- Animal Behaviour and Cognition Lab, School of Psychology, University of Newcastle, Callaghan, NSW, Australia
Wildlife species are harvested for a variety of purposes. This is known to drive phenotypic change, particularly in the context of exploitative harvesting. As the impact of invasive alien species grows, and new conflicts with nuisance species arise, management of these problematic species has emerged as a key topic. Yet there is little to no attention directed to whether and how species are changing as a consequence of lethal control, particularly in terms of their behavior. In this synthesis, we draw attention to the fact that nuisance and invasive species undergoing control are likely to exhibit behavioral change. First, we highlight the potential consequences of behavioral responses to control on species’ management and ecological impact. Second, we provide a framework of mechanisms that can lead to behavioral responses to lethal control. Three categories are described: evolutionary mechanisms, cognitive mechanisms, and stress mechanisms. Understanding which mechanism underlies a behavioral response is paramount as it allows to predict how prevalent the response will be in the population. We argue for increased monitoring of behavior by managers and more research efforts into the mechanisms of behavioral responses to novel threats in order to better predict and mitigate unforeseen and unwanted behavioral change.
Introduction
Humans have had profound effects on wildlife for millennia (Burney and Flannery, 2005; Sullivan et al., 2017), yet anthropogenic impacts are now reaching unprecedented global scales. Habitat transformation and long-range translocation of animal species are modifying entire ecosystems, altering biological communities and trophic relationships and creating new selective landscapes to which organisms must adapt or go extinct. Compounded by climate change and global contamination, these effects make humans the most impactful and fastest driver of phenotypic change in wild animals (Palumbi, 2001; Hendry et al., 2008; Darimont et al., 2009).
Exploitative harvesting of wildlife by humans for food, clothes, medicines, and entertainment is a direct driver of phenotypic change. Unlike habitat transformation and animal translocation, which modify the environments that sustain them, exploitative harvesting, a form of anthropogenic predation, is known to shape the demography, morphology, population dynamics, life-history, and behavior of harvested species (Allendorf and Hard, 2009; Palkovacs et al., 2018). Well documented examples include trophy hunting, which has led to the evolution of smaller horns in bighorn sheep, Ovis canadensis (Pigeon et al., 2016), and passive fishing gear, such as angling and gill-netting, which induces behavioral timidity in harvested fish populations (Díaz Pauli et al., 2015; Arlinghaus et al., 2017; Andersen et al., 2018).
Despite being an evolutionarily more recent form of anthropogenic predation than exploitative harvesting, wildlife control is, as we argue here, equally likely to produce phenotypical trait change. While many species are struggling to adjust to rapidly-changing and human-dominated landscapes, a few are thriving to a point where populations need to be controlled (Figure 1). Nuisance species are local species, which often take advantage of anthropogenic resources and come into conflict with the surrounding human communities (Barrett et al., 2019). Invasive alien species are non-indigenous species, which have been introduced to a novel environment and come to proliferate (Blackburn et al., 2011). In the United States alone, nuisance and invasive animals cause billions of dollars of ecological and economical damage each year (Pimentel et al., 2000; Pimentel et al., 2005). As a consequence, many nuisance and invasive populations are the target of control programs which aim to contain or eradicate their populations (Mack et al., 2000).
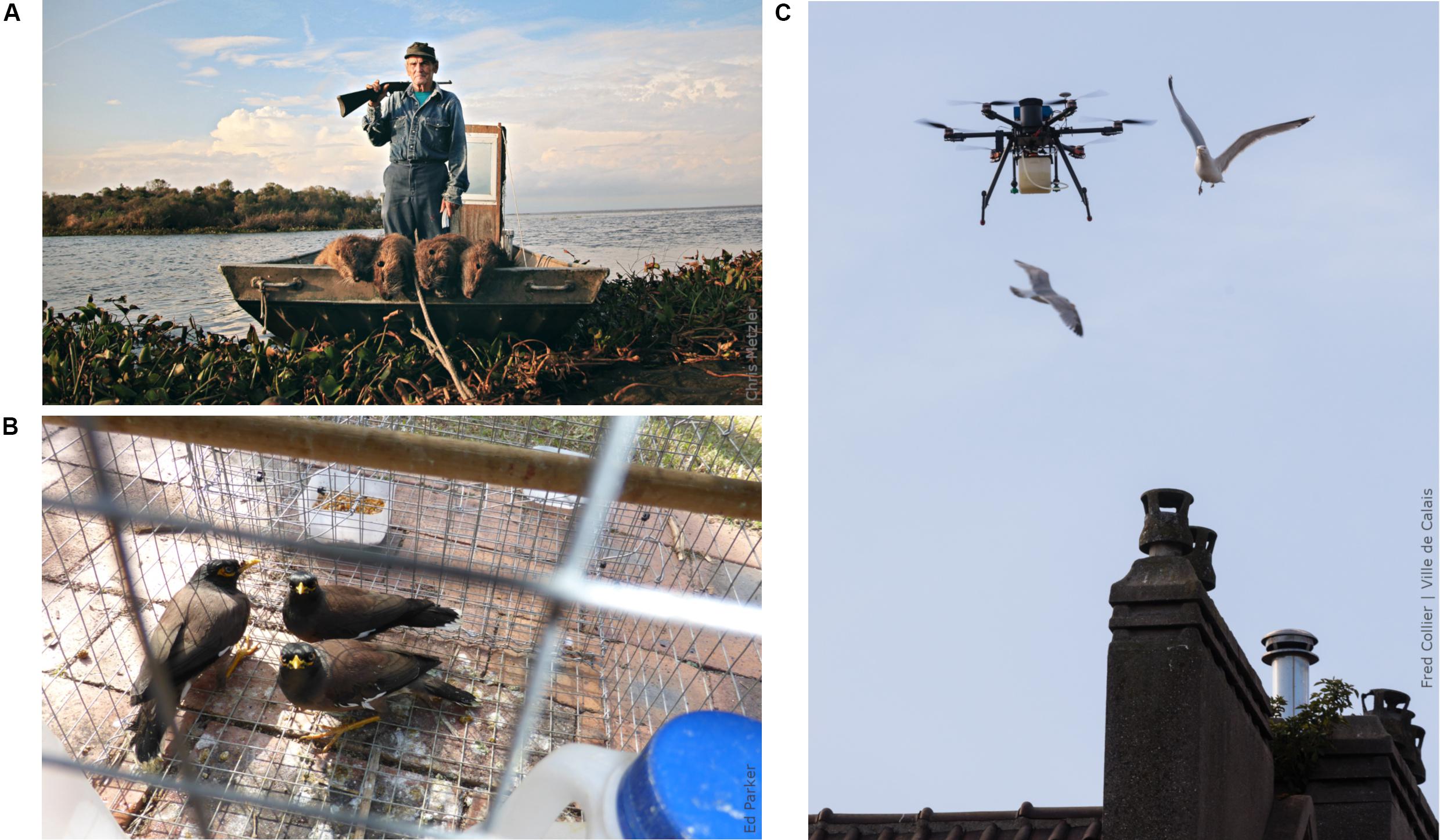
Figure 1. Nuisance and invasive species are controlled by a range of methods and devices. (A) Nutria (Myocastor coypus) hunter in Louisiana, United States. Nutria have been introduced to North America, Europe and Asia from South America. Picture from “rodents of unusual size,” documentary by Quinn Costello, Chris Metzler and Jeff Springer. (B) Common mynas (Acridotheres tristis) in a walk-in baited trap in Queensland, Australia. Common mynas are invasive across the east coast of Australia, many local control programs rely exclusively on such traps. Picture by Ed Parker. (C) Drone intervention to sterilize seagull eggs in Calais, France. A number of French cities control urban seagull populations to reduce nuisance. Picture by Fred Collier | Ville de Calais.
