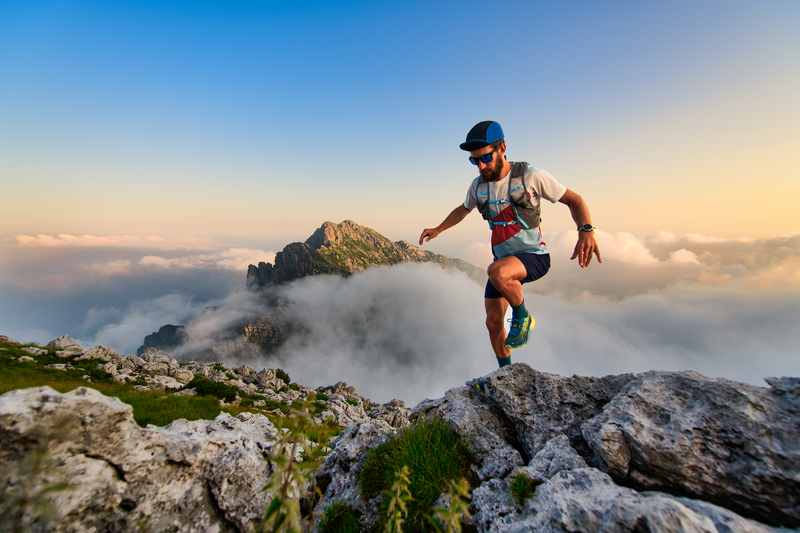
95% of researchers rate our articles as excellent or good
Learn more about the work of our research integrity team to safeguard the quality of each article we publish.
Find out more
MINI REVIEW article
Front. Ecol. Evol. , 18 August 2020
Sec. Evolutionary and Population Genetics
Volume 8 - 2020 | https://doi.org/10.3389/fevo.2020.00271
This article is part of the Research Topic Coping with Climate Change: A Genomic Perspective on Thermal Adaptation View all 11 articles
Phenotypic plasticity, the property by which living organisms express different phenotypes depending on environmental conditions, can impact their response to environmental perturbation, including that resulting from climate change. When exposed to altered environmental conditions, phenotypic plasticity might help or might hinder both immediate survival and future adaptation. Because climate change will cause more than a global rise in mean temperatures, it is valuable to consider the combined effects of temperature and other environmental variables on trait expression (thermal plasticity), as well as trait evolution (thermal adaptation). In this review, we focus primarily on thermal developmental plasticity in insects. We discuss the genomics of thermal plasticity and its relationship to thermal adaptation and thermal tolerance, and to climate change and multifactorial environments.
The ability of natural populations to react to environmental change will depend on the level and type of perturbation organisms experience, and also on their intrinsic capability to respond to it (Parmesan, 2006; Johnston et al., 2019). Phenotypic plasticity, the property by which living organisms express different phenotypes depending on environmental conditions, can impact their response to environmental perturbation, including that resulting from global climate change (Reed et al., 2011; Chevin et al., 2013; Merilä and Hendry, 2014; Sgrò et al., 2016; Bonamour et al., 2019). Considering thermal plasticity, in addition to thermal tolerance and thermal adaptation, will be crucial to assessing how organisms might cope with climate change. And because climate change is not only about increasing mean ambient temperature, it is also clear that it is important to consider effects of multifactorial environments, combining temperature with other environmental variables, both on trait expression and on trait evolution (Kaunisto et al., 2016; Westneat et al., 2019).
Here, we focus on effects of temperature and its combination with other environmental factors on phenotypic plasticity in terrestrial/flying insects, a taxon of ectothermal animals that includes many compelling examples of thermal plasticity. This is a large and ecologically central group of organisms whose geographic ranges, behaviors, and life histories are very much affected by ambient temperature (Colinet et al., 2015). It is also a group with recent worrying trends: steep global population declines (Sánchez-Bayo and Wyckhuys, 2019; Didham et al., 2020; Wagner, 2020), as well as expansions of agricultural pests and disease vectors (Song et al., 2017; Ryan et al., 2019). We direct our attention primarily toward recent examples, and to studies focused on the genomics of thermal plasticity of potential relevance to responses to climate change.
Phenotypic diversity, within and across species, is shaped by interactions between organisms and their environment, which occur at different levels and different time scales. Environmental conditions determine cross-generation changes in phenotype frequencies in populations (notably through natural selection), and affect intra-generation phenotype expression of individuals (via phenotypic plasticity). In this section, we will focus on examples of phenotypic plasticity and how it can evolve and impact adaptive evolution, including in the context of climate change.
The effect of external environmental conditions on phenotype expression can happen at distinct time-scales: (1) change in progeny phenotype that depends on parental environment (trans-generational plasticity; Woestmann and Saastamoinen, 2016; Donelson et al., 2018), (2) change in adult phenotype in response to adult environment, often reversible changes in labile traits, such as behavior, and including what is called acclimation (Stillman, 2003; Sgrò et al., 2016), and (3) change in phenotype that depends on the conditions experienced during development, often leading to irreversible adult phenotypes (the main focus of this review). Indeed, the environmental conditions experienced during development can alter developmental rates and/or trajectories and result in the production of different adult phenotypes from the same genotype, in a phenomenon called developmental plasticity (reviewed in Beldade et al., 2011; Nettle and Bateson, 2015). The study of developmental plasticity, which integrates ecology, evolutionary biology, and developmental biology (eco-evo-devo, Gilbert et al., 2015), is key to understanding how organisms interact with their changing environments.
Plasticity can match organismal phenotypes to their ecological conditions and, as such, be favored by natural selection (Nettle and Bateson, 2015). Plasticity is thought to benefit populations that face distinct challenges imposed by environmental heterogeneity (e.g., Chevin et al., 2010), such as that resulting from alternating seasons (Buckley et al., 2017). Seasonal polyphenism, where alternative seasonal conditions lead to the production of distinct seasonal phenotypes, is common in insects (Nijhout, 2003; Moczek, 2010; Simpson et al., 2011; Yang and Pospisilik, 2019). Their relatively short life cycles allow for multiple generations within the year and, consequently, exposure to conditions that can differ substantially between generations. Seasonally variable environmental factors, often temperature, can induce changes in sets of integrated traits associated to distinct strategies for survival and/or reproduction, suited to the respective seasonal conditions. For example, in the butterfly Bicyclus anynana, the temperature during development anticipates upcoming seasonal conditions in vegetation cover, and induces changes in various adult traits associated with distinct seasonal strategies for predator avoidance and pace-of-life (Box 1).
BOX 1. Seasonal polyphenism in B. anynana.
Development under cooler temperatures leads to the production of dry-season form adults, while development under warmer temperatures leads to wet-season form adults. The seasons differ in vegetation cover and the alternative seasonal forms differ in their strategies for reproduction (solid line box on right side) and for escaping predators (dashed line box). B. anynana drawings by Joana Carvalho (joana_gcc).
A case study of thermal plasticity: Bicyclus anynana butterflies.
The afro-tropical butterfly Bicyclus anynana has become a valuable model of seasonal polyphenism, where an understanding of the ecological significance of alternative seasonal phenotypes can be integrated with knowledge about the developmental basis and evolution of thermal plasticity (Brakefield et al., 2009; Beldade and Peralta, 2017). B. anynana seasonal plasticity is believed to be an adaptation to the strongly contrasting wet versus dry seasons of its African savannah habitat. The temperature experienced during development, which anticipates the upcoming season and conditions adults will have to live in, results in adult phenotypes adjusted to each of the seasons’ conditions (Figure).
Developmental temperature affects a suite of traits, including wing pigmentation (e.g., Brakefield, 1996; Mateus et al., 2014; Wasik et al., 2014), life histories (e.g., Pijpe et al., 2006; Fischer et al., 2007; Oostra et al., 2011, 2014), secondary sexual traits (e.g., Muller et al., 2019), and various behavioral traits (e.g., Prudic et al., 2011; Bear and Monteiro, 2013; Westerman and Monteiro, 2016; van Bergen and Beldade, 2019). Alternative phenotypes correspond to distinct seasonal strategies for predator avoidance and for reproduction, associated to the distinct seasonal status of the habitat’s vegetation cover on which adults perch and larvae feed. Dry-season form adults have dull brown wings, which are cryptic against the background of dry foliage, and have increased body reserves, which sustain longer lifespan and the delay reproduction until the end of the season (Brakefield and Reitsma, 1991; Halali et al., 2020). A raise in ambient temperature anticipates the rainy season, when abundant vegetation provides food for rapid larval growth and adult reproduction. Wet-season form butterflies have a fast pace of life (Brakefield et al., 2009; Halali et al., 2020) and display wings with conspicuous marginal eyespots believed to deflect the attack of predators away from the more fragile body (Lyytinen et al., 2004; Olofsson et al., 2010; Prudic et al., 2015).
