- Department of Organismic and Evolutionary Biology, Harvard University, Cambridge, MA, United States
Bisporic embryo sacs contain nuclei derived from two members of a megaspore tetrad whereas tetrasporic embryo sacs contain nuclei derived from all four members of a tetrad. Megaspores from the same tetrad are less genetically similar than are megaspores from different tetrads. Therefore, cells derived from different megaspores in bisporic and tetrasporic embryo sacs are expected to compete with each other rather than cooperate. The tacit assumption of the plant embryological literature that bisporic and tetrasporic embryo sacs function as integrated organisms is demonstrably false, both theoretically and empirically. This competition within embryo sacs can be expressed as the formation of eggs by descendants of more than one megaspore or by the suppression of the descendants of all but one megaspore. Both phenomena have evolved multiple times. In contrast, all nuclei of monosporic female gametophytes are genetically identical. Consequently, monosporic development is predicted to be more evolutionarily stable than bisporic or tetrasporic development. The triploid endosperm produced by most monosporic gametophytes was probably derived from an ancestrally diploid endosperm and has been considered a key adaptation of the most successful lineages of flowering plants. The greater weight given to the doubled maternal genome in triploid endosperm may have facilitated a more efficient distribution of resources among a mother’s seeds because it reduced the influence of competition for maternal resources among unrelated paternal genomes of endosperm.
Introduction
In the 1970s, plant embryology was primarily a morphological discipline concerned with the structuralist ‘what?’ rather than functionalist ‘why?’ or mechanist ‘how?’. Textbooks and reviews contained an obligatory diagram of the different ‘types’ of female gametophytes (Polygonum-type, Fritillaria-type, etc.), conceived as archetypical forms of how development ‘ideally’ proceeded rather than representions of how development actually proceeded in real organisms. The Drusa-type, for example, had eleven antipodal cells, because this brought the total number of nuclei to four times four, even though this number had rarely, if ever, been observed (Haig, 1990). Some of these haploid ‘organisms’ contained nuclei that were derived from different products of female meiosis, and the mature seed contained multiple tissues of different genetic constitution (diploid seed coat, diploid embryo, and triploid endosperm), but these were formal details without functional meaning. The theory of kin selection reconceptualized the seed, not as a stage in the life cycle of a unitary organism, but as a kin group with all the complexities of shared and conflicting interests found within families.
The first wave of papers applying kin selection to plant embryology broke forty years ago on the sleepy shore of phytomorphology. Papers from that time addressed many aspects of seed development with a particular focus on the triploid endosperm. Charnov (1979) led the way by proposing that the pollen-derived genome of endosperm raised the relatedness of the seed’s nutrient-garnering tissue to its associated embryo thus promoting the father’s interests in the context of ovule abortion. Westoby and Rice (1982) contended that the second maternal genome increased endosperm’s relatedness to embryos in other seeds giving the mother greater control over resource allocation among offspring. For Queller (1983), the extra maternal genome meant that endosperm favored its associated embryo over other embryos more strongly than would the female gametophyte but less strongly than would the embryo itself. Willson and Burley (1983) framed their discussion in terms of male–female conflict rather than kin selection. Post-zygotic abortion of seeds was presented as a mechanism of mate choice. They viewed ‘female’ genomes of endosperm as favoring the interests of mothers and ‘male’ genomes as favoring the interests of fathers.
Although these papers focused on the triploid endosperm of monosporic female gametophytes, in which all nuclei were derived from a single product of meiosis, Willson and Burley (1983) and Queller (1983) both briefly discussed relatednesses of the endosperms of bisporic and tetrasporic gametophytes that contained nuclei derived from more than one product of meiosis. Their calculations assumed that meiosis I was reductional for all loci, an assumption that is mostly true for centromeres but false for loci distal to points of crossing-over. Haig (1986) wrote a brief paper on implications of kin selection for non-monosporic development that took account of crossing-over. Haig (1990) applied these ideas to the diversity of embryo-sac development. This paper is a return to these ideas, thirty years later, in the light of subsequent developments. The paper first reviews the theory of competition within megaspore tetrads, before interpreting unusual features of bisporic and tetrasporic embryo sacs in light of these conflicts. In particular, attention is drawn to the frequent loss of antipodal function and the occurrence of ‘antipodal eggs’ in non-monosporic embryo sacs. The paper then addresses the question of the adaptive significance of endosperm ploidy: why are most endosperms triploid but some are diploid, tetraploid, or other unusual genetic constitutions?
Relatedness Within Megaspore Tetrads
This paper will employ positional and functional labels to identify the members of a megaspore tetrad. The positional labels refer to a megaspore’s position relative to the micropylar and chalazal poles of its ovule. (The micropyle is the end at which pollen tubes enter the ovule. The chalaza is the end nearest the vascular supply of the ovule.) At meiosis I, a diploid megasporocyte divides to produce a micropylar dyad nucleus (D1) and a chalazal dyad nucleus (D2). At meiosis II, D1 divides to produce two megaspore nuclei (M1 toward the micropyle and M2 toward the chalaza) and D2 divides to produce two megaspore nuclei (M3 toward the micropyle and M4 toward the chalaza). Thus, a linear tetrad of megaspore nuclei are denoted M1–M2–M3–M4 proceeding from micropyle to chalaza (Figure 1A). One or more of these megaspores undergo mitotic divisions to produce the cells of the mature gametophyte (Figure 1B).
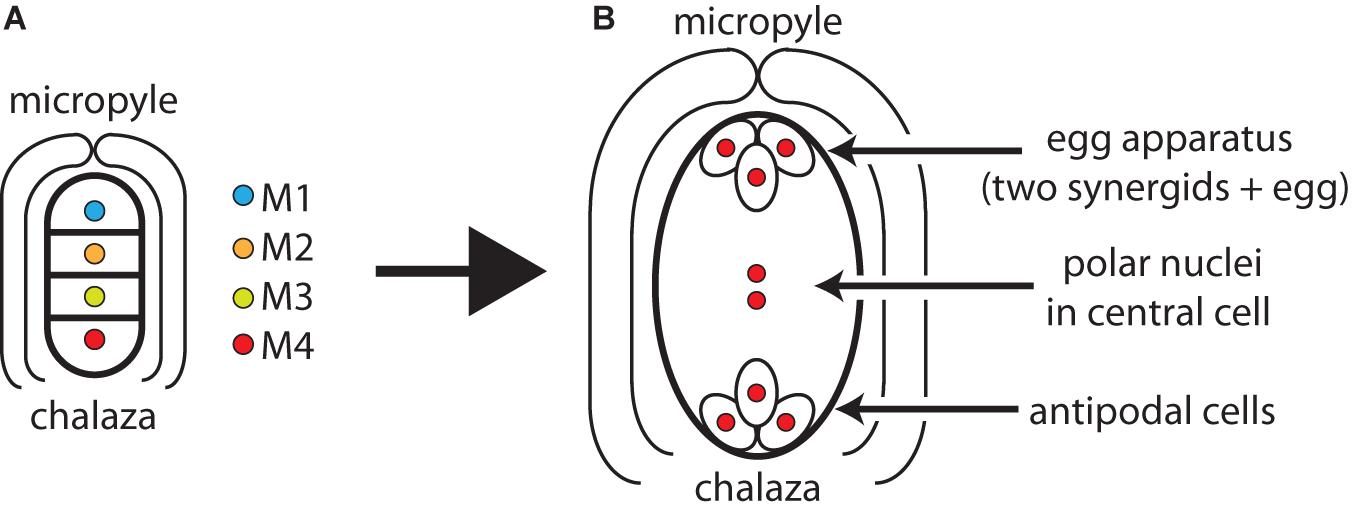
Figure 1. (A) Orientation of the four megaspore nuclei of a meiotic tetrad within an ovule. The megaspore closest to the micropyle is M1 and the megaspore closest to the chalaza is M4. (B) Cell types of a trimitotic monosporic gametophyte derived from M4 (M1–M3 suppressed): two synergids and an egg at the micropylar pole, two polar nuclei in central cell, antipodal cells at the chalazal pole.
The functional labels distinguish germinal from somatic megaspores. Germinal megaspores produce eggs and are therefore on the ‘germ-track’ whereas somatic megaspores (like somatic cells of the body) do not produce eggs and therefore cannot leave direct descendants (Haig, 1986, 1990). In most angiosperms with monosporic development, the megaspore nearest the chalaza (M4) is the germinal megaspore and the somatic megaspores (M1–M3) degenerate soon after meiosis. In angiosperms with bisporic development, either M1 or M3 is the germinal megaspore. In angiosperms with tetrasporic development, M1 is the germinal megaspore. Sometimes multiple members of a tetrad can give rise to an egg. In these cases, there is more than one germinal spore.
Three kinds of relationship exist among the megaspores produced by an outbred sporophyte: (i) megaspores from the same tetrad are ‘sisters’ if their nuclei separated at meiosis II (M1 and M2 are sisters; M3 and M4 are sisters); (ii) megaspores are ‘cousins’ if their nuclei separated at meiosis I (M1 and M2 are cousins of M3 and M4); (iii) megaspores from different meiotic tetrads are ‘half-giblings’ (gibling from ‘gametophytic sibling’). Each megaspore has one sister and two cousins but may have many half-giblings. The probability that two randomly chosen megaspores from the same tetrad share an identical-by-descent copy of a nuclear gene is one-third but this probability is one-half for megaspores from different tetrads (hence half-gibling). These probabilities can be considered the average relatedness of one megaspore to the other.
The relatednesses of sister-megaspores and cousin-megaspores differ for loci at different chromosomal locations. At centromeres, the relatedness between sisters equals one but the relatedness between cousins equals zero (under the assumption of first-division segregation of maternal and paternal centromeres). The relatednesses of sisters and cousins converge on one-third as the number of crossovers between a locus and its centromere increases (Haig, 1986, 2010). As one moves telomeric from a centromere, the relatedness of sisters first falls below one-half once there is a more-than-even chance of a crossover between the locus and its centromere. Thus, spores from different tetrads (half-giblings) have higher relatedness than spores from the same tetrad (sisters or cousins) with the exception of loci close to centromeres in sister-megaspores (Figure 2).
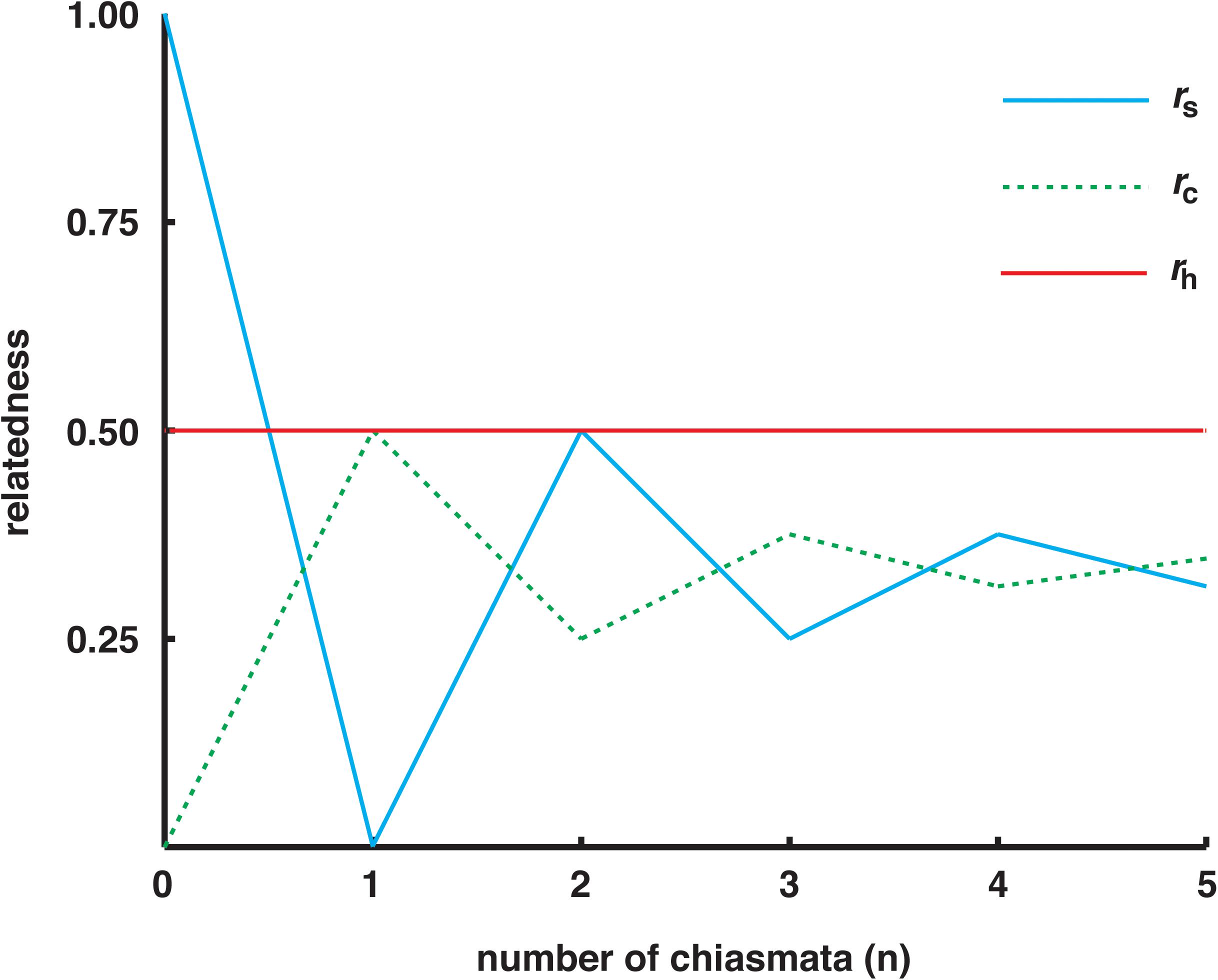
Figure 2. Probability that two megaspores share the same allele because of identity by recent common descent (‘relatedness’) as a function of the number of chiasmata between a locus and its centromere. Megaspores from different tetrads are half-giblings. Megaspores descended from the same dyad cell within a tetrad are sisters. Megaspores descended from different dyad cells within a tetrad are cousins. Relatedness to half-giblings (rh) is one-half. Relatedness to sisters (rs) and to cousins (rc) depend on the probability of second-division segregation (calculations of Mather, 1935; based on Haig, 2010).
