- Department of Biology, University of York, York, United Kingdom
Environmental conditions affect insect fitness, with many species constrained by specific temperature ranges. Aphids are limited to temperate climates and it is hypothesized that this is partly due to their heat-susceptible obligate nutritional symbiont Buchnera. Aphids often carry additional facultative symbionts which can increase the host's fitness after heat stress. Here we used the pea aphid (Acyrthosiphon pisum) and three of its facultative endosymbionts (Candidatus Regiella insecticola, Candidatus Fukatsuia symbiotica (X-type; PAXS), and Candidatus Hamiltonella defensa) to investigate how these species respond to heat stress and whether their presence affects the fitness of the host or the obligate symbiont. We exposed aphid lines to a single high temperature event and measured lifetime fecundity and population densities of both obligate and facultative symbionts. Heat shock reduced aphid fecundity, but for aphids infected with two of the facultative symbionts (Regiella or Fukatsuia), this reduction was less than in uninfected aphids. The population density of Buchnera was also reduced after heat shock, and only recovered in aphids infected with Regiella or Fukatsuia but not in uninfected aphids or those with Hamiltonella. Although heat shock initially reduced the densities of two of the facultative symbionts (Hamiltonella and Fukatsuia), all facultative symbiont densities recovered by adulthood. Two of the facultative symbionts tested therefore aided the recovery of the obligate symbiont and the host, and we discuss possible underlying mechanisms. Our work highlights the beneficial effects of protective symbionts on obligate symbiont recovery after heat stress and how facultative symbionts may affect the wider ecological community.
Introduction
It is well-established that infection with bacterial symbionts can affect an insect host's biology. Reproductive fitness, insect behavior, immune pathway function, and responses to natural enemies may all be influenced by the presence of endosymbionts (Dion et al., 2011; Gerardo and Parker, 2014; Vorburger, 2014; Martinez et al., 2015). By improving the ecological fitness of a host through raising its immunity to natural enemies, or by enhancing its tolerance to environmental stress, a vertically transmitted symbiont increases its own fitness (Oliver et al., 2005; Brownlie and Johnson, 2009).
Rising global temperatures are already affecting insect populations; there is evidence of range shifts (Parmesan and Yohe, 2003), changes in phenology (Walther et al., 2002) and interactions with predators and parasitoids (Harrington et al., 1999; Schmitz and Barton, 2014). For several insect groups infection with various bacterial symbionts has been shown to enhance resistance to temperature stress (Corbin et al., 2017). These effects may be direct symbiont-mediated host protection (Montllor et al., 2002; Neelakanta et al., 2010; Brumin et al., 2011) or indirect effects of temperature on the symbiont itself (Chen et al., 2009; Bordenstein and Bordenstein, 2011).
There are a number of hypotheses for the mechanisms underlying indirect symbiont-mediated protection from heat. Infection with symbionts has been shown to increase the expression of immune system genes (Laughton et al., 2013), and it is hypothesized that this immune response controls the bacteria, restricting growth or location and protecting the host from microbial over-proliferation (Kwong et al., 2017; Maire et al., 2018). It may also bestow temperature tolerance as a by-product. For example, infection of Rickettsia in whiteflies leads to the upregulation of stress-response genes in the host and thus increases survival of the insect under heat shock (Brumin et al., 2011); similarly, insects infected with bacterial symbionts often produce more immune cells than those that are uninfected (Schmitz et al., 2012; Weiss et al., 2012; Laughton et al., 2013; Kim et al., 2015). There are close links between insect responses to heat and to infection–many heat shock proteins are chaperones that aid protein production and refolding post-stress, and they may also enhance immune responses (Young et al., 1993).
Instead of indirectly affecting the host's stress or immune responses, symbionts may themselves produce and release heat shock proteins or metabolites that directly protect the host or other microbes that the host depends on (i.e., obligate symbionts) (Burke G. et al., 2010). Obligate symbionts are often a thermal “weak link” and more susceptible to temperature extremes than their hosts (Corbin et al., 2017; Shan et al., 2017; Zhang et al., 2019). Shielding an obligate symbiont from thermal damage would therefore benefit both the host and all of its symbionts. For example, in pea aphids that experience heat shock, the density of the obligate nutritional symbiont Buchnera aphidicola is usually reduced, but is maintained at near normal levels in aphids that carry the facultative symbiont Candidatus Serratia insecticola (hereafter Serratia) (Burke G. et al., 2010). It is also plausible that facultative symbionts might be directly protecting the host by replacing an obligate symbiont that is no longer able to perform its function. When the obligate symbiont Buchnera is removed using antibiotics at benign temperatures, Serratia in pea aphids moved into the bacteriocytes vacated by Buchnera and subsequently allow the stressed aphid to survive and reproduce (Koga et al., 2003, 2007).
Pea aphids, Acyrthosiphon pisum, are a model system for understanding how facultative symbionts protect their hosts from thermal stress. They and their obligate symbiont are typically intolerant to heat in laboratory populations (Dixon et al., 1987; Dunbar et al., 2007), but three of their eight potential facultative symbionts (Serratia, Candidatus Fukatsuia symbiotica and Candidatus Hamiltonella defensa; hereafter Fukatsuia and Hamiltonella, respectively) are known to improve survival or reproduction after heat shock (Montllor et al., 2002; Koga et al., 2003; Russell and Moran, 2006; Heyworth and Ferrari, 2015). The obligate symbiont Buchnera synthesizes essential amino acids for the host, which are required for aphids to thrive on their imbalanced diet of plant phloem sap (Douglas, 1998). Buchnera has a highly reduced genome (Moran, 1996; Gómez-Valero et al., 2007) and some genotypes are susceptible to high temperatures; under heat stress, just five protective heat shock proteins are deployed (Wilcox et al., 2003) compared to over 75 in its free living relative Escherichia coli (Carruthers and Minion, 2009) and during severe heat shock Buchnera can be killed.
While the costs and benefits of infection are being explored in a broad spectrum of insect species, relatively little is known about how different facultative symbionts confer increased heat tolerance to their hosts, and how these mechanisms vary depending on symbiont species. Understanding how insects can and will respond to increases in temperature is vital to accurately model current and future populations. We investigate whether three common facultative symbionts of the pea aphid [Candidatus Regiella insecticola (hereafter Regiella), Fukatsuia and Hamiltonella] protect the host and how they respond to heat stress themselves. Importantly, we test whether the protection from the effects of heat co-occur with the protection of the obligate symbiont Buchnera. We test whether the facultative symbionts directly protect Buchnera, allow it to recover after heat stress or protect the host by replacing Buchnera and whether this mode of protection is similar for all tested facultative symbionts.
