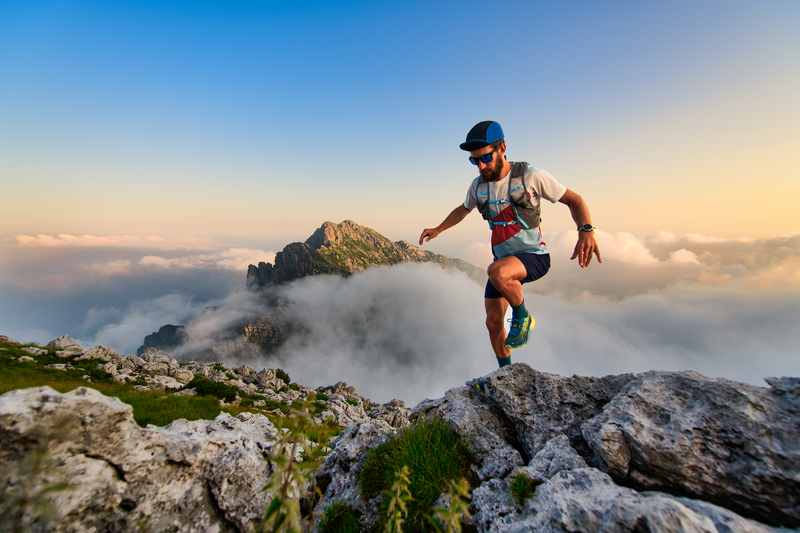
95% of researchers rate our articles as excellent or good
Learn more about the work of our research integrity team to safeguard the quality of each article we publish.
Find out more
ORIGINAL RESEARCH article
Front. Ecol. Evol. , 23 October 2020
Sec. Behavioral and Evolutionary Ecology
Volume 8 - 2020 | https://doi.org/10.3389/fevo.2020.570034
This article is part of the Research Topic The Impact of Weather on the Behavior and Ecology of Birds View all 17 articles
Across taxa, offspring size traits are linked to survival, and life-time fitness. Inclement weather can be a major constraint on offspring growth and parental care. Despite the adaptive benefits of larger offspring, we have a limited understanding of the effects of severe environmental conditions across developmental stages and how coping strategies differ among species. We assessed the influence of inclement weather on offspring size and mass traits within populations of three alpine breeding songbirds in British Columbia: (1) horned lark (Eremophila alpestris), (2) dark-eyed junco (Junco hyemalis), and (3) savannah sparrow (Passerculus sandwichensis). Specifically, we investigated at which stages during early-life development offspring are most vulnerable to inclement weather and whether thresholds exist in the developmental response to severe weather events. Across species, we identified two critical periods that best predicted offspring size: (1) clutch initiation, and (2) the nestling stage. Colder temperatures experienced by the female during clutch initiation were associated with larger, heavier offspring in horned larks but smaller offspring for savannah sparrows, indicating the potential for maternal effects, albeit acting through different mechanisms. Additionally, horned lark offspring were resilient to colder average temperatures during the nestling stage but were vulnerable to extreme cold events and multi-day storms. In contrast, dark-eyed junco nestlings were robust to storms, but smaller size and mass traits were associated with lower daily maximum temperatures (i.e., more mild temperature challenges). We suggest species differences may be linked to life-history traits, such as: (1) the thermoregulatory benefits of larger body mass in horned larks, (2) the benefits of greater nest cover to buffer dark-eyed junco against precipitation events, and (3) delayed clutch initiation for savannah sparrows to limit exposure to cold storms. We provide evidence for stage-specific impacts of inclement weather on offspring development with implications for reproductive success. These results advance our understanding of early-life resilience to stochastic environments, as we may be able to predict differences in the vulnerability of alpine species to increasingly variable and severe weather conditions.
Inclement weather and temperature regimes can strongly influence size and mass development in homeothermic vertebrates (Gillooly et al., 2002; Nord and Giroud, 2020). Since larger offspring size is often associated with greater survival and life-time reproductive success (Marshall et al., 2018), the developmental period represents a prime target for selection to maximize fitness for both offspring and their parents (Rollinson and Rowe, 2015; Vindenes and Langangen, 2015). Altricial songbird development occurs across several well-defined stages: ovum development (internal), egg incubation (external; warmth required), and the nestling stage (warmth and food required). While distinct, these stages are not compartmentalized, as conditions that affect development in one stage can influence subsequent stages (Monaghan, 2008; O’Connor et al., 2014). In addition, songbird offspring are dependent on parental care, such that the development of offspring size traits reflects both parental and offspring responses to prevailing conditions (Auer and Martin, 2017). Inclement weather can stimulate adaptive coping mechanisms in both parents and offspring, reallocating limited resources and promoting or constraining development (Williams, 2012; Wingfield et al., 2017).
Nestlings grow rapidly over a compressed period, such that even short disruptions in growth can negatively influence size at fledging and post-fledging survival (McCarty and Winkler, 1999; Naef-Daenzer and Keller, 1999; Cox et al., 2014). Inclement weather can constrain size trait growth by imposing thermoregulatory challenges that force investment in mass and thermoregulation (Arendt, 1997). Because the physiological and skeletal muscle development required to achieve and maintain endothermy is energetically costly (Price and Dzialowski, 2018), the onset of endothermy and investment in thermoregulation may occur at the expense of size trait growth (i.e., wing length; Olson, 1992; Ricklefs et al., 1994; Węgrzyn, 2013). For example, tree swallow (Tachycineta bicolor) nestlings from experimentally heated nests increase wing growth (Dawson et al., 2005), suggesting optimal conditions can release energy allocation constraints and maximize size growth. In addition, weather effects on nestling growth are often linked to food resources, either by altering resource availability or by affecting the ability of parents to capture and deliver food (Stodola et al., 2010; Pipoly et al., 2013; Tuero et al., 2018). As such, altricial offspring are highly dependent on parental investment to mitigate their developmental responses to inclement weather (Auer and Martin, 2017; de Zwaan et al., 2019).
During reproduction, adults must partition resources between parental care and self-maintenance (i.e., survival; van Noordwijk and de Jong, 1986). Resource-challenged individuals may reduce investment in incubation, brooding, or provisioning nestlings in favor of self-preservation activities like foraging; thus, increasing nest exposure to inclement weather (Williams, 2012). Reduced nest attentiveness during incubation can lead to cooled embryos (Coe et al., 2015) and subsequently constrained nestling growth (Nord and Nilsson, 2011; Ospina et al., 2018; Mueller et al., 2019). In addition, environmental conditions experienced by the female during egg formation have the potential to influence offspring size and mass traits through “maternal effects” (Wolf and Wade, 2009; Moore et al., 2019). Challenging environmental conditions can elevate female glucocorticoid levels (e.g., corticosterone), which may flow passively into the developing egg yolk and reduce nestling growth (Love et al., 2005; Saino et al., 2005). Alternatively, if suboptimal conditions are anticipated, females may invest in larger eggs or elevated yolk testosterone levels to increase nestling size, potentially improving offspring resilience to challenging developmental conditions (Mousseau and Fox, 1998; Marshall and Uller, 2007; Bentz et al., 2016). Maternal effects may therefore have particularly important fitness consequences in harsh, variable environments with limited breeding opportunities, and uncertain resource availability (Crino and Breuner, 2015; Kuijper and Hoyle, 2015).
