- 1Instituto de Ciencias Biológicas, Universidad de Talca, Talca, Chile
- 2Departamento de Genética Molecular y Microbiología, Facultad de Ciencias Biológicas, Pontificia Universidad Católica de Chile, Santiago, Chile
- 3IFEVA, Ecología, CONICET, Facultad de Agronomía, Universidad de Buenos Aires, Buenos Aires, Argentina
- 4Centro de Biotecnología y Genómica de Plantas, Instituto Nacional de Investigación y Tecnología Agraria y Alimentaria (INIA), Universidad Politécnica de Madrid (UPM), Pozuelo de Alarcón, Spain
- 5Centro de Estudios Avanzados en Zonas Áridas (CEAZA), Universidad Católica del Norte, Coquimbo, Chile
- 6Centro de Investigación de Estudios Avanzados del Maule, Universidad Católica del Maule, Talca, Chile
- 7Núcleo Científico Multidisciplinario-DI, Universidad de Talca, Talca, Chile
Antarctic plants have developed mechanisms to deal with one or more adverse factors which allow them to successfully survive such extreme environment. Certain effective mechanisms to face adverse stress factors can arise from the establishment of functional symbiosis with endophytic fungi. In this work, we explored the role of fungal endophytes on host plant performance under high level of UV-B radiation, a harmful factor known to damage structure and function of cell components. In order to unveil the underlying mechanisms, we characterized the expression of genes associated to UV-B photoreception, accumulation of key flavonoids, and physiological responses of Colobanthus quitensis plants with (E+) and without (E−) fungal endophytes, under contrasting levels of UV-B radiation. The deduced proteins of CqUVR8, CqHY5, and CqFLS share the characteristic domains and display high degrees of similarity with other corresponding proteins in plants. Endophyte symbiotic plants showed lower lipid peroxidation and higher photosynthesis efficiency under high UV-B radiation. In comparison with E−, E+ plants showed lower CqUVR8, CqHY5, and CqFLS transcript levels. The content of quercetin, a ROS-scavenger flavonoid, in leaves of E- plants exposed to high UV-B was almost 8-fold higher than that in E+ plants 48 h after treatment. Our results suggest that endophyte fungi minimize cell damage and boost physiological performance in the Antarctic plants increasing the tolerance to UV-B radiation. Fungal endophytes appear as fundamental biological partners for plants to cope with the highly damaging UV-B radiation of Antarctica.
Introduction
Although ultraviolet B radiation (UV-B) is a minor component of the solar radiation, it has a disproportionately harmful effect on plants (Jansen et al., 1998). At global level, UV-B radiation (280 to 315 nm) is absorbed by the ozone layer, permitting that a small proportion reaches surface of the Earth. However, the levels of UV-B radiation that reach the earth’s surface depends on several factors such as latitude, altitude, season, time of day as well as cloudiness (Seckmeyer et al., 2008). The UV-B radiation affects several aspects of plants such as development, growth, morphology (Beissert and Loser, 2008; Kaling et al., 2015), as well as modulates physiological processes and metabolism (McLeod et al., 2001). At the molecular level, the absorption of UV-B radiation by the leaves is associated with important damage on molecules such as DNA, RNA and proteins with ultimately, have several consequences at physiological level. Thus, plant exposure to high levels of UV-B radiation causes a decrease in the photosynthetic efficiency of photosystem (PS) II (Fv/Fm) due to the degradation of the PSII protein D1 (Caldwell et al., 2007; Shourie et al., 2014). The final consequences at individual level, is a reduction in biomass and reproduction output, while even generating mutations in germinal lines with clear effects on the following generations (Kaling et al., 2015).
The negative effects of UV-B radiation can be prevented in part by accessory pigments such as carotenoids, xanthophylls, and flavonoids, which act in concert as protective screen and regulating the oxidative stress (reactive oxygen species; ROS) (Sequeida et al., 2012). Flavonoids are the most ubiquitous group of natural polyphenols known for their photoprotective and antioxidant role (Henry-Kirk et al., 2018). In particular, the flavonol quercetin has been described as a remarkable ROS scavenger due to its structural properties; thus, it strongly controls oxidative damage (Shourie et al., 2014). Studies in Arabidopsis thaliana have shown that the protein encoded by UV RESISTANT LOCUS 8 (UVR8) controls the expression of numerous genes involved in acclimatization and protection against UV-B radiation (Hayes et al., 2017). The genes regulated by UVR8 include precursors of flavonoid biosynthesis, morphogenic regulation of leaves and defense against herbivory (Morales et al., 2015). Recently, great advances have been made in identifying components involved in this specific UV-B signaling pathway (Yang et al., 2018). These components, in addition to UVR8, include constitutive photomorphogenesis 1 (COP1) and the transcription factors bZIP and elongated hypocotyl 5 (HY5). UVR8 accumulates in the nucleus in response to UV-B radiation, where it recognizes cis-acting responsive elements present in the promoter region of the gene HY5, commanding their expression (Moriconi et al., 2018). COP1 is required for activation of the HY5 gene stimulated by UV-B (Loyola et al., 2016). In UVR8- or COP1-defective A. thaliana mutants, an increase in sensitivity to UV-B radiation has been described (Ballaré et al., 2011). UVR8 regulates, through a HY5-dependent mechanism, most of the flavonoid biosynthetic genes, namely CHS, CHI, and FLS1 (Müller-Xing et al., 2014), and specifically of flavonols (Ayabe et al., 2010; Martens et al., 2010). These compounds absorb UV-B light in the 280–320 nm range, and their concentration increases in plants exposed to abiotic environmental stress, including the UV-B. Thus, it has been suggested that these flavonols act as protection filters for UV-B radiation in plants (Lois, 1994).
Antarctica is considered one of the most inhospitable environments on earth (Korckzak-Abshire et al., 2011). Despite this, there are two species of vascular plants that have been able to successfully colonize this continent, Deschampsia antarctica and Colobanthus quitensis (Parnikoza et al., 2011). The Antarctic plant C. quitensis is a small dicotyledonous perennial herb that lives from southern Mexico to the Antarctic Maritime (68°42′S) (Moore, 1970; Convey, 1996). Due to its wide latitudinal range of distribution and low connectivity between populations, genetically differentiated and ecologically adapted populations can be distinguished (Acuña-Rodríguez et al., 2017). To cope with the hostile Antarctic environment, these plant species have developed different physiological and biochemical mechanisms among which the accumulation of flavonoids and carotenoids play a role in photoprotection against UV radiation (Pereira et al., 2009). In addition, several works showed that the association of plants with endophytic microorganisms (fungi and bacteria) isolated from Antarctica allow other host plants tolerating harmful environmental conditions (Molina-Montenegro et al., 2016; Gallardo-Cerda et al., 2018; Ramos et al., 2018; Acuña-Rodríguez et al., 2019). Although an increasing number of publications are highlighting the role of symbiotic microorganisms boosting plant growth and defense (Hamilton et al., 2012; Pieterse et al., 2014), seldom studies have clearly linked plant performance under a given stress factor, with the symbiont-mediated changes in gene expression modulating such physiological response (see e.g., Hereme et al., 2020). Occurring plants of C. quitenis in Antarctica have been found to host diverse group of fungal endophytes (Rosa et al., 2010). In a previous work with the same species, we recently observed that the endophytic fungi increase total biomass and number of flowers under high UV-B radiation, while modulating the hormonal systems in a complex way (Ramos et al., 2018). Although the expected UV-B mediated activation of defense signaling pathways by the UV-B radiation (Ballaré et al., 2011) was evident in endophyte-free plants, there was not a clear response in endophyte-symbiotic plants. The effect of the UV-B in reducing the levels of the growth hormones (indole-3-acetic acid and abscisic acid) was more pronounced in symbiotic plants than in endophyte-free plants which, counterintuitively, contrasts the observed pattern in biomass and flowers (Ramos et al., 2018).
In order to some shed light on this puzzle, here we present results from an experiment aimed at evaluating the effect of UV-B radiation on the performance of C. quitensis plants with and without fungal endophytes. We sought to link the endophyte-mediated effects on the host plant molecular responses to UV-B radiation with that at biochemical and physiology levels. In particular, we assessed the expression of genes involved in the UV-B signal transduction pathway and in the biosynthesis of photoprotective compounds as flavonols. Finally, the flavonol abundance was quantified in plant leaves of C. quitensis with and without the presence of Antarctic native endophytic fungi.
Materials and Methods
Study Site, Generation of Plant Material and Growth Conditions
Colobanthus quitensis plants were collected at the Polish Antarctic Station “Henryk Arctowski,” South Shetland Islands (62°09′S), during the 2016–2017 growing season. We collected 30 plants with ≈150 g of rhizospheric soil (to be used later to prepare the sterile soil mix), selecting individuals that were separated by at least one meter from each other. The plants were transported in plastic containers to the Plant Ecology Laboratory of the University of Talca, Talca, Chile (35°24′S). In the laboratory, the plants were replicated by vegetative propagation to increase the biological material, based on Zuñiga et al. (2009). Briefly, nodal segments from a plant cushion were transplanted 100 mL pots filled with sterile soil mix of sand:soil:peat (4:4:1). The new leaves were developed around 3 months after propagation, prior to UV-B radiation treatment. With reference to Zuñiga et al. (2009), the 6-month-old plants (2–4 cm diameter) were maintained for 30 days in a growth chamber at 4°C, with a photoperiod of 21/3 light/dark (350 μmol photons m–2s–1), until the experiment. The plants were irrigated every 3 days with 10 mL of tap water and fertilized with the nutrient solution Phostrogen (0.2 mg L–1).
Because we wanted to evaluate the role of just fungal endophytes on the plants, we subjected the biological material to an antibiotic treatment. All the plants were first treated with the broad-spectrum systemic antibiotic rifampicin (50 μg mL–1) to eliminate bacteria [for details, see Torres-Díaz et al., 2016; Gallardo-Cerda et al., 2018). To evaluate the effectiveness, at the end of the antibiotic treatment, a random sample of tissues from ten plants was taken and cultured on LB agar for bacterial growth. Ten Plates were incubated at 28°C for 3 days. No bacterial colonies were observed growing on the culture media.
