- Department of Zoology, University of Oxford, Oxford, United Kingdom
Material-bound vibrations are ubiquitous in the environment and are widely used as an information source by animals, whether they are generated by biotic or abiotic sources. The process of vibration information transfer is subject to a wide range of physical constraints, especially during the vibration transmission phase. This is because vibrations must travel through materials in the environment and body of the animal before reaching embedded mechanosensors. Morphology therefore plays a key and often overlooked role in shaping information flow. Web-building spiders are ideal organisms for studying vibration information transfer due to the level of control they have over morphological traits, both within the web (environment) and body, which can give insights for bioinspired design. Here we investigate the mechanisms governing vibration information transfer, including the relative roles of constraints and control mechanisms. We review the known and theoretical contributions of morphological and behavioral traits to vibration transmission in these spiders, and propose an interdisciplinary framework for considering the effects of these traits from a biomechanical perspective. Whereas morphological traits act as a series of springs, dampers and masses arranged in a specific geometry to influence vibration transmission, behavioral traits influence these morphologies often over small timescales in response to changing conditions. We then explore the relative roles of constraints and control mechanisms in shaping the variation of these traits at various taxonomic levels. This analysis reveals the importance of morphology modification to gain control over vibration transmission to mitigate constraints and essentially promote information transfer. In particular, we hypothesize that morphological computation is used by spiders during vibration information transfer to reduce the amount of processing required by the central nervous system (CNS); a hypothesis that can be tested experimentally in the future. We can take inspiration from how spiders control vibration transmission and apply these insights to bioinspired engineering. In particular, the role of morphological computation for vibration control could open up potential developments for soft robots, which could use multi-scale vibration sensory systems inspired by spiders to quickly and efficiently adapt to changing environments.
Introduction
Material-bound vibrations are an important sensory mode for many animals. These vibrations, which propagate through and along the surfaces of materials known as substrates, are ubiquitous in the environment and the study of the biological use of these vibrations is known as “biotremology” (Hill and Wessel, 2016). They can either be produced intentionally as a signal (e.g., in courtship) for communication, or as an unintentional cue (e.g., through the movement of a prey item) that can be used for information by receivers (Mortimer, 2017). Traditionally these vibrations have been overlooked as a potential source of biologically relevant information, with modern techniques unveiling the importance of this sensory modality. This is especially true for terrestrial arthropods, the context of this review, where material-bound vibration information transfer was likely used long before acoustic information transfer (or hearing) evolved (Cocroft and Rodríguez, 2005; Hill, 2009).
Like other sensory modalities, the biological use of material-bound vibrations for information transfer involves the generation, transmission and sensing (here used to refer to sensory transduction) of vibrations. This review focuses on the role of vibration transmission as an important, but relatively overlooked stage in this process, which is the stage encompassing everything between generating a vibration and transduction within sensory cells. This stage is interesting because although the theory of how vibrations propagate through materials is well developed, its application within this biological context is in its infancy and the role of natural selection in shaping or controlling this process is not well understood. In terrestrial arthropods, vibrations first propagate through the substrate before passing into the arthropod’s body where its leg couples with the substrate. Following coupling, vibrations propagate to mechanosensors that are typically embedded in the leg (Figure 1; Pringle, 1955). Mechanical transmission of vibrations therefore involves both propagation of the vibration within a material in the environment (or substrate) and within the animal’s body. Not covered here, at mechanosensors, mechanical stimuli (such as vibrations) lead to the generation of an action potential in the sensory neurons innervating the sensor, and the wave is transformed into an electrical signal that travels along this neuron (Barth and Pickelmann, 1975). For detail and discussion on the effect of neuronal filtering and sensory transduction on the transformation of vibrational information, we refer readers to an alternative review (Barth, 2019).
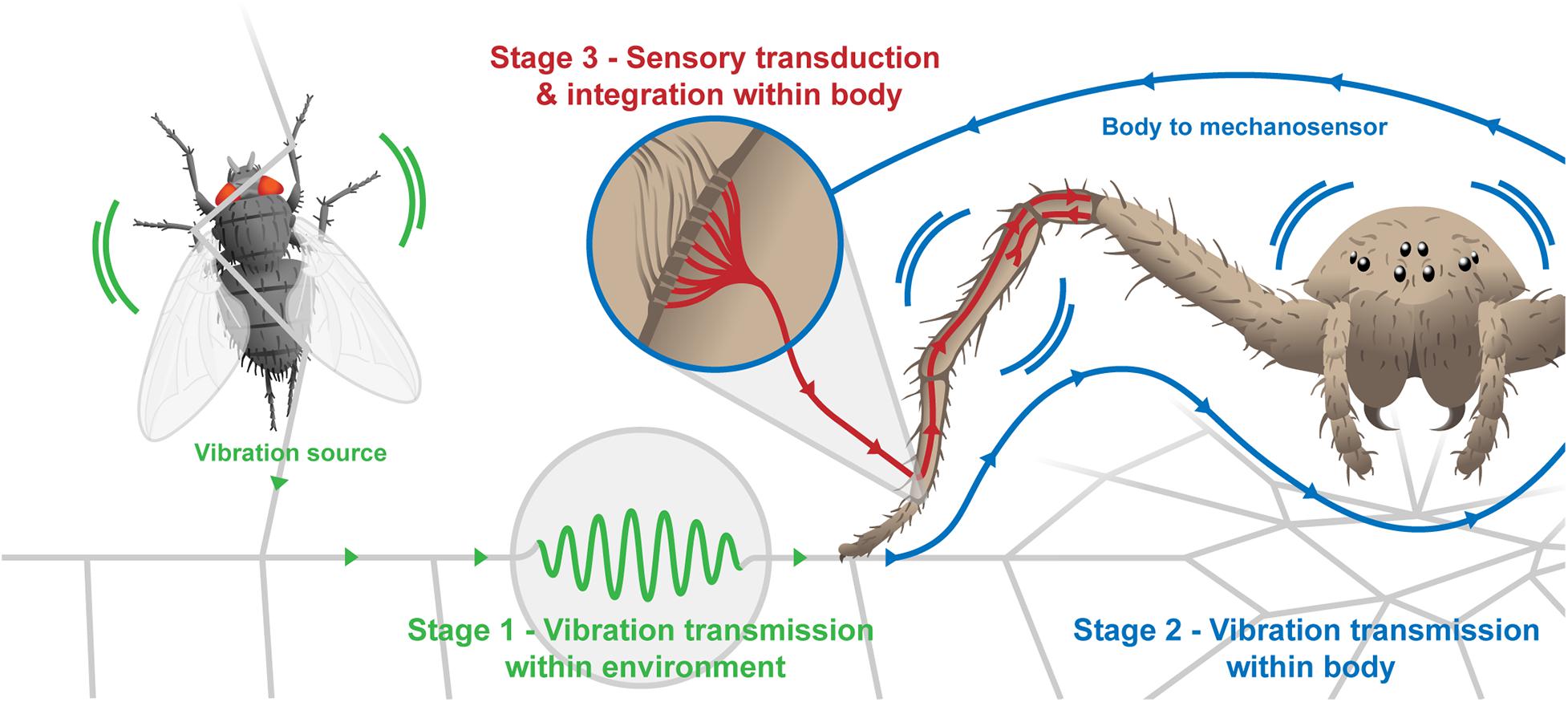
Figure 1. diagrammatic representation of the stages in vibration sensing detailed in Table 1. Stage 1—transmission from source of vibration through environmental substrates (in this case the web) to the body. Stage 2—body transformation of input wave before it reaches the mechanosensor (lyriform organ shown in insert). Stage 3—sensory transduction of vibrational information, which feeds back to determine behavior, in turn influencing Stages 1 and 2.
Vibration transmission is shaped by the interplay between natural selection, evolutionary, and physical constraints, yet the interactions between these processes in this context are not well understood. Both morphological and behavioral traits have been shaped by natural selection to influence vibration transmission (Table 1), which are under evolutionary constraints, e.g., trade-offs and developmental constraints. Each stage of transmission also varies in the degree to which physical constraints are acting. These physical constraints are imposed by their biomechanics and include damping (energy loss) and filtering of vibrational information (Mortimer, 2017). Control mechanisms can be used to mitigate these constraints (see section “Discussion”).
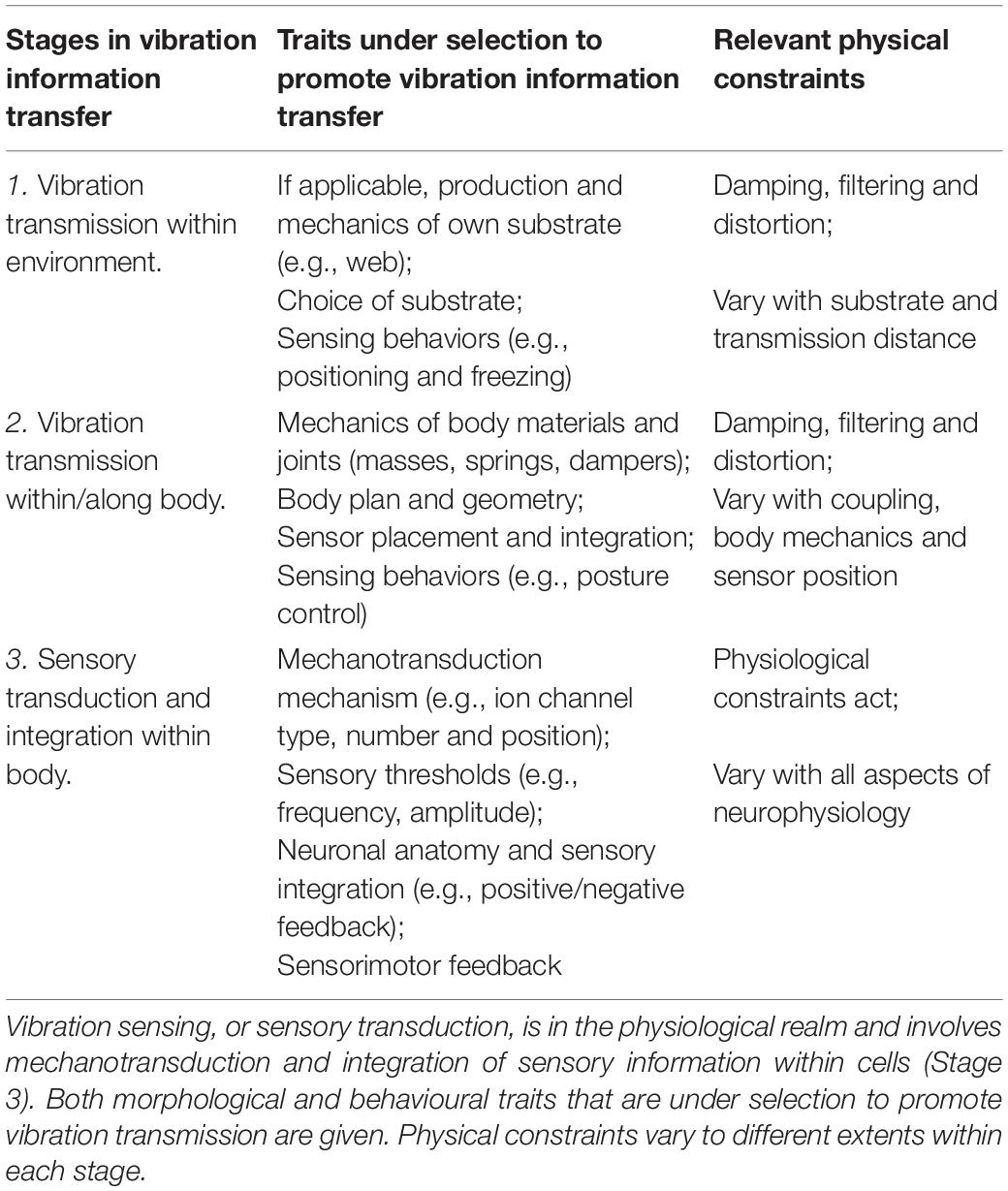
Table 1. The focus of this review is vibration transmission, which is in the physical realm and involves mechanical vibrations propagating through the environment (Stage 1) and body (Stage 2).
