- Department of Zoology, University of Otago, Dunedin, New Zealand
Animals adopt a range of avoidance strategies to reduce their exposure to parasites and the associated cost of infection. If strong selective pressures from parasites are sustained over many generations, avoidance strategies may gradually evolve from phenotypically plastic, or individually variable, to fixed, species-wide traits. Over time, host species possessing effective infection avoidance traits may lose parasite species. Indeed, if overcoming the avoidance strategies of a host species is too costly, i.e., if individuals of that species become too rarely encountered or difficult to infect, a generalist parasite may opt out of this particular arms race. From the host’s perspective, if avoidance traits are not costly or have been co-opted for other functions, they may persist in extant species even if ancestral parasites are lost, as signatures of past selection by parasites. Here, we develop the “ghost of parasitism past” hypothesis. We discuss how animal species with a lower number of parasite species than expected based on their ecological properties or phylogenetic affinities are a good starting point in the search for traces of past parasite-mediated selection. We then argue that the hypothesis explains the dynamic and inconsistent nature of the relationship between the expression of avoidance traits and relative infection risk in comparative analyses across host species. Finally, we propose some approaches to test the predictions of the hypothesis. Animal morphology and behavior show clear evidence of past selective pressures from predators; we argue that past selection from parasites has also left its imprint, though in more subtle ways.
Introduction
The idea that the non-consumptive effects of predators can match or even exceed those of their direct consumption of prey has gained much evidential support in recent years (Preisser et al., 2005; Suraci et al., 2016). In particular, the fear experienced by prey and manifested as distinct behavioral or physiological responses to avoid predation can be costly, leading to reductions in reproductive output and impacts on population dynamics (Clinchy et al., 2013; Zanette and Clinchy, 2019). Similarly, the fear (or disgust) of parasites and the associated costs of infection might cause hosts to adopt particular strategies that reduce the risk of infection (Buck et al., 2018; Weinstein et al., 2018). Parasite avoidance strategies include all steps involved in preventing infection, from early detection and recognition of parasites, all the way to active evasion of infective stages. These strategies are costly, for instance by causing animals to miss out on foraging, mating or socializing opportunities (Norris, 1999; Fritzsche and Allan, 2012; Kavaliers and Choleris, 2018). As we write these lines, the threat of infection by the virus responsible for the COVID-19 disease is causing major changes worldwide to our social behavior with major repercussions on the economy, employment security, and the availability of goods and services. Who then can doubt the costs associated with the fear of infection? Despite these costs, however, avoidance strategies must confer overall net fitness gains, or else they would not be maintained.
In addition to their immediate ecological consequences, the adoption of avoidance strategies by animals and other non-consumptive effects of parasites could have evolutionary implications. Given that avoidance strategies must have overall positive fitness effects, these benefits could drive the evolution of avoidance strategies from phenotypically plastic, or individually variable, to fixed species-wide traits. For this to happen, two conditions must be met. First, the risk of infection must be high enough to represent a real danger for any individual in the population. In other words, the prevalence of infection by a costly parasite, or the diversity of parasite species that may infect a host by a particular route, must be locally high enough to lead to a high probability of incurring a cost from parasite infection. Secondly, the high infection risk must persist over time, thus exerting strong selective pressures over several generations. Situations in which both conditions are met are likely to be common (Wilson et al., 2019), and we may therefore expect some of the traits of extant animals to be the product of past selection to avoid parasite infection. These traits may not be easy to identify, however, as they may now be associated with different functions, or so ubiquitous that they are assumed to have other origins. For example, sexual reproduction is so widespread among living organisms that its potential evolutionary maintenance as a defense against fast-evolving pathogens was long overlooked (Hamilton, 1980).
Here, we discuss how strong and sustained selection of avoidance strategies against parasites in the past may have left its mark in extant species, whether or not this signature is easily detectable. In a parallel with the idea that the ghost of competition past is still visible in the character displacement and non-overlapping niches of extant species living in sympatry (Connell, 1980), we propose that the ghost of parasitism past has shaped host evolution. As examples of evolutionary anachronisms (Barlow, 2000), many behavioral, physiological or morphological traits of extant species may be ascribed to past selective pressures from parasites, i.e., pressures that are no longer measurable. We first discuss how animal species that are nowadays used by a disproportionately low number of parasite species may offer clues in the search for traces of past parasite-mediated selection. Then, we argue that the relationship between the expression of avoidance traits and relative infection risk across host species can change over evolutionary time, and thereby explain the inconsistent results from comparative analyses that have attempted to link these variables. Finally, we acknowledge the challenges of demonstrating cause-and-effect in testing the “ghost of parasitism past” hypothesis, and propose different ways to overcome these difficulties. Our focus is on long-term evolution and interspecific differences, i.e., we look at variation in avoidance strategies among different species, and how this relates to the risks of infection they now face. We consider the abundance and pathogenicity of specific parasites, as well as parasite species richness, as measures of selective pressures imposed by parasites (Bordes and Morand, 2009).