Attention to whether and how wildlife is changing as a consequence of lethal control is quasi inexistent, even though some have begun examining how removing invasive and pest species might alter ecological function, and warning that such interventions might have unexpected/undesirable consequences (Zavaleta et al., 2001; Wallach et al., 2010; Kopf et al., 2017). The possibility that wildlife responds adaptively, which is relevant to both maintaining the effectiveness of population management, and understanding more generally how wild populations respond to anthropic influences, has been almost completely neglected until very recently (Côté et al., 2014; Diquelou et al., 2018; Diquelou and Griffin, 2019). Furthermore, a broad understanding of the proximate mechanisms underpinning change and how this knowledge can be used by managers has so far been overlooked (Diquelou et al., 2018; Závorka et al., 2018).
Our goal is to increase attention to behavioral responses to lethal control. We focus on behavior because behavior constitutes the interface by which animals respond to changes in their environment, including novel threats such as human predation (Wong and Candolin, 2015). First, we examine why behavioral responses to lethal control are an important issue by listing their potential consequences for management programs and the surrounding wildlife. Second, we disentangle the possible proximate causes of these behavioral responses and organize them into a cohesive framework, which allows to predict the scope of their impact. We conclude by encouraging scientists to undertake more research on the topic and urging managers to integrate the potential for behavioral change into nuisance species management strategies.
Potential Consequences of Behavioral Responses
The first and most obvious type of behavioral response is one that reduces the likelihood that individuals are removed or captured, thereby jeopardizing the long-term sustainability of management interventions. This effect is well documented in the context of exploitative harvesting, but is not being considered in a management context. For example, passive fishing gear selectively captures behaviorally bold and active individuals, leaving behind more timid individuals, and lowering yields (Díaz Pauli et al., 2015; Andersen et al., 2018). Similarly, a number of examples including elks (Cervus elaphus), brown bear (Ursus arctos), and pheasants (Phasianus colchicus) show that shyer and slower individuals survive the hunting season better (Ciuti et al., 2012a; Madden and Whiteside, 2014; Leclerc et al., 2019). In the Bahamas, on reefs where lionfish (Pterois volitans) are controlled by spearfishers, the invasive predatory fish change their behavior in a way that makes them harder to control (Côté et al., 2014). Overall, as behavior mediates the vulnerability of individuals to capture, behavioral responses to control represent a major risk for managers’ ability to reliably remove individuals from populations.
The second, subtler, category of behavioral response is one that erroneously inflates the apparent effectiveness of an intervention. Monitoring a species abundance in order to evaluate the effectiveness of a control effort should form part of any well-designed control program. Changes in behavior that make a species more cryptic can cause abundance to be underestimated, however. For example, in areas of Australia where common mynas have been heavily trapped, individuals are less detectable during surveys (Diquelou et al., 2018). While reduced detectability might be a desirable outcome for a species which is merely a pest to humans, it would be highly detrimental to reduce management pressure based on erroneous abundance trends for a species that poses a threat to the ecosystem.
The third and potentially most concerning category of behavioral responses to control includes behavioral changes that modify, potentially for the worse, the impact of the very species for which control is being undertaken. This can occur if a species changes the timing of its activities. For example, control by spearfishers has made lionfish not only harder to control, the practice has also shifted their activity patterns toward twilight. This has increased the risk of encounters between lionfish and native species which were previously unaffected (Côté et al., 2014). Changes in space use can also alter the ecological impact of a species. For example, common mynas undergo heavy trapping pressure in suburban habitats where the human demographic is particularly intolerant of the species (Diquelou, 2017). In the long-term, this could cause mynas to invade more pristine habitats to escape trapping pressure, which would increase the likelihood of competitive interactions with native secondary cavity-nesting birds. Managers need to become aware that such changes in behavior are not only possible, but likely.
A Mechanistic Framework of Behavioral Responses to Lethal Control
Faced with a novel lethal threat, populations can adjust their behavior via a range of evolutionary, cognitive and hormonal mechanisms. Here, we review and organize behavioral responses to control and their underlying mechanisms into a cohesive and comprehensive framework and use the framework to predict the prevalence and speed of behavioral responses in controlled populations. We identify three types of compensatory responses: those that involve evolutionary selection, those that involve cognition, and those that involve stress. The organizing framework not only helps make sense of the relationships between mechanism and behavioral change; it also generates the novel prediction that different mechanisms will cause variation in the prevalence of behavioral change (Figure 2).