In order to obtain plants free of endophytic fungi, half of the clones from each plant were treated with fungicide to obtain plants free of endophytic fungi. Endophytic fungi-free plants (E-) were obtained by applying 2 g L–1 of the commercial fungicide Benlate every 3 days [containing Benomyl [methyl 1-[(1-butylamino) carbonyl]-1H-benzimidazol-2-yl] carbamate (DuPont, Wilmington, DE, United States)]. This fungicide treatment has been previously tested on plants of Colobanthus quitensis and Lolium perenne (Dernoeden and McIntosh, 1991; Torres-Díaz et al., 2016; Hereme et al., 2020) showing very high effectiveness in removing fungi from the whole plant and no apparent phytotoxic effects has been observed. Water was used as control of the fungicide treatment in E+ plants. Two weeks after the fungicide treatment, five E+ and five E− plants were inspected under microscope to verify the presence and/or absence of endophytes; root and shoot tissues were stained with Pelikan blue writing ink (Supplementary Figure S1; Hosseini et al., 2017; Oses Pedraza et al., 2018). Only after passing the set criteria (Gallardo-Cerda et al., 2018), fungicide-treated and untreated plants from each group were considered as endophytic (E+) and non-endophytic (E−) clones, respectively. Previously, the five most abundant fungal endophytes isolated from samples obtained from Colobanthus quitensis were identified as Penicillium chrysogenum (62%), Penicillium brevicompactum (27%), Alternaria sp. (6%), Phaeosphaeria sp. (3%), and Eupenicillium osmophilum (2%) (Hereme et al., 2020).
Fungal Endophyte Effect on the Physiological and Biochemical Performance of C. quitensis Under UV-B Radiation
All the E+ and E− C. quitensis plants were acclimated for 2 days at 4°C prior to UV-B radiation treatment based on Ramos et al. (2018). Briefly, treatment of 46 μmol photons m–2 s–1 of UV-B radiation (10 μW cm–2), provided by fluorescent tubes (PL-L 36W/01/4P UV-B, Hammond, IN, United States), which are narrowband UV-B tubes with a narrow peak of UV-B at approximately 313 nm was applied (Sager and McFarlane, 1997). Control plants without UV-B radiation treatment, were generated by the presence of normal fluorescent tubes used in the culture chambers with Mylar film (Du-Pont, Wilmington, DE, United States) to absorb the radiation of wavelengths less than 320 nm. The intensity of UV-B radiation was measured with a UV light meter (Sentry ST-513, Taiwan, China). UV-B intensity treatment was considered based on natural conditions from the average UV-B radiation reported in Antarctica (Day et al., 2001; Navarrete-Gallegos et al., 2012). A total of 160 C. quitensis plants were randomly assigned to any of the two groups of treatments: I and II) 80 plants with and without endophytes (40 plants E+ and 40 plants E− both without UV-B radiation, respectively) were used as control of UV-B radiation treatment, while III and IV) 80 plants with and without endophytes (40 plants E+ and 40 plants E− 46 μmol photons m–2 s–1, respectively) were used for the UV-B radiation treatment. All treatments were performed with a 21/3 light/dark photoperiod. The evaluation of the different parameters on the plants were carried out at the beginning (0), 6, 24, and 48 h since the radiation treatments were imposed. In each time and UV-B intensity, ten plants were removed from each treatment and shoot material was collected, frozen in liquid nitrogen and maintained at −80°C until further analysis.
To assess whether the fungal endophytes increased the tolerance of C. quitensis to UV-B radiation, biochemical and physiological parameters were analyzed. All the analytical determinations were performed on shoot tissues from each plant. The oxidative degradation of lipid molecules that leads to high levels of malondialdehyde (MDA) is considered a useful index of general lipid peroxidation (Møller et al., 2007). The level of MDA was determined by the thiobarbituric acid (TBA) reaction method (Heath and Packer, 1968). For this purpose, three biological replicates (single plant at each) were assessed from each condition (time of exposure and presence/absence of endophyte). One milliliter of 20% trichloroacetic acid reagent (TCA) was added to the tissue previously pulverized in liquid nitrogen (0.1 g) and mixed by agitation for 10 mins. The homogenate was centrifuged at 10,000 × g for 10 min at room temperature and the supernatant was used for the MDA determination. One milliliter of TCA (20%) and TBA (0.5%) (w/v) was added to 250 μL of the supernatant, which was then incubated at 100°C for 30 min in a dry bath (Thermolyne 16500, Marshall Scientific) before being chilled on ice. The complex formed by MDA and TBA produces a colorimetric reaction, and absorbance was determined in a spectrophotometer (Unicam model UV/Vis Spectrometer) at 532 and 600 nm. The level of MDA was calculated using an extinction coefficient of 155 mm–1 cm–1 using the standard equation for weight in grams for each sample: MDA = [((A532 − A600) × 1/155)/g] × 1,000,000.
During the exposure of the plants to UV-B radiation, the photochemical efficiency of PSII was estimated in accordance with Maxwell and Johnson (2000). For this purpose ten plants were assessed for each condition (time of exposure and presence/absence of endophyte) using a pulse-modulated amplitude fluorimeter (FMS 2, Hansatech, Instrument Ltd., Norfolk, United Kingdom). Plant adaptation was carried out for 30 min prior to UV-B treatment using a black box (30 × 20 × 15 cm) to ensure maximum photochemical efficiency based on Torres-Díaz et al. (2016).
Sequence Analysis
Sequences of interest (UVR8, HY5, and FLS) were obtained from an RNAseq transcriptome from C. quitensis plants exposed to UV-B radiation (unpublished data). Full-length transcripts (see Table 1) were translated using the ExPASy Translate Tool1. The amino acid sequences were analyzed by a multi-alignment carried out using ClustalW and BioEdit Sequence Alignment Editor v7.0 software. Phylogenetic tree construction was performed by neighbor-joining (with 1,000 bootstrap replicates) using MEGA X software (Kumar et al., 2018). For each deduced protein, the isoelectric point and molecular mass were predicted using the Compute pI/Mw tool2.
Relative Expression Analysis of Genes Involved in Response to UV-B Radiation
Total RNA from shoot tissue was extracted with PureLink® Plant RNA Reagent (Invitrogen, United States). DNA traces were removed using the TURBO DNA-freeTM kit (Thermo Fisher Scientific, Waltham, MA, United States) according to the manufacturer’s instructions. Three independent RNA extractions (a single plants at each) were performed from each group of frozen samples. cDNA synthesis was performed using the First-Strand cDNA Synthesis Kit (Thermo Fisher Scientific, Waltham, MA, United States) following the manufacturer’s instructions.
The transcription levels were measured by quantitative PCR (qRT-PCR) using an Mx3000P system (Agilent Technologies, Santa Clara, CA, United States) based on Ramos et al. (2012). All reactions were performed using the Brilliant SYBR Green Master Mix (Agilent Technologies, Santa Clara, CA, United States) according to the procedure described by the manufacturer. For each biological replicate of cDNA (n = 3), qRT-PCR reactions were carried out in triplicates (technical replicates) using 10 μL of Master Mix, 10 μM of each primer, 1 μL of diluted cDNA and nuclease-free water to a final volume of 20 μl. Fluorescence was measured at the end of each amplification cycle. The amplification was followed by a fusion curve analysis with continuous acquisition of fluorescence data during melting at 65–95°C.
The raw data were analyzed manually, and the expression was normalized with the genes coding for α-tubulin and β-tubulin, which were obtained from GenBank3. The primers used for qRT-PCR analysis are shown in Table 1. For each gene, a standard curve was generated using a serial cDNA dilution, and the resulting PCR efficiency calculations were imported into the analysis of the relative expression data. The Excel macro (Microsoft) GENEX v1.10 (gene expression analysis for iCycler iQ® Real-time PCR detection system, v1.10, 2004; Bio-Rad Laboratories, United States) that is based on the algorithm of Vandesompele et al. (2002) was used to analyze the raw data obtained from the thermocycler.
Determination of Flavonol Contents
The extraction of flavonols was carried out based on a previous report (Ramos et al., 2016). Briefly, leaf samples were pulverized in liquid nitrogen using a mortar. Each biological replicate consisted of frozen shoot tissue (0.1 g) from one plant. All experiments were conducted using three biological replicates. Samples were treated with 1.5 mL of methanol/H2O (4: 1, v/v) and sonicated for 10 min. The supernatant obtained after centrifugation at 3,000 × g for 10 min was filtered through 0.22 m cellulose disks. The supernatant containing glycosylated flavonols was hydrolyzed with one volume of 2N HCl and subjected to 70°C for 40 min to obtain the aglycones. The aglycones of flavonols were obtained by treatment with an equal volume of ethyl acetate and centrifugation at 15,600 × g for 10 min, which separated the compounds of interest from the aqueous phase. The organic phase was evaporated in a CentriVap® (Labconco., Kansas City, United States) and obtained pellets were dissolved in 0.5 mL dimethylsulfoxide (DMSO). The analysis was performed in an Agilent 1100 series HPLC system provided with a photodiode array detector (DAD) based on Ramos et al. (2016). Compounds were separated on a reverse-phase C18 analytical column (Kromasil 100, 25 cm × 4.6 mm × 5 μm), and data were analyzed with a ChemStation software chromatography system (Hewlett-Packard). Quantifications were carried out between the wavelengths of 280 and 600 nm, monitoring flavonols at 345 nm.
Statistical Analysis
For the determination of significant differences in the levels of MDA, Fv/Fm, relative expression and flavonol content, two-way ANOVA was used with the factors exposure time (h) and presence of endophytic fungi. For all analyses, the assumptions of normality and homogeneity of variances were tested using the Shapiro-Wilks and Bartlett tests, respectively. Subsequently, Tukey’s HSD multiple comparisons analysis was performed. Differences were considered significant at P ≤ 0.05. The analyses were performed with GraphPad Prism 7 (GraphPad Software Inc., San Diego, CA, United States).
Results
UV-B Radiation Affects Photochemical Performance and Reduce Oxidative Damage of C. quitensis Depending on Endophyte Presence
The presence of fungal endophytes improved the tolerance to UV-B radiation by decreasing cell membrane lipid peroxidation. Under high UV-B radiation, the presence of fungal endophytes decreased MDA production (Figure 1). In addition, plants without endophytes (E-) showed significantly higher content of thiobarbituric acid reactivity and, thus, cell damage caused by exposure to UV-B radiation (Table 2). The accumulation of MDA significantly increased over the course of the experiment (0, 6, 24, and 48 h). This pattern was observed in both control plants and plants exposed to UV-B radiation with and without endophytes (Figures 1A,B). However, without symbionts, the values were significantly higher. When examining plants exposed to UV-B radiation, E+ plants showed significantly lower levels of MDA reactivity compared to E- plants (Table 2).