In this review, we will use spiders as a case study to probe the mechanisms influencing vibration transmission during the process of vibration information transfer, building on previous reviews that discuss physical constraints acting during vibration transmission (Mortimer, 2017, 2019; Barth, 2019). This is the dominant sensory modality for many spiders (Barth, 2002a), and is used for communication with conspecifics during courtship (Schüch and Barth, 1985, 1990; Elias et al., 2003, 2005), as well as sensing prey and predators (Klärner and Barth, 1982; Masters, 1984b; Landolfa and Barth, 1996). As such, mechanisms to influence vibration transmission apply to varied biological contexts with spiders, including prey capture, and conspecific communication. The mechanosensors involved in spider vibration sensing are slit sensilla—some of which are grouped into distinct structures known as lyriform organs (Barth, 2002a). In particular, the metatarsal lyriform organ has been intensively studied for its role in vibration sensing (Barth, 2002a). In addition to sensing externally generated vibrations, spiders use slit sensilla to sense internally generated vibrations, known as proprioception (Seyfarth, 1978).
Morphological and behavioral traits in spiders are extremely variable and the mechanisms causing this variation and the implications for information transfer, whether natural or engineered, have been largely unexplored. Web-building spiders are an excellent model species to study this due to the impressive control mechanisms that can influence vibration transmission both within the environment and body, which in theory allow them to mitigate physical constraints. For spiders, morphological traits include the structures and materials of both the body of the spider and the extended phenotype of the web, and behavioral traits act to influence morphology during vibration transmission (Table 1). This is exemplified by orb-weaving spiders that generate aerial orb webs that act as a snare for prey capture and a vibration transmission platform (Mortimer, 2019). Through modifying the extended phenotype of the web, these spiders are thought to influence vibration transmission outside the body (Mortimer et al., 2016). Most literature on orb weaver vibration transmission is on prey capture, although the web is also used to transmit vibrations during courtship (Wignall and Heberstein, 2013).
An aim of this review is to combine perspectives from across biology and the physical sciences to explore the mechanisms governing vibration transmission. One concept that we will come back to in the Discussion is the idea of morphological computation, which is a term more typically applied to artificial systems (such as robots). Morphological computation is where the body, rather than central nervous system (CNS), is used to control actions or information, thus reducing the total level of processing required by the CNS. Recently, there has been increasing recognition of its role in biological systems—for example, animals rely heavily on the material properties of their leg muscles and tendons to walk/run, rather than these leg movements being closely controlled by the CNS (Mo et al., 2020). In sensory systems, morphological computation could be used to pre-process information before sensory transduction occurs, which in our context would be during the vibration transmission stage. This is thought to occur in the insect eye, where the arrangement of light-sensitive cells allows insects to accurately gauge distance without additional processing in the CNS (Franceschini et al., 1992). During vibration information transfer in terrestrial arthropods, since mechanosensors are embedded into the cuticle or leg (Yack, 2004), vibratory waves must travel through the body of the animal before they are sensed. This means vibration information transfer is more heavily influenced by morphology than other senses (whether acoustic vibration, olfaction or visual), and morphological computation is likely to play a role in this context.
Using orb weaving spiders as a case study, this review will overview the known and theoretical contributions of morphological and behavioral traits to the biomechanics of vibration transmission (sections “Influence of Morphological Traits” and “Influence of Behavioral Traits,” respectively). We deconstruct the effect that these traits have on three key parameters that can be used to model the system—mass/density, springs/dampers (energy storage/loss), and geometry. We explore the interactions and trade-offs between these three parameters, morphological and behavioral traits in the Discussion. Using this approach, we show that orb-weaving spiders possess a high level of control over morphological and behavioral traits during vibration transmission over multiple timescales. We follow with a discussion of how variation in these traits (within an individual, between individuals of the same species, and between species) can be explained by the action of control mechanisms and constraints (Discussion). We suggest that within a species, morphology is controlled flexibly via behavior, which acts to mitigate against various constraints in variable contexts. We conclude that morphology likely plays a key role in transforming information before the vibrational wave reaches a mechanosensor, and hypothesize a role for morphological computation (section “Discussion”). The review provides insights into the relative roles of natural selection, evolutionary and physical constraints in shaping vibration sensing in terrestrial arthropods, but also reveals the mechanisms that can be applied to bioinspired design of devices that use material-bound vibrations for information.
Influence of Morphological Traits
From a biomechanical perspective, morphological traits can be seen as systems with a series of masses, springs, and dampers arranged in a specific geometry that influences its motion over time, whether in the environment (here the web) or within/through the body (here the spider’s body). These three parameters are not mutually exclusive and they interact with each other to influence vibration propagation. Yet thinking of vibrating systems, including animals, as these constituent parameters provides a framework to help us to understand the underlying biomechanics governing its motion and compare between biological systems that vary to different degrees to gain insights into its evolution. Within this section (“Influence of Morphological Traits”), each component will be discussed separately, with the aim that the importance of each component to vibration transmission can be outlined.
Mass is distributed both within the substrate and within the body and is particularly important due to physical constraints acting to dampen (or dissipate energy), filter, and distort vibrational information (i.e., influence the relationship between frequency components). Density is a measure of distributed mass, so is discussed within the section on Masses (section “Masses”). Whereas springs govern energy storage in the system when the springs are compressed or stretched, dampers govern the energy lost from the system, usually due to friction. In our orb-weaver spider context, springs/dampers are the silk threads, the cuticle that forms the exoskeleton of the spider and the spider’s joints. Finally, these parameters come together into a specific geometrical arrangement (web shape, body plan, and mechanosensor structure) to govern vibration transmission and shape the information that can be extracted by the animal due to sensor placement. Here we discuss the known and theoretical contributions of masses, springs, dampers, and geometry to vibration transmission, using this interdisciplinary framework in the context of orb-weaving spiders, where the behaviors that influence these factors are reviewed in section “Influence of Behavioral Traits.”
Masses
Vibration transmission through a material is influenced by mass distribution, including changes in density of a material through which a wave propagates. In our spider context, we will focus on masses in the web, as the spider’s engineered substrate, and in the body, through which vibrations need to propagate before reaching embedded mechanosensors (section “Web and Body Transmission”). Mass and density (in combination with material properties and geometry) govern the speed of vibration transmission, how it loses energy over distance and time, and how the wave is filtered (section “Mechanical Impedance and Resonance”) (Mortimer, 2017). Masses also interact with the other biomechanical parameters of springs and dampers (section “Springs and Dampers”), according to their geometry (section “Geometry”).
Web and Body Transmission
The influence that masses have on vibration transmission differs markedly between the web and the body. The mass of the silk threads comprising the web is negligible compared with the spider’s body and prey items in the web—therefore, the dynamics of vibration transmission are largely dependent on the masses suspended by the silk threads, rather than the web itself. All spider body plans consist of a fused head and body segment (cephalothorax) joined to an abdomen segment via the waist-like pedicel, and eight legs, all of which vary in their mass and geometry (Foelix, 1979). In particular, these masses modulate how vibrations are transmitted through the legs at different joint angles, where higher masses lead to greater forces exerted around the leg joints (via gravity), influencing their springs and dampers (see section “Springs and Dampers”).
The mass distribution of a spider and its web is stochastic, with the mass of objects caught in the web (such as prey and debris), as well as the mass of the spider’s body, varying over time. The mass of the spider’s cephalothorax will fluctuate due to factors such as starvation and dehydration, which will reduce the volume of internal fluid (haemolymph) in the abdomen, as well as the production of eggs by female spiders (Foelix, 1979). Similarly, the amount of silk protein stored in the silk glands will deplete when the spider spins a new web and will then gradually refill (Vollrath, 1999). This is variation that spiders have some control over, but largely cannot avoid, and thus mass fluctuation imposes a physical constraint. Mhatre et al. (2018) simulated the vibrational response of a female black widow spider to vibration, which showed that abdomen mass could vary from 20% to 150% of its in vivo value without a measurable effect on the vibrational response to stimuli between 5 and 200 Hz. Spiders likely possess mechanisms that enable them to account for wave transformation due to their own mass fluctuation, which may be “known” by the spider—this is a topic requiring further research.
Mechanical Impedance and Resonance
As a vibratory wave propagates through a system, the masses it encounters will resist changes in motion that will act to transform the vibratory wave. This tendency for a mass to resist changes in velocity as it is oscillated is known as mechanical impedance, which leads to energy loss and/or reflection of vibrations as a wave passes from a material of one density to another (e.g., from silk to cuticle) (Main, 1993). From a biological perspective, it may therefore be desirable to minimize changes in density and material properties (such as stiffness) throughout transmission, as energy loss will lead to reduction in signal amplitude and therefore make vibrations more difficult to detect. This is especially important in two contexts where waves are transmitted between disparate materials: within a heterogenous substrate (or multi-material substrate) (Elias and Mason, 2014); and also at the coupling points between the substrate and the body.
