- 1Soil, Water and Land Use Team, Wageningen Environmental Research, Wageningen University and Research, Wageningen, Netherlands
- 2Sustainability Research Institute, School of Earth and Environment, University of Leeds, Leeds, United Kingdom
- 3Department of Environment and Geography, University of York, York, United Kingdom
- 4ISEM, CNRS, Univ. Montpellier, IRD, EPHE, Montpellier, France
- 5Institute for Social-Ecological Research, Biodiversity and People, Frankfurt am Main, Germany
- 6Senckenberg Biodiversity and Climate Research Center BiK-F, Ecosystem Services and Climate, Frankfurt am Main, Germany
- 7Department of Ecology and Multidisciplinary Institute for Environmental Studies, University of Alicante, Alicante, Spain
- 8Copernicus Institute of Sustainable Development, Environmental Science Group, Utrecht University, Utrecht, Netherlands
- 9Fundación de la Comunidad Valenciana Centro de Estudios Ambientales del Mediterráneo, Valencia, Spain
- 10Unidad Mixta de Investigación Universidad de Alicante − Fundación CEAM, Alicante, Spain
- 11Department of Evolutionary Biology, Ecology and Environmental Sciences, University of Barcelona, Barcelona, Spain
- 12Fondazione MEDES, Sicignano degli Alburni, Italy
- 13Soil Physics and Land Management Group, Wageningen University and Research, Wageningen, Netherlands
- 14Biometris, Department of Mathematical and Statistical Methods, Wageningen University, Wageningen, Netherlands
- 15Joint Research Centre, European Commission, Ispra, Italy
- 16Centre for Environmental and Marine Studies, Department of Environment and Planning, University of Aveiro, Aveiro, Portugal
- 17Centre for Development and Environment, University of Bern, Bern, Switzerland
- 18Soil Section, Federal Office for the Environment, Bern, Switzerland
- 19Bern School of Applied Sciences, Bern University of Applied Sciences, Bern, Switzerland
- 20Earth System Analysis, Potsdam Institute for Climate Impact Research, Member of the Leibniz Association, Potsdam, Germany
- 21Department of Civil Engineering and Geomatics, Remote Sensing and Geo-Environment Lab, Faculty of Engineering and Technology, Cyprus University of Technology, Limassol, Cyprus
- 22ERATOSTHENES Centre of Excellence, Limassol, Cyprus
- 23Department of Mathematics, Computer Science and Economics, University of Basilicata, Basilicata, Italy
- 24School of Environmental Engineering, Technical University of Crete, Chania, Greece
- 25Department of Agriculture, Hellenic Mediterranean University, Heraklion, Greece
- 26Corepage, Utrecht, Netherlands
- 27Institute for Biodiversity and Ecosystem Dynamics, University of Amsterdam, Amsterdam, Netherlands
One of the most challenging issues in Mediterranean ecosystems to date has been to understand the emergence of discontinuous changes or catastrophic shifts. In the era of the 2030 Sustainable Development Goals, which encompass ideas around Land Degradation Neutrality, advancing this understanding has become even more critical and urgent. The aim of this paper is to synthesize insights into the drivers, processes and management of catastrophic shifts to highlight ways forward for the management of Mediterranean ecosystems. We use a multidisciplinary approach that extends beyond the typical single site, single scale, single approach studies in the current literature. We link applied and theoretical ecology at multiple scales with analyses and modeling of human–environment–climate relations and stakeholder engagement in six field sites in Mediterranean ecosystems to address three key questions:
i) How do major degradation drivers affect ecosystem functioning and services in Mediterranean ecosystems?
ii) What processes happen in the soil and vegetation during a catastrophic shift?
iii) How can management of vulnerable ecosystems be optimized using these findings?
Drawing together the findings from the use of different approaches allows us to address the whole pipeline of changes from drivers through to action. We highlight ways to assess ecosystem vulnerability that can help to prevent ecosystem shifts to undesirable states; identify cost-effective management measures that align with the vision and plans of land users; and evaluate the timing of these measures to enable optimization of their application before thresholds are reached. Such a multidisciplinary approach enables improved identification of early warning signals for discontinuous changes informing more timely and cost-effective management, allowing anticipation of, adaptation to, or even prevention of, undesirable catastrophic ecosystem shifts.
Introduction
Human impacts on the environment have become more acute, and the need to address them more urgent, with catastrophic shifts in ecosystem structure and function becoming a major research focus in recent decades (Scheffer et al., 2001; Scheffer and Carpenter, 2003; Andersen et al., 2009). Critical thresholds, at which ecosystems shift from a desired to an undesired, degraded state (with e.g., bare ground or unwanted plant composition), imply major losses of biological diversity, ecosystem functioning and resilience, as well as potential to undermine livelihoods, greatly reducing the benefits humans obtain from ecosystems (Maestre et al., 2016). In some cases, catastrophic shifts can trigger land abandonment, particularly in sensitive environments such as drylands (Bestelmeyer et al., 2015). However, such shifts are difficult to predict (Guttal and Jayaprakash, 2009; Berdugo et al., 2020). Even if a system has remained stable for a long period, a shift can occur suddenly, with very limited warning (Brock and Carpenter, 2012).
While catastrophic shifts have been observed and analyzed for a wide variety of ecological systems, including lakes (Scheffer et al., 2001), peatlands (Swindles et al., 2018), marine systems (Lees et al., 2006), mussel beds (Génin et al., 2018a) forests (Magnuszewski et al., 2015), and rangelands (Kéfi et al., 2007), using a range of different approaches and frameworks at different scales, persistent challenges remain, and different approaches and methods are rarely sufficiently brought together. In particular, there is a need to understand more clearly the thresholds or tipping points that may result in catastrophic shifts, and to identify the early warning signs and overall impacts, such that appropriate measures can be put in place to manage environmental resources more sustainably. This requires a multidisciplinary approach. In the era of the 2030 Sustainable Development Goals (SDGs), which seek Land Degradation Neutrality (LDN) within SDG target 15.3 [i.e., any reduction in land quality is to be balanced by restoration or rehabilitation of already degraded areas of the same land type, resulting in zero net degradation (Barkemeyer et al., 2015)], advancing this understanding becomes even more critical. Better identification of early warning signals for such changes can inform the development of better-timed and more cost-effective solutions, allowing anticipation of, adaptation to, or even prevention of, undesirable ecosystem shifts (Sietz et al., 2017a).
This paper targets these challenges and presents an integrated study that necessarily took an ambitious, multidisciplinary approach considering the whole pipeline from drivers of catastrophic shifts through to actions to prevent and manage them. This comprehensive view offers considerably novelty to the literature, as most studies focus on just a single aspect of change. The research team comprised soil scientists, (spatial) ecologists, botanists, and specialists in land degradation, desertification, climate change, environmental engineering, stakeholder engagement, and environmental and economic modeling. Such diversity allowed us to conduct research from patch scale (plant–soil interactions) through to regional scale (modeling of management options). Patch scale work focused on six European landscapes that included degraded and reference (not necessarily pristine) ecosystem states with different aridity levels. We formulated broad research questions that we could answer more comprehensively by including data and findings from multiple disciplinary perspectives. This paper synthesizes the results from this multi-site, multi-disciplinary approach to answer three key questions:
(1) How do major degradation drivers affect ecosystem functioning and services in Mediterranean ecosystems?
(2) What processes happen in the soil and vegetation during a catastrophic shift?
(3) How can management of vulnerable ecosystems be optimized using these findings?
Addressing these questions, we draw heavily on the final summary report of our project, synthesizing from that (see CASCADE, 2017). The next section sets out our conceptual framework and the overarching research design. We then present the types of methods used, and the headline results from each type of method. Necessarily in such a multi-disciplinary paper it is not possible to provide all the methodological details, so we keep this at a broad level and point the reader toward more detailed methodological explanations in related papers. We then discuss the wider, integrated implications of our findings and approach.
Framing Catastrophic Shifts
The fold catastrophe framework (Figure 1) shows how increased pressure leading to unfavorable external conditions can cause an ecosystem to degrade (moving from A to B) and slowly shift toward a degraded state (C). This framework underpins our study. The maximum pressure that can be absorbed by the ecosystem is viewed as the ecosystem’s resilience. As long as the environmental pressure does not surpass the resilience, the system has the capacity to return to its previous state when the pressure decreases or ceases (Daliakopoulos and Tsanis, 2014). Sensitivity to environmental pressure increases when the ecosystem approaches a tipping point (Figure 1-B). For example, an increase in grazing may lead to only a marginal decrease in vegetation cover, but it can make the system more sensitive to external pressure (e.g., drought) until a tipping-point is reached. At this point the ecosystem shifts to an alternative stable state (Figure 1-C), characterized by a different structure and functioning, and which may no longer be able to provide sufficient food for herbivores. Knowing the resilience a priori is impossible, as it usually depends on feedback loops between biotic and abiotic components of the ecosystem. This makes catastrophic shifts difficult to predict (Walker et al., 2004). Furthermore, catastrophic shifts can be triggered by changes that operate across scales (e.g., climate change causes an increase in fire frequency and intensity which has knock-on implications for vegetation and soil), which can lead to cascading regime shifts (Rocha et al., 2018). This adds further complexity in attempts to understand catastrophic shifts.
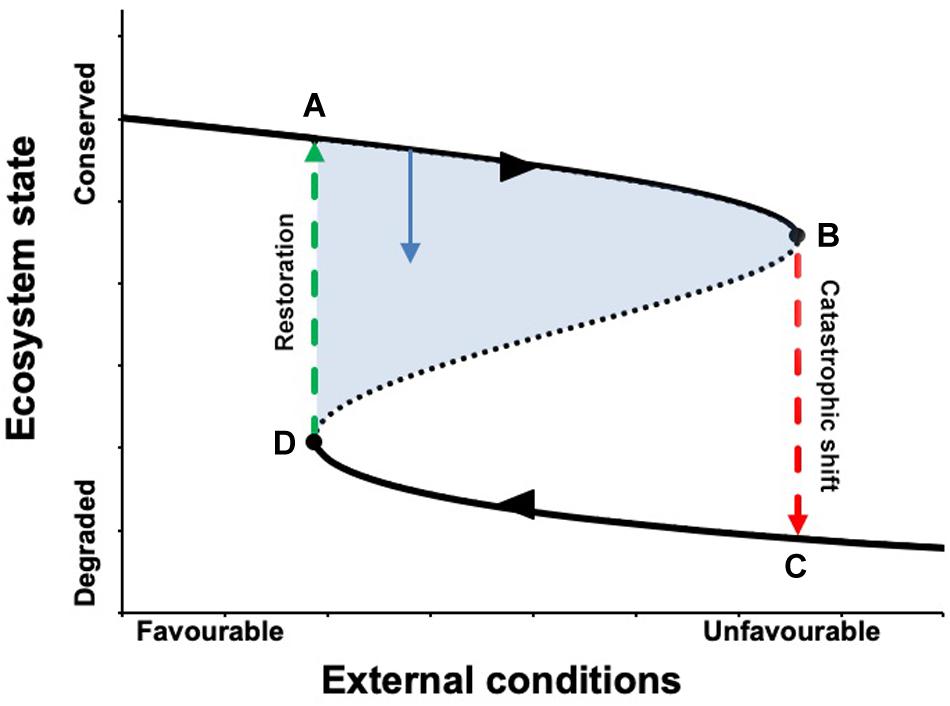
Figure 1. The catastrophic shifts concept. The upper solid line following the arrow represents the gradual decline of the ecosystem as the external pressures become increasingly unfavorable (section A–B). Point ‘B’ represents a point where the ecosystem cannot maintain a conserved ecosystem state and is undergoing a catastrophic shift to a degraded state (point ‘C’). The lower solid line following the arrow represents the gradual restoration of the already degraded ecosystem when the external conditions become more favorable (section C–D). Point ‘D’ represents a point at which the ecosystem is restored to a conserved state (‘restoration’). The latter transition can almost only be achieved under active restoration. The blue area represents resilience, the blue arrow a disturbance, and the black dots tipping points. Degradation can occur anywhere in the figure, while restoration can occur from any point on the upward lower branch.
Catastrophic shifts occur under external stresses, pressures or disturbances, such as decreased water availability or increased grazing (Kéfi et al., 2007). The timeframe over which a catastrophic shift occurs can have a broad range, and varies between ecosystems. For example, Demenocal et al. (2000), found the catastrophic shift of the Saharan region from annual grasses and shrubs to desert conditions took around 500–600 years, while Scheffer et al. (1993), recorded that transitions between stable states in shallow lakes can take between 1 and 5 years. The time over which a catastrophic shift occurs depends on the size and nature of the ecosystem (Biggs et al., 2009), and can take place over timescales from days to years. A slow ecological change may result in an abrupt economic change if the shift is toward a degraded state, as slow moving and fast variables occur concurrently (Reynolds et al., 2007; Stringer et al., 2017).
Indeed, a catastrophic shift to a degraded state is often accompanied by a loss of more than one resource, both socio-economic and in terms of natural capital (Scheffer and Carpenter, 2003; Fraser and Stringer, 2009), and restoration does not affect these resources such that a shift back to the original desirable state happens. The absence of a resource may prevent transition to a conserved state, particularly in dryland systems (Jeltsch et al., 2000, 2014). The alternative situation is not always desirable and may hold inferior ecosystem value compared to the initial system, both ecologically and in relation to human demands placed upon that system (Figure 1). For example, similar transitions can take place between different states of a forest where high fire frequency and loss of resources (e.g., seed bank or soil) combine, leading to changes in the phenology of the vegetation, and ultimately to a stable shrub land. As long as resources do not recover, the system would remain locked in this state.
Predictions of catastrophic ecosystem shifts tend to use either a single indicator approach [e.g., considering the frequency distribution of vegetation patch sizes in arid Mediterranean ecosystems (Kéfi et al., 2007)] or a systems approach, taking into account multiple ecosystem properties, functions and outcomes (e.g., Kuiper et al.’s 2015 study on shallow lakes). Our study takes a systems approach, harnessing the multidisciplinarity of the team to focus on multiple elements of our study ecosystems.
Study Sites
We worked in six study sites across the entire Euro-Mediterranean region (Figure 2) covering a range of aridity levels, land uses, and degradation drivers [Table 1; with land cover classes relevant to the study extracted from CORINE Land Cover (CLC) 2006 (European Environmental Agency [EEA], 2007)]. Figure 3 depicts a typical landscape in each of the study sites for both non-degraded (left) and degraded (right) locations. While forested areas (Ayora and Várzea) are susceptible to both the direct and indirect impacts of recurrent wildfires (Hosseini et al., 2016, 2017a; Santana et al., 2016), rangelands face soil erosion owing to poor vegetation cover (Albatera-Santomera, Messara and Randi) or shrub encroachment (Castelsaraceno), due to overgrazing and inadequate grazing management (leading to undergrazing in Castelsaraceno). Both forest and rangelands are affected by drought and land abandonment. The main causes of degradation at each site differ, but are always associated with the accumulated impact of a driver: forest fires, marginal agriculture and grazing, and long-term poor land management. Less often, causes are related to climate, which acts as a catalyst by making the system more vulnerable to disturbance, stress or pressure. This accumulated impact might cause a catastrophic ecosystem shift (Müller et al., 2014), which can lead to abandonment. In the Mediterranean, land abandonment is widespread and increasing (Bielsa et al., 2005; Sluiter and de Jong, 2007; Duarte et al., 2008). After abandonment, the land might not supply the same types or quantities of ecosystem services as before (Novara et al., 2017). All study sites are affected by summer drought. The historical development and land use of the sites are described in Daliakopoulos and Tsanis (2014), along with the effect of the changing climate (Daliakopoulos et al., 2017). Legacies from past land management have shaped current land uses to either be focused on wood production or marginal grazing.
