- 1Environment Research Institute, Shandong University, Qingdao, China
- 2State Key Laboratory of Eco-hydraulics in Northwest Arid Region of China, Xi′an University of Technology, Xi′an, China
Wetlands have been considered as a vital reservoir of global carbon and are vulnerable to plant invasion. However, the influence mechanism of plant invasion on soil organic carbon (SOC) in wetlands remains unclear. In this study, we investigated SOC, soil physicochemical properties, and soil microbes in the invaded and non-invaded sites in Xinxue River and Xinxue River Constructed Wetland to research the effects of Alternanthera philoxeroides invasion on SOC and explore the related mechanisms. The invasion of A. philoxeroides increased SOC content and density in both the river and the constructed wetland. SOC in the two types of wetlands was positively correlated with moisture content and negatively correlated with bulk density, dissolved oxygen, water temperature, soil temperature, and pH. The invasion decreased the dissolved oxygen in the river and the soil temperature in the constructed wetland which might be the explanation for the increase of SOC in the two types of wetlands. In the river, the decrease of dissolved oxygen caused by plant invasion could alter the microbial community and limit the decomposition of SOC by microbes, which was confirmed by the increase of microbial α diversity indices and the correlations between soil microbial community in the river and dissolved oxygen showed by RDA analysis. The invasion of A. philoxeroides significantly shifted the soil microbial community in the constructed wetland. Redundancy analysis showed that the variation of soil microbial community structure in the constructed wetland could be mainly explained by soil pH and soil temperature. The decrease of soil temperature in the constructed wetland might inhibit microbial activities and result in the accumulation of SOC. In addition, the indicator species reflecting soil microbial community shifted by plant invasion were highly correlated with SOC, suggesting that the variation of indicator species between invaded sites and non-invaded sites might be another reason for the difference in SOC. The study indicates that A. philoxeroides invasion might increase the carbon storage in rivers and constructed wetlands with different mechanisms.
Introduction
As a vital reservoir of carbon, wetlands can offset partial release of carbon dioxide into the atmosphere (Dixon and Krankina, 1995; Mitsch et al., 2013; Nag et al., 2019). The carbon sequestration function of wetland soil plays an important role in mitigating global climate change (Nag et al., 2017; Zhao et al., 2017). According to the Convention on Wetlands signed in 1971, wetlands could be divided into natural wetlands and constructed wetlands (Hawkins and Smith, 1983; Ramsar Convention Secretariat, 2004). Rivers are an example of typical natural wetlands, and a constructed wetland is a combination of water, plants, and substrates, which is modeled on natural wetlands (Hui et al., 2009; Zhang et al., 2009). Rivers and constructed wetlands are important carbon reservoirs (Zhang L. et al., 2015; Reddy et al., 2016). The increase of exotic plant invasion in wetlands, which threatens ecological functions, is gaining increasing attention in ecology research (van der Putten et al., 2007; Seebens et al., 2017; Vaz et al., 2019). Invasive plants can affect the carbon cycle of wetlands’ ecosystems, but the mechanisms remain unclear (McKinley and Blair, 2008; Jin et al., 2017; Bobul’ská et al., 2019; Qi et al., 2019).
Plant invasion has direct and indirect effects on the soil organic carbon (SOC) storage of wetlands. Plant invasion can directly affect SOC storage attributed to the high net primary productivity of invasive plants (Burke et al., 1998; Ehrenfeld, 2003; Tamura and Tharayil, 2014). Plant invasion may also affect SOC via the root exudation and litter decomposition (Craig et al., 2015). Plant invasion could indirectly impact SOC storage by altering soil physicochemical properties (Yang et al., 2017; Qi et al., 2019; Yang, 2019). A study by Yang (2019) demonstrated that Spartina alterniflora invasion not only directly increased SOC content, but also indirectly affected SOC through mediating the effects of soil moisture content. Moreover, the decrease of soil temperature could also enhance SOC storage (Yang, 2019).
The indirect effect also includes the impact of plant invasion on microorganisms. Microorganisms, as natural decomposers, directly play a part in the carbon cycle of wetlands (Huang et al., 2014). Dramatic shifts in soil nutrient content caused by plant invasion could alter the richness and diversity of soil microbial communities (Lorenzo et al., 2010; Yang et al., 2013; Lazzaro et al., 2014). Fontaine et al. (2003) reported that when soil nutrient availability highly increased, the microbial decomposition of fresh organic matter increased while the microbial decomposition of soil organic matter decreased, resulting in SOC accumulation. In addition, the change of soil physicochemical properties such as pH and temperature caused by plant invasion can indirectly affect SOC storage by affecting microbial activities (Bainard et al., 2016; Nguyen et al., 2018). The carbon storage of wetlands was affected by certain microbes such as Betaproteobacteria and Planctomycetacia (Xin and Qin, 2019). The carbon metabolic rate could be altered by the variation of the microbial community. For example, the abundance of heterotrophic bacteria such as Bacteroidetes was increased with Spartina alterniflora invasion, which increased microbial respiration and resulted in the decrease of SOC (Song et al., 2020). Therefore, the indirect effect of plant invasion on SOC storage in wetlands is the key part of the influence mechanism of plant invasion on SOC (Zhang G. et al., 2019; Zhang P. et al., 2020; Yang et al., 2020).
Alternanthera philoxeroides (Mart.) Griseb., a species native to South America, is widely distributed in Oceania, South America, North America, Africa, and many other countries and regions (Telesnicki et al., 2011). In the 1930s, it was first introduced, widely planted and disseminated as feed in South China. In 2003, it was listed in the first batch of invasive alien species in China by the State Environmental Protection Administration of China (Pang et al., 2007). At present, A. philoxeroides is mainly distributed in low altitude, relatively warm, and humid climate areas east of 97°E and south of 44°N in China (Pang et al., 2007).
To explore the influence mechanism of plant invasion on SOC in rivers and constructed wetlands, we chose Nansi Lake Basin, where the invasion was serious, as the research site. We hypothesized that A. philoxeroides invasion could increase SOC storage by altering soil physicochemical properties and the soil microbial community. To test this hypothesis, we examined the SOC content and density, compared the soil physicochemical properties and soil microbial community composition in the invaded sites and non-invaded sites in Xinxue River (XR) and Xinxue River Constructed Wetland (XC) in 2018. We also analyzed the correlations between SOC, soil physicochemical properties and soil microorganisms. This study is of great significance to understand the impact of the invasion of A. philoxeroides and other similar invasive plants on the wetland carbon stock.
Materials and Methods
Study Area and Field Sampling
This study was conducted in the second largest freshwater lake in Huaihe River Basin, Nansi Lake, Shandong Province, China (116°34′–117°21′E, 34°27′–35°20′N). Nansi Lake, the largest freshwater lake in North China, serves as an important hub for water transfer and storage in the East Route of the South-to-North Water Transfer Project in China (Wu et al., 2010). Xinxue River (XR) is one of the principal inflow rivers of Nansi Lake. The Xinxue River Constructed Wetland (XC) was built in 2007 and is located on the southern bank of Xinxue River.
In July 2018, soil samples for physicochemical properties analyses and microbial samples in surface soil for microbial analyses were collected in the sediments in XR and XC (Figure 1). To explore the effects of A. philoxeroides invasion on SOC in wetlands, samples in non-invaded sites were assumed to substitute for the samples in the pre-invasion condition, and this experimental design was consistent with Chen et al. (2018). In XR, nine invaded sites were sampled uniformly and randomly throughout the river. And due to the less non-invaded places in the middle and lower reaches of XR, we only chose three non-invaded sites in the upstream to collect soil samples and microbial samples. In XC, we chose seven invaded sites and five non-invaded sites to collect soil and microbial samples. All collected samples were labeled in sealed bags. Soil samples were stored at 4°C and analyzed in 48 h (Fang et al., 2019; Boscutti et al., 2020; Zhang P. et al., 2020). Microbial samples were stored at −80°C before microbial sequencing.
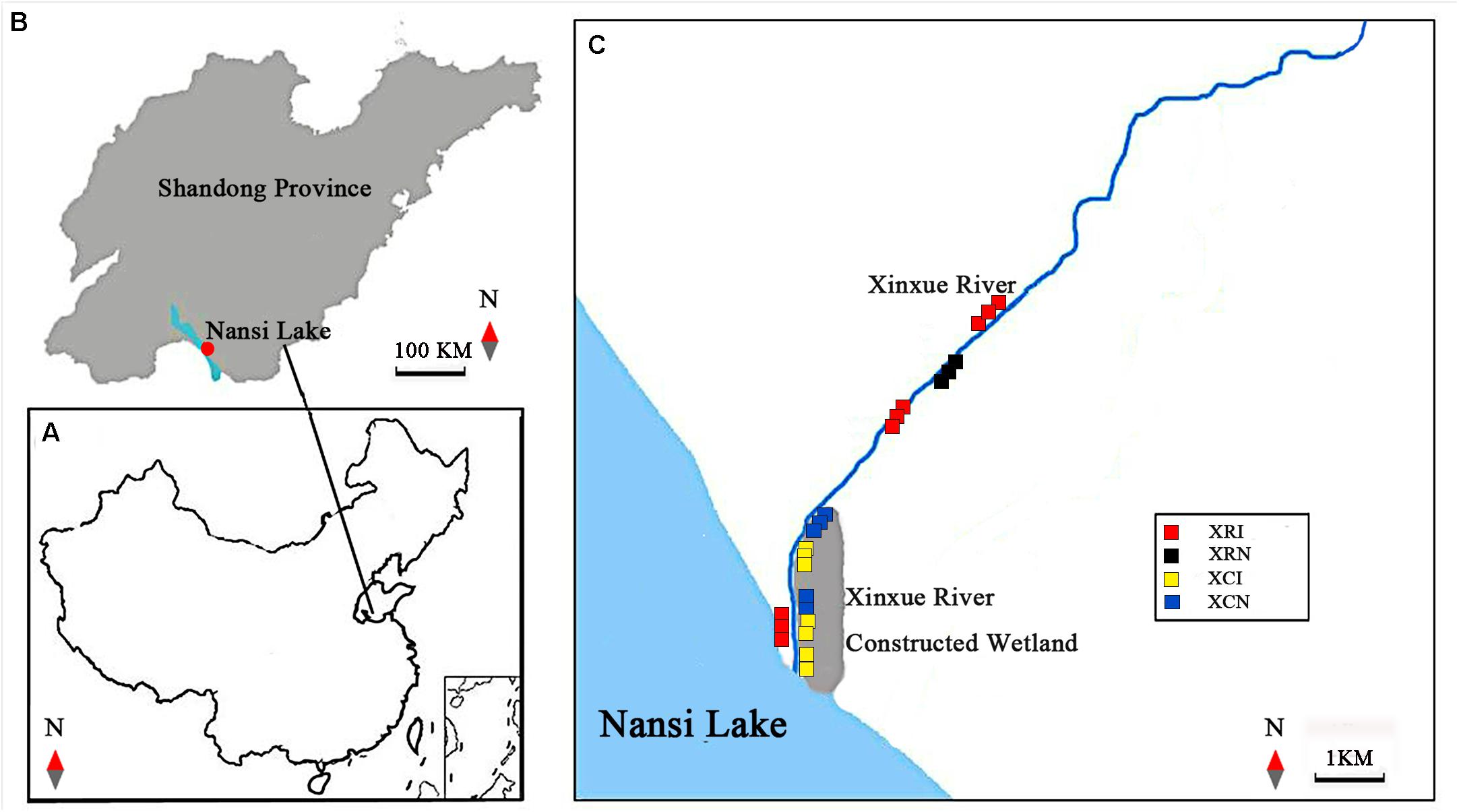
Figure 1. Locations of Xinxue River and Xinxue River Constructed Wetland in Shandong Province and the distribution of invaded and non-invaded sites in the two types of wetlands. (A,B) are the locations of the Nansi Lake Basin in China and Shandong Province, respectively; (C) the distribution of invaded and non-invaded sites in Xinxue River and Xinxue River Constructed Wetland. XRI, Invaded sites in Xinxue River; XRN, Non-invaded sites in Xinxue River; XCI, Invaded sites in Xinxue River Constructed Wetland; XCN, Non-invaded sites in Xinxue River Constructed Wetland.
