2016) and non-additive (e.g., Galloway, 2001; Valtonen et al., 2012; Guillaume et al., 2016) TGP patterns have been observed. Our results show that all past (maternal, parental, and developmental) and immediate environmental experiences had an influence on the offspring anti-predator behaviors resulting in a complex non-additive TGP patterns.
The maternal, paternal, and developmental environments had an influence on escape behavior but only in the immediate context of predation risk. This means that snails integrated the cues from parental and developmental environments only in the light of the immediate environment (Figure 2B). The immediate environment being the most accurate information about the predation risk, the snails did not exhibit differences in escape behavior when the immediate environment was safe (without predator cues), even if they or their parents were exposed to predator cues. Indeed, the maternal exposure decreased the escape behavior of offspring only in the immediate predation environment whatever the offspring developmental environment (Figure 2B). In the immediate safe environment (Figure 2A), this negative maternal effect is likely masked by an overall absence of behavioral response. Indeed, the time to crawl-out in immediate safe environment was ca. 150 s for all snails (Figure 2A) while in the immediate predation environment (Figure 2B), only the snails from exposed mothers took 150 s to escape, the others snails escaping faster (ca. 90 s). This negative maternal effect is likely revealed only when offspring must express behavioral defenses against predators (faster escape in immediate predation environment).
For activity of offspring, we found consistently that maternal, paternal, and developmental environments interacted. However, we did not identify significant patterns resulting from this triple interaction. As for escape behavior, where we did not observe significant differences among snails in the immediate environment without predator cues, the immediate environment is likely the first to be integrated by offspring. In absence of immediate predator cues, offspring did not alter their activity according to parental and developmental environments while in the presence of predator cues, activity cannot be measured as all snails exhibited an escape behavior.
In our predator-prey system, we did not expect sex-specific TGP as P. acuta is a simultaneous hermaphrodite with an internal fertilization and does not provide any parental care. The mating partners therefore have the same morphology, behavior, ecology, and gamete dispersal; they just differ in the reproduction function used at the time of mating (female: eggs and mucus; male: sperm and seminal fluid). Hence, they experience the same predation pressures and may have the same phenotypic optima in response to the current predation risk. This suggests that (1) both sexes would exert similar effects on offspring traits and (2) offspring would similarly benefit to process the maternal or paternal cues (Kamel et al., 2010; Bell and Hellmann, 2019). Surprisingly, we found sex-specific TGP on escape behavior in the immediate context of predation risk, meaning that female and male functions have distinct effects on this trait depending on the immediate environment. For activity (only measured without predator cues), no distinct effects of maternal or paternal environments were found. Investigations of such sex-specific TGP in simultaneous hermaphrodites is restricted to plant species. However, in plants, huge differences in gamete and seed dispersals between male and female functions may promote sex-specific TGP (Galloway, 2001; but see Akkerman et al., 2016). In animals, evidence of sex-specific TGP often used species with sex-specific selection pressures or species providing uniparental care (e.g., Shama et al., 2014; Emborski and Mikheyev, 2019; Lehto and Tinghitella, 2020). Our results suggest that sex-specific TGP may be driven by other factors than sex-specific selection pressures and uniparental care.
Firstly, sex-specific TGP in P. acuta may arise from differences in inheritance mechanisms between female and male functions (Akkerman et al., 2016; Guillaume et al., 2016; Bell and Hellmann, 2019). Female function has a narrow relationship with the subsequent generation and can add informative molecules (e.g., hormones, non-coding RNA) or nutrients in eggs during the production of oocytes or during the offspring very early development (after fertilization and before laying). By contrast, male function can only influence offspring via sperm cells and seminal fluid and therefore has a limited number of molecular pathways to transmit information to the subsequent generation (e.g., epigenetic mechanisms). Although we do not know the specific inheritance mechanisms acting in our study, we can suppose that female and male functions transmit environmental cues via different bearers of information that would explain this sex-specific TGP for escape behavior. For example, in yellow monkeyflower (Mimulus guttatus), Akkerman et al. (2016) have shown that demethylation erased the effect of maternal but not paternal environment, suggesting that only maternal information is transmitted via DNA methylation.
