- Department of Systematic and Evolutionary Botany, University of Zurich, Zurich, Switzerland
Despite the paramount role of floral fragrance in pollinator attraction and reproduction in flowering plants, we know little about its evolution under natural conditions. Here we show that by reducing herbivore load with pesticide application, plants showed evolutionary changes in their floral fragrance within 4 generations when compared to plants with natural levels of herbivory. We interpret this finding in the context of relaxed physiological and ecological trade-offs with fewer herbivores, potentially facilitating selection by bees on specific aromatic volatiles. Our study confirms earlier findings of experimental evolution under highly controlled and simplified conditions in the greenhouse.
Introduction
Flowering plants have evolved an extraordinary diversity of flower colors, shapes, sizes, and fragrances that attract animal pollinators which play a key role in plants’ sexual reproduction (Harder and Johnson, 2009; Schiestl and Johnson, 2013). Among these multiple floral signals, floral fragrance stands out not only because of its chemical complexity and variation, but also for the little we know about the ecological factors affecting its evolution (Raguso, 2008; Schiestl, 2010). Nevertheless, we now know that floral fragrance fulfills the basic requirements for evolution driven by natural selection, specifically, (i) floral volatiles are heritable (Zu et al., 2016), (ii) pollinators can impose selection on some of these volatile compounds (Schiestl et al., 2011; Parachnowitsch et al., 2012; Gross et al., 2016; Knauer and Schiestl, 2017), and (iii) pollinator-mediated selection can result in the evolution of stronger floral fragrances within few generations (Gervasi and Schiestl, 2017; Ramos and Schiestl, 2019).
The evolution of floral fragrance and other signals have been traditionally attributed to the preferences and behavior of animal pollinators (Schiestl and Johnson, 2013). However, in nature, plants also interact with a diverse community of specialist and generalist herbivores that may as well affect the evolution of floral fragrance (Strauss and Whittall, 2006; Theis, 2006). Herbivores can have a role in the evolution of fragrance because floral traits not only mediate interactions with mutualists but also with antagonists, provoking patterns of conflicting selection (Strauss and Whittall, 2006; Bronstein et al., 2007; Kessler and Halitschke, 2009; Schiestl et al., 2014; Sletvold et al., 2015; Knauer and Schiestl, 2017; Chapurlat et al., 2019). In addition, because of physiological cross-talk and/or genetic linkage between reproduction- and defense-related traits (reviewed by references Lucas-Barbosa, 2016; Rusman et al., 2019), they do not evolve independently from each other.
For these reasons, floral fragrance is expected to evolve under direct and indirect selection by both pollinators and herbivores. However, disentangling their relative contribution, and quantifying the evolutionary outcomes of their isolated and combined effects has remained challenging. Recently, using fast-cycling Brassica rapa plants and manipulating hand/bee pollination by Bombus terrestris and herbivory by Pieris brassicae caterpillars, we showed that plants under bee pollination and herbivory evolved reduced floral fragrance and lower pollinator attraction compared to plants that evolved under bee pollination only, suggesting that adaptation to pollinators was constrained by a resource allocation trade-off between reproduction and defense (Ramos and Schiestl, 2019). Although this previous study provided a direct experimental proof for the role of pollinators and herbivores in the rapid evolution of floral fragrance, its controlled conditions, and simplification of the pollinator- and herbivore-community may limit its extrapolation to the evolution of floral fragrance under natural conditions.
In this study we aimed to fill this gap by conducting experimental evolution in the field during 4 plant generations using wild-type self-incompatible B. rapa as model system. We used this plant due to the available knowledge on the identity of the floral volatile compounds and their role to attracting pollinators and herbivores (Schiestl et al., 2014; Knauer and Schiestl, 2015, 2017), and because its reliance on pollinators makes it a good system to study pollinator-mediated evolution (Schiestl et al., 2014). In a meadow (Figure 1A), we established plots planted with B. rapa plants which were subjected to two selection treatments, namely (i) plants with regular application of pesticide, to limit herbivore damage, and (ii) plants without pesticide application, with natural levels of herbivory. In both treatments, the pollinator community was allowed to access the plants. Plants with pesticide were treated once a month with a systemic pesticide that did not show any effects on floral traits and pollinator attraction (see section “Materials and Methods”), whereas plants without pesticide were treated with water. At the end of the experiment we collected seeds from plants of the generation 4 to grow and phenotype plants representing generation 5 under greenhouse conditions. These plants were used for analysis of evolutionary change of floral scent and morphometric floral traits.