Monosporic Development
During megasporogenesis of most seed plants, female gametophytes develop from a single member of the megaspore tetrad and the other members of the tetrad degenerate (monosporic development). All members of a tetrad would be expected to develop as female gametophytes if left to their own devices because a megaspore would not be expected to commit suicide in favor of a sister-megaspore or cousin-megaspore. Therefore, the elimination of three out of four megaspores is predicted to be imposed by the maternal sporophyte (Haig, 1986).
If segregation is unbiased, the two alleles at a diploid locus are equally likely to be inherited by each germinal megaspore (M4). Any allele that could bias its segregation to M4 would have a strong selective advantage. Abnormal chromosome 10 (Ab10) of maize is transmitted to more than 70% of the female gametophytes of heterozygous sporophytes (Rhoades, 1942, 1952). Ab10 is located in the middle of a chromosome arm and is believed to act by biasing its own segregation to outer poles (M1 and M4) at meiosis II (Rhoades, 1952). The driving haplotype encodes a minus-directed kinesin that associates with a family of 180-bp DNA repeats upon which the kinesin confers neocentromeric activity. Ab10 is believed to have originated 12 million years ago and to have driven the accumulation of more than 500 Mb of repeats in the maize genome (Dawe et al., 2018). Despite its strong transmission advantage, Ab10 has not spread to fixation because it is associated with countervailing fitness costs of reduced seed set, reduced seed weight, and reduced pollen viability (Higgins et al., 2018).
Whereas Ab10 undergoes biased transmission at meiosis II, Drive (D) of Mimulus guttatus appears to undergo biased transmission at meiosis I. D is transmitted to 60% of the offspring of female heterozygotes, balanced by costs to male fertility and seed set (Fishman and Kelly, 2015). Because D maps close to the centromere of its chromosome, it probably causes preferential transmission of both its copies to the chalazal dyad nucleus (D2) at meiosis I resulting in transmission of one of its copies to the chalazal megaspore nucleus (M4) at meiosis II.
Ab10 and D are believed to be preferentially transmitted to M4 during meiosis but similar distortion of Mendelian ratios could be attained by preferential survival of female gametophytes in competition with giblings, or of competition among sibling embryos on the same maternal sporophyte. In a study of competition within fruits of Korthalsella, Greta Stevenson (1934) realized the implications: “Characters which thus influence but one stage, e.g., rate of metabolism controlling growth of fertilized embryo sacs will be preserved, together with any characters to which they have become linked. It is thus conceivable how useless and even detrimental characters may become preserved through linkage to other characters.” There is a close conceptual relation between segregation distortion (meiotic drive) and parent–offspring conflict. Both involve an allele obtaining more than its ‘fair share’ of the reproductive investment of a heterozygous sporophyte (Haig, 1996).
Mitotic divisions of the germinal megaspore nucleus produce a multinucleate coenocyte that cellularizes to produce a multicellular haploid gametophyte. I will use the adjectives bimitotic and trimitotic to indicate the number of nuclear divisions that intervene between a megaspore nucleus and egg nucleus. The commonest forms of monosporic development among angiosperms are bimitotic (two rounds of mitosis produce a four-nucleate, four-celled gametophyte) or trimotic (three rounds of mitosis produce an eight-nucleate, seven-celled gametophyte). Bimitotic gametophytes contain two synergids and an egg cell at the micropylar end and a ‘central cell’ with ‘polar nucleus’ at the chalazal end (Figure 3A). Trimitotic gametophytes contain two synergids and an egg cell at the micropylar end, a central cell containing two polar nuclei, and three antipodal cells at the chalazal end (Figure 3B). Bimitotic gametophytes are found in early-diverging lineages of angiosperms (Williams and Friedman, 2002) and in the Onagraceae (Tobe and Raven, 1996). Most angiosperms possess trimitotic gametophytes (Friedman and Ryerson, 2009).
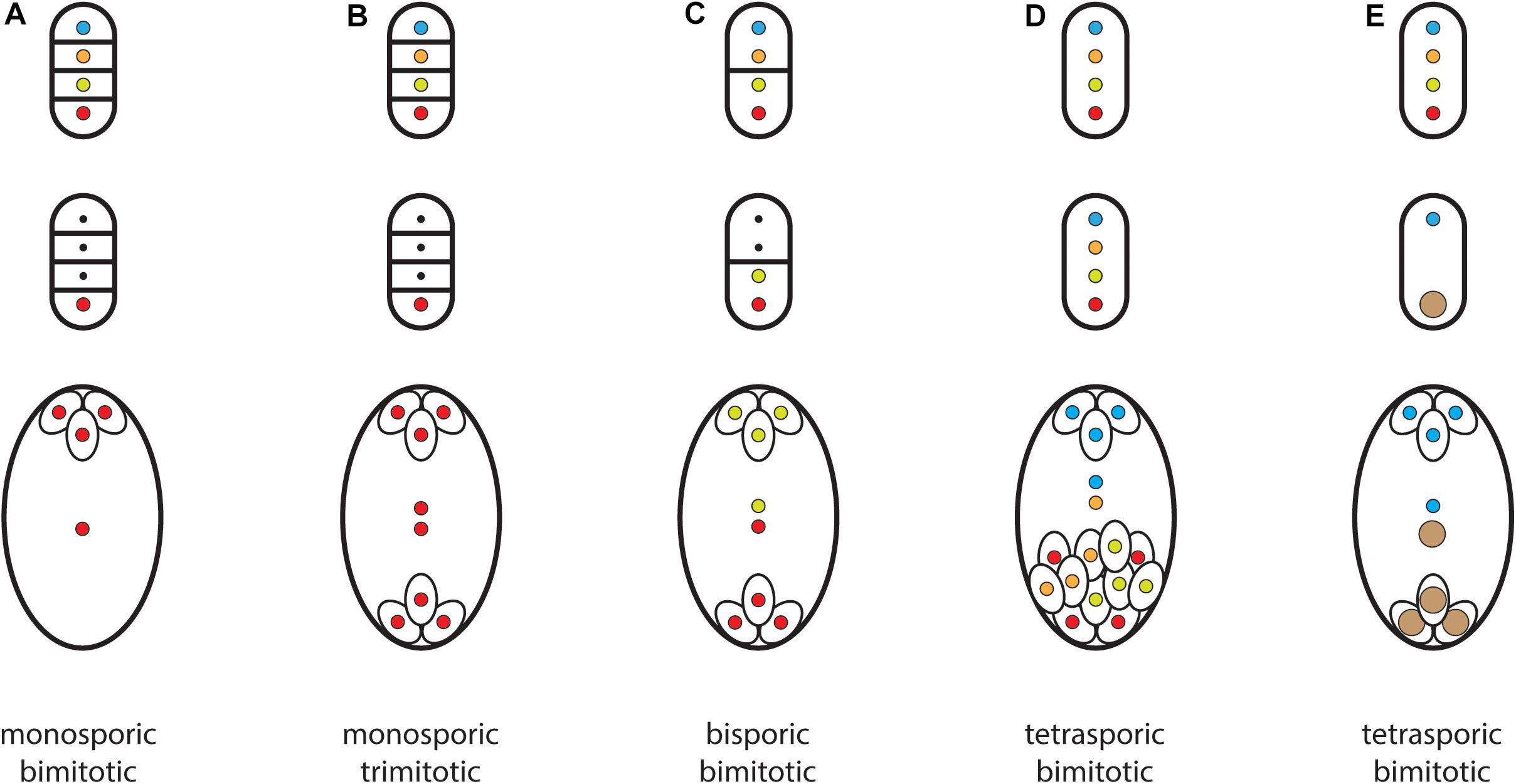
Figure 3. A sample of different kinds of embryo sacs. Top row: megaspore tetrads showing location of cell walls after meiotic divisions. Middle row: (A–C) degeneration of three or two megaspore nuclei; (E) fusion of three chalazal megaspore nuclei to produce a triploid nucleus. Bottom row: mature embryo sacs after mitotic divisions and cellularization. Nuclei are color coded to match their megaspore of origin. (A) four-celled gametophyte found in early-diverging angiosperms; (B) seven-celled gametophyte possessed by most angiosperms (‘Polygonum-type’); (C) bisporic gametophyte (‘Allium-type’); (D) tetrasporic gametophyte (‘Drusa-type’); (E) tetrasporic gametophyte (‘Fritillaria-type’).
Origin of Bisporic and Tetrasporic Development
A three-celled egg apparatus (two synergids and egg) develops at the micropylar end of monosporic female gametophytes (Haig, 1990; Tekleyohans et al., 2017; Skinner and Sundaresan, 2018). Because monosporic gametophytes usually develop from M4, the egg is located at the micropylar pole of a gametophyte that develops from a chalazal megaspore. A three-celled egg apparatus also develops at the micropylar pole of most bisporic and tetrasporic embryo sacs but now the germinal megaspore is M1 or M3 rather than M4. Therefore, most (if not all) evolutionary changes from monosporic development to bisporic or tetrasporic development changed which megaspore nucleus produces the egg apparatus (Figure 3).
Bisporic development is associated with the suppression of cytokinesis after meiosis I (Figure 3C). Tetrasporic development is associated with the suppression of cytokinesis after both meiosis I and II (Figure 3D). As a result two (or four) megaspore nuclei are present in a syncytium that develops into a chimeric embryo sac (Haig, 1990). I refer to the structures produced by bisporic and tetrasporic development as embryo sacs rather than ‘gametophytes’ because I view these chimeric structures as containing two (bisporic) or four (tetrasporic) haploid organisms.
In monosporic development, the egg apparatus is organized from a group of four nuclei at the micropylar pole of the syncytial embryo sac. These nuclei are derived from the chalazal megaspore of the tetrad (M4). In bisporic and tetrasporic embryo sacs, the products of the M4 are restricted to the chalazal pole and the egg apparatus develops from a different megaspore, either M3 in bisporic development or M1 in tetrasporic development. Thus, what would otherwise have been an aborted somatic spore in monosporic development, now produces the functional egg of a bisporic or tetrasporic embryo sac. This suggests a scenario for the origin of bisporic and tetrasporic development from monosporic development. In a population polymorphic for a costly driver that preferentially segregated to M4, genes in megasporocytes would have benefited from the suppression of meiotic cytokinesis because this changed which megaspore produced the functional egg and would have increased the likelihood of non-driving alleles segregating to functional eggs. Variations of this scenario can be imagined. For example, a dominant mutation could cause the suppression of meiotic cytokinesis and benefit by being preferentially transmitted to the new germinal megaspore, or a mutation that suppressed meiotic cytokinesis could result in more than one megaspore developing as a germinal spore thus negating preferential transmission of a driving haplotype to a particular position within the tetrad.
