- 1Hawai‘i Institute of Marine Biology, University of Hawai‘i at Mānoa, Kaneohe, HI, United States
- 2Sea Grant College Program, University of Hawai‘i at Mānoa, Honolulu, HI, United States
- 3Department of Biology, University of Pennsylvania, Philadelphia, PA, United States
Marine heat waves instigated by anthropogenic climate change are causing increasingly frequent and severe coral bleaching events that often lead to widespread coral mortality. While community-wide increases in coral mortality following bleaching events have been documented on reefs around the world, the ecological consequences for conspecific individual colonies exhibiting contrasting phenotypes during heat stress (e.g., bleached vs. not bleached) are not well understood. Here we describe the ecological outcomes of the two dominant reef-building coral species in Kāne‘ohe Bay, Hawai‘i, Montipora capitata and Porites compressa, by monitoring the fates of individuals that exhibited either a bleaching susceptible phenotype (bleached) or resistant phenotype (non-bleached) following the second of two consecutive coral bleaching events in Hawai‘i in 2015. Conspecific pairs of adjacent bleaching susceptible vs. resistant corals were tagged on patch reefs in two regions of Kāne‘ohe Bay with different seawater residence times and terrestrial influence. The ecological consequences (symbiont recovery and mortality) were monitored for 2 years following the peak of the bleaching event. Bleaching susceptible corals suffered higher partial mortality than bleaching resistant corals of the same species in the first 6 months following heat stress. Surprisingly, P. compressa had greater resilience following bleaching (faster pigment recovery and lower post-bleaching mortality) than M. capitata, despite having less resistance to bleaching (higher bleaching prevalence and severity). These differences indicate that bleaching susceptibility of a species is not always a good predictor of mortality following a bleaching event. By tracking the fate of individual colonies of resistant and susceptible phenotypes, contrasting ecological consequences of heat stress were revealed that were undetectable at the population level. Furthermore, this approach revealed individuals that underwent particularly rapid recovery from mortality, including some colonies over a meter in diameter that recovered all live tissue cover from >60% partial mortality within just 1 year. These coral pairs (44 pairs of each species) continue to be maintained and monitored in the field, serving as a “living library” for future investigations on the ecology and physiology of coral bleaching.
Introduction
Ocean warming due to anthropogenic climate change has caused an increase in the frequency and severity of coral bleaching, a visually striking stress response where the coral host expels its endosymbiotic algae (dinoflagellates of the family Symbiodiniaceae) thus revealing the white coral skeleton beneath the translucent animal tissue (Gates et al., 1992; Putnam et al., 2017). In this nutritional symbiosis, tropical corals are obligate partners that require the algae for the majority of their energy (Muscatine and Porter, 1977; Muller-Parker et al., 2015). Because of coral dependence on this partnership, prolonged heat waves that cause sustained coral bleaching can lead to depletion of the host’s energy supply and reserves (Grottoli et al., 2004; Rodrigues and Grottoli, 2007; Imbs and Yakovleva, 2012; Wall et al., 2019). This stress can elicit a variety of sublethal effects, including declines in growth and reproduction (Ward et al., 2000; Baird and Marshall, 2002; Baker et al., 2008; Hughes et al., 2019a), and at worst can result in widespread coral mortality (Loya et al., 2001; McClanahan, 2004; Baker et al., 2008; Eakin et al., 2010; Hughes et al., 2017; Sully et al., 2019). The loss of live coral cover is often followed by rapid erosion of the structural framework of the reef (Couch et al., 2017; Hughes et al., 2018b; Fordyce et al., 2019; Leggat et al., 2019), reducing habitat complexity (Magel et al., 2019) and negatively impacting the diversity of the broader reef community (e.g., fish (Pratchett et al., 2011; Darling et al., 2017; Richardson et al., 2018). Coral mortality following bleaching can also alter the structure of the coral community itself, as bleaching-susceptible species are lost from the community (Loya et al., 2001; McClanahan, 2004; Baker et al., 2008; Bahr et al., 2017; Hughes et al., 2017) while stress-tolerant species remain (Edmunds, 2018; Hughes et al., 2018b) and “weedy” genera that are better suited for rapid recovery following bleaching become dominant (Darling et al., 2012; Edmunds, 2018). These changes in community composition alter the ecological function of the reef (Alvarez-Filip et al., 2013), which alongside the structural degradation following bleaching leads to declines in ecosystem goods and services ranging from fisheries production to coastal protection and tourism (Costanza et al., 2014).
The susceptibility of a reef system to coral bleaching during a marine heat wave depends on a combination of physical and biological factors (Swain et al., 2016). Differences in the local microenvironment (e.g., flow, turbidity, surface reflectance, internal waves, etc.) can ameliorate or exacerbate the magnitude of heat stress and lead to differential bleaching and mortality on nearby reefs (Anthony et al., 2007; Wyatt et al., 2019). Local temperature dynamics also can influence coral bleaching by altering the physiological tolerance of coral populations to heat stress. For example, corals exposed to high diel temperature variability often have higher heat tolerance and greater resistance to bleaching than nearby conspecifics in more stable regimes (Putnam and Edmunds, 2011; Palumbi et al., 2014; Schoepf et al., 2015, 2019; Barshis et al., 2018). These data indicate that environmental history of individuals and populations plays a significant role in determining coral responses to stress (Hughes et al., 2019b), and is likely driven by a combination of both acclimatization (Bay et al., 2013; Bay and Palumbi, 2015; Kenkel and Matz, 2016) and adaptation (Barshis et al., 2013, 2018; Palumbi et al., 2014; Matz et al., 2018). On a global scale, there is recent evidence that the temperature threshold for coral bleaching has risen in correspondence with global warming, suggesting widespread acclimatization and/or adaptation (Coles et al., 2018; DeCarlo et al., 2019). Alternatively, differences in the composition of coral communities between reefs can also influence bleaching extent due to species-specific differences in thermal tolerance (Rowan et al., 1997; Berkelmans and Van Oppen, 2006; Sampayo et al., 2008; Swain et al., 2016; Edmunds, 2018). Indeed, the global loss of many heat sensitive coral species from reef communities (e.g., Loya et al., 2001; McClanahan, 2004; Hughes et al., 2018b; Kim et al., 2019), may be a contributing factor in rising bleaching thresholds. Similarly, intraspecific diversity may also contribute to changes in the bleaching threshold if tolerant genotypes persist in populations and sensitive genotypes are lost. While this would promote reef resistance to bleaching, the ecological consequences of corresponding reductions in genetic diversity remain to be seen.
The effect of intraspecific diversity in bleaching susceptibility (i.e., presence of individuals with contrasting bleached vs. not bleached phenotypes) on downstream ecological outcomes is not well understood, despite an abundance of studies concluding that interspecific differences in bleaching susceptibility are predictive of mortality such that species resistant to bleaching have lower mortality than susceptible species (Loya et al., 2001; McClanahan, 2004; Baker et al., 2008; Bahr et al., 2017; Hughes et al., 2017). Kāne‘ohe Bay, Hawai‘i, located on the northeast coast of O‘ahu, is an opportune system for investigating this question of how intraspecific variability in coral responses to coral bleaching events driven by climate change influence coral survival. The two dominant reef-building coral species in the bay, Montipora capitata and Porites compressa, both exhibit differences in heat stress responses within and between species during bleaching (Grottoli et al., 2006; Cunning et al., 2016; Wall et al., 2019). Differences in symbiont associations and nutritional plasticity are hypothesized to influence bleaching resistance (ability to withstand heat stress without bleaching) and resilience (ability to recover from bleaching) between these two species (Grottoli et al., 2006; Cunning et al., 2016; Wall et al., 2019), while within M. capitata the species of symbionts associated with different individual colonies contributes to differences in heat tolerance (Cunning et al., 2016). However, the influence of intraspecific variation in bleaching susceptibility on ecological outcomes following bleaching events remains unexplored. Understanding how intraspecific variation influences differential outcomes following heat stress is critical for understanding how the increasing frequency and severity of coral bleaching events will impact the function of these important ecosystems.
To better understand how individual colony responses to heat stress can influence ecological outcomes following a coral bleaching event, we conducted a 2-year monitoring study to characterize the recovery dynamics of bleaching susceptible and bleaching resistant coral colonies of M. capitata and P. compressa following a regional bleaching event in Kāne‘ohe Bay in 2015. Hawai‘i experienced anomalously high seawater temperatures in late summer (August–September) of 2015, resulting in widespread coral bleaching throughout the region (Bahr et al., 2017). This was the first consecutive coral bleaching event ever observed on Hawaiian reefs, occurring 1 year following a regional bleaching event in 2014 (Bahr et al., 2015). A total of 22 conspecific pairs of adjacent corals with contrasting bleaching phenotypes (bleaching susceptible or bleaching resistant) of each species were tagged and georeferenced at each of two reefs in the bay with different environmental conditions. Coral pigmentation recovery and mortality were monitored periodically over the following 2 years to examine how bleaching influences the ecological trajectories within and between species. These colonies are visited on a semiannual basis to maintain visibility of the tags for the purpose of establishing these corals as a living library archived in situ for use in future research on coral bleaching mechanisms, thermal physiology, and climate change resilience.
Materials and Methods
Site Selection and Characterization
This study was initiated during the peak of the 2015 coral bleaching event in Kāne‘ohe Bay, O‘ahu. The lagoon of Kāne‘ohe Bay consists of a shallow network of fringing and patch reefs protected by a barrier reef (Bahr et al., 2015), which restricts seawater exchange with the open ocean leading to reefs within the bay with high and low seawater residence times (Lowe et al., 2009). Two patch reefs dominated by the reef-building corals Montipora capitata and Porites compressa from two different regions of the bay were selected for this study: (1) Inner Lagoon region: patch reef 4 (PR4; 21.4339°N, 157.7984°W), an inshore reef with relatively high terrestrial influence (e.g., sedimentation, fresh water and nutrient run-off) and long (>30 days) seawater residence time (Lowe et al., 2009) and (2) Outer Lagoon region: patch reef 13 (PR13; 21.4515°N, 157.7966°W), a seaward reef with less terrestrial influence and short (<24 h) seawater residence time (Lowe et al., 2009; Figures 1A,B). Temperature loggers (Onset U22 Hobo; 0.21°C accuracy; 0.02°C resolution) were deployed on the benthos of each reef at 2–3 m depth on September 24, 2015, and seawater temperature was recorded in 15-minute intervals. The loggers were cross calibrated in an aquarium prior to deployment. Temperature data were collected through October 6, 2016 at the Inner Lagoon reef, and through July 20, 2016 at the Outer Lagoon reef. Because these loggers were not deployed until after the peak of the heatwave, data from nearby monitoring stations within each region were used to determine if there were any differences in cumulative heat stress between the two regions. These data were collected from PR12, located ∼100 m from PR13 in Outer Lagoon (Figure 1D and Table 1; data courtesy of the Division of Aquatic Resources of the State of Hawai‘i1), and PR1 (Coconut Island; data from PACIOOS2), in the same Inner Lagoon flow regime as PR4. Overlapping time series data showed seawater temperatures were similar and highly correlated within each lagoon region (Supplementary Figure S1). Degree heating weeks (DHW) were calculated for the Inner and Outer lagoon reefs from the PR1 and PR12 data, respectively, according to the methods described by Liu et al. (2013) with minor modification. Specifically, DHW were calculated as the hourly accumulation of temperatures ≥1°C above the local mean monthly maximum seawater temperature (MMM) in Kāne‘ohe Bay (28.0°C; Jury and Toonen, 2019), which is ∼1°C higher than the MMM of the reefs of the Main Hawaiian Islands (Jokiel and Brown, 2004; Jury and Toonen, 2019).