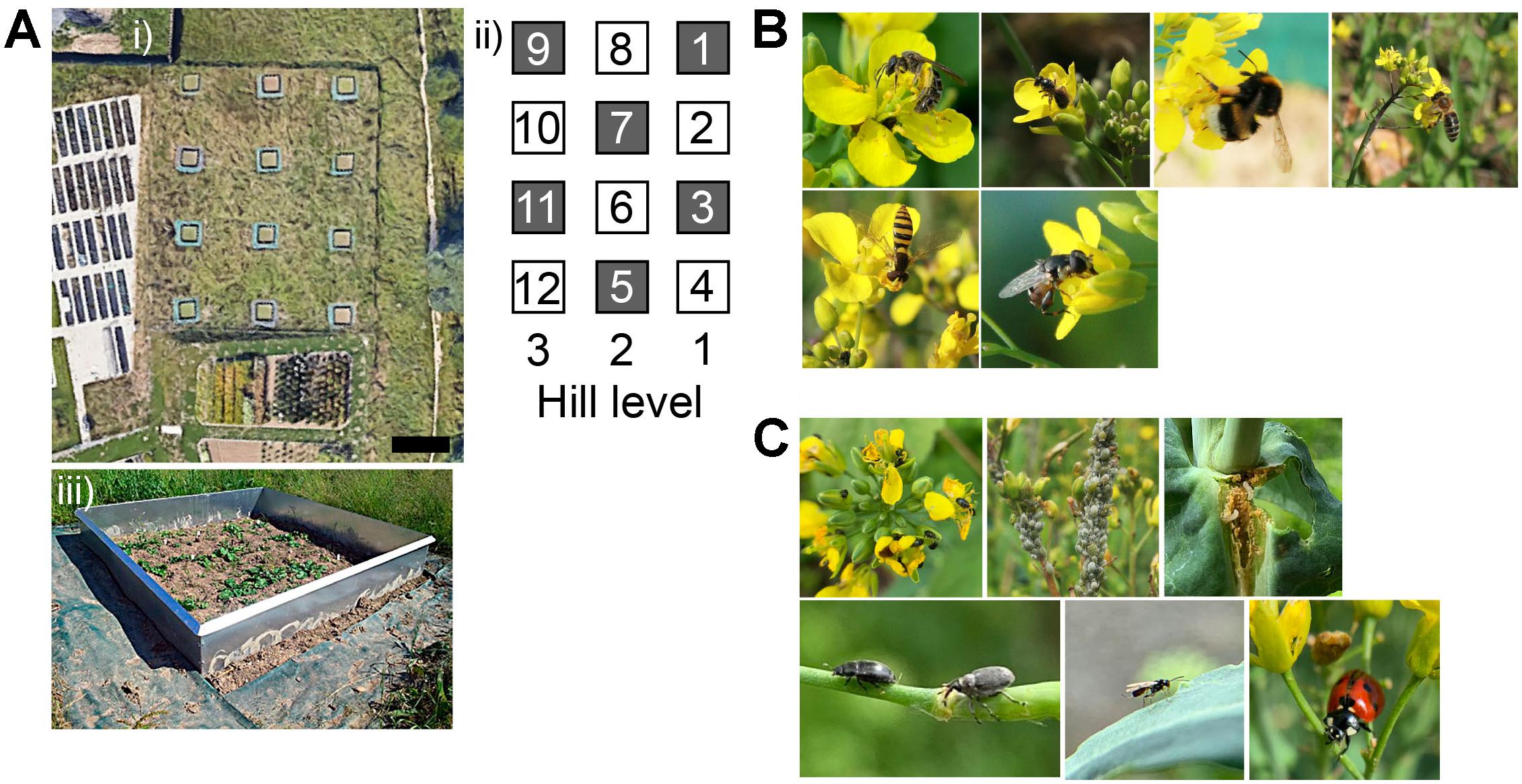
Figure 1. Experimental plots and interacting insects. (A,i) Aerial view of the experimental meadow showing the experimental plots of 2.5 × 2.5 m each. Image obtained from Google Maps (Google, LLC). The black bar at the bottom right depicts a 6 m scale. (ii) Graphic description of the spatial arrangement of plots in the meadow; plants without pesticide (gray squares), and plants with pesticide (white squares) were arranged in an alternating way. Numbers outside the squares indicate hill levels in the meadow, and numbers inside the squares indicate plot number (see section “Materials and Methods). (iii) Example of a weeded plot with pre-flowering B. rapa plants. (B) Some of the insect pollinators present during the experiment. Four bee species are shown on the top row (from left to right: solitary bee spp.1, solitary bee spp. 2, Bombus spp., and Apis mellifera), and two fly species on the bottom row (from left to right: Episyrphus spp., and syphid fly spp.). (C) Some of the insect herbivores and their predators present during the experiment on plants without pesticide. Top row, from left to right: pollen beetles on flowers, aphids on inflorescences, and larvae feeding inside the tissue. Bottom row, from left to right: a pollen beetle and a seedpod weevil, a parasitoid wasp on a leaf, and a ladybug.
Materials and Methods
Plants and Experimental Design
We used seeds of B. rapa from 54 full-sib families that were created by artificial crosses under greenhouse conditions (Schiestl et al., 2014); the original seed stock was obtained from a natural population near Maarssen, the Netherlands, from about 100 individual plants, where the species grows naturally as annual (Schiestl et al., 2014; Knauer and Schiestl, 2015). B. rapa is considered as common self-incompatible weed that occurs naturally throughout Europe and North America (de Jong and Hesse, 2012). Eight individuals from each of 54 full-sib families were sown out during March 2014 in a greenhouse under standardized soil, light and watering conditions. These plants were used at the onset of our experiment and constituted the first generation (G1).
First generation plants at the start of flowering were individually bagged, transported and planted in our experimental field site in 12 evenly spaced plots that had been prepared previously. We established the plots in a meadow situated on the south side of the Irchel Campus of the University of Zurich, Switzerland. The meadow was 40 m long × 35 m width and surrounded by a fence. Each plot consisted of a square of 2.5 × 2.5 m and was separated from each other plot by 6 m (Figure 1A, i). For plot preparation we removed all plants, plowed the soil, fixed plastic foil around the plots to prevent and delay weed recovering, and set an anti-slug metal fence around each plot (Figure 1A, iii). Each plot consisted of 36 individual plants of 36 different families randomly chosen out of the 54 available families. A total of 432 plants were planted in the field for G1. Note that such a sample size corresponds to the use of eight individuals per each of the 54 families, and thus, families were not represented in each of the 12 plots. We did so to increase the number of families and genetic variation, as opposed to have used only 36 families with one individual represented in every plot. Given the spatial arrangement of the plots, the two selection treatments (plants with and without pesticide) were assigned in an alternating way and thus evenly spread throughout the ecologically heterogeneous meadow; the plots of plants without pesticide had uneven numbers 1, 3, 5, 7, 9, 11, and the ones with pesticide had even numbers 2, 4, 6, 8, 10 and 12. Throughout the experiment, a very low number of B. rapa plants grew and reached the flowering stage in the plots 9, 11 without pesticide, and 10, 12 with pesticide, and at the end of the experiment we had not enough data from these plots, and therefore excluded them from the statistical analyses.