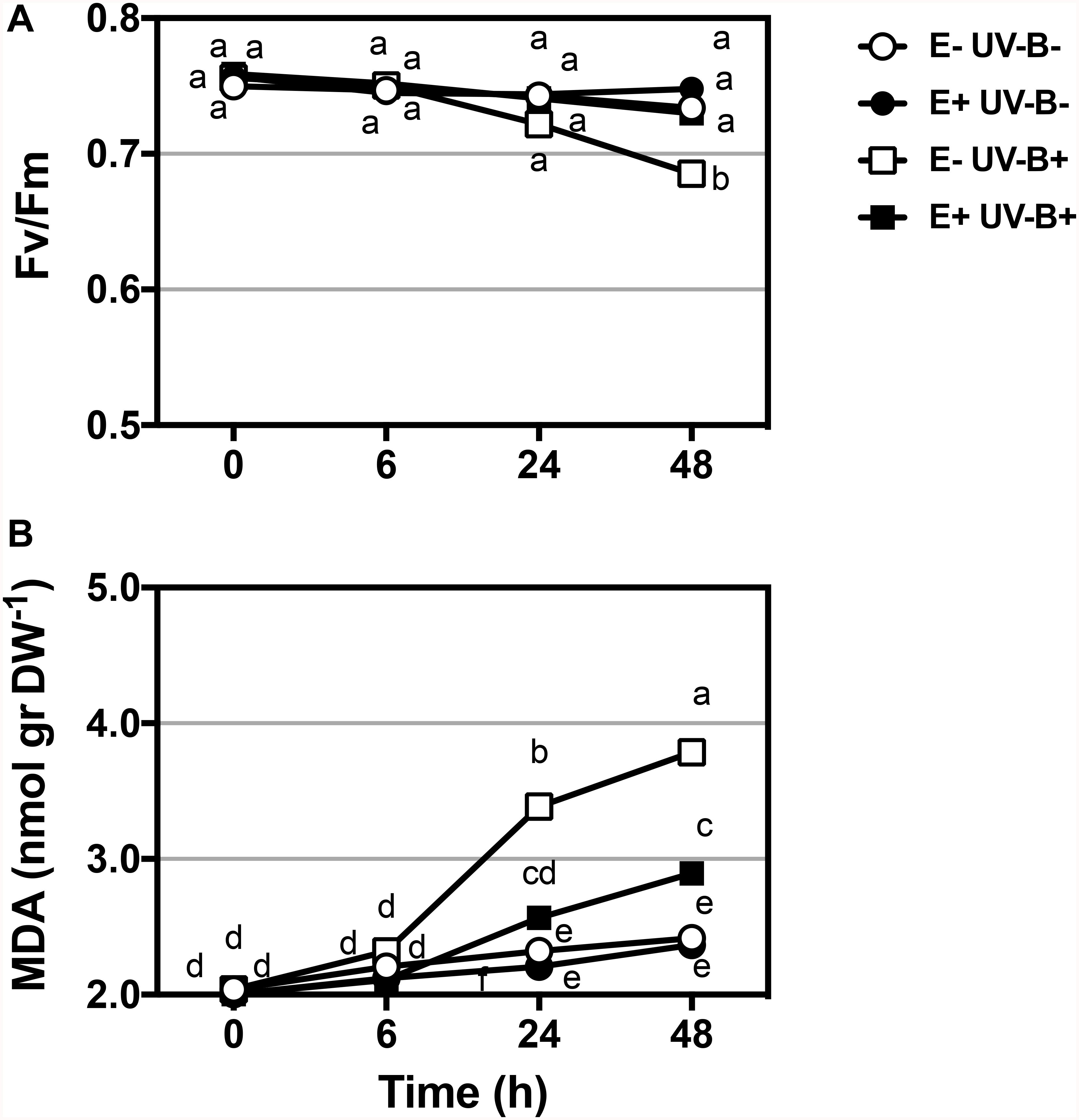
Figure 1. Physiological performance (lipid peroxidation and PSII photochemical efficiency - Fv/Fm) in Colobanthus quitensis exposed to UV-B radiation with and without endophytes. PSII photochemical efficiency (Fv/Fm) (A) and Malondialdehyde MDA (B) variation in the leaves of Colobanthus quitensis were evaluated under UV-B radiation. Control (without radiation UV-B radiation) and UV-B (46 μmol m–2 s–1) radiation in plants with and without endophytes (E+ and E-) were analyzed. Different letters indicate significant differences (P < 0.05; two-way ANOVA). Bars represent means ± SE from three independent experiments.
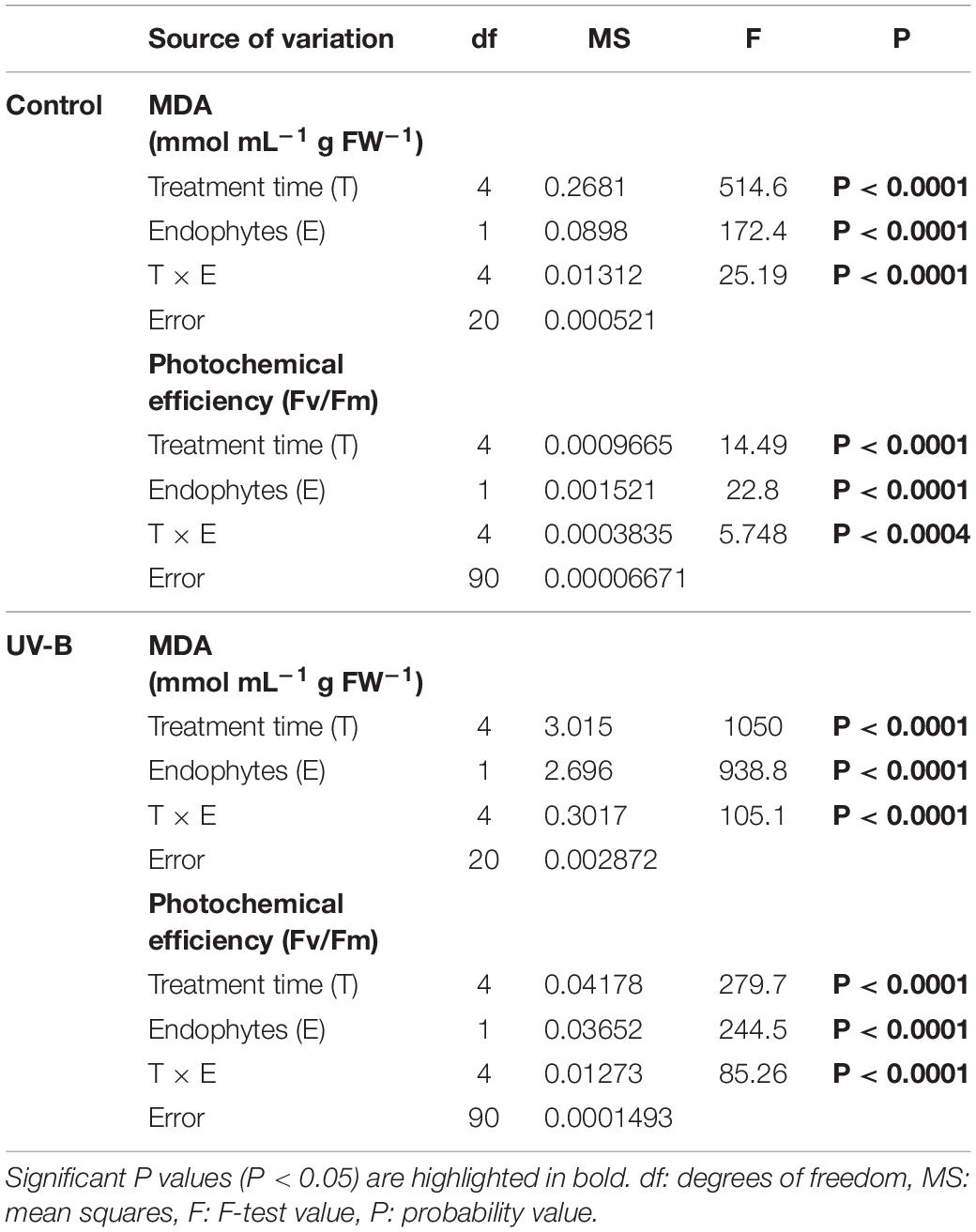
Table 2. Results of factorial two-ways ANOVA evaluating the interactive effects of endophytes presence (E) and different times of treatment for (control: without radiation UV-B radiation, UV-B: 46 μmol m–2 s–1) for photochemical performance, measured as maximum quantum yield (Fv/Fm) of photosystem II (PSII) and MDA (mmol mL g FW–1) of Colobanthus quitensis.
The physiological parameter photochemical efficiency of PSII under UV-B radiation was significantly affected by the UV-B treatment, with clear differences between E− and E+ plants (Table 2). In UV-B radiation control plants, the values of photochemical efficiencies were similar for both E− and E+ plants. However, E− plants exposed to UV-B radiation displayed a significant reduction in photochemical efficiency compared to both, E- plants under UV-B radiation control and to E+ plants in any of the UV-B situations (Table 2). This latter effect was mainly evident after 48 h of UV-B treatment.
Identity and Expression Analysis of Signal Transduction and Flavonol Biosynthesis Related Genes
Three sequences encoding UVR8, FLS, and HY5 proteins were identified in a transcriptomic analysis of C. quitensis exposed to high UV-B radiation and are now available in GeneBank (Table 1). These were highly represented in the RNAseq library (partially annotated) from UV-B-exposed plants and showed strong identity to the UVR8, FLS, and HY5 plant proteins. Multiple alignment analysis and phylogenetic classification of the full-length deduced protein sequences displayed high sequence homology to other UVR8, FLS, and HY5 proteins (Figure 2). The deduced UVR8 protein shares 81.3% identity with the reported protein in Spinacea oleracea and 79.3% identity with a protein from Chenopodium quinoa (Supplementary Figure S2). The deduced FLS protein shares 66% identity with the sequence reported in Nicotiana tabacum and 65% identity with the one from Glycine max. The multiple sequence alignment confirms the presence of the conserved 2-ODD motifs 1 and 2 and also the conserved iron-binding sites (Supplementary Figure S3). The deduced HY5 protein shares 79% identity to S. oleracea and 72% to the reported A. thaliana sequences. Multiple alignment analysis shows that there are conserved domains to the DNA-binding and dimerization domains of the bZIP-class proteins (Supplementary Figure S4).
