- Department of Biological Sciences, California State University Los Angeles, Los Angeles, CA, United States
The evolution of avian flight is one of the great transformations in vertebrate history, marked by striking anatomical changes that presumably help meet the demands of aerial locomotion. These changes did not occur simultaneously, and are challenging to decipher. Although extinct theropods are most often compared to adult birds, studies show that developing birds can uniquely address certain challenges and provide powerful insights into the evolution of avian flight: unlike adults, immature birds have rudimentary, somewhat “dinosaur-like” flight apparatuses and can reveal relationships between form, function, performance, and behavior during flightless to flight-capable transitions. Here, we focus on the musculoskeletal apparatus and use CT scans coupled with a three-dimensional musculoskeletal modeling approach to analyze how ontogenetic changes in skeletal anatomy influence muscle size, leverage, orientation, and corresponding function during the development of flight in a precocial ground bird (Alectoris chukar). Our results demonstrate that immature and adult birds use different functional solutions to execute similar locomotor behaviors: in spite of dramatic changes in skeletal morphology, muscle paths and subsequent functions are largely maintained through ontogeny, because shifts in one bone are offset by changes in others. These findings help provide a viable mechanism for how extinct winged theropods with rudimentary pectoral skeletons might have achieved bird-like behaviors before acquiring fully bird-like anatomies. These findings also emphasize the importance of a holistic, whole-body perspective, and the need for extant validation of extinct behaviors and performance. As empirical studies on locomotor ontogeny accumulate, it is becoming apparent that traditional, isolated interpretations of skeletal anatomy mask the reality that integrated whole systems function in frequently unexpected yet effective ways. Collaborative and integrative efforts that address this challenge will surely strengthen our exploration of life and its evolutionary history.
Introduction
Locomotion is an integral element in the lives of most vertebrates, and reconstructing locomotor behaviors in fossil taxa is crucial for understanding many evolutionary transitions. Form-function relationships in extant organisms are key to deciphering anatomical features in extinct organisms and fundamental to this process. For example, attributes such as posture, muscle morphology, and potential joint movements and corresponding locomotor behaviors can be inferred, to varying degrees, based on physical principles and relationships derived from extant homologs or analogs (Hutchinson and Garcia, 2002; Jasinoski et al., 2006; Pierce et al., 2012; Brassey et al., 2017; Otero et al., 2017). However, most fossils lack a living counterpart with an identical suite of morphological features, and are often characterized by mosaics of ancestral and derived anatomical structures. Assessing locomotor capacity in light of these differences is challenging. Although techniques such as musculoskeletal modeling can account for anatomical differences, all methods of inferring functional attributes of extinct organisms must be validated—for example, by constructing models of extant organisms to establish that inferred characteristics, like muscle function or locomotor capacity, match known values (Hutchinson, 2011). Understanding the functional implications of evolutionary transformations therefore requires a thorough exploration of form-function relationships in extant organisms.
One of evolution's great and puzzling transformations is the acquisition of flight in the theropod-avian lineage. Flight is the most power-demanding mode of locomotion (Alexander, 2002), and its evolution has culminated in a suite of specializations that presumably are adaptations or exaptations (Gould and Vrba, 1982) for meeting aerial challenges. The most conspicuous of these specializations—large wings with asymmetrical primary feathers and a robust pectoral skeleton anchoring large flight muscles—are generally present in volant birds and absent in flightless ones, and have long been viewed as hallmarks of flight capacity. However, these hallmarks did not evolve simultaneously. Feathers appeared very early in the theropod lineage, initially as down-like filaments (Coelurosauria or earlier) that were later complemented by pennaceous feathers arranged as “protowings” on the distal forelimb (Pennaraptora). Larger and more bird-like “wings” appeared among paravians, and fully modern feathers were probably present in ornithurines (Norell and Xu, 2005; Foth et al., 2014; Godefroit et al., 2014; Lefèvre et al., 2020; Rauhut and Foth, 2020; Xu, 2020). In contrast to this early (albeit complex) emergence of feathers, changes in the skeletal apparatus occurred much later. For example, Microraptor, Archaeopteryx, and many other paravians and early avialans had relatively bird-like wings yet lacked the robust pectoral apparatus and specialized forelimb of extant volant birds (Ostrom, 1976; Xu et al., 2003; Wellnhofer, 2009; Zheng et al., 2014; Mayr, 2017) (but see Nudds and Dyke, 2010; Longrich et al., 2012; Nudds, 2014, for discussion of feather morphology). Theropod fossils thus reveal that bird-like wings appeared before bird-like skeletons (Dececchi et al., 2016), and deciphering the functional attributes of these unique, “transitional” fossils is extremely challenging.
Although extinct theropods like Archaeopteryx have most often been compared to extant flight-capable or flightless adult birds, a growing number of studies demonstrate that developing birds can uniquely address certain challenges and provide powerful insights into the evolution of avian flight (Dial, 2003; Heers and Dial, 2012; Heers et al., 2014, 2016). Unlike adult birds, immature birds have rudimentary, somewhat “dinosaur-like” wings coupled with less developed pectoral girdles, and rely on unique pre-flight behaviors that bridge the transition from leg-based terrestrial locomotion to wing-based aerial locomotion (Figure 1). For example, developing birds recruit their growing wings to flap-run up inclines [wing-assisted incline running, i.e., WAIR (Dial, 2003)], improve jumping performance (Heers and Dial, 2015), slow aerial descents (Dial et al., 2008), and/or swim to safety (Dial and Carrier, 2012). Though the skeletons of these immature birds are not identical to those of extinct theropods, many of the skeletal features that changed during the evolution of flight change in similar fashion during the development of flight. For instance, preliminary observations suggest that in both cases, elements of the pectoral girdle (sternum, coracoid, scapula, furcula) increase in relative size and/or change orientation, while the humerus acquires a more complex shape due to enlargement of muscle attachment sites and deflection of the humeral head (Ostrom et al., 1999; Heers and Dial, 2012; Mayr, 2017; Rauhut et al., 2019; Serrano et al., 2020). Such changes might be expected to influence locomotion by affecting muscle size, leverage (moment arm length), and orientation (Hutchinson and Garcia, 2002; Hutchinson et al., 2005; Jasinoski et al., 2006; Brassey et al., 2017; Otero et al., 2017), as well as flapping kinematics and range of motion (Jenkins, 1993; Pierce et al., 2012). By examining how skeletal ontogeny relates to muscle function and wing movement in developing birds, we may therefore gain insight into the functional implications of similar skeletal changes that occurred during the evolution of flight. In short, developing birds can uniquely contribute to our understanding of avian evolution by revealing relationships between form, function, performance, and behavior during flightless to flight-capable transitions.
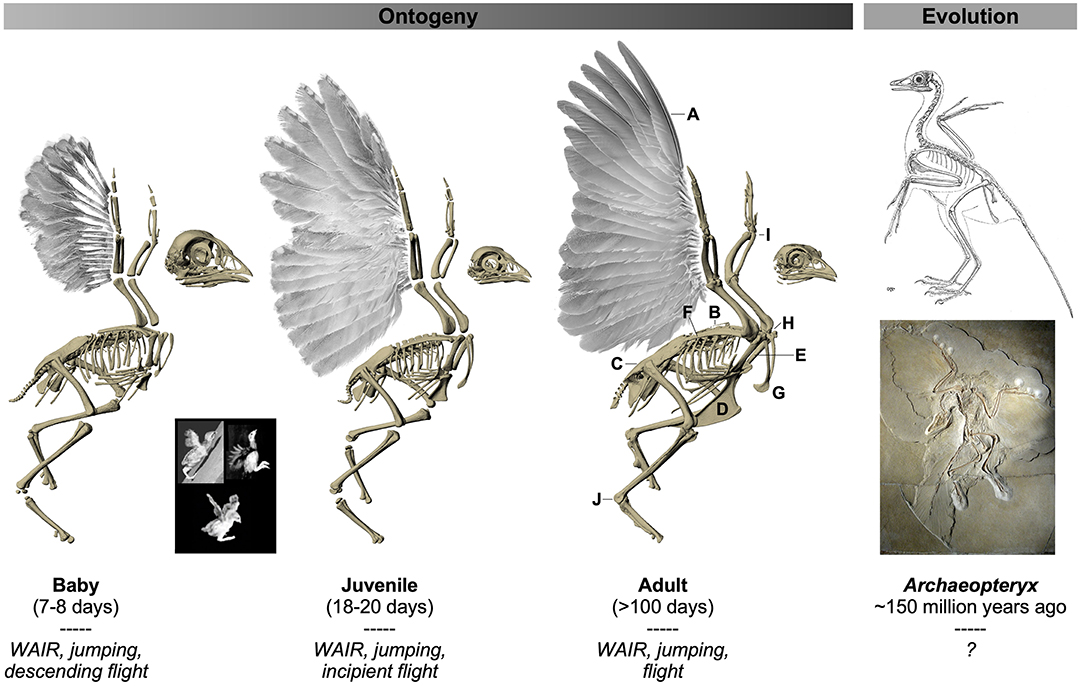
Figure 1. Ontogeny and evolution of the avian flight apparatus. Flight-capable adult birds are characterized by a suite of anatomical features that presumably are adaptations or exaptations for flight. Some of these structures are functionally intuitive, whereas others have no known or demonstrated function(s) but are considered aptations based on their presence in flight-capable birds and their absence in flightless birds and extinct theropods. (A) Stiff, cohesive, and asymmetrical (primaries only) feathers presumably provide stability and reduce permeability for aerodynamic force production (Norberg, 1985; Muller and Patone, 1998; Nudds and Dyke, 2010; Heers et al., 2011; Dial et al., 2012). Fused vertebrae are thought to stabilize the trunk while transferring wing- or leg-generated forces to the body (notarium, B), and/or absorb shock (synsacrum, C) (Ostrom, 1976, 1979; Bock, 1986; Kisia, 2011). A robust flight apparatus [e.g., keeled sternum (D), long and firmly-articulated coracoids (E), long scapulae (F), furcula (G)] permits the attachment and contraction of large, powerful muscles (e.g., pectoralis, supracoracoideus) (George and Berger, 1966; Ostrom, 1974, 1976, 1979, 1986; Bock, 1986), while the triosseal canal (H) (not visible, but present in all age classes shown) allows the supracoracoideus muscle to function similarly to a pulley and elevate and rotate the wing during upstroke (Walker, 1972; Ostrom, 1976, 1979; Rayner, 1988; Norberg, 1990; Poore et al., 1997; Ostrom et al., 1999). Reduced and fused elements in the distal limbs, coupled with channelized joints (I,J), likely reduce weight and facilitate swift limb oscillation, help coordinate joint movements, and restrict joint motion to keep the wing in a planar orientation during downstroke, or the ankle confined to movements in the direction of motion (Ostrom, 1974, 1976, 1979; Coombs, 1978; Vazquez, 1992). Unlike adult birds, developing birds and early winged dinosaurs like Archaeopteryx lack many of these flight aptations: their wings are smaller and/or less aerodynamically effective, and their skeletons are more gracile and less constrained (Heers et al., 2011, 2014; Heers and Dial, 2012). Immature birds nevertheless recruit their rudimentary wings during a variety of locomotor behaviors [italicized text, and inset: wing-assisted incline running, i.e., WAIR (top left); wing-assisted jumping (top right), varying degrees of flight (bottom)], and achieve flight capacity long before flight aptations are fully acquired (Dial, 2003; Dial et al., 2008, 2015; Dial and Carrier, 2012; Heers and Dial, 2015; Heers et al., 2016). Images of Chukar Partridges modified, with permission, from Heers and Dial (2012) and Heers et al. (2018); images of Archaeopteryx (top: by Gerhard Heilmann (Heilmann, 1926); bottom: by H. Raab, licensed under CC BY-SA 3.0) in public domain. Not to scale.
