- Department of Geography, Texas A&M University, College Station, TX, United States
Fens and bogs are distinct in terms of their biogeochemistry, water table behavior, and net peat-accumulation regimes. While most peatlands start developing as fens, a large fraction of them eventually shift to bogs in a step-like ecosystem shift. This transition has traditionally been assumed to be primarily controlled by the ecosystem itself (autogenic control). Here we use 90 peat profiles from southernmost South America (SSA) as a case study that illustrates a synchronous, regional-scale shift from fen to bog around 4200 years ago. In light of these results, we propose and discuss conceptual models that link environmental change (allogenic control) as a trigger to the fen-bog transition (FBT). In addition, our stratigraphic analyses show that Sphagnum deposits are associated with greater peat masses, larger soil-carbon stocks, and higher rates of peat-carbon accumulation than their non-Sphagnum counterparts, with Sphagnum bogs being characterized by soil-carbon densities over twice that of non-Sphagnum peatlands (medians = 141 vs. 56 kgC/m2). Since fens and bogs also behave differently in terms of their carbon exchanges with the atmosphere, a better appraisal of the processes involved in the FBT could help elucidate the role of this critical ecosystem shift in the past and future global carbon cycle.
Introduction
Peatlands cover about 3% of the global land area and account for roughly half of the wetlands worldwide (Rydin and Jeglum, 2013; Mitsch and Gosselink, 2015). Peatland soils are water-saturated; as a result, plant decomposition occurs slowly, which causes the net accumulation of partly decomposed plant matter over centennial to millennial timescales. Peatlands are predominantly found across the mid- and high-latitude regions (∼45–70°), though they can be regionally abundant in the subtropical belt (∼15–30°) (Xu et al., 2018).
Peatlands are classified into two main types, fens and bogs, on the basis of their hydrological and bioclimatic conditions, chemistry and nutrient status, and floristic composition (Charman, 2002; Vitt, 2006). While fens depend on mineral-rich water inputs from groundwater and overland flow, bogs are ombrotrophic, i.e., their water supply comes exclusively from rain and snow (Damman, 1986; Vitt et al., 2003; Mitsch and Gosselink, 2015). Bogs tend to be acidic, while fens are typically characterized by neutral or alkaline pH conditions (Rydin and Jeglum, 2013). This trophic gradient, from rich fens to poor bogs, influences plant community dynamics, including biomass growth and floristic composition (e.g., Malmer, 1986; Glaser, 1992). Plant communities in fens tend to be dominated by sedges, grasses, rushes, and brown moss species. Bogs often harbor continuous Sphagnum moss carpets with some woody shrubs and sedge clusters. In continental regions, drier bogs can also be colonized by small trees. In general, fens are characterized by higher net primary productivity, greater plant diversity, and more rapid peat decomposition rates than bogs (Zoltai and Vitt, 1995; Granath et al., 2010).
Fens and bogs also differ in terms of their ecosystem functions. It has been repeatedly shown (e.g., Blodau, 2002; Turetsky et al., 2014; Abdalla et al., 2016) that fens emit substantially more methane (CH4) than bogs [100:1 according to Laine and Vasander (1996)]. Fens are characterized by high water table levels, which limit CH4 oxidation within the unsaturated (“acrotelm”) upper peat layer (Moore and Roulet, 1993; Whiting and Chanton, 2001), thus facilitating CH4 emission to the atmosphere. Fen vegetation cover, often dominated by sedges, acts as CH4 conduits from deep peats to the atmosphere, further contributing to elevated CH4 emissions from these peatlands (Bubier, 1995; Segers, 1998). Bogs, on the other hand, are typically characterized by lower water table levels and significantly less sedges than fens (Turetsky et al., 2014). Overall, it can be argued that fens and bogs are also distinct in terms of their biogeochemistry and water table (and moisture) behavior.
The estimated extent of fens and bogs at the global scale remains unclear. Best estimates put the global fen and bog areas around 1.1 and 2.1 Mkm2, respectively (Loisel et al., 2017a). This knowledge is based on limited country inventories combined with broad geographic distribution patterns. For example, across the temperate, boreal, and arctic biomes, peatland distribution tends to follow climatic gradients of precipitation and temperature, from oceanic to continental, wet to dry, and mild to cold (Vitt, 2006; Parviainen and Luoto, 2007). For example, in Canada, most bogs are found in the boreal region (though there are permafrost bogs in the Arctic), while fens are typically clustered along the southern edge of the peatland distribution, in mountainous areas, and across the (sub-)Arctic lowlands (Tarnocai et al., 2002).
The classic peatland hydroseral succession that leads to the formation of an ombrotrophic peatland (bog) consists of a transition from an aquatic environment (pond, marsh) to a minerotrophic peatland (fen) and, subsequently, to a peat bog (Zobel, 1988). This general developmental scheme from fen to bog has been attributed to one key autogenic process, namely long-term peat accumulation that eventually reaches an elevation beyond the influence of the mineral groundwater (Kuhry et al., 1993). This autogenic succession paradigm is engrained in our understanding of the fen-bog transition (FBT); as such, process-based peatland models such as the Holocene Peat Model assume that the FBT occurs when 1–2 m of peat has accumulated (Frolking et al., 2001, 2010). In nature, an intermediate stage between the fen and bog states does not really exist (e.g., Walker, 1970; Kuhry et al., 1993; Bunting and Warner, 1998), suggesting that the transition from fen to bog might occur relatively quickly, likely over few decades to centuries; this approximation is based on the thinness (typically only a few cm) of fen-bog transitional layers (e.g., Hughes and Barber, 2003; Ronkainen et al., 2014). Also noteworthy is that alternative developmental pathways have been observed. For example, in permafrost regions, an ombrotrophic peat plateau can “revert” or “cycle” back to a fen following thermokarst collapse (Treat et al., 2015); such cyclical succession between fen and bog has been observed across the discontinuous permafrost zone (e.g., Zoltai, 1993; Camill and Clark, 2000). That said, for the purpose of this paper, the focus remains on the classic succession from fen to bog.
In contrast to the autogenic succession theory, a few studies have proposed that hydroclimatic conditions (allogenic factors) may exert a major influence on the passage from a wetter and richer fen to a poorer and drier bog (e.g., Hughes, 2000; Hughes and Dumayne-Peaty, 2002; Hughes and Barber, 2003). In these cases, the ombrotrophication process is triggered by small changes in allogenic controls such as increased effective moisture (the “wet route”) or prolonged droughts (the “dry route”). An allogenic change can thus “speed up” autogenic succession (e.g., Väliranta et al., 2017); succession could also make the system more susceptible to allogenic factors. For example, in oceanic settings, it has been suggested that an increase in effective precipitation (precipitation minus evaporation) could flush mineral-rich water and enhance Sphagnum growth, which would lead to the formation of a perched water table and the initiation of ombrotrophy (Hughes and Barber, 2003). Under this “wet route” scenario, Sphagnum species isolate themselves from the groundwater by growing “upward.” While the ombrotrophication process is ultimately driven by Sphagnum growth (autogenic control), it is triggered by increased moisture levels (allogenic control). Another example was observed in continental settings, where droughts could trigger ombrotrophication processes by enhancing peat decomposition, which leads to the creation of dense peat layers and to a relatively impermeable substrate, which ultimately isolates living biomass from minerotrophic groundwaters. Sedge remnants (e.g., Eriophorum vaginatum) have been identified along cores, at the FBT, across continental regions (Hughes, 2000; Hughes and Barber, 2004). This plant type, which creates dense tussocks, exhibits strong resistance to decay (Hughes and Barber, 2004) and has a great water-holding capacity (Hughes, 2000). Under this “dry route” scenario, sedge tussocks could provide Sphagnum species with micro-habitats above the groundwater influence, potentially allowing Sphagnum to rapidly grow upward, leading to a quick switch to ombrotrophy.
Both the autogenic and allogenic FBT pathways described above are in line with a growing body of evidence suggesting that peatland dynamics are non-linear. Rather than displaying gradual changes in structure and function that match the frequency of external forcing, peatlands can show long periods of little change that are punctuated with step-like transitions to alternative states in response to seemingly small internal or external forcing mechanisms (Levin, 1998; Belyea and Baird, 2006; Andersen et al., 2009; Belyea, 2009). Such abrupt transitions from one steady state to another are described as bifurcations (or tipping points), and they occur once the stability properties of an ecosystem are suddenly lost to the expense of a new (or alternative) stable state (Scheffer et al., 2001; Lenton, 2013; Milner et al., in press). The alternative ecosystem state may be maintained by self-regulating physical, biological, and/or chemical negative feedbacks, making it difficult or impossible to reverse (Scheffer and Carpenter, 2003). The difficulty to foresee these non-linear responses is concerning because changes in ecosystem structure may lead to critical shifts in ecosystem function (Lenton, 2013). As abrupt changes in peatland dynamics may directly impact peat-C sequestration rates, significant effects on the global C cycle and the climate system can result from such a non-linear behavior.
Rationale and Research Objectives
In an effort to broadly understand the response of peatlands to past, present, and future environmental change, a predictive modeling approach that links fen vs. bog traits with C exchange functions is needed. Although C dynamics are spatially variable within a single peatland due to small-scale heterogeneity associated with hummock-hollow microtopographic gradients (Waddington et al., 1996; Baird et al., 2009), there is merit in distinguishing between fens and bogs for the general purpose of coupling dynamic peatland models with Earth System Models (Frolking et al., 2009). Likewise, landscape-scale mapping of peatland types would lead to better estimates of peatland-atmosphere C exchanges (Baird et al., 2009). Also, knowing the timing of critical ecosystem shifts such as the FBT, both in the past and the future, could help model changes in peatland C dynamics and their role in the global C cycle. In particular, knowing how the extent of fens and bogs has changed throughout the Holocene would provide constraints on peatland-atmosphere greenhouse gas exchange (e.g., Frolking and Roulet, 2007; Yu et al., 2013). Indeed, it has been suggested that the progressive decrease in atmospheric CH4 concentration that occurred from 8000 to 4000 years ago might relate to the FBT across the boreal biome (MacDonald et al., 2006; Loisel et al., 2015). Likewise, Yu (2011) suggested that peat-C accumulation could have contributed to the Holocene changes in atmospheric carbon dioxide (CO2) concentration and δ13CO2 observed along Antarctic ice cores. It is also possible that, in the near future, large swaths of wet arctic tundra switch to bogs, following landscape-scale permafrost thaw and drainage (Yu et al., 2009; Hugelius et al., in press). Despite its key role in the Holocene global C cycle, little is known about the timing and mechanisms of the FBT across the mid- and high-latitude biomes.
In this study, a data synthesis of FBT timing across 90 southern Patagonian peatlands is presented. We show a regional-scale switch from fens to bogs across the region around 4200 years ago, pointing toward a regional-scale control on the FBT. We present two non-exclusive hypotheses that could explain this regional shift in peatland type. A conceptual model of the FBT is then discussed. To our knowledge, no models provide a conceptual understanding of the FBT [but see Loisel et al. (2012), where a preliminary version of this model was presented]. In this model, allogenic factors push the fen beyond its resilience capacity, inducing a regime shift to ombrotrophy. Overall, this information may be of use to improve ecological models via the identification and quantification of ecosystem functional traits between bogs and fens; it also has applications for peatland management efforts such as rehabilitation, restoration, and conservation.
Materials and Methods
Study Region
The study region is southernmost South America (SSA), which in this case refers to the landmass located between ∼ 50 and 56°S. This area includes Chile’s Magallanes region and Argentina’s portion of Tierra del Fuego (Figure 1). The regional climate is heavily influenced by the southern westerly winds, which carry moisture and heat from the Pacific Ocean onto the continent, in a west-to-east direction (Garreaud, 2009). By forcing the maritime air upward, the Andes Mountains create a strong precipitation gradient across the region (Paruelo et al., 1998). Three main peatland types are found across this gradient. First, cushion bogs are established under hyper-oceanic conditions. These ecosystems are dominated by Astelia pumila and Donatia fascicularis, two species that are tolerant of windy, stormy, and cold conditions (Auer, 1958; Moore, 1983; Pisano, 1983). Second, ombrotrophic peat bogs are located under humid to dry conditions (∼ 400–1000 mm/yr); these ecosystems are dominated by Sphagnum magellanicum carpets and scarce stunted trees (Pisano, 1983; Grootjans et al., 2010). Lastly, groundwater-fed herbaceous fens are found under dry to arid conditions (<400 mm/yr) and are usually dominated by grasses, sedges (Carex spp.), rushes (Marsippospermum grandiflorum), and a few shrubs. Peat bogs today thus occur within a narrow band of land located along the lee side of the Andes (Figure 1), where mean annual temperature is around 5°C (Loisel and Yu, 2013a), the temperature seasonality (difference between the warmest and coldest months) is around 10°C (Loisel and Yu, 2013a), and frost is rare (Schneider et al., 2003; Arroyo et al., 2005). These peat bogs are embedded within the deciduous and evergreen forests of southern Patagonia; the bogs are rain-fed and are typically found in valley bottoms atop lacustrine sediments or marine clays and silts (Bentley and McCulloch, 2005; Rabassa et al., 2006).