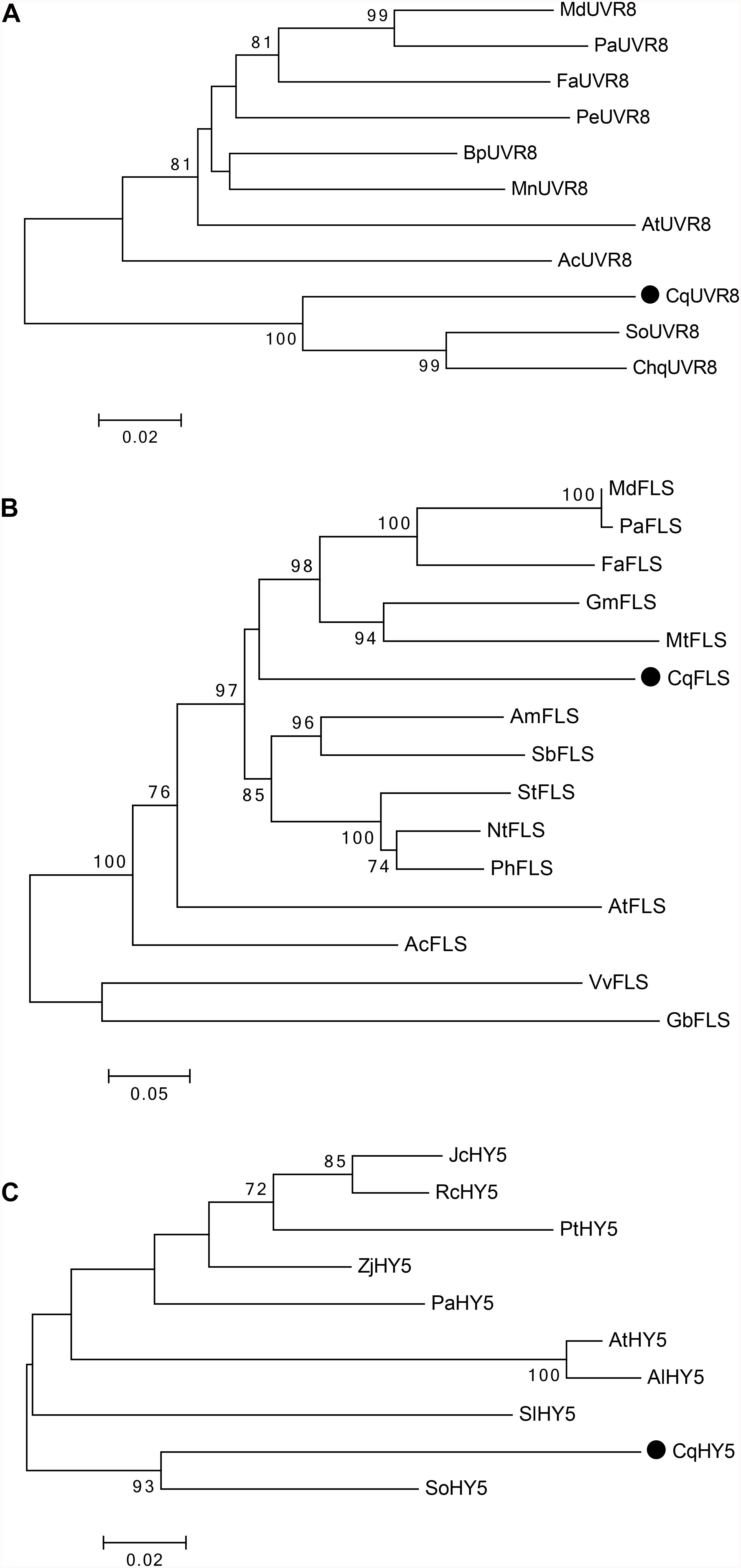
Figure 2. Phylogenetic relationships of the deduced UVR8, FLS, and HY5 proteins from C. quitensis with several plant UVR8, FLS and HY5 proteins. (A) Phylogenetic analysis was performed using the following plant UVR8 proteins: Ananas comosus (XP_020107421), Arabidopsis thaliana (AAD43920), Betula platyphylla (AHY02156), Chenopodium quinoa (XP_021734620), Fragaria x ananassa (AOD75227), Malus domestica (NP_001315969), Morus notabilis (XP_024019180), Populus euphratica (AKJ54489), Spinacea oleracea (XP_021836012), and Prunus avium (XP_021829535). The deduced UVR8 protein of Colobanthus is indicated with a black dot. (B) For FLS, the following proteins were used for the phylogenetic analysis: Glycine max (NP_001237419), Allium cepa (AAT68476), Vitis vinifera (BAE75810), Ginkgo biloba (ACY00393), Arabidopsis thaliana (AAB41504), Malus domestica (AAD26261), Fragaria x ananassa (AAZ78661), Nicotiana tabacum (ABE28017), Antirrhinum majus (ABB53382), Petunia x hybrida (CAA80264), Solanum tuberosum (CAA63092), Prunus avium (AFO67943), Medicago truncatula (AES71332), and Scutellaria baicalensis (AHA14501). The deduced FLS protein of Colobanthus is indicated with a black dot. (C) For HY5, phylogenetic analysis was performed with the following plant HY5 proteins: Arabidopsis thaliana (BAA21116), Arabidopsis lyrata (XP_002873502), Jatropha curcas (XP_012076602), Populus trichocarpa (XP_002308656), Ziziphus jujuba (XP_015885857), Ricinus communis (XP_002515537), Spinacea oleracea (XP_021837612), Solanum lycopersicum (NP_001234820), and Prunus avium (XP_021827650). The deduced HY5 protein of Colobanthus is indicated with a black dot.
CqUVR8 displays an open reading frame (ORF) of 1,308 bp that encodes a protein of 435 amino acids with a molecular weight (Mw) 46.43 kDa and an isoelectric point (pI) 5.50. In the case of CqFLS, an ORF of 1,019 bp encoding a protein of 339 amino acids with a calculated Mw of 38.99 kDa and a pI of 6.68 was observed. CqHY5 has an ORF of 515 bp, encoding a protein of 171 amino acids with a Mw of 18.54 kDa and a pI of 9.74.
Expression of UVR8 and HY5 in plants exposed to UV-B radiation was significantly affected by the presence of fungal endophytes, exposure time and the interaction between both factors (Table 3). The qRT-PCR analysis of UVR8 showed an increase in expression levels at 24 h compared to time 0, but no significant differences between E− and E+ plants were observed under UV-B radiation control conditions (Figure 3A). In plants exposed to UV-B radiation, the expression levels of UVR8 showed a significant upregulation at 6 h compared to that of the control, with the highest expression at 24 h in E−, which was 2.5-fold higher than the expression observed in E+ plants. As can be observed in Figure 3 in E+ plants after 24 h compared to the E− plants UVR8 levels remain low, suggesting that fungal infection protects the plants avoiding the upregulation of this genes (Figure 3B).
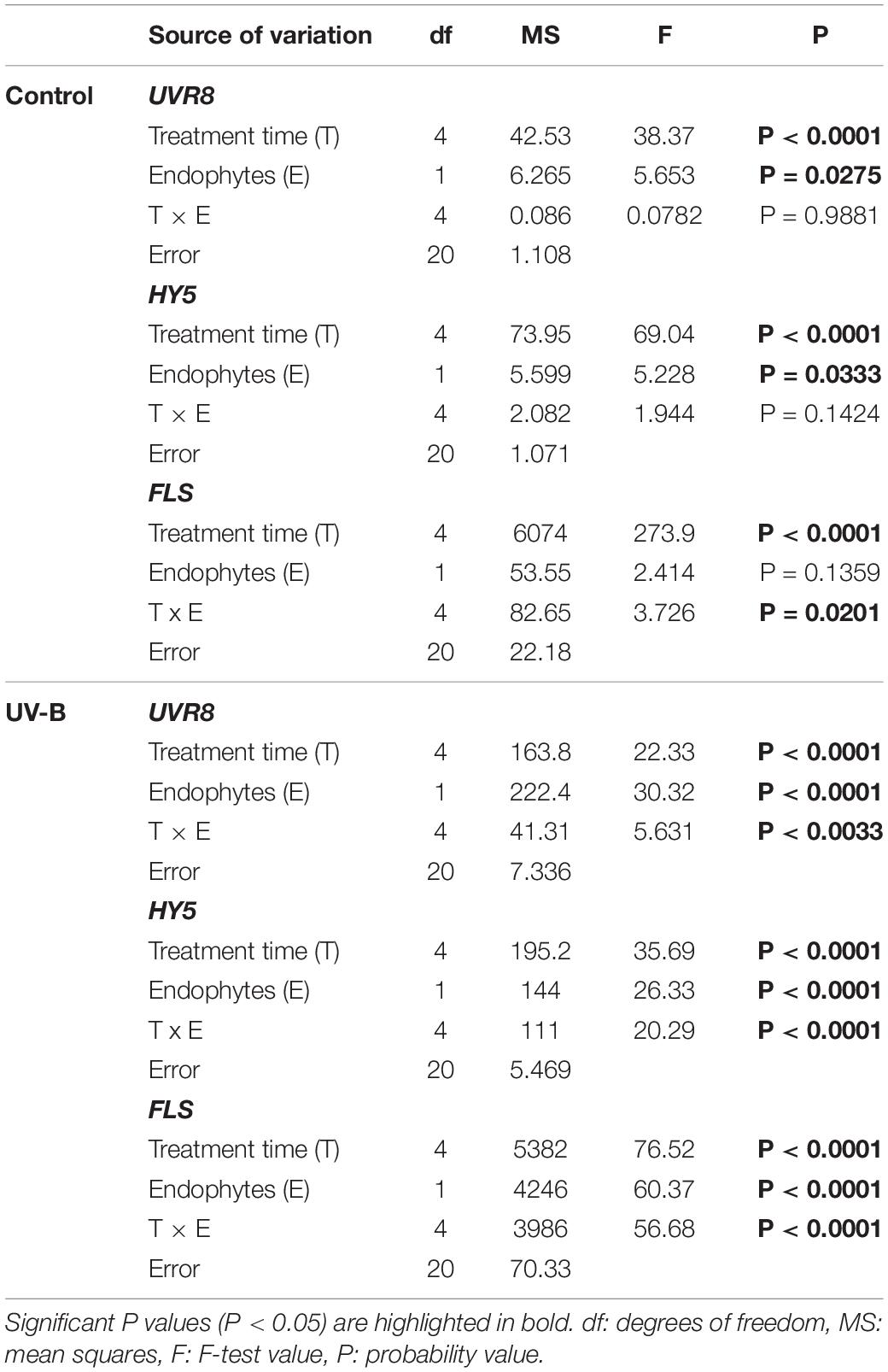
Table 3. Results of factorial ANOVAs evaluating the interactive effects of endophytes presence (E) and different treatment times for (control: without radiation UV-B radiation, UV-B: 46 μmol m–2 s–1) for relative level of expression of genes responding to UV-B by qPCR in the leaf of Colobanthus quitensis.