Laboratory studies have characterized thermal reaction norms for various traits (e.g., Oostra et al., 2011). Revealingly, lab thermal phenotypes do not include phenotypes as extreme as those seen in nature, where other variables combined with temperature might affect development outcomes (Bauerfeind and Fischer, 2005; Rodrigues et al., 2018; Singh et al., 2019), but do include intermediate phenotypes between the typical dry- and wet-season forms, which are rarely found in nature (Brakefield and Reitsma, 1991; Windig et al., 1994; Muller et al., 2019). Lab studies have also allowed characterization of the physiological and genetic basis of thermal plasticity. Measurement and manipulation of ecdysone levels in pupae developing in different temperatures implicated temperature-induced changes in the dynamics of this hormone in the regulation of B. anynana plasticity (Brakefield et al., 1998; Mateus et al., 2014; Oostra et al., 2014; Monteiro et al., 2015; Bear et al., 2017; Bhardwaj et al., 2020). Expression of ecdysone receptor in eyespot organizers has, furthermore, been proposed to account for differences in levels of plasticity between eyespots (Brakefield, 1996; Mateus et al., 2014; Monteiro et al., 2015), and for the evolutionary origin of thermal plasticity in eyespot development (Bhardwaj et al., 2020). Transcriptomic studies targeting individuals from different developmental temperatures have started identifying temperature-regulated genes, potentially responsible different seasonally plastic traits (Macias-Munoz et al., 2016; Oostra et al., 2018). Finally, lab studies have also shed light onto the genetic architecture and constraints on the evolution of thermal reaction norms (Holloway and Brakefield, 1995; Brakefield, 1996; Wijngaarden and Brakefield, 2001; Wijngaarden et al., 2002). On the other hand, field collections allowed for characterization of differences in reaction norms between geographical populations of B. anynana (de Jong et al., 2010) and between Bicyclus species (van Bergen et al., 2017; Balmer et al., 2018).
A number of studies have explored ideas about B. anynana’s vulnerability to climate change. These include assessing effects of increased temperature on organismal performance (Klockmann et al., 2017) and the evolutionary potential for populations to cope with warming (Fischer et al., 2010). They also include discussions about the species’ thermal developmental plasticity possibly becoming a disadvantage if climate change breaks the correlation between the inducing (temperature) and selective (vegetation) environmental variables, and leads to a mismatch between phenotype and environment (de Jong et al., 2010; Oostra et al., 2018), especially if genetic variation for plasticity is depleted (Oostra et al., 2018). Studies for this and other thermal plasticity models, which allow integration of temperature effects across levels (from gene expression, to physiology and development, to multiple adult traits, to individual fitness, to population performance), will be valuable to achieve a better understanding of the impact of phenotypic plasticity in the response to climate change.
Plasticity can be targeted by selection and evolve, and can, in turn, impact adaptive evolution (reviewed in Lafuente and Beldade, 2019). It has been argued that developmental plasticity can help (or hinder; e.g., Cenzer, 2017; Oostra et al., 2018) not only the immediate survival, but also future adaptation of populations facing environmental perturbation (Reed et al., 2011; Bonamour et al., 2019) and colonizing novel environments (Ghalambor et al., 2007; Wang and Althoff, 2019; Bilandžija et al., 2020). In addition, it has been proposed that plasticity can promote phenotypic and taxonomic diversification (Moczek, 2010; Pfennig et al., 2010; Schneider and Meyer, 2017). Whether plasticity can have an impact specifically in responses to climate change has also raised significant attention (Sgrò et al., 2016; Bonamour et al., 2019). Upon change in local environmental conditions, particularly of temperature, organisms that are thermally plastic might display phenotypic change that allows them to rapidly adjust to the new conditions, without genetic change. This type of phenotypic adjustment has been reported for some insect populations, along with other types of population responses to climate change (Figure 1): (1) phenotypic change resulting from genetic change, as populations adapt to new local conditions, (2) shifts in distribution range, as populations track favorable conditions, and (3) population declines that might lead to extinctions. These responses are not mutually exclusive scenarios; they can be combined in different manners (Valladares et al., 2014) and can also be hard to disentangle, as illustrated in the examples below. Shifts in species distributions can result from populations actually migrating to new locations, but can also result from population extinctions on one or multiple distribution edges. Occupation of new locations is generally followed by adaptation to the local conditions (e.g., butterflies that move up along an altitudinal gradient adapted to a host plant in the new habitat; Parmesan et al., 2015). Adaptation to climate change can involve changes in plasticity (e.g., Kingsolver and Buckley, 2018), and plastic responses can facilitate adaptation involving genetic change (e.g., Kelly, 2019) or anticipate extinction (e.g., Manfredini et al., 2019).
Figure 1. Insect examples of responses to climate change. Populations facing warming temperatures that result from climate change can respond in different manners, including moving to new locations where temperature is closer to their optimum (blue), changing genetic composition as they adapt to new local conditions (yellow), expressing suitable temperature-induced phenotype without genetic change (green), or failing to adjust leading to decline and potential extinction (red). Examples from: 1Umina et al., 2005; 2Bradshaw and Holzapfel, 2001; 2001; 3Kearney et al., 2010; 4Jönsson et al., 2007; 5Bentz and Powell, 2014; 6Kollberg et al., 2013; 7Condamine and Sperling, 2018; 8Soroye et al., 2020; 9Faldyn et al., 2018; 10Ryan et al., 2019; 11Parmesan et al., 1999; 12Parkash et al., 2013.
Phenotypic plasticity can impact species distribution and vulnerability (Foden et al., 2019), and might also impact (positively or negatively) population persistence and ability to adapt to challenges arising from climate change (Leonard and Lancaster, 2020). If plasticity leads to changes in phenotype expression in a direction that maintains/improves fitness in the new conditions, it can, indeed, allow organisms to keep pace with environmental change, preventing immediate population extinction (Merilä and Hendry, 2014) and effectively “buy time” for adaptation to occur (Chevin et al., 2010; Snell-Rood et al., 2018). While the positive impact of plasticity in a response to climate change might go beyond buying time (Levis and Pfennig, 2016; Fox et al., 2019), it is also apparent that plasticity can have a negative impact, both by compromising immediate survival (Ghalambor et al., 2007; Manfredini et al., 2019) or by slowing-down future adaptation (discussed in Beldade et al., 2011). Adaptation will be slower if developmental plasticity somehow shields genetic variation from the action of natural selection, but this can be hard to assess (Fox et al., 2019). Survival will be compromised when plasticity leads to expression of phenotypes that, while possibly adaptive in the historical context, are maladaptive in the new conditions (Manfredini et al., 2019). For example, under unusually warm conditions, thermal plasticity in developmental rate in the bark beetle Ips typographus may result in a second generation of beetles consisting of immature stages that are poorly adapted to winter conditions (Dworschak et al., 2014). Maladaptive plasticity seems more common in new habitats, presumably because there has been no evolutionary adjustment of the link between the environmental cues and physiological responses (Ghalambor et al., 2007; Chevin and Hoffmann, 2017). If the environmental cues that leads to change in phenotype expression no longer accurately predict future selective environment, plasticity actually can result in a mis-match between phenotype and environmental conditions. Climate change-related failure in the accuracy of cue predictions can lead to an aggravation of maladaptive phenotype-environment mismatches (e.g., Ghalambor et al., 2007; Bonamour et al., 2019).
Temperature is a key factor determining the geographical distribution, abundance and physiology of insects (Colinet et al., 2015). As small ectotherms whose body temperature closely matches ambient temperature, insects are particularly susceptible to thermal perturbation. Climate change-related temperature variation has been implicated in altered phenology, distribution range, and population abundance of many insect species around the world (Parmesan, 2006; Buckley et al., 2017; Cohen et al., 2018; Macgregor et al., 2019). In this section, we focus on insects’ capability to tolerate, adjust, and adapt to temperature change, which their response to climate change will greatly depend on. We address the relationship between the processes and between their genomic bases.
Temperature acts both as an agent of natural selection (resulting in thermal adaptation), and as a factor affecting phenotype expression (in cases of thermal plasticity). There are any examples of thermal plasticity in insects, including developmental effects and adult acclimation. Temperature-dependence has been described for many processes and traits, including sex determination (Blackmon et al., 2017), induction of diapause (Saunders, 2014), body pigmentation (Sibilia et al., 2018), behavior (Abram et al., 2017). Likewise, thermal adaptation and thermal tolerance have also been extensively studied in various insect species (Tobler et al., 2015; Mallard et al., 2018; Kellermann and van Heerwaarden, 2019). Thermal tolerance, corresponding to a favorable range of temperatures for performance, can be assessed by measuring survival and/or recovery from acute or chronic exposure to temperature extremes (e.g., Kingsolver et al., 2016). As it reflects the capability to cope with adverse temperature conditions, thermal tolerance is very obviously and very directly relevant to how organisms respond to climate change. Moreover, thermal tolerance has also been shown to be associated to other fitness related traits (e.g., tolerance of high temperatures affects dispersal in the Glanville fritillary; Saastamoinen and Hanski, 2008; Mattila, 2015), and to vary between populations and between species (e.g., Hamblin et al., 2017; Oyen and Dillon, 2018).
Thermal plasticity, thermal tolerance, and thermal adaptation are very closely intertwined. Thermal tolerance can be thermally plastic (Schou et al., 2017), but it is unclear how much plasticity in thermal tolerance will impact insects’ response to climate change (Mitchell et al., 2011; Gunderson and Stillman, 2015). Thermal plasticity and thermal tolerance can facilitate thermal adaptation (e.g., Mitchell et al., 2011; Noh et al., 2017). Conversely, thermal adaptation can entail changes in thermal plasticity (discussed above), as well as in thermal tolerance. The evolution of thermal tolerance as a result of adaptation to different thermal regimes is compellingly illustrated by differences between populations along climatic clines, including the negative correlation between heat tolerance and both altitude (e.g., in Heliconius butterflies; Montejo-Kovacevich et al., 2020) and latitude (e.g., in Drosophila flies; van Heerwaarden et al., 2014).