Have Host Species With Few Parasites Evolved a Solution?
Have animal species with disproportionately few parasite species found the secret to parasite avoidance? Some species are exploited by fewer parasite species than what would be expected based on their ecological properties (e.g., body mass, population density, longevity, and social behavior) or phylogenetic affinities. For example, among seabirds with similar habitats, lifestyles and body sizes, members of the order Pelecaniformes (pelicans, gannets, boobies, and cormorants) consistently harbor fewer species of ectoparasitic lice than members of the orders Charadriiformes (gulls, skuas, and auks) and Procellariiformes (albatrosses and petrels) (Hughes and Page, 2007). Similarly, in anthropoid primates (monkeys and apes), even after controlling for uneven sampling effort, the species richness of helminth and protozoan parasites varied substantially among host species, with only a modest proportion of that variance explained by the combined effects of ecological variables such as body mass, population density or longevity (Nunn et al., 2003). Some primate species simply appear to be under-parasitized. Can the “ghost of parasitism past” hypothesis explain why some species have so few parasites? Do species with relatively fewer parasites possess intrinsic features that we are yet to recognize as parasite avoidance strategies? If so, what might these be?
Poulin et al. (2011b) used species-area relationship (SAR) models on several large datasets on parasite species richness in vertebrates to identify host species with more or fewer parasite species than expected based on sampling effort and body mass. Host species lying below the 80% confidence interval of the averaged SAR function were considered as hosts with disproportionately low numbers of parasites for their body mass. In most datasets, there was clear evidence of phylogenetic clustering, i.e., host species from the same family were more likely to all fall above, within or below the confidence interval of the SAR curve (Poulin et al., 2011b). Key ecological traits within a phylogenetic group also played a role. For instance, among shark species, the likelihood that a host species had a disproportionately low number of cestode parasites was influenced by the species’ median depth range, although in different ways depending on the family it belonged to Poulin et al. (2011b). Depth range is indeed known to be an important determinant of cestode species richness in sharks, although depth preferences are assumed to have evolved for other reasons, and not as a strategy to avoid parasite-laden prey (Randhawa and Poulin, 2010). However, if animals perceive their environment as a landscape of risk and move preferentially to safer zones where exposure to infection is lowest (Weinstein et al., 2018), we would expect some of these habitat preferences to have become fixed traits over evolutionary time.
As the physical environment represents a multi-dimensional space in which organisms occupy particular zones, diet, morphology, behavioral, and physiological traits also form continuums along which natural selection can position any given species based on net fitness benefits. If both axes of a two-dimensional space represent the full range of possible values for two behavioral traits, all potential behavioral responses to a detected infection risk can be visualized as a continuous landscape along which there are peaks, i.e., combinations of behavioral patterns corresponding to an elevated infection risk, and valleys representing safe behavioral patterns (Figure 1). Over time, in species selected to avoid pressures from parasites, natural selection may have narrowed down the range of possible responses displayed by a given host species to safe ones only. Effective parasite avoidance strategies could therefore be identified by carefully investigating the properties of animal species with unusually few parasites compared to related species with rich parasite faunas.