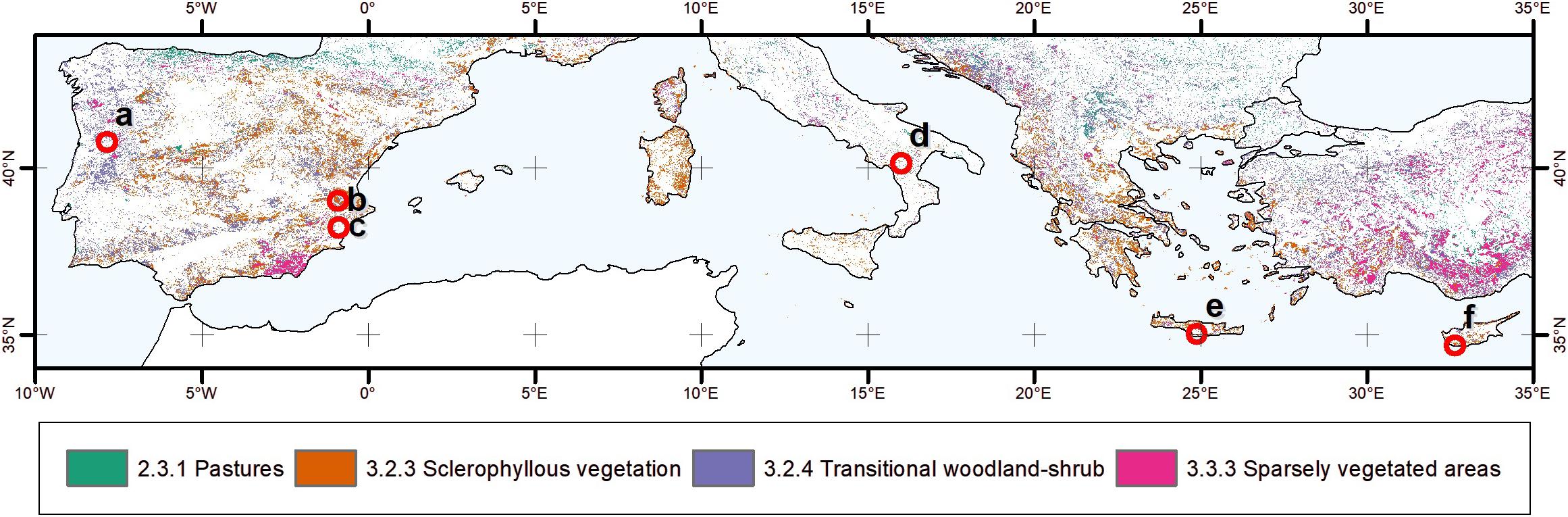
Figure 2. The CASCADE study site locations (a. Várzea, b. Ayora, c. Albatera-Santomera, d. Castelsaraceno, e. Messara, f. Randi), depicted on selected land cover classes relevant to dryland ecosystems. Land cover class nomenclature adopted from CLC (2018). Figure adapted from Daliakopoulos and Tsanis (2013).
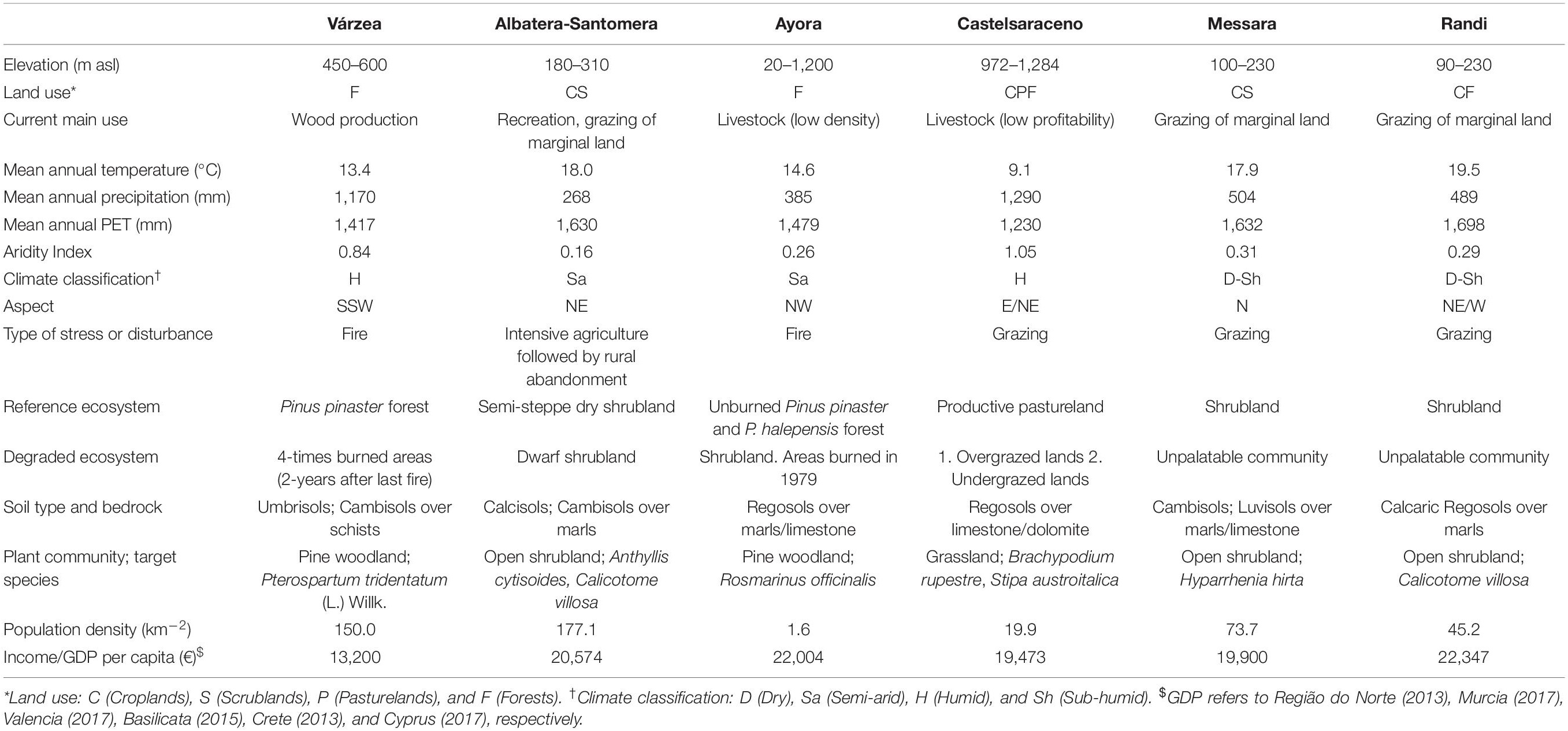
Table 1. Key characteristics of the study sites (adapted from Daliakopoulos and Tsanis, 2013).
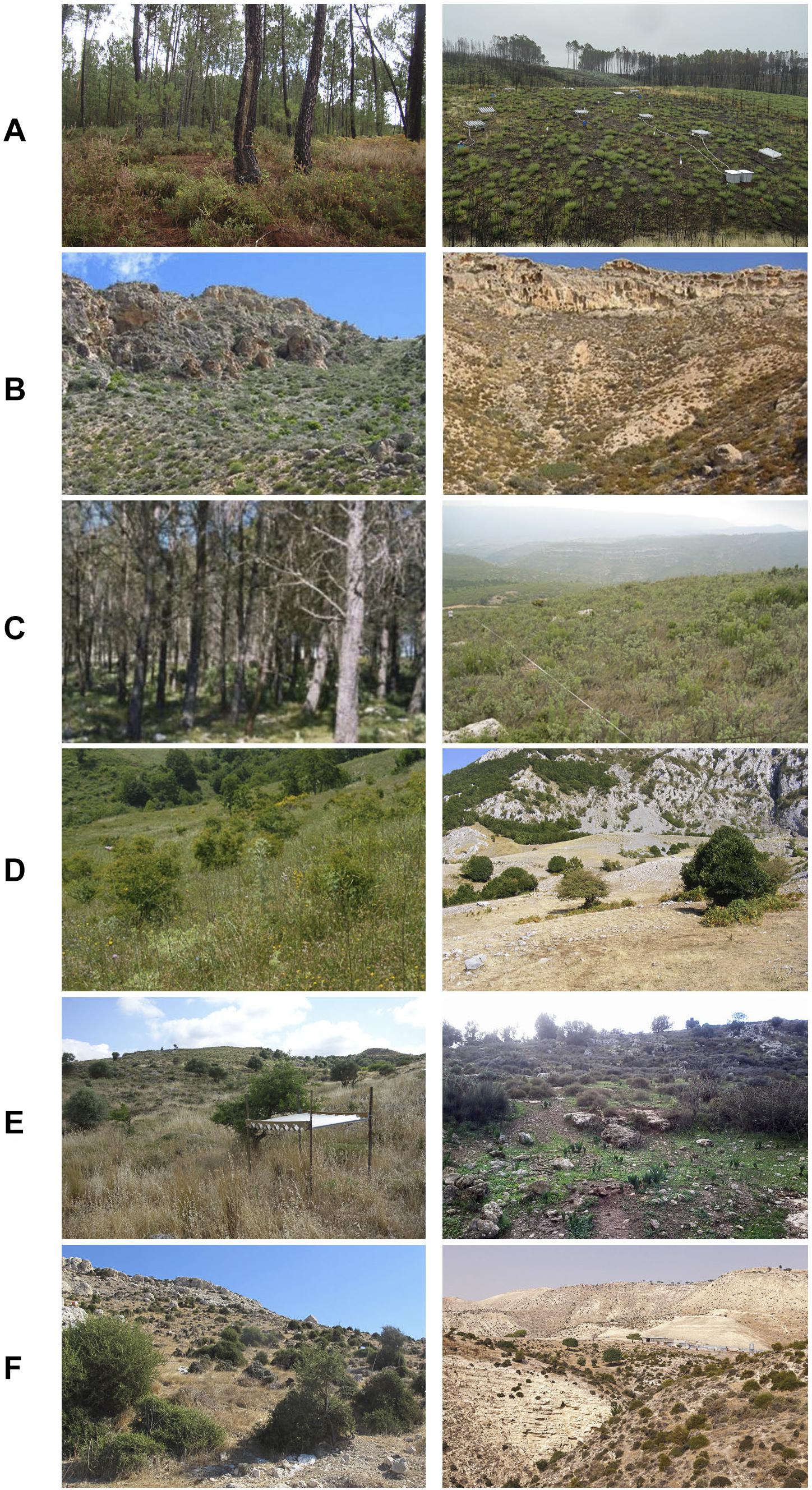
Figure 3. Images of non-degraded and degraded locations in each of the study sites. Panels (A–F) correspond with the locations on the map in Figure 2. (A) Várzea study site images: non-degraded (left) and degraded (right) photos by O. González-Pelayo. (B) Albatera-Santomera study site images: non-degraded (left – photo by S. Bautista) and degraded (right – photo by S. Bautista). (C) Ayora study site images: non-degraded (left – photo credit: CEAM Foundation) and degraded (right– photo credit: CEAM Foundation). (D) Castelsaraceno study site images: non-degraded (left) and degraded (right), photos by R. Salvia. (E) Messara study site images: non-degraded (left – photo by E. van den Elsen) and degraded (right – photo by I. Daliakopoulos). (F) Randi study site images: partly degraded (left – photo by M. Christophorou) and degraded (right – photo by E. van den Elsen).
Research Design and Methodological Approaches
This section sets out the range of methodological approaches used to address our three key research questions. Overall, we combined biophysical assessments and experiments, biophysical and socioeconomic modeling and participatory approaches. Several approaches cross-cut more than one research question, so we point toward the information each approach provides to address the various questions. References are provided should readers wish to follow up on any of the methodology and statistical analyses in more detail.
We began by selecting reference ecosystems and then identified drivers and stress levels within the six study sites (see Tsanis and Daliakopoulos, 2014). Stakeholders and the relevant policies that related to land use and land management were then identified for each site to provide framing information, helping to fine-tune the design of the experimental, modeling and participatory aspects.
Cross-Site Comparative Assessment of Ecosystem Services Provision
Question 1 (effects on ecosystem services) was addressed through a large scale observational study, replicated across the six study sites. At each site, we assessed the supporting and regulating ecosystem services provided by the studied ecosystems at different levels of degradation: Reference (healthy state), Degraded, and Restored (Valdecantos and Vallejo, 2015). Supporting and regulating ecosystem service categories were focused on because together with biodiversity, they are considered to be baseline services and properties that underpin other types of services (Bautista and Lamb, 2013), while most provisioning and cultural ecosystem services are considered to be context dependent (Rojo et al., 2012). We identified key properties of the ecosystems (and derived services) and assessed the degree to which they were affected by the degradation drivers. The three aspects of the evaluation process were: (1) determination of plant composition of the ecosystem, (2) quantification of standing plant biomass, litter and belowground biomass, and (3) application of the Landscape and Function Analysis (LFA) (Tongway and Hindley, 2005) methodology, which combines spatial distribution of vegetation and assessment of soil surface properties. Selection of these indicators was based on previous work (Bautista et al., 2017), with indicators representing a limited number of essential variables that can characterize ecosystem functioning for a majority of drylands worldwide, focusing on five aspects: water conservation, soil conservation, nutrient cycling, carbon sequestration, and diversity of vascular plants. The methodology was applied in all field sites for at least two ecosystem conditions representative of a healthy reference and a degraded state, and where possible, also in a restored area.
Biophysical Field Experiments
To investigate key biophysical processes that may happen in the soil and plants during a transition toward a degraded state (question 2: mechanisms and processes), we combined both observational and manipulative experiments in the plant–soil system across the six study sites, alongside an additional mesocosm experiment at community scale on specific processes and mechanisms of interest. Specifically, we investigated the response of the plant–soil system to increasing stress level by monitoring soil and plant characteristics along various stress gradients (Mayor et al., 2015, 2017), aiming to identify functional thresholds (Bestelmeyer, 2006) and their underlying processes in the soil–plant system. Two experiments were undertaken in the six study sites to identify soil and plant features that could indicate (imminent) ecosystem shifts. In a first ‘stress gradient’ experiment, we compared soil quality and performance of targeted plant species at three levels of environmental stress (low, medium, and high) due to grazing intensity, as they occurred under field conditions. We measured soil organic carbon and nutrient content underneath the plant patches and outside plant patches (inter-patches), including canopy cover, basal diameter and height growth, among other plant and soil characteristics (Mayor et al., 2015). A second, manipulative ‘drought stress’ experiment was conducted to assess the effect of increasing drought on soil condition and plant performance (Mayor et al., 2017). Using ∽2 m2 translucent roofs, we completely excluded direct rainfall from single vegetation patches and associated upslope interpatches. The duration of the experiment varied between sites to guarantee that the rainfall-exclusion plots received around or below the 1st percentile of the mean annual rainfall for at least 1-year period. The roofs were made from two layers of V-profile transparent polycarbonate gutters, which drained the rainfall into an external gutter and storage tank (Figure 4). As controls, we used roofs placed upside-down that let the rain fall through (i.e., roofed controls), as well as vegetation patches without roofs (open controls). We performed the drought-stress experiment for areas under medium grazing or fire-frequency stress, and monitored the same set of soil and plant variables as in the previous stress-gradient experiment.
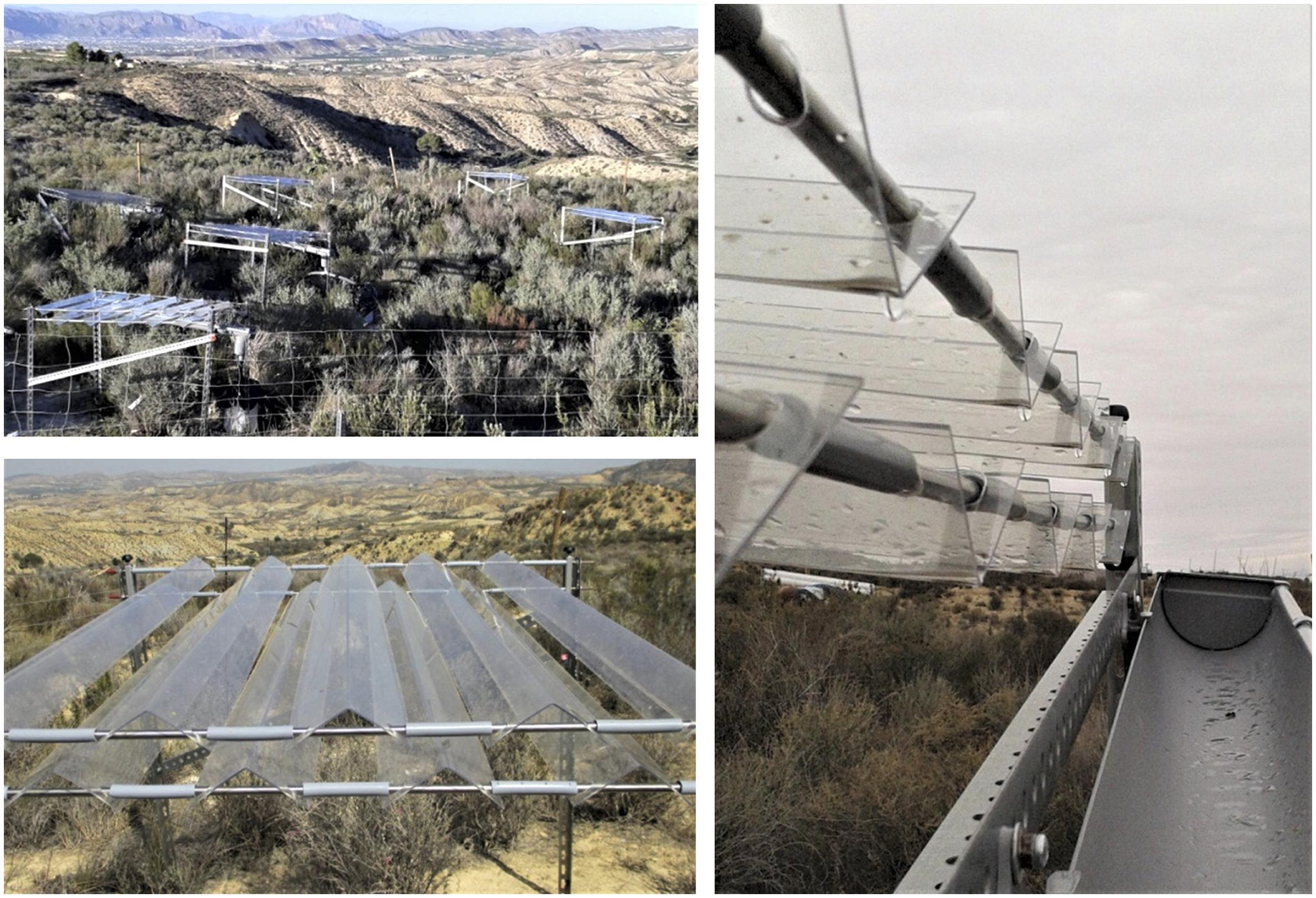
Figure 4. General view of the drought-stress experiment in Albatera-Santomera (above left) and details of a roofed-control plot (bottom left) and a closer view on the roof with the gutter that captures the excluded rainfall (right).