Materials and Methods
Aphids and Symbionts
Rapid, asexual reproduction results in clonal lines of aphids that can be kept indefinitely under long-day conditions. This allows the manipulation of facultative symbiont presence through antibiotic curing or artificial infections while maintaining an essentially identical aphid genotype. Two pea aphid genotypes were used for this study, both collected from the UK (Supplementary Table S1). Genotype 218 was collected naturally infected with Fukatsuia and Hamiltonella, and was cured more than a year before use. This was achieved by feeding young aphids with broad bean leaves suspended in a tube of antibiotic solution (0.5% Gentomicin, 1% Ampicillin, 0.5% Cefotaxime in distilled water) over 4 days (McLean et al., 2011). Genotype 200 was collected naturally uninfected, harboring no known facultative symbionts. All aphid lines were screened for Hamiltonella, Regiella, Serratia symbiotica, Fukatsuia, Spiroplasma sp., Rickettsia sp., and Rickettsiella viridis following protocols in Tsuchida et al. (2010) and Ferrari et al. (2012) to ensure that they had the appropriate symbiont infection and were not infected with any other known facultative symbionts. The symbiont infections were regularly checked to detect possible contamination. The symbiont-specific PCR primers can be found in Supplementary Table S2. The PCR mix comprised 6.25 μl BioMix (Bioline), 0.1 μl (20 μM) of forward and 0.1 μl (20 μM) reverse primer, 5.55 μl distilled water and 1.0 μl sample DNA. The PCR reaction was performed at 94°C for 2 min, followed by 35 cycles of: 94°C for 30 s, 55°C for 30 s, and 72°C for 1 min. It concluded with 6 min at 72°C and then cooled the sample to 4°C indefinitely. PCR products were run on a 1% agarose gel and the presence of a band confirmed the presence of the symbiont.
Five aphid lines were used in the experiment, two uninfected with facultative symbionts (200 and 218) and the remainder infected singly with one of three facultative symbionts, Regiella, Fukatsuia, and Hamiltonella (Supplementary Table S1). Regiella was injected into aphid genotype 200, while the other two symbionts were injected singly into genotype 218. These five aphid lines comprise three pairwise comparisons between uninfected and infected aphids, with the uninfected line of 218 used in two comparisons. This design aimed to compare host fitness and symbiont densities within each pair across the same aphid genetic background and thus we conducted no analyses across multiple pairs. These specific isolates of symbiont were chosen because preliminary results indicated that they were likely to provide heat shock protection.
To produce these infections of Hamiltonella, Regiella, and Fukatsuia we used hemolymph injections from infected donor aphids (Supplementary Table S1). Hemolymph was extracted from donor aphids under a microscope using glass needles and re-injected into the appropriate aphid line and surviving aphids raised to adulthood. Glass needles were pulled from Kwik-Fil™ borosilicate glass capillaries (1B100-4, World Precision Instruments, 1 mm diameter) using a P-97 Flaming/Brown micropipette Puller (Sutter Instrument Co.). The offspring of the surviving aphids were tested for the successful establishment of the new infection when they were adults, and these aphid lines were retested regularly to ensure the maintenance of the new aphid-symbiont combinations. All injected lines had been maintained in the laboratory for at least a year before being used for experimental assays.
Heat Shock Protocol
This assay was designed to understand symbiont dynamics after aphids have been exposed to heat shock. Aphids were exposed to either a single peak of high temperatures or were maintained at a steady control temperature. The “heat shock” temperature chosen was 38.5°C, which was based on a series of pilot studies (data not shown). Our aim was to find a temperature that had a strong negative effect on fitness, but at which approximately half the individuals in an aphid population still survived. The aim was to explore the phenotypes of the symbionts and not to model natural situations directly. The temperature experienced by aphids near the ground is often considerably higher than meteorological records and depends, for example, on aspect and slope of the site, but it is likely that aphids are exposed to similarly high temperatures in Northern England on hot summer days (Bennie et al., 2008; Suggitt et al., 2011).
To produce age-controlled populations of each of the five lines, groups of young adults were placed into petri dishes (9 cm diameter) that contained a single broad bean (Vicia faba var. Sutton Dwarf) leaf, placed in 2% agar. V. faba is a host plant that almost all pea aphids perform on (Ferrari et al., 2008). The adults were left to reproduce for 24 h at 20°C, and the offspring were subsequently put onto 2 week old V. faba plants in groups of 50 and enclosed in a vented, transparent cage. On the following day (aphid age 24–48 h) the populations were moved into cabinets where they were either exposed to heat stress or 20°C as a control. Temperature cabinets were rigorously checked before and during the experiments to ensure even distribution of heat and the same relative humidity (50%) in both cabinets. Plants containing aphids were also placed in a randomized block pattern within the cabinet to remove any potential effects of uneven heat distribution.
While the control treatment was left at 20°C, the temperature in the heat treatment was increased from 20 to 38.5°C steadily over the course of 2 h, held at 38.5°C for 4 h, and then decreased back to 20°C over a further 2 h. Surviving aphids from both treatments were moved onto fresh 2-week-old plants on the day after heat shock to mitigate any temperature effects on the plant itself. These plants were moved into a different controlled-temperature room (20°C), where aphids from both heat treatments were kept together until being collected for analysis.
Aphids were removed to measure symbiont density at two time points. The first was 24–26 h after the start of the peak heat shock period (when the aphids were 3 days old), and the second 11 days post-heat shock (when the aphids were 14 days old, ~6 days after an aphid would usually begin reproducing). These two time points were chosen to investigate symbiont densities immediately after stress, and to test if recovery by the onset of reproduction was possible. Buchnera densities are known to decrease as aphids age (Simonet et al., 2016) and so this second time point was chosen to be the potential highest density of Buchnera during an aphid's development.
The aphids were flash frozen using dry ice and kept at −80°C until DNA extraction. In addition, one surviving apterous individual from each group was placed on a petri dish with a V. faba leaf (as above) to measure the number of offspring produced. These dishes were refreshed every 3–4 days to ensure healthy V. faba leaves. Offspring counts continued until all aphids had died, measuring total lifetime fecundity. There were 5–6 replicates for fecundity counts and symbiont density for each of the five aphid lines in each treatment (i.e., 10–12 replicates in total for each line), these were performed in two temporal blocks with approximately half the replicates of each treatment in each block.
qPCR Protocol
DNA was extracted from the aphids after samples were defrosted at room temperature. Aphids were homogenized in a 200 μl 5% Chelex solution made in distilled water. Ten microliters of proteinase K (10 mg/ml) was added per sample, and samples were incubated for 6 h at 56°C to facilitate digestion. They were then “boiled” at 100°C for 10 min before being centrifuged at 13,000 rpm for 3 min and the supernatant containing the DNA pipetted into a clean 1.5 ml Eppendorf tube which was stored at −20°C until use. Five aphids per replicate were pooled to generate a sample for the first time point (24–26 h after heat shock) and one aphid for the second time point (11 days later).
Samples were run in duplicate using SYBR® Green reagent on a StepOnePlus™ Real Time PCR machine (Applied Biosystems). Each reaction consisted of 10 μl FAST SYBR 2 × mastermix (Applied Biosystems), 1 μl forward primer (7 μM), 1 μl reverse primer (7 μM), 6 μl nuclease-free water, and 2 μl DNA sample. qPCR primers for Regiella, Fukatsuia, Hamiltonella, and the aphid housekeeping gene elongation factor-1 alpha (EF-1α) (Supplementary Table S2) were tested to ensure high efficiency and similarity between primer sets. Melt curves were performed on each plate to ensure the primers were specific to each target and only bound once. Cycling conditions were 95°C for 20 s, followed by 40 cycles of 95°C for 3 s and 60°C for 30 s. The melt curve involved a further 95°C for 15 s, 60°C for 1 min and then a gradual increase to 95°C over 15 min. Each 96-well qPCR plate was analyzed using StepOne Software v2.2.2 (Applied Biosystems) and Cycle threshold (Ct) values were obtained by comparing each primer sample to a single standard curve of known concentration and using identical threshold and baseline levels for each primer target across plates. Standard curves were created by amplifying positive control samples using PCR, calculating DNA concentrations using a High Sensitivity DNA Assay on a 2100 Bioanalyzer system (Agilent), and then serially diluting the sample 1:10 with distilled water to create a 5-sample curve comprising known concentrations decreasing from 10 pmol/ml. Samples with Ct values over 30 were classed as negative, confirmed by our negative controls. This corresponds to a copy number of <52 per 2 μl sample for all primers, and is below the threshold of detection. Where the difference in Ct values between technical replicates was >1.5, the sample was either rerun or not used in the analysis.
