- 1DST-NRF Centre of Excellence, FitzPatrick Institute of African Ornithology, University of Cape Town, Cape Town, South Africa
- 2Biodiversity Research Centre, Department of Zoology, University of British Columbia, Vancouver, BC, Canada
- 3Section of Ecology, Department of Biology, University of Turku, Turku, Finland
The primary role of nests as structural support for eggs, nestlings, and incubating parents is well established, but our understanding of their secondary roles and their adaptive features is still limited. Nests can serve a particularly important role in protecting or buffering birds from weather. In hot, arid environments, maximum daily temperatures can exceed a species’ upper critical temperature threshold and during the non-breeding season temperatures may also drop below freezing. Nest structures that help buffer against extreme temperatures may play a crucial role in managing the costs of thermoregulation, especially those nests that are used and maintained year-round. We use extensive year-round data to investigate the thermal benefits of massive colonial structures built by sociable weavers in the arid savannahs of the Kalahari, South Africa. These colonies consist of multiple nesting chambers and are used as roosts when birds are not breeding. We explored whether these structures provide thermal buffering throughout the year and how individual chamber placement within the colony and features of the chambers influenced their thermal buffering capacity. We also investigated whether nest chambers occupied and modified by an obligate nest parasite, the African pygmy falcon provided additional thermal buffering. Our results show that sociable weaver colonies provide thermal benefits throughout the year, buffering both hot and cold ambient extremes. Chambers with longer entrance tunnels provided better insulation than chambers with shorter entrance tunnels, and chambers located toward the center of a colony provided greater insulation than chambers at the edge. Chambers occupied by falcons did not display additional thermal benefits, which may be due to falcons choosing chambers with shorter entrance tunnels. Because falcons are larger than weavers, they may find it harder to enter chambers with longer entrance tunnels, and/or because weavers cease maintenance of those chambers. In conclusion, the communal nests of sociable weaver provide thermal benefits to weavers and heterospecifics alike, creating a more optimal environment for breeding, roosting and reducing thermal stress. In a landscape that is becoming increasingly harsh under climate change, the importance of these structures to the local animal communities may also increase.
Introduction
All birds are oviparous and require nesting sites to lay their eggs (Mainwaring, 2015). The primary role of nests as structural support for eggs, nestlings, and incubating parents is well established, but our understanding of their secondary roles and their adaptive features is still limited (Heenan and Seymour, 2011; Reynolds and Deeming, 2015). However, secondary roles have been identified and include extending phenotypic signals, reducing parasite loads, reducing predation via crypsis, and moderating the micro-environment within the nests (Heenan, 2013; Mainwaring et al., 2014). These roles demonstrate that nests are multifunctional structures and are far more sophisticated than originally thought (Heenan, 2013; Mainwaring et al., 2014). Yet, studies exploring secondary roles are far less frequent than those investigating other nesting behaviors, that include incubation, brood provisioning, and nest construction (Reynolds and Deeming, 2015). This is surprising, especially when considering the great variation of nests across avian taxa, that include simple scrapes on the ground, cup nests in vegetation, nests in burrows below ground and large communal structures (Maclean, 1973a; Mainwaring et al., 2015).
The diversity of nest types suggests that functional traits of the structure may also differ between species and on the environmental conditions the species encounter. Nevertheless, our understanding of the functional properties of bird nests is still in its infancy, with the majority of studies being carried out using nest-boxes to investigate cavity nesting species (Deeming and Mainwaring, 2015). Many of these studies focus on the nests’ ability to prevent eggs from cooling too quickly (Heenan, 2013; Mainwaring et al., 2014). However, in tropic, sub-tropic and desert climates, there may also be a need to prevent eggs from overheating, yet this question has received little attention (Walsberg and Voss-Roberts, 1983; Mainwaring, 2015). The frequency and duration of hot-weather events are predicted to increase in arid environments as the effects of climate change advance (Meehl and Tebaldi, 2004; Akoon et al., 2011). This has been predicted to have negative effects on avian communities (Conradie et al., 2019), that can range from increasing temperatures reducing foraging efficiency to the increased number of heat waves causing catastrophic mortality events (McKechnie and Wolf, 2010; Cunningham et al., 2015). As a result, our line of research will prove valuable for strengthening our understanding of how birds may cope with climate change.
Birds living in hot arid environments frequently face harsh climatic conditions, with temperatures repeatedly exceeding upper critical thresholds of many species (Smith et al., 2017). For example, six out of the seven desert song birds tested by Smith et al. (2017) demonstrated temperature upper thresholds that sat within a relatively narrow range (36.2–39.7°C), while the largest species tested (70 g) had a considerable higher threshold (42.6°C). Species may adopt behavioral or physiological adaptations that help buffer against these extreme temperatures, including moving to the shade to reduce their heat load (Tieleman et al., 2003; Cunningham et al., 2015). However, behavioral responses may be constrained if birds are restricted to a nest for large portions of the day whilst breeding. Furthermore, eggs, and nestlings may also be susceptible to thermal damage (Walsberg and Voss-Roberts, 1983). Most species maintain eggs at temperatures between 32–35°C, independent of environment or body size (Webb, 1987; Williams, 1996) but temperatures start to become lethal at about 42°C, with embryos dying quickly if they reach 44°C (Webb, 1987; Williams, 1996). As a result, parents must try to prevent their eggs from overheating by regulating the microclimate within the nest (Tieleman et al., 2008). This come with costs because birds in arid environments often experience considerable heat stress during incubation and will subsequently suffer increased water loss (Tieleman et al., 2008). Furthermore, high temperatures can slow nestling growth and increase the risk of nest failure (Cunningham et al., 2013; Rodríguez and Barba, 2016). As a result, thermal stress can be a critical factor influencing reproductive success (Walsberg and Voss-Roberts, 1983).
Temperatures during winter in arid environments can also drop below the optimal incubation temperature, and occasionally fall below freezing (Schwimmer and Haim, 2009). If birds are breeding at this time of the year, low temperatures can lead to longer incubation periods, and therefore increased risk of predation (DuRant et al., 2013). Incubating eggs below optimal temperatures can lead to nestlings with lower body mass (Ardia et al., 2010). If unattended, egg temperatures can drop rapidly (Haftorn, 1988; Weathers and Sullivan, 1989), and if they fall to 25–27°C or below for extended periods, then embryonic development stops, although short intervals of cooling are not always harmful (Webb, 1987; Williams, 1996). Furthermore, incubation is energetically costly for adults, often as expensive as chick rearing, and these costs increase at lower temperatures (Nord and Williams, 2015). For example, at experimentally lowered nest temperatures in tree swallows (Tachycineta bicolor), adults were unable to maintain optimal incubation temperatures and spent more time away from the nest (Ardia et al., 2010). Conversely, at experimentally heated nests, the cost of incubation for tree swallows was reduced and adults increased incubation nest attentiveness (Ardia et al., 2009). It is therefore important for incubating parents to provide a nest that can moderate temperature stress in environments where extremes in temperatures are possible (Medina, 2019). If the breeding season encompasses both hot and/or cold extremes then a nest that buffers against these will facilitate reproductive success.
Nest location, architecture, and the materials used during construction have all been shown to be important factors for buffering external ambient temperatures (Mainwaring et al., 2014). Shaded sites can reduce heat stress during the hottest times of the day or year (Orr, 1970; Tieleman et al., 2008). Enclosed nests provide protection against direct sunlight or prevailing winds (Sidis et al., 1994), with the “roof” of the nest providing shade for the eggs or chicks, during periods of high temperatures, while retaining heat during cold periods (Ricklefs and Hainsworth, 1969; Töpfer and Gedeon, 2012; Martin et al., 2017). Within species studies have demonstrated that nests built in colder climates have better heat retaining characteristics (Rohwer and Law, 2010; Crossman et al., 2011), at least partly due to the materials used to build nests (Kern and van Ripper, 1984; Briskie, 1995; Rohwer and Law, 2010). Nests in environments with frequent strong winds are built with thicker nest-walls to minimize convective heat loss (Schaefer, 1976). Mainwaring and Hartley (2008) found that as the breeding season progressed and temperature increased, the mass of the lining materials declined. Nests in wet environments lacked liners and were more porous, absorbing little water and drying rapidly (Kern and van Ripper, 1984). These studies demonstrate that selective pressures of the local climate and weather may drive much some of the variation observed between nests, and also that nests are adapted to the conditions encountered.
Some species use their nests year-round for reasons other than breeding (Forshaw, 2010). Using nest structures for roosting should provide benefits to individuals throughout the year, including the maintenance of homeothermy. This will be especially important to small species in arid environments, where the costs of endothermy are particularly pronounced (McKechnie and Lovegrove, 2002; McKechnie and Mzilikazi, 2011). When temperatures are low, birds can reduce energy demands while roosting through physiological processes including facultative heterothermic responses such as torpor, or through behaviors such as communal roosting and the use of sheltered roost sites (Lyman, 2013). When temperatures are high, birds can use the structure as a refuge, returning during hot periods to avoid direct sunlight (Maclean, 1973a). Year-round nests can also provide a permanent shelter from storms and strong winds. Furthermore, vegetation in arid environments respond strongly to rainfall and many species rely on these resources to trigger the onset of breeding (Dean et al., 2009), however, often rainfall in these areas is highly unpredictable and variable. Maintaining a year-round or permanent nesting structure should allow these species to respond quickly and appropriately to rain events. Consequently, selective pressures may differ considerably for these species and their nest/roost structures would need to deal with a range of daily and seasonal weather conditions.
Sociable weavers (Philetairus socius; henceforth weavers), small passerine birds (approx. 27 g), endemic to the semi-arid and arid Kalahari in the western parts of southern Africa, build massive colonial nest structures (Maclean, 1973a; Mendelsohn and Anderson, 1997). These large colonies are built using Stipagrostis grasses, and maintenance by weavers means these structures can exist for decades and host many generations of weaver (Collias and Collias, 1964). Each colony can contain between two and 250 chambers and hundreds of weaver individuals (Maclean, 1973a). Each nest chamber is accessed through its own entrance tunnel situated on the underside of the colony (Figure 1). Nest chambers are located at different depths (<25 cm) within the colony and therefore entrance tunnels vary in length (Maclean, 1973a). Internal nest temperatures are cooler than external temperatures in summer and warmer than external temperatures in winter (Batholomew et al., 1975; White et al., 1975; van Dijk et al., 2013; Leighton and Echeverri, 2014). It has also been demonstrated that chambers toward the center provide better thermal buffering against external temperatures than those on the edge (van Dijk et al., 2013; Leighton and Echeverri, 2014). This influences the weaver social dynamics, as dominant individuals occupy central chambers with greater insulation (van Dijk et al., 2013). Furthermore, when weavers are not breeding and external temperatures are low, multiple individuals (up to 8) will roost in a single chamber, this further increases the nest temperature reducing the effects of cold ambient temperatures (Paquet et al., 2016).