Laboratory Analyses
Soil samples were air-dried at room temperature (∼25°C) and then sieved by a 0.15 mm sieve. Soil samples (0.05–0.3 g, accurate to 0.0001 g) were added into hard glass tubes and mixed with 10.00 mL potassium dichromate-sulfuric acid solution (0.4 mol L–1). The glass tubes were inserted into the wire cage and then heated in the oil bath at 170–180°C for 5 min. The liquid and soil residue in the tube was transferred to a 250 mL triangular bottle, the tube and funnel were washed with distilled water, and the washing liquid was poured into the triangular bottle. Three drops of o-phenanthroline indicator were added into the triangular bottle, the residual K2CrO7 was titrated by 0.2 mol L–1 ferrous sulfate solution. The titration was finished when the solution changed from orange to blue-green and then to brown-red. About 0.2 g of SiO2 was selected as blank control, and about 0.2 g of the standard soil GBW 07413a (ASA-2) was selected as the standard control. The volume of consumed ferrous sulfate was recorded and substituted into the following formula:
OM: mass fraction of soil organic matter (g kg–1); V0: standard solution volume of ferrous sulfate consumed by blank experiment (mL); V: standard solution volume of ferrous sulfate consumed by sample determination (mL); c: concentration of ferrous sulfate standard solution (mol L–1); m: weight of the drying sample (g); 0.003: the mass of 1/4 mmol of carbon (g mmol–1); 1.724: conversion factor of organic carbon to organic matter; 1.10: oxidation correction coefficient; 1,000: conversion factor from g to kg.
SOC content and density were calculated by the formulae:
SOC: mass fraction of soil organic carbon (g kg–1); SOCD: mass fraction of soil organic carbon per unit volume (kg m–3); bulk density: weight of soil per unit volume (g cm–3) (Rudiyanto, Minasny and Setiawan, 2016). The content of soil organic matter was determined by potassium dichromate oxidation method, referring to the determination of soil organic matter in the Agricultural Industry Standard of the People’s Republic of China published in 2006.
The pH of soil was obtained by using the determination of pH in soil (NY/T 1377-2007). The bulk density and moisture content were determined by using the ring knife method (Li and Zheng, 2019).
Microbial Analyses
The diversity and richness of the soil microbial samples in the invaded sites and non-invaded sites in XR and XC were analyzed by 16S rRNA amplicon sequencing. First, a rapid CTAB DNA isolation technique was used to extract DNA in microbial samples (Stewart and Via, 1993). The 515F (5′-GTGCCAGCMGCCGCGGTAA-3′) and 806R (5′-GGACTACHVGGGTWTCTAAT-3′) primers were used for the amplification of the microbial (bacterial and archaeal) 16S rRNA V4 fragments (Wu et al., 2016). The truseq DNA PCR free sample preparation kit (Illumina, San Diego, CA, United States) was used to construct the sequencing library and add tags. Quantitation and detection of the constructed library were carried out utilizing a Qubit Fluorescence Quantitative Analyzer (Thermo Fisher Scientific) and an Agilent 2100 Biological Analyzer (Agilent Technologies, Palo Alto, CA, United States). Based on the Illumina HiSeq sequencing platform, the library which met the standard was sequenced to get raw data (Jiao et al., 2016). Splicing, filtering, quality control, and chimeric filtering were carried out to get effective tags (Caporaso et al., 2010). The effective tags with similarities greater than 97% were clustered into Operational Taxonomic Units (OTUs), and the representative sequences of OTUs were annotated to obtain the community composition of microbial samples.
Statistical Analyses
All statistical analyses of soil properties were performed using SPSS software (version 21.0), R (v4.0.1), Canoco 4.5, and Origin 2017. A two-way analysis of variance (ANOVA) was used to analyze the effects of plant invasion, wetland types, and their interactions on SOC and other soil physicochemical properties. One-way ANOVA was performed to analyze the differences in the effects of plant invasion on SOC and other soil physicochemical properties in the river and the constructed wetland, and it was also conducted when the interaction effects were significant in the two-way ANOVA (Nitao, 1989; Qi et al., 2019; Yang et al., 2020). Spearman correlation analyses were conducted in R (v4.0.1) to study the relationships between SOC and other soil physicochemical properties. The microbial data analysis was performed by QIIME, R (v4.0.1), and Galaxy platform1. The microbial α diversity indices including richness indices (Chao1, ACE) and diversity indices (Shannon, Simpson), were calculated using QIIME (Zhang H. et al., 2020). Redundancy analysis (RDA) was conducted in Canoco 4.5 for the relationships between soil properties and microbial composition at the phyla-level. Monte Carlo permutation test (999 permutations) was used to test the statistical significance of the variables of RDA at the 0.05 level (Zhang G. et al., 2020). The differences in microbial composition between invaded sites and non-invaded sites were analyzed and visualized by linear discriminant analysis effect size (LEfSe) using Galaxy platform (Liu et al., 2018). Spearman correlation analyses of SOC and other soil physicochemical properties with indicator species were also carried out in R (v4.0.1).
Accession Number
The raw sequencing data have been submitted to NCBI Sequence Read Archive (SRA) with the Accession Number of SRP2679622.
Results
Effects of A. philoxeroides Invasion on SOC Content and Density
A. philoxeroides invasion strongly affected SOC in the two types of wetlands (Figure 2). SOC content was significantly higher in invaded sites than in non-invaded sites both in XR and XC (PXR = 0.003, PXC = 0.029, Figure 2A). In XR, the SOC density in invaded sites (XRI) was 63% higher than that in non-invaded sites (XRN) (P = 0.002, Figure 2B), while the density of SOC in the invaded sites (XCI) was 30% higher than that in non-invaded sites (XCN) in XC (Figure 2B). The content of SOC in XC was 93% higher than that in XR in both invaded sites and non-invaded sites (Figure 2A). SOC density in XCI was 55% higher than that in XRI (P < 0.0001, Figure 2B), while in the non-invaded sites, SOC density in XCN was 94% higher than that in XRN (P = 0.006, Figure 2B).
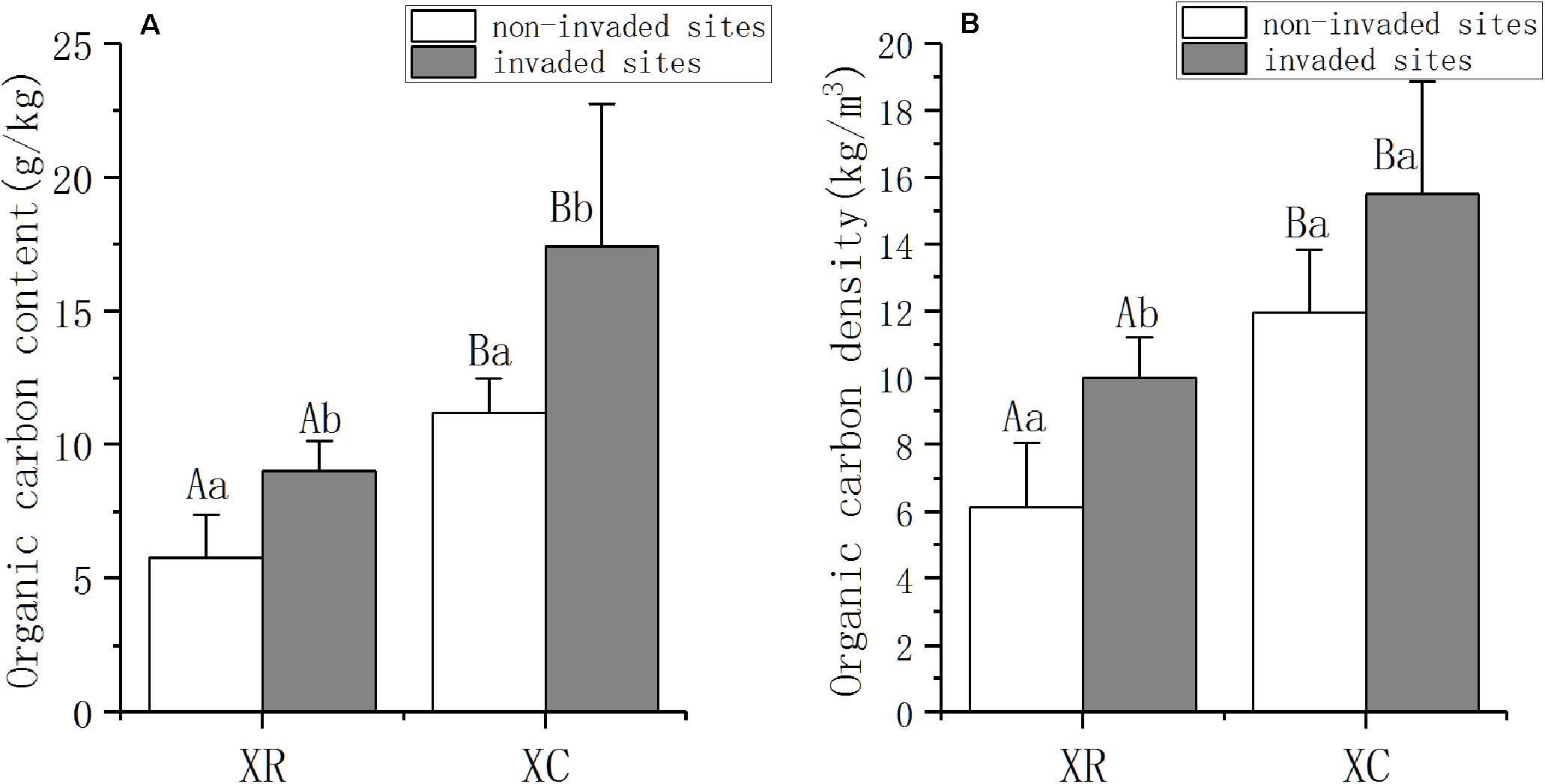
Figure 2. SOC content (A) and density (B) (mean ± SD, standard deviation) in Xinxue River and Xinxue River Constructed Wetland in 2018. XR, Xinxue River; XC, Xinxue River Constructed wetland. Different superscript uppercase letters indicate statistically significant differences at α = 0.05 level between the river and constructed wetland. Different superscript lowercase letters indicate statistically significant differences at α = 0.05 level between invaded sites and non-invaded sites.