Secondly, sex-specific TGP on escape behavior may result from stronger effects of stress on female function than on male function. The single maternal exposure to predator cues induced a decrease in escape behavior (offspring took longer to crawl-out the water) while the single paternal exposure to predator cues did not influence the escape behavior. These different effects between female and male parental functions on offspring behavior may result from differences in reproductive investment. As in numerous organisms, the female function is likely more costly than the male one in P. acuta, although it is difficult to evaluate it in a simultaneous hermaphrodite. Therefore, stressful environments (such as with predation risk) that negatively influence the snail’s state (DeWitt, 1998) are more likely to be passed on by the female function than by the male function. This hypothesis is corroborated by the decrease in escape behavior induced by the maternal exposure. Although in this study we did not test offspring survival in the face of a real predator, our results (Appendix 3) and other studies (e.g., DeWitt et al., 1999; Beaty et al., 2016) have shown that a faster escape behavior of snails exposed to predator-cues in their immediate environment is adaptive. Consequently, the decrease in snail escape behavior induced by maternal exposure may have deleterious fitness effects on offspring in response to a predator. The negative direction of the maternal effect therefore suggests that the maternal effect is a state-based effect rather than an anticipatory one (see also Coslovsky and Richner, 2011; McGhee et al., 2012). Finally, the combination of stressful environments and difference in reproductive investment between sexes may be sufficient to generate a state-based TGP that is sex-specific.
In the immediate predation environment (Figure 2B), the effect of paternal cues depended on whether they were integrated alone or in combination with maternal and developmental cues (see also Galloway, 2001). This results in a complex interaction between paternal, maternal and offspring environments that we can dissect as follows: (1) The single paternal exposure never affected the offspring escape behavior whatever the offspring developmental environment; (2) When both mother and father were exposed, paternal cues were integrated by offspring according to their own developmental environment. In a developmental environment without predator cues (Figure 2Bi), the paternal exposure mitigated the reduction in escape behavior due to the maternal exposure. Consequently, offspring from both exposed parents escaped as fast as offspring from unexposed parents. This mitigation suggests that offspring can process the cues from exposed fathers to buffer the maternal state-based effect, which we can interpret as an information-based TGP of the paternal cues. Similar results have been observed in sticklebacks (Gasterosteus aculeatus) where the negative effect of paternal exposure on offspring survival in the face of a real predator was mitigated by maternal exposure (Hellmann et al., 2019). In this species, as males are the sole providers of paternal care, a paternal exposure to predators leads to negative stress-mediated effects. In a developmental environment with predator cues (Figure 2Bii), the paternal exposure combined with the maternal one did not compensate the negative maternal effect resulting in a slow behavioral response of snails, similar as observed from exposed mother alone. The exposure to predator cues during offspring development might influence the offspring state (DeWitt, 1998) that might be then no longer able to process the paternal cues to mitigate the negative effect of their exposed mothers.
The non-additive patterns we observed here do not fit with the responses already observed in the literature (threshold hypothesis: Stein et al., 2018; Bell and Hellmann, 2019; Lehto and Tinghitella, 2019, 2020; back-up hypothesis: Bell and Hellmann, 2019; Lehto and Tinghitella, 2019; bayesien hypothesis: Stamps and Krishnan, 2014). We showed that the non-additive responses of offspring for escape behavior might result from a hierarchical integration of cues (i.e., information-based) constrained by state-based effects. Immediate and paternal exposures to predator cues might led to information-based effects on the escape behavior of offspring while developmental and maternal effects might have negative state-based effects. Hence, offspring might first integrate the cues from their immediate environment (the most accurate predictor of predation risk) before to integrate then the cues from their fathers (mitigation of the negative maternal effect) if their state is not constrained by their own developmental environment (without predator cues).