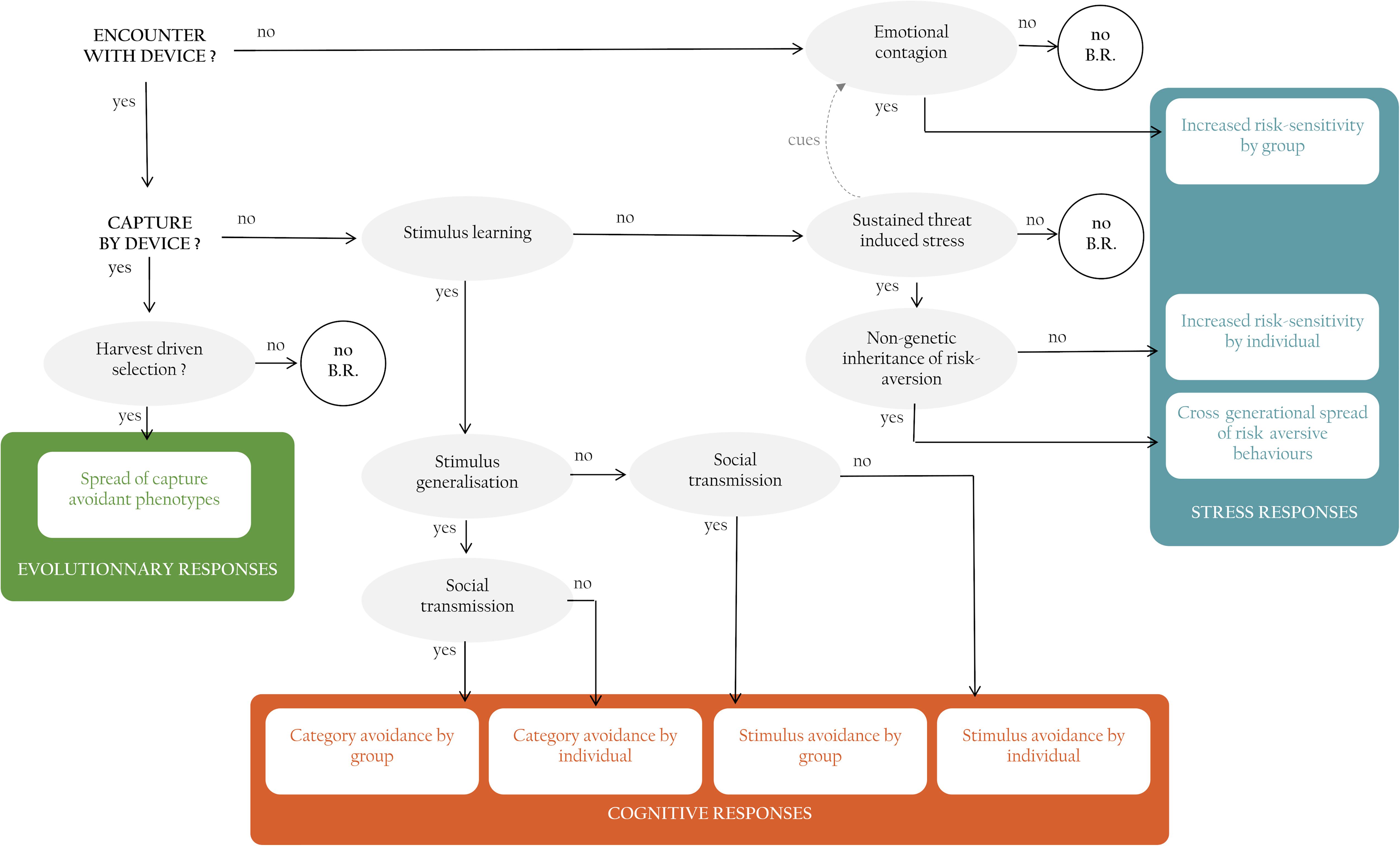
Figure 2. Framework linking interactions of individuals with management devices (in bold black capitals), to mechanisms (in gray circles) to the different types of behavioral responses possible displayed by individuals, and/or populations (in colored boxes). Behavioral responses are grouped into three categories (see text), in some cases, combinations of events and mechanisms do not elicit a behavioral response (white circles with NO B.R.).
We begin with the hypothetical scenario where a management intervention is put into place, targeting a population of invasive or nuisance animals. We refer to this novel threat as a “device” but envisage that this can be any control procedure from a person with a gun, a trap, to a poisoned bait. We employ the term capture but mean it to refer more broadly to any type of outcome whereby an animal is removed from the population, including but not only by death.
Evolutionary Responses
Upon first encounter with a control device an animal may either be captured or not (Figure 2). If it is captured, any genetically based attributes of the captured individual, will be removed from the population. If capture devices target individuals with some heritable behavioral attribute preferentially, then there is scope for directional selection to occur (Uusi-Heikkilä et al., 2008; Allendorf and Hard, 2009). As individuals with behaviors that make them vulnerable to capture are removed, across many generations, this process has the potential to lead to capture-resistant populations. Evolutionarily-driven behavioral change only occurs under limited conditions, however (Festa-Bianchet, 2017). Without a strong directional selective pressure applied to a behavioral trait with a strong heritable component, evolutionarily-driven behavioral change cannot arise (Figure 2).
Although, directional selection of fish based on their behavior is suspected to occur in the context of commercial and recreational fishing (Uusi-Heikkilä et al., 2008; Díaz Pauli and Sih, 2017; Andersen et al., 2018), whether this leads to evolutionary-driven behavioral change is sometimes contested. Even for personality traits, which are presumed to have a strong heritability component, heritability can range from relatively high (Dingemanse et al., 2002; Drent et al., 2003) to very low (Morinay et al., 2019). Estimating the heritability of capture-resistant behavior or personalities is one of the key challenges laying ahead of researchers in order to demonstrate evolutionary behavioral responses to harvesting (Díaz Pauli and Sih, 2017). Yet, long-term pedigree studies such as the ones that would be needed may prove to be difficult to fund in the field of management ecology.
Cognitive Responses
If an individual encounters a capture device but is not caught or escapes, it can change its behavior via a suite of cognitive mechanisms. First, it can learn the cues that predict the control device, and therefore avoid it. The ability to learn predictive relationships between arbitrary novel cues, such as lights and sounds, and biologically important outcomes such as food or pain, is ubiquitous amongst animals (Dukas, 1998; Brembs, 2003; Heyes, 2012). Animals have the potential to learn the features of the capture device (Mineka and Cook, 1988; van Heezik et al., 1999; Griffin et al., 2001; Shier and Owings, 2007; Griffin, 2008), the contextual cues that predict the capture device (Fanselow, 2000), and the place and time in which the capture device occurs (O’Brien and Sutherland, 2007; Ferrari and Chivers, 2009; Griffin and Boyce, 2009). If the capture device is a bait, then conditioned taste aversion is another form of prediction learning which will allow individuals to learn the olfactory and taste cues associated with the lethal chemical (Garcia et al., 1955; Domjan, 1980; Gustavson and Gustavson, 1982).