Offspring size traits can be impacted by a broad range of weather events and severities experienced across developmental stages. Developing offspring may be robust to some suboptimal conditions, but exposure to severe, frequent, or prolonged weather events may cross an energy-challenge threshold beyond which constrained development may occur (Cunningham et al., 2013; Wingfield et al., 2017). In stochastic habitats like the alpine, weather conditions fluctuate greatly within and among seasons (Martin et al., 2017), resulting in significant variation in the early-life exposure and timing of extreme weather events among nests even within the same general period (e.g., first clutches). Variable exposure provides the opportunity to pinpoint periods during early-life development where offspring are most susceptible to extreme weather and to identify potential threshold events. Additionally, comparisons among sympatric species can reveal evolved differences in the developmental response to challenging weather which may reflect differences in key life-history traits that regulate offspring exposure to the environment (i.e., nest cover, parental care). When considered in a life-history context, assessing the influence of severe weather events on offspring development within and among species can highlight the capacity for species to respond to the prevailing environment, as well as inform predictions of species vulnerability under increasingly variable climatic conditions.
We investigated the influence of inclement weather on offspring size trait variation within populations of three ground-nesting songbirds breeding in alpine habitats: (1) horned lark (Eremophila alpestris), (2) dark-eyed junco (Junco hyemalis), and (3) savannah sparrow (Passerculus sandwichensis). Specifically, we assessed: (i) the relative effect of temperature and precipitation variables on nestling size traits, (ii) the importance of severity (i.e., extreme weather events), and (iii) how the timing of inclement weather across developmental stages (ova development, incubation, and nestling stage) impacts offspring development. We predicted that all species would respond most strongly to severe weather events like storms and extreme cold (Wingfield et al., 2017). We also expected the strongest effects to occur during the late incubation and early nestling stage when females must balance time on the nest with self-feeding and provisioning nestlings (Nord and Williams, 2015).
Finally, while our three focal species share comparable life-history traits (e.g., ground-nesters, similar development rates), they differ in two key traits that are associated with the thermal environment of the nest and potentially resilience to challenging weather conditions (Table 1). Specifically, nest cover and body mass may influence environmental exposure and parental investment requirements. Therefore, we also investigated: (iv) differences among species in relative weather effects across developmental stages. Minimal nest cover for horned larks may make offspring more susceptible to heavy precipitation events, particularly in combination with low temperatures, or “cold storms” (Martin et al., 2017). However, a lower brood mass for dark-eyed junco and savannah sparrows may reduce heat retention within the nest, potentially forcing an earlier investment in the development and maintenance of endothermy while also requiring females to invest more in brooding behavior and less in provisioning offspring (Nord and Nilsson, 2012; Andreasson et al., 2016). A larger body mass may reduce the severity of this trade-off, such that horned larks may be less susceptible to thermoregulatory challenges with a greater capacity to buffer offspring against severe or prolonged weather events (Wendeln and Becker, 1999; McNamara et al., 2004).
Table 1. Comparison of select life-history traits among horned lark, dark-eyed junco, and savannah sparrow which may contribute to differences in the thermal environment of the nest and offspring resilience to prevailing weather.
We studied high elevation populations of horned lark, savannah sparrow, and Oregon dark-eyed junco (J. h. oreganus) in British Columbia, Canada. Horned larks are open-country songbirds that breed in sparsely vegetated habitats such as short-grass prairies, desert, and tundra from 0 to over 4,000 m above sea level (a.s.l.; Beason, 1995). Savannah sparrow are also open-country specialists that inhabit cultivated fields, meadows, and alpine tundra >2,000 m a.s.l. in British Columbia (Ryder, 2015), but, unlike horned lark, associate with taller grasses and shrubs (e.g., Salix sp.; MacDonald et al., 2016). Dark-eyed junco breed in open-forest and shrub-dominated habitats from 0 to 3,775 m a.s.l. (Nolan et al., 2002).
For these species, alpine populations predominantly raise one complete brood per season, with evidence for occasional double brooding (Bears et al., 2009; Martin et al., 2009; Camfield et al., 2010). Horned lark and dark-eyed junco begin initiating clutches by mid-May, while savannah sparrows initiate later, from early- to mid-June (Bears et al., 2009; Martin et al., 2017; de Zwaan et al., 2019). Females of each species lay one egg a day and usually begin incubation on the penultimate egg (Beason, 1995; Nolan et al., 2002; Wheelwright and Rising, 2008). See Table 1 for more details on nest traits, development rates, and parental care.
We studied savannah sparrows from 2003 to 2004 and horned larks from 2015 to 2018 in approximately 4 km2 of subalpine and alpine habitat on Hudson Bay Mountain (HBM) near Smithers, British Columbia, Canada (54.8°N, 127.3°W; Figure 1). Savannah sparrows nested primarily between 1,500 and 1,800 m a.s.l. in both alpine tundra and open sub-alpine habitat consisting of scattered krummolz subalpine fir (Abies lasiocarpa) and willow shrubs (Salix sp.) interspersed with alpine meadows. Horned larks nested entirely above treeline from 1,650 to 2,000 m a.s.l. This site is characterized by high winds and fluctuating temperatures (Camfield and Martin, 2009). Snowmelt varies considerably but often extends into mid-June, resulting in compressed breeding seasons (Camfield et al., 2010).
Figure 1. Map of the two alpine study sites within British Columbia, Canada: Hudson Bay Mountain, and Mount Mackenzie. Each study species is associated with the site and elevational range where they were studied. Data were collected for savannah sparrow from 2003–2004, horned lark from 2015–2018, and dark-eyed junco from 2013–2015. Maps: Leaflet JavaScript library with base map and data from OpenStreetMap contributors. Illustrations: A. Drake.
From 2013 to 2015, we studied Oregon dark-eyed juncos between 1,900 and 2,200 m a.s.l. on Mount Mackenzie near Revelstoke, BC, Canada (51.0°N, 118.2°W; Figure 1). This habitat is considered predominantly subalpine, including stands of Engelmann spruce (Picea engelmannii) and subalpine fir with patches of transitional alpine meadows and tundra. Snowmelt occurred from June to early July, slightly later than at HBM. This is likely because seasonal precipitation was considerably greater at Mount Mackenzie over the study period coupled with colder early-season temperatures (Table 2). Otherwise, climate conditions were comparable between sites, particularly the extensive within and among year variability (Table 2).
For all species, nests were located by systematic territory searches and behavioral observation. Nests were monitored every 2–3 days except for near hatch and fledge when we switched to daily nest visits. At 7-days post-hatch (day 0 = hatch date), we measured wing length (±0.5 mm), tarsus length (±0.02 mm), and mass (±0.01 g). For savannah sparrows, only tarsus length and mass were measured. Junco nestlings were measured every 2 days starting at hatch; however, we limited our consideration to measurements at 7-days for this study to allow for comparisons among species. Infrequently, nestlings were measured at 6- or 8-days post-hatch, and thus we also recorded age of measurement to control for this variation. Each nestling was banded with one United States Geological Survey (USGS) numbered aluminum band and 2–3 plastic color bands for subsequent identification.
Precipitation and temperature variables were recorded using two HOBO weather stations (Onset Computer Co., Pocaset, MA, United States): (1) a U30-NRC station for 2015 and 2016, and (2) an RX3000 satellite station for 2018. Both stations were located at 1,695 m a.s.l., within 1.2 km of all nests, and should therefore be representative of ambient conditions within the observed elevational ranges of both species (horned lark: 1,650–2,000 m; savannah sparrow: 1,500–1,800 m). Temperature sensors were positioned approximately 3 m above ground. Raw weather data were recorded every 4 min and then averaged hourly.