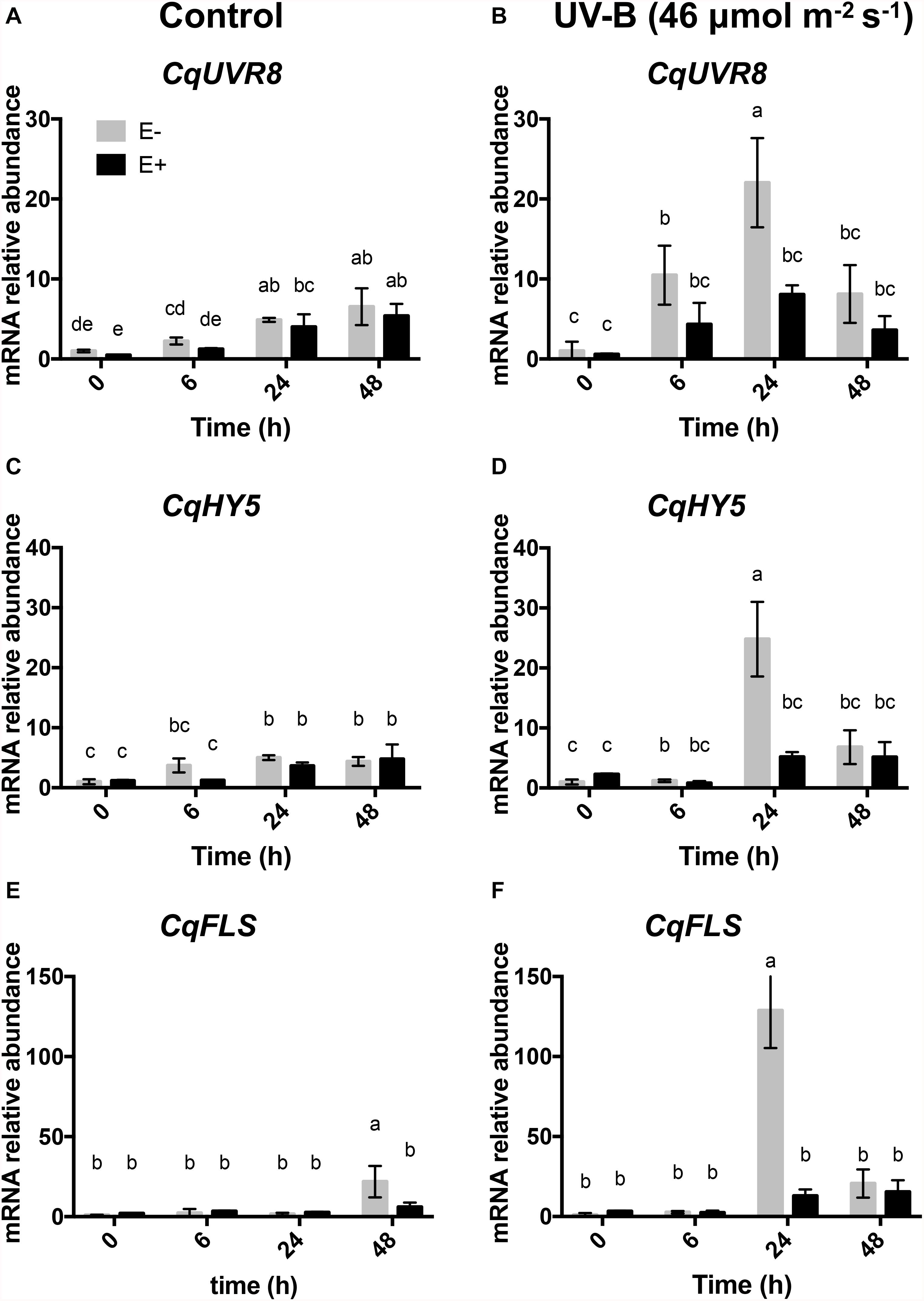
Figure 3. Transcript profile of genes in response to UV-B treatment in the leaves of C. quitensis. Transcript levels of UVR8, HY5, and FLS were evaluated in plants exposed to control [without radiation UV-B radiation (A,C,E)] and with UV-B [46 (μmol m–2 s–1 (B,D,F)] radiation and with and without endophytes (E+ and E–). For relative transcript levels, normalization was performed against housekeeping genes (α-tubulin and β-tubulin) in all samples. Different letters indicate significant differences (P < 0.05; two-way ANOVA). Bars represent the means ± SE of three independent experiments.
Analysis of the relative expression levels of HY5 in control plants showed a significant increase after 24 h compared to 0 h, in both E− and E+ plants, indicating that the fungus did not induce changes in its expression (Figure 3C). Under UV-B radiation treatment, HY5 expression significantly increased at 24 h in E− compared to E+ plants, to then decrease. In addition, no differences were observed in E+ plants during the time-course experiment (Figure 3D).
Finally, expression levels of FLS were analyzed, learning that in the control treatment they were significantly higher at 48 h in E− compared to E+ (Figure 3E). In plants exposed to UV-B radiation, a significant upregulation was observed at 24 h compared to the other times of UV exposure in E− compared to E+ plants, but no differences were observed between different times treatment in E+ plants (Figure 3F).
Endophytes Affects Flavonols Accumulation in Response to UV-B Radiation
To evaluate changes in flavonol contents, plants exposed to UV-B radiation with and without endophytes were analyzed. In the control and treatment groups, the accumulation of kaempferol remained stable throughout the time course experiment in both E− and E+ plants (Figures 4A,B).
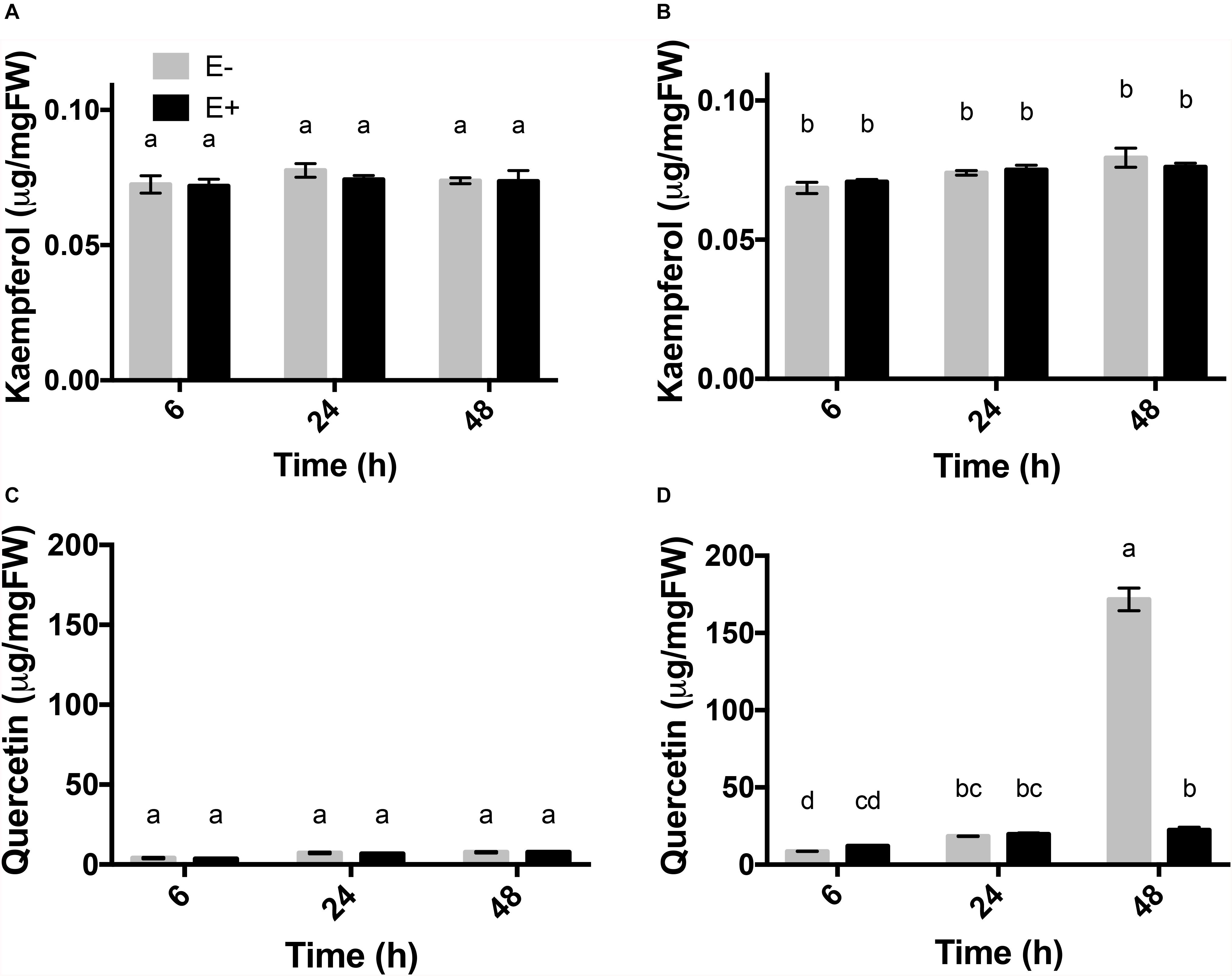
Figure 4. Flavonols accumulation profile in response to UV-B radiation in leaves of C. quitensis. Flavonols content (μg/mg fresh weight) of kaempferol (A,B) and quercetin (C,D) in the leaves of C. quitensis obtained at different times of UV-B exposure were analyzed. Plants exposed to control [without radiation UV-B radiation (A,C)] and UV-B [46 μmol m–2 s–1 (B,D)] radiation and with and without endophytes (E+ and E-) were evaluated. Different letters indicate significant differences (P < 0.05; two-way ANOVA). Bars represent the means ± SE of three independent experiments.
Regarding quercetin levels, in the control group, the accumulation remained stable (Figure 4C). The plants exposed to UV-B showed a significant increase in the levels of quercetin, specifically at 48 h, which displayed an 8-fold higher content in E- plants (171.72 μg mg FW–1) compared E + (22.37 μg mg FW–1) (Figure 4D). Accumulation of quercetin in plants exposed to UV-B radiation was significantly affected by the presence of fungal endophytes, exposure time and the interaction between both factors (Table 4).