In the plants without pesticide, the natural community of herbivores was allowed to infest the plants; these plants were sprayed only with water. Throughout the generations, damage by chewing and piercing herbivores was observed in plants without pesticide, however, we did not quantify levels of herbivory. In the plants with pesticide, plants were sprayed once per month with the pesticide Biscaya® (Bayer GmbH, Austria), in a concentration of 2.5 ml per 5 L of water. Spraying of pesticide/water was performed with gentle movements following a zigzag pattern from the top of the plants at approx. 1 m distance when they were in vegetative stage. When the plants were flowering, spraying was done below the inflorescences in order to avoid spraying the flowers. After a plot was sprayed, we verified that all plants had their leaves covered evenly with pesticide/water in similar amounts, as a way to standardize the volume of pesticide sprayed in each plot. G1 plants were treated for the first time with either water or pesticide on the day that they were planted in the field. The water/pesticide application was repeated for every generation. At the onset of the experiment, we noted that the first application of pesticide in G1 plants generally worked well against insect herbivores, but herbivory by slugs was observed in more than 50% of the plants of both pesticide and non-pesticide plots. Thus, we also decided to apply 5 grams of slug pellets (Ferramol®, Neudorff, Germany) per plot in the plots of plants with pesticide spraying, and we set-up a slug-proof fence to all plots. The experiment spanned four successive generations, indexed as generations 1, 2, 3, and 4, and was performed from March 2014 to November 2017 (one generation in the field per year).
Despite 36 plants were planted in each plot from the onset of the experiment throughout the generations up to generation 4, several plants per plot did not reach reproductive stage, either due to mortality or because they remained in vegetative stage. Thus, as a way to standardize our fruit sampling procedure, we opted to collect five fruits from 10 to 15 plants per plot, and only the biggest plants were chosen. The criteria that we followed to consider a plant as big was based on a visual inspection of the plant height, number of inflorescences and presence of fruits. In wild-type B. rapa plants, plants with more inflorescences tend to produce more flowers, and produce more fruits through insect-mediated pollination (S. Ramos pers. obs.). This procedure was repeated every generation, up to generation 4.
Our sampling procedure may have introduced a bias (selection for tall plants) that could have affected our results, however, the same procedure was applied to plants in both selection treatments (plants with and without pesticide).
With the collected fruits per plant per plot, 100 fully developed seeds we randomly chosen and germinated in the greenhouse, from which 36 plants were randomly chosen and planted in the same plots from where the seeds were collected. Seed germination occurred simultaneously and was evident at the second day after sowing out. Planting in the field was done 20 days after germination in early spring (during April) each generation. Weeds were removed from the plots at least 1 week before B. rapa plants were planted in the field in order to facilitate their growth by reducing competition for resources with other herb species. The above described procedure allowed us to facilitate germination, speed up the growth of the plants in the early stages, and standardize the age of the plants among plots and treatments for every generation.
In 2017, plants of generation 4 (G4) were planted in early spring, and the fruits were collected at the end of August that year. For this last round of fruit collection from the field –in contrast to our above-mentioned method of collecting fruits from the biggest plants per plot during the experiment–, we collected fruits from all available plants within each plot independently of their size. In autumn 2017, 100 randomly chosen seeds per plot from G4 plants were sown out in the greenhouse in common garden conditions, in order to produce the plants for generation five (G5) for phenotyping. Standardized soil was used (Einheitserde, Germany). We then randomly chose between 28 and 37 plants per plot (sample size depended upon availability of germinated plants per plot), which were kept in the greenhouse under 16 h light, 22/23°C, 54% humidity and watered 2–3 times a day until flowering. These plants were used to evaluate the evolutionary changes.
Insect Pollinators and Herbivores
Throughout the experiment at the Irchel Campus, pollinator and herbivore insects that interacted with the plants were observed, although not systematically so no statistical analysis was done. We usually observed the insects once a month when pesticide and water were applied. Different studies have documented that Brassica species tend to attract similar or even the same species of insect pollinators and herbivores across different geographical regions (Root, 1973; Rader et al., 2009; Reddy, 2017; Rusman et al., 2018). Due to such well documented knowledge of the associated insects in Brassica plants and to the ease of identifying these insects through pictures, we identified them by comparing our photographs with those of the literature, and not by using a taxonomic identification key.
In addition, given that B. rapa is a self-incompatible species (de Jong and Hesse, 2012) that require pollen transfer via insect floral visitors to set seeds, and because stigmas and anthers are openly presented in its flowers, almost all visitors of a given size can act as pollinators. Thus, the floral visitors that we observed were considered as pollinators, in spite of not having quantified pollen transfer.