The taxonomic ubiquity of prediction learning makes it highly likely that learning will occur in the control context with some conditions. For such learning to occur, the novel cue must be paired with a reinforcer such as the individual’s own fear elicited by a near escape of the capture device. For bait learning through taste aversion, the animal must experience gastro-intestinal sickness within a few hours of ingesting the bait (Domjan, 1980). Learning device-related cues is more likely if learning is not limited by evolutionary predispositions that constrain which associations can be learned. Biological predispositions to learn facilitate associations of fear with fear-relevant stimuli and inhibit associations of fear with fear-irrelevant stimuli (Cook and Mineka, 1989; Griffin et al., 2002; Brown et al., 2011). For example, tammar wallabies (Macropus eugenii) acquire an antipredator response to a fear-relevant stimulus (fox), but not to a fear-irrelevant stimulus (goat; Griffin et al., 2002).
Whether or not stimulus learning will lead to a capture-avoidant individuals depends not only on individual learning capacity, however. It will depend on whether the learner also expresses acquired avoidance to other, similar-looking cues. This cognitive ability known as stimulus generalization is widespread in the context of threat learning. Prey species show adaptive patterns of generalization from learned to related cues without further pairings of these cues with indicators of risk (e.g., social alarm signals) or attack (Griffin et al., 2001; Brown et al., 2011). Generalization gradients depend on the level of risk associated with the threat (Ferrari et al., 2008) and its certainty (Ferrari et al., 2016). Generalization has important implications in the case of learning about control devices. If individuals learn to avoid one specific device then they might still fall victim to a slightly different one. However, if individuals generalize to all devices, then the effectiveness of a management program that relies solely on this method could be reduced.
Although generalization appears common in the threat context, the extent to which generalization will alter an individual’s response to lethal control will depend upon multiple factors (Shettleworth, 2010). For example, the more perceptually similar two control devices are, the more likely generalization of avoidance from one to the other will be. Experience with certain types of objects increases individuals’ ability to differentiate and categorize objects of that type. Considering, many nuisance and invasive species rely on anthropogenic resources, their extensive experience of anthropogenic structures makes it more likely that they will finely categorize control devices. These species will have the capacity to place control devices into one perceptual category, making it more likely that they will avoid not only the one with which they had an aversive encounter, but all of them. Whether humans associated with trapping might also become avoided is more difficult to predict. On one hand, humans have frontally placed eyes, a predator-typical feature for many species of prey (Caro, 2005). Hence, humans could engage evolutionary established patterns of predator generalization. On the other hand, for species that also rely upon human provisioning, generalizing avoidance to all humans could carry a cost in terms of missed foraging opportunities. Accordingly, several urbanized bird species discriminate between humans that provide food and humans that represent a threat (Levey et al., 2009; Belguermi et al., 2011; Lee et al., 2019), including those directly involved in captures (Marzluff et al., 2010; Diquelou and Griffin, 2019). Overall, commensal species that rely on anthropogenic resources and live in proximity with anthropogenic structures are expected to generalize avoidance across control devices and to discriminate safe from dangerous humans.
Learning and generalization will have population-level effects as long as personal experience with a threat can spread to naïve individuals via social learning (Figure 2). Acquisition of threat-related information from conspecifics is commonplace across taxa (Brown and Laland, 2003; Griffin, 2004). Many animals modify their behavior after witnessing a conspecific undergoing a negative interaction with a threat. Of course, opportunities for learning from conspecifics will increase as the level of sociality increases and interactions between group members are more numerous. In management programs, social learning about control devices has the potential to alter management success dramatically as more and more individuals become avoidant of devices which they have associated with injured, distressed or dead conspecifics. For example, free-ranging common mynas become avoidant of control-like situations after witnessing conspecifics being captured in trap-like structures (Diquelou and Griffin, 2019). Considering walk-in traps are virtually the only method employed to manage this species, social learning could lead to a population-wide decrease in control success.
Stress Responses
Rather than learning about the cues that predict control devices, animals might also spontaneously recognize some aspect of the control context as threatening, which in turn can cause sustained threat-induced stress (Figure 2). When encountering a threat (generally a predator) animals can experience high stress which can induce long lasting effects such as hypervigilance and increased physiological stress levels, akin to certain human stress disorders (Clinchy et al., 2013; Zanette et al., 2019). This response relies on spontaneous recognition of the threat as a danger. Most control procedures, such as traps and poison, are unlikely to appear threatening upon the first encounter as they lack similarity with threats with which animals have eco-evolutionary experience (Carthey and Blumstein, 2018). On the other hand, many species respond to humans as predators with for consequence that human-operated devices may elicit spontaneous fear (Frid and Dill, 2002; Ciuti et al., 2012b). For example, in Great Britain, badgers (Meles meles) are hunted for sport and controlled for the damage they inflict on farms. Badgers are more afraid of humans than of their other current or past natural predators (Clinchy et al., 2016) which could reflect sustained human-induced stress. Overall, sustained control-induced stress could change the behavior of controlled animals by inducing increased sensitivity and responsiveness to danger. This could lead to generalized risk-aversion and a state of hypervigilance in individuals, leading these individuals to be less likely to approach and be caught by control devices.