Previous work on form-function relationships has tended to focus on anatomical structures in isolation [e.g., wings (Burgers and Chiappe, 1999; Nudds and Dyke, 2010), tails (Gatesy and Dial, 1996), muscles (Poore et al., 1997), joints (Jenkins, 1993)], and often from a static, two-dimensional perspective. Such studies have provided valuable information and a strong foundation for further analyses. However, locomotion is a highly dynamic, three-dimensional behavior that involves the entire body. In order to build upon previous work and gain more quantitative insight into the functional implications of skeletal evolution, techniques that provide a more dynamic, holistic, and three-dimensional perspective are required.
Here, we use CT scans coupled with a three-dimensional musculoskeletal modeling approach to analyze how ontogenetic changes in skeletal anatomy—the pectoral girdle and humerus—influence muscle size, leverage, orientation, and locomotor function during the development of flight in a precocial ground bird, the Chukar Partridge (Alectoris chukar). Chukars are a well-studied model system for exploring locomotor ontogeny. At hatching, chicks have somewhat “dinosaur-like” anatomy and are entirely dependent on leg-based locomotion, but within a few days they begin to recruit their wings to jump and to flap-run up inclines (WAIR), then control their descent back down (Figure 1). As their anatomy becomes more bird-like and wing performance improves, chukars are able to flap-run up steeper slopes, jump higher, and eventually fly (Dial, 2003; Dial et al., 2006, 2008; Tobalske and Dial, 2007; Heers et al., 2011, 2016; Heers and Dial, 2012, Dial, 2015). This study focuses on three age classes (7–8 days, herein “baby”: wings used for WAIR, jumping, and descending flight; 18–20 days, herein “juvenile”: wings used for WAIR, jumping, and incipient flight; >100 days, herein “adult”: wings used for WAIR, jumping, and flight) and builds upon previous work to quantitatively explore relationships between skeletal anatomy and muscle morphology and function during flightless to flight-capable transitions.
Materials and Methods
Skeletal Reconstructions, Scaling, and Alignment
We imported image slices from previously collected CT scans of baby, juvenile, and adult chukars (1 bird per age class) (Heers et al., 2016, 2018) into Mimics software (Materialise, Inc.; Leuven, Belgium) and used density thresholds to isolate skeletal elements of the pectoral apparatus and forelimb. The sternum, coracoids, scapulae, furcula, and humeri for each bird were then exported as STL files and imported into 3-matic (Materialise, Inc.; Leuven, Belgium) for analysis. Because birds varied substantially in size (34.6 g baby → 500 g adult) and were scanned in different positions, we scaled and aligned each specimen prior to analysis. Immature (baby and juvenile) birds were scaled to adult size by using the “Measure Distance” tool in 3-matic to measure the length of the notarium (i.e., summed length of the bodies of the thoracic vertebrae that fuse into the notarium) and calculate scale factors, which were checked by overlaying the notaria of the scaled baby and juvenile specimens and confirming that their scaled sizes matched that of the adult. We chose to scale by notarium length partially because the vertebral body components are completely ossified in immature birds (and thus completely visible in CT data), and partially because notarium length provides a proxy for body size that is independent of limb size. Following scaling, the pectoral girdle of each specimen was oriented with the notarium in a horizontal plane and the keel in a vertical plane. Humeri were oriented with the shaft and the deltopectoral crest in a horizontal plane.
Bone Measurements
Following scaling and alignment, we used 3-matic coupled with measurements of freshly dissected and/or cleared and stained specimens to quantify relative changes in bone size, orientation, and complexity. Staining methods for visualizing cartilage in CT (Gignac et al., 2016) were not an option at the time scans were taken, but may simplify future work following this workflow. Where applicable, measurements of the left and right side were averaged for ontogenetic comparisons. Sketches of different measurements are included with graphs (see Results).
Bone Size
• Sternum. Because the keel of immature birds is largely cartilaginous, we used freshly dissected specimens of the same age class (one 7–8 day bird, one 18–20 day bird) to help quantify changes in keel size. The sterna of the dissected specimens were photographed in lateral view and then scaled to the size of the baby or juvenile skeletal model by aligning the ossified portions of the keel, coracoid, and furcula with those in the skeletal model (ossified and cartilaginous components are visually distinguishable during dissection). The “scaled” photographs of the baby and juvenile bird, along with a lateral view of the adult bird exported from 3-matic, were then imported into ImageJ (National Institutes of Health, Bethesda, MD, USA) and the area of the keel was outlined and measured. Note that for other bones (below) we used cleared and stained specimens rather than freshly dissected specimens, partially because cartilage and bone are more distinguishable and partially because cartilage rapidly shrinks following dissection and thus must be photographed immediately. However, we found that the most accurate way to scale the keels of additional specimens to those of the skeletal models was to scale and align based on the entire pectoral girdle rather than the keel alone, since the keel increases in size more rapidly than other bones (i.e., since a slightly older bird may have a similar coracoid and scapula but a larger keel). Visualizing the entire pectoral girdle simultaneously was not possible with cleared and stained specimens because they collapsed on themselves when we attempted to photograph the pectoral girdle in lateral view.
For the remaining bones of the flight apparatus, we measured their lengths in 3-matic and then adjusted the measurements to account for cartilage in immature birds, using cleared and stained specimens (one 7–8 day bird, one 18–20 day bird). To confirm the validity of our cartilage adjustments, we also used X-ray Reconstruction of Moving Morphology (“XROMM”; xromm.org) to measure distances between joints during in vivo flapping kinematics. Previous work had aligned skeletal models (like those here) with x-ray videos of flapping birds to determine in vivo flapping postures (Heers et al., 2016). Cartilage was not visible in the skeletal models or the x-rays, but by estimating the positions of the shoulder and elbow joints as halfway between the humerus and glenoid (shoulder joint) or humerus and radius/ulna (elbow joint), we were able to measure the distance between these two joints as a proxy for humerus length. These measurements were nearly identical to those measured and adjusted in 3-matic (below).
• Coracoids. For each specimen, the left and right coracoids were fit with a cylinder using the “Analytical Cylinder” tool, and the length of the cylinder was measured. To account for cartilaginous components that were not visible in the baby and juvenile skeletal models, we additionally photographed the coracoid of a cleared and stained specimen for each age class, imported the photograph into ImageJ, measured the total length as well as the ossified length, and used this to adjust our measurements in 3-matic:
where 3m refers to lengths of skeletal models measured in 3-matic and cs refers to lengths measured for cleared and stained specimens in ImageJ.
• Furcula. The furcula appeared to be nearly completely ossified and was only measured in 3-matic. We used the “Measure Distance” tool to measure the distance between the ventral extreme of the hypocleidum and the left and right proximal extremes adjacent to the coracoid, and defined the average of these distances as the length of the furcula.
• Scapulae. The lengths of the left and right scapulae were measured in 3-matic using the same “Measure Distance” tool to quantify the straight-line distance between the cranial end (coracoid process of Ghetie et al., 1976) and the caudal tip of the scapula. As with the coracoid, we accounted for cartilage by photographing the scapula of a cleared and stained specimen for both immature age classes, measuring total vs. ossified lengths in ImageJ, and using those measurements to calculate the true lengths of the scapulae (Equation 1).
• Humeri. To measure the lengths of the left and right humeri, we created two parallel, vertical planes, aligned one plane with the proximal end (humeral head) and one with the distal end [ventral or “ulnaris” condyle (Ghetie et al., 1976; Baumel and Witmer, 1993)] of each humerus, and measured the distance between the two planes (same tool as above). Cartilage was accounted for as described above (Equation 1).
• Deltopectoral crest of humerus (DPC). The length of the DPC was quantified as a percentage of humeral length, by measuring the distance between the humeral head and the distal end of the DPC, and dividing this measurement by the distance between the humeral head and the distal end of the humerus [ventral or “ulnaris” condyle (Ghetie et al., 1976; Baumel and Witmer, 1993)]. Both measurements were taken parallel to the shaft of the humerus. For immature birds, we used photographs of the cleared and stained specimens and took measurements in ImageJ; for the adult bird we used 3-matic. Cleared and stained measurements were very similar to measurements made in 3-matic using in vivo postures derived from XROMM (Heers et al., 2016), as described above for the humerus.
• Total forelimb length. To measure total forelimb length, we imported the remainder of the forelimb (radius, ulna, manus) into 3-matic and positioned each bone in mid-downstroke posture, as recorded during in vivo flapping using XROMM (Heers et al., 2016). Because XROMM involves matching bone models with bone x-rays, these in vivo positions correctly position the ossified portions of each bone, such that the gaps between bones represent missing cartilage. To measure total forelimb length, we therefore used the in vivo positions to measure the distances between the shoulder and elbow joints, elbow and wrist joints, and wrist joint and tip of the manus (completely ossified), by creating two planes for each set of joints (one plane at each joint, perpendicular to bones in question) and measuring the distance between them. The sum of these distances was defined as the total forelimb length. Total forelimb length could also have been measured using cleared and stained specimens to account for missing cartilage, but this would have involved more measurements (i.e., 3-matic plus cleared and stained adjustments with Equation 1) and thus more potential for error.