Phenotyping of Plants at Generation 5
For phenotyping, we measured six morphological floral traits, namely petal length, petal width, pistil length, long stamen length, short stamen length, and herkogamy (calculated as the subtraction of pistil length – long stamen length). Three consecutive fully open flowers from the main inflorescence per plant were measured with a digital caliper of the nearest 0.01 mm (Toolcraft, Japan), and nectar was removed using 5 μL micropipettes (Blaubrand, Wertheim, Germany). The mean per trait per plant was then used for subsequent analyses. Plant height was measured at the flowering peak in all plants. For flower volatile collection we sampled from 24 to 36 plants per plot. Inflorescences with at least seven open flowers were enclosed in glass cylinders previously treated with Sigmacote (Sigma-Aldrich), and the number of fully opened flowers were counted. Sigmacote is a silanizing reagent for glassware that creates a neutral hydrophobic film that reduces adsorption of volatiles on the glass surface1. The glass cylinders were closed with Teflon plates that had a central hole to avoid injuring the petiole. Volatiles were collected for 35 min using a push-pull system, where cleaned air with activated charcoal filters was pushed into the glass cylinder at a rate of 150 ml min–1, while air was pulled out of the cylinder at the same flow rate through a glass tube filled with 20 mg Tenax TA (60/80 mesh; Supelco, Bellefonte, PA, United States). Volatile collection was performed between 12:00 and 15:00 h within 2–4 days after the onset of flowering. Samples from empty glass cylinders were collected as air controls. Quantification of volatiles was conducted by gas chromatography with mass selective detection (GC–MSD). Samples were injected into a gas chromatograph (GC; Agilent 6890N; Agilent Technologies, Palo Alto, United States) with a Gerstel thermal desorption unit (TDU3, Gerstel, Mühlheim, Germany) and a cold injection system (CIS; Gerstel). For thermodesorption, the TDU was heated from 30 to 240°C at a rate of 60°C min–1 and held at a final temperature for 1 min. The CIS was set to −150°C during the trapping of eluting compounds from the TDU. For injection, the CIS was heated to 250°C at a rate of 12°Cs–1, and the final temperature was held for 3 min. The GC was equipped with a HP-5 column (0.25 mm diameter, 0.25 mm film thickness, 15 m length), and helium was used as carrier gas at a flow rate of 2 ml min–1. Compound identification and quantification were done with the Agilent MSD chemstation software (v.E.01.00, Agilent Technologies AG, Santa Clara, United States), by comparing the mass spectra of the samples with those of the reference (NIST) database and those of previously injected authentic standards for all compounds. Quantification of compounds was obtained through measurement of peak areas of selected target ions specific to the individual scent compounds; peak areas were converted into absolute amounts using calibration curves previously obtained for each compound using synthetic reference compounds in three different concentrations (Schiestl et al., 2014). For data analysis we only included VOCs that were present in significantly higher amounts than in the air controls following a t-test (in total 13 compounds). Absolute amounts of compounds were divided by the number of open flowers in the inflorescence at the time of scent collection. VOCs are shown in units of picograms per flower per liter of sampled air (liters of sampled air were calculated with data of the duration of collection, –35 min–, and the amount of pushed air, –150 ml of pushed air per minute–). These units also correspond to pg/flower/6.667 min of sampling time (to collect one liter of air it takes 6.667 min at a rate of 150 ml min–1).
The Effect of the Pesticide Alone
We tested the effects of the pesticide alone in an independent experiment by using 21 randomly chosen families from the same genetic families used in the generation 1. For this experiment, we grew between 2 and 4 full-sibling plants per family, totaling 84 plants. Half of the sibling plants per family were sprayed with pesticide while the other half was sprayed with water. We again used the pesticide Biscaya® (Bayer GmbH, Austria), in a concentration of 2.5 ml per 5 L of water, and application of pesticide/water was performed once a month with gently movements following a zigzag pattern from the top of the plants at approx. 1 m distance. To be able to test the effects of the pesticide alone on floral and vegetative traits (see Supplementary Table S1 for a list of traits), we kept these plants in the greenhouse until flowering to avoid herbivory by insects or mechanical damage, especially in the plants sprayed with water. Phenotyping was performed in the greenhouse (as described above in the subsection of phenotyping of plants at generation 5) in a total of 69 sibling plants; N = 35 plants without pesticide, and N = 34 plants with pesticide (for some traits, sample sizes are not the same due to loss of data, see Supplementary Table S1). In addition, we also wanted to test the effect of the pesticide alone on the behavior of insect pollinators. To do this, all of the 84 plants were taken out to a meadow located in the Botanical Garden of University of Zürich 2 months after germination and pollinator observations were performed for a full day from 8:00 to 15:00. In the meadow, the plants were arranged into eight plots with 10–11 plants each separated by 30 cm from each other. The distance between plots was 2 m. Pollinator observations were done in periods of 5 min per plot and the number of individuals landing on plants were recorded, accumulating 10–11 observation periods per plot (N = 84 observation periods). In the single day of pollinator observations bees were the most abundant floral visitors. Different fly species were also observed, however, due to their very low numbers, we did not include them in the analysis.
Statistical Analyses
We performed univariate linear mixed models (LMMs) on 22 phenotypic traits, including the total volatile emission (sum of all volatiles). Flower nectar, volatiles and seeds per flowers were ln(x + 1) transformed beforehand to achieve normal data distribution. Given that our experimental meadow included a smooth hill with a moisture gradient (the lower area was moister) we considered this ecological heterogeneity in the model by a variable termed “hill” (hill consisted of two levels, since level “3” including plots no. 9, 10, 11, and 12 were discarded from the analyses because they had too few plants, see description above; see Figure 1A, ii). We then combined the factors plot (plots 1–8) and hill (hill 1 and 2) by creating a new factor called “plot–hill,” so that each treatment (plants with and without pesticide) was replicated twice within each of the four levels of plot-hill; level 1 of plot-hill included plots 1 and 8 from hill 1 and 2, respectively, level 2 of plot-hill included plots 2 and 7 from hill 1 and 2, respectively, level 3 of plot-hill included plots 3 and 6 from hill 1 and 2, respectively, and level 4 of plot-hill included plots 4 and 5 from hill 1 and 2, respectively. Thus, the model included “treatment” and the interaction of “treatment × plot-hill” as fixed factors, and “plot–hill” as random factor. In such LMMs, a significant “treatment” effect indicates trait differences between selection treatments. We used this criterion as an indication of evolution driven by herbivore/pollinator selection. LMMs were fitted with restricted maximum likelihood (REML) (JMP® v.14, SAS institute Inc.). We estimated correlations among floral traits separately for each selection treatment in order to help with interpretation of the floral traits that showed significant evolutionary change in the same direction (i.e., increase of aromatic volatiles). We used the “corrplot” function from the corrplot package in R (R v.3.4.2, R Development Core Team). P-values were estimated with Bonferroni multiple testing correction.