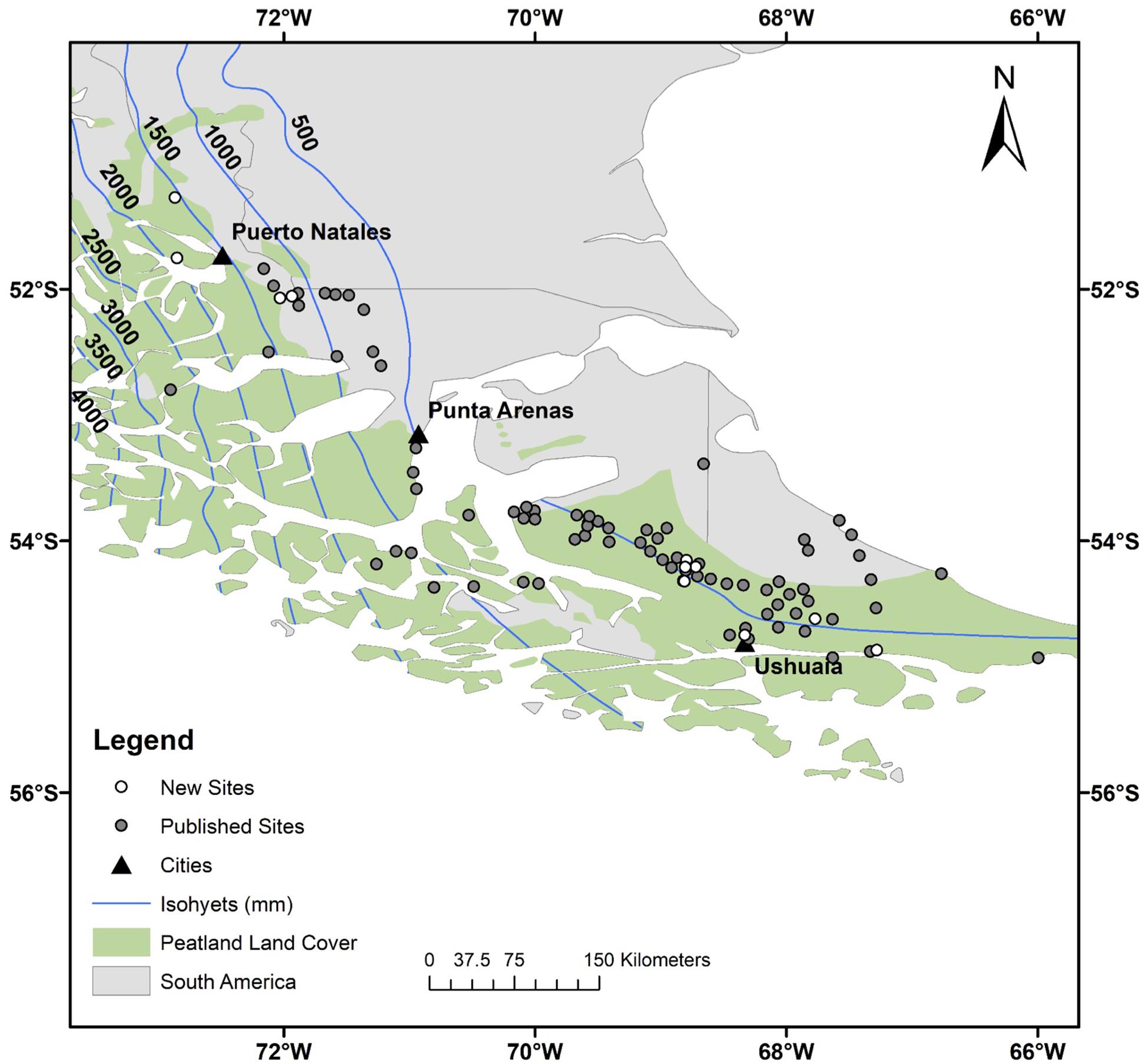
Figure 1. Study region, southern South America. The open circles indicate our 12 new cores, the gray circles show the location of the 78 sites from the literature review, and the black triangles locate the main towns. The peatland area is from Yu et al. (2010) and the dataset used to generate the isohyets is from New et al. (2002). Detailed maps with site names can be found in the Supplementary Information (Supplementary Figure S1).
New Peat Core Data
This study includes a total of 90 peatlands (Supplementary Figure S1), of which 12 were sampled by our team over the course of two field seasons (Supplementary Table S1). In 2010 (PAT-10), four peat bogs were visited and a core was retrieved at each one of the following sites (unofficial names): Harberton (HB), Cerro Negro (CN), Upper Andorra Valley (UAV), and Escondido (ESC). Some results from these cores have been published elsewhere (Loisel and Yu, 2013a, b; Loisel, 2015). In 2018 (PAT-18), eight new sites were cored: Beef-Penguin (BP), Mercedes (MP), Jen (JB), Rasmussen (RAS), Ariel (AP), Cura (CP), Pat-Andy-Nat (PAN), and Flarks (FP). Some of the results from cores MP and BP are published (Bunsen and Loisel, 2020), while those from the other cores remain unpublished at the moment (but see Bunsen, 2020). Note that Ariel Peatland has also been studied by Xia et al. (2018, 2020); likewise, Harberton Bog was the object of previous studies (e.g., Markgraf, 1991; Pendall et al., 2001).
Eleven of the twelve sites are S. magellanicum peat bogs that are characterized by microtopographic gradients, from dry hummocks to wet hollows. Shrubs Empetrum rubrum and Nothofagus antarctica were identified at all sites, with the exception of Jen Bog and Mercedes Peatland, where N. antarctica was replaced by cypress tree Pilgerodendron uviferum, a conifer only found in hyper-humid habitats of Patagonia (Moore, 1983). The sedge and grass communities typically included Marsippospermum grandiflorum, Tetroncium magellanicum, Alopecurus magellanicus, Carex magellanica, and Carex curta. The 12th site (Cura Peatland) is a spring-fed fen dominated by herbaceous plants and Bryales. Cores were extracted in 50-cm increments using a Russian auger. The stratigraphy of each section was described in the field in terms of main peat types (moss, herbaceous, ligneous, etc.) and soil properties (texture, particle size, color). The cores were then wrapped in plastic and foil, and stored in PVC pipe for shipping. Cores were kept at 4°C until lab analyses. Inception age varies between 15,550 and 1450 calibrated years Before Present (cal. yr BP). All 11 peat bog cores exhibit a clear, sharp switch from herbaceous-dominated fen peat to Sphagnum-dominated bog peat (Figure 2). The FBT has thus occurred at some point during the Holocene at these sites.
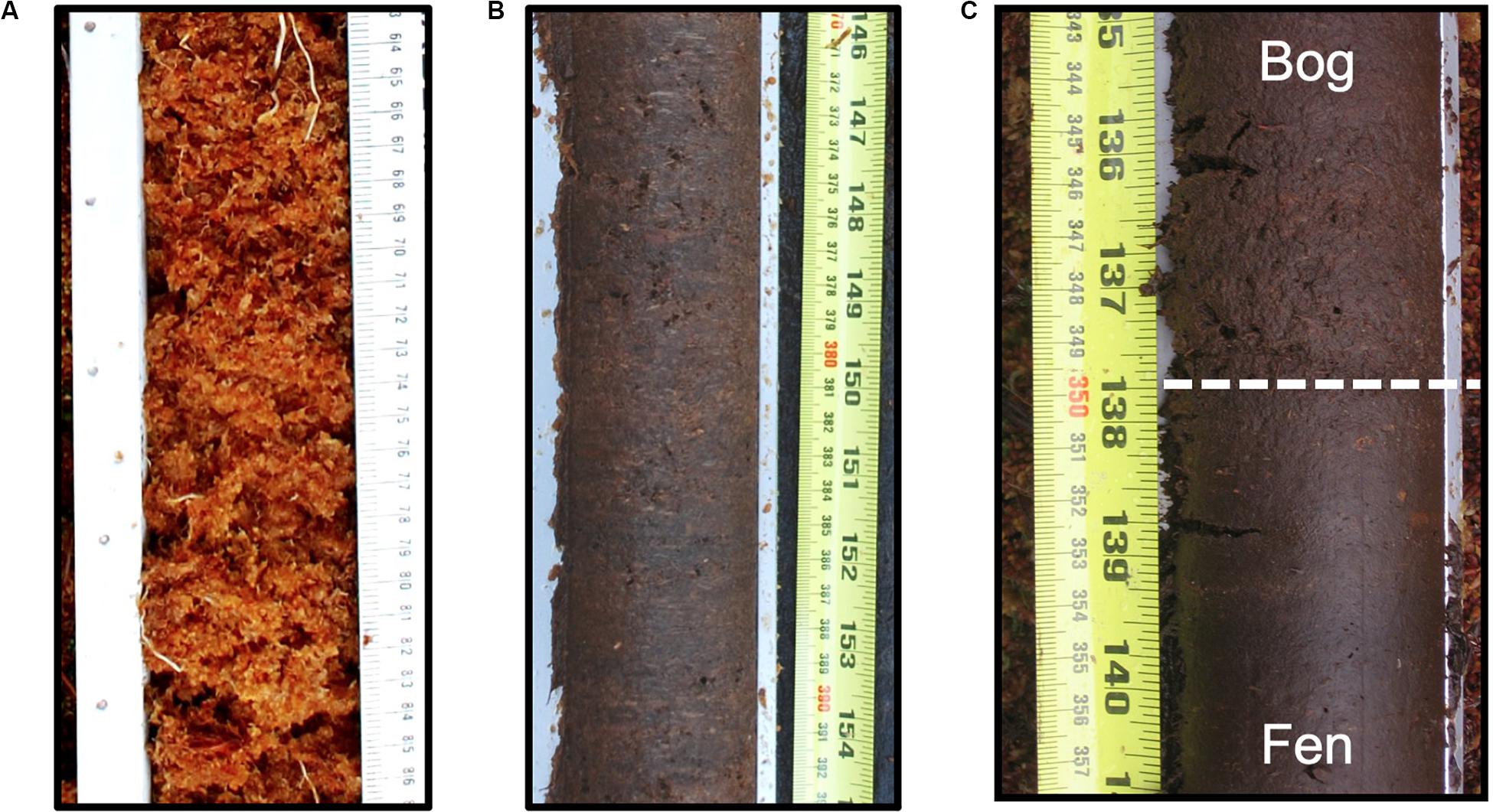
Figure 2. Peat stratigraphic characteristics. (A) Sphagnum peat, poorly decomposed (core PAT10-HB). (B) Herbaceous peat, highly decomposed (core PAT10-CN). (C) Fen-bog transition along a peat core (site PAT10-CN).
Peat core chronology was constrained using radiocarbon (14C) dating (Supplementary Table S2). Hand-picked plant macrofossils were cleaned with deionized water, dried, and 14C-dated using AMS at the University of California – Irvine’s Keck AMS Carbon Cycle Lab (samples collected in 2010) and at Lawrence Livermore National Lab’s CAMS Radiocarbon Lab (samples collected in 2018). When the samples were too decomposed for the identification and cleaning of plant macrofossils, root-free bulk peat samples (63–125 μm) were used instead. As the regional tephrochronology is relatively well known (e.g., Stern, 2008), a few volcanic tephras were identified along our peat profiles on the basis of visual examination (in the field) as well as changes in bulk density (in the lab). The tephra layers that were identified are: Aguilera I, Burney I and II, Hudson I, and Reclus I, as well as cryptotephras Hudson a and b (Mansilla et al., 2016; Supplementary Table S3). While they were not used to build the chronologies, the tephras were used to confirm our age-depth models and compare core stratigraphy between sites.
Age-depth models for the four PAT-10 cores (HB, CN, UAV, and ESC) have been published in Loisel and Yu (2013a) but have been updated for this study, in light of more recent calibration curves being available. Age-depth models for the eight PAT-18 cores (BP, MP, JB, AP, CP, FP PAN, and RAS) are available in Bunsen (2020). All raw and calibrated 14C data, as well as our final age-depth models, are included in the Supplements (Supplementary Table S2 and Supplementary Figure S2), along with tephra ages obtained from the literature (Supplementary Table S3) and bulk density profiles from all 12 cores to show the stratigraphic location of those tephras (Supplementary Figure S3). We used the SHCal13 (Hogg et al., 2013; Reimer et al., 2013) and SHZ 1-2 calibration curves (Hua and Barbetti, 2004), along with Bacon version 2.3.5 (Blaauw and Christen, 2011) to constrain peat chronologies.
Geochemical measurements were performed at high resolution along all 12 cores (Supplementary Figure S3). Along the PAT-10 cores, contiguous sub-samples (1 cm3) were analyzed every cm; the sample interval was increased to 2-cm increments along the PAT-18 cores, though sub-samples (2 cm3) were still measured contiguously. Loss-on-ignition (LOI) was performed to determine peat water content, organic matter content, dry bulk density, and organic matter density on each sub-sample (total = 3854 samples); standard procedures were followed (Dean, 1974). Changes in peat water content and organic matter density were used to infer stratigraphic changes in peat type along the cores for which plant macrofossil analysis has not yet been performed. This inference is based on a statistical analysis that was performed on 438 Patagonian peat samples for which plant fossil analysis as well as geochemical measurements were performed (see Loisel and Yu, 2013a). Results indicate that low water content and high density are associated with herbaceous peat, and high water content and low density are correlated with Sphagnum peat (Loisel and Yu, 2013a). Lastly, peat-carbon accumulation rates (PCAR) were obtained by multiplying the organic matter density of each depth increment (in g OM/cm3) by the interpolated deposition rate of each sample (from the age-depth models, in cm/yr) and by an assumed 50% C content (Loisel and Yu, 2013a).
Data Synthesis
A compilation of 78 peat core stratigraphies from southernmost Patagonia was used to infer the timing of the FBT across the region. The quality of data varies across the different sources, from visual descriptions of peat stratigraphic changes (Auer, 1965) to semi-quantitative plant macrofossil-based reconstructions (De Vleeschouwer et al., 2014). Radiocarbon-based age-depth models were unavailable for Auer’s (1965) cores; instead, peat stratigraphic changes are described in relation with three tephra layers of known ages (Stern, 2008): Reclus I (15,780–14,040 cal. yr BP; median age = 14,900 cal. yr BP), Hudson I (7960–7420 cal. yr BP; median age = 7700 cal. yr BP), and Burney II (5290–3230 cal. yr BP; median age = 4200 cal. yr BP). We used these three tephra layers to compare changes in peat type, total peat mass (gOM/cm2), and PCAR (gC/m2/yr) between all 78 cores from the literature as well as our 12 new cores. Lastly, peat depths were converted to organic matter density, peat mass, and PCAR using the following values: 0.05 g OM cm3 for Sphagnum peat and 0.07 g OM cm3 for herbaceous (i.e., Carex spp., Marsippospermum sp.) and other non-Sphagnum (i.e., Bryales) peat types (Loisel and Yu, 2013a).
Results
Peat Stratigraphy and FBT Timing Across Southern Patagonia
New Cores
The stratigraphic records from our 12 new cores are presented in Figure 3. Each site started its development as a fen, and 11 out of 12 sites evolved into a bog during the mid- and late-Holocene time periods (Figure 3). Of these 11 sites, six switched to bogs right after the deposition of tephra layers (CN, MP, FP, PAN, RAS, and AP). While the FBT at site AP followed the Hudson I eruption (7960–7420 cal. yr BP), the transition occurred after the Burney II eruption (5290–3230 cal. yr BP) at the other five sites. Sites ESC and BP switched to bogs prior to those eruptions, around 4700 and 10,000 cal. yr BP, respectively. One site (HB) is too young for having been affected by any eruption and records a FBT around 1000 cal. yr BP. The last two sites (JB and UAV) transitioned into bogs around 1200 and 2300 cal. yr BP, respectively.