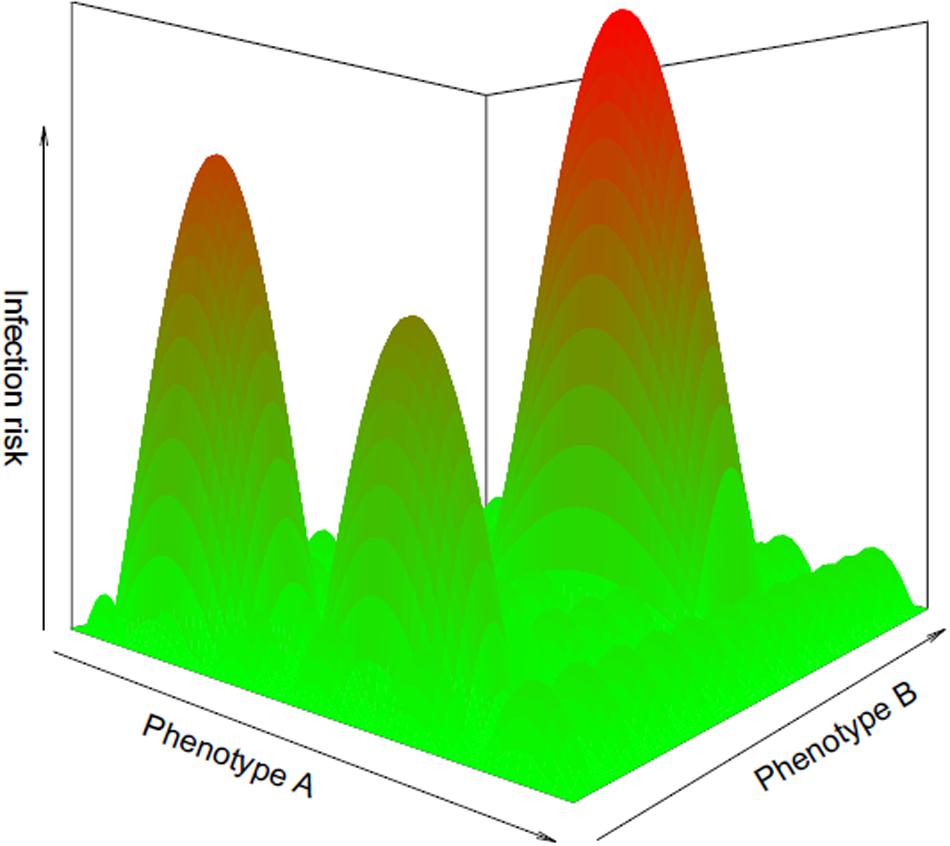
Figure 1. Phenotypic landscape of infection risk, illustrating the infection risk associated with all possible combinations of values for two hypothetical phenotypic traits, A and B. Red peaks correspond to phenotypes exposing individuals to a high risk of infection, whereas green valleys correspond to safe phenotypic strategies. Species whose phenotypes settle in valleys over evolutionary time may eventually lose parasite species.
Evolutionary Covariance of Avoidance Traits Versus Infection Risk
Could the fixation of parasite avoidance strategies, originally evolved out of “fear” and to counter immediate risks of infection, but later maintained because they serve other beneficial functions, obscure the relationship between host traits and parasite species richness? Many host traits, such as diet specialization, whether they are parasite avoidance strategies or coincidental determinants of parasite exposure, are expected to correlate with parasite species richness across host species. However, comparative analyses reveal no consistent correlations between diet breadth and parasite species richness across various groups of vertebrate hosts: the relationship is significant in some studies, but not others (e.g., Gregory et al., 1991; Watve and Sukumar, 1995; Sasal et al., 1997; Morand et al., 2000; Nunn et al., 2003). The same is true of many other host traits thought to be associated with parasite species richness (Kamiya et al., 2014; Morand, 2015).
We suggest that the relationship between parasite infection risk and the phenotypic expression of any given parasite avoidance trait may be reversed over evolutionary time (Figure 2). When a clade of hosts first encounters a new set of parasites, following a host shift or habitat invasion, host populations or host species facing the highest infection risk, i.e., the most parasite species, will evolve the strongest avoidance response. This should produce a positive relationship, across host populations or species, between infection risk and the degree to which avoidance strategies are expressed. However, this situation is dynamic over evolutionary time, as hosts and parasites are involved in a coevolutionary arms race. Just as hosts evolve parasite avoidance strategies, parasites will evolve counter-adaptations. Although, if the costs of overcoming the avoidance strategies of a host species are too high, a parasite may simply opt out of the race. Thus, if members of a host species become too rarely encountered or too difficult to infect, thanks to their avoidance strategies, selection may favor parasites that drop this host species from the range of hosts they can exploit, saving themselves the cost of species-specific adaptations against certain host defensive systems. Indeed, there are costs of being a generalist parasite, because maintaining adaptations to exploit multiple host species often limits performance on any of them: jacks of all trades are usually master of none (Straub et al., 2011; Arbiv et al., 2012; Pinheiro et al., 2016). Thus, short-term success at avoiding parasites could lead to a long-term reduction of the number of parasites actually exploiting a host species, and thus a negative relationship, across host populations or species, between infection risk and the expression of avoidance strategies (Figure 2). This may not only apply to traits playing a role pre-infection, such as avoidance strategies, but also to traits acting post-infection, such as those involved in immunocompetence like relative spleen sizes in birds (e.g., Morand and Poulin, 2000).