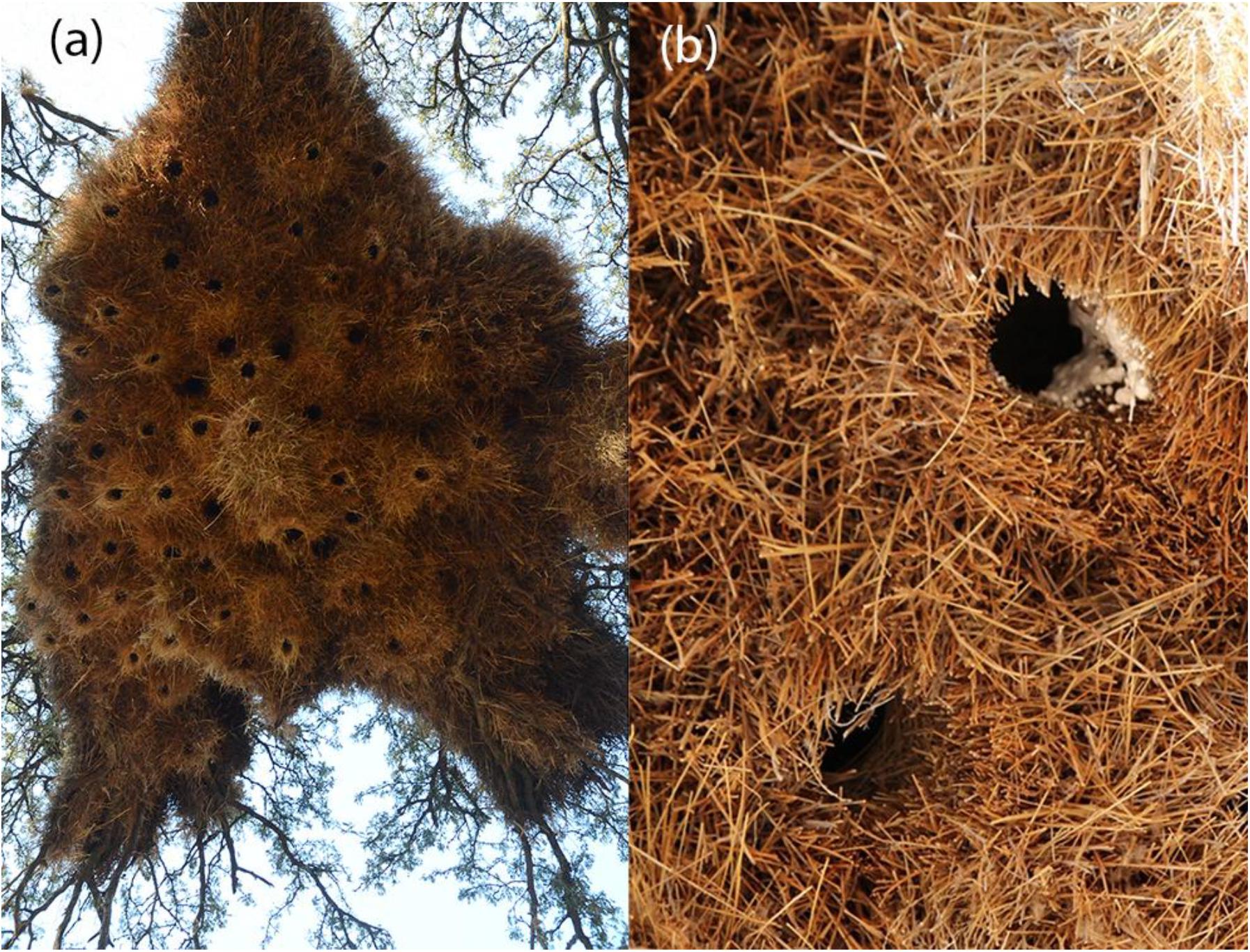
Figure 1. The underside of a sociable weaver colony. These structures can contain many nesting chambers that are entered through the underside of the colony (a). Those occupied by pygmy falcons are conspicuous due to the chalk-like fecal mat pasted around the entrance (b).
Sociable weaver colonies host other species, both avian and non-avian (Maclean, 1973b). Several bird species roost in the weaver chambers, while others also use the chambers for their own reproduction (Maclean, 1970, 1973b; Bolopo et al., 2019). Therefore, the thermal properties of chambers should be important, especially for those species that use the chambers for breeding. An obligate associate of weaver colonies is the pygmy falcon (Polihierax semitorquatus; henceforth “falcons”). Falcons are Africa’s smallest diurnal raptor (approx. 60 g), and in southern Africa they breed and roost exclusively within weaver colonies causing their distribution within this region to overlap with weavers (Maclean, 1970). Both weavers and falcons use the colonies year-round, creating a nesting association with costs and benefits to both species (Maclean, 1970). Falcons prey on weavers’ adults and chicks (Maclean, 1970; Covas et al., 2004; Spiby, 2014), and weavers alarm and often disperse when falcons are present (Lowney et al., 2020). Therefore, falcons can likely choose a chamber in the weaver colonies that are optimal for their requirements. However, chamber selection by falcons and other heterospecifics has not yet been explored. Multiple falcons may use the same chamber, with up to three individuals huddling together during winter (Lund et al., 2020), and family groups may use multiple chambers within a given colony (Bolopo et al., 2019). Interestingly, falcons defecate at their chosen chamber entrances leaving a conspicuous thick white fecal mat, though the reasons behind this remain unknown (Figure 1; Krochuk et al., 2018). Whether these fecal mats impact internal conditions within the chamber is unclear. The whiteness of the fecal mats at chamber entrances may reflect heat or the extra layer may provide further insulation (Mayer et al., 2009). Alternatively, the insulation qualities of the chamber may be diminished when compared to adjacent weaver chambers, but these possibilities remain unexplored.
Here we investigate the thermal properties of chambers in weaver colonies across a calendar year. Previous studies offered a first insight into the thermal properties of these colonial nests but have been limited to short sampling periods (from a few days up to 2 months), low sample sizes, or night-time measurements only. Here, we compiled the most extensive record of year-round day and night temperatures of nest chambers in weaver colonies to investigate (i) how chambers located in different parts of the nest mass (center to periphery) differ in insulation properties, and (ii) the yearly temporal dynamics of the chamber buffering relative to ambient temperatures. Furthermore, we explored the possible thermal properties of the chamber modifications made by the falcons depositing their feces at the entrance of the chamber. We hypothesized that the thick chalk-like fecal mat around the entrance of the chamber might provide extra amelioration against cold and hot temperature peaks. We also explore the chamber selection by falcons.
Materials and Methods
Study Site
Work was conducted at Tswalu Kalahari, a reserve in the Northern Cape Province, South Africa (27°13’30?S, 22°28’40?E). Tswalu Kalahari has a hot and arid climate with mean annual temperatures of 16.8 to 18.2°C that can exceed 40°C in summer and drop below 0°C during winter. For January and July, the mean daily maximum temperatures are 35.6°C and 21.7°C, and the mean minimum temperature is 19°C and 0.6°C, respectively. On average frost occurs 27–33 days per year (van Rooyen and van Rooyen, 2017). In the period of this study we recorded 50 days where temperatures exceeded 40°C and 36 days when temperatures dropped below 5°C. Our study area consisted of 130 km2 within the 960 km2 reserve and contained over 250 weaver colonies, mostly in the two dominant tree species: camelthorn (Vachellia erioloba) and Shephard’s tree (Boscia albitrunca). Weaver colonies at Tswalu Kalahari vary dramatically in size, and their height from the ground, in our study site, weaver nests contained an average of 50 chambers (±43 SD, range 2–244) and were on average 2.45 m from the ground (±1.07 SD, range 1–9 m).
Survey Methods
To monitor the thermal properties of weaver colonies we used Fourtec Microlite temperature loggers to record internal and ambient temperatures once every 5 min. Internal loggers were placed within the cup of nest chambers within a focal colony. Prior to placement, we inspected suitable chambers for logger insertion (see below), using a Rolson 60515 Two LED Telescopic Inspection Mirror. If eggs or chicks were observed, we would place the logger in an adjacent chamber. After placing loggers, we sealed off the chambers using chicken wire, allowing air to flow as normal and simultaneously prevent birds from entering the monitored chambers.
Sociable Weaver Chambers
We collected data on temperature within weaver chambers between 24 December 2015 and 6 January 2017. We used the R statistical package to randomly select 48 colonies to survey. For each colony sampled, we counted the number of chambers, which serve as a good proxy of nest size area (Leighton and Echeverri, 2014). In total each colony was surveyed for approximately 14 days. Initially we planned to survey each colony twice, giving 7 days of sampling each time. However, this was not always possible, therefore, some colonies were only surveyed once, and on these occasions, loggers were placed for approximately 14 consecutive days. Two colonies were sampled concurrently at any given time. After a colony had been surveyed, loggers were removed, data were downloaded and loggers were then placed in a different colony on that same day when possible. If this was not possible, then loggers were placed the following day. This meant that we increased the likelihood of acquiring max and minimum recording from every-day throughout the calendar year. As most colonies were visited twice, we usually surveyed those in opposite seasons (summer and winter, autumn and spring).
We used four loggers to record temperatures at each colony; three internally in chambers and one externally placed. Each logger was placed in a nest chamber in three different positions within a colony. The first was placed in a chamber closest to the edge of the colony, while a second was placed in a central chamber that was mid-way between the colony edges (equal distances to two edges). The third logger was placed in a chamber mid-way between the edge and center chambers. To record the ambient temperature the fourth logger was attached to the underside of a branch on the tree containing the surveyed colony, this logger was placed so that it did not receive direct sunlight. In order to understand how the location of a chamber within the nest affects its insulation properties, we recorded the depth of the chamber by measuring the length of the entrance tunnel, and the distance from the nearest edge of the colony, this was done for chambers that received temperature loggers only.