For the data on the independent experiment to test the effect of the pesticide alone on plant traits and bee visitation, we also performed LMMs, where “treatment,” a factor with two levels (plants with and without pesticide), and the interaction of “treatment × family” were fixed factors, and “family” the random factor (Supplementary Table S1). For bee visitation, an LMM was performed with “total visits” as response variable, “treatment” as fixed effect and “plot” as random effect. The total number of bees were ln(1 + x) transformed beforehand to achieve normality (Supplementary Table S1). For all of our models, we verified that statistical assumptions (e.g., homogeneity of variance and normality) were met, following the protocol suggested by Zuur et al. (2010).
Results and Discussion
In the onset of the experiment, we tested the effect of the pesticide alone on floral morphological traits and volatiles, as well as on vegetative traits (27 traits in total), to figure out potential confounding effects of the pesticide itself on our experimental evolution study. We found no evidence that the pesticide alone had an effect on any phenotypic trait, except for the length of the short stamen, which seem to be increased in plants sprayed with pesticide (Supplementary Table S1). In addition, the pesticide alone had no effects on the number of bees visiting plants (Supplementary Table S1).
During the experiment, we recorded the presence of pollinator and herbivore species that are naturally associated with B. rapa plants (Supplementary Table S2). Overall, different bee species were the predominant pollinators, while the herbivore community in the plants without pesticide was diverse in both identity and feeding habits (Figures 1B,C). We did not quantify the levels of herbivory on our plants, however, we observed that the application of pesticide together with slug pellets was successful in reducing herbivore load in each generation. As a main result, we found that five aromatic volatile compounds, out of the thirteen analyzed, showed higher emission in plants with pesticide application, ranging from 11.4 to 33.2% compared to plants without pesticide application (Figure 2A).
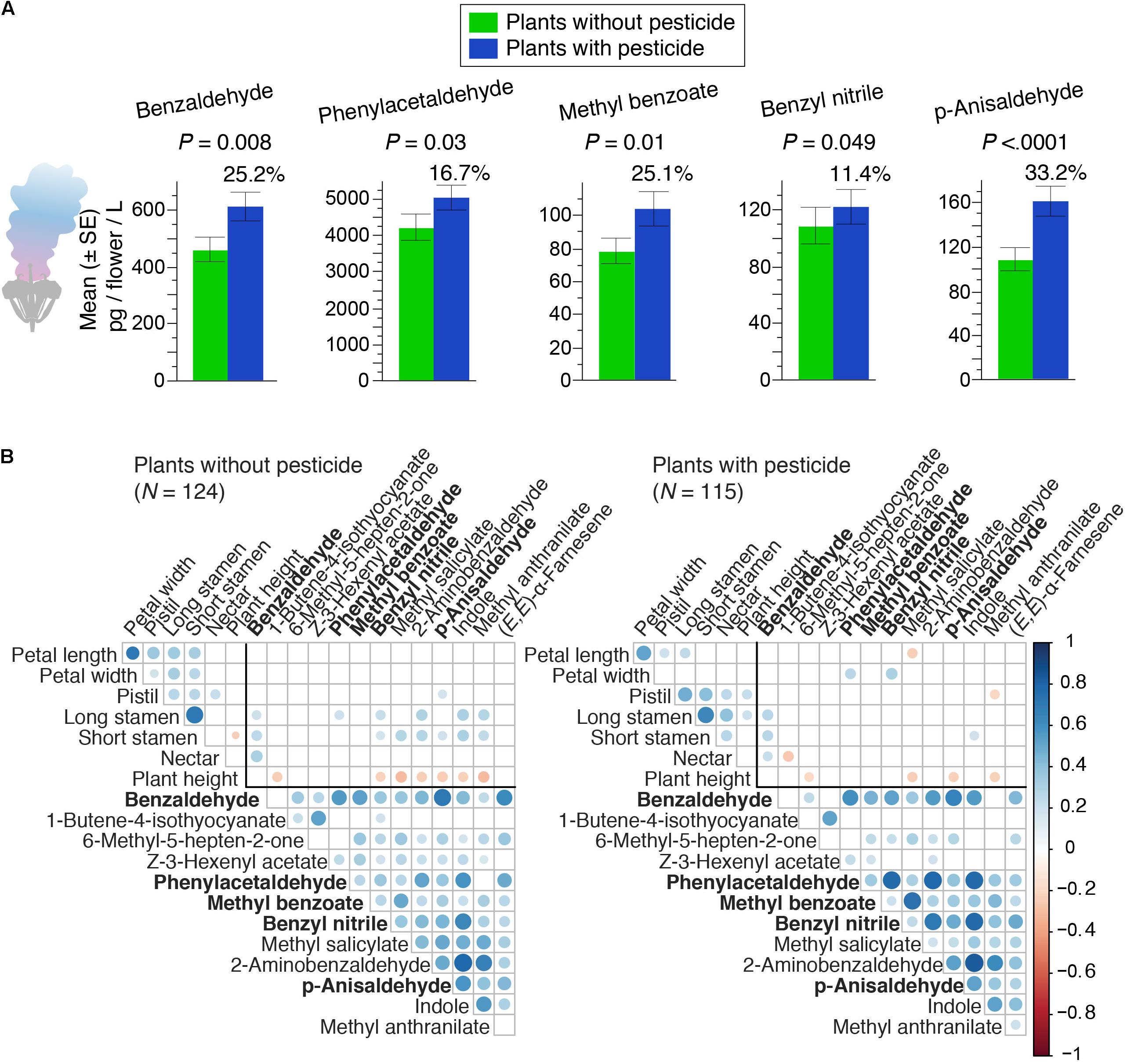
Figure 2. Evolutionary changes in plants with and without pesticide throughout experimental evolution in the field. (A) The five aromatic volatile compounds that evolved an increase in plants with pesticide after four generations of experimental evolution. The percentage value above the blue bars indicates the relative increased emission in plants with pesticide compared to plants without pesticide. P-values from linear mixed models are shown on top of each barplot (see Supplementary Table S3 for mean ± S.D. values in pg/flower/L, which corresponds to pg/flower/6.667 min, and statistics; N = 240 observations per volatile). (B) Correlation plots by selection treatment. In the plots, the size of the circles and the intensity of the color indicate the strength of the correlation (blue for positive and red for negative) according to the color bar at the right side. Cells with a circle indicate correlations (P < 0.05); blank cells indicate no correlation. Sample size is indicated within brackets. The thick line within each plot separates correlations between groups of traits, i.e., flower morphology, scent volatiles, and between morphology and scent (see Supplementary Table S4 for all correlation coefficients). Volatile names in bold are the ones that showed evolutionary change.