So far, we have considered scenarios in which behavioral responses are triggered by an encounter with a control device (Figure 2). It is important to realize that behavioral responses may also occur in individuals that do not encounter the device, however (Figure 2). The perceived risk of encountering predators varies across space, forming what is referred to as the landscape of fear, which affects behavior and habitat selection of prey (Laundré et al., 2001; Thomson et al., 2006; Ciuti et al., 2012b). This perceived landscape of fear can be informed by social partners. It is well established that animals can display social cues that inform conspecifics about the level of danger in the environment beyond the context of the specific threat (Danchin et al., 2004). Emotional contagion is the process through which the emotional state of one individual influences that of other individuals it encounters (Preston and de Waal, 2002; Špinka, 2012; Nakahashi and Ohtsuki, 2018). Fear contagion is a fast occurring phenomenon whereby an observer becomes fearful in response to unconditional social stimuli such as cues or signals of fear emitted by a conspecific (Špinka, 2012). For example zebrafish (Danio rerio) that observe distressed conspecifics, even without seeing the source of the danger, become alarmed and show increases in anti-predator behavior (Fernandes Silva et al., 2019). Control devices, just like predators, could form landscapes of variable perceived predation risk, and conspecifics familiar with the threat and heightened general wariness (e.g., presenting high levels of vigilance, emitting alarm calls, or chemical signals) could cause individuals with no experience of the control threat to become more fearful and behave more cautiously. As a result, naïve individuals might become more reluctant to interact with unfamiliar structures such as traps or taste novel foods such as bait, making them more difficult to attract to control devices. Fear contagion is enhanced by exposure to familiar conspecifics (Fernandes Silva et al., 2019), meaning that social contagion will likely be strongest in species that live is stable social groupings.
There is also now increasing evidence that fear and stress can be passed on from one generation to the next via maternal effects and non-genetic inheritance. Parents living in stressful environments, such as a high-predation environment, can produce young with different behaviors than those from non-stressed parents (Hayward and Wingfield, 2004; Storm and Lima, 2010; Adkins-Regan et al., 2013). In birds these effects can be caused by maternal yolk hormones and parental behavior, including through pre-hatching communication (Henriksen et al., 2011; Tilgar et al., 2011; Adkins-Regan et al., 2013; Mariette and Buchanan, 2016). In some cases, molecular processes regulate the gene expression of affected behaviors, these processes are then called epigenetic and can lead to heritable changes in phenotype (Jablonka and Raz, 2009; Ledón-Rettig et al., 2013). If fearful risk-avoidant individuals are more likely to produce fearful, risk-avoidant offspring that are less likely to approach unfamiliar elements such as control devices, then management interventions may become less and less effective over time.
Conclusion
For the first time, we draw attention to the fact that invasive and nuisance populations that are subject to lethal control are likely to display behavioral change in response. Those responses, while currently mostly overlooked can have important consequences for management and conservation purposes. Behavioral responses can counteract control measures thereby limiting the efficiency and sustainability of control. But less straightforward effects are also likely to occur, in particular behavioral responses can modify, for better or for worse, the ecological impact of the controlled species. Additionally, behavioral change of controlled species can bias counts, thus leading to erroneous abundance estimates.
We propose an exhaustive framework of mechanisms that can lead to behavioral change in response to lethal control. These mechanisms are organized, depending on the pre-requisite events and factors, into one of three categories: evolutionary, stress and cognitive mechanisms (Figure 2). While we explain each of these categories separately, these mechanisms need not be exclusive from one-another and can in fact interact with each other. For example, physiological stress responses can in some cases facilitate, or hinder cognitive mechanisms such as learning (Thaker et al., 2010). Our analysis shows that evolutionary, stress and cognitive mechanism can lead to a range of behavioral changes in response to lethal control. Some behavioral responses will only occur in a limited number of individuals that have encountered and escaped the capture device, such as stimulus avoidance following individual stimulus learning. While on the other hand some mechanisms can lead to population-wide behavioral responses (e.g., emotional contagion, social learning) and even cross-generational responses that spread through harvest-driven selection or non-genetic inheritance. Which mechanism is responsible for the observed behavioral response thus has important consequences for how prevalent the response will be in the controlled population, how fast it will spread and how easy it will be to counteract.
Overall, we argue for increased monitoring of behavior in the management of nuisance and invasive species in order to minimize unwanted and unforeseen behavioral adjustments, but also to advance our understanding of the mechanisms of behavioral changes in response to novel threats.
Author Contributions
MD and AG conceived the manuscript, reviewed published literature, and wrote the manuscript.
Funding
The empirical work that led to the development of this framework was supported by the Australian Pest Animal Research Program, the Ecological Society of Australia, and the Wyong Shire Council.
Conflict of Interest
The authors declare that the research was conducted in the absence of any commercial or financial relationships that could be construed as a potential conflict of interest.
References
Adkins-Regan, E., Banerjee, S. B., Correa, S. M., and Schweitzer, C. (2013). Maternal effects in quail and zebra finches: behavior and hormones. Gen. Comp. Endocrinol. 190, 34–41. doi: 10.1016/j.ygcen.2013.03.002
Allendorf, F. W., and Hard, J. J. (2009). Human-induced evolution caused by unnatural selection through harvest of wild animals. Proc. Natl. Acad. Sci. U.S.A. 106(Suppl. 1) 9987–9994. doi: 10.1073/pnas.0901069106
Andersen, K. H., Marty, L., and Arlinghaus, R. (2018). Evolution of boldness and life history in response to selective harvesting. Can. J. Fish. Aquat. Sci. 75, 271–281. doi: 10.1139/cjfas-2016-0350
Arlinghaus, R., Laskowski, K. L., Alós, J., Klefoth, T., Monk, C. T., Nakayama, S., et al. (2017). Passive gear-induced timidity syndrome in wild fish populations and its potential ecological and managerial implications. Fish Fish. 18, 360–373. doi: 10.1111/faf.12176
Barrett, L. P., Stanton, L. A., and Benson-Amram, S. (2019). The cognition of ‘nuisance’ species. Anim. Behav. 147, 167–177. doi: 10.1016/j.anbehav.2018.05.005
Belguermi, A., Bovet, D., Pascal, A., Prévot-Julliard, A. C., Jalme, M. S., Rat-Fischer, L., et al. (2011). Pigeons discriminate between human feeders. Anim. Cogn. 14, 909–914. doi: 10.1007/s10071-011-0420-7
Blackburn, T. M., Pyšek, P., Bacher, S., Carlton, J. T., Duncan, R. P., Jarošík, V., et al. (2011). A proposed unified framework for biological invasions. Trends Ecol. Evol. 26, 333–339. doi: 10.1016/j.tree.2011.03.023
Brembs, B. (2003). Operant conditioning in invertebrates. Curr. Opin. Neurobiol. 13, 710–717. doi: 10.1016/j.conb.2003.10.002
Brown, C., and Laland, K. N. (2003). Social learning in fishes: a review. Fish Fish. 4, 280–288. doi: 10.1046/j.1467-2979.2003.00122.x
Brown, G. E., Ferrari, M. C. O., Malka, P. H., Russo, S., Tressider, M., and Chivers, D. P. (2011). Generalization of predators and nonpredators by juvenile rainbow trout: learning what is and is not a threat. Anim. Behav. 81, 1249–1256. doi: 10.1016/j.anbehav.2011.03.013
Burney, D. A., and Flannery, T. F. (2005). Fifty millennia of catastrophic extinctions after human contact. Trends Ecol. Evol. 20, 395–401. doi: 10.1016/j.tree.2005.04.022
Caro, T. M. (2005). Antipredator Defenses in Birds and Mammals. London: University of Chicago Press.