Deciphering the genetic basis of thermal plasticity involves asking about the genes involved in regulating the expression of thermally-dependent phenotypes, as well as about the genes contributing to inter-genotype variation in plasticity that can fuel its evolution (Lafuente and Beldade, 2019). Genomic-level studies of different types have made crucial contributions to both ends. First, investigating the genetic basis of the regulation of thermal plasticity requires identifying genes whose expression and/or function depends on temperature, and, among those, the genes that actually account for changes in thermally-sensitive phenotype expression. Transcriptome-profiling studies in a variety of species have documented thermal plasticity in gene expression levels, including assessment of how many and which genes are differentially expressed between temperatures. The important effect of temperature on transcription has been particularly well studied in the genetic model Drosophila melanogaster (e.g., Chen et al., 2015; Sørensen et al., 2016), but also in other insect examples of thermal plasticity (e.g., Oostra et al., 2018). Importantly, while transcriptome-wide scans allow us to identify many genes whose expression depends on temperature, targeted candidate gene analysis facilitates making the connection between differential gene expression and plastic trait development (e.g., thermal plasticity for body pigmentation in D. melanogaster; Gibert et al., 2016). Second, investigating the genetic basis of the variation in thermal plasticity involves identifying genes that harbor allelic variation contributing for differences in plasticity, and, among those, which actually fuel the evolution of plasticity. Differences between genotypes in levels of thermal developmental plastic, which correspond to significant genotype-by-environment interactions, document the existence of genetic variation for plasticity and offer the opportunity to characterize its nature. Here too, candidate gene studies are quickly being replaced by less-biased whole genome analysis, including genome-wide association studies that identify QTLs for inter-genotype differences in thermal plasticity for specific plastic traits (e.g., QTLs for thermal plasticity for body size in D. melanogaster; Lafuente et al., 2018). These loci can provide the raw material for the evolution of plasticity, including level, direction and inducing cues (discussed in (Lafuente and Beldade, 2019).
The rapid rate of current global climate change, with strong effects on many species, provides both a unique opportunity and a pressing need to study the genetic bases of adaptation, tolerance, and plasticity in natural populations (Franks and Hoffmann, 2012). The extent to which the same genes are involved in thermal adaptation, thermal plasticity, and thermal tolerance has also been addressed both by focusing on candidate genes and by using genomic-level approaches. Perhaps unsurprisingly, several candidate gene studies have focused on genes encoding heat-shock proteins, which have been shown to be thermally plastic, impact thermal tolerance, and differ between populations from different thermal conditions (e.g., Sørensen et al., 2001, 2019; Mattila, 2015; Liu et al., 2017). Heat-shock genes also come up as significant hits in some (e.g., Wang et al., 2019) but not all (e.g., Mallard et al., 2018) genomic-level searches. Accumulating genomic studies in Drosophila melanogaster, using different natural and experimental populations and different approaches (Klepsatel et al., 2013; Tobler et al., 2014; Gerken et al., 2015; Machado et al., 2016; Porcelli et al., 2016; Fabian et al., 2017; Lafuente et al., 2018; Rolandi et al., 2018; Kapun et al., 2020), are building an unprecedently powerful body of data to assess the genomic basis of thermal adaptation, and its repeatability and relationship to thermal plasticity and thermal tolerance. In the future, integration of studies covering different species, different geographical and temporal scales, and different approaches will undoubtedly help shed much needed light onto the genomics of thermal plasticity, as well as its overlap with the genomics of thermal tolerance and thermal adaptation.
Climate change entails changes in mean global temperature, but also in temperature dynamics and in other environmental variables. As such, to assess the potential impact of climate change on natural populations, it is relevant to consider the combined effects of change in temperature with change in other variables. This section considers effects of temperature and other environmental variables on both plasticity and adaptation.
As illustrated above, effects of the environment on developmental outcome have been amply documented for various phenotypes and species. Indeed, phenotypic plasticity (phenotypic differences attributable to environmental variation) and genotype-by-environment interactions (i.e., genetic differences in how organisms respond to environmental conditions) are very common. Unlike what happens for genetic variation, though, where evolutionary biology explicitly considers interaction effects (dominance and epistasis), potential environment-by-environment interactions received considerable less attention. Traditionally, experimental studies of plasticity focused on the analysis of single, isolated environmental factors, held constant during the time it takes organisms to complete their life-cycle. This is in stark contrast with natural situations, where complex environments include variation in multiple and highly dynamic environmental cues. These different variables may act additively on phenotype expression, but may also act redundantly, synergistically, or antagonistically (Piggott et al., 2015; Westneat et al., 2019). Climate change has brought substantial attention to the analysis of multi-stressor effects in populations (Kaunisto et al., 2016), albeit with the majority of studies focused on plants or aquatic systems (e.g., Byrne and Przeslawski, 2013; Gunderson et al., 2016).
Focusing exclusively on environmental factors considered to be individually (i.e., on their own, independently of other environmental factors) and universally (i.e., always, for all genotypes) stressful fails to acknowledge that what is and is not “stressful” might depend on environmental and genetic context. For example, what is a stressful temperature under some photoperiod (or for some genotype) might not be stressful under another photoperiod (or for another genotype). Studies of thermal plasticity in multifactorial environments are increasing, including for different insect species. These studies search to investigate phenotypic effects when variation in temperature is combined with variation in other environmental variables (Figure 2), including biotic and abiotic factors (Bubliy et al., 2013; Schou et al., 2013; Arambourou and Stoks, 2015; Saeed et al., 2018; Kutz et al., 2019). Some studies extend the analysis of plasticity in multifactorial environments to include: (1) multiple traits and/or to multiple genotypes (e.g,., Saastamoinen et al., 2013; Verspagen et al., 2020), (2) three-way environmental interactions (e.g., temperature × humidity × food; Bomble and Nath, 2019), and (3) quantifying underlying changes in gene expression (e.g., candidate genes, Rivas et al., 2018, and whole transcriptome, Koch and Guillaume, 2020). The results to date paint a complex picture, with distinct types of additive (e.g., Koch and Guillaume, 2020) and non-additive (e.g., Yoshii et al., 2009; Arambourou and Stoks, 2015; Piggott et al., 2015) effects of multifactorial environments, and differences between traits and between genotypes. This is an area that will, undoubtedly, know much progress in the near future.
Figure 2. Insect examples of studies of effects of temperature combined with other environmental factors on phenotypic plasticity. Examples from: 1Ahmadi et al., 2018; 2Liefting et al., 2017; 3Schou et al., 2013; 4Arambourou and Stoks, 2015; 5Saeed et al., 2018; 6Bubliy et al., 2013; 7Kutz et al., 2019.
Aside effects on phenotype expression, multifactorial environments will obviously also affect adaptive evolution in ways that might be unpredictable based on variation for single environmental factors. Adaptation to novel combinations of environmental variables might be harder or impossible – for example, if phenotypic change favored by one cue is at odds with that favored in relation to the other cue. Such trade-offs are illustrated by studies where adaptation to specific environments entailed costs in performance in other environments (e.g. Callahan et al., 2008; Nunney, 2016; Fox et al., 2019). In natural populations, different environmental factors act in concert as agents of selection, and can co-vary more or less independently and unpredictably. The fact that associations between environmental variables, as well as their dynamics, are likely to change as a result of climate change further endorses the interest in studying the impact of complex environments on the tempo and mode of adaptive evolution.
Our understanding of the phenotypic and genotypic change that accompanies adaptation of insects to complex environments relies on different types of studies. Studies of natural populations include both “snap-shot” and longitudinal comparisons between populations living in different environments (Reinhardt et al., 2014; Manenti et al., 2017; Lerat et al., 2019; Kapun et al., 2020). While studies of natural populations make it possible to detect genetic and phenotypic differentiation and, sometimes, associate the two, it is generally very difficult to know exactly which environmental variables explain divergence and how. Conversely, in studies of experimental populations forced to evolve in different complex environment (e.g., Tomkins et al., 2011; Tobler et al., 2015; Mallard et al., 2018), we typically know exactly which environmental variables differ between selection lines and can identify genetic differences between those lines, but it is not always easy to know which organismal phenotypes were altered and how. It will be valuable to be able to integrate studies from different types of approaches, and for different species and species groups, to have a better understanding of the mechanisms and limitations of adaptation to complex environments.
Throughout the review, we highlighted what we believe are some areas of particular interest for our understanding of the relevance of thermal plasticity to climate change biology. In light of the topic of this special issue, we discussed recent studies on the genomics of thermal plasticity, distinguishing between those identifying the genes whose expression depends on temperature (and might underlie temperature-induced change in developmental outcome), and in terms of the genes that harbor allelic variants contributing to inter-genotype variation in plasticity (and can feed the evolution of thermal plasticity) (see Lafuente and Beldade, 2019). As data accumulates for different systems, we can hope to deepen our knowledge about what those genes are and about the overlap between them, as well as the overlap between them and the those underlying thermal tolerance and thermal adaptation. We also emphasized the relevance of focusing on temperature in the context of complex multifactorial environments, and the importance of considering that variation in response to temperature can depend on genetic and environmental context.
