- Department of Animal and Plant Sciences, University of Sheffield, Sheffield, United Kingdom
The timing of breeding often has a profound influence on the reproductive success of birds living in seasonal environments with rapidly changing nestling food availability. Timing is typically investigated with reference to lay dates, but it is the time of hatching that determines the ambient conditions and food availability that nestlings experience. Thus, in addition to lay date, phenological studies may also have to take account of variation in the length of the incubation period, which is likely to depend on both environmental conditions and parental traits. The primary aim of this study was to use a 24-year dataset to investigate the abiotic and biotic factors influencing variation in incubation duration in long-tailed tits (Aegithalos caudatus), a species in which incubation duration varies substantially (range: 12–26 days). We found support for our predictions that drier conditions, later breeding attempts and larger clutches were associated with shorter incubation periods. Larger clutches were also more resilient to increases in incubation duration associated with wet conditions. Surprisingly, warmer ambient conditions were associated with longer incubation periods. Secondly, we assessed the consequences of variation in the length of incubation periods for the risk of nest predation and the hatching success of surviving clutches. We show that longer incubation periods are likely to be costly due to increased exposure to nest predators. In contrast, we found only marginal effects of environmental conditions or incubation duration on hatching success, implying that wet conditions cause slower embryo growth and hence longer incubation periods, rather than causing embryo fatality. We suggest that long-tailed tits’ nests and parental behavior protect eggs from mortality arising directly from adverse weather conditions.
Introduction
Reproductive success of birds is often dependent upon timing of breeding (Daan et al., 1997; Houston and McNamara, 1999), with individuals that breed earlier in the season typically having higher fitness (Perrins, 1970; Both, 2010). For many bird species living in seasonal environments, it is critical for reproduction to be timed to match temporally ephemeral food resources, with hatching expected to coincide with the peak of food abundance (Perrins, 1970; Monros et al., 1998; Naef-Daenzer et al., 2004; Simmonds et al., 2017). A large number of studies have focused on variation in clutch initiation date (e.g., Visser et al., 1998; Charmantier et al., 2008; Schaper et al., 2012), but other mechanisms for altering the timing of hatching exist. Females may adjust the length of the egg-laying period by altering clutch size, or by increasing the intervals between laying (Haftorn, 1981; Nilsson and Svensson, 1993; Simmonds et al., 2017). Alternatively, the length of the incubation period could be adjusted by starting incubation prior to clutch completion or by delaying the start of incubation (Haftorn, 1981; García-Navas and Sanz, 2011; Nord and Nilsson, 2011; Álvarez and Barba, 2014; Simmonds et al., 2017), and by changing nest attentiveness once incubation has started (Martin et al., 2007; MacDonald et al., 2013; Coe et al., 2015).
Incubation is typically an energy- and time-consuming component of avian reproduction (Tatner and Bryant, 1993; Williams, 1996; DuRant et al., 2013a; Nord and Williams, 2015) due to the relatively high temperatures (34–40°C) at which eggs must be maintained to ensure optimal embryonic development (Webb, 1987; DuRant et al., 2013b). Investment in incubation reduces the energy and time available for parents to spend on self-maintenance (Stearns, 1989; Reznick et al., 2000; Zera and Harshman, 2001), especially in species in which one parent is solely responsible for incubation (Deeming, 2002). Consequently, incubation can reduce parental body condition (Tombre and Erikstad, 1996; Hanssen et al., 2005) and immune function (Knowles et al., 2009), thereby lowering fitness by reducing adult survival (Visser and Lessells, 2001) and future reproductive success (Reid et al., 2000a; Hanssen et al., 2005).
The ability of incubating parents to maintain suitable conditions for embryo development may also be affected by environmental factors. For example, lower ambient temperatures can alter the nest microclimate, causing eggs to cool at a faster rate and reach lower temperatures when left unattended (Reid et al., 2000b). This means that an incubating parent must expend more energy re-heating cooled eggs and maintaining eggs at the optimal temperature (Jarvinen, 1993; Sheaffer and Malecki, 1996; Skinner et al., 1998). Eggs that experience low or fluctuating temperatures not only suffer from reduced hatching success, but also from slower embryonic growth (Olson et al., 2006), production of poorer quality offspring (Nord and Nilsson, 2011; DuRant et al., 2013a) and reduced long-term survival of offspring (Berntsen and Bech, 2016). In addition, heavy rain also leads to reduced hatching success and nest failure in some passerine species (Wesołowski et al., 2002; Martin et al., 2017), an effect that has been attributed to enhanced heat loss from eggs via increased conductance and reduced insulating properties of damp nest materials (Reid et al., 2002; Hilton et al., 2004; Heenan, 2013). Thus adverse weather conditions can have profound consequences for both the length of the incubation period and hatching success.
However, there are ways in which negative environmental impacts may be mitigated, such as by building well-insulated nests (Deeming and Gray, 2016), or by increasing incubation attendance when temperatures are lower (Conway and Martin, 2000; Amininasab et al., 2016). In addition, clutches of different sizes have different thermal properties; the thermal inertia hypothesis predicting that larger clutches should cool more slowly, while also taking longer to re-heat (Reid et al., 2000b; Cooper, 2005). This hypothesis is supported by the observation that large clutches in tree swallows Tachycineta bicolor have shorter incubation periods (Ardia et al., 2006), although most studies have found no effect of clutch size on the length of the incubation period (Székely et al., 1994; Siikamiiki, 1995; Sandercock, 1997; Reid et al., 2000a; Wiebe and Martin, 2000), suggesting that, in general, the thermal properties of the clutch do not have a significant effect on the duration of the incubation period.
The extent to which females can mitigate the negative impacts of climate may depend on their age (e.g., female age was positively correlated with nest temperature in blue tits Cyanistes caeruleus; Amininasab et al., 2016), or their own quality or body condition. For example, Ardia and Clotfelter (2007) found that young female tree swallows that had their feathers clipped to induce poor condition passed the costs of this on to their offspring through lower egg temperatures. Older females also suffered self-maintenance costs from this experimental treatment, but were able to maintain higher egg temperatures and produce higher quality offspring. These studies indicate that older females may be more willing to bear self-maintenance costs and not pass these on to their offspring.
We investigated the effects of timing of breeding and environmental conditions on incubation periods and hatching success in the long-tailed tit Aegithalos caudatus. Long-tailed tits build domed nests that require a large investment of time and energy (McGowan et al., 2004). The nest is constructed of moss and fibers bound together with spiders’ silk, covered on the outside with flakes of lichen and lined with up to 2,500 feathers, with a small entrance hole (Hansell, 1996; McGowan et al., 2004). Only females incubate the eggs, spending about 65% of daylight hours on the nest, while males bring food to incubating females on average twice an hour (Hatchwell et al., 1999). Long-tailed tits are facultative cooperative breeders, but helpers assist only in the feeding of nestlings and fledglings (Gaston, 1973; Hatchwell et al., 2004) and are not present during incubation. Long-tailed tits are well suited to this study because there is much natural variation in the length of time between their last egg being laid and the hatching of their eggs, henceforth referred to as the incubation period (range: 12 to 26 days), the causes and consequences of which are unknown. Also, there is a high rate of nest predation (72%; Gullett et al., 2013; Hatchwell et al., 2013), so longer incubation periods could significantly increase the risk of nest failure.
In this study, first we used a 24-year dataset to test whether incubation period was associated with weather variables (temperature and precipitation), timing of breeding (clutch completion date and attempt number), clutch size and female age. We predicted that incubation periods would: (i) be longer in cold and wet weather conditions; (ii) decrease later in the season and in replacement nests; and (iii) that clutch size would influence incubation duration – either increasing it for larger clutches because more energy is required for incubation, or decreasing it for larger clutch sizes because eggs retain more heat during female foraging bouts, as predicted by the thermal inertia hypothesis. We also predicted: (iv) that older females would be better incubators, thus reducing incubation period duration. Secondly, we tested whether environmental conditions or incubation period duration influenced hatching success. We predicted that cooler, wetter conditions and longer incubation periods would increase hatching failure rates because they were likely to be associated with lower egg temperatures that reduce embryo viability. Finally, we calculated the daily predation risk of nests during incubation to determine the consequences of variation in the length of the incubation period for the probability of nest failure.
Materials and Methods
Study System
Long-tailed tits were studied between 1995 and 2018 in the Rivelin Valley, Sheffield (53°23′N, 1°34′W). Each year the study site contained 25–72 pairs that had 33–114 monitored breeding attempts; c. 95% of breeders were ringed with a British Trust for Ornithology (BTO) ring and a unique combination of two color rings (under BTO license). Nestlings were ringed 11 days after hatching and unringed immigrants to the study site were captured using mist nets and ringed at the beginning of the breeding season.
Long-tailed tits are single-brooded, but breeding attempts frequently fail prior to fledging and if there is sufficient time remaining in the breeding season pairs will initiate another breeding attempt (MacColl and Hatchwell, 2002). The major reason for these failures is predation by both avian and mammalian predators (Hatchwell et al., 1999). Due to the high rate of breeding failure and to maximize the sample size for each analysis the sample sizes vary as follows. For this study, 540 breeding attempts that reached the incubation stage were monitored; of these, 372 clutches hatched and 230 broods survived until day 11 when nestlings were counted. Nests were routinely monitored every 2 days, and daily around the time of hatching, to obtain accurate reproductive parameters, i.e., first egg date, clutch size, hatching date, and either fledging or failure date. A very small percentage of nests, estimated to be <5%, are not found in each year but these undetected nesting attempts typically fail early in the breeding cycle (Sharp et al., 2008).