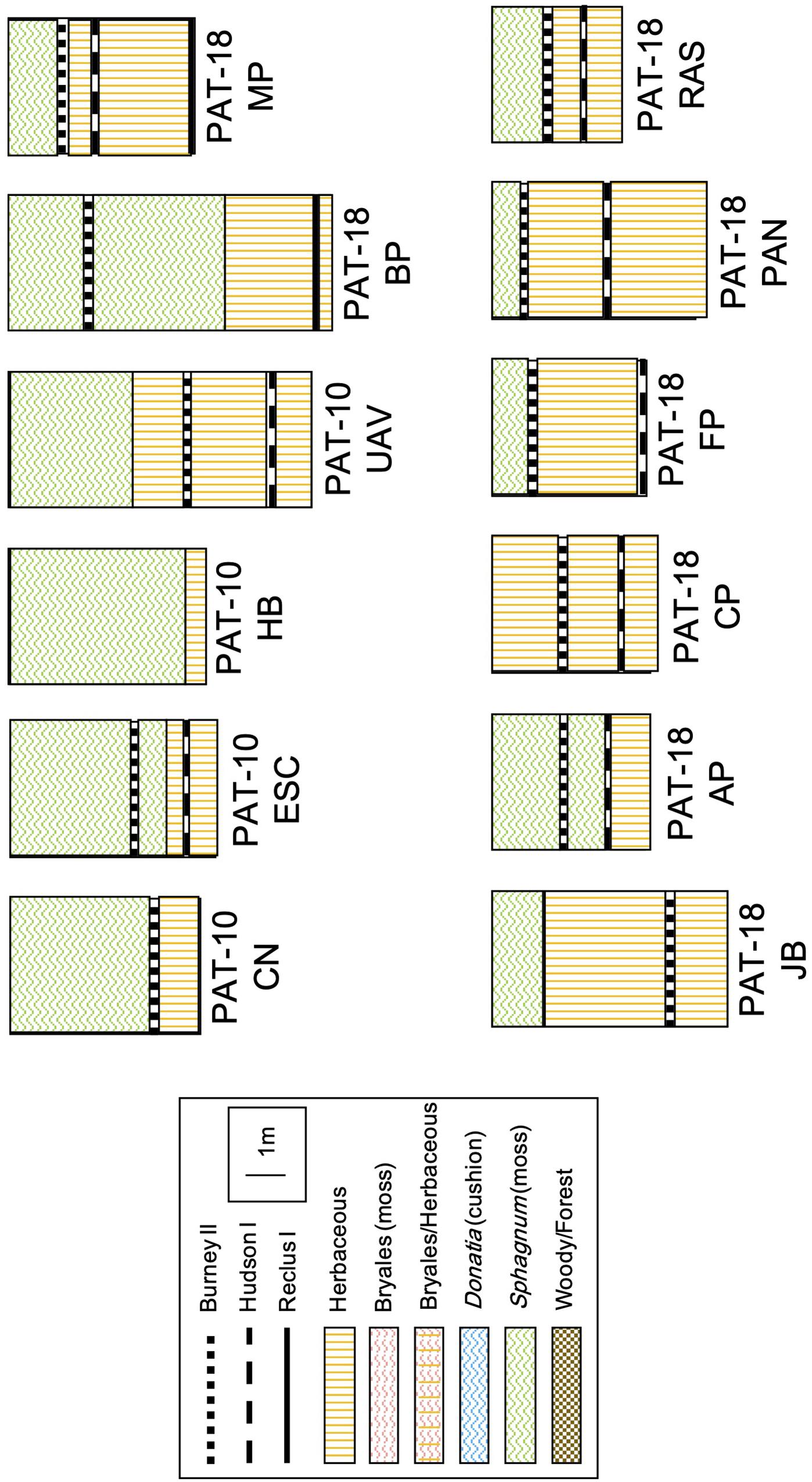
Figure 3. Peat stratigraphic profiles for the 12 new sites included in this study. The sketches are true to scale. The remaining 78 peat profiles from the literature review can be found in the Supplementary Information (Supplementary Figure S4).
Data Synthesis
The synthesis included 78 peat profiles; the majority (63 out of 78 sites) started their development as herbaceous-dominated or Bryales-dominated fens (Supplementary Figure S4). Of the remainder, two sites started as Donatia-dominated cushion bogs, another one began its development as a forested peatland, and the remainder (11 out of 78 sites) started as Sphagnum bogs. As for today’s vegetation communities, 47 of the 78 sites are now Sphagnum-dominated bogs, five are cushion bogs, and 26 are fens. At a few sites (T1, T2, and T3), Sphagnum deposits that pre-date the Reclus I eruption (14,900 cal. yr BP) have been identified (Supplementary Figure S4). Those are exceptional cases, with most Sphagnum deposits developing during the mid- and late-Holocene. Indeed, 35 out of the 47 Sphagnum-dominated sites transitioned from fen to bog right after the deposition of tephra layers. As is the case along our own cores, some peatlands switched to bogs following the Hudson I eruption (7960–7420 cal. yr BP; n = 5), though the majority transitioned after the Burney II eruption (5290–3230 cal. yr BP; n = 30). While sites T3, T5, and HAB switched to bogs prior to those eruptions, five other sites switched between those events (T14, T15, T56, T62, and P52); T29 and Onamonte transitioned after the Burney II eruption (Supplementary Figure S4).
Peat Accumulation Across the FBT in Southern Patagonia
New Cores
In general, our 12 study peatlands have been effective C sinks over the Holocene (Figure 3 and Supplementary Table S4). Core analysis reveals a median thickness of 452 cm (min = 290, max = 770) and a median soil C density of 130 kgC/m2 (min = 89, max = 220). The rate of peat accumulation has not been constant through time across our sites (refer to Supplementary Figure S3 for details). For instance, mean PCAR for the time period between Reclus I (14,900 cal. yr BP) and Hudson I (7700 cal. yr BP) amounts to 7 gC/m2/yr (Supplementary Table S4). This figure reaches 13 gC/m2/yr during the time period between Hudson 1 and Burney II (4200 cal. yr BP) eruptions. It then rises up to 22 gC/m2/yr for the last 4200 cal. yr BP (Supplementary Table S4). While these figures account differentially for long-term decomposition and peat compaction on the basis of their age (i.e., the young peat has not decomposed or compacted as much as the old one), they are still informative.
Data Synthesis
There are large differences in terms of peat depth and peat C density across the 78 sites described in the literature (Supplementary Figure S4 and Supplementary Table S4). Median peat thickness amounts to 345 cm (min = 60, max = 1400), which translates to a median soil C density of 119 kgC/m2 (min = 21, max = 375). Temporal changes in the rate of peat accumulation have also occurred across the 78 sites (refer to Supplementary Figure S3 for detailed results). In general, and similar to the findings from our own cores, PCARs were lower than average between the Reclus I (14,900 cal. yr BP) and Hudson I (7700 cal. yr BP) eruptions, with a mean of 7 gC/m2/yr (Supplementary Table S4). This figure increases to 11 gC/m2/yr during the time period between Hudson 1 and Burney II (4200 cal. yr BP) eruptions, and remains about the same (12 gC/m2/yr) over the past 4200 cal. yr BP (Supplementary Table S4).
Discussion
Spatial and Temporal Patterns of the FBT Across Southern Patagonia
Altogether, 58 out of the 90 surveyed peatlands are bogs today (Figure 3 and Supplementary Figure S4). Out of these 58 Sphagnum-dominated bogs, 35 transitioned from their fen state at 4200 cal. yr BP (Burney II eruption), six switched to bogs at 7700 cal. yr BP (Hudson I eruption), and 13 underwent the FBT at other times (Figure 4). As for the sites that did not respond to those eruptions and switched to bogs at later stages (e.g., JB, UAV, ONA, and RR), it is possible that the eruption (or its tephra) was not sufficient to destabilize the fen conditions. As for the sites that switched prior to those eruptions (e.g., ESC, BP, and HAB), we do not have sufficient evidence to suggest alternative triggering mechanisms at this time, though drying conditions during the mid-Holocene might have facilitated the colonization by Sphagnum (e.g., Hughes, 2000; Hughes and Barber, 2004). Of the remaining 32 sites, 27 have persisted as fens for their entire history while the other five are Donatia-dominated cushion bogs today (Supplementary Figure S4). The temporal pattern offered by the FBT is striking, with a majority of sites simultaneously switching to bogs across SSA (Figure 4).
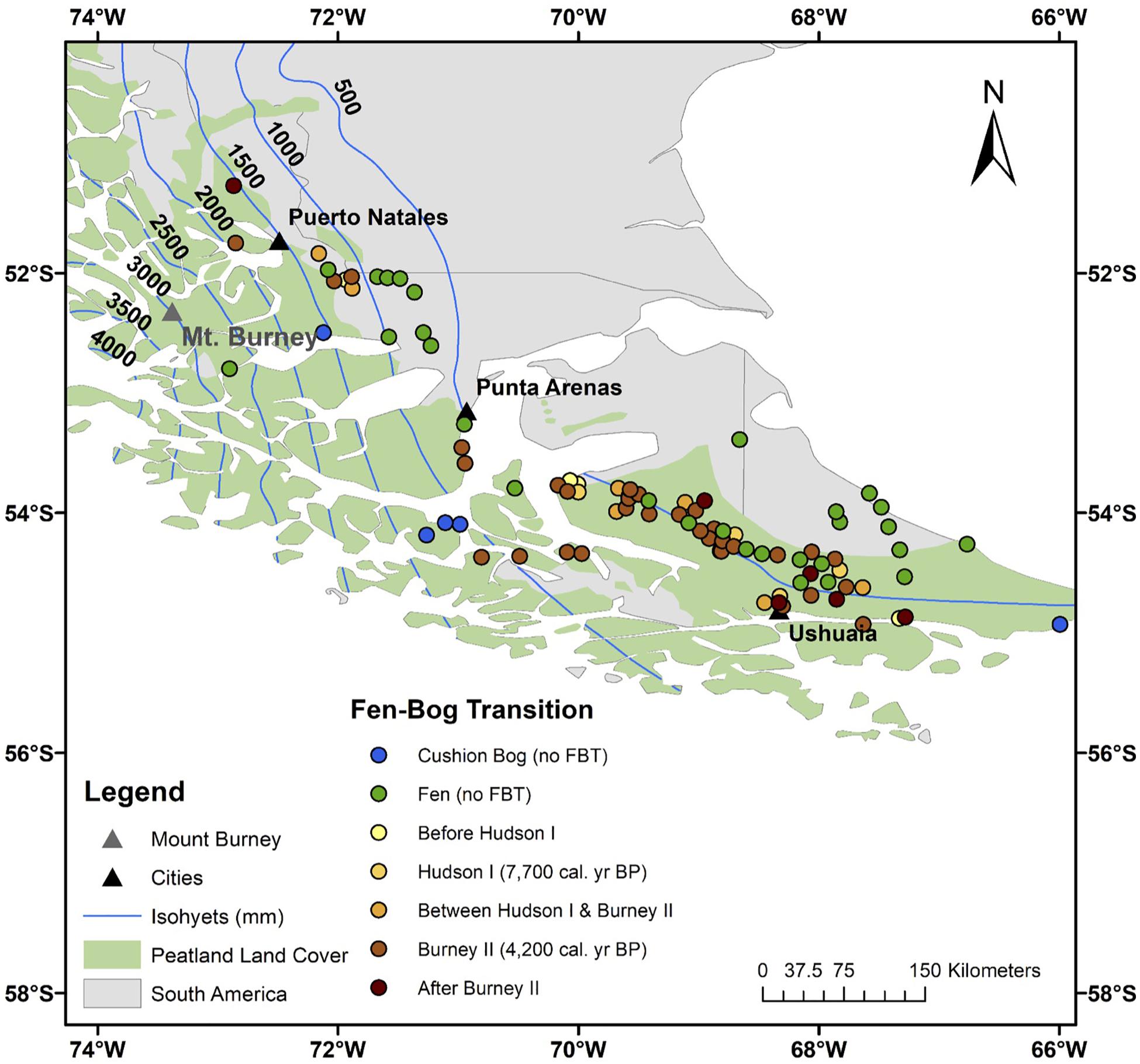
Figure 4. Fen-bog transition timing across southern South America. Non-Sphagnum peatlands (fens and cushion bogs) are also shown. The location of Mount Burney is indicated by a gray triangle. Mount Hudson (45.9 S, 72.97 W) is located outside the area shown on the map. The peatland area is from Yu et al. (2010) and the dataset used to generate the isohyets is from New et al. (2002).
Some peatland-rich areas of southernmost Patagonia are found outside the Sphagnum moss domain. As such, the five cushion bogs cataloged in this study (T40, T41, T42, T60, and SKY-2) are located off the western Chilean coast and along the Strait of Magellan, in areas of either very high precipitation, high wind, or storminess (Supplementary Figure S4; see Supplementary Figure S1 for detailed location of these sites). Though tephra layers were identified along these core profiles, Sphagnum moss is not typically encountered in these two regions today, due to climatic limitations (Pisano, 1983). That said, the co-occurrence of Donatia and Sphagnum has been observed in a few peatlands located near the current limit of Sphagnum bogs (near sites PAT-18-MP, between sites PAT-10-HB and T60, and at site SKY). In this area, to our knowledge, at least one peat core harbors switches between cushion- and Sphagnum-dominated assemblages (Mathijssen et al., 2019). But for the sake of this study, which focuses on the FBT, we will not investigate this topic further.
Another outstanding spatial pattern from our analysis is the location of 27 “persistent fens.” These peatlands have not undergone the FBT despite having been influenced by volcanic eruptions (Figure 3 and Supplementary Figure S4). Of these persistent fens, 13 sites are clearly found in the steppe ecoregion (T36, T37, T51, T64, T65, T66, T67, T69, P57, P58, P60, P61, and P65), making it unlikely for Sphagnum to colonize these areas due to local hydroclimatic limitations (Supplementary Figure S4; see Supplementary Figure S1 for detailed locations). That said, several sites that are either located near the modern-day boundary between the steppe and forest ecoregions (T7, T19, T30, T31, T32, T63, and P63) or within the Sphagnum bog domain (PAT-18-CP, T23, T26, T58, T61, P53, and P56) also persisted as fens. In those cases, a number of local conditions that would prohibit oligotrophy, including insufficient peat thickness, the presence of mineral-rich springs or surface runoff from surrounding hillslopes, and other conditions such as inadequate parent material or topography, could have prevented the switch to bog conditions. In particular, many of these peatlands are found in complex moraine basins that are probably strongly influenced by groundwater (Auer, 1965; Coronato et al., 2006; Rabassa et al., 2006).
Spatial and Temporal Patterns of Peat Accumulation Across the FBT
A comparative analysis of Sphagnum vs. non-Sphagnum peat mass revealed that Sphagnum-dominated peatlands are characterized by greater peat stores, and thus larger soil C stocks, than their non-Sphagnum counterparts (Tukey’s HSD: p < 0.001, except for the period prior to 14,900 cal. yr BP, where the difference between the two peat types was not statistically significant). This finding is consistent through the different time periods (Figure 5). We observed relatively consistent peat mass values for both Sphagnum (median ∼ 12 g/cm2) and non-Sphagnum (median ∼4 g/cm2) peat types through time, though these figures should not be directly compared, as each time period has a different duration. To alleviate this issue, PCAR was calculated to standardize changes in peat mass over time.