This evolutionary change in the volatiles was not independent, as most volatiles of a specific compound group were correlated with each other (Figure 2B). This finding is consistent with the results of our previous study performed under controlled and simplified conditions (Ramos and Schiestl, 2019), where the volatile compounds benzaldehyde, benzyl nitrile and p-anisaldehyde showed increased emission in plants that evolved under bee pollination without herbivory, compared to plants that evolved with bee pollination and herbivory (Ramos and Schiestl, 2019). The collective evidence therefore suggests that absence of herbivores relaxes the trade-offs between reproduction and defense and allows for rapid evolutionary change of the floral fragrance, likely due to pollinator-mediated selection.
The natural pollinator community in our experiment was dominated by bees, and bees have been shown in previous studies to impose positive directional selection on fragrance (Parachnowitsch et al., 2012; Gervasi and Schiestl, 2017; Knauer and Schiestl, 2017). Specifically, the volatile compounds phenylacetaldehyde, p-anisaldehyde, benzaldehyde, methyl benzoate and benzyl nitrile have been previously found to be under significant and generally positive selection by bumblebees in controlled experiments (Gervasi and Schiestl, 2017; Knauer and Schiestl, 2017). Studies performing electroantennographic recording (EAD) (Dötterl and Vereecken, 2010; Knauer and Schiestl, 2015; Zito et al., 2019) suggest that pollinator-mediated selection on certain floral VOCs is likely if pollinating insects can detect them (Schiestl et al., 2011; Schiestl and Dötterl, 2012; Parachnowitsch, 2014). In an thoroughly review, Dötterl and Vereecken (2010) documented several floral volatiles that have been shown to elicit positive behavioral responses in bees. Among these floral volatiles, they report p-anisaldehyde, methyl benzoate and phenylacetaldehyde, which showed evolutionary change in our study. In addition, benzaldehyde has also been reported to elicit positive antennal responses in Apis mellifera (Zito et al., 2019). Furthermore, it has been found that bees can readily associate floral rewards (pollen and nectar) with phenylacetaldehyde in wild-type B. rapa plants, and prefer these honest signals when foraging (Knauer and Schiestl, 2015, 2017). Overall, the ability of bees to detect these aromatic floral volatiles (benzaldehyde, phenylacetaldehyde, methyl benzoate p-anisaldehyde), suggest that the evolutionary changes observed could be explained by positive selection by pollinators together with a relaxation of herbivore-induced trade-offs.
Our interpretation that the change in fragrance represents an increased emission in plants with pesticide, rather than a decreased emission in plants without pesticide is preliminary, because of the lack of data on the fragrance emission in plants of the starting generation and phenotypic selection throughout the experiment. Furthermore, because we did not grow our experimental plants for one generation without herbivory before phenotyping, maternal effects could have impacted our findings. For individual floral scent compounds, however, transgenerational inheritance due to maternal effects and/or to epigenetic mechanisms were not detectable in an earlier study using herbivory in an inbred accession of B. rapa (Kellenberger et al., 2018), whereas seed production did show maternal effects with a significant increase one generation after herbivory had stopped. The relative contribution of maternal effects or epigenetic changes on the evolutionary change of floral volatiles –and in general in floral evolution–, are topics that deserve further investigation.
Apart from floral fragrance, none of the other analyzed traits showed differences between plants of the two selection treatments (Supplementary Table S3). This result is consistent with previous short-term evolution studies performed under controlled conditions showing no detectable evolutionary change in some floral morphological traits (e.g., flower number, corolla size), in spite of significant positive selection (Gervasi and Schiestl, 2017; Knauer and Schiestl, 2017; Ramos and Schiestl, 2019). Such finding might be caused by differences in the available standing genetic variation between morphological and chemical floral traits and/or their patterns of genetic covariation, which may constrain or enhance their evolutionary response (Cai et al., 2016; Zu et al., 2019). Indeed, recent evidence suggests that natural and artificial selection and genetic variance-covariance (G-matrix) can predict the rapid evolution of floral fragrance with more accuracy than that of morphological floral traits (Zu et al., 2019).