Soil Physicochemical Properties and the Relationships Between SOC and Soil Physicochemical Properties
In XR, dissolved oxygen in invaded sites was significantly lower than that in non-invaded sites (Table 1). Water temperature and soil temperature in invaded sites in XC were significantly lower than those in non-invaded sites (Table 1). In the invaded sites in the two types of wetlands, soil moisture content in XRI was significantly lower than that in XCI (Table 1), while bulk density, dissolved oxygen, water temperature, soil temperature, and pH in XRI were significantly higher than those in XCI (Table 1). However, there was no significant difference in moisture content and bulk density in the non-invaded sites in the two types of wetlands (Table 1). Spearman correlation analysis showed that SOC content was significantly positively correlated with moisture content, but significantly negatively related to bulk density, dissolved oxygen, water temperature, soil temperature, and pH (Figure 3). The correlations of SOC density were basically the same with SOC content, except for bulk density.
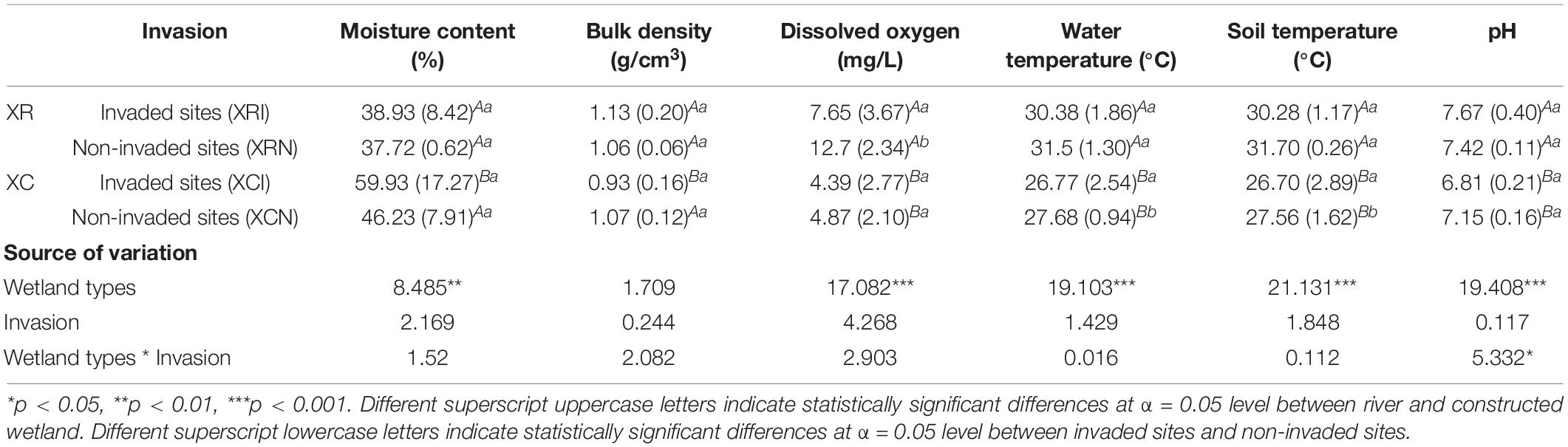
Table 1. Soil physicochemical properties (mean, SD) in Xinxue River (XR) and Xinxue River Constructed Wetland (XC).
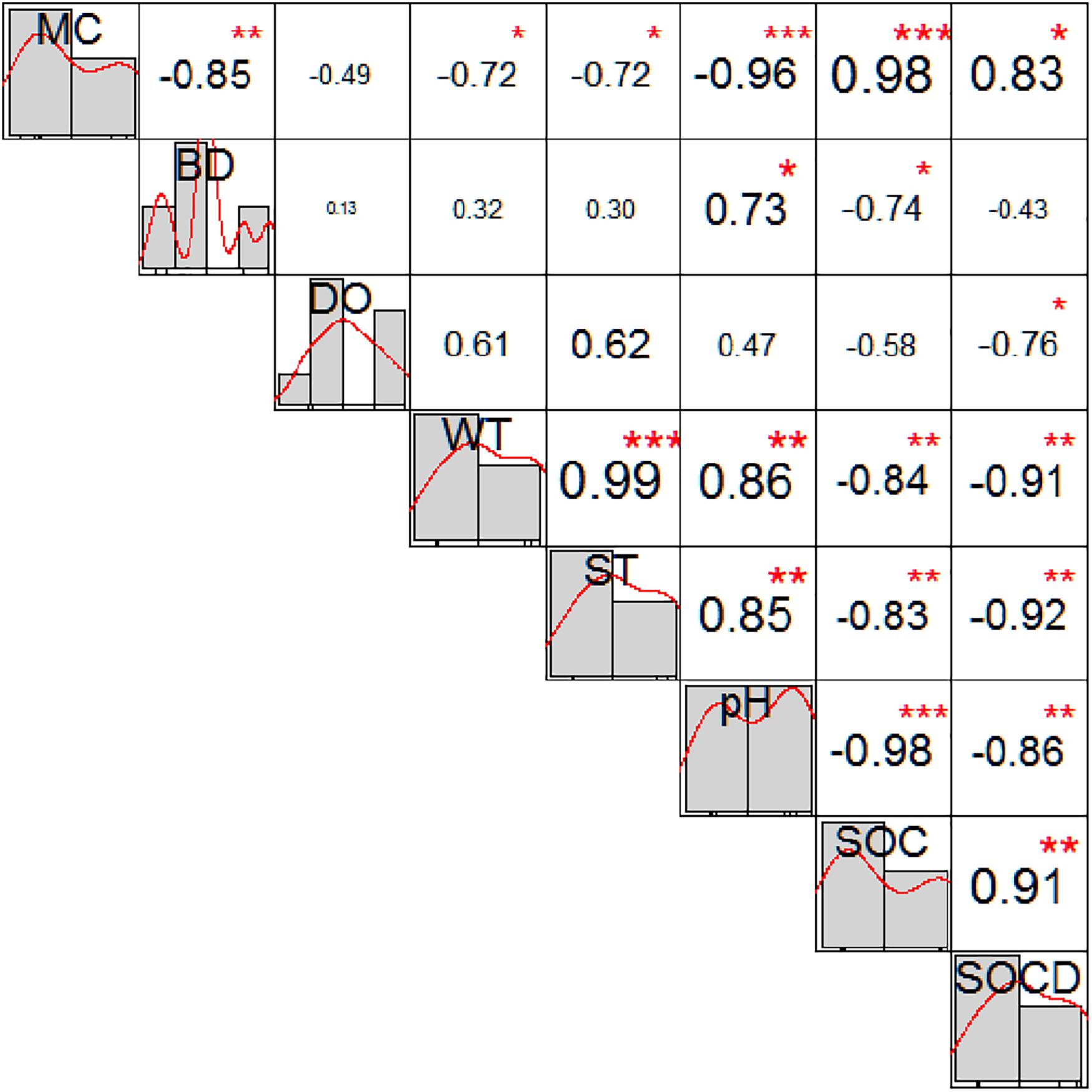
Figure 3. Spearman correlation matrix for SOC and soil physicochemical properties in Xinxue River (XR) and Xinxue River Constructed Wetland (XC). SOC, Soil organic carbon content; SOCD, soil organic carbon density; MC, moisture content; BD, bulk density; DO, dissolved oxygen; WT, water temperature; ST, soil temperature. Diagonal shows the variable distribution diagram; the top right of the diagonal shows the Spearman correlation coefficient and the level of significance, the size of the number reflects the value of the correlation (*p < 0.05; **p < 0.01; ***p < 0.001).
Soil Microbial Community and the Relationships Between Soil Microbial Community and Soil Properties
Proteobacteria was the most dominant phylum among all the studied sites, which accounted for 46.4–54.4% of total bacterial community composition (Figure 4). Chloroflexi, Bacteroidetes, Acidobacteria, Gemmatimonadetes, Actinobacteria, and Verrucomicrobia were also abundant phyla. Euryarchaeota, Bathyarchaeota, and Elusimicrobia were important Archaea in the studied area.