Precipitation data were missing for 2003 and 2004 (savannahsparrows), and both temperature and precipitation data were missing for parts of 2017 (horned lark). To estimate precipitation for these periods, we used values from the Smithers Regional Airport ∼8 km from our site (station ID = SA10774981; elevation = 522 m). Total precipitation was poorly correlated between stations, but station SA10774981 correctly identified days on HBM with or without precipitation 81% of the time and days with ≥10 mm of precipitation 90% of the time (Martin et al., 2017). Therefore, to be consistent across all years, whether exact precipitation amounts were available or not, we classified each day as a precipitation day (≥1 mm) or storm event (≥10 mm) using a binomial 0 or 1. To estimate missing temperature values, we interpolated hourly estimates of air surface temperature from the 8 nearest grid points in the National Centers for Environmental Prediction (NCEP) R-1 dataset using the R package “RNCEP” (Kemp et al., 2012). Comparisons of the interpolated estimates with existing measurements from the study site were highly correlated (rp = 0.91), validating this method. We regressed recorded temperatures at HBM on interpolated temperature for all years with existing data (2003–2018) in order to correct for elevational effects. The resulting equation was used to convert interpolated temperature to better align with true temperature measurements:
For 2013 and 2014, we used hourly temperature data from a weather station owned by the local ski resort (Revelstoke Mountain Resort, Inc) situated at 1,950 m a.s.l. within our study site (dark-eyed junco elevation range = 1,900–2,200 m a.s.l.). Temperature data were missing for 2015, so we retrieved hourly recordings from a nearby station located at 1,850 m a.s.l. on neighboring Mount Revelstoke (∼8.5 km from Mount Mackenzie), part of the Provincial Snow Survey Network (station ID: 2A06P; B.C. Ministry of Environment and Climate Change Strategy 2019). Values from the two sites were highly correlated in 2013 and 2014 (rp = 0.98) but differed by an intercept, so we used the following equation for conversion:
We also extracted precipitation values from station 2A06P as precipitation data were not available from the ski resort weather station at our site. We compared station 2A06P values to those from the Revelstoke airport (WMO station ID: 1176745), located ∼3.5 km from our study site but at 445 m in elevation. While total precipitation correlated poorly, recorded precipitation and storm events matched for 90% of days during the breeding season. Since station 2A06P is at approximately the same elevation as our study site and displays high concordance with weather patterns within the proximate region, we concluded that its precipitation data accurately represented conditions experienced at our study site.
Due to the use of multiple weather stations, we restricted the weather variables we considered to five for which we had a high degree of confidence and that were comparable among study sites: (1) average daily temperature, (2) daily hours ≤10°C, (3) daily hours ≤5°C, (4) precipitation days (≥1 mm/day), and (5) storm events (≥10 mm/day). Daily hours below the 10°C and 5°C threshold reflect cumulative temperature challenges for developing eggs and young. In larks, 10°C is an ecologically relevant threshold, below which females increase incubation efforts (MacDonald et al., 2014) and, during the nestling stage, can prolong offspring development (de Zwaan et al., 2019). However, species or individuals may vary in their ability to respond to moderate temperature thresholds like 10°C, so we also considered hours ≤5°C to address more extreme conditions that may have pronounced effects on early-life development (Pérez et al., 2016). Daily average temperatures were calculated as the average of each day between dawn and dusk (0400–2200 h) to reflect the period when nest contents were most likely to be exposed to ambient temperatures, as night-time incubation attentivity is >90% (Camfield and Martin, 2009). Hours below 5 and 10°C were the sum of hours below each threshold within the same exposure period.
To assess the influence of weather on offspring development and identify the most critical time periods across early-life stages (clutch initiation, incubation, and nestling stage), we used a two-step process. First, for each candidate weather variable, we used a sliding window sensitivity analysis to identify the time periods where each variable showed the strongest relationship with nestling size. Then, for each size trait (wing length, tarsus length, and mass), we fit a global General Additive Mixed-effects Model (GAMM) which included all selected weather variables. Penalized regression splines allowed us to examine non-linear associations between weather variables and size traits within a modeling framework that reduced weather variables to linear effects or dropped them entirely from the model where added complexity did not contribute to overall fit. All weather variables were standardized to allow comparisons among traits and species. All analyses were conducted in R 3.6.3 (R Core Team, 2020).
Sliding window approaches systematically test associations between weather metrics and biological variables of interest across all possible time windows within a specified period, and then rank each subsequent model with Akaike Information Criterion (AIC; van de Pol and Cockburn, 2011; van de Pol et al., 2016). With nestling size traits as the response variables, we built models that assessed all windows within a 30-day period prior to nestling measurement at 7-days post-hatch for each nest. This time period encompasses the incubation and nestling stage, as well as, an average of 8–10 days prior to clutch initiation for each species. For many songbirds, ova development takes approximately 3–5 days when nutrients and hormones are transferred from female to offspring (Williams, 2012). Therefore, 8–10 days prior to clutch initiation should be sufficient to capture the ova development period across species. We constrained the tested time windows to a minimum of 3 days and a maximum of the full 30 days. The minimum window was chosen to avoid spurious correlations with single weather events and to allow for weather patterns prolonged enough to stimulate physiological and behavioral responses in females and nestlings.
For temperature variables (average daily temperature, hours ≤5°C, and hours ≤10°C), we calculated the mean, minimum, and maximum values, as well as the variance across all days within each time window. This allowed us to evaluate the relative influence of average conditions, extremes or variability on nestling size and mass traits. For precipitation variables (precipitation days, storm events), we calculated the sum and variance within each time window to assess cumulative effects and variability, respectively.
For each weather variable, all possible time windows were ranked using AIC relative to the null model. The null model included age of measurement, brood size, and clutch initiation date as fixed effects, and nest ID as a random effect to account for non-independence among nestlings of the same nest. The top time windows were chosen based on the lowest AIC if it was a significantly better fit than the null (ΔAIC < –2). If more than one window occurred within 2 AIC of the top window, the one with the strongest β-coefficient was chosen. If distinctly different time windows occurred within the top models (e.g., 30–20 and 7–0 days), then both windows were selected for that weather variable. Due to the large number of comparisons inherent to sliding window approaches, we additionally ran each model on 100 randomized datasets to determine the likelihood of selecting the same top models by chance (Type 1 error; van de Pol et al., 2016). Only weather variables where the observed results were different from the randomized analyses (P < 0.10) were selected as candidate variables. An α-value of 0.10 was used at this stage as a conservative approach to maximize the number of candidate variables retained for model selection (see next section). The sliding window analysis was conducted using R package “climwin” (Bailey and van de Pol, 2015).
For each size trait, we used all selected candidate weather variables to build a global GAMM using the “mgcv” package (Wood, 2011). Age at measurement, brood size, and clutch initiation date were included as covariates in each model, with nest ID as a random effect. For each weather variable, we fit thin-plate regression splines with a maximum of 3 possible knots to test for potential non-linear associations. Models were fit using Restricted Maximum Likelihood (REML) and incorporating penalties for both smoothing factors and the null space. Based on the fit to the data, this process determines whether a variable should be a smoothed term (2 or 3 knots), a linear term, or be removed from the model (Wood, 2003). Variables were removed from the model if their estimated degrees of freedom (edf) were less than 0.7, retained as a linear term between 0.7 and 1.7, and retained as a smoothed term if greater than 1.7. If all weather variables were linear, a linear mixed-effects model was fit to the selected model structure using “lme4” (Bates et al., 2015). We evaluated collinearity among linear terms using the Variance Inflation Factor (VIF) and retained weather variables with a VIF < 3. Standardized β-coefficients were extracted as effect sizes and associations were considered significant if the 95% confidence interval did not include zero.
Data and R code are available from the Figshare Digital Repository: data http://doi.org/10.6084/m9.figshare.13070276 (de Zwaan et al., 2020a); R code http://doi.org/10.6084/m9.figshare.13070267 (de Zwaan et al., 2020b).