Bone Orientation
With the exception of the glenoid, bone orientations were quantified solely in 3-matic.
• Coracoid angle. We define “coracoid angle” as the obtuse angle between a horizontal plane and the shaft of the coracoid. As described above, we used the “Analytical Cylinder” tool to fit cylinders to the left and right coracoids, and then measured the angle between each cylinder and a horizontal plane, using the “Measure Angle” tool.
• Scapulocoracoid angle. The scapulocoracoid angle is the angle between the scapula and coracoid. We used the coracoid cylinders described above to represent each coracoid. For each scapula, we first fitted an arc (“Create Arc” tool) to the ventral edge of the bone, and then fit a cylinder to the portion of the arc that intersected the cylinder of the coracoid. Finally, we measured the angle between the coracoid cylinder and the scapula cylinder to determine the scapulocoracoid angle.
• Furculocoracoid angle. We define “furculocoracoid angle” as the angle between the furcula and the coracoids. Again, we used the coracoid cylinders described above to represent each coracoid. We used a plane to quantify the orientation of the furcula (“Datum Plane” tool with 3 points: cranial surface of intersection between left and right clavicles and hypocleidum + cranial surfaces of clavicles at articulations with the coracoids). We then used the “Measure Angle” tool to quantify the angle between the plane of the furcula and each coracoid cylinder, and took the average of these measurements as the furculocoracoid angle.
• Glenoid. The glenoid is partially cartilaginous, especially in immature birds. To quantify the orientation of this structure, we therefore photographed the shoulder joint in dissected specimens in lateral view, as described for the sternum in section Bone Size above (glenoid difficult to photograph in cleared and stained specimens). Photographs were aligned to skeletal models (i.e., with the vertebral column horizontal), then measured. For each bird we took two measurements with respect to a horizontal plane: the angle made by a line connecting the dorsal surfaces of the labra of the scapula and coracoid, and the angle made by a line connecting the ventral surfaces of the labra of the scapula and coracoid. These two measurements were averaged for each bird. We did not notice any other obvious, evolutionarily-relevant differences (i.e., whether glenoid was directed more vertically in older birds).
Bone Complexity
The humerus becomes more complex through avian ontogeny and evolution. To capture some of this complexity, we quantified three conspicuous morphological features, in 3-matic.
• Deflection of humeral head. We measured the deflection of the humeral head away from the humeral shaft in ventral view, because the deltopectoral crest makes measurements in dorsal view more challenging. For the left and right humeri of each bird, a vertical plane was created, aligned with the shaft of the humerus, and positioned at the intersection between the midline (long axis) of the shaft and the distal end of the deltopectoral crest. This plane was then duplicated, and rotated until it intersected the center of the humeral head. We measured the angle between these two planes (“Measure Angle” tool) to quantify deflection of the humeral head.
• Depth of deltopectoral crest (DPC). Although the DPC is partially cartilaginous in immature birds, dissected and cleared and stained specimens show that the pectoralis and supracoracoideus insert mainly on the ossified portions of this structure. We therefore measured the maximal depth of the ossified DPC in 3-matic. This is an underestimate of the size in immature birds, but functionally more relevant. To measure the depth of the DPC on left and right humeri, we created a vertical plane on the cranial surface of the proximal humerus (“Datum Plane” tool), duplicated the plane, aligned the duplicate to the edge of the DPC at its maximal width, and measured the distance between the two planes.
• Offset of margo caudalis. The margo caudalis is a muscle attachment site on the caudal surface of the proximal humerus that grows away from the long axis of the humerus as the bicipital crest expands through ontogeny. To quantify this offset on left and right humeri, we created two horizontal planes: one passing through the dorsal surface of the shaft of the humerus, parallel to the long axis of the bone, and one passing through the margo caudalis. The distance between these planes was defined as the offset or expansion of the margo caudalis.
Muscle Analysis
To explore relationships between skeletal and muscular development, we used previously collected data and musculoskeletal models (Heers and Dial, 2015; Heers et al., 2016, 2018) to document ontogenetic changes in muscle mass, as well as the leverage (moment arm length) and orientation or “pull” of shoulder muscles during wing-assisted incline running (WAIR). All of these studies used the same age classes (7–8 day baby, 18–20 day juvenile, >100 day adult) and, as much as possible, the same specimens.
Muscle Mass
Muscle masses were obtained by dissecting birds (three birds per age class). For full details, see (Heers and Dial, 2015).
Muscle Leverage (Moment Arm Length)
The length of a moment arm is defined as the perpendicular distance between a muscle's line of action and the joint it acts upon. Moment arms determine how muscle force is transformed into limb motion: a muscle's effect is the product of its force multiplied by its moment arm. Thus, the longer the moment arm, the greater the muscle leverage. We analyzed moment arms that were previously calculated using musculoskeletal models (made in SIMM, i.e., Software for Interactive Musculoskeletal Modeling; Musculographics, Inc, CA; http://www.musculographics.com/) and simulations [done in OpenSim; https://opensim.stanford.edu/ (Delp et al., 2007)] of WAIR. For full details, see (Heers et al., 2018).
Muscle Orientation (Direction of Pull)
Although each muscle has one moment arm per joint, this three-dimensional moment arm can be decomposed into z, y, and x vectors to determine a muscle's leverage in different directions [i.e., elevation/depression (z) vs. protraction/retraction (y) vs. long axis rotation (x)]. To assess each shoulder muscle's direction of pull, we calculated the average vector sum of each muscle's z (elevation/depression) and y (protraction/retraction) moment arms, under three conditions: in vivo kinematics during maximal effort WAIR, adult kinematics during WAIR, and a standardized downstroke position (for downstroke muscles) or upstroke position (for upstroke muscles). Ontogenetic differences in muscle pull during in vivo kinematics could result from anatomical or kinematic differences, whereas differences during adult and/or standardized kinematics would result solely from anatomical differences.
Results
Bones Change Dramatically in Size, Orientation, and Complexity During Bird Development
Elements of the flight apparatus in 7–8 day old (“baby”), 18–20 day old (“juvenile”), and adult chukars differ substantially in relative size, orientation, and complexity (Figure 2). When standardized by notarium length, components of the pectoral girdle (coracoid, scapula, furcula, and especially sternum) increase in size during ontogeny (Figure 3A), whereas the humerus and forelimb are proportionally longest in the juvenile bird and the deltopectoral crest is proportionally long in both immature age classes (Figure 3B). Bones of the pectoral girdle also change in orientation. During ontogeny, the keel of the sternum shifts ~15°, from a slightly more vertical orientation to an orientation more parallel to the vertebral column. This is partially due to a shift in the angle of the sternum and partially due to greater growth in the anterior portion of the keel (Figure 2). The angles between the coracoid and body axis, and coracoid and furcula, increase through ontogeny, while the scapulocoracoid angle decreases and the glenoid becomes more parallel to the vertebral column (Figure 4). Simultaneously, the humerus becomes more complex (Figures 2, 5): muscle attachment sites like the deltopectoral crest become more pronounced, the bicipital crest expands, and the humeral head is deflected away from the shaft and becomes more globular.
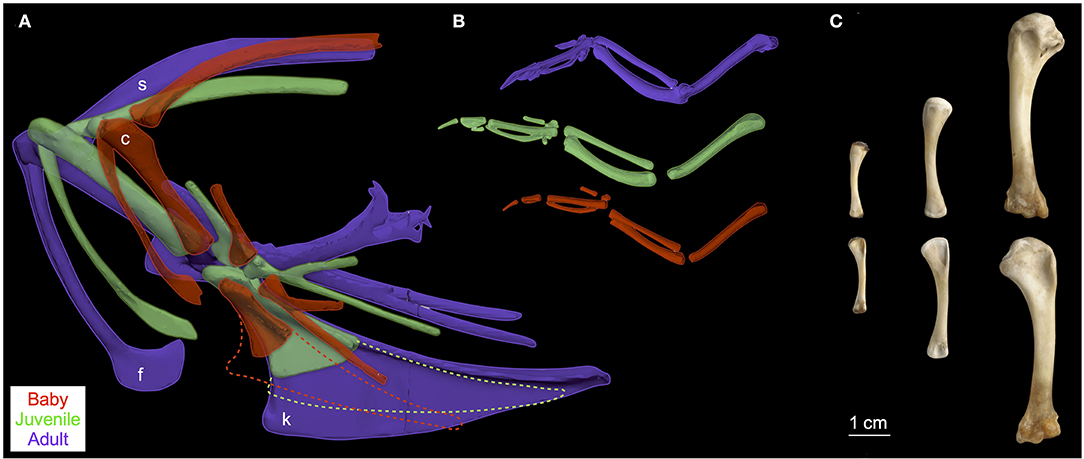
Figure 2. Pectoral girdle and forelimb ontogeny. During avian ontogeny, elements of the pectoral girdle (A) and forelimb (B,C) change in relative size, orientation, and/or complexity. Pectoral girdles of chukars (A) shown in left lateral view and standardized by notarium length; notaria (not shown) oriented horizontally and positioned by aligning the coracosternal joints to clearly show changes in bone orientation; k (keel of sternum; cartilaginous components dashed), f (furcula), c (coracoid), s (scapula). Left forelimbs of chukars (B) shown in dorsal view (~ perpendicular to deltopectoral crest) and mid-downstroke posture, also standardized by notarium length but not to scale with (A). Left humeri (C) of baby, juvenile, and adult chukars shown in caudal (top) and cranial (bottom) views; photo by Nicole Wong.
