- 1Department of Biology and Wildlife, University of Alaska Fairbanks, Fairbanks, AK, United States
- 2Gates of the Arctic National Park and Preserve, Arctic Inventory and Monitoring Network, National Park Service, Fairbanks, AK, United States
- 3Institute of Arctic Biology, University of Alaska Fairbanks, Fairbanks, AK, United States
A distinguishing characteristic of many migratory animals is their annual return to distinct calving (birthing) areas in the spring, yet the navigational mechanisms employed during migration that result in this pattern are poorly understood. Effective conservation of these species requires reliable delineation of such areas, quantifying the factors that influence their selection, and understanding the underlying mechanisms resulting in use of calving areas. We used barren-ground caribou (Rangifer tarandus granti) as a study species and identified calving sites of the Western Arctic Herd in Alaska using GPS collar data from 2010–2017. We assessed variability in calving areas by comparing spatial delineations across all combinations of years. To understand calving area selection at a landscape scale, we performed a resource selection analysis comparing calving sites to available locations across the herd’s range and incorporated time-varying, remotely sensed metrics of vegetation quality and quantity. We found that whereas calving areas varied from year to year, this annual variation was centered on an area of recurring attraction consistent with previous studies covering the last six decades. Calving sites were characterized by high-quality forage at the average time of calving, but not peak calving that year, and by a narrow range of distinct physiographic factors. Each year, calving sites were located on areas of above-average conditions based on our predictive model. Our findings indicate that the pattern of spring migration for pregnant females was to migrate to areas that consistently provide high-quality forage when averaged across years, and then upon arriving at this calving ground, refine selection using their perception of annually varying conditions that are driven by environmental stochasticity. We suggest that the well-documented and widespread pattern of fidelity to calving grounds by caribou is supportive of a navigational mechanism based on spatial memory at a broad scale to optimize foraging and energy acquisition at a critical life-history stage. The extent to which migrants depend on memory to reach their spring destinations has implications for the adaptability of populations to changing climate and human impacts.
Introduction
Migration is a behavioral adaptation to seasonal environmental conditions and resource availability (Alerstam et al., 2003; Avgar et al., 2014). How animal movement relates to resource conditions is scale dependent (Bailey et al., 1996), such that movements within patches of resources (MacArthur and Pianka, 1966; Charnov, 1976) can scale up to landscape-scale use patterns and can result in the dramatic movements characteristic of migratory animals (Shaw and Couzin, 2013). A current challenge in the field of animal ecology is to understand the influence of the navigational mechanisms responsible for large-scale movements such as migration. These mechanisms broadly fall into two domains: perception-based movements, where animals follow immediately perceived resource gradients to track high-quality resources as they arise, or memory-based movements, where animals use previous experience to direct their movements to areas of high-quality resources outside of the immediately perceptible zone (Avgar et al., 2013; Fagan et al., 2013). Examples of perception-based movement include animals “surfing a green wave” of high-quality forage as it moves across a spatial gradient (van der Graaf et al., 2006; Merkle et al., 2016; Aikens et al., 2017), whereas memory-based movements are characterized by animals moving independently of proximal resource gradients and moving to distant areas of high-quality resources (Howery et al., 1999; Polansky et al., 2015; Bracis and Mueller, 2017). Because these navigational processes cannot be directly measured in wild animals, inferring their relative influence from movement data requires integrating empirical observations with theoretical and experimental findings (Fagan et al., 2013).
Spring migration of females in many migratory ungulate species culminates with parturition (hereafter calving), with females often aggregating on calving areas. Such species include blue wildebeest (Connochaetes taurinus; Estes, 1976), Tibetan antelope (Pantholops hodgsonii; Schaller et al., 2006), Saiga antelope (Saiga tatarica; Bekenov et al., 1998), and barren-ground caribou (Rangifer tarandus; Kelsall, 1968). Calving begins a period of heightened nutritional demand for pregnant females caused by the high energetic cost of lactation (Chan-McLeod et al., 1994). Owing to this demand, females are hypothesized to synchronize calving with periods of high vegetative quality (Oftedal, 1985; Miller-Rushing et al., 2010) and, indeed, selection for calving areas has been linked to vegetative productivity for some species [Tibetan antelope, Ganzorig et al., 2011; Mongolian gazelle (Procapra gutturosa), Leimgruber et al., 2001]. Spring vegetative productivity has also been positively associated with offspring condition (Pettorelli et al., 2005b, 2006). An alternative explanation for calving area selection is that females attempt to space away from predators (Bergerud, 1996; Creel et al., 2005), but testing the influence of these two hypotheses is often difficult.
Calving aggregations are typically highly vulnerable to human disturbance because a large percentage of the population is concentrated in relatively small areas at calving and anthropogenic influences can strongly alter female behavior (Nellemann and Cameron, 1998; Joly et al., 2004; Singh et al., 2010). Survival of neonates is a critical factor in population dynamics (Gaillard et al., 2000) and lactation performance directly affects offspring growth (White, 1992; Crête and Huot, 1993), meaning conservation of calving areas is likely a key component to managing these populations (Taillon et al., 2012). Considering that calving areas typically exhibit some level of annual variability (Lent, 1966; Skoog, 1968; Griffith et al., 2002), documenting annual use at decadal scales and understanding the mechanistic processes driving selection of these areas are critical for effective, long-term conservation (Singh and Milner-Gulland, 2011; Wilson et al., 2012). Understanding these processes before anthropogenic development has taken place is essential; inferences about calving selection after development has taken place will likely be confounded by risk effects and avoidance behavior, introducing potentially large and unknown biases to calving site selection (Harju et al., 2011).
To address these issues in a unified approach, we used migratory barren-ground caribou as a study species and investigated the use of calving areas across 8 years for the Western Arctic Herd (WAH) in Alaska, one of the largest caribou herds in the world. Barren-ground caribou are an excellent study species for this approach, for calving marks the destination of long-distance migration in the spring for pregnant females, which aggregate around calving and generally exhibit inter-annual fidelity to their calving grounds (Kelsall, 1968). We defined three scales of calving: individual calving sites in a given year (first scale) comprise an annual calving area (second scale), that in turn constitute a calving ground when considered across numerous years (third scale; Figure 1; Gunn and Miller, 1986). Our goals were to (1) document spatial trends in the calving areas of the WAH, (2) investigate the landscape-level factors influencing selection for calving sites to better understand the emergent spatial patterns of calving areas, and (3) interpret our findings to better understand what navigational mechanisms could explain the phenomenon of fidelity of caribou to their calving grounds. We hypothesized that if females exhibited primarily perception-based selection, calving sites would be characterized by low interannual consistency and track high-quality vegetation for each year. Alternatively, if selection were primarily memory-based, calving sites would be characterized by high interannual consistency and high-quality vegetation, as averaged across the study period, but not necessarily the best site in any given year.
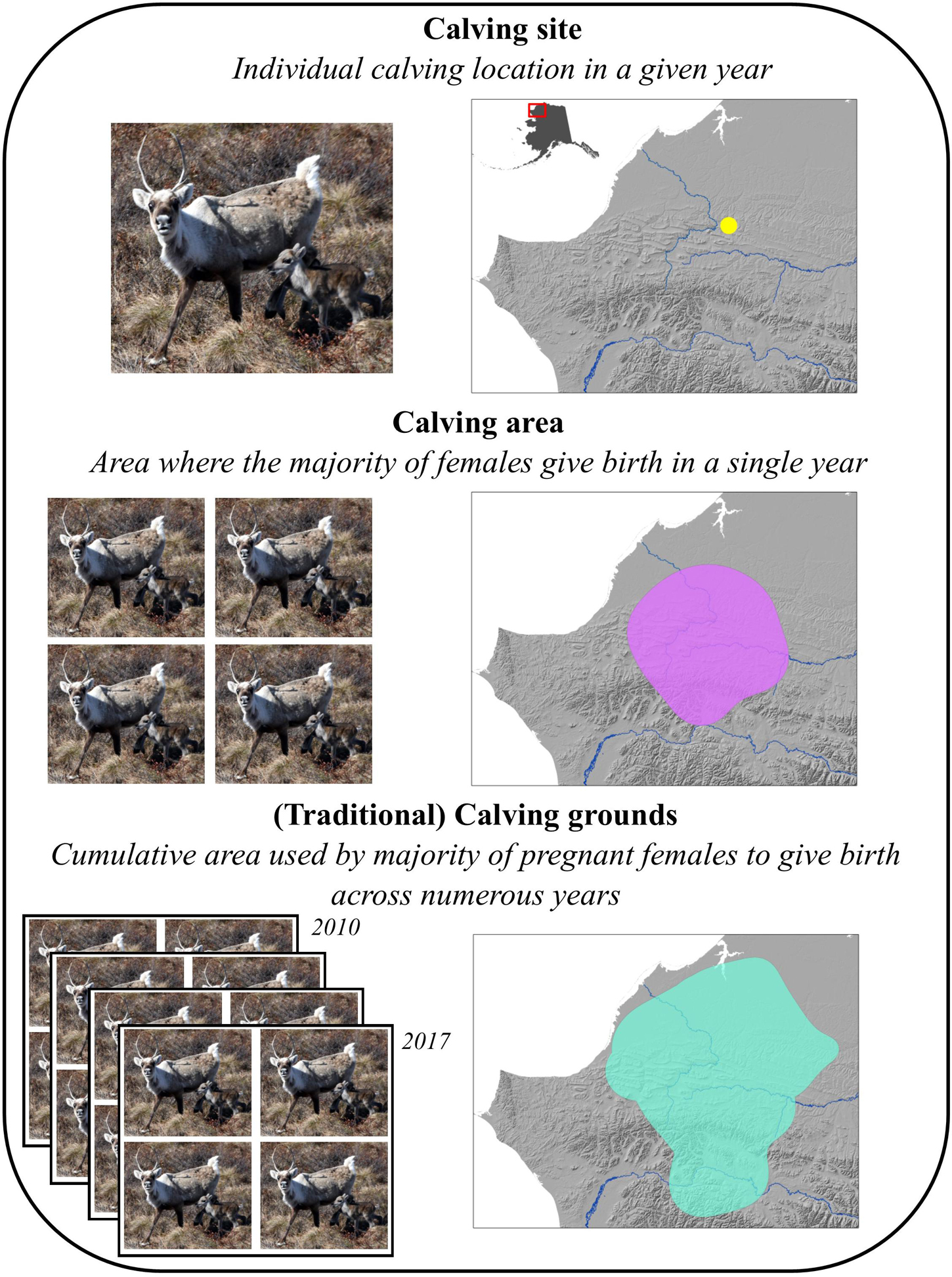
Figure 1. The scales and definitions of calving considered in this analysis of the Western Arctic Herd. The calving location (yellow point) was from an individual in 2010, the calving area (purple polygon) was based on all detected calving events in 2010, and the calving ground (teal polygon) was the extent of all calving areas from 2010–2017 combined. Definitions adapted from Gunn and Miller (1986).