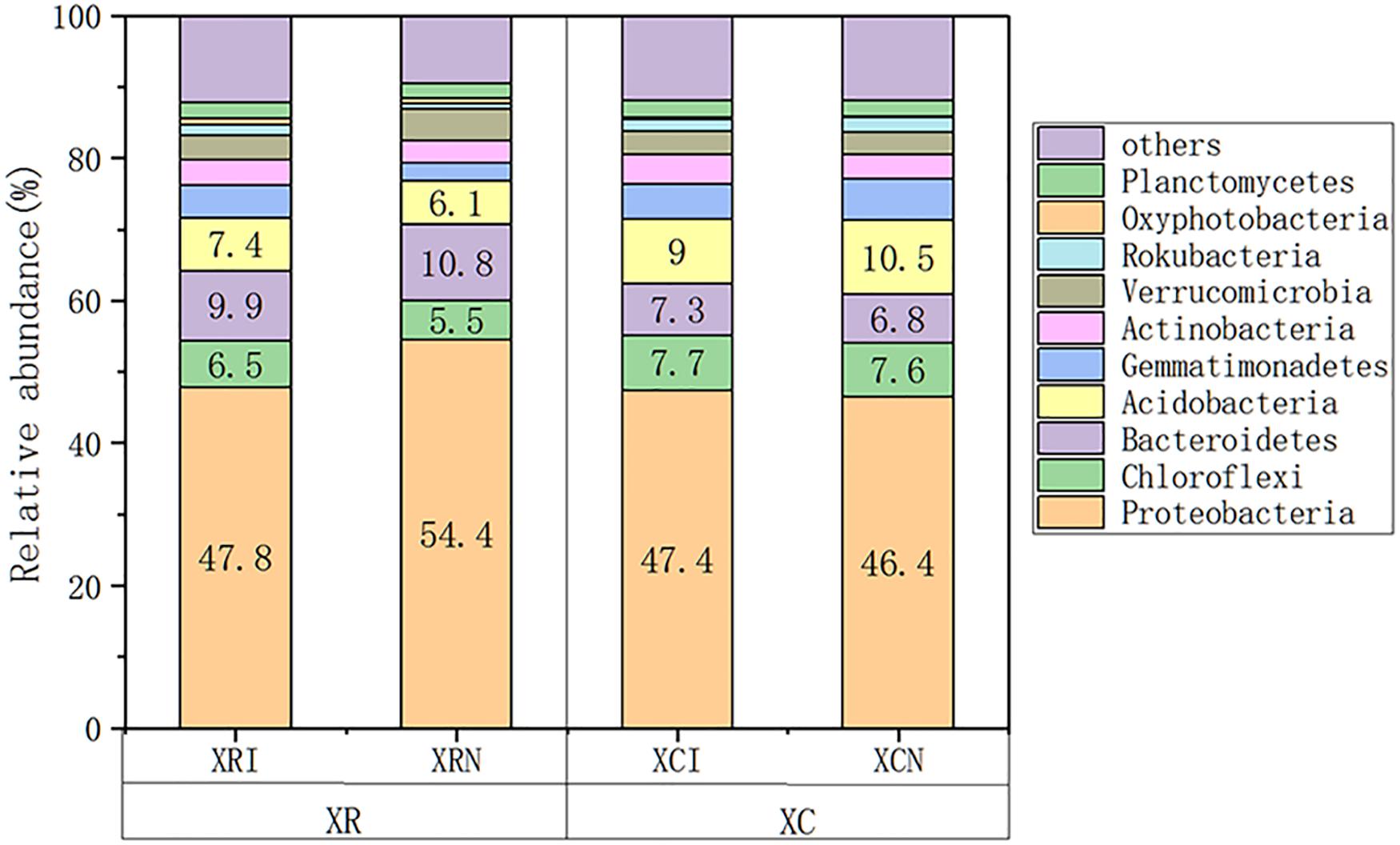
Figure 4. Relative abundance of soil microbial species at invaded sites and non-invaded sites in the two types of wetlands. XRI, Xinxue River invaded sites; XRN, Xinxue River non-invaded sites; XCI, Xinxue River Constructed Wetland invaded sites; XCN, Xinxue River Constructed Wetland non-invaded sites.
The microbial diversity indices (Shannon and Simpson) were significantly lower in XRN than in XRI and XCN (Table 2). The microbial richness indices (Chao1 and ACE) and observed species were also lower in XRN than in XRI and XCN, but there was no significant difference between invaded sites and non-invaded sites in the two types of wetlands. Based on Spearman correlation analysis, the microbial diversity indices had significantly positive correlations with the content and density of SOC and significantly negative correlations with dissolved oxygen, water temperature, and soil temperature.

Table 2. Alpha Diversity analysis index of invaded sites and non-invaded sites of two types of wetlands.
The relationships between microbial community structure and soil properties in the invaded sites and non-invaded sites in the two types of wetlands were revealed by the Redundancy analysis (RDA) (Figure 5). The first two axes explained 34.1% of the total variation in soil microbial community at the phyla-level (Figure 5). The soil microbial community was significantly correlated with dissolved oxygen (P < 0.05), dissolved oxygen explained 19.4% of the variation in the microbial community structure. Meanwhile, there was a clear separation between XR (XRI, XRN) and XC (XCI, XCN). SOC showed a positive relation with the samples in XR, but a negative relation with the samples in XC.
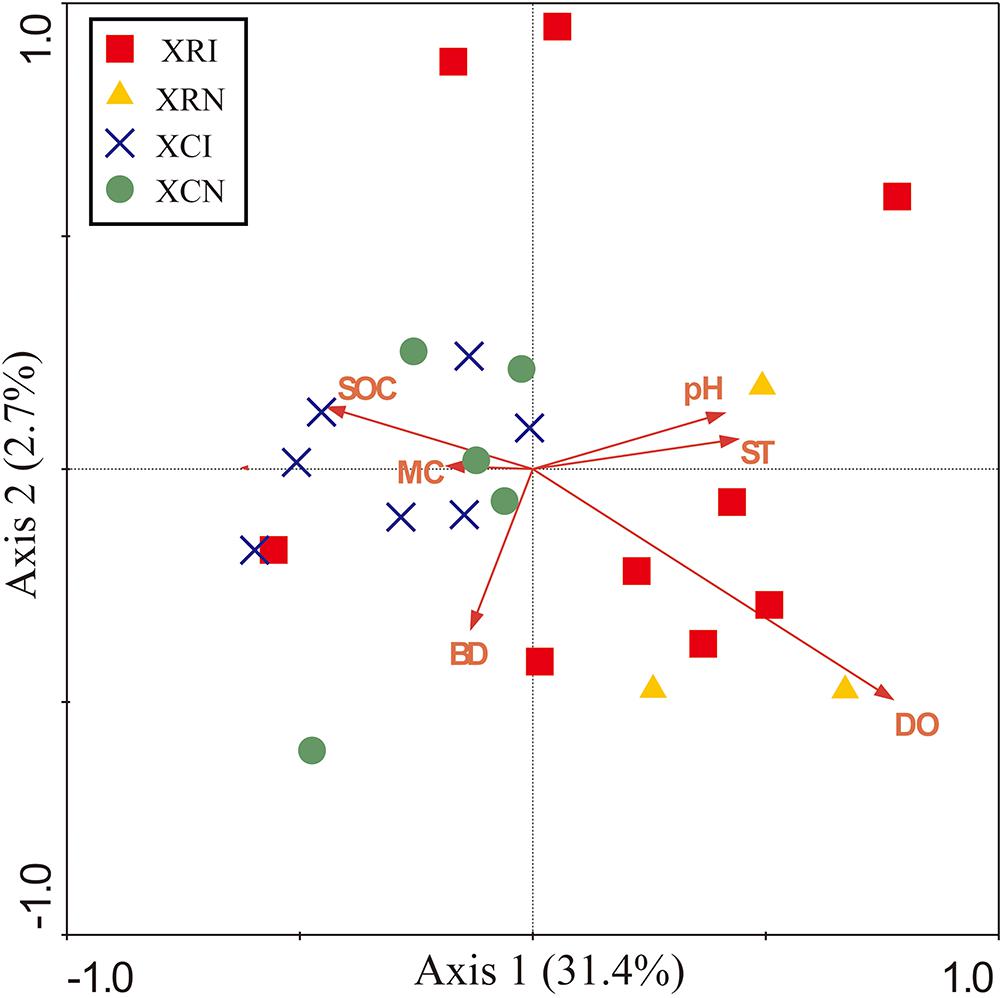
Figure 5. Redundancy analysis (RDA) diagram illustrating the relationships between the soil bacterial community in invaded sites and non-invaded sites in the two types of wetlands and soil physicochemical properties. Axis 1 explained the maximum variation at 31.4%, and Axis 2 explained 2.7%. Points represent the soil samples. XRI, Xinxue River invaded sites; XRN, Xinxue River non-invaded sites; XCI, Xinxue River Constructed Wetland invaded sites; XCN, Xinxue River Constructed Wetland non-invaded sites. Red arrows represent the soil physicochemical properties: pH, SOC, soil organic carbon content; SOCD, soil organic carbon density; MC, moisture content; BD, bulk density; DO, dissolved oxygen; ST, soil temperature.
The RDA results of XR and XC were shown in Figures 6A,B, respectively. In XR, the first two axes explained about 63.1% of the total variation (Figure 6A). The microbial community significantly correlated with bulk density and dissolved oxygen. They respectively explained 23% and 20.5% of the total variation of microbial community structure. The first two axes in XC explained about 53.4% of the total variation (Figure 6B). Microbial community significantly correlated with pH and soil temperature. They respectively explained 19.8 and 15.6% of the total variation in microbial community structure.