At the plant community scale, we conducted a manipulative mesocosm experiment to study the effects of vegetation pattern on resource conservation, as well as the hypothesized eco-hydrological mechanisms that modulate dryland dynamics (Bautista et al., 2015). The intention was that the mesocosm experiment would provide information linking that from the field experiments and models (discussed later) to address particular general processes that are common across Mediterranean ecosystems. On a set of 18 (2 × 1 m) runoff plots, we created a variety of plant cover types by planting three contrasting species with either a coarse-grained pattern (six large patches of nine individual plants each) or a fine-grained pattern (18 small patches of three plants each). The species used were one perennial grass (Lygeum spartum L.) and two shrubs (Atriplex halimus L., and Phillyrea angustifolia L.). There were three plot replicates for each treatment combination (2 spatial patterns × 3 plant species). Over a 1-year period, we monitored, runoff and sediment yields from each plot, bare-soil connectivity, and plant growth (Bautista et al., 2015).
Modeling of Vegetation Dynamics
A number of mechanistic models of vegetation dynamics were developed to understand and predict catastrophic shifts in Mediterranean ecosystems, particularly drylands. These developments focused on two axes of improvement to current models relevant to studying resilience: (i) the way external stresses are incorporated, focusing on three types of stress: grazing, drought and fire; and (ii) the way vegetation (the ‘biotic component’) is modeled, incorporating species, and species-species interactions in vegetation models. These models contributed to question 2 (mechanisms and processes), linking plant–soil and landscape responses to stress, and provided useful insights to question 3 (management implications) The models were of different types: (i) spatially explicit models of dryland vegetation dynamics that describe feedbacks between vegetation pattern and local environmental conditions, incorporating either grazing as a spatially explicit stress (Schneider and Kéfi, 2016) or ecohydrological feedbacks between spatial pattern and resource redistribution (Mayor et al., 2019); (ii) a spatially explicit version of the water limitation model by Rietkerk et al. (1997) that was coupled with a hydrological hillslope model that explicitly describes depth independent infiltration and infiltration excess runoff generation (Siteur et al., 2014); (iii) a spatially implicit model for vegetation cover including different plant functional types with different fire-responses, and their feedback with fire occurrence (Baudena et al., 2020); and (iv) a spatially implicit model including the coupled dynamics of different plant functional types and soil moisture, with facilitation between plants due to grazing protection and infiltration improvement, and competition for water (Verwijmeren, 2016). We investigated how these ecological mechanisms affected the response of the ecosystem to stress, looking for shift behaviors and identifying the conditions that favored the emergence of shifts at the ecosystem scale.
Participatory Assessment
Stakeholders (land managers, land users, local administrators, and experts/consultants) were engaged from the very beginning of the project, in line with good practices in the literature (Reed et al., 2014). This participatory approach was especially important in addressing research question 3 (management implications). Perceived current and future changes in environmental conditions and adaptations to those conditions were explored through focus groups held near the start of the research. Existing land management practices were subsequently assessed using the standard World Overview of Conservation Approaches and Technologies (WOCAT) technology questionnaire (Wocat, 2008) with stakeholder involvement. Findings from this assessment formed the basis of an in-depth study on sustainability and resilience of land management practices vis-à-vis ecosystem thresholds and disturbances (Jucker Riva et al., 2016, 2018).
Economic Modeling
Experimental and modeling results, as well as the WOCAT questionnaires, were used to model the economic effects of different management scenarios (De Ita et al., 2017), following the rationale described in Sietz et al. (2017a). This contributed toward answering research question 3 (management implications). The socio-ecological effectiveness of land management was also evaluated by linking non-linear ecosystem behavior to an economic evaluation of land management options, to provide essential insights into appropriate timings, climate-induced windows of opportunity and risk and financial viability of investments (Sietz et al., 2017a). These considerations informed an integrated modeling assessment in which the effects of a set of management scenarios were evaluated regarding their ecological impact, i.e., the likelihood that vegetation cover remains above a critical threshold, as well as their economic impact, i.e., net present value over a period of 10 years (Sietz et al., 2017c).
Future changes and expectations expressed by stakeholders make management scenarios more realistic and coherent with their willingness to act (de Vente et al., 2016), underscoring the need to ensure stakeholder engagement formed a fundamental part of our integrated assessment. Economic modeling results, information on past and present land management practices obtained using the WOCAT questionnaires, and information on relevant policies, were then combined to formulate management guidelines. The guidelines were discussed with stakeholders in workshops held in each of the six study sites. Feedback from stakeholders was used to fine-tune the scenarios. Finally, project results were presented to, and discussed with, policy stakeholders from all sites in a policy workshop, as well as being made available in an information hub that includes relevant information regarding catastrophic ecosystem shifts, soil and plant processes, the study sites, and management information in the form of videos and documents directed to the general public.1
Results
This section presents our key findings in relation to each of the research questions.
1. How Do Major Degradation Drivers Affect Ecosystem Functioning and Services in Mediterranean Ecosystems?
Overall, results showed that degradation pressures severely impacted ecosystem properties and services of the selected Mediterranean ecosystems. Losses of ecosystem services tend to be higher for drier climates (Figure 5). Some observed changes from reference toward degraded states suggest that certain degradation thresholds might have been passed. Catastrophic ecosystem shifts in Mediterranean ecosystems are therefore transitions that generally result in decreased plant cover and diversity, accompanied by increased loss of resources and reduced delivery of ecosystem services.
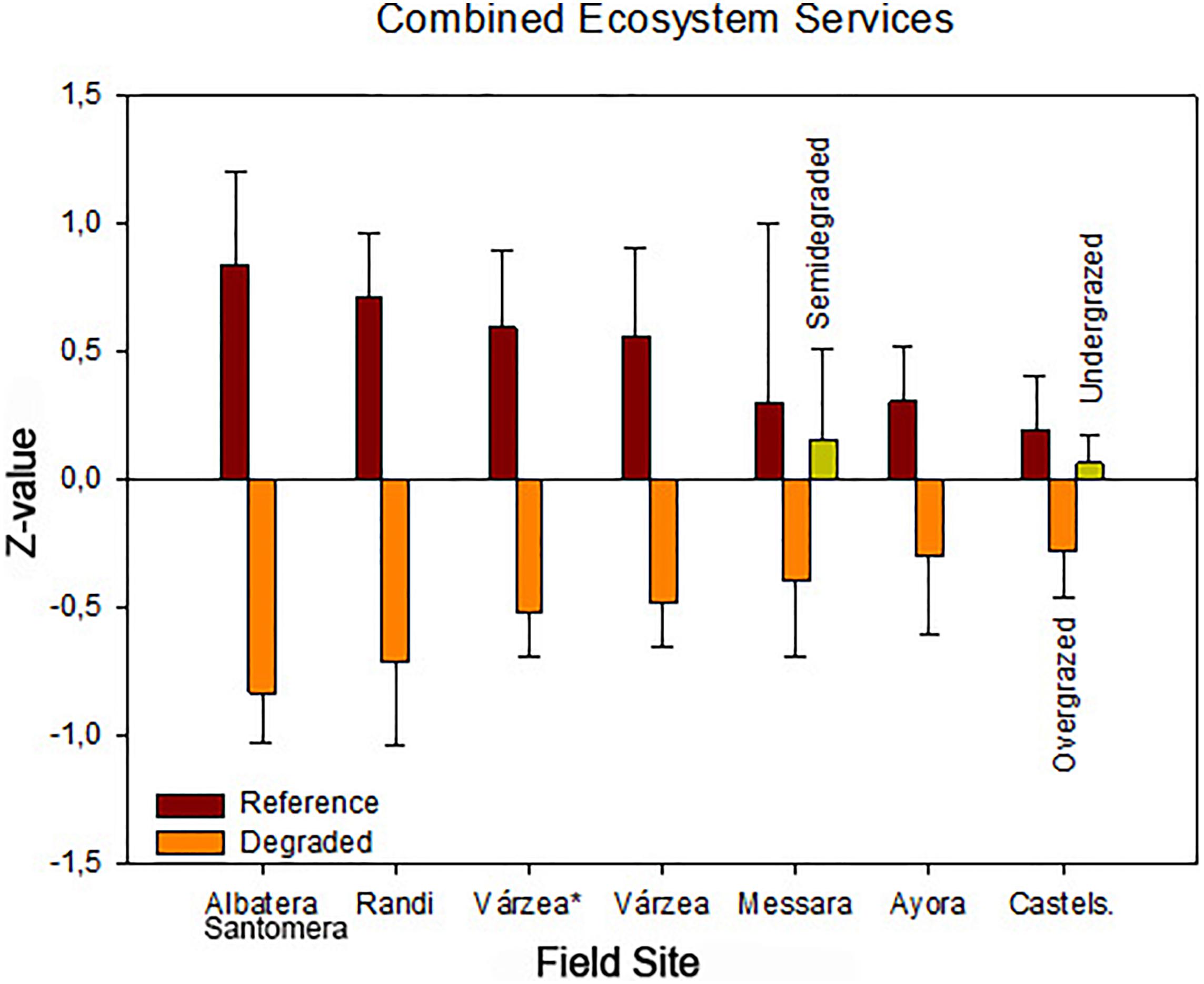
Figure 5. Summary of the loss of standardized ecosystem services (water and soil conservation, nutrient cycling, C sequestration, and fire risk reduction, the latter only in Ayora) and biodiversity due to the local degradation stress in all field sites. Bars represent an average of all five ecosystem services evaluated. Várzea* refers to the C sequestration service including estimated biomass of the overstory with bibliographic data.
Although the field sites include different Mediterranean ecosystems with different biogeographical and historical characteristics, in general the plant communities in the degraded situations were very different from the respective non-degraded references, both in composition and species abundance (Valdecantos and Vallejo, 2015; Valdecantos et al., 2016). Higher stress resulted in more homogeneous communities than in undisturbed states, as reflected by lower values of species diversity and evenness (Table 2). Field sites affected by grazing showed a general decrease in plant diversity with grazing pressure, and most can therefore be described as overgrazed (Perevolotsky and Seligman, 1998). We further observed a profound change in species composition in all grazed sites, though more modest in Castelsaraceno. Aboveground biomass was reduced in two out of three grazed sites (Castelsaraceno overgrazed and Randi) but increased in Messara (Table 2). Plant spatial pattern and distribution in the grazed states was markedly different than in the un-grazed ones, with more open areas and lower length and width of the plant patches in the grazed plots. These changes reduce the capacity to retain resources (sink effect) as observed in other areas subjected to grazing (Papanastasis et al., 2015) and, hence, reduce resource conservation. Similarly, LFA derived indices (infiltration, stability and nutrient cycling) were lower in all degraded sites than in their respective references, suggesting degradation of soil surface conditions and, thus, soil, water and nutrient degradation in the system (Papanastasis et al., 2015). Nevertheless, grazing, especially when intense, represents an important tool to reduce fire risk in areas with prolonged drought periods by reducing the amount of fuel available. In these cases, grazing systems provide another service to people as they reduce the fire hazard.
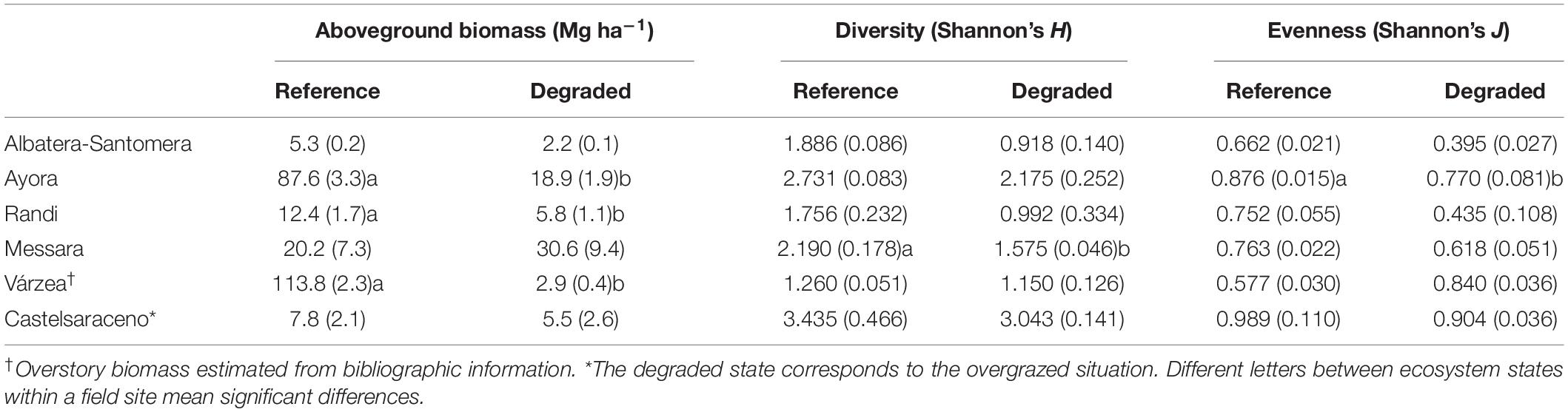
Table 2. Plant biomass and diversity indexes (mean values and standard errors in brackets) in the undisturbed and degraded states of the six CASCADE field sites, ordered from most to least arid.
The sites with fire pressure offer two complementary pictures of secondary succession after wildfires: a very initial stage of vegetation recovery in Várzea and a mature continuous shrubland without tree-canopy recovery in Ayora. In the short term, the ecosystem shows important reduction in species richness, biomass, vegetation patches, stability, infiltration and nutrient cycling (Table 2). These result in an overall significant loss of ecosystem services, but this Maritime Pine forest ecosystem has the ability to recover most, if not all of them, with time, provided pine regeneration rates are adequate. Thirty-five years after fire, the Ayora burned areas recovered ecosystem functionality to values of the reference pine forest and showed a spatial arrangement of vegetation that better conserves resources, alongside similar accumulated amounts of understory and belowground biomass and litter. Pine regeneration after fire, and hence catastrophic shifts, depend on factors such as fire-interval, pre-fire basal area, slope aspect, land use history or competition with grasses at the seedling stage (Pausas et al., 2002, 2004; Baeza et al., 2007; Maia et al., 2012, 2014). Scarce presence of pines in the degraded states of Ayora field site resulted in a significant reduction of C sequestration and could be improved by appropriate post-fire management. The observed shift from forest to non-forest (shrubland) vegetation observed in Ayora is not uncommon, especially in drylands, and is likely to occur in the very high fire recurrence of the degraded plots in Várzea. The short interval between the two latest fires (2005 and 2012) may cause the change from forest to non-forest vegetation in this area as the time for the first flowering in Pinus pinaster takes 4–10 years (Tapias et al., 2004). Landscape was also shown to be a determinant in allowing spontaneous regeneration of pines or reforestation success: Jucker Riva et al. (2017) detected a sharp difference in pine regeneration after fire between south-facing, exposed, steep areas (more challenging abiotic conditions: decreased humidity, increase erosion) compared to north-facing exposed areas, even when these areas were exposed to the same wildfires. This imbalance between fire regime and dominant plant species’ life histories or unfavorable post-fire conditions may result in a failure to recover pre-fire carbon stocks and hence the C sequestration service (Rocca et al., 2014; Santana et al., 2016). Stephens et al. (2013) suggest that this shift might not be catastrophic but would affect most ecosystem services. All ecosystem services showed significant short-term losses after the fire (Várzea) but only biodiversity and C sequestration losses lasted in the long term (Ayora).