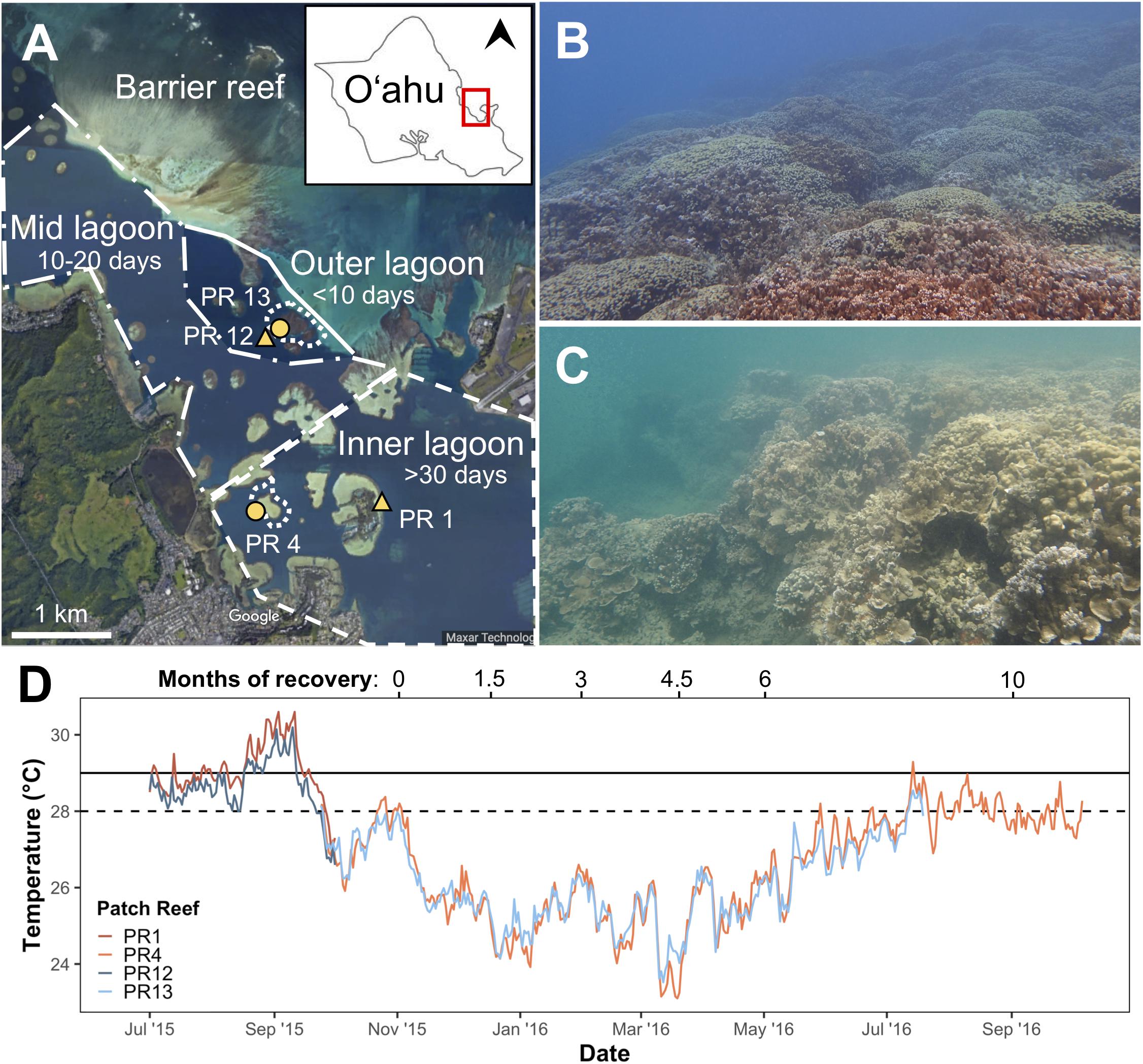
Figure 1. (A) Map of the southern region of Kāne‘ohe Bay, O‘ahu. Inset shows the island of O‘ahu, with north indicated by the arrowhead and the red square indicating the southern region of Kāne‘ohe Bay enlarged in detail. Distinct hydrodynamic regimes within the lagoon are indicated by the polygons: the dashed line surrounds the Inner Lagoon region where seawater residence times are >30 days; the dash-dot line surrounds the Mid Lagoon region where seawater residence times are 10–20 days; the solid line surrounds the Outer Lagoon region where seawater residence times are <10 days (from Lowe et al., 2009). Yellow symbols indicate locations of in situ temperature loggers. Representative images of each reef are shown for (B) Outer lagoon (PR13) and (C) Inner lagoon (PR4). (D) Maximum daily temperatures at each reef. Dashed horizontal line indicates local MMM; solid horizontal line indicates coral bleaching threshold (MMM+1).
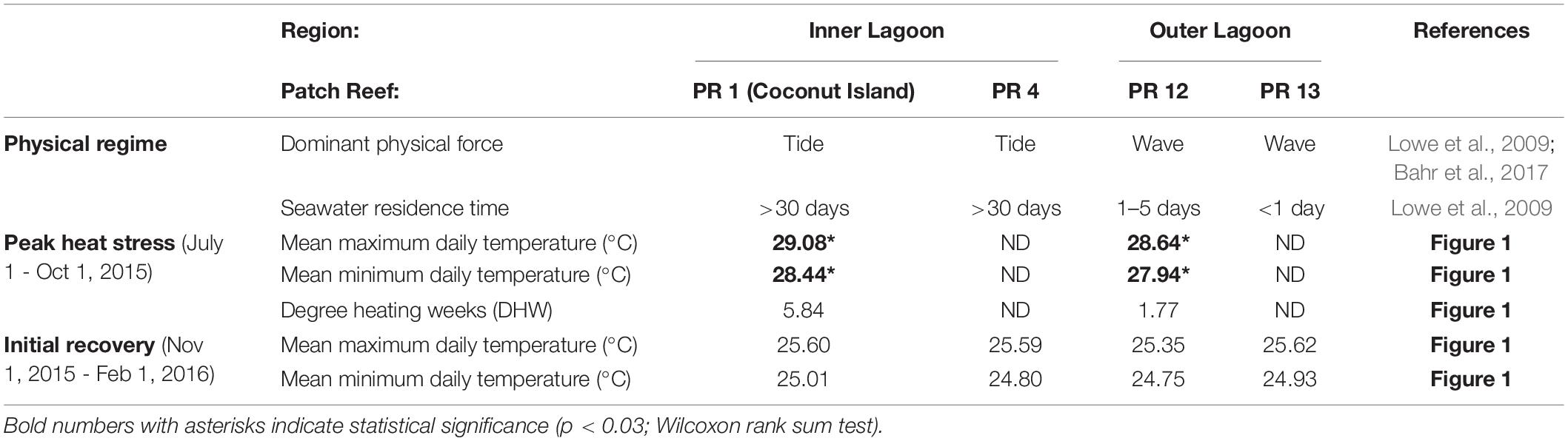
Table 1. Summary of seawater hydrodynamics and temperature conditions within the Inner and Outer lagoon regions in Kāne‘ohe Bay, Hawai‘i during the peak of the heat stress event and the initial recovery period.
Characterization of Benthic Community Composition and Coral Bleaching Responses
Surveys were performed to assess benthic community composition and coral bleaching responses during the 2015 bleaching event, and again after 1.5, 4, 10, and 18–19 months of recovery. Four surveys were conducted at each site for each time point along the reef slope in parallel to the reef crest at a depth of 2 m (± 1 m). Each survey was carried out along a 40 m transect laid parallel to the reef crest. A photoquadrat (0.33 m2) was placed on the benthos and images were taken every 2 m along the transect using an underwater camera (Canon PowerShot or Olympus TG5). Images were analyzed using Coral Point Count with Excel extensions (CPCe)3 (100 points per image). Corals were identified to species, and bleaching severity was recorded. Bleaching severity of the coral tissue under each point was assessed visually, and categorized as either white (severely bleached), pale (moderately bleached), or fully pigmented (not bleached). Bleaching prevalence was calculated as the proportion of live coral affected by severe to moderate bleaching (i.e., cumulative proportion of white or pale out of total). All other organisms were classified into functional groups (turf algae, crustose coralline algae (CCA), macroalgae, sediment and sand, sponges).
Monitoring of Bleaching Resistant and Susceptible Corals
Individual coral colonies of M. capitata and P. compressa with contrasting bleaching phenotypes were identified at the peak of the bleaching event from late September through the first week of November 2015. Bleaching resistant corals were defined as those that remained pigmented during the bleaching event, while bleaching susceptible corals were defined as those that appeared completely white. All corals were located along the edge of the reef crest (approximately 1 m depth) and down the reef slope (up to approximately 3 m depth). A plastic cattle tag with a unique identification number was attached to each colony, then the colony was photographed and its GPS location was recorded. All tagged colonies were conspecific pairs of colonies with contrasting bleaching phenotypes that were located adjacent to one another on the reef (e.g., Figure 2). A total of 22 pairs (44 colonies) of each species were tagged at each of two reefs (PR4 in the Inner Lagoon and PR13 in the Outer Lagoon; Supplementary Data S1). This paired design eliminated the potential for differences in the local microenvironment experienced by one phenotype but not the other (e.g., light intensity, flow) to confound interpretation of differences in their bleaching susceptibility. This paired design is also powerful because it can distinguish between mortality related to heat stress (both phenotypes) and mortality related to symbiont loss (bleached phenotypes). Bleaching recovery (where applicable) and partial mortality of tagged individuals were monitored every ∼6 weeks for the first 6 months following the bleaching event, then once every 6 months up to 24 months following the bleaching event. At each time point, colonies were photographed and visual observations of their bleaching status were recorded on a three-point scale (as in Guest et al., 2016; Coles et al., 2018; Ritson-Williams and Gates, 2020). Corals were given a color score based on their visual color: (1) white (>80% of colony white with no visible pigmentation); (2) pale (>10% colony affected by pigment loss); or (3) fully pigmented (<10% colony with any pale coloration). Partial mortality was quantified in 20% intervals (0–100%) from the photographs. The mean color scores and partial mortality for each species were calculated for each bleaching phenotype and site at each time point. For colonies with a missing observation, mortality scores were estimated using the mortality score of the previous time point. This is a conservative estimate of mortality (i.e., minimum possible mortality at that time point), and in most cases mortality scores did not change surrounding the missing observation. Missing observations of color scores were never interpolated across the time series due to the possibility for rapid loss or recovery of pigmentation.
