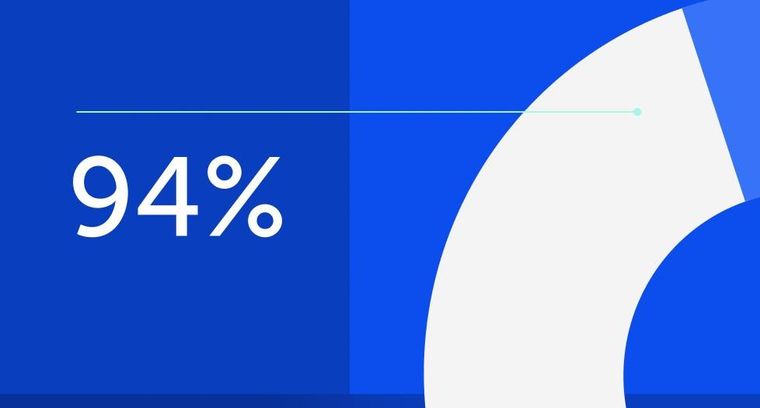
94% of researchers rate our articles as excellent or good
Learn more about the work of our research integrity team to safeguard the quality of each article we publish.
Find out more
ORIGINAL RESEARCH article
Front. Ecol. Evol., 27 May 2020
Sec. Evolutionary Developmental Biology
Volume 8 - 2020 | https://doi.org/10.3389/fevo.2020.00112
This article is part of the Research TopicEvo-Devo of Color Pattern FormationView all 20 articles
Eyespots are wing color pattern elements repeatedly observed in many butterflies, whose developmental genetics has been extensively documented. Nevertheless, the evolutionary forces shaping their diversification across species still remain largely unknown. Here we investigate the evolution of eyespots in the 30 species of the neotropical genus Morpho. Morpho butterflies display a series of eyespots located on the ventral side of their wings, highly variable among species, ranging from large, conspicuous structures to vestigial spots. Applying geometric morphometrics to a large sample (N = 910) spanning all Morpho species and both sexes, we assess eyespot number, position, size, and shape. We detect a divergence in eyespot position between understory and canopy species, with an L-shaped configuration in canopy species and a line pattern in understory species, where the eyespots tend to fuse and form a stripe in white and pale species. This effect is stronger than expected based on a Brownian motion model of phylogenetic divergence, suggesting an adaptation to the microhabitat and an influence of the wing color on the evolution of pattern elements. Remarkably, this shift in color pattern is strongly correlated with a shift in wing shape. However, using a thin-plate spline interpolation, we show that the shape change is insufficient to explain the evolution of eyespot position as a developmental side effect of wing shape evolution, also pointing at an adaptive effect. Finally, we find a significant negative correlation between eyespot relative size and the within-species variation in eyespot number, position, size, and shape, suggesting a relaxed or apostatic selection on small eyespots (rare phenotypes being favored as they are less likely to be remembered and thus detected by predators). We hypothesize that contrasted ecology may explain the observed differences between species: large and phenotypically stable eyespots might act as attack deflectors, small, variable faded eyespots might rather enhance crypsis, and pale species stripe pattern might disrupt the outline of the wing.
Eyespots are among the most studied wing color pattern elements in butterflies, and their developmental genetics is therefore known in great detail [e.g., Nijhout, 1991; Brakefield et al., 1996; reviewed in Monteiro (2015)]: their development has been suggested to be controlled by reaction–diffusion processes involving the focal expression of morphogens typically located in between wing veins. The evolution of eyespots may thus be influenced by variation in wing shape (e.g., Monteiro et al., 1997; Breuker et al., 2007). Direct selection on eyespots has also been demonstrated in some lepidopteran species (reviewed in Stevens, 2005; Kodandaramaiah, 2011). For instance, large conspicuous dorsal eyespots were shown to intimidate or deter predators in some butterfly and moth species (e.g., Vallin et al., 2005); marginal ventral eyespots can deflect predator attacks away from the vital body parts (e.g., Olofsson et al., 2010); in Bicyclus anynana, a role in mate choice was shown for both dorsal (e.g., Robertson and Monteiro, 2005) and ventral eyespots (Huq et al., 2019). Yet the relative roles of natural and sexual selection, developmental constraints linked to wing shape and neutral processes, have not been investigated in a comparative framework. In particular, a prominent role for selection is often assumed (Kodandaramaiah, 2011), and the importance of neutral factors is seldom mentioned (but see Beldade et al., 2002; Allen, 2008). This is surprising as eyespot number and morphology can be highly variable within species (e.g., Maniola jurtina; Brakefield, 1984), suggesting a weak selection. Many species with a cryptic ventral color pattern harbor reduced, inconspicuous, and very variable eyespots, evocative of neutral variation, or apostatic selection, a form of selection where rare phenotypes are favored as they are less likely to be remembered by predators and therefore detected (Clarke, 1969; Bond and Kamil, 2002).