An alternative scenario posits that the initial change from monosporic to bisporic or tetrasporic development was selectively neutral. Because of the loss of cytokinesis at meiosis I or at both meiotic divisions, gametophytic development was initiated from two or four megaspore nuclei rather than one megaspore nucleus. All megaspore nuclei initially responded to the positional cues within the embryo sac cytoplasm to produce a functional ‘gametophyte’ with a single micropylar egg. However, the functional development of somatic spores was then subject to relaxed selection because of reduced relatedness to the egg or positive selection to become a germinal spore. Under this scenario, bisporic and tetrasporic embryo sacs initially formed coordinated chimeric ‘gametophytes’ but this initial state of cooperation was evolutionarily unstable. Under either scenario, bisporic and tetrasporic embryo sacs evolve toward ‘functional monospory’ because of deterioration of functions performed by the descendants of somatic spores or toward competition between two or more ‘monosporic’ gametophytes, each forming an egg, within a single embryo sac.
Bisporic Bimitotic Embryo Sacs
Bisporic development results from failure of cytokinesis after meiosis II. As a result, the megaspore tetrad is comprised of two cells each containing two megaspore nuclei. The embryo sac develops from one of these dyad cells by two rounds of mitotic division and the other dyad cell is suppressed. More commonly it is the micropylar dyad cell (D1 containing nuclei M1 and M2) that is suppressed and the chalazal dyad cell (D2 containing nuclei M3 and M4) that undergoes further development. After two rounds of mitotic division of M3 and M4, the embryo sac contains a micropylar quartet of nuclei (derived from M3) and a chalazal quartet of nuclei (derived from M4). Sometimes, one or more divisions of M4 is suppressed.
Bisporic bimitotic embryo sacs are idealized as the ‘Allium-type.’ This idealized type has the same arrangement of cells and nuclei as ‘Polygonum-type’ monosporic trimitotic gametophytes except that the micropylar quartet of nuclei are derived from M3 (egg apparatus and upper polar nucleus) and the chalazal quartet of nuclei are derived from M4 (lower polar nucleus and antipodal cells) (Figure 3C). The ‘Endymion-type’ is similar except that the micropylar quartet develops from M1 and the chalazal quartet from M2. My discussion will refer to the ‘Allium-type’ but applies equally to the ‘Endymion-type’ with M1 substituted for M3 and M2 for M4.
Why does the evolution of bisporic development involve a direct transition from monosporic trimitotic development to bisporic bimitotic development (seemingly changing two things at once) rather than via an intermediate stage of bisporic trimitotic development? Haig (1990) proposed a simple explanation. Monosporic trimitotic development has two divisions with cytokinesis (meiosis I and II), then two divisions without cytokinesis (first and second mitosis), followed by cellularization after third mitosis. Bisporic bimitotic development has one division with cytokinesis (meiosis I), then two divisions without cytokinesis (meiosis II and first mitosis), followed by cellularization after second mitosis. Mechanistically, meiosis II is a form of mitosis (although with different genetic consequences because of crossing-over in prophase of the preceding division). The evolutionary transition to bisporic bimitotic development simply ‘skips’ one cytokinetic division after meiosis I.
All nuclei of monosporic gametophytes are descended from the same megaspore nucleus and have therefore evolved to work together to further the fitness of the embryo produced by the egg-cell. Consider the action of natural selection on mutations that affect antipodal function. A recent mutation will be expressed in 50% of monosporic embryo-sacs. The mutation will be present in all nuclei of monosporic embryo-sacs, including the egg cell. Such a gene benefits from the successful production of embryos by the embryo-sacs in which it is expressed. Beneficial mutations will be favored by natural selection, deleterious mutations disfavored. In monosporic development, antipodal functions will be maintained by natural selection when they benefit the egg-cell’s embryo.
Now consider the fate of a recent mutation that affects antipodal function in species with bisporic development. The mutant allele will initially be present in heterozygous sporophytes. The allele present in the egg nucleus will frequently be different from the allele present in antipodal nuclei because the egg nucleus and antipodal nuclei of bisporic embryo sacs are descended from sister-megaspore nuclei. The strength of selection to maintain antipodal functions is diminished in proportion to this mismatch. For a mutation at an ‘average locus,’ the egg and antipodal nuclei will possess matching alleles one-third of the time and mismatching alleles two-thirds of the time (Figure 2). Therefore selection to maintain antipodal function is weaker in bisporic than monosporic development. Moreover, if embryos of different ovules compete for maternal investment, there may be positive selection to impair antipodal function in ovules in which a mutant allele is transmitted to M4 if this benefits embryos in other ovules where the mutant allele is transmitted to M3. For these reasons, antipodal functions of bisporic embryo sacs will be poorly maintained by natural selection.
Mutations that convert M4 into a germinal spore would cause the development of an egg-apparatus at the chalazal pole of the embryo sac (descended from M4) to compete for fertilizations with the egg-apparatus at the micropylar pole (descended from M3). The mutation could increase in frequency in the gene pool if chalazal eggs were occasionally fertilized in the place of micropylar eggs. By contrast, there is no selective advantage for a similar allele expressed at the chalazal pole of a monosporic gametophyte because the egg apparatus and antipodals are derived from the same megaspore and necessarily carry the same allele (Haig, 1990).
I will illustrate these evolutionary dynamics with examples from the Amaryllidaceae, with a particular focus on the genus Scilla which contains species with monosporic, bisporic, and tetrasporic development and the genus Allium characterized by bisporic development. Scilla species with monosporic development have endopolyploid antipodal cells that are proposed to play a role in embryo sac nutrition (Battaglia and Feeley, 1959; Nagl, 1976). Monosporic development and endopolyploid antipodals are ancestral features of the genus (Svoma, 1981). However, in species with bisporic (or tetrasporic) embryo sacs, antipodal cells rapidly degenerate without polyploidization (Svoma, 1981; Svoma and Greilhuber, 1988). The monophyletic Scilla siberica group have bisporic gametophytes with usually ephemeral antipodal cells, but sometimes the three cells at the chalazal pole (descended from M4) resemble the egg cell with two synergids (descended from M3) at the micropylar pole (Svoma and Greilhuber, 1989). The obvious interpretation is that descendants of M3 and M4 are competing to produce an embryo.
Ephemeral antipodals are typical in bisporic Allium species (Elmore, 1898; Porter, 1936; Murphy, 1946; Syamasundar and Panchaksharappa, 1975; Khalelle and Mitchell, 1982; Ashurmetov et al., 2001; Nadirashvili et al., 2006; Ismailoglu et al., 2010) and are presumably ancestral characters of the genus. Often, one of the synergids is highly polyploid and is believed to perform a nutritive function for the early embryo. These patterns are consistent with a model in which antipodal functions were lost at the chalazal pole of bisporic embryo sacs and a synergid has evolved to replace these functions.
At first sight, this interpretation is challenged by Hasitschka-Jenschke’s (1957) report of an endopolyploid antipodal cell in Allium ursinum. A close inspection of her figures reveals a striking symmetry between the micropylar and chalazal poles of the embryo sac: at both poles there are two cells with haploid nuclei and one cell with a highly polyploid nucleus. Hasitschka-Jenschke interpreted the micropylar polyploid cell as a synergid and the chalazal polyploid cell as an antipodal. My alternative interpretation is that the two megaspore nuclei of the bisporic embryo sac have both developed as germinal spores. Each megaspore has given rise to one egg cell, one haploid synergid, one polyploid synergid, and has contributed a polar nucleus to the central cell.
In a subsequent paper, Hasitschka-Jenschke (1958) reported two species with polyploid synergids and ephemeral haploid antipodals (Allium ammophilum, Allium angulosum) and one species with two polyploid synergids at the micropylar pole and two polyploid antipodals at the chalazal pole (Allium pulchellum; note again the symmetry between the poles). I conjecture that ancestral antipodal functions have been lost from the derivatives of a somatic nucleus at the chalazal pole in Allium ammophilum and Allium angulosum but that the chalazal megaspore nucleus has differentiated as a germinal spore in Allium pulchellum. In this case, each of the two megaspore nuclei has developed into an egg cell, two polyploid synergids, and one polar nucleus.
The development of eggs and embryos at the chalazal pole of Allium embryo sacs has been documented many times since Tretjakow (1895) reported antipodal embryos in Allium odorum. Weber (1929) reported that antipodals degenerated before fertilization in most Allium species but were persistent and resembled an egg and two synergids in Allium paradoxum. Håkansson (1951) reported “In most embryosacs [of Allium senescens] the antipodals were differentiated as an egg-apparatus, one antipodal having the appearance of an egg-cell while two were more or less similar to synergids. … The close similarity of the two groups of cells is striking. One synergid in each group has increased strongly in size, as is usual in unfertilized embryosacs of Allium. The nucleus and the cytoplasm of these antipodal cells are changed as in normal synergids.” Vinogradova (2018), similarly, reported the regular formation of an egg-apparatus at both the micropylar and chalazal poles of Allium ramosum embryo sacs. Specht et al. (2001) observed twin seedlings emerging from seeds in 25 out of 26 Allium taxa. The highest level of twinning was observed in Allium splendens with twin seedlings germinating from 32% seeds. The two seedlings emerged from opposite ends of the former embryo sac in cleared ovules of Allium tuberosum. The interpretation of twinning as the outcome of fertilization of two sexual egg cells is complicated by the widespread occurrence of apomictic polyembryony in Allium.
These taxonomic patterns are compatible with a model in which antipodal functions deteriorate in lineages with bisporic embryo sacs. Concurrently, mutations may be favored that convert chalazal somatic spores into germinal spores that produce a functional egg. The rapid degeneration of cells at the chalazal pole in many species with bisporic embryo sacs has been proposed to reflect positive selection by genes expressed in chalazal spores to ‘sabotage’ development in favor of other embryo sacs (Haig, 1986) or as ‘suppression’ of chalazal cells by genes expressed in the germinal spore to prevent the formation of chalazal eggs (Haig, 1990). These are not mutually exclusive hypotheses: genes expressed in chalazal nuclei may have been selected both to suppress ancestral antipodal functions and to form an egg apparatus; genes expressed in micropylar nuclei may have been selected both to compensate for lost antipodal functions and to suppress activities of chalazal nuclei.
Tetrasporic Embryo Sacs
Tetrasporic development occurs when neither meiotic division is followed by cytokinesis. As a result, the megaspore tetrad consists of four nuclei in a shared cytoplasm. The micropylar megaspore nucleus (M1) is a germinal spore and divides twice before organizing as an egg apparatus (two synergids and an egg) and a polar nucleus (Figure 3D). The nuclear descendants of somatic megaspore nuclei (M2–M4) have divergent genetic interests from the nuclear descendants of M1. Therefore, similar perturbations of ‘regular’ development are predicted at the chalazal pole of tetrasporic embryo sacs to those observed in bisporic embryo sacs.
A mutation that affected antipodal function would be expressed in all embryo sacs of heterozygous sporophytes with tetrasporic development of the gametophyte generation. In 50% of ovules, the mutation would be present in M1 and one other megaspore. In 50% of ovules, the mutation would be absent from M1 but present in two of the three chalazal megaspores. Precise predictions depend on the degree of ‘dominance’ of the mutation, the location of the mutation relative to its centromere, and whether expression differs in descendants of M2 (sister of M1) versus descendants of M3 and M4 (cousins of M1). I conjecture (i) that selection to maintain antipodal function is weaker in tetrasporic development than in monosporic development but stronger than in bisporic development and (ii) that mutations that cause development of antipodal eggs are favored in those forms of tetrasporic development in which descendants of the somatic megaspores remain haploid.
Most tetrasporic embryo sacs are bimitotic. In the ‘Drusa-type’ of development, the megaspore nuclei each divide twice to generate a 16-nucleate embryo sac that cellularizes as two synergids plus an egg and upper polar nucleus descended from M1 and a lower polar nucleus and eleven antipodal cells descended from M2–M4 (Figure 3D). This idealized ‘type’ is rarely, if ever, observed in real plants. In particular, the ‘typical’ number of eleven antipodal cells is highly atypical because of failures to divide and degeneration of nuclei at the chalazal pole (Haig, 1990). For example, species of Scilla (Svoma, 1981; Svoma and Greilhuber, 1989) and Ulmus (Shattuck, 1905; Ekdahl, 1941; Walker, 1950) with ‘Drusa-type’ embryo sacs usually possess fewer than eleven antipodal cells.