Materials and Methods
Study Species
The WAH utilizes over 350,000 km2 of northwestern Alaska, typically migrating from wintering areas in the south, which vary by year and individual, to the calving ground and summer range in the north (Figure 2; Lent, 1966; Dau, 2015, Joly and Cameron, 2019). Calving generally occurs May 31–June 13 (Cameron et al., 2018). Beginning in 2009, GPS collars (Telonics, Mesa, AZ) were deployed annually on adult female caribou (≥2years old) as they swam across the Kobuk River during fall migration (Dau, 1997; Joly et al., 2012). Captures were conducted using procedures approved by the State of Alaska Institutional Animal Care and Use Committee (IACUC; 0040-2017-40). Collars were programmed to record locations every 8 h and by 2017, 203 collars had been deployed. From 2003 to 2016, the herd decreased from a high of 490,000 to 201,000 caribou and then increased to 244,000 in 2019 (Alaska Department of Fish and Game, 2019). Caribou populations are known to fluctuate at decadal time scales, and this is generally linked with large-scale climate patterns (Gunn, 2003; Joly et al., 2011). The northeast extent of the WAH range overlaps with the neighboring Teshekpuk Herd, and individuals between the two herds have been known to mix (Mager et al., 2013; Prichard et al., 2020).
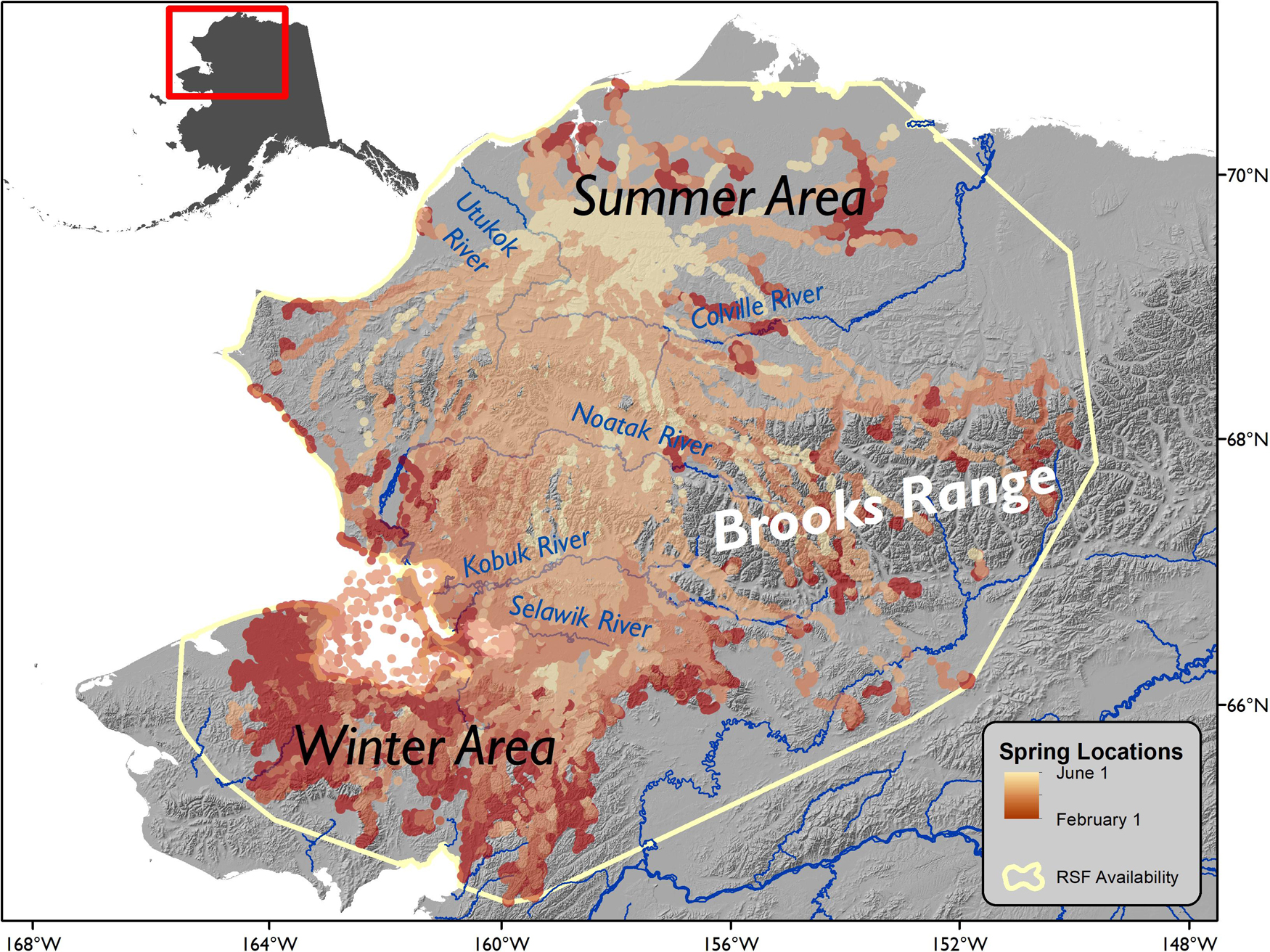
Figure 2. Annual range of Western Arctic Herd caribou, Alaska. All GPS points from February 1 (dark red) to June 1 (yellow) are displayed from 2009–2017. The 100% minimum convex polygon, indicating the extent of the available area for the resource selection function (RSF), is presented as a pale yellow line and was generated using all annual locations in the same span of years.
Identifying Calving Events
We applied two different approaches to infer calving events from the 2010–2017 GPS data: an individual-based method and a population-based method (DeMars et al., 2013; Cameron et al., 2018). The former fit two a priori movement models (parturient and non-parturient) to movement rate data and model fit was evaluated using information criteria. The second method established a herd-specific movement rate threshold for calving from known events and then analyzed movement rates for individuals that dropped below the threshold using a 3-day smoothing parameter (DeMars et al., 2013). Using instances when the two methods agreed resulted in accurately classifying calving events 89% of the time (n = 119) when compared to aerial observation data (Cameron et al., 2018).
For data spanning 2010–2015, we used the calving events as reported in Cameron et al. (2018), in which aerial data were used to validate identified calving events from the movement-based approaches. For the data spanning the calving period of 2016–2017, we followed the procedures outlined in Cameron et al. (2018) to identify calving events without relying on supporting aerial data. However, because there was a record number of non-migratory individuals during the winter of 2016–2017 (Joly and Cameron, 2019) and the individual-based method is ill-suited for individuals not exhibiting migration movements prior to calving (Cameron et al., 2018), we incorporated a designation of migratory and non-migratory for each individual and adjusted the analysis as follows. For individuals that migrated (identified as crossing at least one of the three major rivers separating summer and wintering areas), we used the calving events from instances of method agreement. For individuals that did not migrate to a southern wintering area that year and that the two model results disagreed, we used calving events identified by the population-based method. For calving sites, we used the GPS location that corresponded with the identified calving event from the population-based method, because the individual-based method appeared to label events one GPS interval early (Cameron et al., 2018).
Spatial Patterns in Calving Areas
To address our first goal of spatial trends in calving areas, we defined an annual calving area as the area used by the majority (>80%) of individuals for calving in the herd in a given year (Gunn and Miller, 1986). We calculated a kernel utilization distribution (Worton, 1989) based on the calving sites for each year using the package “adehabitatHR” version 0.4.14 (Calenge, 2006) in the R statistical program version 3.4.3 (R Core Team, 2017). In this approach, a bivariate normal probability distribution is centered over each calving site in a given year and averaged together, resulting in one distribution (the kernel) for each year. Kernels were generated using a 500 × 500 m grid in an Albers equal area projection, which minimizes distortion along the latitudinal gradient given the relatively high latitude of our study area and ensured valid comparisons between years (Snyder, 1987). All kernels were generated using the same bandwidth smoothing parameter (h = 25,000) and we used the 95% contour as they resulted in unbroken range delineations for all years (Hooten et al., 2017). This approach, which is based on the explicit calving sites, minimizes potential bias in delineating calving areas that can be introduced by mismatches between calving timing and aerial observation timing during traditional surveys (Gunn and Miller, 1986).
To test the null hypothesis that the spatial distribution of annual calving areas did not vary by year, we employed a kernel randomization analysis outlined by Breed et al. (2006). For comparisons between 2 years, we randomly assigned (without replacement) a year designation to each calving site. Then, kernels for both years were generated using the same grid and smoothing parameters as outlined above. The area of both randomized kernels was then computed, as well as the area of overlap between the two kernels. Last, we calculated the test statistic as the area of the kernel overlap divided by the largest area of the two kernel regions. We repeated this process 250 times without duplicating any random year assignments. The p-value was calculated as the proportion of random overlaps smaller than the observed overlap for the 2 years being considered, so that if the observed overlap was smaller than all observed overlap values, the p-value was <0.004 (see Supplementary Figure 1 for illustration). We performed this analysis for all combinations of annual comparisons, ranging from sequential up to 7-year intervals, and considered our alpha level as 0.05 for a one-tailed test.
Range-Wide Calving Site Selection
Our other goals were to understand the biotic and abiotic factors driving caribou calving site selection at the landscape level and the navigational mechanisms caribou employ to arrive there. We performed a resource selection function analysis (RSF; Manly et al., 2002) using the calving sites each year and compared them with random locations from the herd’s range, representing the third-order of selection (Johnson, 1980). To define range-wide availability, we drew a 100% minimum-convex polygon, constrained to the coastal boundary, around all GPS locations during the study period. Defining availability is a particular challenge for resource selection studies, with the implicit assumption that available points are unused and available to all individuals (Keating and Cherry, 2004; Aarts et al., 2008). We focused on a range-wide scale for this analysis because individuals in the herd used the polygon area throughout the 8 years of study and we detected calving events at the extreme southwestern and northeastern extent of the range, far outside of the traditionally defined calving area. For each of the 8 years from 2010 to 2017, we created 10,000 random locations within the polygon, for a total of 80,000 available points.
We attributed both used and available points with a combination of physiographic attributes and annually varying environmental indices. We attributed elevation values from a 5 m resolution digital terrain model derived from the Interferometric Synthetic Aperture Radar (U.S Geological Survey, 2017) and calculated a solar radiation index (Keating et al., 2007) for each point using slope and aspect derived from the terrain model. This index ranges from −1 to 1, with low index values corresponding to north-facing steep slopes, high values south-facing steep slopes, and flatter slopes around 0.35. We calculated a vector ruggedness measure (VRM; Sappington et al., 2007), which is a measure of the ruggedness of the terrain, for each point using the digital terrain model and a 15 × 15 m swath. We used a land cover classification map (Boggs et al., 2016) to attribute all points with land cover type and reduced the classifications into four categories from the original 20 based on diet categories of the predominant vegetation (forest, shrub, herbaceous, and lichen/sparse; Supplementary Table 1). We filtered points that occurred in pixels originally categorized as bare ground, fire scar, ice/snow, and water.
For environmental indices, we attributed the annual snow off date (day of year) specific to that year for each point as determined from Moderate Resolution Imaging Spectroradiometer (MODIS) data (Macander et al., 2015). We included two measures of primary productivity at multiple time intervals using the normalized difference vegetative index (NDVI, for review see Pettorelli et al., 2005a) acquired from the MODIS V6 and compiled into 7-day composites with 250 m resolution (data available from the U.S. Geological Survey; Jenkerson et al., 2010). For an index of forage quantity, we used the raw NDVI value at a weekly temporal resolution and as an index of forage quality we calculated the change in NDVI values between sequential NDVI composites (NDVI rate) for the same time period by calculating the difference between sequential coverages (denoted “ΔNDVI”). ΔNDVI has been used in prior studies as an index for forage quality, including in Africa (Boone et al., 2006) and Alaska (Griffith et al., 2002), and also used to calculate a similar measure, the Instantaneous Rate of Green-up (Bischof et al., 2012). For arctic vegetation, a positive change in NDVI during spring corresponds with phenological periods of high nutrient concentrations and rapid vegetation growth (Finstad, 2008; Gustine et al., 2017).