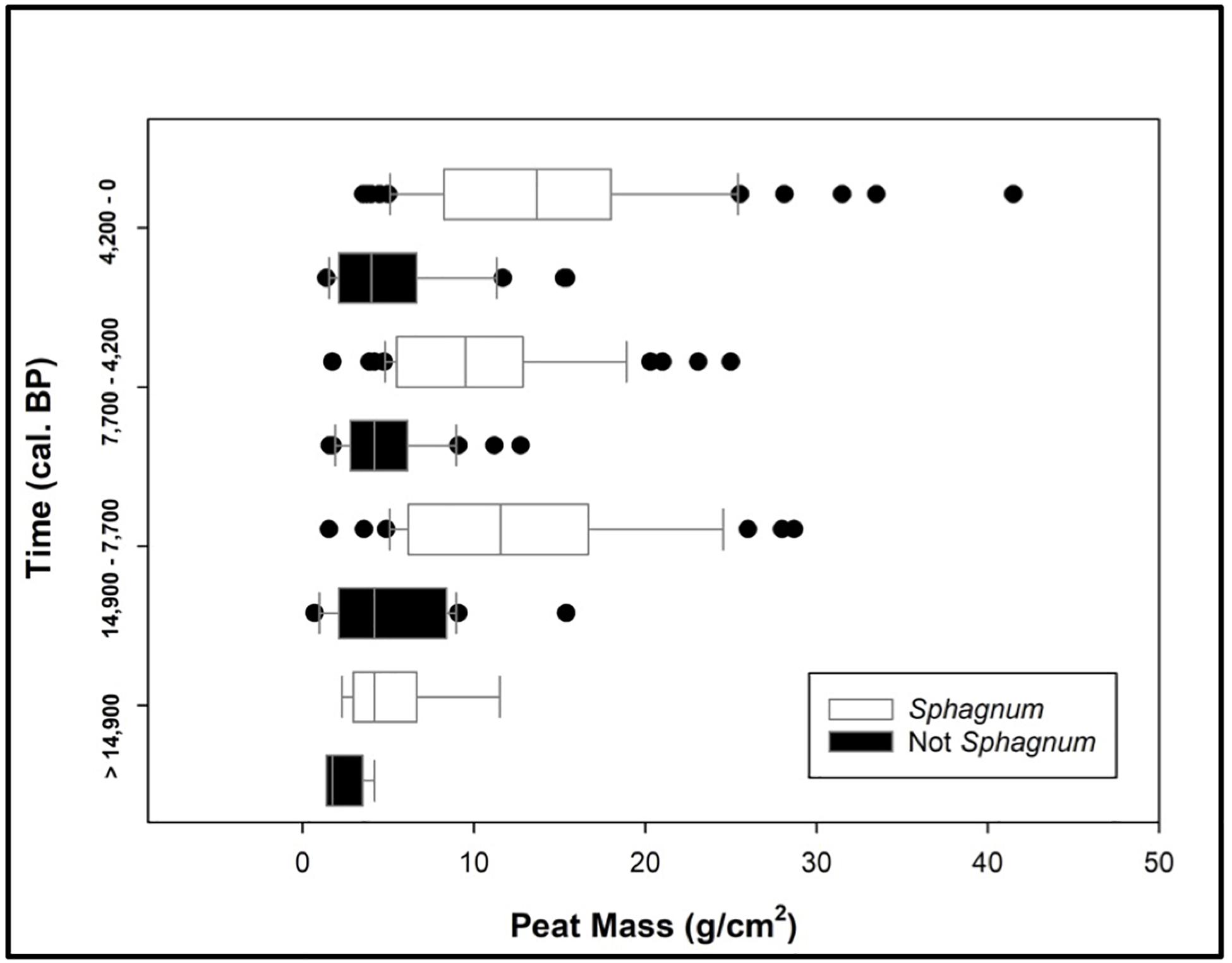
Figure 5. A comparative analysis of Sphagnum vs. non-Sphagnum peat masses over time. The time periods are dictated by three tephra layers: Reclus I (14,900 cal. yr BP), Hudson I (7700 cal. yr BP), and Burney II (4200 cal. yr BP). Sphagnum and non-Sphagnum peat masses are statistically different for every time period (Tukey’s HSD: p < 0.0001), except prior to 14,900 cal. yr BP.
There are two outstanding patterns that come out of the PCAR data (Figure 6). First, Sphagnum peat is consistently accumulating at greater rates than its non-Sphagnum counterpart (Tukey’s HSD: p < 0.001, except for the period between 14,900 and 7700 cal. yr BP, where the difference between the two peat types was not statistically significant), with median apparent rates of C accumulation that progressively increase through time, from 10 gC/m2/yr between Reclus I and Hudson I, to 16 gC/m2/yr between Hudson I and Burney II, and to 20 gC/m2/yr from 4200 cal. yr BP to modern times (Figure 6). Second, non-Sphagnum PCARs remain uniform throughout all three time periods, with a median of 7 gC/m2/yr (Figure 6). To normalize PCAR values for each peatland developmental history, time-weighted PCARs were calculated for the Sphagnum and non-Sphagnum peatland sites (Figure 7). This calculation multiplies the PCAR from each time period by its duration to ensure that each period is adequately represented in the “whole core PCAR.” We find, once again, that Sphagnum sites have been characterized by an overall greater C-sink capacity than non-Sphagnum sites (Tukey’s HSD: p < 0.00001), with median time-weighted PCAR values of 17 gC/m2/yr (min = 4, max = 49) for Sphagnum and 8 gC/m2/yr (min = 2, max = 33) for non-Sphagnum. Note that, amongst the non-Sphagnum sites, time-weighted PCAR ranged from 7 to 10 gC/m2/yr for cushion bogs and from 2 to 33 gC/m2/yr for persistent fens.
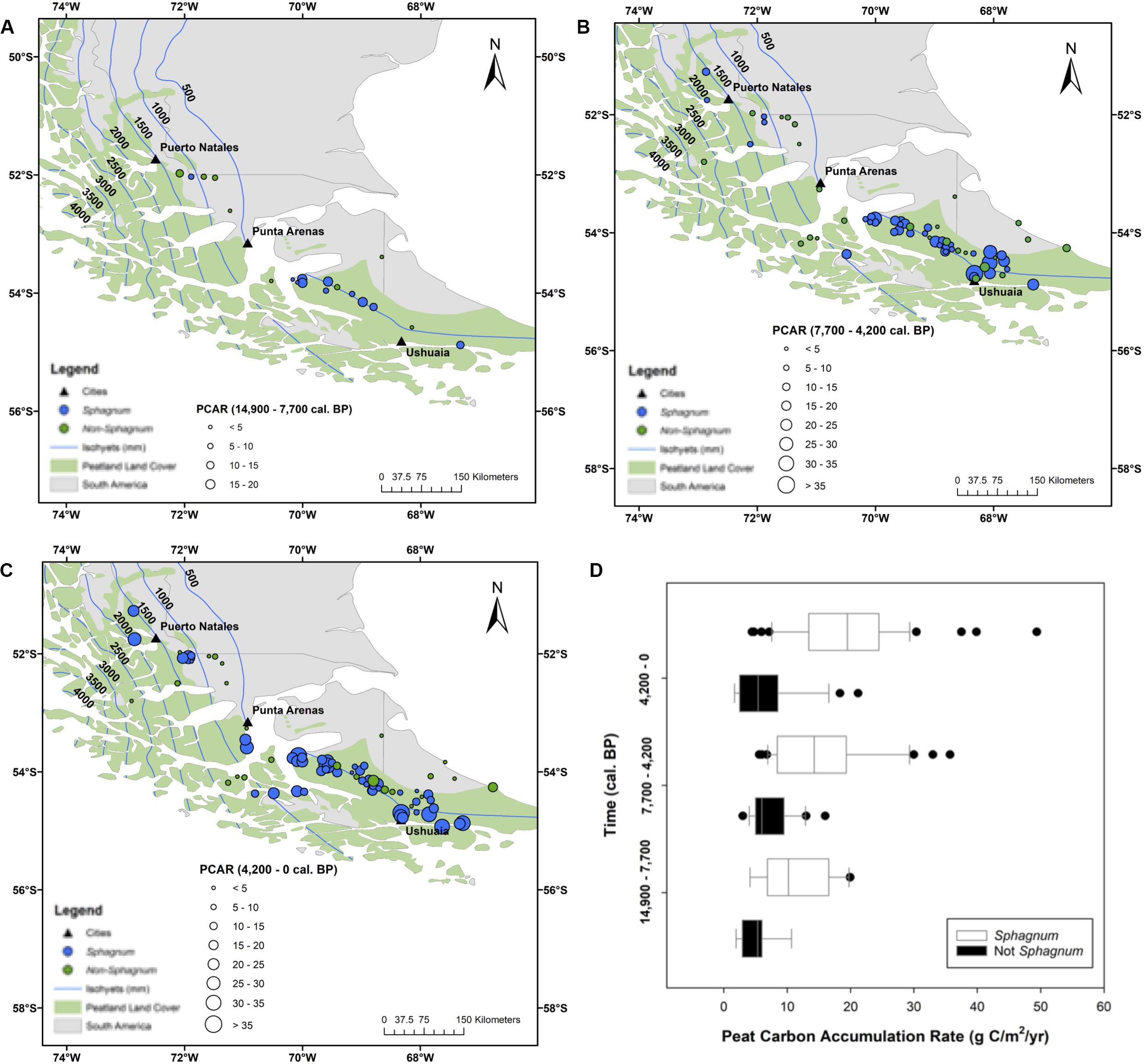
Figure 6. A comparative analysis of Sphagnum vs. non-Sphagnum peat-carbon accumulation rates (PCAR) over time. (A–C) Spatial distribution of Sphagnum (blue circles) and non-Sphagnum (in green) peatlands and associated PCAR (circle size). (D) Temporal distribution of PCAR changes between Sphagnum (white boxes) and non-Sphagnum (black boxes) peat types. The time periods are dictated by three tephra layers: Reclus I (14,900 cal. yr BP), Hudson I (7700 cal. yr BP), and Burney II (4200 yr cal. BP). Sphagnum and non-Sphagnum peat masses are statistically different for every time period (Tukey’s HSD: p < 0.001), except from 14,900 to 7700 cal. yr BP. The peatland area is from Yu et al. (2010) and the dataset used to generate the isohyets is from New et al. (2002).
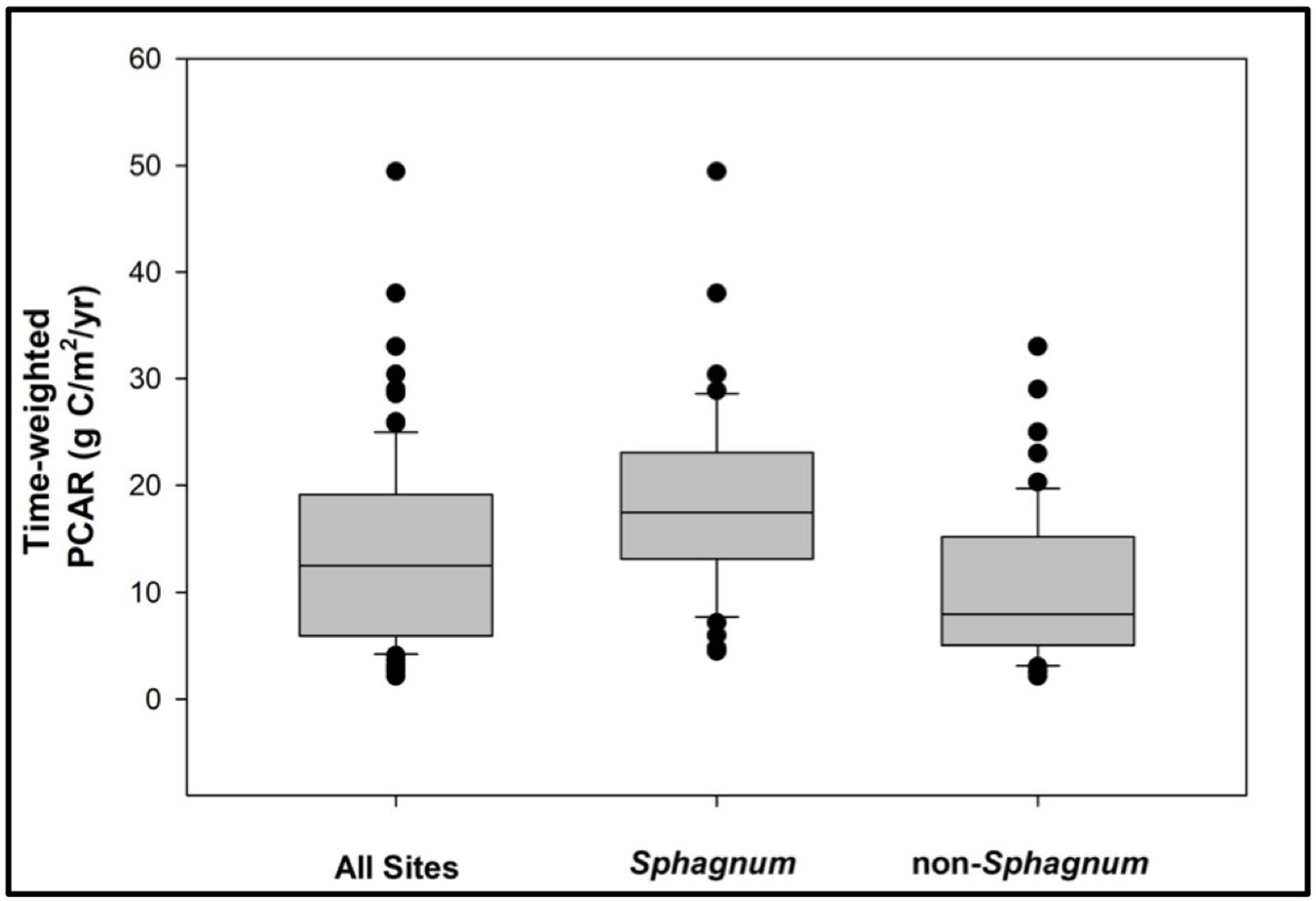
Figure 7. A comparative analysis of time-weighted peat-carbon accumulation rates (PCAR) for Sphagnum vs. non-Sphagnum peat types. The Sphagnum sites have been characterized by an overall greater C-sink capacity than the non-Sphagnum sites (Tukey’s HSD: p < 0.00001).