Carthey, A. J. R., and Blumstein, D. T. (2018). Predicting predator recognition in a changing world. Trends Ecol. Evol. 33, 106–115. doi: 10.1016/j.tree.2017.10.00
Ciuti, S., Muhly, T. B., Paton, D. G., McDevitt, A. D., Musiani, M., and Boyce, M. S. (2012a). Human selection of elk behavioural traits in a landscape of fear. Proc. R. Soc. London B Biol. Sci. 279, 4407–4416. doi: 10.1098/rspb.2012.1483
Ciuti, S., Northrup, J. M., Muhly, T. B., Simi, S., Musiani, M., Pitt, J. A., et al. (2012b). Effects of humans on behaviour of wildlife exceed those of natural predators in a landscape of fear. PLoS One 7:e50611. doi: 10.1371/journal.pone.0050611
Clinchy, M., Sheriff, M. J., and Zanette, L. Y. (2013). Predator-induced stress and the ecology of fear. Funct. Ecol. 27, 56–65. doi: 10.1111/1365-2435.12007
Clinchy, M., Zanette, L. Y., Roberts, D., Suraci, J. P., Buesching, C. D., Newman, C., et al. (2016). Fear of the human “super predator” far exceeds the fear of large carnivores in a model mesocarnivore. Behav. Ecol. 27:arw117. doi: 10.1093/beheco/arw117
Cook, M., and Mineka, S. (1989). Observational conditioning of fear to fear-relevant versus fear-irrelevant stimuli in rhesus monkeys. J. Abnorm. Psychol. 98, 448–459. doi: 10.1037/0021-843X.98.4.448
Côté, I. M., Darling, E. S., Malpica-Cruz, L., Smith, N. S., Green, S. J., Curtis-Quick, J., et al. (2014). What doesn’t kill you makes you wary? Effect of repeated culling on the behaviour of an invasive predator. PLoS One 9:e94248. doi: 10.1371/journal.pone.0094248
Danchin, É, Giraldeau, L.-A., Valone, T. J., and Wagner, R. H. (2004). Public information: from nosy neighbors to cultural evolution. Science 305, 487–491. doi: 10.1126/science.1098254
Darimont, C. T., Carlson, S. M., Kinnison, M. T., Paquet, P. C., Reimchen, T. E., and Wilmers, C. C. (2009). Human predators outpace other agents of trait change in the wild. Proc. Natl. Acad. Sci. U.S.A. 106, 952–954. doi: 10.1073/pnas.0809235106
Díaz Pauli, B., and Sih, A. (2017). Behavioural responses to human-induced change: why fishing should not be ignored. Evol. Appl. 10, 231–240. doi: 10.1111/eva.12456
Díaz Pauli, B., Wiech, M., Heino, M., and Utne-Palm, A. C. (2015). Opposite selection on behavioural types by active and passive fishing gears in a simulated guppy Poecilia reticulata fishery. J. Fish. Biol. 86, 1030–1045. doi: 10.1111/jfb.12620
Dingemanse, N. J., Both, C., Drent, P. J., van Oers, K., and van Noordwijk, A. J. (2002). Repeatability and heritability of exploratory behaviour in great tits from the wild. Anim. Behav. 64, 929–938. doi: 10.1006/anbe.2002.2006
Diquelou, M. C. (2017). Responses of Invasive Birds to Control: the Case of Common Mynas in Australia. Newcastle: University of Newcastle.
Diquelou, M. C., and Griffin, A. S. (2019). It’s a trap! Invasive common mynas learn socially about control-related cues. Behav. Ecol. 30, 1314–1323. doi: 10.1093/beheco/arz079
Diquelou, M. C., MacFarlane, G. R., and Griffin, A. S. (2018). Investigating responses to control: a comparison of common myna behaviour across areas of high and low trapping pressure. Biol. Invasions 20, 3591–3604. doi: 10.1007/s10530-018-1798-9
Domjan, M. (1980). “Ingestional aversion learning: unique and general processes,” in Advances in the Study of Behavior, Vol. 11, eds J. S. Rosenblatt, R. A. Hinde, C. Beer, and M. C. Busnel (New York, NY: Academic Press), 275–336. doi: 10.1016/s0065-3454(08)60120-5
Drent, P. J., van Oers, K., and van Noordwijk, A. J. (2003). Realized heritability of personalities in the great tit (Parus major). Proc. R. Soc. London B Biol. Sci. 270, 45–51. doi: 10.1098/rspb.2002.2168
Dukas, R. (1998). “Evolutionary ecology of learning,” in Cognitive Ecology. The Evolutionary Ecology of Information Processing and Decision Making, ed. R. Dukas (Chicago, IL: The University of Chicago Press), 129–174.