The original contributions presented in the study are included in the article/Supplementary Material, further inquiries can be directed to the corresponding author/s.
SP and ÉL designed the project and carried out the experiments. JT carried out the data analysis and drafted the manuscript. All authors strongly revised the manuscript and approved the manuscript for publication.
The authors declare that the research was conducted in the absence of any commercial or financial relationships that could be construed as a potential conflict of interest.
We would like to thank the following students for their great support for data collection: Laurent Bensoussan, Justine Boutry, Rachel Arnaud, Marie Bouilloud, and Anaïs Seve-Minnaert. This work was performed within the framework of the EUR H2O’Lyon (ANR-17-EURE-0018) of Université de Lyon (UdL), within the program “Investissements d’Avenir” operated by the French National Research Agency (ANR).
The Supplementary Material for this article can be found online at: https://www.frontiersin.org/articles/10.3389/fevo.2020.591074/full#supplementary-material
Agrawal, A. A., Laforsch, C., and Tollrian, R. (1999). Transgenerational induction of defences in animals and plants. Nature 401, 60–63. doi: 10.1038/43425
Akkerman, K. C., Sattarin, A., Kelly, J. K., and Scoville, A. G. (2016). Transgenerational plasticity is sex-dependent and persistent in yellow monkeyflower (Mimulus guttatus). Environ. Epigenet. 2:dvw003. doi: 10.1093/eep/dvw003
Alexander, J. E., and Covich, A. P. (1991). Predator avoidance by the freshwater snail Physella virgata in response to the crayfish Procambarus simulans. Oecologia 87, 435–442. doi: 10.1007/BF00634603
Bates, D., Mächler, M., Bolker, B., and Walker, S. (2015). Fitting linear mixed-effects models using lme4. J. Stat. Softw. 67, 1–48. doi: 10.18637/jss.v067.i01
Beaty, L. E., Wormington, J. D., Kensinger, B. J., Bayley, K. N., Goeppner, S. R., Gustafson, K. D., et al. (2016). Shaped by the past, acting in the present: transgenerational plasticity of anti-predatory traits. Oikos 125, 1570–1576. doi: 10.1111/oik.03114
Bell, A. M., and Hellmann, J. (2019). An integrative framework for understanding the mechanisms and multigenerational consequences of transgenerational plasticity. Annu. Rev. Ecol. Evol. Syst. 50, 97–118. doi: 10.1146/annurev-ecolsys-110218-024613
Blumstein, D. T., and Daniel, J. C. (2007). Quantifying Behavior the JWatcher Way. Sunderland, MA: Sinauer Associates.