In this study, we investigate the evolution of eyespots in Morpho butterflies, a genus that displays a particularly marked diversity of eyespot number, color, size, and shape (Blandin, 2007). This Neotropical genus is famous for its large species, some of which have bright iridescent blue wings (e.g., Debat et al., 2018). In sharp contrast with the bright colors of the dorsal wing surface, the ventral side is in most cases brownish and rather cryptic—although a few species are very pale and three even have a white background. The ventral color patterns include a series of eyespots that broadly vary among species, from large and highly conspicuous to small and barely visible (Supplementary Figure S1). The phylogeny of the 30 recognized Morpho species was recently updated (Cassildé et al., 2010, 2012; Penz et al., 2012; Chazot et al., 2016). The genus includes a basal clade of two species and two larger clades (Figure 1A). One clade is composed of species that mostly fly at the canopy level, often seen gliding high in the forest strata. All other species, including the two early diverging species, are mostly found flying in the understory, with a typical flapping–gliding behavior (DeVries et al., 2010). Although this binary division between canopy and understory species is likely oversimplifying, it nevertheless seems a meaningful ecological approximation. It was indeed shown that the divergence of wing shape between species from these two microhabitats was stronger than expected from a phylogenetic effect alone (DeVries et al., 2010; Chazot et al., 2016), suggesting an adaptation to those different ecological conditions, possibly via selection of contrasted flight behaviors.
Figure 1. Phylogeny and position of eyespots in Morphos. (A) Phylogeny of the Morpho genus (modified from Chazot et al., 2016). Green, understory species; blue, canopy species. The position of eyespots found in the different species and their frequencies are shown on the right. (B) The 15 possible positions of eyespots on Morpho wings and their frequency, all species confounded. (C) The four landmarks depicting eyespot position, and the eyespot of which the outline shape was quantified. The data are from males only.
Here we explore the diversification of Morpho eyespots and, in particular, the possible roles of neutral evolution and developmental constraints in relation to wing shape evolution and ecology. We focus on eyespot number, presence and absence at each putative location, eyespot position relative to wing veins and outline, and finally on eyespot size and shape using geometric morphometrics to finely depict and quantify phenotypic variation.
We investigate whether neutral divergence is sufficient to explain the observed patterns of eyespot evolution between species. We test adaptive hypotheses against this null hypothesis of neutral evolution: (1) is there any sign of adaptive divergence among microhabitats, possibly in relation with the existence of different predator communities in the canopy and the understory habitats? (2) How much variation of eyespot position can be attributed to variation of wing shape via a passive structural correlation (i.e., a developmental constraint)? (3) Does variation in eyespot size and shape reflect differential selective regimes? In particular, we hypothesize that the largest eyespots might be under stabilizing selection while the smallest might be under relaxed selection. We predict accordingly that small eyespots should be more variable within species than large ones. We briefly discuss our finding in the context of our (limited) knowledge of Morpho ecology.
We sampled specimens from the collection of Paris Museum of Natural History to cover the 30 recognized species and include both sexes within species. We thus photographed 910 individual butterflies (642 males and 268 females) in standardized conditions using a NikonD90 camera (see Chazot et al., 2016). All measurements were performed on the ventral surface of the wings.
The full sample was used to quantify eyespot number and position. Eyespots can occur on both hindwing and forewing at 15 different positions defined by compartments between veins (Figure 1B). For each individual, the presence of eyespot was assessed at every possible location, coded as 0 (absence) or 1 (presence) in a data matrix. We computed the average number of eyespots per wing and per species as well as their average positions and frequency.
We estimated the phylogenetic signal using the K statistic (Blomberg et al., 2003). We then tested the possible occurrence of a difference in eyespot number between canopy (10 species) and understory (20 species) species using a phylogenetic ANOVA as performed in the phytools package (Revell, 2012). Accounting for the phylogeny is particularly important as the distribution of species across microhabitats mostly matches the phylogenetic structure in two main clades (Figure 1A). We also investigated the evolution of sexual dimorphism by computing the difference of mean eyespot number between sexes (a dimorphic species could thus be categorized as monomorphic if the two sexes have the same number of eyespots but in different positions, but this does not occur in the genus Morpho).
Female specimens are few in the collection as males are more often collected than females in the field, so we focused our investigation of eyespot configurations on male specimens alone. Eyespot configuration was defined as the combined presence and absence data at each of the 15 putative locations. We applied a principal component analysis (PCA) on eyespot frequencies at each of the 15 positions within species and projected the phylogenetic tree onto the first PC plane, explaining most of the variance in eyespot configurations. This allowed depicting the similarity in eyespot configuration among species together with the phylogenetic relationships between species. We then quantified the phylogenetic signal of eyespot configuration and tested its significance using the multivariate generalization of the Blomberg K [K-mult function of the R package geomorph (Adams, 2014; Adams et al., 2019)]. We then specifically compared the eyespot configurations of canopy and understory species using a phylogenetic MANOVA to disentangle the effects of neutral divergence from contrasted selective pressures exerted in these two habitats.