Measuring Incubation Period
We followed many previous studies by measuring incubation period as the number of days between clutch completion and hatching (Nilsson and Smith, 1988; Wiebe and Martin, 2000; Martin, 2002; Martin et al., 2007; Rohwer et al., 2015; Bueno-Enciso et al., 2017). Our procedure for determining incubation period was as follows. The date the first egg of each clutch was laid (hereafter referred to as first egg date) was recorded as a date within the year, where 1st March is set as day 1. Long-tailed tits lay a single egg per day, around dawn, and we counted the number of eggs in a clutch on or around the day the 12th egg would have been laid; this is the largest clutch size recorded in the 26 years of our study. From this we calculated the date of clutch completion. The assumption that females commenced incubation only after clutch completion is justified by observations that females do not start incubation until their last egg was laid (Glen, 1985; B. J. Hatchwell personal observation) and also by the observation that hatching is synchronous. We confirmed that short incubation periods were not a consequence of incubation starting prior to clutch completion by examining whether nestling size hierarchies were greater in broods with shorter incubation periods, which would be the expected result of early incubation and hence asynchronous hatching (Slagsvold et al., 1995; Stenning, 2008). We calculated asynchrony as the mass of the heaviest nestling in a brood minus the mass of the lightest nestling in a brood, divided by the mean mass of the brood (Kluen et al., 2011). There was no significant difference in the degree of asynchrony of broods in the lower (range: 12 to 15 days) and upper (range: 17 to 26 days) quartiles of incubation period (F(1,73) = 0.03, P = 0.20). Hatching date was recorded by checking nests at daily intervals from the earliest expected date of hatching; given that eggs could hatch shortly after one nest-check and hence up to 1 day before being checked again, hatch dates were accurate to within 1 day.
Our measure of incubation duration is thus dominated by the period during which females incubated eggs, but it also includes any delay in the start of incubation after laying the final egg. Such delays do sometimes occur in long-tailed tits, but it was not logistically feasible to routinely record the date on which females started incubation for all nests. However, we were able to estimate how frequent a delayed start to incubation was by noting whether the eggs were warm to the touch when eggs were counted 12 (n = 103), 11 (n = 35) or 10 (n = 22) days after the first egg date. For this sub-sample of breeding attempts, 8.1% (n = 160) of females had not begun incubation when the nest was checked. Of the remaining 147 breeding attempts we calculated the maximum potential delay in starting incubation as the difference between the day the last egg was laid and the day the eggs were counted. We then compared this to the delay in hatching (number of days beyond the shortest incubation in our study) and found that 87.1% of them had a greater delay in hatching than could be accounted for by simply delaying the start of incubation. This indicates that while some of the longer incubation periods may be due to delays in the start of incubation, most are indeed due to active incubation by females lasting longer. Moreover, given that any delay in the start of incubation may be attributable to poor weather (Rowe and Weatherhead, 2009), any effect would be consistent with our hypothesis that adverse environmental conditions prolong the incubation period and extend the period of predation risk.
To account for the considerable inter-annual variation in the timing of breeding caused by variable early spring temperatures (Gullett et al., 2013), we calculated relative first egg date and relative clutch completion date. For each breeding attempt, we calculated the number of days between the dates of these events for a given nest and the earliest first egg and earliest incubation start dates recorded in that year. Only breeding attempts for which first egg date, clutch size and hatch date information was available were used in analyses, leaving 372 breeding attempts by 289 females remaining in our dataset. The incubation period for these breeding attempts ranged from 12 to 26 days (mean ± SD = 16.4 days ± 1.56, median = 16 days). The dataset contained two outliers (incubation durations of 23 and 26 days) and analyses were conducted with and without outliers. We report analyses including outliers in the main text, but the results from analyses with and without outliers are reported in Supplementary Tables S1 and S2, respectively.
Measuring Hatching Success
Brood size when nestlings were 11 days old was used to estimate the number of eggs that had successfully hatched. Long-tailed tits have a low nestling mortality rate due to starvation between hatching and day 11 (2.3% of nestlings; Hatchwell et al., 2004), and partial nest predation is also rare. Thus, it is very likely that a small brood size on day 11 is due to hatching failure rather than nestling mortality; and this was confirmed in the majority of cases by the presence of unhatched but intact eggs in the nest on day 11. Hatching success was variable (range = 12.5–100%) but high on average (mean = 81.8%; median = 88.9%). Note that since some broods were completely depredated between hatching and day 11 our sample size for this analysis was smaller than for the incubation period study (n = 230).
Weather Data
Weather data for 1995–2018 were obtained from Weston Park Meteorological Station (Museums Sheffield, 2019), located 5 km east of the center of the study site and at a similar elevation (131 m above sea level compared with mean field site altitude of 168 m). Temperatures at the weather station are significantly positively correlated with those recorded at the field site (Gullett et al., 2014), and were used because on-site temperature data were not available for all years. Given strong spatial autocorrelation in precipitation patterns (Burton et al., 2013) the close proximity of the weather station to the study site also means that the difference in precipitation between the weather station and field site is minor (Gullett et al., 2014), although we have no direct measurements of precipitation from the field site to compare to the weather station data.
We calculated mean daily temperature, mean daily minimum temperature, mean daily maximum temperature, mean daily rainfall, and the proportion of rainy days during the incubation period of each nest (n = 372). Initially, we defined the proportion of rainy days in three ways: (i) the proportion of days with any rain (>0 mm), (ii) the proportion of days with >0.35 mm of rain (which excludes the least rainy 10% of days), and (iii) the proportion of days with >3 mm of rain (which excludes the least rainy 75% of days). However, mean daily rainfall during the incubation period, and all three measures of the proportion of rainy days were closely positively correlated (Pearson’s correlation: r ≥ 0.78, df = 370, P < 0.001 in all cases). Similarly, minimum and maximum temperatures were highly positively correlated with mean temperature (Pearson’s correlation: r ≥ 0.87, df = 370, P < 0.001 in both cases). Therefore, we used only the mean temperature and the proportion of rainy days (>0 mm) in our main statistical models. These variables were significantly negatively correlated (Pearson’s correlation: r = −0.47, df = 370, P < 0.001), but this collinearity was well within the threshold to which information theoretic approaches are robust (VIF < 2; Freckleton, 2011). The proportion of rainy days variable was used instead of mean rainfall because it provides a better indicator of daily rainfall patterns, which we considered more likely to affect the nest’s insulation quality throughout the incubation period than total rainfall. However, the analysis was also conducted using mean daily maximum temperature, mean daily minimum temperature, mean daily rainfall and the proportion of days with >3 mm of rain and the results were qualitatively similar (Supplementary Tables S3–S5).
Statistical Analysis
All statistical analyses were conducted in R 3.3.1 (R Core Team, 2016). We used an information theoretic approach to model selection and constructed all possible models given our predictor variables but retained year (as a random effect) in all models. We used Akaike’s Information Criterion corrected for small sample size (AICc) to compare model fit (Burnham and Anderson, 2002). We report the results of all models within 2 AICc points of the model with the lowest AICc value. This methodology allows us to compare all possible models and identify competing models that could equally well describe our data. To test how well the models fit our data, we used the MuMIn package (Barton, 2018) to calculate marginal and conditional pseudo-R2 using the methods described by Nakagawa and Schielzeth (2013). The model-averaged estimates (mean and 95% confidence intervals) were also calculated. Effects were considered statistically significant when the 95% confidence intervals for a parameter estimate did not span zero (Burnham and Anderson, 2002).
Factors Affecting Incubation Period
To investigate factors affecting incubation period we constructed restricted maximum likelihood linear mixed models using the lmer function in the lme4 package (Bates et al., 2015). Incubation period was modeled as a function of the proportion of rainy days, mean daily temperature, relative incubation start date, clutch size, attempt (whether it was a first or replacement nest as a binary factor) and female age (in years from ringing as a nestling for philopatric recruits and assuming that immigrant recruits were yearlings when first ringed (McGowan et al., 2003); year was included as a random factor. We also included six interactions, although only one interaction was ever present in any single model. The six interactions were between mean daily temperature and the proportion of rainy days, mean daily temperature and relative incubation start date, mean daily temperature and clutch size, proportion of rainy days and incubation period start date, proportion of rainy days and clutch size, and clutch size and incubation period start date. All continuous variables were scaled and centered. Female identity was not included in the models because 77.7% of the data points came from unique females and no single female contributed more than 1.36% of the data to the analysis. In addition, we separately investigated the repeatability of incubation period in females using the rptR package (Nakagawa and Schielzeth, 2010) and found that it was not repeatable (R ± SE = 0.00 ± 0.03, P = 1.00). Likewise, neither lay date (R ± SE = 0.02 ± 0.07, P = 0.41) nor clutch size (R ± SE = 0 ± 0.03, P = 1) were significantly repeatable for females.
Factors Affecting Hatching Success
We built generalized linear mixed-effects models with a binomial error structure and logit link of hatching success using the glmer function in the lme4 package (Bates et al., 2015). Hatching success was modeled as a function of incubation period, proportion of rainy days, mean daily temperature, incubation period start date, clutch size, attempt and female age (defined as above), including year as a random factor. In addition, we included the same six interactions as in the duration of incubation period models. All continuous variables were scaled and centered. Again, female identity was not used as a random factor given the high percentage of unique individuals in the dataset (81.1%).