To determine the thermal properties of different weaver chambers, we extracted the highest and lowest temperature readings from each logger for each day of the survey. Additionally, we extracted the two recordings taken before and after each maximum and minimum recording (five readings in total, i.e., a 25 min period). This was to allow for a greater representation of the colony’s thermal properties.
Pygmy Falcon Chambers
To determine whether falcon fecal mats at chamber entrances effect the thermal properties of weaver chambers we placed two temperature loggers in colonies hosting falcons. The first logger was placed inside an active or recently active falcon chamber. Recently used falcon chambers are characterized by the presence of white, rather than pink, fecal mats (Maclean, 1970; Krochuk et al., 2018). The second logger was placed in a chamber immediately adjacent to the falcon chamber. Data were collected during the Austral autumn (17 April – 1 May) and winter (26 June – 12 July) of 2017 and the summer (December) of 2018. Loggers took measurements every 5 min for between 6 and 8 days. We surveyed 13 colonies, five in winter, and seven in summer. Data were extracted as detailed above. Measurements regarding the size of each colony, the chambers depth and distance from the edge of the colony were also taken.
To investigate pygmy falcon chamber location selection within colonies we used data of the falcon chambers receiving temperature loggers, here we measured the chamber depth (length of entrance tunnel), and distance from the nearest outside edge as well as counting the number of colony chambers (colony size). We added falcon chamber location data from ten additional different colonies that hosted falcons, where the same chambers measurements were taken (loggers were not deployed).
Statistical Analyses
We analyzed all data using the R statistical package 3.6.3 (R Core Team, 2020). To compare temperatures between the different logger locations (external, edge chamber, intermediate chamber, and center chamber), we carried out linear mixed models (LMMs) using the lme4 package (Bates et al., 2015). Temperature was used as the response variable for all analyses, using “day” as the sampling unit; summarizing data so that each of the response variables was calculated per logger location, per day. We compared maximum and minimum temperatures for each of the logger locations. These were obtained by calculating the mean maximum and minimum temperatures for each day, from the five readings extracted. Residual distributions of the models were inspected to assess model fit. For analyses where interactions were fitted, we explored interactions where p values < 0.05 using post hoc tests. Interactions with p > 0.05 were subsequently removed from the models. We used the emmeans and emtrends functions from the emmeans package (Lenth, 2018) to undertake post hoc analyses to check for differences between factor levels. For each response variable, the full model terms and structure, and the error distribution used are detailed in Appendix Table A1.
To compare if thermal properties of weaver colonies differed across seasons, we initially tested for differences between the three internal chamber location temperatures and the ambient temperature. The logger location (external, edge chamber, intermediate chamber, and center chamber), the size of the colony (the number of chambers), and the season (spring, summer, autumn, and winter) were used as explanatory variables. Seasons were categorized as the temperature readings taken between 1 September – 30 November as spring, 1 December – 28 February as summer, 1 March – 31 May as autumn, and 1 June – 31 August as winter. To determine if the logger location differently influenced the temperature during different seasons, we fitted interactions between logger location and season. Each colony and chamber were given a unique ID, and these were both used as random effects, with chamber ID being nested within colony ID. As two colonies were sampled at a given time, date was also used as a random effect.
Secondly, we investigated if chamber properties may explain temperature variation of weaver chambers (response variable). As explanatory variables we entered the ambient temperature, the chamber location, chamber depth, and colony size. To determine if the chamber characteristics differently influence the temperature during different seasons, we fitted interactions between the external temperature and chamber depth, the external temperature and colony size, and the external temperature and location.
To compare the thermal properties of falcon chambers with adjacent weaver chambers, we focused on the seasons with the extreme temperatures, therefore maximum temperatures collected in summer and minimum temperatures collected in winter were fitted as response variables. The logger location (falcon chamber or adjacent weaver chamber), colony size, colony depth, and the distance from the edge of the colony were used as explanatory variables. Each colony was given a unique ID that was used as a random factor. However, due to the small number of falcon chambers sampled, we used paired t-tests to determine differences between the independent chamber characteristics. No differences were observed between chamber depth, and the distance from the edge, therefore these variables were omitted from all LMMs. Furthermore, colony size shared identical variation with Colony ID, therefore colony size was also omitted from our analyses.
Results
Sociable Weaver Chambers
We collected temperature data for 703 days (mean days per tree = 14.9 ± 0.6 SE) from the 48 colonies. The maximum ambient and internal temperatures were 45.0°C (mean 31.1°C ± SE 0.3), and 44.0°C (28.0°C ± 0.15), respectively (Table 1). The maximum temperatures for chambers located at the edge of the colony were 44.0°C (28.4°C ± 0.3), while intermediate and centrally located chambers were 41.8°C (28.0°C ± 0.7) and 43.0°C (27.5°C ± 0.3), respectively (Table 1). The minimum ambient and internal temperatures were −3.1°C (16.7°C ± 0.3), and −2.1°C (19.6°C ± 0.2), respectively. The minimum temperatures for chambers located at the edge of the colony were −2.1°C (18.9°C ± 0.3), while intermediate and centrally located chambers were −0.4°C (20.0°C ± 0.3) and 0.4°C (19.8°C ± 0.3), respectively (Table 1).
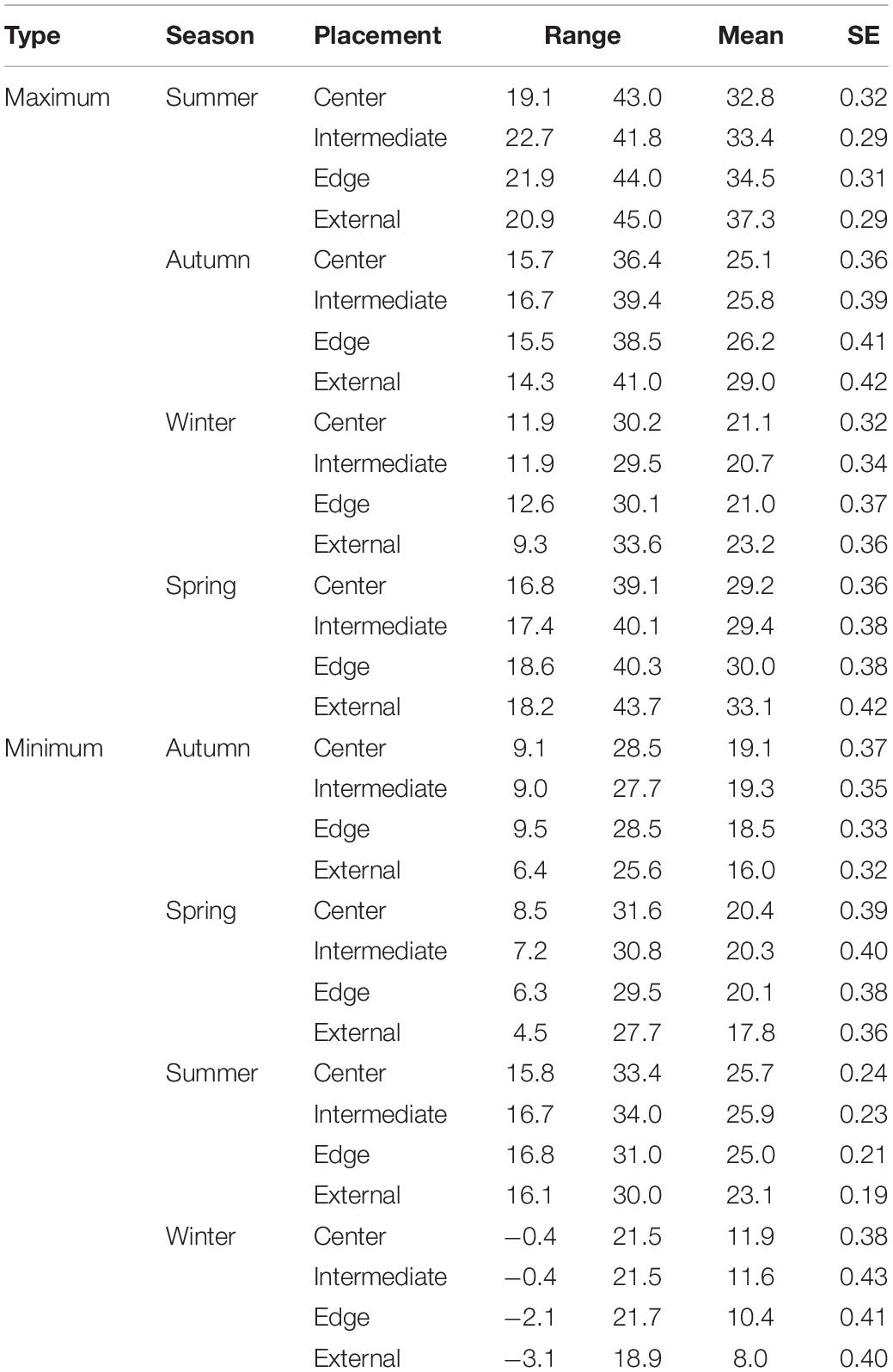
Table 1. Seasonal temperature readings including the minimum, maximum, mean, and standard error for each logger placement for maximum and minimum comparisons.
Ambient vs Internal Temperatures
Logger location and season explained significant amounts of variation of both maximum and minimum temperatures recorded (Appendix Table A1). Post hoc analyses revealed that, year-round, maximum temperatures inside colony chambers were all significantly cooler than the maximum ambient temperature, and that the differences between internal and external temperatures did not vary between seasons. Chambers on the edge of the colony were on average 2.8°C cooler (±SE 0.3, t = -13.59, and p < 0.001) than ambient temperatures. Whereas, central and intermediate chambers were 3.7°C (±SE 0.3, t = -18.22, and p < 0.001), and 3.3°C cooler (±SE 0.3, t = -15.89, and p < 0.001) than ambient temperatures, respectively. Furthermore, central chambers were on average 1.1°C cooler (±SE 0.2, t = 4.79, and p < 0.001) than chambers on the edge of the colony (Figure 2). Year-round minimum temperatures were all significantly warmer inside colony chambers compared to the external ambient temperature. Chambers on the edge of the colony were on average 2.3°C warmer (±SE 0.2) than ambient temperatures, whereas central and intermediate chambers were 3.1°C (±SE 0.2) and 3.2°C warmer (±SE 0.2) than ambient temperatures, respectively. Central and intermediate chambers were also on average 0.8°C (±SE 0.2, t = 4.446, and p < 0.001) and 0.9°C (±SE 0.2, t = 5.013, and p < 0.001) warmer than edge chambers (Figure 2). Season also explained significant variation (χ2 = 472.6, p < 0.001), with temperatures being warmer during summer and colder in winter. The interactions between logger location and season did not explain the variation and was removed from final models (Appendix Table A1).
