- Univ Lyon, Université Claude Bernard Lyon 1, CNRS, ENTPE, UMR 5023 LEHNA, Villeurbanne, France
Almost all animal species are engaged in predator-prey interactions. These interactions, variable in time and space, favor the emergence and evolution of phenotypic plasticity, which allows prey to fine-tune their phenotype to the current risk of predation. A famous example is the induction of defensive neck-teeth, spines or helmets in some water fleas when they detect cues of predator presence. In general, the response may involve different types of traits (behavioral, morphological, physiological, and life-history traits), alone or in combination. The induced traits may be adaptive anti-predator defenses or reflect more general stress-based responses. Recently, it has been found that predator-induced plasticity occurs not only within but also across generations (transgenerational plasticity), i.e., the phenotype of a generation is influenced by the detection of predator-cues in previous generation(s), even if the current generation is not itself exposed to these cues. In this paper, we aim to review this accumulating literature and propose a current state of key aspects of predator-induced transgenerational plasticity in metazoans. In particular, we review whether patterns of predator-induced transgenerational plasticity depend on the type of traits. We analyze the adaptive value of predator-induced transgenerational plasticity and explore the evidence for its evolution and underlying mechanisms. We also consider its temporal dynamics: what are the time windows during which predator-cues must be detected to be transmitted across generations? Are transgenerational responses in offspring stage-dependent? How many generations does transgenerational plasticity persist? Finally, we discuss other factors highlighted in the literature that influence predator-induced transgenerational plasticity: what are the relative contributions of maternal and paternal exposure to predator-cues in generating transgenerational plasticity? Do transgenerational responses depend on offspring sex? Do they scale with the perceived level of predation risk? This review shows that we are only at the beginning of understanding the processes of predator-induced transgenerational plasticity, and it encourages future research to fill the lack of knowledge highlighted here.
Introduction
Research on predator-prey interactions and phenotypic plasticity has been intimately linked for a long time, merging ecological and evolutionary considerations. At the beginning of the 20th century, the mutation theory of de Vries and MacDougal (1905) was predominant, stating that organismal characters change because of relatively rare and heritable changes in the genetic material. Stimulated by the short-comings of the mutation theory, Richard Woltereck was the first to conceptualize that the phenotype is also influenced by the environment (Woltereck, 1909). Using Daphnia water fleas as a model system, he experimentally demonstrated that morphological shape (the relative size of the head) varied in relation to different nutrient levels (Nicoglou, 2018). He drew “phenotypic curves” to describe the phenomenon and coined the term reaction norm (“Reaktionsnorm”). Using experimental approaches on a model that is easy and cheap to raise, he thus paved the way for future studies on phenotypic plasticity—now generally defined as the ability of a given genotype to express alternative phenotypes under different environmental conditions (Pigliucci, 2005; namely within-generational plasticity, WGP hereafter). Particularly, his findings inspired studies on plastic responses in predator-prey systems, of which morphological change of Daphnia in presence of predators is the most famous example (Riessen, 1999; Tollrian and Harvell, 1999). A rich literature [starting with Gilbert’s (1966) study on rotifers and Jacobs (1967) on daphnids] has demonstrated predator-induced defenses in prey (e.g., mammals: Hunter and Skinner, 1998; amphibians: Van Buskirk, 2002; insects: Li and Lee, 2004; birds: Eggers et al., 2006; mollusks: Auld and Relyea, 2011; actinopterygii: Torres-Dowdall et al., 2012; reviewed by Riessen, 1999; Tollrian and Harvell, 1999) as well as, to a lesser degree, prey-induced offenses in predators (e.g., Padilla, 2001; Kopp and Tollrian, 2003; Kishida et al., 2010). Theory suggests that the evolution of phenotypic plasticity is favored by the highly variable nature of predator-prey interactions in both time and space (e.g., Lima, 1998; Sih et al., 2000; Svanbäck et al., 2009). In this review, we will focus on predator-induced plasticity in prey, so-called inducible defenses, which remains the most common case of phenotypic plasticity in predator-prey systems (Tollrian and Harvell, 1999). These anti-predator responses are triggered when prey detect specific (mechanical, visual, auditory, chemical) cues of predator presence in their environment—chemical cues being the most common (predator-specific odor, dietary cues or alarm cues from injured prey; Mitchell et al., 2017). The response to predator-cues can involve different types of traits (behavioral, morphological, physiological and life-history traits), alone or in combination. These traits can confer defensive value or reflect stress-based responses. The optimal defense strategies depend on the relative speed between the expression of traits and both the onset and duration of risk (Steiner and Pfeiffer, 2007; Mitchell et al., 2017).
Ninety years after the presentation of R. Woltereck at the German Society of Zoology, and after a rich literature on inducible defenses in water fleas had accumulated, Agrawal et al. (1999) showed that Daphnia cucullata exposed to cues from dipteran larvae not only express morphological defenses, but also produce offspring that are better defended than those from unthreatened parents. This study pioneered the general idea that phenotypic plasticity can occur across generations and that such transgenerational plasticity (hereafter TGP) may play an important role in predator-prey interactions (Agrawal et al., 1999). Since then, a growing number of studies has examined the ecological and evolutionary importance of TGP both in general (Figure 1A) and in the context of predator-prey interactions (Figure 1B). In the present review, we aim to propose a synthesis of the key aspects of predator-induced TGP in metazoans. We define TGP as all phenotypic changes in a new generation that are triggered by the environment experienced by the previous generation(s). This broad definition (also used in very recent reviews: Bell and Hellmann, 2019; Yin et al., 2019; Donelan et al., 2020) allows to encompass effects on offspring phenotype due to the effect of predator exposure on maternal or paternal conditions (state-based TGP) and due to signals transmitted by parents (adaptive TGP). To search for relevant studies, we used the following keywords in the Web Of Science Core collection: (“trans?generational plasticity” OR “trans?generational effect?” OR ‘trans?generational response?” OR “inter?generational effect?” OR “inter?generational plasticity” OR “inter?generational response?” OR “maternal effect?” OR “paternal effect?” OR “parental effect?” OR “grand?parental effect?” OR “maternal programming” OR “paternal programming” OR “parental programming” OR “maternal care” OR “paternal care” OR “parental care” OR “maternal environment” OR “paternal environment” OR “parental environment” OR “grand?parental environment” OR “across generations”) AND (anti?predator OR predation OR predator) and we filtered the results in the subject areas ‘environmental sciences ecology’ and ‘evolutionary biology.’ Our review includes (1) studies that do not explicitly use the term ‘transgenerational plasticity’ but that fit in our definition of TGP, (2) studies in which transgenerational and within-generational responses interact (non-additive effects; e.g., Agrawal et al., 1999; Beaty et al., 2016) or not (additive effects; e.g., Salinas et al., 2013; Luquet and Tariel, 2016), (3) studies involving adaptive (e.g., inducible defenses) and/or non-adaptive (e.g., state-based TGP) responses, and (4) studies in which parental exposure to predator-cues occurred after fertilization, but in which it seems unlikely that the early developmental stages of offspring were able to directly perceive predator-cues on their own (e.g., exposure to olfactory predator-cues of gravid females, visual predator-cues during parental care on eggs). However, we discarded (1) studies that focus on offspring traits without considering the eco-evolutionary framework of predator-prey interactions (e.g., cognitive abilities: Coutellier and Würbel, 2009; Roche et al., 2012; response to contaminants: Plautz et al., 2013), using predation risk only as a stressor, and (2) studies that focus only on the reproductive performance of mothers (e.g., egg size or clutch size) without evaluating later effects on offspring traits (e.g., Segers and Taborsky, 2012; Tigreros et al., 2019). Moreover, this review, specifically focused on TGP in the context of predator-prey interactions, benefits from the very recent reviews on TGP in general (Bell and Hellmann, 2019; Yin et al., 2019) and in other ecological contexts (climate change: Donelson et al., 2018; human-altered environment: Donelan et al., 2020).
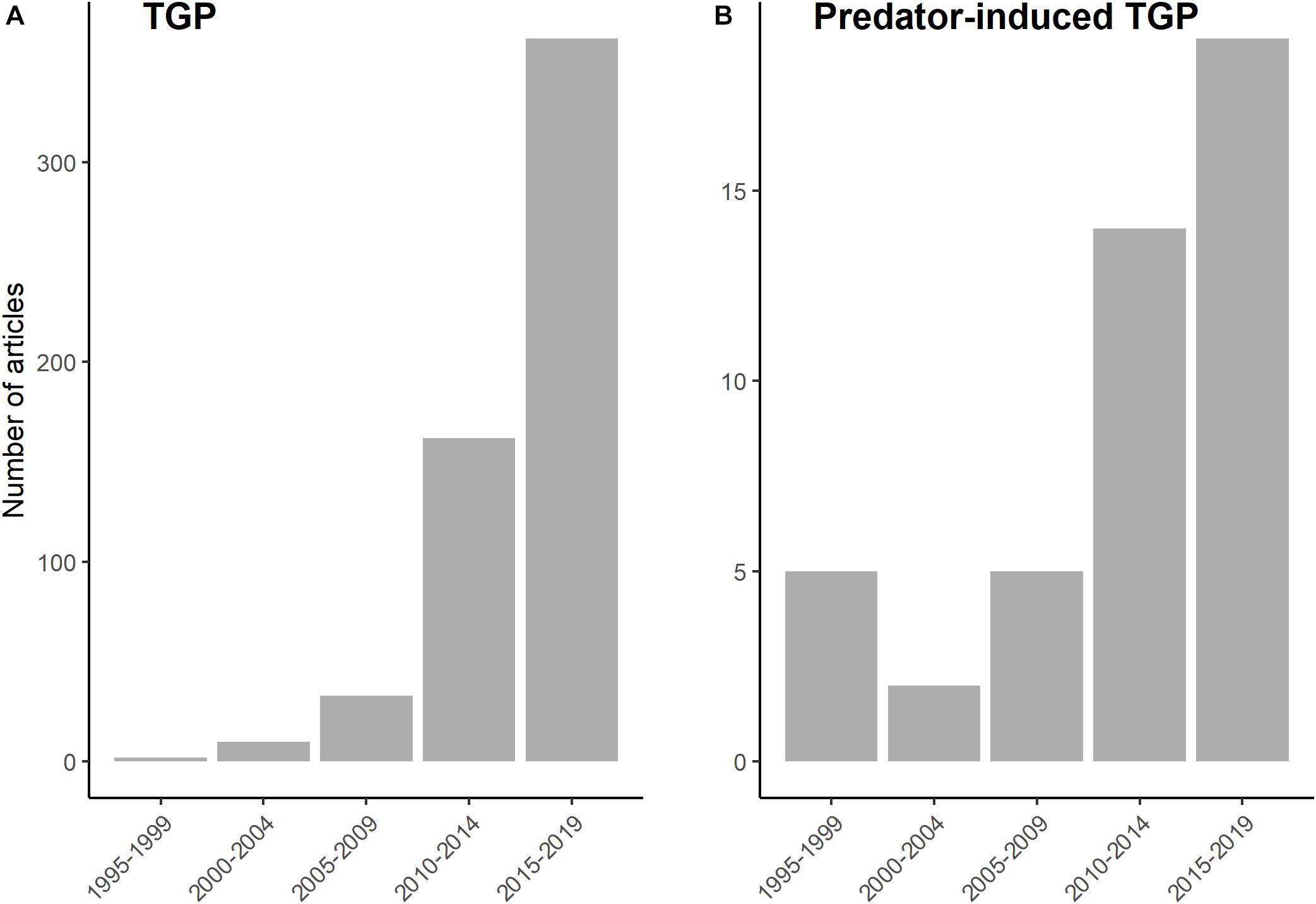
Figure 1. Growing interest in (A) transgenerational plasticity and (B) in predator-induced transgenerational plasticity. (A) List of articles extracted from the Core Collection of Web of science using the keyword “transgenerational plasticity.” (B) List of articles included in our review (Table 1), see the Introduction for the detailled selection procedure.
In total, we reviewed 55 empirical studies investigating predator-induced TGP using different predator signals (27 chemical, 17 visual, 5 auditory, 13 real predator) in various prey species (Table 1). Of these, 40 studies are empirical demonstrations of predator-induced TGP, while the other 15, mainly from the past 2 years, tested more specific hypotheses about TGP. TGP is therefore a growing research field, where the underlying processes are just starting to be explored. Our review is structured as follows: first, we summarize the types of traits involved in predator-induced TGP. Second, we focus on the evolutionary aspects of predator-induced TGP: what is its adaptive value? Can transgenerational responses evolve? What are the underlying mechanisms of inheritance? Third, we analyze the temporal dynamics of predator-induced TGP: what are the sensitive developmental windows in parents and offspring? How many generations does the influence of predators persist in prey? Fourth, we discuss other key aspects of predator-induced TGP: sex-specific patterns and adjustment to the intensity of predation.