Analysis of Predation During the Incubation Period
To assess quantitatively how variation in incubation period affected predation risk we used the model of incubation period to predict how focal predictors, such as relative incubation start date, changed the length of the incubation period and then used the daily nest predation rate to infer predicted changes in likelihood of nest predation in a typical year. This approach assumes that predation risk is uniform through time and space so that the probability of predation is purely a function of the time at risk. We calculated the daily nest predation rate during the incubation period using the Mayfield (1975) method. This method calculated the daily predation risk facing a nest by taking the total number of nests predated during the incubation period and dividing by the total number of active nest days during the incubation period for all nests. This estimate is based on 540 nests where clutches were completed and incubation started, of which 27.4% were depredated during incubation.
Results
Factors Affecting Incubation Period
An information theoretic approach to modeling incubation period identified three well-supported models (Supplementary Table S1). These models explained a moderate amount of variation with the random effect of year explaining approximately half of this variation (conditional R2 = 34.5%; marginal R2 = 17.2%). The relative incubation period start date was present in all models, with pairs breeding later in the year having shorter incubation periods (Table 1 and Figure 1A). Weather variables were also important because they were present in all four top models, with incubation periods increasing at higher temperatures (Table 1 and Figure 1B) and as the proportion of rainy days increased (Table 1 and Figure 1C). Larger clutch sizes were associated with shorter incubation periods (Table 1 and Figure 1D). All of the top models also contained an interaction term between clutch size and proportion of rainy days, which indicated that the effect of increased rainfall on incubation period was lessened by having a larger clutch (Figure 1C). Breeding attempt and female age were each present in two of the four top models (Supplementary Table S1), suggesting that the incubation period was shorter for replacement nests and younger females, but the confidence intervals for these effects’ parameter estimates overlapped zero suggesting that effects were negligible (Table 1).
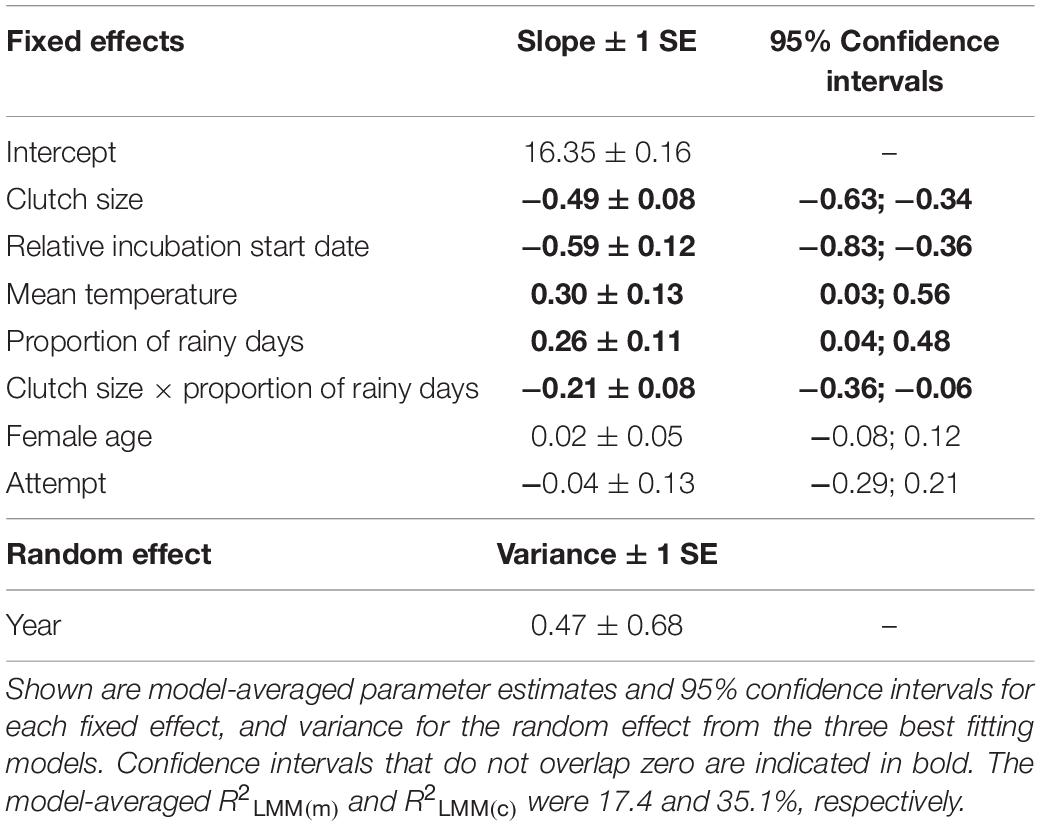
Table 1. The effects of clutch size, relative incubation start date, mean temperature, proportion of rainy days, female age, attempt and the interaction between clutch size and the proportion of rainy days on the duration of the incubation period in long-tailed tits.
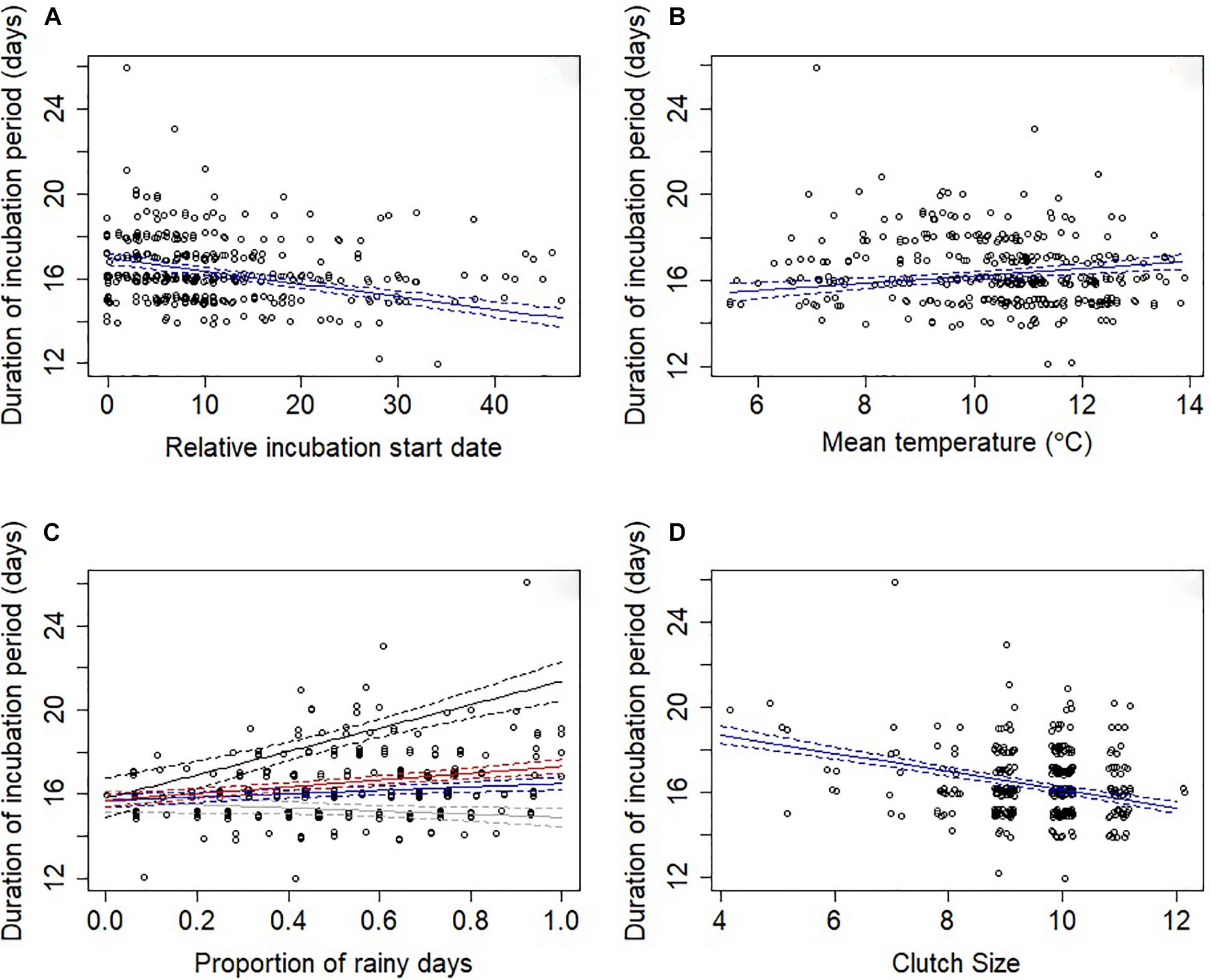
Figure 1. Duration of the incubation period (days) in relation to (A) relative incubation start date, (B) mean temperature, (C) proportion of rainy days and (D) clutch size. Points have been offset so that overlapping points can be better seen. The solid lines indicate the predicted values from model-averaged parameters and dashed lines indicate the standard error. In (C) due to an interaction between proportion of rainy days and clutch size lines represent the model-averaged parameters when the clutch size was set to the lower quartile value (nine eggs; blue), to the upper quartile value (10 eggs; red), to the minimum value (four eggs; black) and to the maximum value (12 eggs; gray).
Factors Affecting Hatching Success
Three models were retained in the top subset (Supplementary Table S6), although these models explained only a small amount of variation in hatching success (conditional R2 = 7.2%; marginal R2 = 4.9%). Longer incubation periods had a negative effect on hatching success (Table 2 and Figure 2A). Greater hatching success was also associated with larger clutch sizes (Figure 2B), younger females (Figure 2C) and first breeding attempts (Table 2), all of which were present in each of the top set of models. Lower mean temperatures and more rainy days were associated with greater hatching success, although only in one of the three models (Supplementary Table S6) and the confidence intervals for these effects’ parameter estimates overlapped zero indicating that their effects were negligible (Table 2).