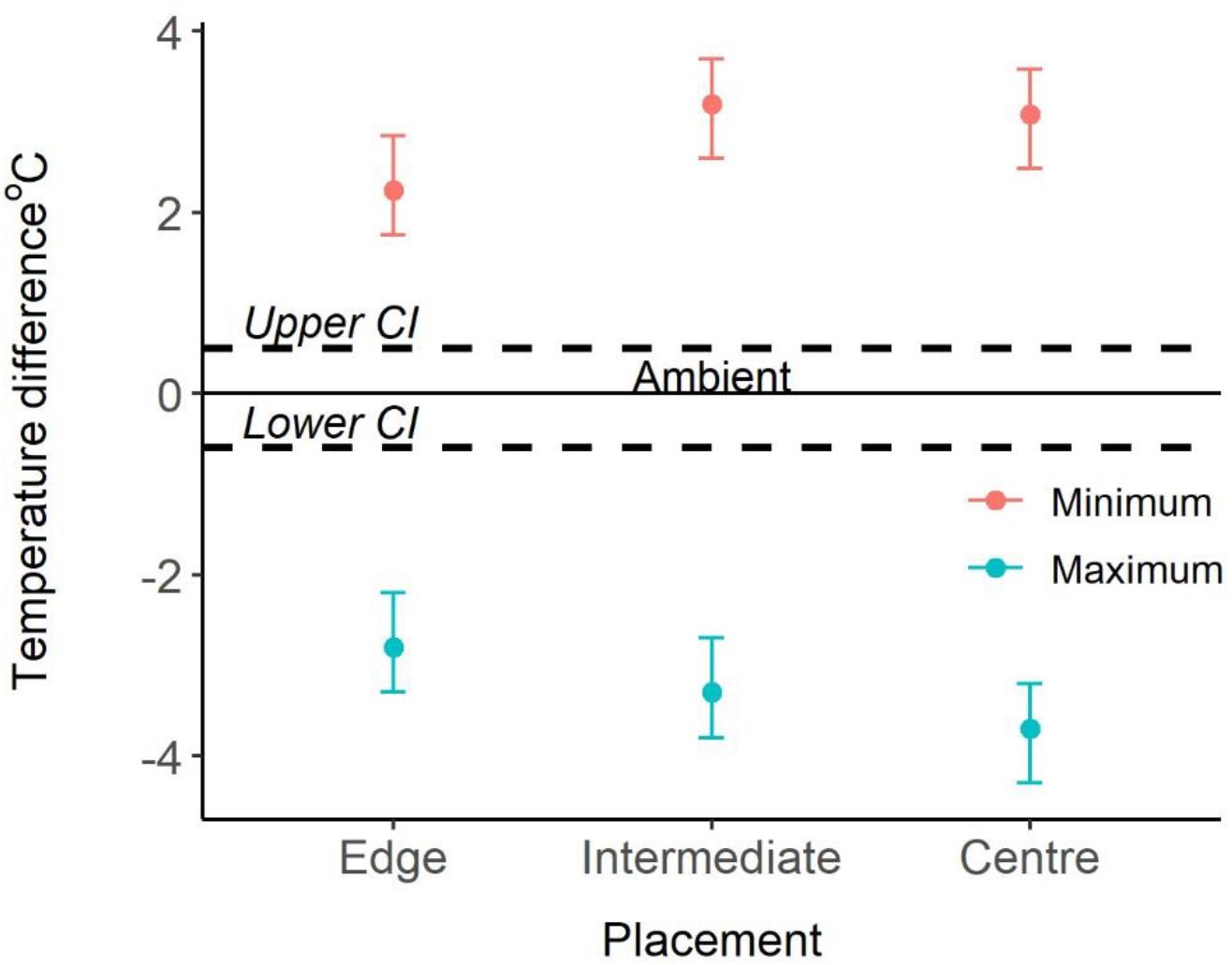
Figure 2. Comparison of ambient and internal temperatures. 0°C and the solid line represent the ambient temperature, the dotted lines represent the confidence intervals, and the points represent the difference to ambient temperatures (mean ± 95% CI).
Comparison of Colony Chambers
We collected data on the characteristics of 48 colonies and 332 nesting chambers. Colony size averaged 42 chambers (±SE 2.49, range 10–102). Edge, intermediate and central chambers were 14.0 cm ± 1.0 (mean ± SE), 46.4 cm (±2.0), and 70.5 cm (±2.8) from the periphery of the colony, respectively. While chamber depth was 16.6 (±0.4), 18.6 cm (±0.4), and 19.2 cm (±0.4), respectively. Pearson’s tests did not reveal any correlations between colony size and the chamber distance from the edge (|r| = 0.05), colony size and the depth of the chamber (| r| = 0.11), or between the chamber distance from the edge and the chamber depth (| r| = 0.16).
When we explored how the different chamber characteristics influenced temperatures within colonies, we discovered that the interactions between ambient temperatures and chamber location, and ambient temperature and chamber depth explained significant amounts of variation for both maximum and minimum temperature (Appendix Table A1). Moreover, the interaction between ambient temperature and colony size also explained significant variation between minimum temperatures (Appendix Table A1). Post hoc comparisons between chamber locations revealed that central and intermediate chambers provide stronger insulation than edge chambers for both maximum (central t = −3.01, p < 0.01; intermediate t = −2.53, p < 0.05) and minimum temperatures (central t = −2.806, p < 0.05; intermediate t = −2.42, p < 0.05). These chambers were cooler against high, and warmer against low ambient temperatures.
At the highest maximum ambient temperature (45.0°C), chambers on the edge of the colony were predicted by our models to be 7.5°C (±0.3) cooler, while intermediate and central chambers were 8.5°C (±0.4) and 9.0°C (±0.4) cooler, respectively (Figure 3). When maximum temperatures were at their lowest (9.3°C), internal chambers were all warmer. Chambers on the edge were estimated by the model to be 4.5°C (±0.5) warmer, while intermediate and central chambers were 5.1°C (±0.5) and 5°C (±0.5) warmer, respectively (Figure 3). When the ambient maximum temperatures were at 20°C, the maximum temperatures for all chambers was also 20°C (Figure 3).
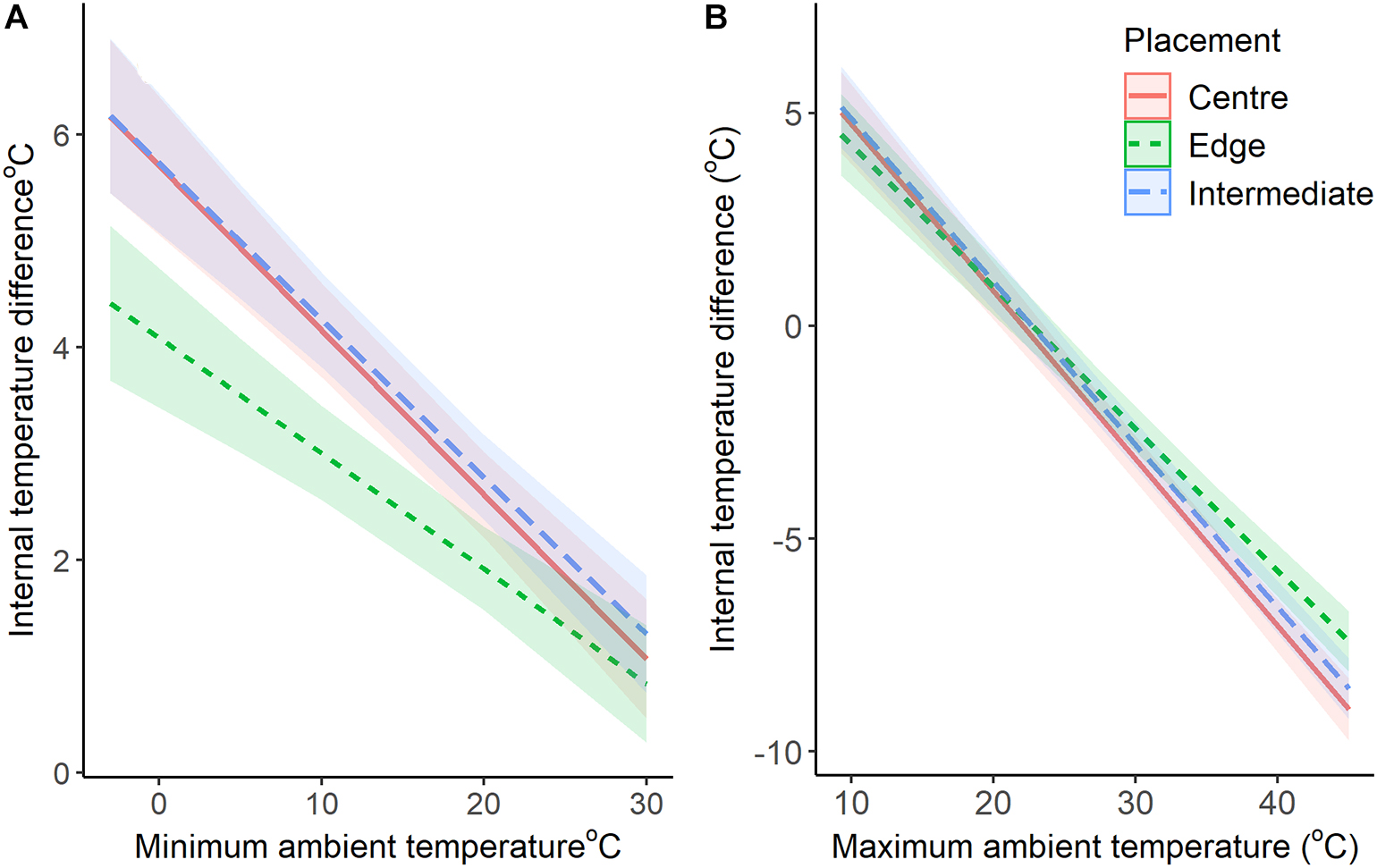
Figure 3. Comparison of the buffering capabilities of nest chambers (+95% CI) against maximum (A) and minimum (B) ambient temperatures. The lines represent the difference between internal and ambient temperatures (model predicted values with 95% confidence intervals).