We included five temporal windows (1 week before peak calving, the week of the peak, and the following 3 weeks after peak calving) for both NDVI metrics to assess at what temporal scale caribou may be responding to vegetation signals. To evaluate support for perception-based selection, we assigned the five temporal windows for both NDVI metrics relative to peak calving for that specific year, with the effect that the week of peak calving NDVI values differed between years and corresponded to the timing of calving observed the given year (perception of current conditions). To evaluate the potential for memory-based selection, we assigned these temporal windows relative to the herd’s average peak calving across all 8 years (June 3, see section “Results”), such that regardless of when peak calving was in a given year, both NDVI metrics represented consistent weeks across years (average conditions). This framework is similar to work assessing the influence of perception and memory in zebra (Equus burchelli) migration (Bracis and Mueller, 2017).
We tested the influence of these biotic and abiotic factors on caribou calving site selection using mixed-effects logistic regression, with use of a calving site as the response. We log-transformed elevation and VRM to approximate a normal distribution and standardized continuous covariates (mean centered and divided by the standard deviation) for model fitting. Correlation coefficients among physiographic attributes were under 0.5, and they all were under 0.2 when compared with environmental variables. We included a random intercept term for year to account for sampling across time and considered random slope terms for the environmental variables to account for stochastic annual variability (Gillies et al., 2006). We performed model selection at two stages – the first to select a random effect structure and the second to select fixed effect variables and structures (Bolker et al., 2009) using model selection based on Akaike’s Information Criterion corrected for small sample sizes (AICc; Hurvich and Tsai, 1989; Burnham and Anderson, 2002). For all NDVI, ΔNDVI, and snow-free variables, we fitted full fixed-effects models with a random slope term for each environmental covariate (including a random intercept for year) and compared performance with an intercept-only random effects model. In the second stage of model selection, we proceeded with fixed-effects selection using the top-performing random effect structure from the previous stage and included all biologically justifiable interactions and combinations. All analyses were performed in the R statistical program using the package “lme4” (Bates et al., 2015). We used our top model to generate a predictive map for calving sites by averaging the selected environmental covariate raster across the 8 years, as well as generated year-specific predictive maps with the corresponding environmental data for that year. We calculated the average of the year-specific predictive values within each annual calving area and compared these with the calving site values for the given year.
Results
Identifying Calving Events
From 2010 to 2017, we detected 214 total calving events, ranging from 15 to 52 in a given year, and the average calving date was June 3 (Table 1). We identified calving in one non-migratory individual in 2016 and 14 in 2017 for which we used results from only the population-based model. We detected four calving events outside of the historical calving grounds: one in 2012 for an individual that remained on the winter range of the Seward Peninsula and three in 2017 for WAH individuals that calved in the Teshekpuk Herd calving area to the east. For the subsequent analyses of calving area trends and selection, we excluded these four events because they greatly skewed calving distribution estimates, leaving us with 210 total calving events across 8 years.
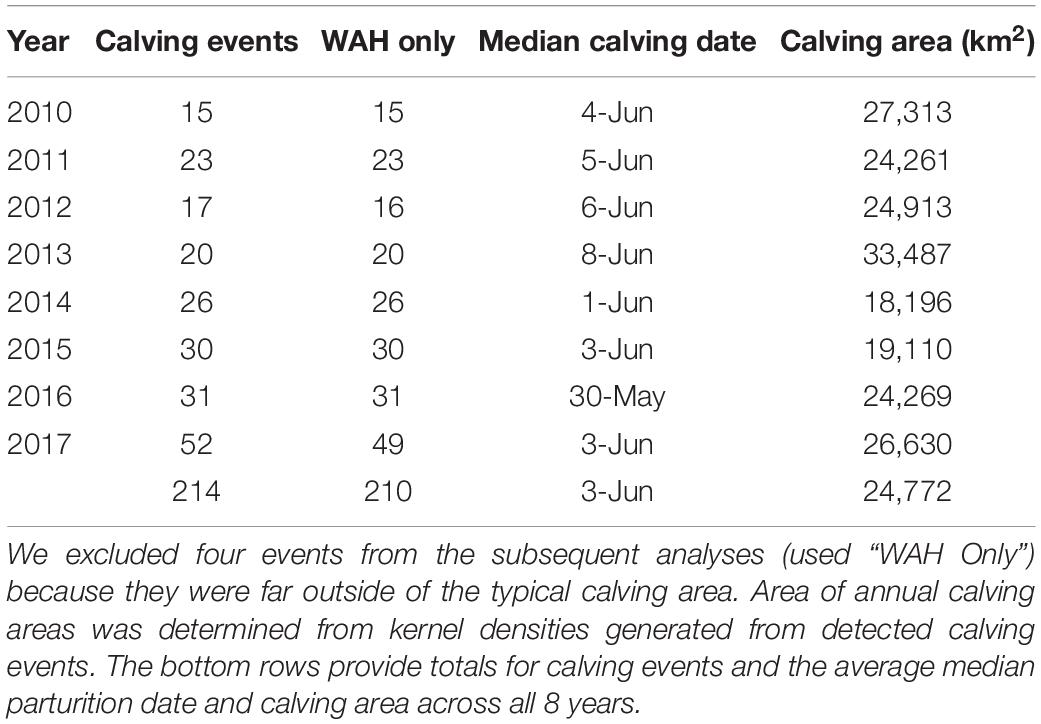
Table 1. Detected calving events based on movement data for the Western Arctic Herd (WAH), 2010–2017, Alaska.
Spatial Patterns in Calving Areas
Across the 8 years we analyzed, the WAH calving areas exhibited variation at the annual scale, but the general area was characterized by remarkable fidelity. The average extent of the calving area for the herd in a given year was 24,772 km2 (Table 1). Calving areas exhibited both latitudinal and longitudinal variation across years, with calving occurring in the Brooks Range and as far south as the Noatak River in some years (Figure 3). On an annual basis, calving areas had significantly less overlap than expected by chance three out of seven times (p < 0.05; Table 2). This trend of significant differentiation among years was evident at all further levels of comparison: at 2-year (p < 0.05 for five out of six), 3-year (p < 0.05 for four out of five), 4-year (p < 0.05 for three out of four), 5-year (p < 0.05 for one out of three), 6-year (p < 0.05 for one out of two), and 7-year intervals (p < 0.05 for the one comparison). When considered across years, the calving area of WAH females always shared a 7,281 km2 core area of overlapping extent that was used every year of the study, with calving areas of less frequent use stretching as far away as the Noatak River (Figure 4) for a total calving ground extent of 53,330 km2.
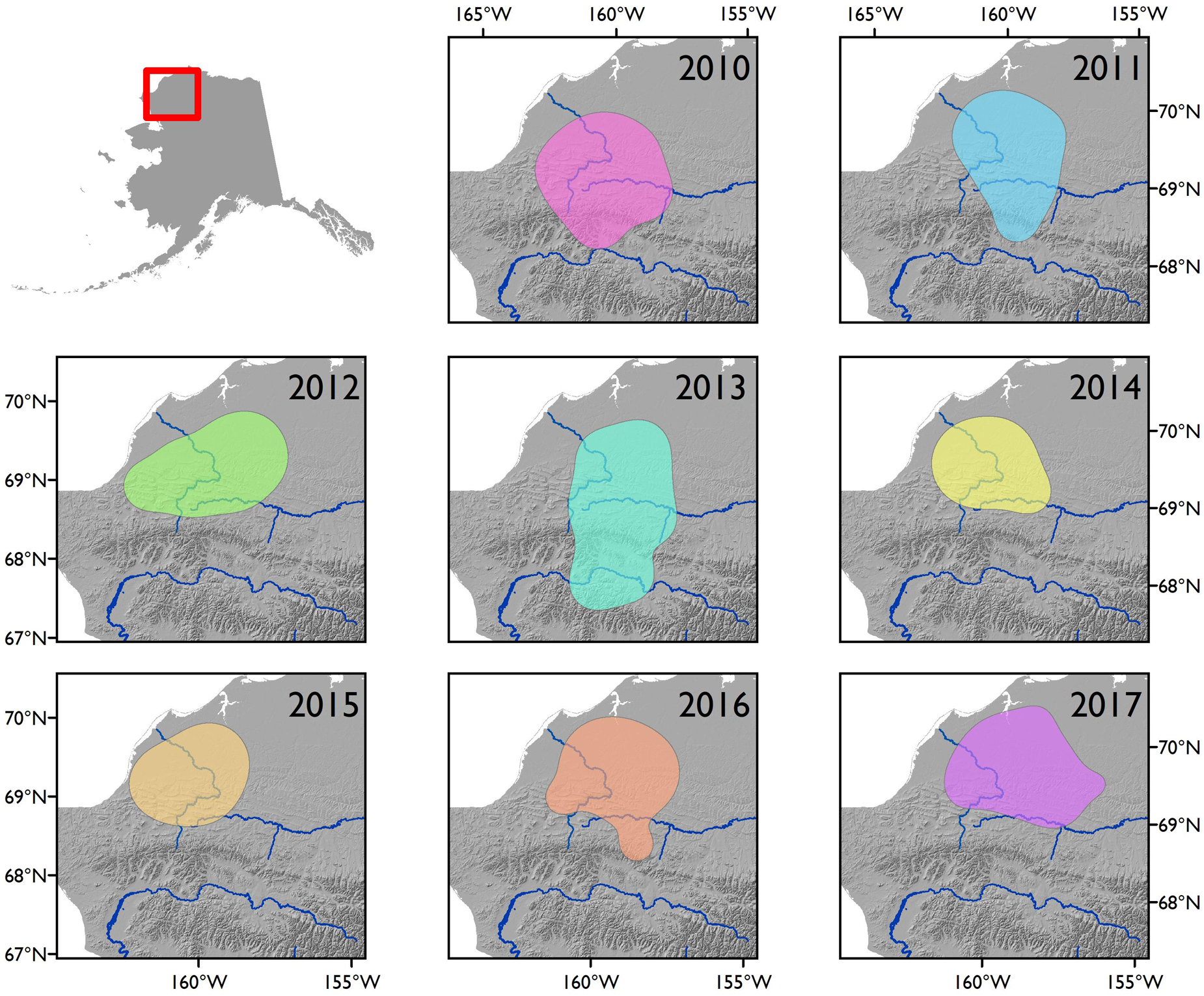
Figure 3. Annual calving areas of the Western Arctic Herd, 2010–2017, Alaska. Calving areas were delineated using the 95% contour of a kernel utilization distribution generated from parturition locations, which were inferred from GPS data.