Albatera-Santomera showed the highest relative losses of all individual ecosystem services of all field sites. It is the most stressed site as reflected by the very low aridity index (0.16; Table 1) and multiple diffuse pressures, stresses and disturbances that are and have been acting on the system for long periods. The main ecosystem properties affected by degradation were those related to the spatial distribution of vegetation and open areas (sink/source spatial pattern) that finally determine the conservation of resources. The degraded landscape showed a reduction of vegetation cover, with fewer and smaller patches of vegetation at longer distances from each other, and higher proportions of bare soil, which in turn, reduce the capacity of water infiltration and nutrient cycling, decrease water and soil conservation, and consequently reduce productivity (Boeschoten, 2013).
Informal field observations also indicate biodiversity was also substantially reduced in the degraded areas (Table 2), probably due to the absence of tall shrubs that act as keystone species in these semiarid shrublands (Maestre and Cortina, 2004). The stability index showed the lowest losses in the degraded as compared to the reference state. Previous work in semi-arid Mediterranean areas has shown that the stability index is less sensitive than the other LFA indices to detect differences between land uses and/or degradation levels (Mayor and Bautista, 2012). López et al. (2013) found lower values of the LFA stability index as degradation increased, associated with lower vegetation cover and patch density, length and width, but a further increase of the index with more intense degradation, as the exposed rock surface is higher and the sediment susceptible to being transported is lower. These results could therefore suggest that the system might have passed a threshold of irreversibility (Scheffer and Carpenter, 2003). The field sites affected by grazing showed a generalized decrease in diversity with grazing pressure (Table 2) and, hence, can be described as overgrazed (Perevolotsky and Seligman, 1998). Papanastasis et al. (2015) observed very similar results to those we obtained in the grazed sites, with a significant loss of biodiversity, plant cover, stability, infiltration and nutrient cycling indices, and patch size in heavily grazed areas. They also reported increases in some properties and ecosystem services under moderate grazing stress. Some authors have observed an increase in plant species diversity and composition when disturbed by grazing (Holocheck et al., 1989; Belsky, 1992), partly because of the change of competitive relationships between plant species (Crawley, 1983), especially of those that are unpalatable. However, many dominant species of grazed rangelands present morphological and biochemical mechanisms to withstand grazing providing a relatively high system resilience (Perevolotsky, 1995). Aboveground biomass was reduced in two out of three grazed sites (Castelsaraceno overgrazed and Randi) but increased in Messara (Table 2). In sites which present a long history of grazing, higher levels of pressure may not significantly affect productivity.
Ecosystem services showed important losses due to grazing in the order Randi> Messara> Castelsaraceno following a decreasing order of aridity, although differences between Randi and Messara are smooth. Wang et al. (2014) established 0.32 as the threshold value of the aridity index that determines net N losses or accumulations. Castelsaraceno and Randi are well above and below this value, respectively, while Messara is around this threshold, suggesting that N accumulation processes prevail in the Italian site while N losses do in Cyprus (Table 1).
2. What Processes Happen in the Soil and Vegetation During a Catastrophic Shift?
Our results from the field experiments and from the biophysical modeling provided both independent and complementary insights into the mechanisms and main processes that may lead to a catastrophic shift in response to increasing stress. Findings from the field experiments focused on processes that happen in the soil–plant system; the mesocosm experiment combined with spatially explicit modeling informed us of the ecohydrological processes and feedbacks that link plant pattern and resource loss, and control vegetation dynamics and catastrophic shifts in drylands. Finally, a suite of models provided insights into the long-term ecosystem processes in response to a variety of degradation drivers. Results from each approach are presented in turn, followed by a summary of our findings on potential early warning signals of an imminent shift.
Response of the Soil–Plant System to Increasing Stress: Findings From Field Experiments
At four of the six field sites, we assessed the response of soil quality and plant performance to a grazing-pressure gradient. We found that the response to increasing grazing was dependent on the climatic conditions. In Castelsaraceno and Albatera-Santomera sites, soil quality did not vary significantly between grazing-pressure levels, yet Albatera-Santomera exhibited a gradual trend toward higher nutrient availability and larger amounts of soil organic N and C from high-stress to low-stress plots. These two sites represented the wettest and the driest field sites, respectively, and were subjected to grazing pressures that were, overall, low to moderate. The relatively wetter climate of Castelsaraceno, together with its high vegetation cover, might make its soil–plant system robust enough to deal with the current grazing levels. In the case of Albatera-Santomera with its very dry conditions and reduced overall vegetation cover, one might expect that soil conditions are mainly affected by water availability, being the limiting factor for vegetation cover and growth and, ultimately, soil quality. Conversely, Messara and Randi showed noticeable effects of increasing grazing on several soil quality indicators, with a general decrease in soil quality with increasing grazing. However, no clear pattern emerged as to how grazing-pressure levels were linked to threshold values of soil quality indicators, with gradual changes in most sites and sharp changes in Randi, the site with the highest overall grazing pressure. Most soil variables showed higher values in soils underneath plant patches than in the interpatches, with the ratios patch/interpatch generally increasing with increasing stress.
The effects of the different grazing-pressure levels on the performance of the targeted plant species differed markedly between the four grazing study sites. In Albatera-Santomera, canopy cover and basal twig diameter of Anthyllis cytisoides did not vary noticeably with grazing-stress level, although there was a tendency for twig growth to be higher at the high stress plots, probably due to compensatory growth (Oesterheld and McNaughton, 1991). In Randi, canopy cover and branch basal diameter of Calicotome villosa showed higher values for the low and intermediate pressure levels than for the high pressure level. In Castelsaraceno and Messara, neither plant cover nor biomass of the target grass species showed clear differences among the three grazing stress levels.
A possible explanation for these findings is that grazing impacts are reduced not only in marginal ecosystems that are essentially controlled by lack of water availability (Albatera-Santomera) but also in productive ecosystems with plenty of water available and high vegetation cover (Castelsaraceno), except perhaps, if grazing stresses become much higher than at present. From the two sites with less extreme conditions, Messara showed a noticeable impact of grazing stress level on soil quality but not on plant performance, while Randi revealed a consistent grazing effect on both soil quality and plant performance.
The drought-stress manipulative experiment, performed at all six field sites, yielded no consistent results across sites. For the driest sites, Randi and Albatera-Santomera, which also experienced a severe natural drought during the study period, we found increased nutrient availability and, to a lesser extent, higher carbon contents with increased drought stress. This is probably due to an increased amount of litter and dead roots being degraded (López-Poma and Bautista, 2014). However, none of the other four field sites showed any relevant effect of increased drought. Similarly, only Randi and Albatera-Santomera showed a decreasing trend in plant performance with increasing drought stress. These results indicate an extraordinary capacity of the plant–soil systems in Mediterranean areas to cope with drought, at least in the short-term, as only the combined effect of a severe natural drought plus the additional experimentally induced drought produced a decrease in plant performance.
Pattern-Dependent Ecohydrological Processes and Catastrophic Shifts: Combined Mesocosm and Ecohydrological Modeling Results
Our findings from the plant-pattern mesocosm experiment (Bautista et al., 2015) demonstrated that both plant cover and plant pattern exert a critical role in controlling water and soil conservation in patchy ecosystems. The connectivity of bare soil emerged as the most critical pattern attribute for explaining the hydrological behavior of patchy ecosystems, as it reflects and depends on both cover and pattern (Rodríguez et al., 2018). Greater bare-soil connectivity implies larger water and sediment losses from semiarid slopes, but also implies larger inter-patch areas, which benefits the downslope patch (Urgeghe and Bautista, 2015).
Although plant cover and biomass are the most common vegetation properties used as indicators of hydrological functioning, our results suggest that indices based on capturing the connectivity of the bare-soil matrix in patchy ecosystems, such as the Flowlength index (Mayor et al., 2008), have great potential as surrogates for the hydrologic functioning in drylands. These indices can be easily obtained from aerial photographs and incorporated into hydrological and erosion models at the hillslope and catchment scales.
By incorporating the Flowlength index into a spatially explicit model of dryland vegetation dynamics, we investigated the role of connectivity-mediated feedbacks that link vegetation pattern, resource redistribution and productivity in modulating the potential for catastrophic shifts. We found that a positive connectivity-mediated feedback between resource loss and decreasing vegetation cover decreased the amount of stress required to cause a catastrophic shift to a degraded state (Mayor et al., 2013). However, our modeling results suggest that such catastrophic shifts are less likely in drylands with a strong response of vegetation to source–sink dynamics (i.e., enhanced vegetation growth in response to a local increase in runoff from bare-soil areas to the plant patches) (Mayor et al., 2019).
Modeling Results
We developed a suite of different vegetation models that contributed to the fundamental understanding of the plant–soil responses of Mediterranean ecosystems to degradation drivers (Mayor et al., 2013, 2019; Siteur et al., 2014; Schneider and Kéfi, 2016; Génin et al., 2018a; Baudena et al., 2020). Models that incorporate spatially explicit grazing (i.e., that grazers tend to eat at the borders of vegetation patches rather than in their centers), predicted a higher probability and magnitude of catastrophic shifts under increasing stress (drought or grazing intensity) than previous models assuming homogeneous grazing (Schneider and Kéfi, 2016). Thus, the small-scale interactions between grazing and drought further decreased ecosystem resilience. A spatially implicit fire model revealed that while Mediterranean forests are resilient to fires under historical climate conditions, increases in drought, coupled with recurrent fires, may lead the Mediterranean forests to pass a tipping point and degrade into an open-shrubland (Baudena et al., 2020). This confirms earlier results from our spatially explicit water limitation model on how rainfall patterns under upcoming climate change in Mediterranean drylands induce and enhance the occurrence of catastrophic shifts in those ecosystems (Siteur et al., 2014).
Model results overall confirmed the importance of positive feedbacks in driving the emergence of alternative stable states at the ecosystem scale, and helped us to better understand how different interactions and drivers control Mediterranean ecosystems and their changes through time. Such knowledge can be extremely useful in supporting management decisions. For example, the model results from Baudena et al. (2020) underline the importance of planting seedlings from late successional, resprouting species, to increase resistance and resilience to forest fires (Valdecantos et al., 2016). Moreover, the essential role of facilitation for both species coexistence and ecosystem resilience highlighted by the models suggests that it may provide a good opportunity for ecosystem restoration. Thus, in degraded rangelands, remaining adult individuals of nurse species adapted against grazing can be used to improve the survival of planted seedlings (Verwijmeren et al., 2014; Verwijmeren, 2016).
Indicators and Early Warning Signals of Catastrophic Shifts
The model studies also explored the potential for spatial early warning signals of imminent catastrophic shifts in drylands, as the patterns of vegetation that arise as a result of near-neighbor interactions such as facilitation and competition. This follows the theory that certain vegetation pattern metrics may offer possibilities in identifying an upcoming critical transition in semi-arid ecosystems (Rietkerk et al., 2004; Kéfi et al., 2007, 2011, 2014).
The grazing model used by Schneider and Kéfi (2016) explored the predictive potential of indicators of spatial structure of a landscape (namely, the deviation from a power-law in patch-size-distributions) for catastrophic shifts under combined drought and grazing stress (Figure 6).
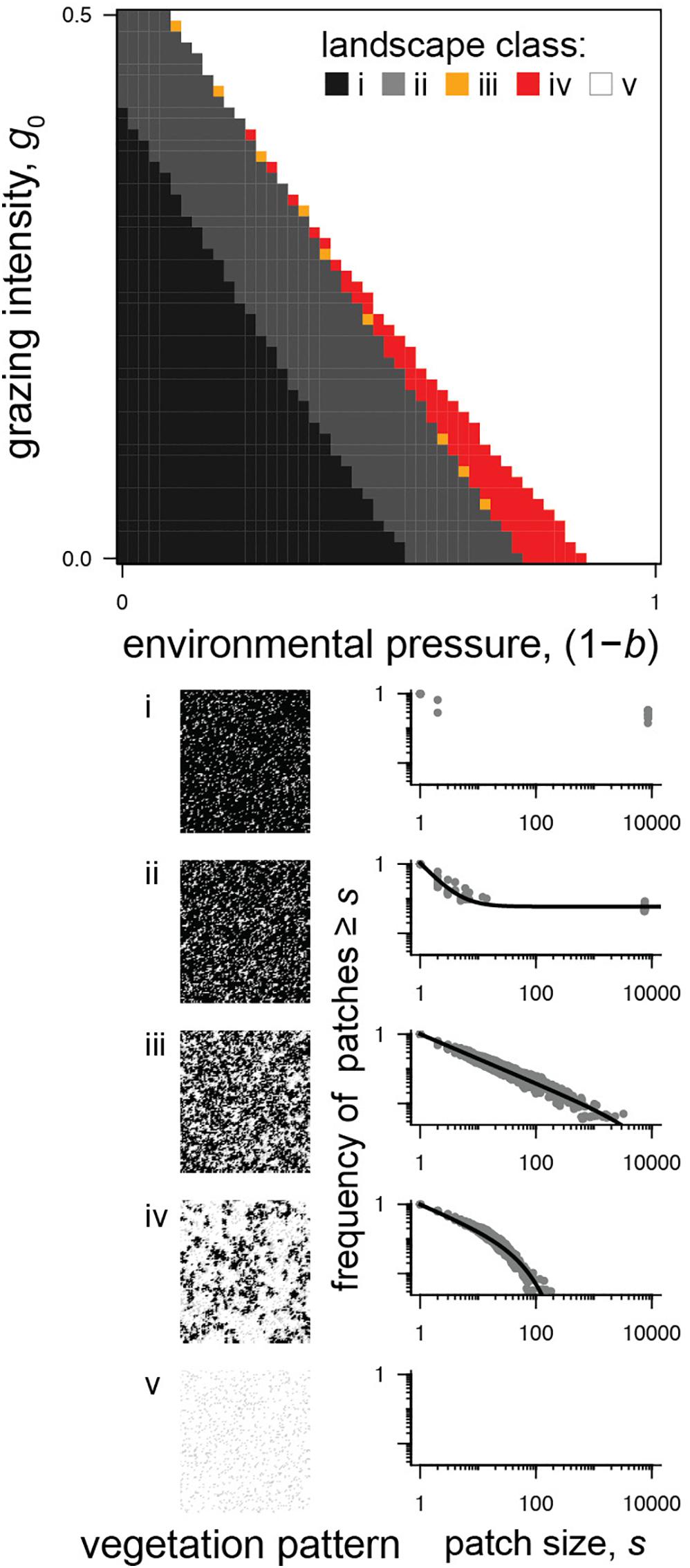
Figure 6. Model results of dryland grazing model by Schneider and Kéfi (2016). Model landscapes were classified based on the shape of the inverse cumulative patch–size distributions obtained from repeated simulation runs along gradients of environmental and grazing pressures. If crossing the tipping point, the system transitions from vegetated (i.e., shaded area) to desert (white area). Classes are i: full cover; ii: up-bent power-law with spanning clusters; iii: straight power-law; iv: down-bent power law (the early-warning-sign of a catastrophic shift); v: desert. Note that at high grazing pressure, a vegetation collapse was not preceded by down-bent power laws (iv).
Addition of spatially heterogeneous grazing (in contrast to uniform pressure) not only altered ecosystem stability (by increasing the probability of catastrophic shift) but also blurred the early warning signals at high grazing pressure. This suggests that we need to be cautious regarding the use of early warning signals of ecosystem degradation when the pressure at play has a spatially explicit component (see also Génin et al., 2018a). It also suggests that additional degradation indicators need to be developed taking into account the spatial component of the stressor.
Model simulations of our spatially explicit ecohydrological model (Mayor et al., 2013) suggested that changes in bare-soil connectivity (e.g., Flowlength index; Mayor et al., 2008) may be more informative as an early warning indicator of dryland degradation than changes in vegetation cover. We found that positive deviations of the Flowlength index from the expected values for a random vegetation cover pattern sharply increased in the proximity to transitions toward degraded states. This result suggests that an extraordinary increase in bare-soil connectivity may lead to unavoidable degradation (Rodríguez et al., 2018).
By exploring different types of spatial and temporal indicators as potential early warning signs of catastrophic shifts, the model studies provide a deeper understanding of when and where the indicators might be applicable in real world systems (Kéfi et al., 2014; Schneider and Kéfi, 2016; Génin et al., 2018a, b). Quantifying those indicators derived from model studies on field data, particularly with the prospect of increasingly affordable remote sensing technology, will help identify sites at higher risk of catastrophic shifts, allowing prioritization of those for conservation measures.