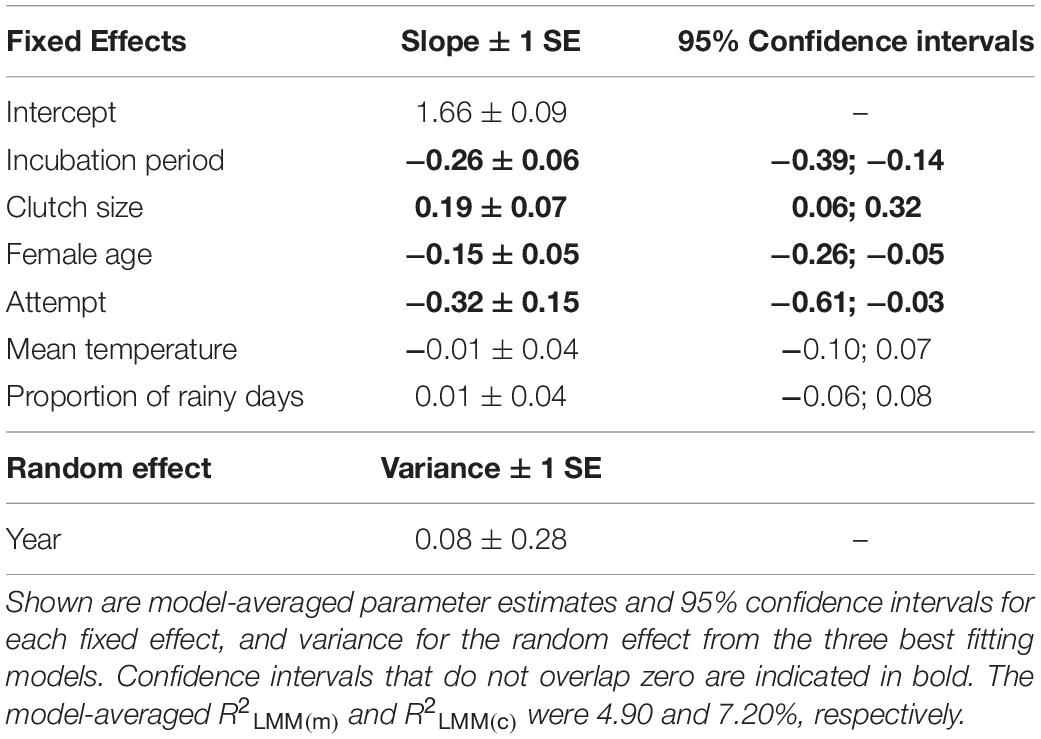
Table 2. The effects of incubation period, clutch size, female age, attempt, mean temperature and proportion of rainy days on hatching success in long-tailed tits.
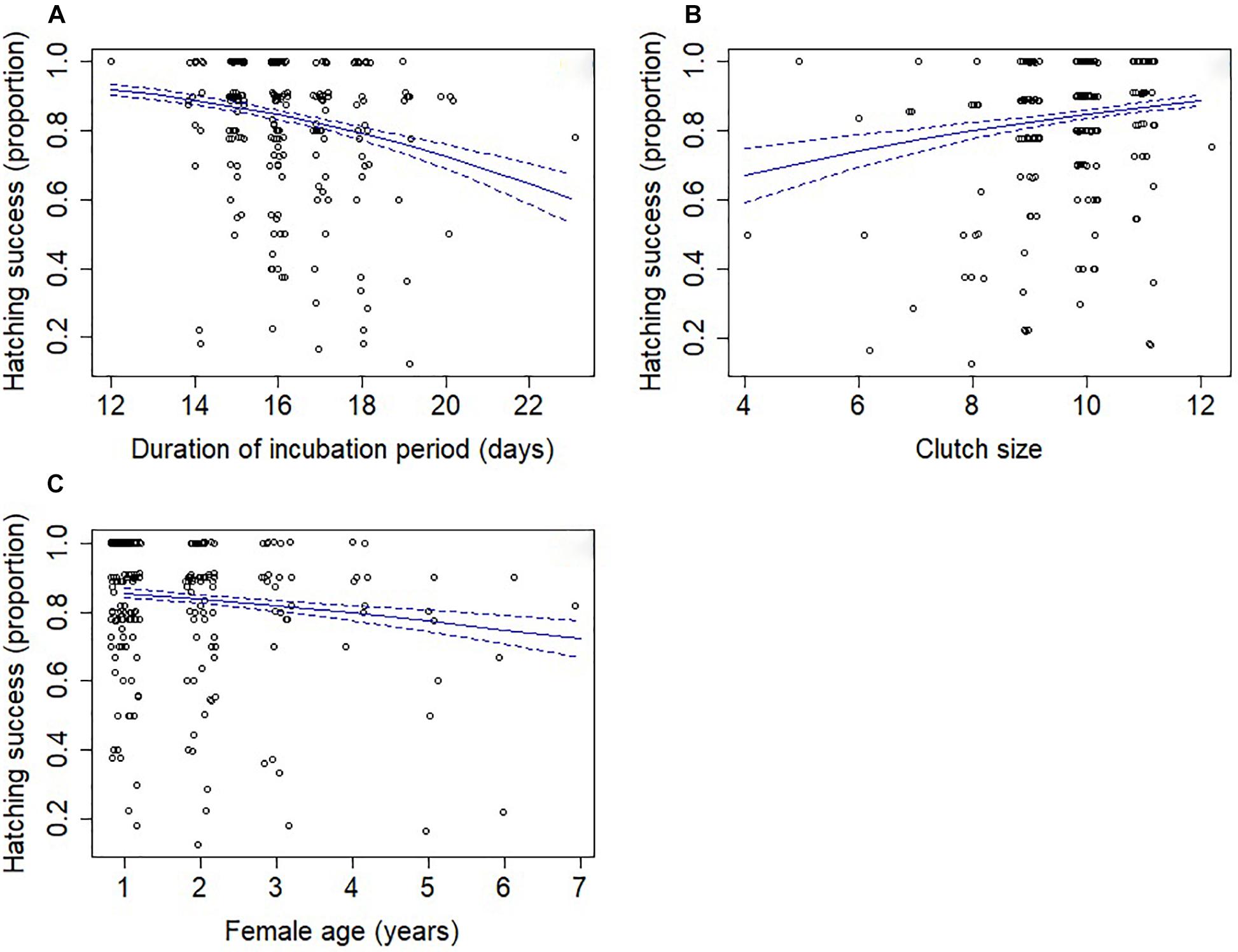
Figure 2. Hatching success (proportion) in relation to (A) the length of the incubation period, (B) clutch size and (C) female age. Points have been offset so that overlapping points can be better seen. The solid lines indicate the predicted values from model-averaged parameters and dashed lines indicate the standard error.
Effects of Incubation Period on Nest Predation Risk
Through a typical breeding season, the incubation period was predicted to decrease from 17.0 to 14.1 days (Figure 1a). Given a Mayfield daily nest predation rate during incubation of 0.02 this reduction in incubation period equates to the probability of predation reducing from 0.34 to 0.28 (i.e., an 18% reduction in predation). For the observed range of mean temperatures during incubation from 5.5 to 13.9°C, the models predict a change in the duration of the incubation period from 15.5 to 16.9 days (Figure 1B), which represents a change in predation risk probability from 0.31 to 0.34 (i.e., a 10% increase in predation). Similarly, the proportion of rainy days experienced during the incubation period ranged from 0 to 1, resulting in a change of incubation period from 15.7 to 16.8 days and an increase in predation risk probability from 0.31 to 0.34 (i.e., a 10% increase in predation). Clutch size ranged from 4 to 12 eggs in our sample of nests, which corresponds to a reduction in the incubation period from 18.7 to 15.3 days, under mean precipitation levels, with each additional egg resulting in the incubation period being shortened by 0.43 days and the probability of predation risk decreasing from 0.37 for 4 egg clutches to 0.31 for 12 egg clutches (i.e., a 16% reduction in predation).
Discussion
Variation in the incubation period of long-tailed tits over this 24-year study was influenced by clutch size, clutch completion date, mean daily temperature, and proportion of rainy days. Incubation period was longer for smaller clutches, for breeding attempts initiated earlier in the breeding season, under warmer temperatures, and when the proportion of rainy days was higher. However, the effect of rainy days varied with clutch size because larger clutch sizes were less affected by increases in the proportion of rainy days. In addition, incubation periods were shorter in replacement nesting attempts and when females were younger, although these effects were negligible. Changes in incubation duration influenced the risk of nest predation during incubation with each additional day of incubation leading to predation probabilities increasing by 0.02. Modeling indicated that timing of breeding and clutch size were the most influential factors moderating nest predation risk by influencing incubation durations. Models of hatching success had limited explanatory power, but we found evidence that greater hatching success was associated with shorter incubation periods, and to a lesser extent younger females, larger clutch sizes and first breeding attempts. Mean temperature and rainfall had minimal effects on hatching success.