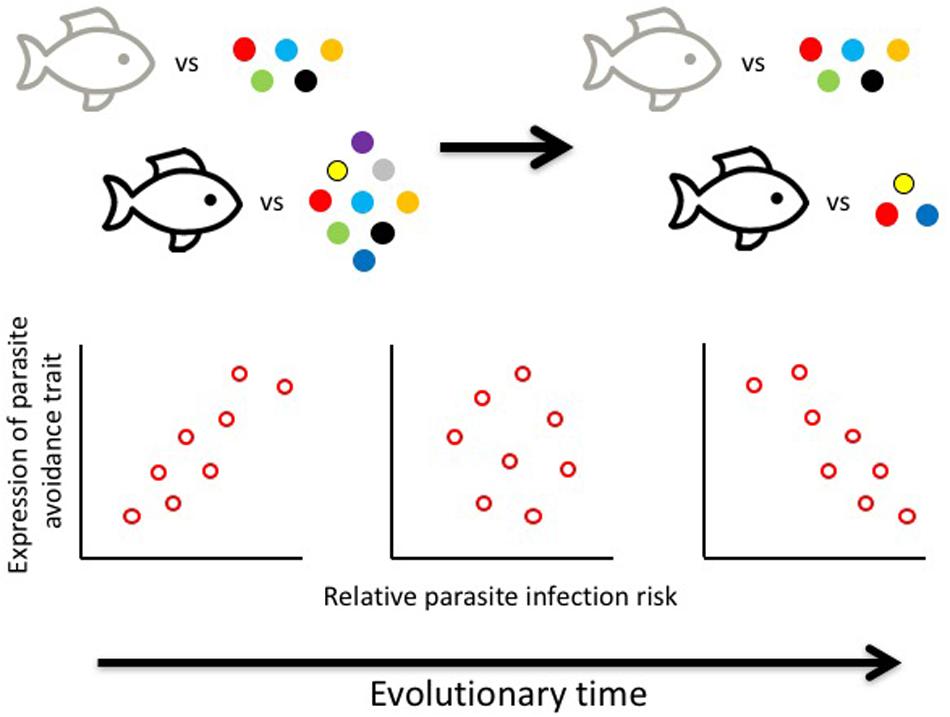
Figure 2. Evolutionary dynamic relationship between infection risk and avoidance traits, as it shifts from positive to negative over evolutionary time. Species facing multiple parasite species (colored points) are under stronger selection to develop more pronounced avoidance strategies (dark shape outline) than those facing few parasites; over time, however, strong avoidance strategies may lead to the loss of parasite species. The different points on the graphs represent either distinct host species, or distinct populations of the same host species. NB: Scale on x-axis shows relative risk (e.g., parasite species richness), and not absolute risk; it allows comparison among points on the same graph, but not among graphs.
Of course, the above scenario is not the only possible evolutionary direction. If a particular avoidance trait is costly and traded-off against other functions, and if pressure from infection risk is relaxed, we might expect the expression of the trait to be reduced with time. In contrast, if it is co-opted for other functions and continues to confer benefits, it may persist and become disconnected from its original parasite avoidance function. Or, if it is fitness neutral, the trait may persist even with no clear function. Nevertheless, the scenario we propose could explain the lack of consistent relationships found between parasite species richness and host traits thought to be associated with infection risk: the various studies focusing on different taxa may simply capture snapshots of different stages in a dynamic evolutionary process.
The Causality Chain
Any association among extant species between a particular trait and some measure of infection risk like parasite species richness can have at least two evolutionary explanations. Some host species may have few parasite species because that trait provides them with limited exposure, with the trait having evolved for other reasons or functions in the first place. Alternatively, the trait may have evolved to its full extent in those host species that faced a high infection risk and specifically for the purpose of lowering that risk, even if these hosts now experience a much lower infection risk. For instance, among Amazonian bats, omnivorous species have much higher infection levels by foodborne helminths than species that specialize strictly on nectar or fruits (Albuquerque et al., 2016). One possible explanation is that the narrow diet of a specialized forager could coincidentally protect it from exposure to many foodborne parasites, even if that diet has evolved for other ecological or physiological reasons. Alternatively, a forager living in an environment rich in foodborne parasites may have been selected for a narrow diet as a parasite avoidance strategy. How can we distinguish between these two scenarios?
Correlative evidence from field studies can only support the “ghost of parasitism past” hypothesis, but it cannot refute it. As an example, consider the link between fish migration and infection risk. In a field study of sympatric and congeneric species of galaxiid fishes in New Zealand, species that leave freshwater habitats and migrate downstream to spend their early life in coastal habitats incur significantly lower risks of infection by a range of trematode species, compared to their close relatives which do not migrate and reside in freshwaters for their whole lives (Poulin et al., 2012). There are clear benefits gained by fish that avoid trematode infection in early life: infection of young fish <40 mm in length causes malformations and greatly increases mortality (Kelly et al., 2010). So why do these fish migrate? Is the migratory behavior truly acting as an ongoing strategy to reduce exposure to parasites during a critical life stage? Or is it the legacy of an ancestral parasite avoidance strategy retained by selection for the other benefits it confers? Or did it evolve for completely different reasons and only coincidentally provides reduced infection risk as a side-effect? Theoretical models suggest that parasitism can indeed exert selective pressures strong enough to drive the evolution of migration, but only if it causes a reduction in prevalence by costly parasites rather than a reduction in the number of parasite species faced by the fish (Shaw et al., 2018, 2019).