3. How Can We Better Manage Vulnerable Ecosystems?
At the ecosystem scale, land management measures adopted by land users to utilize, enhance or maintain the resources provided by the environment, provide an important link between the ecological and socio-economic aspects of the system (Hurni, 2000). To assess measures and improve upon them required us to understand the values and perception of land users and managers, as well as their livelihood strategies and possibilities for action. Based on information provided by stakeholders on perceived and future environmental changes and adaptation to those conditions, we identified promising management practices within the study sites, assessed them and described them through a standardized methodology. This information was then used as a basis for modeling the economic effects of different scenarios (Sietz et al., 2017c) and their ecological impacts. Management guidelines building on these findings were developed and evaluated with stakeholders in each study site, then shared with policy stakeholders from all sites in a policy workshop. This section synthesizes the key findings from these activities.
Stakeholders’ responses to regime changes suggested that they adapt their land management mostly in a reactive way. It remains difficult to accurately identify tipping points and forecast regime shifts in ways that are useful for land users and managers to prevent a shift. Measures or adaptations that increase ecosystem resilience, nudging the tipping points further away from the system’s current state, appeared to be the most effective strategy.
Across the study sites, adaptations encompassed environmental management, socio-political measures and economic measures. Environmental management changes were reported most often (forming 67% of all measures across sites) and varied across sites. For example in Cyprus, stakeholders mentioned environmental management measures such as road clearing, building terraces to reduce erosion, and growing drought resistant plants; while in Italy stakeholders mentioned that they had to actively manage the forests by removing drought affected trees and planting fast growing trees, and relocating beehives (De Ita et al., 2017). Across all study sites socio-political measures constituted 15% of measures and comprised improvement of land use and environmental management through cross-sector organizations (Italy), advancing or creating policies for land use (Spain and Portugal) and patrolling to prevent illegal practices (Cyprus). Under socio-economic and cultural measures (constituting 18% of measures), stakeholders mentioned subsidies (Spain, Greece, Portugal, and Cyprus) and migration (Italy) as ways in which catastrophic shifts have been managed.
Understanding how we can better manage ecosystems vulnerable to catastrophic shifts requires an appreciation of how land management practices contribute to or reduce resilience to stresses, mitigate land degradation or rehabilitate degraded areas with long lasting effects (Jucker Riva et al., 2016, 2018). Research participants and local stakeholders identified, assessed and evaluated relevant management practices in each study site (Figure 7).
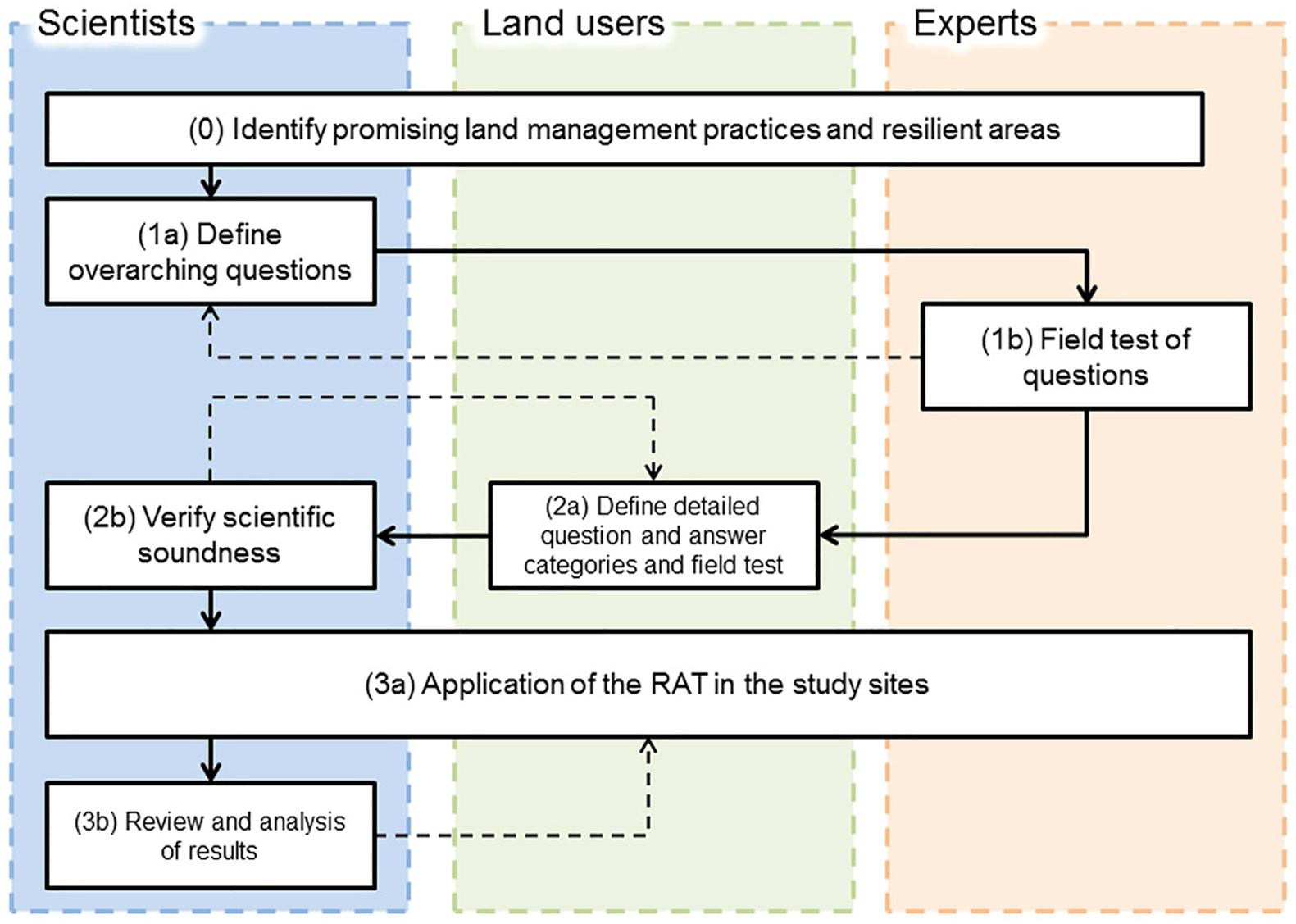
Figure 7. Development and application of the Resilience Assessment Tool (RAT) within this research and interaction with different stakeholder groups. “Experts” refer to local experts or land management advisors; “land users” includes land owners, shepherds, and forest workers. Adapted from Jucker Riva et al. (2018).
Using the Resilience Assessment Tool (RAT) in Figure 7, researchers were able to compare land management practices with regard to resilience. Almost all the management practices assessed have a positive impact on the resilience of the systems. However, three quarters have at least some negative impacts as they decrease the resilience toward some other stresses, pressures or disturbances. Our assessment suggests that different management objectives require widely different practices: practices that increase resilience to fire (e.g., clearing of vegetation, firebreaks), may reduce resilience to drought, while there is evidence that practices that reduce land degradation caused by land use (e.g., pine afforestation, conservation logging) can increase vulnerability to disturbances (fire and pest outbreaks, respectively). Thus, combining different land management practices (to reduce negative impacts of land use or to tackle different stresses) appears to be the best strategy to reduce the risk of regime shift while increasing sustainability of land use. Below we present our findings from the different contexts linked to the different stresses and pressures in our study sites (forest fire, grazing and land abandonment).
Forest Fire Context
Our findings indicate that stresses, especially fire, can induce catastrophic shifts in Mediterranean forest (Mayor et al., 2016). We identified and assessed three different ways in which Mediterranean forests can recover after stresses (Jucker Riva et al., 2016) and how these can be fostered up to 20 years before the stress occurs. While forest regeneration from seed banks is only possible with sufficiently long time intervals between fires, regeneration from resprouting individuals fosters a quick recovery, especially under beneficial conditions such as north-facing and gentle slopes (Jucker Riva et al., 2016). Our studies have also highlighted that establishing vegetation and especially mulch cover immediately after fire has an important role in preventing soil erosion and thus retaining nutrients and maintaining soil fertility (Mayor et al., 2016; Hosseini et al., 2017b). Remote-sensing analysis of the Ayora site (Jucker Riva et al., 2017) showed how recovery of Mediterranean forests after fire is highly dependent upon landscape and land use history, suggesting the need for adaptive, context specific management plans. Stakeholder engagement in developing and implementing such plans is vital. In depth, participatory assessment of land management practices (Jucker Riva et al., 2018) indicated that fuel management increases forest resilience without significant negative impacts, appearing to be the most important practice. These results led us to conclude that: (i) minimizing fuel load and connectivity reduces fire spread and intensity, increasing chances of recovery after fire; (ii) diversity of species reduces flammability, as well as outbreaks of pests, increasing resilience to multiple stresses; and (iii) sufficient protective soil cover shortly after a fire reduces soil erosion, reducing the likelihood of regime shift and long-term degradation.
Overgrazing Context
Grazing areas in the Mediterranean are mostly dominated by shrublands that appear very resilient. However, the combination of long-term grazing stress, climate instability and disturbances can reduce the biomass density in the long-term, triggering soil degradation and hydrological changes, decreasing fodder provision, slope stability and increasing offsite damage from runoff and sediment. Our observations and land users’ experiences suggest removing grazing completely may increase fire frequency and lead to vegetation changes. Thus, maintaining moderate grazing appears to be the most efficient strategy to maintain pasture productivity while avoiding catastrophic ecosystem shifts.
Using the RAT, we assessed beneficial management practices to mitigate grazing impacts while maintaining land use, finding these to include rotational grazing, fodder provision and area closure. The effectiveness of these practices was heavily dependent on climate: in the most arid areas, vegetation recovery can be slow or null, especially in the dry season. In Randi and in the overgrazed state in Castelsaraceno we observed a general improvement of ecosystem services by temporary grazing exclusion (5–10 years), especially in Randi where plant cover, litter accumulation and aboveground biomass recovered to similar levels found in the undisturbed reference areas.
Active restoration of degraded pastures in Messara aimed to transform land use from grazing to carob tree orchards as a silvopastoral system, rather than to recover the pre-disturbance state of the ecosystem. This practice was assessed through the RAT as providing multiple benefits such as additional fodder and shade for animals, decreased soil erosion, improved soil fertility and additional income through tree products. Controlled, moderate grazing was shown to be effective in reducing the risk of fire (Jucker Riva et al., 2018). However, once a fire has occurred on grazing land, it is important to allow a minimum of 2 years for resting (while providing supplementary fodder) or to actively revegetate, in order to prevent a catastrophic shift (Jucker Riva et al., 2018). Remaining plant individuals can be used as nurses to increase the recruitment of seedlings planted below.
Land Abandonment Context
In the study sites in which land abandonment was widespread, affecting mainly degraded, low productivity and remote areas, abandoned areas were found to have significant trade-off effects that may contribute to catastrophic shifts. Forested areas (Ayora, Várzea) and grazing areas (Messara, Randi) after abandonment have increased flammable biomass, leading to more frequent and intense fires, a known trigger of catastrophic shifts in forests. The grazing systems in Randi and Castelsaraceno are affected by animal pests (rats, boars), resulting from uncontrolled growth after abandonment, increasing shrub encroachment in grass pastures (Castelsaraceno) and Carob tree die-off (Randi). They are mitigated by short-term management options like tree protection and pasture fencing, but would require an integrated ecosystem approach to promote natural predators. Another problem identified by stakeholders is pasture degradation by invasive and/or unpalatable species or thorny shrubs, observed on intensively grazed areas but also on abandoned or marginal ones. Beside diversifying herds, options include manuring pastures, seeding fodder species and mechanically removing thorny shrubs.
Management options for abandoned land further include revegetation, rotational grazing or alternative land uses, such as bee-keeping, biodiversity management, tourism or wind/solar energy production. Furthermore, land that is not used economically at present can become valuable in future and thus knowledge and infrastructure might need to be maintained. Cooperation and new forms of management (e.g., silviculture) can help to overcome labor availability constraints.
Complementing the ecological assessment of the rangeland resilience model with insights into investment costs (e.g., costs to purchase supplementary fodder) and income through livestock production, we assessed different land management scenarios. Scenarios represented common management strategies including opportunistic and conservational grazing management in combination with varying levels of risk aversion (Table 2). Scenarios were designed in consultation with land users in the study areas reflecting destocking and restocking strategies with which land users respond to low and variable rainfall and associated vegetation dynamics. Opportunistic strategies, e.g., adjusting livestock density to pasture productivity in each year (Westoby et al., 1989; Lategan, 1995) allow land users to directly benefit from annual productivity changes but without considering the time span necessary for vegetation recovery. In contrast, land users using conservation strategies exclude livestock from part of the land in wet years while fully grazing the land in other years (Müller et al., 2007; Quaas et al., 2007). This ‘resting’ means that stocking rates stay below the grazing capacity in wet years facilitating vegetation recovery and potentially higher stocking rates in the following years. In our modeling approach, we distinguished degraded and restored starting conditions and particularly considered the emergence of windows of opportunities and risks (Figure 8) to capture critical land management timings that realize ecological benefits at minimum risk and cost (Sietz et al., 2017a). These windows reflect strong variations in environmental conditions, presenting particular opportunities for restoration or prevention of catastrophic shifts [e.g., exceptionally wet episodes, such as those associated with the El Niño Southern Oscillation (Holmgren and Scheffer, 2001)] and risks (e.g., severe drought; Staal et al., 2015). To illustrate major differences in the costs and benefits arising from management interventions depending on the stability domains, we considered seeding as a key restoration measure that directly affects an ecosystem’s state. For example, if a degraded ecosystem is positioned in a bi-stable domain (see unfavorable state in Figure 1), a priority situation for restoration, investments in land management coinciding with a window of opportunity have a greater chance of passing a critical threshold and generating higher gross benefits than those outside such a window of opportunity. If a critical threshold is passed, the ecosystem state (e.g., vegetation cover) improves naturally without any further maintenance costs (Figure 9).
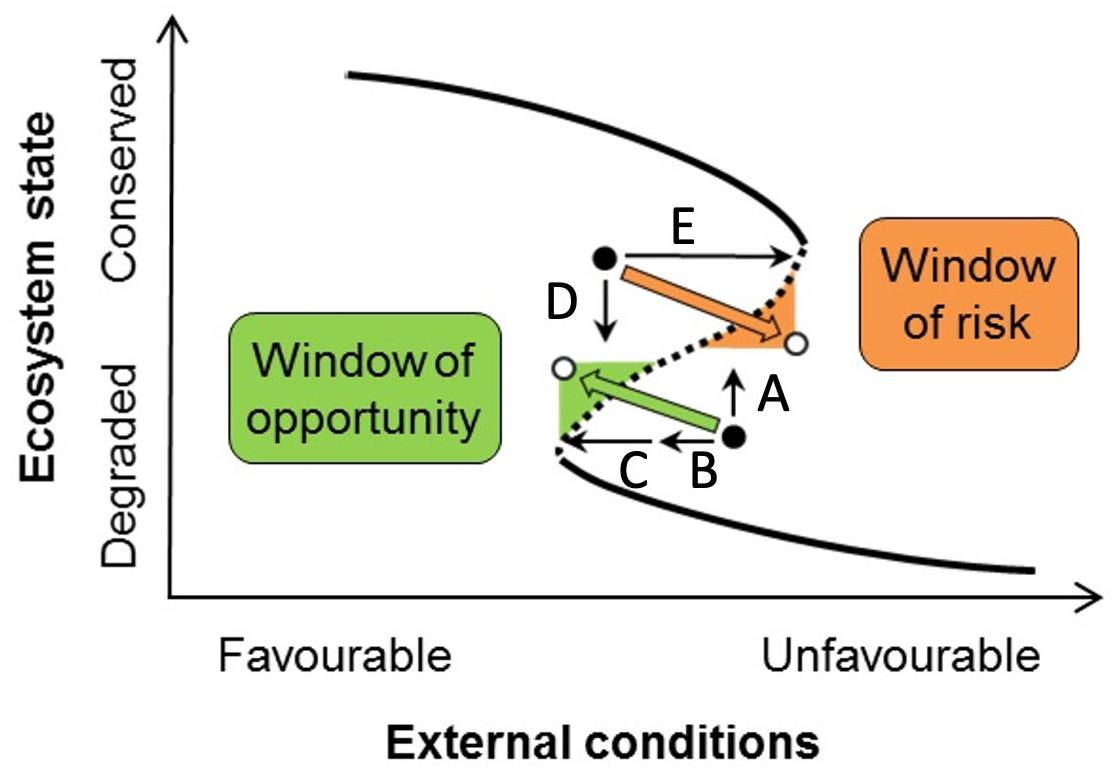
Figure 8. Windows of opportunity and risk (see Figure 1 for explanation of the concept of catastrophic shifts). Arrows exemplify effects of different types of management practices and external climate drivers: A, seeding; B, reduced grazing pressure; C, extremely wet episode; D, deforestation; E, drought (adapted from Sietz et al., 2017a).