Shorter incubation periods toward the end of the breeding season may be advantageous by allowing nestling provisioning to occur closer to the peak abundance of insects, especially of caterpillars that constitute the major component of nestling diet at this time of year (Gullett et al., 2014). Caterpillar abundance in the Rivelin Valley typically peaks around 23 May, i.e., during the long-tailed nestling period, and nestlings in relatively early and relatively late nests are provisioned with fewer caterpillars (Gullett, 2014). Other woodland passerines that predominantly provision offspring with caterpillars also appear to alter incubation behavior in order to better match the date of caterpillar peak abundance, e.g., blue tit (Visser et al., 1998), great tit Parus major (Simmonds et al., 2017) and European pied flycatcher Ficedula hypoleuca (Both and Visser, 2005). Adult long-tailed tits have more variable diets than their nestlings and the reduction in length of the incubation period later in the season could also be due to increased abundance of other insects that enables incubating females to increase daytime nest attendance (Dewey and Kennedy, 2001; Duncan Rastogi et al., 2006) and hence reduce incubation periods (Lyon and Montgomerie, 1985; Martin, 2002; Martin et al., 2007). In addition, the rate at which males provision females on the nest during the incubation period could increase later in the breeding season leading to greater female nest attentiveness and hence shorter incubation periods, as has been shown in other passerines (Martin and Ghalambor, 1999; Eikenaar et al., 2003; Matysioková et al., 2011).
Laying a larger clutch is thought to be costly because of the additional investment in eggs and because larger broods may increase activity around the nest leading to increased predation (Skutch, 1949; Johnsgard, 1973; Perrins, 1977; Martin et al., 2000). These costs may be partially compensated for by the reduction in incubation period with larger clutches; an increase in clutch size by one egg was associated with a reduction in incubation period of approximately half a day. Ardia et al. (2006) also found that larger clutches had shorter incubation periods in tree swallows, and it has been suggested that under temperate or cold conditions it is better to have a larger clutch size as this increases thermal inertia, reducing cooling rates relative to smaller clutches (Reid et al., 2000b; Cooper, 2005). This relationship between clutch size and incubation period contrasts with experimental evidence that increased clutch sizes do not reduce incubation duration (Székely et al., 1994; Siikamiiki, 1995; Sandercock, 1997; Reid et al., 2000a; Wiebe and Martin, 2000), a discrepancy that may be due to females being unable to effectively incubate enlarged clutches in experimental treatments. Our results represent natural variation where females would presumably be able to incubate the whole clutch effectively. It may be that better quality females are able to simultaneously produce naturally larger clutches while incubating more efficiently than poorer quality females, and hence reduce the incubation period for their clutches. However, further experimental work that monitored female quality while experimentally altering clutch sizes and measuring the duration of the incubation periods would be required to test this idea.
The interaction between clutch size and the proportion of rainy days indicated that larger clutches seem to be particularly advantageous when the proportion of rainy days increased. During periods of rainfall the nest is likely to lose heat via increased conductance through damp nesting materials (Reid et al., 2002; Hilton et al., 2004; Heenan, 2013), so our result may indicate that larger clutches buffer the effects of rainfall because a smaller clutch surface area to volume ratio reduces exposure to damp nesting materials or humid and cold air. However, it is also important to consider the potential impact of weather on the behavior of incubating birds; for example, any effects of increased conductance may be confounded by females adjusting their nest attentiveness during periods of heavy rainfall and storms (MacDonald et al., 2013; Fu et al., 2017).
Contrary to our predictions, higher ambient temperatures were associated with longer incubation periods. This result is especially surprising given that previous studies have found that other species, including cavity-nesting species whose nests tend to be well insulated (Massaro et al., 2013), are likely to delay the start of incubation at colder temperatures, thus increasing the duration of incubation (great tits: Monros et al., 1998; blue tits: Kluen et al., 2011). A likely mechanism for higher temperatures increasing the duration of the incubation period is adjustment of incubation behavior under different ambient temperatures. For example, incubating females may leave the nest for shorter periods in cold conditions (Voss et al., 2006; Amininasab et al., 2016; Walters et al., 2016), so eggs remain within the optimal incubation temperature range for a greater proportion of the day. However, other studies have reported greater nest attendance when ambient temperatures are higher (Morton and Pereyra, 1985; Ardia et al., 2010; MacDonald et al., 2013; Simmonds et al., 2017), perhaps because lower costs of heating eggs and/or foraging allow females to incubate for longer before they need to forage again. Regardless of the mechanism generating the positive association we observed between temperature and incubation duration in long-tailed tits, this effect suggests that climate change will not only lead to changes in the timing of breeding (Gullett et al., 2013), but may also lead to an increase in the incubation period of long-tailed tits, potentially increasing the risk that hatching is mis-timed relative to peak availability of caterpillars (Burgess et al., 2018).
Weather variables had marginal negative consequences because they had negligible effects on hatching success and led to a smaller change in the probability of nest predation, through increased exposure time, compared with the changes due to clutch size and timing of breeding. While, exposure time only provides limited information about the predation risk that a brood may face as predator behavior can change in relation to weather conditions (Morrison and Bolger, 2002; Preston and Rotenberry, 2006) there is no evidence that predation rates of long-tailed tit nests is related to weather in our study system (Gullett et al., 2015).
The negligible effect of temperature on hatching success is somewhat surprising given that other studies have found that lower ambient temperature can affect egg viability (Beissinger et al., 2005; Ardia et al., 2006). Contrary to our predictions and other studies (MacDonald et al., 2013; Martin et al., 2017), rainfall also had only a negligible effect on hatching success. These minor effects of environmental conditions on hatching success suggest that long-tailed tits may be able to buffer adverse weather conditions, either through females altering their incubation behavior or through the construction of well-insulated and water-resistant nests. Alternatively, it may be that for long-tailed tit embryos a reduction in temperature does not lead to the death of the embryo, but does lead to slower embryo development, which causes a lengthening of the incubation period. Further investigation is required to test these hypotheses.
Other factors had important impacts on hatching success. First, hatching success decreased as the incubation period increased, a finding consistent with previous studies of blue tits (Kluen et al., 2011; Nord and Nilsson, 2011), tree swallows (Lombardo et al., 1995), and great tits (Diez-Méndez et al., 2020). These results indicate that while birds may extend incubation to delay hatching so that it coincides better with the peak of food abundance, there is a cost to this strategic decision if it reduces hatching success. The relationship between hatching success and incubation period could be the result of either decreasing egg viability with time or increased fluctuation in egg temperature causing a reduction in egg viability. Support for the incubation inefficiency explanation is equivocal. An experimental study of house wrens Troglodytes aedon that used cross-fostering of eggs to extend or reduce the length of time females had to incubate a clutch had no effect on hatching success (Sakaluk et al., 2018), suggesting that the inefficient incubation hypothesis is unlikely. However, females in lower body condition incubate for longer and have reduced hatching success due to lower incubation temperatures (Hepp et al., 2006) and increased incubation recesses (Bueno-Enciso et al., 2017). In addition, Nord and Nilsson (2011) found that when incubation temperature was low, the incubation period was extended and the hatching success was lower. These mechanisms are not mutually exclusive, and either could result in the observed decrease in long-tailed tit hatching success.
We also found a weak, positive relationship between hatching success and clutch size. This contrasts with experimental studies reporting lower hatching success with increased clutch sizes (Siikamiiki, 1995; Reid et al., 2000b), which was presumed to be attributable to energetic constraints on successful incubation of experimentally enlarged clutches, as already discussed. The contrast between our observational findings and previous experimental studies may be explained by better quality females having larger clutch sizes and being better able to maintain the incubation temperatures (Hepp et al., 2006), leading to higher hatching success.
Finally, we found that hatching success was lower for older females. Previous work on putative age effects is equivocal. Some studies report increasing hatching success with age, e.g., prothonotary warblers Protonotaria citrea (Blem et al., 1999), while others have found no effect, e.g., European starlings Sturnus vulgaris (Komdeur et al., 2005), blue tit (Lambrechts et al., 2012) and house sparrows Passer domesticus (Stewart and Westneat, 2013). Our results are consistent with senescence, which has been widely reported in passerine reproductive performance (Monaghan et al., 2008; Robertson and Rendellt, 2012; Jankowiak and Wysocki, 2016), but it should be noted that in previous studies, albeit based on smaller sample sizes, we have not detected senescence in long-tailed tit life history traits (Hatchwell et al., 2004; Meade and Hatchwell, 2010).
In conclusion, our long-term analysis showed that mean daily temperature, proportion of rainy days, clutch size and relative incubation start date explained variation in the duration of the incubation period of long-tailed tits. In contrast, we found only marginal effects of environmental conditions on hatching success. This suggests that wet conditions cause slower growth of embryos and hence longer incubation periods, rather than directly causing embryo fatality. Finally, long incubation periods were likely to be costly due to reduced hatching success and increased exposure to predation risk.
Data Availability Statement
The datasets generated for this study are available on request to the corresponding author.
Ethics Statement
The animal study was reviewed and approved by University of Sheffield Ethical Review Committee (Project Applications and Amendments Sub-Committee) and a Project License is held for taking blood samples from the UK Home Office (PPL 70/8434).
Author Contributions
CGH is supervised by KLE and BJH who initiated and manages the long-term long-tailed tit study system. CGH led the data analysis and wrote the first draft of the manuscript. KLE and BJH contributed to the conception and design of the data analysis, interpretation, and writing the manuscript. All authors contributed to the article and approved the submitted version.
Funding
We are grateful for the support of NERC, for funding the long-tailed tit study for 24 years and funding CGH through “Adapting to the Challenges of a Changing Environment” (ACCE), a NERC-funded doctoral training partnership (NE/L002450/1).
Conflict of Interest
The authors declare that the research was conducted in the absence of any commercial or financial relationships that could be construed as a potential conflict of interest.