Similar comparative studies among related species that differ in a key trait also hint at past selective pressures from parasites, with species possessing particular traits successfully escaping from parasitism. For instance, lepidopteran species with hairy caterpillars face a much lower risk of attack by parasitoid wasps than those with smooth caterpillars (Kageyama and Sugiura, 2016), as do species of gall wasps which produce ornate and bumpy galls compared to those that produce simpler galls (Bailey et al., 2009). The huge variation in behavioral and morphological defenses among insects (Gross, 1993) provides excellent material for tests of the “ghost of parasitism past” hypothesis.
Although intriguing, the results of comparative studies on extant species provide only hints of what may have happened over evolutionary time. Studies that focus on changes across long time scales would be more convincing, but these also have their limitations. In particular, we lack data on the strength of selective pressures exerted by parasites on extinct animals in the deep past. Data on infection risk or parasite species richness in extinct host species are almost impossible to obtain, except from glimpses obtained from coprolites (e.g., Wood et al., 2013) or traces left on fossils (e.g., Huntley et al., 2014). Therefore, we are blind to past selection pressures, just as we are blind to most parasite avoidance strategies of extinct animals involving behavior or other phenotypes that leave no fossil traces.
Some approaches nevertheless allow large-scale and convincing ways of testing the “ghost of parasitism past” hypothesis. One of them would be to test for covariation between the geographical distribution of a putative parasite avoidance trait and the distribution of past selective pressures from parasites, estimated as accurately as possible, among populations of the same or closely related species. For example, the sickle cell hemoglobin gene causes malformation of red blood cells and reduces their ability to bind and transport oxygen (Serjeant and Serjeant, 2001). The gene provides some resistance to malaria in heterozygous carriers, but causes sickle cell anemia and is usually fatal in individuals carrying two copies of the gene. Geographical variation in the frequency of the gene among human populations shows a strong association with the historical distribution of malaria endemicity, dating back to the times before any interventions or control measures were first implemented (Piel et al., 2010). Yet the gene still persists in human populations from areas where malaria is no longer a problem. This provides strong evidence that even where an otherwise deleterious anti-parasite trait is no longer needed nor beneficial in any other way, it can persist as a signature of past selective pressures from parasites. A similar approach could be used to determine whether the expression of any presumed parasite avoidance trait shows a spatial match with estimates of past selective pressures. Threespine sticklebacks, Gasterosteus aculeatus, may be the ideal system for such an approach. They have proven an excellent model system for studies of host-parasite interactions (Barber, 2013), the diversity and abundance of parasites vary widely among stickleback populations (Poulin et al., 2011a), and the genetic and phenotypic signature of selection pressures from parasites has proven tractable in stickleback populations (Brunner et al., 2017; Weber et al., 2017).
Alternatively, one might use a comparative approach across host species that vary in the expression of the presumed avoidance trait. Using dated phylogenies as a basis for the comparative analysis, it may be possible to reconstruct the ancestral origin and evolution of the presumed avoidance trait, and use this information to predict whether a positive or negative correlation would be expected between trait expression and relative parasite infection risk (see previous section). None of the approaches summarized above is ideal on its own, but in combination they offer a way forward to put the hypothesis to the test.
Conclusion
In this essay, we have argued that what begins as an adaptation providing immediate protection from infection may in the long run promote the loss of parasites and lead to a host species with fewer natural enemies. An inducible parasite avoidance mechanism showing variation among individuals could produce, if parasite pressures are sustained over many generations, fixed species-wide traits and/or an evolutionary niche shift. These can manifest in many ways. For instance, one could even argue that the excessive propensity for hygiene among people in industrialized countries is a legacy of pressures from parasites no longer plaguing human populations. In homage to previous hypotheses explaining niche segregation in the absence of measurable competition among sympatric species from the same guild (Connell, 1980), or anti-predator adaptations displayed by populations no longer facing predation (Peckarsky and Penton, 1988; Gliwicz and Jachner, 1992), we dub this the ghost of parasitism past.