We focused on thermal plasticity in insects, its potential role in response to climate change, its genomic basis, and the interactions between temperature and other environmental factors. Each of these issues, along with related topics that we did not cover at all, is attracting substantial research attention and, we expect, will know much progress in the near future. Below we highlight the topics complementary to those we covered that are also relevant for the discussion about the relevance of thermal plasticity to climate change biology.
First, we focused primarily on developmental plasticity, which, especially in holometabolous insects, often leads to fixed adult phenotypes. We paid less attention to effects of temperature directly on adult traits, which often lead to reversible phenotypes. These include phenomena that are key to climate change biology, such as acclimation, through physiological and/or behavioral plasticity (Huey et al., 2003; Stillman, 2003). These can mitigate the immediate effect of variation in thermal environments, but can also constrain adaptation to permanent/directional temperature perturbation.
Second, we focused on effects of climate change and of multifactorial environments on molecular-level processes (e.g., thermal plasticity in gene expression), organismal-level processes (e.g., thermal plasticity in developmental outcomes), and population-level processes (e.g., thermal adaptation in experimental and natural populations). We did not discuss supra-population effects of climate change or of multifactorial environments (Fordyce, 2006), such as effects on the species composition of communities (e.g., Chown et al., 2015; de Vries et al., 2019) and on inter-specific interactions (e.g., Williams et al., 2008; Cornelissen, 2011; Wernegreen, 2012; Cahill et al., 2013), both of which can have substantial ramification effects (Grimm et al., 2013).
Finally, we focused exclusively on insect examples, but effects of climate change and multifactorial environments on phenotype expression and adaptation are also being studied in other groups (e.g., Byrne and Przeslawski, 2013; Gunderson et al., 2016; Lange and Marshall, 2017). It will be crucial to integrate different examples both to uncover unique responses, as well as to derive general principles about biological responses to climate change.
YR researched the literature. YR and PB conceived and wrote the manuscript. Both authors contributed to the article and approved the submitted version.
Financial support for this work was provided by the Portuguese science funding agency, Fundação para a Ciência e Tecnologia, FCT: Ph.D. fellowship to YR (FCT SFRH/BD/114404/2016), and research support to PB ((PTDC/BIA-EVF/0017/2014 and PTDC/BEX-BID/5340/2014).
The authors declare that the research was conducted in the absence of any commercial or financial relationships that could be construed as a potential conflict of interest.
We wish to thank the issue and journal editors for the invitation and for accommodating delays due to the pandemics, Joana Carvalho (@joana_gcc) for the Bicyclus anynana drawings in Box 1, and various colleagues for discussions who helped shape our ideas on this topic, including Christian Braendle, Roberto Arbore, Elvira Lafuente, and Erik van Bergen.
Abram, P. K., Boivin, G., Moiroux, J., and Brodeur, J. (2017). Behavioural effects of temperature on ectothermic animals: unifying thermal physiology and behavioural plasticity. Biol. Rev. 92, 1859–1876. doi: 10.1111/brv.12312
Ahmadi, F., Moharramipour, S., and Mikani, A. (2018). The effect of temperature and photoperiod on diapause induction in pupae of Scrobipalpa ocellatella (Lepidoptera: Gelechiidae). Environ. Entomol. 47, 1314–1322. doi: 10.1093/ee/nvy082
Arambourou, H., and Stoks, R. (2015). Combined effects of larval exposure to a heat wave and chlorpyrifos in northern and southern populations of the damselfly Ischnura elegans. Chemosphere 128, 148–154. doi: 10.1016/j.chemosphere.2015.01.044
Balmer, A. J., Brakefield, P. M., Brattström, O., and van Bergen, E. (2018). Developmental plasticity for male secondary sexual traits in a group of polyphenic tropical butterflies. Oikos 127, 1812–1821. doi: 10.1111/oik.05291
Bauerfeind, S. S., and Fischer, K. (2005). Effects of food stress and density in different life stages on reproduction in a butterfly. Oikos 111, 514–524. doi: 10.1111/j.0030-1299.2005.13888.x
Bear, A., and Monteiro, A. (2013). Male Courtship rate plasticity in the butterfly Bicyclus anynana is controlled by temperature experienced during the pupal and adult Stages. PLoS One 8:e064061. doi: 10.1371/journal.pone.0064061
Bear, A., Prudic, K. L., and Monteiro, A. (2017). Steroid hormone signaling during development has a latent effect on adult male sexual behavior in the butterfly Bicyclus anynana. PLoS One 12:e0174403. doi: 10.1371/journal.pone.0174403
Beldade, P., Mateus, A. R. A., and Keller, R. A. (2011). Evolution and molecular mechanisms of adaptive developmental plasticity. Mol. Ecol. 20, 1347–1363. doi: 10.1111/j.1365-294X.2011.05016.x
Beldade, P., and Peralta, C. M. (2017). Developmental and evolutionary mechanisms shaping butterfly eyespots. Curr. Opin. Insect Sci. 19, 22–29. doi: 10.1016/j.cois.2016.10.006
Bentz, B. J., and Powell, J. A. (2014). Mountain pine beetle seasonal timing and constraints to bivoltinism: a comment on Mitton and Ferrenberg, Mountain pine beetle develops an unprecedented summer generation in response to climate warming. Am. Nat. 184, 787–796. doi: 10.1086/678405
Bhardwaj, S., Jolander, L. S. H., Wenk, M. R., Oliver, J. C., Nijhout, H. F., and Monteiro, A. (2020). Origin of the mechanism of phenotypic plasticity in satyrid butterfly eyespots. eLife 9, 1–13. doi: 10.7554/eLife.49544
Bilandžija, H., Hollifield, B., Steck, M., Meng, G., Ng, M., Koch, A. D., et al. (2020). Phenotypic plasticity as a mechanism of cave colonization and adaptation. eLife 9, 1–27. doi: 10.7554/eLife.51830
Blackmon, H., Ross, L., and Bachtrog, D. (2017). Sex determination, sex chromosomes, and karyotype evolution in insects. J. Hered. 108, 78–93. doi: 10.1093/jhered/esw047
Bomble, P., and Nath, B. B. (2019). Comparative susceptibility of chironomus and Drosophila to exposure to each and combinations of the following stressors: desiccation, heat stress and starvation. Eur. J. Environ. Sci. 9, 41–46. doi: 10.14712/23361964.2019.5
Bonamour, S., Chevin, L. M., Charmantier, A., and Teplitsky, C. (2019). Phenotypic plasticity in response to climate change: the importance of cue variation. Philos. Trans. R. Soc. B Biol. Sci. 374:20180178. doi: 10.1098/rstb.2018.0178
Bradshaw, W. E., and Holzapfel, C. M. (2001). Genetic shift in photoperiodic response correlated with global warming. Proc. Natl. Acad. Sci. U.S.A. 98, 14509–14511. doi: 10.1073/PNAS.241391498
Brakefield, P. M. (1996). Seasonal polyphenism in butterflies and natural selection. Trends Ecol. Evol. 11, 275–277. doi: 10.1016/0169-5347(96)30025-30026
Brakefield, P. M., Beldade, P., and Zwaan, B. J. (2009). The African butterfly Bicyclus anynana: a model for evolutionary genetics and evolutionary developmental biology. Cold Spring Harb. Protoc. 4:pdb.emo122. doi: 10.1101/pdb.emo122
Brakefield, P. M., Kesbeke, F., and Koch, P. B. (1998). The regulation of phenotypic plasticity of eyespots in the butterfly Bicyclus anynana. Am. Nat. 152, 853–860. doi: 10.1086/286213
Brakefield, P. M., and Reitsma, N. (1991). Phenotypic plasticity, seasonal climate and the population biology of Bicyclus butterflies (Satyridae) in Malawi. Ecol. Entomol. 16, 291–303. doi: 10.1111/j.1365-2311.1991.tb00220.x
Bubliy, O. A., Kristensen, T. N., and Loeschcke, V. (2013). Stress-induced plastic responses in Drosophila simulans following exposure to combinations of temperature and humidity levels. J. Exp. Biol. 216, 4601–4607. doi: 10.1242/jeb.092502
Buckley, L. B., Arakaki, A. J., Cannistra, A. F., Kharouba, H. M., and Kingsolver, J. G. (2017). Insect development, thermal plasticity and fitness implications in changing, seasonal environments. Integr. Comp. Biol. 57, 988–998. doi: 10.1093/icb/icx032
Byrne, M., and Przeslawski, R. (2013). Multistressor impacts of warming and acidification of the ocean on marine invertebrates’ life histories. Integr. Comp. Biol. 53, 582–596. doi: 10.1093/icb/ict049
Cahill, A. E., Aiello-Lammens, M. E., Caitlin Fisher-Reid, M., Hua, X., Karanewsky, C. J., Ryu, H. Y., et al. (2013). How does climate change cause extinction? Proc. R. Soc. B Biol. Sci. 280:890. doi: 10.1098/rspb.2012.1890
Callahan, H. S., Maughan, H., and Steiner, U. K. (2008). Phenotypic plasticity, costs of phenotypes, and costs of plasticity: toward an integrative view. Ann. N. Y. Acad. Sci. 1133, 44–66. doi: 10.1196/annals.1438.008
Cenzer, M. L. (2017). Maladaptive plasticity masks the effects of natural selection in the red-shouldered soapberry bug. Am. Nat. 190, 521–533. doi: 10.1086/693456
Chen, J., Nolte, V., and Schlotterer, C. (2015). Temperature-related reaction norms of gene expression: regulatory architecture and functional implications. Mol. Biol. Evol. 32, 2393–2402. doi: 10.1093/molbev/msv120
Chevin, L. M., Collins, S., and Lefèvre, F. (2013). Phenotypic plasticity and evolutionary demographic responses to climate change: taking theory out to the field. Funct. Ecol. 27, 967–979. doi: 10.1111/j.1365-2435.2012.02043.x
Chevin, L. M., and Hoffmann, A. A. (2017). Evolution of phenotypic plasticity in extreme environments. Philos. Trans. R. Soc. B Biol. Sci. 372:20160138. doi: 10.1098/rstb.2016.0138
Chevin, L. M., Lande, R., and Mace, G. M. (2010). Adaptation, plasticity, and extinction in a changing environment: towards a predictive theory. PLoS Biol. 8:1000357. doi: 10.1371/journal.pbio.1000357
Chown, S. L., Hodgins, K. A., Griffin, P. C., Oakeshott, J. G., Byrne, M., and Hoffmann, A. A. (2015). Biological invasions, climate change and genomics. Evol. Appl. 8, 23–46. doi: 10.1111/eva.12234
Cohen, J. M., Lajeunesse, M. J., and Rohr, J. R. (2018). A global synthesis of animal phenological responses to climate change/631/158/2165/2457/631/158/2039/129/141/139 letter. Nat. Clim. Chang. 8, 224–228. doi: 10.1038/s41558-018-0067-63
Colinet, H., Sinclair, B. J., Vernon, P., and Renault, D. (2015). Insects in fluctuating thermal environments. Annu. Rev. Entomol. 60, 123–140. doi: 10.1146/annurev-ento-010814-021017
Condamine, F. L., and Sperling, F. A. H. (2018). Anthropogenic threats to high-altitude parnassian diversity. News Lepid. Soc. 60, 94–99.