In conclusion, our study demonstrates that floral fragrance can exhibit rapid evolution likely through pollinator-mediated selection when the physiological and ecological compromises imposed by herbivores are relaxed. Furthermore, in spite of the complexity of plant-insect interactions in nature, our findings support previous experimental evolution performed under highly controlled and simplified pollinator and herbivore conditions. It thus reinforces that relevant findings can be achieved with such an ecologically simplified setting, which allows the control of deterministic factors and the elimination of variation in abiotic factors. Altogether, flowers, as we see them today, might reflect the result of evolutionary compromises between reproduction and defense, owing to the selection caused by both pollinators, herbivores, and other factors poorly studied (e.g., microorganisms, see Rebolleda-Gómez et al., 2019).
Data Availability Statement
The datasets generated for this study are available on request to the corresponding author.
Author Contributions
FS and SR designed the study, interpreted the data, and reviewed and edited the manuscript. SR performed the experiments, collected and analyzed the data, and wrote the manuscript.
Funding
This research was funded by grants to FS from the European Union’s Seventh Framework Program (FP7/2007-2013 and FP7/2007-2011) under grant agreement number 281093, and a full graduate fellowship funded by the Consejo de Ciencia y Tecnología CONACYT-México to SR (CVU-346745).
Conflict of Interest
The authors declare that the research was conducted in the absence of any commercial or financial relationships that could be construed as a potential conflict of interest.
Acknowledgments
We deeply thank the help of Alice Balmer, Luca Arrigo, Rayko Jonas, Markus Mierhofer, and Daniel Schlagenhauf, as well as a number of undergrad students for their help during the 4 years of this experiment; Franz Huber helped with GC-MS analysis. Bernhard Schmid helped with the establishment of field plots at the Irchel campus of UZH. The comments of the two reviewers and the handling editor greatly improved the quality and clarity of our manuscript, thus we appreciate their time and advice.
Supplementary Material
The Supplementary Material for this article can be found online at: https://www.frontiersin.org/articles/10.3389/fevo.2020.00030/full#supplementary-material
Footnotes
References
Bronstein, J. L., Huxman, T. E., and Davidowitz, G. (2007). “Plant-mediated effects linking herbivory and pollination,” in Ecological Communities: Plant Mediation in Indirect Interaction Webs, eds T. Ohgushi, T. P. Craig, and P. W. Price, (Cambridge: Cambridge University Press), 75–103.
Cai, J., Zu, P., and Schiestl, F. P. (2016). The molecular bases of floral scent evolution under artificial selection: insights from a transcriptome analysis in Brassica rapa. Sci. Rep. 6, 1–8. doi: 10.1038/srep36966
Chapurlat, E., Ågren, J., Anderson, J., Friberg, M., and Sletvold, N. (2019). Conflicting selection on floral scent emission in the orchid Gymnadenia conopsea. New Phytol. 222, 2009–2022. doi: 10.1111/nph.15747
de Jong, T. J., and Hesse, E. (2012). Selection against hybrids in mixed populations of Brassica rapa and Brassica napus: model and synthesis. New Phytol. 194, 1134–1142. doi: 10.1111/j.1469-8137.2012.04122.x
Dötterl, S., and Vereecken, N. J. (2010). The chemical ecology and evolution of bee–flower interactions: a review and perspectives. The present review is one in the special series of reviews on animal–plant interactions. Can. J. Zool. 88, 668–697. doi: 10.1139/Z10-031
Gervasi, D. D. L., and Schiestl, F. P. (2017). Real-time divergent evolution in plants driven by pollinators. Nat. Commun. 8:14691. doi: 10.1038/ncomms14691
Gross, K., Sun, M., and Schiestl, F. P. (2016). Why do floral perfumes become different? Region-specific selection on floral scent in a terrestrial orchid. PLoS One 11:e0147975. doi: 10.1371/journal.pone.0147975
Harder, L. D., and Johnson, S. D. (2009). Darwin’s beautiful contrivances: evolutionary and functional evidence for floral adaptation. New Phytol. 183, 530–545. doi: 10.1111/j.1469-8137.2009.02914.x
Kellenberger, R. T., Desurmont, G. A., Schlüter, P. M., and Schiestl, F. P. (2018). Trans-generational inheritance of herbivory-induced phenotypic changes in Brassica rapa. Sci. Rep. 8, 1–9. doi: 10.1038/s41598-018-21880-2
Kessler, A., and Halitschke, R. (2009). Testing the potential for conflicting selection on floral chemical traits by pollinators and herbivores: predictions and case study. Funct. Ecol. 23, 901–912. doi: 10.1111/j.1365-2435.2009.01639.x
Knauer, A. C., and Schiestl, F. P. (2015). Bees use honest floral signals as indicators of reward when visiting flowers. Ecol. Lett. 18, 135–143. doi: 10.1111/ele.12386
Knauer, A. C., and Schiestl, F. P. (2017). The effect of pollinators and herbivores on selection for floral signals: a case study in Brassica rapa. Evol. Ecol. 31, 285–304. doi: 10.1007/s10682-016-9878-8
Lucas-Barbosa, D. (2016). Integrating studies on plant-pollinator and plant-herbivore interactions. Trends Plant Sci. 21, 125–133. doi: 10.1016/j.tplants.2015.10.013
Parachnowitsch, A. L. (2014). New synthesis: the evolutionary ecology of floral volatiles. J. Chem. Ecol. 40:859. doi: 10.1007/s10886-014-0491-4
Parachnowitsch, A. L., Raguso, R. A., and Kessler, A. (2012). Phenotypic selection to increase floral scent emission, but not flower size or colour in bee-pollinated Penstemon digitalis. New Phytol. 195, 667–675. doi: 10.1111/j.1469-8137.2012.04188.x
Rader, R., Howlett, B. G., Cunningham, S. A., Westcott, D. A., Newstrom-Lloyd, L. E., Walker, M. K., et al. (2009). Alternative pollinator taxa are equally efficient but not as effective as the honeybee in a mass flowering crop. J. Appl. Ecol. 46, 1080–1087. doi: 10.1111/j.1365-2664.2009.01700.x
Raguso, R. A. (2008). Wake up and smell the roses: the ecology and evolution of floral scent. Annu. Rev. Ecol. Evol. Syst. 39, 549–569. doi: 10.1146/annurev.ecolsys.38.091206.095601
Ramos, S. E., and Schiestl, F. P. (2019). Rapid plant evolution driven by the interaction of pollination and herbivory. Science 364, 193–196. doi: 10.1126/SCIENCE.AAV6962
Rebolleda-Gómez, M., Forrester, N. J., Russell, A. L., Wei, N., Fetters, A. M., Jessica, D., et al. (2019). Gazing into the anthosphere: considering how microbes influence floral evolution. New Phytol. 224, 1012–1020. doi: 10.1111/nph.16137
Reddy, G. V. P. (2017). Integrated Management of Insect Pests on Canola and other Brassica Oilseed Crops. Oxfordshire: Centre for Agriculture and Bioscience International.