Of course, some caveats apply to the arguments we propose. For instance, a strategy protecting hosts against a parasite that is no longer present may nevertheless persist if it also protects the host against other parasites. Alternatively, an avoidance strategy that serves against parasites that go extinct may be underpinned by the same neurobiological and physiological mechanisms that allow other avoidance strategies acting against other natural enemies that are still around; that strategy would therefore not necessarily be abandoned. The general avoidance of feces shown by many animals belongs to this category (Weinstein et al., 2018). The “ghost of parasitism past” hypothesis thus requires a more nuanced interpretation than what we presented in some of the earlier examples.
Due to the nature of the phenomenon, the “ghost of parasitism past” hypothesis cannot be tested within an experimental framework allowing strong inference of causality. However, we provide plausible examples of situations where putative avoidance strategies may now be species characteristics associated with fewer parasite species than one would expect based on a host species’ other ecological features or phylogenetic affinities. We also propose some analytical approaches which, though not perfect, can serve to support the hypothesis. The morphology and behavior of extant animals display an array of adaptations that evolved in response to sustained pressures from predators, ranging from cryptic coloration and spines, to vigilance and alarm calls. Some of these persist in populations where predation pressures have been relaxed. We argue that similar adaptations against past pressures from parasites must be widespread in the animal kingdom. These are perhaps more subtle and more difficult to identify than adaptations against predators, but they are no less worthy of study.
Author Contributions
All authors actively participated in developing the concepts presented in the manuscript. RP wrote the manuscript, with input from all other authors.
Funding
JB, DA, J-FD, AF, EP, and BR were funded by Doctoral Scholarships from the University of Otago. J-FD was also funded by a scholarship (PGSD3-530445-2019) from the Natural Sciences and Engineering Research Council of Canada (NSERC).
Conflict of Interest
The authors declare that the research was conducted in the absence of any commercial or financial relationships that could be construed as a potential conflict of interest.
Acknowledgments
We thank Janet Koprivnikar for inviting us (on behalf of the other editors) to contribute to this special issue. We also thank Tommy Leung and Chris Selbach for comments on an earlier version of the manuscript.
References
Albuquerque, A. C. A., Moraes, M. F. D., Silva, A. C., Lapera, I. M., Tebaldi, J. H., and Hoppe, E. G. L. (2016). Helminth fauna of chiropterans in Amazonia: biological interactions between parasite and host. Parasitol. Res. 115, 3229–3237. doi: 10.1007/s00436-016-5085-3
Arbiv, A., Khokhlova, I. S., Ovadia, O., Novoplansky, A., and Krasnov, B. R. (2012). Use it or lose it: reproductive implications of ecological specialization in a haematophagous ectoparasite. J. Evol. Biol. 25, 1140–1148. doi: 10.1111/j.1420-9101.2012.02499.x
Bailey, R., Schönrogge, K., Cook, J. M., Melika, G., Csoka, G., Thuroczy, C., et al. (2009). Host niches and defensive extended phenotypes structure parasitoid wasp communities. PLoS Biol. 7:e1000179. doi: 10.1371/journal.pbio.1000179
Barber, I. (2013). Sticklebacks as model hosts in ecological and evolutionary parasitology. Trends Parasitol. 29, 556–566. doi: 10.1016/j.pt.2013.09.004
Bordes, F., and Morand, S. (2009). Parasite diversity: an overlooked metric of parasite pressures? Oikos 118, 801–806. doi: 10.1111/j.1600-0706.2008.17169.x
Brunner, F. S., Anaya-Rojas, J. M., Matthews, B., and Eizaguirre, C. (2017). Experimental evidence that parasites drive eco-evolutionary feedbacks. Proc. Natl. Acad. Sci. U.S.A. 114, 3678–3683. doi: 10.1073/pnas.1619147114
Buck, J. C., Weinstein, S. B., and Young, H. S. (2018). Ecological and evolutionary consequences of parasite avoidance. Trends Ecol. Evol. 33, 619–632. doi: 10.1016/j.tree.2018.05.001
Clinchy, M., Sheriff, M. J., and Zanette, L. Y. (2013). Predator-induced stress and the ecology of fear. Funct. Ecol. 27, 56–65. doi: 10.1111/1365-2435.12007
Connell, J. H. (1980). Diversity and the coevolution of competitors, or the ghost of competition past. Oikos 35, 131–138.
Fritzsche, A., and Allan, B. F. (2012). The ecology of fear: Host foraging behavior varies with the spatio-temporal abundance of a dominant ectoparasite. Ecohealth 9, 70–74. doi: 10.1007/s10393-012-0744-z
Gliwicz, Z. M., and Jachner, A. (1992). Diel migrations of juvenile fish: a ghost of predation past or present. Arch. Hydrobiol. 124, 385–410.