We measured 361 horned lark, 120 dark-eyed junco, and 96 savannah sparrow nestlings from a combined 170 nests. At 7-days post-hatch, horned lark nestlings were considerably larger and heavier than dark-eyed junco and savannah sparrow (Table 3). First nests for horned lark and dark-eyed junco were initiated at approximately the same time (mid-May) and had a breeding season length of about 50 days (first to last clutch initiation date; Table 3). Savannah sparrows began breeding nearly 3 weeks later than larks, resulting in a breeding season that was >50% shorter (Table 3). During the 30-day period prior to nestling measurement, horned larks experienced colder temperatures but fewer storms and precipitation days, while dark-eyed junco and savannah sparrow experienced comparable weather conditions (Table 3).
Table 3. Nestling size traits at 7-days post-hatch, clutch initiation date, and weather conditions experienced over the 30-day window of each individual nesting attempt across species.
Across species, the sliding window analysis identified two general time periods where weather influenced offspring development: (1) from several days before clutch initiation to early incubation (approximately 30–16 days prior to nestling measurement at 7-days post-hatch), and (2) the nestling stage (7–0 days; Figure 2). The type of weather variable operating within these time windows and extent of its influence differed among species and size traits (Appendix S1: Supplementary Table 1). Neither temperature nor precipitation variance were selected in any of the top time windows, suggesting offspring development was not impacted by weather variability itself. Rather, depending on the species, offspring size and mass traits responded most strongly to the average or extreme temperatures within a given window, as well as the cumulative effects of precipitation and storm events (Figure 2). When both average daily temperature and hours ≤10°C were selected, they occurred within the same time window and were strongly correlated (rp > 0.90). Thus, we hereafter report only daily temperature as an indicator of mild temperature effects and hours ≤5°C to reflect periods of extreme cold.
Figure 2. Sliding window results for the top temperature and precipitation variables that influence offspring development across developmental stages for horned lark (A; purple), dark-eyed junco (B; light blue), and savannah sparrow (C; orange). Only traits that passed the randomization test and time windows that had a better fit than the null (≤ 2AIC) are included. Each cell represents 1 day. A darker color intensity indicates greater support for an association between the weather variable and size trait, or a greater number of models that selected that time window.
Average daily temperatures, particularly the coldest day prior-to and during the clutch initiation period (27–16 days prior to measurement), as well as extreme cold (hours ≤5°C) during the nestling stage (7–0 days) were the most influential temperature predictors for the development of size and mass traits in lark nestlings (Figure 3). There was a negative relationship between multi-day average temperatures near clutch initiation and wing length (β = −2.6, 95% confidence interval = –4.2, –0.9) and mass (β = −1.2, 95% CI = −2.0, −0.3), such that colder average temperatures were associated with larger and heavier nestlings (Figure 4A). During this period, the minimum average daily temperature (coldest day) was also negatively associated with mass (β = −1.5, 95% CI: −2.1, −0.8), and in fact, was a better predictor compared to the multi-day average (ΔAIC = −8.6). Thus, cold days during clutch initiation were associated with heavier nestlings. In contrast, at the nestling stage, an increase in the number of hours ≤5°C was linked to smaller nestlings across all size and mass traits (Figure 4B).
Figure 3. Standardized model effect sizes for temperature and precipitation weather variables for each species. The approximate windows in brackets (clutch initiation, incubation, and nestling stage) are generalizations to facilitate comparisons among species and size traits. Error bars depict 95% confidence intervals and an effect is considered significant if the error bars do not overlap zero (gray dashed line). Mean, min and max labels indicate whether it was the average daily temperature, minimum daily average, or maximum daily average within the selected window that best predicted the size trait. The “1” or “2” labels mark the effect for one storm over the time window or two storms relative to zero storms. Gray points with an effect size of zero and standard deviation of 2 indicate the variable did not pass the sliding window randomization test and therefore was not a candidate variable for model selection.
Figure 4. Temperature associations for horned lark, dark-eyed junco, and savannah sparrow. Panels depict (A) daily average temperature prior-to and during the clutch initiation period (27–16 days prior to measurement date), (B) periods of extreme cold during the nestling stage (7–0 days), (C) the maximum daily averages during the nestling stage for dark-eyed junco, and (D) average clutch initiation temperatures for savannah sparrows. Lines represent the predicted trends from the linear mixed effects models, controlling for variation within nests, and the shaded areas are 95% confidence intervals of the partial residuals. All response axes represent relative trait size, where zero indicates the mean value and a 1-unit change equals 1 standard deviation.
For dark-eyed juncos, only temperatures during the nestling stage (7–0 days prior to measurement) were associated with size and mass traits (Figure 3). However, unlike larks which responded to periods of extreme cold (hours ≤5°C), dark-eyed junco nestlings responded most strongly to changes in the maximum average daily temperature (warmest day), or an upper temperature limit (Figure 3). A warmer maximum during the nestling period was linked to greater wing length (β = 2.5, 95% CI = 1.5, 3.5), tarsus length (β = 0.8, 95% CI = 0.4, 1.1), and mass (β = 0.7, 95% CI = 0.1, 1.0) at 7-days post-hatch (Figure 4C).
For savannah sparrows, nestling size trait development was linked to average daily temperatures prior to clutch initiation (30–23 days prior to measurement; Figure 3). Greater temperatures were associated with longer tarsi (β = 0.7, 95% CI = 0.2, 1.2; Figure 4D), but there was no effect on mass.
Horned lark nestlings responded most strongly to storm events during the late incubation and nestling periods. Greater frequencies of storms 12–2 days and 20–2 days prior to measurement were associated with reduced wing and tarsus length, respectively. However, there was no evidence that storm frequency influenced mass (Figure 3). Importantly, only multiple storm events during this time period influenced size trait development, as there was no observable response to a single storm, indicating a possible resilience threshold (Figure 5A).
Figure 5. Influence of storm events during the nestling stage on (A) horned lark wing and tarsus length, and (B) dark-eyed junco mass. Points represent the raw data points and an asterisk indicates a significant difference from zero storms. The scaled trait length was standardized such that each unit is one standard deviation change from the mean at zero.
In contrast, changes in dark-eyed junco nestling size traits (wing, tarsus) were not associated with storm events (Figure 3). A model that included storms during the nestling stage (5-0 days) had similar support to the top mass model (ΔAIC = 1.8); however, the observed negative trend was not significant for either single (β = −0.3, 95% CI = −1.3, −0.7) or multiple storm events (β = −0.4, 95% CI = −1.7, 1.0; Figure 5B).
Savannah sparrow size trait development was also not associated with storms. Instead, a greater frequency of precipitation days prior to clutch initiation and during early incubation (30–12 days) was associated with greater nestling mass (β = 1.8, 95% CI = 1.1, 2.5; Figure 3). During this period, precipitation days and average daily temperature were highly correlated (rp = 0.72) and in the absence of precipitation, greater temperature was positively associated with mass (β = 0.9, 95% CI = 0.2, 1.2). Therefore, while precipitation was the better predictor, it was not possible to separate the influence of precipitation and temperature on nestling mass development for savannah sparrows during this period. See Appendix S1: Supplementary Table 2 for full model outputs.
We identified two general periods where offspring size traits were most influenced by inclement weather: (1) clutch initiation, and (2) the nestling stage. We demonstrated greater resilience to cold temperature challenges in horned lark, but also greater susceptibility to precipitation events than in dark-eyed junco and savannah sparrow; particularly the cumulative effects of multiple storms. Further, both horned larks and savannah sparrows exhibited relatively strong associations between temperature during the clutch initiation process and offspring development, indicating the potential influence of maternal effects. By considering differences among species in a life-history context, our results may be generalizable to other alpine breeding species, allowing us to predict how an increasingly variable and extreme climate may influence reproductive success in alpine bird populations.