Starting with the former, damping in heterogenous materials may be reduced through morphological adaptations such as impedance matching (O’Connell-Rodwell, 2007), where materials with similar properties are used to minimize changes in mechanical impedance. The degree of sclerotization (cross-linking) in a spider’s exoskeleton varies across different parts of the body and legs (Blickhan and Barth, 1985), therefore altering the material properties through which vibrational waves propagate. Gradients in material properties of the cuticle are often found [e.g., elastic modulus varying from 8.3 (±1.1) to 2.8 (±1.3) GPa across the cuticular pad] (Young et al., 2014; Erko et al., 2015), which would reduce mechanical impedance changes and so reduce damping and filtering of the vibration. Impedance matching mechanisms via gradients in material properties in terrestrial arthropods require further research.
Moving on to the effect of coupling, the energy loss caused by the coupling between silk and the spider claw (tarsus) is expected to differ between wave types, which is the type of vibrational wave propagating through the material. In the spider web, wave types vary in the direction of oscillation, where longitudinal waves oscillate within the axis of the fiber and transverse waves oscillate perpendicular to the fiber axis. Whilst only longitudinal wave transmission to the tarsus has been measured, the fact that transmission of longitudinal waves through the web shows less damping compared transverse (c. −2 dB on the same silk thread compared with c. −16 at 20 Hz) (Masters and Markl, 1981; Masters, 1984a), means that we can estimate the results for transmission to the tarsus. These differences are expected to become more pronounced above 100 Hz, after which transverse wave damping increases (down to c. −50 dB on the same silk thread) (Masters, 1984a; Landolfa and Barth, 1996). Since longitudinal vibrations are particularly important for initiating predatory behavior in orb weavers (Klärner and Barth, 1982), these observations support the idea that coupling functions to preserve longitudinal wave transmission between the silk and tarsus.
Changes in mechanical impedance also affect vibration transmission when objects sit in the web, such as the spider, prey or debris. Evidence from modeling suggests that the presence of the spider itself at the web centre (hub) leads to significant damping compared with empty webs—c. −16/−36 dB for transverse/longitudinal waves, respectively (Mortimer et al., 2018). Often the presence of these objects cannot be avoided and so present a constraint on vibration transmission. However, some spiders may be able to use the mechanical impedance of their body mass functionally in vibration information transfer. This is because a mass at the hub alters both the speeds and amplitudes of vibrations in the web (Mortimer et al., 2018), which provide information on the location of a vibration source (Mortimer et al., 2019). Mechanical impedance of prey items may also be used by orb weavers as a prey localization cue (Mortimer et al., 2019).
Vibrations often contain multiple frequencies at once. Resonance is where the frequency of an input vibration matches the natural frequency of the system through which it travels, resulting in these frequencies being amplified. The natural frequency of the system is governed by its masses and geometry (Balachandran and Magrab, 2008)—in this case it includes objects in the web and the silk threads comprising the web. At low frequencies (<30 Hz), in theory resonance may occur in the body of the spider itself, or other large objects (such as prey bundles) in the web (Frohlich and Buskirk, 1982). Frequencies in this range contain noise from environmental factors (such as wind) (Masters, 1984b; Wu and Elias, 2014), so resonance of objects in the web is therefore unlikely to be used functionally by the spider. However, spiders may be able to use resonance of silk threads [predicted to be in the hundreds to thousands of Hertz range (Frohlich and Buskirk, 1982)] to locate small objects, by “plucking” silk threads and detecting vibrational cues specific to the thread on which the object sits (Klärner and Barth, 1982). A hypothesized mechanism for this object localization method is the use of wave reflection off the object due to mechanical impedance changes that result in high frequency vibrations on these specific threads (Mortimer, 2019).
Springs and Dampers
The springs and dampers in a vibrating system governs the energy stored and lost during vibration transmission. This is determined by the material and structural properties of the material through which a vibrational wave travels. Most biological tissues contain biopolymers, meaning that they behave as viscoelastic materials (Vincent, 1990). Many biological viscoelastic materials behave like springs at low deformations, meaning they have a linear relationship between force and displacement up to a certain displacement. In this range, they can store and return energy like a spring. Outside this deformation range, they no longer behave as springs, but the material deforms to dissipate energy from the system, which is viscous behavior. This energy dissipation, or damping, depends on the time that the material has to deform (e.g., it can extend more over longer timescales) and the temperature (e.g., it can extend more at higher temperatures), as both influence the energy introduced to the biopolymer, which alters its structure beyond a transition point (the glass transition temperature of the biopolymer) (Guan et al., 2012). This makes energy dissipation time-, frequency-, and temperature-dependent. Viscoelastic materials therefore act as both springs and dampers, where this behavior changes with both deformation range and frequency (Vincent, 1990).
In context, the most important viscoelastic materials for spiders are the silk threads that make up the web, and the spider’s body tissues—especially the cuticle of the exoskeleton, in which the slit sensilla vibration sensors are embedded (Barth, 2019). The springs/dampers within the materials interact with mass to influence vibration transmission: the stiffness and density of these materials are linked to wavespeed and damping, which in turn are involved in frequency filtering and hence determine the shape of the vibrational wave that arrives at the sensor (Mortimer, 2017). These materials also make up structures such as joints that have their own springs and dampers as they move. The properties of the materials and structures therefore have a profound effect on the transmission of vibrational waves.
Vibration Transmission Within Web Environment
The spring-like behavior of silk at low deformations governs the transmission of vibrations through the web. A key property of a spring is its stiffness, which is the gradient of the relationship between force and extension, which governs the tension or force on the silk when a displacement is applied (Vollrath, 2000). Spider dragline silk is unique in the range of stiffness available, due to an unusual property called supercontraction, where high humidity [>70% relative humidity (RH)] dramatically alters the silk structure and reduces its stiffness (Liu et al., 2008). Here, we will briefly review the effects of silk tension, stiffness, and supercontraction on vibration transmission in webs, whilst the behaviors that are able to control these features are reviewed later (section “Influence of Behavior”).
Silk tension both controls web geometry and is determined by it. This feedback system is vital for maintaining the mechanical integrity of the web, with mooring threads that attach to the environment being under the greatest tension (Wirth and Barth, 1992). Silk tension is particularly important for the transmission of transverse waves as it directly affects wavespeed and amplitude when interacting with mass per unit length and geometry (Mortimer et al., 2014, 2016). Conversely, longitudinal wave transmission is influenced by stiffness (Frohlich and Buskirk, 1982), which is of particular importance for predatory behavior due to its low damping in the web (almost no damping, > −2 dB, from prey-capture region to hub along same silk thread in the biologically relevant range of 1–10,000 Hz) (Masters and Markl, 1981). Stiffness is influenced by supercontraction, which decreases the order of the protein structure and reduces stiffness (Liu et al., 2008; Guan et al., 2011). The degree of supercontraction is altered by the proportion of proline amino acid in the silk proteins—silks from different species of spider contain varying amounts of proline (ranging from as little as 0.6 to 14.3 mole%), and thus experience different degrees of supercontraction (Liu et al., 2008).
Vibration Transmission Within/Along Body
As slit sensilla are embedded sensors, the material properties of the cuticle directly influence vibrations before they reach the sensors, and also govern how the slit sensilla deform in response to vibrational waves. Since the cuticle behaves like a spring at low deformations, the slit sensilla are able to deform when extended and then return to their original state (Hössl et al., 2006). The exoskeleton covering the legs, where the largest concentrations of slit sensilla are found, is composed of stiff exocuticle. The opisthosoma (posterior part of the abdomen), on the other hand, is covered by less stiff mesocuticle, and therefore the slit sensilla found here will undergo greater deformation in response to a vibrational wave compared with those surrounded by a stiffer material (Barth, 2002a).
A cuticular pad situated distally (toward the claw) to the metatarsal lyriform organ is involved in transmitting and filtering vibrations as they propagate from the tarsus to the metatarsus leg segments (McConney et al., 2007; Young et al., 2014; Erko et al., 2015). The viscoelasticity of the pad allows it to act as a high-pass filter, meaning it filters out low-frequency background noise (e.g., vibrations of c. < 10 Hz). At low frequencies, the pad behaves in a viscous manner, producing maximum damping properties. This means that large tarsal deflections (angular displacements c. 10° and above) are required to activate the slit sensilla, which also acts to prevent damage to the organ at high deflections (Young et al., 2014; Erko et al., 2015). At higher frequencies (>10 Hz), the pad transitions to a spring-like state, becoming stiffer and transmitting vibrations much more effectively. These properties are temperature-dependent, with the pad damping vibrations at higher temperatures (>21°C at 10 Hz) (McConney et al., 2007; Young et al., 2014).
In addition to the cuticle, the joints between leg segments themselves act as springs and dampers. For some joint motions (loading along the leg axis), joints behave as springs (Blickhan, 1986). For other joint motions (dorsoventral and lateral deflections), increased joint deflection stretches the articular membrane, which is thought to increase the alignment within the material, increasing stiffness (Blickhan, 1986). This results in energy being lost as the joint is loaded and unloaded, so the joint acts as a damper under certain conditions (Blickhan, 1986). As the lyriform organs are typically embedded near the leg joints, their mechanical sensitivity (here taken as the ratio of input force to cuticle deformation, i.e., the strain) is influenced by the strains and changes in stiffness in the cuticle that is generated by joint movement. When the legs are loaded axially, the deformation in the cuticle is negligible around 170° (between c. −0.1 and 0.1 με/mN), but the deformation, and presumably lyriform organ sensitivity, increases above/below this joint angle as the cuticle is put under tension/compression, respectively (Blickhan and Barth, 1985). There are some exceptions to this (e.g., VS-5 lyriform organ), where organs are compressed (and therefore stimulated) during leg extension (Blickhan and Barth, 1985).
Geometry
The vibrational motion of a system made of masses, springs, and dampers is determined by its overall geometrical structure. Diversity in web shapes and body plans influences vibration transmission through these materials. Variation in the geometry of morphological traits between species can be explained by the evolution of divergent hunting strategies, shaped in part by various constraints. In addition, mechanosensor geometry and placement varies within an individual, both spatially over different areas of the body, and temporally due to development and regeneration.
Vibration Transmission Within Web and Body
Web geometry is highly variable both within and between individuals. Individual variation in web geometry usually arises due to external and internal factors that change over time. For example, orb webs built at 20% relative humidity have c. 80% the capture area of webs built at 70% relative humidity (Vollrath et al., 1997). Starvation has also been shown to affect the variability of web geometry—when sufficiently fed, spiders use web modifications (such as increased capture area) to reduce variability in web geometry, which is thought to produce a more optimal phenotype for prey capture effectiveness (Vollrath and Samu, 1997). Whilst this variation has been well quantified, the trade-offs between mechanical functions vs. vibration transmission when geometry is modified have largely not been investigated, and future experiments should aim to quantify both mechanical and vibration transmission properties as web geometry changes and their implications for prey capture.