Root, R. B. (1973). Organization of a plant-arthropod association in simple and diverse habitats: the fauna of collards (Brassica oleracea). Ecol. Monogr. 43, 95–124. doi: 10.2307/1942161
Rusman, Q., Lucas-Barbosa, D., and Poelman, E. H. (2018). Dealing with mutualists and antagonists: specificity of plant-mediated interactions between herbivores and flower visitors, and consequences for plant fitness. Funct. Ecol. 32, 1022–1035. doi: 10.1111/1365-2435.13035
Rusman, Q., Lucas-Barbosa, D., Poelman, E. H., and Dicke, M. (2019). Ecology of plastic flowers. Trends Plant Sci. 24, 725–740. doi: 10.1016/j.tplants.2019.04.007
Schiestl, F. P. (2010). The evolution of floral scent and insect chemical communication. Ecol. Lett. 13, 643–656. doi: 10.1111/j.1461-0248.2010.01451.x
Schiestl, F. P., and Dötterl, S. (2012). The evolution of floral scent and olfactory preferences in pollinators: coevolution or pre-existing bias? Evolution 66, 2042–2055. doi: 10.1111/j.1558-5646.2012.01593.x
Schiestl, F. P., Huber, F. K., and Gómez, J. M. (2011). Phenotypic selection on floral scent: trade-off between attraction and deterrence? Evol. Ecol. 25, 237–248. doi: 10.1007/s10682-010-9409-y
Schiestl, F. P., and Johnson, S. D. (2013). Pollinator-mediated evolution of floral signals. Trends Ecol. Evol. 28, 307–315. doi: 10.1016/j.tree.2013.01.019
Schiestl, F. P., Kirk, H., Bigler, L., Cozzolino, S., and Desurmont, G. A. (2014). Herbivory and floral signaling: phenotypic plasticity and tradeoffs between reproduction and indirect defense. New Phytol. 203, 257–266. doi: 10.1111/nph.12783
Sletvold, N., Moritz, K. K., and Agren, J. (2015). Additive effects of pollinators and herbivores result in both conflicting and reinforcing selection on floral traits. Ecology 96, 214–221. doi: 10.1890/14-0119.1
Strauss, S. Y., and Whittall, J. B. (2006). “Non-pollinator agents of selection on floral traits,” in Ecology and Evolution of Flowers, eds L. D. Harder, and S. C. H. Barrett, (New York, NY: Oxford University Press), 120–139.
Theis, N. (2006). Fragrance of Canada thistle (Cirsium arvense) attracts both floral herbivores and pollinators. J. Chem. Ecol. 32, 917–927. doi: 10.1007/s10886-006-9051-x
Zito, P., Tavella, F., Pacifico, D., Campanella, V., Sajeva, M., Carimi, F., et al. (2019). Interspecific variation of inflorescence scents and insect visitors in Allium (Amaryllidaceae: Allioideae). Plant Syst. Evol. 305, 727–741. doi: 10.1007/s00606-019-01601-6
Zu, P., Blanckenhorn, W. U., and Schiestl, F. P. (2016). Heritability of floral volatiles and pleiotropic responses to artificial selection in Brassica rapa. New Phytol. 209, 1208–1219. doi: 10.1111/nph.13652
Zu, P., Schiestl, F. P., Gervasi, D., Li, X., Runcie, D., et al. (2019). Floral signals evolve in a predictable way under artificial and pollinator selection in Brassica rapa using a G-matrix. bioRxiv [Preprint]. doi: 10.1101/675413
Keywords: floral signals, scent, VOCs, pollination, herbivory, trade-off, Brassica rapa (Brassicaceae), experimental evolution
Citation: Ramos SE and Schiestl FP (2020) Evolution of Floral Fragrance Is Compromised by Herbivory. Front. Ecol. Evol. 8:30. doi: 10.3389/fevo.2020.00030
Received: 10 October 2019; Accepted: 03 February 2020;
Published: 19 February 2020.
Edited by:
Thomas Seth Davis, Colorado State University, United StatesReviewed by:
Stefan Dötterl, University of Salzburg, AustriaSalvatore Cozzolino, University of Naples Federico II, Italy
Copyright © 2020 Ramos and Schiestl. This is an open-access article distributed under the terms of the Creative Commons Attribution License (CC BY). The use, distribution or reproduction in other forums is permitted, provided the original author(s) and the copyright owner(s) are credited and that the original publication in this journal is cited, in accordance with accepted academic practice. No use, distribution or reproduction is permitted which does not comply with these terms.
*Correspondence: Florian P. Schiestl, Zmxvcmlhbi5zY2hpZXN0bEBzeXN0Ym90LnV6aC5jaA==
†Present address: Sergio E. Ramos, Department of Biological Sciences, University of Pittsburgh, Pittsburgh, PA, United States