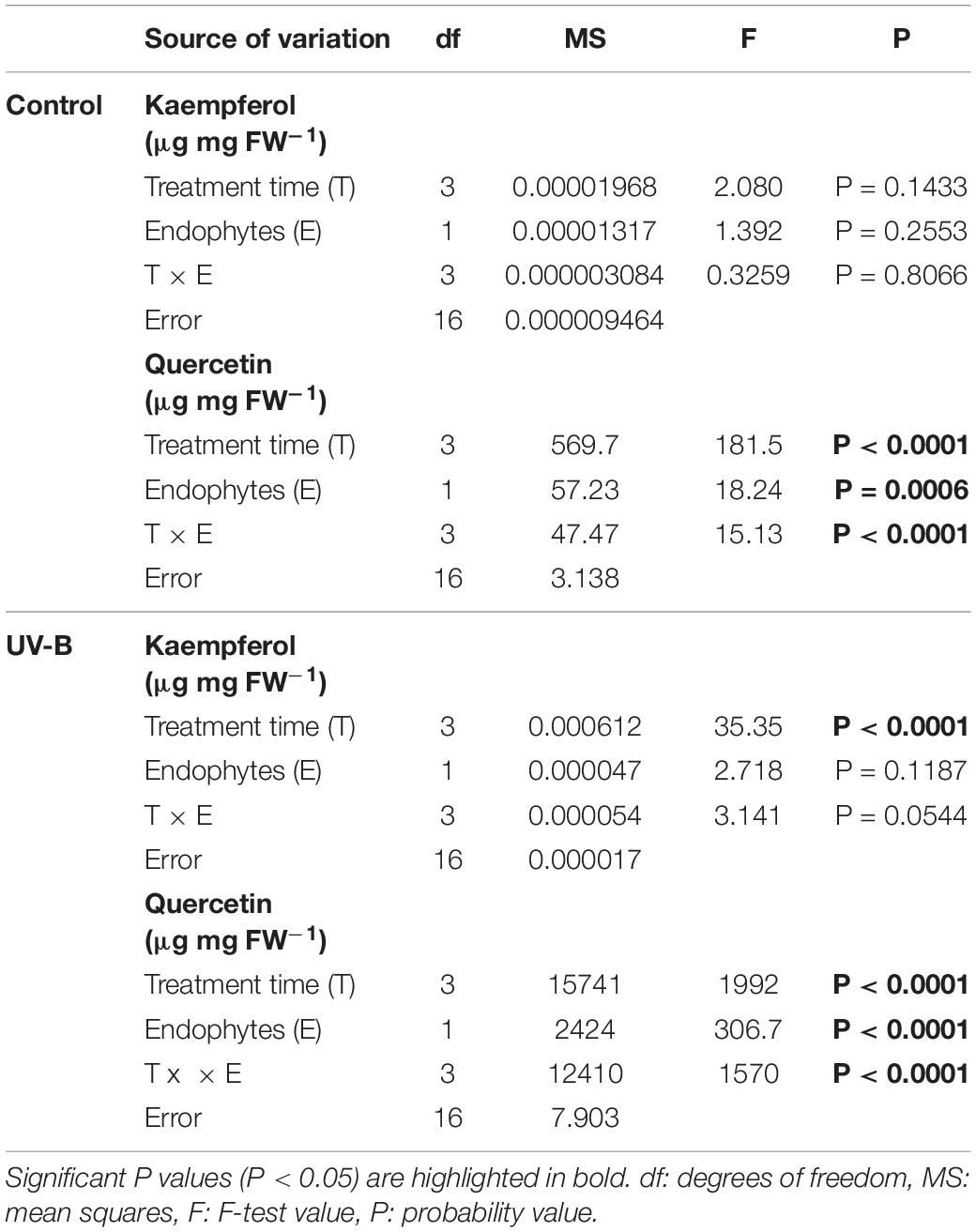
Table 4. Results of factorial ANOVAs evaluating the interactive effects of endophytes presence (E) and different treatment times for (control: without radiation UV-B radiation, UV-B: 46 μmol m–2 s–1) for flavonol content (μg mg fresh weight–1) of kaempferol and quercetin in the leaf of Colobanthus quitensis.
Discussion
The decrease in stratospheric ozone since the mid-1970s has led to a significant increase in UV-B radiation over Antarctica (Ryan et al., 2009). Such changes add extra stress to the plants present in this region, which has boosted additional interest in elucidating the response mechanisms that could be involved in facilitating the survival of sessile organisms such as plants under these unfavorable conditions. Thus far, most studies focused on the physiological, morphological and reproductive performance effects of UV-B radiation in plants, including C. quitensis (Navarrete-Gallegos et al., 2012; Ramos et al., 2018). However, the underlying molecular mechanisms remain poorly understood. In order to improve our knowledge on the underlying molecular mechanisms, we evaluated the UV-B effects on C. quitensis at the ecophysiological level. Hereby, we were able to describe some of the molecular mechanisms involved in the response to UV-B stress. At the same time, we assessed how extrinsic biotic factors, such as the presence of fungal endophytes, can modulate the plant response to UV-B stress.
The presented data underline the fact that the presence of a fungal endophyte can positively affect UV-B tolerance in C. quitensis plants. Plants that contain the fungal endophytes appear to improve their photochemical efficiency. In addition, we observed changes in gene expression and metabolite production, that can help to explain how this Antarctic plant copes with stress conditions exerted by UV-B radiation.
At the biochemical level, the presence of fungal endophytes improved the tolerance to UV-B radiation by decreasing cell membrane lipid peroxidation, as accounted by MDA production (Figure 1). At the molecular level, this study is the first one to evaluate genes that respond to UV-B radiation, and genes associated with the biosynthesis of flavonols in plants of C. quitensis modulated by fungal endophytes. It was observed that UVR8 transcript levels increased earlier than those of HY5 and FLS genes in the leaves of plants exposed to UV-B radiation. Such expression dynamics suggest that UVR8 could control a complex genetic program involved in acclimatization and protection against UV-B radiation. In turn, the increase in expression of HY5 and FLS occurred 24 h after the plant exposure to UV-B radiation, triggering the accumulation of flavonols in the aerial tissues.
In Vitis vinifera L., variation in the expression of UVR8, HY5, and HYH has been described, showing a great dependence on the organ, plant developmental stage as well as the type of radiation (Liu et al., 2015). In consequence, it has been proposed that the UV-B response machinery in V. vinifera would be mainly triggering the accumulation of flavonols in the fruits through the activation of genes, such as HY5 and HYH, both at high and low levels of UV-B exposure (Loyola et al., 2016). In A. thaliana, it has been described that UVR8 controls the expression of several genes involved in acclimatization and protection against UV-B radiation, including those involved in the biosynthesis of flavonoids (phenolic protectants) (reviewed in Ballaré et al., 2011). These metabolites are among the most abundant natural phytochemical compounds with several biological functions that confer tolerance to stress in plants (Henry-Kirk et al., 2018).
In the study by Carrasco-Ríos (2009), it was suggested that high tolerance to UV-B radiation in the Antarctic plants could be attributed to their ability to activate enzymatic and non-enzymatic antioxidant systems, and that secondary metabolites playing an important role in protecting leaves from the highly damaging energy. The increase in the production of secondary metabolites such as polyphenols and flavonoids is considered one of the key mechanisms of adaptation to UV-B radiation based on its antioxidant role against free radicals (Vasela et al., 2013; Liu et al., 2015). These metabolites accumulate in the epidermal cells of many plant and fruit species and have a radiation absorption capacity between 280 and 360 nm. Consequently, these metabolites alleviate the deleterious effect of UV-B light on different cellular components (Carrasco-Ríos, 2009). Our results suggest that the flavonol quercetin plays a relevant role in the response of endophyte-free plants to UV radiation. This is supported by the observation of an 8-fold increase in the levels of this metabolite in leaves 48 h after the exposure of plants to UV-B radiation. This finding is in agreement with previous data showing, in Arabidopsis, an accumulation of flavonols (e.g., saiginol) mainly in floral tissues, correlating with a greater tolerance to UV-B light (Tohge et al., 2016). In V. vinifera, it has been described that flavonols, especially the glycosides of quercetin and kaempferol, increase substantially after exposing the plants to UV-B radiation (Carrasco-Ríos, 2009).
To cope with harsh environmental conditions such as those found in Antarctica, plants have evolved their own mechanisms and those ones that arise from the association with beneficial microbial symbionts. Mutualistic interactions between plants and microorganisms, either fungi or bacteria, can confer several benefits to host plants, such as tolerance to drought, salinity, temperature, and heavy metals, that have been reported to positively influence plant persistence and growth (Rodriguez et al., 2004; Hamilton et al., 2012; Acuña-Rodríguez et al., 2020; Pérez-Alonso et al., 2020). Our results suggest that the presence of endophytic fungi has positive effects on the physiological and biochemical performance of C. quitensis, likely through the transcriptional modulation of key genes related with the plant response to UV-B radiation. Specifically, under UV-B radiation, the levels of lipid peroxidation were higher, and the Fv/Fm ratio was decreased in the absence of endophytes. Considering that a decrease in the Fv/Fm ratio indicates a reduction in the photochemical efficiency of the PSII and likely damage in the photosynthetic apparatus, our results indicate that endophytic fungi improved the capacities of the plant to respond to high UV-B radiation. The expression of the genes UVR8, HY5, and FLS was higher in plants without endophytes exposed to UV-B radiation at early times of exposure (24 h), activating the UV-B specific signaling pathway, but this was not observed in endophyte-symbiotic plants. This observation is in line with reports in which endophytes regulate gene expression in plant hosts to increase stress tolerance (Sun et al., 2010; Xu et al., 2017; Dastogeer et al., 2018; Hereme et al., 2020). Thus, fungal endophytes could be involved in a trade-off to increase the physiological fitness, as demonstrated by higher photochemical efficiency, and to improve the biochemical mechanism, as demonstrated by lower cellular damage by lipid peroxidation. The fact that these differences appeared when plants were exposed to the environmental stress, suggests that the plant-endophyte association activates certain molecular mechanisms to improve the host plant fitness is context-dependent. This is in agreement with our previous study in which C. quitensis associated with extremophile fungal endophytes and exposed to high UV-B radiation displayed a strong decrease in ABA content in shoot tissues (Ramos et al., 2018). In relation with this observation, ABA has been described to promote the biosynthesis and accumulation of flavonols in V. vinifera (Berli et al., 2010, 2011).
It is important to note that plants exposed to UV-B radiation revealed a significant increase in levels of quercetin, specifically at 48 h, with higher levels observed in plants without endophytes compared to those with endophytes (Figure 4). This result is interesting, since it could suggest that until 48 h, the accumulation of quercetin could be the product of an intrinsic response of C. quitensis against UV-B radiation. These results suggest that in the presence of endophytic fungi, as a product of the ecophysiological state of the plant, the responses are delayed or the increase in flavonols are not necessary because other compensatory mechanisms are triggered. However, new studies considering higher levels of UV-B radiation intensity, increase in the evaluation period and the determination of the relative contribution of each member of the association with respect to quercetin could reveal more information to understand this pattern.
The association of plants with microbial endophytes appears to be key in the plant adaptation to stressful conditions. Studies with different degree of detail, show that not only fungal but also bacterial endophytes can be source of novel metabolites and metabolic functions which, in addition to those of host plants, increase the repertory to respond to the environmental variation (Hamilton et al., 2012; Truyens et al., 2015; Bastías et al., 2017, 2020; Acuña-Rodríguez et al., 2020; Pérez-Alonso et al., 2020). As summary, we had previously shown that endophytic foliar fungi associated with C. quitensis under UV-B radiation (5 and 30 μW cm–2), improved the ecophysiological performance of individuals, with higher total biomass and higher number of flowers. Under the same condition, endophyte-symbiotic plants presented lower lipid peroxidation which could be regulated by changes in the hormonal content of salicylic acid, jasmonate, indole-3-acetate and abscisic acid (Ramos et al., 2018).
In this work, we evaluated the effect of fungal endophytes occurring in both above- and below-ground tissues of the plant. Therefore, we cannot rule out that root endophytes have also played a role in modulating the plant tolerance to UV-B radiation. However, we would like to suggest a more relevant role for leaf fungal endophytes. An argument for this is that not only the presence or absence of fungal endophytes are affecting the plant response to UV-B radiation, but it is also expected UV-B to affect the community of microorganisms of the phyllosphere (Jacobs and Sundin, 2001; Santhanam et al., 2017). Therefore, we propose that the modulation of flavonoids in the leaves exposed to sun, results from the positive feedback between the plant-endophyte interaction and the UV-B radiation.
Finally, we were able to link the endophyte presence with UV-B radiation linked gene responses to plant physiological parameters. Although here were separated bacteria from fungi, endophytes are suggested to play part of an integral response of plants to stress factors and should always be considered in future works.