The standard curves were used to calculate the DNA concentration of each sample, and this was converted into copy numbers per 2 μl of DNA extract. To control for aphid size and extraction quality, copy numbers for each sample were presented relative to those of a housekeeping aphid gene as a control, giving a ratio of symbiont copy numbers to aphid copy numbers.
Statistical Analysis
Data were analyzed using the R software v. 3.4.1 (R Core Team, 2018). Since our core question was to test whether the three symbiont species can provide heat shock protection, but not to compare the extent of this protection, the data were analyzed separately for each symbiont species. Thus, in each analysis the infected line was compared with the same uninfected aphid genotype, within and across heat treatments. The data for the uninfected line 218 was therefore used twice, paired with line 218 infected with Hamiltonella or Fukatsuia. Similarly, we analyzed the two time points separately because symbiont densities change during aphid development, which would complicate the interpretation of the analysis.
Lifetime fecundity of the set of Regiella lines was analyzed using a general linear model assuming a normal error distribution. The number of offspring was the response variable, and temporal block, facultative symbiont presence, heat treatment and the interaction between symbiont presence and heat treatment were the explanatory variables. The sets of Fukatsuia and Hamiltonella lines were analyzed with a non-parametric Kruskal-Wallis test, because the model assumptions of parametric models were not met. This was followed by Wilcoxon tests to identify differences between specific treatments.
The densities of the symbionts were also analyzed with a general linear model assuming a normal error distribution. This was split into six analyses, separate for the lines relating to each symbiont species at each time point, to simplify the interpretation. For Buchnera densities, the explanatory variables were again temporal block, facultative symbiont presence and heat treatment as well as the interaction between the latter factors. For the densities of the facultative symbionts, only block and heat treatment were explanatory variables. In most cases model assumptions were met without transforming the data, only the Buchnera densities at the first time point in the Regiella lines were log-transformed. For Regiella densities at the first time point and Buchnera densities in the set of Regiella lines at the second time point, Kruskal Wallis and Wilcoxon tests were used as described for the fecundity data.
For all general linear models, post-hoc tests were only performed when the factor or interaction was significant in the main analysis. This was conducted using the R package “phia” (De Rosario-Martinez, 2015), with Holm's correction for multiple comparisons. All data are available as Supplementary Material (Data Sheets 2–4).
Results
Effects of Facultative Symbionts on Fecundity After Heat Shock
We exposed aphids to a short spike of high temperature and measured facultative and obligate symbiont densities and aphid fitness after 1 and 11 days. As expected, heat shock decreased the number of offspring produced in an aphid's lifetime in all three sets of lines [Regiella F(1, 19) = 103.23, P < 0.001; Fukatsuia: W = 109, P = 0.03, Hamiltonella: W = 136.5, P = 0.001; Figure 1]. However, the extent of this decrease was modified by the presence of Regiella and Fukatsuia [Regiella, symbiont × heat treatment: F(1, 19) = 5.78, P = 0.03; Fukatsuia: heat treatment in uninfected lines W = 36, P = 0.004 and in infected lines W = 13, P = 0.48]. Fukatsuia provided the greatest protection from heat as there was no difference in the fecundity of the infected lines in the control and heat shock treatment, whereas there was a greater reduction in fecundity in the uninfected aphids than in the infected aphids for the Regiella lines. In contrast, there was a similar decrease in fecundity for both uninfected and infected Hamiltonella lines following heat shock (uninfected: W = 36, P = 0.004; infected: W = 34.5, P = 0.008; Figure 1). At benign temperatures, two of the symbionts also affected lifetime fecundity: the presence of Hamiltonella increased lifetime fecundity (W = 4, P = 0.03), whereas Fukatsuia decreased it (W = 34, P = 0.013), and there was no difference for Regiella (Figure 1).
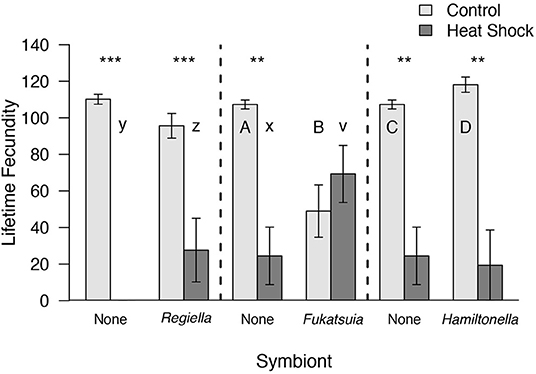
Figure 1. The effect of heat shock and facultative symbiont presence on the number of offspring produced by pea aphids. Aphids from genotype 200 that were uninfected or carrying Regiella are compared in the first panel. Aphids from genotype 218 that were uninfected or carrying Fukatsuia or Hamiltonella are compared separately in the second and third panels, respectively. In all comparisons, there was a significant effect of heat shock compared to controls, but no overall effect of symbiont presence. Means and standard errors are shown. Within each panel separately, upper case letters denote significant differences between aphids with different symbionts within the control treatment and lower case letters denote significant differences in the heat shock treatment. The asterisks show significant differences between heat treatments for aphids of the same symbiont status (**P < 0.01, ***P < 0.001).
Facultative Symbiont Densities After Heat Shock
We measured the densities of the three facultative symbionts at two time points after exposure to heat, 24–26 h and 11 days post-heat shock (Figure 2). Compared to non-heat shocked controls the densities of two of the symbionts, Fukatsuia and Hamiltonella, were lower on the day after heat shock [Fukatsuia: F(1, 8) = 65.05, P < 0.001, Hamiltonella: F(1, 8) = 6.64, P = 0.03], whereas densities of Regiella are unaffected (W = 28, p = 0.13; but note that this is significant in a less conservative parametric test). By the second time point, taken when the aphids were young adults, there was no difference between population densities in heat stressed or control aphids for any of the three facultative symbionts [Fukatsuia: F(1, 8) = 0.35, P = 0.57, Hamiltonella: F(1, 7) = 0.95, P = 0.36, Regiella: F(1, 6) = 0.01, P = 0.91; Figure 2], suggesting that heat did not have long-term effects on facultative symbiont populations.