Web geometry due to the spider’s resting location on the orb web alters vibration transmission, where some individuals sit in a retreat at the edge of their web rather than being positioned at the hub (center). Some spider species are more likely to employ this strategy than others, such as Zygiella x-notata, which senses web vibrations using a signal thread that joins the retreat to the hub, passing through a sector devoid of crossing threads (Klärner and Barth, 1982; Mortimer et al., 2018). Thus, they initially forgo the ability to localize prey on the web in favor of being less conspicuous at the retreat to avoid predation (Pasquet et al., 2007). After sensing prey struggling in the web, Z. x-notata moves along the signal thread to the hub, where it is able to orient toward the source vibration, making prey capture take longer (Klärner and Barth, 1982). The change in resting position does not impose any biologically relevant time cost due to vibration propagation through the web, but signals arriving at the retreat will be more damped than those at the hub (−1.37 ± 0.91 dB on the signal thread relative to the hub) (Mortimer et al., 2018).
There is high interspecific variation in web geometry (Foelix, 1979), and different web types are characteristic of separate taxonomic groups—these include orb webs, funnel webs, tubular webs, sheet webs, and tangle webs. Whilst most studies have focused on orb webs as their regular structure makes them easier to model, other web geometries are a promising area for future research, as each has their own unique transmission properties. For example, whilst the transmission efficiencies of transverse and longitudinal waves through orb webs differ greatly (especially with masses in the web as described previously) (Masters, 1984a; Landolfa and Barth, 1996; Mortimer et al., 2016), in other web geometries, such as sheet and tangle webs, they may propagate equally well (Naftilan, 1999; Vibert et al., 2016). In these web types, there are a large number of points where threads come into contact with each other, and unlike in orb webs, the angles between threads tend to be quite variable (Vibert et al., 2016). This irregular web architecture means that there is a lot of variation in what frequencies are transmitted best within and between webs, suggesting that frequency may not be the most important factor for discriminating between vibratory sources for these spiders. Modeling suggests that resonance of the silk threads may be useful in enhancing the amplitude of prey generated vibrations in funnel/sheet webs, with amplification occurring within some of the frequency range that prey struggles at (2–200 Hz) (Naftilan, 1999).
The influence that the overall body plan of the spider has on vibration transmission is a largely unexplored area, yet is likely to prove vital in our understanding of how evolution and constraints have shaped vibration information transfer. A useful approach will be to model diverse spider body plans as mass/spring/dampers systems to investigate the physical relationships underlying vibration transmission. From a mass/spring/damper perspective, overall body plan matters because it determines the dynamics of the vibrating system (including torques and resonances). We can consider two extremes of spider morphology and compare the body plan of the Pholcidae (cellar spiders) with the Theraphosidae (tarantulas) (Figure 2; Foelix, 1979). In Pholcidae, a pendulous abdomen is connected to a small cephalothorax, which is in turn supported by very long, thin legs. In Theraphosidae, the legs are much shorter and thicker, comprising a larger proportion of the spider’s overall mass. These families have evolved disparate hunting strategies, with the Pholcidae employing a web to trap prey, and Theraphosidae being ambush predators. Therefore their difference in morphology is also correlated with a difference in substrate through which they sense vibrations (ground/tree vs. the web), and a difference in the vibrations of prey. The leg and body shapes can be assumed to be adaptive for their respective hunting strategies, shaped by evolutionary and physical constraints including trade-offs; for example the strong, muscular legs in Theraphosidae have to be able to overpower prey as well as sense vibrations. Whether the shapes of the legs and body are adaptive in vibration transmission remains to be seen, but in theory these differences will influence vibration transmission.
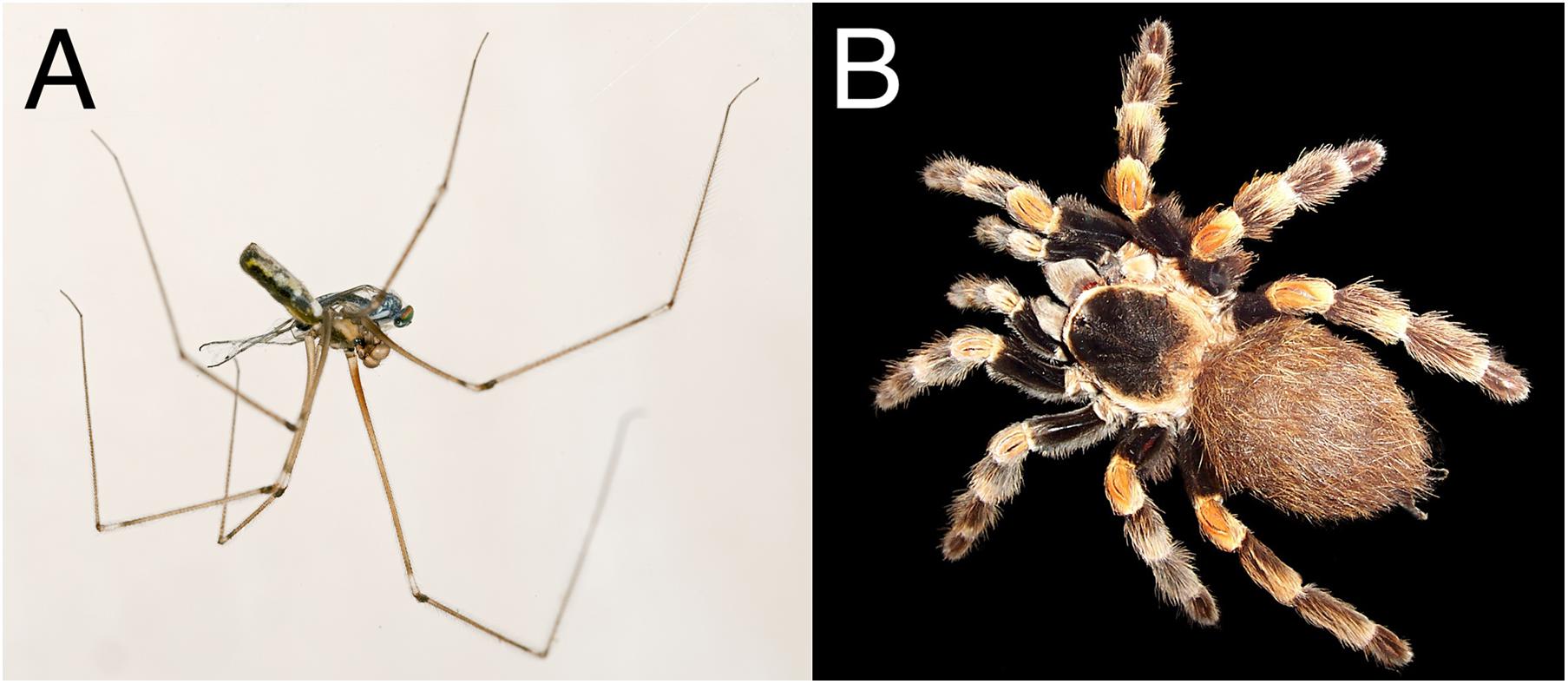
Figure 2. Comparison of the body geometries of Pholcus phalangioides (A) and Brachypelma smithi (B). Open source images: A—Filename “Pholcus phalangiodes MHNT male. jpg,” courtesy of Didier Descouens, License CC BY-SA 4.0, B—Filename “Brachypelma edit. jpg,” courtesy of user “Fir0002,” License CC BY-SA 3.0.
Mechanosensor Geometry and Placement
Sensor geometry and placement is key to how mechanosensors respond to deformations in the exoskeleton, from the position of individual slits, through to the geometry and position of the sensors on the body. The spatial and temporal variation in slit sensilla geometry across individual spiders has been extensively studied in a single species—Cupiennius salei (Barth, 2019). Understanding interspecific variation in sensor geometry is currently limited, but further research would reveal how spiders in different contexts solve the problems of sensor placement to promote vibrational information transfer. More research is needed on the distributions of slit sensilla across all parts of the spiders’ bodies on a range of spider species other than the well-studied Cupiennius salei.
We can first consider the shape and structure of individual slits. Variation in slit length (5–200 μm) affects the sensitivity of the organ, as a larger slit will deform more than a smaller slit. This induces a greater deflection in the covering membrane that spans the slit and which sensory cells are attached to, which in turn makes vibrations easier to sense and decode (Barth, 1972; Barth and Pickelmann, 1975). The base curvature of the covering membrane also varies, with some forming a deeper trough within the slit than others—again, this affects sensitivity as greater curvature will mean that the membrane deflects more as the slit deforms (Barth and Bohnenberger, 1978). This is linked to the position of the neurons that innervate the membrane, with most dendrites connecting near the middle, where the greatest deformation occurs (Molina et al., 2009).
The arrangement of slits within the lyriform organs also has implications for vibration sensing. Slit arrangements and overall organ shape differ between lyriform organs located on different areas of the leg, which give each organ subtly different sensing capabilities (Figure 3A; Barth et al., 1984; Hössl et al., 2007, 2009). Heart-shaped arrangements found on the trochanter (leg segment), for example, are particularly sensitive to load direction (Barth et al., 1984), whilst slightly asymmetrical triangular arrangements can be used for measuring different response ranges (Hössl et al., 2009). The orientation of these slits relative to the leg axis is also important for vibration sensing, as the greatest deformation will be induced when the long axis is perpendicular to the direction of the input force. Because of this, most slit sensilla, including those in the lyriform organs, are oriented parallel to the long axis of the legs—which is the direction of vibrational wave transmission. However, not all of them are, and the reasons for this variation are currently unclear. This could be investigated by comparing slit orientation in different species and correlating this with morphological/life strategy differences.