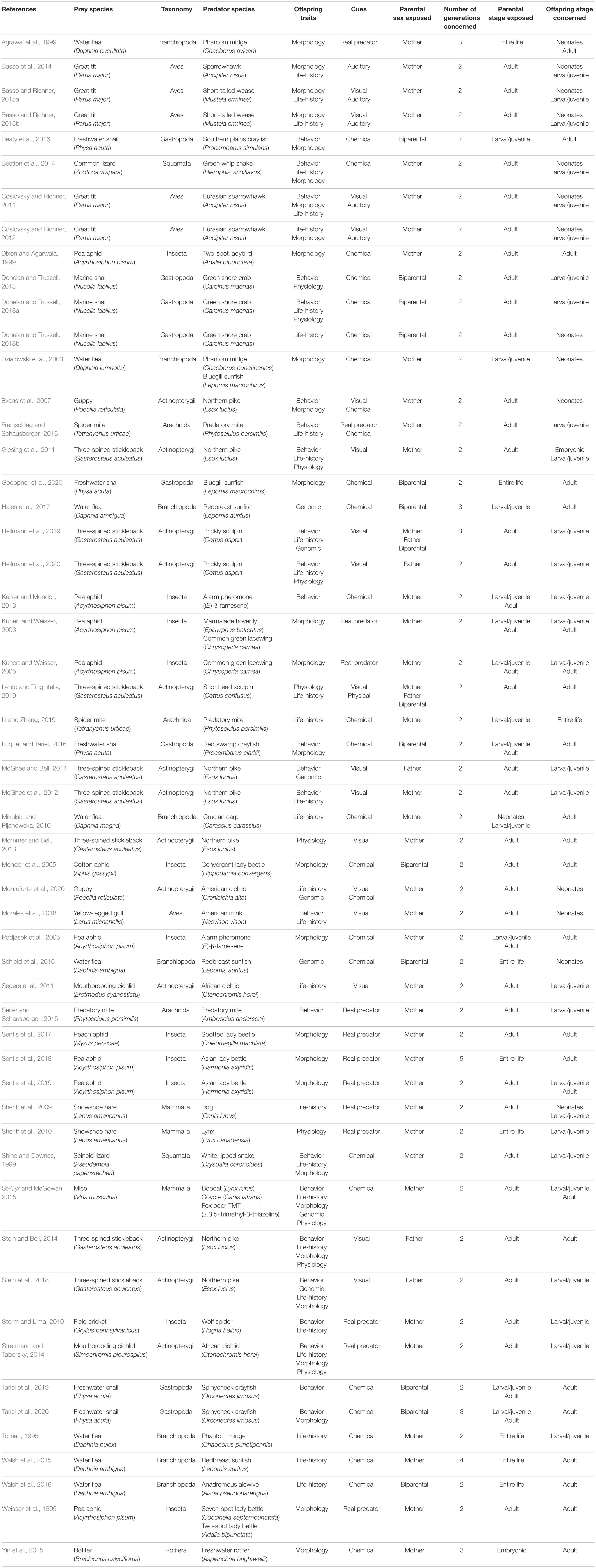
Table 1. Relevant information of the 55 papers studying transgenerational plasticity in the context of predator-prey interactions.
Type of Traits Involved in Predator-Induced Transgenerational Plasticity
Different types of traits (morphological, behavioral, life-history, and physiological) are involved in within-generational responses to predation (Tollrian and Harvell, 1999). These same traits can be influenced in offspring by parental exposure to predator-cues (Tables 2, 3), as for instance shell thickness in freshwater snails (Beaty et al., 2016; Luquet and Tariel, 2016), activity in sticklebacks (Stein et al., 2018; Hellmann et al., 2019), size at maturation in water fleas (Tollrian, 1995; Walsh et al., 2016) or corticosteroïds in hares (Sheriff et al., 2010). In the TGP studies we reviewed, 45% of traits are anti-predator defenses (e.g., longer helmet in Daphnia, crawling-out behavior in Physa freshwater snails), whereas the remaining 55% are more general responses (life-history: e.g., body size and mass; physiological stress-response: e.g., plasma cortisol; (epi)genomic modifications: e.g., brain gene expression; Tables 2, 3). Some of these more general responses may nevertheless contribute to anti-predator strategy, such as larger eggs (McGhee et al., 2012) or higher size at birth of offspring prey (Donelan and Trussell, 2018b), which allow to faster reach a refuge-size. In other cases, the responses are more likely to be by-products of carry-over effects of parental exposure to predator-cues (stress-based responses, e.g., smaller egg size: McGhee et al., 2012; smaller size at birth: Monteforte et al., 2020) or reflect trade-offs between anti-predator defenses and other functions in offspring (e.g., induction of morphological defenses at the expense of growth rate or investment in reproduction: DeWitt et al., 1998). For example, two studies (Stein and Bell, 2014; Stein et al., 2018) showed that stickleback offspring produced by fathers exposed to predation risk were lighter and smaller. It is possible that these offspring received less fanning (oxygen) from predator-exposed fathers, altering growth patterns during embryonic development (carry-over effect), or that increased timidity (a transgenerationally induced anti-predator behavior) involved a trade-off with foraging, limiting feeding of offspring from predator-exposed fathers. In these cases, we followed the study authors in classifying the traits as anti-predator defenses (i.e., participating in anti-predator strategy) or not (Table 3). The empirical studies included in this review reported statistically significant predator-induced TGP for 55 of the 68 (81%) anti-predator defenses and for 50 of the 82 (61%) more general responses (Tables 2, 3), although this rather high prevalence may be influenced by publication bias.
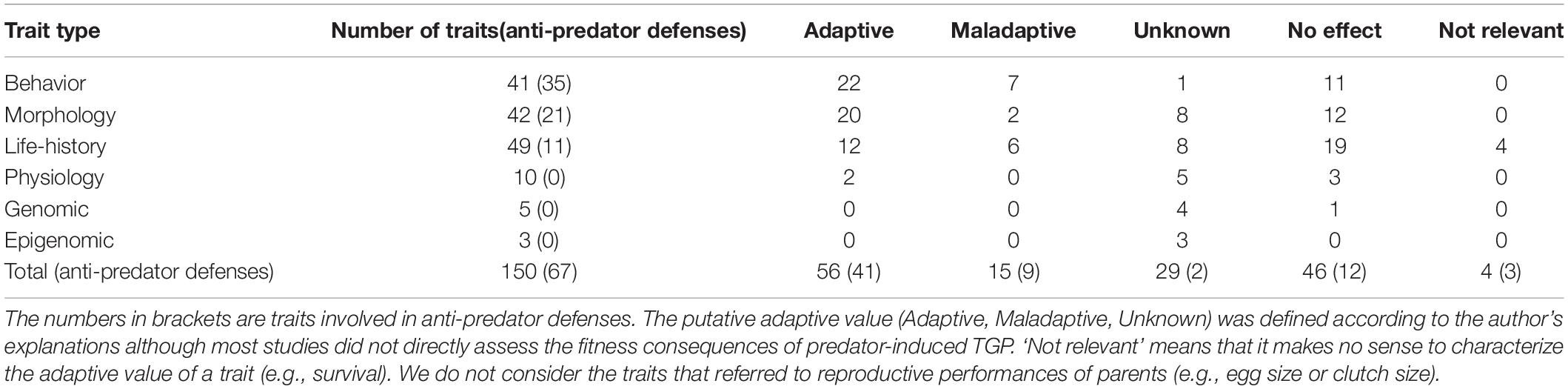
Table 2. Number of traits investigated across the 55 studies reviewed and putative adaptive value of the predator-induced transgenerational effects according to the trait type.
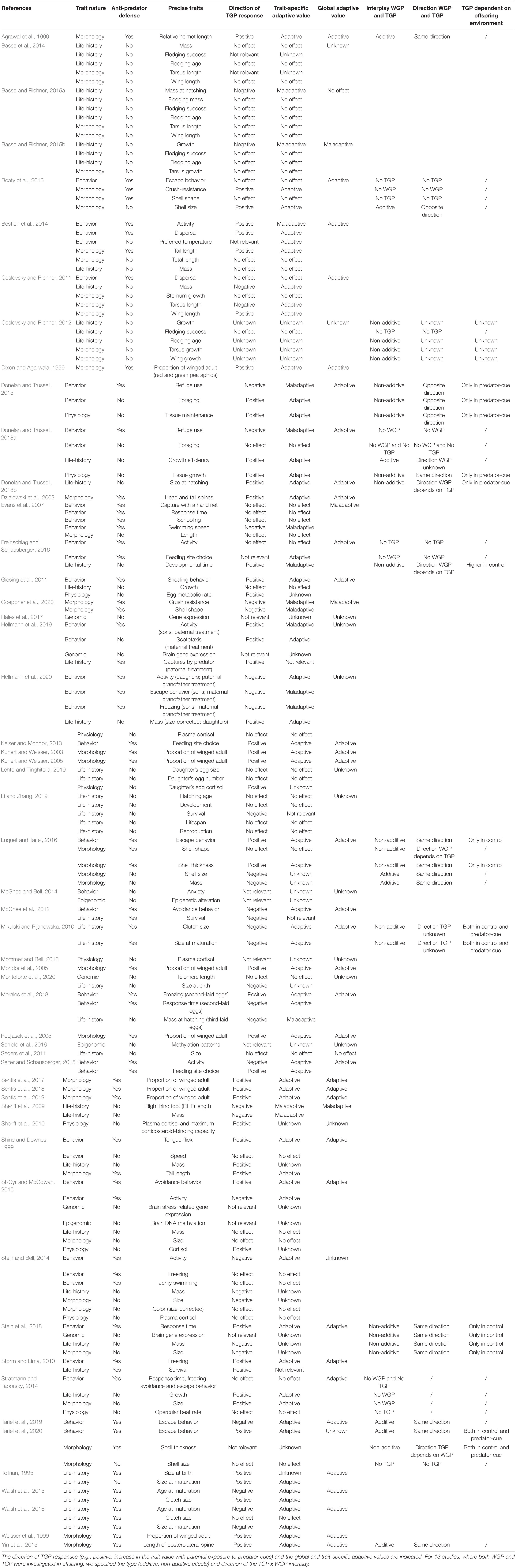
Table 3. Nature and details of all traits involved in predator-induced TGP (anti-predator defenses themselves or not) in the 55 studies.
Induction of anti-predator defenses both within and across generations depends mainly on the cost/benefit balance in different environments (with and without predators) (Uller, 2008; Auld et al., 2010; Murren et al., 2015). Benefits of induced responses can rely on (1) a decrease in the predator’s ability to perceive the prey (behavioral traits: e.g., increased refuge use, decreased activity); for example, Freinschlag and Schausberger (2016) showed in the two-spotted spider mite (Tetranychus urticae) that maternal exposure to predator-cues changes offspring preference for feeding sites from the leaf vein (predator-free mothers) to the leaf blade (predator-exposed mothers), reducing the likelihood of encountering predators; (2) a deviation from the predator’s preferred prey size (morphological and life-history traits: e.g., refuge-size, acceleration in development time, growth rate; Sogard, 1997); for example, Stratmann and Taborsky (2014) showed in a mouthbrooding cichlid (Simochromis pleurospilus) that offspring from predator-exposed mothers grow faster, protecting them from gape-limited fish predators; (3) an increased escape ability (morphological traits: e.g., improving locomotion ability or increasing handling time of predators to increase escape probability); for example, Bestion et al. (2014) exposed gravid females of common lizards (Zootoca vivipara) to snake cues and observed that offspring from predator-exposed mothers had longer tails (luring effect). Costs associated with induction of defenses within a generation arise mainly from changes in energy allocation. To our knowledge, costs of transgenerationally induced defenses have never been investigated, but are most likely similar to those of WGP. In addition, an interesting open question is whether the fact of transmitting defenses to offspring incurs costs to the parents.
Induction of anti-predator defenses also depends on trait lability, i.e., how fast the trait can change (induction and reversion) relative to predation risk; for example, behavioral traits are more labile and more easily reversible than morphological traits, which are in most cases more constrained during development and exhibit irreversible variations (West-Eberhard, 2003; Ghalambor et al., 2010). Theory therefore predicts that TGP should be found more frequently for morphological traits (allowing offspring to orient their early development toward an anti-predator response based on parental cues, before the morphological traits are fixed) than in behavioral traits (which should be more likely to be influenced by current cues) (Piersma and Drent, 2003; Dingemanse and Wolf, 2013; Kuijper and Hoyle, 2015). However, contrary to this theoretical prediction, the empirical studies included in this review found significant predator-induced TGP for all kinds of anti-predator defenses, including behavior [18 out of the 21 (86%) morphological defenses, 25 out of the 35 (71%) behavioral defenses and 10 out of the 11 (91%) life-history defenses, Tables 2, 3]. The benefit of inducing anti-predator behavior across generations may be that, precisely because they are highly labile, they can be quickly “switched off” (due to WGP) in case of a mismatch between parental and offspring environments (Beaman et al., 2016). Conversely, TGP involving morphological defensive traits or life-history traits is likely to irreversibly engage the offspring in certain developmental trajectories, even if predation risk decreases.
Overall, our review shows that the effects of parental exposure to predation are not restricted to specific types of traits. However, within studies, the transgenerational effects vary strongly among traits (significance and direction: e.g., Luquet and Tariel, 2016; Donelan and Trussell, 2018a; Stein et al., 2018), meaning that interpretations based on single traits will often be meaningless. Instead, predator-induced TGP should be interpreted in the context of a global anti-predator strategy, including several types of traits, and if relevant, their anti-predator role, and how they trade off with each other.
Evolutionary Aspects of Predator-Induced Transgenerational Plasticity
A key question for the study of TGP in predator-prey interactions is whether the transgenerationally induced phenotype actually increases offspring fitness. Indeed, the evolutionary potential of TGP depends on its adaptive value, the correlation between parental and offspring predation risk and on how the cue of predation risk is imprinted at a molecular level and inherited over generations.