The extreme form of chalazal suppression occurs in species in which the three somatic megaspores degenerate without further division and the mature embryo sac contains four uninucleate cells (two synergids, an egg and central cell) descended from the germinal spore (M1) perhaps containing remnants of the degenerated megaspore nuclei. Such embryo sacs closely resemble the monosporic bimitotic gametophytes of Figure 3A. They have been classed among tetrasporic embryo sacs solely because of the failure of cytokinesis after the meiotic divisions. Gametophytes of this kind have been described in Clintonia (Smith, 1911; Pahuja and Kumar, 1970) and Melampyrum (Greilhuber, 1973).
As was the case for bisporic embryo sacs, mutations that are expressed in the descendants of somatic spores in tetrasporic embryos sacs will be favored that cause chalazal nuclei to develop as eggs that compete with eggs descended from germinal megaspore nuclei. Consistent with this expectation, additional eggs and embryos have been observed at the chalazal pole of Ulmus embryo sacs (Shattuck, 1905; Ekdahl, 1941). In some taxa, all four megaspore nuclei function as germinal spores and produce four egg apparatuses in a tetrapolar arrangement (Stephens, 1909; Sateishi, 1927; Maheshwari and Johri, 1941; Mukherjee, 1958). Embryo sacs of this kind are known as ‘Penaea-type’ or ‘Acalypha indica-type’ (the differences between these ‘types’ need not concern us here).
In several tetrasporic bimitotic taxa, the three somatic spores of the megaspore tetrad fuse to form a triploid nucleus (Figure 3E). This has been known as the ‘Fritillaria-type’ (Maheshwari, 1937, 1946b) since Bambacioni (1928) described the triple fusion of chalazal megaspore nuclei in Fritillaria persica. Triple fusion is characteristic of many members of the Liliaceae and similar fusions have independently evolved in Hyacinthoides vincentia (Ebert and Greilhuber, 2005) and several species of Piper (Madrid and Friedman, 2009) among other taxa. Chalazal triploidy may stabilize the development of tetrasporic embryo sacs because it forecloses the possibility of chalazal megaspore nuclei leaving sexual descendants by their conversion into egg-producing germinal spores. Contrary to this expectation, Battaglia (1947) reported development of triploid antipodal eggs in Rudbeckia flava.
In the tetrasporic bimitotic embryo sacs discussed so far, synergid nuclei are derived from the same megaspore nucleus as the egg nucleus and upper polar nucleus. Therefore, synergids are expected to function for the benefit of their associated egg cell. In the so-called ‘Adoxa-type,’ there is only one mitotic division of the four megaspore nuclei to produce an eight-nucleate embryo sac. In the idealized form of these tetrasporic unimitotic embryo sacs, synergids are derived from a different megaspore nucleus than the egg nucleus and upper polar nucleus. This is evolutionarily problematic because synergids would be selected to impede fertilization of the associated egg or to develop as an alternative egg that competed for fertilization. Historically, many species that had been described as possessing ‘Adoxa-type’ embryo sacs were subsequently reinterpreted as possessing bimitotic development so that the micropylar quartet of synergids, egg and upper polar nucleus are derived from a single megaspore (Fagerlind, 1939b; Maheshwari, 1946a).
The embryo sacs of Adoxa and Sambucus are the only generally accepted exemplars of the ‘Adoxa-type.’ A single mitotic division of the megaspore nuclei produces an eight-nucleate embryo sac. In Adoxa moschatellina, pollen tubes do not enter the embryo sac via a ‘synergid’ and embryo sacs may produce multiple embryos (Czapik, 1976). In Sambucus racemosa, multiple pollen tubes enter individual embryo sacs. The groups of cells at micropylar and chalazal poles have very similar structure and eggs cells can only be identified retrospectively by fertilization. Up to three embryos can be found at the micropylar pole and embryos have been observed at both the micropylar and chalazal pole of an embryo sac (Tokc, 1980). I hypothesize that most cells of Adoxa and Sambucus embryo sacs are potential eggs and dedicated synergids are absent.
Plumbaginaceae is the sister family to Polygonaceae in which monosporic trimitotic development was first clearly described in Polygonum divaricatum (Strasburger, 1879, pp. 5–7). Tetrasporic development is present in all members of the Plumbaginaceae but differs between the two subfamilies: bimitotic in Staticeae; unimitotic in Plumbagineae (Boyes and Battaglia, 1951a). The most parsimonious interpretation is that tetrasporic bimitotic development evolved from monosporic trimitotic development in an ancestor of the Plumbaginaceae, then tetrasporic unimitotic development evolved from tetrasporic bimitotic development in an ancestor of the Plumbagineae.
In the Staticeae, M1 divides twice to produce the standard quartet of two synergids, an egg and upper polar nucleus but the behavior of the descendants of M2–M4 shows great variation. In several species, the three lower megaspore nuclei fuse to produce a triploid nucleus (Fagerlind, 1939a, b; D’Amato, 1940; Roís et al., 2016) as also observed in the Liliaceae, Euphorbiaceae and Piperaceae. In at least one species, Statice japonica, the second sperm fuses only with the haploid upper polar nucleus (descended from M1) and not with the triploid lower polar nucleus (descended from M2–M4) (Ya-e, 1941). This could be considered a pseudomonosporic gametophyte with diploid endosperm. ‘Anomalous’ embryo sacs are reported with more than one egg (Dahlgren, 1916; D’Amato, 1940). Fagerlind (1938) reported a 16-nucleate embryo sac in Statice eu-limonium in which there were four egg apparatuses and four polar nuclei in the central cell.
Tetrasporic unimitotic development in the Plumbagineae differs from that observed in Adoxa and Sambucus (Adoxaceae). Each megaspore nucleus divides once to produce a single peripheral cell and a polar nucleus in the central cell. These peripheral cells are usually interpreted as an egg, two lateral cells on opposite sides of the embryo sac, and a single chalazal antipodal cell (Boyes and Battaglia, 1951b). The egg possesses synergid-like characters such as wall ingrowths resembling a filiform apparatus (Cass and Karas, 1974; Russell and Cass, 1988). Sometimes more than one of the peripheral cells has egg-like properties (Dahlgren, 1916, 1937; Haupt, 1934; D’Amato, 1940; Mathur and Khan, 1941; Boyes and Battaglia, 1951b). Often the peripheral cells degenerate so that the mature embryo sac is two-celled: an egg-synergid and a central cell with tetraploid fusion nucleus (Haupt, 1934; Boyes and Battaglia, 1951b; Cass, 1972). In Plumbagella micrantha, three megaspore nuclei at the chalazal end of the embryo sac fuse to from a triploid nucleus. After the single mitotic division, the mature embryo sac is three-celled: an egg synergid, a central cell containing a haploid and triploid polar nucleus and a triploid antipodal cell (Boyes, 1939; Russell and Cass, 1988).
The two major themes of this section are reprised in the above review of tetrasporic development in Plumbaginaceae. First, the descendants of M2–M4 exhibit highly variable behavior and often are ephemeral. Second, the descendants of M2–M4 differentiate as egg-like cells in some embryo sacs.
Monosporic and Tetrasporic Development in Gymnosperms
Female gametophytes of angiosperms usually produce a single egg per ovule. Therefore, competition for maternal resources occurs among embryos in different ovules. By contrast, most gymnosperms have female gametophytes with multiple eggs that give rise to multiple embryos, only one of which survives in the mature seed (Haig, 1992a). Therefore, competition for maternal resources occurs among embryos within gymnosperm ovules.
Mature female gametophytes of gymnosperms are substantial structures. An early coenocytic stage of nuclear proliferation produces hundreds to thousands of nuclei distributed around a large central vacuole. This coenocytic stage is followed by a process of cellularization in which the vacuole is centripetally ‘filled in.’ Multiple egg-containing archegonia are differentiated, usually at the micropylar pole of the gametophyte (Singh, 1978). Development is usually monosporic. All of the eggs of a monosporic gametophyte are descended from a single germinal megaspore (usually M4) and therefore possess identical haploid genomes. After fertilization of multiple eggs by multiple pollen tubes, the resulting embryos compete for control of the ovule (Haig, 1992a), selecting among the pollen-derived nuclear genomes of the embryos because all embryos possess the same egg-derived nuclear genomes. Many conifers inherit their plastids, and sometime their mitochondria, from their pollen-parent (Mogensen, 1996). In these cases, competition among embryos also tests paternal nucleocytoplasmic combinations.
Among extant Gnetales, Ephedra has monosporic development with regular cellularization of the central vacuole and formation of multicellular archegonia (Berridge and Sanday, 1907; Maheshwari, 1935; Lehmann-Baerts, 1967) but Gnetum and Welwitschia exhibit tetrasporic development, with irregular cellularization of the central vacuole and the formation of ‘naked’ egg cells without organized archegonia (Vasil, 1959; Martens, 1963, 1971; Carmichael and Friedman, 1996; Friedman and Carmichael, 1998; Friedman, 2015a). The irregular features of these tetrasporic ‘embryo sacs,’ including multinucleate cells and nuclear fusions, are probably evolutionary consequences of the destabilization of regular development by competition among nuclear descendants of the four megaspore nuclei to produce the successful embryo of the ovule. This competition is most evident in Welwitschia mirabilis where egg nuclei form ‘prothallial tubes’ (Friedman, 2015b) that grow upwards to meet pollen tubes growing down from the micropyle. After fertilization, Welwitschia embryos race back into the nutritive tissue of the embryo sac (Pearson, 1929; Martens, 1971; Haig, 1987; Friedman, 2015a).
Tetrasporic development has independently evolved in Cupressus sempervirum (El Maâtaoui et al., 1998) but is absent in other members of the genus (Ceccherini and Raddi, 2009). Unfortunately, female gametophytic development has not been described in detail in this species beyond reports of an initial free nuclear phase followed by cellularization and organization of archegonia (El Maâtaoui et al., 1998; Mehra and Malhotra, 1947). There are hints of perturbed development of the female gametophyte relative to related species with monosporic development (as also seen in the comparison between tetrasporic Gnetum and Welwitschia and monosporic Ephedra). Sugihara (1956) briefly noted that archegonial complexes lacked the common jacket layer characteristic of other members of the Cupressaceae. Mehra and Malhotra (1947) reported proliferation of more than 6000 nuclei before cellularization, the highest number recorded in a gymnosperm, and that “an attempt at the formation of an archegonial complex such as is characteristic of the genus takes place at the micropylar end of the gametophyte, but nowhere well formed archegonia have been observed.” Moreover, “In rare cases attempts at formation of isolated archegonia or a complex of a few archegonia in the lateral region beside their occurrence at the micropylar end have been observed.” Flow cytometry of the nutritive tissue of female gametophytes provides evidence of multinuclear compartments and nuclear fusions (Pichot and El Maâtaoui, 1997; El Maâtaoui and Pichot, 1999). Once again, gametophyte development has become ‘disorderly’ once it contains nuclei of different genotypes.
Polar Nuclei and Endosperm
Polar nuclei are located in the central cell of angiosperm embryo sacs. The historical reason for this peculiar nomenclature appears to have been that one nucleus originated at each pole before both moved to meet each other at an equatorial location (Fischer, 1880, p. 95). Nawaschin (1898) showed that fertilization of the egg cell in Lilium martagon and Fritillaria tenella was accompanied by fusion of a second sperm cell with the two polar nuclei. He considered the embryo and endosperm produced by this double fertilization to be a pair of unequally developing twins (“einer Paares sich ungleich entwickelnder Zwillinge”). This section will consider the implications of different forms of embryo sac development for the genetic constitution of the polar nuclei and resulting endosperms.
Endosperm of Monosporic Gametophytes
In monosporic bimitotic gametophytes, the egg and polar nucleus are mitotic sisters. The two sperm nuclei released by a pollen tube are also mitotic sisters. Therefore, the diploid embryo and endosperm are genotypically identical but phenotypically distinct. In evolutionary terms, the embryo and endosperm of a seed can be considered parts of a an integrated diploid organism. I will use ‘embryosperm’ to refer to the combination of a diploid embryo and genetically identical diploid endosperm. In an embryosperm, evolutionary conflicts are absent between embryo and endosperm but are present within either tissue between maternally derived and paternally derived genomes.