Bonduriansky, R., Runagall-McNaull, A., and Crean, A. J. (2016). The nutritional geometry of parental effects: maternal and paternal macronutrient consumption and offspring phenotype in a neriid fly. Funct. Ecol. 30, 1675–1686. doi: 10.1111/1365-2435.12643
Burgess, S. C., and Marshall, D. J. (2014). Adaptive parental effects: the importance of estimating environmental predictability and offspring fitness appropriately. Oikos 123, 769–776. doi: 10.1111/oik.01235
Burke, N. W., Nakagawa, S., and Bonduriansky, R. (2020). Sexual conflict mediated by ecological sex differences can generate diverse patterns of transgenerational plasticity. bioRxiv [Preprint]. doi: 10.1101/846287 bioRxiv: 84 6287
Coslovsky, M., and Richner, H. (2011). Predation risk affects offspring growth via maternal effects: maternal effects due to predation risk. Funct. Ecol. 25, 878–888. doi: 10.1111/j.1365-2435.2011.01834.x
Crean, A. J., and Bonduriansky, R. (2014). What is a paternal effect? Trends Ecol. Evol. 29, 554–559. doi: 10.1016/j.tree.2014.07.009
DeWitt, T. J. (1998). Costs and limits of phenotypic plasticity: tests with predator-induced morphology and life history in a freshwater snail. J. Evol. Biol. 11, 465–480. doi: 10.1046/j.1420-9101.1998.11040465.x
DeWitt, T. J., Sih, A., and Hucko, J. A. (1999). Trait compensation and cospecialization in a freshwater snail: size, shape and antipredator behaviour. Anim. Behav. 58, 397–407. doi: 10.1006/anbe.1999.1158
Donelan, S. C., Hellmann, J. K., Bell, A. M., Luttbeg, B., Sheriff, M. J., and Sih, A. (2020). Transgenerational plasticity in human-altered environments. Trends Ecol. Evol. 35, 115–124. doi: 10.1016/j.tree.2019.09.003
Donelson, J. M., Salinas, S., Munday, P. L., and Shama, L. N. S. (2018). Transgenerational plasticity and climate change experiments: where do we go from here? Glob. Change Biol. 24, 13–34. doi: 10.1111/gcb.13903
Ducatez, S., Baguette, M., Stevens, V. M., Legrand, D., and Fréville, H. (2012). Complex interactions between paternal and maternal effects: parental experience and age at reproduction affect fecundity and offspring performance in a butterfly. Evolution 66, 3558–3569. doi: 10.1111/j.1558-5646.2012.01704.x
Emborski, C., and Mikheyev, A. S. (2019). Ancestral diet transgenerationally influences offspring in a parent-of-origin and sex-specific manner. Philos. Trans. R. Soc. B Biol. Sci. 374:20180181. doi: 10.1098/rstb.2018.0181
Galloway, L. F. (2001). The effect of maternal and paternal environments on seed characters in the herbaceous plant Campanula americana (Campanulaceae). Am. J. Bot. 88, 832–840. doi: 10.2307/2657035
Galloway, L. F., and Etterson, J. R. (2007). Transgenerational plasticity is adaptive in the wild. Science 318, 1134–1136. doi: 10.1126/science.1148766
Gilad, T., and Scharf, I. (2019). Separation between maternal and paternal effects on offspring following exposure of adult red flour beetles to two stressors. Ecol. Entomol. 44, 494–501. doi: 10.1111/een.12726
Guillaume, A. S., Monro, K., and Marshall, D. J. (2016). Transgenerational plasticity and environmental stress: do paternal effects act as a conduit or a buffer? Funct. Ecol. 30, 1175–1184. doi: 10.1111/1365-2435.12604
Hellmann, J. K., Bukhari, S. A., Deno, J., and Bell, A. M. (2019). Sex-specific transgenerational plasticity in threespined sticklebacks. bioRxiv [Preprint]. doi: 10.1101/763862 bioRxiv: 763862
Henry, P.-Y., and Jarne, P. (2007). Marking hard-shelled gastropods: tag loss, impact on life-history traits, and perspectives in biology. Invertebr. Biol. 126, 138–153. doi: 10.1111/j.1744-7410.2007.00084.x
Immler, S. (2018). The sperm factor: paternal impact beyond genes. Heredity 121, 239–247. doi: 10.1038/s41437-018-0111-0
Kamel, S. J., Grosberg, R. K., and Marshall, D. J. (2010). Family conflicts in the sea. Trends Ecol. Evol. 25, 442–449. doi: 10.1016/j.tree.2010.05.008
Krawetz, S. A. (2005). Paternal contribution: new insights and future challenges. Nat. Rev. Genet. 6, 633–642. doi: 10.1038/nrg1654
Kuznetsova, A., Brockhoff, P. B., and Christensen, R. H. B. (2017). lmertest package: tests in linear mixed effects models. J. Stat. Softw. 82, 1–26. doi: 10.18637/jss.v082.i13
Lehto, W. R., and Tinghitella, R. M. (2019). Joint maternal and paternal stress increases the cortisol in their daughters’ eggs. Evol. Ecol. Res. 20, 133–144.