To investigate the evolution of eyespot position beyond their presence/absence within each wing compartment, we defined a landmark at the focus of the four most common eyespots of the hindwing (Figure 1C), allowing to precisely quantify their position on the wing. We then analyzed the variation of the resulting sets of landmarks using geometric morphometrics. The sample used for this analysis included 763 individuals from 25 species because some species do not exhibit these four eyespots simultaneously (Morpho marcus, Morpho eugenia, Morpho portis, Morpho aega, and Morpho zephyritis were discarded). The landmarks were superimposed using a generalized Procrustes superimposition (Rohlf and Slice, 1990). We applied a PCA on the species mean coordinates and projected the phylogenetic tree onto the first PC plane. The phylogenetic signal was computed and the canopy and the understory species were again compared using a phylogenetic MANOVA.
To investigate the possible link between the position of eyespot and wing shape evolution, we used another dataset previously obtained on the very same specimens (Chazot et al., 2016), describing wing size and shape using semi-landmarks placed on the wing outline and landmarks on vein intersections. We specifically quantified the covariation between hindwing shape and the position of the four eyespots by applying two-block partial least squares (PLS) regression (Rohlf and Corti, 2000) while accounting for the phylogenetic relationship among species [phylo-integration function of geomorph (Adams et al., 2019)].
We then explored whether the change in eyespot positions was a consequence of a change in wing shape—the eyespot position shifting due to a shift in vein positions. We applied an interpolation algorithm to the eyespot coordinates using the average shape of understory species as the reference (i.e., starting point) and that of the canopy species as a target (i.e., end-point) using the tps2D function of Claude (2008). This procedure allowed us to predict the shift in eyespot positions generated by a shift in wing shape between understory and canopy species. Contrasting the predicted and the observed shifts allows testing whether the eyespot position observed in the canopy species results from the sole evolution of wing shape or whether specific evolutionary forces are acting on the eyespot relative position on the wing.
Finally, we quantified the variation in size and shape of one specific eyespot across the genus. We selected the posterior radial eyespot (Rs) present in all individuals (Figures 1B,C).
A subsample of ca. 15 individuals per species was selected, including the two sexes at an average of 2:1 proportion in favor of males, for a total of 403 individuals. The eyespot morphology was quantified by 149 semi-landmarks equidistantly spaced along the outline, plus two landmarks, one positioned at the center of the eyespot and the other located at the proximal intersection of the outline and the direction parallel to the adjacent veins running through the eyespot center. The landmark and the semi-landmark configurations were superimposed using a generalized Procrustes superimposition (Rohlf and Slice, 1990). The semi-landmarks were sled in TPSrelw (Rohlf, 2015) by minimizing the bending energy (e.g., Gunz and Mitteroecker, 2013). The resulting coordinates in the tangent space were used as shape data, and centroid size was used as a size estimate. The phylogenetic signal associated with these variables describing eyespot shape and size was assessed by the K-mult and the divergence between canopy and understory species tested using a phylogenetic MANOVA.
We then investigated the distribution of eyespot variability across the genus: in particular, we tested whether large eyespots are less variable than smaller ones as would be expected if large eyespots are under a stronger stabilizing selection than the small ones. To do so, we quantified the within-species coefficient of variation for eyespot size and the trace of the within-species covariance matrix for eyespot shape and assessed their correlation with eyespot relative size, i.e., the eyespot size standardized by wing size. This standardization was applied to account for the fact that species with large wings tend to have larger eyespots. The significance of the covariation was tested using phylogenetic regressions as performed in phytools (Revell, 2012).
We also explored the association between eyespot relative size and the variation of other morphological traits as eyespot number and eyespot position.
The most common configuration of eyespots includes three eyespots on the forewing and four eyespots on the hindwing (Figures 1A,B). These seven eyespots are observed in the same positions defined by the veins in all species in most individuals (>84% of individuals). The presence of an eyespot at each of the eight other possible locations on the wings is less frequent (2–27% of species). The total number of eyespots ranges from six (in Morpho rhodopteron) to 11 (in some Morpho lympharis individuals), involving variation of both forewing eyespots (from two in M. rhodopteron and M. portis to five in M. lympharis) and hindwing eyespots (from two in some M. aega and M. zephyritis individuals to seven in Morpho cypris and Morpho rhetenor). A significant phylogenetic signal in eyespot number was detected in both sexes, with consistently stronger values in the hindwing close to the pattern expected under constant Brownian motion divergence K = 1 (Kmale forewing = 0.56 p = 0.014; Kmale hindwing = 0.95, p < 0.001; Kfemale forewing = 0.58, p = 0.015; Kfemale hindwing = 0.89, p < 0.001). No difference between canopy and understory species was detected when accounting for the phylogeny, neither for the total number of eyespots (F = 2.14, p = 0.52) and the forewing (F = 0.49, p = 0.79) nor the hindwing (F = 4.58, p = 0.344). Sexual dimorphism in eyespot number ranged from 0 (in Morpho deidamia, Morpho helenor, and M. zephyritis) to 1.5 in M. cypris, with most species presenting absolute values lower than 0.5 (Supplementary Figure S2). No difference in sexual dimorphism was detected between the hindwings and the forewings (t = −0.08, df = 55.1, p-value = 0.9). Canopy species were found to be more sexually dimorphic than understory species (difference between male and females mean numbers: 0.70 ± 017 SE in canopy vs. 0.26 ± 0.05 SE in understory), but this difference is not larger than expected given the phylogeny (F = 7.98, p = 0.18).