A comparison of the Sphagnum vs. non-Sphagnum portions of the cores reveals that, since the Reclus I eruption, approximately 42% of the total peat mass accumulated at these 90 sites is made of Sphagnum peat (Figure 3). This figure considers organic matter density differences between Sphagnum (0.05 gOM/cm3) and non-Sphagnum peat (0.07 gOM/cm3). For comparison, Sphagnum peat has dominated the regional peatland landscapes during approximately 30% of the entire peat history, as reconstructed from the cores. In other words, though Sphagnum peat is less dense than its non-Sphagnum counterpart, it has built a peat deposit that is greater (42%) than the total amount of time (30%) these peatlands have been Sphagnum-dominated bogs. Likewise, core analysis reveals a median peat C mass (or median soil C density) of 119 kgC/m2 (Figure 8 and Supplementary Table S4), with modern-day Sphagnum bogs having significantly greater C storage (141 kgC/m2) than their non-Sphagnum counterparts (56 kgC/m2; Tukey’s HSD: p < 0.00001). A regional map of peatland C density, which also reports whether each site is a Sphagnum or non-Sphagnum peatland, suggests that the greatest peat C stocks might be found along the eastern and southern portions of Isla Grande, in Tierra del Fuego (Figure 8).
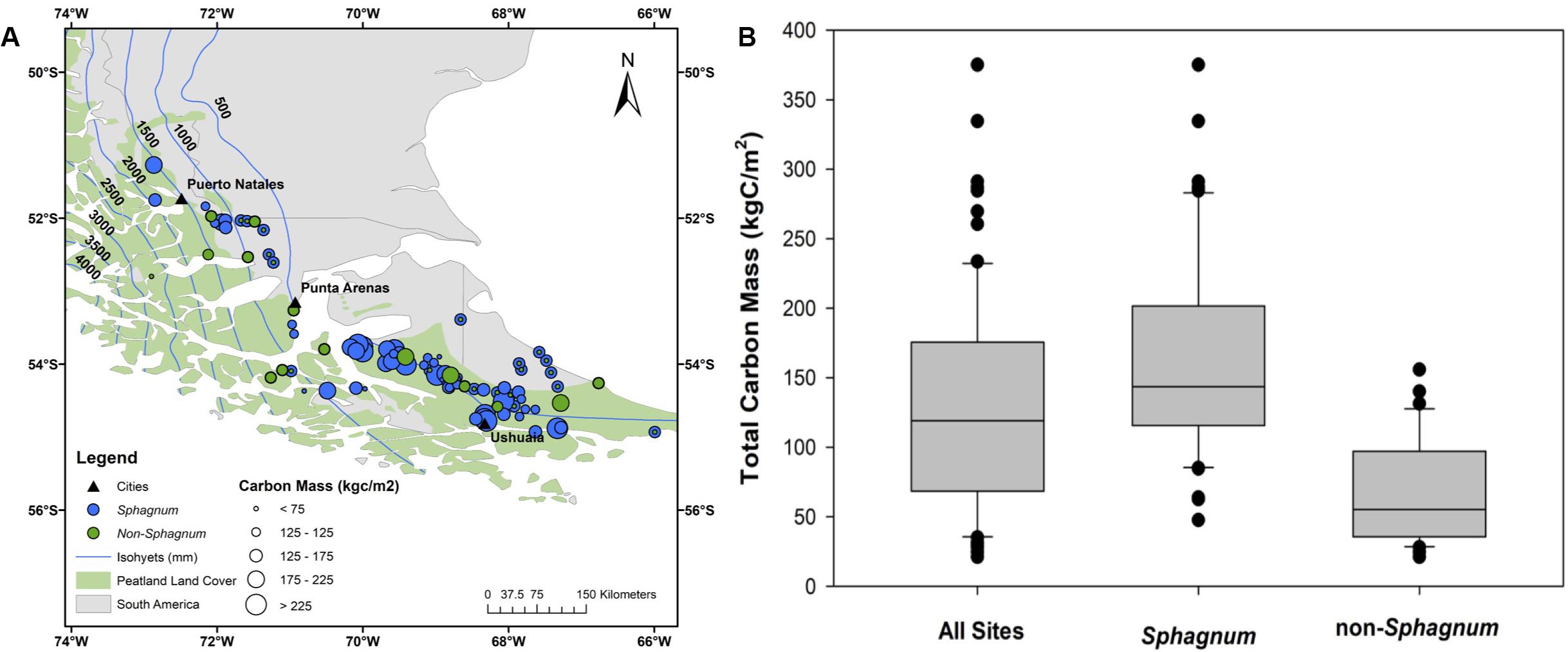
Figure 8. A comparative analysis of Sphagnum vs. non-Sphagnum peat carbon mass per unit area (also referred to as soil carbon density in the text). (A) Spatial distribution of Sphagnum (blue circles) and non-Sphagnum (in green) peatlands and associated carbon mass (circle size). (B) Statistical representation of carbon mass for Sphagnum and non-Sphagnum peat types compared to all sites combined. The peatland area is from Yu et al. (2010) and the dataset used to generate the isohyets is from New et al. (2002).
Given the high proportion of sites that exhibit their FBT at 4200 cal. yr BP, we specifically analyzed peat mass and PCAR differences between sites that switched to bogs after the Burney II eruption vs. those that did not (Figure 9). We find that, on average, sites that switched to bogs accumulated significantly more peat (and stored more C) than sites that remained fens or cushion bogs (Tukey’s HSD: p < 0.00001). These figures amount to a median peat mass of 15 g/cm2, equivalent to 19 gC/m2/yr for the 58 bogs, vs. a median peat mass of 4 g/cm2 or 5 gC/m2/yr for the other 32 sites (Figure 9).
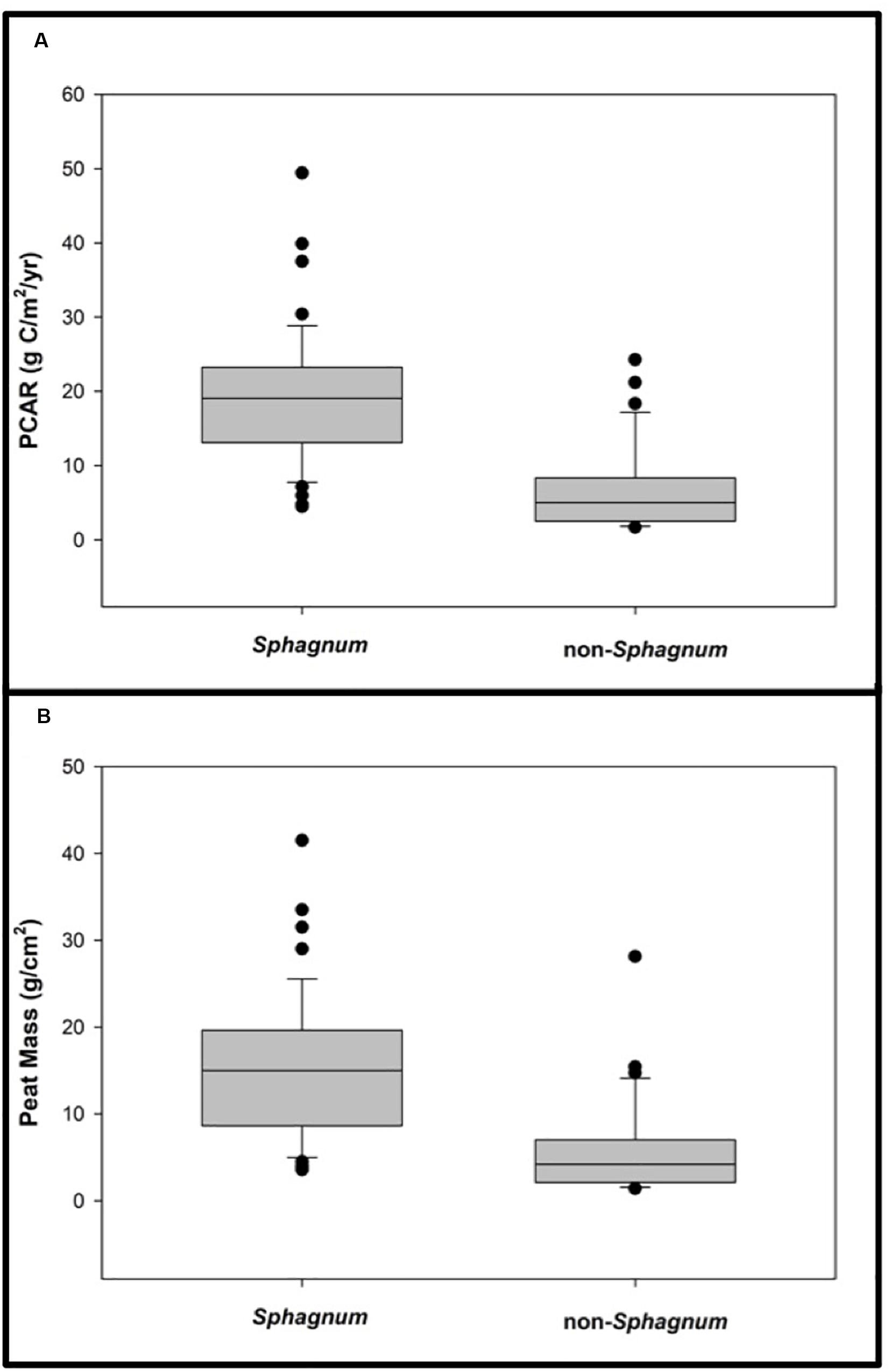
Figure 9. A comparative analysis of Sphagnum vs. non-Sphagnum peat properties since the Mount Burney II eruption (4200 cal. yr BP). (A) Sphagnum vs. non-Sphagnum peat carbon accumulation rates since 4200 cal. yr BP. (B) Sphagnum vs. non-Sphagnum peat masses. Both analyses reveal that sites that switched to bogs accumulated significantly more peat (and stored more carbon) than sites that remained fens or cushion bogs (Tukey’s HSD: p < 0.00001).
Potential Mechanisms for the FBT
In light of tipping point theory, we argue that destabilizing forces are responsible for the FBT. These positive feedbacks consist of autogenic processes that can be triggered by a perturbation that helps “tip” a fen system into a bog state, by either dampening or amplifying a number of peatland processes. We propose two mechanisms that could explain the surge of Sphagnum during the late-Holocene: (1) volcanic impact, and (2) change in hydroclimate.
Volcanic Impact
Auer (1965) was the first to suggest that tephra layers might have aided the formation of Sphagnum bogs. He speculated that these tephra layers might have provided a dry and acidic substrate that also prevented evaporation across large swaths of land, thereby facilitating Sphagnum invasion. We postulate that the idea of a volcanic impact capable of inducing a regional-scale FBT is plausible. In fact, Kilian et al. (2006) suggested that the Burney II eruption led to long-lasting damage to forest, aquatic and peatland ecosystems in the vicinity of the stratovolcano. Our scenario implies a much broader, regional-scale impact associated with this eruption. We consider a scenario under which the combined effects of volcanic fallout (i.e., tephra) and sulfur dioxide (SO2) plumes caused intense peatland acidification because of fens weak buffering capacity (Gorham et al., 1984), which led to nutrient seepage and an abrupt ecosystem shift to bog. While it is possible that the tephra itself provided a “dry” substrate for Sphagnum, as proposed by Auer (1965), we are less comfortable with this idea, given that our stratigraphic evidence suggests that the Burney II ash layer was often less than 1 cm in thickness. Conversely, the potential impact of an acidic SO2 plume on the regional vegetation communities is worth considering, as those plumes could take place over the course of many years as the volcano is degassing, dissimilar to the 1-time tephra fallout event. This “volcanic impact” scenario suggests that a disturbance induced an abrupt acidification of the peatland surface, which facilitated Sphagnum establishment, which led to the FBT. In light of tipping point theory, this scenario implies that the new conditions brought about by the tephra and/or the SO2 plume were able to promote the bog state at the expense of the fen state.
Change in Hydroclimate
While the broad-scale synchronicity of the FBT with a tephra layer makes the volcanic scenario a strong candidate for the observed pattern, the influence of climate on the FBT cannot be overlooked. The Burney II tephra was deposited toward the end of the co-called sub-Boreal time period (Auer, 1965), which was characterized by generally dry conditions. It is indeed possible that fens were drying up and that peat accumulation was slowing down due to enhanced decomposition. While the volcanic eruption might have facilitated the FBT, the underlying dry conditions could have been essential to the establishment of bog conditions. This dry climate scenario could also explain why a number of sites switched to bogs between Hudson I and Burney II, without “help” from a volcanic eruption. These ideas make intuitive sense and are corroborated by recent paleoclimate reconstructions. In particular, we know that many glaciers in the Patagonian Icefield, on South Georgia and James Ross Islands, and along the Antarctic Peninsula receded between ∼ 4500 and 2700 cal. yr BP (Mulvaney et al., 2012; Sterken et al., 2012; Anyia, 2013; Strelin et al., 2014; Kaplan et al., 2016; Oppedal et al., 2018). Peatlands expanded across sub-Antarctica during these times as well (e.g., Yu et al., 2016; Loisel et al., 2017b). Another aspect of the regional paleoclimate is a subsequent increase in effective moisture around 2700 cal. yr BP (e.g., Moreno et al., 2018; Oppedal et al., 2018). Glacial advances have been described for this time period; it is possible that Sphagnum moss benefited from wetter conditions, which could also explain the recent increases in peat mass and PCAR (Figure 9). The FBT across southern Patagonia is also contemporaneous with the “4.2 ka event,” which has been observed in many parts of the world (e.g., Bond et al., 2001; Wanner et al., 2008) and associated with dry and cool climatic conditions. In light of tipping point theory, this “climate” scenario implies that the dry conditions brought about by the change in regional hydroclimate were able to promote the bog state at the expense of the fen state.
Wind
One last effect that should be discussed is the importance of wind in controlling peatland dynamics in southern Patagonia. Anyone who has seen bog surfaces from this region probably noticed the very tall and often desiccated Sphagnum hummocks that dot these peatlands. Often, the shape and orientation of these hummocks are determined by the main wind direction. While Sphagnum might be able to develop over fen deposits during dry periods, Sphagnum communities also have to persist amid strong directional winds. In many cases, Sphagnum is eventually destroyed by the influence of such strong winds and associated dry conditions, leading to retrogressive bog patches that give way to shrubby landscapes (Auer, 1965). Considering the negative impact of wind on bog development, the ∼ 2700 cal. yr BP increase in effective moisture becomes even more important in maintaining, and perhaps promoting, Sphagnum growth across the region.
A Conceptual Model for the FBT
In light of complex adaptive system theory, a peatland could respond in a step-like way when a slowly varying internal driver (here: peat thickness) exceeds a threshold value, but where the threshold value depends upon the direction of the driver (increase or decrease). In other words, the transition from fen to bog could take place at a different threshold than the transition from bog to fen would, inducing a hysteresis loop that makes the fen and bog possible “alternative states” under a series of conditions (Figure 10). This model considers the resilience and ecological memory of the fen and bog stable states (Belyea, 2009). Allogenic factors can push a mature fen beyond its resilience capacity and induce a shift to bog conditions, and vice versa, but at different thresholds values (Hughes and Dumayne-Peaty, 2002). We postulate that changes in climate (moisture and temperature) and stochastic disturbance events (e.g., drought, flood, volcanic eruption, fire, pollution) become increasingly important over time in facilitating the FBT (i.e., the fen state becomes less stable over time). In other words, the stabilizing forces that were promoting the fen persistence eventually lose to a perturbation that promotes the bog state. The bog is then maintained by another set of stabilizing forces, and a return to the fen state would require different driver conditions than those that promoted the FBT.