Cold temperatures can constrain nestling size growth (Dawson et al., 2005), with variation in response to cold among species and populations potentially reflecting differences in nestling resource allocation or parental investment (Eeva et al., 2002; Mainwaring and Hartley, 2016; Auer and Martin, 2017). While colder temperatures during the nestling stage were associated with smaller offspring size traits in horned larks, this effect was only expressed during extended periods of extreme cold (hours ≤5°C), indicating general resilience to alpine weather conditions. In contrast, dark-eyed junco nestlings exhibited reduced growth in response to colder daily average temperatures (i.e., more moderate temperature challenges). Nestling horned lark at 7-days post-hatch were nearly 66% heavier than dark-eyed junco (Table 3) and therefore may be better able to conserve heat and delay the onset of endothermy to invest in size trait development, even under suboptimal conditions (Calder, 1984; Węgrzyn, 2013; Andreasson et al., 2016). The larger body mass of adult horned lark compared to dark-eyed junco (Table 1) may also provide female larks with greater energy reserves, allowing them to brood longer and at colder temperatures and making them better able to buffer their offspring against prevailing conditions (Wendeln and Becker, 1999; Nord and Williams, 2015).
Interestingly, nestling dark-eyed junco size and mass traits were best predicted by the warmest daily average temperature during the nestling stage (i.e., changes in exposure to the upper thermal range). The ability for adult birds to capture prey and provision nestlings should increase with insect activity (Avery and Krebs, 1984). In cold, seasonal environments like the alpine or arctic, daily fluctuations in insect activity and offspring provisioning rates are likely closely linked to ambient temperatures (Low et al., 2008; Tulp and Schekkerman, 2008). Therefore, maximum daily average temperature experienced during the nestling stage may reflect an ecological signal of elevated insect activity and thus offspring food availability, with benefits for nestling growth. Additionally, dark-eyed junco nests have greater cover than both horned lark and savannah sparrows (Table 1). Greater nest cover offers better concealment from predators, but at the expense of a colder microclimate (Marzluff, 1988; de Zwaan and Martin, 2018). Temperature extremes, like higher maximum temperatures, may be more representative of reduced thermoregulatory challenges for offspring in nests with less sun exposure. Ultimately, detailed information on temperature-specific nestling diet or nest microclimate dynamics among species is required to address these possible mechanisms.
There was no association between temperature during the nestling stage and offspring size traits in savannah sparrows. This is surprising given their previously documented higher nest mortality during periods of suboptimal weather (Martin et al., 2017). However, because the earliest savannah sparrow nests were initiated nearly 3 weeks later than both horned lark (sympatric breeder) and dark-eyed junco, the minimum average temperature experienced during any savannah sparrow nesting attempt was significantly higher (Table 3). Martin et al. (2017) proposed that this delayed onset of breeding was a potential strategy for savannah sparrow to avoid cold storms and reduce the probability of nest failure. Our results indicate that the warmer temperatures associated with later breeding may also benefit offspring development.
While there was no observable influence of a single storm, multiple storms during the nestling stage constrained offspring growth in horned larks. This aligns with the concept of multiple or cumulative stressors where individuals may be resilient to suboptimal conditions up to a specific threshold (allostasis; Wingfield et al., 1998). We found no storm effect for dark-eyed junco, suggesting they may be more robust to precipitation events. Horned larks have highly exposed nests (16% mean overhead cover) compared to dark-eyed junco (90–100%) where nests were often dug into the bank with an earthen or rock overhang (Table 1). Nest placement may therefore underlie differences between species in their susceptibility to storms. Horned lark females brood their nestlings immediately upon onset of rain or snow and remain on the nest for the duration of the weather event, relying on the male to provision nestlings; thus reducing total provisioning rate by at least one half (Goullaud et al., 2018). Prolonged storm events likely impose a significant constraint on nestling food intake and ultimately negatively impact growth rate. For dark-eyed juncos, greater nest cover may make brooding less critical to protecting offspring against precipitation, enabling parents to maintain provisioning rates.
For savannah sparrows, storm events did not influence offspring mass or size traits, despite cold storms being particularly detrimental to their nest success (Martin et al., 2017). Breeding later in the season may not reduce the number of storms birds experience, but it would limit exposure to the more energetically taxing “cold storm” events (Martin et al., 2017; Wingfield et al., 2017). Additionally, we found that warm precipitation prior to and during incubation positively influenced nestling mass in savannah sparrows. While precipitation during the nestling stage can be detrimental to offspring growth (Morganti et al., 2017), warm, wet conditions promote insect abundance (Tuero et al., 2018) and can increase nestling growth if precipitation occurs prior to hatch (Pipoly et al., 2013). Therefore, higher temperatures combined with precipitation may reflect greater food availability during peak nestling growth.
Environmental conditions experienced by the female prior-to or during clutch initiation have strong potential to impact offspring development and life-time fitness (Mousseau and Fox, 1998; Moore et al., 2019). Counterintuitively, colder temperatures during clutch initiation were associated with larger, heavier offspring for horned lark. While we lack the data to address maternal effects in this study, it is worth noting that this association could be adaptive if larger offspring are more robust to suboptimal conditions and if the maternal environment predicts the nestling development environment (Marshall and Uller, 2007; Weber et al., 2018). In the context of alpine birds, our results highlight that conditions experienced during clutch initiation may be an important component of offspring development and reproductive success. Research evaluating the propensity for adaptive maternal effects in alpine species would improve our understanding of how birds cope with stochastic environments.
In this study, we focused predominantly on the influence of weather conditions on offspring size and mass at 7-days post-hatch with the expectation that disrupted growth will have negative fitness consequences. Fledglings with smaller wings or tarsi are less mobile, impacting predator evasion (Martin et al., 2018). Smaller fledglings may exhibit delayed foraging independence and potentially are less likely to endure challenging weather conditions beyond the protection of the nest (Sullivan, 1989; Nord and Nilsson, 2016). Importantly, poor environmental conditions may simply delay fledging, such that nestlings leave the nest at a similar size and mass (i.e., catch-up growth; Aldredge, 2016). However, delayed fledging increases nest exposure and thus the probability of nest predation (Remes and Martin, 2002). Beyond these short-term consequences, poor developmental conditions have the potential to influence future thermal tolerance, longevity and life-time reproductive success (Lindström, 1999; Monaghan, 2008; Andreasson et al., 2018; Nord and Giroud, 2020). Longitudinal studies on individuals that evaluate these latent effects are rare, particularly in free-living populations, but are necessary to fully understand the fitness consequences of weather conditions at different stages of offspring development.
Extreme weather events are becoming increasingly common, particularly in already stochastic habitats like the alpine and arctic (IPCC, 2018). Identifying critical stages where alpine songbird reproduction is most vulnerable to inclement weather is fundamental to predicting future reproductive success under a changing climate. Horned larks were resilient to colder average temperatures but were vulnerable to prolonged periods of extreme cold and multi-day storms. Dark-eyed junco and savannah sparrow appeared less resilient to temperature challenges but were robust to storm events. Different response thresholds and susceptibilities likely reflect differences in life-history traits such as nest cover, body mass, and breeding phenology. For example, savannah sparrows may evade environmental constraints by nesting later in the season when conditions are more benign, but at the expense of a shorter breeding season. We provide evidence for stage-specific impacts of inclement weather on offspring development which advances our understanding of early-life resilience to stochastic environments. We also highlight that key life-history traits may correlate with differences among alpine species in their vulnerability to extreme weather events, such that their capacity to cope with an increasingly variable environment may be predictable.
The data and code are available from the Figshare data repository. Data: doi: 10.6084/m9.figshare.13070276. R code: Doi: 10.6084/m9.figshare.13070267.
All procedures and protocols for this study were approved by the University of British Columbia’s Animal Care Committee (A03-0095, A13-0073, and A15-0027) and are in accordance with the Canadian Council on Animal Care’s national guidelines. All data were also collected under a Scientific Permit for Capture and Banding of Migratory Birds from Environment and Climate Change Canada (10365 BO, 10365 DS, and 10761 J).