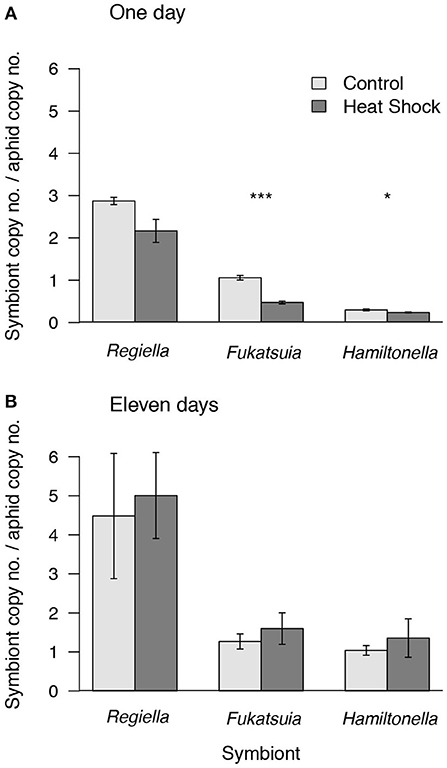
Figure 2. Densities of facultative symbionts in pea aphids after heat shock or in the control treatment (A) 24–26 h after the onset of the heat shock and (B) 11 days after heat shock. Densities are shown as the copy number of the gyrB gene of the facultative symbiont relative to copy number of the aphid gene EF1-α. Means and standard errors are shown. Asterisks denote differences between heat treatments for aphids carrying a given symbiont (*P < 0.05, ***P < 0.001).
Obligate Symbiont Densities Under Heat Shock
Compared to the control treatment densities of Buchnera were decreased on the day after heat shock in each of the three pairs of lines, regardless of facultative symbiont infection [Regiella lines: F(1, 19) = 80.36, P < 0.001, Fukatsuia lines: F(1, 18) = 70.71, P < 0.001, Hamiltonella lines: F(1, 18) = 56.07, P < 0.001; Figure 3]. Regardless of treatment, Buchnera densities were higher in the lines harboring Fukatsuia [F(1, 18) = 33.86, P < 0.001] or Hamiltonella [F(1, 18) = 24.52, P < 0.001] compared to uninfected lines, an effect that was not seen in the Regiella lines [F(1, 19) = 0.15, P = 0.71]. For the Fukatsuia and Hamiltonella lines there was also a significant interaction between symbiont presence and heat treatment [Fukatsuia: F(1, 18) = 9.57, P = 0.006; Hamiltonella: F(1, 18) = 4.80, P = 0.04]: in both cases, Buchnera densities in the control treatment were higher in lines with facultative symbionts compared to uninfected aphids but there was no difference between these lines after heat shock. The interaction was not significant for the Regiella lines [F(1, 19) = 2.58, P = 0.13] where the extent of the loss of Buchnera did not differ between infected and uninfected lines.
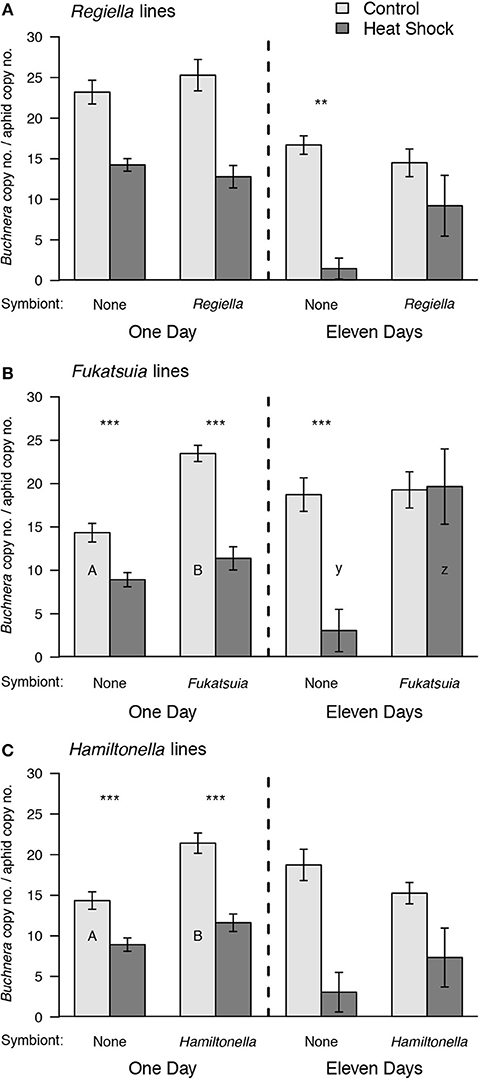
Figure 3. The effect of heat shock and facultative symbiont presence on densities of the primary symbiont Buchnera in pea aphids. In each panel Buchnera densities are shown 1 or 11 days after heat shock. Uninfected aphids are compared to aphids carrying (A) Regiella, (B) Fukatsuia, and (C) Hamiltonella. The uninfected replicates are the same for the Fukatsuia and Hamiltonella lines. Means and standard errors are shown. In all cases, there was a significant overall difference between the heat shock and control treatments. There was also a significant overall effect of symbiont presence for the Fukatsuia lines at both time points, and Hamiltonella lines 1 day after heat shock. These main effects are not illustrated on the figure. post-hoc results from significant interactions are denoted by upper case letters for significant differences between aphids with different symbionts within the control treatment, and lower-case letters denote significant differences in the heat shock treatment. The asterisks show significant post-hoc differences between heat treatments for aphids of the same symbiont status (**P < 0.01, ***P < 0.001).
At the later time point, when aphids were young adults, heat shock again reduced Buchnera densities on average [Regiella lines: W = 105, P = 0.004; Fukatsuia lines: F(1, 19) = 6.85, P = 0.02; Hamiltonella lines: F(1, 17) = 24.84, P < 0.001]. Fukatsuia presence on average also significantly increased the density of Buchnera regardless of treatment which was due to high Buchnera densities in the heat shocked aphids [F(1, 19) = 7.89, P = 0.01]; a difference that was not found for Regiella (W = 46, P = 0.37) or Hamiltonella presence [F(1, 17) = 0.17, P = 0.69]. Importantly, there was a significant interaction between symbiont infection and temperature in the Fukatsuia lines [F(1, 19) = 7.55, P = 0.01] and an equivalent effect in the Regiella lines (heat treatment in uninfected lines: W = 36, P = 0.004 and in infected lines: W = 17, P = 0.42) that was not seen for the Hamiltonella lines [F(1, 17) = 1.95, P = 0.18]: Buchnera densities after heat shock were significantly reduced in uninfected aphids but not when Fukatsuia or Regiella were present.
Discussion
Our results show that different aphid symbionts can protect the aphid from heat and help the obligate symbiont to recover after heat shock. Infection with Regiella and Fukatsuia was closely linked to Buchnera recovery after heat shock and led to increased production of offspring compared to uninfected controls whereas there was no such protection in aphids infected with Hamiltonella. This pattern differs from other studies (Russell and Moran, 2006; Doremus and Oliver, 2017), which found that Hamiltonella but not Regiella or Fukatsuia provided heat protection. As different lines of insects and symbionts were used in these studies, it is likely that these protective effects are dependent on symbiont, host genotype or their interaction and are thus not a universal feature of symbiont infection. The prevalence of heat protection may be overestimated here since we chose genotypes based on preliminary results.
Buchnera densities are closely linked to aphid fitness. Disrupting the obligate symbiosis by removing Buchnera leads to large reductions in offspring production and often host death (Koga et al., 2003; Akman Gündüz and Douglas, 2009) Overly high densities of Buchnera can also lead to a reduction in fitness (Chong and Moran, 2016), meaning that the relationship between density of the symbiont and number of offspring produced is not directly proportional. However, removal of Buchnera, via antibiotics or heat, as also shown in our results, generally leads to aphid sterility in the absence of facultative symbionts (Dunbar et al., 2007; Koga et al., 2007).