Eyespot configuration displayed a significant phylogenetic signal consistent with neutral divergence among species (K-mult = 0.971, p = 0.001). No difference between canopy and understory species was detected when accounting for the phylogeny (Wilks = 0.43, F = 1.8, p = 0.99). The PCA on male species mean eyespot frequencies is shown in Figure 2. Species with a stable and low number of eyespots are found on the negative PC1 values and species with a high and variable number of eyespots are found on the positive values, with a group of species displaying the “canonical” 3:4 configuration lying in the mid-PC plane. Interestingly, the three white species (Morpho polyphemus, Morpho iphitus, and Morpho epistrophus) cluster together with two very pale species (Morpho sulkowskyi and M. lympharis) due to a common high number of contiguous eyespots on the hindwing.
Figure 2. First two principal components explaining the variation in ventral eyespot presence/absence. The eyespot colors figure their frequency within species.
The PCA applied on the species mean posterior eyespot position (Figure 3) shows a remarkable contrast between canopy species, where the four eyespots form an “L,” and understory species, where they tend to form a line. The phylogenetic signal was strong (K-mult = 1.62, p < 0.001), but the difference between the two microhabitats remained significant when accounting for the phylogeny (F = 7.08, p < 0.001), suggesting a stronger effect than expected under a neutral Brownian model of evolution. Interestingly, the white species M. epistrophus and M. iphitus, (and to a lesser degree M. polyphemus) and the pale species M. sulkowskyi, M. lympharis, and M. rhodopteron are all found on the positive values of PC1, harboring strikingly aligned eyespots.
Figure 3. Phylomorphospace of the four landmark eyespot configurations. PC1 clearly contrasts the L-shaped configuration of canopy species and the line configuration of the understory species. Note the extreme position of the white and the pale species on PC1 positive values.
The correlation between the wing shape and the position of the eyespots was strong (r-PLS = 0.88, p < 0.001) and triggered by the joint divergence for both wing shape and eyespot position between canopy and understory species (Supplementary Figure S3). The correlation was reduced when accounting for the phylogeny but remained significant (r-PLS = 0.64, p = 0.049; Supplementary Figure S3).
The interpolation of the wing shape change from understory to canopy, applied to the understory configuration of eyespots, is shown in Figure 4. The expected effect of the wing shape change is a shift from the “aligned” understory mean eyespot position to a more “L-shaped” disposition. However, the induced shift is clearly less marked than the observed difference between understory and canopy mean eyespot positions, albeit in the same direction. The observed change in eyespot position therefore cannot be fully accounted for by a correlated response of eyespot position to the evolution of wing shape, pointing at a specific selective effect on eyespots.
Figure 4. Interpolation of the position of the four eyespots relative to shape change between understory and canopy mean wing shapes. The red arrows point at the observed (right) and interpolated (left) shifts in eyespot positions.
The PCA on the first posterior eyespot shape (Figure 5) mainly contrasts eyespots that are elongated in the opposite direction: on the positive PC1 values, the white species M. epistrophus and M. iphitus display an eyespot markedly elongated transversally to the veins, while M. telemachus or M. theseus eyespots are slightly elongated longitudinally, on the negative values of PC1. There is a significant phylogenetic signal in eyespot shape (K-mult = 0.77, p = 0.001) and a significant difference between canopy and understory species when accounting for the phylogeny (F = 0.42, p = 0.05).
The analysis of eyespot variability suggested that relatively small eyespots tend to be more variable than larger ones: a strong negative correlation between the relative spot size and the within-species coefficient of variation of spot size was detected, which remained significant when accounting for the phylogeny (r = −0.587, t = −3.39, p = 0.002; Figure 6). Similarly, the shape of the small eyespots was found to be more variable (larger trace of the within-species covariance matrix) than that of the large eyespots, a trend that was higher than expected given the phylogenetic structure (r = −0.37; t = −4.1, p < 0.001; Figure 6). A similar pattern was found for eyespot number and position, whose variability was negatively correlated to eyespot relative size (Supplementary Figure S4). The species whose eyespots are the most variable include the white species (M. iphitus, M. epistrophus, and M. polyphemus) and two pigmented species whose eyespots tend to fade and almost disappear in some individuals (M. aega and Morpho anaxibia; see Supplementary Figure S1).
Figure 6. Correlation between eyespot mean relative size and eyespot variation. Top: within-species coefficient of variation of size. Bottom: within-species trace of the shape covariance matrix (shape variation).
Overall our results suggest that the evolution of eyespots in Morpho butterflies results from a combination of neutral divergence and selection. While the number of eyespots appears rather labile, with a strong phylogenetic signal and no divergence among microhabitats, possibly indicative of neutral evolution, their precise position and relative size are more strongly structured by ecology, which might stem from selective effects.