At the lowest minimum temperature (−3.1°C), chambers on the edge were predicted to be 4.4°C (±0.4) warmer, while intermediate and central chambers were 6.2°C (±0.4) and 6.2°C (±0.4) warmer, respectively (Figure 3). When minimum temperatures were at their highest (30°C), chambers on the edge were estimated to be 0.8°C (±0.3) warmer, while intermediate and central chambers were 1.3°C (±0.3) and 1.2°C (±0.3) warmer, respectively (Figure 3).
Chambers with deeper entrances also provided greater insulation, being cooler during high, and warmer during low ambient temperatures (Figure 4). At the highest maximum ambient temperature (45.0°C), the deepest chambers (30 cm) were predicted to be 10.0°C (±0.6) cooler, while the shallowest chambers were predicted to be 6.5°C (±0.6) cooler (Figure 4). When maximum temperatures were at their lowest (9.3°C), deep and shallow chambers were estimated to be 5.5°C (±0.8) and 4.1°C (±0.5) warmer, respectively (Figure 4). At the lowest minimum temperature (−3.1°C), the deepest and shallowest chambers were predicted to be 6.9°C (±0.6) and 4.0°C (±0.7) warmer, respectively (Figure 4). While at the highest minimum temperatures (30°C), deeper chambers were estimated to be 0.4°C (±0.5) warmer, while shallower chambers were 1.8°C (±0.50) warmer (Figure 4).
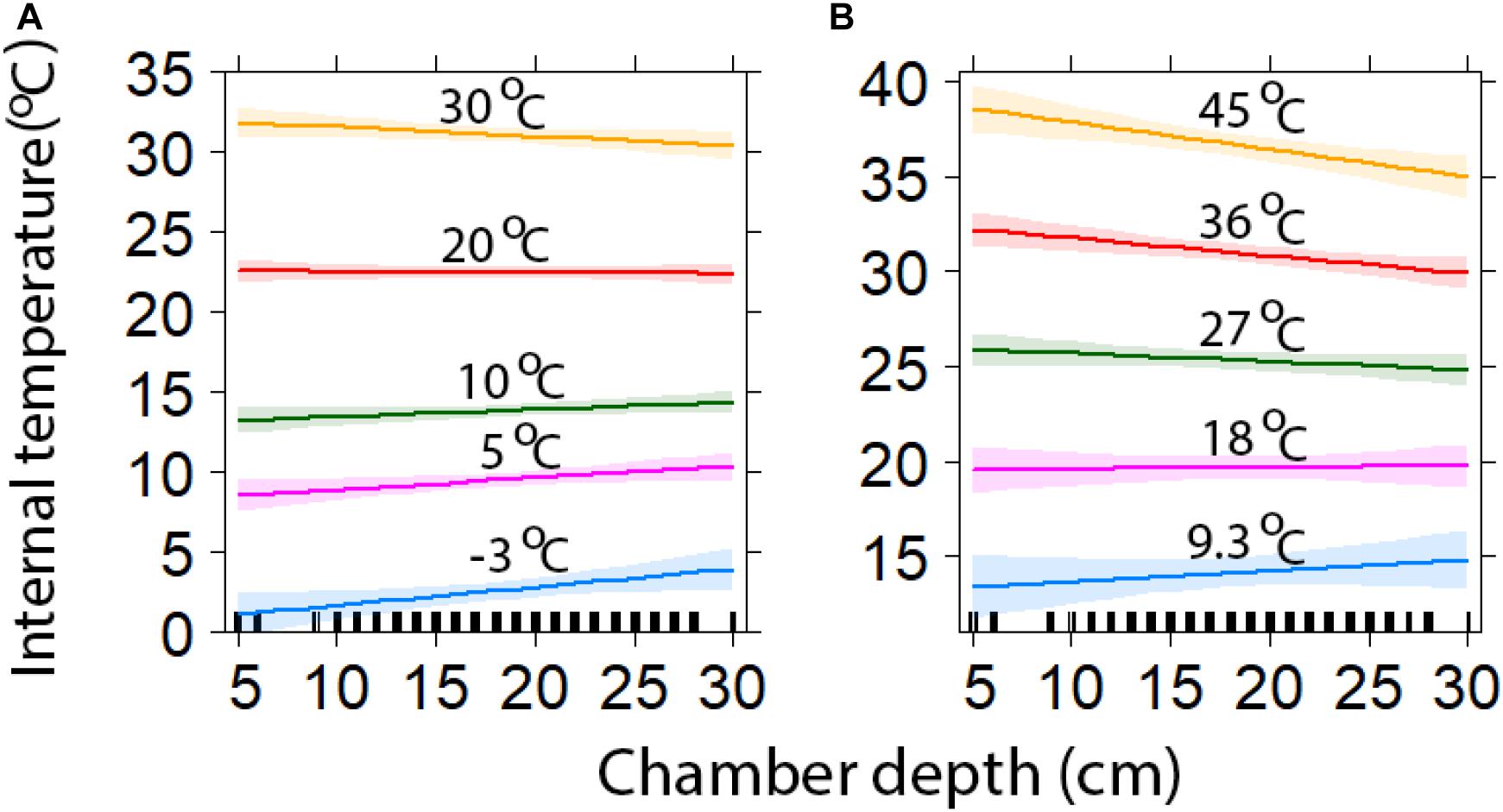
Figure 4. Comparison of chamber depth insulation against minimum (A) and maximum temperatures (±95% CI). The lines represent a range of external temperatures for a range of minimum (A) and maximum (B) values (model predicted values with 95% confidence intervals).
Chambers inside larger colonies were cooler than those inside smaller colonies when ambient temperatures were at their coldest. However, when minimum temperatures were at their highest, chambers in larger colonies were warmer than in smaller colonies (Figure 5). At lowest minimum temperature (−3.1°C), the largest and smallest colonies were predicted to be 3.8°C (±0.7) and 4.0°C (±0.7) warmer, respectively (Figure 5). While at the highest minimum temperatures (30°C), the largest and smallest colonies were estimated to be 2.4°C (±0.6) and 0.3°C (±0.4) warmer, respectively (Figure 5).
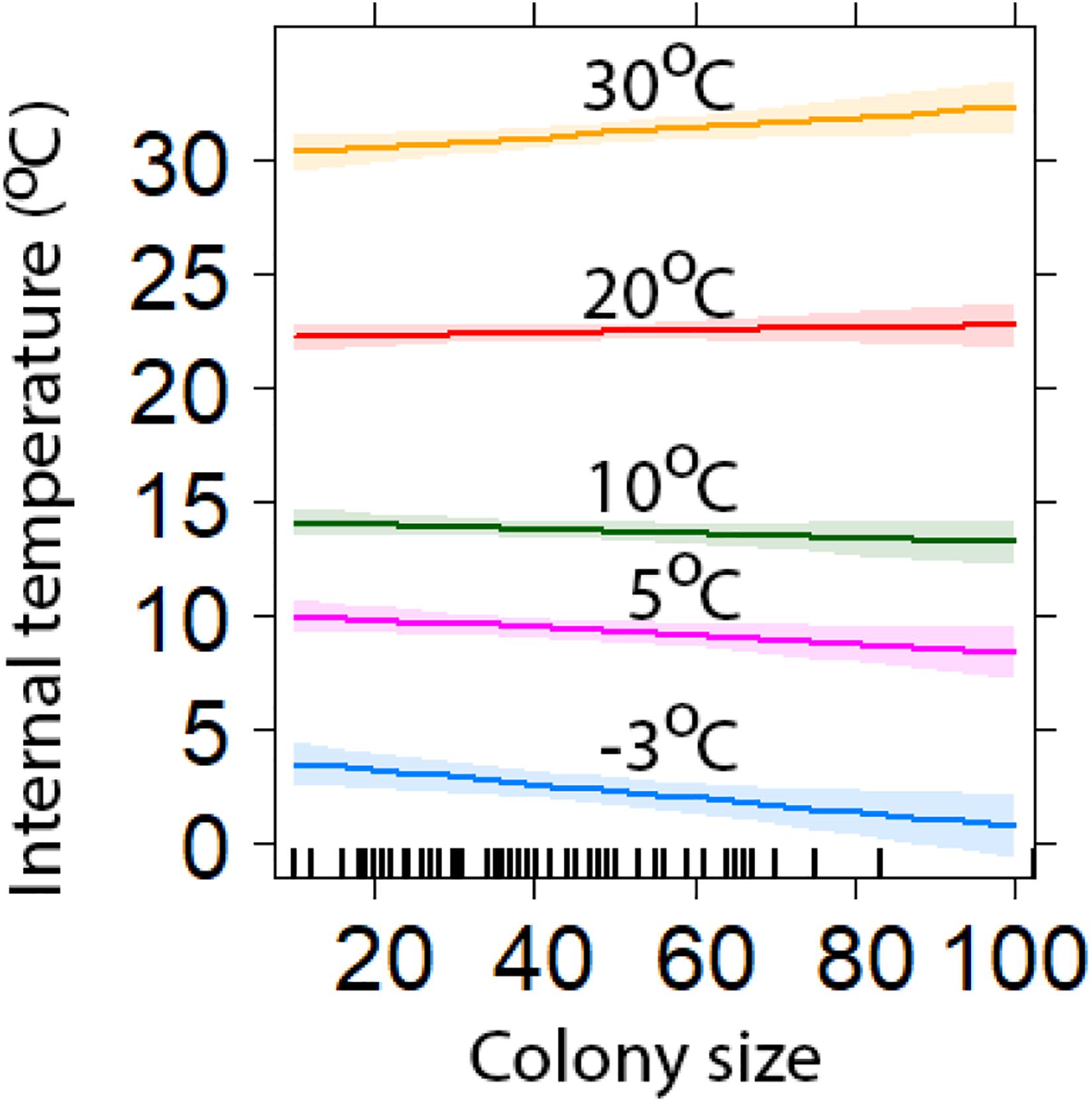
Figure 5. Comparison of the insulation capabilities of different sized colonies compared with minimum temperatures (±95% CI). Colony Size is determined by the number of chambers. The lines represent a range of external temperatures for a range of minimum values (model predicted values with 95% confidence intervals).