Fanselow, M. S. (2000). Contextual fear, gestalt memories, and the hippocampus. Behav. Brain Res. 110, 73–81. doi: 10.1016/s0166-4328(99)00186-2
Fernandes Silva, P., Garcia de Leaniz, C., and Luchiari, A. C. (2019). Fear contagion in zebrafish: a behaviour affected by familiarity. Anim. Behav. 153, 95–103. doi: 10.1016/j.anbehav.2019.05.004
Ferrari, M. C. O., and Chivers, D. P. (2009). Temporal variability, threat sensitivity and conflicting information about the nature of risk: understanding the dynamics of tadpole antipredator behaviour. Anim. Behav. 78, 11–16. doi: 10.1016/j.anbehav.2009.03.016
Ferrari, M. C. O., Crane, A. L., and Chivers, D. P. (2016). Certainty and the cognitive ecology of generalization of predator recognition. Anim. Behav. 111, 207–211. doi: 10.1016/j.anbehav.2015.10.026
Ferrari, M. C. O., Messier, F., and Chivers, D. P. (2008). Can prey exhibit threat-sensitive generalization of predator recognition? Extending the predator recognition continuum hypothesis. Proc. R. Soc. London B Biol. Sci. 275, 1811–1816. doi: 10.1098/rspb.2008.0305
Festa-Bianchet, M. (2017). When does selective hunting select, how can we tell, and what should we do about it? Mamm. Rev. 47, 76–81. doi: 10.1111/mam.12078
Frid, A., and Dill, L. M. (2002). Human-caused disturbance as a form of predation risk. Conserv. Ecol. 6:11. doi: 10.1002/jwmg.631
Garcia, J., Kimeldorf, D. J., and Koelling, R. A. (1955). Conditioned aversion to saccharin resulting from exposure to gamma radiation. Science 122, 157–158. doi: 10.1126/science.122.3160.157
Griffin, A. S. (2004). Social learning about predators: a review and prospectus. Anim. Learn. Behav. 32, 131–140. doi: 10.3758/BF03196014
Griffin, A. S. (2008). Social learning about predators: is it just Pavlovian conditioning? Brain Res. Bull. 76, 264–271.
Griffin, A. S., and Boyce, H. M. (2009). Indian mynahs, Acridotheres tristis, learn about dangerous places by observing the fate of others. Anim. Behav. 78, 79–84. doi: 10.1016/j.anbehav.2009.03.012
Griffin, A. S., Evans, C. S., and Blumstein, D. T. (2001). Learning specificity in acquired predator recognition. Anim. Behav. 62, 577–589. doi: 10.1006/anbe.2001.1781
Griffin, A. S., Evans, C. S., and Blumstein, D. T. (2002). Selective learning in a marsupial. Ethology 108, 1103–1114. doi: 10.1046/j.1439-0310.2002.00840.x
Gustavson, C. R., and Gustavson, J. C. (1982). Food avoidance in rats: the differential effects of shock, illness and repellents. Appetite 3, 335–340. doi: 10.1016/S0195-6663(82)80051-2
Hayward, L. S., and Wingfield, J. C. (2004). Maternal corticosterone is transferred to avian yolk and may alter offspring growth and adult phenotype. Gen. Comp. Endocrinol. 135, 365–371. doi: 10.1016/j.ygcen.2003.11.002
Hendry, A. P., Farrugia, T. J., and Kinnison, M. T. (2008). Human influences on rates of phenotypic change in wild animal populations. Mol. Ecol. 17, 20–29. doi: 10.1111/j.1365-294X.2007.03428.x
Henriksen, R., Rettenbacher, S., and Groothuis, T. G. G. (2011). Prenatal stress in birds: pathways, effects, function and perspectives. Neurosci. Biobehav. Rev. 35, 1484–1501. doi: 10.1016/j.neubiorev.2011.04.010
Heyes, C. (2012). Simple minds: a qualified defence of associative learning. Philos. Trans. R. Soc. B Biol. Sci. 367, 2695–2703. doi: 10.1098/rstb.2012.0217
Jablonka, E., and Raz, G. (2009). Transgenerational epigenetic inheritance: prevalence, mechanisms, and implications for the study of heredity and evolution. Quartely Rev. Biol. 84, 1–46.
Kopf, R. K., Nimmo, D. G., Humphries, P., Baumgartner, L. J., Bode, M., Bond, N. R., et al. (2017). Confronting the risks of large scale invasive species control. Nat. Ecol. Evol. 1:0172. doi: 10.1038/s41559-017-0172
Laundré, J. W., Hernández, L., and Altendorf, K. B. (2001). Wolves, elk, and bison: reestablishing the “landscape of fear” in yellowstone national park, U.S.A. Can. J. Zool. 79, 1401–1409. doi: 10.1139/z01-094
Leclerc, M., Zedrosser, A., Swenson, J. E., and Pelletier, F. (2019). Hunters select for behavioral traits in a large carnivore. Sci. Rep. 9:12371. doi: 10.1038/s41598-019-48853-3
Ledón-Rettig, C. C., Richards, C. L., and Martin, L. B. (2013). Epigenetics for behavioral ecologists. Behav. Ecol. 24, 311–324. doi: 10.1093/beheco/ars145
Lee, V. E., Régli, N., McIvor, G. E., and Thornton, A. (2019). Social learning about dangerous people by wild jackdaws. R. Soc. Open Sci. 6:191031. doi: 10.1098/rsos.191031
Levey, D. J., Londoño, G. A., Ungvari-Martin, J., Hiersoux, M. R., Jankowski, J. E., Poulsen, J. R., et al. (2009). Urban mockingbirds quickly learn to identify individual humans. Proc. Natl. Acad. Sci. U.S.A. 106, 8959–8962. doi: 10.1073/pnas.0811422106
Mack, R. N., Simberloff, D., Mark Lonsdale, W., Evans, H., Clout, M., and Bazzaz, F. A. (2000). Biotic invasions: causes, epidemiology, global consequences, and control. Ecol. Appl. 10, 689–710. doi: 10.1890/1051-0761(2000)010
Madden, J. R., and Whiteside, M. A. (2014). Selection on behavioural traits during “unselective” harvest. Anim. Behav. 87, 129–136. doi: 10.1016/j.anbehav.2013.10.021
Mariette, M. M., and Buchanan, K. L. (2016). Prenatal acoustic communication programs offspring for high posthatching temperatures in a songbird. Science 353, 812–814. doi: 10.1126/science.aaf7049
Marzluff, J. M., Walls, J., Cornell, H. N., Withey, J. C., and Craig, D. P. (2010). Lasting recognition of threatening people by wild American crows. Anim. Behav. 79, 699–707. doi: 10.1016/j.anbehav.2009.12.022
Mineka, S., and Cook, M. (1988). “Social learning and the acquisition of snake fear in monkeys,” in Social Learning: Psychological and Biological Perspectives. Hillsdale, eds T. R. Zentall and B. G. J. Galef (New Jersey: Lawrence Erlbaum Associates), 51–73.