Adaptive Value
Determining the adaptive value of TGP is not an easy task, because depending on studies, parental exposure to predation can have very different outcomes with regard to offspring fitness. In some cases, transgenerational responses appear clearly adaptive, for example, when offspring of predator-exposed parents exhibit stronger inducible defenses (e.g., Agrawal et al., 1999; Storm and Lima, 2010; Luquet and Tariel, 2016; Table 3 – “Adaptive value” columns). In other cases, transgenerational responses are more complex and depend on interactions between ancestral and offspring environments, the trait under consideration (§2; Tariel et al., 2020), developmental stage of offspring (§4.1.1; Li and Zhang, 2019) and sex (§5.1; Hellmann et al., 2019), and are therefore more difficult to relate to self-explanatory anti-predator scenarios with clear adaptive advantages. In addition, detection of predator presence may generate stress, which not only negatively impacts parental condition, but may also generate negative carry-over effects on offspring (state-based TGP, e.g., reduced body condition of progeny; Stein et al., 2018). As only three studies measured offspring fitness experimentally (for instance, with a survival test against a lethal exposure to predators; Storm and Lima, 2010; McGhee et al., 2012; Hellmann et al., 2019), we determined putative adaptive values of the transgenerational responses to predation by following the opinion of the study authors (Tables 2, 3). 64% of studies reported potentially adaptive responses, while 7% reported maladaptive responses; for 25% of studies, we were not able to determine the adaptive nature of the induced trait(s), and two studies did not show any transgenerational effects at all (Table 3 – “Global adaptive value” column). Although this imbalance may be due to publication bias in favor of systems with well-characterized anti-predator defenses, the above pattern nevertheless shows that predator-induced TGP has the potential to play an important role in predator-prey interactions, by allowing prey to pre-adapt their phenotype to future predation risk. Concerning interactions between ancestral and offspring environments, 14 studies report such interactions using full factorial experiments (Table 3 – “Interplay WGP and TGP” column). Results were very varied: WGP and TGP can operate in the same or opposite directions (Table 3 – “Direction WGP and TGP” column). Moreover, the direction of WGP can depend on parental environments, and conversely, the direction of TGP can depend on offspring environments (Table 3 – “Direction WGP and TGP” column). Interestingly, WGP can mask TGP, i.e., the effects of parental environment are only observed in predator-free offspring environments, or reveal it, i.e., the effects of parental environment are only observed in offspring experiencing predator presence (Table 3 – “TGP dependent on offspring environment” column), while TGP can override WGP, i.e., offspring do not respond to their own environment (e.g., Beaty et al., 2016; Freinschlag and Schausberger, 2016; Luquet and Tariel, 2016).
Evolutionary Implications
The next question is then to know whether predator-induced TGP can evolve and under what environmental conditions. In general, phenotypic plasticity is selected when the environment is variable in time and/or space and provides reliable and accurate cues about future selection pressures that will act on the induced phenotype (Kuijper and Hoyle, 2015; Leimar and McNamara, 2015). This is challenging for TGP, because cue perception and expression of the induced phenotype are distant in time with a minimum lag time of one generation. Empirical examples showing that TGP can evolve are rare, but some examples come indeed from the context of predator-induced defenses. Two studies have demonstrated local adaptation of transgenerational responses to predators in wild populations. Storm and Lima (2010) showed that gravid crickets (Gryllus pennsylvanicus) from populations with predators produce offspring that are more responsive to predator-cues than those from populations without predators. Walsh et al. (2016) studied induced defenses of Daphnia ambigua in populations under three regimes of fish predation (consistently strong, consistently weak, or variable predation risk). They demonstrated that consistently strong (or weak) predation risk selected for TGP, while variable risk favored WGP. These two examples confirm that TGP may evolve in the wild, and that temporal variability and predictability of predation risk are key forces driving evolution of predator-induced TGP. In contrast, Goeppner et al. (2020) found Physa acuta snails from a population with predators to be more crush-resistant than snails from a population without predators (local adaptation), but no differential patterns of predator-induced TGP between the two populations (same transgenerational responses in both populations, and opposite to WGP). Finally, evolution of predator-induced TGP is suggested by two studies on aphids (Acyrthosiphon pisum). Sentis et al. (2019) compared clonal lineages specialized on two host plants characterized by contrasting predation risk. They found that lineages specialized on plants associated with high predation risk had a stronger transgenerational response to predators (increased frequency of winged offspring) relative to those from plants associated with low predation risk. Importantly, the authors showed that the proportion of winged offspring has high heritability, indicating that this defense and its plasticity can potentially evolve by selection. In another study, Sentis et al. (2018) exposed aphids from the same clone to predator presence or absence for 27 generations. They observed that predator-induced TGP was similar between treatments after 16 generations of predator exposure, but decreased after 25 generations of predator exposure in the predator treatment compared to the predator-free treatment, suggesting that TGP was counter-selected (probably because production costs of defenses were not compensated by higher survival to predators in their experimental system where dispersal was limited). With so few studies, it seems difficult to draw general conclusions about the evolutionary potential of predator-induced TGP, but both theoretical and empirical studies point in the same direction. Therefore, it is crucial to investigate the evolutionary potential of predator-induced TGP by (1) assessing the heritability of transgenerational responses to predators, (2) demonstrating in the laboratory (e.g., by experimental evolution) that TGP can be selected according to the variability and predictability of predation risk, and (3) confirming local adaptation in transgenerational responses to predators in wild populations.
Inheritance Mechanisms
While it is now well-established that predation can induce defensive responses that persist for several generations, the mechanisms underlying this persistence remain a black box. For example, the relationship between shifts in gene-expression patterns and defense induction has only been described within a generation (Miyakawa et al., 2010; Tollrian and Leese, 2010). For transmission of information across generations, many authors evoke non-genetic sources of heritability, especially epigenetic ones (Boškoviæ and Rando, 2018; Norouzitallab et al., 2019; Duempelmann et al., 2020). However, both genetic and epigenetic mechanisms of predator-induced TGP have rarely been investigated in detail. Indeed, we found only six studies that explored predator-induced TGP at the genomic level (McGhee and Bell, 2014; St-Cyr and McGowan, 2015; Schield et al., 2016; Hales et al., 2017; Stein et al., 2018; Hellmann et al., 2019). Hales et al. (2017) tested the influence of chemical cues from fish predators on gene expression patterns within and across three generations (F0, F1, and F2) in D. ambigua. The clone they used was known to show strong predator-induced TGP, with phenotypic changes opposite to those induced by WGP (Walsh et al., 2015). They found that TGP and WGP involved changes in expression level in different sets of genes, indicating divergent underlying mechanisms. In contrast, Stein et al. (2018) showed that TGP and WGP involved changes in identical sets of genes in offspring of three-spined stickleback (Gasterosteus aculeatus), and that changes in expression level of these genes were identical whether predation risk was experienced by the father (TGP), the offspring (WGP) or both. At the phenotypic level, WGP and TGP also involved identical responses. These two studies suggest that predation risk induces similar genomic responses within and across generations when the phenotypic responses are themselves similar. In addition, genomic mechanisms may differ between the sexes. Hellmann et al. (2019) demonstrated in G. aculeatus that maternal and paternal exposure to predation risk had distinct effects on gene expression patterns in the offspring brain, and that these effects varied between male and female offspring. A similar result was observed in mice, where only the gene expression patterns of female offspring were impacted by maternal exposure to predator-cues (St-Cyr and McGowan, 2015).
Interestingly, Hales et al. (2017) observed a decrease in the number of differentially expressed genes between the F1 and F2 generations—a trend consistent with the observed decrease in transgenerational responses (§4.2; Walsh et al., 2015) and the lability of inherited epigenetic marks (Fallet et al., 2020). In a companion methodological paper, Schield et al. (2016) found shifts in the methylation state of sampled loci between F0 (with predator-cues) and F1 (without predator-cues) in D. ambigua, suggesting that DNA methylation patterns can vary between generations experiencing different predation environments. However, their experimental design dit not allow to demonstrate whether the epigenetic modifications are (1) sensitive to predator-cues (with vs. without predator-cues within a generation) and (2) related to the transmission of predation risk across generations (this would require a fully factorial design across two generations). In addition, Hellmann et al. (2020) showed that F0 exposure to predator-cues influenced the phenotype of F2 but not F1, indicating that epigenetic transmission and phenotypic consequences can be decoupled. Individuals could be carriers of epigenetic information and transmit altered phenotypes to their offspring without displaying the phenotypes themselves. Finally, McGhee and Bell (2014) showed in G. aculeatus that the amount of direct care provided by fathers, when modulated by predation risk, was linked to differential expression in offspring brains of a DNA methyltransferase (Dnmt3a) responsible for de novo methylation. Although this is not evidence of transgenerational epigenetic inheritance, it suggests that predation-risk driven behavior of fathers may influence the epigenetic programming of their offspring, which might in turn be transmitted to the next generation. To our knowledge, how and to what extent the epigenome is related to phenotype across generations is still an open question both in predator-prey systems and in general (see Fallet et al., 2020 for a detailed discussion). Taken together, the above results highlight the need for future work examining predator-induced TGP and WGP simultaneously, at both the (epi)genomic and phenotypic levels.
Temporal Dynamics of Predator-Induced Transgenerational Plasticity
Transgenerational plasticity is a temporal process that is initiated in past generations, but has consequences in current and future generations. Temporal dynamics are thus a key aspect of this process: what are the time windows during which an environmental signal must be detected to be transmitted across generations? When are the transgenerational responses expressed in offspring? How long can predator-induced TGP persist in prey across generations?
Critical Time Windows to Trigger Transgenerational Plasticity
Evolution of TGP depends on how accurately the parental cue eliciting TGP predicts the environment that will exert selection on the offspring phenotype (Auge et al., 2017; Bell and Hellmann, 2019; Donelan et al., 2020). Cue accuracy depends on the time lag between cue perception and expression of offspring phenotypes (§3.2). Therefore, two developmental aspects may influence the presence, strength and direction of predator-induced TGP: (1) the life-history stage at which parents perceive environmental cues (induction time), and (2) the life-history stage at which the offspring initiate responses (expression time) (Figure 2; Burton and Metcalfe, 2014; Donelson et al., 2018; Bell and Hellmann, 2019; Yin et al., 2019; Donelan et al., 2020). Among the 55 studies that explore transgenerational effects in response to predation risk, 18 investigate patterns of TGP with different timings of exposure in parents (four studies) or expression in offspring (14 studies).
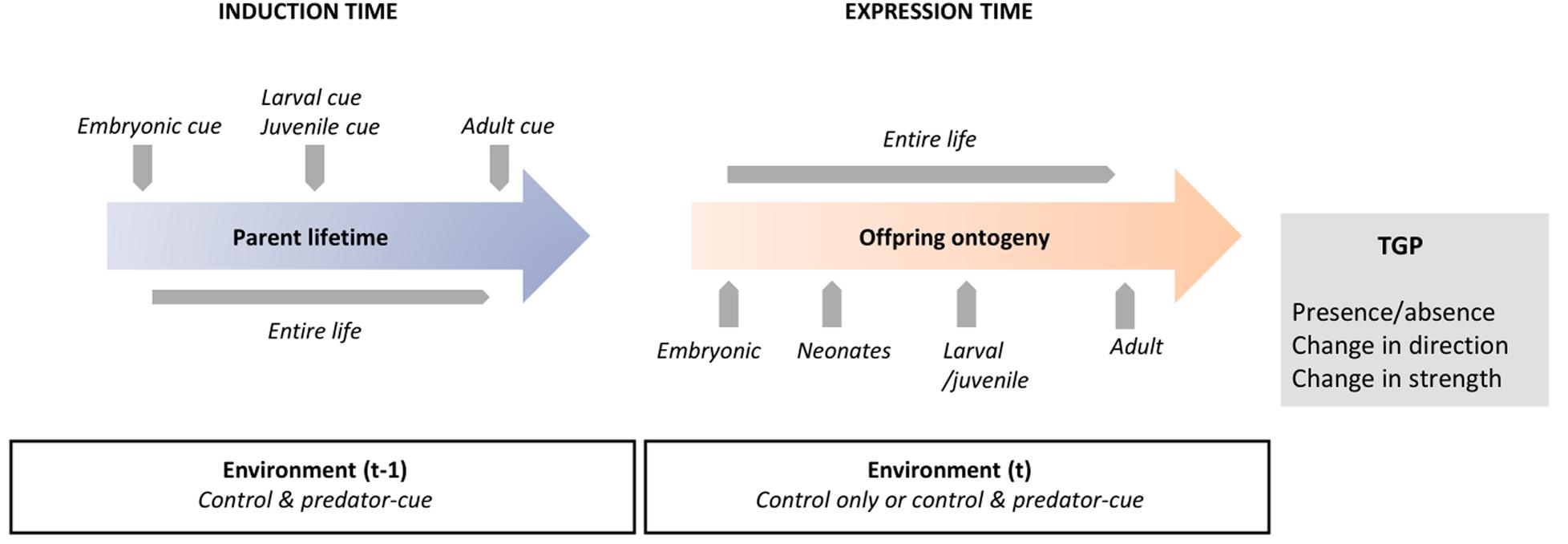
Figure 2. Sensitive windows in parental and offspring lifetimes for transgenerational plasticity induction and expression.