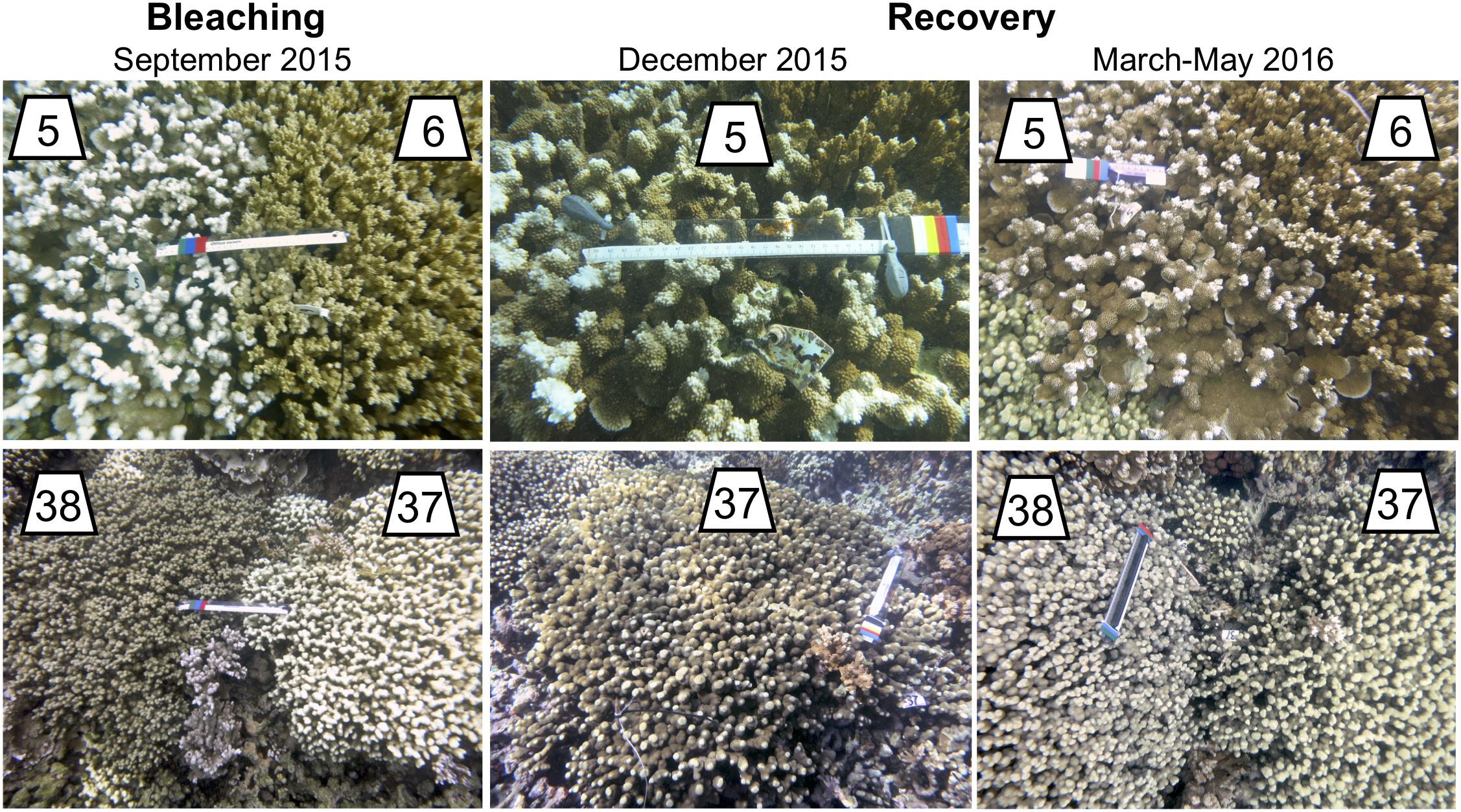
Figure 2. Representative images of tagged bleached and non-bleached corals. Top row: M. capitata pairs 5 and 6; Bottom row: P. compressa pairs 37 and 38. Left column shows pairs during the bleaching event (Sept. 2015). Center column shows the bleaching susceptible colony of the pair after 1.5 months of recovery (Dec. 2015). Right column shows the same pairs after 3–6 months of recovery [Mar. 2016 (colonies 5 and 6); May 2016 (colonies 37 and 38)].
Statistical Analyses
Statistical analyses were conducted in R and RStudio v. 3.6.1 (R Core Team, 2019). Seawater temperature means were compared using Wilcoxon rank sum tests. Linear mixed effects models (lme4 package, Bates et al., 2015) were used to analyze four different response variables from the benthic survey time series: (1) proportion of live coral cover, (2) prevalence of bleached coral cover [proportion of coral cover showing symptoms of bleaching (white or pale)], (3) prevalence of severely bleached coral cover (white only), and (4) bleaching severity (proportion of symptomatic live coral exhibiting severe bleaching). We hypothesized that corals with different bleaching phenotypes would recover at different rates and that these rates would differ between reefs, and therefore our models reflect a priori hypotheses. Survey depth was included as a random intercept. For coral cover, lagoon and time point were the main effects. For coral bleaching prevalence and severity, we used a full factorial design with coral species, lagoon, and time point as the main effects. To test hypotheses about the differential recovery of individually monitored bleaching susceptible versus bleaching resistant corals, we used linear mixed effects models to examine how (1) mean color score and (2) mean tissue loss in each species differed over time, lagoon, and bleaching phenotype as fixed effects (Tables 2, 3). Montipora capitata and P. compressa were analyzed separately, with coral identification number as a random effect to account for repeated measures. For models in which the three-way interaction between lagoon, bleaching phenotype, and time point was significant, we further analyzed the effect of lagoon and bleaching phenotype and their interaction with separate linear mixed models subset at each time point. Because not every colony within a pair was found at every time point, we tested for the effects of intermittent partial pairs by analyzing a subset of the dataset that included only data from pairs where both partners were found at a time point (“pairs” models). We tested the effect of lagoon and bleaching phenotype and their interaction on the differences in pigmentation and mortality scores using the models described above, with the addition of the random effect of pair identification number to test for spatial heterogeneity within lagoons. For all analyses, p-values were calculated using Type II analysis of variance (ANOVA) with Satterthwaite’s approximation method. Tukey honest significant difference post hoc tests (R package emmeans, Lenth, 2019) were used to test for significant pairwise differences in main effects. Results are presented with p-values specifying the main effects of bleaching phenotype (P), recovery month (M), and lagoon (L).
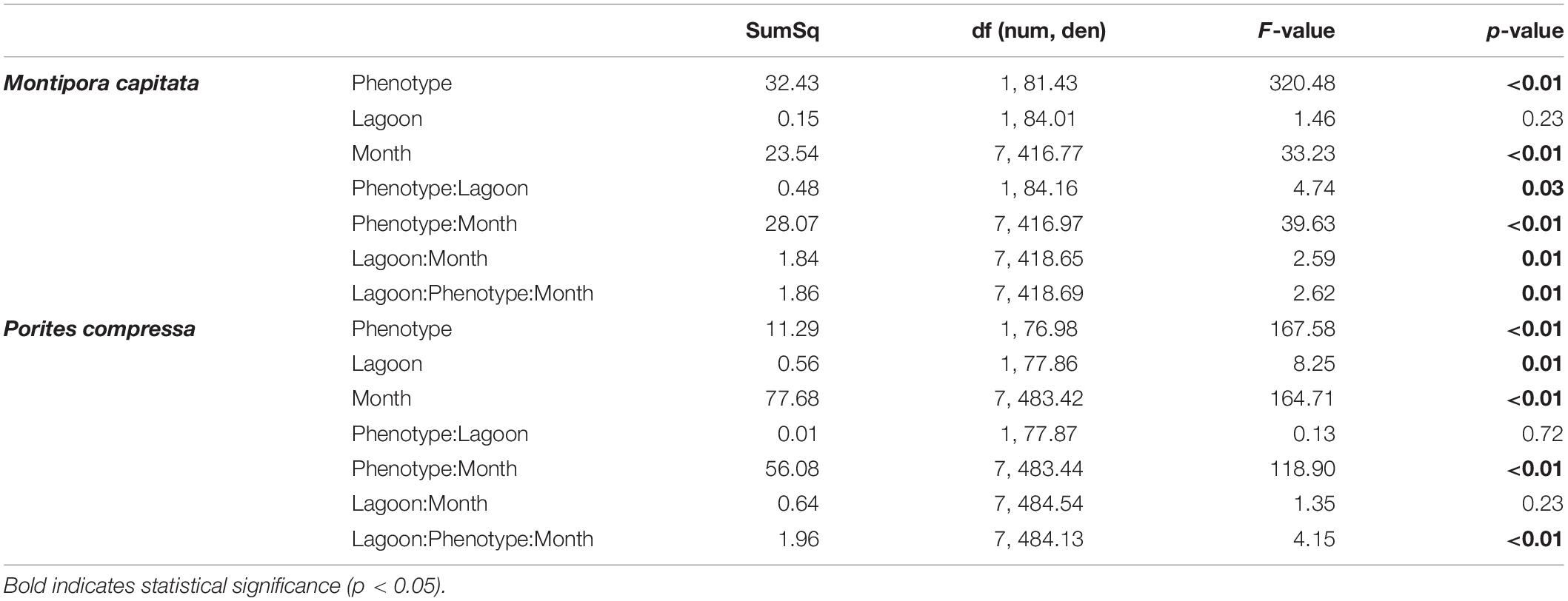
Table 2. Type II Analysis of Variance Table with Satterthwaite approximation for the effects of bleaching phenotype, lagoon, and recovery time across the course of the study, on pigmentation recovery of bleaching susceptible and resistant colonies for Montipora capitata and Porites compressa at Inner and Outer lagoons (Color Score∼Phenotype × Lagoon × Month+(1|Coral Tag ID).
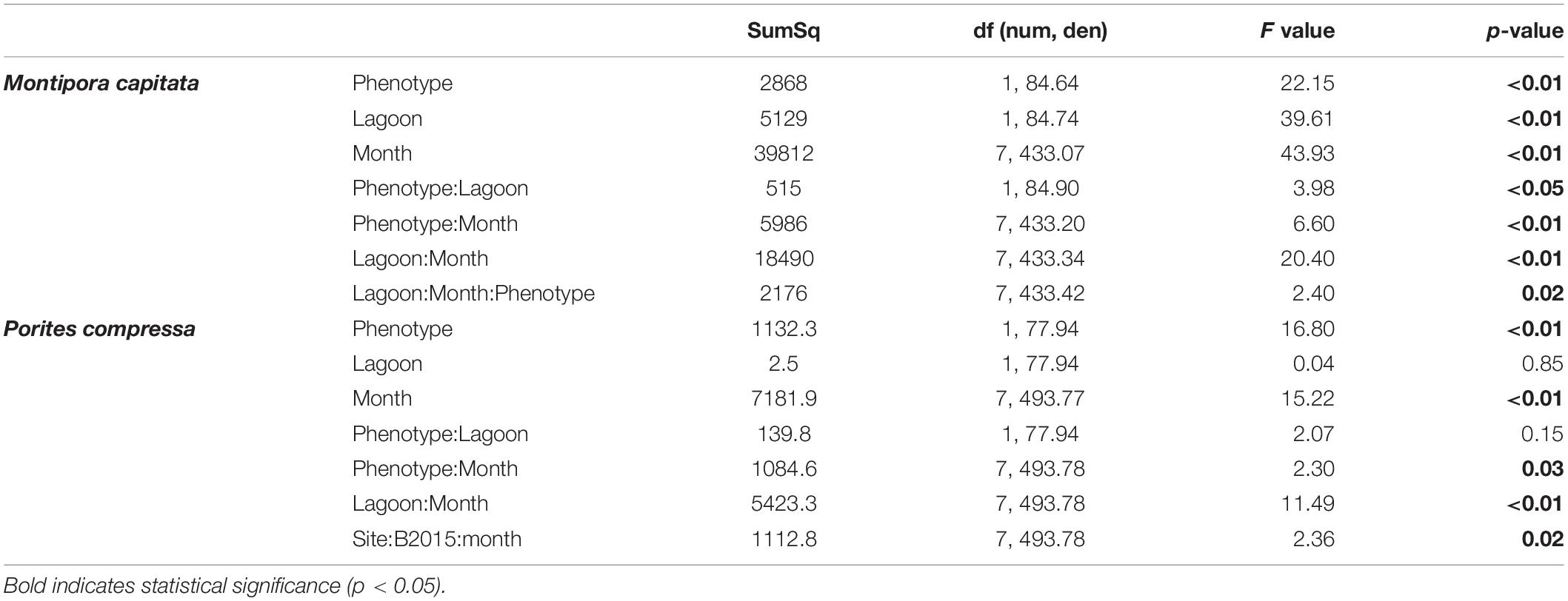
Table 3. Type II Analysis of Variance Table with Satterthwaite approximation for the effects of bleaching phenotype, lagoon, and recovery time across the course of the study, on partial mortality of bleaching susceptible and resistant colonies for Montipora capitata and Porites compressa at Inner and Outer lagoons (Partial Mortality∼Phenotype × Lagoon × Month+(1|Coral Tag ID).
Results
Temperature Dynamics Throughout Bleaching and Recovery
Midday seawater temperatures exceeded the local bleaching threshold of 29.0°C throughout Kāne‘ohe Bay in September 2015 (Figure 1; Bahr et al., 2017). Daily highs met or exceeded 29°C for a total of 46 days at the Inner Lagoon reef (PR1) versus 24 days at the Outer Lagoon reef (PR12; Figure 1D). The average maximum daily seawater temperature during the heatwave was 29.08°C in the Inner Lagoon, which was on average 0.44°C warmer than the Outer Lagoon (28.64°C, p < 0.01, Wilcoxon test; Table 1). The average minimum daily seawater temperature was also higher at the Inner Lagoon than the Outer Lagoon during this time (+0.50°C, p < 0.01, Wilcoxon test; Table 1), while the daily temperature range did not differ significantly between the two sites (p = 0.118, Wilcoxon test). This resulted in higher accumulated heat stress at Inner Lagoon relative to the Outer Lagoon, with a total of 5.84 degree heating weeks (DHW) at Inner Lagoon versus 1.77 DHW at the Outer Lagoon (Table 1). In contrast, during the first 3 months following the heatwave, the average daily maximum and minimum seawater temperatures were not significantly different between the two regions (Figure 1D and Table 1).