Female gametophytes in different ovules of the same sporophyte are half-giblings (rh = ½). Consider the simple case in which two ovules are fertilized by unrelated pollen grains (rp = 0). The embryosperms of the two seeds are half-siblings. What is the relatedness of half-siblings? The traditional answer was r = ¼, the average of rh = ½ and rp = 0. This averaging assumed that maternally derived and paternally derived alleles were indistinguishable in their effects. However, if genes have different effects when maternally derived and paternally derived, as occurs with genomic imprinting, then alleles will evolve to express different genetic interests in the two roles. Maternally derived alleles expressed in embryosperms will evolve to treat their own embryo (re = 1) as twice as valuable as a half-sibling (rh = ½) whereas paternally derived alleles in embryosperms will evolve as if maternal half-siblings were without value (rp = 0).
Most angiosperms possess monosporic trimitotic gametophytes and triploid endosperms. The egg nucleus and upper polar nucleus are mitotic sisters as are the two sperm nuclei that fertilize the egg and polar nuclei. The lower polar nucleus is descended from the same megaspore nucleus as the egg nucleus and upper polar nucleus. What is the evolutionary significance of a triploid endosperm as compared to a diploid endosperm? Westoby and Rice (1982) and Queller (1983) argued that addition of the second polar nucleus increased the relatedness of the endosperm to embryos in other seeds with the same mother but different fathers. Both polar nuclei’s relatednesses to the eggs of other ovules are one-half (rh = ½) whereas a sperm’s relatedness to the sperm of unrelated pollen tubes is zero (rp = 0). These authors averaged the relatednesses of the three nuclei that contributed to endosperm to obtain an average relatedness of one-third. This gave double weight to the maternal relatedness (rh = ½) and single weight to the paternal relatedness (rp = 0). Since, the average relatedness of one-third was greater than r = ¼ for half-siblings, they argued that maternal provisioning of a triploid endosperm reduced competition among offspring and therefore alleviated mother–offspring conflict as compared to direct provisioning of a diploid embryo.
The idea that triploidy reduced mother–offspring conflict is appealing but the averaging of relatednesses bothered me when I considered this problem as a doctoral student with Westoby in the 1980s. One worry was the averaging of maternal and paternal coefficients of relatedness. This concern led to my recognition of an internal conflict between maternally derived and paternally derived genomes of offspring in all organisms with postzygotic maternal care (Haig and Westoby, 1989). A more perplexing problem was the double weight given to the two polar nuclei. The relatedness of a polar nucleus to an egg in another ovule (rh = ½) should be the same for both polar nuclei and should remain unchanged whether the two polar nuclei sit side-by-side or fuse to form a diploid secondary nucleus. Why should the fusion of polar nuclei with a sperm nucleus change their relatedness to diploid embryos? My preferred answer to this question is that triploidy does not change relatedness: maternally derived genes in endosperm have relatedness rh = ½ to maternal half-siblings whereas paternally derived genes have relatedness rp = 0 to maternal half-siblings. The addition of an extra maternally derived genome does not change relatedness but increases the power of maternally derived genes to exert their interests simply because of their numerical advantage relative to paternally derived genes (Willson and Burley, 1983; Haig, 2016). These reservations about ‘average relatedness’ also apply to the coefficients of relatedness derived by Friedman et al. (2008) for various endosperm types.
As belatedly noted by Haig and Westoby (2006), our theory of ‘parent-specific gene expression’ was prefigured in passages from section 1.2 of Mate choice in plants (Willson and Burley, 1983) that argue for the evolution of ‘sex-limited expression’ of alleles in response to genetic conflict between the ‘male portions’ and ‘female portions’ of offspring (p. 19). As a subtle difference between the two presentations, Willson and Burley (1983) equated the interests of ‘female’ portions of zygotes (or endosperms) with those of mothers (e.g., “the interests of the female portion of the zygote should be coincident with those of its mother”, p. 19) and equated the interests of ‘male’ portions with those of fathers. Haig and Westoby (1989), on the other hand, framed their theory as a conflict between ‘maternally derived’ and ‘paternally derived’ genes expressed in offspring because we recognized that these had distinct interests from genes expressed in mothers and fathers (see the distinction between ‘madumnal/padumnal’ and ‘maternal/paternal’ in Haig, 1996). In the final stages of writing the present paper, I re-read section 3.3 of Mate choice in plants. In that section, Willson and Burley (1983) apply their ideas to the evolution of triploid endosperm and conclude that duplication of the ‘female’ genome increased female ‘influence’ without changing patterns of relatedness (p. 83) and that “increases in ploidy are most likely the result of male-female conflict operating at the level of the gene” (p. 87). These conclusions anticipate interpretations of Haig (2016) and the current paper. I apologize for this additional oversight.
Endosperm of Bisporic Embryo Sacs
The upper and lower polar nuclei of monosporic trimotic female gametophytes are genetically identical but may exhibit a ‘division of labor.’ Thus, the lower polar nucleus of monosporic Arabidopsis thaliana carries the CKI1 histidine kinase to the upper polar nucleus to enable nuclear fusion and subsequent development as endosperm (Yuan et al., 2016). The upper and lower polar nuclei of bisporic embryo sacs may also express different functions but, in this case, the nuclei are genetically distinct and cannot be assumed to be ‘on the same side.’
The polar nuclei of bisporic embryo sacs are descended from sister-megaspores. The upper polar nucleus is the mitotic-sister of the egg nucleus but the lower polar nucleus is derived from a different megaspore with an ‘average’ relatedness of a third to its own egg and a half to eggs in other ovules. Triploid endosperms of species with bisporic development will have three different genetic factions (Rutishauser, 1956): genes derived from the upper polar nucleus, from the lower polar nucleus, and from the sperm nucleus. Genes derived from the sperm nucleus and the upper polar nucleus will value their own embryo more highly than its half-siblings, but to different degrees, whereas genes derived from the lower polar nucleus will value other embryos more highly than their own embryo. Three-party relationships are inherently less stable than two-party relationships.
In Allium atroviolaceum and Allium rotundum, the lower polar nucleus is heterochromatic and transciptionally inactive before it fuses with the upper polar nucleus. After fusion of the polar nuclei, the heterochromatic chromosomes derived from the lower polar nucleus are then extruded from nuclei early in endosperm development so that the mature endosperm is diploid rather than triploid (Gvaladze, 1979; Gvaladze et al., 2002). Therefore, the embryo and mature endosperm are parts of a single genetic individual, an embryosperm, descended from a four-nucleate monosporic gametophyte. Perhaps, the maternal genome of the upper polar nucleus and the paternal genome of the second sperm nucleus have ‘ganged-up’ against the lower polar nucleus to exclude it from their relationship.
Endosperm of Tetrasporic Embryo Sacs
The genetic composition of endosperm is variable among tetrasporic embryo sacs. Diploid endosperms are produced in some taxa with tetrasporic development. In the genus Clintonia (Liliaceae), no walls separate the nuclei of the megaspore tetrad but derivatives of the three lower megaspore nuclei degenerate, and a diploid endosperm is formed by fertilization of a single polar nucleus that is mitotic sister of the egg (Smith, 1911; Pahuja and Kumar, 1970). Similarly, in the tetrasporic embryo sacs of Statice japonica (Plumbaginaceae) and the genus Melampyrum (Scrophulariaceae), the second sperm fuses only with the upper polar nucleus (Ya-e, 1941; Greilhuber, 1973). These female gametophytes can be considered ‘functionally monosporic’ with genetic identity of embryo and diploid endosperm (an embryosperm).
The central cell of the 16-nucleate embryo sacs of Manekia naranjoana (Piperaceae) contains two polar nuclei that would give rise to a triploid endosperm after double fertilization. This condition has been characterized as ‘functionally bisporic’ (Arias and Williams, 2008). The central cell of the 16-nucleate tetrasporic embryo sacs of Penaeaceae contains four polar nuclei (one derived from each of the megaspore nuclei) (Stephens, 1909). This arrangement would give rise to a pentaploid endosperm after double fertilization. The central cell of Peperomia hispidula contains 14 ‘polar nuclei’ (Johnson, 1914) that would produce a 15-ploid endosperm if fertilized. Examples of many other genetic compositions of tetrasporic endosperm could be given but I will limit myself to consideration of the endosperm of species from the Liliaceae in which the lower polar nucleus is triploid.
One of the ironies of plant embryology is that double fertilization was first described in highly atypical Lilium and Fritillaria. These embryo sacs were subsequently found to produce a pentaploid endosperm because the lower polar nucleus derived from the fusion of three chalazal megaspore nuclei. Every endosperm within every seed of a maternal sporophyte contains a genome derived from each of the megaspores of a meiotic tetrad plus a genome derived from a sperm nucleus that is the mitotic sister of the sperm that fertilized the egg. All of these endosperms are genetically identical with respect to their tetraploid maternally derived genotype but differ with respect to their haploid paternally derived genotypes (Rutishauser, 1956).
Genes expressed in maternal sporophytes are expected to be impartial among embryos of different seeds. The same might be expected of the combined maternally derived genomes of the upper (haploid) and lower (triploid) polar nuclei of the ‘Fritillaria-type’ because the polar nuclei combine all four products of female meiosis and thus collectively duplicate the maternal sporophytic genome. However, the polar nuclei are descended from haploid products of meiosis and may have acquired cues, subsequent to meiosis, as to whether they are descended from the micropylar germinal spore (M1) or chalazal somatic spores (M2, M3, M4). As a consequence, the genome of the haploid upper polar nucleus may not be on the ‘same side’ as the genome of the triploid lower polar nucleus after these fuse with the second sperm to form a pentaoploid endosperm.
Pentaploid endosperm in Gagea (Liliaceae) is formed by the fusion of the second sperm with a haploid upper polar nucleus and triploid lower polar nucleus but, in some species, the genomes derived from the lower polar nucleus are heterochromatic (Romanov, 1962; Buzek et al., 1998; Greilhuber et al., 2000). If the heterochromatic genomes are transcriptionally silent, the resulting endosperm is functionally diploid. In some species of Tulipa (Liliaceae), triple fusion of chalazal megaspore nuclei occurs but the second sperm fuses only with the haploid upper polar nucleus (Pechenitsyn, 1972; Mizuochi et al., 2009). The resulting embryo and endosperm are diploid ‘identical twins’ and comprise an ‘embryosperm.’
The fruits of lilies contain long rows of seeds each with a pentaploid endosperm associated with a diploid embryo. A curious convergence has occurred in some insects that line up in similar rows along branches of host plants. Mealy-bugs and diaspid scale-insects contain a nutritive tissue housing endosymbiotic bacteria. This tissue, the bacteriome, is derived from fusion of both polar bodies of the egg with an early cleavage nucleus of the embryo and is thus pentaploid, or higher-ploid if the polar bodies fuse with multiple cleavage nuclei (Schrader, 1923; Brown and Bennett, 1957; Brown, 1965; Nur, 1977). Pentaploid bacteriome nuclei have a similar genetic constitution to endosperm nuclei of lily seeds (a tetraploid maternal genome reconstituted from all products of a meiotic tetrad combined with a haploid sperm-derived genome). Normark (2004) has suggested that the high genetic relatedness of their bacteriomes may mitigate intrafamilial conflicts among siblings. In some scale insects, the three maternally derived genomes derived from the polar-bodies are heterochromatic so that the euchromatic genomes of the bacteriome match the genotype of the diploid embryo (Brown and Nur, 1964; Brown, 1965). In others, the pentaploid bacteriome is replaced during development by a diploid tissue (Brown, 1965). Parallel phenomena of ‘diploidization’ occur in the endosperms of some lilies and their relatives (see above).
Why Is Endosperm (Mostly) Triploid?
The littoral landscape has been transformed since the first wave of papers that applied kin-selection to seed development (Haig, 2013; Pires, 2014). Medea was the first gene to be identified with imprinted expression in Arabidopsis endosperm (Grossniklaus et al., 1998; Kinoshita et al., 1999). Since then many imprinted genes have been discovered in Arabidopsis thaliana (and its relatives) and in agricultural cereals. The study of ‘maternal excess’ and ‘paternal excess’ endosperms (Haig and Westoby, 1991) has been a focus of research with the ratio of maternal to paternal genomes in endosperms now widely accepted as making a difference during development. The mechanisms of these effects remain areas of active research and debate (Hornslien et al., 2019; Satyaki and Gehring, 2019).