DZ, KM, and AD conceived the ideas. DZ, JG, and KM collected the data. DZ and AD analyzed the data and led writing of the manuscript. All authors contributed critically to the drafts and gave final approval for publication.
Funding for this research was provided to DZ by the Northern Scientific Training Program, American Ornithological Society, Society of Canadian Ornithologists, Hesse Research Award, and Northwest Science Association, with financial support to DZ by the Natural Sciences and Engineering Research Council of Canada (NSERC) and University of British Columbia, and to KM by NSERC and Environment and Climate Change Canada.
The authors declare that the research was conducted in the absence of any commercial or financial relationships that could be construed as a potential conflict of interest.
We thank T. Altamirano, N. Bennett, A. Camfield, A. Clason, N. Froese, E. Gow, J. Green, S. Hudson, J. Lee, M. Martin, D. Maucieri, N. Morrel, M. Mossop, C. Rivas, E. Smith, and A. Sulemanji for their contributions to data collection.
The Supplementary Material for this article can be found online at: https://www.frontiersin.org/articles/10.3389/fevo.2020.570034/full#supplementary-material
Aldredge, R. A. (2016). Using non-linear mixed effects models to identify patterns of chick growth in house sparrows Passer domesticus. Ibis 158, 16–27. doi: 10.1111/ibi.12312
Andreasson, F., Nord, A., and Nilsson, J. Å (2016). Brood size constrains the development of endothermy in blue tits. J. Exp. Biol. 219, 2212–2219. doi: 10.1242/jeb.135350
Andreasson, F., Nord, A., and Nilsson, J. Å (2018). Experimentally increased nest temperature affects body temperature, growth and apparent survival in blue tit nestlings. J. Avian Biol. 49:e01620. doi: 10.1111/jav.01620
Arendt, J. D. (1997). Adaptive intrinsic growth rates: an integration across taxa. Q. Rev. Biol. 72, 149–177. doi: 10.1086/419764
Auer, S. K., and Martin, T. E. (2017). Parental care mitigates carry-over effects of poor early conditions on offspring growth. Behav. Ecol. 28, 1176–1182. doi: 10.1093/beheco/arx082
Avery, M. I., and Krebs, J. R. (1984). Temperature and foraging success of great tits Parus major hunting for spiders. Ibis 126, 33–38. doi: 10.1111/j.1474-919X.1984.tb03661.x
Bailey, L., and van de Pol, M. (2015). climwin: Climate Window Analysis. Avaliable at: http://cran.r-project.org/web/packages/climwin/index.html (accessed May 26, 2020).
Bates, D., Maechler, M., Bolker, B., and Walker, S. (2015). Fitting linear mixed-effects models using lme4. J. Stat. Softw. 67, 1–48. doi: 10.18637/jss.v067.i01
Bears, H., Martin, K., and White, G. C. (2009). Breeding in high-elevation habitat results in shift to slower life-history strategy within a single species. J. Anim. Ecol. 78, 365–375. doi: 10.1111/j.1365-2656.2008.01491.x
Beason, R. C. (1995). “Horned Lark (Eremophila alpestris) Version 1.0 published online March 2020,” in Birds of the World, ed. S. M. Billerman (Ithaca, NY: Cornell Lab of Ornithology), doi: 10.2173/bow.horlar.01
Bentz, A. B., Becker, D. J., and Navara, K. J. (2016). Evolutionary implications of interspecific variation in a maternal effect: a metaanalysis of yolk testosterone response to competition. Royal Soc. Open Sci. 3:160499. doi: 10.1098/rsos.160499
S. M. Billerman, B. K. Keeney, P. G. Rodewald, and T. S. Schulenberg (eds.). (2020). “Birds of the world,” in Cornell Laboratory of Ornithology (Ithaca, NY). Available online at: https://birdsoftheworld.org/bow/home
Camfield, A. F., and Martin, K. (2009). The influence of ambient temperature on horned lark incubation behaviour in an alpine environment. Behaviour 146, 1615–1633. doi: 10.1163/156853909X463335
Camfield, A. F., Pearson, S. F., and Martin, K. (2010). Life history variation between high and low elevation subspecies of horned larks Eremophila spp. J. Avian Biol. 41, 273–281. doi: 10.1111/j.1600-048X.2009.04816.x
Coe, B. H., Beck, M. L., Chin, S. Y., Jachowski, C. M. B., and Hopkins, W. A. (2015). Local variation in weather conditions influences incubation behavior and temperature in a passerine bird. J. Avian Biol. 46, 385–394. doi: 10.1111/jav.00581
Cox, W. A., Thompson, F. R. III, Cox, A. S., and Faaborg, J. (2014). Post-fledging survival in passerine birds and the value of post-fledging studies to conservation. J. Wildl. Manag. 78, 183–193. doi: 10.1002/jwmg.670
Crino, O. L., and Breuner, C. W. (2015). Developmental stress: evidence for positive phenotypic and fitness effects in birds. J. Ornithol. 156, 389–398. doi: 10.1007/s10336-015-1236-z
Cunningham, S. J., Martin, R. O., Hojem, C. L., and Hockey, P. A. (2013). Temperatures in excess of critical thresholds threaten nestling growth and survival in a rapidly-warming arid savanna: a study of common fiscals. PLoS One 8:e74613. doi: 10.1371/journal.pone.0074613
Dawson, R. D., Lawrie, C. C., and O’Brien, E. L. (2005). The importance of microclimate variation in determining size, growth and survival of avian offspring: experimental evidence from a cavity nesting passerine. Oecologia 144, 499–507. doi: 10.1007/s00442-005-0075-7
de Zwaan, D. R., Camfield, A. F., MacDonald, E. C., and Martin, K. (2019). Variation in offspring development is driven more by weather and maternal condition than predation risk. Funct. Ecol. 33, 447–456. doi: 10.1111/1365-2435.13273
de Zwaan, D. R., Drake, A., Greenwood, J. L., and Martin, K. (2020a). Data from: Timing and intensity of weather events shape nestling development strategies in three alpine breeding songbirds. Figshare Digital Respository doi: 10.6084/m9.figshare.13070276
de Zwaan, D. R., Drake, A., Greenwood, J. L., and Martin, K. (2020b). R code from: Timing and intensity of weather events shape nestling development strategies in three alpine breeding songbirds. Figshare Digital Respository doi: 10.6084/m9.figshare.13070267
de Zwaan, D. R., and Martin, K. (2018). Substrate and structure of ground nests have fitness consequences for an alpine songbird. Ibis 160, 790–804. doi: 10.1111/ibi.12582
Eeva, T., Lehikoinen, E., Rönkä, M., Lummaa, V., and Currie, D. (2002). Different responses to cold weather in two pied flycatcher populations. Ecography 25, 705–713. doi: 10.1034/j.1600-0587.2002.250606.x
Gillooly, J. F., Charnov, E. L., West, G. B., Savage, V. M., and Brown, J. H. (2002). Effects of size and temperature on developmental time. Nature 417, 70–73. doi: 10.1038/417070a
Goullaud, E. L., de Zwaan, D. R., and Martin, K. (2018). Predation risk-induced adjustments in provisioning behavior for Horned Lark (Eremophila alpestris) in British Columbia. Wilson J. Ornithol. 130, 180–190. doi: 10.1676/16-150.1
IPCC (2018). “Summary for Policymakers,” in Global Warming of 1.5°C. An IPCC Special Report on the Impacts of Global Warming of 1.5°C Above Pre-Industrial Levels and Related Global Greenhouse Gas Emission Pathways, in the Context of Strengthening the Global Response to the Threat of Climate Change, Sustainable Development, and Efforts to Eradicate Poverty, eds V. Masson-Delmotte, P. Zhai, H.-O. Pörtner, D. Roberts, J. Skea, P. R. Shukla, et al. (Geneva: World Meteorological Organization).