Benthic Community Composition
Coral cover was significantly different between the Inner and Outer Lagoon reefs (pL < 0.01, Supplementary Table S1), but did not change significantly over the course of the 2-year bleaching recovery period (pL:M = 0.1, Figure 3 and Supplementary Table S1. At the initiation of this study, coral cover at PR13 in the Outer Lagoon was 80 ± 4.2% (Figure 3B), and at PR4 in the Inner Lagoon was 52 ± 6.0% (Figure 3A). Community composition of each reef is reported below as the range from min to max across the entire time series. The coral community of the Outer Lagoon reef was dominated by P. compressa throughout the time series (62–77% of live coral cover) relative to M. capitata (23–38% of live coral cover). The relative abundance of each species at the Inner Lagoon reef was 54–63% for P. compressa vs. 37–46% for M. capitata. Turf algae were the second most abundant functional group at both reefs, comprising 6–10% of the benthos at the Outer Lagoon reef and 25–40% at the Inner Lagoon reef. Sediments were also common along the benthos at the Inner Lagoon reef (8.5–21%; Figure 3A), but were found in low abundance at the Outer Lagoon reef (<1%; Figure 3B). Both reefs had a low abundance (<5%) of macroalgae, crustose coralline algae (CCA) and sponges. The Inner Lagoon reef had an abundance of small filter feeders (e.g., boring oysters and sponges) that were not readily apparent on photoquadrat images but were observed by divers/snorkelers during surveys. This reef also typically had low visibility (<5 m; Figure 1C), whereas the Outer Lagoon reef tended to have higher visibility (∼20–30 m, personal observations; Figure 1B).
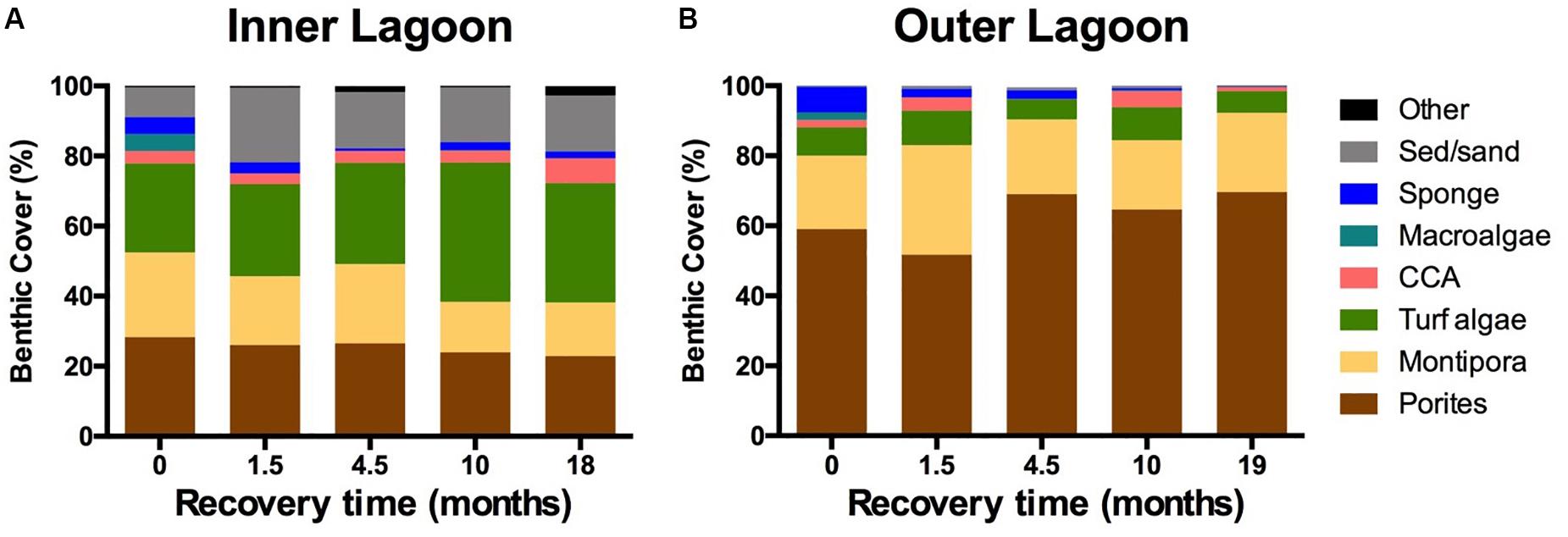
Figure 3. Benthic community composition from the peak of the coral bleaching event (Oct. 2015) and after 1.5, 4.5, 10, and 18–19 months of recovery for (A) the Inner lagoon (PR4) and (B) the Outer lagoon (PR13) in Kāne‘ohe Bay, O‘ahu. Data represent the means of four replicate transects.
Initial Coral Bleaching Prevalence and Severity Differed Between Species and Reefs
Coral tagging and benthic surveys were initiated during the peak extent of bleaching in Kāne‘ohe Bay in late September through October 2015 (Bahr et al., 2017). There were two significant interactions for bleaching prevalence: lagoon and recovery time (pL:M < 0.01) and species and recovery time (pS:M < 0.01; Supplementary Table S2). Additionally, there was a significant three-way interaction of lagoon, species and recovery time for both the prevalence of severely bleached tissue (pL:S:M < 0.01; Supplementary Table S3) and bleaching severity (the proportion of completely bleached tissue out of all affected corals; pS:L:M < 0.01; Supplementary Table S4). At peak bleaching, there was a higher prevalence of coral bleaching (proportion of live coral that was symptomatic, either white or pale) at the Inner Lagoon reef (79%) than the Outer Lagoon reef (44%; post hoc p < 0.01). Separated by species, initial coral bleaching prevalence was 69 ± 3% at the Inner Lagoon reef vs. 39 ± 12% at the Outer Lagoon reef for M. capitata, and 87 ± 7% at the Inner Lagoon reef vs. 45 ± 5% at the Outer Lagoon reef for P. compressa (Figure 4). Within reefs, the prevalence of severely bleached (white) tissue was lower for M. capitata (26 ± 6% of all live tissue) than for P. compressa (71 ± 10% of all live tissue) at the Inner Lagoon reef (post hoc p < 0.01; Supplementary Figure S2), whereas there was no significant difference in severe bleaching prevalence between species at the Outer Lagoon reef (post hoc p > 0.05; Figure 4). Bleaching severity (the proportion of symptomatic tissue that was completely bleached) was higher at the Inner Lagoon reef for P. compressa (80 ± 5%) than for M. capitata (37 ± 7%, Figure 4; post hoc p < 0.01). Bleaching severity for P. compressa was substantially lower at the Outer Lagoon reef (29 ± 10%; post hoc p < 0.01) than the Inner Lagoon reef (80 ± 5%), whereas bleaching severity did not differ significantly between sites for M. capitata (33 ± 5% at the Outer Lagoon reef vs. 37 ± 7% at the Inner Lagoon reef; post hoc p < 0.05).
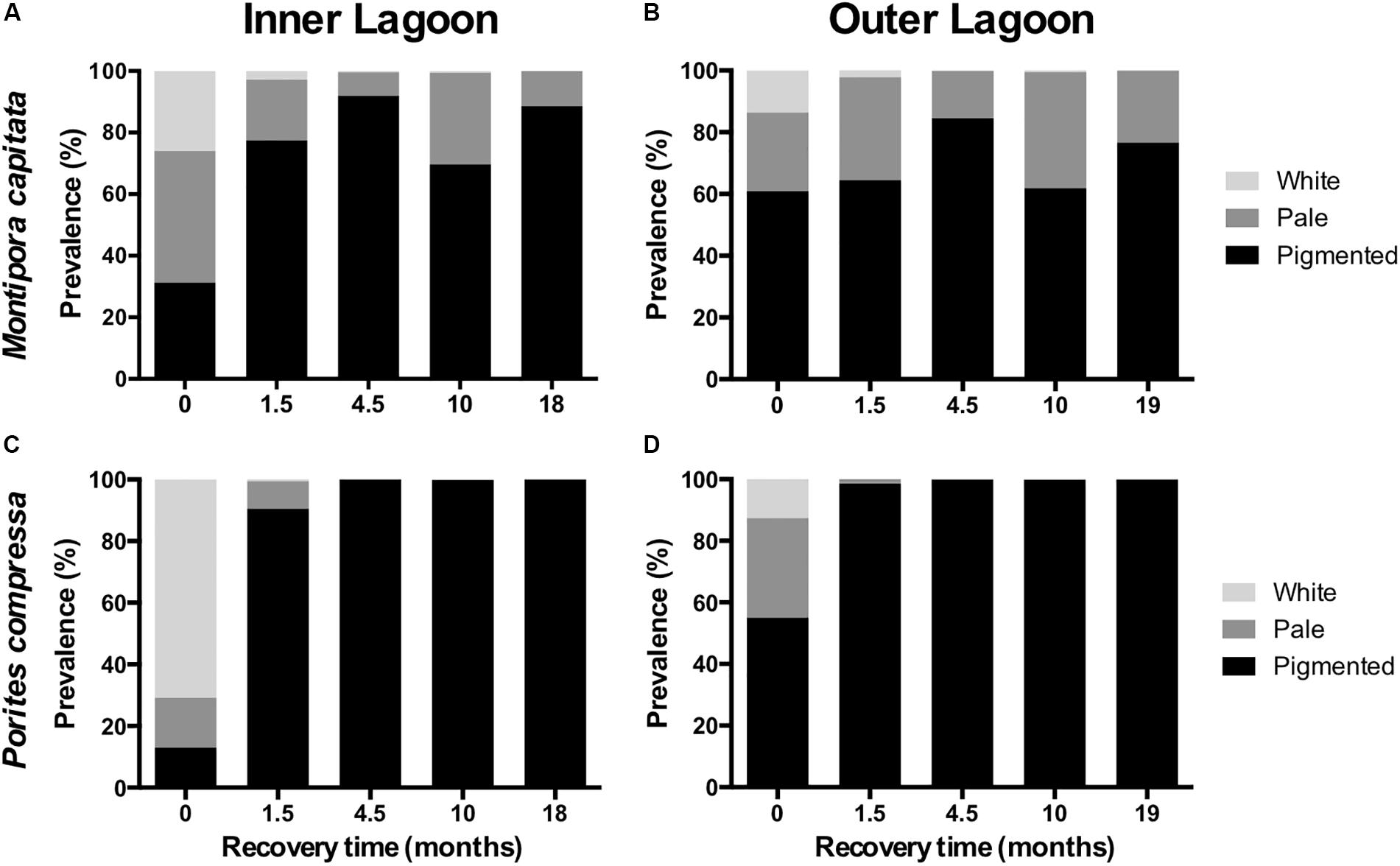
Figure 4. Prevalence of coral bleaching from the peak of the bleaching event (Oct. 2015) and after 1.5, 4.5, 10, and 18–19 months of recovery for Montipora capitata (A,B) and Porites compressa (C,D) at the Inner lagoon (left column) and Outer lagoon (right column). Data represent the means of four replicate transects.