Induction Time of Transgenerational Plasticity: Parental Sensitive Windows
The time at which an environmental change is experienced can directly influence phenotypic responses in both the exposed individual (WGP) and its offspring (TGP) (Burton and Metcalfe, 2014; Donelson et al., 2018; Donelan et al., 2020). Some theoretical models predict that early-perceived cues in parental development should have weaker effects on offspring than late-perceived cues (Ezard et al., 2014; Prizak et al., 2014). Indeed, later parental environments are better predictors of offspring environment because of the shorter time lag between perception of cues and the predicted environment. Therefore, the period before and during reproduction in parental development is expected to be a critical period for TGP induction (Donelan et al., 2020). In contrast, some empirical studies rather identify the early parental development (i.e., embryonic or neonate phases) as particularly sensitive to influence TGP (Burton and Metcalfe, 2014; Fawcett and Frankenhuis, 2015; Donelson et al., 2018; Yin et al., 2019), which might be linked to a higher proportion of early embryonic cells that are highly sensitive to environmental cues (Burton and Metcalfe, 2014).
In the context of predator-induced TGP, most studies (62%) focused on parental exposure during adult life only (Table 1). A few studies explored the effects of parental exposure during the post-embryonic period (neonates + larval/juvenile phase, larval/juvenile phase, larval/juvenile phase + adults; 2, 7, and 13% of studies, respectively) or TGP responses when parents were confronted with predator presence during their entire life including the embryonic phase (15%). Only one study was carried out with parents exposed to predator-cues only in the embryonic phase (Yin et al., 2015). Finally, we found only four studies (7%) that compared the influence of different exposure timings (Agrawal et al., 1999; Mikulski and Pijanowska, 2010; Walsh et al., 2015; Yin et al., 2015). Three of these studies are focused on water fleas (D. cucullata, D. magna, D. ambigua; Agrawal et al., 1999; Mikulski and Pijanowska, 2010; Walsh et al., 2015), while the fourth one is on the rotifer Brachionus calyciflorus (Yin et al., 2015). Considering these four studies, three patterns emerge (Figure 2).
Two studies demonstrated that the expression of predator-induced TGP depended on the parental life stage in which the cues were perceived, but timing differed between the two. Agrawal et al. (1999) showed that a defensive morphology in water fleas (D. cucullata) was induced in offspring from mothers exposed to predator-cues before becoming pregnant, but not in offspring from mothers exposed later. Walsh et al. (2015), working on D. ambigua, found that offspring from mothers exposed to predator-cues during the juvenile stage did not show TGP with respect to the age of maturation. In contrast, offspring from mothers that had been exposed during their entire life or only late in their development (i.e., at maturation) matured faster than those originated from predator-free mothers.
Two other studies highlighted that the strength of predator-induced TGP depended on the parental life stage in which the cues were perceived. Offspring from D. magna mothers exposed to predator-cues at a late juvenile stage exhibited broader life-history responses to predation (i.e., maturity at a smaller size, lower fecundity) than offspring from mothers confronted with predation at earlier or later life stages (Mikulski and Pijanowska, 2010). Similarly, offspring from B. calyciflorus mothers exposed to predator cues at a late embryonic stage exhibited broader morphological responses to predation (i.e., longer spine and higher posterolateral spine-body length ratio) during two generations than offspring from mothers exposed at earlier embryonic stages (Yin et al., 2015).
To our knowledge, no study has found evidence that the direction of predator-induced TGP depends on the parental life stage in which the cues are perceived. However, an element of a response emerges when two different studies on sticklebacks (G. aculeatus) are combined. While paternal exposure to predator-cues during sperm formation decreased offspring anti-predator behavior and survival in the presence of a real predator (Hellmann et al., 2019), paternal exposure later during egg care increased offspring anti-predator behavior and decreased body size and condition (Stein and Bell, 2014). These results suggest that, in the first case, fathers transmitted negative effects of predator-induced stress to their offspring, while in the second case, fathers pre-adapted their offspring to predation risk.
All these results confirm the importance of considering how the timing of parental exposure affects the presence, strength and direction of transgenerational phenotypic changes. Results are not consistent across studies and show that several parental life stages can be critical periods for influencing the next generation (embryonic stage, late juvenile stage, or at maturation), sometimes with a narrow window of sensitivity (early and late embryonic stage, or at maturation before or after being pregnant).
Expression Time of Transgenerational Plasticity: Offspring Sensitive Windows in Ontogeny
From the offspring’s perspective, timing of cue perception is important because it determines when offspring can initiate an appropriate response to information transmitted by their parents. Some researchers expect a stronger effect on offspring phenotype when parental cues are received early in embryonic development (Bell and Hellmann, 2019). However, it is difficult to know when cues are actually perceived or integrated by the offspring, and studies generally only report the offspring stage at which TGP is expressed. The meta-analysis of Yin et al. (2019) found that TGP is most strongly expressed in offspring juvenile stages, and less strongly in embryonic and adult stages. In the context of predation, the majority of studies measured transgenerational responses only once during offspring development: during early development (neonates) in 11% of studies, later during the larval-juvenile stage in 29% of studies, and during the adult stage in 35% of studies (Table 1). No studies examined TGP expression only in the embryonic stage. To our knowledge, 14 studies (25%) explored TGP expression in response to predation risk at different times in offspring life, either within an offspring stage or among different stages (Tollrian, 1995; Agrawal et al., 1999; Sheriff et al., 2010; Coslovsky and Richner, 2011, 2012; Giesing et al., 2011; Basso et al., 2014; Bestion et al., 2014; Stratmann and Taborsky, 2014; Basso and Richner, 2015a, b; St-Cyr and McGowan, 2015; Freinschlag and Schausberger, 2016; Li and Zhang, 2019). Screening these studies, different scenarios are observed (Figure 2).
Five studies described that parental experience with predation risk can influence offspring traits early in development, but the effect dissipates later in life. Two studies on spider mites (T. urticae) showed that maternal predation experience retarded offspring development in embryonic, larval and early juvenile stages, but the effect disappeared in the late juvenile stage and for reproductive parameters in adults (Freinschlag and Schausberger, 2016; Li and Zhang, 2019). In the same way, offspring of predator-exposed mothers were smaller and lighter during the early juvenile stage in great tits (Coslovsky and Richner, 2011, 2012; Basso and Richner, 2015a) and grew faster in an African cichlid (Stratmann and Taborsky, 2014), whereas no TGP of these traits was found later in the juvenile and adult stages for both models.
In the study of Agrawal et al. (1999), TGP was also expressed early in offspring development, but decreased later without disappearing completely. Indeed, differences in helmet length in D. cucullata offspring (second and third brood of the same generation) from mothers exposed to predator-cues relative to those from control mothers were stronger in the neonate stage than later in the adult stage. In contrast, Bestion et al. (2014) showed an increasing strength of TGP expression over offspring development: common lizard juveniles (Z. vivipara) born to mothers exposed to predator-cues had longer tails relative to body length already at birth, but the difference was stronger later in development. In the study of Basso and Richner (2015b) on great tits, maternal exposure to predator-cues did not significantly alter offspring growth rate at birth, but later during juvenile development.
Three other studies also showed that parental predator experience induces defenses in early life-history stages that persist over offspring development with approximately the same strength. For example, in Tollrian (1995), the difference in body size of D. pulex offspring from mothers exposed to predator-cues relative to offspring from control mothers remained significant and at approximately the same strength throughout juvenile development (six instars). In another species of water flea (D. cucullata), neonates (first brood) born from mothers exposed to predator-cues produced stronger induced defenses (i.e., higher relative helmet length) than neonates from mothers raised in a predator-free environment (Agrawal et al., 1999), and this effect persisted with the same strength when offspring reached maturity. In the same way, Sheriff et al. (2010) found identical patterns of TGP responses (increased fecal corticosteroid metabolite concentration, a stress index) for juvenile snowshoe hares (L. americanus) irrespective of their age.
In contrast, only one study (Coslovsky and Richner, 2011), on wing development in great tits (P. major), found transgenerational responses changing direction over the course of offspring development. The authors found that, just after birth, offspring from predator-exposed mothers had shorter wings than those from predator-free mothers. Later, however, wing growth in offspring from predator-exposed mothers was accelerated. Consequently, on day 14, the difference between the two treatments had vanished, and at age of maturity, offspring from predator-exposed mothers had longer wings than those from predator-free mothers.
Finally, to our knowledge, no studies to date found that parental experience of predator-cues can shape offspring phenotype strictly late in life. While many studies (42%) investigated and found TGP in adults, it is not possible to determine if these transgenerational effects appeared earlier and persisted across development or if they appeared only in the adult stage.
All these studies on expression time suggest that offspring can integrate past experiences of predation into the expression of their phenotype at different stages of development. Transgenerational effects in offspring may be expressed in a single stage or throughout the entire life, and their strength and direction can change during development. Contrary to the meta-analysis of Yin et al. (2019), which found that TGP tends to be weak in early offspring development and stronger later on during the juvenile stage, most studies reviewed here show that predator-induced TGP is stronger in early offspring stages.
In conclusion, empirical studies on the timing of TGP showed equivocal and often inconsistent results. Indeed, TGP may be induced by predator-cues perceived during different developmental windows in parents and may be expressed at different life stages in offspring. Missing these critical windows in experiments may lead to underestimation of the importance of TGP in predator-prey interactions.
Persistence Across Generations
How and for how long predator-induced TGP can persist in prey across generations is crucial information, because it determines the extent to which transgenerational responses contribute to long-term evolutionary changes. Since TGP is characterized by a lag time between cue perception and expression of the induced phenotype (§3.2), its persistence across generations should depend on the reliability of cues in predicting predation risk in subsequent generations. Based on the patterns proposed in the review of Bell and Hellmann (2019) on general TGP, we can propose three scenarios: (1) Cues are reliable indicators of predation risk only for the next generation: F1 offspring produced by parents exposed to predator-cues (F0 generation) express phenotypic changes, which dissipate in the F2 and subsequent generations (pattern 1 in Figure 3); (2) Cues are reliable indicators for several generations: the induced phenotype of the F1 generation persists in a similar way (strength and direction) across one (F2) or multiple generations (pattern 2 in Figure 3); (3) The reliability of cues decreases over time: the induced phenotype of the F1 generation persists across multiple generations, but with decreasing mean effects between consecutive generations (pattern 3 in Figure 3).
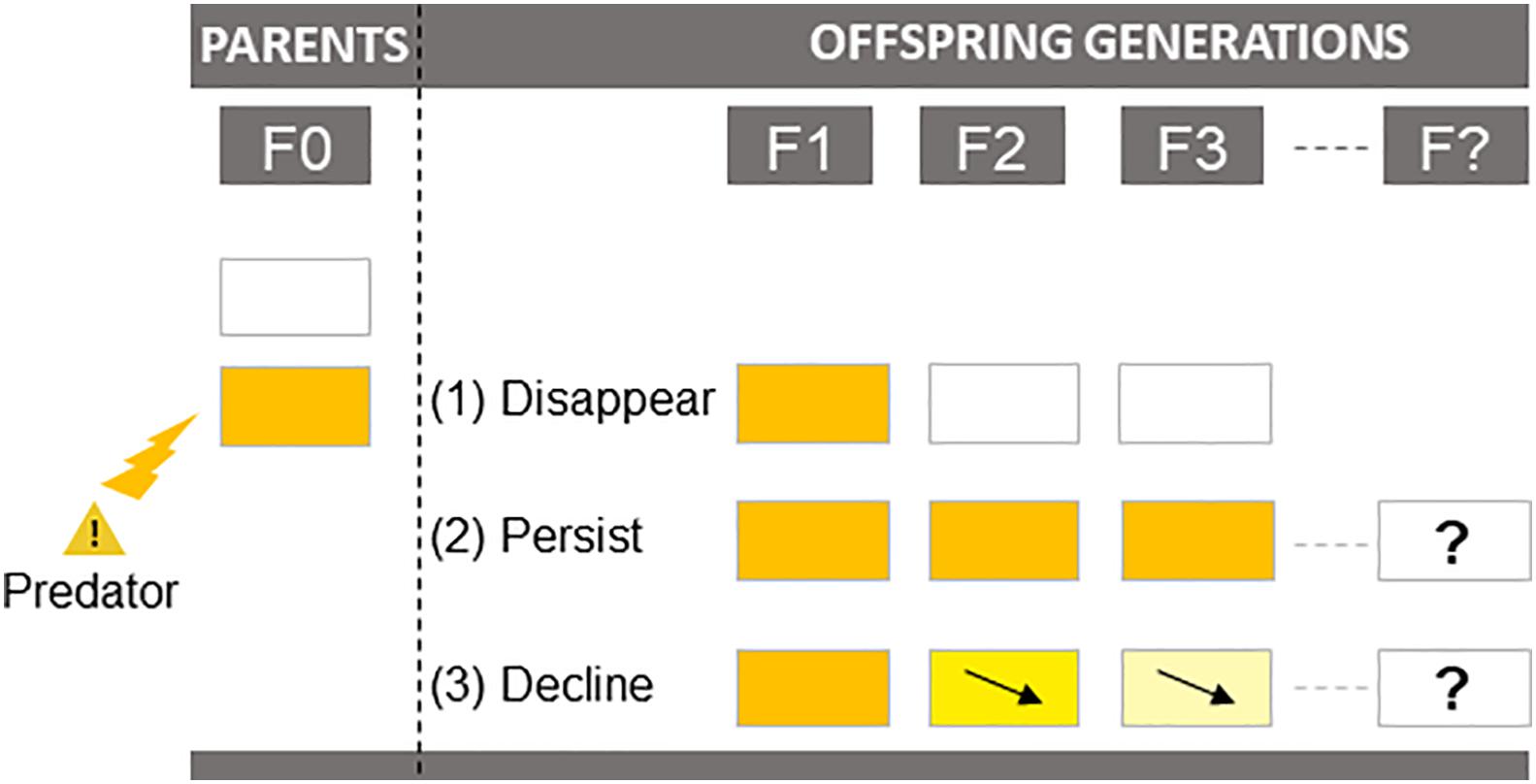
Figure 3. Scenarios of transgenerational plasticity persistence over generations. Prey are exposed to predator cues only at the F0 generation.