Acknowledgments
We thank all fieldworkers who aided in collection of long-term data.
Supplementary Material
The Supplementary Material for this article can be found online at: https://www.frontiersin.org/articles/10.3389/fevo.2020.542179/full#supplementary-material
References
Álvarez, E., and Barba, E. (2014). Incubation and hatching periods in a Mediterranean great tit Parus major population. Bird Stud. 61, 152–161. doi: 10.1080/00063657.2014.908819
Amininasab, S. M., Kingma, S. A., Birker, M., Hildenbrandt, H., and Komdeur, J. (2016). The effect of ambient temperature, habitat quality and individual age on incubation behaviour and incubation feeding in a socially monogamous songbird. Behav. Ecol. Sociobiol. 70, 1591–1600. doi: 10.1007/s00265-016-2167-2
Ardia, D. R., and Clotfelter, E. D. (2007). Individual quality and age affect responses to an energetic constraint in a cavity-nesting bird. Behav. Ecol. 18, 259–266. doi: 10.1093/beheco/arl078
Ardia, D. R., Cooper, C. B., and Dhondt, A. A. (2006). Warm temperatures lead to early onset of incubation, shorter incubation periods and greater hatching asynchrony in tree swallows Tachycineta bicolor at the extremes of their range. J. Avian Biol. 37, 137–142. doi: 10.1111/j.0908-8857.2006.03747.x
Ardia, D. R., Pérez, J. H., and Clotfelter, E. D. (2010). Experimental cooling during incubation leads to reduced innate immunity and body condition in nestling tree swallows. Proc. R. Soc Biol. Sci. 277, 1881–1888. doi: 10.1098/rspb.2009.2138
Barton, K. (2018). MuMIn: Multi-Model Inference. R package version 1.42.1. Available at: https://CRAN.R-project.org/package=MuMIn (accessed September 24, 2018).
Bates, D., Machler, M., Bolker, B. M., and Walker, S. (2015). Fitting linear mixed-effects models using lme4. J. Statist. Softw. 67, 1–48.
Beissinger, S. R., Cook, M. I., and Arendt, W. J. (2005). The shelf life of bird eggs: testing egg viability using a tropical climate gradient. Ecology 86, 2164–2175. doi: 10.1890/04-1624
Berntsen, H. H., and Bech, C. (2016). Incubation temperature influences survival in a small passerine bird. J. Avian Biol. 47, 141–145. doi: 10.1111/jav.00688
Blem, C. R., Blem, L. B., and Barrientos, C. I. (1999). Relationships of clutch size and hatching success to age of female prothonotary warblers. Wilson Bull. 111, 577–581.
Both, C. (2010). “Food availability, mistiming and climatic change,” in Effects of Climate Change on Birds, eds A. P. Møller, and Fiedler (Oxford: Oxford University Press), 129–147.
Both, C., and Visser, M. E. (2005). The effect of climate change on the correlation between avian life-history traits. Glob. Change Biol. 11, 1606–1613. doi: 10.1111/j.1365-2486.2005.01038.x
Bueno-Enciso, J., Barrientos, R., and Sanz, J. J. (2017). Incubation behaviour of blue Cyanistes caeruleus and great tits Parus major in a Mediterranean habitat. Acta Ornithol. 52, 21–34. doi: 10.3161/00016454AO2017.52.1.003
Burgess, M. D., Smith, K. W., Evans, K. L., Leech, D., Pearce-Higgins, J. W., Branston, C. J., et al. (2018). Tritrophic phenological match-mismatch in space and time. Nat. Ecol. Evol. 2, 970–975. doi: 10.1038/s41559-018-0543-1
Burnham, K. P., and Anderson, D. R. (2002). Model Selection and Multimodel Inference: A Practical Information-Theoretic Approach, 2nd Edn. New York, NY: Springer-Verlag New York Inc.
Burton, A., Glenis, V., Jones, M., and Kilsby, C. (2013). Models of daily rainfall cross-correlation for the United Kingdom. Environ. Model. Softw. 49, 22–33. doi: 10.1016/j.envsoft.2013.06.001
Charmantier, A., Mccleery, R. H., Cole, L. R., Perrins, C., Kruuk, L. E. B., and Sheldon, B. C. (2008). Adaptive phenotypic plasticity in response to climate change in a wild bird population. Science 320, 800–804. doi: 10.1126/science.1157174
Coe, B. H., Beck, M. L., Chin, S. Y., Jachowski, C. M. B., and Hopkins, W. A. (2015). Local variation in weather conditions influences incubation behavior and temperature in a passerine bird. J. Avian Biol. 46, 385–394. doi: 10.1111/jav.00581
Conway, C. J., and Martin, T. E. (2000). Effects of ambient temperature on avian incubation behavior. Behav. Ecol. 11, 178–188. doi: 10.1093/beheco/11.2.178
Cooper, C. (2005). Seasonal and latitudinal trends in clutch size: thermal constraints during laying and incubation. Ecology 86, 2018-2031. doi: 10.1890/03-8028
Core Team, R. (2016). R: A language and Environment for Statistical Computing. Vienna: R Foundation for Statistical Computing.
Daan, S., Tinbergen, J. M., and Davies, N. B. (eds) (1997). “Adaptation of Life Histories,” in Behavioural Ecology, An Evolutionary Approach, ed. J. R. Krebs (Oxford: Blackwell), 311–333.
Deeming, D. C., and Gray, L. A. (2016). Incubation attentiveness and nest insulatory values correlate in songbirds. Avian Biol. Res. 9, 32–36. doi: 10.3184/175815516X14454107215458
Dewey, S. R., and Kennedy, P. L. (2001). Effects of supplemental food on parental-care strategies and juvenile survival of northern goshawks. Auk 118, 352–365. doi: 10.1093/auk/118.2.352
Diez-Méndez, D., Rodríguez, S., Álvarez, E., and Barba, E. (2020). The role of partial incubation and egg repositioning within the clutch in hatching asynchrony and subsequent effects on breeding success. Ibis 162, 63–74. doi: 10.1111/ibi.12730
Duncan Rastogi, A., Zanette, L., and Clinchy, M. (2006). Food availability affects diurnal nest predation and adult antipredator behaviour in song sparrows, Melospiza melodia. Animal Behav. 72, 933–940. doi: 10.1016/j.anbehav.2006.03.006
DuRant, S. E., Hopkins, W. A., Hepp, G. R., and Romero, L. M. (2013a). Energetic constraints and parental care: is corticosterone indicative of energetic costs of incubation in a precocial bird? Horm. Behav. 63, 385–391. doi: 10.1016/j.yhbeh.2012.12.001
DuRant, S. E., Hopkins, W. A., Hepp, G. R., and Walters, J. R. (2013b). Ecological, evolutionary, and conservation implications of incubation temperature-dependent phenotypes in birds. Biol. Rev. 88, 499–509. doi: 10.1111/brv.12015
Eikenaar, C., Berg, M. L., and Komdeur, J. (2003). Experimental evidence for the influence of food availability on incubation attendance and hatching asynchrony in the Australian reed warbler Acrocephalus australis. J. Avian Biol. 34, 419–427. doi: 10.1111/j.0908-8857.2003.03147.x
Freckleton, R. P. (2011). Dealing with collinearity in behavioural and ecological data: model averaging and the problems of measurement error. Behav. Ecol. Sociobiol. 65, 91–101. doi: 10.1007/s00265-010-1045-6
Fu, Y., Dai, B., Wen, L., Chen, B., Dowell, S., and Zhang, Z. (2017). Unusual incubation behavior and embryonic tolerance of hypothermia in the Sichuan partridge (Arborophila rufipectus). J. Ornithol. 158, 707–715. doi: 10.1007/s10336-016-1422-7
García-Navas, V., and Sanz, J. J. (2011). Short-term alterations in songbird breeding schedule lead to better synchronization with food availability. Auk 128, 146–155. doi: 10.1525/auk.2010.10094
Gaston, A. J. (1973). The ecology and behaviour of long-tailed tit. Ibis 115:330. doi: 10.1111/j.1474-919X.1973.tb01974.x
Glen, N. W. (1985). The Cooperative Breeding Behavior of the Long-Tailed tit (Aegithalos caudatus). D.Phil. thesis, University of Oxford, Oxford.
Gullett, P., Evans, K. L., Robinson, R. A., and Hatchwell, B. J. (2014). Climate change and annual survival in a temperate passerine: partitioning seasonal effects and predicting future patterns. Oikos 123, 389–400. doi: 10.1111/j.1600-0706.2013.00620.x
Gullett, P., Hatchwell, B. J., Robinson, R. A., and Evans, K. L. (2013). Phenological indices of avian reproduction: cryptic shifts and prediction across large spatial and temporal scales. Ecol. Evol. 3, 1864–1877. doi: 10.1002/ece3.558
Gullett, P. R. (2014). Demographic of Avian Responses to Climate Change: Long-Tailed Tits Aegithalos caudatus As A Case Study. PhD thesis, University of Sheffield, Sheffield.