Lehto, W. R., and Tinghitella, R. M. (2020). Predator-induced maternal and paternal effects independently alter sexual selection. Evolution 74, 404–418. doi: 10.1111/evo.13906
Leimar, O., and McNamara, J. M. (2015). The evolution of transgenerational integration of information in heterogeneous environments. Am. Nat. 185, E55–E69. doi: 10.1086/679575
Lenth, R. (2019). emmeans: Estimated Marginal Means, aka Least-Squares Means. Available online at: https://CRAN.R-project.org/package=emmeans.
Lima, S. L., and Dill, L. M. (1990). Behavioral decisions made under the risk of predation: a review and prospectus. Can. J. Zool. 68, 619–640. doi: 10.1139/z90-092
Luquet, E., and Tariel, J. (2016). Offspring reaction norms shaped by parental environment: interaction between within- and trans-generational plasticity of inducible defenses. BMC Evol. Biol. 16:209. doi: 10.1186/s12862-016-0795-9
Lydeard, C., Campbell, D., and Golz, M. (2016). Physa acuta Draparnaud, 1805 should be treated as a native of North America. not Europe. Malacologia 59, 347–350. doi: 10.4002/040.059.0213
Magiafoglou, A., and Hoffmann, A. A. (2003). Cross-generation effects due to cold exposure in Drosophila serrata. Funct. Ecol. 17, 664–672. doi: 10.1046/j.1365-2435.2003.00774.x
Marshall, D. J. (2008). Transgenerational plasticity in the sea: context-dependent maternal effects across the life history. Ecology 89, 418–427. doi: 10.1890/07-0449.1
McGhee, K. E., Pintor, L. M., Suhr, E. L., and Bell, A. M. (2012). Maternal exposure to predation risk decreases offspring antipredator behaviour and survival in threespined stickleback. Funct. Ecol. 26, 932–940. doi: 10.1111/j.1365-2435.2012.02008.x
R Development Core Team (2019). R: A Language and Environment for Statistical Computing. Vienna: R Foundation for Statistical Computing.
Shama, L. N. S., Strobel, A., Mark, F. C., and Wegner, K. M. (2014). Transgenerational plasticity in marine sticklebacks: maternal effects mediate impacts of a warming ocean. Funct. Ecol. 28, 1482–1493. doi: 10.1111/1365-2435.12280
Sih, A., and McCarthy, T. M. (2002). Prey responses to pulses of risk and safety: testing the risk allocation hypothesis. Anim. Behav. 63, 437–443. doi: 10.1006/anbe.2001.1921
Stamps, J. A., and Krishnan, V. V. (2014). Combining information from ancestors and personal experiences to predict individual differences in developmental trajectories. Am. Nat. 184, 647–657. doi: 10.1086/678116
Stein, L. R., Bukhari, S. A., and Bell, A. M. (2018). Personal and transgenerational cues are nonadditive at the phenotypic and molecular level. Nat. Ecol. Evol. 2, 1306–1311. doi: 10.1038/s41559-018-0605-4
Tariel, J., Plénet, S., and Luquet, É (2020). Transgenerational plasticity of inducible defences: combined effects of grand-parental, parental and current environments. Ecol. Evol. 10, 2367–2376. doi: 10.1002/ece3.6046
Tsitrone, A., Jarne, P., and David, P. (2003). Delayed selfing and resource reallocations in relation to mate availability in the freshwater snail Physa acuta. Am. Nat. 162, 474–488. doi: 10.1086/376889
Turner, A. M., Fetterolf, S. A., and Bernot, R. J. (1999). Predator identity and consumer behavior: differential effects of fish and crayfish on the habitat use of a freshwater snail. Oecologia 118, 242–247. doi: 10.1007/s004420050724
Valtonen, T. M., Kangassalo, K., Pölkki, M., and Rantala, M. J. (2012). Transgenerational effects of parental larval diet on offspring development time, adult body size and pathogen resistance in Drosophila melanogaster. PLoS One 7:e31611. doi: 10.1371/journal.pone.0031611