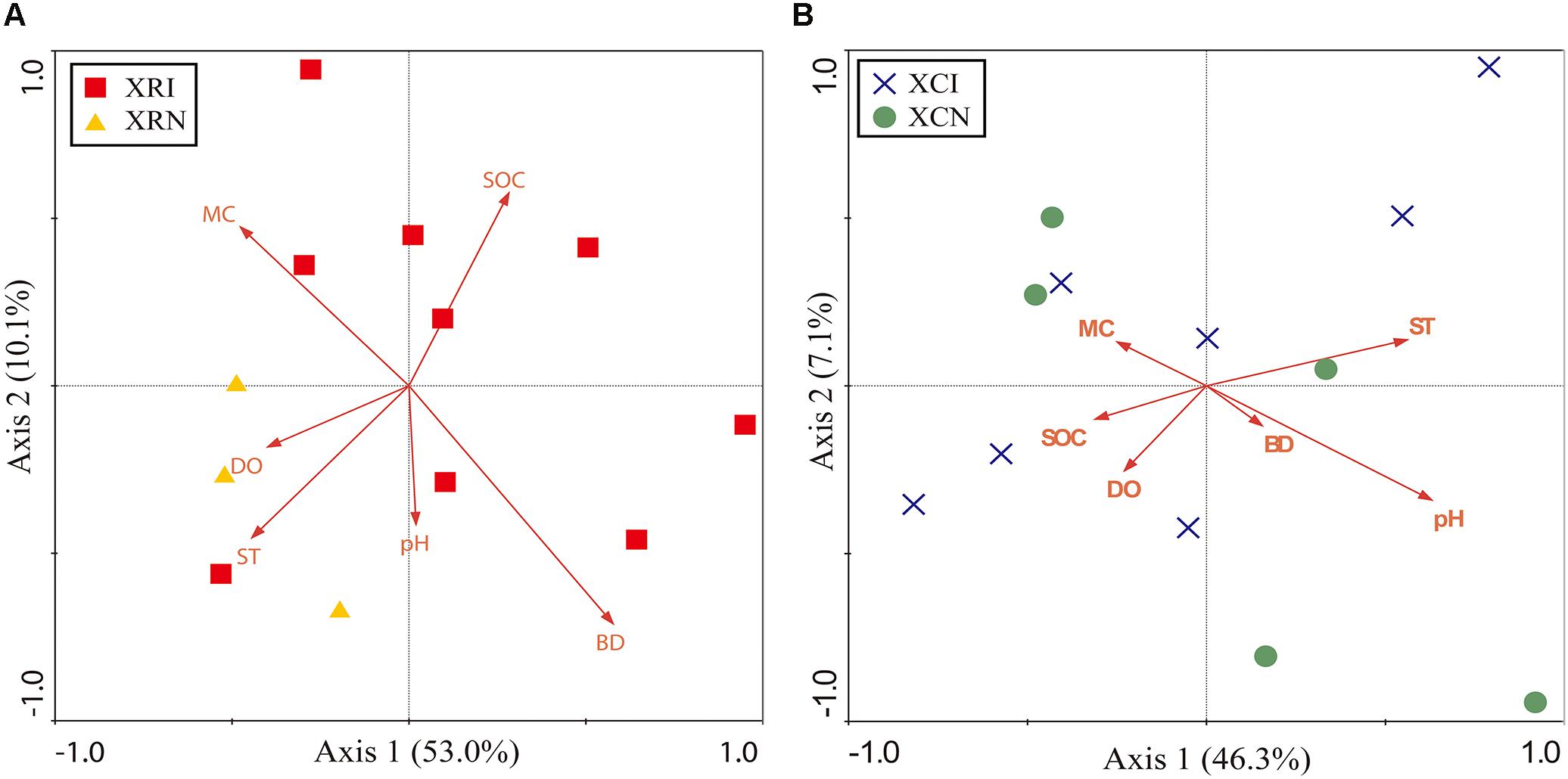
Figure 6. Redundancy analysis (RDA) diagram illustrating the relationship between soil physicochemical properties and the soil bacterial community in XR (A) and XC (B). In XR, Axis 1 explained the maximum variation at 53.0%, and Axis 2 explained 10.1% (A). Axis 1 in XC explained the maximum variation at 46.3%, and Axis 2 explained 7.1% (B). Points represent the soil samples. XRI, Xinxue River invaded sites; XRN, Xinxue River non-invaded sites; XCI, Xinxue River Constructed Wetland invaded sites; XCN, Xinxue River Constructed Wetland non-invaded sites. Red arrows represent the soil physicochemical properties: pH, SOC, soil organic carbon content; SOCD, soil organic carbon density; MC, moisture content; BD, bulk density; DO, dissolved oxygen; ST, soil temperature.
We quantified the indicator species reflecting soil microbial community shifted by plant invasion in XR and XC (Figure 7). In XR, phylum Gemmatimonadetes was enriched in invaded sites (Figure 7A). And 12 bacterial groups were abundant in non-invaded sites of XR, such as the phyla Proteobacteria and Verrucomicrobia. For Proteobacteria, the orders Xanthomonadales and Cellvibrionales, family Xanthomonadaceae, genera Denitratisoma and Arenimonas within the class Gammaproteobacteria and family Haliangiaceae within the class Deltaproteobacteria were more abundant in non-invaded sites than in invaded sites. For Verrucomicrobia, the relative abundance of the class Verrucomicrobiae, the family Rubritaleaceae, and the genus Luteolibacter were higher in XRN than in XRI. In XC, five bacteria lineages were higher in invaded sites than in non-invaded sites, including class Bacilli, families Planococcaceae, Microbacteriaceae, and Sandaracinaceae, and genus Geothermobacter. However, the relative abundance of family unidentified_Solibacterales and genus Bryobacter within the order Solibacterales, family unidentified_Acidobacteriales within the order Acidobacteriales, genus Sphingomonas within the phylum Bacteroidetes, and genus Terrimonas within the phylum Proteobacteria were higher in XCN than in XCI (Figure 7B).
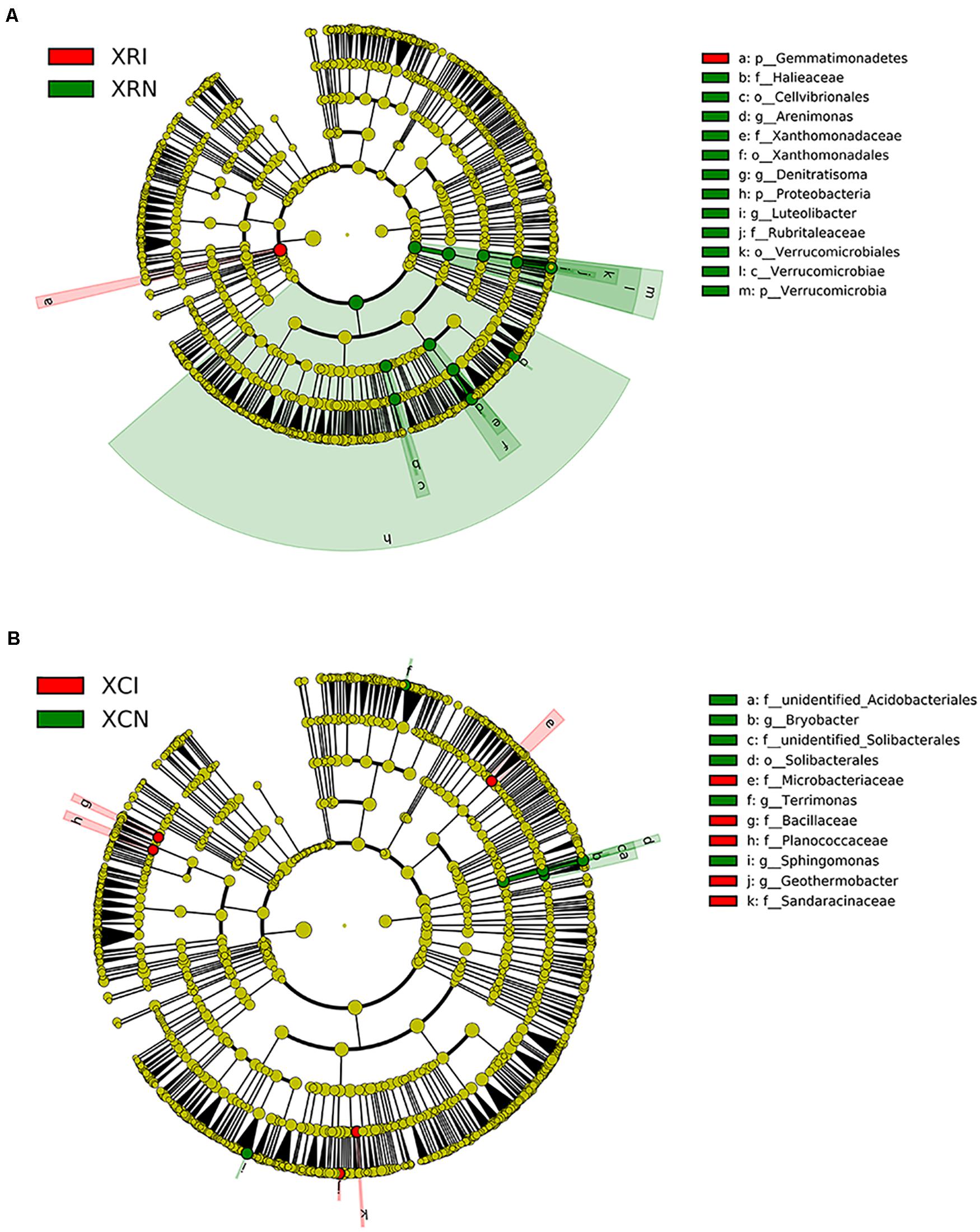
Figure 7. The differences in microbial lineages between invaded sites and non-invaded sites analyzed by linear discriminant analysis effect size (LEfSe) in Xinxue River (A) and Xinxue River Constructed Wetland (B). The cladogram shows the lineages with linear discriminant analysis score higher than 2.0. The different taxonomy units are represented by rings with different diameters. Yellow nodes show no significant difference in invaded sites and non-invaded sites, while red means the higher units in invaded sites and green means the higher units in non-invaded sites. XRI, Xinxue River invaded sites; XRN, Xinxue River non-invaded sites; XCI, Xinxue River Constructed Wetland invaded sites; XCN, Xinxue River Constructed Wetland non-invaded sites.
Spearman correlation analysis showed that the indicator species were highly related with soil properties in XR and XC (Figure 8). In XR, the relative abundance of Gemmatimonadetes enriched in invaded sites had positive correlations with SOC content and density, but negative correlations with dissolved oxygen and soil temperature (Figure 8A). In XR, the species showed negative correlations with SOC content and density and positive correlations with dissolved oxygen and soil temperature, which were more abundant in non-invaded sites than in invaded sites. In XC, the indicator species in invaded sites positively correlated with SOC content and density, but negatively correlated with pH. And the indicator species in non-invaded sites of XC showed negative correlations with the content and density of SOC and moisture content, and positive correlations with pH and bulk density (Figure 8B).