Most of the studies reviewed here (87%) investigated predator-induced TGP over two generations only (F0 and F1). In the following, we summarize the results of seven studies that explored the influence of past exposure to predation over three (Agrawal et al., 1999; Yin et al., 2015; Hales et al., 2017; Hellmann et al., 2020; Tariel et al., 2020), four (Walsh et al., 2015) or five generations (Sentis et al., 2018). Agrawal et al. (1999) showed that the morphological defense of D. cucullata (relative helmet length) induced in F1 offspring from F0 predator-exposed parents disappeared in F2 offspring (pattern 1 in Figure 3). A similar pattern was found for clutch size in D. ambigua (Walsh et al., 2015), total length and stress-induced cortisol in the three-spined stickleback G. aculeatus (Hellmann et al., 2020), and for body mass and shell size in the freshwater snail P. acuta (Tariel et al., 2020): in all cases, the transgenerational effect disappeared in the F2 generation (no effect of the grand-parental environment). In contrast, Yin et al. (2015) demonstrated grand-maternal induction of defensive morphology (posterolateral spine) in rotifers B. calyciflorus. Similarly, Tariel et al. (2020) found that grand-parental exposure to predator-cues influenced escape behavior and shell thickness in P. acuta. For age at maturation in D. ambigua, predator-induced TGP (earlier maturation) was detectable two generations following cue removal (i.e., until the F2 generation), and finally disappeared in the F3 (Walsh et al., 2015). Interestingly, the transgenerational effect in the F2 generation was lower than in the F1 in all studies (Walsh et al., 2015; Yin et al., 2015; Tariel et al., 2020), indicating a decline of transgenerational response to predation over time (pattern 3 in Figure 3). Moreover, Hellmann et al. (2020) observed in G. aculeatus that predator-induced TGP persisted in a lineage-specific (through the grand-maternal or grand-paternal lineage) and in a sex-specific (only in male or female grand-offspring) way: F2 females were heavier and had a reduced anti-predator response (reduced activity after a simulated predator attack) when their paternal grandfather was exposed to predator-cues, while F2 males had a reduced anti-predator response (frozen and escape behavior) when their maternal grandfather was exposed to predator-cues. This means that transgenerational effects may selectively persist across generations in only a subset of individuals, which can make it very difficult to assess the persistence of predator-induced TGP, since most studies focused on average responses only. To our knowledge, the experimental-evolution experiment by Sentis et al. (2018) is the only study that investigated predator-induced TGP over five generations. They exposed genetically identical pea aphids (A. pisum) to predator presence for 27 generations, but removed predators at three points (after 3, 13, and 22 generations of exposure) and monitored predator-induced TGP for five generations after predator removal. They found that the defensive phenotype—a high frequency of winged aphids in the population—persisted for one generation after predator removal, but then fell for two to three generations below the level of the predator-free treatment (lower proportion of aphids with defenses). This example illustrates not only that TGP can persist over several generations, but also that the effects can change direction (potentially going from adaptive to non-adaptive) over generations. Interestingly, the number of generations needed to come back to the level of the predator-free treatment increased with the number of generations previously exposed to predators. This suggests that the accumulation of exposures to predators over generations may increase the persistence of predator-induced TGP.
Although there are too few studies to draw a general conclusion, all studies to date show that predator-induced TGP can extend beyond the generation following predator exposure, but seems to decline gradually with each generation and eventually disappear (pattern 3 on Figure 3). This highlights the need for empirical studies on longer timescales to determine how long the signals of predators are embedded across generations.
Other Key Aspects of Predator-Induced Transgenerational Plasticity
According to the literature, two other aspects can influence the induction, strength and direction of predator-induced TGP: sex and the strength of predation risk. The key questions are: (1) Do paternal and maternal environments influence offspring phenotype in the same way or not? And do transgenerational responses depend on offspring sex? (2) Do prey invest in anti-predator responses according to the level of predation risk in the parental environment?
Sex-Specific Predator-Induced Transgenerational Plasticity
TGP can be sex-specific: its induction in offspring may depend on which parent transmits the environmental signal (parental level) and its expression by offspring may depend on their sex (offspring level). At the parental level, TGP can be induced in offspring by the maternal environment, the paternal environment, or both (e.g., Bonduriansky and Head, 2007; Triggs and Knell, 2012; Guillaume et al., 2016) (left panel on Figure 4). Parental cues can lead to (1) different information allowing offspring to trigger different responses in terms of strength or direction or at different developmental stages (multiple messages hypothesis), or (2) similar information allowing offspring to only respond to cues when they receive information through more than one modality (threshold hypothesis) or just to have a backup (backup hypothesis) (Bell and Hellmann, 2019). At the offspring level, the parental environment can impact only daughters, only sons, or both daughters and sons, which can lead to different responses in terms of strength and/or direction (review in Glover and Hill, 2012 for the effects of parental stress) (right panel on Figure 4). What favors sex-specific patterns at parental and offspring levels remains unexplored, but potentially has strong evolutionary implications (Bell and Hellmann, 2019).
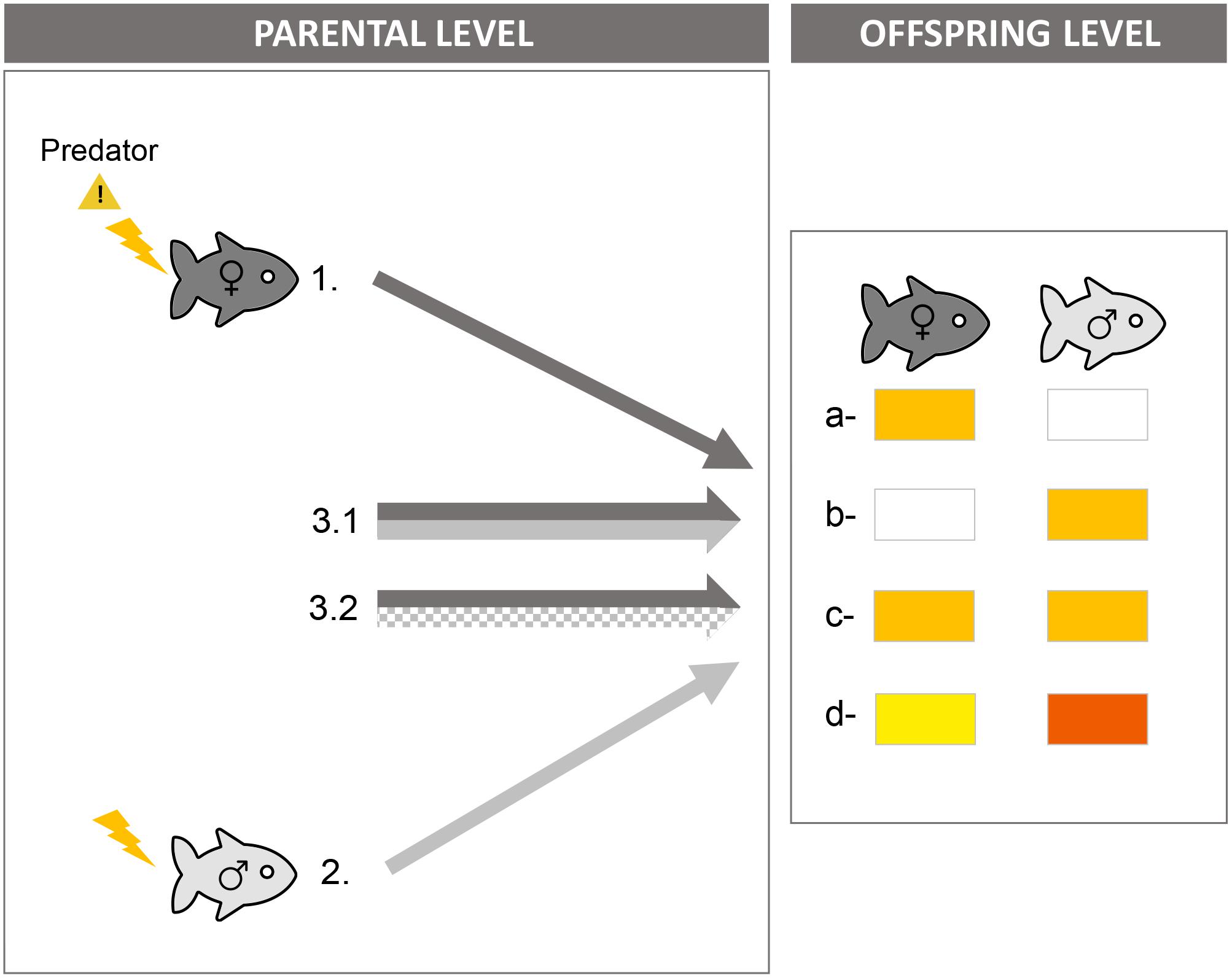
Figure 4. Sex-specific predator-induced transgenerational plasticity. Even if both parents detected cues of predator presence, offspring may respond only to maternal cues (pattern 1), only to paternal cues (2) or to both parental cues with similar (3.1) or different effects on strength and/or direction between maternal and paternal cues (3.2). Furthermore, the response to parental cues may be expressed in daughters only (pattern a), in sons only (b) or in both daughters and sons but with similar (c) or different responses in terms of strength and/or direction (d).
Parental Sex-Specific Transgenerational Plasticity
It has long been assumed that induction of TGP is mostly driven by the maternal environment (Crean and Bonduriansky, 2014). Consequently, sex-specificity of TGP at the parental level has been largely overlooked. In the context of predator-induced TGP, it has only been investigated in two studies on sticklebacks (Hellmann et al., 2019; Lehto and Tinghitella, 2019). In these studies, parental sex-specific TGP patterns were highly dependent on offspring traits. Some offspring traits were affected only by maternal exposure to predator-cues (cautiousness) or only by paternal exposure (activity) (patterns 1 and 2 on Figure 4). For the other traits (survival, cortisol level in the daughter’s egg), effects of paternal exposure interacted with effects of maternal exposure, adding yet another layer of complexity. In other words, the presence and pattern of paternal effects depended on maternal exposure to predator-cues (and vice-versa). For example, in Hellmann et al. (2019), paternal exposure decreased offspring survival to a real predator, but only when mothers had not been exposed to predator-cues. When mothers had been exposed, paternal exposure did not affect offspring survival (multiple message hypothesis). In Lehto and Tinghitella (2019), only exposure of both parents increased cortisol level of daughter’s egg, while paternal exposure or maternal exposure alone had no effect (threshold hypothesis).
Offspring Sex-Specific Transgenerational Plasticity
In the context of predator-induced TGP, sex-specific TGP at the offspring level has been investigated in seven studies: three on great tits (Coslovsky and Richner, 2011; Basso and Richner, 2015a, b), two on sticklebacks (Stein and Bell, 2014; Hellmann et al., 2019), one on spider mites (Li and Zhang, 2019) and one on mice (St-Cyr and McGowan, 2015). As at the parental level, sex-specific TGP patterns were highly dependent on offspring traits. Most traits (e.g., weight, body size, survival, cautiousness) did not show any sex-specific pattern: daughters and sons were equally affected by the parental environment (Coslovsky and Richner, 2011; Stein and Bell, 2014; Basso and Richner, 2015a, b; Hellmann et al., 2019) (pattern c in Figure 4). For the other traits, parental exposure influenced only one sex and not the other (patterns a and b in Figure 4: Coslovsky and Richner, 2011; Basso and Richner, 2015a; Hellmann et al., 2019; Li and Zhang, 2019). For instance, paternal exposure to predator-cues increased activity of sons but not daughters—this could be adaptive, as only males may benefit from higher activity under predation risk in sticklebacks. Only one study showed that the paternal environment can influence both daughters and sons but with different strength: daughters increased their anti-predator behavior and cortisol level more than sons following maternal exposure to predator-cues in mice (pattern d in Figure 4; St-Cyr and McGowan, 2015).
At the parental level, as all results are on sticklebacks, more species remain to be described to generalize the existence and patterns of parental sex-specific TGP. However, these few results suggest that offspring integrate cues from both parents, raising questions about how parental cues combine together (i.e., whether they are additive, non-additive, repetitious, etc. Bell and Hellmann, 2019). At the offspring level, daughters and sons tend to react similarly, but when sex-specific TGP is present, transgenerational responses are often observed in one sex and not the other.
In conclusion, all these results suggest that sex of both offspring and parents may shape how TGP impacts predator-induced traits. We still do not know what favors sex-specific TGP at both parental and offspring levels. Sexual conflict may play a role if males and females have different phenotypic optima when facing predation risk (Christe et al., 2006; Meuthen et al., 2018; Burke et al., 2019). Sex-differences in ecology, gamete dispersal or offspring investment may also play a role if, for example, maternal and paternal environments predict offspring environment differently (§3.2; Bell and Hellmann, 2019).