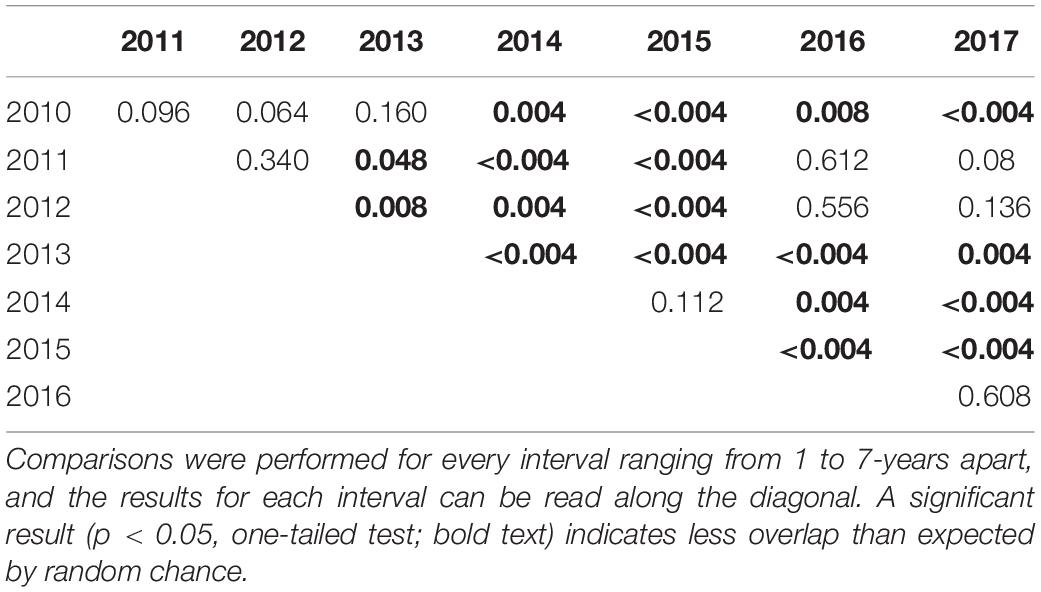
Table 2. Kernel overlap tests comparing annual calving areas of Western Arctic Herd caribou, 2010–2017, Alaska.
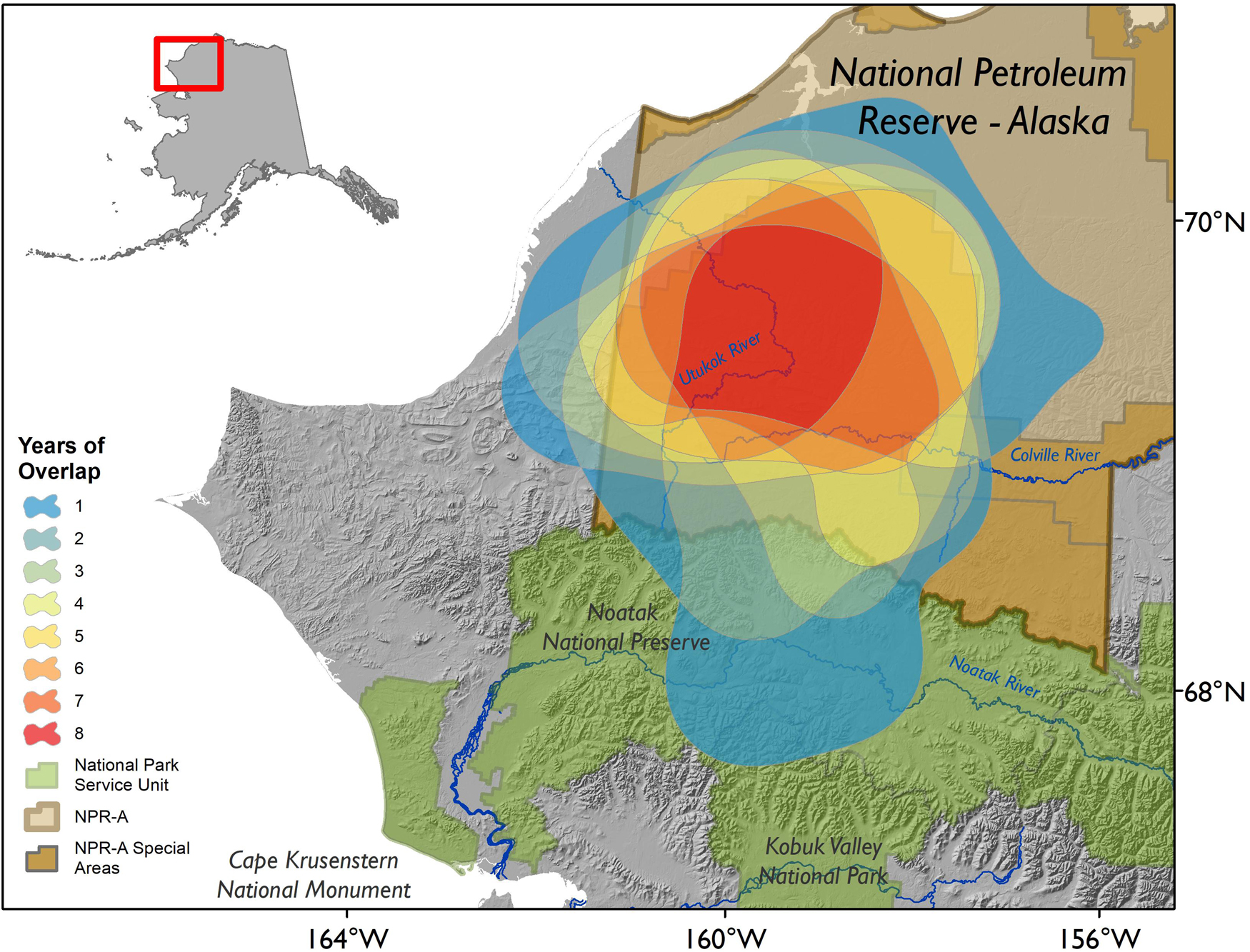
Figure 4. Overlap of all observed annual calving areas for caribou of the Western Arctic Herd, 2010–2017, Alaska. Calving areas were delineated using the 95% contour of a kernel utilization distribution generated from parturition locations, which were inferred from GPS data. Special Areas of the National Petroleum Reserve – Alaska (NPR-A; brown) include the Utukok River Uplands and Colville River Special Areas, as defined in the 2013 Integrated Activity Plan (BLM, 2012).
Range-Wide Calving Site Selection
The selection of calving sites was characterized by mostly flat tundra within a band of elevation that was greening up at the time of average calving for the herd across all years of the study. The environmental covariate that explained the most variance in calving site selection was ΔNDVI at the average peak calving date for the study (“ΔNDVI.148”), from May 21–27 to May 28–June 3 every year (day of year 141–147 to 148–154; Supplementary Table 2) and substantially outperformed the next best model in random effect selection, which included a random slope for ΔNDVI at peak calving specific to each year (ΔNDVI.Calve; Δ AICc = 29.2). In model selection for fixed effects, the top performing model included terms for land cover, quadratic terms for elevation and solar radiation that indicate selection for intermediate values for both, an interaction between elevation and ΔNDVI.148, and terrain ruggedness (Supplementary Table 3). Females strongly selected for sites with high ΔNDVI at the time of peak calving (Table 3).
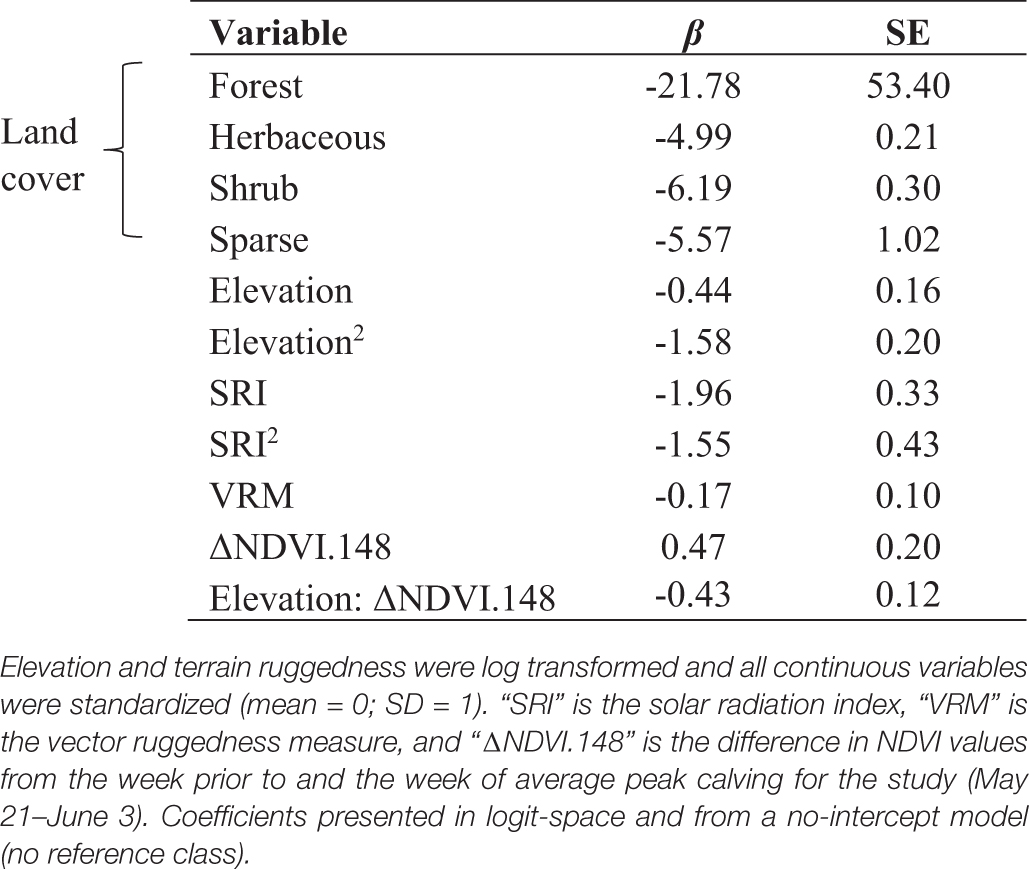
Table 3. Coefficient estimates from the top RSF model for female caribou calving site selection, Western Arctic Herd, 2010–2017, Alaska.
Calving sites were associated with a band of low elevation areas, indicating selection of elevations between approximately 50–600 m above sea level. Elevation and ΔNDVI exhibited an interactive effect, with females most strongly selecting for elevations approximately 100–175 m that were experiencing the fastest green-up at the time of peak calving. Of the four land cover classes we considered, we did not detect calving in any forested sites and found the strongest selection for herbaceous cover at calving (Table 3). The solar radiation index also exhibited a quadratic relationship for calving site selection (Table 3), indicating selection of sites with a positive index ranging from approximately 0.15 to 0.5, which correspond to lower angle slopes and encompass nearly all aspects. The negative linear coefficient for terrain ruggedness supported this result, indicating that females selected for less rugged terrain (Table 3). Our predictive map of calving habitat indicates that calving for the WAH occurs in the largest, continuous expanse of habitat characterized by these unique factors within their range, and that the attributes associated with calving sites extend to the east beyond documented calving areas (Figure 5). Importantly, calving occurred on sites with higher predicted value from the top model compared to the average of the calving area in the given year (Supplementary Figure 2).