A key question that we addressed was whether the facultative symbionts protect Buchnera from the effects of heat, and if so whether Buchnera is directly protected or its recovery facilitated. The densities of both Buchnera and the facultative symbionts were reduced 24 h after heat shock. In aphids carrying Fukatsuia or Regiella, these densities returned to levels that were similar to those in non-heat shocked controls, thus demonstrating a clear role of the facultative symbionts in the recovery of Buchnera. The most parsimonious interpretation of the observed pattern is that the facultative symbionts do not provide immediate protection, although it is possible that the decline in Buchnera DNA density occurs due to different processes in aphids with and without facultative symbionts. It is conceivable that Buchnera is only truly killed by heat inside the latter and growth is merely arrested in the former and thus some immediate protection occurs.
The patterns of obligate and facultative symbiont densities observed here suggest that a different mechanism underlies the heat protection provided by Regiella and Fukatsuia compared to that provided by Serratia (Montllor et al., 2002; Burke G. et al., 2010). After heat shock, Serratia in pea aphids lyse and this coincides with metabolomic changes (Burke G. et al., 2010). At the same time Buchnera densities are maintained at similar levels to those at benign temperatures (Burke G. et al., 2010). In our experiments, in aphids with Regiella or Fukatsuia, Buchnera densities initially decrease. This demonstrates that the protection provided by the facultative symbionts is not instant and suggests that the protection is probably not due to a constitutively activated aphid stress response, but it is still possible that the facultative symbionts prime a stress response by the aphid that helps recovery later on.
The provision of heat-protective compounds, through either lysis or release from a live cell, is also consistent with our observations. Burke G. et al. (2010) explored which metabolites are affected by heat treatment when Serratia lyse. They found three metabolic changes linked to the presence of the protective Serratia after heat stress, one of which is a decrease in concentration of the antioxidant indole-3-lactate, as well as two other unidentified metabolites (Burke G. et al., 2010). The initial decline of Fukatsuia in our study suggest that lysis is likely in this symbiont (and possibly in Regiella where the decline is significant using less conservative statistics). However, the protective compounds released during this process appear to act later than in Serratia (Burke G. et al., 2010) as Buchnera also declines initially. In some cases, facultative symbionts can replace the function of an obligate symbiont (Koga et al., 2003, 2007) and this could be a way of protecting the host from the effects of heat. In our system, both Buchnera and the facultative symbionts recover demonstrating that this functional replacement is not a likely mechanism here.
Some strains of Buchnera are more resistant to heat than others (Dunbar et al., 2007; Moran and Yun, 2015) and it is possible that the two aphid genotypes here carry different Buchnera genotypes. Aphids in laboratory populations often carry Buchnera strains with the ibpA12 mutant allele that are more sensitive to heat but have higher fitness (Burke, G. R. et al., 2010). We did not sequence ibpA in the genotypes used here since we were interested in the effects of facultative symbionts and the Buchnera strain is the same within all our comparisons. It is worth noting that the Fukatsuia and Hamiltonella lines both had the same aphid and Buchnera genotype but only Fukatsuia protected, suggesting that the Buchnera strain does not bias our conclusions. However, in natural populations the absence of facultative symbiont infections is correlated with a higher incidence of this mutation (Burke, G. R. et al., 2010). Aphids thus have two mechanisms which protect Buchnera from heat: the absence of the heat-sensitive ibpA12 mutant allele and the presence of protective facultative symbionts. Facultative symbionts appear to confer low fitness in the presence of ibpA12 and are thus likely selected against in aphids with this mutation (Burke, G. R. et al., 2010). It thus suggests that in natural populations facultative symbionts may only be able to rescue the aphid Buchnera from heat in aphids that carry the heat-tolerant ibpA13 allele and this may explain the scarcity of ibpA12 in natural populations.
As well as the protective effect of Fukatsuia and Regiella, we observed interesting changes in symbiont densities at benign temperatures. Fukatsuia decreased fecundity, as shown previously (Heyworth and Ferrari, 2015; Doremus and Oliver, 2017); the densities of Buchnera confirm that this is not due to suppression of the obligate symbiont (Koga et al., 2003), which has been observed for a costly infection by Rickettsia in pea aphids (Sakurai et al., 2005). Surprisingly, infection with Fukatsuia or Hamiltonella leads to an increase of Buchnera population levels in younger aphids. This may benefit both the aphid and Buchnera when facultative symbionts are present, because additional nutrients may be required. It is possible that either the aphid upregulates Buchnera densities or that this strain of Buchnera responds to the presence of facultative symbionts by increasing its growth rate. In either case, the density of Buchnera cells in infected aphids is comparable to uninfected aphids once the aphids are adult.
The ability of facultative symbionts to protect obligate nutritional symbionts from heat stress has implications for the frequencies and spread of both the microbes and the insects themselves. Many phytophagous insects rely on obligate symbionts to provide essential nutrition, but these are often vulnerable to ecologically stressful situations (Bennett and Moran, 2015; Kikuchi et al., 2016) due to severe genome reduction during coevolution with their hosts (McCutcheon and Moran, 2011). This genome reduction probably led to the heat sensitivity that some facultative symbionts ameliorate, an example of a symbiosis rescuing another symbiosis. It seems improbable that this rescue resulted from close coevolution due to the relatively transient nature of facultative symbiosis infections (Smith et al., 2015).
As we and others (Montllor et al., 2002; Burke G. et al., 2010) have shown, carrying certain isolates of facultative symbionts can protect obligate symbionts from a single, short exposure to heat, but it remains to be investigated whether this protection is also effective under long-term or regular exposure to extreme temperatures. The temperature-dependent fitness effects are likely to alter the frequencies of facultative symbionts in natural populations, but will do so in concert with other abiotic and biotic factors, including the frequencies of heat-tolerant Buchnera strains. The symbionts' ability to affect interactions between host and natural enemies is also well-documented (Hrček et al., 2016). These interactions can be affected by a change in temperature, through an effect of temperature on the natural enemy itself (Roux et al., 2010; Nguyen et al., 2013) or on the interaction between host, symbiont and natural enemy (Guay et al., 2009; Jeffs and Lewis, 2013; Heyworth and Ferrari, 2016). In addition, both vertical and horizontal transmission frequencies of symbionts can be affected by temperature (Anbutsu et al., 2008; Osaka et al., 2008; Liu et al., 2019). A combination of temperature-dependent fitness effects and transmission dynamics is therefore a likely reason for the lack of a clear correlation between symbiont mediated benefits seen in laboratory experiments and symbiont frequencies observed in the field (Oliver et al., 2014), and probably contributes to the geographic variation in the composition of facultative symbiont communities (Montllor et al., 2002; Tsuchida et al., 2002; Sepúlveda et al., 2017). There are, however, examples where the patterns based on laboratory experiments are observed: the frequencies of the aphid heat-protective symbiont Serratia are high in host populations in the warmer climes of Southern USA (Chen and Purcell, 1997; Montllor et al., 2002) and more generally in arid compared to temperate regions (Henry et al., 2013). Similarly, land temperature correlates with symbiont prevalence in midges (Morag et al., 2012).
Facultative symbionts alter insect fitness under stressful conditions and can affect not just the host, but also species that the host interacts with directly and indirectly (McLean and Godfray, 2016; Doremus et al., 2018). In extreme cases, hosting a defensive symbiont can lead to cascading extinctions and the collapse of entire communities (Sanders et al., 2016). Our work highlights how the host and its symbiont community is affected by temperature and that this temperature-dependency might result in changes of community interactions under climate change.