Adjustment of Transgenerational Plasticity to Predation Risk
In the context of WGP, anti-predator defenses have long been considered as polyphenism between defended and undefended morphologies (Figure 5A; Harvell, 1990). For instance, a field experiment on the acorn barnacle Chthamalus anisopoma showed polyphenism with two distinct shell morphologies (straight vs. curved shape) in response to predation (Lively, 1986). In reality, however, level of expression of anti-predator defenses is often correlated with intensity of current predation risk (i.e., phenotypic modulation; Figure 5B; e.g., Tollrian, 1993; Van Buskirk and Arioli, 2002; Laurila et al., 2004), allowing prey to be protected from predators while limiting the costs of over-expression of defense (Tollrian et al., 2015). For example, Teplitsky et al. (2005) found a positive correlation between morphological changes and fish predator density in Rana dalmatina tadpoles, and Yin et al. (2015) found a positive correlation between spine development and concentration of predator-cues in the rotifer B. calyciflorus. We can therefore reasonably suggest that the level of TGP (i.e., level of expression of offspring defenses) should also be proportional to the level of predation risk perceived by ancestors (Figure 5B). This hypothesis has been investigated in only three papers. Podjasek et al. (2005) clearly found a positive and relatively linear correlation between production of winged offspring in the pea aphid A. pisum and concentration of alarm pheromones, a reliable cue of predation risk. Also in the pea aphid, Kunert and Weisser (2003) found a non-linear relation between transgenerational response and parental predation risk, with the strongest response (i.e., highest percentage of winged offspring) being observed at an intermediate predation risk and the lowest response at very high and very low predation risk. Finally, Freinschlag and Schausberger (2016) did not find any correlation between developmental time or anti-predator behavior of juvenile spider mites (T. urticae) and the intensity of predation risk (no, low, moderate, severe) experienced by their mothers. To get a consistent picture, more empirical studies are needed on how the intensity of parental predation risk modulates the expression level of offspring defenses.
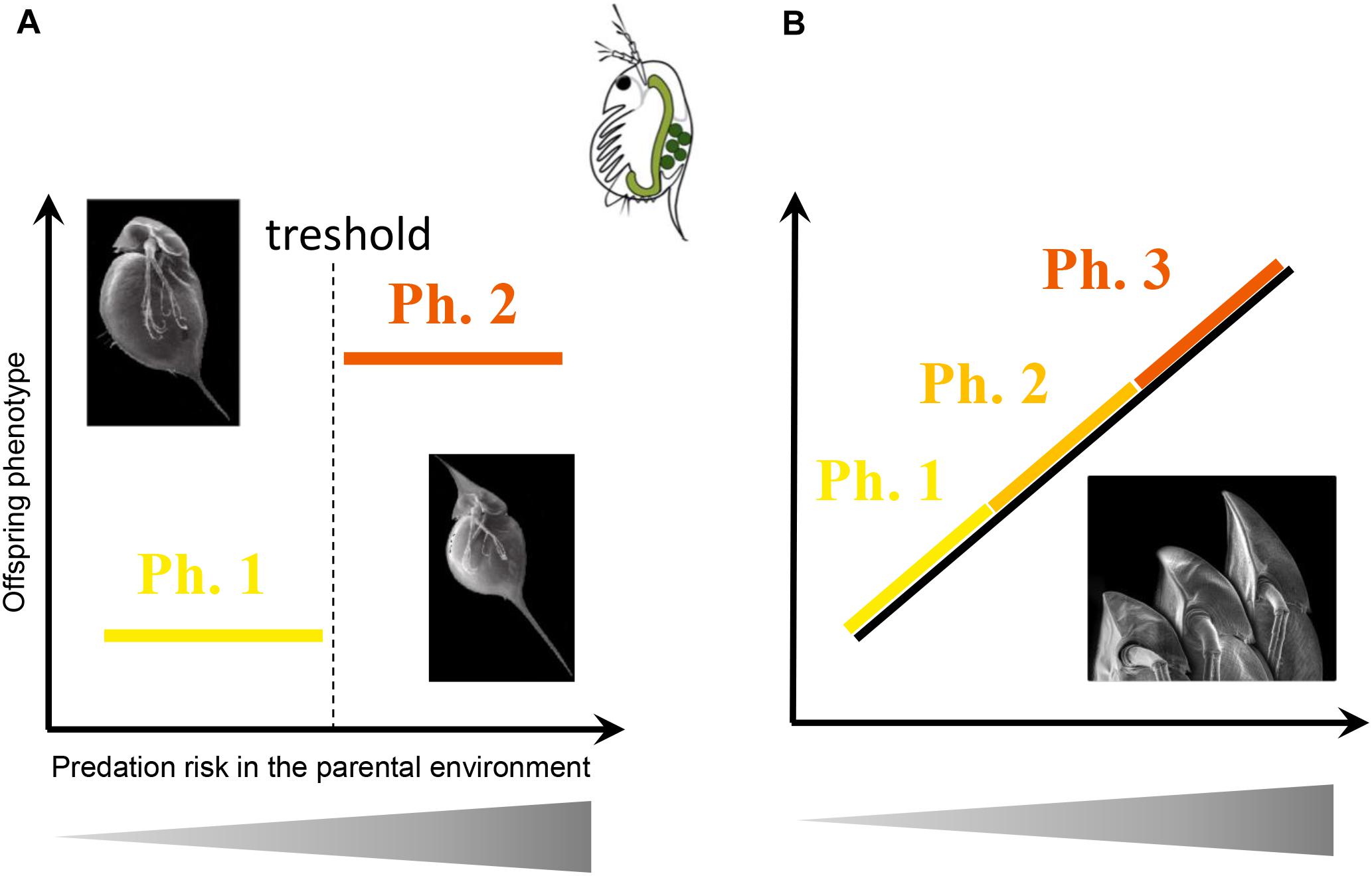
Figure 5. Offspring trait expression according to increasing predation risk in the parental environment. When the risk increases, (A) there is no interest to be half protected against a predator, the expected response is a polyphenism (threshold response), or (B) the strength of the response is expected to be proportional to actual risk (phenotypic modulation). Photographs are from Agrawal (2001), (reprinted with permission from AAAS) and from Herzog and Laforsch (2013).
Conclusion
Predator-prey interactions have long been a focus of ecological and evolutionary studies, likely because almost all species are engaged in such interactions. The literature has accumulated a solid knowledge of within-generation plasticity (WGP) in prey and predators, and it is commonly observed that prey develop defensive phenotypes when they detect predation risk. Over the past two decades, some studies have also shown that prey exposed to predator-cues can produce offspring with better defenses than offspring from predator-free parents [transgenerational plasticity (TGP)]. This review summarizes current knowledge on predator-induced TGP in metazoans. Most of the 55 studies we reviewed focused on five model taxa: fish (24%) aphids (16%), water fleas (15%), aquatic snails (15%), and birds (11%). Although a more diverse set of taxa would allow for more robust generalizations, the study of TGP requires rearing animals over at least two generations, which limits the study to taxa with short generation times. This explains why some taxa that are widely used to study predator-induced WGP, such as amphibians, are still lacking in TGP studies.
Our review highlights that all kinds of traits are prone to exhibit predator-induced TGP, even the most labile ones such as behavior. Predator-induced TGP seems to confer increased fitness in most studies, is evolvable (e.g., shows signatures of local adaptation) and can involve epigenetic mechanisms of inheritance, although these aspects are still too rarely evaluated. However, predator-induced TGP is often characterized by complex phenotypic patterns that can be difficult to interpret and do not always fit with simple explanatory scenarios invoking clear adaptive advantages. The induction, strength, and direction of predator-induced TGP depend on several factors and their interplay:
(1) Timing is important, but no general sensitive or critical periods can be defined, either in terms of parental information or offspring expression.
(2) Predator-induced TGP can persist for more than one generation (three generations on average in studies conducted over a sufficiently long time-frame), meaning that past environments may interact with each other and with offspring environmental conditions in shaping the anti-predator phenotype.
(3) The induction of TGP can depend on which parent (mother or father) has been exposed to predation risk, and its expression can be different in daughters and sons.
(4) While prey are often able to adjust their within-generational defenses to the level of predation risk, evidence of such scaling is lacking for TGP, but the number of relevant studies is still very limited.
Despite these general conclusions, we are only at the beginning of understanding the processes involved in predator-induced TGP. Indeed, most current studies only describe the existence of TGP in response to predation risk, while very few unravel the underlying processes. This makes TGP an exciting and challenging research topic for future studies. Such studies will be necessary (1) to add more examples of predator-induced TGP, (2) to study TGP in different offspring environments to account for the interplay between past and current predation risk, and (3) to disentangle the complexity of TGP (i.e., dissect processes and underlying mechanisms of induction and expression) in order to evaluate its adaptive value and its ecological and evolutionary impacts in predator-prey interactions. A relevant perspective would be to consider implications of TGP at the population level. Particularly, how predator-induced TGP may buffer the top-down effects of predators on prey population size, allowing better prediction of population dynamics of predator and prey and dynamics of food webs in general.
Author Contributions
All authors have contributed equally to the work, and approved it for publication.
Funding
This work was performed within the framework of the EUR H2O’Lyon (ANR-17-EURE-0018) of Université de Lyon (UdL), within the program “Investissements d’Avenir” operated by the French National Research Agency (ANR).
Conflict of Interest
The authors declare that the research was conducted in the absence of any commercial or financial relationships that could be construed as a potential conflict of interest.
Acknowledgments
We would like to thank Michael Kopp and Andreas Walzer for their helpful and thorough comments on our manuscript.
References
Agrawal, A. A. (2001). Phenotypic plasticity in the interactions and evolution of species. Science 294, 321–326. doi: 10.1126/science.1060701
Agrawal, A. A., Laforsch, C., and Tollrian, R. (1999). Transgenerational induction of defences in animals and plants. Nature 401, 60–63. doi: 10.1038/43425
Auge, G. A., Leverett, L. D., Edwards, B. R., and Donohue, K. (2017). Adjusting phenotypes via within- and across-generational plasticity. New Phytol. 216, 343–349. doi: 10.1111/nph.14495
Auld, J. R., Agrawal, A. A., and Relyea, R. A. (2010). Re-evaluating the costs and limits of adaptive phenotypic plasticity. Proc. R. Soc. B Biol. Sci. 277, 503–511. doi: 10.1098/rspb.2009.1355
Auld, J. R., and Relyea, R. A. (2011). Adaptive plasticity in predator-induced defenses in a common freshwater snail: altered selection and mode of predation due to prey phenotype. Evol. Ecol. 25, 189–202. doi: 10.1007/s10682-010-9394-1
Basso, A., Coslovsky, M., and Richner, H. (2014). Parasite- and predator-induced maternal effects in the great tit (Parus major). Behav. Ecol. 25, 1105–1114. doi: 10.1093/beheco/aru088
Basso, A., and Richner, H. (2015a). Effects of nest predation risk on female incubation behavior and offspring growth in great tits. Behav. Ecol. Sociobiol. 69, 977–989. doi: 10.1007/s00265-015-1910-4
Basso, A., and Richner, H. (2015b). Predator-specific effects on incubation behaviour and offspring growth in great tits. PLoS One 10:e0121088. doi: 10.1371/journal.pone.0121088
Beaman, J. E., White, C. R., and Seebacher, F. (2016). Evolution of plasticity: mechanistic link between development and reversible acclimation. Trends Ecol. Evol. 31, 237–249. doi: 10.1016/j.tree.2016.01.004
Beaty, L. E., Wormington, J. D., Kensinger, B. J., Bayley, K. N., Goeppner, S. R., Gustafson, K. D., et al. (2016). Shaped by the past, acting in the present: transgenerational plasticity of anti-predatory traits. Oikos 125, 1570–1576. doi: 10.1111/oik.03114
Bell, A. M., and Hellmann, J. (2019). An integrative framework for understanding the mechanisms and multigenerational consequences of transgenerational plasticity. Annu. Rev. Ecol. Evol. Syst. 50, 97–118. doi: 10.1146/annurev-ecolsys-110218-024613
Bestion, E., Teyssier, A., Aubret, F., Clobert, J., and Cote, J. (2014). Maternal exposure to predator scents: offspring phenotypic adjustment and dispersal. Proc. R. Soc. B Biol. Sci. 281:20140701. doi: 10.1098/rspb.2014.0701
Bonduriansky, R., and Head, M. (2007). Maternal and paternal condition effects on offspring phenotype in Telostylinus angusticollis (Diptera: Neriidae). J. Evol. Biol. 20, 2379–2388. doi: 10.1111/j.1420-9101.2007.01419.x
Boškoviæ, A., and Rando, O. J. (2018). Transgenerational Epigenetic Inheritance. Annu. Rev. Genet. 52, 21–41. doi: 10.1146/annurev-genet-120417-031404
Burke, N. W., Nakagawa, S., and Bonduriansky, R. (2019). Sexual conflict explains diverse patterns of transgenerational plasticity. bioRxiv [Preprint]. doi: 10.1101/846287
Burton, T., and Metcalfe, N. B. (2014). Can environmental conditions experienced in early life influence future generations? Proc. R. Soc. B Biol. Sci. 281:20140311. doi: 10.1098/rspb.2014.0311
Christe, P., Keller, L., and Roulin, A. (2006). The predation cost of being a male: implications for sex-specific rates of ageing. Oikos 114, 381–384. doi: 10.1111/j.2006.0030-1299.15130.x
Coslovsky, M., and Richner, H. (2011). Predation risk affects offspring growth via maternal effects: maternal effects due to predation risk. Funct. Ecol. 25, 878–888. doi: 10.1111/j.1365-2435.2011.01834.x
Coslovsky, M., and Richner, H. (2012). Preparing offspring for a dangerous world: potential costs of being wrong. PLoS One 7:e48840. doi: 10.1371/journal.pone.0048840
Coutellier, L., and Würbel, H. (2009). Early environmental cues affect object recognition memory in adult female but not male C57BL/6 mice. Behav. Brain Res. 203, 312–315. doi: 10.1016/j.bbr.2009.05.001
Crean, A. J., and Bonduriansky, R. (2014). What is a paternal effect? Trends Ecol. Evol. 29, 554–559. doi: 10.1016/j.tree.2014.07.009
de Vries, H., and MacDougal, D. T. (1905). Species and Varieties, Their Origin by Mutation: Lectures Delivered at the University of California. Chicago, IL: The Open court publishing company.