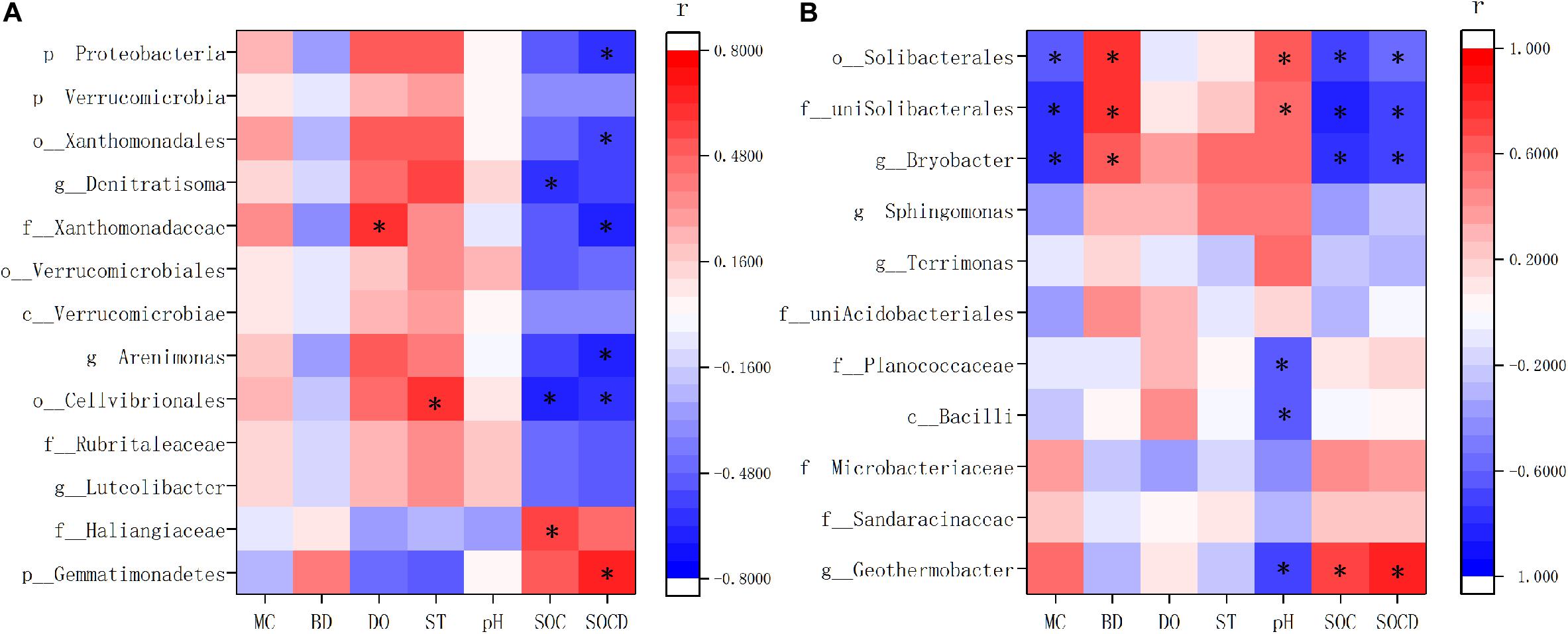
Figure 8. Correlation matrix based on Spearman correlation analysis between soil properties and indicator species in Xinxue River (A) and Xinxue River Constructed Wetland (B). MC, Moisture content; BD, bulk density; DO, dissolved oxygen; ST, soil temperature; SOC, soil organic carbon content; SOCD, soil organic carbon density. The different taxonomy units are represented by: p, phyla; c, class; o, order; f, family; g, genus. *p < 0.05.
Discussion
Previous studies demonstrated that plant invasion greatly impacts the soil carbon cycle (Ehrenfeld, 2003; Drenovsky and Batten, 2007; Tamura and Tharayil, 2014), but the influence mechanism of plant invasion on SOC in wetlands remains unclear. In our study, in both the river and the constructed wetland, A. philoxeroides-invaded sites had higher SOC content and density than non-invaded sites. Our result showed that the plant invasion could increase the content of SOC, which was consistent with previous studies (He et al., 2019; Zhang G. et al., 2019). Invasive plants with high net primary productivity could increase the content of SOC through root exudation and litter decomposition (Craig et al., 2015; Zhou et al., 2015). When the soil nutrients increased, microbes decomposed the fresh organic matter instead of soil organic matter, resulting in the accumulation of SOC (Fontaine et al., 2003). It might be an explanation for the increase of SOC in the river and the constructed wetland with A. philoxeroides invasion.
Plant invasion can indirectly affect the carbon cycle by changing soil physicochemical properties including moisture content, pH, bulk density, dissolved oxygen, and temperature (Huang et al., 2014; Qi et al., 2019; Yang, 2019). In our study, the positive correlations between SOC and moisture content and the negative correlations between SOC and bulk density, dissolved oxygen, water temperature, soil temperature, and pH (Figure 3) conformed with the results of Qi et al. (2019). The effect of A. philoxeroides invasion on the storage of SOC might be related to the alternation of different physicochemical properties in the river and the constructed wetland. In the river, dissolved oxygen was significantly decreased in invaded sites. The negative correlation of SOC with this reduced dissolved oxygen could partially explain the higher content of SOC in invaded sites. A previous study reported that dissolved oxygen could be taken up by microbes for microbial activities (Higashino et al., 2008). The consumption of dissolved oxygen in invaded sites in the river might be due to the increase of the activities and reproduction of microorganisms, which was confirmed by the fact that invaded sites had a higher richness of microbes than non-invaded sites. The decreased dissolved oxygen with plant invasion in the river correlated with the alteration of the microbial community (Figure 6A), which might limit the consumption of SOC by aerobic heterotrophic microorganisms such as Betaproteobacteria (Zhang Y. et al., 2015), and might indirectly result in the accumulation of SOC. Hence, we inferred that in the river invaded by plants, dissolved oxygen might be the dominant factor affecting the consumption of SOC by altering microbial respiration and composition. However, plant invasion in the constructed wetland altered different soil properties. The significant decrease of soil and water temperature might be due to the shielding effect of invasive plants on the water surface (Wang et al., 2009). Lower temperatures might inhibit microbial growth and even alter soil microbial community structure (Supramaniam et al., 2016). In the constructed wetland, soil temperature was related in the variation of microbial community structure (Figure 6B), which could be a reason for the difference in SOC between invaded sites and non-invaded sites. In the river and the constructed wetland, A. philoxeroides invasion could change different soil properties including dissolved oxygen and temperature to affect the storage of SOC indirectly.
Plant invasion can affect soil microorganisms, which act as one of the main factors of SOC formation (Bradford et al., 2013). The root exudates of invasive plants are an important carbon source for soil microbes (Craig et al., 2015). A previous study has shown that plant invasion could elevate soil microbial abundance and diversity (Yang et al., 2019). In our study, A. philoxeroides-invaded sites in the river had higher microbial diversity indices (Shannon and Simpson) than the non-invaded sites, which might be due to the plant invasion. But there was no difference in the microbial diversity in the constructed wetland. The stable microbial diversity indices in the constructed wetland might be because of the compensation from other microbial groups for some microbial variations or the limited impacts of plant invasion on microbial α diversity indices (Custer and van Diepen, 2020). The result of LEfSe showed that the microbial community structure in the river and the constructed wetland was significantly different between the invaded and non-invaded sites (Figure 7). The microbial community structure might be changed by plant invasion. In our study area, the indicator species in A. philoxeroides-invaded sites and non-invaded sites showed positive and negative correlations with SOC. Phylum Gemmatimonadetes, reported to be capable of photosynthetic reaction, served as the indicator species in invaded sites of river (Zeng et al., 2014). The indicator species in non-invaded sites of the river including classes Gammaproteobacteria and Deltaproteobacteria, and family Rubritaleaceae could degrade polysaccharides (Martinez-Garcia et al., 2012; Desta et al., 2014; Rosenberg et al., 2014). By querying KEGG3, the indicator species in invaded sites of the constructed wetland have M00169, M00345, and M00579 metabolic pathways of carbon fixation. However, the indicator species in non-invaded sites including order Acidobacteriales, genera Sphingomonas and Terrimonas have been reported to be able to degrade cellulose and other polysaccharides (Desta et al., 2014; Rosenberg et al., 2014). Order Solibacterales could participate in the mineralization of phosphorus (Bergkemper et al., 2016). The indicator species might be an important factor for the increase of SOC caused by plant invasion.
Furthermore, the results indicate that plant invasion might have a greater impact on SOC in the river than in the constructed wetland. This might be because rivers are vulnerable to plant invasion and constructed wetlands have higher SOC content and density than rivers. The lower content and density of SOC in rivers might be due to physicochemical properties, water velocities, and vegetation coverage (Braskerud, 2001; Cao et al., 2015). Moreover, the positive correlation between SOC and dissolved oxygen in the constructed wetland was different from the river and other studies (Cao et al., 2015), which might be because the roots of invasive plants import root exudates and oxygen into the soil. However, the degrees of plant invasion in the river and the constructed wetland are not experimentally controlled, and the lack of data before and after the plant invasion of the same area is also a limitation of this study. More experimental studies are needed to validate the influence mechanisms of plant invasion on SOC.
Conclusion
The study found that the invasion of A. philoxeroides significantly increased SOC content and density in both the river and the constructed wetland. SOC in the two types of wetlands had positive correlation with moisture content and negative correlations with bulk density, dissolved oxygen, water temperature, soil temperature, and pH. The invasion decreased the dissolved oxygen in the river and the soil temperature in the constructed wetland. It is possible that the alternation of different soil properties in the two types of wetlands with plant invasion resulted in the difference in SOC. With plant invasion, the decrease of dissolved oxygen in the river might alter the microbial community and limit the decomposition of organic carbon by microbes. The increase of microbial α diversity indices and the correlations between soil microbial community in the river and dissolved oxygen verified the effect of plant invasion on SOC. In the constructed wetland, plant invasion only changed the microbial community structure. And RDA analysis showed that the variation in soil microbial community structure in the constructed wetland could be mainly explained by pH and soil temperature. We suggest that the decrease of soil and water temperature in the constructed wetland might inhibit the microbial growth and reduce microbial respiration. Furthermore, the variation in indicator species caused by plant invasion might be related to the difference in SOC, as confirmed by their correlations. This study is of great significance to understand the impact of the invasion of A. philoxeroides and other similar invasive plants on the wetland carbon stock, and provides a foundation for further research on the relationship between global change and plant invasion.
Data Availability Statement
The datasets generated for this study can be found in the online repositories. The names of the repository/repositories and accession number(s) can be found below: https://www.ncbi.nlm.nih.gov/, SRP267962.
Ethics Statement
The sample collection in this study was approved by the Management Committee of the Xinxue River Constructed Wetland.