Gregory, R. D., Keymer, A. E., and Harvey, P. H. (1991). Life history, ecology and parasite community structure in Soviet birds. Biol. J. Linn. Soc. 43, 249–262. doi: 10.1111/j.1095-8312.1991.tb00597.x
Gross, P. (1993). Insect behavioral and morphological defences against parasitoids. Annu. Rev. Entomol. 38, 251–273. doi: 10.1146/annurev.en.38.010193.001343
Hughes, J., and Page, R. D. M. (2007). Comparative tests of ectoparasite species richness in seabirds. BMC Evol. Biol. 7:227. doi: 10.1186/1471-2148-7-227
Huntley, J. W., Fürsich, F. T., Alberti, M., Hethke, M., and Liu, C. (2014). A complete Holocene record of trematode-bivalve infection and implications for the response of parasitism to climate change. Proc. Nat. Acad. Sci. U.S.A. 111, 18150–18155. doi: 10.1073/pnas.1416747111
Kageyama, A., and Sugiura, S. (2016). Caterpillar hairs as an anti-parasitoid defence. Sci. Nat. 103, 86.
Kamiya, T., O’Dwyer, K., Nakagawa, S., and Poulin, R. (2014). What determines species richness of parasitic organisms? A meta-analysis across animal, plant and fungal hosts. Biol. Rev. 89, 123–134. doi: 10.1111/brv.12046
Kavaliers, M., and Choleris, E. (2018). The role of social cognition in parasite and pathogen avoidance. Philos. Trans. R. Soc. B 373:20170206. doi: 10.1098/rstb.2017.0206
Kelly, D. W., Thomas, H., Thieltges, D. W., Poulin, R., and Tompkins, D. M. (2010). Trematode infection causes malformations and population effects in a declining New Zealand fish. J. Anim. Ecol. 79, 445–452. doi: 10.1111/j.1365-2656.2009.01636.x
Morand, S. (2015). (macro-)Evolutionary ecology of parasite diversity: from determinants of parasite species richness to host diversification. Int. J. Parasitol. Parasit. Wildl. 4, 80–87. doi: 10.1016/j.ijppaw.2015.01.001
Morand, S., Cribb, T. H., Kulbicki, M., Chauvet, C., Dufour, V., Faliex, E., et al. (2000). Determinants of endoparasite species richness of New Caledonian Chaetodontidae. Parasitology 121, 65–73. doi: 10.1017/s0031182099006058
Morand, S., and Poulin, R. (2000). Nematode parasite species richness and the evolution of spleen size in birds. Can. J. Zool. 78, 1356–1360. doi: 10.1139/z00-076
Norris, K. (1999). A trade-off between energy intake and exposure to parasites in oystercatchers feeding on a bivalve mollusc. Proc. R. Soc. B 266, 1703–1709. doi: 10.1098/rspb.1999.0835
Nunn, C. L., Altizer, S., Jones, K. E., and Sechrest, W. (2003). Comparative tests of parasite species richness in primates. Am. Nat. 162, 597–614. doi: 10.1086/378721
Peckarsky, B. L., and Penton, M. A. (1988). Why do Ephemerella nymphs scorpion posture: a “ghost of predation past”? Oikos 53, 185–193.
Piel, F. B., Patil, A. P., Howes, R. E., Nyangiri, O. A., Gething, P. W., Williams, T. N., et al. (2010). Global distribution of the sickle cell gene and geographical confirmation of the malaria hypothesis. Nat. Commun. 1:104.