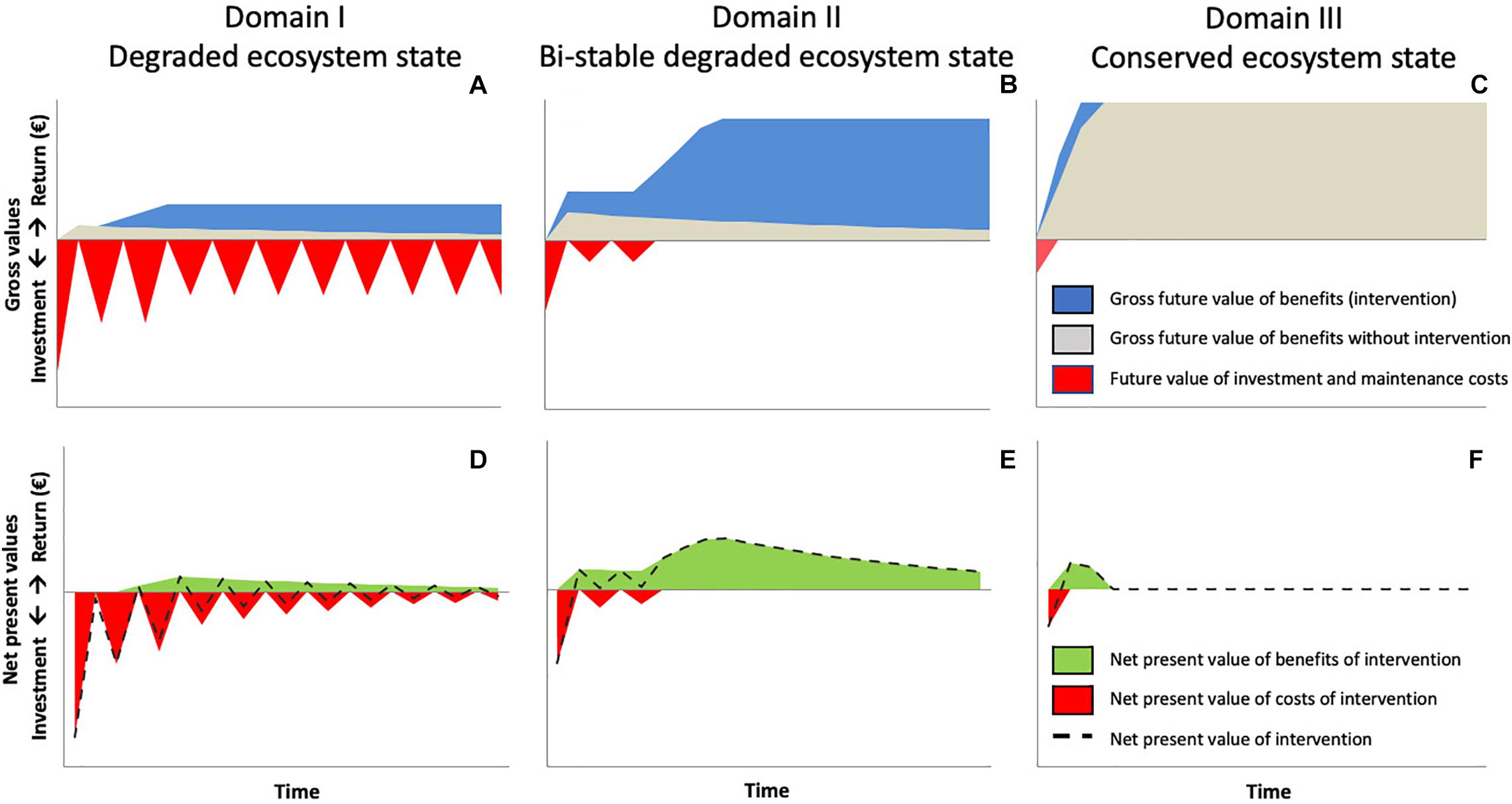
Figure 9. Cost-efficiency of management interventions dependent on stability domains and window of opportunity. Blue, gray, and red areas in panels (A–C) represent gross future values of the benefits of intervention, the benefits that would have occurred without intervention and costs of intervention, respectively. Gross future benefits depend on productivity levels, which vary between stability domains. In panels (D–F), the same investments of panels (A–C) are converted to discounted (present) value of investment costs and benefits. Dotted lines refer to net present value of intervention (i.e., subtracting from gross benefits the intervention costs and any benefits that would have been obtained without the intervention). The middle panel indicates management effects concurring with a window of opportunity. Note the declining level of initial investment costs and recurrent maintenance costs going from Domain I to Domain III. Investment in Domain I is economically unattractive, in Domain III it can be slightly attractive, while under a window of opportunity, investment in Domain II is economically very attractive. Source: Adapted from Sietz et al. (2017a).
The socio-ecological effectiveness of our management scenarios differed largely according to windows of opportunities and risks, starting conditions and investment and capital costs (e.g., for supplementary fodder and hired labor). For example, higher risk aversion and resting in wet years/extreme risk aversion scenarios (Table 3) implied a significant increase in the likelihood of maintaining vegetation cover above 40%, though only at restored sites. Yet, although the conservation scenario S2 effectively prevented degradation catastrophic shift, the economic loss was greater (−370 Euro/ha) than in S1 (−140 Euro/ha). This indicated that policy incentives such as subsidies would be useful to increase land users’ motivation to implement this type of management. In contrast, scenario S1 yielded higher economic returns but could not effectively prevent degradation, as stocking rates were insufficiently reduced.
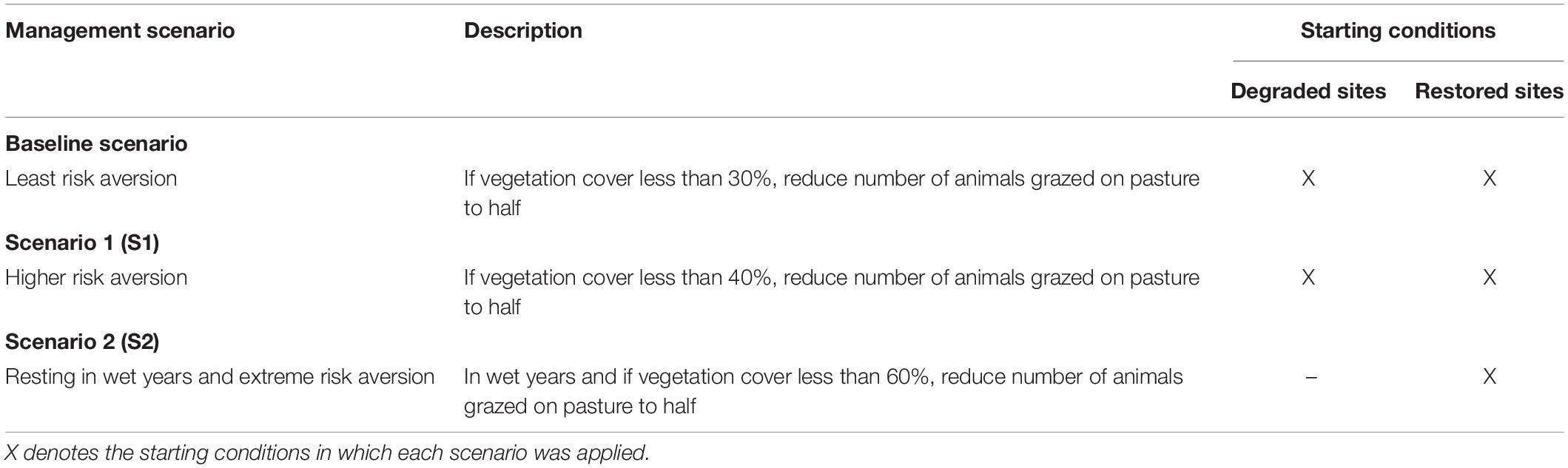
Table 3. Land management scenarios [adapted from Sietz et al. (2017c)].
The variety of promising measures within the study sites in this research and the information about their sustainability and resilience was used as the basis for guiding natural resource managers in improving the management of dryland ecosystems, in particular with regard to preventing pressures, stresses and disturbances, mitigating their negative impact and ensuring recovery. Although generalizing impacts of land management remains challenging as practices can be extremely diverse depending on the area, actors and timing (Schwilch et al., 2014; Marques et al., 2016), we worked with stakeholders to understand their responses to change and elaborate some key principles for the management of forest fires, overgrazing and land abandonment (see Schwilch et al., 2016). Each set of principles has a number of ecological grounding statements that draw on findings from questions 1 and 2, and related land management recommendations, each taking into account that ecosystems are also affected by the occurrence of droughts (noting the impact of the driver may vary depending on drought conditions).
The management of vulnerable ecosystems is a challenging task. It includes maintaining or enhancing the natural resource base as well as sustaining productivity. It requires the maintenance and restoration of vital ecosystem components that provide resilience to climate change, disasters and other threats and risks. In this way, catastrophic shifts might be prevented. Cost-effective management interventions, acceptable to stakeholders, should thus be applied before thresholds are reached, or when windows of opportunity occur. Findings from our research support other assessments that it is cheaper to prevent degradation than it is to reduce or reverse it (ELD Initiative and UNEP, 2015). By considering investment costs together with expected benefits and windows of opportunities and risks, our findings advance understanding of socio-ecological determinants of specific land use strategies, e.g., farm size, household characteristics and stakeholders’ perceptions (Sietz and Van Dijk, 2015) and the evaluation of threshold behavior (Suding and Hobbs, 2009). These insights can facilitate land-based management decisions aimed at addressing heterogeneity in global sustainability challenges such as loss of biosphere integrity, livelihood insecurity and vulnerability (Sietz, 2014; Steffen et al., 2015; Kok et al., 2016).
Overall, our study provided two key lessons for managing Mediterranean ecosystems in cost-effective and efficient ways. These are: (i) that there is a need for reliably predicting windows of opportunities and risks linked with Sustainable Land Management (SLM) and restoration advice tailored to land users’ needs and (ii) there is a need for managerial flexibility enabling continuous and rapid adaptation of management decisions according to emerging opportunities or risks. Interventions should include bundles of different practices used in combination to both mitigate the pressure and reduce vulnerability, and be supported by policy. Only if we manage to effectively avoid catastrophic ecosystem shifts, reduce land degradation and reverse the negative consequences of both, will we be able to contribute to a land degradation neutral world.
Discussion
Findings from this study addressing our three key research questions confirm that land degradation is occurring in various Mediterranean ecosystems. Observed changes in vegetation diversity, vegetation composition and soil quality suggest that a point may be reached where ecosystems are no longer able to provide services as they are doing now, or as they once could. Monitoring and modeling provided hints as to which changes in the plant–soil and plant–plant systems might occur during shifts. Some field experiments would benefit from observations over a greater number of seasons, but advances in modeling allowed us to examine the ecosystems, discover ways to avoid catastrophic shifts, to make ecosystems more resilient, and to educate end users in adapting to shifts that possibly have already taken place. Models effectively provided an additional time dimension to our analyses. An understanding of what happens during shifts can help define appropriate management strategies that can prevent the occurrence of shifts, or if they have already occurred, can assist in the recovery of the ecosystem. Predictions of the proximity of ecosystems to thresholds may be used by policymakers and land users to inform wider sustainable management of Mediterranean ecosystems, to prioritize areas for action, and inform the use of both proactive and reactive policy mechanisms to support land managers.
Taking the research findings as a whole provides far more substantive insights than is possible to ascertain from addressing any of our three research questions alone, and the participatory approach in which stakeholder engagement is central was vital in enabling the research to inform changes on the ground, underscoring best practices in the stakeholder engagement literature (Stringer and Dougill, 2013; Reed et al., 2014; de Vente et al., 2016). The learning and knowledge sharing process was considered particularly important by the project, especially where land users and land managers have dissenting positions, such was the case in Crete, where policy makers and shepherds were not able to attend the same workshops due to conflicting views (Sotirov et al., 2017). As shared goals are more feasible to be pursued, participatory approaches can be key in identifying common ground, connecting long and short-term ways of thinking through improving understanding. By taking a stakeholder engagement approach throughout, it was not possible only to share information, but also to share priorities, visions, and barriers. This was recognized as a tool to further design and elaborate outreach and management programs. It also opened new channels of communication between researchers and stakeholders. In a similar way, the multidisciplinary approach of the study supported learning across disciplines, with researchers from a variety of academic backgrounds working together to address the three broad research questions from different angles; each offering valuable insights through their approaches to help build a more complete understanding of catastrophic shifts and their management.
Overall, advanced tools for sustainable land management in drylands such as WOCAT (World Overview of Conservation Approaches and Technologies2) have been developed and promising practices can be up-scaled based on similarities between socio-ecological systems (Kok et al., 2016; Sietz et al., 2017b). Yet, tailoring measures to particular socio-environmental systems is necessary. Conditions vary within sites and over time (Schwilch et al., 2012), and the effectiveness of programs and measures depend on their capacity to address particular local and external issues affecting land degradation. However, stakeholders’ involvement in the research does not guarantee the identification of appropriate management measures (Schwilch et al., 2012) or the adoption of new technologies. There are various barriers to the adoption of new and innovative land management measures (Fleskens et al., 2014; Sietz and Van Dijk, 2015), and even when measures are adopted they can swiftly be dis-adopted (see e.g., Chinseu et al., 2019). Policy research and literature state that decision making is mainly driven by beliefs, values and experience, which in turn can interact with particular sets of goals and perceptions and resolve related issues and challenges (Hall, 1993; Sotirov et al., 2017). These can all affect the ways in which research is used, no matter how participatory the approach has been.
Conclusion
Overall, we have synthesized the findings from experiments, modeling and stakeholder engagement across six European study sites. While each component part yielded information that contributed toward answering our research questions, by taking the research as a multidisciplinary whole, and engaging stakeholders from the study sites, we were able to target our findings toward those who can use them. Our integrated study highlighted that there is still more work to be done in terms of gaining a complete picture of catastrophic shifts. However, by improving integrated understanding of: (i) the impacts of degradation drivers on ecosystem functioning and services, (ii) the processes that happen in the soil and plants during transition toward a catastrophic shift and (iii) how the management of vulnerable ecosystems can be optimized through derivation of key principles, bundles of approaches and assessments of windows of opportunity, we have put forward measures that can be utilized by stakeholders including land users and policymakers, to better manage change and avoid catastrophic shifts. Such on-the-ground efforts can, in turn, support progress toward the SDGs and global targets seeking to achieve Land Degradation Neutrality.
Data Availability Statement
Data used for writing this publication is available from the following DropBox repository: https://www.dropbox.com/sh/vza6mop3rlq3vei/AAAKVsTPWQiOzkS4cmH3z3qua?dl=00.
Ethics Statement
Our collaboration agreement identified that any participation of humans in the study would adhere to principles of informed consent and anonymity. Given the research was not collecting any personal information, the local legislation and institutional requirements in the locations in which data were collected did not regard any additional ethical approvals to be obtained. Participants were informed about how the data would be collected, who would have access, how long data would be stored and that it would be used in publications stemming from the work without any identifying information, in line with good research ethical practice. Informed consent was sought. Verbal consent was considered appropriate, taking into the cultural context of some of the sites, where requesting written consent would have made participants uncomfortable.
Author Contributions
PR and SB conceptualized the project. LS, RH, SK, SB, AM, MB, MR, AV, VV, NG, CB, LF, LH, PP, JK, GS, MJ, DS, GQ, IT, ID, PR, and EE designed the methodologies. SK, FS, SB, AM, MB, MR, AV, VV, LF, SV, JK, GS, MJ, DS, MC, GQ, RS, ID, and EE collected the data. SK, FS, SB, AM, MB, MR, AV, VV, LF, LH, NG, JB, SV, JK, GS, MJ, DS, MC, GQ, RS, ID, and EE analyzed the data. LS and EE led the manuscript writing. EE and RH led the project. All authors contributed critically to the drafts and final approval for publication.
Conflict of Interest
The authors declare that the research was conducted in the absence of any commercial or financial relationships that could be construed as a potential conflict of interest.
Funding
This research was conducted under the auspices of the CASCADE Project that is funded by the European Commission FP7 program, ENV.2011.2.1.4−2 – ‘Behavior of ecosystems, thresholds and tipping points,’ EU Grant agreement: 283068. This manuscript synthesizes our findings from across this project and in doing so draws heavily on our final report to the EU, which is archived at: https://library.wur.nl/WebQuery/wurpubs/fulltext/441608. SV and JK also thank FCT/MCTES for the financial support to CESAM (UIDP/50017/2020 + UIDB/50017/2020), through national funds. SB also thanks the Spanish Ministry of Science and Innovation for financial support (CGL2017-89804-R).