Conclusion
The presented results strongly suggest that the functional symbiosis of C. quitensis plants with extremophile antarctic endophytic fungi modulate mechanisms in plants to allow them to cope with the stress caused by UV-B radiation. The presence of endophytic fungi improves the physiological performance of C. quitensis by reducing cellular damage by means of eliciting the metabolic pathways involved in flavonol biosynthesis. At the molecular level, the presence of endophytic fungi in plants caused a late transcriptional response of the components of the UVR8 pathway, which include the genes UVR8, HY5, and FLS. However, the investigation on the genetic and biochemical basis of symbiotic plant-fungus communication is still in its infancy and the underlying mechanisms are poorly understood (Ramos et al., 2018; Acuña-Rodríguez et al., 2019).
Data Availability Statement
The datasets generated for this study are available in GenBank and can be found in the NCBI database under the accession numbers: GCIB01004125.1, GCIB01008463.1, MH643782, MH643781, and MH643780.
Author Contributions
AB, PR, and MM-M designed the experiments. AB performed the experiments. AB, MM-M and PR analyzed the data. RH prepared the plant material. AB, RH, SR-L, LL, PG, SP, MM-M and PR wrote the paper and all authors reviewed the manuscript.
Funding
We acknowledge the financial, authorization and logistic support of the Chilean Antarctic Institute (INACH). MM-M was supported by project FONDECYT 1181034 and PII 20150126. REDES #190078 from Conicyt. PR acknowledges “Núcleo Científico Multidisciplinario” from Universidad de Talca. This article contributes to the SCAR biological research programs “Antarctic Thresholds - Ecosystem Resilience and Adaptation” (AnT-ERA) and “State of the Antarctic Ecosystem” (AntEco).
Conflict of Interest
The authors declare that the research was conducted in the absence of any commercial or financial relationships that could be construed as a potential conflict of interest.
Acknowledgments
We thank Daniel Zunino for support with plant maintenance and design of experimental devices.
Supplementary Material
The Supplementary Material for this article can be found online at: https://www.frontiersin.org/articles/10.3389/fevo.2020.00122/full#supplementary-material
Footnotes
References
Acuña-Rodríguez, I. S., Hansen, H., Gallardo-Cerda, J., Atala, C., and Molina-Montenegro, M. A. (2019). Antarctic extremophiles: biotechnological alternative to crop productivity in saline soils. Front. Bioeng. Biotechnol. 7:22. doi: 10.3389/fbioe.2019.00022
Acuña-Rodríguez, I. S., Newsham, K. K., Gundel, P. E., Torres-Díaz, C., and Molina-Montenegro, M. A. (2020). Functional roles of microbial symbionts in plant cold tolerance. Ecol. Lett. 12:2020. doi: 10.1111/ele.13502
Acuña-Rodríguez, I. S., Torres-Díaz, C., Hereme, R., and Molina-Montenegro, M. A. (2017). Asymmetric responses to simulated global warming by populations of Colobanthus quitensis along a latitudinal gradient. Peer J 5:e3718. doi: 10.7717/peerj.3718
Ayabe, S., Uchiyama, H., Aoki, T., and Akashi, T. (2010). “plant phenolics: phenylpropanoids,” in Comprehensive Natural Products II, eds H.-W. Liu and L. Mander (Oxford: Elsevier), 929–976.
Ballaré, C. L., Caldwell, M. M., Flint, S. D., Robinson, S. A., and Bornman, J. F. (2011). Effects of solar ultraviolet radiation on terrestrial ecosystems. Patterns, mechanisms, and interactions with climate change. Photochem. Photobiol. Sci. 10, 226–241. doi: 10.1039/c0pp90035d
Bastías, D. A., Johnson, L. J., and Card, S. D. (2020). Symbiotic bacteria of plant-associated fungi: friends or foes? Curr. Opin. Plant Biol. 56, 1–8. doi: 10.1016/j.pbi.2019.10.010
Bastías, D. A., Martínez-Ghersa, M. A., Ballaré, C. L., and Gundel, P. E. (2017). Epichloë fungal endophytes and plant defenses: not just alkaloids. Trends Plant Sci. 22, 939–948. doi: 10.1016/j.tplants.2017.08.005
Beissert, S., and Loser, K. (2008). Review molecular and cellular mechanisms of photocarcinogenesis. Photochem. Photobiol. 19, 29–34.
Berli, F. J., Fanzone, M., Piccoli, P., and Bottini, R. (2011). Solar UV-B and ABA are involved in phenol metabolism of Vitis vinifera L. Increasing biosynthesis of berry skin polyphenols. J. Agric. Food Chem. 59, 4874–4884. doi: 10.1021/jf200040z
Berli, F. J., Moreno, D., Piccoli, P., Hespanhol-Viana, L., Silva, M. F., Bressan-Smith, R., et al. (2010). Abscisic acid is involved in the response of grape (Vitis vinifera L.) cv. malbec leaf tissues to ultraviolet-B radiation by enhancing ultraviolet-absorbing compounds, antioxidant enzymes and membrane sterols. Plant Cell Environ. 33, 1–10. doi: 10.1111/j.1365-3040.2009.02044.x
Caldwell, M. M., Bornman, J. F., Ballaré, C. L., Flint, S. D., and Kulandaivelu, G. (2007). Terrestrial ecosystems, increased solar ultraviolet radiation, and interactions with other climate change factors. Photochem. Photobiol. Sci. 6, 252–266. doi: 10.1039/b700019g
Carrasco-Ríos, L. (2009). Efecto de la radiación ultravioleta-B en plantas. Idesia 27, 59–76. doi: 10.1186/s12864-015-2225-6
Convey, P. (1996). The influence of environmental characteristics on life history attributes of antarctic terrestrial biota. Biol. Rev. 71, 191–225.
Dastogeer, K. M. G., Li, H., Sivasithamparam, K., Jones, M. G. K., and Wylie, S. J. (2018). Fungal endophytes and a virus confer drought tolerance to Nicotiana benthamiana plants through modulating osmolytes, antioxidant enzymes and expression of host drought responsive genes. Environ. Exp. Bot. 149, 95–108.
Day, T. A., Ruhland, C. T., and Xiong, F. S. (2001). Influence of solar ultraviolet-B radiation on Antarctic terrestrial plants: results from a 4-year field study. J. Photochem. Photobiol. B 62, 78–87. doi: 10.1016/s1011-1344(01)00161-0
Dernoeden, P. H., and McIntosh, M. S. (1991). Seasonal responses of perennial ryegrass as influenced by fungicides. Hortscience 26, 1181–1183.
Gallardo-Cerda, J., Levihuan, J., Lavín, P., Oses, R., Atala, C., Torres-Díaz, C., et al. (2018). Antarctic rhizobacteria improve salt tolerance and physiological performance of the Antarctic vascular plants. Polar Biol. 41, 1973–1982.
Hamilton, C. E., Gundel, P. E., Helander, M., and Saikkonen, K. (2012). Endophytic mediation of reactive oxygen species and antioxidant activity in plants: a review. Fungal Divers. 54, 1–10.
Hayes, S., Sharma, A., Fraser, D. P., Trevisan, M., Cragg-Barber, C. K., Tavridou, E., et al. (2017). UV-B perceived by the UVR8 photoreceptor inhibits plant thermomorphogenesis. Curr. Biol. 27, 120–127. doi: 10.1016/j.cub.2016.11.004
Heath, R. L., and Packer, L. (1968). Photoperoxidation in isolated chloroplasts. Arch. Biochem. Biophys. 125, 189–198.
Henry-Kirk, R. A., Plunkett, B., Hall, M., McGhie, T., Allan, A. C., Wargent, J. J., et al. (2018). Solar UV light regulates flavonoid metabolism in apple (Malus x domestica). Plant Cell Environ. 41, 675–688. doi: 10.1111/pce.13125
Hereme, R., Morales-Navarro, S., Ballesteros, G., Barrera, A., Ramos, P., Gundel, P. E., et al. (2020). Fungal endophytes exert positive effects on Colobanthus quitensis under water stress but neutral under a projected climate change scenario in Antarctica. Front. Microbiol. 11:264. doi: 10.3389/fmicb.2020.00264
Hosseini, F., Mosaddeghi, M. R., and Dexter, A. R. (2017). Effect of the fungus Piriformospora indica on physiological characteristics and root morphology of wheat under combined drought and mechanical stresses. Plant Physiol. Biochem. 118, 107–120. doi: 10.1016/j.plaphy.2017.06.005
Jacobs, J. L., and Sundin, G. W. (2001). Effect of solar UV-B radiation on a phyllosphere bacterial community. Appl. Environ. Microb. 67, 5488–5496. doi: 10.1128/AEM.67.12.5488-5496.2001
Jansen, M. A. K., Gaba, V., and Greenberg, B. M. (1998). Higher plants and UV-B radiation: balancing damage, repair and acclimation. Trends Plant Sci. 3, 131–135.
Kaling, M., Kanawati, B., Ghirardo, A., Albert, A., Winkler, J. B., Heller, W., et al. (2015). UV-B mediated metabolic rearrangements in poplar revealed by non-targeted metabolomics. Plant Cell Environ. 38, 892–904. doi: 10.1111/pce.12348
Korckzak-Abshire, M., Lees, A. C., and Jojczyk, A. (2011). First documented record of barn swallow (Hirundo rustica). Pol. Polar Res. 32, 1–6.
Kumar, S., Stecher, G., Li, M., Knyaz, C., and Tamura, K. (2018). MEGA X: molecular evolutionary genetics analysis across computing platforms. Mol. Biol. Evol. 35, 1547–1549. doi: 10.1093/molbev/msy096
Liu, L., Gregan, S., Winefield, C., and Jordan, B. (2015). From UVR8 to flavonol synthase: UV-B- induced gene expression in Sauvignon blanc grape berry. Plant Cell Environ. 38, 905–919. doi: 10.1111/pce.12349
Lois, R. (1994). Accumulation of UV-absorbing flavonoids induced by UV-B radiation in Arabidopsis thaliana L. Planta 194, 498–503.
Loyola, R., Herrera, D., Mas, A., Wong, D. C. J., Höll, J., Cavallini, E., et al. (2016). The photomorphogenic factors UV-B RECEPTOR 1, ELONGATED HYPOCOTYL 5, and HY5 HOMOLOGUE are part of the UV-B signalling pathway in grapevine and mediate flavonol accumulation in response to the environment. J. Exp. Bot. 67, 5429–5445. doi: 10.1093/jxb/erw307
Martens, S., Preuß, A., and Matern, U. (2010). Multifunctional flavonoid dioxygenases: flavonol and anthocyanin biosynthesis in Arabidopsis thaliana L. Phytochemistry 71, 1040–1049. doi: 10.1016/j.phytochem.2010.04.016
Maxwell, K., and Johnson, G. N. (2000). Chlorophyll fluorescence—a practical guide. J. Exp. Bot. 51, 659–668.