Cornelissen, T. (2011). Climate change and its effects on terrestrial insects and herbivory patterns. Neotrop. Entomol. 40, 155–163. doi: 10.1590/S1519-566X2011000200001
de Jong, M. A., Kesbeke, F. M. N. H., Brakefield, P. M., and Zwaan, B. J. (2010). Geographic variation in thermal plasticity of life history and wing pattern in Bicyclus anynana. Clim. Res. 43, 91–102. doi: 10.3354/cr00881
de Vries, J., Kraak, M. H. S., Verdonschot, R. C. M., and Verdonschot, P. F. M. (2019). Quantifying cumulative stress acting on macroinvertebrate assemblages in lowland streams. Sci. Total Environ. 694:133630. doi: 10.1016/j.scitotenv.2019.133630
Didham, R. K., Basset, Y., Collins, C. M., Leather, S. R., Littlewood, N. A., Menz, M. H. M., et al. (2020). Interpreting insect declines: seven challenges and a way forward. Insect. Conserv. Divers. 13, 103–114. doi: 10.1111/icad.12408
Donelson, J. M., Salinas, S., Munday, P. L., and Shama, L. N. S. (2018). Transgenerational plasticity and climate change experiments: where do we go from here? Glob. Chang. Biol. 24, 13–34. doi: 10.1111/gcb.13903
Dworschak, K., Gruppe, A., and Schopf, R. (2014). Survivability and post-diapause fitness in a scolytid beetle as a function of overwintering developmental stage and the implications for population dynamics. Ecol. Entomol. 39, 519–526. doi: 10.1111/een.12127
Fabian, D. K., Lack, J. B., Mathur, V., Schlötterer, C., Schmidt, P. S., Pool, J. E., et al. (2017). Spatially varying selection shapes life history clines among populations of Drosophila melanogaster from sub-Saharan Africa. J. Evol. Biol. 28, 826–840. doi: 10.1016/j.physbeh.2017.03.040
Faldyn, M. J., Hunter, M. D., and Elderd, B. D. (2018). Climate change and an invasive, tropical milkweed: an ecological trap for monarch butterflies. Ecology 99, 1031–1038. doi: 10.1002/ecy.2198
Fischer, K., Dierks, A., Franke, K., Geister, T. L., Liszka, M., Winter, S., et al. (2010). Environmental effects on temperature stress resistance in the tropical butterfly Bicyclus anynana. PLoS One 5:e15284. doi: 10.1371/journal.pone.0015284
Fischer, K., Zwaan, B. J., and Brakefield, P. M. (2007). Realized correlated responses to artificial selection on pre-adult life-history traits in a butterfly. Heredity 98, 157–164. doi: 10.1038/sj.hdy.6800919
Foden, W. B., Young, B. E., Akçakaya, H. R., Garcia, R. A., Hoffmann, A. A., Stein, B. A., et al. (2019). Climate change vulnerability assessment of species. Wiley Interdiscip. Rev. Clim. Chang. 10, 1–36. doi: 10.1002/wcc.551
Fordyce, J. A. (2006). The evolutionary consequences of ecological interactions mediated through phenotypic plasticity. J. Exp. Biol. 209, 2377–2383. doi: 10.1242/jeb.02271
Fox, R. J., Donelson, J. M., Schunter, C., Ravasi, T., and Gaitán-Espitia, J. D. (2019). Beyond buying time: the role of plasticity in phenotypic adaptation to rapid environmental change. Philos. Trans. R. Soc. B Biol. Sci. 374:174. doi: 10.1098/rstb.2018.0174
Franks, S. J., and Hoffmann, A. A. (2012). Genetics of climate change adaptation. Annu. Rev. Genet. 46, 185–208. doi: 10.1146/annurev-genet-110711-155511
Gerken, A. R., Eller, O. C., Hahn, D. A., and Morgan, T. J. (2015). Constraints, independence, and evolution of thermal plasticity: probing genetic architecture of long-and short-term thermal acclimation. Proc. Natl. Acad. Sci. U.S.A. 112, 4399–4404. doi: 10.1073/pnas.1503456112
Ghalambor, C. K., McKay, J. K., Carroll, S. P., and Reznick, D. N. (2007). Adaptive versus non-adaptive phenotypic plasticity and the potential for contemporary adaptation in new environments. Funct. Ecol. 21, 394–407. doi: 10.1111/j.1365-2435.2007.01283.x
Gibert, J. M., Mouchel-Vielh, E., De Castro, S., and Peronnet, F. (2016). Phenotypic plasticity through transcriptional regulation of the evolutionary hotspot gene tan in Drosophila melanogaster. PLoS Genet. 12:1006218. doi: 10.1371/journal.pgen.1006218
Gilbert, S. F., Bosch, T. C. G., and Ledón-Rettig, C. (2015). Eco-Evo-Devo: developmental symbiosis and developmental plasticity as evolutionary agents. Nat. Rev. Genet. 16, 611–622. doi: 10.1038/nrg3982
Grimm, N. B., Chapin, F. S., Bierwagen, B., Gonzalez, P., Groffman, P. M., Luo, Y., et al. (2013). The impacts of climate change on ecosystem structure and function. Front. Ecol. Environ. 11, 474–482. doi: 10.1890/120282
Gunderson, A. R., Armstrong, E. J., and Stillman, J. H. (2016). Multiple stressors in a changing world: The need for an improved perspective on physiological responses to the dynamic marine environment. Ann. Rev. Mar. Sci. 8, 357–378. doi: 10.1146/annurev-marine-122414-133953
Gunderson, A. R., and Stillman, J. H. (2015). Plasticity in thermal tolerance has limited potential to buffer ectotherms from global warming. Proc. R. Soc. B Biol. Sci. 282:401. doi: 10.1098/rspb.2015.0401
Halali, S., Brakefield, P. M., Collins, S. C., and Brattström, O. (2020). To mate, or not to mate: the evolution of reproductive diapause facilitates insect radiation into African savannahs in the Late Miocene. J. Anim. Ecol. 89, 1230–1241. doi: 10.1111/1365-2656.13178
Hamblin, A. L., Youngsteadt, E., López-Uribe, M. M., and Frank, S. D. (2017). Physiological thermal limits predict differential responses of bees to urban heat-island effects. Biol. Lett. 13:125. doi: 10.1098/rsbl.2017.0125
Holloway, G. J., and Brakefield, P. M. (1995). Artificial selection of reaction norms of wing pattern elements in Bicyclus anynana. Heredity 74, 91–99. doi: 10.1038/hdy.1995.11
Huey, R. B., Hertz, P. E., and Sinervo, B. (2003). Behavioral drive versus behavioral inertia in evolution: a null model approach. Amer. Nat. 161, 357–366.