The number and the distribution of eyespots are highly variable among species. The two early diverging species, M. marcus and M. eugenia display a 3:3 configuration on average, which is less than most other species with a typical 3:4 configuration (Figure 1). Interestingly, their two most posterior eyespots on the hindwing are located on positions Cu1 and Cu2, contrasting with all other species harboring only three posterior eyespots that are located on M3 and Cu1. This difference suggests a certain lability in the distribution of eyespots across species. The phylogenetic signal was close to the neutral K = 1 and no difference was detected between understory and canopy species. These results are compatible with the hypothesis of a neutral evolution of eyespot number and distribution throughout species diversification. Such an evolutionary lability is also congruent with the known developmental basis of eyespot formation. Eyespots are indeed developmental serial homologs (e.g., Allen, 2008; Monteiro, 2015), i.e., they consist in the repeated expression at different locations of the same genetic network (Oliver et al., 2014). The presence of an eyespot at a specific position then depends on the local concentration of morphogens that triggers the expression of the eyespot genetic network [see Monteiro (2015) for a review]. Changes in size, shape, or color composition of a specific eyespot, which involve changes in the eyespot genetic network, have strong pleiotropic effects on other eyespots (e.g., Brakefield, 2001). In contrast, it is conceivable that adding or removing an eyespot, relying on a simple switch on or off of the network expression (for example, by a local change in a morphogen concentration), would be less developmentally constrained [but see Beldade and Brakefield (2003)]. The evolution of eyespot number and configuration in Morpho would thus be characterized by an important role of drift and a low impact of developmental constraints.
The absence of association between the number of eyespots and microhabitat strikingly contrasts with the evolution of the position of the four most common eyespots on the hindwing, which is driven by a strong divergence between microhabitats (Figure 3). Although partly explained by the phylogenetic signal, the divergence is stronger than expected from a neutral evolution, suggesting that selective forces are involved. The results of the interpolation analysis (Figure 4) concur with this interpretation. We had previously shown that wing shape has diverged between microhabitats (Chazot et al., 2016), a divergence interpreted as the mark of a selection on flight behavior, favoring gliding flight in the open canopy and a maneuverable flapping flight in the dense understory (DeVries et al., 2010; Chazot et al., 2016; Le Roy et al., 2019). The interpolated shift in eyespot position induced by the wing shape change, while in the same direction as the observed difference between understory and canopy species, is markedly less pronounced (Figure 4). This demonstrates that the difference in color pattern is more than a simple by-product of a difference in wing shape that would impose a shift in eyespot position via a developmental correlation.
What selective pressure might thus be involved in this divergence between understory-aligned to canopy-L-shaped configurations of eyespots? The answer might partly lie in the apparent convergence between white and pale understory species, which is clear not only for the eyespot position (Figure 3) but also for their number and configuration (Figure 2). In these species, the aligned eyespots tend to form a striped pattern (Figure 7), which even involves the transversal elongation of the eyespots as shown in our shape analysis (Figure 5). This is particularly marked in the two white species M. epistrophus and M. iphitus, but remarkably a similar transversal elongation is found on the subsequent posterior eyespots in the pale species (Figure 7). Altogether these suggest that in pale and white species a dark stripe pattern is favored over circular eyespots that tend to lose their individuality and form a stripe. Such an alignment and elongation seem to extend to two other species (M. portis and M. zephyritis) that were not included in our position analysis due their reduced number of posterior eyespots (typically 3) and that also display marked stripes in association with their aligned eyespots. Interestingly, M. portis is a rather pale species, the ventral side of M. zephyritis males is also quite pale, with white and brown stripes, and the female M. zephyritis are white. The selective force promoting such a stripe pattern in the white and pale species is unknown, but stripes parallel to the wing margin have been suggested to disrupt the animal’s outline and increase the difficulty of capture [e.g., Seymoure and Aiello, 2015; see Stevens and Merilaita (2009) for review]. The evolution of a pale coloration in Morpho may modify the way natural and sexual selection act on color pattern, possibly explaining the shift from separate eyespots to a single, fused ‘stripe’. Specific ecological situations may promote the evolution of striped patterns in different Morpho clades: for example, all pale species are part of a clade of small-sized species that feed on bamboos (Chazot et al., in revision) and typically fly amidst very dense bushes where their striped color pattern might be cryptic. Estimating the effect of color pattern variation on predation risk in different microhabitats is now required to clarify the selective forces involved in the evolution of the striped patterns in Morpho.
Figure 7. White and pale species configurations of posterior eyespots, showing their aligned disposition. Note the strongly elongated shape of all eyespots in M. iphitus and in posterior eyespots in M. sulkowskyi and M. lympharis.