Gullett, P. R., Hatchwell, B. J., Robinson, R. A., and Evans, K. L. (2015). Breeding season weather determines long-tailed tit reproductive success through impacts on recruitment. J. Avian Biol. 46, 441–451. doi: 10.1111/jav.00560
Haftorn, S. (1981). Incubation during the egg-laying period in relation to clutch-size and other aspects of reproduction in the great tit Parus major. Ornis Scand. 12, 169–185. doi: 10.2307/3676074
Hansell, M. H. (1996). The function of lichen flakes and white spider cocoons on the outer surface of birds’ nests. J. Nat. History 30, 303–311. doi: 10.1080/00222939600771181
Hanssen, S. A., Hasselquist, D., Folstad, I., and Erikstad, K. E. (2005). Cost of reproduction in a long-lived bird: incubation effort reduces immune function and future reproduction. Proc. R. Soc. B Biol. Sci. 272, 1039–1046. doi: 10.1098/rspb.2005.3057
Hatchwell, B. J., Russell, A. F., Fowlie, M. K., and Ross, D. J. (1999). Reproductive success and nest-site selection in a cooperative breeder: effect of experience and a direct benefit of helping. Auk 116, 355–363. doi: 10.2307/4089370
Hatchwell, B. J., Russell, A. F., MacColl, A. D. C., Ross, D. J., Fowlie, M. K., and McGowan, A. (2004). Helpers increase long-term but not short-term productivity in cooperatively breeding long-tailed tits. Behav. Ecol. 15, 1–10. doi: 10.1093/beheco/arg091
Hatchwell, B. J., Sharp, S. P., Beckerman, A. P., and Meade, J. (2013). Ecological and demographic correlates of helping behaviour in a cooperatively breeding bird. J. Anim. Ecol. 82, 486–494. doi: 10.1111/1365-2656.12017
Heenan, C. B. (2013). An overview of the factors influencing the morphology and thermal properties of avian nests. Avian Biol. Res. 6, 104–118. doi: 10.3184/003685013X13614670646299
Hepp, G. R., Kennamer, R. A., and Johnson, M. H. (2006). Maternal effects in wood ducks: incubation temperature influences incubation period and neonate phenotype. Funct. Ecol. 20, 307–314. doi: 10.1111/j.1365-2435.2006.01108.x
Hilton, G. M., Hansell, M. H., Ruxton, G. D., Reid, J. M., and Monaghan, P. (2004). Using artificial nests to test importance of nesting material and nest shelter for incubation energetics. Auk 121, 777–787. doi: 10.2307/4090314
Houston, A. I., and McNamara, J. M. (1999). Models of Adaptive Behavior: An Approach Based on State. Cambridge: Cambridge University Press.
Jankowiak, Ł, and Wysocki, D. (2016). Do individual breeding experience and parental effort affect breeding season length in blackbirds? Behav. Ecol. 27, 829–834. doi: 10.1093/beheco/arv227
Jarvinen, A. (1993). Spatial and temporal variation in reproductive traits of adjacent northern pied flycatcher Ficedula hypoleuca populations. Ornis Scand. 24, 33–40. doi: 10.2307/3676407
Kluen, E., de Heij, M. E., and Brommer, J. E. (2011). Adjusting the timing of hatching to changing environmental conditions has fitness costs in blue tits. Behav. Ecol. Sociobiol. 65, 2091–2103. doi: 10.1007/s00265-011-1218-y
Knowles, S. C. L., Nakagawa, S., and Sheldon, B. C. (2009). Elevated reproductive effort increases blood parasitaemia and decreases immune function in birds: a meta-regression approach. Funct. Ecol. 23, 405–415. doi: 10.1111/j.1365-2435.2008.01507.x
Komdeur, J., Oorebeek, M., Van Overveld, T., and Cuthill, I. C. (2005). Mutual ornamentation, age, and reproductive performance in the European starling. Behav. Ecol. 16, 805–817. doi: 10.1093/beheco/ari059
Lambrechts, M. M., Aimé, C., Midamegbe, A., Galan, M. J., Perret, P., Grégoire, A., et al. (2012). Nest size and breeding success in first and replacement clutches: an experimental study in blue tits Cyanistes caeruleus. J. Ornithol. 153, 173–179. doi: 10.1007/s10336-011-0722-1
Lombardo, M., Bosman, R. M., Faro, C. A., Houtteman, S. G., and Kluisza, T. S. (1995). Effect of feathers as nest insulation on incubation behaviour and reproductive performance of tree swallows (Tachycineta bicolor). Auk 112, 973–981. doi: 10.2307/4089028
Lyon, B. E., and Montgomerie, R. D. (1985). Incubation feeding in snow buntings: female manipulation or indirect male parental care? Behav. Ecol. Sociobiol. 17, 279–284. doi: 10.1007/BF00300147
MacColl, A. D. C., and Hatchwell, B. J. (2002). Temporal variation in fitness payoffs promotes cooperative breeding in long-tailed tits Aegithalos caudatus. Am. Nat. 160, 186–194. doi: 10.1086/341013
MacDonald, E. C., Camfield, A. F., Jankowski, J. E., and Martin, K. (2013). Extended incubation recesses by alpine-breeding horned larks: a strategy for dealing with inclement weather? J. Field Ornithol. 84, 58–68. doi: 10.1111/jofo.12006
Martin, K., Wilson, S., Macdonald, E. C., Camfield, A. F., Martin, M., and Trefry, S. A. (2017). Effects of severe weather on reproduction for sympatric songbirds in an alpine environment: interactions of climate extremes influence nesting success. Auk 134, 696–709. doi: 10.1642/AUK-16-271.1
Martin, T. E. (2002). A new view of avian life-history evolution tested on an incubation paradox. Proc. R. Soc. B Biol. Sci. Biol. Sci. 269, 309–316. doi: 10.1098/rspb.2001.1879
Martin, T. E., Auer, S. K., Bassar, R. D., Niklison, A. M., and Lloyd, P. (2007). Geographic variation in avian incubation periods and parental influences on embryonic temperature. Evolution 61, 2558–2569. doi: 10.1111/j.1558-5646.2007.00204.x
Martin, T. E., and Ghalambor, C. K. (1999). Males feeding females during inclubation. I. Required by microclimate or condtrained by predation? Am. Nat. 153, 131–139. doi: 10.1086/303153
Martin, T. E., Scott, J., and Menge, C. (2000). Nest predation increases with parental activity: separating nest site and parental activity effects. Proc. R. Soc. B Biol. Sci. 267, 2287–2293. doi: 10.1098/rspb.2000.1281
Massaro, M., Stanbury, M., and Briskie, J. V. (2013). Nest site selection by the endangered black robin increases vulnerability to predation by an invasive bird. Anim. Conserv. 16, 404–411. doi: 10.1111/acv.12007
Matysioková, B., Cockburn, A., and Remeš, V. (2011). Male incubation feeding in songbirds responds differently to nest predation risk across hemispheres. Anim. Behav. 82, 1347–1356. doi: 10.1016/j.anbehav.2011.09.018
McGowan, A., Hatchwell, B. J., and Woodburn, R. J. W. (2003). The effect of helping behaviour on the survival of juvenile and adult long-tailed tits Aegithalos caudatus. J. Anim. Ecol. 72, 491–499. doi: 10.1046/j.1365-2656.2003.00719.x
McGowan, A., Sharp, S. P., and Hatchwell, B. J. (2004). The structure and function of nests of long-tailed tits Aegithalos caudatus. Funct. Ecol. 18, 578–583. doi: 10.1111/j.0269-8463.2004.00883.x
Meade, J., and Hatchwell, B. J. (2010). No direct fitness benefits of helping in a cooperative breeder despite higher survival of helpers. Behav. Ecol. 21, 1186–1194. doi: 10.1093/beheco/arq137
Monaghan, P., Charmantier, A., Nussey, D. H., and Ricklefs, R. E. (2008). The evolutionary ecology of senescence. Funct. Ecol. 22, 371–378. doi: 10.1111/j.1365-2435.2008.01418.x
Monros, J. S., Belda, E. J., and Barba, E. (1998). Delays of the hatching dates in great tits Parus major: effects on breeding performance. Ardea 86, 213–220.
Morrison, S. A., and Bolger, D. T. (2002). Variation in a sparrow’s reproductive success with rainfall: food and predator-mediated processes. Oecologia 133, 315–324. doi: 10.1007/s00442-002-1040-3
Morton, M. L., and Pereyra, M. E. (1985). The regulation of egg temperatures and attentiveness patterns in the dusky flycatcher (Empidonax oberholseri). Auk 102, 25–37. doi: 10.2307/4086819
Naef-Daenzer, L., Nager, R. G., Keller, L. F., and Naef-Daenzer, B. (2004). Are hatching delays a cost or a benefit for great tit Parus major parents? Ardea 92, 229–237.
Nakagawa, S., and Schielzeth, H. (2010). Repeatability for gaussian and non-gaussian data: a practical guide for biologists. Biol. Rev. 85, 935–956. doi: 10.1111/j.1469-185X.2010.00141.x
Nakagawa, S., and Schielzeth, H. (2013). A general and simple method for obtaining R2 from generalized linear mixed-effects models. Methods Ecol. Evol. 4, 133–142. doi: 10.1111/j.2041-210x.2012.00261.x
Nilsson, J. Å, and Smith, H. G. (1988). Incubation feeding as a male tactic for early hatching. Anim. Behav. 36, 641–647. doi: 10.1016/S0003-3472(88)80145-3
Nilsson, J. Å, and Svensson, E. (1993). The frequency and timing of laying gaps. Ornis Scand. 24, 122–126. doi: 10.2307/3676361
Nord, A., and Nilsson, J. Å (2011). Incubation temperature affects growth and energy metabolism in blue tit nestlings. Am. Nat. 178, 639–651. doi: 10.1086/662172
Nord, A., and Williams, J. B. (2015). “The energetic costs of incubation,” in Nests, Eggs, and Incubation: New Ideas About Avian Reproduction, eds D. C. Deeming and S. J. Reynolds (Oxford: Oxford University Press), 152–170. doi: 10.1093/acprof:oso/9780198718666.003.0013
Olson, C. R., Vleck, C. M., and Vleck, D. (2006). Periodic cooling of bird eggs reduces embryonic growth efficiency. Physiol. Biochem. Zool. 79, 927–936. doi: 10.1086/506003
Perrins, C. M. (1970). The timing of birds’ breeding seasons. Ibis 112, 242–255. doi: 10.1111/j.1474-919X.1970.tb00096.x
Perrins, C. M. (1977). “The role of predation in the evolution of clutch size,” in Evolutionary Ecology, eds B. Stonehouse and C. M. Perrins (Baltimore, MD: University Park Press), 181–191. doi: 10.1007/978-1-349-05226-4_16
Preston, K. L., and Rotenberry, J. T. (2006). Independent effects of food and predator- mediated processes on annual fecundity in a songbird. Ecology 87, 160–168.