Footnotes
References
Andersen, T., Carstensen, J., Hernandez-Garcia, E., and Duarte, C. M. (2009). Ecological thresholds and regime shifts: approaches to identification. Trends Ecol. Evol. 24, 49–57. doi: 10.1016/j.tree.2008.07.014
Baeza, M., Valdecantos, A., Alloza, J., and Vallejo, V. (2007). Human disturbance and environmental factors as drivers of long-term post-fire regeneration patterns in Mediterranean forests. J. Veg. Sci. 18, 243–252. doi: 10.1111/j.1654-1103.2007.tb02535.x
Barkemeyer, R., Stringer, L. C., Hollins, J. A., and Josephi, F. (2015). Corporate reporting on solutions to wicked problems: Sustainable land management in the mining sector. Environ. Sci. Policy 48, 196–209. doi: 10.1016/j.envsci.2014.12.021
Baudena, M., Santana, V. M., Baeza, M. J., Bautista, S., Eppinga, M. B., Hemerik, L., et al. (2020). Increased aridity drives post-fire recovery of Mediterranean forests towards open shrublands. New Phytol. 225, 1500–1515. doi: 10.1111/nph.16252
Bautista, S., and Lamb, D. (2013). “Ecosystem services,” in Encyclopedia of Environmental Management, ed S. E. Jorgensen (New York, NY: Taylor & Francis Group). doi: 10.1081/E-Eem-120046612
Bautista, S., Llovet, J., Ocampo-Melgar, A., Vilagrosa, A., Mayor, A. G., Murias, C., et al. (2017). Integrating knowledge exchange and the assessment of dryland management alternatives - A learning-centered participatory approach. J. Environ. Manage. 195, 35–45. doi: 10.1016/j.jenvman.2016.11.050
Bautista, S., Urghege, A. M., Morcillo, L., López-Poma, R., Camacho, A., Turrión, D., et al. (2015). Report on the potential for sudden shifts. Cascade Project Deliverable 4.1.
Belsky, A. J. (1992). Effects of grazing, competition, disturbance and fire on species composition and diversity in grassland communities. J. Veg. Sci. 3, 187–200. doi: 10.2307/3235679
Berdugo, M., Delgado-Baquerizo, M., Soliveres, S., Hernández-Clemente, R., Zhao, Y., Gaitán, J. J., et al. (2020). Global ecosystem thresholds driven by aridity. Science 367, 787–790. doi: 10.1126/science.aay5958
Bestelmeyer, B. T. (2006). Threshold concepts and their use in rangeland management and restoration: the good, the bad, and the insidious. Restor. Ecol. 14, 325–329. doi: 10.1111/j.1526-100x.2006.00140.x
Bestelmeyer, B. T., Okin, G. S., Duniway, M. C., Archer, S. R., Sayre, N. F., Williamson, J. C., et al. (2015). Desertification, land use, and the transformation of global drylands. Front. Ecol. Environ. 13, 28–36. doi: 10.1890/140162
Bielsa, I., Pons, X., and Bunce, B. (2005). Agricultural Abandonment in the North Eastern Iberian Peninsula: the use of basic landscape metrics to support planning. J. Environ. Plan. Manage. 48, 85–102. doi: 10.1080/0964056042000308166
Biggs, R., Carpenter, S. R., and Brock, W. A. (2009). Turning back from the brink: detecting an impending regime shift in time to avert it. Proc. Natl. Acad. Sci. U.S.A. 106, 826–831. doi: 10.1073/pnas.0811729106
Boeschoten, M. J. C. (2013). The Impact of Land Degradation on Two Contrasting Dryland Ecosystems and the Ecosystem Services they Provide and Their Potentials for Restoration. Ph. D. thesis, University of Utrecht, Utrecht.
Brock, W. A., and Carpenter, S. R. (2012). Early warnings of regime shift when the ecosystem structure is unknown. PLoS One 7:e45586. doi: 10.1371/journal.pone.0045586
CASCADE (2017). Catastrophic Shifts in Drylands: How Can we Prevent Ecosystem Degradation? Final Publishable Summary. Available online at: https://library.wur.nl/WebQuery/wurpubs/fulltext/441608 (accessed September 23, 2020).
Chinseu, E., Dougill, A., and Stringer, L. (2019). Why do smallholder farmers dis-adopt conservation agriculture? Insights from Malawi. Land Degrad. Dev. 30, 533–543. doi: 10.1002/ldr.3190
Crawley, M. J. (1983). Herbivory: The Dynamics of Animal–Plant Interactions. Oxford: Blackwell Scientific Publications.
Daliakopoulos, I. N., Panagea, I. S., Tsanis, I. K., Grillakis, M. G., Koutroulis, A. G., Hessel, R., et al. (2017). Yield response of Mediterranean rangelands under a changing climate. Land Degrad. Dev. 28, 1962–1972. doi: 10.1002/ldr.2717
Daliakopoulos, I. N., and Tsanis, I. K. (2013). Historical Evolution of Dryland Ecosystems. Cascade Project Deliverable 2.1. Available online at: https://www.cascadis-project.eu/study-sites/26-varzea-portugal (accessed September 23, 2020).
Daliakopoulos, I. N., and Tsanis, I. K. (2014). Climate-induced catastrophic shifts in pastoralism systems managed under the maximum sustainable yield model. Oper. Res. 14, 177–188. doi: 10.1007/s12351-014-0156-7
De Ita, C., Stringer, L., Fleskens, L., and Sietz, D. (2017). Report on Multi-Scale Evaluation of Cascade’s Management Principles and Grazing Model Scenarios with Stakeholders and Policy Makers. Cascade Project Deliverable 8.3. Available online at: https://www.cascadis-project.eu/multi-scale-evaluation/157-references (accessed September 23, 2020).
de Vente, J., Reed, M., Stringer, L., Valente, S., and Newig, J. (2016). How does the context and design of participatory decision making processes affect their outcomes? Evidence from sustainable land management in global drylands. Ecol. Soc. 21. doi: 10.5751/ES-08053-210224
Demenocal, P., Ortiz, J., Guilderson, T., Adkins, J., Sarnthein, M., Baker, L., et al. (2000). Abrupt onset and termination of the African Humid Period:: rapid climate responses to gradual insolation forcing. Quat. Sci. Rev. 19, 347–361. doi: 10.1016/S0277-3791(99)00081-5
Duarte, F., Jones, N., and Fleskens, L. (2008). Traditional olive orchards on sloping land: sustainability or abandonment? J. Environ. Manage. 89, 86–98. doi: 10.1016/j.jenvman.2007.05.024
ELD Initiative and UNEP (2015). The Economics of Land Degradation in Africa: Benefits of Action Outweigh the Costs. Available online at: www.eld-initiative.org
Fleskens, L., Nainggolan, D., and Stringer, L. (2014). An exploration of scenarios to support sustainable land management using integrated environmental socio-economic models. Environ. Manage. 54, 1005–1021. doi: 10.1007/s00267-013-0202-x
Fraser, E. D. G., and Stringer, L. C. (2009). Explaining agricultural collapse: macro-forces, micro-crises and the emergence of land use vulnerability in southern Romania. Glob. Environ. Change 19, 45–53. doi: 10.1016/j.gloenvcha.2008.11.001
Génin, A., Majumder, S., Sankaran, S., Schneider, F. D., Danet, A., Berdugo, M., et al. (2018a). Spatially heterogeneous stressors can alter the performance of indicators of regime shifts. Ecol. Indic. 94, 520–533. doi: 10.1016/j.ecolind.2017.10.071
Génin, A., S., Majumder, S., Sankaran, A., Danet, V., Guttal, F. D., Schneider, S., et al. (2018b). Monitoring ecosystem degradation using spatial data and the R package spatial warnings. Methods Ecol. Evol. 9, 2067–2075. doi: 10.1111/2041-210X.13058
Guttal, V., and Jayaprakash, C. (2009). Spatial variance and spatial skewness: leading indicators of regime shifts in spatial ecological systems. Theor. Ecol. 2, 3–12. doi: 10.1007/s12080-008-0033-1
Hall, P. A. (1993). Policy paradigms, social learning, and the state: the case of economic policymaking in Britain. Comp. Pol. 275–296. doi: 10.2307/422246
Holmgren, M., and Scheffer, M. (2001). El Niño as a window of opportunity for the restoration of degraded arid ecosystems. Ecosystems 4, 151–159. doi: 10.1007/s100210000065
Holocheck, J. I., Pieper, R. D., and And Herbel, C. H. (1989). Range management principles and practices. Englewood Cliffs, NJ: Prentice-Hall.
Hosseini, M., Geissen, V., Gonzalez Pelayo, O., Serpa, D., Machado, A. I., Ritsema, C., et al. (2017a). Effects of fire occurrence and recurrence on nitrogen and phosphorus losses by overland flow in maritime pine plantations in north-central Portugal. Geoderma 289, 97–106. doi: 10.1016/j.geoderma.2016.11.033
Hosseini, M., Gonzalez Pelayo, O., Vasques, A. R., Ritsema, C., Geissen, V., and Keizer, J. J. (2017b). The short-term effectiveness of surfactant seed coating and mulching treatment in reducing post-fire runoff and erosion. Geoderma 307, 231–237. doi: 10.1016/j.geoderma.2017.08.008
Hosseini, M., Keizer, J. J., Gonzalez Pelayo, O., Prats, S. A., Ritsema, C., and Geissen, V. (2016). Effect of fire frequency on runoff, soil erosion, and loss of organic matter at the micro-plot scale in north-central Portugal. Geoderma 269, 126–137. doi: 10.1016/j.geoderma.2016.02.004
Hurni, H. (2000). Assessing sustainable land management (Slm). Agric. Ecosyst. Environ. 81, 83–92. doi: 10.1016/S0167-8809(00)00182-1
Jeltsch, F., Turnbull, L., Scarsoglio, S., Alados, C. L., Gallart, F., Mueller, E. N., et al. (2014). “Resilience, Self-Organization, Complexity and Pattern Formation,” in Patterns of Land Degradation in Drylands, eds E. Mueller, J. Wainwright, A. Parsons, and L. Turnbull (Dordrecht: Springer). doi: 10.1007/978-94-007-5727-1_3
Jeltsch, F., Weber, G. E., and Grimm, V. (2000). Ecological buffering mechanisms in savannas: a unifying theory of long-term tree-grass coexistence. Plant Ecol. 150, 161–171. doi: 10.1023/A:1026590806682
Jucker Riva, M., Baeza, J., Bautista, S., Christoforou, M., Daliakopoulos, I. N., Hadjimitsis, D., et al. (2018). How does land management contribute to the resilience of Mediterranean forests and rangelands? A participatory assessment. Land Degrad. Dev. 29, 3721–3735. doi: 10.1002/ldr.3104
Jucker Riva, M., Daliakopoulos, I. N., Eckert, S., Hodel, E., and Liniger, H. (2017). Assessment of land degradation in Mediterranean forests and grazing lands using a landscape unit approach and the normalized difference vegetation index. Appl. Geogr. 86, 8–21. doi: 10.1016/j.apgeog.2017.06.017
Jucker Riva, M., Liniger, H., Valdecantos, A., and Schwilch, G. (2016). Impacts of land management on the resilience of Mediterranean dry forests to fire. Sustainability 8:981. doi: 10.3390/su8100981
Kéfi, S., Guttal, V., Brock, W. A., Carpenter, S. R., Ellison, A. M., Livina, V., et al. (2014). Early Warning signals of ecological transitions: methods for spatial patterns. PLoS One 9:2097. doi: 10.1371/journal.pone.0092097
Kéfi, S., Rietkerk, M., Alados, C. L., Pueyo, Y., Papanastasis, V. P., Elaich, A., et al. (2007). Spatial vegetation patterns and imminent desertification in Mediterranean arid ecosystems. Nature 449, 213–217. doi: 10.1038/nature06111
Kéfi, S., Rietkerk, M., Roy, M., Franc, A., de Ruiter, P. C., and Pascual M. (2011). Robust scaling in ecosystems and the meltdown of patch size distributions before extinction. Ecol. Lett. 14, 29–35.
Kok, M., Lüdeke, M., Lucas, P., Sterzel, T., Walther, C., Janssen, P., et al. (2016). A new method for analysing socio-ecological patterns of vulnerability. Reg. Environ. Change 16, 229–243. doi: 10.1007/s10113-014-0746-1
Kuiper, J. J., Van Altena, C., De Ruiter, P. C., Van Gerven, L. P., Janse, J. H., and Mooij, W. M. (2015). Food-web stability signals critical transitions in temperate shallow lakes. Nat. Commun. 6:7727. doi: 10.1038/ncomms8727
Lategan, F. S. (1995). Range ecology at disequilibrium: new models of natural variability and pastoral adaptation in African savannas, edited by RH Behnke Jr, I Scoones & C Kerven. Dev. South. Afr. 12, 145–146. doi: 10.1080/03768359508439798
Lees, K., Pitois, S., Scott, C., Frid, C., and Mackinson, S. (2006). Characterizing regime shifts in the marine environment. Fish Fish. 7, 104–127. doi: 10.1111/j.1467-2979.2006.00215.x
López, D. R., Brizuela, M. A., Willems, P., Aguiar, M. R., Siffredi, G., and Bran, D. (2013). Linking ecosystem resistance, resilience, and stability in steppes of North Patagonia. Ecol. Indic. 24, 1–11. doi: 10.1016/j.ecolind.2012.05.014
López-Poma, R., and Bautista, S. (2014). Plant regeneration functional groups modulate the response to fire of soil enzyme activities in a Mediterranean shrubland. Soil Biol. Biochem. 79, 5–13. doi: 10.1016/j.soilbio.2014.08.016
Maestre, F. T., and Cortina, J. (2004). Insights into ecosystem composition and function in a sequence of degraded semiarid steppes. Restor. Ecol. 12, 494–502. doi: /10.1111/j.1061-2971.2004.03106.x
Maestre, F. T., Eldridge, D. J., Soliveres, S., Kéfi, S., Delgado-Baquerizo, M., Bowker, M. A., et al. (2016). Structure and functioning of dryland ecosystems in a changing world. Annu. Rev. Ecol. Evol. Syst. 47, 215–237. doi: 10.1146/annurev-ecolsys-121415-032311
Magnuszewski, P., Ostasiewicz, K., Chazdon, R., Salk, C., Pajak, M., Sendzimir, J., et al. (2015). Resilience and alternative stable states of tropical forest landscapes under shifting cultivation regimes. PLoS One 10:e0137497. doi: 10.1371/journal.pone.0137497
Maia, P., Keizer, J., Vasques, A., Abrantes, N., Roxo, L., Fernandes, P., et al. (2014). Post-fire plant diversity and abundance in pine and eucalypt stands in Portugal: effects of biogeography, topography, forest type and post-fire management. For. Ecol. Manage. 334, 154–162. doi: 10.1016/j.foreco.2014.08.030
Maia, P., Pausas, J., Vasques, A., and Keizer, J. J. (2012). Fire severity as a key factor in post-fire regeneration of Pinus pinaster (Ait.) in Central Portugal. Ann. For. Sci. 69, 489–498. doi: 10.1007/s13595-012-0203-6
Marques, M. J., Schwilch, G., Lauterburg, N., Crittenden, S., Tesfai, M., Stolte, J., et al. (2016). Multifaceted impacts of sustainable land management in drylands: a review. Sustainability 8:177. doi: 10.3390/su8020177
Mayor, A. G., Bautista, S., Rodriguez, F., and Kéfi, S. (2019). Connectivity-mediated ecohydrological feedbacks and regime shifts in drylands. Ecosystems 22, 1497–1511. doi: 10.1007/s10021-019-00366-w
Mayor, A. G., Valdecantos, A., Vallejo, V., Keizer, J., Bloem, J., Baeza, J., et al. (2016). Fire-induced pine woodland to shrubland transitions in Southern Europe may promote shifts in soil fertility. Sci. Total Environ. 573, 1232–1241. doi: 10.1016/j.scitotenv.2016.03.243
Mayor, A. G., Vallejo, R., Bautista, S., Ruiter, P., Hemerik, L., Geissen, V., et al. (2017). Identification of critical changes preceding catastrophic shifts: ecosystems affected by increasing grazing intensity and severe drought. Cascade Project Deliverable 3.1b. Available online at: https://www.dropbox.com/s/mujsupwinuurzxh/D3.1b%20-%20REPORT%2021%20-%20WB3%20-%20Identification%20of%20critical%20changes%20preceding%20shifts~%20-%20Del.3.1b%20-%20FULL.pdf?dl=0 (accessed September 23, 2020).