Stabilizing selection tends to reduce phenotypic variation both by removing genetic variants and by favoring canalized phenotypes, i.e., invariant to mutations and/or environmental influences (e.g., Waddington, 1957; Wagner et al., 1997; Meiklejohn and Hartl, 2002); relaxed selection is in turn expected to be associated with a relatively high phenotypic variation (e.g., Crespi and Vanderkist, 1997; Billet et al., 2012). The general negative association detected in this study between eyespot mean relative size and its variation, both in size, shape, number, and location (Figure 5 and Supplementary Figure S4), might suggest that small eyespots experience reduced selective pressure relatively to large eyespots. Interspecific correlations of variability patterns are difficult to interpret as selection operates within species that may differ in ecology, demography, and genetic control of the studied phenotype. A similar selection regime might thus have contrasted effects on phenotypic variation in different species because of such differences. The observed correlation—that accounts for the phylogenetic relatedness—is nevertheless consistent with a differential selection on large and small eyespots. Large conspicuous eyespots have been suggested to have an intimidating effect upon predators, either due to their resemblance to vertebrate’s eye or simply to their conspicuousness [reviewed in Stevens (2005)]. This, however, seems unlikely for ventral eyespots that are always visible when the butterfly is at rest. Alternatively, ventral eyespots might deflect predator attacks away from the body (e.g., Olofsson et al., 2010; Prudic et al., 2015). Huq et al. (2019) have recently shown that ventral eyespot color plays a role in mate choice in B. anynana, leaving open the possibility that eyespot size and shape might be under sexual selection. Conspicuous eyespots are thus expected to be under tight selection, which might lead to a reduced morphological variation (e.g., Nieberding et al., 2018). Notably, species exhibiting the smallest and most variable eyespots (Figure 6 and Supplementary Figure S4) are either white species (Figure 7) or species wherein the eyespots are extremely faded and sometimes barely qualify as eyespots (M. aega and M. anaxibia; Supplementary Figure S1). In the former case, the increased variation might be related to a hypothetical modification of the selection regime that would no longer stabilize the individual eyespot size and shape but the overall stripe pattern. In the latter case, the cryptic color pattern rather than the eyespot conspicuousness might be favored by selection. The reduction in size, the fading, and the increased variation of eyespots in these species might thus reflect a relaxed selection. It has been suggested that crypsis is improved when color patterns are more variable, making it more difficult for the predators to remember and identify, a phenomenon called apostatic selection [reviewed in Bond (2007)]. Rare cryptic patterns could thus be favored by negative frequency-dependent selection, therefore promoting their variation [see Johannesson and Butlin (2017) for a discussion]. Although fully speculative, the occurrence of apostatic selection in species presenting reduced eyespots might explain their particularly strong variation. Additional research documenting the ecology of Morpho, and in particular the predation pressures acting on color pattern variations, are needed to investigate those hypotheses.
All data are available at DRYAD (accession 10.5061/dryad.q573n5tfs).
VD, VL, and NC conceived the study. SJ acquired the data. VD and SJ analyzed the data. VD drafted the manuscript. All authors reviewed and approved the manuscript.
Financial support was provided to VD by the Museum ATM Blanche 2016. Labex BCDiv (ANR-10-LABX-0003) to VD.
The authors declare that the research was conducted in the absence of any commercial or financial relationships that could be construed as a potential conflict of interest.
We would like to thank Stephen Panara and Nicolas Zilbermann for their help with the picture acquisition, Marianne Elias for helpful discussions, and two reviewers for their constructive comments on previous drafts of the manuscript.
The Supplementary Material for this article can be found online at: https://www.frontiersin.org/articles/10.3389/fevo.2020.00112/full#supplementary-material
FIGURE S1 | Examples of large and small eyespots. (A) Large conspicuous and stable eyespots in M. hecuba and M. deidamia. (B) Small, faded, and variable eyespots in M. anaxibia and M. aega.
FIGURE S2 | Anterior and posterior eyespot numbers in males and females.
FIGURE S3 | Covariation between wing shape and the position of the four posterior eyespots. Top: two-blocks partial least squares (PLS) on the total dataset. Bottom: two-blocks phylogenetic PLS on species means.
FIGURE S4 | Covariation between eyespot size and eyespot position variation (top) and eyespot number variation (bottom).
Adams, D. C. (2014). A generalized K statistic for estimating phylogenetic signal from shape and other high-dimensional multivariate data. Syst. Biol. 63, 685–697. doi: 10.1093/sysbio/syu030
Adams, D. C., Collyer, M., and Kaliontzopoulou, A. (2019). Geomorph: Software for Geometric Morphometric Analyses. R Package Version 3.1.0.