Pygmy Falcon Chambers
Falcon chambers were located 40.7 cm ± 4.87 (mean ± SE) from the edge of the colony and had a mean depth of 15.0 cm (±0.1). The average maximum internal temperature in summer for falcon chambers was 36.9°C (±0.4), while for adjacent weaver chambers it was 36.3°C (±0.3). The average minimum values in winter were 13.9°C (±0.5) and 14.6°C (±0.5), respectively. Paired t-tests found no difference between the falcon and adjacent weaver chambers in depth and distance from the edge, likely due to non-random selection of chambers adjacent to each other. Therefore, these two explanatory variables were omitted from the analyses. Chamber type (falcon/adjacent) did not explain significant variation in chamber temperatures for maximum (χ2 = 3.25, df = 1, and p = 0.07) and minimum (χ2 = 2.68, df = 1, and p = 0.1) temperatures. Falcon chambers were, on average 0.6°C (±0.4) warmer and 0.7°C (±0.4) cooler in summer and winter, respectively. These differences were not statistically significant; however, the p values are likely influenced by the low sample size (Appendix Table A2).
Discussion
Our study demonstrates that weaver nests provide a thermal buffer across all seasons, thus providing a more stable environment to roost, lay eggs, and raise chicks. Our results agree with previous studies demonstrating the importance of chamber positioning with regard to the quality of temperature buffering (van Dijk et al., 2013; Leighton and Echeverri, 2014). During summer, temperatures in chambers at the center of the colony were as much as 20% cooler than maximum ambient temperatures, and chambers at the edge were nearly 16% cooler. Furthermore, the deepest chambers were up to 24% cooler whereas shallower ones were 14% cooler. During winter, central chambers were up to three times warmer, and edge chambers twice as warm. These temperature differences likely result in substantial reductions in rest-phase energy expenditure (McKechnie and Lovegrove, 2001; Whitfield et al., 2015). In addition, as a permanent structure, weaver chambers also provide thermal buffering and shelter for heterospecifics. However, while pygmy falcons modify the chambers they occupy, by adding a fecal mat at the entrance, the thermal properties of these chambers did not differ from immediately adjacent chambers.
Year-Round Thermal Benefits for Sociable Weavers
The capacity of the colonies to buffer harsh ambient temperatures year-round is important for a species whose breeding depends on highly variable and unpredictable rainfall. The breeding season of weavers can last between 3 and 9 months (Covas, 2002). However, late rains can also mean that this breeding season is extended further and into the Austral winter, as was the case during the period of this study. Therefore, having a nesting environment that provides thermal protection throughout the year allows the weavers to respond appropriately and breed shortly after rains, even when ambient temperatures are outside viable incubation temperatures (25–42°C). Although all chambers provide refuge against hot and cold ambient temperatures, those located nearer the center and deeper into the nest mass provide greater insulation, and therefore have more stable temperatures, than those with short entrance tunnels and those located at the edge of a colony. Consequently, most weaver breeding occurs in those chambers with greater insulation (van Dijk et al., 2013).
The thermal properties of weaver colonies also provide benefits for roosting individuals, year-round (van Dijk et al., 2013; Leighton and Echeverri, 2014). This will allow birds to use chambers as an insulated refuge to conserve important water and energy supplies during times of extreme temperatures. During high temperatures, weavers can use the cooler chambers to reduce their heat load, while during cold temperatures they may be able to maintain body temperature and conserve important energy supplies (Paquet et al., 2016). However, when temperatures inside chambers are low, multiple weavers can huddle together in a single chamber to conserve body temperature, and further reduce the energetic requirements of thermoregulation (Paquet et al., 2016). Colonies not only provide thermal buffering against extreme hot and cold temperatures, they also provide refuge from strong winds and heavy rain-storms (personal observations), demonstrating that colonies are used by weavers to mitigate the harsh weather conditions of the arid environment. However, large communal nests are uncommon in arid environments, suggesting that there are negative aspects to building such structures and therefore warrants further investigation.
Our results suggest that as colony size increases, the insulation capability of the structure against low temperatures deteriorate. During cold spells, minimum temperatures inside larger colonies were lower than those recorded inside smaller colonies. However, when ambient minimum temperatures remained above 18°C the chambers inside larger colonies were warmer. Birds in warm environments have been shown to build nests that buffer against warm temperatures and lose heat quicker than those in colder environments (Rohwer and Law, 2010); we provide further support of this in our study. Moreover, birds in colder environments build nests that are better insulated against low temperatures and retain heat for longer periods (Rohwer and Law, 2010; Crossman et al., 2011). We are unsure why larger weaver colonies appear to have greater convective heat loss than smaller colonies. We speculate that in cold weather, weaver individuals may move from outer chambers to huddle in groups in more central chambers. This would cause central chambers to be warmer than those on the edge, and larger colonies may have more empty edge chambers that could increase the rate of heat loss. Additionally, it may be that our random colony selection had a disproportionate number of smaller colonies that were sampled during hot periods, however, correlation tests failed to demonstrate this. Therefore, how and why larger nests are colder than smaller nests during low temperatures remains uncertain, suggesting that selection for larger colonies is driven by factors other than insulation against local weather conditions and warrants further investigation. For example, roosting with potentially hundreds of other conspecifics, may reduce an individual’s risk of predation, via a dilution effect (Eiserer, 1984; Beauchamp, 1999).
Year-Round Benefits for Pygmy Falcons
The temperatures in falcon chambers were not statistically different from adjacent weaver chambers. However, our analyses may have lacked power to detect a difference due to small sample size (summer n = 7; winter n = 5). Our analyses suggested that falcon-modified chambers were on average 0.6°C warmer in summer and 0.7°C cooler in winter than adjacent chambers, therefore falcon modification decreased the buffering potential of the chambers. Despite this, falcons still gain benefits from weaver colonies, including an insulated nest and a year-round roost that they often use to avoid storms and high temperatures during the day (personal observation). In addition, falcons are obligate users of weaver colonies; therefore, they experience all of the benefits these nests provide without the energetic cost of building or maintaining them. Falcons may have the ability to defend colonies from certain predators (Maclean, 1970), but are also will also likely suffer many of the costs that weavers do, including high nest predation and failures due to colonies collapsing (Maclean, 1973a,b). Weavers do not maintain nest chambers occupied by falcons, increasing the likelihood that these particular chambers will break off the main colony (personal observation). This lack of maintenance may also explain the insulation differences between falcon and adjacent chambers. However, falcons appear to have a preference for chambers away from the center of the colony and with shorter entrance tunnels. Although it is likely that falcons would benefit from selecting chambers with better insulation, they are larger than weavers and as a result may struggle to access chambers through longer entrance tunnels. It may also be that falcon chamber selection is also driven by other factors, such as predation risk, although this is untested. Alternatively, falcons may not need chambers with the highest buffering quality, as they may have other behaviors that help them cope with extreme temperatures. For example, Sapsford (1986) reported that the falcons can enter torpor and lower their body temperature during winter, though a recent study failed to repeat these results (Lund et al., 2020). Furthermore, falcons huddle together, with up to three individuals occupying a single chamber (Lund et al., 2020).
In summary, we use extensive year-round data to demonstrate that weaver colonies provide a refuge against hot and cold temperatures for weavers and falcons, and that this insultation varies between chamber characteristics and location. Weavers and falcons not only use these structures for breeding and roosting, but also as a refuge against the sun and inclement weather (Maclean, 1973a; Bolopo et al., 2019; Lund et al., 2020). Weaver colonies are multi-functional structures, whose thermal properties create a more optimal environment for roosting, incubating and raising chicks, making this possible throughout much of the year. This is especially important for weavers, as food required for provisioning is positively linked to the unpredictable rainfall that this area experiences, and as a result weaver breeding is strongly linked to rainfall (Covas, 2002). The falcon breeding season normally occurs during spring and into summer, and does not appear to be tied so strongly to rainfall (Maclean, 1970; Bolopo et al., 2019). This means that falcons will face high temperatures during breeding; weaver chambers provide refuge from this. Additionally, many more species use weaver colonies for breeding and/or roosting, and these would be expected to gain the similar costs and benefits as weavers and falcons. However, nothing is yet known about chamber selection by other heterospecifics and whether there is a dominance hierarchy to access favored chambers. Our results demonstrate that weaver colonies provide a thermal refuge and appear to be a nest structure evolved to provide these benefits. In a landscape that is becoming increasingly harsh under climate changes (Akoon et al., 2011), the importance of these structures to the local animal communities may also increase. Due to their sheer size, sociable weaver colonies may represent an extreme case of a nest structure that buffers the impacts of seasonal weather. However, several bird species especially in arid environments construct nests that can persist year-round, for example white-browed sparrow-weavers (Plocepasser mahali) and red-billed buffalo weavers (Bubalornis niger), and we encourage more work investigating the buffering effects of these structures.
Data Availability Statement
The datasets underlying all analyses presented in this study have been archived at the University of Cape Town’s open access institutional data repository, ZivaHub (a figshare platform), where they are publicly available at https://doi.org/10.25375/uct.13042169.v1.
Ethics Statement
The animal study was reviewed and approved by University of Cape Town, South Africa (2015/V14/RT).
Author Contributions
All authors contributed to conceiving the project. AL, BK, and DB conducted the fieldwork. AL, DB, and RT contributed to analysis and interpretation of the data. AL was primarily responsible for writing the manuscript and all other authors contributed to writing, editing and, ultimately, approving of the manuscript for publication.
Funding
All authors were supported by DST-NRF Center of Excellence of the African FitzPatrick Institute of African Ornithology, and by a Tswalu Foundation grant. RT was further supported by the Academy of Finland, and the University of Turku Collegium for Science and Technology grant in setting up the study site.
Conflict of Interest
The authors declare that the research was conducted in the absence of any commercial or financial relationships that could be construed as a potential conflict of interest.
Acknowledgments
We thank two reviewers for their time in making comments and suggestions that improved this manuscript. We thank the Oppenheimer family, Tswalu Foundation, and Tswalu Kalahari for permitting us to conduct research on their property and Clement Motau for assistance with data collection. This study is part of the Kalahari Endangered Ecosystem Project (KEEP) and was supported by its sponsors.
References
Akoon, I., Archer, E., Colvin, C., Davis, C., and Diedricks, G. P. J. (2011). South African Risk and Vulnerability Atlas. Pretoria: Department of Science and Technology.