DeWitt, T. J., Sih, A., and Wilson, D. S. (1998). Costs and limits of phenotypic plasticity. Trends Ecol. Evol. 13, 77–81. doi: 10.1016/S0169-5347(97)01274-3
Dingemanse, N. J., and Wolf, M. (2013). Between-individual differences in behavioural plasticity within populations: causes and consequences. Anim. Behav. 85, 1031–1039. doi: 10.1016/j.anbehav.2012.12.032
Dixon, A. F. G., and Agarwala, B. K. (1999). Ladybird-induced life–history changes in aphids. Proc. R. Soc. Lond. B Biol. Sci. 266, 1549–1553. doi: 10.1098/rspb.1999.0814
Donelan, S. C., Hellmann, J. K., Bell, A. M., Luttbeg, B., Sheriff, M. J., and Sih, A. (2020). Transgenerational plasticity in human-altered environments. Trends Ecol. Evol. 35, 115–124. doi: 10.1016/j.tree.2019.09.003
Donelan, S. C., and Trussell, G. C. (2015). Parental effects enhance risk tolerance and performance in offspring. Ecology 96, 2049–2055. doi: 10.1890/14-1773.1
Donelan, S. C., and Trussell, G. C. (2018a). Parental and embryonic experiences with predation risk affect prey offspring behaviour and performance. Proc. R. Soc. B Biol. Sci. 285:20180034. doi: 10.1098/rspb.2018.0034
Donelan, S. C., and Trussell, G. C. (2018b). Synergistic effects of parental and embryonic exposure to predation risk on prey offspring size at emergence. Ecology 99, 68–78. doi: 10.1002/ecy.2067
Donelson, J. M., Salinas, S., Munday, P. L., and Shama, L. N. S. (2018). Transgenerational plasticity and climate change experiments: where do we go from here? Glob. Change Biol. 24, 13–34. doi: 10.1111/gcb.13903
Duempelmann, L., Skribbe, M., and Bühler, M. (2020). Small RNAs in the transgenerational inheritance of epigenetic information. Trends Genet. 36, 203–214. doi: 10.1016/j.tig.2019.12.001
Dzialowski, A. R., Lennon, J. T., O’Brien, W. J., and Smith, V. H. (2003). Predator-induced phenotypic plasticity in the exotic cladoceran Daphnia lumholtzi. Freshw. Biol. 48, 1593–1602. doi: 10.1046/j.1365-2427.2003.01111.x
Eggers, S., Griesser, M., Nystrand, M., and Ekman, J. (2006). Predation risk induces changes in nest-site selection and clutch size in the Siberian jay. Proc. R. Soc. B Biol. Sci. 273, 701–706. doi: 10.1098/rspb.2005.3373
Evans, J. P., Gasparini, C., and Pilastro, A. (2007). Female guppies shorten brood retention in response to predator cues. Behav. Ecol. Sociobiol. 61, 719–727. doi: 10.1007/s00265-006-0302-1
Ezard, T. H. G., Prizak, R., and Hoyle, R. B. (2014). The fitness costs of adaptation via phenotypic plasticity and maternal effects. Funct. Ecol. 28, 693–701. doi: 10.1111/1365-2435.12207
Fallet, M., Luquet, E., David, P., and Cosseau, C. (2020). Epigenetic inheritance and intergenerational effects in mollusks. Gene 729:144166. doi: 10.1016/j.gene.2019.144166
Fawcett, T. W., and Frankenhuis, W. E. (2015). Adaptive explanations for sensitive windows in development. Front. Zool. 12:S3. doi: 10.1186/1742-9994-12-S1-S3
Freinschlag, J., and Schausberger, P. (2016). Predation risk-mediated maternal effects in the two-spotted spider mite, Tetranychus urticae. Exp. Appl. Acarol. 69, 35–47. doi: 10.1007/s10493-016-0014-9
Ghalambor, C., Angeloni, L. M., and Carroll, S. (2010). “Behavior as phenotypic plasticity,” in Evolutionary Behavioral Ecology, eds C. Fox and D. Westneat (Oxford: Oxford University Press), 90–107.
Giesing, E. R., Suski, C. D., Warner, R. E., and Bell, A. M. (2011). Female sticklebacks transfer information via eggs: effects of maternal experience with predators on offspring. Proc. R. Soc. B Biol. Sci. 278, 1753–1759. doi: 10.1098/rspb.2010.1819
Gilbert, J. J. (1966). Rotifer ecology and embryological induction. Science 151, 1234–1237. doi: 10.1126/science.151.3715.1234
Glover, V., and Hill, J. (2012). Sex differences in the programming effects of prenatal stress on psychopathology and stress responses: An evolutionary perspective. Physiol. Behav. 106, 736–740. doi: 10.1016/j.physbeh.2012.02.011
Goeppner, S. R., Roberts, M. E., Beaty, L. E., and Luttbeg, B. (2020). Freshwater snail responses to fish predation integrate phenotypic plasticity and local adaptation. Aquat. Ecol. 54, 309–322. doi: 10.1007/s10452-019-09744-x
Guillaume, A. S., Monro, K., and Marshall, D. J. (2016). Transgenerational plasticity and environmental stress: do paternal effects act as a conduit or a buffer? Funct. Ecol. 30, 1175–1184. doi: 10.1111/1365-2435.12604
Hales, N. R., Schield, D. R., Andrew, A. L., Card, D. C., Walsh, M. R., and Castoe, T. A. (2017). Contrasting gene expression programs correspond with predator-induced phenotypic plasticity within and across generations in Daphnia. Mol. Ecol. 26, 5003–5015. doi: 10.1111/mec.14213
Harvell, C. D. (1990). The ecology and evolution of inducible defenses. Q. Rev. Biol. 65, 323–340. doi: 10.1086/416841
Hellmann, J. K., Bukhari, S. A., Deno, J., and Bell, A. M. (2019). Sex-specific transgenerational plasticity in threespined sticklebacks. bioRxiv [Preprint]. doi: 10.1101/763862
Hellmann, J. K., Carlson, E. R., and Bell, A. M. (2020). Sex-specific transgenerational plasticity II: Grandpaternal effects are lineage- and sex-specific in threespined sticklebacks. bioRxiv 796995. doi: 10.1101/796995
Herzog, Q., and Laforsch, C. (2013). Modality matters for the expression of inducible defenses: introducing a concept of predator modality. BMC Biol. 11:113. doi: 10.1186/1741-7007-11-113
Hunter, L. T. B., and Skinner, J. D. (1998). Vigilance behaviour in african ungulates: the role of predation pressure. Behaviour 135, 195–211. doi: 10.1163/156853998793066320
Jacobs, J. (1967). Untersuchungen zur Funktion and Evolution der Zyklomorphose bei Daphnia, mit besonderer Berucksichtigung der Selecktion durch Fischc. Arch. Hydrobiol. 62, 467–541.
Keiser, C. N., and Mondor, E. B. (2013). Transgenerational behavioral plasticity in a parthenogenetic insect in response to increased predation risk. J. Insect Behav. 26, 603–613. doi: 10.1007/s10905-013-9376-6
Kishida, O., Trussell, G. C., Mougi, A., and Nishimura, K. (2010). Evolutionary ecology of inducible morphological plasticity in predator–prey interaction: toward the practical links with population ecology. Popul. Ecol. 52, 37–46. doi: 10.1007/s10144-009-0182-0
Kopp, M., and Tollrian, R. (2003). Reciprocal phenotypic plasticity in a predator–prey system: inducible offences against inducible defences? Ecol. Lett. 6, 742–748. doi: 10.1046/j.1461-0248.2003.00485.x
Kuijper, B., and Hoyle, R. B. (2015). When to rely on maternal effects and when on phenotypic plasticity? Evolution 69, 950–968. doi: 10.1111/evo.12635
Kunert, G., and Weisser, W. W. (2003). The interplay between density- and trait-mediated effects in predator-prey interactions: a case study in aphid wing polymorphism. Oecologia 135, 304–312. doi: 10.1007/s00442-003-1185-8
Kunert, G., and Weisser, W. W. (2005). The importance of antennae for pea aphid wing induction in the presence of natural enemies. Bull. Entomol. Res. 95, 125–131. doi: 10.1079/BER2004342
Laurila, A., Järvi-Laturi, M., Pakkasmaa, S., and Merilä, J. (2004). Temporal variation in predation risk: stage-dependency, graded responses and fitness costs in tadpole antipredator defences. Oikos 107, 90–99. doi: 10.1111/j.0030-1299.2004.13126.x
Lehto, W. R., and Tinghitella, R. M. (2019). Joint maternal and paternal stress increases the cortisol in their daughters’ eggs. Evol. Ecol. Res. 20, 133–144.
Leimar, O., and McNamara, J. M. (2015). The evolution of transgenerational integration of information in heterogeneous environments. Am. Nat. 185, E55–E69. doi: 10.1086/679575
Li, D., and Lee, W. S. (2004). Predator-induced plasticity in web-building behaviour. Anim. Behav. 67, 309–318. doi: 10.1016/j.anbehav.2003.06.011
Li, G.-Y., and Zhang, Z.-Q. (2019). Development, lifespan and reproduction of spider mites exposed to predator-induced stress across generations. Biogerontology 20, 871–882. doi: 10.1007/s10522-019-09835-0
Lima, S. L. (1998). Nonlethal effects in the ecology of predator-prey interactions. what are the ecological effects of anti-predator decision-making? Bioscience 48, 25–34. doi: 10.2307/1313225
Lively, C. M. (1986). Predator-induced shell dimorphism in the acorn barnacle chthamalus anisopoma. Evolution 40, 232–242. doi: 10.1111/j.1558-5646.1986.tb00466.x
Luquet, E., and Tariel, J. (2016). Offspring reaction norms shaped by parental environment: interaction between within- and trans-generational plasticity of inducible defenses. BMC Evol. Biol. 16:209. doi: 10.1186/s12862-016-0795-9
McGhee, K. E., and Bell, A. M. (2014). Paternal care in a fish: epigenetics and fitness enhancing effects on offspring anxiety. Proc. R. Soc. B Biol. Sci. 281:20141146. doi: 10.1098/rspb.2014.1146
McGhee, K. E., Pintor, L. M., Suhr, E. L., and Bell, A. M. (2012). Maternal exposure to predation risk decreases offspring antipredator behaviour and survival in threespined stickleback. Funct. Ecol. 26, 932–940. doi: 10.1111/j.1365-2435.2012.02008.x
Meuthen, D., Baldauf, S. A., Bakker, T. C. M., and Thünken, T. (2018). Neglected patterns of variation in phenotypic plasticity: age- and sex-specific antipredator plasticity in a cichlid fish. Am. Nat. 191, 475–490. doi: 10.1086/696264
Mikulski, A., and Pijanowska, J. (2010). When and how can Daphnia prepare their offspring for the threat of predation? Hydrobiologia 643, 21–26. doi: 10.1007/s10750-010-0131-0
Mitchell, M. D., Bairos-Novak, K. R., and Ferrari, M. C. O. (2017). Mechanisms underlying the control of responses to predator odours in aquatic prey. J. Exp. Biol. 220, 1937–1946. doi: 10.1242/jeb.135137
Miyakawa, H., Imai, M., Sugimoto, N., Ishikawa, Y., Ishikawa, A., Ishigaki, H., et al. (2010). Gene up-regulation in response to predator kairomones in the water flea, Daphnia pulex. BMC Dev. Biol. 10:45. doi: 10.1186/1471-213X-10-45
Mommer, B. C., and Bell, A. M. (2013). A test of maternal programming of offspring stress response to predation risk in threespine sticklebacks. Physiol. Behav. 122, 222–227. doi: 10.1016/j.physbeh.2013.04.004
Mondor, E. B., Rosenheim, J. A., and Addicott, J. F. (2005). Predator-induced transgenerational phenotypic plasticity in the cotton aphid. Oecologia 142, 104–108. doi: 10.1007/s00442-004-1710-4
Monteforte, S., Cattelan, S., Morosinotto, C., Pilastro, A., and Grapputo, A. (2020). Maternal predator-exposure affects offspring size at birth but not telomere length in a live-bearing fish. Ecol. Evol. 10, 2030–2039. doi: 10.1002/ece3.6035
Morales, J., Lucas, A., and Velando, A. (2018). Maternal programming of offspring antipredator behavior in a seabird. Behav. Ecol. 29, 479–485. doi: 10.1093/beheco/arx197
Murren, C. J., Auld, J. R., Callahan, H., Ghalambor, C. K., Handelsman, C. A., Heskel, M. A., et al. (2015). Constraints on the evolution of phenotypic plasticity: limits and costs of phenotype and plasticity. Heredity 115, 293–301. doi: 10.1038/hdy.2015.8
Nicoglou, A. (2018). “The concept of plasticity in the history of the nature-nurture debate in the early twentieth century,” in The Palgrave Handbook of Biology and Society, eds M. Meloni, J. Cromby, D. Fitzgerald, and S. Lloyd (London: Palgrave Macmillan), 97–122. doi: 10.1057/978-1-137-52879-7_5
Norouzitallab, P., Baruah, K., Vanrompay, D., and Bossier, P. (2019). Can epigenetics translate environmental cues into phenotypes? Sci. Total Environ. 647, 1281–1293. doi: 10.1016/j.scitotenv.2018.08.063
Padilla, D. (2001). Food and environmental cues trigger an inducible offence. Evol. Ecol. Res. 3, 15–25.