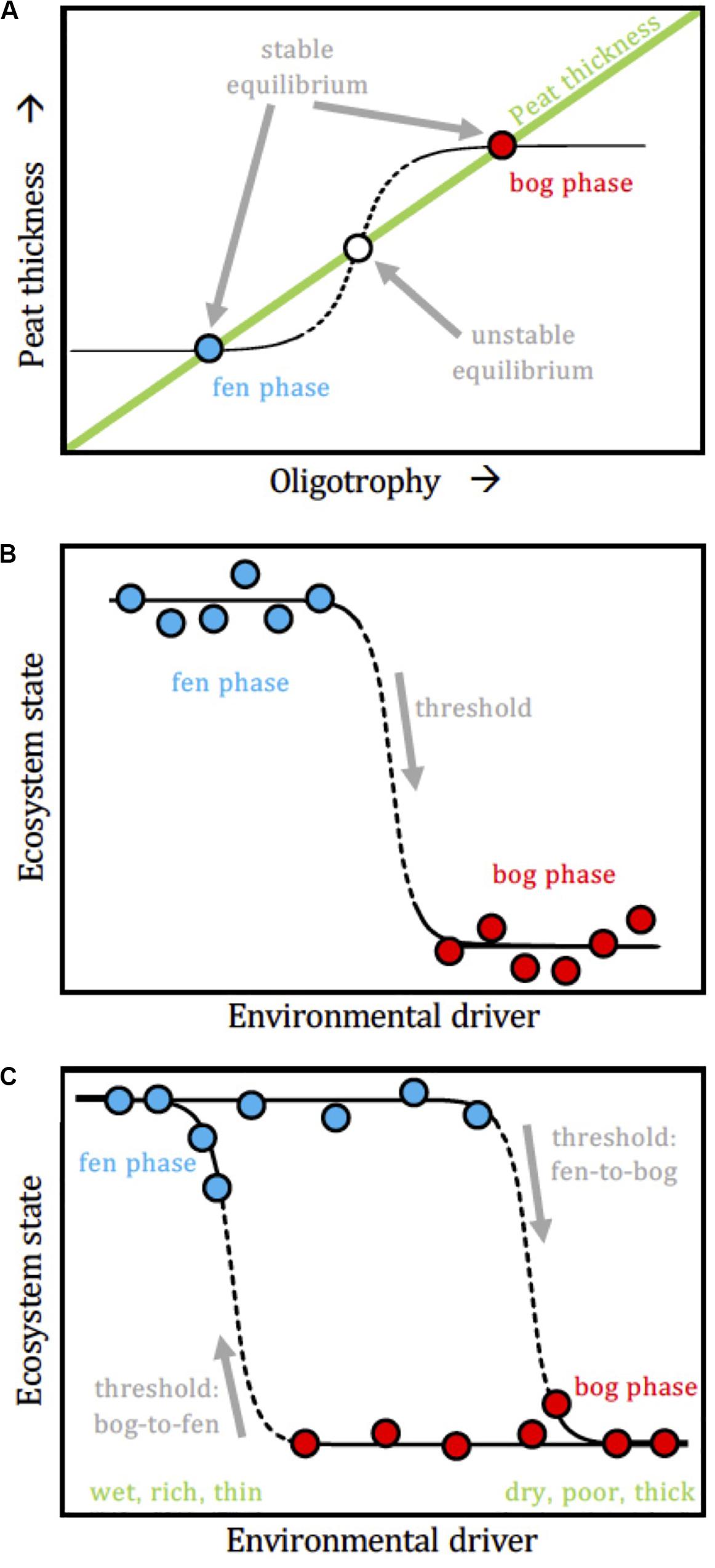
Figure 10. Conceptual models for non-linear fen-bog transition scenarios. (A) Overall pattern, where the peatland state (fen vs. bog) responds non-linearly as oligotrophy increases along with peat thickness. There is no “intermediate” state between fen and bog but rather, once a threshold is passed, the system switches from one state to the other. (B) State-threshold model, where the regime shift in the ecosystem state takes place once a threshold in an internal driver (e.g., peat thickness) is exceeded. (C) Driver-state hysteresis, where a loop links the ecosystem state to the environmental driver, which results in jumps between two alternative stable states.
Bog Stabilizing Properties
The stabilizing properties of bogs occur over multiple spatial scales, from microforms (100–101 m) to mesoforms (101–102 m) and whole ecosystems (102–103 m). These stabilizing forces include a number of negative feedback mechanisms that relate to the presence of Sphagnum, changes in peat water storage, vegetation mosaics, and the rate of peat formation, which are briefly described below.
Presence of Sphagnum
The colonization by Sphagnum helps shape and maintain an acidic, nutrient-poor, and insulated habitat that further promotes Sphagnum persistence (Rydin et al., 2006); it can also absorb pollutants. This genus has a great water-holding capacity and is resistant to decay, making it an excellent peat builder that drives peat formation (van Breemen, 1995). The colonization by Sphagnum is likely associated to a local increase in soil wetness, which may be caused by decreased soil permeability (Rydin and Jeglum, 2013) or the formation of a groundwater mound (Ingram, 1982), both of which can be autogenically- or allogenically-induced.
Peat Water Storage
Following rainfall and snowmelt, excess water can be stored in the acrotelm (the unsaturated uppermost portion of the peat profile), where near-surface peat is pliable and pore spaces are large (Belyea, 2009; Rydin and Jeglum, 2013). By the same token, any superfluous water can be rapidly discharged, thus helping the bog maintain an adequate water supply without exceeding it, which could otherwise change the nature of soil water chemistry. In contrast, during a drought, water table levels drop, but near-surface transmissivity is also reduced, leading to water conservation. This process is accompanied by surface peat contraction, which also limits water loss (Glaser et al., 2004). In addition, mosses can lose their pigments, leading to high albedo and thus increased reflectance that results in slower water loss via evaporation (Gerdol et al., 1996).
Mosaics of Hummocks and Hollows
Vegetation mosaics can increase peat bog resilience by promoting water storage (drought persistence) and by easing drainage (flood persistence). Indeed, flooded hollows can expand to either store or discharge excess water during floods; conversely, hollows can shrink during droughts to limit water loss to evaporation (Quinton and Roulet, 1998; Belyea, 2009). These local water reallocation strategies seem to benefit the system as a whole when compared to a homogenous vegetation cover (Quinton and Roulet, 1998). However, it has been argued that vegetation mosaics can promote perturbations over long timescales. This is particularly the case in continental settings, as they could lead to the expansion of dry hummocks with vascular plants that pump water from the adjacent hollows (Eppinga et al., 2009). In this case, hummocks do not act as drought buffers; rather, they amplify the initial condition, which can lead to self-perpetuating dry conditions.
Rate of Peat Formation
In itself, peat formation plays a self-regulating role in maintaining bog conditions. Vertical peat accretion is a result of the net positive balance between plant production and peat decomposition. Under optimal growth conditions, which have been observed in “lawns” and low hummocks (Belyea and Clymo, 2001), plant production is maximized, leading to vertical peat accumulation that is accompanied by a rising groundwater mound (Belyea and Baird, 2006). If the rate of peat formation was to “outpace” the capacity of the peatland to keep itself wet enough, a hummock would form. Plant production would then be reduced and peat decomposition would increase due to aerobic conditions, thereby constraining vertical peat accretion and bringing the system back to the low hummock/lawn conditions of optimal growth. In this case, the non-linear relationship between the thickness of the acrotelm and the rate of peat formation brings stability to the system (e.g., Belyea and Clymo, 2001; Belyea, 2009). Conversely, if wet years were to occur, the lawns would become wetter, perhaps turning into hollows, which would decrease both the rate of peat formation and the acrotelm thickness, which could tip the system into a self-perpetuating wet state.
System Properties Leading to Fen Persistence
While it can be said that a number of negative feedbacks promote the persistence and homeostasis of the bog state (see above), the situation is different for the fen state. In the literature, it has been suggested that peatlands in general, and bogs in particular, are complex adaptive systems (Belyea and Baird, 2006). But not much has been said about the persistence capabilities of fens. Indeed, fens tend to be topographically constrained, that is, they develop in wet depressions and can “swamp” low slopes as they expand horizontally (e.g., Korhola et al., 2010; Loisel et al., 2013). It has also been suggested that they tend to be more sensitive to climate and hydrological changes then bogs (e.g., Hilbert et al., 2000), perhaps as a result of their incapacity to self-regulate the way bogs do. From this perspective, the stabilizing forces that promote a fen persistence are more difficult to identify. In an attempt to fill this knowledge gap, we propose that lateral expansion and intensified peat decay are potential properties that lead to persistence, albeit being “passive” negative feedbacks, as both these processes contribute to maintaining high surface wetness, particularly near the center of the fen.
Lateral Expansion
Peat accumulation in a fen basin raises the water table locally. Due to an increase in the hydraulic head caused by greater vertical peat accumulation near the center, subsurface water has been shown to flow from the center of the fen toward the margins (Morris et al., 2011), allowing peat to spread laterally through “swamping” of adjacent mineral soils (Belyea, 2009; Korhola et al., 2010; Ruppel et al., 2013). The resulting effect is an increase in the total fen area, which further increases water availability and stability toward the center, thereby maintaining high surface wetness (Loisel et al., 2013). This prevents colonizing Sphagnum species from invading the fen (Granath et al., 2010). By the same token, when a fen reaches a stage where it can no longer spread laterally, its capacity to maintain wet conditions might be lost and perturbations could lead to a regime shift to dry/bog conditions.
Intensified Decay
Despite their high surface wetness, peat decay is intensive in most fens due to highly fluctuating water table levels and nutrient-rich water, which promote microbial diversity and activity, respectively (Vitt, 2006). Intensified aerobic decay has the effect of slowing down net vertical accumulation, which reduces hydraulic conductivity by creating dense peat deposits, further promoting a thin acrotelm and wet surface conditions. In addition, water table depth variability has been found to be most pronounced near the edges of fens (e.g., Loisel et al., 2013), which could lead to intensified peat decay (and thus higher bulk density) near the margins, which then reduces lateral water losses but maintains high surface wetness toward the center of the fen (Morris et al., 2011).
Conclusion
In light of complex adaptive system theory, we propose that allogenic controls can trigger the FBT in peatland systems. The case study presented here indicates that, out of 58 Sphagnum-dominated bogs from southern Patagonia, 35 transitioned from their fen state at 4200 cal. yr BP (Burney II eruption) while another six switched to bogs at 7700 cal. yr BP (Hudson I eruption); the remainder underwent the FBT at other times. Given this striking pattern, it is plausible that the volcanic eruptions facilitated the FBT. Pertaining to the Burney II eruption in particular, which is also synchronous with large-scale climate cooling and drying, it is possible that fens were drying up and that peat accumulation was slowing down due to enhanced decomposition. While the volcanic eruption might have facilitated the FBT, the underlying dry conditions could have been essential to the establishment of bog conditions. In any case, there is strong evidence that those external factors induced the FBT.
In terms of carbon storage, the stratigraphic analyses presented in this study show that, across southern Patagonia, Sphagnum deposits are associated with greater peat masses, larger soil carbon stocks, and higher peat-carbon accumulation rate values than their non-Sphagnum counterparts. The median peat mass for modern-day Sphagnum bogs is over twice that of non-Sphagnum peatlands (141 vs. 56 kgC/m2). Focusing on the peat deposits that have accumulated since Burney II allows us to confirm these long-term trends. Indeed, we find that, on average, bogs stored carbon at a rate almost four times greater than the sites that remained fens or cushion bogs (median = 19 gC/m2/yr for the 58 bogs vs. 5 gC/m2/yr for the other 32 sites). Our dataset demonstrates that the carbon-sink capacity of those peatlands that switched to bogs is much greater than those that did not.
Overall, our paleoecological study demonstrates that fens and bogs across SSA are characterized by distinct soil carbon masses, densities, and accumulation rates. By governing the peatland long-term carbon sequestration, the bog vs. fen status of a peatland can be seen as an ecosystem trait that can be translated into a functional ecosystem property, that is, long-term carbon storage in peatlands; it can thus be used for integrating peatland types into process-based and physics-based ecological models. Additional studies that analyze the timing and driving mechanisms of the FBT, as well as its impacts on carbon sequestration, are needed to provide a broader perspective on this ecosystem transition and to better constrain such models. Additional functional properties such as carbon dioxide and methane gas exchange differences between fens and bogs could similarly be inferred and used to predict spatial and temporal variations in peatland carbon fluxes. This information may be of use for peatland management efforts, in particular, those interested in rehabilitation, restoration, and conservation.
Data Availability Statement
The original contributions presented in the study are included in the article/Supplementary Material, further inquiries can be directed to the corresponding author.
Author Contributions
JL conceived the study and wrote most of the manuscript. MB contributed to data and analyses, helped writing the text, and made the majority of the figures and tables presented in the study. Both authors contributed to the article and approved the submitted version.
Funding
This research and data collection were funded by a Faculty Innovation Grant from the Lehigh University to Z. Yu, a US-NSF Doctoral Dissertation Improvement Grant (DEB-1110665) to JL, an Exploration Grant from the National Geographic Society to JL, Texas A&M University’s College of Geosciences via their High-Impact Learning Experiences Program to JL, and graduate support from Texas A&M University’s Department of Geography to MB.
Conflict of Interest
The authors declare that the research was conducted in the absence of any commercial or financial relationships that could be construed as a potential conflict of interest.
Acknowledgments
The authors acknowledge Kirsten Bevan Rydell, Daniel Brosseau, Patrick Campbell, Julia Hillin, Natalie Jacobs, Eric Nutt, Prof. Zicheng Yu (Lehigh University), Dr. Andy Parsekian (University of Wyoming), and Dr. Clau Mansilla (University of Magallanes, Chile) for their help in the field in 2010 and 2018; Rodrigo Munzenmayer, Alejandro Kusch, Melissa Carmody, and their staff at the Wildlife Conservation Society’s Karukinka Park (Chile) for access to the Park and logistical support (2018); Liliana K. Marusic from Estancia Cerro Negro (Chile) and R. Natalie P. Goodall from Estancia Harberton (Argentina) for access to their properties (2010); Julia Hillin and Greg Sills for their help in the laboratory; Dr. Tom Guilderson for his direction and mentoring with radiocarbon dating at LLNL’s CAMS; Profs. Zicheng Yu (Lehigh University), Robert Booth (Lehigh University), and Paolo D’Odorico (University of Virginia), as well as the C-PEAT community for helpful discussions about the FBT over the years.