Allen, C. E. (2008). The “Eyespot Module” and eyespots as modules: development, evolution, and integration of a complex phenotype. J. Exp. Zool. B Mol. Dev. Evol. 310, 179–190. doi: 10.1002/jez.b.21186
Beldade, P., and Brakefield, P. M. (2003). Concerted evolution and developmental integration in modular butterfly wing patterns. Evol. Dev. 5, 169–179. doi: 10.1046/j.1525-142x.2003.03025.x
Beldade, P., Koops, K., and Brakefield, P. M. (2002). Developmental constraints versus flexibility in morphological evolution. Nature 416:844. doi: 10.1038/416844a
Billet, G., Hautier, L., Asher, R. J., Schwarz, C., Crumpton, N., Martin, T., et al. (2012). High morphological variation of vestibular system accompanies slow and infrequent locomotion in three-toed sloths. Proc. R. Soc. B Biol. Sci. 279, 3932–3939. doi: 10.1098/rspb.2012.1212
Blomberg, S. P., Garland, T. Jr., and Ives, A. R. (2003). Testing for phylogenetic signal in comparative data: behavioral traits are more labile. Evolution 57, 717–745. doi: 10.1111/j.0014-3820.2003.tb00285.x
Bond, A. B. (2007). The evolution of color polymorphism: crypticity, searching images, and apostatic selection. Annu. Rev. Ecol. Evol. Syst. 38, 489–514.
Bond, A. B., and Kamil, A. C. (2002). Visual predators select for crypticity and polymorphism in virtual prey. Nature 415, 609–613. doi: 10.1038/415609a
Brakefield, P. M. (1984). “Ecological genetics of quantitative characters of Maniola jurtina and other butterflies,” in Proceedings of the Symposia of the Royal Entomological Society of London, Rock Hill, SC.
Brakefield, P. M. (2001). Structure of a character and the evolution of butterfly eyespot patterns. J. Exp. Zool. 291, 93–104. doi: 10.1002/jez.1062
Brakefield, P. M., Gates, J., Keys, D., Kesbeke, F., Wijngaarden, P. J., Montelro, A., et al. (1996). Development, plasticity and evolution of butterfly eyespot patterns. Nature 384:236. doi: 10.1038/384236a0
Breuker, C. J., Gibbs, M., Van Dyck, H., Brakefield, P. M., Klingenberg, C. P., and Van Dongen, S. (2007). Integration of wings and their eyespots in the speckled wood butterfly Pararge aegeria. J. Exp. Zool. B Mol. Dev. Evol. 308, 454–463. doi: 10.1002/jez.b.21171
Cassildé, C., Blandin, P., Pierre, J., and Bourgoin, T. (2010). Phylogeny of the genus Morpho fabricius, 1807, revisited (Lepidoptera, Nymphalidae). Bull. Soc. Entomol. France 115, 225–250.
Cassildé, C., Blandin, P., and Silvain, J. F. (2012). Phylogeny of the genus Morpho fabricius 1807: insights from two mitochondrial genes (Lepidoptera: Nymphalidae). Ann. Soc. Entomol. France 48, 173–188.
Chazot, N., Panara, S., Zilbermann, N., Blandin, P., Le Poul, Y., Cornette, R., et al. (2016). Morpho morphometrics: shared ancestry and selection drive the evolution of wing size and shape in Morpho butterflies. Evolution 70, 181–194. doi: 10.1111/evo.12842
Chazot, N., Blandin, P., Elias, M., Debat, V., and Condamine, F. L. (in revision). Are rates of species, and phenotypic diversification correlated in (the) Morpho butterflies?Google Scholar
Crespi, B. J., and Vanderkist, B. A. (1997). Fluctuating asymmetry in vestigial and functional traits of a haplodiploid insect. Heredity 79:624.
Debat, V., Berthier, S., Blandin, P., Chazot, N., Elias, M., Gomez, D., et al. (2018). Why are Morpho Blue? In Biodiversity and Evolution. Amsterdam: Elsevier, 139–174.
DeVries, P. J., Penz, C. M., and Hill, R. I. (2010). Vertical distribution, flight behaviour and evolution of wing morphology in Morpho butterflies. J. Anim. Ecol. 79, 1077–1085. doi: 10.1111/j.1365-2656.2010.01710.x
Gunz, P., and Mitteroecker, P. (2013). Semi-landmarks: a method for quantifying curves and surfaces. Hystrix Ital. J. Mammal. 24, 103–109.
Huq, M., Bhardwaj, S., and Monteiro, A. (2019). Male Bicyclus anynana butterflies choose females on the basis of their ventral UV-reflective eyespot centers. J. Insect Sci. 19:25. doi: 10.1093/jisesa/iez014
Johannesson, K., and Butlin, R. K. (2017). What explains rare and conspicuous colours in a snail? A test of time-series data against models of drift, migration or selection. Heredity 118, 21–30. doi: 10.1038/hdy.2016.77
Kodandaramaiah, U. (2011). The evolutionary significance of butterfly eyespots. Behav. Ecol. 22, 1264–1271.
Le Roy, C., Debat, V., and Llaurens, V. (2019). Adaptive evolution of butterfly wing shape: from morphology to behaviour. Biol. Rev. 94, 1261–1281. doi: 10.1111/brv.12500
Meiklejohn, C. D., and Hartl, D. L. (2002). A single mode of canalization. Trends Ecol. Evol. 17, 468–473.