Johnston, A. S. A., Boyd, R. J., Watson, J. W., Paul, A., Evans, L. C., Gardner, E. L., et al. (2019). Predicting population responses to environmental change from individual-level mechanisms: Towards a standardized mechanistic approach. Proc. R. Soc. B Biol. Sci. 286:1916. doi: 10.1098/rspb.2019.1916
Jönsson, A. M., Harding, S., Bärring, L., and Ravn, H. P. (2007). Impact of climate change on the population dynamics of Ips typographus in southern Sweden. Agric. For. Meteorol. 146, 70–81. doi: 10.1016/j.agrformet.2007.05.006
Kapun, M., Barrón, M. G., Staubach, F., Obbard, D. J., Wiberg, R. A. W., Vieira, J., et al. (2020). Genomic analysis of European Drosophila melanogaster populations reveals longitudinal structure, continent-wide selection, and previously unknown DNA viruses. Mol. Biol. Evol. 2020:msaa120. doi: 10.1093/molbev/msaa120
Kaunisto, S., Ferguson, L. V., and Sinclair, B. J. (2016). Can we predict the effects of multiple stressors on insects in a changing climate? Curr. Opin. Insect. Sci. 17, 55–61. doi: 10.1016/j.cois.2016.07.001
Kearney, M. R., Briscoe, N. J., Karoly, D. J., Porter, W. P., Norgate, M., and Sunnucks, P. (2010). Early emergence in a butterfly causally linked to anthropogenic warming. Biol. Lett. 6, 674–677. doi: 10.1098/rsbl.2010.0053
Kellermann, V., and van Heerwaarden, B. (2019). Terrestrial insects and climate change: adaptive responses in key traits. Physiol. Entomol. 44, 99–115. doi: 10.1111/phen.12282
Kelly, M. (2019). Adaptation to climate change through genetic accommodation and assimilation of plastic phenotypes. Philos. Trans. R. Soc. B Biol. Sci. 374:176. doi: 10.1098/rstb.2018.0176
Kingsolver, J. G., and Buckley, L. B. (2018). How do phenology, plasticity, and evolution determine the fitness consequences of climate change for montane butterflies? Evol. Appl. 11, 1231–1244. doi: 10.1111/eva.12618
Kingsolver, J. G., MacLean, H. J., Goddin, S. B., and Augustine, K. E. (2016). Plasticity of upper thermal limits to acute and chronic temperature variation in Manduca sexta larvae Joel. J. Exp. Biol. 219, 1290–1294. doi: 10.1242/jeb.138321
Klepsatel, P., Gáliková, M., De Maio, N., Huber, C. D., Schlötterer, C., and Flatt, T. (2013). Variation in thermal performance and reaction norms among populations of Drosophila melanogaster. Evolution 67, 3573–3587. doi: 10.1111/evo.12221
Klockmann, M., Kleinschmidt, F., and Fischer, K. (2017). Carried over: heat stress in the egg stage reduces subsequent performance in a butterfly. PLoS One 12:180968. doi: 10.1371/journal.pone.0180968
Koch, E. L., and Guillaume, F. (2020). Additive and mostly adaptive plastic responses of gene expression to multiple stress in Tribolium castaneum. PLoS Genet. 16:e1008768. doi: 10.1371/journal.pgen.1008768
Kollberg, I., Bylund, H., Schmidt, A., Gershenzon, J., and Björkman, C. (2013). Multiple effects of temperature, photoperiod and food quality on the performance of a pine sawfly. Ecol. Entomol. 38, 201–208. doi: 10.1111/een.12005
Kutz, T. C., Sgrò, C. M., and Mirth, C. K. (2019). Interacting with change: diet mediates how larvae respond to their thermal environment. Funct. Ecol. 33, 1940–1951. doi: 10.1111/1365-2435.13414
Lafuente, E., and Beldade, P. (2019). Genomics of developmental plasticity in animals. Front. Genet. 10:720. doi: 10.3389/fgene.2019.00720
Lafuente, E., Duneau, D., and Beldade, P. (2018). Genetic basis of thermal plasticity variation in Drosophila melanogaster body size. PLoS Genet. 14:e1007686. doi: 10.1371/journal.pgen.1007686
Lange, R., and Marshall, D. (2017). Ecologically relevant levels of multiple, common marine stressors suggest antagonistic effects. Sci. Rep. 7, 1–9. doi: 10.1038/s41598-017-06373-y
Leonard, A. M., and Lancaster, L. T. (2020). Maladaptive plasticity facilitates evolution of thermal tolerance during an experimental range shift. BMC Evol. Biol. 20:1587. doi: 10.1186/s12862-020-1589-1587
Lerat, E., Goubert, C., Guirao-Rico, S., Merenciano, M., Dufour, A. B., Vieira, C., et al. (2019). Population-specific dynamics and selection patterns of transposable element insertions in European natural populations. Mol. Ecol. 28, 1506–1522. doi: 10.1111/mec.14963
Levis, N. A., and Pfennig, D. W. (2016). Evaluating “Plasticity-First” evolution in Nature: key criteria and empirical approaches. Trends Ecol. Evol. 31, 563–574. doi: 10.1016/j.tree.2016.03.012
Liefting, M., Cosijn, J., and Ellers, J. (2017). Synergistic effect of daily temperature fluctuations and matching light-dark cycle enhances population growth and synchronizes oviposition behavior in a soil arthropod. J. Insect Physiol. 96, 108–114. doi: 10.1016/j.jinsphys.2016.10.002
Liu, Y., Su, H., Li, R., Li, X., Xu, Y., Dai, X., et al. (2017). Comparative transcriptome analysis of Glyphodes pyloalis walker (Lepidoptera: Pyralidae) reveals novel insights into heat stress tolerance in insects. BMC Genomics 18:974. doi: 10.1186/s12864-017-4355-4355
Lyytinen, A., Brakefield, P. M., Lindstrom, L., and Mappes, J. (2004). Does predation maintain eyespot plasticity in Bicyclus anynana? Proc. R. Soc. B Biol. Sci. 271, 279–283. doi: 10.1098/rspb.2003.2571
Macgregor, C. J., Thomas, C. D., Roy, D. B., Beaumont, M. A., Bell, J. R., Brereton, T., et al. (2019). Climate-induced phenology shifts linked to range expansions in species with multiple reproductive cycles per year. Nat. Commun. 10:4455. doi: 10.1038/s41467-019-12479-w
Machado, H. E., Bergland, A. O., O’Brien, K. R., Behrman, E. L., Schmidt, P. S., and Petrov, D. A. (2016). Comparative population genomics of latitudinal variation in Drosophila simulans and Drosophila melanogaster. Mol. Ecol. 25, 723–740. doi: 10.1111/mec.13446
Macias-Munoz, A., Smith, G., Monteiro, A., and Briscoe, A. D. (2016). Transcriptome-wide differential gene expression in Bicyclus anynana butterflies: female vision-related genes are more plastic. Mol. Biol. Evol. 33, 79–92. doi: 10.1093/molbev/msv197
Mallard, F., Nolte, V., Tobler, R., Kapun, M., and Schlötterer, C. (2018). A simple genetic basis of adaptation to a novel thermal environment results in complex metabolic rewiring in Drosophila. Genome Biol. 19, 1–15. doi: 10.1186/s13059-018-1503-1504
Manenti, T., Sørensen, J. G., and Loeschcke, V. (2017). Environmental heterogeneity does not affect levels of phenotypic plasticity in natural populations of three Drosophila species. Ecol. Evol. 7, 2716–2724. doi: 10.1002/ece3.2904
Manfredini, F., Arbetman, M., and Toth, A. L. (2019). A potential role for phenotypic plasticity in invasions and declines of social insects. Front. Ecol. Evol. 7:375. doi: 10.3389/fevo.2019.00375
Mateus, A. R. A., Marques-Pita, M., Oostra, V., Lafuente, E., Brakefield, P. M., Zwaan, B. J., et al. (2014). Adaptive developmental plasticity: compartmentalized responses to environmental cues and to corresponding internal signals provide phenotypic flexibility. BMC Biol. 12:97. doi: 10.1186/s12915-014-0097-x
Mattila, A. L. K. (2015). Thermal biology of flight in a butterfly: genotype, flight metabolism, and environmental conditions. Ecol. Evol. 5, 5539–5551. doi: 10.1002/ece3.1758
Merilä, J., and Hendry, A. P. (2014). Climate change, adaptation, and phenotypic plasticity: the problem and the evidence. Evol. Appl. 7, 1–14. doi: 10.1111/eva.12137
Mitchell, K. A., Sgrò, C. M., and Hoffmann, A. A. (2011). Phenotypic plasticity in upper thermal limits is weakly related to Drosophila species distributions. Funct. Ecol. 25, 661–670. doi: 10.1111/j.1365-2435.2010.01821.x