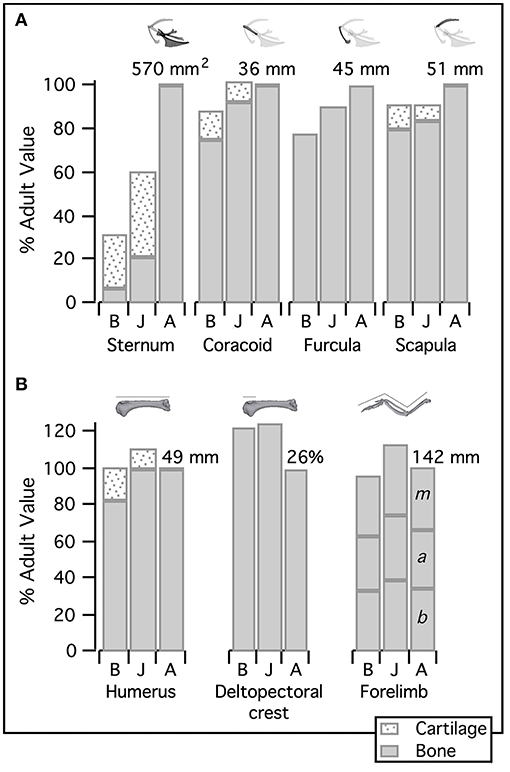
Figure 3. Relative size of the flight apparatus. (A) Elements of the pectoral girdle become proportionally larger during avian ontogeny: bones are typically smallest in baby birds (though the scapula is equally small in juveniles) and largest in adults (though the coracoid reaches adult size in juveniles). (B) In contrast, humeral as well as total forelimb length peaks in juveniles, and the deltopectoral crest is proportionally longer in both immature age classes than in adults. Bone measurements first standardized by notarium length to determine proportions relative to body size, then standardized by adult values to assess the relative development of different components of the flight apparatus. Coracoid, furcula, scapula, humerus, and (total) forelimb quantified by length; deltopectoral crest quantified as a percentage of humeral length; keel of sternum quantified by area; adult values indicated above respective bar. B, Baby chukar; J, Juvenile chukar; A, Adult chukar; b, brachium; a, antebrachium; m, manus.
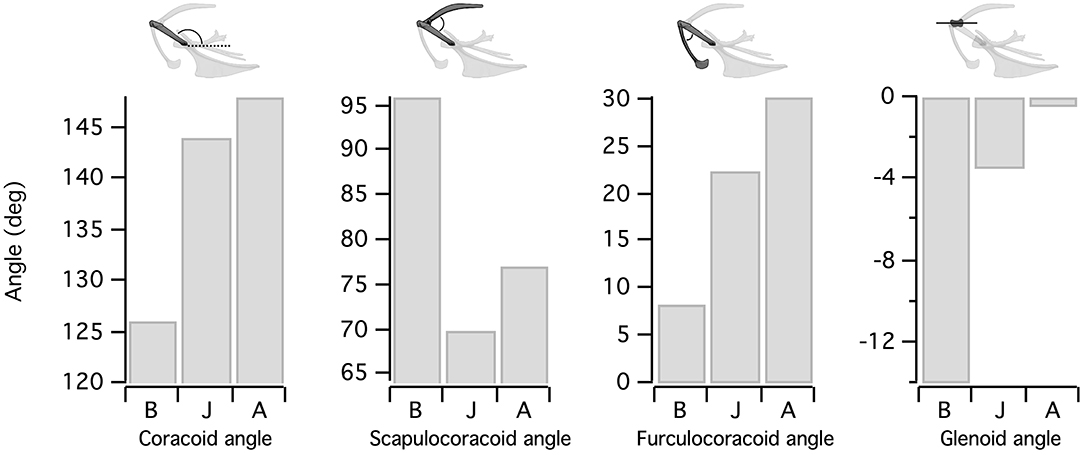
Figure 4. Orientation of the flight apparatus. Elements of the pectoral girdle change orientation during avian ontogeny. Because the scapula remains close to the vertebral column, increases in coracoid angle are accompanied by decreases in scapulocoracoid angle. Simultaneously, the angle between the furcula and coracoid increases. Shifts in coracoid orientation also bring the glenoid more parallel to the vertebral column. B, Baby chukar; J, Juvenile chukar; A, Adult chukar.
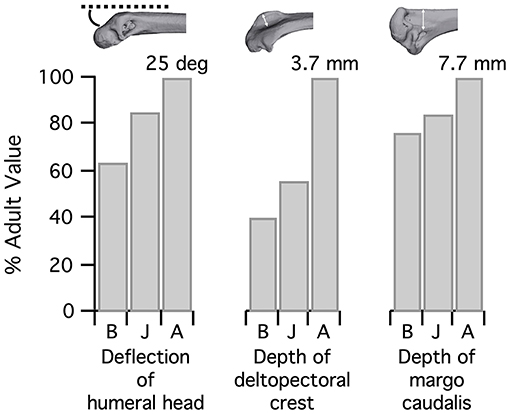
Figure 5. Complexity of the humerus. The humerus grows more complex during ontogeny, as the humeral head is deflected away from the shaft and muscle attachment sites like the deltopectoral crest and bicipital crest (as measured by the margo caudalis, where several shoulder muscles attach) become exaggerated. Note that the depth of the deltopectoral crest is underestimated in baby and juvenile birds because cartilage was not measured here; however, muscles appear to attach to the ossified portion, so this measurement is the most functionally relevant. B, Baby chukar; J, Juvenile chukar; A, Adult chukar.
Ontogenetic Changes in Skeletal Anatomy Do Not Result in Very Different Flapping Kinematics, at Least During Wing-Assisted Incline Running (WAIR)
Though differences in skeletal anatomy might be expected to result in different flapping kinematics, due to differing muscle and/or joint morphology, previous work demonstrates that during maximal effort wing-assisted incline running, developing and adult chukars have very similar kinematics (Heers et al., 2016). Differences that do exist may compensate for the underdeveloped flight apparatus of immature birds (below).
Ontogenetic Changes in Skeletal Anatomy Are Associated With Increases in Muscle Mass and Leverage (Moment Arm Length), but Not Necessarily Orientation or Function
Differences in skeletal anatomy might also be expected to influence muscle morphology. Consistent with this expectation, relative muscle mass (% body mass) increases through ontogeny (Figure 6). These increases are most substantial in muscles that originate from the pectoral girdle (e.g., pectoralis, supracoracoideus) and drive shoulder movements (~30% of adult values in baby chukars capable of WAIR, ~60% of adult values in juvenile chukars capable of incipient flight). Relative moment arm lengths—and thus muscle leverage—show a more complicated pattern. When standardized by notarium length, moment arms about the z (elevation-depression) and y (protraction-retraction) axes show a general increase through ontogeny but tend to peak in juveniles (for 6 out of 10 muscles about z axis, 7 about y axis), whereas moment arms for long axis rotation (x) tend to increase through ontogeny and peak in adults (for 6 out 10 muscles) (Figure 7). The proportionally large z and y moment arms in the juvenile are likely an outcome of its proportionally long forelimbs and deltopectoral crest (Figures 2, 3B), whereas the large x moment arms in the adult probably result from skeletal features like the deflected humeral head, expanded bicipital crest, and wider deltopectoral crest, all of which shift muscle attachment sites away from the long axis of the humerus (Figures 2, 5) (Heers et al., 2018).
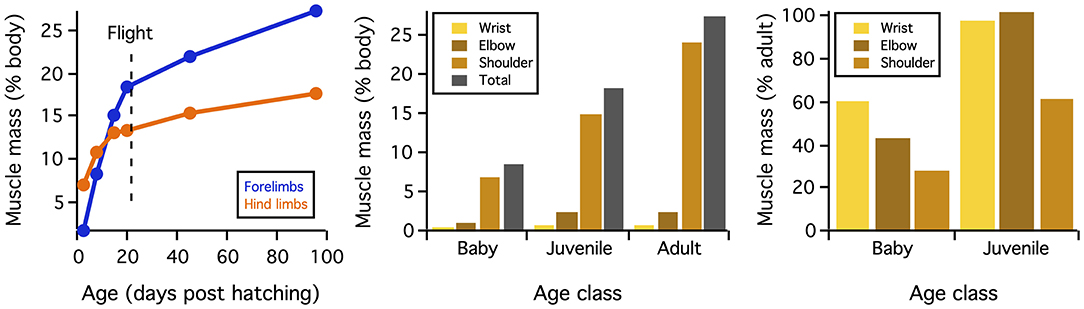
Figure 6. Muscle mass. When standardized by body mass, muscle mass increases through ontogeny. These increases are most extreme for shoulder muscles that originate from the pectoral girdle (Figure 3A) and drive shoulder movements. Figure from Heers et al. (2018).
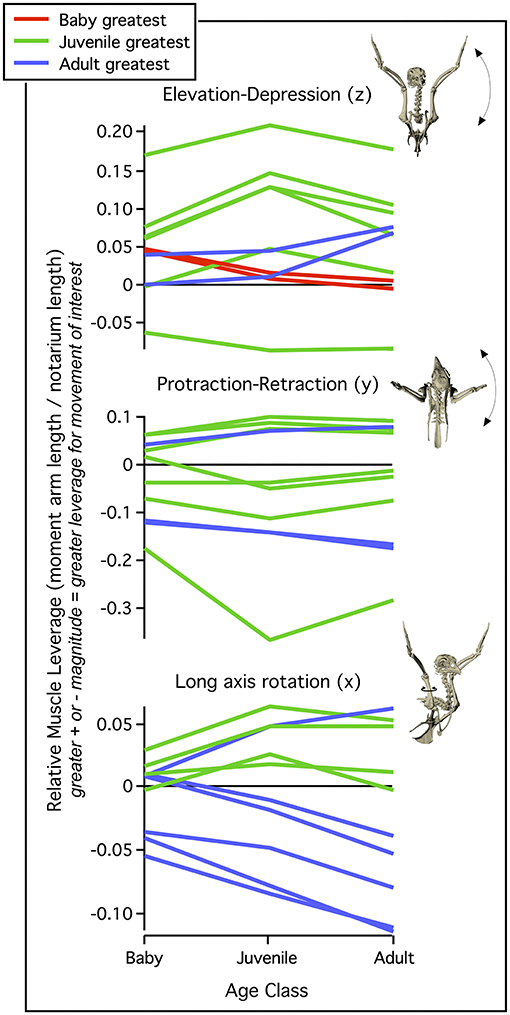
Figure 7. Muscle leverage (moment arm length). When standardized by notarium length, moment arm lengths generally increase through ontogeny but show different developmental patterns, depending on the direction of motion. Muscle leverage for elevation/depression (z axis) and protraction/retraction (y axis) tends to peak in juveniles, most likely due to their proportionally long forelimbs and deltopectoral crests (Figures 2, 3B). In contrast, moment arms for long axis rotation (x axis) tend to peak in adults, whose exaggerated muscle attachment sites help pull muscles away from the long axis of the humerus (Figures 2, 5). Each line represents the relative moment arm length (leverage) for one shoulder muscle about the z, y, or x axis, during maximal effort wing-assisted incline running (WAIR) in chukars. Figure modified from Heers et al. (2018).