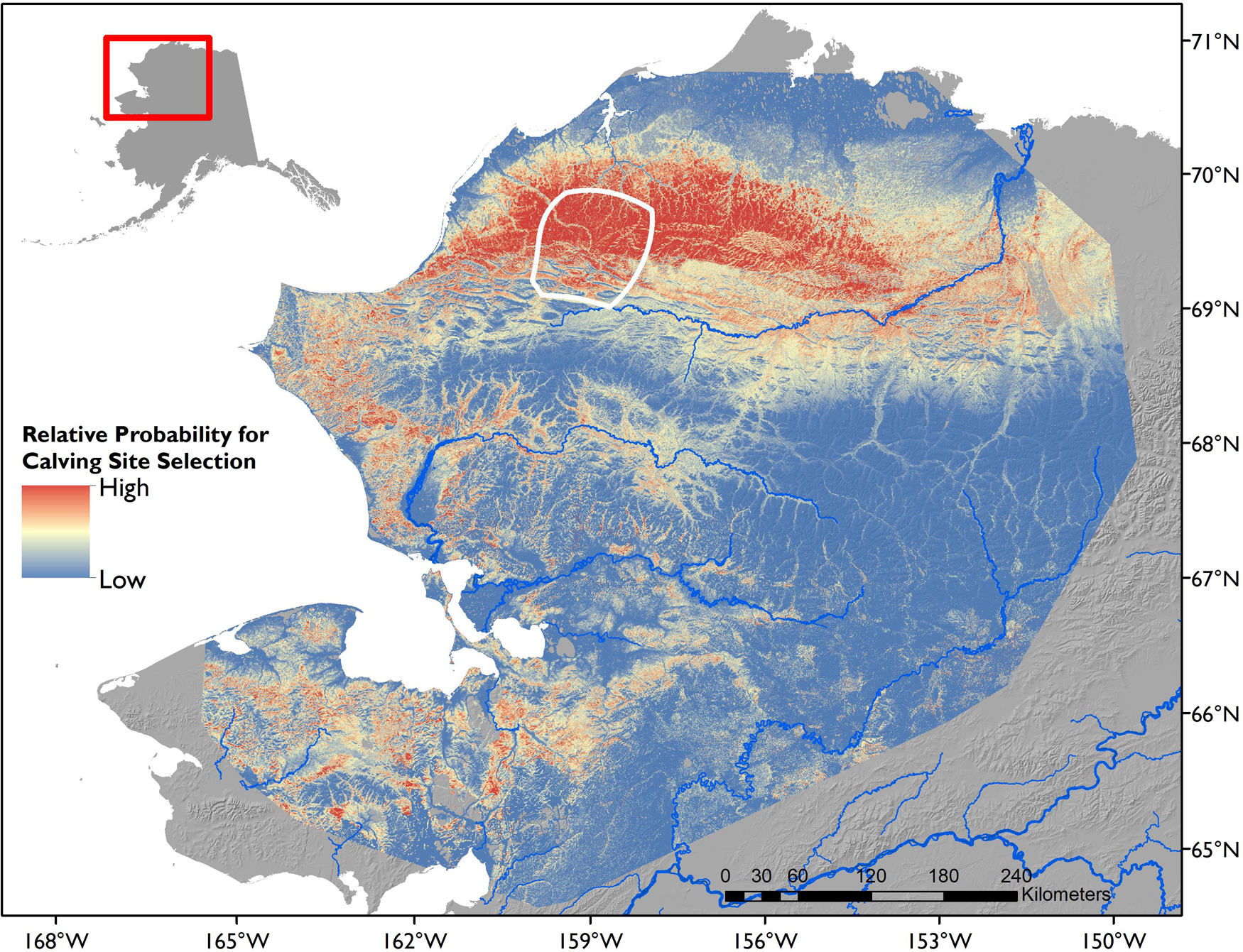
Figure 5. Predictive map of high-quality calving habitat for caribou of the Western Arctic Herd, Alaska. Map was made from the top performing resource selection function model including land cover, elevation, solar radiation, terrain ruggedness, and rate of NDVI increase from the week before to the week of average peak calving. Data for NDVI rate at peak calving were averaged across the 8 years of NDVI composites from the analysis for map generation. White polygon indicates the core area in which calving was detected for all 8 years in the study.
Discussion
Mounting evidence indicates that animals use memory to make movement decisions that improve resource acquisition in a heterogeneous landscape (Bailey et al., 1996; Fagan et al., 2013; Bracis et al., 2015; Abrahms et al., 2019; Merkle et al., 2019). For example, bison (Bison bison) base foraging on their memory of patch location and quality that result in observed home-range spatial patterns (Merkle et al., 2014). Elephants (Loxodonta africana) rely on spatial memory to minimize long-distance travel to perennial waterholes in an arid environment (Polansky et al., 2015). In an explicit test of the relative importance of memory versus perception using zebras, simulations of migration paths based on memory mechanisms reached the actual migration destination more accurately than simulations based on perception mechanisms, even when the perceptual range was increased to omniscience (Bracis and Mueller, 2017). Considering that less productive regions are associated with longer annual movements of large terrestrial mammals (Joly et al., 2019), the extreme variability of arctic conditions could conceivably promote an adaptation for memory-based capabilities in caribou.
Our results highlight the strong fidelity of a highly migratory ungulate to its calving ground within an extensive range across the nearly decade-long study period. Notably, pregnant females selected calving sites that were characterized by high-quality forage at the average time of peak calving. High fidelity is particularly impressive considering the highly variable winter ranges of individuals in this (Joly and Cameron, 2019) and other herds (Schaefer et al., 2000; Faille et al., 2010; Peignier et al., 2019), and thus females must routinely travel different routes between winter ranges and the calving area (Kelsall, 1968; Nicholson et al., 2016; Baltensperger and Joly, 2019). Spring migration routes for pregnant females are typically snow covered (Boelman et al., 2019; Gurarie et al., 2019), so these segments of the migration occur well before green-up and are unlikely to be a product of perception-based movement along the way used by other ungulates (e.g., Merkle et al., 2016). Considering the spatial consistency of use and selection for average conditions, we suggest that the fidelity of caribou to their calving grounds is supportive of memory-based movement at the landscape scale.
The use of perception-based versus memory-based movement are not necessarily mutually exclusive and may depend on the scale being considered (Bailey et al., 1996). Trial studies with sheep (Ovis spp.) revealed that individuals can remember the locations of resources between trials and use spatial memory to improve foraging efficiency. Impressively, sheep could also associate a cue with resource locations, such that when a resource patch was moved between trials, the sheep went to the original location first, then directed movement to the cue (and thus the resource; Edwards et al., 1996). Spatial consistency in calving areas for the WAH did not appear to be driven exclusively by memory of a specific place – calving sites for individuals were approximately 55 km apart across years on average (Joly et al., in preparation), which is similar to findings for other herds (Fancy and Whitten, 1991; Schaefer et al., 2000). Our finding that specific calving sites had higher forage quality than the overall average for that year’s calving area suggests that females refine calving site selection based on updated information perceived after arriving on the calving ground. In other words, our results suggest that memory guides pregnant female caribou to the general calving grounds during spring migration but then the individual’s perception of local, contemporary conditions each year refines their movement, resulting in the annual variability in calving sites and thus the characteristic annual variability of the calving areas of many herds.
Weather conditions, such as precipitation (Le Corre et al., 2017), can influence spring arrival timing, and deeper snow increases the cost of movement for caribou (Fancy and White, 1987) and is hypothesized to delay migration in other arctic caribou herds (Duquette, 1988; Gurarie et al., 2019). We suspect some of the southerly calving sites reported here were caused by such snowy spring conditions impeding migratory movement and delaying arrival to the main calving ground, which resulted in birth en route. The spring of 2013 had unusually cold temperatures and heavy late spring snowfall (Sousanes and Hill, 2013), as well as the most southerly calving sites of our study. Such snow-related delays have occurred before: some calves were born south of the Brooks Range during the unseasonably late spring of 1962 (Lent, 1966), and late snowmelt has correlated with southerly calving events in the nearby Teshekpuk Herd (Carroll et al., 2005). Based on the influence of forage quality to calving sites we detected, we attribute the observed east-west spatial variation to caribou adjusting their calving sites to annual vegetative conditions they found upon arriving to the calving ground. Variability in the annual calving area has been linked to variation in forage quality for the Porcupine Herd (Griffith et al., 2002), as well as variation in snow conditions (Fancy and Whitten, 1991). Considered cumulatively, WAH caribou utilized an area seven times larger than the core calving area across nearly a decade, likely responding to experienced annual environmental stochasticity.
Our finding of selection for an index of vegetation phenology (NDVI rate from weekly composites) supports previous studies documenting selection for ΔNDVI after calving (Kelleyhouse, 2001; Griffith et al., 2002) and aligns with recent work suggesting that raw NDVI is a poor metric of forage nutrients (Johnson et al., 2018). For many ungulates, calving and subsequent lactation are the most energetically demanding periods of the year (Clutton-Brock et al., 1989; Barboza and Parker, 2008). Female caribou exhibit a strong preference for immature floral heads of cottongrass (Eriophorum vaginatum) at calving (Kuropat and Bryant, 1980; Thompson and McCourt, 1981; Griffith et al., 2002), which offers one of the greatest sources of digestible nitrogen and protein at the beginning of the arctic growing season (Kuropat and Bryant, 1980; Johnstone et al., 2002; Cebrian et al., 2008; Gustine et al., 2017). Cottongrass is adapted to early spring growth relative to other tundra plant communities (Chapin et al., 1979), with initiation of the floral heads the autumn before allowing elongation to resume shortly after snow ablation (Wein, 1973; Cebrian et al., 2008). Considering the dominance of tussock-tundra communities (of which cottongrass is the primary component) in the foothills north of the Brooks Range (Boggs et al., 2016), we posit that the forage quality signal we identified in calving site selection by the WAH is largely influenced by cottongrass flowering, though early leaf flush of deciduous shrubs such as willow species (such as Salix pulchra) may also occur during the calving period (Borner et al., 2008). The absence of calving in the large area of predicted high-quality habitat to the east of the calving ground (Figure 5) is notable. One explanation is that following calving, the herd reliably moves to the southwest and toward the coast to avoid insect harassment, an activity that exerts large energetic costs as well as lost foraging opportunities (Witter et al., 2012; Dau, 2015; Joly and Cameron, 2019; Joly et al., 2020). Potentially, the selection of calving sites balances the nutritional need for access to high-quality resources at calving with distance to insect relief areas that will be critical in July. If so, this would suggest that selection of calving sites can also be influenced by the expectation of conditions to come after calves are born.
Another possible interpretation for our results of calving area consistency, and the most widely accepted alternative explanation for migratory ungulates to synchronously give birth in distinct calving areas, is to escape predation (Bergerud, 1974, 1996; Estes, 1976; Fancy and Whitten, 1991). The principal predators for caribou calves are wolves (Canis lupus), brown bears (Ursus arctos), and golden eagles (Aquila chrysaetos; Whitten et al., 1992). If predation risk primarily motivates selection of the calving area, then we would expect calving to occur in areas of the lowest predator densities across the range. Indeed, coarse estimates indicate that densities of wolves and brown bears are higher south of the Brooks Range compared to the north. However, in the northern portion of the herd’s range, densities for both predators are greater in the Brooks Range foothills, where WAH calving is centered, compared to the coastal plain to the north (Supplementary Table 4). Thus, the location of the core WAH calving ground is not consistent with predation risk as the primary driver of calving site selection. Our findings support the alternative hypothesis that migratory species match the increased metabolic demands of calving with favorable foraging conditions (Baker, 1938), and fit within a growing body of literature that links bottom-up signals to calving area selection by migratory ungulates. In Mongolia, calving areas for Mongolian gazelles exhibited higher NDVI values than the rest of the range at the time of use (Leimgruber et al., 2001). In Kazakhstan, Saiga antelope calving was found to be synchronized with peak productivity based on NDVI, and calving areas were characterized by low variability, and thus high reliability, of vegetative productivity (Singh et al., 2010). A preliminary study on the Tibetan Plateau suggested that Tibetan antelopes synchronize use of calving areas with peaks in primary productivity as well (Ganzorig et al., 2011). Whereas none of these studies directly tested for predator avoidance effects, there is mounting evidence from around the globe that bottom-up forces influence calving site selection for ungulates and that the motivation of selection cannot be simplified without considering scale and the potential that predation risk plays a lesser role than has been previously suggested (Fancy and Whitten, 1991; Bergerud, 1996).