Reid, J. M., Cresswell, W., Holt, S., Mellanby, R. J., Whitfield, D. P., and Ruxton, G. D. (2002). Nest scrape design and clutch heat loss in pectoral sandpipers (Calidris melanotos). Funct. Ecol. 16, 305–312. doi: 10.1046/j.1365-2435.2002.00632.x
Reid, J. M., Monaghan, P., and Ruxton, G. D. (2000a). Resource allocation between reproductive phases: the importance of thermal conditions in determining the cost of incubation. Proc. R. Soc. B Biol. Sci. 267, 37–41. doi: 10.1098/rspb.2000.0963
Reid, J. M., Monaghan, P., and Ruxton, G. D. (2000b). The consequences of clutch size for incubation conditons and hatching success in starlings. Funct. Ecol. 14, 560–565. doi: 10.1046/j.1365-2435.2000.t01-1-00446.x
Reznick, D., Nunney, L., and Tessier, A. (2000). Big houses, big cars, superfleas and the costs of reproduction. Trends Ecol. Evol. 15, 421–425. doi: 10.1016/S0169-5347(00)01941-8
Robertson, J., and Rendellt, B. (2012). A long-term study of reproductive performance in tree swallows: the influence of age and senescence the influence on output. J. Anim. Ecol. 70, 1014–1031. doi: 10.1046/j.0021-8790.2001.00555.x
Rohwer, V. G., Bonier, F., and Martin, P. R. (2015). Conflict between biotic and climatic selective pressures acting on an extended phenotype in a subarctic, but not temperate, environment. Proc. R. Soc. B Biol. Sci. 282, 20151585. doi: 10.1098/rspb.2015.1585
Rowe, K. M. C., and Weatherhead, P. J. (2009). A third incubation tactic: delayed incubation by American robins (Turdus migratorius). Auk 126, 141–146. doi: 10.1525/auk.2009.07210
Sakaluk, S. K., Thompson, C. F., and Bowers, E. K. (2018). Experimental manipulation of incubation period reveals no apparent costs of incubation in house wrens. Anim. Behav. 137, 169–177. doi: 10.1016/j.anbehav.2018.01.006
Sandercock, B. K. (1997). Incubation capacity and clutch size determination in two calidrine sandpipers: a test of the four-egg threshold. Oecologia 110, 50–59. doi: 10.1007/s004420050132
Schaper, S. V., Dawson, A., Sharp, P. J., Gienapp, P., Caro, S. P., and Visser, M. E. (2012). Increasing temperature, not mean temperature, is a cue for avian timing of reproduction. Am. Nat. 179, 55–69. doi: 10.1086/663675
Sharp, S. P., Baker, M. B., Hadfield, J. D., Simeoni, M., and Hatchwell, B. J. (2008). Natal dispersal and recruitment in a cooperatively breeding bird. Oikos 117, 1371–1379. doi: 10.1111/j.2008.0030-1299.16392.x
Sheaffer, S. E., and Malecki, R. A. (1996). Predicting breeding success of Atlantic population canada geese from meteorological variables. J. Wildl. Manag. 60, 882–890. doi: 10.2307/3802389
Siikamiiki, P. (1995). Are large clutches costly to incubate - the case of the pied flycatcher. J. Avian Biol. 26, 76–80. doi: 10.2307/3677215
Simmonds, E. G., Sheldon, B. C., Coulson, T., and Cole, E. F. (2017). Incubation behavior adjustments, driven by ambient temperature variation, improve synchrony between hatch dates and caterpillar peak in a wild bird population. Ecol. Evol. 7, 1–11. doi: 10.1002/ece3.3446
Skinner, W. R., Jefferies, R. L., Carleton, T. J., Rockwell, R. F., and Abraham, K. F. (1998). Prediction of reproductive success and failure in lesser snow geese based on early season climatic variables. Glob. Change Biol. 4, 3–16. doi: 10.1046/j.1365-2486.1998.00097.x
Skutch, A. (1949). Do tropical birds rear as many young as they can nourish? Ibis 91, 430–455. doi: 10.1111/j.1474-919X.1949.tb02293.x
Slagsvold, T., Amundsen, T., and Dale, S. (1995). Costs and benefits of hatching asynchrony in blue tits Parus caeruleus. J. Anim. Ecol. 64:563. doi: 10.2307/5800
Stearns, S. C. (1989). Trade-offs in life-history evolution. Funct. Ecol. 3, 259–268. doi: 10.2307/2389364
Stenning, M. J. (2008). Hatching asynchrony and brood reduction in blue tits Cyanistes caeruleus may be a plastic response to local oak Quercus robur bud burst and caterpillar emergence. Acta Ornithol. 43, 97–106. doi: 10.3161/000164508x345383
Stewart, I. R. K., and Westneat, D. F. (2013). Patterns of hatching failure in the house sparrow Passer domesticus. J. Avian Biol. 44, 69–79. doi: 10.1111/j.1600-048X.2012.05795.x
Székely, T., Karsai, I., and Williams, T. D. (1994). Determination of clutch-size in the kentish plover Charadrius alexandrinus. Ibis 136, 341–348. doi: 10.1111/j.1474-919X.1994.tb01105.x
Tatner, P., and Bryant, D. M. (1993). Interspecific variation in daily energy expenditure during avian incubation. J. Zool. 231, 215–232. doi: 10.1111/j.1469-7998.1993.tb01913.x
Tombre, I. M., and Erikstad, K. E. (1996). An experimental study of incubation effort in high-Arctic barnacle geese. J. Anim. Ecol. 65, 325–331. doi: 10.2307/5878
Visser, M. E., and Lessells, C. M. (2001). The costs of egg production and incubation in great tits (Parus major). Proc. R. Soc. B Biol. Sci. 268, 1271–1277. doi: 10.1098/rspb.2001.1661
Visser, M. E., van Noordwijk, A. J., Tinbergen, J. M., and Lessells, C. M. (1998). Warmer springs lead to mistimed reproduction in great tits (Parus major). Proc. R. Soc. B Biol. Sci. 265, 1867–1870. doi: 10.1098/rspb.1998.0514
Voss, M. A., Reed Hainsworth, F., and Ellis-Felege, S. N. (2006). Use of a new model to quantify compromises between embryo development and parental self-maintenance in three species of intermittently incubating passerines. J. Ther. Biol. 31, 453–460. doi: 10.1016/j.jtherbio.2006.03.002
Walters, L. A., Webber, J. A., Jones, B. A., and Volker, C. L. (2016). Taking a break: the relationship between ambient temperature and nest attendance patterns of incubating Carolina chickadees (Poecile carolinensis). Wilson J. Ornithol. 128, 719–726. doi: 10.1676/15-115.1
Webb, D. R. (1987). Thermal tolerance of avian embryos: a review. Condor 89, 874–898. doi: 10.2307/1368537
Wesołowski, T., Czeszczewik, D., Rowiñski, P., and Walankiewicz, W. (2002). Nest soaking in natural holes - a serious cause of breeding failure? Ornis Fennica 79, 132–138.
Wiebe, K. L., and Martin, K. (2000). The use of incubation behavior to adjust avian reproductive costs after egg laying. Behav. Ecol. Sociobiol. 48, 463–470. doi: 10.1007/s002650000259
Williams, J. B. (1996). “Energetics of avian incubation,” in Avian Energetics and Nutritional Ecology, ed. C. Carey (Boston, MA: Springer), 375–416. doi: 10.1007/978-1-4613-0425-8_11
Keywords: climate, incubation length, microclimate, parental investment, thermal environment, seasonal variation
Citation: Higgott CG, Evans KL and Hatchwell BJ (2020) Incubation in a Temperate Passerine: Do Environmental Conditions Affect Incubation Period Duration and Hatching Success? Front. Ecol. Evol. 8:542179. doi: 10.3389/fevo.2020.542179
Received: 11 March 2020; Accepted: 02 September 2020;
Published: 22 September 2020.
Edited by:
Mark C. Mainwaring, University of Montana, United StatesReviewed by:
Michał Glądalski, University of Łódź, PolandAlejandro Cantarero, University of Turku, Finland
Copyright © 2020 Higgott, Evans and Hatchwell. This is an open-access article distributed under the terms of the Creative Commons Attribution License (CC BY). The use, distribution or reproduction in other forums is permitted, provided the original author(s) and the copyright owner(s) are credited and that the original publication in this journal is cited, in accordance with accepted academic practice. No use, distribution or reproduction is permitted which does not comply with these terms.
*Correspondence: Ben J. Hatchwell, Yi5oYXRjaHdlbGxAc2hlZmZpZWxkLmFjLnVr