Supplementary Material
The Supplementary Material for this article can be found online at: https://www.frontiersin.org/articles/10.3389/fevo.2020.00273/full#supplementary-material
References
Abdalla, M., Hastings, A., Truu, J., Espenberg, M., Mander, Ü, and Smith, P. (2016). Emissions of methane from northern peatlands: a review of management impacts and implications for future management options. Ecol. Evol. 6, 7080–7102. doi: 10.1002/ece3.2469
Andersen, T., Carstensen, J., Hernández-Garcia, E., and Duarte, C. M. (2009). Ecological thresholds and regime shifts: approaches and identification. Trends Ecol. Evol. 24, 49–57. doi: 10.1016/j.tree.2008.07.014
Anyia, M. (2013). Holocene glaciations of Hielo Patagónico (Patagonian Icefield), South America: a brief review. Geochem. J. 47, 98–105. doi: 10.2343/geochemj.1.0171
Arroyo, M. T. K., Mihoc, M., Pliscoff, P., and Arroyo-Kalin, M. (2005). “The Magellanic moorland,” in The World’s Largest Wetlands: Ecology and Conservation, eds L. H. Fraser and P. A. Keddy (Cambridge, MA: Cambridge University Press), 424–445. doi: 10.1017/cbo9780511542091.013
Auer, V. (1958). The Pleistocene of Fuego- Patagonia. Part II: The history of the flora and vegetation, Series A III, Geologica-Geographica 50. Helsinki: Anales Academiae Scientiarum Fennicea.
Auer, V. (1965). The Pleistocene of Fuego- Patagonia, Part IV: Bog profiles, Series A III, Geologica-Geographica 80. Helsinki: Anales Academiae Scientiarum Fennicea.
Baird, A. J., Belyea, L. R., and Morris, P. J. (2009). “Upscaling of peatland-atmosphere fluxes of methane: small-scale heterogeneity in process rates,” in Northern Peatlands and Carbon Cycling, eds A. J. Baird, L. R. Belyea, X. Comas, A.S. Reeve, and D. Lee (Washington, DC: American Geophysical Union), 37–53. doi: 10.1029/2008gm000826
Belyea, L. R. (2009). “Non-linear dynamics of peatlands and potential feedbacks on the climate system,” in Northern Peatlands and Carbon Cycling, eds A. Baird, L. Belyea, X. Comas, A. Reeve, and L. Slater (Washington, DC: American Geophysical Union Monograph Series), 5–18. doi: 10.1029/2008gm000829
Belyea, L. R., and Baird, A. J. (2006). Beyond “The limits to peat bog growth”: cross-scale feedback in peatland development. Ecol. Monogr. 76, 299–322. doi: 10.1890/0012-9615(2006)076[0299:btltpb]2.0.co;2
Belyea, L. R., and Clymo, R. S. (2001). Feedback control of the rate of peat formation. Proc. Natl. Soc. Lond. B 268, 1315–1321. doi: 10.1098/rspb.2001.1665
Bentley, M. J., and McCulloch, R. (2005). Impact of neotectonics on the record of glacier and sea level fluctuations, Strait of Magellan, southern Chile. Geografiska Ann. Ser. A Phys. Geogr. 87, 393–402. doi: 10.1111/j.0435-3676.2005.00265.x
Blaauw, M., and Christen, J. A. (2011). Flexible paleoclimate age-depth models using an autoregressive gamma process. Bayesian Anal. 6, 457–474. doi: 10.1214/ba/1339616472
Blodau, C. (2002). Carbon cycling in peatlands: a review of processes and controls. Environ. Rev. 10, 111–134. doi: 10.1139/a02-004
Bond, G., Kromer, B., Beer, J., Muscheler, R., Evans, M. N., Showers, W., et al. (2001). Persistent solar influence on North Atlantic climate during the Holocene. Science 294, 2130–2136. doi: 10.1126/science.1065680
Bubier, J. L. (1995). The relationship of vegetation to methane emission and hydrochemical gradients in northern peatlands. J. Ecol. 83, 403–420.
Bunsen, M. S. (2020). Peatland Carbon Storage in Patagonia: From Long-Term Legacies to Short-Term Dynamics. M.Sc. thesis, Department of Geography, Texas A&M University, College Station, TX.
Bunsen, M. S., and Loisel, J. (2020). Carbon storage dynamics in peatlands: comparing recent and long-term accumulation histories in Southern Patagonia. Glob. Change Biol. doi: 10.1111/gcb.15262
CrossRef Full Text [Epub ahed of print].PubMed Abstract | Google Scholar
Bunting, M. J., and Warner, B. G. (1998). Hydroseral development in southern Ontario: patterns and controls. J. Biogeogr. 25, 3–18. doi: 10.1046/j.1365-2699.1998.251195.x
Camill, P., and Clark, J. S. (2000). Long-term perspectives on lagged ecosystem responses to climate change: permafrost in boreal peatlands and the grassland/woodland boundary. Ecosystems 3, 534–544. doi: 10.1007/s100210000047
Coronato, A., Roig, C., Collado, L., and Roig, F. (2006). “Geomorphologic emplacement and vegetation characteristics of Fuegian peatlands, southernmost Argentina, South America,” in Peatlands: Evolution and Records of Environmental and Climate Changes, eds I. P. Martini, A. Martinez Cortizas, and W. Chesworth (Amsterdam: Elsevier), 111–128. doi: 10.1016/s0928-2025(06)09005-5
Damman, A. W. H. (1986). Hydrology, development, and biogeochemistry of ombrogenous peat bogs with special reference to nutrient relocation in a western Newfoundland bog. Can. J. Bot. 64, 384–394. doi: 10.1139/b86-055
De Vleeschouwer, F., Vanneste, H., Mauquoy, D., Piotrowska, N., Torrejo, F., Roland, T., et al. (2014). Emissions from pre-Hispanic metallurgy in the South American atmosphere. PLoS One 9:e111315. doi: 10.1371/journal.pone.0111315
Dean, W. E. Jr. (1974). Determination of carbonate and organic matter in calcareous sediments and sedimentary rocks by loss on ignition: comparison with other methods. J. Sediment. Petrol. 44, 242–248.
Eppinga, M. B., de Ruiter, P. C., Wassen, M. J., and Rietkerk, M. (2009). Nutrients and hydrology indicate the driving mechanisms of peatland surface patterning. Am. Nat. 173, 803–818. doi: 10.1086/598487
Fesq-Martin, M., Friedmann, A., Peters, M., Behrmann, J., and Kilian, R. (2004). Late-glacial and Holocene vegetation history of the Magellanic rain forest in southwestern Patagonia, Chile. Veg. History Archaeobot. 13, 249–255. doi: 10.1007/s00334-004-0047-6
Frolking, S., and Roulet, N. T. (2007). Holocene radiative forcing impact of northern peatland carbon accumulation and methane emissions. Glob. Change Biol. 13, 1–10.
Frolking, S., Roulet, N. T., and Lawrence, D. (2009). “Issues related to incorporating northern peatlands into global climate models,” in Northern Peatlands and Carbon Cycling, Geophysical Monograph 184, eds A. Baird, L. Belyea, X. Comas, A. Reeve, and L. Slater (Washington DC: American Geophysical Union), 19–35. doi: 10.1029/2008gm000809
Frolking, S., Roulet, N. T., Moore, T. R., Richard, P. J. H., Lavoie, M., and Muller, S. D. (2001). Modeling northern peatland decomposition and peat accumulation. Ecosystems 4, 479–498. doi: 10.1007/s10021-001-0105-1
Frolking, S., Roulet, N. T., Tuittila, E., Bubier, J. L., Quillet, A., Talbot, J., et al. (2010). A new model of Holocene peatland net primary production, decomposition, water balance, and peat accumulation. Earth Syst. Dyn. 1, 1–21. doi: 10.5194/esd-1-1-2010
Gerdol, R., Bonora, A., Gualandri, R., and Pancaldi, S. (1996). CO2 exchange, photosynthetic pigment composition, and cell ultrastructure of Sphagnum mosses during dehydration and subsequent rehydration. Can. J. Bot. 74, 726–734. doi: 10.1139/b96-091
Glaser, P. H. (1992). Raised bogs in eastern North America–regional controls for species richness and floristic assemblages. J. Ecol. 1, 535–554.
Glaser, P. H., Chanton, J. P., Morin, P., Rosenberry, D. O., Siegel, D. I., Ruud, O., et al. (2004). Surface deformations as indicators of deep ebullition fluxes in a large northern peatland. Glob. Biogeochem. Cycles 18:GB1003. doi: 10.1029/2003GB002069
Gorham, E., Bayley, S. E., and Schindler, D. W. (1984). Ecological effects of acid rain deposition upon peatlands: a neglected field in ‘acid-rain’ research. Can. J. Fish. Aquat. Sci. 41, 1256–1268. doi: 10.1139/f84-152
Granath, G., Strengbom, J., and Rydin, H. (2010). Rapid ecosystem shifts in peatlands: linking plant physiology and succession. Ecology 91, 3047–3056. doi: 10.1890/09-2267.1
Grootjans, A., Iturraspe, R., Lanting, A., Fritz, C., and Joosten, H. (2010). Ecohydrological features of some contrasting mires in Tierra del Fuego, Argentina. Mires Peat. 5, 1–14.
Heusser, C. J. (1989). Late Quaternary vegetation and climate of southern Tierra del Fuego. Quatern. Res. 31, 396–406. doi: 10.1016/0033-5894(89)90047-1
Heusser, C. J. (1993). Late Quaternary forest-steppe contact zone, Isla Grande de Tierra del Fuego, subantarctic South America. Quatern. Sci. Rev. 12, 169–177. doi: 10.1016/0277-3791(93)90051-m
Heusser, C. J. (1998). Deglacial paleoclimate of the American sector of the Southern Ocean: late Glacial–Holocene records from the latitude of Canal Beagle (55 S), Argentine Tierra del Fuego. Palaeogeogr. Palaeoclimatol. Palaeoecol. 141, 277–301. doi: 10.1016/s0031-0182(98)00053-4
Hilbert, D. W., Roulet, N. T., and Moore, T. R. (2000). Modelling and analysis of peatlands as dynamical systems. J. Ecol. 88, 230–242. doi: 10.1046/j.1365-2745.2000.00438.x
Hogg, A., Turney, C., Palmer, J., Cook, E., and Buckley, B. (2013). Is there any evidence for regional atmospheric 14C offsets in the southern hemisphere? Radiocarbon 55, 2029–2034. doi: 10.2458/azu_js_rc.v55i2.16104
Hua, Q., and Barbetti, M. (2004). Review of tropospheric bomb 14C data for carbon cycle modeling and age calibration purposes. Radiocarbon 46, 1273–1298. doi: 10.1017/s0033822200033142
Huber, U. M. (2003). Linkages Among Climate, Vegetation and Fire in Fuego-Patagonia during the Late-Glacial and Holocene. PhD dissertation, University of Colorado, Boulder, CO.
Hugelius, G., Loisel, J., Chadburn, S., Jackson, R., MacDonald, G., Marushchak, M., et al. (in press). Northern peatlands and permafrost thaw: effects on carbon and nitrogen stocks and fluxes. Proc. Natl. Acad. Sci. U.S.A.
Hughes, P. D. M. (2000). A reappraisal of the mechanisms leading to ombrotrophy in British raised mires. Ecol. Lett. 3, 7–9. doi: 10.1046/j.1461-0248.2000.00118.x
Hughes, P. D. M., and Barber, K. E. (2003). Mire development across the fen-bog transition on the Teifi foodplain at Tuegaron Bog, Cenedigion, Wales, and a comparison with 13 other raised bogs. J. Ecol. 91, 253–264. doi: 10.1046/j.1365-2745.2003.00762.x
Hughes, P. D. M., and Barber, K. E. (2004). Contrasting pathways to ombrotrophy in three raised bogs from Ireland and Cumbria, England. Holocene 14, 65–77. doi: 10.1191/0959683604hl690rp
Hughes, P. D. M., and Dumayne-Peaty, L. (2002). Testing theories of mire development using multiple successions at Crymlyn Bog, West Glamorgan, South Wales, UK. J. Ecol. 90, 456–471. doi: 10.1046/j.1365-2745.2002.00677.x
Ingram, H. A. P. (1982). Size and shape in raised mire ecosystems: a geophysical model. Nature 297, 300–303. doi: 10.1038/297300a0
Kaplan, M., Schaefer, J., Strelin, J. A., Denton, G., Anderson, R., Vandergoes, M., et al. (2016). Patagonian and southern South Atlantic view of Holocene climate. Quatern. Sci. Rev. 141, 112–125. doi: 10.1016/j.quascirev.2016.03.014
Kilian, R., Biester, H., Behrmann, J., Baeza, O., Fesq-Martin, M., Hohner, M., et al. (2006). Millennium-scale volcanic impact on a superhumid and pristine ecosystem. Geology 34, 609–612.
Korhola, A., Ruppel, M., Seppa, H., Valiranta, M., Virtanen, T., and Weckstrom, J. (2010). The importance of northern peatland expansion to the late-Holocene rise of atmospheric methane. Quatern. Sci. Rev. 29, 611–617. doi: 10.1016/j.quascirev.2009.12.010
Kuhry, P., Nicholson, B. J., Gignac, L. D., Vitt, D. H., and Bayley, S. E. (1993). Development of Sphagnum-dominated peatlands in boreal continental Canada. Can. J. Bot. 71, 10–22. doi: 10.1139/b93-002
Laine, J., and Vasander, H. (1996). “Ecology and vegetation gradients of peatlands,” in Peatlands in Finland, ed. H. Vasander (Finland: Finnish Peatland Society).
Lenton, T. M. (2013). Environmental tipping points. Annu. Rev. Environ. Resour. 38, 1–29. doi: 10.1146/annurev-environ-102511-084654
Levin, S. A. (1998). Ecosystems and the biosphere as complex adaptive systems. Ecosystems 1, 431–436. doi: 10.1007/s100219900037
Loisel, J. (2015). “Peatlands as carbon sinks / Las turberas como sumideros de carbono,” in Funciones y Servicios Ecosistémicos de las Turberas en Magallanes, INIA N° 33, eds E. Domínguez and D. Vega-Valdés (Chile: Punta Arenas), 297–315.
Loisel, J., MacDonald, G., Kremenetski, K., and Holmquist, J. (2015). Timing of Fen-Bog Transition Across the Northern Peatland Domain. Nagoya: INQUA Quadrennial Congress.