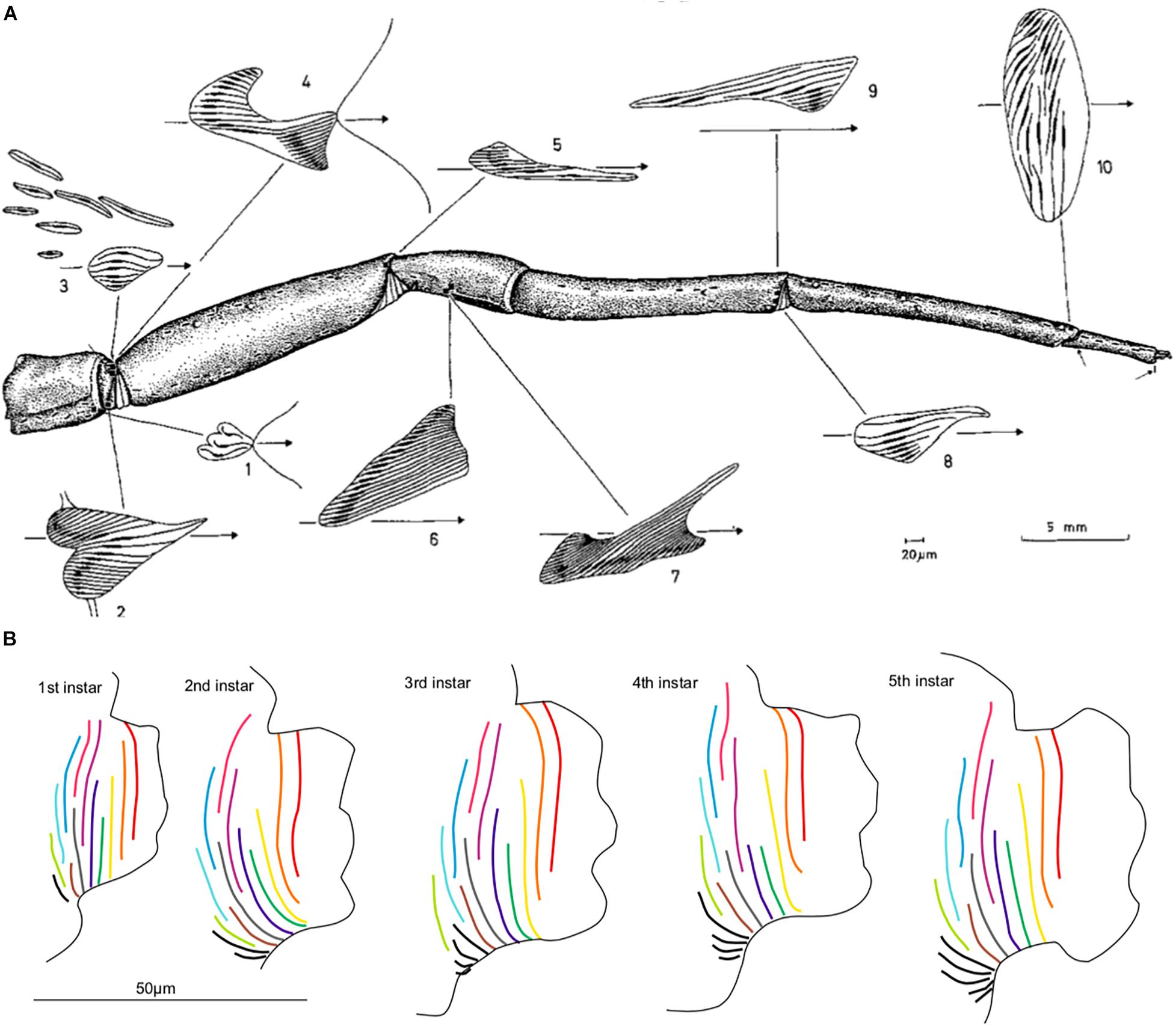
Figure 3. A—spatial variation in lyriform organ geometry on the posterior of the first leg of Cupiennius salei, including heart-shaped, triangular, oval and irregular arrangements of slits. B—temporal variation in the HS10 lyriform organ on the first leg over the first 5 juvenile instars of Latrodectus hesperus, demonstrating changes in shape as well as increase in overall number of slits through growth. (A) Reprinted by permission from Springer Nature Copyright Clearance Centre: Springer Nature, Zoomorphologie (Slit sense-organs of arachnids - Comparative-study of their topography on walking legs (Chelicerata. Arachnida), Barth, F. G. and Stagl, J.) [Copyright], (1976). (B) Reprinted by permission from Springer Nature Copyright Clearance Centre: Springer Nature, Zoomorphology (Developmental morphology of a lyriform organ in the Western black widow (Latrodectus hesperus), Morley, E. L., et al.) [Copyright], (2016).
Lyriform organ geometry changes over development, where the lyriform organs and metatarsal pads grow at different rates (Figure 3B; Morley et al., 2016). The pad shows isometric scaling (proportional) relative to metatarsal length, as the pad grows at the same rate as the rest of the leg. However, the lyriform organ starts out relatively large and grows slowly using hypoallometric scaling in order to preserve function (Morley et al., 2016). This results in these two components being mismatched during early molt stages due to developmental constraints and trade-offs, with the small pad meaning that juveniles are less able to filter low-frequency environmental noise (<40 Hz) (Morley et al., 2016). Whether the effect of this mismatch is compensated for by the nervous system, handled by morphology or mitigated through behavior, remains an open question.
Both slit length and lyriform organ geometry are also affected by limb regeneration. Some spiders lose limbs and regrow them during successive molts. However, it can take several molts before the limb regains normal morphology, where the regenerated leg from the first molt may be only half the length of the others, yet appears to function normally (Vollrath, 1995). Crucially, the shape of the lyriform organs on regenerated legs differs from normal legs—the triangular organ, for example, has a lower apex whilst legs are regenerating (Speck-Hergenröder and Barth, 1988). Further research is needed to determine whether differences in the shape of the lyriform organ are adaptive, whether due to regeneration, development, and interspecific differences. Using modeling, evidence could be provided that this variation is adaptive by demonstrating that changes in lyriform organ morphology produce a compensatory transformation in the input wave.
Finally, the location of the slit sensilla on the body determines how they respond to forces, and may be linked to their function (Barth and Stagl, 1976). Slit sensilla are distributed throughout the spider’s body and are extremely numerous, with around 3,500 in Cupiennius salei (Barth, 2002b). In general, the highest concentrations of slits (both single slits, loose groups, and lyriform organs) are found in the proximal segments of the legs (closer to body), which is correlated with increased musculature in this region. Lyriform organs are typically located at or near the leg joints (Barth, 2019), where the forces through the cuticle are likely to be highest. Single slit sensilla, on the other hand, are often found well away from the joints in the middle of the leg segments. They are, however, frequently located near where the muscle attaches to the cuticle, which supports the idea that these organs function in proprioception, through the sensing of internally generated strains from muscular contraction (Seyfarth et al., 1985). Other organs are located toward the distal ends of the legs, such as the metatarsal lyriform organ and the tarsal slits (Barth, 2002a). These organs respond to external vibrations (Barth and Bohnenberger, 1978; Speck and Barth, 1982), which makes sense given they are located closer to the coupling point with the substrate. However, vibrations will continue to propagate up through the leg and body after reaching the metatarsal lyriform organ and hence more proximally located organs are likely to be involved as well, which is supported by evidence that spiders still orient toward vibration sources (albeit with reduced accuracy) when the metatarsal lyriform organs are ablated (Seyfarth and Barth, 1972; Seyfarth et al., 1982; Bleckmann and Barth, 1984).
Influence of Behavioral Traits
Behavior is a key mechanism influencing vibration transmission as it acts to influence morphology in different biological contexts. This includes altering mass distribution, the parameters of springs and dampers through stiffness and tension, and changing overall geometry—both in the extended phenotype of the web and in the spider’s body.
Vibration Transmission Within Web
The extended phenotype of the spider web is an excellent example of using behavior to control an individual’s vibratory environment. Different elements of the web can be modified to alter different aspects of vibration transmission, whilst maintaining other functions such as prey capture ability. We have already discussed how masses, springs, dampers, and geometry within the web may influence vibration transmission, and here we discuss the degree of behavioral control spiders have over these factors, and link these with the biological contexts that drive spiders to make these changes.
Starting with the effect of masses, some spiders will actively remove foreign objects from their web (Cloudsley-Thompson, 1995; Pasquet et al., 2007). Whilst these tidying behaviors may be driven by a number of different selection pressures [e.g., avoiding damage to the web, hygienic reasons (Straus and Avilés, 2018)], they will also affect vibration transmission by modifying mechanical impedance on the web. Orb weavers have been shown to locate these objects using a “plucking” behavior (Klärner and Barth, 1982). The presence of some masses in the web (such as stored prey items) is, however, unavoidable, despite their impact on vibration transmission. Behaviors such as caching prey (storing food e.g., in Nephila) are used to cope with a variable food supply (de Crespigny et al., 2001) and also attract more insects into the web (Henaut et al., 2010). However, caching prey only within a small area may mitigate the impact of stored food on vibration transmission as their effect on vibration transmission would be location specific.
Orb weaving spiders have behavioral mechanisms to control the springs and dampers of the web, via changing tension, stiffness, and supercontraction. Spiders may adjust silk tension in order to deliberately modulate the transmission of vibrations through the web in response to a change in conditions (Watanabe, 2000). As well as controlling silk tension, spiders are also able to modulate silk stiffness. Orb weavers can adjust silk stiffness during web construction [by spinning silk more quickly (Vollrath et al., 2001)] and after the web is complete (by applying a force to a thread). Both of these processes result in increased ordering of the protein structure and hence increase stiffness (Guan et al., 2012). Spiders can modulate supercontraction to a certain degree by changing silk tension, as threads will not supercontract at high tensions (>140 MPa) (Boutry and Blackledge, 2010; Guan et al., 2011). Therefore, leaving threads slack will lead to increased supercontraction. Threads may also end up being unintentionally slack due to web damage, where supercontraction could act to increase thread tension in these areas. Spiders can also stretch out supercontracted threads to increase stiffness following supercontraction (Guinea et al., 2005). Spiders may therefore modify stiffness using these mechanisms to modulate trade-offs, for example increasing silk stiffness to prioritize mechanical function at the expense of prey sensing ability, as increased stiffness reduces longitudinal wave amplitude (Mortimer et al., 2016). Although these are mechanisms in theory, whether orb weavers actually apply their ability to modulate stiffness to control web properties and vibration transmission is currently unclear. Experiments would be needed to link sensory cues with spider control mechanisms and the resulting mechanical and vibrational properties of the web would need to be quantified.
Individual spiders will modify the geometry of their webs to influence trade-offs in response to environmental variables (Vollrath et al., 1997). When it is windy, garden cross spiders will produce a smaller, rounder orb; with fewer and more widely spaced capture spiral threads (40,686 mm2 total area and 14,545 mm spiral length in still conditions vs. 35,235 mm2 total area and 13,039 mm spiral length in windy conditions) (Vollrath and Samu, 1997)—this geometry is less likely to catch the wind like a sail and get damaged (Zaera et al., 2014). We hypothesize that in these situations, spiders prioritize the mechanical integrity of their web over vibration transmission, as fewer capture spirals reduce transverse wave amplitude (Mortimer et al., 2016). However, there are other relevant trade-offs at play here—these same modifications are also likely to reduce the prey-capture effectiveness of the web.
Orb weavers can also alter the transmission properties of their web in response to their energetic state (Watanabe, 2000). Starved spiders are more likely to target smaller prey items that would usually be ignored when satiated (Herberstein et al., 1998). Some orb weavers (e.g., Octonoba sybotides) modify web geometry when starved by forming webs with spiral rather than linear stabilimenta (web decorations). This is thought to increase radial thread tension (Eberhard, 1972), which makes low amplitude transverse vibrations produced by small prey easier to detect (Mortimer et al., 2016). In turn, this allows starved spiders to respond to small prey more quickly (Watanabe, 2000).