Another major development was the description of four-celled (monosporic bimitotic) gametophytes in lineages of early-diverging angiosperms (Williams and Friedman, 2002). The ubiquitous seven-celled female gametophyte, with triploid endosperm, may have evolved from a four-celled ancestor, with diploid endosperm. Diploid endosperms have a 1m:1p ratio of maternal to paternal genomes with the antagonistic effects of maternally expressed genes (MEGs) and paternally expressed genes (PEGs) expected to have reached some kind of evolutionary equilibrium (Wilkins and Haig, 2001; Povilus et al., 2018). Friedman and Williams (2003) present a model in which seven-celled gametophytes arose by movement of one nucleus to the chalazal pole at the two-nucleate stage of monosporic development followed by two mitoses with duplication of the micropylar module of an egg apparatus and polar nucleus at the chalazal pole of the gametophyte. Below I speculate about (i) the immediate effects of a change from diploid to triploid endosperm and (ii) delayed evolutionary responses to these immediate effects.
Triploid endosperm (2m:1p) doubled the maternal dosage of ancestral diploid endosperm (1m:1p). Therefore, the immediate effects of such a change may have resembled the known effects of ‘maternal excess’ caused by the doubling of maternal dosage in crosses of tetraploid mothers to diploid fathers (Haig and Westoby, 1991). Viable ‘maternal excess’ endosperms are associated with smaller seeds and reduced demands on maternal resources. Such an innovation would have been more likely to become established if it were caused by a mutation with sporophytic effects rather than gametophytic effects. This is because a sporophytic effect would reduce levels of demand in all of a mother’s brood whereas a gametophytic effect (in the broods of heterozygous mothers) would reduce the demand of the half of the brood with the mutation for the benefit of the half of the brood without the mutation. An immediate effect of the origin of 2m:1p endosperm would have been to shift the relative expression of imprinted MEGs and PEGs, away from a former balance, toward the maternal sporophytic optimum. The delayed evolutionary response to this perturbation would have been the downregulation of MEGs and the upregulation of PEGs until a new balance was attained in 2m:1p endosperms.
A reversion from a triploid endosperm (2m:1p) to diploid endosperm (1m:1p) appears to be more difficult evolutionarily than the initial change in the other direction. My reasoning here is that diploid revertants (1m:1p) are predicted to resemble the ‘paternal excess’ endosperms (2m:2p) produced by crosses of diploid mothers to tetraploid fathers. In reciprocal crosses between diploids and tetraploids of species with 2m:1p endosperms, ‘paternal excess’ endosperms (2m:2p) are less viable than ‘maternal excess’ endosperms (4m:1p) (Haig and Westoby, 1991). If this analogy is valid, then there should be greater ease in changing from a diploid to a triploid endosperm than the reverse change from triploid to diploid. The reason for this asymmetry are not fully understood. The variable fates of antipodal cells among monosporic trimitotic gametophytes compared to the strict conservation of two polar nuclei in the central cell suggests that the 2m:1p ratio in endosperm has been the key factor accounting for the evolutionary conservation of this form of development (Haig, 1990, p. 268).
Another reason for the conservation of triploid 2m:1p endosperm is that maternally derived genomes in endosperm favor a more efficient distribution of resources among siblings than paternally derived genomes because competing siblings have higher matrilineal than patrilineal relatedness (Haig, 1992b; Haig and Wilkins, 2000). Thus, species with maternally weighted endosperms may suffer less from the costs of sibling conflict than species with equally weighted endosperms. This is closely related to the advantages of greater maternal control over nutrient allocation invoked by Westoby and Rice (1982). I have phrased this as an advantage to the species because I am assuming a context of interspecific competition for ecological niches among lineages with different endosperm ploidies.
Monosporic trimitotic development has reverted once to monosporic bimitotic development in the Onagraceae (Friedman et al., 2008). The Onagraceae exhibit a number of other unusual embryological features including the development of gametophytes from M1 in most species (Tobe and Raven, 1996), regular competition between monosporic gametophytes derived from M1 and M4 in the ovules of other species (Sniezko and Harte, 1984), the formation of ‘ring complexes’ one of which undergoes ‘gametic drive’ in male reproduction and the other ‘gametic drive’ in female reproduction (Cleland, 1962; Harte, 1994), and the restriction of crossing-over to chromosomal ends (Golczyk et al., 2014). Whether these peculiarities can all be fitted into a single coherent evolutionary picture is a task for future work.
Diploid endosperm has evolved from higher-ploid endosperm in multiple species with bisporic or tetrasporic development, some of them reviewed in this paper. Such changes appear to have occurred multiple times in species with non-monosporic development whereas there has been only a single origin of diploid endosperm from triploid endosperm in monosporic development which occurs in many more species. Haig (1986, 1990) argued that non-monosporic development is inherently evolutionarily unstable because of conflicts between descendants of different megaspore nuclei.
The derivation of bisporic bimitotic development from monosporic trimitotic development does not change endosperm ploidy. Therefore, bisporic triploid endosperm (2m:1p) would initially have expressed the pre-existing balance between the effects of MEGs and PEGs. Because the lower polar nucleus has lower relatedness to the associated embryo, its genome would be expected to evolve to reduce demands on mothers when expressed in endosperm (for example, by upregulating MEGs). The evolution of a diploid endosperm in Allium atroviolaceum and Allium rotundum (see above) may have been a response of the genomes of the upper polar nucleus and sperm to eliminate the third genome derived from a somatic megaspore because this genome strongly inhibited endosperm development and reduced embryo fitness. In species with tetrasporic pentaploid endosperms (4m:1p) the initial effect of the addition of two maternally derived genomes to a 2m:1p endosperm would be a ‘maternal excess’ endosperm with reduced effective demand. The evolutionary response of PEGs would be to increase their expression. The evolutionary response of MEGs would be to increase their expression from the haploid genome derived from the germinal megaspore but not from the three haploid genomes derived from somatic megaspores. Therefore, embryos in seeds that were able to exclude these genomes to produce a diploid endosperm would be selectively favored. The presence of alleles in endosperm that are absent in the associated embryo is inherently evolutionarily unstable, resulting in multiple origins of diploid ‘embryosperms’ from bisporic and tetrasporic development.
Another question raised by the ubiquity of monosporic triploid endosperms is: why are there no monosporic higher-ploid endosperms? My current conjecture is that 2m:1p is an evolutionary ‘sweet spot’ that reduces sibling competition for maternal resources within the constraints of conserved features of angiosperm developmental and molecular biology.
Discussion
It is thirty years since I first attempted to bring order to the diversity of embryo sac development in Haig (1990). My principal aims in that paper were two-fold. First, I desired to move the field away from a classification based on morphological ideal ‘types’ to a focus on developmental ‘algorithms’ and how one form can arise from another by minimal genetic changes (evo-devo). Second, I desired plant embryologists to recognize that bisporic and tetrasporic embryo sacs were not integrated individuals with coordinated adaptations. Rather, they were chimeric assemblages of different genotypes with divergent evolutionary ‘interests.’
With regard to the first aim, I initially intended to describe developmental processes without offering a classification of the resulting forms but I was persuaded by reviewers that I needed to offer something in the place of existing classifications. Thankfully nobody has adopted the classification of Haig (1990). Two aspects of my classification have been retained in this paper. The first is the traditional distinction between monosporic, bisporic, and tetrasporic development. This distinction is important because it determines how many genetic individuals are present in the embryo sac. The second is the number of mitotic divisions of the germinal megaspore between the completion of meiosis and the differentiation of an egg. This is important because it determines the number of nuclei of the germinal genotype within the embryo sac. Haig (1990) used ‘one-phasic,’ ‘two-phasic,’ and ‘three-phasic— a translation of Fagerlind’s (1944) 1-phasische, 2-phasische, 3-phasische — to indicate the number of mitotic divisions. In this paper, I adopt unimitotic, bimitotic, and trimitotic as more intuitive substitutes. These adjectives (tetrasporic, bimitotic, etc.) appertain to developmental processes and are not intended to be defining characters of morphological ‘types.’
In 1990, very little was known about the molecular genetics of female gametophyte development. We now know much more about the molecular mechanisms of monosporic trimitotic development, especially in the model organism Arabidopsis and in agricultural crops, especially rice and maize. Another area of active research has been into the molecular genetics of apomictic development because of the (unrealized) promise of apomixis as a way of fixing elite agricultural genotypes. Unfortunately we are almost as ignorant of the molecular genetics of non-monosporic sexual development today as we were thirty years ago. Perhaps someone will be funded to dissect the molecular embryology of onions or chives (species with bisporic embryo sacs).
With regard to my second aim, Haig (1990) was a total failure. This paper is another attempt to show how an appreciation of conflict among the nuclei of non-monosporic embryo sacs can make sense of what is observed under the microscope. Many phenomena that have hitherto been no more than miscellaneous observations, recorded as deviations from ‘type,’ make sense from this perspective. SC Maheshwari (1955), for example, noted a frequent tendency for a reduction in the number of nuclei at the chalazal end of bisporic embryo sacs, and Rutishauser (1969, p. 32) noted an association between antipodal eggs and embryo sacs with bisporic or tetrasporic development. These empirical correlations remained unexplained, but the kin-conflict interpretation offers causal explanations for these patterns, albeit explanations in terms of the ‘final’ causation of adaptive function (or loss of function) rather than the ‘efficient’ causation of molecular mechanism (Haig, 2020).
A third, more quixotic, aim of the current paper is to interest non-botanical readers in the fascinations of endosperm. The details of double fertilization remain botanical arcana for most biologists, considered of no interest to anyone but a narrow specialist. But this is chauvinistic. A large proportion of the calories consumed by the human population come from endosperm, either directly by the consumption of grains or indirectly by the consumption of meat from grain-fed animals. Nawaschin’s (1898) discovery of double fertilization contributed to the ‘rediscovery’ of Mendel’s experiments by Correns and de Vries who were studying paternal effects in seeds (Dunn, 1973). Arguments about how to calculate parent-specific relatedness in endosperm parallel philosophers’ debates about ‘self-locating beliefs’ (Haig, 2016). Endosperm pops up in odd places, not only as popcorn. It provides food for thought.
Author Contributions
The author confirms being the sole contributor of this work and has approved it for publication.
Conflict of Interest
The author declares that the research was conducted in the absence of any commercial or financial relationships that could be construed as a potential conflict of interest.
References
Arias, T., and Williams, J. H. (2008). Embryology of Manekia naranjoana (Piperaceae) and the origin of the tetrasporic, 16-nucleate female gametophytes in Piperales. Am. J. Bot. 95, 272–285. doi: 10.3732/ajb.95.3.272
Ashurmetov, O. A., Yangalycheva, S. S., and Fritsch, R. M. (2001). Morphological and embryological characters of three middle Asian Allium L. species (Alliaceae). Bot. J. Linn. Soc. 137, 51–64. doi: 10.1111/j.1095-8339.2001.tb01104.x
Bambacioni, V. (1928). Ricerche sulla ecologia e sulla embriologia di Fritillaria persica. Ann. Bot. 18, 7–37. doi: 10.2307/j.ctvb1hsgb.2
Battaglia, E. (1947). Ricerche cariologiche e embriologiche sul genere Rudbeckia (Asteraceae). XII. Il gametofito femminile e maschile di Rudbeckia flava Greene, con particolare riguardo al suo comportamento di ibrido strutturale. Nuovo G. Bot. Ital. 54, 560–567. doi: 10.1080/11263504709440455
Battaglia, E., and Feeley, E. (1959). The embryo sac of Scilla pratensis Waldst. et Kit. (Liliaceae). Caryologia 11, 407–414. doi: 10.1080/00087114.1959.10797061
Berridge, E. M., and Sanday, E. (1907). Oogenesis and embryogeny in Ephedra distachya. New Phytol. 6, 167–174. doi: 10.1111/j.1469-8137.1907.tb06054.x
Boyes, J. W. (1939). Development of the embryo sac of Plumbagella micrantha. Am. J. Bot. 26, 539–547. doi: 10.1002/j.1537-2197.1939.tb09316.x
Boyes, J. W., and Battaglia, E. (1951a). Embryo-sac development in the Plumbaginaceae. Caryologia 3, 305–310. doi: 10.1080/00087114.1951.10797167
Boyes, J. W., and Battaglia, E. (1951b). The tetrasporic embryo sacs of Plumbago coccinea, P. scandens, and Ceratostigma willmottianum. Bot. Gaz. 112, 485–489. doi: 10.1086/335679
Brown, S. W. (1965). Chromosomal survey of the armored and palm scale insects (Coccoïdea: Diaspididae and Phoenicoccidae). Hilgardia 36, 189–294.