Kemp, M. U., van Loon, E., Shamoun-Baranes, J., and Bouten, W. (2012). RNCEP: global weather and climate data at your fingertips. Methods Ecol. Evol. 3, 65–70. doi: 10.1111/j.2041-210X.2011.00138.x
Kuijper, B., and Hoyle, R. B. (2015). When to rely on maternal effects and when on phenotypic plasticity? Evolution 69, 950–968. doi: 10.1111/evo.12635
Lindström, J. (1999). Early development and fitness in birds and mammals. Trends Ecol. Evol. 14, 343–348. doi: 10.1016/S0169-5347(99)01639-0
Love, O. P., Chin, E. H., Wynne-Edwards, K. E., and Williams, T. D. (2005). Stress hormones: a link between maternal condition and sex-biased reproductive investment. Am. Nat. 166, 751–766. doi: 10.1086/497440
Low, M., Eggers, S., Arlt, D., and Pärt, T. (2008). Daily patterns of nest visits are correlated with ambient temperature in the Northern Wheatear. J. Ornithol. 149, 515–519. doi: 10.1007/s10336-008-0300-3
MacDonald, E. C., Camfield, A. F., Jankowski, J. E., and Martin, K. (2014). An alpine-breeding songbird can adjust dawn incubation rhythms to annual thermal regimes. Auk 131, 495–506. doi: 10.1642/AUK-13-234.1
MacDonald, E. C., Camfield, A. F., Martin, M., Wilson, S., and Martin, K. (2016). Nest-site selection and consequences for nest survival among three sympatric songbirds in an alpine environment. J. Ornithol. 157, 393–405. doi: 10.1007/s10336-015-1286-2
Mainwaring, M. C., and Hartley, I. R. (2016). Local weather conditions have complex effects on the growth of blue tit nestlings. J. Therm. Biol. 60, 12–19. doi: 10.1016/j.jtherbio.2016.05.005
Marshall, D. J., Pettersen, A. K., and Cameron, H. (2018). A global synthesis of offspring size variation, its eco-evolutionary causes and consequences. Funct. Ecol. 32, 1436–1446. doi: 10.1111/1365-2435.13099
Marshall, D. J., and Uller, T. (2007). When is a maternal effect adaptive? Oikos 116, 1957–1963. doi: 10.1111/j.2007.0030-1299.16203.x
Martin, K., Wilson, S., MacDonald, E. C., Camfield, A. F., Martin, M., and Trefry, S. A. (2017). Effects of severe weather on reproduction for sympatric songbirds in an alpine environment: interactions of climate extremes influence nesting success. Auk 134, 696–709. doi: 10.1642/AUK-16-271.1
Martin, M., Camfield, A. F., and Martin, K. (2009). Demography of an alpine population of Savannah Sparrows. J. Field Ornithol. 80, 253–264. doi: 10.1111/j.1557-9263.2009.00228.x
Martin, T. E., Tobalske, B., Riordan, M. M., Case, S. B., and Dial, K. P. (2018). Age and performance at fledging are a cause and consequence of juvenile mortality between life stages. Sci. Adv. 4, 1–8. doi: 10.1126/sciadv.aar1988
Marzluff, J. M. (1988). Do Pinyon Jays alter nest placement based on prior experience? Anim. Behav. 36, 1–10. doi: 10.1016/S0003-3472(88)80244-6
McCarty, J. P., and Winkler, D. W. (1999). The relative importance of short term fluctuations in environmental conditions in determining the growth rates of nestling Tree Swallows: an investigation of natural variation using path analysis. Ibis 141, 286–296. doi: 10.1111/j.1474-919X.1999.tb07551.x
McNamara, J. M., Ekman, J., and Houston, A. I. (2004). The effect of thermoregulatory substitution on optimal energy reserves of small birds in winter. Oikos 105, 192–196. doi: 10.1111/j.0030-1299.2004.12188.x
Monaghan, P. (2008). Early growth conditions, phenotypic development and environmental change. Philos. Trans. R. Soc. B 363, 1635–1645. doi: 10.1098/rstb.2007.0011
Moore, M. P., Whiteman, H. H., and Martin, R. A. (2019). A mother’s legacy: the strength of maternal effects in animal populations. Ecol. Lett. 22, 1620–1628. doi: 10.1111/ele.13351
Morganti, M., Rubolini, D., Caprioli, M., Saino, N., and Ambrosini, R. (2017). Rainfall, but not temperature, negatively affects the growth of Blue Tit Cyanistes caeruleus nestlings. Bird Study. 64, 159–167. doi: 10.1080/00063657.2017.1309006
Mousseau, T. A., and Fox, C. W. (1998). The adaptive significance of maternal effects. Trends Ecol. Evol. 13, 403–407. doi: 10.1016/S0169-5347(98)01472-4
Mueller, A. J., Miller, K. D., and Bowers, E. K. (2019). Nest microclimate during incubation affects posthatching development and parental care in wild birds. Sci. Rep. 9, 1–11. doi: 10.1038/s41598-019-41690-4
Naef-Daenzer, B., and Keller, L. F. (1999). The foraging performance of great and blue tits (Parus major and P. caeruleus) in relation to caterpillar development, and its consequences for nestling growth and fledging weight. J. Anim. Ecol. 68, 708–718. doi: 10.1046/j.1365-2656.1999.00318.x
Nolan, V. Jr., Ketterson, E. D., Cristol, D. A., Rogers, C. M., Clotfelter, E. D., et al. (2002). “Dark-eyed Junco (Junco hyemalis) Version 1.0 published online March 2020,” in Birds of the World, eds A. F. Poole and F. B. Gill (Ithaca, NY: Cornell Lab of Ornithology), doi: 10.2173/bow.daejun.01
Nord, A., and Giroud, S. (2020). Lifelong effects of thermal challenges during development in birds and mammals. Front. Physiol. 11:419. doi: 10.3389/fphys.2020.00419
Nord, A., and Nilsson, J. Å (2011). Incubation temperature affects growth and energy metabolism in blue tit nestlings. Am. Nat. 178, 639–651. doi: 10.1086/662172
Nord, A., and Nilsson, J. Å (2012). Context-dependent costs of incubation in the pied flycatcher. Anim. Behav. 84, 427–436. doi: 10.1016/j.anbehav.2012.05.017
Nord, A., and Nilsson, J. Å (2016). Long-term consequences of high incubation temperature in a wild bird population. Biol. Lett. 12:20160087. doi: 10.1098/rsbl.2016.0087
Nord, A., and Williams, J. B. (2015). “The energetic costs of incubation,” in Nests, Eggs, and Incubation: New Ideas About Avian Reproduction, eds D. C. Deeming and S. J. Reynolds (Oxford: Oxford University Press), 152–170.
O’Connor, C. M., Norris, D. R., Crossin, G. T., and Cooke, S. J. (2014). Biological carryover effects: linking common concepts and mechanisms in ecology and evolution. Ecosphere 5, 1–11. doi: 10.1890/ES13-00388.1
Olson, J. M. (1992). Growth, the development of endothermy, and the allocation of energy in red-winged blackbird Agelaius phoeniceus during the nestling period. Physiol. Zool. 65, 124–152.