Ardia, D. R., Pérez, J. H., Chad, E. K., Voss, M. A., and Clotfelter, E. D. (2009). Temperature and life history: experimental heating leads female tree swallows to modulate egg temperature and incubation behaviour. J. Anim. Ecol. 78, 4–13. doi: 10.1111/j.1365-2656.2008.01453.x
Ardia, D. R., Pérez, J. H., and Clotfelter, E. D. (2010). Experimental cooling during incubation leads to reduced innate immunity and body condition in nestling tree swallows. Proc. R. Soc. B Biol. Sci. 277, 1881–1888. doi: 10.1098/rspb.2009.2138
Bates, D., Maechler, M., Bolker, B., and Walker, S. (2015). lme4: Linear mixed-effects Models Using Eigen and S4. R Package Version 1.1–7.2014.
Batholomew, G. A., White, F. N., and Howell, T. R. (1975). The thermal significance of the nest of the sociable weaver Philetairus socius: summer observations. Ibis 118, 402–410. doi: 10.1111/j.1474-919x.1976.tb02027.x
Beauchamp, G. (1999). The evolution of communal roosting in birds: origin and secondary losses. Behav. Ecol. 10, 675–687. doi: 10.1093/beheco/10.6.675
Bolopo, D., Lowney, A. M., and Thomson, R. L. (2019). Helpers improve fledgling body condition in bigger broods of cooperatively breeding African pygmy falcon. Behav. Ecol. Sociobiol. 73, 1–9. doi: 10.1007/s00265-018-2630-3
Briskie, J. V. (1995). Nesting biology of the yellow warbler at the northern limit of Its range. J. Field. Ornithol. 66, 531–543.
Collias, N. E., and Collias, E. C. (1964). Evolution of Nest-Building in the Weaverbirds (Ploceidae). Berkeley, CA: University of California Press.
Conradie, S. R., Woodborne, S. M., Cunningham, S. J., and McKechnie, A. E. (2019). Chronic, sublethal effects of high temperatures will cause severe declines in southern African arid-zone birds during the 21st century. Proc. Natl. Acad. Sci. U.S.A. 116, 14065–14070. doi: 10.1073/pnas.1821312116
Covas, R. (2002). Life History, Evolution and Cooperative Breeding in the Sociable Weaver. PhD thesis, University of Cape Town, Cape Town, doi: 10.1016/B0-12-226865-2/00175-9.
Covas, R., Huyser, O., and Doutrelant, C. (2004). Pygmy Falcon predation of nestlings of their obligate host, the Sociable Weaver. Ostrich 75, 325–326. doi: 10.2989/00306520409485463
Crossman, C. A., Rohwer, V. G., and Martin, P. R. (2011). Variation in the structure of bird nests between northern manitoba and southeastern ontario. PLoS One 6:e0019086. doi: 10.1371/journal.pone.0019086
Cunningham, S. J., Martin, R. O., and Hockey, P. A. R. (2015). Can behaviour buffer the impacts of climate change on an arid-zone bird? Ostrich 86, 119–126. doi: 10.2989/00306525.2015.1016469
Cunningham, S. J., Martin, R. O., Hojem, C. L., and Hockey, P. A. R. (2013). Temperatures in excess of critical thresholds threaten nestling growth and survival in a rapidly-warming arid savanna: a study of common fiscals. PLoS One 8:e0074613. doi: 10.1371/journal.pone.0074613
Dean, W. R. J., Barnard, P., and Anderson, M. D. (2009). When to stay, when to go: trade-offs for southern african arid-zone birds in times of drought. S. Afr. J. Sci. 105, 24–28. doi: 10.1590/S0038-23532009000100016
Deeming, D. C., and Mainwaring, M. C. (2015). “Functional properties of nests,” in Nests, Eggs Incubation. New Ideas About Avian Reproduction, eds D. C. Deeming and S. J. Reynolds (Oxford: Oxford University Press), 29–49. doi: 10.1093/acprof:oso/9780198718666.003.0004
DuRant, S. E., Hopkins, W. A., Hepp, G. R., and Walters, J. R. (2013). Ecological, evolutionary, and conservation implications of incubation temperature-dependent phenotypes in birds. Biol. Rev. 88, 499–509. doi: 10.1111/brv.12015
Haftorn, S. (1988). Nordic society oikos incubating female passerines do not let the egg temperature fall below the physiological zero temperature during their absences from the nest. Ornis Scand. 19, 97–110. doi: 10.2307/3676458
Heenan, C. B. (2013). An overview of the factors influencing the morphology and thermal properties of avian nests. Avian Biol. Res. 6, 104–118. doi: 10.3184/003685013X13614670646299
Heenan, C. B., and Seymour, R. S. (2011). Structural support, not insulation, is the primary driver for avian cup-shaped nest design. Proc. R. Soc. B Biol. Sci. 278, 2924–2929. doi: 10.1098/rspb.2010.2798
Kern, M. D., and van Ripper, C. III (1984). Altitudinal variations in nests of the Hawaiian honeycreeper Hemignathus virens virens. Condor 86, 443–454. doi: 10.2307/1366825
Krochuk, B. A., Bolopo, D., Lowney, A. M., Meyers, P. R., Spottiswoode, C. N., Raman, R. M. G., et al. (2018). Why defaecate on your doorstep? Investigating an unusual behaviour in Africa’s smallest falcon. Ostrich 89, 1–8. doi: 10.2989/00306525.2018.1529001
Leighton, G. M., and Echeverri, S. (2014). Non-linear influence of nest size on thermal buffering of sociable weaver nests and the maintenance of cooperative nest construction. Avian Biol. Res. 7, 255–260. doi: 10.3184/175815514X14151918723245
Lowney, A. M., Flower, T. P., and Thomson, R. L. (2020). Kalahari skinks eavesdrop on sociable weavers to manage predation by pygmy falcons and expand their realized niche. Behav. Ecol. doi: 10.1093/beheco/araa057
Lund, J., Bolopo, D., Thomson, R. L., Elliott, D. L., Arnot, L. F., Kemp, R., et al. (2020). Winter thermoregulation in free-ranging pygmy falcons in the Kalahari Desert. J. Ornithol. 161, 549–555. doi: 10.1007/s10336-020-01755-y
Maclean, G. L. (1973a). The sociable weaver, Part 2: nest architecture and social organization. Ostrich 44, 191–218. doi: 10.1080/00306525.1973.9639159
Maclean, G. L. (1973b). The sociable weaver, Part 4: predators, parasites and symbionts. Ostrich 44, 241–253. doi: 10.1080/00306525.1973.9639161
Mainwaring, M. C. (2015). “Nest construction and incubation in a changing climate,” in Nests, Eggs Incubation. New Ideas About Avian Reproduction, eds D. C. Deeming and S. J. Reynolds (Oxford: Oxford University Press), 65–74. doi: 10.1093/acprof:oso/9780198718666.003.0006
Mainwaring, M. C., and Hartley, I. R. (2008). Seasonal adjustments in nest cup lining in blue tits cyanistes caeruleus. Ardea 96, 278–282. doi: 10.5253/078.096.0213
Mainwaring, M. C., Hartley, I. R., Lambrechts, M. M., and Deeming, D. C. (2014). The design and function of birds’ nests. Ecol. Evol. 4, 3909–3928. doi: 10.1002/ece3.1054
Mainwaring, M. C., Reynolds, S. J., and Weidinger, K. (2015). “The influence of predation on the location and design of nests,” in Nests, Eggs Incubation. New Ideas About Avian Reproduction, eds D. C. Deeming and S. J. Reynolds (Oxford: Oxford University Press), 65–74.
Martin, T. E., Boyce, A. J., Fierro-Calderón, K., Mitchell, A. E., Armstad, C. E., Mouton, J. C., et al. (2017). Enclosed nests may provide greater thermal than nest predation benefits compared with open nests across latitudes. Funct. Ecol. 31, 1231–1240. doi: 10.1111/1365-2435.12819
Mayer, P. M., Smith, L. M., Ford, R. G., Watterson, D. C., McCutchen, M. D., and Ryan, M. R. (2009). Nest construction by a ground-nesting bird represents a potential trade-off between egg crypticity and thermoregulation. Oecologia 159, 893–901. doi: 10.1007/s00442-008-1266-9
McKechnie, A. E., and Lovegrove, B. G. (2001). Thermoregulation and the energetic significance of clustering behavior in the white-backed mousebird (Colius colius). Physiol. Biochem. Zool. 74, 238–249. doi: 10.1086/319669
McKechnie, A. E., and Lovegrove, B. G. (2002). Avian facultative hypothermic responses: a review. Condor 104, 705–724. doi: 10.1093/condor/104.4.705
McKechnie, A. E., and Mzilikazi, N. (2011). Heterothermy in afrotropical mammals and birds: a review. Integr. Comp. Biol. 51, 349–363. doi: 10.1093/icb/icr035
McKechnie, A. E., and Wolf, B. O. (2010). Climate change increases the likelihood of catastrophic avian mortality events during extreme heat waves. Biol. Lett. 6, 253–256. doi: 10.1098/rsbl.2009.0702
Medina, I. (2019). The role of the environment in the evolution of nest shape in Australian passerines. Sci. Rep. 9, 1–10. doi: 10.1038/s41598-019-41948-x
Meehl, G. A., and Tebaldi, C. (2004). More intense, more frequent, and longer lasting heat waves in the 21st century. Science 305, 994–998. doi: 10.1126/science.1098704
Mendelsohn, J. M., and Anderson, M. D. (1997). “Sociable weaver,” in The Atlas of Southern. African Birds, eds J. A. Harrison, D. G. Allan, L. G. Underhill, M. Herremans, A. J. Tree, V. Park, et al. (Johannesburg: Birdlife South Africa), 534–535.
Nord, A., and Williams, J. B. (2015). “The energetic costs of incubation,” in Nests, Eggs Incubation. New Ideas About Avian Reproduction, eds D. C. Deeming and S. J. Reynolds (Oxford: Oxford University Press), 152–170. doi: 10.1093/acprof:oso/9780198718666.003.0013
Orr, Y. (1970). Temperature measurements at the nest of the desert lark (Ammomanes deserti deserti). Condor 72, 476–478.