Loisel, J., van Bellen, S., Pelletier, L., Talbot, J., Hugelius, G., Karran, D., et al. (2017a). Insights and issues with estimating northern peatland carbon stocks and fluxes since the Last Glacial Maximum. Earth Sci. Rev. 165, 59–80. doi: 10.1016/j.earscirev.2016.12.001
Loisel, J., Yu, Z., Beilman, D. W., Kaiser, K., and Parnikoza, I. (2017b). Past and present peatland development in Antarctica under warm climates. Sci. Rep. 7:12344. doi: 10.1038/s41598-017-12479-0
Loisel, J., and Yu, Z. (2013a). Holocene peatland dynamics in Patagonia. Quatern. Sci. Rev. 69, 125–141. doi: 10.1016/j.quascirev.2013.02.023
Loisel, J., and Yu, Z. (2013b). Surface vegetation patterning controls carbon accumulation in peatlands. Geophys. Res. Lett. 40, 5508–5513. doi: 10.1002/grl.50744
Loisel, J., Yu, Z., and D’Odorico, P. (2012). “Peatland dynamics in Patagonia: abrupt mid-Holocene fen-to-bog transition and carbon sequestration implications,” in Proceedings, 14th International Peat Congress, Stockholm.
Loisel, J., Yu, Z., Parsekian, A., Nolan, J., and Slater, L. (2013). Quantifying landscape morphology influence on peatland lateral expansion using ground penetrating radar (GPR) and peat core analysis. J. Geophys. Res. Biogeosci. 118, 373–384. doi: 10.1002/jgrg.20029
MacDonald, G. M., Beilman, D. W., Kremenetski, K. V., Sheng, Y., Smith, L., and Valichko, A. A. (2006). Rapid early development of circum-arctic peatlands and atmospheric CH4 and CO2 variations. Science 314, 285–288. doi: 10.1126/science.1131722
Malmer, N. (1986). Vegetational gradients in relation to environmental conditions in northwestern European mires. Can. J. Bot. 2, 375–383. doi: 10.1139/b86-054
Mansilla, C., McCulloch, R. D., and Morello, F. (2016). “Extending the tephrochronology of fuego-patagonia, Southern South America (~53°s),” in Proceedings of the VII Southern Connections at Punta Arenas, Punta Arenas.
Markgraf, V., and Huber, U. M. (2010). Late and postglacial vegetation and fire history in Southern Patagonia and Tierra del Fuego. Palaeogeogr. Palaeoclimatol. Palaeoecol. 297, 351–366. doi: 10.1016/j.palaeo.2010.08.013
Mathijssen, P. J., Gałka, M., Borken, W., and Knorr, K. H. (2019). Plant communities control long term carbon accumulation and biogeochemical gradients in a Patagonian bog. Sci. Tot. Environ. 684, 670–681. doi: 10.1016/j.scitotenv.2019.05.310
Milner, A., Baird, A., Green, S., Swindles, G., Young, D., and Sanderson, N., et al. (in press). A regime shift from erosion to carbon accumulation in a temperate northern peatland. J. Ecol. doi: 10.1111/1365?2745.13453
Moore, T. R., and Roulet, N. T. (1993). Methane flux: water table position relations in northern peatlands. Geophys. Res. Lett. 20, 587–590. doi: 10.1029/93gl00208
Moreno, P., Vilanova, I., Villa-Martinez, R., Dunbar, R., Mucciarone, D., Kaplan, M., et al. (2018). Onset and evolution of southern annular mode-like changes at centennial timescale. Sci. Rep. 8, 1–9.
Moreno, P. I., Francois, J.-P., Moy, C., and Villa-Martinez, R. (2010). Covariability of the Southern Westerlies and atmospheric CO2 during the Holocene. Geology 38, 727–730. doi: 10.1130/g30962.1
Morris, P. J., Belyea, L. R., and Baird, A. J. (2011). Ecohydrological feedbacks in peatland development: a theoretical modelling study. J. Ecol. 99, 1190–1201. doi: 10.1111/j.1365-2745.2011.01842.x
Mulvaney, R., Abram, N. J., Hindmarsh, R. C., Arrowsmith, C., Fleet, L., and Triest, J. (2012). Recent Antarctic peninsula warming relative to Holocene climate and ice-shelf history. Nature 489, 141–144. doi: 10.1038/nature11391
New, M., Lister, D., Hulme, M., and Makin, I. (2002). A high-resolution data set of surface climate over global land areas. Clim. Res. 21, 1–25. doi: 10.3354/cr021001
Oppedal, L. T., Bakke, J., Paasche, Ø, Werner, J. P., and van der Bilt, W. G. M. (2018). Cirque glacier on South Georgia shows centennial variability over the last 7000 years. Front. Earth Sci. 6:2. doi: 10.3389/feart.2018.00002
Paruelo, J. M., Beltrán, A., Jobbágy, E. G., Sala, O. E., and Golluscio, R. A. (1998). The climate of Patagonia: general patterns and controls on biotic processes. Ecol. Austral. 8, 85–101.
Parviainen, M., and Luoto, M. (2007). Climate envelopes of mire complex types in Fennoscandia. Geografiska Ann. Ser. A Phys. Geogr. 89, 137–151. doi: 10.1111/j.1468-0459.2007.00314.x
Pendall, E., Markgraf, V., White, J. W., Dreier, M., and Kenny, R. (2001). Multiproxy record of late Pleistocene–Holocene climate and vegetation changes from a peat bog in Patagonia. Quatern. Res. 55, 168–178. doi: 10.1006/qres.2000.2206
Pisano, E. (1983). “The Magellanic tundra complex,” in Mires: Swamp, Bog, Fen and Moor, Vol. 2, ed. A. J. P. Gore (Amsterdam: Elsevier), 295–329.
Quinton, W. L., and Roulet, N. T. (1998). Spring and summer runoff hydrology of a subarctic patterned wetland. Arctic Alpine Res. 30, 285–294.
Rabassa, J., Coronato, A., Heusser, C., Junent, F. R., Borromei, A., Roig, C., et al. (2006). The peatlands of Argentine Tierra del Fuego as a source for paleoclimatic and paleoenvironmental information. Dev. Earth Surface Process. 9, 129–144. doi: 10.1016/s0928-2025(06)09006-7
Reimer, P. J., Bard, E., Bayliss, A., Beck, J. W., Blackwell, P. G., Ramsey, C. B., et al. (2013). IntCal13 and Marine13 radiocarbon age calibration curves 0–50,000 years cal BP. Radiocarbon 55, 1869–1887. doi: 10.2458/azu_js_rc.55.16947
Ronkainen, T., McClymont, E. L., Tuittila, E. S., and Väliranta, M. (2014). Plant macrofossil and biomarker evidence of fen–bog transition and associated changes in vegetation in two Finnish peatlands. Holocene 24, 828–841. doi: 10.1177/0959683614530442
Ruppel, M., Väliranta, M., Virtanen, T., and Korhola, A. (2013). Postglacial spatiotemporal peatland initiation and lateral expansion dynamics in North America and northern Europe. Holocene 23, 1596–1606. doi: 10.1177/0959683613499053
Rydin, H., Gunnarsson, U., and Sundberg, S. (2006). The role of Sphagnum in peatland development and persistence. Boreal Peatland Ecosyst. Ecol. Stud. 188, 47–65. doi: 10.1007/978-3-540-31913-9_4
Rydin, H., and Jeglum, J. (eds) (2013). The Biology. (of)Peatlands. Oxford: Oxford University Press.
Scheffer, M., Carpenter, S., Foley, J. A., Folke, C., and Walker, B. (2001). Catastrophic shifts in ecosystems. Nature 413, 591–596. doi: 10.1038/35098000
Scheffer, M., and Carpenter, S. R. (2003). Catastrophic regime shifts in ecosystems: linking theory to observation. Trends Ecol. Evol. 18, 648–656. doi: 10.1016/j.tree.2003.09.002
Schneider, C., Glaser, M., Kilian, R., Santana, A., Butorovic, N., and Casassa, G. (2003). Weather observations across the Southern Andes at 53°S. Phys. Geogr. 24, 97–119. doi: 10.2747/0272-3646.24.2.97
Segers, R. (1998). Methane production and methane consumption: a review of processes underlying wetland methane fluxes. Biogeochemistry 41, 23–51.
Sterken, M., Roberts, S., Hodgson, D. A., Vyverman, W., Balbo, A. L., Sabbe, K., et al. (2012). Holocene glacial and climate history of Prince Gustav Channel, northeastern Antarctic Peninsula. Quatern. Sci. Rev. 31, 93–111. doi: 10.1016/j.quascirev.2011.10.017
Stern, C. R. (2008). Holocene tephrochronology record of large explosive eruptions in the southernmost Patagonian Andes. Bull. Volcanol. 70, 435–454. doi: 10.1007/s00445-007-0148-z
Strelin, J. A., Kaplan, M. R., Vandergoes, M. J., Denton, G. H., and Schaefer, J. M. (2014). Holocene glacier history of the Lago Argentino basin, southern Patagonian Icefield. Quatern. Sci. Rev. 101, 124–145. doi: 10.1016/j.quascirev.2014.06.026
Tarnocai, C., Kettles, I. M., and Lacelle, B. (2002). Peatlands of Canada Database. Geological Survey of Canada, open file report 3834. Montreal: McGill University.
Treat, C. C., Jones, M. C., Camill, P., Garneau, M., Gallego-Sala, A., Harden, J. W., et al. (2015). Effects of permafrost aggradation on peat properties as determined from a pan-arctic synthesis of plant macrofossils. J. Geophys. Res. Biogeosci. 121, 78–94. doi: 10.1002/2015JG003061
Turetsky, M. R., Kotowska, A., Bubier, J. L., Dise, N. B., Crill, P., Hornibrook, E. R. C., et al. (2014). A synthesis of methane emissions from 71 northern, temperate, and subtropical wetlands. Glob. Change Biol. 20, 2183–2197. doi: 10.1111/gcb.12580
Väliranta, M., Salojärvi, N., Vuorsalo, A., Juutinen, S., Korhola, A., Luoto, M., et al. (2017). Holocene fen–bog transitions, current status in Finland and future perspectives. Holocene 27, 752–764. doi: 10.1177/0959683616670471
van Breemen, N. (1995). How Sphagnum bogs down other plants. Trends Ecol. Evol. 10, 270–275. doi: 10.1016/0169-5347(95)90007-1
Vitt, D. H. (2006). Functional characteristics and indicators of boreal peatlands. Boreal Peatland Ecosyst. Ecol. Stud. 188, 9–24. doi: 10.1007/978-3-540-31913-9_2
Vitt, D. H., Halsey, L. A., Bray, J., and Kinser, A. (2003). Patterns of bryophyte richness in a complex boreal landscape: identifying key habitats at McClelland Lake wetland. Bryologist 106, 372–382. doi: 10.1639/03
Waddington, J. M., Roulet, N. T., and Swanson, R. V. (1996). Water table control of CH4 emission enhancement by vascular plants in boreal peatlands. J. Geophys. Res. 101, 775–785.
Walker, D. (1970). “Direction and rate in some British postglacial hydroseres,” in Studies in the Vegetational History of the British Isles, eds D. Walker and R. G. West (Cambridge, MA: Cambridge University Press), 117–139.
Wanner, H., Beer, J., Bütikofer, J., Crowley, T. J., Cubasch, U., Flückiger, J., et al. (2008). Mid-to Late Holocene climate change: an overview. Quatern. Sci. Rev. 27, 1791–1828.
Whiting, G. J., and Chanton, J. P. (2001). Greenhouse carbon balance of wetlands: methane emission versus carbon sequestration. Tellus 53B, 521–528. doi: 10.1034/j.1600-0889.2001.530501.x
Xia, Z., Yu, Z., and Loisel, J. (2018). Centennial-scale dynamics of the Southern Hemisphere Westerly Winds across the Drake Passage over the past two millennia. Geology 46, 855–858. doi: 10.1130/g40187.1
Xia, Z., Zheng, Y., Stelling, J., Loisel, J., Huang, Y., and Yu, Z. (2020). Understanding environmental controls on the carbon and water isotopes in peatland Sphagnum mosses. Geochim. Cosmochim. Acta 277, 265–284. doi: 10.1016/j.gca.2020.03.034
Xu, J., Morris, P. J., Liu, J., and Holden, J. (2018). PEATMAP: refining estimates of global peatland distribution based on a meta-analysis. Catena 160, 134–140. doi: 10.1016/j.catena.2017.09.010
Yu, Z. (2011). Holocene carbon flux histories of the world’s peatlands: global carbon-cycle implications. Holocene 21, 761–774. doi: 10.1177/0959683610386982
Yu, Z., Beilman, D. W., and Jones, M. C. (2009). “Sensitivity of northern peatland carbon dynamics to Holocene climate change,” in Northern Peatlands and Carbon Cycling, eds A. Baird, L. Belyea, X. Comas, A. Reeve, and L. Slater (Washington, DC: American Geophysical Union Monograph Series), 55–69. doi: 10.1029/2008gm000822
Yu, Z., Beilman, D. W., and Loisel, J. (2016). Transformations of landscape and peat-forming ecosystems in response to late Holocene climate change in the western Antarctic Peninsula. Geophys. Res. Lett. 43, 7186–7195. doi: 10.1002/2016GL069380
Yu, Z., Loisel, J., Brosseau, D. P., Beilman, D. W., and Hunt, S. J. (2010). Global peatland dynamics since the Last Glacial Maximum. Geophys. Res. Lett. 37:L13402.
Yu, Z., Loisel, J., Turetsky, M. R., Cai, S., Zhao, Y., Frolking, S., et al. (2013). Evidence for elevated emissions from high-latitude wetlands contributing to high atmospheric CH4 concentration in the early Holocene. Glob. Biogeochem. Cycles 27, 131–140. doi: 10.1002/gbc.20025
Zobel, M. (1988). Autogenic succession in boreal mires – a review. Folia Geobotanica Phytotaxonomica 28, 417–445. doi: 10.1007/bf02853361
Zoltai, S. C. (1993). Cyclic development of permafrost in the peatlands of northwestern Alberta, Canada. Arctic Alpine Res. 25, 240–246.
Keywords: carbon sink function, Holocene, Mires, tipping point, regime shift, complex adaptive system, Paleoecology
Citation: Loisel J and Bunsen M (2020) Abrupt Fen-Bog Transition Across Southern Patagonia: Timing, Causes, and Impacts on Carbon Sequestration. Front. Ecol. Evol. 8:273. doi: 10.3389/fevo.2020.00273
Received: 23 April 2020; Accepted: 31 July 2020;
Published: 18 August 2020.
Edited by:
Alistair William Robin Seddon, University of Bergen, NorwayReviewed by:
Paul Mathijssen, University of Münster, GermanyXu Chen, China University of Geosciences, China
Copyright © 2020 Loisel and Bunsen. This is an open-access article distributed under the terms of the Creative Commons Attribution License (CC BY). The use, distribution or reproduction in other forums is permitted, provided the original author(s) and the copyright owner(s) are credited and that the original publication in this journal is cited, in accordance with accepted academic practice. No use, distribution or reproduction is permitted which does not comply with these terms.
*Correspondence: Julie Loisel, anVsaWVsb2lzZWxAdGFtdS5lZHU=