In contrast to the substantial changes observed in muscle mass and leverage, average muscle moment arm orientations during flapping are fairly consistent in chukars (i.e., muscles pull in similar directions through ontogeny). Though many shoulder muscles show small changes coincident with shifts in bone orientation and/or complexity (Table 1), moment arm orientations for the two most important flight muscles—the pectoralis and the supracoracoideus—do not appear to shift in conjunction with shifts in bone orientation:
• Supracoracoideus (main upstroke muscle; origin on sternum, coracoid, and coracoclavicular membrane; insertion on dorsal surface of deltopectoral crest via triosseal canal). The supracoracoideus appears to pull more dorsally in juveniles than in babies or adults, irrespective of kinematics (Table 1). The proportionally long forelimbs of juveniles—combined with the proportionally long deltopectoral crest (Figure 3B)— presumably enhance elevation. If this interpretation is correct, the more dorsal pull of the supracoracoideus in juveniles results from differences in bone size rather than differences in bone orientation. The long humerus and deltopectoral crest of juveniles also enhance pectoralis leverage, although not above adult values, potentially due to other changes that enhance pectoralis leverage in adults (below).
• Pectoralis (main downstroke muscle; origin on sternum, ribs, furcula, and sternocoracoclavicular membrane; insertion on ventral surface of deltopectoral crest). During in vivo kinematics for maximal effort WAIR, the pectoralis appears to pull more caudally in immature birds (Table 1, column A). However, this difference is largely eliminated when kinematics are standardized (columns B, C), especially during the start of the downstroke when the pectoralis is active (column C). This indicates that differences in moment arm orientation observed during in vivo kinematics are due to subtle differences in flapping kinematics rather than anatomy. Kinematic differences do not appear to result from restrictions or differences in joint morphology—immature chukars actually have a greater range of motion than adults (due to their less ossified and more flexible joints) (Heers et al., 2016), and the glenoid is more steeply angled away from the vertebral column (Figure 4) indicating that, if anything, the wing would be expected to move more cranially during the downstroke rather than more caudally. Kinematic subtleties also do not appear to optimize muscle leverage for elevation/depression or protraction/retraction, but may improve leverage for long axis rotation, especially in baby birds that have less complex humeri and therefore less leverage for rotation (Figure 8A). In addition, kinematic differences may serve to improve aerodynamic performance (Figure 8B). Previous work demonstrates that the wings of developing chukars produce proportionally more drag than the wings of adults (Heers et al., 2011). During WAIR, baby chukars adopt a more vertical global stroke plane angle (102° vs. 110° in older birds), such that drag contributes substantially to weight support (Jackson et al., 2009; Heers et al., 2011). This steep stroke plane angle results at least partially from the more pitched posture of younger birds (Heers et al., 2016), but probably also from the more caudally-directed pull of the pectoralis during in vivo kinematics. In short, ontogenetic differences in pectoralis pull during in vivo flapping kinematics do not appear to result from ontogenetic shifts in skeletal anatomy, but may be adaptive in improving muscle leverage (for rotation) and aerodynamic performance in young birds with draggy wings.
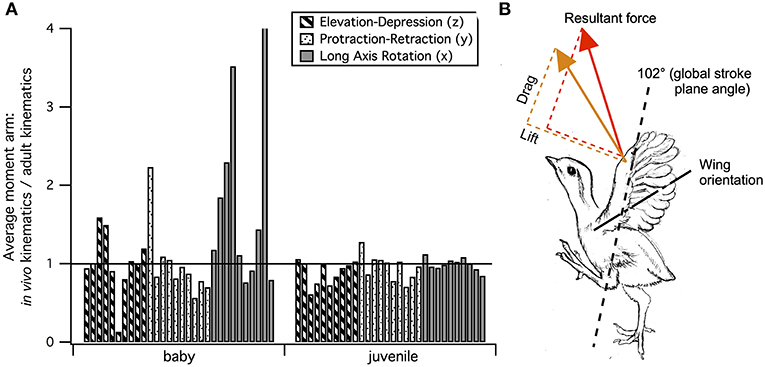
Figure 8. Kinematic optimization? In spite of changes in skeletal morphology, flapping kinematics during maximal effort wing-assisted incline running (WAIR) are very similar in immature and adult chukars (Heers et al., 2016). (A) Differences that do exist may help improve muscle leverage for long axis rotation in baby birds (Figure 7), whose humeri lack the exaggerated muscle attachment sites of adults (Figures 2, 5) (7 out of 10 muscles have greater leverage for long axis rotation during in vivo than adult kinematics, compared to 4 out of 10 for elevation/depression and 3 out of 10 for protraction/retraction). In juveniles, muscle leverage for long axis rotation during in vivo and adult kinematics is more similar (5 out of 10 muscles have greater leverage for long axis rotation during in vivo than adult kinematics, but differences are not as substantial; 4 out of 10 are greater for elevation/depression and 5 out of 10 are greater for protraction/retraction). (B) Kinematic differences may also improve wing performance: 8 (red arrow) and 10 (orange arrow) day old chukars have a more vertical global stroke plane angle than older birds, which helps compensate for their draggy wings by orienting drag in a direction that contributes substantially to weight support (Jackson et al., 2009; Heers et al., 2011). (A) Each bar represents the average moment arm of one muscle during in vivo kinematics divided by its average moment arm during adult kinematics; pectoralis and supracoracoideus are the first and second bars, respectively, for each grouping. (B) Adapted from Heers et al. (2011); sketch by Robert Petty.
Given that bones of the pectoral girdle show substantial changes in orientation during ontogeny, the fact that these changes do not seem to be associated with changes in orientation of the supracoracoideus or the pectoralis is, at first glance, surprising. For example, as the sternum becomes more parallel to the vertebral column and the angle of the coracoid increases during ontogeny, the pectoralis and supracoracoideus might be expected to take on a more cranio-caudal orientation as their origins shift more caudally to the coracosternal joint and their insertions shift cranially to it (Figure 2). In reality, however, the glenoid, furcula, and scapula maintain their positions relative to the vertebral column through ontogeny (Figure 9), because shifts in coracoid angle are counteracted by changes in furculocoracoid and scapulocoracoid angles (i.e., the coracoid changes orientation but the furcula and scapula do not). Simultaneously, the cranial portion of the keel expands more than the caudal portion and at least partially counteracts ontogenetic shifts in sternum orientation. Thus, as the coracoid changes orientation and positions the growing sternum more caudally, the furcula and cranial portion of the keel expand and maintain the cranial origins of the supracoracoideus and especially the pectoralis. Coupled with the triosseal canal, which helps constrain the supracoracoideus, these skeletal changes allow for an expansion of muscle attachment sites (keel, interosseous membranes) and increases in muscle mass, without changes in muscle function. In short, muscle paths converge near the shoulder joint and functions (Table 1; Table 2 in Heers et al., 2018) remain similar through ontogeny, in spite of underlying changes in the skeletal apparatus.
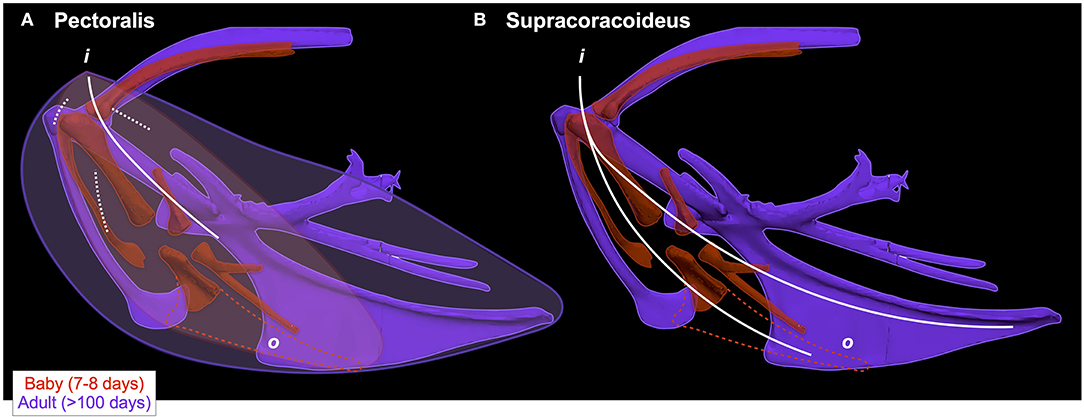
Figure 9. Different skeletons underlie similar muscles. In spite of underlying changes in skeletal morphology, the pectoralis (left) and supracoracoideus (right) maintain similar paths (indicated by white lines; o = origin, i = insertion) through ontogeny because changes in one bone are offset by changes in others. When baby and adult chukars are aligned by the vertebral column (i.e., notaria in same position and orientation; not shown for clarity of other structures) rather than by the coracosternal joint (as in Figure 2), it becomes clear that ontogenetic shifts in coracoid angle (Figure 4) are counteracted by changes in furculo- and scapulo-coracoid angles, such that the glenoid, furcula, and scapula maintain similar positions relative to the vertebral column. As the coracoid changes orientation and positions the growing sternum more caudally, the furcula and cranial portion of the keel expand and maintain the cranial origins of the supracoracoideus and especially the pectoralis. (A) Though the pectoralis has a very broad origin on multiple bones, the bulk of the muscle is concentrated cranially in adult birds and muscle fibers converge near the shoulder joint, resulting in similar paths in babies and adults. Due to its large size and complex architecture, this muscle is extremely challenging to model. The path shown here was determined by tracking the central tendon (solid white line) and fibers (dashed white lines) in dissected adult birds and overlaying these paths onto the skeleton; though the central tendon is not clearly defined in immature birds, muscle fibers appear to be oriented similarly to those of adult birds. Paths were confirmed by elevating and depressing the wing of a deceased bird and observing fiber orientations. (B) The supracoracoideus more closely tracks the sternum and coracoid but is constrained by the triosseal canal, similarly causing muscle paths to converge near the shoulder joint. White lines show the path of the pectoralis (left; solid = central tendon, dashed = fibers) or the supracoracoideus tendon (right; this tendon passes medial to the shoulder joint through the triosseal canal, but is shown so that the entire pathway is visible); red or purple lines show the approximate outline of the pectoralis muscle. Baby and adult aligned by the vertebral column, showing that (i) the glenoid does not change position, (ii) the scapula and furcula have similar orientations in spite of different coracoid orientations, and (iii) the keel in baby birds is functionally equivalent to the interosseous membrane between the sternum and furcula in adults.