Moczek, A. P. (2010). Phenotypic plasticity and diversity in insects. Philos. Trans. R. Soc. B Biol. Sci. 365, 593–603. doi: 10.1098/rstb.2009.0263
Monteiro, A., Tong, X., Bear, A., Liew, S. F., Bhardwaj, S., Wasik, B. R., et al. (2015). Differential expression of ecdysone receptor leads to variation in phenotypic plasticity across serial homologs. PLoS Genet. 11:1005529. doi: 10.1371/journal.pgen.1005529
Montejo-Kovacevich, G., Martin, S. H., Meier, J. I., Bacquet, C. N., Monllor, M., Jiggins, C. D., et al. (2020). Microclimate buffering and thermal tolerance across elevations in a tropical butterfly. J. Exp. Biol. 223:jeb220426. doi: 10.1242/jeb.220426
Muller, D., Elias, B., Collard, L., Pels, C., Holveck, M. J., and Nieberding, C. M. (2019). Polyphenism of visual and chemical secondary sexually-selected wing traits in the butterfly Bicyclus anynana: how different is the intermediate phenotype? PLoS One 14:225003. doi: 10.1371/journal.pone.0225003
Nettle, D., and Bateson, M. (2015). Adaptive developmental plasticity: what is it, how can we recognize it and when can it evolve? Proc. R. Soc. B Biol. Sci. 282:1005. doi: 10.1098/rspb.2015.1005
Nijhout, H. F. (2003). Development and evolution of adaptive polyphenisms. Evol. Dev. 5, 9–18. doi: 10.1046/j.1525-142X.2003.03003.x
Noh, S., Everman, E. R., Berger, C. M., and Morgan, T. J. (2017). Seasonal variation in basal and plastic cold tolerance: adaptation is influenced by both long- and short-term phenotypic plasticity. Ecol. Evol. 7, 5248–5257. doi: 10.1002/ece3.3112
Nunney, L. (2016). Adapting to a changing environment: modeling the interaction of directional selection and plasticity. J. Hered. 107, 15–24. doi: 10.1093/jhered/esv084
Olofsson, M., Vallin, A., Jakobsson, S., and Wiklund, C. (2010). Marginal eyespots on butterfly wings deflect bird attacks under low light intensities with UV wavelengths. PLoS One 5:10798. doi: 10.1371/journal.pone.0010798
Oostra, V., de Jong, M. A., Invergo, B. M., Kesbeke, F., Wende, F., Brakefield, P. M., et al. (2011). Translating environmental gradients into discontinuous reaction norms via hormone signalling in a polyphenic butterfly. Proc. R. Soc. B Biol. Sci. 278, 789–797. doi: 10.1098/rspb.2010.1560
Oostra, V., Mateus, A. R. A., van der Burg, K. R. L., Piessens, T., Eijk, M., Van Brakefield, P. M., et al. (2014). Ecdysteroid hormones link the juvenile environment to alternative adult life histories in a seasonal insect. Am. Nat. 184, E79–E92. doi: 10.1086/677260
Oostra, V., Saastamoinen, M., Zwaan, B. J., and Wheat, C. W. (2018). Strong phenotypic plasticity limits potential for evolutionary responses to climate change. Nat. Commun. 9:1005. doi: 10.1038/s41467-018-03384-3389
Oyen, K. J., and Dillon, M. E. (2018). Critical thermal limits of bumblebees (Bombus impatiens) are marked by stereotypical behaviors and are unchanged by acclimation, age or feeding status. J. Exp. Biol. 221:jeb165589. doi: 10.1242/jeb.165589
Parkash, R., Ramniwas, S., and Kajla, B. (2013). Climate warming mediates range shift of two differentially adapted stenothermal Drosophila species in the Western Himalayas. J. Asia. Pac. Entomol. 16, 147–153. doi: 10.1016/j.aspen.2012.12.004
Parmesan, C. (2006). Ecological and evolutionary responses to recent climate change. Annu. Rev. Ecol. Evol. Syst. 37, 637–669. doi: 10.2307/annurev.ecolsys.37.091305.30000024
Parmesan, C., Ryrholm, N., Stefanescu, C., Hill, J. K., Thomas, C. D., Descimon, H., et al. (1999). Poleward shifts in geographical ranges of butterfly species associated with regional warming. Nature 399, 579–583. doi: 10.1038/21181
Parmesan, C., Williams-Anderson, A., Moskwik, M., Mikheyev, A. S., and Singer, M. C. (2015). Endangered Quino checkerspot butterfly and climate change: short-term success but long-term vulnerability? J. Insect Conserv. 19, 185–204. doi: 10.1007/s10841-014-9743-9744
Pfennig, D. W., Wund, M. A., Snell-Rood, E. C., Cruickshank, T., Schlichting, C. D., and Moczek, A. P. (2010). Phenotypic plasticity’s impacts on diversification and speciation. Trends Ecol. Evol. 25, 459–467. doi: 10.1016/j.tree.2010.05.006
Piggott, J. J., Townsend, C. R., and Matthaei, C. D. (2015). Reconceptualizing synergism and antagonism among multiple stressors. Ecol. Evol. 5, 1538–1547. doi: 10.1002/ece3.1465
Pijpe, J., Fischer, K., Brakefield, P. M., and Zwaan, B. J. (2006). Consequences of artificial selection on pre-adult development for adult lifespan under benign conditions in the butterfly Bicyclus anynana. Mech. Ageing Dev. 127, 802–807. doi: 10.1016/j.mad.2006.07.006
Porcelli, D., Westram, A. M., Pascual, M., Gaston, K. J., Butlin, R. K., and Snook, R. R. (2016). Gene expression clines reveal local adaptation and associated trade-offs at a continental scale. Sci. Rep. 6, 1–12. doi: 10.1038/srep32975
Prudic, K. L., Jeon, C., Cao, H., and Monteiro, A. (2011). Developmental plasticity in sexual roles of butterfly species drives mutual sexual ornamentation. Science 331, 73–75. doi: 10.1126/science.1197114
Prudic, K. L., Stoehr, A. M., Wasik, B. R., and Monteiro, A. (2015). Eyespots deflect predator attack increasing fitness and promoting the evolution of phenotypic plasticity. Proc. R. Soc. B Biol. Sci. 282:1531. doi: 10.1098/rspb.2014.1531
Reed, T. E., Schindler, D. E., and Waples, R. S. (2011). Interacting effects of phenotypic plasticity and evolution on population persistence in a changing climate. Conserv. Biol. 25, 56–63. doi: 10.1111/j.1523-1739.2010.01552.x
Reinhardt, J. A., Kolaczkowski, B., Jones, C. D., Begun, D. J., and Kern, A. D. (2014). Parallel geographic variation in Drosophila melanogaster. Genetics 197, 361–373. doi: 10.1534/genetics.114.161463
Rivas, M. J., Saura, M., Pérez-Figueroa, A., Panova, M., Johansson, T., André, C., et al. (2018). Population genomics of parallel evolution in gene expression and gene sequence during ecological adaptation. Sci. Rep. 8, 1–12. doi: 10.1038/s41598-018-33897-33898
Rodrigues, Y. K., van Bergen, E., Alves, F., Duneau, D., and Beldade, P. (2018). Complex effects of day and night temperature fluctuations on thermally plastic traits in an experimental model of adaptive seasonal plasticity. bioRxiv [Preprint], doi: 10.1101/207258
Rolandi, C., Lighton, J. R. B., de la Vega, G. J., Schilman, P. E., and Mensch, J. (2018). Genetic variation for tolerance to high temperatures in a population of Drosophila melanogaster. Ecol. Evol. 8, 10374–10383. doi: 10.1002/ece3.4409
Ryan, S. J., Carlson, C. J., Mordecai, E. A., and Johnson, L. R. (2019). Global expansion and redistribution of Aedes-borne virus transmission risk with climate change. PLoS Negl. Trop. Dis. 13:7213. doi: 10.1371/journal.pntd.0007213
Saastamoinen, M., Brommer, J. E., Brakefield, P. M., and Zwaan, B. J. (2013). Quantitative genetic analysis of responses to larval food limitation in a polyphenic butterfly indicates environmentand trait-specific effects. Ecol. Evol. 3, 3565–3575. doi: 10.1002/ece3.684
Saastamoinen, M., and Hanski, I. (2008). Genotypic and environmental effects on flight activity and oviposition in the glanville fritillary butterfly. Am. Nat. 171, 701–712. doi: 10.1086/587531
Saeed, N., Battisti, A., Martinez-Sañudo, I., and Mori, N. (2018). Combined effect of temperature and Wolbachia infection on the fitness of Drosophila suzukii. Bull. Insectol. 71, 161–169.