Recent studies indicate that animal movement is strongly affected by social interactions when animals are in groups, termed collective movement (Westley et al., 2018). In a collective movement framework, individual group members may hold different levels of information about the environment (Couzin et al., 2005) and more informed individuals can act as group leaders in movement processes (Huse et al., 2002; Couzin et al., 2005; Guttal and Couzin, 2010; Berdahl et al., 2018). Given that caribou migrate in the spring in groups, we speculate that collective movement processes are likely at play (Duquette and Klein, 1987). This concept has a long history with local indigenous knowledge about caribou, which recommends “let the leaders pass” during migration (Padilla and Kofinas, 2014). If so, determining at what level information is held in caribou groups (such as age classes) and what proportion of informed individuals are necessary to result in the observed calving patterns, are promising avenues for future research.
Management Implications
Migratory ungulates rely on large expanses of range to maximize fitness (Hebblewhite et al., 2008; Joly et al., 2019) and migration routes of animals that rely on spatial memory are more susceptible to disturbance as they are likely more inflexible (Bracis and Mueller, 2017). Once lost, migratory patterns can take many generations for a population to learn and re-establish (Jesmer et al., 2018). Previous studies recommend that to fully conserve calving grounds for species such as caribou, managers should consider the full extent of calving at a decadal scale as the goal (Carroll et al., 2005; Taillon et al., 2012). Across 8 years of study, the WAH used an approximately 7,000 km2 core area along the Utukok River for calving and a broader area of 53,330 km2 to respond to environmental variability experienced each year on the calving ground. Comparing our findings with previous studies of the WAH up to six decades prior highlights the remarkable fidelity of this herd to its general calving ground (Supplementary Figure 3; Lent, 1966; Kelleyhouse, 2001) and local indigenous knowledge suggests this pattern extends before the 20th century (Lent, 1966; Burch, 2012). We recommend managers adopt the extent of the calving ground as the management goal for migratory caribou herds such as the WAH to ensure adequate space to respond to the annual environmental variability faced by caribou populations. We expect this recommendation has immediate utility for WAH management, for the area where the majority of calving occurs is on the National Petroleum Reserve – Alaska and specifically within the Utukok River Special Area. The Bureau of Land Management is currently revising the Integrated Activity Plan, which will designate conservation areas within the Reserve and stipulations on development in the greater area, and decisions made now have the potential to impact the WAH calving grounds for decades.
Data Availability Statement
Movement data used in this manuscript is stored in a public repository called IRMA, but access is restricted due to the sensitivity of a harvestable species’ location data. Contact authors for access. https://irma.nps.gov/Datastore/Reference/Profile/2260262.
Ethics Statement
The animal study was reviewed and approved by the State of Alaska Institutional Animal Care and Use Committee (IACUC; 0040-2017-40).
Author Contributions
MC and KJ managed the data. MC, KJ, GB, CM, and KK analyzed and interpreted the data, and contributed to manuscript revision. MC wrote the first draft of the manuscript. All authors read and approved the submitted version.
Funding
National Park Service (NPS) and Alaska Department of Fish and Game fund the on-going caribou monitoring program and NPS funded personnel time for this project.
Conflict of Interest
The authors declare that the research was conducted in the absence of any commercial or financial relationships that could be construed as a potential conflict of interest.
Acknowledgments
We thank Lincoln Parrett and Alex Hansen of the Alaska Department of Fish and Game (ADF&G), as well as their colleagues, for their continued efforts and collaboration in the long-term monitoring of the Western Arctic Herd, as well as countless insightful discussions on caribou. We also thank Regan Sarwas of the National Park Service (NPS) for his invaluable contributions to data management as well as Dave Swanson of NPS for his input and numerous discussions on remote sensing data. We are indebted to Lincoln Parrett, Luke Sanford, Julia McMahon, Eric Wald, and our reviewers for their insightful input on previous versions of this manuscript.
Supplementary Material
The Supplementary Material for this article can be found online at: https://www.frontiersin.org/articles/10.3389/fevo.2020.564567/full#supplementary-material
References
Aarts, G., MacKenzie, M., McConnell, B., Fedak, M., and Matthiopoulos, J. (2008). Estimating space-use and habitat preference from wildlife telemetry data. Ecography 31, 140–160. doi: 10.1111/j.2007.0906-7590.05236.x
Abrahms, B., Hazen, E. L., Aikens, E. O., Savoca, M. S., Goldbogen, J. A., and Bograd, S. J. (2019). Memory and resource tracking drive blue whale migrations. Proc. Natl. Acad. Sci. U.S.A. 116, 5582–5587. doi: 10.1073/pnas.1819031116
Aikens, E. O., Kauffman, M. J., Merkle, J. A., Dwinnell, S. P. H., Fralick, G. L., and Monteith, K. L. (2017). The greenscape shapes surfing of resource waves in a large migratory herbivore. Ecol. Lett. 20, 741–750. doi: 10.1111/ele.12772
Alaska Department of Fish and Game (2019). Western Arctic Caribou Herd Working Group – 2019 Meeting Summary. Available online at: https://westernarcticcaribou.net/wp-content/uploads/2020/03/2019-WACH-WG-Meeting-Summary-DRAFT-for-WACH-WG-approval-at-2020-mtg.pdf (accessed April 15, 2020).
Alerstam, T., Hedenström, A., and Åkesson, S. (2003). Long-distance migration: evolution and determinants. Oikos 103, 247–260.
Avgar, T., Deardon, R., and Fryxell, J. M. (2013). An empirically parameterized individual based model of animal movement, perception, and memory. Ecol. Modell. 251, 158–172. doi: 10.1016/j.ecolmodel.2012.12.002
Avgar, T., Street, G., and Fryxell, J. M. (2014). On the adaptive benefits of mammal migration. Can. J. Zool. 92, 481–490. doi: 10.1139/cjz-2013-0076
Bailey, D. W., Gross, J. E., Laca, E. A., Rittenhouse, L. R., Coughenour, M. B., Swift, D. M., et al. (1996). Mechanisms that result in large herbivore grazing distribution patterns. J. Range Manag. 49, 386–400. doi: 10.2307/4002919
Baker, J. R. (1938). “The evolution of breeding seasons,” in Evolution: Essays on Aspects of Evolutionary Biology Presented to Professor E. S. Goodrich, ed. G. R. de Beer (Oxford: Clarendon), 161–177.
Baltensperger, A. P., and Joly, K. (2019). Using seasonal landscape models to predict space use and migratory patterns of an arctic ungulate. Mov. Ecol. 8, 1–19.
Barboza, P. S., and Parker, K. L. (2008). Allocating protein to reproduction in arctic reindeer and caribou. Physiol. Biochem. Zool. 81, 835–855. doi: 10.1086/590414
Bates, D., Maechler, M., Bolker, B., and Walker, S. (2015). Fitting linear mixed-effects models using lme4. J. Stat. Softw. 67, 1–48. doi: 10.18637/jss.v067.i01
Bekenov, A. B., Grachevand, I. A., and Milner-Gulland, E. J. (1998). The ecology and management of the Saiga antelope in Kazakhstan. Mamm. Rev. 28, 1–52.
Berdahl, A. M., Kao, A. B., Flack, A., Westley, P. A. H., Codling, E. A., Couzin, I. D., et al. (2018). Collective animal navigation and migratory culture: from theoretical models to empirical evidence. Philos. Trans. R. Soc. B Biol. Sci. 373:20170009. doi: 10.1098/rstb.2017.0009
Bergerud, A. T. (1974). “The role of the environment in the aggregation, movement and disturbance behaviour of caribou,” in The Behaviour of Ungulates and Its Relation to Management, Vol. 2, eds V. Gesit and F. Walther (Gland: International Union for Conservation of Nature and Natural Resources), 552–584.
Bergerud, A. T. (1996). Evolving perspectives on caribou population dynamics, have we got it right yet? Rangifer 16, 95–116. doi: 10.7557/2.16.4.1225
Bischof, R., Loe, L. E., Meisingset, E. L., Zimmermann, B., Van Moorter, B., and Mysterud, A. (2012). A migratory northern ungulate in the pursuit of spring: jumping or surfing the green wave? Am. Nat. 180, 407–424. doi: 10.1086/667590
BLM (2012). National Petroleum Reserve-Alaska Integrated Activity Plan/Environmental Impact Statement. Available online at: https://eplanning.blm.gov/epl-front-office/projects/nepa/5251/41003/43153/Vol1_NPR-A_Final_IAP_FEIS.pdf (accessed April 15, 2020).
Boelman, N., Liston, G. E., Gurarie, E., Meddens, A. J. H., Mahoney, P. J., Kirchner, P. B., et al. (2019). Integrating snow science and wildlife ecology in Arctic-boreal North America. Environ. Res. Lett. 14:010401. doi: 10.1088/1748-9326/aaeec1
Boggs, K., Flagstad, L., Boucher, T., Kuo, T., Fehringer, D., Guyer, S., et al. (2016). Vegetation Map and Classification: Northern, Western, and Interior Alaska - Second Edition. Anchorage: Alaska Center for Conservation Science, University of Alaska Anchorage.
Bolker, B. M., Brooks, M. E., Clark, C. J., Geange, S. W., Poulsen, J. R., Stevens, M. H. H., et al. (2009). Generalized linear mixed models: a practical guide for ecology and evolution. Trends Ecol. Evol. 24, 127–135. doi: 10.1016/j.tree.2008.10.008
Boone, R. B., Thirgood, S. J., and Hopcraft, J. G. C. (2006). Serengeti wildebeest migratory patterns modeled from rainfall and new vegetation growth. Ecology 87, 1987–1994.
Borner, A. P., Kielland, K., and Walker, M. D. (2008). Effects of simulated climate change on plant phenology and nitrogen mineralization in Alaskan arctic tundra. Arctic Antarct. Alp. Res. 40, 27–38. doi: 10.1657/1523-0430(06-099)
Bracis, C., Gurarie, E., Van Moorter, B., and Goodwin, R. A. (2015). Memory effects on movement behavior in animal foraging. PLoS One 10:e0136057. doi: 10.1371/journal.pone.0136057
Bracis, C., and Mueller, T. (2017). Memory, not just perception, plays an important role in terrestrial mammalian migration. Proc. R. Soc. B Biol. Sci. 284:20170449. doi: 10.1098/rspb.2017.0449
Breed, G. A., Bowen, W., McMillan, J., and Leonard, M. (2006). Sexual segregation of seasonal foraging habitats in a non-migratory marine mammal. Proc. R. Soc. B Biol. Sci. 273, 2319–2326. doi: 10.1098/rspb.2006.3581
Burch, E. S. (2012). Caribou Herds of Nothwest Alaska, 1850-2000. Fairbanks, AK: University of Alaska Press.
Burnham, K. P., and Anderson, D. R. (2002). Model Selection and Multimodel Inference: A Practical Information-Theoretic Approach. New York: Springer.
Calenge, C. (2006). The package adehabitat for the R software: a tool for the analysis of space and habitat use by animals. Ecol. Modell. 197, 516–519.