Data Availability Statement
All datasets generated for this study are included in the article/Supplementary Material.
Author Contributions
EH, MS, and JF conceived the ideas and designed methodology, collected the data, wrote the manuscript, and gave final approval for publication. EH and JF analyzed the data.
Funding
EH was supported by a studentship from the Biotechnology and Biological Sciences Research Council (BBSRC, award BB/F016751/1) and JF and MS by award BB/J00524X/1 from BBSRC.
Conflict of Interest
The authors declare that the research was conducted in the absence of any commercial or financial relationships that could be construed as a potential conflict of interest.
Acknowledgments
We would like to thank Alison Fenwick, Chris Lancaster, and Paul Scott for setting up and monitoring the cabinets for the heat shock treatment, Sally James in the Technology Facility at the University of York for support with the qPCR, and Sally Raines for helping with aphid culture and helpful discussions.
Supplementary Material
The Supplementary Material for this article can be found online at: https://www.frontiersin.org/articles/10.3389/fevo.2020.00056/full#supplementary-material
Data Sheet 2. Densities of the obligate symbiont Buchnera aphidicola in the two heat treatments in the presence and absence of facultative symbionts.
Data Sheet 3. Densities of the facultative symbionts in the two heat treatments.
Data Sheet 4. Lifetime fecundity of pea aphids in the two heat treatments in the presence and absence of facultative symbionts.
References
Akman Gündüz, E., and Douglas, A. E. (2009). Symbiotic bacteria enable insect to use a nutritionally inadequate diet. Proc. Biol. Sci. 276, 987–991. doi: 10.1098/rspb.2008.1476
Anbutsu, H., Goto, S., and Fukatsu, T. (2008). High and low temperatures differently affect infection density and vertical transmission of male-killing Spiroplasma symbionts in Drosophila hosts. Appl. Env. Microbiol. 74, 6053–6059. doi: 10.1128/AEM.01503-08
Bennett, G. M., and Moran, N. A. (2015). Heritable symbiosis: the advantages and perils of an evolutionary rabbit hole. Proc. Natl. Acad. Sci. U.S.A. 112, 10169–10176. doi: 10.1073/pnas.1421388112
Bennie, J., Huntley, B., Wiltshire, A., Hill, M. O., and Baxter, R. (2008). Slope, aspect and climate: spatially explicit and implicit models of topographic microclimate in chalk grassland. Ecol. Model. 216, 47–59. doi: 10.1016/j.ecolmodel.2008.04.010
Bordenstein, S. R., and Bordenstein, S. R. (2011). Temperature affects the tripartite interactions between bacteriophage WO, Wolbachia, and cytoplasmic incompatibility. PLoS ONE 6:e29106. doi: 10.1371/journal.pone.0029106
Brownlie, J. C., and Johnson, K. N. (2009). Symbiont-mediated protection in insect hosts. Trends Microbiol. 17, 348–354. doi: 10.1016/j.tim.2009.05.005
Brumin, M., Kontsedalov, S., and Ghanim, M. (2011). Rickettsia influences thermotolerance in the whitefly Bemisia tabaci B biotype. Insect Sci. 18, 57–66. doi: 10.1111/j.1744-7917.2010.01396.x
Burke, G., Fiehn, O., and Moran, N. (2010). Effects of facultative symbionts and heat stress on the metabolome of pea aphids. ISME J. 4, 242–252. doi: 10.1038/ismej.2009.114
Burke, G. R., McLaughlin, H. J., Simon, J. C., and Moran, N. A. (2010). Dynamics of a recurrent Buchnera mutation that affects thermal tolerance of pea aphid hosts. Genetics 186, 367–372. doi: 10.1534/genetics.110.117440
Carruthers, M. D., and Minion, C. (2009). Transcriptome analysis of Escherichia coli O157:H7 EDL933 during heat shock. FEMS Microbiol. Lett. 295, 96–102. doi: 10.1111/j.1574-6968.2009.01587.x
Chen, C. Y., Lai, C. Y., and Kuo, M. H. (2009). Temperature effect on the growth of Buchnera endosymbiont in Aphis craccivora (Hemiptera: Aphididae). Symbiosis 49, 53–59. doi: 10.1007/s13199-009-0011-4
Chen, D. Q., and Purcell, A. H. (1997). Occurrence and transmission of facultative endosymbionts in aphids. Curr. Microbiol. 34, 220–225. doi: 10.1007/s002849900172
Chong, R. A., and Moran, N. A. (2016). Intraspecific genetic variation in hosts affects regulation of obligate heritable symbionts. Proc. Natl. Acad. Sci. U.S.A. 113, 13114–13119. doi: 10.1073/pnas.1610749113
Corbin, C., Heyworth, E. R., Ferrari, J., and Hurst, G. D. D. (2017). Heritable symbionts in a world of varying temperature. Heredity 118, 10–20. doi: 10.1038/hdy.2016.71
De Rosario-Martinez, H. (2015). phia: post-hoc interaction analysis. R package version 0.2-1. Available online at: https://CRAN.R-project.org/package=phia
Dion, E., Polin, S. E., Simon, J. C., and Outreman, Y. (2011). Symbiont infection affects aphid defensive behaviours. Biol. Lett. 7, 743–746. doi: 10.1098/rsbl.2011.0249
Dixon, A. F. G., Kindlmann, P., Leps, J., and Holman, J. (1987). Why there are so few species of aphids, especially in the tropics. Am. Nat. 129, 580–592. doi: 10.1086/284659
Doremus, M. R., and Oliver, K. M. (2017). Aphid heritable symbiont exploits defensive mutualism. Appl. Environ. Microbiol. 83:e03276-16. doi: 10.1128/AEM.03276-16
Doremus, M. R., Smith, A. H., Kim, K. L., Holder, A. J., Russell, J. A., and Oliver, K. M. (2018). Breakdown of a defensive symbiosis, but not endogenous defences, at elevated temperatures. Mol. Ecol. 27, 2138–2151. doi: 10.1111/mec.14399
Douglas, A. E. (1998). Nutritional interactions in insect-microbial symbioses: aphids and their symbiotic bacteria Buchnera. Annu. Rev. Entomol. 43, 17–37. doi: 10.1146/annurev.ento.43.1.17
Dunbar, H. E., Wilson, A. C. C., Ferguson, N. R., and Moran, N. A. (2007). Aphid thermal tolerance is governed by a point mutation in bacterial symbionts. PLoS Biol. 5:e0050096. doi: 10.1371/journal.pbio.0050096
Ferrari, J., Via, S., and Godfray, H. C. J. (2008). Population differentiation and genetic variation in performance on eight hosts in the pea aphid complex. Evolution 62, 2508–2524. doi: 10.1111/j.1558-5646.2008.00468.x
Ferrari, J., West, J. A., Via, S., and Godfray, H. C. J. (2012). Population genetic structure and secondary symbionts in host-associated populations of the pea aphid complex. Evolution 66, 375–390. doi: 10.1111/j.1558-5646.2011.01436.x
Gerardo, N. M., and Parker, B. J. (2014). Mechanisms of symbiont-conferred protection against natural enemies: an ecological and evolutionary framework. Curr. Opin. Insect Sci. 4, 8–14. doi: 10.1016/j.cois.2014.08.002
Gómez-Valero, L., Silva, F. J., Simon, J. C., and Latorre, A. (2007). Genome reduction of the aphid endosymbiont Buchnera aphidicola in a recent evolutionary time scale. Gene 389, 87–95. doi: 10.1016/j.gene.2006.10.001