Yin, J., Zhou, M., Lin, Z., Li, Q. Q., and Zhang, Y. (2019). Transgenerational effects benefit offspring across diverse environments: a meta-analysis in plants and animals. Ecol. Lett. 22, 1976–1986. doi: 10.1111/ele.13373
Zizzari, Z. V., van Straalen, N. M., and Ellers, J. (2016). Transgenerational effects of nutrition are different for sons and daughters. J. Evol. Biol. 29, 1317–1327. doi: 10.1111/jeb.12872
Zuccolo, L., DeRoo, L. A., Wills, A. K., Davey Smith, G., Suren, P., Roth, C., et al. (2016). Pre-conception and prenatal alcohol exposure from mothers and fathers drinking and head circumference: results from the Norwegian Mother-Child Study (MoBa). Sci. Rep. 6:39535. doi: 10.1038/srep39535
Zuur, A., Ieno, E. N., Walker, N., Saveliev, A. A., and Smith, G. M. (2009). Mixed Effects Models and Extensions in Ecology with R. New York, NY: Springer-Verlag. doi: 10.1007/978-0-387-87458-6
Note: “C” stands for control environment and “P” for predator-cue environment. “Dev” stands for developmental environment. P-values are corrected for multiple testing with Holm method. Bold p-values indicate significant pairwise comparisons (corrected P-value ≤ 0.05).
Most snails escape faster in the immediate predator-cue environment (“P”) than in the immediate control environment (“C”). The “C” developmental environment is shown on the left panel (i) and the “P” developmental environment is shown on the right panel (ii). Text indicates the four combinations of maternal x paternal environments (CxC, PxC, CxP, and PxP). Dots are for snails from non-exposed mothers while squares are for snails from exposed mothers. White shapes are for snails from non-exposed fathers while gray shapes are for snails from exposed fathers. Points are mean ± SE.
Keywords: maternal effect, paternal effect, sex-specific, predator-prey interactions, cue integration, non-additive response, Physa acuta
Citation: Tariel J, Luquet É and Plénet S (2020) Interactions Between Maternal, Paternal, Developmental, and Immediate Environmental Effects on Anti-predator Behavior of the Snail Physa acuta. Front. Ecol. Evol. 08:591074. doi: 10.3389/fevo.2020.591074
Received: 03 August 2020; Accepted: 10 November 2020;
Published: 07 December 2020.
Edited by:
Olivia Roth, GEOMAR Helmholtz Center for Ocean Research Kiel, GermanyReviewed by:
Kyle Gustafson, Arkansas State University, United StatesCopyright © 2020 Tariel, Luquet and Plénet. This is an open-access article distributed under the terms of the Creative Commons Attribution License (CC BY). The use, distribution or reproduction in other forums is permitted, provided the original author(s) and the copyright owner(s) are credited and that the original publication in this journal is cited, in accordance with accepted academic practice. No use, distribution or reproduction is permitted which does not comply with these terms.
*Correspondence: Juliette Tariel, anVsaWV0dGV0YXJpZWxAZ21haWwuY29t; Sandrine Plénet, c2FuZHJpbmUucGxlbmV0QHVuaXYtbHlvbjEuZnI=
Disclaimer: All claims expressed in this article are solely those of the authors and do not necessarily represent those of their affiliated organizations, or those of the publisher, the editors and the reviewers. Any product that may be evaluated in this article or claim that may be made by its manufacturer is not guaranteed or endorsed by the publisher.
Research integrity at Frontiers
Learn more about the work of our research integrity team to safeguard the quality of each article we publish.