Cameron, M. D., Joly, K., Breed, G. A., Parrett, L. S., and Kielland, K. (2018). Movement-based methods to infer parturition events in migratory ungulates. Can. J. Zool. 96, 1187–1195. doi: 10.1139/cjz-2017-0314
Carroll, G. M., Parrett, L. S., George, J. C., and Yokel, D. A. (2005). Calving distribution of the Teshekpuk caribou herd, 1994-2003. Rangifer 16, 27–35. doi: 10.7557/2.25.4.1767
Cebrian, M. R., Kielland, K., and Finstad, G. (2008). Forage quality and reindeer productivity: multiplier effects amplified by climate change. Arctic Antarct. Alp. Res. 40, 48–54. doi: 10.1657/1523-0430(07-069)
Chan-McLeod, A. C. A., White, R. G., and Holleman, D. F. (1994). Effects of protein and energy intake, body condition, and season on nutrient partitioning and milk production in caribou and reindeer. Can. J. Zool. 72, 938–947. doi: 10.1139/z94-127
Chapin, F. S. III, van Cleve, K., and Chapin, M. C. (1979). Soil temperature and nutrient cycling in the tussock growth form of Eriophorum vaginatum. J. Ecol. 67, 169–189. doi: 10.2307/2259343
Charnov, E. L. (1976). Optimal foraging theory: the marginal value theorem. Theor. Popul. Biol. 9, 129–136.
Clutton-Brock, T. H., Albon, S. D., and Guiness, F. E. (1989). Fitness costs of gestation and lactation in wild mammals. Nature 337, 260–262. doi: 10.1038/337260a0
Couzin, I. D., Krause, J., Franks, N. R., and Levin, S. A. (2005). Effective leadership and decision-making in animal groups on the move. Nature 433, 513–516. doi: 10.1038/nature03236
Creel, S., Winnie, J. Jr., Maxwell, B., Hamlin, K., and Creel, M. (2005). Elk alter habitat selection as an antipredator response to wolves. Ecology 86, 3387–3397.
Crête, M., and Huot, J. (1993). Regulation of a large herd of migratory caribou: summer nutrition affects calf growth and body reserves of dams. Can. J. Zool. 71, 2291–2296. doi: 10.1139/z93-321
Dau, J. R. (1997). “Units 21D, 22A, 22B, 23, 24, 26A caribou management report,” in Caribou Management Report of Survey-Inventory Activities 1 July 1994-1930 June 1996, ed. M. V. Hicks (Alaska: Alaska Department Fish and Game, Division of Wildlife Conservation).
Dau, J. R. (2015). “Units 21D, 22A, 22B, 22C, 22D, 22E, 23, 24 and 26A. Chapter 14, pages 14-1 through 14-89,” in Caribou Management Report of Survey And Inventory Activities 1 July 2012-2030 June 2014, eds P. Harper and L. A. McCarthy (Alaska: Alaska Department of Fish and Game).
DeMars, C. A., Auger-Méthé, M., Schlägel, U. E., and Boutin, S. (2013). Inferring parturition and neonate survival from movement patterns of female ungulates: a case study using woodland caribou. Ecol. Evol. 3, 4149–4160. doi: 10.1002/ece3.785
Duquette, L. S. (1988). Snow characteristics along caribou trails and within feeding areas during spring migration. Arctic 41, 143–144.
Duquette, L. S., and Klein, D. R. (1987). Activity budgets and group size of caribou during spring migration. Can. J. Zool. 65, 164–168. doi: 10.1139/z87-023
Edwards, G. R., Newman, J. A., Parsons, A. J., and Krebs, J. R. (1996). Use of cues by grazing animals to locate food patches: an example with sheep. Appl. Anim. Behav. Sci. 50, 147–160. doi: 10.1016/S0168-1591(96)01095-7
Estes, R. D. (1976). The significance of breeding synchrony in the wildebeest. East Afr. Wildl. J. 14, 135–152.
Fagan, W. F., Lewis, M. A., Auger-Méthé, M., Avgar, T., Benhamou, S., Breed, G. A., et al. (2013). Spatial memory and animal movement. Ecol. Lett. 16, 1316–1329. doi: 10.1111/ele.12165
Faille, G., Dussault, C., Ouellet, J. P., Fortin, D., Courtois, R., St-Laurent, M. H., et al. (2010). Range fidelity: the missing link between caribou decline and habitat alteration? Biol. Conserv. 143, 2840–2850. doi: 10.1016/j.biocon.2010.08.001
Fancy, S. G., and White, R. G. (1987). Energy expenditures for locomotion by barren-ground caribou. Can. J. Zool. 65, 122–128. doi: 10.1139/z87-018
Fancy, S. G., and Whitten, K. R. (1991). Selection of calving sites by Porcupine herd caribou. Can. J. Zool. 69, 1736–1743. doi: 10.1139/z91-242
Finstad, G. L. (2008). Applied Range Ecology of Reindeer (Rangifer tarandus tarandus) on the Seward Peninsula, Alaska. Available online at: https://search.proquest.com/docview/304684271?accountid=43793 (accessed April 15, 2020).
Gaillard, J. M., Festa-Bianchet, M., Yoccoz, N. G., Loison, A., and Toïgo, C. (2000). Temporal variation in fitness components and population dynamics of large herbivores. Annu. Rev. Ecol. Syst. 31, 367–393. doi: 10.1146/annurev.ecolsys.31.1.367
Ganzorig, S., Hoshino, B., Manaeva, K., and Igota, H. (2011). Seasonal migration of Tibetan antelope (Pantholops hodgsonii) and its relation with spatial patterns of relative primary productivity (NDVI). Proceeding 32nd Asian Conf. Remote Sens. 3, 1493–1497.
Gillies, C. S., Hebblewhite, M., Nielsen, S. E., Krawchuk, M. A., Aldridge, C. L., Frair, J. L., et al. (2006). Application of random effects to the study of resource selection by animals. J. Anim. Ecol. 75, 887–898. doi: 10.1111/j.1365-2656.2006.01106.x
Griffith, B., Douglas, D. C., Walsh, N. E., Young, D. D., McCabe, T. R., Russell, D. E., et al. (2002). “The porcupine caribou herd,” in Arctic Refuge Coastal Plain Terrestrial Wildlife Research Summaries, eds D. C. Douglas, P. E. Reynolds, and E. B. Rhode (Reston: U. S. Geological Survey), 8–37.
Gunn, A. (2003). Voles lemmings and caribou - population cycles revisited? Rangifer 23, 105–111. doi: 10.7557/2.23.5.1689
Gunn, A., and Miller, F. L. (1986). Traditional behaviour and fidelity to caribou calving grounds by barren-ground caribou. Rangifer 6, 151–158. doi: 10.7557/2.6.2.640
Gurarie, E., Hebblewhiteb, M., Joly, K., Kelly, A. P., Adamczewski, J., Davidson, S. C., et al. (2019). Tactical departures and strategic arrivals: divergent effects of climate and weather on caribou spring migrations. Ecosphere 10:e02971. doi: 10.1002/ecs2.2971
Gustine, D. D., Barboza, P. S., Adams, L., Griffith, B., Cameron, R., and Whitten, K. (2017). Advancing the match-mismatch framework for large herbivores in the Arctic: evaluating the evidence for a trophic mismatch in caribou. PLoS One 12:e0171807. doi: 10.1371/journal.pone.0171807
Guttal, V., and Couzin, I. D. (2010). Social interactions, information use, and the evolution of collective migration. Proc. Natl. Acad. Sci. U.S.A. 107, 16172–16177. doi: 10.1073/pnas.1006874107
Harju, S. M., Dzialak, M. R., Osborn, R. G., Hayden-Wing, L. D., and Winstead, J. B. (2011). Conservation planning using resource selection models: altered selection in the presence of human activity changes spatial prediction of resource use. Anim. Conserv. 14, 502–511. doi: 10.1111/j.1469-1795.2011.00456.x
Hebblewhite, M., Merrill, E. H., and McDermid, G. J. (2008). A multi-scale test of the forage maturation hypothesis in a partially migrating ungulate population. Ecol. Monogr. 78, 141–166. doi: 10.1890/06-1708.1
Hooten, M. B., Johnson, D. S., Mcclintock, B. T., and Morales, J. M. (2017). Animal Movement: Statistical Models for Telemetry Data. Boca Raton, FL: CRC Press, Inc.
Howery, L. D., Bailey, D. W., and Laca, E. A. (1999). Impact of spatial memory on habitat use. Grazing Behav. Livest. Wildl. 3, 91–100.
Hurvich, C. M., and Tsai, C.-L. (1989). Regression and time series model selection in small samples. Biometrika 76, 297–307.
Huse, G., Railsback, S., and Fernö, A. (2002). Modelling changes in migration pattern of herring: collective behaviour and numerical domination. J. Fish Biol. 60, 571–582. doi: 10.1006/jfbi.2002.1874
Jenkerson, C., Maiersperger, T., and Schmidt, G. (2010). eMODIS: A User-Friendly Data Source. Reston: U. S. Geological Survey Open-File Report.
Jesmer, B. R., Merkle, J. A., Goheen, J. R., Aikens, E. O., Beck, J. L., Courtemanch, A. B., et al. (2018). Is ungulate migration culturally transmitted? Evidence of social learning from translocated animals. Science 361, 1023–1025. doi: 10.1126/science.aat0985
Johnson, D. H. (1980). The comparison of usage and availability measurements for evaluating resource preference. Ecology 61, 65–71. doi: 10.2307/1937156
Johnson, H. E., Gustine, D. D., Golden, T. S., Adams, L. G., Parrett, L. S., Lenart, E. A., et al. (2018). NDVI exhibits mixed success in predicting spatiotemporal variation in caribou summer forage quality and quantity. Ecosphere 9:e02461. doi: 10.1002/ecs2.2461
Johnstone, J., Russell, D. E., and Griffith, B. (2002). Variations in plant forage quality in the range of the Porcupine caribou herd. Rangifer 22, 83–91. doi: 10.7557/2.22.1.693
Joly, K., and Cameron, M. D. (2019). Caribou vital sign annual report for the Arctic Network Inventory and Monitoring Program: September 2018-August 2019. Fort Collins, CO: Collins.
Joly, K., Couriot, O., Cameron, M. D., and Gurarie, E. (2020). Behavioral, physiological, demographic and ecological impacts of hematophagous and endoparasitic insects on an arctic ungulate. Toxins 12:334. doi: 10.3390/toxins12050334
Joly, K., Gurarie, E., Sorum, M. S., Kaczensky, P., Cameron, M. D., Jakes, A. F., et al. (2019). Longest terrestrial migrations and movements around the world. Sci. Rep. 9:15333. doi: 10.1038/s41598-019-51884-5
Joly, K., Klein, D. R., Verbyla, D. L., Rupp, T. S., and Chapin, F. S. (2011). Linkages between large-scale climate patterns and the dynamics of Arctic caribou populations. Ecography 34, 345–352. doi: 10.1111/j.1600-0587.2010.06377.x
Joly, K., Miller, S. D., and Shults, B. S. (2012). Caribou Monitoring Protocol for the Arctic Network Inventory and Monitoring Program. Natural Resource Report NPS/ARCN/NRR—2012/564. Fort Collins, CO: National Park Service.
Joly, K., Nellemann, C., and Vistnes, I. (2004). A reevaluation of caribou distribution near an oilfield road on Alaska’s North Slope. Wildl. Soc. Bull. 34, 866–869.
Keating, K. A., and Cherry, S. (2004). Use and interpretation of logistic regression in habitat-selection studies. J. Wildl. Manage. 68, 774–789.
Keating, K. A., Gogan, P. J. P., Vore, J. M., and Irby, L. R. (2007). A simple solar radiation index for wildlife habitat studies. J. Wildl. Manage. 71, 1344–1348. doi: 10.2193/2006-359
Kelleyhouse, R. A. (2001). Calving Ground Selection and Fidelity: Teshekpuk Lake and Western Arctic Caribou Herds. Fairbanks, AK: University of Alaska Fairbanks.
Kelsall, J. P. (1968). The Migratory Barren-Ground Caribou of North America. Ottawa: Queen’s Printer.