Piersma, T., and Drent, J. (2003). Phenotypic flexibility and the evolution of organismal design. Trends Ecol. Evol. 18, 228–233. doi: 10.1016/S0169-5347(03)00036-3
Pigliucci, M. (2005). Evolution of phenotypic plasticity: Where are we going now? Trends Ecol. Evol. 20, 481–486. doi: 10.1016/j.tree.2005.06.001
Plautz, S. C., Guest, T., Funkhouser, M. A., and Salice, C. J. (2013). Transgenerational cross-tolerance to stress: parental exposure to predators increases offspring contaminant tolerance. Ecotoxicology 22, 854–861. doi: 10.1007/s10646-013-1056-y
Podjasek, J. O., Bosnjak, L. M., Brooker, D. J., and Mondor, E. B. (2005). Alarm pheromone induces a transgenerational wing polyphenism in the pea aphid, Acyrthosiphon pisum. Can. J. Zool. 83, 1138–1141. doi: 10.1139/z05-108
Prizak, R., Ezard, T. H. G., and Hoyle, R. B. (2014). Fitness consequences of maternal and grandmaternal effects. Ecol. Evol. 4, 3139–3145. doi: 10.1002/ece3.1150
Riessen, H. P. (1999). Predator-induced life history shifts in Daphnia: a synthesis of studies using meta-analysis. Can. J. Fish. Aquat. Sci. 56, 2487–2494. doi: 10.1139/f99-155
Roche, D. P., McGhee, K. E., and Bell, A. M. (2012). Maternal predator-exposure has lifelong consequences for offspring learning in threespined sticklebacks. Biol. Lett. 8, 932–935. doi: 10.1098/rsbl.2012.0685
Salinas, S., Brown, S. C., Mangel, M., and Munch, S. B. (2013). Non-genetic inheritance and changing environments. Non Genet. Inherit. 1, 38–50. doi: 10.2478/ngi-2013-0005
Schield, D. R., Walsh, M. R., Card, D. C., Andrew, A. L., Adams, R. H., and Castoe, T. A. (2016). EpiRADseq: scalable analysis of genomewide patterns of methylation using next-generation sequencing. Methods Ecol. Evol. 7, 60–69. doi: 10.1111/2041-210X.12435
Segers, F. H. I. D., Gerber, B., and Taborsky, B. (2011). Do maternal food deprivation and offspring predator cues interactively affect maternal effort in fish? Ethology 117, 708–721. doi: 10.1111/j.1439-0310.2011.01922.x
Segers, F. H. I. D., and Taborsky, B. (2012). Juvenile exposure to predator cues induces a larger egg size in fish. Proc. R. Soc. B Biol. Sci. 279, 1241–1248. doi: 10.1098/rspb.2011.1290
Seiter, M., and Schausberger, P. (2015). Maternal intraguild predation risk affects offspring anti-predator behavior and learning in mites. Sci. Rep. 5:15046. doi: 10.1038/srep15046
Sentis, A., Bertram, R., Dardenne, N., Ramon-Portugal, F., Espinasse, G., Louit, I., et al. (2018). Evolution without standing genetic variation: change in transgenerational plastic response under persistent predation pressure. Heredity 121, 266–281. doi: 10.1038/s41437-018-0108-8
Sentis, A., Bertram, R., Dardenne, N., Ramon-Portugal, F., Louit, I., Le Trionnaire, G., et al. (2019). Different phenotypic plastic responses to predators observed among aphid lineages specialized on different host plants. Sci. Rep. 9:9017. doi: 10.1038/s41598-019-45220-0
Sentis, A., Hemptinne, J.-L., and Brodeur, J. (2017). Non-additive effects of simulated heat waves and predators on prey phenotype and transgenerational phenotypic plasticity. Glob. Change Biol. 23, 4598–4608. doi: 10.1111/gcb.13674
Sheriff, M. J., Krebs, C. J., and Boonstra, R. (2009). The sensitive hare: sublethal effects of predator stress on reproduction in snowshoe hares. J. Anim. Ecol. 78, 1249–1258. doi: 10.1111/j.1365-2656.2009.01552.x
Sheriff, M. J., Krebs, C. J., and Boonstra, R. (2010). The ghosts of predators past: population cycles and the role of maternal programming under fluctuating predation risk. Ecology 91, 2983–2994. doi: 10.1890/09-1108.1
Shine, R., and Downes, S. J. (1999). Can pregnant lizards adjust their offspring phenotypes to environmental conditions? Oecologia 119, 1–8. doi: 10.1007/s004420050754
Sih, A., Ziemba, R., and Harding, K. C. (2000). New insights on how temporal variation in predation risk shapes prey behavior. Trends Ecol. Evol. 15, 3–4. doi: 10.1016/S0169-5347(99)01766-8
Sogard, S. M. (1997). Size-selective mortality in the juvenile stage of teleost fishes: a review. Bull. Mar. Sci. 60, 1129–1157.
St-Cyr, S., and McGowan, P. O. (2015). Programming of stress-related behavior and epigenetic neural gene regulation in mice offspring through maternal exposure to predator odor. Front. Behav. Neurosci. 9:145. doi: 10.3389/fnbeh.2015.00145
Stein, L. R., and Bell, A. M. (2014). Paternal programming in sticklebacks. Anim. Behav. 95, 165–171. doi: 10.1016/j.anbehav.2014.07.010
Stein, L. R., Bukhari, S. A., and Bell, A. M. (2018). Personal and transgenerational cues are nonadditive at the phenotypic and molecular level. Nat. Ecol. Evol. 2, 1306–1311. doi: 10.1038/s41559-018-0605-4
Steiner, U. K., and Pfeiffer, T. (2007). Optimizing time and resource allocation trade-offs for investment into morphological and behavioral defense. Am. Nat. 169, 118–129. doi: 10.1086/509939
Storm, J. J., and Lima, S. L. (2010). Mothers forewarn offspring about predators: a transgenerational maternal effect on behavior. Am. Nat. 175, 382–390. doi: 10.1086/650443
Stratmann, A., and Taborsky, B. (2014). Antipredator defences of young are independently determined by genetic inheritance, maternal effects and own early experience in mouthbrooding cichlids. Funct. Ecol. 28, 944–953. doi: 10.1111/1365-2435.12224
Svanbäck, R., Pineda-Krch, M., and Doebeli, M. (2009). Fluctuating population dynamics promotes the evolution of phenotypic plasticity. Am. Nat 174, 176–189. doi: 10.1086/600112
Tariel, J., Plénet, S., and Luquet, É (2019). How past exposure to predation affects personality and behavioural plasticity? bioRxiv [Preprint]. doi: 10.1101/844258
Tariel, J., Plénet, S., and Luquet, É (2020). Transgenerational plasticity of inducible defences: combined effects of grand-parental, parental and current environments. Ecol. Evol. 10, 2367–2376. doi: 10.1002/ece3.6046
Teplitsky, C., Plenet, S., Léna, J.-P., Mermet, N., Malet, E., and Joly, P. (2005). Escape behaviour and ultimate causes of specific induced defences in an anuran tadpole. J. Evol. Biol. 18, 180–190. doi: 10.1111/j.1420-9101.2004.00790.x
Tigreros, N., Norris, R. H., and Thaler, J. S. (2019). Maternal effects across life stages: larvae experiencing predation risk increase offspring provisioning. Ecol. Entomol. 44, 738–744. doi: 10.1111/een.12752
Tollrian, R. (1993). Neckteeth formation in Daphnia pulex as an example of continuous phenotypic plasticity: morphological effects of Chaoborus kairomone concentration and their quantification. J. Plankton Res. 15, 1309–1318. doi: 10.1093/plankt/15.11.1309
Tollrian, R. (1995). Predator-induced morphological defenses: costs, life history shifts, and maternal effects in Daphnia Pulex. Ecology 76, 1691–1705. doi: 10.2307/1940703
Tollrian, R., Duggen, S., Weiss, L. C., Laforsch, C., and Kopp, M. (2015). Density-dependent adjustment of inducible defenses. Sci. Rep. 5:12736. doi: 10.1038/srep12736
Tollrian, R., and Harvell, C. D. (eds) (1999). The Ecology and Evolution of Inducible Defenses. Princeton, NJ: Princeton University Press.
Tollrian, R., and Leese, F. (2010). Ecological genomics: steps towards unraveling the genetic basis of inducible defenses in Daphnia. BMC Biol. 8:51. doi: 10.1186/1741-7007-8-51
Torres-Dowdall, J., Handelsman, C. A., Reznick, D. N., and Ghalambor, C. K. (2012). Local adaptation and the evolution of phenotypic plasticity in Trinidadian Guppies (poecilia Reticulata). Evolution 66, 3432–3443. doi: 10.1111/j.1558-5646.2012.01694.x
Triggs, A. M., and Knell, R. J. (2012). Parental diet has strong transgenerational effects on offspring immunity. Funct. Ecol. 26, 1409–1417. doi: 10.1111/j.1365-2435.2012.02051.x
Uller, T. (2008). Developmental plasticity and the evolution of parental effects. Trends Ecol. Evol. 23, 432–438. doi: 10.1016/j.tree.2008.04.005
Van Buskirk, J. (2002). A Comparative test of the adaptive plasticity hypothesis: relationships between habitat and phenotype in anuran larvae. Am. Nat. 160, 87–102. doi: 10.1086/340599
Van Buskirk, J., and Arioli, M. (2002). Dosage response of an induced defense: how sensitive are tadpoles to predation risk? Ecology 83, 1580–1585. doi: 10.2307/3071977
Walsh, M. R., Castoe, T., Holmes, J., Packer, M., Biles, K., Walsh, M., et al. (2016). Local adaptation in transgenerational responses to predators. Proc. R. Soc. B Biol. Sci. 283:20152271. doi: 10.1098/rspb.2015.2271
Walsh, M. R., Cooley, F., Biles, K., and Munch, S. B. (2015). Predator-induced phenotypic plasticity within- and across-generations: a challenge for theory? Proc. R. Soc. B Biol. Sci. 282:20142205. doi: 10.1098/rspb.2014.2205
Weisser, W. W., Braendle, C., and Minoretti, N. (1999). Predator-induced morphological shift in the pea aphid. Proc. R. Soc. Lond. B Biol. Sci. 266, 1175–1181. doi: 10.1098/rspb.1999.0760
West-Eberhard, M. J. (2003). Developmental Plasticity and Evolution. Oxford: Oxford University Press.
Woltereck, R. (1909). Weitere experimentelle Untersuchungen über Artveränderung, speziell über das Wesen quantitativer Artunterschiede bei Daphniden. Vers. Dtsch. Zool. Geselleschaft 110–172.
Yin, J., Zhou, M., Lin, Z., Li, Q. Q., and Zhang, Y. (2019). Transgenerational effects benefit offspring across diverse environments: a meta-analysis in plants and animals. Ecol. Lett. 22, 1976–1986. doi: 10.1111/ele.13373
Keywords: non-genetic inheritance, inducible defense, intergenerational plasticity, parental effects, sex dependence, sensitive windows
Citation: Tariel J, Plénet S and Luquet É (2020) Transgenerational Plasticity in the Context of Predator-Prey Interactions. Front. Ecol. Evol. 8:548660. doi: 10.3389/fevo.2020.548660
Received: 03 April 2020; Accepted: 03 September 2020;
Published: 25 September 2020.
Edited by:
Olivia Roth, GEOMAR Helmholtz Center for Ocean Research Kiel, GermanyReviewed by:
Michael Kopp, Aix-Marseille Université, FranceAndreas Walzer, University of Natural Resources and Life Sciences Vienna, Austria
Copyright © 2020 Tariel, Plénet and Luquet. This is an open-access article distributed under the terms of the Creative Commons Attribution License (CC BY). The use, distribution or reproduction in other forums is permitted, provided the original author(s) and the copyright owner(s) are credited and that the original publication in this journal is cited, in accordance with accepted academic practice. No use, distribution or reproduction is permitted which does not comply with these terms.
*Correspondence: Juliette Tariel, anVsaWV0dGV0YXJpZWxAZ21haWwuY29t; Émilien Luquet, ZW1pbGllbi5sdXF1ZXRAdW5pdi1seW9uMS5mcg==
†These authors have contributed equally to this work