Discussion
The evolution of avian flight is one of the great transformations in vertebrate history, marked by striking anatomical changes that presumably are adaptations or exaptations (Gould and Vrba, 1982) (“aptations”) for meeting the demands of aerial locomotion (Figure 1). Some of these aptations are functionally intuitive—for example, the keel of the sternum clearly provides a large attachment site for important flight muscles, while the triosseal canal constrains the tendon of the supracoracoideus muscle. However, many aptations are categorized as such based on their presence in flight-capable birds and their absence in flightless birds and extinct theropods, with no known or demonstrated function(s). For instance, the angle between the scapula and the coracoid is acute (or close to acute) in volant birds but obtuse in avian embryos and secondarily flightless birds (Livezey, 1989, 2003, 2008), and these differences have been used to discuss potential flight capacity in extinct organisms (Olson and Feduccia, 1979; Feduccia, 1986; Chatterjee and Templin, 2003). Yet the functional relevance of this angle has not been demonstrated. Similarly, the humerus of modern birds shows greater complexity (more exaggerated muscle attachment sites, globular and deflected head) than the humeri of extinct theropods like Archaeopteryx. Though the degree of complexity is likely related to muscle morphology and function, and/or to flight kinematics, these ideas have not been assessed. In short, the avian body plan is highly specialized for flight, and though widely discussed, many specializations are not well-understood.
Skeletal Anatomy vs. Muscle Morphology
To better understand how changes in the pectoral girdle and humerus influence muscle morphology and function during flightless to flight-capable transitions, we quantified how ontogenetic changes in bone size, orientation, and complexity influence muscle size, leverage, and orientation or pull in a developing ground bird, the Chukar Partridge. The skeletons of developing birds are not identical to those of extinct theropods but nevertheless can provide important functional insights, because many features that changed during the evolution of flight also change during the development of flight, which allows their form-function relationships to be assessed. Our results demonstrate that, like the evolution of flight, the development of flight is accompanied by striking changes in skeletal anatomy (Figures 1, 2). When standardized by body (notarium) length, elements of the chukar flight apparatus increase in relative size (Figure 3) and complexity (Figure 5) during ontogeny, while simultaneously changing substantially in orientation (Figure 4):
• The sternum grows larger and more parallel to the vertebral column as it ossifies. This element is often not preserved in extinct paravians (Zheng et al., 2014), perhaps due to late ossification during ontogeny and/or cartilage decay (Figure 10). If reconstructions showing a small, more vertically-angled sternum (similar to that of secondarily flightless birds; e.g., Chiappe and Dyke, 2006; Hartman, 2013; Xu and Qin, 2017) are correct, then a similar shift occurred during the evolution of flight.
• The coracoid angle increases, the scapulocoracoid angle decreases, and the coracoid and scapula lengthen. All of these changes are readily apparent in the fossil record (e.g., Figure 4 in Jenkins, 1993), albeit to a greater degree (e.g., the coracoid lengthens much more between paravians and modern birds than between hatchling and adult birds).
• The furcula grows longer and the angle between the coracoid and furcula increases. Both of these changes can be observed in the fossil record (see reconstructions in Chiappe and Dyke, 2006), albeit again on a more striking scale.
• The forelimb increases in length but is proportionally longest in juveniles, whereas the deltopectoral crest is proportionally long in both baby and juvenile birds. Growth of the bicipital crest, elaboration of muscle attachment sites, and deflection and expansion of the humeral head simultaneously contribute to increases in complexity. Many of these changes occurred during the evolution of flight as well: the forelimb lengthens, even after the appearance of wings (Middleton and Gatesy, 2000; Dececchi and Larsson, 2013), the humeral head becomes more deflected (Rauhut et al., 2019), and bone complexity generally increases. The size of the deltopectoral crest has been related to flight style in Sapeornis (Serrano and Chiappe, 2017), but its relative proportions have not been assessed in other extinct taxa.
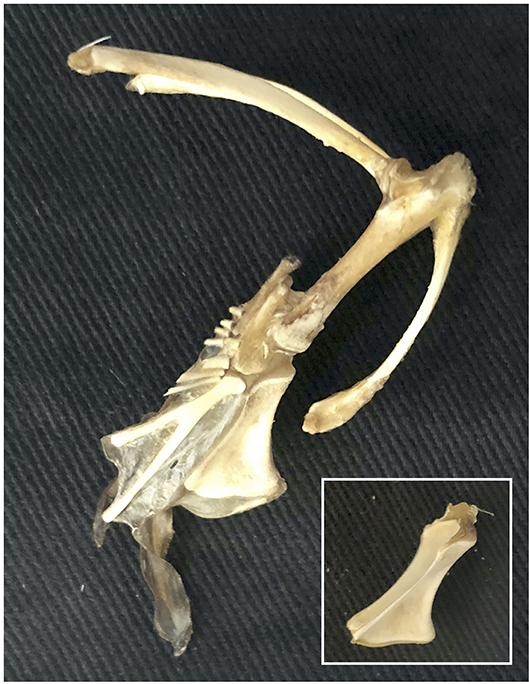
Figure 10. Cartilaginous keel. The cartilaginous keel of immature birds quickly shrinks and deforms following dissection, is completely degraded by skeletal preparation (warm water bath with protein-digesting enzymes, or dermestid beetles) (inset), and thus would easily be lost in the fossil record.
Whether on ontogenetic or evolutionary timescales, these transitions in skeletal anatomy change the size, position, and/or orientation of joint surfaces and/or muscle attachment sites, and therefore might be expected to influence muscle size, leverage, and orientation. Consistent with this expectation, relative muscle mass (Figure 6) and relative muscle leverage (Figure 7) generally increase through ontogeny, although moment arms for elevation-depression and protraction-retraction tend to peak in juveniles, likely due to their proportionally long forelimbs and deltopectoral crests (Heers et al., 2018). As anticipated, ontogenetic changes in skeletal anatomy also result in slight shifts in orientation for some shoulder muscles. However, for the two most important flight muscles—the pectoralis and supracoracoideus—muscle paths and subsequent functions (Table 1; Table 2 in Heers et al., 2018) remain similar in spite of underlying changes in the skeletal apparatus. This is surprising, because the pectoralis and supracoracoideus might be expected to take on a more cranio-caudal orientation as the sternum becomes more parallel to the vertebral column and the coracoid changes orientation (Figure 2). However, our results demonstrate that, in chukars, developmental shifts in one bone are counteracted by developmental shifts in others: changes in furculocoracoid and scapulocoracoid angles counteract changes in coracoid angle, such that the glenoid, furcula, and scapula maintain their positions relative to the vertebral column (Figure 9). As the coracoid changes orientation and displaces the growing sternum more caudally, the furcula and cranial portion of the keel expand and maintain the cranial positions of the supracoracoideus and especially the pectoralis. These changes allow for increases in muscle mass without changes in muscle function, because muscle paths converge near the shoulder joint and remain similar through ontogeny in spite of underlying skeletal changes (Figure 9). Collectively, such findings may suggest that evolutionary changes in skeletal anatomy were associated with increases in muscle size and leverage, but perhaps only minor changes in orientation or pull of the pectoralis and supracoracoideus [but see discussions of the triosseal canal (Ostrom, 1976; Mayr, 2017)]. In short, multiple skeletal solutions yield similar muscle pathways.
Our results also provide explanations for why aptations like humeral complexity and scapulocoracoid angle are related to flight capability. Humeral complexity increases both during ontogeny and evolution, as muscle attachment sites become more exaggerated and the humeral head is deflected. Skeletal features that are most exaggerated in adult birds—the deflected humeral head, expanded bicipital crest, and generally more complex bone processes—likely improve muscle leverage (moment arms) for long axis rotation (Figures 2, 5, 7) by shifting muscle attachment sites away from the long axis of the humerus (Heers et al., 2018). Greater muscle leverage likely improves wing control and may facilitate rapid wing turnaround. For example, the supracoracoideus rapidly supinates the humerus at the start of upstroke (Poore et al., 1997), and although the underdeveloped flight apparatus of baby birds does not prevent them from flapping their wings, this function may be enhanced in older birds as increases in bone complexity improve the supracoracoideus' leverage for rotation.
Whereas increases in humeral complexity likely enhance muscle leverage, the angle between the scapula and coracoid appears to be related to muscle size. As the coracoid angle increases, the scapulocoracoid angle decreases, because the scapula and the glenoid maintain their positions with respect to the vertebral column. This shift in coracoid orientation shifts the sternum to a more caudal position as it grows, and thereby expands the origin of the pectoralis and supracoracoideus caudally (Figure 9). Simultaneously, the furculocoracoid angle increases and the cranial portion of the keel hypertrophies, thereby maintaining the cranial origins of the supracoracoideus and especially the pectoralis. Together, these shifts expand muscle attachment areas and allow for increases in muscle mass while maintaining similar muscle paths (Figure 9). Thus, assessing scapulocoracoid angles is assessing only part of the story—in reality, the coracoid shifts to allow for expansion of muscle attachment sites, while both the scapula and the furcula maintain similar positions and help preserve muscle function. Changes in one bone cannot be analyzed independently of changes in others, reiterating that the functional relevance of many flight aptations is best understood through the lens of a more whole-body perspective.
Skeletal Anatomy vs. Flapping Kinematics
In spite of developmental changes in skeletal anatomy, previous work demonstrates that flapping kinematics in immature and adult chukars are very similar during maximal effort wing-assisted incline running (Heers et al., 2016). Differences that do exist do not appear to be directly caused by developmental changes in skeletal anatomy, but may help compensate for poorer muscle leverage in long axis rotation (Figure 8A), especially in baby birds with less complex humeri (Figures 2, 5). Kinematic differences may also serve to improve locomotor performance in young birds with draggy wings (Figure 8B)—the pectoralis pulls the wing more caudally in baby and juvenile chukars (Table 1) and helps direct drag in a direction that contributes substantially to weight support (Heers et al., 2011). Thus, although flapping kinematics are very similar overall, differences that exist appear to be adaptive for optimizing rotational leverage and aerodynamic performance in young birds with developing flight apparatuses. In conjunction with previous work, these findings show that it is difficult to predict or understand kinematics from skeletal morphology alone, and that similar and effective flapping movements can be produced by animals with very different wings and musculoskeletal apparatuses.