Paquet, M., Doutrelant, C., Loubon, M., Theron, F., Rat, M., and Covas, R. (2016). Communal roosting, thermoregulatory benefits and breeding group size predictability in cooperatively breeding sociable weavers. J. Avian Biol. 47, 749–755. doi: 10.1111/jav.00916
R Core Team (2020). R: A Language and Environment for Statistical Computing (Vienna: R Project). Available online at: http://www.R-project.org
Reynolds, S. J., and Deeming, D. C. (2015). “Incubating new ideas about avian reproduction,” in Nests, Eggs Incubation. New Ideas About Avian Reproduction, eds D. C. Deeming and S. J. Reynolds (Oxford: Oxford University Press), 1–7. doi: 10.1093/acprof:oso/9780198718666.003.0001
Ricklefs, R. E., and Hainsworth, R. F. (1969). Temperature regulation in nestling cactus wrens: the nest environment. Condor 71, 32–37. doi: 10.2307/1366045
Rodríguez, S., and Barba, E. (2016). Nestling growth is impaired by heat stress: an experimental study in a mediterranean great tit population. Zool. Stud. 55:13. doi: 10.6620/ZS.2016.55-40
Rohwer, V. G., and Law, J. S. Y. (2010). Geographic variation in nests of yellow warblers breeding in Churchill, Manitoba, and Elgin, Ontario. Condor 112, 596–604. doi: 10.1525/cond.2010.090229
Sapsford, C. W. (1986). Bioenergetics and hypothermia of Pygmy Falcons in the Kalahari Gemshok National. Gabar 1, 20–24.
Schaefer, V. H. (1976). Geographic variation in the placement and structure of oriole nests. Condor 78, 443–448. doi: 10.2307/1367092
Schwimmer, H., and Haim, A. (2009). Physiological adaptations of small mammals to desert ecosystems. Integr. Zool. 4, 357–366. doi: 10.1111/j.1749-4877.2009.00176.x
Sidis, Y., Ziberman, R., and Ar, A. (1994). thermal aspects of nest placement in the orange-tufted sunbird (Nectarinia osea). Auk 111, 1001–1005. doi: 10.2307/4088835
Smith, E. K., O’Neill, J. J., Gerson, A. R., McKechnie, A. E., and Wolf, B. O. (2017). Avian thermoregulation in the heat: resting metabolism, evaporative cooling and heat tolerance in Sonoran Desert songbirds. J. Exp. Biol. 220, 3290–3300. doi: 10.1242/jeb.161141
Spiby, J. (2014). Not so friendly neighbours: pygmy falcon eating sociable weaver nestling. Ornithol. Obs. 5, 357–360.
Tieleman, B. I., Noordwijk, H. J. V. A., and Williams, J. B. (2008). Nest site selection in a hot desert: trade-off between microclimate and nest site selection in a hot desert: trade-off between microclimate and predation risk? Condor 110, 116–124. doi: 10.1525/cond.2008.110.1.116.116
Tieleman, I. B., Williams, J. B., Buschur, M. E., and Brown, C. R. (2003). Phenotypic variation of larks along an aridity gradient: are desert birds more flexible? Ecology 84, 1800–1815. doi: 10.1890/0012-9658(2003)084[1800:pvolaa]2.0.co;2
Töpfer, T., and Gedeon, K. (2012). The construction and thermal insulation of Ethiopian Bush-crow (Zavattariornis stresemanni) nests: a preliminary study. Avian Biol. Res. 5, 198–202. doi: 10.3184/175815512X13534399582256
van Dijk, R. E., Kaden, J. C., Argüelles-Ticó, A., Beltran, L. M., Paquet, M., Covas, R., et al. (2013). The thermoregulatory benefits of the communal nest of sociable weavers Philetairus socius are spatially structured within nests. J. Avian Biol. 44, 102–110. doi: 10.1111/j.1600-048X.2012.05797.x
van Rooyen, N., and van Rooyen, G. (2017). Ecological Evaluation of Tswalu Kalahari Reserve. Pretoria: Ekotrust CC.
Walsberg, G. E., and Voss-Roberts, K. A. (1983). Incubation in desert-nesting doves: mechanisms for egg cooling. Physiol. Zool. 56, 88–93. doi: 10.1086/physzool.56.1.30159969
Weathers, W. W., and Sullivan, K. A. (1989). Nest attentiveness and egg temperature in the Yellow-eyed Junco. Condor 91, 628–633. doi: 10.2307/1368113
Webb, D. R. (1987). Thermal tolerance of avian embryos: a review. Condor 89, 874–898. doi: 10.2307/1368537
White, F. N., Batholomew, G. A., and Howell, T. R. (1975). The thermal significance of the nest of the sociable weaver Philetairus socius: winter observations. Ibis 117, 171–179. doi: 10.1111/j.1474-919x.1975.tb04205.x
Whitfield, M. C., Smit, B., McKechnie, A. E., and Wolf, B. O. (2015). Avian thermoregulation in the heat: scaling of heat tolerance and evaporative cooling capacity in three southern African arid-zone passerines. J. Exp. Biol. 218, 1705–1714. doi: 10.1242/jeb.121749
Williams, J. B. (1996). ““Energetics of avian incubation,” in Avian Energetics and Nutritional Ecology, ed. C. Carey (New York, NY: Champan and Hall), 375–415. doi: 10.1007/978-1-4613-0425-8_11
Appendix
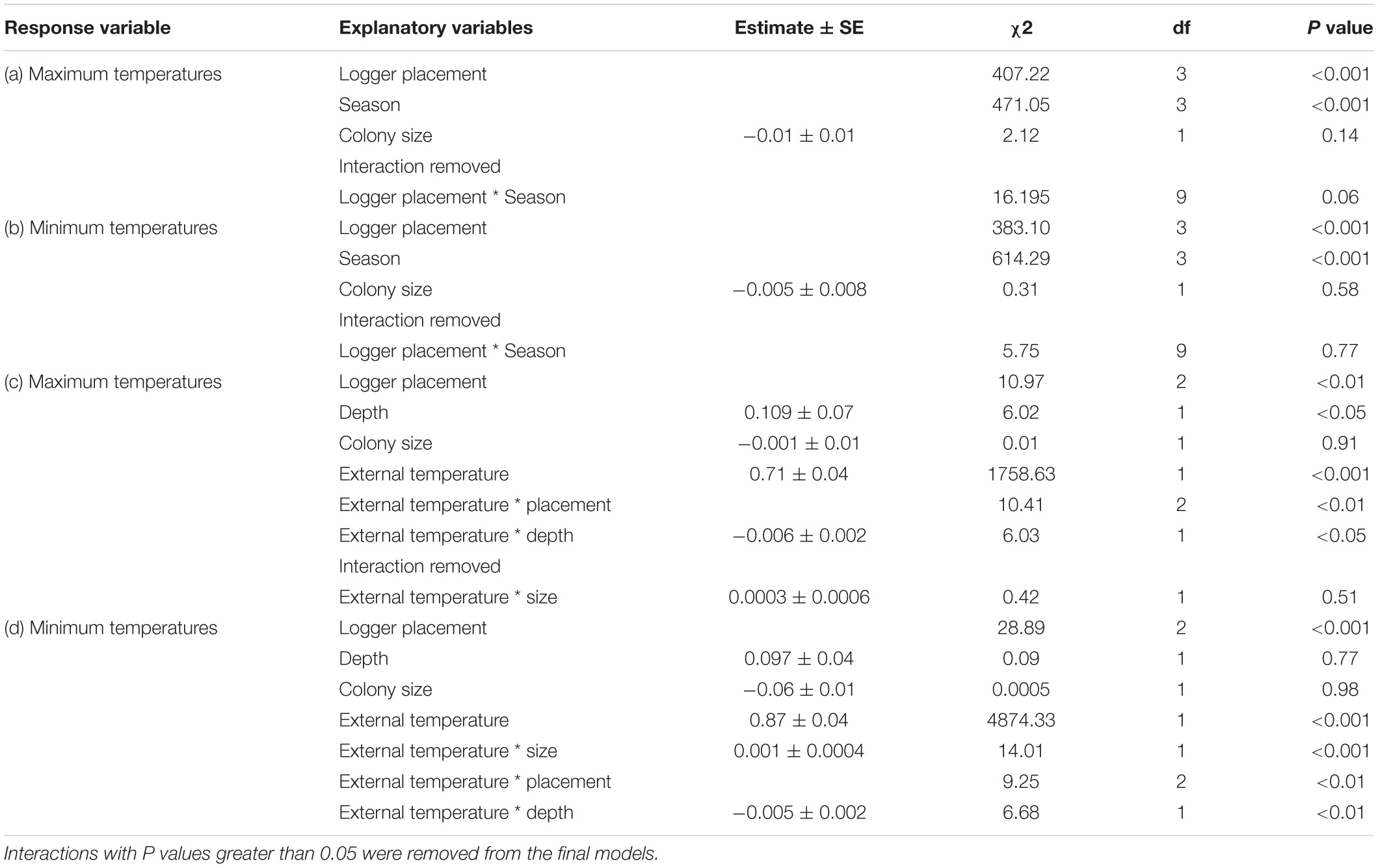
TABLE A1. Linear mixed models investigating the maximum and minimum temperature variables to logger placement, season, and colony size.
Keywords: ecosystem engineers, community ecology, positive interactions, thermal refuge, environmental adjustment, shelter, Harsh climate, Environmental adaptations
Citation: Lowney AM, Bolopo D, Krochuk BA and Thomson RL (2020) The Large Communal Nests of Sociable Weavers Provide Year-Round Insulated Refuge for Weavers and Pygmy Falcons. Front. Ecol. Evol. 8:570006. doi: 10.3389/fevo.2020.570006
Received: 05 June 2020; Accepted: 25 September 2020;
Published: 03 November 2020.
Edited by:
Stuart Peter Sharp, Lancaster University, United KingdomReviewed by:
Charles Deeming, University of Lincoln, United KingdomMark C. Mainwaring, University of Montana, United States
Copyright © 2020 Lowney, Bolopo, Krochuk and Thomson. This is an open-access article distributed under the terms of the Creative Commons Attribution License (CC BY). The use, distribution or reproduction in other forums is permitted, provided the original author(s) and the copyright owner(s) are credited and that the original publication in this journal is cited, in accordance with accepted academic practice. No use, distribution or reproduction is permitted which does not comply with these terms.
*Correspondence: Anthony M. Lowney, YW50aG9ueW0ubG93bmV5QGdtYWlsLmNvbQ==