Sánchez-Bayo, F., and Wyckhuys, K. A. G. (2019). Worldwide decline of the entomofauna: a review of its drivers. Biol. Conserv. 232, 8–27. doi: 10.1016/j.biocon.2019.01.020
Saunders, D. S. (2014). Insect photoperiodism: effects of temperature on the induction of insect diapause and diverse roles for the circadian system in the photoperiodic response. Entomol. Sci. 17, 25–40. doi: 10.1111/ens.12059
Schneider, R. F., and Meyer, A. (2017). How plasticity, genetic assimilation and cryptic genetic variation may contribute to adaptive radiations. Mol. Ecol. 26, 330–350. doi: 10.1111/mec.13880
Schou, M. F., Mouridsen, M. B., Sørensen, J. G., and Loeschcke, V. (2017). Linear reaction norms of thermal limits in Drosophila: predictable plasticity in cold but not in heat tolerance. Funct. Ecol. 31, 934–945. doi: 10.1111/1365-2435.12782
Schou, T. M., Faurby, S., Kjaersgaard, A., Pertoldi, C., Loeschcke, V., Hald, B., et al. (2013). Temperature and population density effects on locomotor activity of Musca domestica (Diptera: Muscidae). Environ. Entomol. 42, 1322–1328. doi: 10.1603/en13039
Sgrò, C. M., Terblanche, J. S., and Hoffmann, A. A. (2016). What can plasticity contribute to insect responses to climate change? Annu. Rev. Entomol. 61, 433–451. doi: 10.1146/annurev-ento-010715-023859
Sibilia, C. D., Brosko, K. A., Hickling, C. J., Thompson, L. M., Grayson, K. L., and Olson, J. R. (2018). Thermal physiology and developmental plasticity of pigmentation in the harlequin bug (Hemiptera: Pentatomidae). J. Insect Sci. 18:iey066. doi: 10.1093/jisesa/iey066
Simpson, S. J., Sword, G. A., and Lo, N. (2011). Polyphenism in insects. Curr. Biol. 21, 738–749. doi: 10.1016/j.cub.2011.06.006
Singh, P., Bergen, E., Van Brattström, O., Osbaldeston, D., and Paul, M. (2019). Complex multi-trait responses to multivariate environmental cues in a seasonal butterfly. bioRxiv [Preprint], doi: 10.1101/772749
Snell-Rood, E. C., Kobiela, M. E., Sikkink, K. L., and Shephard, A. M. (2018). Mechanisms of plastic rescue in novel environments. Annu. Rev. Ecol. Evol. Syst. 49, 331–354. doi: 10.1146/annurev-ecolsys-110617-062622
Song, H., Foquet, B., Mariño-Pérez, R., and Woller, D. A. (2017). Phylogeny of locusts and grasshoppers reveals complex evolution of density-dependent phenotypic plasticity. Sci. Rep. 7, 1–13. doi: 10.1038/s41598-017-07105-y
Sørensen, J. G., Dahlgaard, J., and Loeschcke, V. (2001). Genetic variation in thermal tolerance among natural populations of Drosophila buzzatii: down regulation of Hsp70 expression and variation in heat stress resistance traits. Funct. Ecol. 15, 289–296. doi: 10.1046/j.1365-2435.2001.00525.x
Sørensen, J. G., Giribets, M. P., Tarrío, R., Rodríguez-Trelles, F., Schou, M. F., and Loeschcke, V. (2019). Expression of thermal tolerance genes in two Drosophila species with different acclimation capacities. J. Therm. Biol. 84, 200–207. doi: 10.1016/j.jtherbio.2019.07.005
Sørensen, J. G., Schou, M. F., Kristensen, T. N., and Loeschcke, V. (2016). Thermal fluctuations affect the transcriptome through mechanisms independent of average temperature. Sci. Rep. 6, 1–11. doi: 10.1038/srep30975
Soroye, P., Newbold, T., and Kerr, J. (2020). Climate change contributes to widespread declines among bumble bees across continents. Science 367, 685–688. doi: 10.1126/science.aax8591
Stillman, J. H. (2003). Acclimation capacity underlies susceptibility to climate change. Science 301, 65. doi: 10.1126/science.1083073
Tobler, R., Franssen, S. U., Kofler, R., Orozco-Terwengel, P., Nolte, V., Hermisson, J., et al. (2014). Massive habitat-specific genomic response in D. melanogaster populations during experimental evolution in hot and cold environments. Mol. Biol. Evol. 31, 364–375. doi: 10.1093/molbev/mst205
Tobler, R., Hermisson, J., and Schlötterer, C. (2015). Parallel trait adaptation across valaopposing thermal environments in experimental Drosophila melanogaster populations. Evolution 69, 1745–1759. doi: 10.1111/evo.12705
Tomkins, J. L., Hazel, W. N., Penrose, M. A., Radwan, J. W., and Lebas, N. R. (2011). Habitat complexity drives experimental evolution of a conditionally expressed secondary sexual trait. Curr. Biol. 21, 569–573. doi: 10.1016/j.cub.2011.02.032
Umina, P. A., Weeks, A. R., Kearney, M. R., McKechnie, S. W., and Hoffmann, A. A. (2005). Evolution: a rapid shift in a classic clinal pattern in Drosophila reflecting climate change. Science 308, 691–693. doi: 10.1126/science.1109523
Valladares, F., Matesanz, S., Guilhaumon, F., Araújo, M. B., Balaguer, L., Benito-Garzón, M., et al. (2014). The effects of phenotypic plasticity and local adaptation on forecasts of species range shifts under climate change. Ecol. Lett. 17, 1351–1364. doi: 10.1111/ele.12348
van Bergen, E., and Beldade, P. (2019). Seasonal plasticity in anti-predatory strategies: matching of color and color preference for effective crypsis. Evol. Lett. 3, 313–320. doi: 10.1002/evl3.113
van Bergen, E., Osbaldeston, D., Kodandaramaiah, U., Brattström, O., Aduse-Poku, K., and Brakefield, P. M. (2017). Conserved patterns of integrated developmental plasticity in a group of polyphenic tropical butterflies. BMC Evol. Biol. 17:59. doi: 10.1186/s12862-017-0907-901
van Heerwaarden, B., Lee, R. F. H., Overgaard, J., and Sgrò, C. M. (2014). No patterns in thermal plasticity along a latitudinal gradient in Drosophila simulans from eastern Australia. J. Evol. Biol. 27, 2541–2553. doi: 10.1111/jeb.12510
Verspagen, N., Suvi, I., Marjo, S., and Erik van, B. (2020). Multidimensional plasticity in the glanville fritillary butterfly: larval performance curves are temperature, host and family specific. bioRxiv [Preprint], doi: 10.1101/2020.05.05.065698
Wagner, D. L. (2020). Insect declines in the anthropocene. Annu. Rev. Entomol. 65, 457–480. doi: 10.1146/annurev-ento-011019-025151
Wang, S. P., and Althoff, D. M. (2019). Phenotypic plasticity facilitates initial colonization of a novel environment. Evolution 73, 303–316. doi: 10.1111/evo.13676
Wang, X. R., Wang, C., Ban, F. X., Zhu, D. T., Liu, S. S., and Wang, X. W. (2019). Genome-wide identification and characterization of HSP gene superfamily in whitefly (Bemisia tabaci) and expression profiling analysis under temperature stress. Insect Sci. 26, 44–57. doi: 10.1111/1744-7917.12505
Wasik, B. R., Liew, S. F., Lilien, D. A., Dinwiddie, A. J., Noh, H., Cao, H., et al. (2014). Artificial selection for structural color on butterfly wings and comparison with natural evolution. Proc. Natl. Acad. Sci. U.S.A. 111, 12109–12114. doi: 10.1073/pnas.1402770111
Wernegreen, J. J. (2012). Mutualism meltdown in insects: bacteria constrain thermal adaptation. Curr. Opin. Microbiol. 15, 255–262. doi: 10.1016/j.mib.2012.02.001
Westerman, E., and Monteiro, A. (2016). Rearing temperature influences adult response to changes in mating status. PLoS One 11:e0146546. doi: 10.1371/journal.pone.0146546
Westneat, D. F., Potts, L. J., Sasser, K. L., and Shaffer, J. D. (2019). Causes and consequences of phenotypic plasticity in complex environments. Trends Ecol Evol 34, 555–568. doi: 10.1016/j.tree.2019.02.010
Wijngaarden, P. J., and Brakefield, P. M. (2001). Lack of response to artificial selection on the slope of reaction norms for seasonal polyphenism in the butterfly Bicyclus anynana. Heredity 87, 410–420. doi: 10.1046/j.1365-2540.2001.00933.x
Wijngaarden, P. J., Koch, P. B., and Brakefield, P. M. (2002). Artificial selection on the shape of reaction norms for eyespot size in the butterfly Bicyclus anynana: direct and correlated responses. J. Evol. Biol. 15, 290–300. doi: 10.1046/j.1420-9101.2002.00380.x
Williams, S. E., Shoo, L. P., Isaac, J. L., Hoffmann, A. A., and Langham, G. (2008). Towards an integrated framework for assessing the vulnerability of species to climate change. PLoS Biol. 6:e0060325. doi: 10.1371/journal.pbio.0060325
Windig, J. J., Brakefield, P. M., Reitsma, N., and Wilson, J. G. M. (1994). Seasonal polyphenism in the wild: survey of wing patterns in five species of Bicyclus butterflies in Malawi. Ecol. Entomol. 19, 285–298. doi: 10.1111/j.1365-2311.1994.tb00420.x
Woestmann, L., and Saastamoinen, M. (2016). The importance of trans-generational effects in lepidoptera. Curr. Zool. 62, 489–499. doi: 10.1093/cz/zow029
Yang, C. H., and Pospisilik, J. A. (2019). Polyphenism - A window into gene-environment interactions and phenotypic plasticity. Front. Genet. 10:132. doi: 10.3389/fgene.2019.00132
Keywords: developmental plasticity, thermal adaptation, multifactorial environments, environment-by-environment interactions, climate change
Citation: Rodrigues YK and Beldade P (2020) Thermal Plasticity in Insects’ Response to Climate Change and to Multifactorial Environments. Front. Ecol. Evol. 8:271. doi: 10.3389/fevo.2020.00271
Received: 01 June 2020; Accepted: 30 July 2020;
Published: 18 August 2020.
Edited by:
Mauro Santos, Autonomous University of Barcelona, SpainReviewed by:
Vincenzo Trotta, Università degli Studi della Basilicata, ItalyCopyright © 2020 Rodrigues and Beldade. This is an open-access article distributed under the terms of the Creative Commons Attribution License (CC BY). The use, distribution or reproduction in other forums is permitted, provided the original author(s) and the copyright owner(s) are credited and that the original publication in this journal is cited, in accordance with accepted academic practice. No use, distribution or reproduction is permitted which does not comply with these terms.
*Correspondence: Yara Katia Rodrigues, eXJvZHJpZ3Vlc0BpZ2MuZ3VsYmVua2lhbi5wdA==; Patr cia Beldade, cGJlbGRhZGVAZmMudWwucHQ=
Disclaimer: All claims expressed in this article are solely those of the authors and do not necessarily represent those of their affiliated organizations, or those of the publisher, the editors and the reviewers. Any product that may be evaluated in this article or claim that may be made by its manufacturer is not guaranteed or endorsed by the publisher.
Research integrity at Frontiers
Learn more about the work of our research integrity team to safeguard the quality of each article we publish.