Monteiro, A. (2015). Origin, development, and evolution of butterfly eyespots. Annu. Rev. Entomol. 60, 253–271. doi: 10.1146/annurev-ento-010814-020942
Monteiro, A., Brakefield, P. M., and French, V. (1997). The relationship between eyespot shape and wing shape in the butterfly Bicyclus anynana: a genetic and morphometrical approach. J. Evol. Biol. 10, 787–802.
Nieberding, C. M., San Martin, G., Saenko, S., Allen, C. E., Brakefield, P. M., and Visser, B. (2018). Sexual selection contributes to partial restoration of phenotypic robustness in a butterfly. Sci. Rep. 8:14315. doi: 10.1038/s41598-018-32132-8
Nijhout, H. F. (1991). The Development and Evolution Of Butterfly Wing Patterns. Washington, DC: Smithsonian Institution Press.
Oliver, J. C., Beaulieu, J. M., Gall, L. F., Piel, W. H., and Monteiro, A. (2014). Nymphalid eyespot serial homologues originate as a few individualized modules. Proc. R. Soc. B Biol. Sci. 281:20133262. doi: 10.1098/rspb.2013.3262
Olofsson, M., Vallin, A., Jakobsson, S., and Wiklund, C. (2010). Marginal eyespots on butterfly wings deflect bird attacks under low light intensities with UV wavelengths. PLoS One 5:e10798. doi: 10.1371/journal.pone.0010798
Penz, C. M., Devries, P. J., and Wahlberg, N. (2012). Diversification of Morpho butterflies (Lepidoptera, Nymphalidae): a re-evaluation of morphological characters and new insight from DNA sequence data. Syst. Entomol. 37, 670–685.
Prudic, K. L., Stoehr, A. M., Wasik, B. R., and Monteiro, A. (2015). Eyespots deflect predator attack increasing fitness and promoting the evolution of phenotypic plasticity. Proc. R. Soc. B Biol. Sci. 282:20141531. doi: 10.1098/rspb.2014.1531
Revell, L. J. (2012). phytools: an R package for phylogenetic comparative biology (and other things). Methods Ecol. Evol. 3, 217–223.
Robertson, K. A., and Monteiro, A. (2005). Female Bicyclus anynana butterflies choose males on the basis of their dorsal UV-reflective eyespot pupils. Proc. R. Soc. B Biol. Sci. 272, 1541–1546. doi: 10.1098/rspb.2005.3142
Rohlf, F. J., and Corti, M. (2000). Use of two-block partial least-squares to study covariation in shape. Syst. Biol. 49, 740–753. doi: 10.1080/106351500750049806
Rohlf, F. J., and Slice, D. (1990). Extensions of the Procrustes method for the optimal superimposition of landmarks. Syst. Biol. 39, 40–59.
Seymoure, B. M., and Aiello, A. (2015). Keeping the band together: evidence for false boundary disruptive coloration in a butterfly. J. Evolut. Biol. 28, 1618–1624. doi: 10.1111/jeb.12681
Stevens, M. (2005). The role of eyespots as anti-predator mechanisms, principally demonstrated in the Lepidoptera. Biol. Rev. 80, 573–588. doi: 10.1017/S1464793105006810
Stevens, M., and Merilaita, S. (2009). Defining disruptive coloration and distinguishing its functions. Philos. Trans. R. Soc. B Biol. Sci. 364, 481–488. doi: 10.1098/rstb.2008.0216
Vallin, A., Jakobsson, S., Lind, J., and Wiklund, C. (2005). Prey survival by predator intimidation: an experimental study of peacock butterfly defence against blue tits. Proc. R. Soc. B Biol. Sci. 272, 1203–1207. doi: 10.1098/rspb.2004.3034
Keywords: eyespot, morphometrics, Evo-Devo, evolution, selection
Citation: Debat V, Chazot N, Jarosson S, Blandin P and Llaurens V (2020) What Drives the Diversification of Eyespots in Morpho Butterflies? Disentangling Developmental and Selective Constraints From Neutral Evolution. Front. Ecol. Evol. 8:112. doi: 10.3389/fevo.2020.00112
Received: 13 December 2019; Accepted: 06 April 2020;
Published: 27 May 2020.
Edited by:
Marcus Kronforst, The University of Chicago, United StatesReviewed by:
Patricia Johnston Moore, University of Georgia, United StatesCopyright © 2020 Debat, Chazot, Jarosson, Blandin and Llaurens. This is an open-access article distributed under the terms of the Creative Commons Attribution License (CC BY). The use, distribution or reproduction in other forums is permitted, provided the original author(s) and the copyright owner(s) are credited and that the original publication in this journal is cited, in accordance with accepted academic practice. No use, distribution or reproduction is permitted which does not comply with these terms.
*Correspondence: Vincent Debat, ZGViYXRAbW5obi5mcg==
Disclaimer: All claims expressed in this article are solely those of the authors and do not necessarily represent those of their affiliated organizations, or those of the publisher, the editors and the reviewers. Any product that may be evaluated in this article or claim that may be made by its manufacturer is not guaranteed or endorsed by the publisher.
Research integrity at Frontiers
Learn more about the work of our research integrity team to safeguard the quality of each article we publish.