Brown, S. W., and Bennett, F. D. (1957). On sex determination in the diaspine scale Pseudaulacaspis pentagona (Targ.) (Coccoidea). Genetics 42, 510–523.
Brown, S. W., and Nur, U. (1964). Heterochromatic chromosomes in the coccoids. Science 145, 130–136. doi: 10.1126/science.145.3628.130
Buzek, J., Ebert, I., Ruffini-Catiglione, M., Siroky, J., Vyskot, B., and Greilhuber, J. (1998). Structure and DNA methylation pattern of partially heterochromatinised endosperm nuclei in Gagea lutea (Liliaceae). Planta 204, 506–514. doi: 10.1007/s004250050285
Carmichael, J. S., and Friedman, W. E. (1996). Double fertilization in Gnetum gnemon (Gnetaceae): its bearing on the evolution of sexual reproduction within the Gnetales and the anthophyte clade. Am. J. Bot. 83, 767–780. doi: 10.1002/j.1537-2197.1996.tb12766.x
Cass, D. D. (1972). Occurrence and development of a filiform apparatus in the egg of Plumbago capensis. Am. J. Bot. 59, 279–283. doi: 10.1002/j.1537-2197.1972.tb10093.x
Cass, D. D., and Karas, I. (1974). Ultrastructural organization of the egg of Plumbago zeylanica. Protoplasma 81, 49–62. doi: 10.1007/bf02055773
Ceccherini, L., and Raddi, S. (2009). Anatomical and genetic features of the Cupressus megagametophyte: the diploid pattern in C. sempervirens is an exception for this genus. Plant Biosyst. 143(Suppl.), S1–S5. doi: 10.1080/11263500903187076
Charnov, E. L. (1979). Simultaneous hermaphroditism and sexual selection. Proc. Natl. Acad. Sci. U.S.A. 76, 2480–2484. doi: 10.1073/pnas.76.5.2480
Cleland, R. E. (1962). The cytogenetics of Oenothera. Adv. Genet. 11, 147–237. doi: 10.1016/s0065-2660(08)60287-4
Czapik, R. (1976). Variability in the embryological cycle of Adoxa moschatellina L. Acta Biol. Cracoviensis Ser. Bot. 19, 1–13.
Dahlgren, K. V. O. (1916). Zytologische und embryologische Studien über die Reihen Primulales und Plumbaginales. K. Sven. Vetenskapsakademiens Handl. 56, 1–80.
Dahlgren, K. V. O. (1937). Die entwicklung des embryosackes bei Plumbago zeylanica. Bot. Notiser 1937, 487–498.
D’Amato, F. (1940). Contributo all’embriologia Delle Plumbaginaceae. Nuovo G. Bot. Ital. 47, 349–382. doi: 10.1080/11263504009439779
Dawe, R. K., Lowry, E. G., Gent, J. I., Stitzer, M. C., Swentowsky, K. W., Higgins, D. M., et al. (2018). A kinesin-14 motor activates neocentromeres to promote meiotic drive in maize. Cell 173, 839–850. doi: 10.1016/j.cell.2018.03.009
Ebert, I., and Greilhuber, J. (2005). Developmental switch during embryo sac formation from a bisporic mode to the tetrasporic Fritillaria type in Hyacinthoides vincentina (Hoffmannsegg & Link) Rothm. (Hyacinthaceae). Acta Biol. Cracov. Ser. Bot. 47, 179–184.
Ekdahl, I. (1941). Die entwicklung von embryosack und embryo bei Ulmus glabra Huds. Sven. Bot. Tidskr. 35, 143–156.
El Maâtaoui, M., and Pichot, C. (1999). Nuclear and cell fusion cause polyploidy in the megagametophyte of common cypress Cupressus sempervirens L. Planta 208, 345–351. doi: 10.1007/s004250050568
El Maâtaoui, M., Pichot, C., Alzubi, H., and Grimaud, N. (1998). Cytological basis for a tetraspory in Cupressus sempervirens L. megagametogenesis and its implications in genetic studies. Theor. Appl. Genet. 96, 776–779. doi: 10.1007/s001220050801
Fagerlind, F. (1938). Wo kommen tetrasporische durch drei Teilungsschritte vollentwickelte Embryosäcke unter den Angiospermen vor? Bot. Notiser 1938, 461–498.
Fagerlind, F. (1939b). Kritische und revidierende untersuchungen über das Vorkommen des Adoxa (“Lilium”) Typus. Acta Horti Bergiani 13, 1–49.
Fagerlind, F. (1944). Der tetrasporische angiospermen-embryosack und dessen bedeutung für das verständnis der entwicklungsmechanik und phylogenie des embryosacks. Arkiv Bot. 31A, 1–71. doi: 10.1159/000066897
Fischer, A. (1880). Zur kenntnis der embryosackentwicklung einiger angiospermen. Jena. Z. Naturwiss. 14, 90–132.
Fishman, L., and Kelly, J. K. (2015). Centromere-associated meiotic drive and female fitness variation in Mimulus. Evolution 69, 1208–1218. doi: 10.1111/evo.12661
Friedman, W. E. (2015a). Development and evolution of the female gametophyte and fertilization process in Welwitschia mirabilis (Welwitschiaceae). Am. J. Bot. 102, 312–324. doi: 10.3732/ajb.1400472
Friedman, W. E. (2015b). Evolving words and the egg-bearing tubes of Welwitschia (Welwitschiaceae). Am. J. Bot. 102, 176–179. doi: 10.3732/ajb.1400537
Friedman, W. E., and Carmichael, J. S. (1998). Heterochrony and developmental innovation: evolution of female gametophyte ontogeny in Gnetum, a highly apomorphic seed plant. Evolution 52, 1016–1030. doi: 10.2307/2411233
Friedman, W. E., Madrid, E. N., and Williams, J. H. (2008). Origin of the fittest and survival of the fittest: relating female gametophyte development to endosperm genetics. Int. J. Plant Sci. 169, 79–92. doi: 10.1086/523354
Friedman, W. E., and Ryerson, K. C. (2009). Reconstructing the ancestral female gametophyte of angiosperms: insights from Amborella and other ancient lineages of flowering plants. Am. J. Bot. 96, 129–143. doi: 10.3732/ajb.0800311
Friedman, W. E., and Williams, J. H. (2003). Modularity of the angiosperm female gametophyte and its bearing on the early evolution of endosperm in flowering plants. Evolution 57, 216–230. doi: 10.1111/j.0014-3820.2003.tb00257.x
Golczyk, H., Massouh, A., and Greiner, S. (2014). Translocations of chromosome end-segments and facultative heterochromatin promote meiotic ring formation in evening primroses. Plant Cell 26, 1280–1293. doi: 10.1105/tpc.114.122655
Greilhuber, J. (1973). Über die entwicklung des embryosacks von Melampyrum und Parentucellia latifolia (Scrophulariaceae, Pedicularieae). Österr. Bot. Z. 121, 81–97. doi: 10.1007/bf01373367
Greilhuber, J., Ebert, I., Lorenz, A., and Vyskot, B. (2000). Origin of facultative heterochromatin in the endosperm of Gagea lutea (Liliaceae). Protoplasma 212, 217–226. doi: 10.1007/bf01282922
Grossniklaus, U., Vielle-Calzada, J. P., Hoeppner, M. A., and Gagliano, W. B. (1998). Maternal control of embryogenesis by MEDEA, a Polycomb group gene in Arabidopsis. Science 280, 446–450. doi: 10.1126/science.280.5362.446
Gvaladze, G. (1979). Asynchronous division in embryo and endosperm in Liliaceae. Proc. Indian Natl. Sci. Acad. B 45, 596–604.
Gvaladze, G., Nadirashvilli, N., and Akhalkatsi, M. (2002). Chromatin diminution during endosperm development in Allium atroviolaceum Boiss. (Alliaceae). Bull. Georgian Acad. Sci. 166, 537–540.
Haig, D. (1986). Conflicts among megaspores. J. Theor. Biol. 123, 471–480. doi: 10.1016/s0022-5193(86)80214-4
Haig, D. (1987). Kin conflict in seed plants. Trends Ecol. Evol. 2, 337–340. doi: 10.1016/0169-5347(87)90110-8
Haig, D. (1990). New perspectives on the angiosperm female gametophyte. Bot. Rev. 56, 236–274. doi: 10.1007/bf02858326
Haig, D. (1992a). “Brood reduction in gymnosperms,” in Cannibalism: Ecology and Evolution Among Diverse Taxa, eds M. Elgar and B. Crespi (Oxford: Oxford University Press), 63–84.
Haig, D. (1992b). Genomic imprinting and the theory of parent-offspring conflict. Semin. Dev. Biol. 3, 153–160.
Haig, D. (1996). Gestational drive and the green-bearded placenta. Proc. Natl. Acad. Sci. U.S.A. 93, 6547–6551. doi: 10.1073/pnas.93.13.6547
Haig, D. (2010). Games in tetrads: segregation, recombination, and meiotic drive. Am. Nat. 176, 404–413. doi: 10.1086/656265
Haig, D. (2013). Kin conflict in seed development: an interdependent but fractious collective. Annu. Rev. Cell Dev. Biol. 29, 189–211. doi: 10.1146/annurev-cellbio-101512-122324
Haig, D. (2016). Sleeping Beauty in a grain of rice. Biol. Philos. 31, 23–37. doi: 10.1007/s10539-015-9503-1
Haig, D., and Westoby, M. (1989). Parent-specific gene expression and the triploid endosperm. Am. Nat. 134, 147–155. doi: 10.1086/284971
Haig, D., and Westoby, M. (1991). Genomic imprinting in endosperm: its effects on seed development in crosses between species and between different ploidies of the same species, and its implications for the evolution of apomixis. Philos. Trans. R. Soc. B 33, 2935–2946.
Haig, D., and Westoby, M. (2006). An earlier formulation of the genetic conflict hypothesis of genomic imprinting. Nat. Genet. 38:271. doi: 10.1038/ng0306-271
Haig, D., and Wilkins, J. F. (2000). Genomic imprinting, sibling solidarity, and the logic of collective action. Philos. Trans. R. Soc. B 355, 1593–1597. doi: 10.1098/rstb.2000.0720
Hasitschka-Jenschke, G. (1957). Die entwicklung der samenanlage von Allium ursinum mit besonderer Berücksichtigung der endopolyploiden kerne in synergiden und antipoden. Österr. Bot. Z. 104, 1–24. doi: 10.1007/bf01289119
Hasitschka-Jenschke, G. (1958). Zur Karyologie der samenanlage dreier Allium-Arten. Österr. Bot. Z. 105, 71–82. doi: 10.1007/bf01289002
Haupt, A. W. (1934). Ovule and embryo sac of Plumbago capensis. Bot. Gaz. 95, 649–659. doi: 10.1086/334435
Higgins, D. M., Lowry, E. G., Kanizay, L. B., Becraft, P. W., Hall, D. W., and Dawe, R. K. (2018). Fitness costs and variation in transmission distortion associated with the abnormal chromosome 10 meiotic drive system in maize. Genetics 208, 297–305. doi: 10.1534/genetics.117.300060
Hornslien, K. S., Miller, J. S., and Grini, P. E. (2019). Regulation of parent-of-origin allelic expression in the endosperm. Plant Physiol. 180, 1498–1519. doi: 10.1104/pp.19.00320
Ismailoglu, I., Coskun, Z. M., Keskin, M., and Ünal, M. (2010). Development and cytochemical studies on embryo sac, embryo and endosperm in Allium peroninianum. Pak. J. Bot. 42, 2711–2718.