Decline in Coral Bleaching Prevalence Differs Between Species During Recovery
Bleaching prevalence rapidly decreased for P. compressa at both reefs, with a 97% decrease in bleaching prevalence observed after 1.5 months of recovery at the Outer Lagoon reef to <2% overall prevalence, and an 89% decrease at the Inner Lagoon reef to <10% prevalence (Figure 4). In contrast, bleaching prevalence in M. capitata declined more slowly, with a mean decrease of 67% at the Inner Lagoon reef versus only a 9% decrease at the Outer Lagoon reef after 1.5 months. At that point, <3% of M. capitata remained fully bleached at either site, and 20–33% remained pale. In contrast to peak bleaching, coral bleaching prevalence was significantly higher for M. capitata than P. compressa after 1.5 months of recovery (Figure 4; post hoc p = 0.02) due to its slower recovery from bleaching. By 4.5 months of recovery, bleaching prevalence no longer differed between species (Figure 4). Interestingly, after several months of declining prevalence, the seasonal peak in water temperatures in September 2016 (Month 10; Figure 1D) corresponded with an increase in the prevalence of pale M. capitata (post hoc p = 0.03; Figures 4A,B). Porites compressa pigmentation was not affected by this seasonal warming, and bleaching prevalence remained below 2% from 1.5 months of recovery onward (Figures 4C,D).
Pigmentation Recovery Dynamics of Individual Coral Colonies Following Heat Stress
Bleaching resistant corals remained pigmented throughout the entire 24-month recovery period for both species, whereas bleached corals exhibited increasing pigmentation over the first 3 months of recovery for both species (Figures 2, 5A–D). This resulted in a significant interaction of bleaching phenotype and time point on coral pigmentation for both M. capitata and P. compressa (pP:M < 0.01, Table 2). Additionally, there was a significant three-way interaction of bleaching phenotype, lagoon and month (pP:L:M < 0.01, Table 2), and so we investigated how phenotype differed by lagoon at each time point. This analysis indicated that bleaching recovery times differed between M. capitata and P. compressa. For example, bleaching susceptible colonies of P. compressa remained distinguishable from resistant colonies 1.5 months post peak bleaching (pP < 0.01, Supplementary Table S5), but recovered full visual pigmentation by 3 months at both reefs (pP:L > 0.05; Supplementary Table S5; Figures 5C,D). In contrast, recovery was slower for M. capitata. Only 31% of bleaching susceptible M. capitata fully recovered normal pigmentation at the Inner Lagoon reef after 3 months, while no individuals had fully recovered at the Outer Lagoon reef at that time (Figures 5A,B). The phenotype effect differed by lagoon between three and six months of recovery (pP:L < 0.05, Supplementary Table S5), such that there was a larger difference between phenotypes at the Outer Lagoon than the Inner Lagoon. Full pigmentation recovery for bleaching susceptible M. capitata colonies took as long as 24 months at the Inner Lagoon reef, going from 25% of individuals with full pigmentation at 18 months to 94% at 24 months (Figure 5A). At the Outer Lagoon reef, most bleaching susceptible M. capitata colonies (68%) remained pale for the entire 24-month duration of this study (Figure 5B).
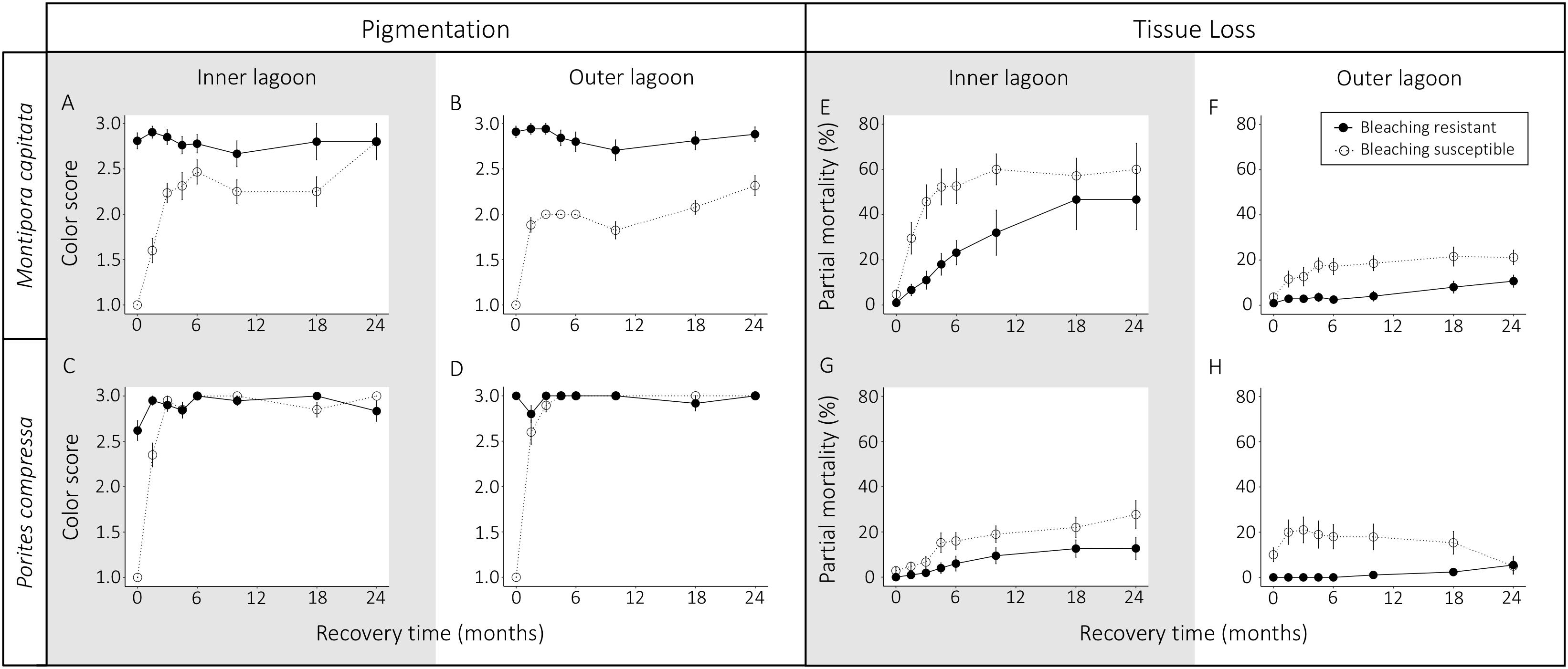
Figure 5. Average color score (A–D) and partial mortality (E–H) of bleaching susceptible versus bleaching resistant colonies of Montipora capitata (A,B,E,F) and Porites compressa (C,D,G,H) at the Inner Lagoon (A,C,E,G) and Outer Lagoon (B,D,F,H) from the peak of the coral bleaching event (September–October 2015) through the following 24 months of recovery. Solid lines indicate bleaching resistant colonies; dashed lines indicate bleaching susceptible colonies. Color scores: 1, white; 2, pale; 3, pigmented. Error bars indicate SEM.
Because both partners of each pair were not located at every time point, models were run on a subset of the data where any coral colony whose pair was not found at a time point was excluded (“pairs” models) in order to test for effects of spatial location on the reef on recovery dynamics. Here, the main effects were the same as for the “full” models. For P. compressa, the statistical patterns were identical to the three-way (phenotype:lagoon:month) and individual time point “full” models (Supplementary Table S6). For M. capitata, the statistical patterns were identical for the three-way model across time, although there were a few differences at individual time points. Specifically, the interaction of lagoon and bleaching phenotype that was significant in the “full” models at months 3–6, was not significant in the “pairs” models (Supplementary Table S6), indicating that differences in color score between adjacent bleached and unbleached corals were equal between lagoons. This indicates that there was no effect of local environment driving differences in bleaching recovery.
Colony Mortality Following Bleaching Differs Between Bleaching Phenotypes
Partial mortality increased significantly for both species over time following the bleaching event, the extent of which differed between bleaching phenotypes (M. capitata: pP:M < 0.01, P. compressa pP:M = 0.03; Table 3 and Figure 5) and site (pP:M:L = 0.02; Table 2). For each species, bleaching susceptible colonies had significantly more partial mortality than bleaching resistant colonies across both sites during the first year of recovery (pP < 0.01, Supplementary Table S7), and most partial mortality of bleaching susceptible colonies occurred in the first 6 months following the heat stress (Figures 5E–H). At three months of recovery, partial mortality of bleaching susceptible and resistant phenotypes differed significantly more at the Inner Lagoon for M. capitata, and at the Outer Lagoon for P. compressa (pL:P = 0.01; Supplementary Table S7). Overall, partial mortality was <20% in the first year following heat stress for both phenotypes of both species at the Outer Lagoon reef and for both phenotypes of P. compressa at the Inner Lagoon reef (Figures 5F–H). In contrast, mean partial mortality for M. capitata at the Inner Lagoon reef was 60% for bleaching susceptible colonies vs. 32% for bleaching resistant colonies at this time (Figure 5E). Mean partial mortality remained significantly higher for M. capitata at the Inner Lagoon reef than the Outer Lagoon reef at every time point across the 24-month recovery for both phenotypes (pL ≤ 0.01; Supplementary Table S7). Similarly, mean partial mortality for P. compressa was also higher at the Inner Lagoon reef than the Outer Lagoon reef regardless of bleaching phenotype after 24 months (pL < 0.01; Supplementary Table S7).
While bleaching resistant corals showed lower partial mortality in the first year following the heat stress than bleaching sensitive corals, bleaching resistant corals showed increasing tissue loss across the second year of recovery. This resulted in no significant differences in mean partial mortality between phenotypes for either species at either site by 24 months (Supplementary Table S7). The one exception to the pattern that drove this convergence was for P. compressa at the Outer Lagoon reef, where bleaching sensitive P. compressa actually showed an average decline in partial mortality (Figure 5H). The most dramatic example of colony regrowth was P. compressa Tag no. 225, which suffered ∼60% mortality in the first 2 months following bleaching but then regrew to nearly 100% live tissue within one year following the heatwave. Only five colonies (one P. compressa and four M. capitata) experienced full mortality during the 2-year recovery period, and all were located at the Inner Lagoon reef. The statistical patterns did not differ when analyzing partial mortality scores from the “pairs” models, suggesting that spatial location on the reef did not greatly influence the difference in partial mortality between phenotypes (Supplementary Table S8).
Discussion
Bleaching Susceptibility of a Species Is Not Predictive of Mortality
Porites compressa experienced higher bleaching prevalence and severity (i.e., lower bleaching resistance) than Montipora capitata at the Inner Lagoon reef, and yet bleaching susceptible individuals of P. compressa experienced significantly less partial mortality (i.e., greater bleaching resilience) than bleaching susceptible individuals of M. capitata at this same site. These results are in contrast to the common pattern of greater bleaching prevalence of a species leading to greater mortality (Baird and Marshall, 2002; Jones, 2008; Hughes et al., 2018b), and may reflect two different species-specific heat stress response strategies. In the case of M. capitata, this species resisted bleaching to a greater extent than P. compressa, but individuals that bleached had lower resilience following bleaching (slower recovery and higher mortality). P. compressa, on the other hand, was more susceptible to bleaching but had greater resilience following bleaching (faster recovery and lower mortality) than M. capitata. The relatively lower resilience (higher partial mortality) of M. capitata than P. compressa following bleaching observed here was somewhat surprising because M. capitata typically replaces lost biomass following bleaching faster than P. compressa (Grottoli et al., 2006; Rodrigues and Grottoli, 2007; Wall et al., 2019), and was thus expected to have lower partial mortality. Faster biomass recovery in M. capitata occurs despite the observation that this species typically recovers pigmentation (chlorophyll a) slower than P. compressa (Grottoli et al., 2006; Rodrigues and Grottoli, 2007; Wall et al., 2019), though not always (Wall et al., 2019). Interestingly, while bleached M. capitata recovered pigmentation faster than P. compressa following the 2014 coral bleaching event (Wall et al., 2019), the reverse was observed following the 2015 event (Figure 5). This suggests that coral recovery rates following heat stress may depend on the frequency of stress events. As our observations describe the responses of these corals to the second of two bleaching events to occur within the span of one year, the discrepancies between the outcomes we observed and those of previous studies could be due in part to the recurrent nature of the stress. This pattern has also been observed in other reef systems, where annual repeat bleaching events turn some Caribbean coral species from winners into losers (Grottoli et al., 2014).