Compensation
This study builds upon previous work demonstrating that developing animals can achieve high levels of locomotor performance by compensating for underdeveloped locomotor structures (Carrier, 1996; Herrel and Gibb, 2006; Dial et al., 2015). Baby (7–8 day) chukars appear to compensate both kinematically and behaviorally. Flapping kinematics appear to improve muscle leverage for long axis rotation and orient drag in a direction that supports body weight (Figure 8) (Heers et al., 2011). Behaviorally, locomotor performance is improved by recruiting wings and legs cooperatively (Dial, 2003; Dial et al., 2015; Heers and Dial, 2015). Such wing-leg cooperation also enhances performance in juvenile (18–20 day) birds. However, juvenile chukars are just becoming flight-capable, and, at this critical stage, anatomical features appear to play an additional and important compensatory role. Incipiently volant juveniles compensate for small flight muscles (Figure 6) with proportionally long forelimbs and deltopectoral crests (Figures 2, 3B), and compensate for less aerodynamically effective feathers by having longer feathers and small bodies (low wing loading) (Jackson et al., 2009; Heers et al., 2011, 2018). In short, anatomic, kinematic, and behavioral compensations allow immature ground birds to acquire flight capacity long before flight aptations are fully developed, playing a key role in avian ontogeny and perhaps a similar role in avian evolution. Flight apparatuses are functional throughout their development—even at rudimentary, more “dinosaur-like” stages.
Collectively
Our results demonstrate that developing and adult ground birds use different functional solutions to execute similar locomotor behaviors. Muscle paths and subsequent functions are largely maintained through ontogeny in spite of dramatic changes in skeletal morphology because shifts in one bone are offset by changes in others. Future studies could build upon this work by examining ontogenetic changes in birds with different life history or locomotor strategies, and by using such data to help reconstruct the musculoskeletal apparatuses of avian predecessors. These preliminary findings nevertheless suggest a possible mechanism for how extinct winged theropods with rudimentary pectoral apparatuses might have achieved bird-like behaviors before acquiring fully bird-like anatomies. These findings also emphasize:
1. The importance of a holistic, whole-body perspective on locomotor performance. Though highly specialized for flight, many aspects of the avian body plan are not well-understood, potentially because they have been viewed in isolation [e.g., scapulocoracoid angle, “What use is half a wing?” (Mivart, 1871)]. Our understanding will be incomplete and possibly misleading unless we consider the entire locomotor apparatus (skeleton + muscles + feathers; wings + legs) on multiple levels (form, function, performance, behavior, ecology).
2. The need for extant validation of extinct behaviors and performance. Although powerful techniques for modeling locomotion in extinct organisms are available and constantly improving (see citations in Pittman et al., 2020), all workflows for inferring function must be validated (Hutchinson, 2011), because animals often do not work the way we expect them to (e.g., developing birds fly long before flight aptations are fully developed; similar muscle paths in spite of different skeletal morphologies). Understanding locomotor evolution requires a thorough exploration of locomotion in extant organisms.
3. The power of ontogeny. Developing birds offer a unique and powerful contribution to studies on avian evolution—only among developing birds can we quantitatively explore real relationships between form, function, performance, and behavior during flightless to flight-capable transitions.
As empirical studies on locomotor ontogeny accumulate, it is becoming apparent that traditional, isolated interpretations of skeletal anatomy mask the reality that integrated whole systems function in frequently unexpected yet effective ways. This perspective is challenging to incorporate into evolutionary studies, because it requires establishing relationships between form, function, performance, behavior, and ecology in extant organisms, and validating workflows for inferring such relationships in extinct organisms. But without such a perspective, our inferences are incomplete and sometimes misleading. Collaborative and integrative efforts that address this challenge will surely strengthen our exploration of life and its evolutionary history.
Data Availability Statement
The original contributions presented in the study are included in the article/supplementary materials, further inquiries can be directed to the corresponding author/s.
Ethics Statement
The animal study was reviewed and approved by University of Montana Institutional Animal Care and Use Committee and the Royal Veterinary College Ethics and Welfare Committee.
Author Contributions
AH, SV, LH, and JC helped collect and analyze data. Manuscript written by AH. All authors contributed to the article and approved the submitted version.
Funding
This project was supported by a New Investigator grant from the California State University Program for Education and Research in Biotechnology (CSUPERB).
Conflict of Interest
The authors declare that the research was conducted in the absence of any commercial or financial relationships that could be construed as a potential conflict of interest.
Acknowledgments
We thank CSUPERB for funding this project, and Ken Dial, Jeffery Rankin, editor Corwin Sullivan, and manuscript reviewers for their time and feedback.
References
Alexander, R. M. (2002). The merits and implications of travel by swimming, flight and running for animals of different sizes. Integr. Comp. Biol. 42, 1060–1064. doi: 10.1093/icb/42.5.1060
Baumel, J. J., and Witmer, L. M. (1993). “Osteologia,” in Handbook of Avian Anatomy: Nomina Anatomica Avium, eds. J. J. Baumel, A. S. King, J. E. Breazile, H. E. Evans, and J. C. Vanden Berge (Cambridge, MA: Nuttall Ornithological Club), 45–132.
Brassey, C. A., Maidment, S. C. R., and Barrett, P. M. (2017). Muscle moment arm analyses applied to vertebrate paleontology: a case study using Stegosaurus stenops Marsh, 1887. J. Vertebr. Paleontol. 37:e1361432. doi: 10.1080/02724634.2017.1361432
Burgers, P., and Chiappe, L. M. (1999). The wing of Archaeopteryx as a primary thrust generator. Nature 399, 60–62. doi: 10.1038/19967
Chatterjee, S., and Templin, R. J. (2003). The flight of Archaeopteryx. Naturwissenschaften 90, 27–32. doi: 10.1007/s00114-002-0385-0
Chiappe, L. M., and Dyke, G. J. (2006). The early evolutionary history of birds. J. Paleontol. Soc. 22, 133–151.
Coombs, W. P. (1978). Theoretical aspects of cursorial adaptations in Dinosaurs. Q. Rev. Biol. 53, 393–418.
Dececchi, T. A., and Larsson, H. C. E. (2013). Body and limb size dissociation at the origin of birds: uncoupling allometric constraints across a macroevolutionary transition. Evolution 67, 2741–2752. doi: 10.1111/evo.12150
Dececchi, T. A., Larsson, H. C. E., and Habib, M. B. (2016). The wings before the bird: an evaluation of flapping-based locomotory hypotheses in bird antecedents. PeerJ 4:e2159. doi: 10.7717/peerj.2159
Delp, S. L., Anderson, F. C., Arnold, A. S., Loan, P., Habib, A., John, C. T., et al. (2007). OpenSim: open-source software to create and analyze dynamic simulations of movement. IEEE Trans. Biomed. Eng. 54, 1940–1950. doi: 10.1109/TBME.2007.901024
Dial, K. P. (1992). Activity patterns of the wing muscles of the Pigeon (Columba livia) during different modes of flight. J. Exp. Zool. 262, 357–373.
Dial, K. P. (2003). Wing-assisted incline running and the evolution of flight. Science 299, 402–404. doi: 10.1126/science.1078237
Dial, K. P., Heers, A. M., and Dial, T. R. (2015). “Ontogenetic and evolutionary transformations: the ecological significance of rudimentary structures,” in Great Transformations in Vertebrate Evolution, eds K. P. Dial, N. Shubin, and E. L. Brainerd (Chicago, IL: University of Chicago Press), 283–301.
Dial, K. P., Jackson, B. E., and Segre, P. (2008). A fundamental avian wing-stroke provides a new perspective on the evolution of flight. Nature 451, 985–989. doi: 10.1038/nature06517
Dial, K. P., Randall, R. J., and Dial, T. R. (2006). What use is half a wing in the ecology and evolution of birds? Bioscience 56, 437–445. doi: 10.1641/0006-3568(2006)056[0437:WUIHAW]2.0.CO;2
Dial, T. R., and Carrier, D. R. (2012). Precocial hindlimbs and altricial forelimbs: partitioning ontogenetic strategies in Mallard ducks (Anas platyrhynchos). J. Exp. Biol. 215, 3703–3710. doi: 10.1242/jeb.057380
Dial, T. R., Heers, A. M., and Tobalske, B. W. (2012). Ontogeny of aerodynamics in mallards: comparative performance and developmental implications. J. Exp. Biol. 215, 3693–3702. doi: 10.1242/jeb.062018
Feduccia, A. (1986). The scapulocoracoid of flightless birds: a primitive avian character similar to that of theropods. Ibis 128, 128–132. doi: 10.1111/j.1474-919X.1986.tb02099.x
Foth, C., Tischlinger, H., and Rauhut, O. W. M. (2014). New specimen of Archaeopteryx provides insights into the evolution of pennaceous feathers. Nature 511, 79–82. doi: 10.1038/nature13467
Gatesy, S. M., and Dial, K. P. (1996). From frond to fan: Archaeopteryx and the evolution of short-tailed birds. Evolution 50, 2037–2048.
Ghetie, V., Chitescu, S.t., Cotofan, V., and Hildebrand, A. (1976). Anatomical Atlas of Domestic Birds. Bucharest: Editura Academiei Republicii Socialiste Romania.
Gignac, P. M., Kley, N. J., Clarke, J. A., Colbert, M. W., Morhardt, A. C., Cerio, D., et al. (2016). Diffusible iodine-based contrast-enhanced computed tomography (diceCT): an emerging tool for rapid, high-resolution, 3-D imaging of metazoan soft tissues. J. Anat. 228, 889–909. doi: 10.1111/joa.12449
Godefroit, P., Sinitsa, S. M., Dhouailly, D., Bolotsky, Y. L., Sizov, A. V., McNamara, M. E., et al. (2014). A Jurassic ornithischian dinosaur from Siberia with both feathers and scales. Science 345, 451–455. doi: 10.1126/science.1253351
Gould, S. J., and Vrba, E. S. (1982). Exaptation-A Missing Term in the Science of Form. Paleobiology 8, 4–15.