Ospina, E. A., Merrill, L., and Benson, T. J. (2018). Incubation temperature impacts nestling growth and survival in an open-cup nesting passerine. Ecol. Evol. 8, 3270–3279. doi: 10.1002/ece3.3911
Pérez, J. H., Krause, J. S., Chmura, H. E., Bowman, S., McGuigan, M., Asmus, A. L., et al. (2016). Nestling growth rates in relation to food abundance and weather in the Arctic. Auk 133, 261–272. doi: 10.1642/AUK-15-111.1
Pipoly, I., Bókony, V., Seress, G., Szabó, K., and Liker, A. (2013). Effects of extreme weather on reproductive success in a temperate-breeding songbird. PLoS One 8:11. doi: 10.1371/journal.pone.0080033
Price, E. R., and Dzialowski, E. M. (2018). Development of endothermy in birds: patterns and mechanisms. J. Comp. Physiol. B 188, 373–391. doi: 10.1007/s00360-017-1135-0
R Core Team (2020). R: A Language and Environment for Statistical Computing. Vienna: R Foundation for Statistical Computing.
Remes, V., and Martin, T. E. (2002). Environmental influences on the evolution of growth and developmental rates in passerines. Evolution 56, 2505–2518. doi: 10.1111/j.0014-3820.2002.tb00175.x
Ricklefs, R. E., Shea, R. E., and Choi, I. H. (1994). Inverse relationship between functional maturity and exponential growth rate of avian skeletal muscle: a constraint on evolutionary response. Evolution 48, 1080–1088. doi: 10.1111/j.1558-5646.1994.tb05295.x
Rollinson, N., and Rowe, L. (2015). Persistent directional selection on body size and a resolution to the paradox of stasis. Evolution 69, 2441–2451. doi: 10.1111/evo.12753
Ryder, J. M. (2015). “Savannah Sparrow,” in The Atlas of the Breeding Birds of British Columbia, 2008–2012, eds P. J. A. Davidson, R. J. Cannings, A. R. Couturier, D. Lepage, and C. M. Di Corrado (Delta, BC: Bird Studies Canada).
Saino, N., Romano, M., Ferrari, R. P., Martinelli, R., and Møller, A. P. (2005). Stressed mothers lay eggs with high corticosterone levels which produce low-quality offspring. J. Exp. Zool. A Comp. Exp. Biol. 303, 998–1006. doi: 10.1002/jez.a.224
Stodola, K. W., Buehler, D. A., Kim, D. H., Franzreb, K. E., and Linder, E. T. (2010). Biotic and abiotic factors governing nestling-period length in the ovenbird (Seiurus aurocapilla). Auk 127, 204–211. doi: 10.1525/auk.2009.09151
Sullivan, K. A. (1989). Predation and starvation: age-specific mortality in juvenile juncos (Junco phaenotus). J. Anim. Ecol. 58, 275–286.
Tuero, D. T., Jahn, A. E., Husak, M. S., Roeder, D. V., Masson, D. A., Pucheta, F. M., et al. (2018). Ecological determinants of Tyrannus flycatcher nestling growth at north-and south-temperate latitudes. Auk 135, 439–448. doi: 10.1642/AUK-17-62.1
Tulp, I., and Schekkerman, H. (2008). Has prey availability for arctic birds advanced with climate change? Hindcasting the abundance of tundra arthropods using weather and seasonal variation. Arctic 61, 48–60.
van de Pol, M., Bailey, L. D., McLean, N., Rijsdijk, L., Lawson, C. R., and Brouwer, L. (2016). Identifying the best climatic predictors in ecology and evolution. Methods Ecol. Evol. 7, 1246–1257. doi: 10.1111/2041-210X.12590
van de Pol, M., and Cockburn, A. (2011). Identifying the critical climatic time window that affects trait expression. Am. Nat. 177, 698–707. doi: 10.1086/659101
van Noordwijk, A. J., and de Jong, G. (1986). Acquisition and allocation of resources: their influence on variation in life history tactics. Am. Nat. 128, 137–142. doi: 10.1086/284547
Vindenes, Y., and Langangen, Ø (2015). Individual heterogeneity in life histories and eco-evolutionary dynamics. Ecol. Lett. 18, 417–432. doi: 10.1111/ele.12421
Weber, B. M., Bowers, E. K., Terrell, K. A., Falcone, J. F., Thompson, C. F., and Sakaluk, S. K. (2018). Pre-and post-natal effects of experimentally manipulated maternal corticosterone on growth, stress reactivity, and survival of nestling house wrens. Funct. Ecol. 32, 1995–2007. doi: 10.1111/1365-2435.13126
Węgrzyn, E. (2013). Resource allocation between growth and endothermy allows rapid nestling development at low feeding rates in a species under high nest predation. J. Avian Biol. 44, 383–389. doi: 10.1111/j.1600-048X.2013.05846.x
Wendeln, H., and Becker, P. H. (1999). Effects of parental quality and effort on the reproduction of common terns. J. Anim. Ecol. 68, 205–214. doi: 10.1046/j.1365-2656.1999.00276.x
Wheelwright, N. T., and Rising, J. D. (2008). ““Savannah sparrow (Passerculus sandwichensis) Version 1.0 published online March 2020,” in Birds of the World, ed. A. F. Poole (Ithaca, NY: Cornell Lab of Ornithology), doi: 10.2173/bow.savspa.01
Williams, T. D. (2012). Physiological Adaptations for Breeding in Birds. Princeton, NJ: Princeton University Press.
Wingfield, J. C., Maney, D. L., Breuner, C. W., Jacobs, J. D., Lynn, S., Ramenofsky, M., et al. (1998). Ecological bases of hormone—behavior interactions: the “emergency life history stage”. Am. Zool. 38, 191–206. doi: 10.1093/icb/38.1.191
Wingfield, J. C., Pérez, J. H., Krause, J. S., Word, K. R., González-Gómez, P. L., Lisovski, S., et al. (2017). How birds cope physiologically and behaviourally with extreme climatic events. Phil. Trans. R. Soc. B 372, 140–149. doi: 10.1098/rstb.2016.0140
Wolf, J. B., and Wade, M. J. (2009). What are maternal effects (and what are they not)? Phil. Trans. R. Soc. B 364, 1107–1115. doi: 10.1098/rstb.2008.0238
Wood, S. N. (2003). Thin plate regression splines. J. R. Stat. Soc. B 65, 95–114. doi: 10.1111/1467-9868.00374
Keywords: dark-eyed junco (Junco hyemalis), extreme weather and climate events, horned lark (Eremophila alpestris), cold storms, savannah sparrow (Passerculus sandwichensis), sympatric breeding, altricial nestling growth rate, high latitude temperate mountains
Citation: de Zwaan DR, Drake A, Greenwood JL and Martin K (2020) Timing and Intensity of Weather Events Shape Nestling Development Strategies in Three Alpine Breeding Songbirds. Front. Ecol. Evol. 8:570034. doi: 10.3389/fevo.2020.570034
Received: 05 June 2020; Accepted: 25 September 2020;
Published: 23 October 2020.
Edited by:
Andreas Nord, Lund University, SwedenReviewed by:
Gary Burness, Trent University, CanadaCopyright © 2020 de Zwaan, Drake, Greenwood and Martin. This is an open-access article distributed under the terms of the Creative Commons Attribution License (CC BY). The use, distribution or reproduction in other forums is permitted, provided the original author(s) and the copyright owner(s) are credited and that the original publication in this journal is cited, in accordance with accepted academic practice. No use, distribution or reproduction is permitted which does not comply with these terms.
*Correspondence: Devin R. de Zwaan, ZHJkZXp3YWFuQGdtYWlsLmNvbQ==
Disclaimer: All claims expressed in this article are solely those of the authors and do not necessarily represent those of their affiliated organizations, or those of the publisher, the editors and the reviewers. Any product that may be evaluated in this article or claim that may be made by its manufacturer is not guaranteed or endorsed by the publisher.
Research integrity at Frontiers
Learn more about the work of our research integrity team to safeguard the quality of each article we publish.