Ecological Outcomes Differ Between Bleaching Susceptible and Resistant Phenotypes
Intraspecific differences in bleaching susceptibility had a significant influence on the ecological outcomes of populations of both coral species in the months following heat stress. For both M. capitata and P. compressa, bleaching susceptible individuals suffered higher partial mortality than bleaching resistant individuals located on the same reef. This resulted in significant losses of live coral cover from the reef, which despite not being detected in reef-wide surveys, likely has a significant impact on the ecological function of each reef. From an evolutionary perspective, these differences in partial mortality are likely to negatively impact reproductive success, as reproductive output is positively correlated with colony size in colonial coral species (Hall and Hughes, 1996) and thus corals suffering tissue loss will likely have lower fecundity in subsequent spawning seasons than they would have had. Differential partial mortality between susceptible and resistant phenotypes therefore likely influences individual fitness and thus the genetic composition of offspring released in subsequent reproductive events. Further exacerbating this loss, corals that have recently undergone bleaching have a lower likelihood of reproducing at all relative to bleaching resistant conspecifics, and those that do manage to reproduce tend to release fewer and less provisioned gametes (Ward et al., 2000; Fisch et al., 2019). Together these factors will likely limit the evolutionary success of bleaching susceptible genotypes and the ecological resilience of the reef by reducing the recruitment pool and live coral cover (Fisch et al., 2019; Hughes et al., 2019a). However, the low frequency of complete colony mortality during this bleaching event indicates the adult gene pool has remained mostly intact, maintaining the genetic diversity of the current population. Intraspecific differences in coral mortality also affect the ecological landscape of coral symbionts. In M. capitata, for example, bleaching resistant phenotypes tend to be dominated by Durusdinium glynii, whereas bleaching susceptible individuals are mostly dominated by Cladocopium sp. (Cunning et al., 2016), and lower partial mortality of D. glynii-dominated individuals would likely increase the relative abundance of D. glynii in the community. As symbiont transmission occurs vertically in this species (Padilla-Gamiño et al., 2012), this would also increase the proportion of larvae inheriting D. glynii, potentially altering the composition of the symbiont community for generations. Furthermore, as heat stress events increase in frequency and severity (Hughes et al., 2018a), the relative growth benefits associated with hosting thermally sensitive symbionts like Cladocopium spp. at non-stressful temperatures may fail to make up for the higher costs of these associations during heat stress, altering the tradeoffs of the association (Cunning et al., 2015). Mechanisms driving differential stress responses in P. compressa are less well understood, particularly as symbiont variation in this species is limited to a single species of Cladocopium (ITS2 clade C15; LaJeunesse et al., 2004). Differential bleaching in this species is thus likely driven by differences in host physiology (Baird et al., 2009), the composition of the microbiome (Morrow et al., 2018), or a combination thereof.
Coral Bleaching and Recovery Dynamics Differed Between Inner and Outer Lagoon Reefs
Montipora capitata and P. compressa both experienced higher bleaching prevalence and severity at the Inner Lagoon reef than the Outer Lagoon reef, which corresponded with higher partial mortality for M. capitata. These results are reflective of coral mortality patterns from bay-wide surveys, which also observed higher cumulative coral mortality on reefs in the Inner Lagoon region (Bahr et al., 2017). Higher rates of bleaching and mortality within the Inner Lagoon were likely due to the higher accumulated heat stress at this site. However, additional environmental factors were likely involved because bleaching resistant M. capitata also had higher mortality at the Inner Lagoon reef relative to either phenotype at the Outer Lagoon reef. These data suggest that differences in coral bleaching and mortality between the two reefs were likely due to a combination of environmental factors, with worse outcomes within the Inner Lagoon potentially driven by the lower flow rates, longer seawater residence times (>30 days; Lowe et al., 2009), and closer proximity to land and associated freshwater runoff relative to the Outer Lagoon reef. Low flow environments can limit nutrient and waste exchange in the coral boundary layer, suppressing coral metabolism (Mass et al., 2010; Putnam et al., 2017). In addition, higher freshwater input (Bahr et al., 2015) and associated allochthonous inorganic nutrients (Stimson, 2015), which can lead to greater rates of bleaching (Wiedenmann et al., 2013; Vega Thurber et al., 2014), may have exacerbated coral stress and bleaching during the heat wave, limiting coral recovery and exacerbating tissue loss in the Inner Lagoon relative to the Outer Lagoon. Indeed, pigmentation recovery rates were slower within the Inner Lagoon in both the 2015 (this study) and 2014 bleaching events (Cunning et al., 2016; Wall et al., 2019). However, we cannot disentangle the role of nutrients versus differences in temperature and light regimes in driving heat stress responses in this study.
Coral Recovery Requires More Time Between Bleaching Events
Consecutive annual bleaching events have become a feature of coral reefs in Hawaii and around the world (Grottoli et al., 2014; Bahr et al., 2017; Hughes et al., 2018a; Raymundo et al., 2019). For example, corals in Hawaii had ∼10 months to recover from the effects of the 2014 heatwave before a second heatwave hit in 2015. This short recovery period is likely one reason the 2015 heatwave caused the highest cumulative coral mortality ever observed in Hawaii following a bleaching event (Bahr et al., 2017). Short intervals between heat waves such as these can lead to higher coral mortality following the second event because they may prevent individual corals from fully recovering energetically from the first heat stress prior to exposure to the second (e.g., Sale et al., 2019), and thus are likely to make individuals less resistant to bleaching and mortality in the second event. This short time interval also likely limits acclimatization, and indeed many colonies in Hawaii that bleached in 2014 tended to bleach again in 2015 (Ritson-Williams and Gates, 2020), suggesting that these corals had not acclimatized to higher temperatures. Longer recovery periods are also important for regrowth of tissue lost during a heatwave, and our data showed that tissue lost due to partial mortality was generally not replaced by live coral cover in the 2 years following the bleaching event. This indicates that a predominance of corals in Kāne‘ohe Bay require longer recovery intervals to replace lost coral cover following bleaching-related mortality. Furthermore, our data indicate that symbiont recovery rates may take longer following repeat bleaching events, as M. capitata recovered visual pigmentation and symbiont abundance in a span of 1–3 months in previous bleaching events (Jokiel and Brown, 2004; Cunning et al., 2016; Wall et al., 2019; Ritson-Williams and Gates, 2020) and recovers in 8 months or less in mesocosm experiments (Rodrigues and Grottoli, 2007), while in 2015 we found that it took as much as 2 years for many bleached M. capitata individuals to fully recover pigmentation. In addition, repetitive bleaching events may influence the differential success of the major reef building species in Kāne‘ohe Bay. We found that P. compressa recovered pigmentation faster than M. capitata and had a lower rate of tissue loss. This was similar to patterns of pigmentation recovery and mortality in these same species at other patch reefs in Kāne‘ohe Bay during both the 2014 and 2015 bleaching events (Ritson-Williams and Gates, 2020), and suggests that P. compressa may gain ecological advantage if bleaching events become more common. Encouragingly, both the 2014 and 2015 events led to low rates of complete colony mortality, with <3% of corals suffering 100% mortality (M. capitata and P. compressa; this study) versus <2% of M. capitata in 2014 (Cunning et al., 2015). Also encouraging, there were individuals that underwent a complete recovery of live tissue cover following significant (>50%) partial mortality, suggesting that at least a few individuals, albeit a minority, are highly resilient and that given a few years of recovery between stress events can rapidly replace lost tissue when mortality is incomplete.
Individual Tracking Uncovers Low Levels of Partial Mortality Following Heat Stress
Both bleaching susceptible and bleaching resistant individuals suffered partial mortality in the months following the bleaching event, indicating that although resistant individuals did not visually bleach, all corals were negatively affected by heat stress. Interestingly, while bleaching-susceptible colonies of both species suffered an average of ∼20% tissue loss in the first 6 months following the bleaching event, these losses did not manifest as significant changes in live coral cover. This discrepancy could be due in part to the low mortality of bleaching resistant phenotypes masking the higher mortality of bleaching-susceptible individuals in community wide surveys. In addition, the high variance of photoquadrat surveys makes it difficult to detect small changes in benthic cover (Jokiel et al., 2015). Photoquadrat surveys are also commonly used to quantify recently dead coral cover during or following a bleaching event, however, this method cannot discern whether the coral that died had in fact bleached, whereas individual colony data revealed the consequences of heat stress for both bleaching phenotypes. While these losses in live coral cover were not detected at the community level, the significant partial mortality observed at the colony level has a negative impact on the ecology and evolution of coral communities as described above. Loss of susceptible individuals may also lower the genotypic richness of the population, which in corals can correlate with lower reproductive success (Baums et al., 2013). That said, the lack of a significant decline in coral cover at these two reefs is encouraging for reef recovery from this bleaching event.
Living Library: Coral Pairs Are a Resource for Future Research and Restoration
By identifying individual coral colonies with distinct heat stress responses and tracking them over a multi-year period, we have generated and continue to maintain a live geo-referenced biological archive in the field as a resource for research on the effects of environmental history and thermal stress responses on the ecology and physiology of reef-building corals. This resource will be particularly valuable in light of predicted increases in the frequency and severity of coral bleaching events, as it allows for prospective sampling of bleaching susceptible vs. resistant phenotypes before, during, and following a bleaching event. This experimental system allows researchers to address questions of coral acclimatization and adaptation to changing oceans, such as examining whether individual coral responses predict tolerance to future stress. In addition, it allows researchers to identify if individual coral responses to subsequent events change relative to the outcomes observed in the current event (e.g., acclimatization, loss of resistance and/or resilience), potentially altering which species within the community are considered the ecological winners. Finally, fully recovered corals of known contrasting heat tolerances can be used to investigate the interaction of temperature tolerance with coral responses to other stressors, including but not limited to ocean acidification, eutrophication, and pathogens, helping to identify potential tradeoffs of heat tolerance in corals and the importance of phenotypic and genetic diversity in coral reef resilience (Stachowicz et al., 2007; Ladd et al., 2017). This will be useful for informing coral restoration strategies, particularly in light of the movement toward human assisted evolution (e.g., assisted gene flow), as it allows assessment of the potential tradeoffs of propagating heat tolerant genotypes (Van Oppen et al., 2015, 2017). From a management perspective, bleaching resistant individuals have the potential to serve as a resource for reef managers interested in propagating climate change resilient genotypes on damaged or degraded coral reefs (Van Oppen et al., 2017). Comparing bleaching responses within species demonstrates the importance of understanding individual colony susceptibility to heat stress and trajectories of recovery in the face of ongoing climate change. These questions are important for understanding the persistence of coral reefs into the future.
Data Availability Statement
The datasets analyzed for this study and R scripts can be found in Zenodo at doi: 10.5281/zenodo.3862838. The raw data supporting the conclusions of this article will be made available by the authors, without undue reservation, to any qualified researcher.
Author Contributions
KB and RG designed the study. EL, JD, and JH helped to collect the data. KB, SM, AP, TI, and AH collected and analyzed the data. KB and SM wrote the manuscript. All authors edited and approved the final version of the manuscript.
Funding
This work was supported by funding from Paul G. Allen Family Foundation to RG, the University of Pennsylvania to KB, the Point Foundation to SM, National Science Foundation Graduate Research Fellowships to AH and EL, and a grant/cooperative agreement from the National Oceanic and Atmospheric Administration, Project R/IR-37 to SM and RG, which is sponsored by the University of Hawaii Sea Grant College Program, SOEST, under Institutional Grant No. NA14OAR4170071 from NOAA Office of Sea Grant, Department of Commerce. The views expressed herein are those of the author(s) and do not necessarily reflect the views of NOAA or any of its sub-agencies.
Conflict of Interest
The authors declare that the research was conducted in the absence of any commercial or financial relationships that could be construed as a potential conflict of interest.
Acknowledgments
We thank Yanitza Grantcharska and George Davies for their help in the field, and the Hawai‘i Institute of Marine Biology staff for logistical support. Thank you also to the State of Hawai‘i Division of Aquatic Resources for sharing data from their temperature sensors, and to Craig Nelson for feedback on statistical models. This is HIMB contribution 1808 and SOEST contribution 10987. bioRxiv preprint first posted online Dec. 18, 2019; doi: https://doi.org/10.1101/2019.12.17.880161.