Guay, J. F., Boudreault, S., Michaud, D., and Cloutier, C. (2009). Impact of environmental stress on aphid clonal resistance to parasitoids: role of Hamiltonella defensa bacterial symbiosis in association with a new facultative symbiont of the pea aphid. J. Insect Physiol. 55, 919–926. doi: 10.1016/j.jinsphys.2009.06.006
Harrington, R., Woiwod, I., and Sparks, T. (1999). Climate change and trophic interactions. Trends Ecol. Evol. 14, 146–150. doi: 10.1016/S0169-5347(99)01604-3
Henry, L. M., Peccoud, J., Simon, J. C., Hadfield, J. D., Maiden, M. J. C., Ferrari, J., et al. (2013). Horizontally transmitted symbionts and host colonization of ecological niches. Curr. Biol. 23, 1713–1717. doi: 10.1016/j.cub.2013.07.029
Heyworth, E. R., and Ferrari, J. (2015). A facultative endosymbiont in aphids can provide diverse ecological benefits. J. Evol. Biol. 28, 1753–1760. doi: 10.1111/jeb.12705
Heyworth, E. R., and Ferrari, J. (2016). Heat stress affects facultative symbiont-mediated protection from a parasitoid wasp. PLoS ONE 11:e0167180. doi: 10.1371/journal.pone.0167180
Hrček, J., McLean, A. H. C., and Godfray, H. C. J. (2016). Symbionts modify interactions between insects and natural enemies in the field. J. Anim. Ecol. 1605–1612. doi: 10.1111/1365-2656.12586
Jeffs, C. T., and Lewis, O. T. (2013). Effects of climate warming on host–parasitoid interactions. Ecol. Entomol. 38, 209–218. doi: 10.1111/een.12026
Kikuchi, Y., Tada, A., Musolin, D. L., Hari, N., Hosokawa, T., Fujisaki, K., et al. (2016). Collapse of insect gut symbiosis under simulated climate change. mBio 7:16. doi: 10.1128/mBio.01578-16
Kim, J. K., Lee, J. B., Huh, Y. R., Jang, H. A., Kim, C. H., Yoo, J. W., et al. (2015). Burkholderia gut symbionts enhance the innate immunity of host Riptortus pedestris. Dev. Comp. Immunol. 53, 265–269. doi: 10.1016/j.dci.2015.07.006
Koga, R., Tsuchida, T., and Fukatsu, T. (2003). Changing partners in an obligate symbiosis: a facultative endosymbiont can compensate for loss of the essential endosymbiont Buchnera in an aphid. Proc. Biol. Sci. 270, 2543–2550. doi: 10.1098/rspb.2003.2537
Koga, R., Tsuchida, T., Sakurai, M., and Fukatsu, T. (2007). Selective elimination of aphid endosymbionts: effects of antibiotic dose and host genotype, and fitness consequences. FEMS Microbiol. Ecol. 60, 229–239. doi: 10.1111/j.1574-6941.2007.00284.x
Kwong, W. K., Mancenido, A. L., and Moran, N. A. (2017). Immune system stimulation by the native gut microbiota of honey bees. R. Soc. Open Sci. 4:170003. doi: 10.1098/rsos.170003
Laughton, A. M., Fan, M. H., and Gerardo, N. M. (2013). The combined effects of bacterial symbionts and ageing on life history traits in the pea aphid Acyrthosiphon pisum. Appl. Environ. Microbiol. 80, 470–477. doi: 10.1128/AEM.02657-13
Liu, X. D., Lei, H. X., and Chen, F. F. (2019). Infection pattern and negative effects of a facultative endosymbiont on its insect host are environment-dependent. Sci. Rep. 9:4013. doi: 10.1038/s41598-019-40607-5
Maire, J., Vincent-Monégat, C., Masson, F., Zaidman-Rémy, A., and Heddi, A. (2018). An IMD-like pathway mediates both endosymbiont control and host immunity in the cereal weevil Sitophilus spp. Microbiome 6:6. doi: 10.1186/s40168-017-0397-9
Martinez, J., Ok, S., Smith, S., Snoeck, K., Day, J. P., and Jiggins, F. M. (2015). Should symbionts be nice or selfish? antiviral effects of Wolbachia are costly but reproductive parasitism is not. PLoS Pathog. 11:e1005021. doi: 10.1371/journal.ppat.1005021
McCutcheon, J. P., and Moran, N. A. (2011). Extreme genome reduction in symbiotic bacteria. Nat. Rev. Microbiol. 10, 13–26. doi: 10.1038/nrmicro2670
McLean, A., Van Asch, M., Ferrari, J., and Godfray, H. C. J. (2011). Effects of bacterial secondary symbionts on host plant use in pea aphids. Proc. Biol. Sci. 278, 760–766. doi: 10.1098/rspb.2010.1654
McLean, A. H. C., and Godfray, H. C. J. (2016). The outcome of competition between two parasitoid species is influenced by a facultative symbiont of their aphid host. Funct. Ecol. 31, 927–933. doi: 10.1111/1365-2435.12781
Montllor, C. B., Maxmen, A., and Purcell, A. H. (2002). Facultative bacterial endosymbionts benefit pea aphids Acyrthosiphon pisum under heat stress. Ecol. Entomol. 27, 189–195. doi: 10.1046/j.1365-2311.2002.00393.x
Morag, N., Klement, E., Saroya, Y., Lensky, I., and Gottlieb, Y. (2012). Prevalence of the symbiont Cardinium in Culicoides (Diptera: Ceratopogonidae) vector species is associated with land surface temperature. FASEB J. 26, 4025–4034. doi: 10.1096/fj.12-210419
Moran, N. A. (1996). Accelerated evolution and Muller's ratchet in endosymbiotic bacteria. Proc. Natl. Acad. Sci. U.S.A. 93, 2873–2878. doi: 10.1073/pnas.93.7.2873
Moran, N. A., and Yun, Y. (2015). Experimental replacement of an obligate insect symbiont. Proc. Natl. Acad. Sci. U.S.A. 112, 2093–2096. doi: 10.1073/pnas.1420037112
Neelakanta, G., Sultana, H., Fish, D., Anderson, J. F., and Fikrig, E. (2010). Anaplasma phagocytophilum induces Ixodes scapularis ticks to express an antifreeze glycoprotein gene that enhances their survival in the cold. J. Clin. Invest. 120, 3179–3190. doi: 10.1172/JCI42868
Nguyen, T. M., Bressac, C., and Chevrier, C. (2013). Heat stress affects male reproduction in a parasitoid wasp. J. Insect Physiol. 59, 248–254. doi: 10.1016/j.jinsphys.2012.12.001
Oliver, K. M., Moran, N. A., and Hunter, M. S. (2005). Variation in resistance to parasitism in aphids is due to symbionts not host genotype. Proc. Natl. Acad. Sci. U.S.A. 102, 12795–12800. doi: 10.1073/pnas.0506131102
Oliver, K. M., Smith, A. H., and Russell, J. A. (2014). Defensive symbiosis in the real world – advancing ecological studies of heritable, protective bacteria in aphids and beyond. Funct. Ecol. 28, 341–355. doi: 10.1111/1365-2435.12133
Osaka, R., Nomura, M., Watada, M., and Kageyama, D. (2008). Negative effects of low temperatures on the vertical transmission and infection density of a Spiroplasma endosymbiont in Drosophila hydei. Curr. Microbiol. 57, 335–339. doi: 10.1007/s00284-008-9199-4
Parmesan, C., and Yohe, G. (2003). A globally coherent fingerprint of climate change impacts across natural systems. Nature 421, 37–42. doi: 10.1038/nature01286
R Core Team (2018). R: A Language and Environment for Statistical Computing. Vienna: R Foundation for Statistical Computing. Available online at: http://www.R-project.org/ (accessed April 9, 2019).