Using all of these mechanisms, orb-weaving spiders in theory are able to adjust the vibration transmission properties of their web at multiple temporal levels, possibly even specifically targeting certain wave types (longitudinal or transverse waves). This high level of control enables them to effectively respond to changes in environment, web damage, fluctuations in body mass, and climatic factors—variation which might otherwise impair vibration information transfer.
Vibration Transmission Within/Along Body
The dynamics of vibration transmission in a spider’s body is strongly influenced by posture, which spiders actively control. We have already discussed how leg angle influences the springs and dampers of the joints, as well as the sensitivity of the lyriform organs, and here we examine how spiders use behavior to control this.
Spiders in theory can use postural changes to alter mass distribution, springs/dampers and geometry in response to different behavioral contexts. For example, the crouching posture tends to be adopted more frequently when a spider is starved (Mhatre et al., 2018). Empirical evidence for the effect of posture is currently limited to the role of springs/dampers at individual leg joints (Blickhan, 1986). Whilst evidence from modeling indicates that full-body geometry and mass distribution is also important for vibration dynamics (Mhatre et al., 2018), this is an area that is yet to be fully investigated. We do know that different postures are correlated with the sensitivity of the lyriform organs, as different joint angles influence the extension and stiffness of the cuticle surrounding the joint (Blickhan, 1986). Spiders adopt different resting postures when sitting at the hub or retreat of their webs, such that their legs are in contact with the relevant silk threads for vibration sensing (Klärner and Barth, 1982). Resting positions are typically used when the spider is sensing externally generated vibrations—in this configuration, the tibia-metatarsus angle is small (<120°), and the sensitivity of the organs is maximized. When the spider is walking, the legs are extended through much of the stepping cycle, reducing the sensitivity of the lyriform organs (Blickhan and Barth, 1985). Therefore, the spider maximizes lyriform organ sensitivity in contexts where they are most likely to show predatory behavior in response to vibrations. During locomotion, other functions are prioritized, where lyriform organs may serve more of a proprioceptive function (Barth, 2002a).
Discussion
So far, this review has covered the mechanisms governing vibration transmission using orb-weaving spiders as examples of animals that influence transmission both within the environment and the body. We have demonstrated that a wide range of morphological and behavioral traits influence vibration transmission via their effects on masses, springs, dampers, and geometry, which interact together to govern vibrational motion. We have also shown that these traits have considerable variation at different levels. How can we understand this variation to uncover the constraints acting on this sensory mode and the evolutionary drivers? Here we discuss the roles that constraints and control have in shaping this trait variation across different time scales and taxonomic levels, and the implications for vibration sensing. Through this discussion, we hypothesize a role for morphological computation in shaping vibration transmission, which can be tested for in future studies and applied within bioinspired engineering.
Firstly, we see that constraints of different types are acting on the morphological traits. The first type is physical constraints—the action of damping, filtering or distortion caused by the physics underlying the dynamics of vibration transmission. Physical constraints are governed by the geometry and properties of masses, springs, and dampers, and act to filter and dampen vibrational information, exemplified by the effects of the spider’s body properties and geometry on vibration transmission both in the web and through the body. These physical constraints ultimately influence propagation distance, the transmission quality of different frequencies, and the ability of the spider to filter information from noise (Mortimer, 2017). The second type is evolutionary trade-offs, given that the web and spider bodies are multifunctional, their morphologies are important not only for vibration transmission, but also other biological functions. For example, web geometry, tension, stiffness, and supercontraction affect both the function of the orb web to capture prey, as well as vibration transmission (Mortimer et al., 2016). Also, the spring and damper properties of spider leg joints are important for locomotion, as well as vibration sensing (Blickhan and Barth, 1985). The third type of constraint is developmental constraints, where the development of the spider’s morphological traits determines their geometry and properties, such as the effects of spider age or leg regeneration. For example, vibration transmission through the body and sensory transduction are affected over the course of development by the different growing rates of the metatarsal lyriform organs and associated metatarsal pads (Morley et al., 2016). These constraints do not act equally on morphological traits; some are more prone to one type of constraint than another.
The constraints do not solely act to limit or fix variation in morphological traits; we found examples of the constraints leading to non-functional variation and even constraints being harnessed for functional uses. For example, some morphological features such as leg length affect vibration transmission, but are relatively fixed within an individual due to development constraints (except over development and regeneration). In terms of non-functional variation, some morphological features will vary, but their variation may not be functionally useful for vibration sensing, such as masses in the web. As we have seen, masses have significant effects on vibration transmission due to the strong influence of mechanical impedance, a physical constraint. There are also a few examples of where apparent physical constraints cause variation that may be harnessed for functional uses in the web. For example, although the spider body mass affects vibration transmission via mechanical impedance, this accentuates orientation cues in the web, which spiders may use to locate prey (Mortimer et al., 2019). As a final thought, few of the morphological traits we have reviewed appear to be free from any form of constraint, with the possible exception of mechanosensor placement. The sheer number of sensors and their wide distribution across spider bodies suggests that evolution was able to come up with this solution repeatedly for functional uses, resulting in apparent redundancy in the system. Investigation of sensor placement differences and functionality across different species that differ in their ecology will be able to solve this mystery.
Even where constraints may be acting on morphology, there are different control mechanisms that can mitigate constraints to maintain functionality for vibration sensing. We can infer a control mechanism is in place when a trait shows variation that is functionally useful, but species comparisons and behavioral studies would provide direct evidence. Control mechanisms can be at the individual level due to plasticity that acts at small time scales to shape trait variation. However, they can also be due to evolution acting over longer timescales driving species differences through niche adaptation. We see that individual spiders are unable to directly control leg and body morphology to mitigate constraints, as these are generally fixed following development. This means that an individual’s vibration transmission system must be robust to variation, which may explain the number of sensors and redundancy in the system. However, spiders can directly control web morphological traits as they have silks with an impressive range of properties, which is functionally useful to the spider. Silk property variation can control the spring and dampers of their extended phenotype at short timescales in response to changing conditions (Mortimer et al., 2014). We also see evidence for control of constraints via niche adaptation in different spider species as certain morphological traits correlate with particular hunting strategies. However, data on the functionality of specific differences between species in body morphology for vibration sensing is lacking. How different species morphologies influence inherent trade-offs, as well as physical and developmental constraints, requires further study. An interdisciplinary approach utilizing modeling (informed through experimental determination of relevant biomechanical parameters) and behavioral trials would be a promising line of research. In combination, we note that spider morphology does not vary as much between species as other terrestrial arthropods, where morphological diversity is more common in silk-use than overall body plan (Vollrath, 1999).
Behavior is an important mechanism controlling morphology. The constraints and control mechanisms above do not apply in the same way to behavioral traits, which in its nature can be more variable, with plasticity at the individual level and niche adaptation at the species level. At the individual level, we see examples of using behavior to mitigate physical constraints by removing masses on the web (Cloudsley-Thompson, 1995), controlling web properties and geometry (Watanabe, 2000; Mortimer et al., 2016), as well as dictating spider position and posture on the web (Mhatre et al., 2018; Mortimer et al., 2018). We also see evidence of using behavior to shape trade-offs between different functions, for example adjusting web geometry in response to changing environmental conditions to shape mechanical verses sensory functions (Vollrath et al., 1997). These behavioral control mechanisms are extremely useful as they effectively harness the multifunctionality of morphological traits that underlie the trade-off, to enable the spider to deal with variable conditions by changing a multifunctional trait (Vollrath, 1999). Whether behavior can be used to mitigate developmental constraints (such as lyriform organ/pad mismatch) is a current research gap, but could be explored by investigating behaviors that juvenile spiders might use to avoid the problem of filtering low-frequency environmental noise.
The high level of involvement that morphology has in vibration transmission suggests a role for morphological computation, where morphology acts to perform useful tasks within a system (Muller and Hoffmann, 2017). Morphological computation is uniquely applicable to our spider context as vibrational information propagates through both the web and body morphology before reaching embedded mechanosensors. In this context, you can see how morphology, or behavior via its action on morphology, could act on vibrational information during propagation to filter and transform vibrational waves, which could be used in theory to promote information transfer. Using morphological computation, the filtering of information from noise is not handled solely by the nervous system, but by the masses, springs/dampers, and geometry of the web/body morphology, with each of these parameters potentially being tuned to transform the input wave differently under varying conditions. We might expect morphological computation to be selected for in terrestrial arthropods as it is in theory an efficient way, in terms of computational cost and potentially energetic cost, to promote information transfer. This is because complex computational tasks that would usually be performed by the CNS are replaced with relatively simple and potentially passive responses that are governed by a system’s morphology (Muller and Hoffmann, 2017). This reduces computational cost as the animal, in theory, can compute more quickly, as CNS processing takes time, but it also reduces the complexity and number of connections required in the nervous system (Pfeifer and Bongard, 2006). It can potentially increase energetic efficiency as the computational tasks could involve passive responses of morphology rather than active metabolic processes in the CNS (Muller and Hoffmann, 2017).
Since morphological computation would be influenced by both morphological and behavioral traits, control of these traits can be selected for in vibration-sensing animals to influence vibration transmission via filtering input waves. Individual plasticity in these traits is likely to influence vibration information transfer through morphological computation. Whether morphology is controlled by an individual to functionally influence vibration transmission through the body warrants further research, but we can make predictions for how control via morphological computation would work in natural contexts. Possible examples of plasticity in spider morphological traits include the shape of the lyriform organs throughout development and after regeneration. If morphological variation here is functional for vibration information transfer, we would predict lyriform organ shape to transform input waves to compensate for leg length changes before sensory transduction occurs, thus avoiding the need for extra processing in the CNS. In this example, both leg length and lyriform organ shape are morphological traits influencing vibration transmission, as these traits alter the mass and material property distribution of the spider’s leg (aspects of mass, springs/dampers, and geometry). Spider behavioral traits potentially involved in morphological computation include posture changes and modifying web geometry, which could be used to functionally influence vibration transmission. If the web uses morphological computation, we would predict that spiders use behavior to modify web properties in response to environmental factors to alter the vibrational information that arrives at the spider, thus avoiding the need for extra processing in the CNS as environment changes. In this example, spider web building is a behavioral trait influencing web geometry as a morphological trait. The geometry of the web in turn influences vibration transmission, as this governs mass and material property distribution of the spider’s web (aspects of mass, springs/dampers, and geometry). Web-building spiders are special in this case; since they control their vibratory environment, they can mitigate the physical constraints faced by many other organisms that are limited by the transmission properties of the substrate upon which they live. This again highlights how web-building spiders are an ideal model organism for studying vibration information transfer, since they have control over morphology in both their vibration transmission platform and their body.