Supplementary Material
The Supplementary Material for this article can be found online at: https://www.frontiersin.org/articles/10.3389/fevo.2020.00178/full#supplementary-material
Footnotes
- ^ Division of Aquatic Resources, State of Hawaii: RExOUi5hcXVhdGljc0BoYXdhaWkuZ292; http://dlnr.hawaii.gov/dar/
- ^ http://www.pacioos.hawaii.edu/weather/obs-mokuoloe/
- ^ https://cnso.nova.edu/cpce/index.html
References
Alvarez-Filip, L., Carricart-Ganivet, J. P., Horta-Puga, G., and Iglesias-Prieto, R. (2013). Shifts in coral-assemblage composition do not ensure persistence of reef functionality. Sci. Rep. 3, 1–5.
Anthony, K. R. N., Connolly, S. R., and Hoegh-Guldberg, O. (2007). Bleaching, energetics, and coral mortality risk: effects of temperature, light, and sediment regime. Limnol. Oceanogr. 52, 716–726. doi: 10.4319/lo.2007.52.2.0716
Bahr, K. D., Jokiel, P. L., and Rodgers, K. S. (2015). The 2014 coral bleaching and freshwater flood events in Kāne‘ohe Bay. Hawai‘i. PeerJ 3:e1136. doi: 10.7717/peerj.1136
Bahr, K. D., Rodgers, K. S., and Jokiel, P. L. (2017). Impact of three bleaching events on the reef resiliency of Kāne‘ohe Bay, Hawai‘i. Front. Mar. Sci. 4:398. doi: 10.3389/fmars.2017.00398
Baird, A. H., Bhagooli, R., Ralph, P. J., and Takahashi, S. (2009). Coral bleaching: the role of the host. Trends Ecol. Evol. 24, 16–20. doi: 10.1016/j.tree.2008.09.005
Baird, A. H., and Marshall, P. A. (2002). Mortality, growth and reproduction in scleractinian corals following bleaching on the great barrier reef. Mar. Ecol. Prog. Ser. 237, 133–141. doi: 10.3354/meps237133
Baker, A. C., Glynn, P. W., and Riegl, B. (2008). Climate change and coral reef bleaching: an ecological assessment of long-term impacts, recovery trends and future outlook. Estuar. Coast. Shelf Sci. 80, 435–471. doi: 10.1016/j.ecss.2008.09.003
Barshis, D. J., Birkeland, C., Toonen, R. J., Gates, R. D., and Stillman, J. H. (2018). High-frequency temperature variability mirrors fixed differences in thermal limits of the massive coral Porites lobata. J. Exp. Biol. 221:eb188581.
Barshis, D. J., Ladner, J. T., Oliver, T. A., Seneca, F. O., Traylor-Knowles, N., and Palumbi, S. R. (2013). Genomic basis for coral resilience to climate change. Proc. Natl. Acad. Sci. U.S.A. 110, 1387–1392. doi: 10.1073/pnas.1210224110
Bates, D., Mächler, M., Bolker, B., and Walker, S. (2015). Fitting linear mixed-effects models using lme4. J. Stat. Softw. 67, 1–48.
Baums, I. B., Devlin-Durante, M. K., Polato, N. R., Xu, D., Giri, S., Altman, N. S., et al. (2013). Genotypic variation influences reproductive success and thermal stress tolerance in the reef building coral. Acropora palmata. Coral Reefs 32, 703–717. doi: 10.1007/s00338-013-1012-6
Bay, L. K., Guérécheau, A., Andreakis, N., Ulstrup, K. E., and Matz, M. V. (2013). Gene expression signatures of energetic acclimatisation in the reef building coral Acropora millepora. PLoS One 8:e61736. doi: 10.1371/journal.pone.0061736
Bay, R. A., and Palumbi, S. R. (2015). Rapid acclimation ability mediated by transcriptome changes in reef-building corals. Genome Biol. Evol. 7, 1602–1612. doi: 10.1093/gbe/evv085
Berkelmans, R., and Van Oppen, M. J. (2006). The role of zooxanthellae in the thermal tolerance of corals: a ‘nugget of hope’ for coral reefs in an era of climate change. Proc. R. Soc. Lond. B. Biol. Sci. 273, 2305–2312. doi: 10.1098/rspb.2006.3567
Coles, S. L., Bahr, K. D., Rodgers, K. S., May, S. L., McGowan, A. E., Tsang, A., et al. (2018). Evidence of acclimatization or adaptation in Hawaiian corals to higher ocean temperatures. PeerJ 6:e5347. doi: 10.7717/peerj.5347
Costanza, R., de Groot, R., Sutton, P., van der Ploeg, S., Anderson, S.J., Kubiszewski, I., et al. (2014). Changes in the global value of ecosystem services. Glob. Environ. Change 26, 152–158. doi: 10.1016/j.gloenvcha.2014.04.002
Couch, C. S., Burns, J. H. R., Liu, G., Steward, K., Gutlay, T. N., Kenyon, J., et al. (2017). Mass coral bleaching due to unprecedented marine heatwave in papahânaumokuâkea marine national monument (Northwestern Hawaiian Islands). PLoS One 12:e0185121. doi: 10.1371/journal.pone.0185121
Cunning, R., Gillette, P., Capo, T., Galvez, K., and Baker, A. C. (2015). Growth tradeoffs associated with thermotolerant symbionts in the coral Pocillopora damicornis are lost in warmer oceans. Coral Reefs 34, 155–160. doi: 10.1007/s00338-014-1216-4
Cunning, R., Ritson-Williams, R., and Gates, R. D. (2016). Patterns of bleaching and recovery of montipora capitata in Kāne ‘ohe Bay. Hawai ‘i, USA. Mar. Ecol. Prog. Ser. 551, 131–139. doi: 10.3354/meps11733
Darling, E. S., Alvarez-Filip, L., Oliver, T. A., McClanahan, T. R., and Côté, I. M. (2012). Evaluating life-history strategies of reef corals from species traits. Ecol. Lett. 15, 1378–1386. doi: 10.1111/j.1461-0248.2012.01861.x
Darling, E. S., Graham, N. A. J., Januchowski-Hartley, F. A., Nash, K. L., Pratchett, M. S., and Wilson, S. K. (2017). Relationships between structural complexity, coral traits, and reef fish assemblages. Coral Reefs 36, 561–575. doi: 10.1007/s00338-017-1539-z
DeCarlo, T. M., Harrison, H. B., Gajdzik, L., Alaguarda, D., Rodolfo-Metalpa, R., D’Olivo, J., et al. (2019). Acclimatization of massive reef-building corals to consecutive heatwaves. Proc. R. Soc. B Biol. Sci. 286:20190235. doi: 10.1098/rspb.2019.0235
Eakin, C. M., Morgan, J. A., Heron, S. F., Smith, T. B., Liu, G., Alvarez-Filip, L., et al. (2010). Caribbean corals in crisis: record thermal stress. bleaching, and mortality in 2005. PLoS One 5:e13969. doi: 10.1371/journal.pone.0013969
Edmunds, P. J. (2018). Implications of high rates of sexual recruitment in driving rapid reef recovery in Mo’orea. French Polynesia. Sci. Rep. 8, 1–11.
Fisch, J., Drury, C., Towle, E. K., Winter, R. N., and Miller, M. W. (2019). Physiological and reproductive repercussions of consecutive summer bleaching events of the threatened Caribbean coral Orbicella faveolata. Coral Reefs 38, 863–876. doi: 10.1007/s00338-019-01817-5
Fordyce, A. J., Ainsworth, T. A., Heron, S. F., and Leggat, W. (2019). Marine heatwave hotspots in coral reef environments: physical drivers, ecophysiological outcomes, and impact upon structural complexity. Front. Mar. Sci. 6:498. doi: 10.3389/fmars.2019.00498
Gates, R. D., Baghdasarian, G., and Muscatine, L. (1992) Temperature stress causes host cell detachment in symbiotic cnidarians: implications for coral bleaching. Biol. Bull. 182, 324–332. doi: 10.2307/1542252
Grottoli, A. G., Rodrigues, L. J., and Juarez, C. (2004). Lipids and stable carbon isotopes in two species of Hawaiian corals, porites compressa and montipora verrucosa, following a bleaching event. Mar. Biol. 145, 621–631.
Grottoli, A. G., Rodrigues, L. J., and Palardy, J. E. (2006). Heterotrophic plasticity and resilience in bleached corals. Nature 440, 1186–1189. doi: 10.1038/nature04565
Grottoli, A. G., Warner, M. E., Levas, S. J., Aschaffenburg, M. D., Schoepf, V., McGinley, M., et al. (2014). The cumulative impact of annual coral bleaching can turn some coral species winners into losers. Glob Change Biol. 20, 3823–3833. doi: 10.1111/gcb.12658
Guest, J. R., Low, J., Tun, K., Wilson, B., Ng, C., Raingeard, D., et al. (2016). Coral community response to bleaching on a highly disturbed reef. Sci. Rep. 6, 1–10.
Hall, V. R., and Hughes, T. P. (1996). Reproductive strategies of modular organisms: comparative studies of reef- building corals. Ecology 77, 950–963. doi: 10.2307/2265514
Hughes, T. P., Anderson, K. D., Connolly, S. R., Heron, S. F., Kerry, J. T., Lough, J. M., et al. (2018a). Spatial and temporal patterns of mass bleaching of corals in the anthropocene. Science 359, 80–83. doi: 10.1126/science.aan8048
Hughes, T. P., Kerry, J. T., Baird, A. H., Connolly, S. R., Dietzel, A., Eakin, C. M., et al. (2018b). Global warming transforms coral reef assemblages. Nature 556, 492–496. doi: 10.1038/s41586-018-0041-2
Hughes, T. P., Kerry, J. T., Álvarez-Noriega, M., Álvarez-Romero, J. G., Anderson, K. D., Baird, A. H., et al. (2017). Global warming and recurrent mass bleaching of corals. Nature 543, 373–377.
Hughes, T. P., Kerry, J. T., Baird, A. H., Connolly, S. R., Chase, T. J., Dietzel, A., et al. (2019a). Global warming impairs stock–recruitment dynamics of corals. Nature 568, 387–390. doi: 10.1038/s41586-019-1081-y
Hughes, T. P., Kerry, J. T., Connolly, S. R., Baird, A. H., Eakin, C. M., Heron, S. F., et al. (2019b). Ecological memory modifies the cumulative impact of recurrent climate extremes. Nat. Clim. Change 9, 40–43. doi: 10.1038/s41558-018-0351-2
Imbs, A. B., and Yakovleva, I. M. (2012). Dynamics of lipid and fatty acid composition of shallow-water corals under thermal stress: an experimental approach. Coral Reefs 31, 41–53. doi: 10.1007/s00338-011-0817-4
Jokiel, P. L., and Brown, E. K. (2004). Global warming, regional trends and inshore environmental conditions influence coral bleaching in Hawaii. Glob Change Biol. 10, 1627–1641. doi: 10.1111/j.1365-2486.2004.00836.x
Jokiel, P. L., Rodgers, K. S., Brown, E. K., Kenyon, J. C., Aeby, G., Smith, W. R., et al. (2015). Comparison of methods used to estimate coral cover in the Hawaiian Islands. PeerJ 3:e954. doi: 10.7717/peerj.954
Jones, R. J. (2008). Coral bleaching, bleaching-induced mortality, and the adaptive significance of the bleaching response. Mar. Biol. 154, 65–80. doi: 10.1007/s00227-007-0900-0
Jury, C. P., and Toonen, R. J. (2019). Adaptive responses and local stressor mitigation drive coral resilience in warmer, more acidic oceans. Proc. R. Soc. B 286:20190614. doi: 10.1098/rspb.2019.0614
Kenkel, C. D., and Matz, M. V. (2016). Gene expression plasticity as a mechanism of coral adaptation to a variable environment. Nat. Ecol. Evol. 1:14.