Roux, O., Le Lann, C., van Alphen, J. J. M., and van Baaren, J. (2010). How does heat shock affect the life history traits of adults and progeny of the aphid parasitoid Aphidius avenae (Hymenoptera: Aphidiidae)? Bull. Entomol. Res. 100, 543–549. doi: 10.1017/S0007485309990575
Russell, J. A., and Moran, N. A. (2006). Costs and benefits of symbiont infection in aphids: variation among symbionts and across temperatures. Proc. Biol. Sci. 273, 603–610. doi: 10.1098/rspb.2005.3348
Sakurai, M., Koga, R., Tsuchida, T., Meng, X. Y., and Fukatsu, T. (2005). Rickettsia symbiont in the pea aphid Acyrthosiphon pisum: novel cellular tropism, effect on host fitness, and interaction with the essential symbiont Buchnera. Appl. Environ. Microbiol. 71, 4069–4075. doi: 10.1128/AEM.71.7.4069-4075.2005
Sanders, D., Kehoe, R., van Veen, F. J. F., McLean, A., Godfray, H. C. J., Dicke, M., et al. (2016). Defensive insect symbiont leads to cascading extinctions and community collapse. Ecol. Lett. 19, 789–799. doi: 10.1111/ele.12616
Schmitz, A., Anselme, C., Ravallec, M., Rebuf, C., Simon, J. C., Gatti, J. L., et al. (2012). The cellular immune response of the pea aphid to foreign intrusion and symbiotic challenge. PLoS ONE 7:e42114. doi: 10.1371/journal.pone.0042114
Schmitz, O. J., and Barton, B. T. (2014). Climate change effects on behavioral and physiological ecology of predator–prey interactions: implications for conservation biological control. Biol. Control 75, 87–96. doi: 10.1016/j.biocontrol.2013.10.001
Sepúlveda, D. A., Zepeda-Paulo, F., Ramírez, C. C., Lavandero, B., and Figueroa, C. C. (2017). Diversity, frequency, and geographic distribution of facultative bacterial endosymbionts in introduced aphid pests. Insect Sci. 24, 511–521. doi: 10.1111/1744-7917.12313
Shan, H. W., Deng, W. H., Luan, J. B., Zhang, M. J., Zhang, Z., Liu, S. S., et al. (2017). Thermal sensitivity of bacteriocytes constrains the persistence of intracellular bacteria in whitefly symbiosis under heat stress. Environ. Microbiol. Rep. 9, 706–716. doi: 10.1111/1758-2229.12554
Simonet, P., Duport, G., Gaget, K., Weiss-Gayet, M., Colella, S., Febvay, G., et al. (2016). Direct flow cytometry measurements reveal a fine-tuning of symbiotic cell dynamics according to the host developmental needs in aphid symbiosis. Sci. Rep. 6:19967. doi: 10.1038/srep19967
Smith, A. H., Łukasik, P., O'Connor, M. P., Lee, A., Mayo, G., Drott, M. T., et al. (2015). Patterns, causes and consequences of defensive microbiome dynamics across multiple scales. Mol. Ecol. 24, 1135–1149. doi: 10.1111/mec.13095
Suggitt, A. J., Gillingham, P. K., Hill, J. K., Huntley, B., Kunin, W. E., Roy, D. B., et al. (2011). Habitat microclimates drive fine-scale variation in extreme temperatures. Oikos 120, 1–8. doi: 10.1111/j.1600-0706.2010.18270.x
Tsuchida, T., Koga, R., Horikawa, M., Tsunoda, T., Maoka, T., Matsumoto, S., et al. (2010). Symbiotic bacterium modifies aphid body color. Science 330, 1102–1104. doi: 10.1126/science.1195463
Tsuchida, T., Koga, R., Shibao, H., Matsumoto, T., and Fukatsu, T. (2002). Diversity and geographic distribution of secondary endosymbiotic bacteria in natural populations of the pea aphid, Acyrthosiphon pisum. Mol. Ecol. 11, 2123–2135. doi: 10.1046/j.1365-294X.2002.01606.x
Vorburger, C. (2014). The evolutionary ecology of symbiont-conferred resistance to parasitoids in aphids. Insect Sci. 21, 251–264. doi: 10.1111/1744-7917.12067
Walther, G. R., Post, E., Convey, P., Menzel, A., Parmesan, C., Beebee, T., et al. (2002). Ecological responses to recent climate change. Nature 416, 389–395. doi: 10.1038/416389a
Weiss, B. L., Maltz, M., and Aksoy, S. (2012). Obligate symbionts activate immune system development in the tsetse fly. J. Immunol. 188, 3395–3403. doi: 10.4049/jimmunol.1103691
Wilcox, J. L., Dunbar, H. E., Wolfinger, R. D., and Moran, N. A. (2003). Consequences of reductive evolution for gene expression in an obligate endosymbiont. Mol. Microbiol. 48, 1491–1500. doi: 10.1046/j.1365-2958.2003.03522.x
Young, D., Roman, E., Moreno, C., O'Brien, R., Born, W., Welch, W. J., et al. (1993). Molecular chaperones and the immune response. Philos. Trans. R. Soc. Lond. B. Biol. Sci. 339, 363–368. doi: 10.1098/rstb.1993.0035
Keywords: Acyrthosiphon pisum, Buchnera aphidicola, facultative symbiont, heat stress, insect symbionts, quantitative PCR, symbiosis
Citation: Heyworth ER, Smee MR and Ferrari J (2020) Aphid Facultative Symbionts Aid Recovery of Their Obligate Symbiont and Their Host After Heat Stress. Front. Ecol. Evol. 8:56. doi: 10.3389/fevo.2020.00056
Received: 18 October 2019; Accepted: 26 February 2020;
Published: 19 March 2020.
Edited by:
Cesar A. Cardenas, Instituto Antártico Chileno (INACH), ChileReviewed by:
Hugo Mathé-Hubert, Université de Lorraine, FranceAlison Karley, The James Hutton Institute, United Kingdom
Christoph Vorburger, Swiss Federal Institute of Aquatic Science and Technology, Switzerland
Copyright © 2020 Heyworth, Smee and Ferrari. This is an open-access article distributed under the terms of the Creative Commons Attribution License (CC BY). The use, distribution or reproduction in other forums is permitted, provided the original author(s) and the copyright owner(s) are credited and that the original publication in this journal is cited, in accordance with accepted academic practice. No use, distribution or reproduction is permitted which does not comply with these terms.
*Correspondence: Julia Ferrari, anVsaWEuZmVycmFyaUB5b3JrLmFjLnVr
†These authors have contributed equally to this work
‡Present address: Melanie R. Smee, Microbiology Department, Cornell University, Ithaca, NY, United States