Author Contributions
KL, RY, and JL: conception and design of study, analysis and interpretation of data. KL and RY: acquisition of data. RY and JL: drafting the manuscript. JF, KL, JL, CZ, and QQ: revising the manuscript critically for important intellectual content. RY, KL, JF, QQ, CZ, and JL: approval of the version of the manuscript to be published. All authors contributed to the article and approved the submitted version.
Funding
This study was supported by the Major Science and Technology Program for Water Pollution Control and Treatment (No. 2017ZX07101003), the National Natural Science Foundation of China (No. 32071523), the Natural Science Foundation of Shaanxi Province (No. 2019JLP-26), and the Natural Science Foundation of Shandong Province (No. ZR2017MC013).
Conflict of Interest
The authors declare that the research was conducted in the absence of any commercial or financial relationships that could be construed as a potential conflict of interest.
Acknowledgments
We would like to thank Lifei Wang for English language editing.
Footnotes
- ^ http://huttenhower.sph.harvard.edu/galaxy/
- ^ https://www.ncbi.nlm.nih.gov/sra/?term=SRP267962
- ^ https://www.genome.jp/kegg/kegg2.html
References
Bainard, L. D., Hamel, C., and Gan, Y. (2016). Edaphic properties override the influence of crops on the composition of the soil bacterial community in a semiarid agroecosystem. Appl. Soil Ecol. 105, 160–168. doi: 10.1016/j.apsoil.2016.03.013
Bergkemper, F., Kublik, S., Lang, F., Krüger, J., Vestergaard, G., Schloter, M., et al. (2016). Novel oligonucleotide primers reveal a high diversity of microbes which drive phosphorous turnover in soil. J. Microbiol. Methods 125, 91–97. doi: 10.1016/j.mimet.2016.04.011
Bobul’ská, L., Demková, L., Čerevková, A., and Renčo, M. (2019). Invasive Goldenrod (Solidago gigantea) influences soil microbial activities in forest and grassland ecosystems in central Europe. Diversity 11:134. doi: 10.3390/d11080134
Boscutti, F., Pellegrini, E., Casolo, V., Nobili, M., Buccheri, M., and Alberti, G. (2020). Cascading effects from plant to soil elucidate how the invasive Amorpha fruticosa L. impacts dry grasslands. J. Veg. Sci. 31, 667–677. doi: 10.1111/jvs.12879
Bradford, M. A., Keiser, A. D., Davies, C. A., Mersmann, C. A., and Strickland, M. S. (2013). Empirical evidence that soil carbon formation from plant inputs is positively related to microbial growth. Biogeochemistry 113, 271–281. doi: 10.1007/s10533-012-9822-0
Braskerud, B. C. (2001). The influence of vegetation on sedimentation and resuspension of soil particles in small constructed wetlands. J. Environ. Qual. 30, 1447–1457. doi: 10.2134/jeq2001.3041447x
Burke, I. C., Lauenroth, W. K., Vinton, M. A., Hook, P. B., Kelly, R. H., Epstein, H. E., et al. (1998). Plant-soil interactions in temperate grasslands. Biogeochemistry 42, 121–143. doi: 10.1007/978-94-017-2691-7_7
Cao, Q., Wang, R., Zhang, H., Ge, X., and Liu, J. (2015). Distribution of organic carbon in the sediments of Xinxue River and the Xinxue River Constructed Wetland, China. PLoS One 10:e134713. doi: 10.1371/journal.pone.0134713
Caporaso, J. G., Kuczynski, J., Stombaugh, J., Bittinger, K., Bushman, F. D., Costello, E. K., et al. (2010). QIIME allows analysis of high-throughput community sequencing data. Nat. Methods 7, 335–336.
Chen, Q., Jian, S., Ma, K., and Chen, P. (2018). Differences in macrobenthic faunal communities in mangrove wetland habitats (Zhanjiang, China) invaded and non-invaded by exotic cordgrass Spartina alterniflora. Ecol. Res. 33, 1113–1123. doi: 10.1007/s11284-018-1624-y
Craig, M. E., Pearson, S. M., and Fraterrigo, J. M. (2015). Grass invasion effects on forest soil carbon depend on landscape-level land use patterns. Ecology 96, 2265–2279. doi: 10.1890/14-1770.1
Custer, G. F., and van Diepen, L. T. A. (2020). Plant invasion has limited impact on soil microbial α-diversity: a meta-analysis. Diversity 12:112. doi: 10.3390/d12030112
Desta, A. F., Assefa, F., Leta, S., Stomeo, F., Wamalwa, M., Njahira, M., et al. (2014). Microbial community structure and diversity in an integrated system of anaerobic-aerobic reactors and a constructed wetland for the treatment of tannery wastewater in Modjo, Ethiopia. PLoS One 9:e115576. doi: 10.1371/journal.pone.0115576
Dixon, R. K., and Krankina, O. N. (1995). “Can the terrestrial biosphere be managed to conserve and sequester carbon?,” in Carbon Sequestration in the Biosphere. NATO ASI Series (Series I: Global Environmental Change, Vol. 33, ed. M. A. Beran (Berlin: Springer), 153–179. doi: 10.1007/978-3-642-79943-3_9
Drenovsky, R. E., and Batten, K. M. (2007). Invasion by Aegilops triuncialis (Barb Goatgrass) slows carbon and nutrient cycling in a serpentine grassland. Biol. Invas. 9, 107–116. doi: 10.1007/s10530-006-0007-4
Ehrenfeld, J. G. (2003). Effects of exotic plant invasions on soil nutrient cycling processes. Ecosystems 6, 503–523. doi: 10.1007/s10021-002-0151-3
Fang, J., Zhao, R., Cao, Q., Quan, Q., Sun, R., and Liu, J. (2019). Effects of emergent aquatic plants on nitrogen transformation processes and related microorganisms in a constructed wetland in northern China. Plant Soil 443, 473–492. doi: 10.1007/s11104-019-04249-w
Fontaine, S., Mariotti, A., and Abbadie, L. (2003). The priming effect of organic matter: a question of microbial competition? Soil Biol. Biochem. 35, 837–843. doi: 10.1016/s0038-0717(03)00123-8
Hawkins, C. F., and Smith, O. E. (1983). The convention on wetlands of international importance especially as waterfowl habitat. Environ. Policy Law 10, 70–71. doi: 10.1016/s0378-777x(83)80165-6
He, Y., Zhou, X., Cheng, W., Zhou, L., Zhang, G., Zhou, G., et al. (2019). Linking improvement of soil structure to soil carbon storage followinginvasion by a C4 plant Spartina alterniflora. Ecosystems 22, 859–872. doi: 10.1007/s10021-018-0308-3
Higashino, M., O’Connor, B. L., Hondzo, M., and Stefan, H. G. (2008). Oxygen transfer from flowing water to microbes in an organic sediment bed. Hydrobiologia 614, 219–231. doi: 10.1007/s10750-008-9508-8
Huang, X., Liu, S., Wang, H., Hu, Z., Li, Z., and You, Y. (2014). Changes of soil microbial biomass carbon and community composition through mixing nitrogen-fixing species with Eucalyptus urophylla in subtropical China. Soil Biol. Biochem. 73, 42–48. doi: 10.1016/j.soilbio.2014.01.021
Hui, Y., Zhang, R., and Li, X. (2009). Classification of wetland from TM imageries based on decision tree. Wseas Trans. Inform. Sci. Appl. 6, 1155–1164.
Jiao, S., Liu, Z., Lin, Y., Yang, J., Chen, W., and Wei, G. (2016). Bacterial communities in oil contaminated soils: biogeography and co-occurrence patterns. Soil Biol. Biochem. 98, 64–73. doi: 10.1016/j.soilbio.2016.04.005
Jin, B., Lai, D. Y. F., Gao, D., Tong, C., and Zeng, C. (2017). Changes in soil organic carbon dynamics in a native C4 plant-dominated tidal marsh following Spartina alterniflora invasion. Pedosphere 27, 856–867. doi: 10.1016/s1002-0160(17)60396-5
Lazzaro, L., Giuliani, C., Fabiani, A., Agnelli, A. E., Pastorelli, R., Lagomarsino, A., et al. (2014). Soil and plant changing after invasion: the case of Acacia dealbata in a Mediterranean ecosystem. Sci. Total Environ. 49, 491–498. doi: 10.1016/j.scitotenv.2014.08.014
Li, C., and Zheng, L. (2019). Influencing factors on measurement accuracy of soil water by ring knife method (in Chinese). Bull. Soil Water Conserv. 039, 118–123.
Liu, X., Li, J., Yu, L., Pan, H., Liu, H., Liu, Y., et al. (2018). Simultaneous measurement of bacterial abundance and composition in response to biochar in soybean field soil using 16S rRNA gene sequencing. Land Degrad. Dev. 29, 2172–2182. doi: 10.1002/ldr.2838
Lorenzo, P., Rodríguez-Echeverría, S., González, L., and Freitas, H. (2010). Effect of invasive Acacia dealbata Link on soil microorganisms as determined by PCR-DGGE. Appl. Soil Ecol. 44, 245–251. doi: 10.1016/j.apsoil.2010.01.001
Martinez-Garcia, M., Brazel, D. M., Swan, B. K., Arnosti, C., and Chain, P. S. G. (2012). Capturing single cell genomes of active polysaccharide degraders: an unexpected contribution of Verrucomicrobia. PLoS One 7:e35314. doi: 10.1371/journal.pone.035314
McKinley, D. C., and Blair, J. M. (2008). Woody plant encroachment by Juniperus virginiana in a mesic native grassland promotes rapid carbon and nitrogen accrual. Ecosystems 11, 454–468. doi: 10.1007/s10021-008-9133-4
Mitsch, W. J., Bernal, B., Nahlik, A. M., Mander, Ü, Zhang, L., Anderson, C. J., et al. (2013). Wetlands, carbon, and climate change. Landsc. Ecol. 28, 583–597.
Nag, S. K., Liu, R., and Lal, R. (2017). Emission of greenhouse gases and soil carbon sequestration in a riparian marsh wetland in central Ohio. Environ. Monit. Assess. 189, 580.1–580.12.