McLeod, A. R., Rey, A., Newsham, K. K., Lewis, G. C., and Wolferstan, P. (2001). Effects of elevated ultraviolet radiation and endophytic fungi on plant growth and insect feeding in Lolium perenne, Festuca rubra, F. arundinacea and F. pratensis. J. Photochem. Photobiol. B. 62, 97–107. doi: 10.1016/s1011-1344(01)00151-8
Molina-Montenegro, M. A., Oses, R., Torres-Díaz, C., Atala, C., Zurita-Silva, A., and Ruíz- Lara, S. (2016). Root-endophytes improve the ecophysiological performance and production of an agricultural species under drought condition. AoB Plants 8:lw062. doi: 10.1093/aobpla/plw062
Møller, I. M., Jensen, P. E., and Hansson, A. (2007). Oxidative modifications to cellular components in plants. Annu. Rev. Plant Biol. 58, 459–481. doi: 10.1146/annurev.arplant.58.032806.103946
Moore, D. M. (1970). Studies in Colobanthus quitensis (Kunth) Bartl. and Deschampsia antarctica Desv. II. Taxonomy, distribution and relationships. Brit. Antarc. Surv. Bull. 23, 63–80.
Morales, L. O., Brosché, M., Vainonen, J. P., Sipari, N., Lindfors, A. V., and Strid, Å, et al. (2015). Are solar UV-B- and UV-A-dependent gene expression and metabolite accumulation in Arabidopsis mediated by the stress response regulator radical-induced cell death1? Plant Cell Environ. 38, 878–891. doi: 10.1111/pce.12341
Moriconi, V., Binkert, M., Costigliolo, C., Sellaro, R., Ulm, R., and Casal, J. J. (2018). Perception of sunflecks by the UV-B photoreceptor UV resistance locus 8. Plant Physiol. 177, 75–81. doi: 10.1104/pp.18.00048
Müller-Xing, R., Xing, Q., and Goodrich, J. (2014). Footprints of the sun: memory of UV and light stress in plants. Front. Plant Sci. 5:474. doi: 10.3389/fpls.2014.00474
Navarrete-Gallegos, A. A., Bravo, L. A., Molina-Montenegro, M. A., and Corcuera, U. J. (2012). Respuestas antioxidantes en dos ecotipos de Colobanthus quitensis (Caryophyllaceae) expuestos a alta radiación UV-B y baja temperatura. Rev. Chil. Hist. Nat. 85, 419–433.
Oses Pedraza, R., Torres-Díaz, C., Lavin, P., Retamales-Molina, P., Atala, C., Acuña-Rodríguez, I., et al. (2018). Root fungal endophytes improve the growth of antarctic plants through an enhanced nitrogen acquisition. PeerJ 6:e26774v1. doi: 10.7287/peerj.preprints.26774v1
Parnikoza, I., Kozeretska, I., and Kunakh, V. (2011). Vascular plants of the maritime antarctic: origin and adaptation. Am. J. Plant Sci. 2, 381–395.
Pereira, B. K., Rosa, R. M., da Silva, J., Guecheva, T. N., Oliveira, I. M., Ianistcki, M., et al. (2009). Protective effects of three extracts from Antarctic plants against ultraviolet radiation in several biological models. J. Photochem. Photobiol. B 96, 117–129. doi: 10.1016/j.jphotobiol.2009.04.011
Pérez-Alonso, M. M., Guerrero-Galán, C., Scholz, S. S., Kiba, T., Sakakibara, H., Ludwig-Müller, J., et al. (2020). Harnessing symbiotic plant-fungus interactions to unleash hidden forces from extreme plant ecosystems. J. Exp. Bot. 24:eraa040. doi: 10.1093/jxb/eraa040
Pieterse, C. M., Zamioudis, C., Berendsen, R. L., Weller, D. M., Van Wees, S. C., and Bakker, P. A. (2014). Induced systemic resistance by beneficial microbes. Annu. Rev. Phytopathol. 52, 347–375. doi: 10.1146/annurev-phyto-082712-102340
Ramos, P., Guajardo, J., Moya-León, M. A., and Herrera, R. (2016). A differential distribution of auxin and flavonols in radiata pine stem seedlings exposed to inclination. Tree Genet. Genomes 12:42.
Ramos, P., Le Provost, G., Gantz, C., Plomion, C., and Herrera, R. (2012). Transcriptional analysis of differentially expressed genes in response to stem inclination in young seedlings of pine. Plant Biol. 14, 923–933. doi: 10.1111/j.1438-8677.2012.00572.x
Ramos, P., Rivas, N., Pollmann, S., Casati, P., and Molina-Montenegro, M. A. (2018). Hormonal and physiological changes driven by fungal endophytes increase Antarctic plant performance under UV-B radiation. Fungal Ecol. 34, 76–82.
Rodriguez, R. J., Redman, R. S., and Henson, J. M. (2004). The role of fungal symbioses in the adaptation of plants to high stress environments. Mitig. Adapt. Strat. Global 9, 261–272.
Rosa, L. H., Almeida Vieira, M. L., Santiago, I. F., and Rosa, C. A. (2010). Endophytic fungi community associated with the dicotyledonous plant Colobanthus quitensis (Kunth) Bartl. (Caryophyllaceae) in Antarctica. FEMS Microbiol. Ecol. 73, 178–189. doi: 10.1111/j.1574-6941.2010.00872.x
Ryan, K. G., Burne, A., and Seppelt, R. D. (2009). Historical ozone concentrations and flavonoid levels in herbarium specimens of the Antarctic moss Bryum argenteum. Glob. Chang Biol. 15, 1694–1702.
Sager, J. C., and McFarlane, J. C. (1997). “Radiation,” in Plant Growth Chamber Handbook, eds R. W. Langhans and T. W. Tibbitts (Ames, IA: Iowa State University Press), 1–29.
Santhanam, R., Oh, Y., Kumar, R., Weinhold, A., Luu, V. T., Groten, K., et al. (2017). Specificity of root microbiomes in native-grown Nicotiana attenuata and plant responses to UVB increase Deinococcus colonization. Mol. Ecol. 26, 2543–2562. doi: 10.1111/mec.14049
Seckmeyer, G., Pissulla, D., Glandorf, M., Henriques, D., Johnsen, B., Webb, A., et al. (2008). Variability of UV irradiance in Europe. Photochem. Photobiol. 84, 172–179. doi: 10.1111/j.1751-1097.2007.00216.x
Sequeida, Á., Tapia, E., Ortega, M., Zamora, P., Castro, Á., Montes, C., et al. (2012). Production of phenolic metabolites by Deschampsia antarctica shoots using UV-B treatments during cultivation in a photobioreactor. Electron. J. Biotechnol. 15:7.
Shourie, A., Tomar, P., Srivastava, D., and Chauhan, R. (2014). Enhanced biosynthesis of quercetin occurs as a photoprotective measure in Lycopersicon esculentum Mill. under acute UV-B exposure. Braz. Arch. Biol. Technol. 57, 317–325.
Sun, C., Johnson, J. M., Cai, D., Sherameti, I., Oelmuller, R., and Lou, B. (2010). Piriformospora indica confers drought tolerance in Chinese cabbage leaves by stimulating antioxidant enzymes, the expression of drought-related genes and the plastid localized CAS protein. J. Plant Physiol. 167, 1009–1017. doi: 10.1016/j.jplph.2010.02.013
Tohge, T., Wendenburg, R., Ishihara, H., Nakabayashi, R., Watanabe, M., Sulpice, R., et al. (2016). Characterization of a recently evolved flavonol-phenylacyltransferase gene provides signatures of natural light selection in Brassicaceae. Nat. Commun. 7:12399. doi: 10.1038/ncomms12399
Torres-Díaz, C., Gallardo-Cerda, J., Lavin, P., Oses, R., Carrasco-Urra, F., Atala, C., et al. (2016). Biological interactions and simulated climate change modulates the ecophysiological performance of Colobanthus quitensis in the Antarctic ecosystem. PLoS One 11:e0164844. doi: 10.1371/journal.pone.0164844
Truyens, S., Weyens, N., Cuypers, A., and Vangronsveld, J. (2015). Bacterial seed endophytes: Genera, vertical transmission and interaction with plants. Environ. Microbiol. Rep. 7, 40–50.
Vandesompele, J., De Preter, K., Pattyn, F., Poppe, B., Van Roy, N., De Paepe, A., et al. (2002). Accurate normalization of real-time quantitative RT-PCR data by geometric averaging of multiple internal control genes. Genome Biol. 3, 34–41. doi: 10.1186/gb-2002-3-7-research0034
Vasela, B., Novotna, K., Rajsnerova, P., and Klem, K. (2013). Effects of UV radiation and drought on the accumulation of Uv-Screening compounds and photosynthetic parameters in selected herbs and grasses of the mountain grassland ecosystem. Mendelnet 468, 391–396.
Xu, L., Wang, A., Wang, J., Wei, Q., and Zhang, W. (2017). Piriformospora indica confers drought tolerance on Zea mays L. through enhancement of antioxidant activity and expression of drought-related genes. Crop J. 5, 251–258.
Yang, Y., Liang, T., Zhang, L., Shao, K., Gu, X., Shang, R., et al. (2018). UVR8 interacts with WRKY36 to regulate HY5 transcription and hypocotyl elongation in Arabidopsis. Nat. Plants 4, 98–107. doi: 10.1038/s41477-017-0099-0
Keywords: UV-B stress, Antarctica, Colobanthus quitensis, molecular response, flavonols, fungal endophytes
Citation: Barrera A, Hereme R, Ruiz-Lara S, Larrondo LF, Gundel PE, Pollmann S, Molina-Montenegro MA and Ramos P (2020) Fungal Endophytes Enhance the Photoprotective Mechanisms and Photochemical Efficiency in the Antarctic Colobanthus quitensis (Kunth) Bartl. Exposed to UV-B Radiation. Front. Ecol. Evol. 8:122. doi: 10.3389/fevo.2020.00122
Received: 10 February 2020; Accepted: 15 April 2020;
Published: 06 May 2020.
Edited by:
Bradley Robert Dotson, Lund University, SwedenReviewed by:
Muhammad Hamayun, Abdul Wali Khan University Mardan, PakistanSophie Trouvelot, Université de Bourgogne, France
Copyright © 2020 Barrera, Hereme, Ruiz-Lara, Larrondo, Gundel, Pollmann, Molina-Montenegro and Ramos. This is an open-access article distributed under the terms of the Creative Commons Attribution License (CC BY). The use, distribution or reproduction in other forums is permitted, provided the original author(s) and the copyright owner(s) are credited and that the original publication in this journal is cited, in accordance with accepted academic practice. No use, distribution or reproduction is permitted which does not comply with these terms.
*Correspondence: Patricio Ramos, cHJhbW9zQHV0YWxjYS5jbA==