Hartman, S. (2013). Scott Hartman's Skeletal Drawing. Available online at: https://www.skeletaldrawing.com/theropods/archaeopteryx
Heers, A. M., Baier, D. B., Jackson, B. E., and Dial, K. P. (2016). Flapping before flight: high resolution, three-dimensional skeletal kinematics of wings and legs during avian development. PLoS ONE 11:e0153446. doi: 10.1371/journal.pone.0153446
Heers, A. M., and Dial, K. P. (2012). From extant to extinct: locomotor ontogeny and the evolution of avian flight. Trends Ecol. Evol. 27, 296–305. doi: 10.1016/j.tree.2011.12.003
Heers, A. M., and Dial, K. P. (2015). Wings versus legs in the avian bauplan: development and evolution of alternative locomotor strategies. Evolution 69, 305–320. doi: 10.1111/evo.12576
Heers, A. M., Dial, K. P., and Tobalske, B. W. (2014). From baby birds to feathered dinosaurs: incipient wings and the evolution of flight. Paleobiology 40, 459–476. doi: 10.1666/13057
Heers, A. M., Rankin, J. W., and Hutchinson, J. R. (2018). Building a bird: musculoskeletal modeling and simulation of wing-assisted incline running during avian ontogeny. Front. Bioeng. Biotechnol. 6:140. doi: 10.3389/fbioe.2018.00140
Heers, A. M., Tobalske, B. W., and Dial, K. P. (2011). Ontogeny of lift and drag production in ground birds. J. Exp. Biol. 214, 717–725. doi: 10.1242/jeb.051177
Herrel, A., and Gibb, A. C. (2006). Ontogeny of performance in vertebrates. Physiol. Biochem. Zool. 79, 1–6. doi: 10.1086/498196
Hutchinson, J. R. (2011). On the inference of function from structure using biomechanical modelling and simulation of extinct organisms. Biol. Lett. 8, 115–118. doi: 10.1098/rsbl.2011.0399
Hutchinson, J. R., Anderson, F. C., Blemker, S. S., and Delp, S. L. (2005). Analysis of hindlimb muscle moment arms in Tyrannosaurus rex using a three-dimensional musculoskeletal computer model: implications for stance, gait, and speed. Paleobiology 31, 676–701. doi: 10.1666/0094-8373(2005)031[0676:AOHMMA]2.0.CO;2
Hutchinson, J. R., and Garcia, M. (2002). Tyrannosaurus was not a fast runner. Nature 415, 1018–1021. doi: 10.1038/4151018a
Jackson, B. E., Segre, P., and Dial, K. P. (2009). Precocial development of locomotor performance in a ground-dwelling bird (Alectoris chukar): negotiating a three-dimensional terrestrial environment. Proc. R. Soc. B Biol. Sci. 276, 3457–3466. doi: 10.1098/rspb.2009.0794
Jasinoski, S. C., Russell, A. P., and Currie, P. J. (2006). An integrative phylogenetic and extrapolatory approach to the reconstruction of dromaeosaur (Theropoda: Eumaniraptora) shoulder musculature. Zool. J. Linn. Soc. 146, 301–344. doi: 10.1111/j.1096-3642.2006.00200.x
Jenkins, F. A. (1993). The evolution of the avian shoulder joint. Am. J. Sci. 293, 253–267. doi: 10.2475/ajs.293.A.253
Lefèvre, U., Cau, A., Hu, D., and Godefroit, P. (2020). “Feather Evolution in Pennaraptora,” in The Evolution of Feathers: From Their Origin to the Present, eds C. Foth and O. W. M. Rauhut (Springer), 103–118.
Livezey, B. C. (1989). Flightlessness in Grebes (Aves, Podicipedidae): its independent evolution in three genera. Evolution 43, 29–54. doi: 10.1111/j.1558-5646.1989.tb04205.x
Livezey, B. C. (2003). Evolution of Flightlessness in Rails (Gruiformes: Rallidae): Phylogenetic, Ecomorphological, and Ontogenetic Perspectives. Lawrence, KS: American Ornithologists' Union.
Livezey, B. C. (2008). Flightlessness in the Galápagos cormorant (Compsohalieus [Nannopterum] harrisi): heterochrony, giantism and specialization. Zool. J. Linn. Soc. 105, 155–224. doi: 10.1111/j.1096-3642.1992.tb01229.x
Longrich, N. R., Vinther, J., Meng, Q., Li, Q., and Russell, A. P. (2012). Primitive wing feather arrangement in Archaeopteryx lithographica and Anchiornis huxleyi. Curr. Biol. 22, 2262–2267. doi: 10.1016/j.cub.2012.09.052
Mayr, G. (2017). Pectoral girdle morphology of Mesozoic birds and the evolution of the avian supracoracoideus muscle. J. Ornithol. 158, 859–867. doi: 10.1007/s10336-017-1451-x
Middleton, K. M., and Gatesy, S. M. (2000). Theropod forelimb design and evolution. Zool. J. Linn. Soc. 128, 149–187. doi: 10.1006/zjls.1998.0193
Norberg, R. A. (1985). “Function of vane asymmetry and shaft curvature in bird flight feathers; inferences on flight ability of Archaeopteryx,” in The Beginnings of Birds, eds J. H. Ostrom, M. K. Hecht, G. Viohl, and P. Wellnhofer (Eichstatt: Jura Museum), 303–318.
Norberg, U. M. (1990). Vertebrate Flight: Mechanics, Physiology, Morphology, Ecology and Evolution. Berlin: Springer-Verlag.
Norell, M. A., and Xu, X. (2005). Feathered dinosaurs. Annu. Rev. Earth Planet. Sci. 33, 277–299. doi: 10.1146/annurev.earth.33.092203.122511
Nudds, R. L. (2014). Reassessment of the wing feathers of Archaeopteryx lithographica suggests no robust evidence for the presence of elongated dorsal wing coverts. PLoS ONE 9:e93963. doi: 10.1371/journal.pone.0093963
Nudds, R. L., and Dyke, G. J. (2010). Narrow primary feather rachises in confuciusornis and archaeopteryx suggest poor flight ability. Science 328, 887–889. doi: 10.1126/science.1188895
Olson, S. L., and Feduccia, A. (1979). Flight capability and the pectoral girdle of Archaeopteryx. Nature 278, 247–248. doi: 10.1038/278247a0
Ostrom, J. H. (1976). Some hypothetical anatomical stages in the evolution of avian flight. Smithson. Contrib. Paleobiol. 27, 1–21.
Ostrom, J. H., Poore, S. O., and Goslow, G. E. (1999). Humeral rotation and wrist supination; important functional complex for the evolution of powered flight in birds? Smithson. Contrib. Paleobiol. 89, 301–309.
Otero, A., Allen, V., Pol, D., and Hutchinson, J. R. (2017). Forelimb muscle and joint actions in Archosauria: insights from Crocodylus johnstoni (Pseudosuchia) and Mussaurus patagonicus (Sauropodomorpha). PeerJ 5:e3976. doi: 10.7717/peerj.3976
Pierce, S. E., Clack, J. A., and Hutchinson, J. R. (2012). Three-dimensional limb joint mobility in the early tetrapod Ichthyostega. Nature 486, 523–526. doi: 10.1038/nature11124
Pittman, M., Heers, A. M., Serrano, F. J., Field, D. J., Habib, M. B., Dececchi, T. A., et al. (2020). Methods of studying early theropod flight. Bull. Am. Mus. Nat. Hist. 440, 277–294.
Poore, S. O., Ashcroft, A., Sánchez-Haiman, A., and Goslow, G. E. (1997). The contractile properties of the M. supracoracoideus in the pigeon and starling: a case for long-axis rotation of the humerus. J. Exp. Biol. 200, 2987–3002.
Rauhut, O. W., Tischlinger, H., and Foth, C. (2019). A non-archaeopterygid avialan theropod from the Late Jurassic of southern Germany. eLife 8:e43789. doi: 10.7554/eLife.43789
Rauhut, O. W. M., and Foth, C. (2020). “The origin of birds: current consensus, controversy, and the occurrence of feathers,” in The Evolution of Feathers: From Their Origin to the Present, eds C. Foth and O. W. M. Rauhut (Springer), 27–46.
Rayner, J. M. V. (1988). The evolution of vertebrate flight. Biol. J. Linn. Soc. 34, 269–287. doi: 10.1111/j.1095-8312.1988.tb01963.x
Serrano, F. J., and Chiappe, L. M. (2017). Aerodynamic modelling of a Cretaceous bird reveals thermal soaring capabilities during early avian evolution. J. R. Soc. Interface 14:20170182. doi: 10.1098/rsif.2017.0182
Serrano, J. F., Costa-Pérez, M., Navalón, G., and Martín-Serra, A. (2020). Morphological disparity of the humerus in modern birds. Diversity 12:173. doi: 10.3390/d12050173
Tobalske, B. W., and Dial, K. P. (2007). Aerodynamics of wing-assisted incline running in birds. J. Exp. Biol. 210, 1742–1751. doi: 10.1242/jeb.001701
Vazquez, R. J. (1992). Functional osteology of the avian wrist and the evolution of flapping flight. J. Morphol. 211, 259–268.
Walker, A. D. (1972). New light on the Origin of Birds and Crocodiles. Nature 237, 257–263. doi: 10.1038/237257a0
Xu, X. (2020). “Filamentous integuments in nonavialan theropods and their kin: advances and future perspectives for understanding the evolution of feathers,” in The Evolution of Feathers: From Their Origin to the Present (Cham: Springer), 67–78.
Xu, X., and Qin, Z.-C. (2017). A new tiny dromaeosaurid dinosaur from the Lower Cretaceous Jehol Group of western Liaoning and niche differentiation among the Jehol dromaeosaurids. Vertebr. Palasiat. 55, 129–144.
Xu, X., Zhou, Z., Wang, X., Kuang, X., Zhang, F., and Du, X. (2003). Four-winged dinosaurs from China. Nature 421, 335–340. doi: 10.1038/nature01342
Keywords: flight, locomotion, ontogeny, evolution, bird, avian, theropod, musculoskeletal modeling
Citation: Heers AM, Varghese SL, Hatier LK and Cabrera JJ (2021) Multiple Functional Solutions During Flightless to Flight-Capable Transitions. Front. Ecol. Evol. 8:573411. doi: 10.3389/fevo.2020.573411
Received: 17 June 2020; Accepted: 16 November 2020;
Published: 10 February 2021.
Edited by:
Corwin Sullivan, University of Alberta, CanadaReviewed by:
Zhiheng Li, Chinese Academy of Sciences, ChinaElizabeth Anne Freedman Fowler, Dickinson State University, United States
Copyright © 2021 Heers, Varghese, Hatier and Cabrera. This is an open-access article distributed under the terms of the Creative Commons Attribution License (CC BY). The use, distribution or reproduction in other forums is permitted, provided the original author(s) and the copyright owner(s) are credited and that the original publication in this journal is cited, in accordance with accepted academic practice. No use, distribution or reproduction is permitted which does not comply with these terms.
*Correspondence: Ashley M. Heers, YXNobWhlZXJzQGdtYWlsLmNvbQ==