Using spiders as inspiration, these control mechanisms could have direct applications for developing new, bioinspired technologies that use material-bound vibrations for information (Lipson, 2014; Barth, 2019)—for example soft robots for search-and-rescue applications (Hawkes et al., 2017). Designing robots to cope with unknown environments in real-time is a challenge for engineers, as environmental variability can pose problems for artificial systems (Hauser et al., 2011). Sensing here is key, where material-bound vibrations can be used to monitor the internal and external environment to respond appropriately in real-time to environmental variability to maintain functionality. Morphological computation is an efficient way to solve these problems for artificial systems as computational tasks can be outsourced to the system’s morphology, which reduces power requirements. If the insights of how animals, such as spiders, achieve this can be applied to robotic design, it can open up new applications for robots that are adaptable to changing environments. A spider-inspired soft robot would use morphological computation to increase not only efficiency and adaptability, but also damage tolerance, for example using “smart” structures to detect and compensate for damage to part of its body (Hauser et al., 2014). To reach this stage, it is necessary to fill the current research gap in our fundamental understanding of how an eight-legged spider-shaped morphology transmits vibrations. What are the most important biomechanical parameters influencing wave transmission? Is there any evidence for morphological computation significantly reducing processing cost in the CNS? Is the system robust enough for morphological computation to still be effective in the face of variation in morphology over time, and could an individual spider exploit this? How does this process vary in different species, with different life strategies and different morphologies? Mathematical modeling of the whole-body system, combined with experimental manipulations of the animal systems, may provide the answer.
Further research is likely to show that morphological computation in nature is far more widespread than has previously been recognized. We suggest that evolution has produced an array of sensory solutions to problems faced by engineers, and that continued research into understanding the mechanisms that natural systems use to promote information transfer will lead to new types of technologies for varied applications.
Author Contributions
TM wrote the first draft and prepared the figures. BM and TM edited the manuscript. BM provided supervision for TM. Both authors contributed to the article and approved the submitted version.
Conflict of Interest
The authors declare that the research was conducted in the absence of any commercial or financial relationships that could be construed as a potential conflict of interest.
Funding
This work was supported by the Royal Society University Research Fellowship to BM (URF\R1\191033).
Acknowledgments
We thank the Royal Society for funding. We thank Tom Mulder for discussions on spider biotremology and also for his comments on the manuscript.
References
Barth, F. G. (1972). Physiology of slit sense organs 2. Functional morphology of a mechanoreceptor. J. Comp. Physiol. 81, 159–186.
Barth, F. G. (2002b). Spider senses - technical perfection and biology. Zoology 105, 271–285. doi: 10.1078/0944-2006-00082
Barth, F. G. (2019). Mechanics to pre-process information for the fine tuning of mechanoreceptors. J. Comp. Physiol. A 205, 661–686. doi: 10.1007/s00359-019-01355-z
Barth, F. G., and Bohnenberger, J. (1978). Lyriform slit sense organ - Thresholds and stimulus amplitude ranges in a multi-unit mechanoreceptor. J. Comp. Physiol. 125, 37–43. doi: 10.1007/bf00656829
Barth, F. G., Ficker, E., and Federle, H. U. (1984). Model studies on the mechanical significance of grouping in compound spider slit sensilla (Chelicerata, Araneida). Zoomorphology 104, 204–215. doi: 10.1007/bf00312032
Barth, F. G., and Pickelmann, P. (1975). Lyriform slit sense-organs - Modeling an arthropod mechanoreceptor. J. Comp. Physiol. 103, 39–54. doi: 10.1007/bf01380043
Barth, F. G., and Stagl, J. (1976). Slit sense-organs of arachnids - Comparative-study of their topography on walking legs (Chelicerata. Arachnida). Zoomorphologie 86, 1–23. doi: 10.1007/bf01006710
Bleckmann, H., and Barth, F. G. (1984). Sensory ecology of a semi-aquatic spider (Dolomedes triton) 2. the release of predatory behavior by water-surface waves. Behav. Ecol. Sociobiol. 14, 303–312. doi: 10.1007/bf00299502
Blickhan, R. (1986). Stiffness of an arthropod leg joint. J. Biomech. 19, 375–384. doi: 10.1016/0021-9290(86)90014-x
Blickhan, R., and Barth, F. G. (1985). Strains in the exoskeleton of spiders. J. Comp. Physiol. A 157, 115–147. doi: 10.1007/bf00611101
Boutry, C., and Blackledge, T. A. (2010). Evolution of supercontraction in spider silk: structure-function relationship from tarantulas to orb-weavers. J. Exp. Biol. 213, 3505–3514. doi: 10.1242/jeb.046110
Cloudsley-Thompson, J. L. (1995). A review of the anti-predator devices of spiders. Bull. Br. Arachnol. Soc. 10, 81–96.
Cocroft, R. B., and Rodríguez, R. L. (2005). The behavioral ecology of insect vibrational communication. Bioscience 55, 323–334. doi: 10.1641/0006-3568(2005)055[0323:tbeoiv]2.0.co;2
de Crespigny, F. E. C., Herberstein, M. E., and Elgar, M. A. (2001). Food caching in orb-web spiders (Araneae : Araneoidea). Sci. Nat. 88, 42–45. doi: 10.1007/s001140000194
Eberhard, W. G. (1972). The web of Uloborus diversus Araneae Uloboridae. J. Zool. 166, 417–465. doi: 10.1111/j.1469-7998.1972.tb04968.x
Elias, D. O., Hebets, E. A., Hoy, R. R., and Mason, A. C. (2005). Seismic signals are crucial for male mating success in a visual specialist jumping spider (Araneae : Salticidae). Anim. Behav. 69, 931–938. doi: 10.1016/j.anbehav.2004.06.024
Elias, D. O., and Mason, A. C. (2014). “The role of wave and substrate heterogeneity in vibratory communication: practical issues in studying the effect of vibratory environments in communication,” in Studying Vibrational Communication, eds R. B. Cocroft, M. Gogala, P. S. M. Hill, and A. Wessel (New York, NY: Springer), 215–248. doi: 10.1007/978-3-662-43607-3_12
Elias, D. O., Mason, A. C., Maddison, W. P., and Hoy, R. R. (2003). Seismic signals in a courting male jumping spider (Araneae : Salticidae). J. Exp. Biol. 206, 4029–4039. doi: 10.1242/jeb.00634
Erko, M., Younes-Metzler, O., Rack, A., Zaslansky, P., Young, S. L., Milliron, G., et al. (2015). Micro- and nano-structural details of a spider’s filter for substrate vibrations: relevance for low-frequency signal transmission. J. R. Soc. Interface 12:20141111. doi: 10.1098/rsif.2014.1111
Franceschini, N., Pichon, J. M., and Blanes, C. (1992). From insect vision to robot vision. Phil. Trans. R. Soc. Lond. B 337, 283–294. doi: 10.1098/rstb.1992.0106
Frohlich, C., and Buskirk, R. E. (1982). Transmission and attenuation of vibration in orb spider webs. J. Theor. Biol. 95, 13–36. doi: 10.1016/0022-5193(82)90284-3
Guan, J., Porter, D., and Vollrath, F. (2012). Silks cope with stress by tuning their mechanical properties under load. Polymer 53, 2717–2726. doi: 10.1016/j.polymer.2012.04.017
Guan, J., Vollrath, F., and Porter, D. (2011). Two mechanisms for supercontraction in Nephila spider dragline silk. Biomacromolecules 12, 4030–4035. doi: 10.1021/bm201032v
Guinea, G. V., Elices, M., Pérez-Rigueiro, J., and Plaza, G. R. (2005). Stretching of supercontracted fibers: a link between spinning and the variability of spider silk. J. Exp. Biol. 208, 25–30. doi: 10.1242/jeb.01344
Hauser, H., Ijspeert, A. J., Füchslin, R. M., Pfeifer, R., and Maass, W. (2011). Towards a theoretical foundation for morphological computation with compliant bodies. Biol. Cybern. 105, 355–370. doi: 10.1007/s00422-012-0471-0
Hauser, H., Nakajima, K., and Füchslin, R. M. (2014). “Morphological computation - The body as a computational resource,” in Opinions and Outlooks on Morphological Computation, eds H. Hauser, R. M. Füchslin, and R. Pfeifer (e-book), 227–244.
Hawkes, E. W., Blumenschein, L. H., Greer, J. D., and Okamura, A. M. (2017). A soft robot that navigates its environment through growth. Sci. Robot. 2:eaan3028. doi: 10.1126/scirobotics.aan3028
Henaut, Y., Machkour-M’Rabet, S., Winterton, P., and Calmé, S. (2010). Insect attraction by webs of Nephila clavipes (Araneae: Nephilidae). J. Arachnol. 38, 135–138. doi: 10.1636/t08-72.1
Herberstein, M. E., Abernethy, K. E., Backhouse, K., Bradford, H., de Crespigny, F. E., Luckock, P. R., et al. (1998). The effect of feeding history on prey capture behaviour in the orbweb spider Argiope keyserlingi Karsch (Araneae: Araneidae). Ethology 104, 565–571. doi: 10.1111/j.1439-0310.1998.tb00091.x
Hill, P. S. M. (2009). How do animals use substrate-borne vibrations as an information source? Sci. Nat. 96, 1355–1371. doi: 10.1007/s00114-009-0588-8
Hössl, B., Böhm, H. J., Rammerstorfer, F. G., and Barth, F. G. (2007). Finite element modeling of arachnid slit sensilla- I. The mechanical significance of different slit arrays. J. Comp. Physiol. A 193, 445–459. doi: 10.1007/s00359-006-0201-y
Hössl, B., Böhm, H. J., Rammerstorfer, F. G., Mullan, R., and Barth, F. G. (2006). Studying the deformation of arachnid slit sensilla by a fracture mechanical approach. J. Biomech. 39, 1761–1768. doi: 10.1016/j.jbiomech.2005.05.031
Hössl, B., Böhm, H. J., Schaber, C. F., Rammerstorfer, F. G., and Barth, F. G. (2009). Finite element modeling of arachnid slit sensilla: II. Actual lyriform organs and the face deformations of the individual slits. J. Comp. Physiol. A 195, 881–894. doi: 10.1007/s00359-009-0467-y
Klärner, D., and Barth, F. G. (1982). Vibratory signals and prey capture in orb-weaving spiders (Zygiella x-notata, Nephila clavipes, Araneidae). J. Comp. Physiol. 148, 445–455. doi: 10.1007/bf00619783
Landolfa, M. A., and Barth, F. G. (1996). Vibrations in the orb web of the spider Nephila clavipes: cues for discrimination and orientation. J. Comp. Physiol. A 179, 493–508.