Mayor, A. G., Vallejo, R., Bautista, S., Ruiter, P. D., Hemerik, L., Geissen, V., et al. (2015). Identification of critical changes preceding catastrophic shifts: ecosystems affected by increasing wildfire recurrence. Cascade Project Deliverable 3.1a. Available online at: https://www.dropbox.com/s/jcxm8u5rx1jcil5/D3.1a%20-%20REPORT%2010%20-%20WB3%20-%20Identification%20of%20critical%20changes%20preceding%20shifts~%20-%20Del.3.1a%20-%20FULL.pdf?dl=0 (accessed September 23, 2020).
Mayor, Á. G., and Bautista, S. (2012). Multi-scale evaluation of soil functional indicators for the assessment of water and soil retention in Mediterranean semiarid landscapes. Ecol. Indic. 20, 332–336. doi: 10.1016/j.ecolind.2012.03.003
Mayor, Á. G., Bautista, S., Small, E. E., Dixon, M., and Bellot, J. (2008). Measurement of the connectivity of runoff source areas as determined by vegetation pattern and topography: a tool for assessing potential water and soil losses in drylands. Water Resour. Res. 44:W10423. doi: 10.1029/2007WR006367
Mayor, Á. G., Kéfi, S., Bautista, S., Rodríguez, F., Cartení, F., and Rietkerk, M. (2013). Feedbacks between vegetation pattern and resource loss dramatically decrease ecosystem resilience and restoration potential in a simple dryland model. Landsc. Ecol. 28, 931–942. doi: 10.1007/s10980-013-9870-4
Müller, B., Frank, K., and Wissel, C. (2007). Relevance of rest periods in non-equilibrium rangeland systems–a modelling analysis. Agric. Syst. 92, 295–317. doi: 10.1016/j.agsy.2006.03.010
Müller, D., Sun, Z., Vongvisouk, T., Pflugmacher, D., Xu, J., and Mertz, O. (2014). Regime shifts limit the predictability of land-system change. Glob. Environ. Change 28, 75–83. doi: 10.1016/j.gloenvcha.2014.06.003
Novara, A., Gristina, L., Sala, G., Galati, A., Crescimanno, M., Cerdà, A., et al. (2017). Agricultural land abandonment in Mediterranean environment provides ecosystem services via soil carbon sequestration. Sci. Total Environ. 576, 420–429. doi: 10.1016/j.scitotenv.2016.10.123
Oesterheld, M., and McNaughton, S. (1991). Effect of stress and time for recovery on the amount of compensatory growth after grazing. Oecologia 85, 305–313. doi: 10.1007/BF00320604
Papanastasis, V. P., Bautista, S., Chouvardas, D., Mantzanas, K., Papadimitriou, M., Mayor, A. G., et al. (2015). Comparative assessment of goods and services provided by grazing regulation and reforestation in degraded Mediterranean rangelands. Land Degrad. Dev. 28, 1178–1187. doi: 10.1002/ldr.2368
Pausas, J., Ouadah, N., Ferran, A., Gimeno, T., and Vallejo, R. (2002). Fire severity and seedling establishment in Pinus halepensis woodlands, eastern Iberian Peninsula. Plant Ecol. 169, 205–213. doi: /10.1023/A:1026019528443
Pausas, J., Ribeiro, E., and Vallejo, R. (2004). Post-fire regeneration variability of Pinus halepensis in the eastern Iberian Peninsula. For. Ecol. Manage. 203, 251–259. doi: 10.1016/j.foreco.2004.07.061
Perevolotsky, A. (1995). What determines grazing preference for Mediterranean woody species. Options Mediterr. 12, 221–224.
Perevolotsky, A., and Seligman, N. A. G. (1998). Role of grazing in Mediterranean rangeland ecosystems. Bioscience 48, 1007–1017. doi: 10.2307/1313457
Quaas, M. F., Baumgärtner, S., Becker, C., Frank, K., and Müller, B. (2007). Uncertainty and sustainability in the management of rangelands. Ecol. Econ. 62, 251–266. doi: 10.1016/j.ecolecon.2006.03.028
Reed, M. S., Stringer, L., Fazey, I., Evely, A., and Kruijsen, J. (2014). Five principles for the practice of knowledge exchange in environmental management. J. Environ. Manage. 146, 337–345. doi: 10.1016/j.jenvman.2014.07.021
Reynolds, J. F., Smith, D. M., Lambin, E. F., Turner, B. L. II, Mortimore, M., Batterbury, S. P., et al. (2007). Global desertification: building a science for dryland development. Science 316, 847–851. doi: 10.1126/science.1131634
Rietkerk, M., Dekker, S. C., De Ruiter, P. C., and Van De Koppel, J. (2004). Self-organized patchiness and catastrophic shifts in ecosystems. Science 305, 1926–1929. doi: 10.1126/science.1101867
Rietkerk, M., van den Bosch, F., and van de Koppel J. (1997). Site-specific properties and irreversible vegetation changes in semi-arid grazing systems. Oikos 80, 241–252. doi: 10.2307/3546592
Rocca, M. E., Brown, P. M., Macdonald, L. H., and Carrico, C. M. (2014). Climate change impacts on fire regimes and key ecosystem services in Rocky Mountain forests. For. Ecol. Manage. 327, 290–305. doi: 10.1016/j.foreco.2014.04.005
Rocha, J. C., Peterson, G., Bodin, Ö., and Levin, S. (2018). Cascading regime shifts within and across scales. Science 362, 1379–1383. doi: 10.1126/science.aat7850
Rodríguez, F., Mayor, Á. G., Rietkerk, M., and Bautista, S. (2018). A null model for assessing the cover-independent role of bare soil connectivity as indicator of dryland functioning and dynamics. Ecol. Indic. 94, 512–519. doi: 10.1016/j.ecolind.2017.10.023
Rojo, L., Bautista, S., Orr, B. J., Vallejo, R., Cortina, J., and Derak, M. (2012). Prevention and restoration actions to combat desertification. An integrated assessment: the Practice Project. Sécheresse 23, 219–226. doi: 10.1684/sec.2012.0351
Santana, V. M., González-Pelayo, O., Maia, P. A. A., Varela, M. E. T., Valdecantos, A., Vallejo, V. R., et al. (2016). Effects of fire recurrence and different salvage logging techniques on carbon storage in Pinus pinaster forests from northern Portugal. Eur. J. For. Res. 135, 1107–1117. doi: 10.1007/s10342-016-0997-0
Scheffer, M., Carpenter, S., Foley, J. A., Folke, C., and Walker, B. (2001). Catastrophic shifts in ecosystems. Nature 413, 591–596. doi: 10.1038/35098000
Scheffer, M., and Carpenter, S. R. (2003). Catastrophic regime shifts in ecosystems: linking theory to observation. Trends Ecol. Evol. 18, 648–656. doi: 10.1016/j.tree.2003.09.002
Scheffer, M., Hosper, S., Meijer, M., Moss, B., and Jeppesen, E. (1993). Alternative equilibria in shallow lakes. Trends Ecol. Evol. 8, 275–279. doi: 10.1016/0169-5347(93)90254-M
Schneider, F. D., and Kéfi, S. (2016). Spatially heterogeneous pressure raises risk of catastrophic shifts. Theor. Ecol. 9, 207–217. doi: 10.1007/s12080-015-0289-1
Schwilch, G., Bachmann, F., and De Graaff, J. (2012). Decision support for selecting Slm technologies with stakeholders. Appl. Geogr. 34, 86–98. doi: 10.1016/j.apgeog.2011.11.002
Schwilch, G., Jucker Riva, M., and Liniger, H. (2016). Comprehensive Guidelines for Natural Resource Managers. Cascade Project Deliverable 7.3. Available online at: https://www.cascadis-project.eu/documents/category/12-better-management?start=20 (accessed September 23, 2020).
Schwilch, G., Liniger, H. P., and Hurni, H. (2014). Sustainable Land Management (Slm) Practices in Drylands: How Do They Address Desertification Threats? Environ. Manage. 54, 983–1004. doi: 10.1007/s00267-013-0071-3
Sietz, D. (2014). Regionalisation of global insights into dryland vulnerability: better reflecting smallholders’ vulnerability in Northeast Brazil. Glob. Environ. Change 25, 173–185. doi: 10.1016/j.gloenvcha.2014.01.010
Sietz, D., Fleskens, L., and Stringer, L. C. (2017a). Learning from non-linear ecosystem dynamics is vital for achieving land degradation neutrality. Land Degrad. Dev. 28, 2308–2314. doi: 10.1002/ldr.2732
Sietz, D., Ordoñez, J. C., Kok, M. T. J., Janssen, P., Hilderink, H. B. M., Tittonell, P., et al. (2017b). Nested archetypes of vulnerability in African drylands: where lies potential for sustainable agricultural intensification? Environ. Res. Lett. 12:095006. doi: 10.1088/1748-9326/aa768b
Sietz, D., Fleskens, L., and Stringer, L. (2017c). Report on Integrated Modelling Strategy. CASCADE Project Deliverable 8.2. Available online at: https://www.dropbox.com/sh/vza6mop3rlq3vei/AAAKVsTPWQiOzkS4cmH3z3qua?dl=00 (accessed September 23, 2020).
Sietz, D., and Van Dijk, H. (2015). Land-based adaptation to global change: What drives soil and water conservation in western Africa? Glob. Environ. Change 33, 131–141. doi: 10.1016/j.gloenvcha.2015.05.001
Siteur, K., Siero, E., Eppinga, M. B., Rademacher, J. D. M., Doelman, A., and Rietkerk, M. (2014). Beyond Turing: the response of patterned ecosystems to environmental change. Ecol. Complex. 20, 81–96. doi: 10.1016/j.ecocom.2014.09.002
Sluiter, R., and de Jong, S. M. (2007). Spatial patterns of Mediterranean land abandonment and related land cover transitions. Landsc. Ecol. 22, 559–576. doi: 10.1007/s10980-006-9049-3
Sotirov, M., Blum, M., Storch, S., Selter, A., and Schraml, U. (2017). Do forest policy actors learn through forward-thinking? Conflict and cooperation relating to the past, present and futures of sustainable forest management in Germany. For. Policy Econ. 85, 256–268. doi: 10.1016/j.forpol.2016.11.011
Staal, A., Dekker, S. C., Hirota, M., and Van Nes, E. H. (2015). Synergistic effects of drought and deforestation on the resilience of the south-eastern Amazon rainforest. Ecol. Complex. 22, 65–75. doi: 10.1016/j.ecocom.2015.01.003
Steffen, W., Richardson, K., Rockström, J., Cornell, S. E., Fetzer, I., Bennett, E. M., et al. (2015). Planetary boundaries: guiding human development on a changing planet. Science 347:1259855. doi: 10.1126/science.1259855
Stephens, S., Agee, J. K., Fulé, P., North, M., Romme, W., Swetnam, T., et al. (2013). Managing forests and fire in changing climates. Science 342, 41–42. doi: 10.1126/science.1240294
Stringer, L. C., and Dougill, A. (2013). Channelling science into policy: enabling best practices from research on land degradation and sustainable land management in dryland Africa. J. Environ. Manage. 114, 328–335. doi: 10.1016/j.jenvman.2012.10.025
Stringer, L. C., Reed, M. S., Fleskens, L., Thomas, R. J., Le, Q. B., and Lala-Pritchard, T. (2017). A New Dryland Development Paradigm Grounded in Empirical Analysis of Dryland Systems Science. Land Degrad. Dev. 28, 1952–1961. doi: 10.1002/ldr.2716
Suding, K. N., and Hobbs, R. J. (2009). Threshold models in restoration and conservation: a developing framework. Trends Ecol. Evol. 24, 271–279. doi: 10.1016/j.tree.2008.11.012
Swindles, G. T., Morris, P. J., Whitney, B., Galloway, J. M., Galka, M., Gallego-Sala, A., et al. (2018). Ecosystem state shifts during long-term development of an Amazonian peatland. Glob. Change Biol. 24, 738–757. doi: 10.1111/gcb.13950
Tapias, R., Climent, J., Pardos, J. A., and Gil, L. (2004). Life histories of Mediterranean pines. Plant Ecol. 171, 53–68. doi: 10.1023/B:VEGE.0000029383.72609.f0
Tongway, D., and Hindley, N. (2005). Landscape Function Analysis Manual: Procedures For Monitoring And Assessing Landscapes. Canberra: CSIRO Sustainable Ecosystems.
Tsanis, I. K., and Daliakopoulos, I. N. (2014). Drivers of Change in the Study Sites. Cascade Project Deliverable 2.2. Cascade Report 06. Available online at: https://www.cascadis-project.eu/randi-forest-cyprus/66-drivers-of-change (accessed September 23, 2020).
Urgeghe, A. M., and Bautista, S. (2015). Size and connectivity of upslope runoff-source areas modulate the performance of woody plants in Mediterranean drylands. Ecohydrology 8, 1292–1303. doi: 10.1002/eco.1582
Valdecantos, A., and Vallejo, R. (2015). Report on Structural and Functional Changes Associated to Regime Shifts in Mediterranean Dryland Ecosystems. Cascade Project Deliverable 5.1. Available online at: https://www.dropbox.com/s/yp56ft5j34q593e/D5.1%20-%20REPORT%2011%20-%20WB5%20-%20Report%20on%20structural%20and%20functional%20changes%20associated%20to%20regime%20shifts%20in%20Mediterranean%20dryland%20ecosystems%20-%20Del.5.1%20-%20FULL.pdf?dl=0 (accessed September 23, 2020).
Valdecantos, A., Vallejo, R., Fuentes, D., and Geeson, N. (2016). Fire Recurrence in E Spain Forests: Natural Dynamics and Restoration Approach. A Cascade Project Case Study in the Ayora, Spain. Cascade Project Booklet. Available online at: https://www.cascadis-project.eu/phocadownload/CASCADE_factsheet_fire_recurrence.pdf
Verwijmeren, M. (2016). Interspecific Facilitation and Critical Transitions in Arid Ecosystems. Ph.D. thesis, Utrecht University, Utrecht.
Verwijmeren, M., Rietkerk, M., Bautista, S., Mayor, A. G., Wassen, M. J., and Smit, C. (2014). Drought and grazing combined: contrasting shifts in plant interactions at species pair and community level. J. Arid Environ. 111, 53–60. doi: 10.1016/j.jaridenv.2014.08.001
Walker, B., Meyers, J., and Society. (2004). Thresholds in ecological and social–ecological systems: a developing database. Ecol. Soc. 9:3. doi: 10.5751/ES-00664-090203
Wang, C., Wang, X., Liu, D., Wu, H., Lü, X., Fang, Y., et al. (2014). Aridity threshold in controlling ecosystem nitrogen cycling in arid and semi-arid grasslands. Nat. Commun. 5, 1–8.
Westoby, M., Walker, B., and Noy-Meir, I. (1989). Opportunistic management for rangelands not at equilibrium. J. Range Manage. 266–274. doi: 10.2307/3899492
Keywords: dryland ecosystems, ecosystem restoration, multidisciplinary, resilience, stakeholder engagement recommendations
Citation: van den Elsen E, Stringer LC, De Ita C, Hessel R, Kéfi S, Schneider FD, Bautista S, Mayor AG, Baudena M, Rietkerk M, Valdecantos A, Vallejo VR, Geeson N, Brandt CJ, Fleskens L, Hemerik L, Panagos P, Valente S, Keizer JJ, Schwilch G, Jucker Riva M, Sietz D, Christoforou M, Hadjimitsis DG, Papoutsa C, Quaranta G, Salvia R, Tsanis IK, Daliakopoulos I, Claringbould H and de Ruiter PC (2020) Advances in Understanding and Managing Catastrophic Ecosystem Shifts in Mediterranean Ecosystems. Front. Ecol. Evol. 8:561101. doi: 10.3389/fevo.2020.561101
Received: 11 May 2020; Accepted: 07 September 2020;
Published: 22 October 2020.
Edited by:
Nadia S. Santini, National Autonomous University of Mexico, MexicoReviewed by:
Carlos Martorell, Universidad Nacional Autónoma de México, MexicoAlice Nunes, University of Lisbon, Portugal
Copyright © 2020 van den Elsen, Stringer, De Ita, Hessel, Kéfi, Schneider, Bautista, Mayor, Baudena, Rietkerk, Valdecantos, Vallejo, Geeson, Brandt, Fleskens, Hemerik, Panagos, Valente, Keizer, Schwilch, Jucker Riva, Sietz, Christoforou, Hadjimitsis, Papoutsa, Quaranta, Salvia, Tsanis, Daliakopoulos, Claringbould and de Ruiter. This is an open-access article distributed under the terms of the Creative Commons Attribution License (CC BY). The use, distribution or reproduction in other forums is permitted, provided the original author(s) and the copyright owner(s) are credited and that the original publication in this journal is cited, in accordance with accepted academic practice. No use, distribution or reproduction is permitted which does not comply with these terms.
*Correspondence: Lindsay C. Stringer, bGluZHNheS5zdHJpbmdlckB5b3JrLmFjLnVr