Pinheiro, R. B. P., Félix, G. M. F., Chaves, A. V., Lacorte, G. A., Santos, F. R., Braga, E. M., et al. (2016). Trade-offs and resource breadth processes as drivers of performance and specificity in a host-parasite system: a new integrative hypothesis. Int. J Parasitol. 46, 115–121. doi: 10.1016/j.ijpara.2015.10.002
Poulin, R., Blanar, C. A., Thieltges, D. W., and Marcogliese, D. J. (2011a). The biogeography of parasitism in sticklebacks: distance, habitat differences and the similarity in parasite occurrence and abundance. Ecography 34, 540–551. doi: 10.1111/j.1600-0587.2010.06826.x
Poulin, R., Guilhaumon, F., Randhawa, H. S., Luque, J. L., and Mouillot, D. (2011b). Identifying hotspots of parasite diversity from species-area relationships: host phylogeny versus host ecology. Oikos 120, 740–747. doi: 10.1111/j.1600-0706.2010.19036.x
Poulin, R., Closs, G. P., Lill, A. W. T., Hicks, A. S., Herrmann, K. K., and Kelly, D. W. (2012). Migration as an escape from parasitism in New Zealand galaxiid fishes. Oecologia 169, 955–963. doi: 10.1007/s00442-012-2251-x
Preisser, E. L., Bolnick, D. I., and Benard, M. F. (2005). Scared to death? The effects of intimidation and consumption in predator-prey interactions. Ecology 87, 501–509. doi: 10.1890/04-0719
Randhawa, H. S., and Poulin, R. (2010). Determinants of tapeworm species richness in elasmobranch fishes: untangling environmental and phylogenetic influences. Ecography 33, 866–877. doi: 10.1111/j.1600-0587.2010.06169.x
Sasal, P., Morand, S., and Guégan, J. F. (1997). Determinants of parasite species richness in Mediterranean marine fish. Mar. Ecol. Progr. Ser. 149, 61–71. doi: 10.3354/meps149061
Shaw, A. K., Craft, M. E., Zuk, M., and Binning, S. A. (2019). Host migration strategy is shaped by forms of parasite transmission and infection cost. J. Anim. Ecol. 88, 1601–1612. doi: 10.1111/1365-2656.13050
Shaw, A. K., Sherman, J., Barker, F. K., and Zuk, M. (2018). Metrics matter: the effect of parasite richness, intensity and prevalence on the evolution of host migration. Proc. R. Soc. B 285:20182147. doi: 10.1098/rspb.2018.2147
Straub, C. S., Ives, A. R., and Gratton, C. (2011). Evidence for a trade-off between host-range breadth and host-use efficiency in aphid parasitoids. Am. Nat. 177, 389–395. doi: 10.1086/658177
Suraci, J. P., Clinchy, M., Dill, L. M., Roberts, D., and Zanette, L. Y. (2016). Fear of large carnivores causes a trophic cascade. Nat. Commun. 7:10698.
Watve, M. G., and Sukumar, R. (1995). Parasite abundance and diversity in mammals: correlates with host ecology. Proc. Nat. Acad. Sci. U.S.A. 92, 8945–8949. doi: 10.1073/pnas.92.19.8945
Weber, J. N., Kalbe, M., Shim, K. C., Erin, N. I., Steinel, N. C., Ma, L., et al. (2017). Resist globally, infect locally: a transcontinental test of adaptation by stickleback and their tapeworm parasite. Am. Nat. 189, 43–57. doi: 10.1086/689597
Weinstein, S. B., Buck, J. C., and Young, H. S. (2018). A landscape of disgust. Science 359, 1213–1214. doi: 10.1126/science.aas8694
Wilson, K., Fenton, A., and Tompkins, D. (2019). Wildlife Disease Ecology. Cambridge: Cambridge University Press.
Wood, J. R., Wilmshurst, J. M., Rawlence, N. J., Bonner, K. I., Worthy, T. H., Kinsella, J. M., et al. (2013). A megafauna’s microfauna: gastrointestinal parasites of New Zealand’s extinct moa (Aves: Dinornithiformes). PLoS One 8:e57315. doi: 10.1371/journal.pone.0057315
Keywords: avoidance behavior, comparative analysis, infection risk, parasite-mediated selection, parasite species richness, selection pressure
Citation: Poulin R, Bennett J, de Angeli Dutra D, Doherty J-F, Filion A, Park E and Ruehle B (2020) Evolutionary Signature of Ancient Parasite Pressures, or the Ghost of Parasitism Past. Front. Ecol. Evol. 8:195. doi: 10.3389/fevo.2020.00195
Received: 09 April 2020; Accepted: 29 May 2020;
Published: 23 June 2020.
Edited by:
Lien Luong, University of Alberta, CanadaReviewed by:
Martin Kavaliers, University of Western Ontario, CanadaTommy Leung, University of New England, Australia
Copyright © 2020 Poulin, Bennett, de Angeli Dutra, Doherty, Filion, Park and Ruehle. This is an open-access article distributed under the terms of the Creative Commons Attribution License (CC BY). The use, distribution or reproduction in other forums is permitted, provided the original author(s) and the copyright owner(s) are credited and that the original publication in this journal is cited, in accordance with accepted academic practice. No use, distribution or reproduction is permitted which does not comply with these terms.
*Correspondence: Robert Poulin, cm9iZXJ0LnBvdWxpbkBvdGFnby5hYy5ueg==
†ORCID: Robert Poulin, orcid.org/0000-0003-1390-1206; Jerusha Bennett, orcid.org/0000-0003-1037-894X; Daniela de Angeli Dutra, orcid.org/0000-0003-2341-2035; Jean-François Doherty, orcid.org/0000-0003-4766-9417; Antoine Filion, orcid.org/0000-0003-1198-3017; Eunji Park, orcid.org/0000-0002-5087-2398; Brandon Ruehle, orcid.org/0000-0002-9898-3169