Lipson, H. (2014). Challenges and opportunities for design, simulation, and fabrication of soft robots. Soft Robotics 1, 21–27. doi: 10.1089/soro.2013.0007
Liu, Y., Sponner, A., Porter, D., and Vollrath, F. (2008). Proline and processing of spider silks. Biomacromolecules 9, 116–121. doi: 10.1021/bm700877g
Main, I. G. (1993). Vibrations and Waves in Physics, 3rd Edn. Cambridge: Cambridge University Press.
Masters, W. M. (1984a). Vibrations in the orbwebs of Nuctenea sclopetaria (Araneidae) 1. Behav. Ecol. Sociobiol. 15, 207–215. doi: 10.1007/bf00292977
Masters, W. M. (1984b). Vibrations in the orbwebs of Nuctenea sclopetaria (Araneidae) 2. Prey and wind signals and the spider’s response threshold. Behav. Ecol. Sociobiol. 15, 217–223. doi: 10.1007/bf00292978
Masters, W. M., and Markl, H. (1981). Vibration signal transmission in spider Nuctenea sclopetaria orb webs. Science 209, 363–365. doi: 10.1126/science.213.4505.363
McConney, M. E., Schaber, C. F., Julian, M. D., Barth, F. G., and Tsukruk, V. V. (2007). Viscoelastic nanoscale properties of cuticle contribute to the high-pass properties of spider vibration receptor (Cupiennius salei Keys). J. R. Soc. Interface 4, 1135–1143. doi: 10.1098/rsif.2007.1000
Mhatre, N., Sivalinghem, S., and Mason, A. C. (2018). Posture controls mechanical tuning in the black widow spider mechanosensory system. bioRxiv [Preprint], Available at: https://www.biorxiv.org/content/10.1101/484238v1 (accessed October 20, 2019).PMIDNOPMID
Mo, A., Izzi, F., Haeufle, D. F. B., and Badri-Spröwitz, A. (2020). Effective viscous damping enables morphological computation in legged locomotion. Front. Robot. AI 7:110. doi: 10.3389/frobt.2020.00110
Molina, J., Schaber, C. F., and Barth, F. G. (2009). In search of differences between the two types of sensory cells innervating spider slit sensilla (Cupiennius salei Keys.). J. Comp. Physiol. A 195, 1031–1041. doi: 10.1007/s00359-009-0477-9
Morley, E. L., Sivalinghem, S., and Mason, A. C. (2016). Developmental morphology of a lyriform organ in the Western black widow (Latrodectus hesperus). Zoomorphology 135, 433–440. doi: 10.1007/s00435-016-0324-9
Mortimer, B. (2017). Biotremology: do physical constraints limit the propagation of vibrational information? Anim. Behav. 130, 165–174. doi: 10.1016/j.anbehav.2017.06.015
Mortimer, B. (2019). A Spider’s vibration landscape: adaptations to promote vibrational information transfer in orb webs. Integr. Comp. Biol. 59, 1636–1645. doi: 10.1093/icb/icz043
Mortimer, B., Gordon, S. D., Holland, C., Siviour, C. R., Vollrath, F., and Windmill, J. F. C. (2014). The speed of sound in silk: linking material performance to biological function. Adv. Mater. 26, 5179–5183. doi: 10.1002/adma.201401027
Mortimer, B., Soler, A., Siviour, C. R., and Vollrath, F. (2018). Remote monitoring of vibrational information in spider webs. Sci. Nat. 105:37.
Mortimer, B., Soler, A., Siviour, C. R., Zaera, R., and Vollrath, F. (2016). Tuning the instrument: sonic properties in the spider’s web. J. R. Soc. Interface 13:20160341. doi: 10.1098/rsif.2016.0341
Mortimer, B., Soler, A., Wilkins, L., and Vollrath, F. (2019). Decoding the locational information in the orb web vibrations of Araneus diadematus and Zygiella x-notata. J. R. Soc. Interface 16:154.
Muller, V. C., and Hoffmann, M. (2017). What is morphological computation? On how the body contributes to cognition and control. Artif. Life 23, 1–24. doi: 10.1162/artl_a_00219
Naftilan, S. A. (1999). Transmission of vibrations in funnel and sheet spider webs. Int. J. Biol. Macromol. 24, 289–293. doi: 10.1016/s0141-8130(98)00092-0
O’Connell-Rodwell, C. E. (2007). Keeping an “Ear” to the ground: seismic communication in elephants. Physiology 22, 287–294. doi: 10.1152/physiol.00008.2007
Pasquet, A., Cardot, J., and Leborgne, R. (2007). Wasp attacks and spider defence in the orb weaving species Zygiella x-notata. J. Insect Behav. 20, 553–564. doi: 10.1007/s10905-007-9098-8
Pfeifer, R., and Bongard, J. (2006). How the Body Shapes the Way we Think: A New View of Intelligence. Cambridge, MA: MIT press.
Pringle, J. W. S. (1955). The function of the lyriform organs of arachnids. J. Exp. Biol. 32, 270–278.
Schüch, W., and Barth, F. G. (1985). Temporal patterns in the vibratory courtship signals of the wandering spider Cupiennius salei Keys. Behav. Ecol. Sociobiol. 16, 263–271. doi: 10.1007/bf00310990
Schüch, W., and Barth, F. G. (1990). Vibratory communication in a spider - Female responses to synthetic male vibrations. J. Comp. Physiol. A 166, 817–826.
Seyfarth, E. A. (1978). Lyriform slit sense-organs and muscle reflexes in spider leg. J. Comp. Physiol. 125, 45–57. doi: 10.1007/bf00656830
Seyfarth, E. A., and Barth, F. G. (1972). Compound slit sense organs on spider leg - Mechanoreceptors involved in kinesthetic orientation. J. Comp. Physiol. 78, 176–191. doi: 10.1007/bf00693611
Seyfarth, E. A., Eckweiler, W., and Hammer, K. (1985). Proprioceptors and sensory nerves in the legs of a spider. Cupiennius salei (Arachnida, Araneida). Zoomorphology 105, 190–196. doi: 10.1007/bf00312156
Seyfarth, E. A., Hergenröder, R., Ebbes, H., and Barth, F. G. (1982). Idiothetic orientation of a wandering spider - Compensation of detours and estimates of goal distance. Behav. Ecol. Sociobiol. 11, 139–148. doi: 10.1007/bf00300103
Speck, J., and Barth, F. G. (1982). Vibration sensitivity of pretarsal slit sensilla in the spider leg. J. Comp. Physiol. 148, 187–194. doi: 10.1007/bf00619125
Speck-Hergenröder, J., and Barth, F. G. (1988). Vibration sensitive hairs on the spider leg. Experientia 44, 13–14. doi: 10.1007/bf01960224
Straus, S., and Avilés, L. (2018). Effects of host colony size and hygiene behaviours on social spider kleptoparasite loads along an elevation gradient. Funct. Ecol. 32, 2707–2716. doi: 10.1111/1365-2435.13225
Vibert, S., Scott, C., and Gries, G. (2016). Vibration transmission through sheet webs of hobo spiders (Eratigena agrestis) and tangle webs of western black widow spiders (Latrodectus hesperus). J. Comp. Physiol. A 202, 749–758. doi: 10.1007/s00359-016-1113-0
Vincent, J. (1990). Structural Biomaterials Revised Edition. New Jersey: Princeton University Press.
Vollrath, F. (1995). Lyriform organs on regenerated spider legs. Bull. Br. Arachnol. Soc. 10, 115–118.
Vollrath, F. (2000). Strength and structure of spiders’ silks. J. Biotechnol. 74, 67–83. doi: 10.1016/s1389-0352(00)00006-4
Vollrath, F., Downes, M., and Krackow, S. (1997). Design variability in web geometry of an orb-weaving spider. Physiol. Behav. 62, 735–743. doi: 10.1016/s0031-9384(97)00186-8
Vollrath, F., Madsen, B., and Shao, Z. Z. (2001). The effect of spinning conditions on the mechanics of a spider’s dragline silk. Proc. R. Soc. B 268, 2339–2346.
Vollrath, F., and Samu, F. (1997). The effect of starvation on web geometry in an orb-weaving spider. Bull. Br. Arachnol. Soc. 10, 295–298.
Watanabe, T. (2000). Web tuning of an orb-web spider, Octonoba sybotides, regulates prey-catching behaviour. Proc. R. Soc. B 267, 565–569. doi: 10.1098/rspb.2000.1038
Wignall, A. E., and Heberstein, M. E. (2013). The influence of vibratory courtship on female mating behaviour in orb-web spiders (Argiope keyserlingi. Karsch 1878). PLoS One 8:e53057. doi: 10.1371/journal.pone.0053057
Wu, C. H., and Elias, D. O. (2014). Vibratory noise in anthropogenic habitats and its effects on prey detection in a web-building spider. Anim. Behav. 90, 47–56. doi: 10.1016/j.anbehav.2014.01.006
Yack, J. E. (2004). The structure and function of auditory chordotonal organs in insects. Microsc. Res. Techniq. 63, 315–337. doi: 10.1002/jemt.20051
Young, S. L., Chyasnavichyus, M., Erko, M., Barth, F. G., Fratzl, P., Zlotnikov, I., et al. (2014). A spider’s biological vibration filter: micromechanical characteristics of a biomaterial surface. Acta Biomater. 10, 4832–4842. doi: 10.1016/j.actbio.2014.07.023
Keywords: biotremology, sensory ecology, physical ecology, morphological computation, Araneae
Citation: Miller TE and Mortimer B (2020) Control vs. Constraint: Understanding the Mechanisms of Vibration Transmission During Material-Bound Information Transfer. Front. Ecol. Evol. 8:587846. doi: 10.3389/fevo.2020.587846
Received: 27 July 2020; Accepted: 26 November 2020;
Published: 11 December 2020.
Edited by:
Fernando Montealegre-Z, University of Lincoln, United KingdomReviewed by:
Joseph Jackson, University of Strathclyde, United KingdomGregory Patrick Sutton, University of Bristol, United Kingdom
Copyright © 2020 Miller and Mortimer. This is an open-access article distributed under the terms of the Creative Commons Attribution License (CC BY). The use, distribution or reproduction in other forums is permitted, provided the original author(s) and the copyright owner(s) are credited and that the original publication in this journal is cited, in accordance with accepted academic practice. No use, distribution or reproduction is permitted which does not comply with these terms.
*Correspondence: Beth Mortimer, YmV0aC5tb3J0aW1lckB6b28ub3guYWMudWs=