Nag, S. K., Nandy, S. K., Roy, K., Sarkar, U. K., and Das, B. K. (2019). Carbon balance of a sewage-fed aquaculture wetland. Wetlands Ecol. Manag. 27, 311–322. doi: 10.1007/s11273-019-09661-8
Nguyen, L. T. T., Osanai, Y., Lai, K., Anderson, I. C., Bange, M. P., Tissue, D. T., et al. (2018). Responses of the soil microbial community to nitrogen fertilizer regimes and historical exposure to extreme weather events: flooding or prolonged-drought. Soil Biol. Biochem. 118, 227–236. doi: 10.1016/j.soilbio.2017.12.016
Nitao, J. K. (1989). Enzymatic adaption in a specialist herbivore for feeding on furanocoumarin- containing. Ecology 70, 629–635. doi: 10.2307/1940214
Pang, X., Geng, Y., Sosa, A., Zhang, W., Li, B., and Chen, J. (2007). Invasive plant Alternanthera philoxeroides: biology, ecology and management (in Chinese). Acta Phytotaxon. Sin. 45, 884–900. doi: 10.1360/aps06134
Qi, X., Liu, H., Lin, Z., Liu, X., and Gong, H. (2019). Impacts of age and expansion direction of invasive Spartina alterniflora on soil organic carbon dynamics in coastal salt marshes along eastern China. Estuar. Coasts 42, 1858–1867. doi: 10.1007/s12237-019-00611-4
Ramsar Convention Secretariat (2004). The Ramsar Convention Manual: a Guide to the Convention on Wetlands (Ramsar, Iran, 1971). 3rd Edn, Gland: Ramsar Convention Secretariat.
Reddy, G. B., Raczkowski, C. W., Cyrus, J. S., and Szogi, A. (2016). Carbon sequestration in a surface flow constructed wetland after 12 years of swine wastewater treatment. Water Sci. Technol. 73, 2501–2508. doi: 10.2166/wst.2016.112
Rosenberg, E., DeLong, E. F., Lory, S., Stackebrandt, E., and Thompson, F. (2014). The Prokaryotes. Berlin: Springer.
Rudiyanto, Minasny, B., and Setiawan, B. I. (2016). Further results on comparison of methods for quantifying soil carbon in tropical peats. Geoderma 269, 108–111. doi: 10.1016/j.geoderma.2016.01.038
Seebens, H., Blackburn, T. M., Dyer, E. E., Genovesi, P., Hulme, P. E., Jeschke, J. M., et al. (2017). No saturation in the accumulation of alien species worldwide. Nat. Commun. 8:14435.
Song, S., Zhang, C., Gao, Y., Zhu, X., Wang, R., Wang, M., et al. (2020). Responses of wetland soil bacterial community and edaphic factors to two-year experimental warming and Spartina alterniflora invasion in Chongming Island. J. Clean. Product. 250:119502. doi: 10.1016/j.jclepro.2019.119502
Stewart, C. N., and Via, L. E. (1993). A rapid CTAB DNA isolation technique useful for RAPD fingerprinting and other PCR applications. Biotechniques 14, 748–750.
Supramaniam, Y., Chong, C. W., Silvaraj, S., and Tan, K. P. (2016). Effect of short term variation in temperature and water content on the bacterial community in a tropical soil. Appl. Soil Ecol. 107, 279–289. doi: 10.1016/j.apsoil.2016.07.003
Tamura, M., and Tharayil, N. (2014). Plant litter chemistry and microbial priming regulate the accrual, composition and stability of soil carbon in invaded ecosystems. New Phytol. 203, 110–124. doi: 10.1111/nph.12795
Telesnicki, M., Sosa, A., Greizerstein, E., and Julien, M. (2011). Cytogenetic effect of Alternanthera philoxeroides (alligator weed) on Agasicles hygrophila (Coleoptera: Chrysomelidae) in its native range. Biol. Control. 57, 138–142. doi: 10.1016/j.biocontrol.2011.02.003
van der Putten, W. H., Klironomos, J. N., and Wardle, D. A. (2007). Microbial ecology of biological invasions. ISME J. 1, 28–37.
Vaz, A. S., Alcaraz-Segura, D., Vicente, J. R., and Honrado, J. P. (2019). The many roles of remote densing in invasion science. Front. Ecol. Evol. 7:370.
Wang, Y., Bao, Z., Zhu, Y., and Hua, J. (2009). Analysis of temperature modulation of plant defense against biotrophic microbes. Mol. Plant Microb. Interact. 22, 498–506. doi: 10.1094/mpmi-22-5-0498
Wu, W., Chen, Y., Faisal, S., Khan, A., Chen, Z., Ling, Z., et al. (2016). Improving methane production in cow dung and corn straw co-fermentation systems via enhanced degradation of cellulose by cabbage addition. Sci. Rep. 6:33628.
Wu, Z., Mu, J., Xie, G., Lu, C., and Zhu, J. (2010). Analysis of water environmental quality variation trends in Nansi Lake and its joined rivers. Res. Environ. Sci. 23, 1167–1173.
Xin, X., and Qin, J. (2019). Rapid start-up of partial nitritation in aerobic granular sludge bioreactor and the analysis of bacterial community dynamics. Bioprocess Biosyst. Eng. 42, 1973–1981. doi: 10.1007/s00449-019-02190-x
Yang, R. (2019). Mechanisms of soil organic carbon storage response to Spartina alterniflora invasion and climate change. Sci. Total Environ. 690, 7–15. doi: 10.1016/j.scitotenv.2019.06.472
Yang, W., Cai, A., Wang, J., Luo, Y., Cheng, X., and An, S. (2020). Exotic Spartina alterniflora Loisel. invasion significantly shifts soil bacterial communities with the successional gradient of saltmarsh in eastern China. Plant Soil 449, 97–115. doi: 10.1007/s11104-020-04470-y
Yang, W., Jeelani, N., Zhu, Z., Luo, Y., Cheng, X., and An, S. (2019). Alterations in soil bacterial community in relation to Spartina alterniflora Loisel. invasion chronosequence in the eastern Chinese coastal wetlands. Appl. Soil Ecol. 135, 38–43. doi: 10.1016/j.apsoil.2018.11.009
Yang, W., Qiao, Y., Li, N., Zhao, H., Yang, R., Leng, X., et al. (2017). Seawall construction alters soil carbon and nitrogen dynamics and soil microbial biomass in an invasive Spartina alterniflora salt marsh in eastern China. Appl. Soil Ecol. 110, 1–11. doi: 10.1016/j.apsoil.2016.11.007
Yang, W., Zhao, H., Chen, X., Yin, S., Cheng, X., and An, S. (2013). Consequences of short-term C4 plant Spartina alterniflora invasions for soil organic carbon dynamics in a coastal wetland of Eastern China. Ecol. Eng. 61, 50–57. doi: 10.1016/j.ecoleng.2013.09.056
Zeng, Y., Feng, F., Medova, H., Dean, J., and Kobli, E. M. (2014). Functional type 2 photosynthetic reaction centers found in the rare bacterial phylum Gemmatimonadetes. Proc. Natl. Acad. Sci. U.S.A. 111, 7795–7800. doi: 10.1073/pnas.1400295111
Zhang, D., Gersberg, R. M., and Tan, S. K. (2009). Constructed wetlands in China. Ecol. Eng. 35, 1367–1378.
Zhang, G., Bai, J., Jia, J., Wang, W., Wang, X., Zhao, Q., et al. (2019). Shifts of soil microbial community composition along a short-term invasion chronosequence of Spartina alterniflora in a Chinese estuary. Sci. Total Environ. 657, 222–233. doi: 10.1016/j.scitotenv.2018.12.061
Zhang, G., Bai, J., Zhao, Q., Jia, J., Wang, W., and Wang, X. (2020). Bacterial succession in salt marsh soils along a short-term invasion chronosequence of Spartina alterniflora in the Yellow River Estuary, China. Microb. Ecol. 79, 644–661. doi: 10.1007/s00248-019-01430-7
Zhang, H., Goncalves, P., Copeland, E., Qi, S., Dai, Z., Li, G., et al. (2020). Invasion by the weed Conyza canadensis alters soil nutrient supply and shifts microbiota structure. Soil Biol. Biochem. 143:107739. doi: 10.1016/j.soilbio.2020.107739
Zhang, L., Qin, X., Liu, P., Huang, Q., Lan, F., and Ji, H. (2015). Estimation of carbon sink fluxes in the Pearl River basin (China) based on a water-rock-gas-organism interaction model. Environ. Earth Sci. 74, 945–952. doi: 10.1007/s12665-014-3788-2
Zhang, P., Scheu, S., Li, B., Lin, G., Zhao, J., and Wu, J. (2020). Litter C transformations of invasive Spartina alterniflora affected by litter type and soil source. Biol. Fertil. Soils 56, 369–379. doi: 10.1007/s00374-019-01429-9
Zhang, Y., Wang, L., Hu, Y., Xi, X., Tang, Y., Chen, J., et al. (2015). Water organic pollution and eutrophication influence soil microbial processes, increasing soil respiration of estuarine wetlands: site study in Jiuduansha Wetland. PLoS One 10:e0126951. doi: 10.1371/journal.pone.0126951
Zhao, G., Ye, S., Li, G., Yu, X., and McClellan, S. A. (2017). Soil organic carbon storage changes in coastal wetlands of the Liaohe Delta, China, based on landscape patterns. Estuar. Coasts 40, 967–976. doi: 10.1007/s12237-016-0194-x
Keywords: Alternanthera philoxeroides, invasion, river, constructed wetland, soil organic carbon, dissloved oxygen, soil temperature, icrobial community
Citation: Yang R, Li K, Fang J, Quan Q, Zhang C and Liu J (2020) The Invasion of Alternanthera philoxeroides Increased Soil Organic Carbon in a River and a Constructed Wetland With Different Mechanisms. Front. Ecol. Evol. 8:574528. doi: 10.3389/fevo.2020.574528
Received: 20 June 2020; Accepted: 22 September 2020;
Published: 06 November 2020.
Edited by:
Bin Zhu, University of Hartford, United StatesCopyright © 2020 Yang, Li, Fang, Quan, Zhang and Liu. This is an open-access article distributed under the terms of the Creative Commons Attribution License (CC BY). The use, distribution or reproduction in other forums is permitted, provided the original author(s) and the copyright owner(s) are credited and that the original publication in this journal is cited, in accordance with accepted academic practice. No use, distribution or reproduction is permitted which does not comply with these terms.
*Correspondence: Jian Liu, ZWNvbG9neUBzZHUuZWR1LmNu