Kim, S. W., Sampayo, E. M., Sommer, B., Sims, C. A., Gómez-Cabrera, M. C., Dalton, S. J., et al. (2019). Refugia under threat: mass bleaching of coral assemblages in high-latitude eastern Australia. Glob. Change Biol. 25, 3918–3931. doi: 10.1111/gcb.14772
Ladd, M. C., Shantz, A. A., Bartels, E., and Burkepile, D. E. (2017). Thermal stress reveals a genotype-specific tradeoff between growth and tissue loss in restored Acropora cervicornis. Mar. Ecol. Prog. Ser. 572, 129–139. doi: 10.3354/meps12169
LaJeunesse, T. C., Thornhill, D. J., Cox, E. F., Stanton, F. G., Fitt, W. K., and Schmidt, G. W. (2004). High diversity and host specificity observed among symbiotic dinoflagellates in reef coral communities from Hawaii. Coral Reefs 23, 596–603.
Leggat, W. P., Camp, E. F., Suggett, D. J., Heron, S. F., Fordyce, A. J., Gardner, S., et al. (2019). Rapid coral decay Is associated with marine heatwave mortality events on reefs. Curr. Biol. 29, 2723.e4–2730.e4.
Lenth, R. (2019). Emmeans: Estimated Marginal Means, Aka Least- Squares Means. R Package; Version 1.3.3. Avaliable online at: https://cran.r-project.org/web/packages/emmeans/index.html
Liu, G., Rauenzahn, J., Heron, S., Eakin, M., Skirving, W., Christensen, T., et al. (2013). NOAA coral reef watch 50 km satellite sea surface temperature-based decision support system for coral bleaching management. NOAA Tech. Rep. Nesdis 143:33.
Lowe, R. J., Falter, J. L., Monismith, S. G., and Atkinson, M. J. (2009). Wave-driven circulation of a coastal reef–lagoon system. J. Phys. Oceanogr. 39, 873–893. doi: 10.1175/2008jpo3958.1
Loya, Y., Sakai, K., Yamazato, K., Nakano, Y., Sambali, H., and van Woesik, R. (2001). Coral bleaching: the winners and the losers. Ecol. Lett. 4, 122–131. doi: 10.1046/j.1461-0248.2001.00203.x
Magel, J. M. T., Burns, J. H. R., Gates, R. D., and Baum, J. K. (2019). Effects of bleaching-associated mass coral mortality on reef structural complexity across a gradient of local disturbance. Sci. Rep. 9, 1–12.
Mass, T., Genin, A., Shavit, U., Grinstein, M., and Tchernov, D. (2010). Flow enhances photosynthesis in marine benthic autotrophs by increasing the efflux of oxygen from the organism to the water. Proc. Natl. Acad. Sci. 107, 2527–2531. doi: 10.1073/pnas.0912348107
Matz, M. V., Treml, E. A., Aglyamova, G. V., and Bay, L. K. (2018). Potential and limits for rapid genetic adaptation to warming in a great barrier reef coral. PLoS Genet. 14:e1007220. doi: 10.1371/journal.pgen.1007220
McClanahan, T. R. (2004). The relationship between bleaching and mortality of common corals. Mar. Biol. 144, 1239–1245. doi: 10.1007/s00227-003-1271-9
Morrow, K. M., Muller, E., and Lesser, M. P. (2018). “How does the coral microbiome cause, respond to, or modulate the bleaching process?,” in Coral Bleaching: Patterns, Processes, Causes and Consequences, Ecological Studies, eds M. J. H. van Oppen, and J. M. Lough, (Cham: Springer International Publishing), 153–188. doi: 10.1007/978-3-319-75393-5_7
Muller-Parker, G., D’Elia, C. F., and Cook, C. B. (2015). “Interactions Between Corals and Their Symbiotic Algae,” in Coral Reefs in the Anthropocene, ed. C. Birkeland, (Netherlands: Springer), 99–116. doi: 10.1007/978-94-017-7249-5_5
Muscatine, L., and Porter, J. W. (1977). Reef corals: mutualistic symbioses adapted to nutrient-poor environments. Bioscience 27, 454–460. doi: 10.2307/1297526
Padilla-Gamiño, J. L., Pochon, X., Bird, C., Concepcion, G. T., and Gates, R. D. (2012). From parent to gamete: vertical transmission of Symbiodinium (Dinophyceae) ITS2 sequence assemblages in the reef building coral Montipora capitata. PLoS One 7:e38440. doi: 10.1371/journal.pone.0038440
Palumbi, S. R., Barshis, D. J., Traylor-Knowles, N., and Bay, R. A. (2014). Mechanisms of reef coral resistance to future climate change. Science 344, 895–898. doi: 10.1126/science.1251336
Pratchett, M. S., Hoey, A. S., Wilson, S. K., Messmer, V., and Graham, N. A. J. (2011). Changes in biodiversity and functioning of reef fish assemblages following coral bleaching and coral loss. Diversity 3, 424–452. doi: 10.3390/d3030424
Putnam, H. M., Barott, K. L., Ainsworth, T. D., and Gates, R. D. (2017). The vulnerability and resilience of reef-building corals. Curr. Biol. 27, R528–R540.
Putnam, H. M., and Edmunds, P. J. (2011). The physiological response of reef corals to diel fluctuations in seawater temperature. J. Exp. Mar. Biol. Ecol. 396, 216–223. doi: 10.1016/j.jembe.2010.10.026
R Core Team, (2019). R: A Language and Environment for Statistical Computing. Vienna: R Found Stat Comput. doi: 10.1016/j.jembe.2010.10.026
Raymundo, L. J., Burdick, D., Hoot, W., Miller, R., Brown, V., Reynolds, T., et al. (2019). Successive bleaching events cause mass coral mortality in Guam. Micronesia. Coral Reefs 38, 677–700. doi: 10.1007/s00338-019-01836-2
Richardson, L. E., Graham, N. A. J., Pratchett, M. S., Eurich, J. G., and Hoey, A. S. (2018). Mass coral bleaching causes biotic homogenization of reef fish assemblages. Glob. Change Biol. 24, 3117–3129.
Ritson-Williams, R. D., and Gates, R. D. (2020). Coral community resilience to successive years of bleaching in K‘ne’ohe Bay, Hawai’i. Coral Reefs. doi: 10.1007/s00338-020-01944-4
Rodrigues, L. J., and Grottoli, A. G. (2007). Energy reserves and metabolism as indicators of coral recovery from bleaching. Limnol. Oceanogr. 52, 1874–1882. doi: 10.4319/lo.2007.52.5.1874
Rowan, R., Knowlton, N., Baker, A., and Jara, J. (1997). Landscape ecology of algal symbionts creates variation in episodes of coral bleaching. Nature 388, 265–269. doi: 10.1038/40843
Sale, T. L., Marko, P. B., Oliver, T. A., and Hunter, C. L. (2019). Assessment of acclimatization and subsequent survival of corals during repeated natural thermal stress events in Hawai‘i. Mar. Ecol. Prog. Ser. 624, 65–76. doi: 10.3354/meps13031
Sampayo, E. M., Ridgway, T., Bongaerts, P., and Hoegh-Guldberg, O. (2008). Bleaching susceptibility and mortality of corals are determined by fine-scale differences in symbiont type. Proc. Natl. Acad. Sci. U.S.A. 105, 10444–10449. doi: 10.1073/pnas.0708049105
Schoepf, V., Carrion, S. A., Pfeifer, S. M., Naugle, M., Dugal, L., Bruyn, J., et al. (2019). Stress-resistant corals may not acclimatize to ocean warming but maintain heat tolerance under cooler temperatures. Nat. Commun. 10, 1–10.
Schoepf, V., Stat, M., Falter, J. L., and McCulloch, M. T. (2015). Limits to the thermal tolerance of corals adapted to a highly fluctuating, naturally extreme temperature environment. Sci. Rep. 5:17639.
Stachowicz, J. J., Bruno, J. F., and Duffy, J. E. (2007). Understanding the effects of marine biodiversity on communities and ecosystems. Annu. Rev. Ecol. Evol. Syst. 38, 739–766. doi: 10.1146/annurev.ecolsys.38.091206.095659
Stimson, J. (2015). Long-term record of nutrient concentrations in Kāne ‘ohe Bay, O ‘ahu, Hawai ‘i, and its relevance to onset and end of a phase shift involving an indigenous alga, Dictyosphaeria cavernosa. Pac. Sci. 69, 319–339. doi: 10.2984/69.3.3
Sully, S., Burkepile, D. E., Donovan, M. K., Hodgson, G., and van Woesik, R. (2019). A global analysis of coral bleaching over the past two decades. Nat. Commun. 10:1264.
Swain, T. D., Vega-Perkins, J. B., Oestreich, W. K., Triebold, C., DuBois, E., Henss, J., et al. (2016). Coral bleaching response index: a new tool to standardize and compare susceptibility to thermal bleaching. Glob. Change Biol. 22, 2475–2488. doi: 10.1111/gcb.13276
Van Oppen, M. J., Gates, R. D., Blackall, L. L., Cantin, N., Chakravarti, L. J., Chan, W. Y., et al. (2017). Shifting paradigms in restoration of the world’s coral reefs. Glob. Change Biol. 23, 3437–3448.
Van Oppen, M. J., Oliver, J. K., Putnam, H. M., and Gates, R. D. (2015). Building coral reef resilience through assisted evolution. Proc. Natl. Acad. Sci. U.S.A. 112, 2307–2313. doi: 10.1073/pnas.1422301112
Vega Thurber, R., Burkepile, D. E., Fuchs, C., Shantz, A. A., McMinds, R., and Zaneveld, J. (2014). Chronic nutrient enrichment increases prevalence and severity of coral disease and bleaching. Glob. Change Biol. 20, 544–554. doi: 10.1111/gcb.12450
Wall, C. B., Ritson-Williams, R., Popp, B. N., and Gates, R. D. (2019). Spatial variation in the biochemical and isotopic composition of corals during bleaching and recovery. Limnol. Oceanogr. 64, 2011–2028. doi: 10.1002/lno.11166
Ward, S., Harrison, P., and Hoegh-Guldberg, O. (2000). Coral bleaching reduces reproduction of scleractinian corals and increases susceptibility to future stress. Proc. Int. Coral Reef Symp. 2, 1123–1128.
Wiedenmann, J., D’Angelo, C., Smith, E. G., Hunt, A. N., Legiret, F., Postle, A. D., et al. (2013). Nutrient enrichment can increase the susceptibility of reef corals to bleaching. Nat. Clim. Change 3, 160–164. doi: 10.1038/nclimate1661
Keywords: Montipora capitata, Porites compressa, coral bleaching, mortality, symbiosis, thermal stress, ecology
Citation: Matsuda SB, Huffmyer AS, Lenz EA, Davidson JM, Hancock JR, Przybylowski A, Innis T, Gates RD and Barott KL (2020) Coral Bleaching Susceptibility Is Predictive of Subsequent Mortality Within but Not Between Coral Species. Front. Ecol. Evol. 8:178. doi: 10.3389/fevo.2020.00178
Received: 12 December 2019; Accepted: 19 May 2020;
Published: 12 June 2020.
Edited by:
Cesar A. Cardenas, Instituto Antártico Chileno (INACH), ChileReviewed by:
C. Mark Eakin, National Oceanic and Atmospheric Administration (NOAA), United StatesJustin H. Baumann, University of North Carolina at Chapel Hill, United States
Sylvain Agostini, University of Tsukuba, Japan
Copyright © 2020 Matsuda, Huffmyer, Lenz, Davidson, Hancock, Przybylowski, Innis, Gates and Barott. This is an open-access article distributed under the terms of the Creative Commons Attribution License (CC BY). The use, distribution or reproduction in other forums is permitted, provided the original author(s) and the copyright owner(s) are credited and that the original publication in this journal is cited, in accordance with accepted academic practice. No use, distribution or reproduction is permitted which does not comply with these terms.
*Correspondence: Katie L. Barott, a2Jhcm90dEBzYXMudXBlbm4uZWR1
†ORCID: Shayle B. Matsuda, orcid.org/0000-0002-4354-0133; Ariana S. Huffmyer, orcid.org/0000-0002-9170-0971