Morinay, J., Daniel, G., Gustafsson, L., and Doligez, B. (2019). No evidence for behavioural syndrome and genetic basis for three personality traits in a wild bird population. Anim. Behav. 153, 69–82. doi: 10.1016/j.anbehav.2019.05.001
Nakahashi, W., and Ohtsuki, H. (2018). Evolution of emotional contagion in group-living animals. J. Theor. Biol. 440, 12–20. doi: 10.1016/j.jtbi.2017.12.015
O’Brien, J., and Sutherland, R. J. (2007). Evidence for episodic memory in a pavlovian conditioning procedure in rats. Hippocampus 17, 1149–1152. doi: 10.1002/hipo.20346
Palkovacs, E. P., Moritsch, M. M., Contolini, G. M., and Pelletier, F. (2018). Ecology of harvest-driven trait changes and implications for ecosystem management. Front. Ecol. Environ. 16, 20–28. doi: 10.1002/fee.1743
Palumbi, S. R. (2001). Humans as the world’s greatest evolutionary force. Science 293, 1786–1790. doi: 10.1126/science.293.5536.1786
Pigeon, G., Festa-Bianchet, M., Coltman, D. W., and Pelletier, F. (2016). Intense selective hunting leads to artificial evolution in horn size. Evol. Appl. 9, 521–530. doi: 10.1111/eva.12358
Pimentel, D., Lach, L., Zuniga, R., and Morrison, D. (2000). Environmental and economic costs of nonindigenous species in the United States. Bioscience 50:53. doi: 10.1641/0006-3568(2000)050
Pimentel, D., Zuniga, R., and Morrison, D. (2005). Update on the environmental and economic costs associated with alien-invasive species in the United States. Ecol. Econ. 52, 273–288. doi: 10.1016/j.ecolecon.2004.10.002
Preston, S. D., and de Waal, F. B. M. (2002). Empathy: its ultimate and proximate bases. Behav. Brain Sci. 25, 1–20. doi: 10.1017/S0140525X02000018
Shettleworth, S. J. (2010). Cognition, Evolution, and Behavior, 2nd Edn. New York, NY: Oxford University Press.
Shier, D. M., and Owings, D. H. (2007). Effects of social learning on predator training and postrelease survival in juvenile black-tailed prairie dogs, Cynomys ludovicianus. Anim. Behav. 73, 567–577. doi: 10.3233/978-1-61499-672-9-1642
Špinka, M. (2012). Social dimension of emotions and its implication for animal welfare. Appl. Anim. Behav. Sci. 138, 170–181. doi: 10.1016/j.applanim.2012.02.005
Storm, J. J., and Lima, S. L. (2010). Mothers forewarn offspring about predators: a transgenerational maternal effect on behavior. Am. Nat. 175, 382–390. doi: 10.1086/650443
Sullivan, A. P., Bird, D. W., and Perry, G. H. (2017). Human behaviour as a long-term ecological driver of non-human evolution. Nat. Ecol. Evol. 1:65. doi: 10.1038/s41559-016-0065
Thaker, M., Vanak, A. T., Lima, S. L., and Hews, D. K. (2010). Stress and aversive learning in a wild vertebrate: the role of corticosterone in mediating escape from a novel stressor. Am. Nat. 175, 50–60. doi: 10.1086/648558
Thomson, R. L., Forsman, J. T., Sardà-Palomera, F., and Mönkkönen, M. (2006). Fear factor: prey habitat selection and its consequences in a predation risk landscape. Ecography 29, 507–514. doi: 10.1111/j.0906-7590.2006.04568.x
Tilgar, V., Moks, K., and Saag, P. (2011). Predator-induced stress changes parental feeding behavior in pied flycatchers. Behav. Ecol. 22, 23–28. doi: 10.1093/beheco/arq164
Uusi-Heikkilä, S., Wolter, C., Klefoth, T., and Arlinghaus, R. (2008). A behavioral perspective on fishing-induced evolution. Trends Ecol. Evol. 23, 419–421. doi: 10.1016/j.tree.2008.04.006
van Heezik, Y., Seddon, P. J., and Maloney, R. F. (1999). Helping reintroduced houbara bustards avoid predation: effective anti-predator training and the predictive value of pre-release behaviour. Anim. Conserv. 2, 155–163. doi: 10.1111/j.1469-1795.1999.tb00061.x
Wallach, A. D., Johnson, C. N., Ritchie, E. G., and O’Neill, A. J. (2010). Predator control promotes invasive dominated ecological states. Ecol. Lett. 13, 1008–1018. doi: 10.1111/j.1461-0248.2010.01492.x
Wong, B. B. M., and Candolin, U. (2015). Behavioral responses to changing environments. Behav. Ecol. 26, 665–673. doi: 10.1093/beheco/aru183
Zanette, L. Y., Hobbs, E. C., Witterick, L. E., MacDougall-Shackleton, S. A., and Clinchy, M. (2019). Predator-induced fear causes PTSD-like changes in the brains and behaviour of wild animals. Sci. Rep. 9:11474. doi: 10.1038/s41598-019-47684-6
Zavaleta, E. S., Hobbs, R. J., and Mooney, H. A. (2001). Viewing invasive species removal in a whole-ecosystem context. Trends Ecol. Evol. 16, 454–459. doi: 10.1016/S0169-5347(01)02194-2
Keywords: species management, learning, stress, evolution, invasive alien species, nuisance species, wildlife harvesting
Citation: Diquelou MC and Griffin AS (2020) Behavioral Responses of Invasive and Nuisance Vertebrates to Harvesting: A Mechanistic Framework. Front. Ecol. Evol. 8:177. doi: 10.3389/fevo.2020.00177
Received: 13 December 2019; Accepted: 19 May 2020;
Published: 25 June 2020.
Edited by:
Shannon J. McCauley, University of Toronto Mississauga, CanadaReviewed by:
Marco Festa-Bianchet, Université de Sherbrooke, CanadaLucas Wauters, University of Insubria, Italy
Copyright © 2020 Diquelou and Griffin. This is an open-access article distributed under the terms of the Creative Commons Attribution License (CC BY). The use, distribution or reproduction in other forums is permitted, provided the original author(s) and the copyright owner(s) are credited and that the original publication in this journal is cited, in accordance with accepted academic practice. No use, distribution or reproduction is permitted which does not comply with these terms.
*Correspondence: Marie C. Diquelou, bS5jLmRpcXVlbG91QGdtYWlsLmNvbQ==