Kuropat, P., and Bryant, J. P. (1980). “Foraging behavior of cow caribou on the Utukok calving grounds in Northwestern Alaska,” in Proceedings of the Second International Reindeer/Caribou Symposium 17-21 September 1979, Roros, Norway, eds E. Reimers, E. Gaare, and S. Skjenneberg (Røros: Direktoratet for Vilt og Ferskvannsfisk), 64–70.
Le Corre, M., Dussault, C., and Côté, S. D. (2017). Weather conditions and variation in timing of spring and fall migrations of migratory caribou. J. Mammal. 98, 260–271. doi: 10.1093/jmammal/gyw177
Leimgruber, P., McShea, W. J., Brookes, C. J., Bolor-Erdene, L., Wemmer, C., and Larson, C. (2001). Spatial patterns in relative primary productivity and gazelle migration in the Eastern Steppes of Mongolia. Biol. Conserv. 102, 205–212. doi: 10.1016/S0006-3207(01)00041-6
Lent, P. C. (1966). Calving and related social behavior in the barren-ground caribou. Z. Tierpsychol. 23, 701–756. doi: 10.1111/j.1439-0310.1966.tb01707.x
Macander, M. J., Swingley, C. S., Joly, K., and Raynolds, M. K. (2015). Landsat-based snow persistence map for northwest Alaska. Remote Sens. Environ. 163, 23–31. doi: 10.1016/j.rse.2015.02.028
MacArthur, R., and Pianka, E. R. (1966). On optimal use of a patchy environment. Am. Nat. 100:603. doi: 10.1086/282454
Mager, K. H., Colson, K. E., and Hundertmark, K. J. (2013). High genetic connectivity and introgression from domestic reindeer characterize northern Alaska caribou herds. Conserv. Genet. 14, 1111–1123. doi: 10.1007/s10592-013-0499-2
Manly, B. F. J., McDonald, L. L., Thomas, D. L., Mcdonald, T. L., and Erickson, W. P. (2002). Resource Selection by Animals: Statistical Design and Analysis for Field Studies, Second Edn. Dordrecht: Springer.
Merkle, J. A., Fortin, D., and Morales, J. M. (2014). A memory-based foraging tactic reveals an adaptive mechanism for restricted space use. Ecol. Lett. 17, 924–931. doi: 10.1111/ele.12294
Merkle, J. A., Monteith, K. L., Aikens, E. O., Hayes, M. M., Hersey, K. R., Middleton, A. D., et al. (2016). Large herbivores surf waves of green-up in spring. Proc. R. Soc. B Biol. Sci. 283:20160456. doi: 10.1098/rspb.2016.0456
Merkle, J. A., Sawyer, H., Monteith, K. L., Dwinnell, S. P. H., Fralick, G. L., and Kauffman, M. J. (2019). Spatial memory shapes migration and its benefits: evidence from a large herbivore. Ecol. Lett. 22, 1797–1805. doi: 10.1111/ele.13362
Miller-Rushing, A. J., Høye, T. T., Inouye, D. W., and Post, E. (2010). The effects of phenological mismatches on demography. Philos. Trans. R. Soc. B Biol. Sci. 365, 3177–3186. doi: 10.1098/rstb.2010.0148
Nellemann, C., and Cameron, R. D. (1998). Cumulative impacts of an evolving oil-field complex on the distribution of calving caribou. Can. J. Zool. 76, 1425–1430. doi: 10.1139/z98-078
Nicholson, K. L., Arthur, S. M., Horne, J. S., Garton, E. O., and Del Vecchio, P. A. (2016). Modeling caribou movements: seasonal ranges and migration routes of the Central Arctic Herd. PLoS One 11:e0150333. doi: 10.1371/journal.pone.0150333
Oftedal, O. T. (1985). “Pregnancy and lactation,” in Bioenergetics of Wild Herbivores, eds R. J. Hudson and R. G. White (Boca Raton, FL: CRC Press, Inc), 215–238.
Padilla, E., and Kofinas, G. P. (2014). “Letting the leaders pass”: barriers to using traditional ecological knowledge in comanagement as the basis of formal hunting regulations. Ecol. Soc. 19:7. doi: 10.5751/ES-05999-190207
Peignier, M., Webber, Q. M. R., Koen, E. L., Laforge, M. P., Robitaille, A. L., and Vander Wal, E. (2019). Space use and social association in a gregarious ungulate: testing the conspecific attraction and resource dispersion hypotheses. Ecol. Evol. 9, 5133–5145. doi: 10.1002/ece3.5071
Pettorelli, N., Gaillard, J., Mysterud, A., Duncan, P., Stenseth, N. C., Delorme, D., et al. (2006). Using a proxy of plant productivity (NDVI) to find key periods for animal performance: the case of roe deer. Oikos 112, 565–572.
Pettorelli, N., Vik, J. O., Mysterud, A., Gaillard, J. M., Tucker, C. J., and Stenseth, N. C. (2005a). Using the satellite-derived NDVI to assess ecological responses to environmental change. Trends Ecol. Evol. 20, 503–510. doi: 10.1016/j.tree.2005.05.011
Pettorelli, N., Weladji, R. B., Holand, Ø, Mysterud, A., Breie, H., and Stenseth, N. C. (2005b). The relative role of winter and spring conditions: linking climate and landscape-scale plant phenology to alpine reindeer body mass. Biol. Lett. 1, 24–26. doi: 10.1098/rsbl.2004.0262
Polansky, L., Kilian, W., and Wittemyer, G. (2015). Elucidating the significance of spatial memory on movement decisions by African savannah elephants using state-space models. Proc. R. Soc. B Biol. Sci. 282:20143042. doi: 10.1098/rspb.2014.3042
Prichard, A. K., Parrett, L. S., Lenart, E. A., Caikoski, J. R., Joly, K., and Person, B. T. (2020). Interchange and overlap among four adjacent arctic caribou herds. J. Wildl. Manage. 84, 1500–1514. doi: 10.1002/jwmg.21934
R Core Team (2017). R: A Language and Environment for Statistical Computing. Vienna: R Foundation for Statistical Computing.
Sappington, J. M., Longshore, K. M., and Thompson, D. B. (2007). Quantifying landscape ruggedness for animal habitat analysis: a case study using bighorn sheep in the Mojave Desert. J. Wildl. Manage. 71, 1419–1426. doi: 10.2193/2005-723
Schaefer, J. A., Bergman, C. M., and Luttich, S. N. (2000). Site fidelity of female caribou at multiple spatial scales. Landsc. Ecol. 15, 731–739. doi: 10.1023/A:1008160408257
Schaller, G. B., Kang, A., Xinbin, C. A. I., and Schaller, G. B. (2006). Migratory and calving behavior of Tibetan antelope population. Acta Theriol. Sin. 26, 105–113.
Shaw, A. K., and Couzin, I. D. (2013). Migration or residency? The evolution of movement behavior and information usage in seasonal environments. Am. Nat. 181, 114–124. doi: 10.1086/668600
Singh, N. J., Grachev, I. A., Bekenov, A. B., and Milner-Gulland, E. J. (2010). Saiga antelope calving site selection is increasingly driven by human disturbance. Biol. Conserv. 143, 1770–1779. doi: 10.1016/j.biocon.2010.04.026
Singh, N. J., and Milner-Gulland, E. J. (2011). Conserving a moving target: planning protection for a migratory species as its distribution changes. J. Appl. Ecol. 48, 35–46. doi: 10.1111/j.1365-2664.2010.01905.x
Skoog, R. O. (1968). Ecology of the Caribou (Rangifer Tarandus Granti) in Alaska. Fairbanks, AK: University of Alaska Fairbanks.
Snyder, J. P. (1987). Map Projections: A Working Manual, Vol. 1395. Washington, D.C: US Government Printing Office.
Sousanes, P., and Hill, K. (2013). Western Arctic Parklands Spring 2013 Weather Summary. Available online at: https://irma.nps.gov/DataStore/DownloadFile/473401 (accessed April 15, 2020).
Taillon, J., Festa-Bianchet, M., and Côté, S. D. (2012). Shifting targets in the tundra: protection of migratory caribou calving grounds must account for spatial changes over time. Biol. Conserv. 147, 163–173. doi: 10.1016/j.biocon.2011.12.027
Thompson, D. C., and McCourt, K. H. (1981). Seasonal diets of the porcupine caribou herd. Am. Midl. Nat. 105, 70–76.
U.S Geological Survey (2017). 5 Meter Alaska Digital Elevation Models (DEMs) - USGS National Map 3DEP Downloadable Data Collection. Reston: U.S. Geological Survey.
van der Graaf, S., Stahl, J., Klimkowska, A., Bakker, J. P., and Drent, R. H. (2006). Surfing on a green wave – how plant growth drives spring migration in the barnacle goose Branta leucopsis. Ardea 94, 567–577.
Wein, R. W. (1973). Eriophorum vaginatum L. J. Ecol. 61, 601–615. doi: 10.1016/j.colsurfb.2011.03.013
Westley, P. A. H., Berdahl, A. M., Torney, C. J., and Biro, D. (2018). Collective movement in ecology: from emerging technologies to conservation and management. Philos. Trans. R. Soc. B Biol. Sci. 373:20170004. doi: 10.1098/rstb.2017.0004
White, R. G. (1992). “Nutritional in relation to season, lactation, and growth of north temperate deer,” in The Biology of Deer, ed. R. D. Brown (New York, NY: Springer), 407–417.
Whitten, K. R., Garner, G. W., Mauer, F. J., and Harris, R. B. (1992). Productivity and early calf survival in the Porcupine caribou herd. J. Wildl. Manage. 56, 201–212. doi: 10.2307/3808814
Wilson, R. R., Prichard, A. K., Parrett, L. S., Person, B. T., Carroll, G. M., Smith, M. A., et al. (2012). Summer resource selection and identification of important habitat prior to industrial development for the Teshekpuk Caribou Herd in Northern Alaska. PLoS One 7:e48697. doi: 10.1371/journal.pone.0048697
Witter, L. A., Johnson, C. J., Croft, B., Gunn, A., and Gillingham, M. P. (2012). Behavioural trade-offs in response to external stimuli: time allocation of an Arctic ungulate during varying intensities of harassment by parasitic flies. J. Anim. Ecol. 81, 284–295. doi: 10.1111/j.1365-2656.2011.01905.x
Keywords: Alaska, calving grounds, caribou, migration, NDVI, Rangifer tarandus
Citation: Cameron MD, Joly K, Breed GA, Mulder CPH and Kielland K (2020) Pronounced Fidelity and Selection for Average Conditions of Calving Area Suggestive of Spatial Memory in a Highly Migratory Ungulate. Front. Ecol. Evol. 8:564567. doi: 10.3389/fevo.2020.564567
Received: 12 June 2020; Accepted: 30 October 2020;
Published: 23 November 2020.
Edited by:
Vernon Bleich, University of Nevada, Reno, United StatesReviewed by:
Inger Suzanne Prange, Appalachian Wildlife Research Institute, United StatesCarl Soulsbury, University of Lincoln, United Kingdom
Copyright © 2020 Cameron, Joly, Breed, Mulder and Kielland. This is an open-access article distributed under the terms of the Creative Commons Attribution License (CC BY). The use, distribution or reproduction in other forums is permitted, provided the original author(s) and the copyright owner(s) are credited and that the original publication in this journal is cited, in accordance with accepted academic practice. No use, distribution or reproduction is permitted which does not comply with these terms.
*Correspondence: Matthew D. Cameron, bWF0dGhld19jYW1lcm9uQG5wcy5nb3Y=