Johnson, D. S. (1914). Studies on the development of the Piperaceae. II. The structure and seed-development of Peperomia hispidula. Am. J. Bot. 1, 357–397. doi: 10.1002/j.1537-2197.1914.tb09375.x
Khalelle, T. F., and Mitchell, B. B. (1982). Cytoembryology of Allium textile Nels. & Macbr. Am. J. Bot. 69, 950–956. doi: 10.1002/j.1537-2197.1982.tb13338.x
Kinoshita, T., Yadegari, R., Harada, J. J., Goldberg, R. B., and Fischer, R. L. (1999). Imprinting of the MEDEA polycomb gene in the Arabidopsis endosperm. Plant Cell 11, 1945–1952. doi: 10.2307/3871089
Lehmann-Baerts, M. (1967). Ovule, gamétophyte femelle et embryogenèse chez Ephedra distachya L. Cellule 67, 51–87.
Madrid, E. N., and Friedman, W. E. (2009). The developmental basis of an evolutionary diversification of female gametophyte structure in Piper and Piperaceae. Ann. Bot. 103, 869–884. doi: 10.1093/aob/mcp011
Maheshwari, P. (1935). Contributions to the morphology of Ephedra foliata. I. The development of male and female gametophytes. Proc. Indian Acad. Sci. B 1, 586–606.
Maheshwari, P. (1937). A critical review of the types of embryo sacs in angiosperms. New Phytol. 36, 359–417. doi: 10.1111/j.1469-8137.1937.tb06921.x
Maheshwari, P. (1946b). The Fritillaria type of embryo sac: a critical review. J. Indian Bot. Soc. 101–119.
Maheshwari, P., and Johri, B. M. (1941). The embryo-sac of Acalypha indica L. Beih. Bot. Centralblatt 61A, 125–136.
Maheshwari, S. C. (1955). The occurrence of bisporic embryo sacs in angiosperms — a critical review. Phytomorphology 5, 67–99.
Martens, P. (1963). Recherches sur Welwitschia mirabilis — III. L’ovule et le sac embryonnaire. Les sacs embryonnaire extra-floraux. Cellule 63, 309–329.
Mather, K. (1935). Reductional and equational separation of the chromosomes in bivalents and multivalents. J. Genet. 30, 53–78. doi: 10.1007/bf02982205
Mathur, K., and Khan, R. (1941). The development of the embryo sac in Vogelia indica, Lamk. Proc. Indian Acad. Sci. B 13, 360–368.
Mehra, P. N., and Malhotra, R. K. (1947). Stages in the embryology of Cupressus sempervirens Linn. with particular reference to the occurrence of multiple male cells in the male gametophyte. Proc. Natl. Acad. Sci. India B 17, 129–153.
Mizuochi, H., Matsuzaki, H., Moue, T., and Okazaki, K. (2009). Diploid endosperm formation in Tulipa spp. and identification of a 1:1 maternal-to-paternal genome ratio in endosperms of T. gesneriana L. Sex. Plant Reprod. 22, 27–36. doi: 10.1007/s00497-008-0088-6
Mogensen, H. L. (1996). The hows and whys of cytoplasmic inheritance in seed plants. Am. J. Bot. 83, 383–404. doi: 10.1002/j.1537-2197.1996.tb12718.x
Mukherjee, P. K. (1958). The female gametophyte of Acalypha malabarica Muell. With a brief discussion on the Penæa type of embryo-sac. J. Indian Bot. Soc. 37, 504–508.
Murphy, J. B. (1946). Megasporogenesis and development of the embryo sac of Allium cernuum. Bot. Gaz. 108, 129–136. doi: 10.1086/335400
Nadirashvili, N., Gvaladze, G. E., and Akhalkatsi, M. S. (2006). Structure and function of the hypertrophic synergid in some species of Allium L. Proc. Georgian Acad. Sci. Biol. Ser. B 4, 53–60.
Nagl, W. (1976). The polytechnic antipodal cells in Scilla bifolia: DNA replication pattern and possibility of nucleolar DNA amplification. Cytobiologie 14, 165–170.
Nawaschin, S. (1898). Resultate einer revision der befruchtungsvorgänge bei Lilium martagon und Fritillaria tenella. Bull. Acad. Impériale Sci. 9, 377–382.
Normark, B. B. (2004). The strange case of the armored scale insect and its bacteriome. PLoS Biol. 2:e43. doi: 10.1371/journal.pbio.0020043
Nur, U. (1977). Maternal inheritance of enzymes in the mealybug Pseudococcus obscurus (Homoptera). Genetics 86, 149–160.
Pahuja, A. N., and Kumar, V. (1970). Embryo sac development, cytology, and systematic position of Clintonia. Phytomorphology 20, 97–102.
Pechenitsyn, V. P. (1972). [The double fertilization in species of Tulipa with Fritillaria-type embryo sac]. Bot. Z. 57, 465–469.
Pichot, C., and El Maâtaoui, M. (1997). Flow cytometric evidence for multiple ploidy levels in the endosperm of some gymnosperm species. Theor. Appl. Genet. 94, 865–870. doi: 10.1007/s001220050488
Pires, N. D. (2014). Seed evolution: parental conflicts in a multi-generational household. Biomol. Concepts 5, 71–86. doi: 10.1515/bmc-2013-0034
Porter, T. R. (1936). Development of the megagametophyte and embryo of Allium mutabile. Bot. Gaz. 98, 317–327. doi: 10.1086/334640
Povilus, R. A., Diggle, P. K., and Friedman, W. E. (2018). Evidence for parent-of-origin effects and interparental conflict in seeds of an ancient flowering plant lineage. Proc. R. Soc. B 285:20172491. doi: 10.1098/rspb.2017.2491
Queller, D. C. (1983). Kin selection and conflict in seed maturation. J. Theor. Biol. 100, 153–172. doi: 10.1016/0022-5193(83)90099-1
Rhoades, M. M. (1952). “Preferential segregation in maize,” in Heterosis, ed. J. W. Gowen (Ames, IA: Iowa State College Press), 66–80.
Roís, A. S., Sádio, F., Teixeira, G., Paes, A. P., Espírito-Santo, D., Sharbel, T. F., et al. (2016). Phylogeography and modes of reproduction in diploid and tetraploid halophytes of Limonium species (Plumbaginaceae): evidence for a pattern of geographical parthenogenesis. Ann. Bot. 117, 37–50. doi: 10.1093/aob/mcv138
Romanov, I. D. (1962). The origin of the unique structure of endosperm nuclei in Gagea. Dokl. Bot. Sci. Sec. 141, 188–190.
Russell, S. D., and Cass, D. D. (1988). Fertilization in Plumbagella micrantha. Am. J. Bot. 75, 778–781. doi: 10.1002/j.1537-2197.1988.tb13500.x
Rutishauser, A. (1969). Embryologie und Fortpflanzungsbiologie der Angiospermen. Vienna: Springer-Verlag.
Sateishi, S. (1927). [On the development of the embryo sac and fertilization of Acalypha australis L. (Preliminary note)]. Bot. Mag. Tokyo 41, 477–485. doi: 10.15281/jplantres1887.41.477
Satyaki, P. R. V., and Gehring, M. (2019). Paternally acting canonical RNA-directed DNA methylation pathway genes sensitize Arabidopsis endosperm to paternal genome dosage. Plant Cell 31, 1563–1578. doi: 10.1105/tpc.19.00047
Schrader, F. (1923). The origin of the mycetocytes in Pseudococcus. Biol. Bull. 45, 279–296. doi: 10.2307/1536727
Shattuck, C. H. (1905). A morphological study of Ulmus americana. Bot. Gaz. 40, 209–223. doi: 10.1086/328668
Skinner, D. J., and Sundaresan, V. (2018). Recent advances in understanding female gametophyte development. F1000Res. 7:F1000 Faculty Rev-804.
Smith, R. W. (1911). The tetranucleate embryo sac of Clintonia. Bot. Gaz. 52, 209–217. doi: 10.1086/330611
Sniezko, R., and Harte, C. (1984). Polarity and competition between megaspores in the ovule of Oenothera hybrids. Plant Syst. Evol. 144, 83–97. doi: 10.1007/bf00986667
Specht, C. E., Meister, A., Keller, E. R. J., Korzun, L., and Börner, A. (2001). Polyembryony in species of the genus Allium. Euphytica 121, 37–44. doi: 10.1023/A:1012013121656
Stephens, E. L. (1909). The embryo-sac and embryo of certain Penaeaceae. Ann. Bot. 23, 363–378. doi: 10.1093/oxfordjournals.aob.a089226
Stevenson, G. B. (1934). The life history of the New Zealand species of the parasitic genus Korthalsella. Trans. R. Soc. N. Z. 64, 175–190.
Sugihara, Y. (1956). The embryogeny of Cupressus sempervirens Linnaeus. Science Reports of the Tôhoku University. Fourth Ser. 22, 1–4. doi: 10.2147/rrmc.s29902
Svoma, E. (1981). Zur systematischen embryologie der Gattung Scilla L. (Liliaceae). Stapfia 9, 1–124.
Svoma, E., and Greilhuber, J. (1988). Studies on systematic embryology in Scilla (Hyacinthaceae). Plant Syst. Evol. 161, 169–181. doi: 10.1007/bf00937297
Svoma, E., and Greilhuber, J. (1989). Systematic embryology of the Scilla siberica alliance (Hyacinthaceae). Nord. J. Bot. 8, 585–600. doi: 10.1111/j.1756-1051.1989.tb01733.x
Syamasundar, J., and Panchaksharappa, M. G. (1975). A cytochemical study of the hypertrophied synergid in Allium cepa L. Cytologia 40, 371–376. doi: 10.1508/cytologia.40.371
Tekleyohans, D. G., Nakel, T., and Groß-Hardt, R. (2017). Patterning the female gametophyte of flowering plants. Plant Physiol. 173, 122–129. doi: 10.1104/pp.16.01472
Tobe, H., and Raven, P. H. (1996). Embryology of Onagraceae (Myrtales): characteristics, variation and relationships. Telopea 6, 667–688. doi: 10.7751/telopea19963030
Tretjakow, S. (1895). Die betheiligung der antipoden in fällen der polyembryonie bei Allium odorum L. Ber. Dtsch. Bot. Ges. 13, 13–17.
Vinogradova, G. Y. (2018). [Endosperm development in Allium schoenoprasum and A. ramosum (Alliaceae)]. Bot. Z. 103, 1451–1471. doi: 10.1134/s0006813618110066
Walker, R. I. (1950). Megasporogenesis and development of megagametophyte in Ulmus. Am. J. Bot. 37, 47–52. doi: 10.1002/j.1537-2197.1950.tb08160.x
Weber, E. (1929). Entwicklungsgeschichtliche untersuchungen über die Gattung Allium. Bot. Arch. 25, 1–44.
Westoby, M., and Rice, B. (1982). Evolution of the seed plants and inclusive fitness of plant tissues. Evolution 36, 713–724. doi: 10.2307/2407884
Wilkins, J. F., and Haig, D. (2001). Genomic imprinting of two antagonistic loci. Proc. R. Soc. B 268, 1861–1867. doi: 10.1098/rspb.2001.1651
Williams, J. H., and Friedman, W. E. (2002). Identification of diploid endosperm in an early angiosperm lineage. Nature 415, 522–526. doi: 10.1038/415522a
Willson, M. F., and Burley, N. (1983). Mate Choice in Plants. Princeton, NJ: Princeton University Press.
Ya-e, M. (1941). Development of the embryo-sac in Statice japonica Siebold et Zuccarini. Sci. Rep. Tôhoku Univ. Ser. IV 16, 297–303.
Keywords: endosperm, double fertilization, genomic imprinting, meiotic drive, antipodal cells, female gametophyte, kin selection
Citation: Haig D (2020) Poles Apart: Monosporic, Bisporic, and Tetrasporic Embryo Sacs Revisited. Front. Ecol. Evol. 8:516640. doi: 10.3389/fevo.2020.516640
Received: 19 December 2019; Accepted: 17 August 2020;
Published: 15 September 2020.
Edited by:
John Richard Pannell, University of Lausanne, SwitzerlandReviewed by:
Jeremy Van Cleve, University of Kentucky, United StatesPamela Diggle, University of Connecticut, United States
Copyright © 2020 Haig. This is an open-access article distributed under the terms of the Creative Commons Attribution License (CC BY). The use, distribution or reproduction in other forums is permitted, provided the original author(s) and the copyright owner(s) are credited and that the original publication in this journal is cited, in accordance with accepted academic practice. No use, distribution or reproduction is permitted which does not comply with these terms.
*Correspondence: David Haig, ZGhhaWdAb2ViLmhhcnZhcmQuZWR1